- 1“Iuliu Hatieganu” University of Medicine and Pharmacy, Cluj-Napoca, Romania
- 2Research Center for Functional Genomics, Biomedicine and Translational Medicine, “Iuliu Hatieganu” University of Medicine and Pharmacy, Cluj-Napoca, Romania
- 3MEDFUTURE–Research Center for Advanced Medicine, “Iuliu-Hatieganu” University of Medicine and Pharmacy, Cluj-Napoca, Romania
- 45th Surgical Department, Municipal Hospital, Cluj-Napoca, Romania
- 511th Department of Oncological Surgery and Gynecological Oncology, University of Medicine and Pharmacy “Iuliu Hatieganu,” Cluj-Napoca, Romania
- 6Department of Surgery, The Oncology Institute “Prof. Dr. Ion Chiricuţǎ,” Cluj-Napoca, Romania
- 7Department of Medical Biochemistry, “Iuliu Hatieganu” University of Medicine and Pharmacy, Cluj-Napoca, Romania
- 8Department of Functional Genomics and Experimental Pathology, The Oncology Institute “Prof Dr. Ion Chiricuţǎ,” Cluj-Napoca, Romania
Hepatocellular carcinoma (HCC) is one of the most common causes of cancer-related death worldwide. Current treatment options for inoperable HCCs have decreased therapeutic efficacy and are associated with systemic toxicity and chemoresistance. Sirtuin 1 (SIRT1) is a nicotinamide adenine dinucleotide–dependent enzyme that is frequently overexpressed in HCC, where it promotes tumorigenicity, metastasis, and chemoresistance. SIRT1 also maintains the tumorigenic and self-renewal proprieties of liver cancer stem cells. Multiple tumor-suppressive microRNAs (miRNAs) are downregulated in HCC and, as a consequence, permit SIRT1-induced tumorigenicity. However, either directly targeting SIRT1, combining conventional chemotherapy with SIRT1 inhibitors, or upregulating tumor-suppressive miRNAs may improve therapeutic efficacy and patient outcomes. Here, we present the interaction between SIRT1, miRNAs, and liver cancer stem cells and discuss the consequences of their interplay for the development and treatment of HCC.
Introduction
Hepatocellular carcinoma (HCC) is the most frequent primary liver malignancy and among the most common causes of cancer-related death worldwide (1, 2). The majority of cases occur due to HCV or alcoholic cirrhosis (3). However, HCC can also develop in obese individuals with non-alcoholic steatohepatitis (NASH) (4–7). The increasing incidence in diet-induced NASH is estimated to upsurge the number of patients with NASH-related HCC (3). The main therapeutic options for HCC include liver transplantation, surgical resection, and chemotherapy (8). However, most patients present with advanced-stage, unresectable HCC. Moreover, first-line treatment compounds such as sorafenib have low response rates (9) and are associated with systemic toxicity and chemoresistance (10, 11). Therefore, a better understanding of the underlying mechanisms that promote HCC development, chemoresistance, and metastases is vital for improving patient outcomes (12).
Mammalian sirtuins (SIRT1-7) are NAD+-dependent deacetylases that are involved in a wide variety of biological processes including energy metabolism and lifespan and health span regulation (13). Mammalian sirtuins possess histone deacetylase, mono-ADP-ribosyltransferase, desuccinylase, demalonylase, demyristoylase, and depalmitoylase activity (14). SIRT1 is chiefly localized in the nucleus and plays a role in genomic stability, telomere maintenance, and cell survival (15, 16). SIRT1 regulates both histones and multiple downstream non-histone targets such as estrogen receptor-alpha (17), PPARγ (18), PGC-1α (19), androgen receptor (20), FOXO transcription factors (21), p53 (22), NF-κB (23), and Survivin (24). SIRT1 can also upregulate oncogenes: β-catenin (25), c-Myc (26), and HIF-1α (27) increasing their activity as a result.
SIRT1 is vital for the physiological function of healthy tissues. For instance, SIRT1 null mice have defects in hepatocyte metabolism and a shortened life span (28). SIRT1 deletion in mice hepatocytes results in hepatitis and hepatic steatosis (29). Oppositely, inducing SIRT1 activity in healthy tissues with synthetic activators or transgenic expression provided a plethora of benefits. SIRT1 overexpression reduced the release of pro-inflammatory cytokines and increased cell viability (23, 30, 31). SIRT1 also preserved the functions of hepatocytes and adipocytes against obesity (32). Overall, SIRT1 can be called a “Master Metabolic Regulator” (33), which is essential for normal hepatic function.
The Expression and Function of SIRT1 in HCC
SIRT1 has a multifaceted relationship with oncogenesis. SIRT1 is overexpressed in multiple malignancies, including human myeloid leukemia (34), colon cancer (35), prostate cancer (36), and squamous cell carcinomas (37). Conversely, SIRT1 expression is reduced in ovarian cancers and glioblastoma (38) when compared to corresponding normal tissues. Overall, SIRT1 may function as both an oncogene and tumor suppressor depending on subcellular localization, age, type of tissue, and concomitant mutations in related signaling pathways.
In HCC, SIRT1 was the only member of the Sirtuin family consistently overexpressed (39) and deemed vital for all stages of HCC tumorigenesis (39). Moreover, it was repeatedly demonstrated that SIRT1 was frequently overexpressed in HCC biopsies when compared to corresponding adjacent non-cancerous liver parenchyma (40–42) and its expression was necessary for the maintenance of HCC tumorigenesis (15, 43–45). Generally, SIRT1 mRNA levels are similar in HCC and non-malignant adjacent tissue, suggesting that SIRT1 is increased in HCC via a post-transcriptional mechanism (15). Hypermethylated in cancer 1 (HIC1) and p53 negatively regulate SIRT1 mRNA transcription and are often mutated or dysfunctional in HCC. Thus, SIRT1 overexpression may be partly accounted for by the decreased inhibition of its transcription. However, SIRT1 protein levels are also preserved post-translationally via reduced degradation and increased stability (15, 46).
Additionally, SIRT1 was overexpressed in a multitude of human HCC cell lines such as HKC1-4, SNU-423, HKC1-2, PLC5 SNU-449, SK-Hep-1, Huh-7, HepG2, and Hep3B (15, 45), when compared to normal liver cell lines (47).
However, there is still some controversy regarding SIRT1's role in HCC, as some reports showed that SIRT1 was downregulated in human HCC samples and hypothesized it had tumor-suppressive roles (38). The multifaceted role of SIRT1 in carcinogenesis suggests (48) that its function is dependent on cancer type and the state of downstream or upstream molecules that influence its oncogenicity (49). The role of SIRT1 in HCC may also depend on its subcellular localization. Although, in HCC cells, SIRT1 had a predominant nuclear localization where its expression promotes tumorigenesis, it was reported that cytoplasmatic SIRT1 may have tumor-suppressive roles (50).
Multiple lines of evidence suggest that SIRT1 expression has survival-promoting effects in both normal hepatocytes and in HCC cells. In healthy mice, SIRT1 overexpression protected against malignancies (51) and basal SIRT1 expression was vital for maintaining physiologic hepatic morphology and normal lifespan (44). However, basal SIRT1 levels were lower in mouse livers compared to other viscera, indicating that the hepatocytes may be more sensitive to the under- or overexpression of SIRT1 (44).
Similarly, SIRT1 expression is vital for the proliferation and survival of HCC cells (44). Malignant cells were shown to enhance their function by hijacking survival signaling pathways of non-malignant cells (52, 53). Therefore, SIRT1 activity may promote cellular function and survival and inhibit cancerous transformation in normal hepatocytes; after malignant transformation, SIRT1's functionality may be employed in promoting tumorigenesis and sustaining HCC survival (15). That is, SIRT1's activity may promote cellular survival independent of the cancerous or non-cancerous state of the hepatocytes. As of yet, there are no reports of experimentally induced oncogenesis via SIRT1 overexpression. Finally, SIRT1 overexpression does not appear to be a cancer-initiating event but rather a cancer-induced adaptive mechanism that promotes survival and proliferation (42). However, because SIRT1 simultaneously regulates a wide spectrum of biological processes, its role in HCC oncogenesis is incompletely understood and further research is warranted in order to clarify at which level and via what mechanisms do HCC cells increase and become dependent on SIRT1 expression. Additionally, the interplay between SIRT1 and the other six sirtuin family members and their role in HCC should be further explored.
Multiple studies evaluated the prognostic value of SIRT1 expression in HCC. SIRT1 overexpression correlated with the development of portal vein tumoral thrombosis, decreased overall survival rates, lower disease-free survival, and advanced TNM stages (54). Patients with SIRT1-positive HCC biopsies had a decreased 10-year survival compared to SIRT1-negative HCC patients. SIRT1 protein levels appear to be positively correlated with HCC grades; specifically, SIRT1 expression is higher in advanced HCC stages. One meta-analysis investigated the prognostic and clinical implications of SIRT1 expression in HCC. It showed that heightened SIRT1 expression was associated with decreased patient overall survival and death-free survival. Moreover, increased SIRT1 expression correlated with larger tumor size, higher p53 expression, high alpha-fetoprotein (AFP) levels and advanced TNM stages (55). However, it was highlighted that, for the studies examined in the meta-analysis, there was no clear cutoff value or unified standard for the measurement of SIRT1 expression. Even though the statistical power was limited, it can be concluded that increased SIRT1 expression correlated with a poor HCC prognosis (26).
The deacetylation function of SIRT1 is vital for its oncogenic role in HCC. When the deacetylation domain of SIRT1 is mutated, the proliferation and colony formation ability of HCC cells are inhibited (40). Inhibition of SIRT1 in HCC cells, either through knockdown or administration of SIRT1 inhibitors, led to decreased tumor development in vitro and in vivo and exerted cytostatic as opposed to a cytotoxic effect (42, 44), while SIRT1 overexpression accelerated HCC growth (44). However, in vivo experiments indicate that other mutations in relevant cancer-related pathways might determine the function of SIRT1, thus, the role of SIRT1 should be viewed as context dependent (56). SIRT1 is also implicated in the malfunction of multiple HCC signaling pathways such as FOXO1, p53, and TGF (57–59). SIRT1 downstream targets involved in HCC progression include YAP (Yes-associated protein) (44, 60), PTEN/PI3K/Akt (61, 62), telomerase, and p53 (63). Overall, in HCC, SIRT1 acts as a potential oncogene (45). Further on, we will elaborate on the interplay between SIRT1 and the aforementioned pathways and molecules.
SIRT1 expression was also shown to prevent malignant development in a mouse model of metabolic-syndrome associated HCC. Communicable infectious diseases have been successfully dealt with in the past decades. However, in the early twenty-first century, non-communicable diseases have become a principal health hazard. The global spread of high calorie and low fiber, Western style foods, coupled with decreases in physical exercise led to a global epidemic of metabolic syndrome. The financial burden inflicted by metabolic syndrome is in the trillions (64). The epidemiology of obesity-associated HCC (65) and in vitro and in vivo experiments suggest that an obesogenic lifestyle, via pro-inflammatory cytokines, insulin resistance, steatosis, and lipotoxicity, may progress from metabolic syndrome to NASH (6) and HCC (65). Overall, diet-induced NASH is estimated to upsurge the number of patients with NASH-related HCC (3, 66–68).
SIRT1 expression promotes genomic stability in normal hepatocytes and appears to be protective against high-fat diet (HFD)-induced HCC. Moreover, the role of SIRT1 as a protector against metabolic syndrome is clear (69, 70). For instance, enhancing SIRT1 activity in a mouse model of type 2 diabetes leads to improved insulin resistance and controls hyperglycemia (7, 71, 72). Moreover, transgenic mice that systemically overexpress SIRT1 were protected from the hazards produced by a HFD (69, 73).
One model of metabolic syndrome-associated cancer examined the effects of a threefold systemic SIRT1 expression on diet-associated HCC.
Mice overexpressing SIRT1 systemically at approximately threefold that of the normal WT mice had measurably increased hepatic SIRT1 deacetylase activity. These mice had improved glucose tolerance, decreased adipose inflammation, and were protected from other negative effects of HFD such as hepatic steatosis. Moreover, compared to the control group, SIRT1-overexpressing mice displayed a lower incidence of HCC after the chronic administration of a HFD. Part of the protective effects of SIRT1 expression in HCC development was attributed to decreasing NF-κB-induced inflammation and malignant transformation (51).
Overall, systemic threefold SIRT1 overexpression protects hepatocytes but not fibroblasts from DNA damage and translates as safeguard against HFD-induced HCC (51).
SIRT 1 may promote protective effects against HCC via its effect on β-catenin (25) —an oncogene associated with epithelial cancer (74). This may account for the carcinoma-selective protection provided by SIRT1 overexpression.
Collectively, the current body of literature suggests that SIRT1 expression has a pro-tumorigenic role in HCC but is not a cancer-initiating event.
Role of SIRT1 in the Tumorigenicity of Liver Cancer Stem Cells
Multiple models have been proposed in order to explain the functional and histological heterogeneity of solid cancers. One of them proposes a hierarchical organization of tumoral cell populations where a minor cell population termed cancer stem cells (CSCs) with self-renewal and differentiation capacities repopulate tumors and establish the histological and functional heterogeneity characteristic of most cancers (75, 76). Intra-tumoral CSCs are capable of differentiation and self-renewal and give rise to tumors identical to the original one in primary and metastatic sites (76, 77). HCC tissue samples possess intra-tumoral heterogeneity (78, 79), and a subpopulation of cells with stem cell-like proprieties might give rise to HCC and accelerate cancerous proliferation (80–82). Therefore, due to their proliferation and differentiation abilities, liver CSC (LCSC) have been incriminated for HCC initiation (83), chemoresistance (84, 85), metastasis (83), recurrence, and overall dismal patient outcome (83, 86–89).
For instance, hepatoblasts are cell progenitors with the ability to differentiate into hepatocytes (90). During chronic liver inflammation, hepatoblasts and other hepatic progenitor cells accrue genetic and epigenetic modifications, leading to their conversion in LCSCs (91). Importantly, through a process called dedifferentiation, hepatocytes can also undergo malignant transformation by acquiring CSC phenotypes (92). HCC CSCs can be identified through multiple biological markers such as CD133, CD90, and CD13 and ubiquitin-specific protease 22 (USP22) (82, 93). It was shown that SIRT1 plays a vital role in the self-renewal and maintenance of embryonic stem cells (94) and hematopoietic stem cells (95) and is also implicated in the biology of LCSC.
SIRT1–MRPS5 Signaling Pathway
Liver cancer stem cells (LCSCs) use enhanced mitochondrial respiration to generate ATP. In contrast, cancer cells primarily rely on aerobic glycolysis (Warburg effect) to generate ATP. Metabolic reprogramming is a hallmark of cancer and plays a vital role in cancer progression (96); however, the regulator of metabolic reprogramming that drives the switch from oxidative phosphorylation to aerobic glycolysis in LCSC is not fully understood.
Mitochondrial ribosomal protein S5 (MRPS5) is required for the enhanced mitochondrial function of LCSCs and promotes cancer commencement and development (97, 98). Acetylation promotes the nuclear translocation of various proteins (99). SIRT1 is highly expressed in LCSCs (100, 100) where it deacetylates MRPS5, thus determining its subcellular localization. In LCSCs, deacetylated MRPS5 is located in the mitochondria where it promoted oxidative phosphorylation, stimulated NAD+ production, and improved mitochondrial function, thus preserving LCSC stemness. Increased NAD+ also maintains SIRT1 activity and promotes a SIRT1–MRPS5 positive feedback loop. In contrast, in HCC cells, acetylated MRPS5 translocates to the nucleus and consequently promotes metabolic flexibility and enhanced glycolysis (101).
Relevantly, multiple acetylated proteins act as metabolic enzymes in the extra nuclear environment and as transcription factors when located inside the nucleus (102). Nuclear translocation of MRPS5 led to enhanced expression of glycolytic proteins and a switch in metabolism, from oxidative phosphorylation to a Warburg-type metabolism. Thus, MRPS5 may function as a transcription factor when localized in the nucleus and consequently regulate the expression of glycolytic genes. However, the exact mechanism by which MRPS5 increases the expression of glycolytic proteins is not clear. Further research should establish whether acetylated MRPS5 acts as a glycolysis promoting transcription factor in HCC.
SIRT1 expression was higher in LCSC compared to HCC cells. The comparatively lower SIRT1 expression in HCC cells may account for the predominant nuclear localization of MRPS5. However, the tumor microenvironment is highly dynamic and SIRT1 expression may be heterogeneous in different cellular subpopulations at different time points. Thus, fluctuating SIRT1 expression may contribute to tumoral heterogeneity and self-renewal by facilitating the transition of LCSCs to HCC cells. This may explain why MRPS5 is found in higher concentration in HCC cell's nucleus even though SIRT1 is frequently overexpressed in HCC cells and it would be expected to deacetylate MRPS5 and thus promote its mitochondrial localization.
SIRT1 also induced the mitochondrial unfolded protein response (UPRmt) that preserves cell longevity and metabolic fitness (103, 104). LCSCs present accelerated oxidative phosphorylation, which is associated with increased ROS production. Therefore, the SIRT1–UPRmt axis maintained LCSC viability by reducing ROS.
Metformin was reported to be beneficial to HCC patients (58). Interestingly, metformin downregulates MRPS5, which inhibits the activity of mitochondrial complex 1, thus decreasing the function of LCSCs (see Figure 1).
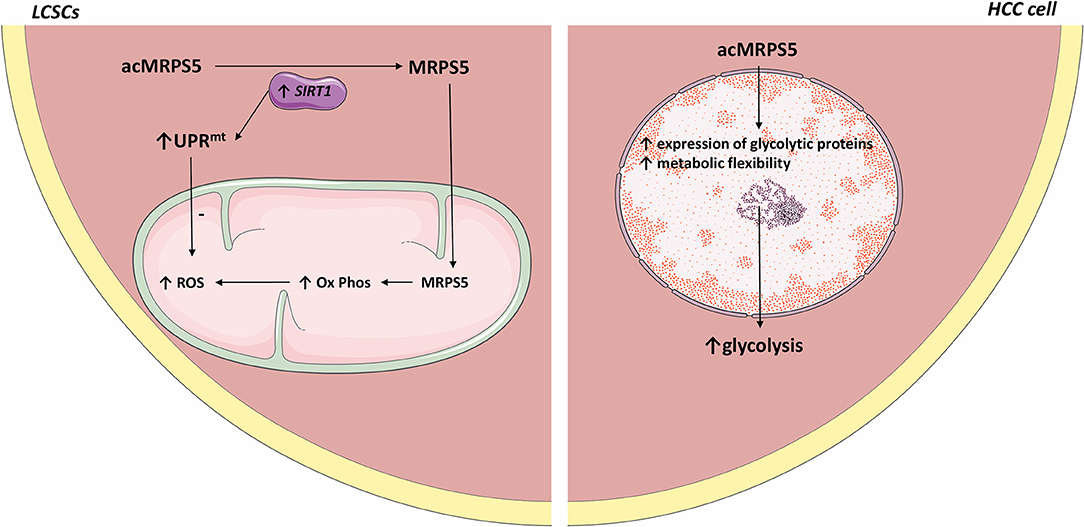
Figure 1. Comparative representation of MRPS5 signaling in liver cancer stem cells and HCC cells. In LCSCs, SIRT1 deacetylates MRPS5, which promotes its mitochondrial localization. Mitochondrial MRPS5 increases oxidative phosphorylation and consequently ROS. However, SIRT1 also promotes UPRmt activity, which decreases ROS levels. Conversely, in HCC cells, MRPS5 is localized in the nucleus where its activity promotes increased cellular glycolysis. All the figures in this article were made using images from http://smart.servier.com.
Human HCC samples with increased SIRT1 expression and high cytoplasmic MRPS5 levels presented more CSCs and were associated with high metastases rates, cancerous embolization, increased tumor size, and decreased survival compared to patients whose HCC biopsies showcased low cellular SIRT1 expression and high nuclear MRPS5 levels. This further indicates that the metabolic reprogramming induced by SIRT1/MRPS5 axis is crucial for the stemness of CSCs.
In summary, the SIRT1/MRPS5 axis augmented the metabolic plasticity and reprogramming of LCSC by ameliorating and maintaining mitochondrial function, consequently promoting hepatocarcinogenesis. Further studies should explore the interaction between SIRT1 and other MRPs. Administration of LCSC-targeted oxidative phosphorylation inhibitors or MRPS5 inhibitors should be explored in future experiments.
SIRT1–SOX Signaling Pathway
In vivo mice models demonstrated that SIRT1 is necessary for maintaining the self-renewal and tumorigenicity of LCSCs. In those models, silencing SIRT1 expression reduced the incidence of HCC compared with the controls (100). SIRT1 is overexpressed in LCSCs where it is necessary for maintaining oncogenesis and self-renewal and is correlated with a poor prognosis of HCC patients.
The core embryonic transcription factor circuitry (SOX2, c-Myc, Oct4, Nanog) is implicated in the self-renewal of CSCs (105–107). SIRT1 induces tumorigenicity in a subpopulation of LCSC in a SOX2-dependent manner. SIRT1 knockdown in LCSCs decreased SOX2, Oct4, and Nanog expression levels. Treating LCSCs with SIRT1 inhibitors TV6 and EX-527 reduced SOX2 and Nanog (100).
SIRT1 regulates SOX2 gene expression thus primes LCSC for self-renewal. DNA (cytosine-5)-methyltransferase 3A (DNMT3A) catalyzes the transfer of methyl groups to CpG DNA structures. In LCSCs, SIRT1 expression inhibited DNMT3A, consequently promoting hypomethylation of the SOX2 promoter and activated SOX2 gene expression, consequently inducing self-renewal and oncogenicity (100). Notably, HCC stage and recurrence were correlated with SIRT1 and SOX2. Thus, in LCSCs, SOX2 is a prime downstream regulator of SIRT1-induced self-renewal and oncogenesis (100).
The Ubiquitin Proteasome Pathway (UPP) is the prime mechanism for protein catabolism in mammals. UPP is also responsible for degrading SIRT1 (108, 109). IGF1 was vital for the self-renewal and tumoral growth of LCSCs. Namely, IGF signaling inhibited the UPP pathway, thus increasing SIRT1 protein levels and function in LCSCs (100). Thus, IGF1 may enhance the self-renewal of LCSCs by mediating SIRT1 levels (100).
Inhibiting SIRT1 in LCSCs reduced SOX2 expression and strongly repressed tumor growth in both in vivo and in vitro models. Overall, SIRT1 deacetylase activity was vital for the oncogenicity and self-renewal of LCSCs. Selective SIRT1 inhibition in LCSCs is a potential therapeutic target hindering HCC development and progression.
SIRT1–MEK Signaling Pathway
Initially, HCC heterogeneity was attributed to hepatocytes since the liver was presumed to lack a distinct stem cell population (110). Nevertheless, accumulating evidence shows that HCCs display multiple cell subpopulations, some of which have stem cell characteristics (111, 112), and increased expression of CSC1 markers (Nanog, SOX2, Oct4) was identified in some HCC subpopulations (113, 114). These subpopulations were associated with increased HCC invasion and chemoresistance (115). The core embryonic transcription factor's circuitry (SOX2, c-Myc, Oct4, Nanog) is essential for LCSCs self-renewal (105–107).
Mitogen-activated protein kinase 1 (MAPK1/MEK1) is an oncogene implicated in cancer development and therapy resistance. Active MEK1 signaling is vital for the proliferation and oncogenic potential of LCSC. The interplay between MEK1 and SIRT1 was crucial for upholding the self-renewal and growth of LCSCs. Decreased MEK1 expression in LCSCs reduced the expression of Oct4, c-Myc, SOX2, and Nanog and significantly decreased LCSC self-renewal and proliferation (46). Mechanistically, MEK1 signaling activation increased SIRT1 expression and protein stability and inhibited the proteasomal degradation of SIRT1; this promoted self-renewal and oncogenicity in LCSCs, resulting in poor prognosis of HCC patients (46).
In a cohort of 148 HCC patients, the expression of the MEK1–SIRT1 pathway was strongly correlated with tumor size, vascular and capsular invasion, clinical tumor stage, and poor prognosis (46). MEK1 knockdown in LCSCs isolated from HCC samples lowered SIRT1's half-life, suppressed oncogenicity and self-renewal, and lessened the expression of stem cell markers. However, these results need to be further replicated and validated in vivo. Inhibiting SIRT1/MEK1 signaling impedes HCC oncogenesis and should be further explored as a possible therapeutic target (see Figure 1).
Notch3–SIRT1–LSD1–SOX2 Signaling Pathway
Lysine demethylase 1 (LSD1) is an epigenetic regulator responsible for demethylating various histones and controls the pluripotency of stem cells (116–118). LSD1 is overexpressed in HCC cells compared to normal hepatic parenchyma. Moreover, LSD1 is overtly expressed in LCSCs where it directly regulated the transcription of the SOX2 gen, promoted self-renewal and tumorigenesis, and was associated with a poor patient prognosis (119). Similar to the effect of SIRT1 via DNMT3A, LSD1 demethylated the SOX2 promoter and consequently increased its expression and improved LCSC stemness. Acetylation inhibits the enzymatic activity of LSD1 and stimulates its degradation via UPP. SIRT1 deacetylated LSD1 and thus increased its stability (see Figure 2).
Notch signaling is vital for cell proliferation and survival (120). In HCC, Notch receptors are mostly overexpressed and their ligand expression was associated with aggressive tumor phenotypes (121). Notch promoted HCC development and metastasis through activating the Wnt/β-catenin pathway (122, 123). Moreover, Notch signaling was shown to promote CSC self-renewal. Notch3 signaling induced SIRT1 expression and facilitated LDS1 deacetylation and activated LSD1, consequently promoting LCSC self-renewal. The Notch3-dependent pathway was crucial for LCSC self-renewal and in vivo tumor dissemination (see Table 1).
SIRT1–CPEB1 Signaling Pathway
Cytoplasmic polyadenylation element-binding protein 1 (CPEB1) mediates mRNA translation and negatively regulates HCC stemness and chemoresistance. Moreover, CPEB1 expression was low in HCC and LCSCs when compared to normal hepatocytes (124).
In HCC, CPEB1 upregulation decreased chemoresistance, accelerated doxorubicin-induced apoptosis, inhibited cell migration and self-renewal and decreased tumoral growth while CPEB1 knockdown had the opposite effect.
The 3′ untranslated region (3′UTR) of SIRT1 mRNA presents two cytoplasmic polyadenylation element (CPE) sequences. CPEB1 binds to SIRT1 mRNA and could curtail the poly(A) extremity of SIRT1 mRNA, thus decreasing SIRT1 protein levels. Thus, CPEB1 regulated SIRT1 expression at the post-transcriptional level. Hence, decreased CPEB1 expression may account for SIRT1 overexpression, which in turn promotes LCSCs self-renewal, chemoresistance, and HCC cell spheroid formation (124).
The Interplay Between miRNAs and SIRT1 in the Development and Treatment of HCC
MicroRNAs (miRNAs/miRs) are endogenous, single-stranded, non-coding regulatory RNAs (131) that exert their biological functions by integrating into the RNA-inducing silencing complex of their target mRNA where they attach to the 3′UTR and either inhibit mRNA translation or induce its degradation (47, 132). The function of multiple miRs was shown to be dysregulated in HCC and in a plethora of other cancers. Depending on the cellular environment and target genes, miRs can function as either oncogenes or tumor suppressors (47, 133, 134). miRs were demonstrated to play a role in HCC development, proliferation metastasis, and therapeutic resistance (121, 135, 136).
p53-miR-34a–SIRT1 Signaling Pathway
0404
0404, is a DNA-damaging compound with no cytotoxic effects on non-cancerous human hepatocytes. 0404 induced apoptosis and decreased growth in an in vivo HepG2 HCC model. However, P53 WT HepG2 cells were more responsive to 0404 compared to the p53 mutant Huh7 cell lines (137).
P53 modulates the transcription of multiple miRs. In turn, numerous miRs target the 3′UTR region of p53 mRNA. Hence, p53 and miRs may form a feedback loop (138).
The miR-34 family is typically silenced in multiple tumors and was identified as the most frequent p53-induced miRs (139, 140). miR-34a was shown to increase p53 transcription and acetylation and induced apoptosis in HCC cells. In HepG2 but not in Huh7 cell lines, 0404 upregulated p53 and miR-34a expression, increased acetylated p53, and downregulated SIRT1 protein expression, which consequently inhibited HCC growth (137). The anticancer mechanisms induced by 0404 and its toxicity and efficacy should be examined in vivo on multiple HCC cell lines.
Quercetin
Quercetin is a flavonoid with anti-cancer proprieties and low toxicity to non-cancerous cells. Quercetin activated apoptosis and cell cycle arrest in HepG2 and Huh7 cells. However, p53 status determined the sensitivity of HCC cells to quercetin. Namely, in p53 WT cell lines, proliferation was reduced by a significantly lower quercetin quantity, when compared to p53 mutants. HepG2 cells treated with quercetin showcased increased p53 expression, miR-34a activity, and SIRT1 inhibition (140, 141).
SIRT1 deacetylates p53, resulting in the cessation of its activity. miR-34a silences SIRT1 mRNA by binding to its 3′UTR region (142). Quercetin activated p53, which induced miR-34a and consequently silenced SIRT1 mRNA expression, leading to increased p53 acetylation, activity, and consequently apoptosis, thus forming a positive feedback loop. In summary, quercetin activates the p53-miR-34-SIRT1 axis and induces a positive feedback loop that suppresses tumor formation (143).
miR-34a-IL-24 Oncolytic Adenoviruses
miR-34a expression was shown to be downregulated in multiple cancers including HCC, where it exerts a tumor-suppressive role. In HCC, miR-34 levels were inversely correlated with vascular invasion, necrosis, and histological staging, and low miR-34a expression was associated with decreased overall survival (144).
miR-34a delivery via oncolytic adenovirus killed HCC cells in vitro, with low toxicity to normal hepatocytes. Importantly, miR-34a expressed in HCC via oncolytic adenoviruses, downregulated SIRT1 and Bcl-2 (144) expression, and induced cancerous cytotoxicity (144).
The cytokine IL-24 is known to inhibit tumoral angiogenesis and activates tumoral apoptosis. It was hypothesized that increasing the expression of both IL-24 and miR-34a would provide synergistic therapeutic benefits. Oncolytic adenovirus-mediated transfer of both miR-34a and IL-24 led to a more potent inhibition of HCC cell growth than administering either miR-34a or IL-24 separately. However, the antitumoral mechanisms of the oncolytic adenoviruses used in the abovementioned study are insufficiently understood and its effects on metastasis should also be explored.
Butyrate–miR-22–SIRT1 Signaling Pathway
Butyrate, a short-chain fatty acid, is produced by the intestinal microbiome via anaerobic fermentation and is subsequently absorbed by the hepatocytes (145). Butyrate was shown to induce apoptosis and decrease tumorigenesis in multiple malignancies (146, 147). Butyrate was reported to inhibit SIRT1 gene expression in some types of cancer, although this has not yet been demonstrated in HCC (148).
miR-22 was shown to be downregulated in HCC and its low levels contributed to tumorigenesis (149). miR-22 expression activated apoptosis and inhibited the in vitro proliferation of the Huh7 cells. Oppositely, SIRT1 expression was high in Huh7 cells and increased the expression of antioxidants such as superoxide dismutase (SOD), consequently maintaining cell proliferation (40).
In Huh7 cells, butyrate induced miR-22, which directly binds the 3′UTR region of SIRT1 and downregulates its expression; this reduced SOD activity and augmented ROS production, increasing caspase 3 and cytochrome c activity, thus promoting apoptosis (150). Furthermore, by downregulating SIRT1, miR-22 increased PTEN and gsk-3 expression and downregulated β-catenin and p-akt expression and thus may promote apoptosis and decrease HCC proliferation (150).
However, multiple aforementioned experiments were only performed with 2D cultures or in vitro (150, 151). 3D cultures better mimic the in vivo environment (152) and it was reported that therapeutic approaches are less effective in 2D cultures when compared to 3D ones. Drug resistance was also reported to be higher in 3D cultures (153–155). Thus, replicating therapeutic interventions performed with monolayer cultures with spheroids, or in vivo, may offer a better understanding of their translational potential (see Figure 3).
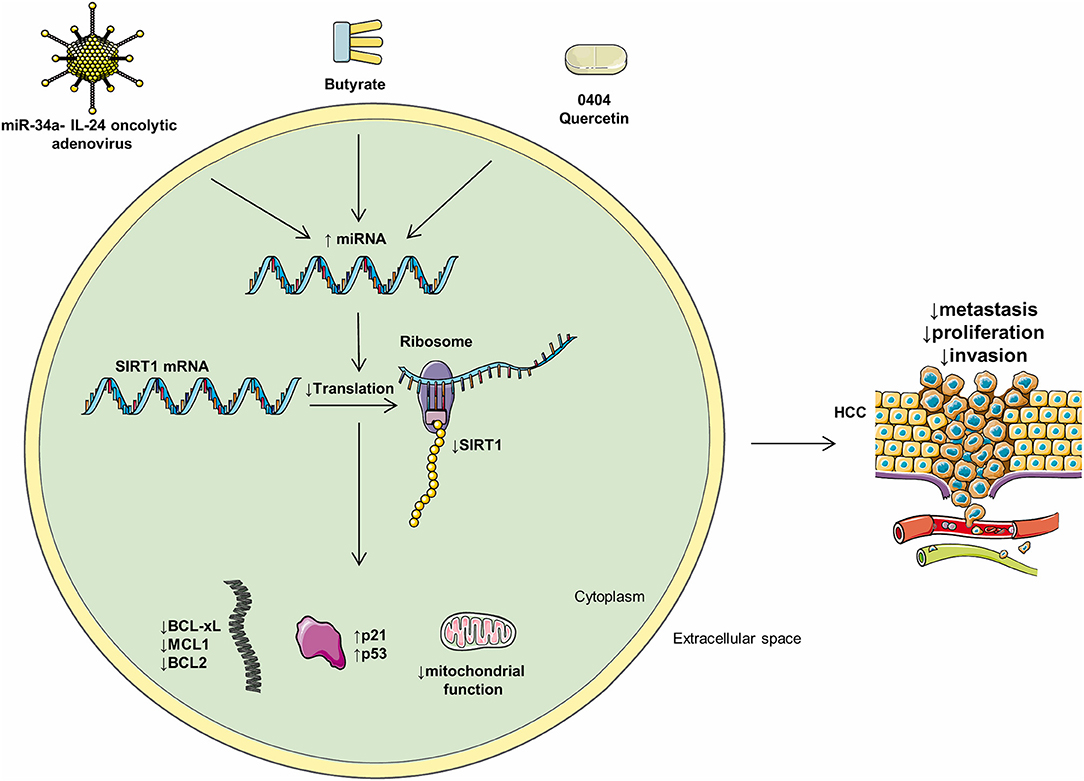
Figure 3. Mediators of miRs and their intracellular effects. Multiple compounds may influence the levels of intracellular miRs and negatively regulate HCC progression. Oncolytic adenoviruses, butyrate, 0404, and quercetin positively regulate multiple HCC miRs and, as a consequence, decrease metastasis, proliferation, and invasion.
miR-133b-Sirt1-GPC3-Wnt/β-Catenin Signaling Pathway
The Wnt/β-catenin pathway is essential for the physiological functioning of the liver (156) and is also implicated in oncogenesis (157). In non-cancerous cells, SIRT1 deacetylates β-catenin, thus constricting it to the cytoplasm and limiting its ability to trigger transcription and induce cell proliferation (25).
The β-catenin gene is commonly mutated in HCC (158). SIRT1 is negatively associated with β-catenin mutation in HCCs, demonstrating that SIRT1 may promote oncogenesis in cancers independent of Wnt/β-catenin.
miR-133b mainly acts as a tumor suppressor and is markedly reduced in a multitude of cancers (159). miR-133b expression was decreased in a majority of HCC biopsies when compared to paired adjacent normal tissue (160). Moreover, miR-133b upregulation in HepG2 cells strongly repressed HCC cell proliferation and invasion and promoted apoptosis (47). Additionally, miR-133b upregulation decreased tumor growth, in nude mice with orthotopic HepG2 cell tumors. miR-133b directly targets and is inversely correlated with SIRT1 in human HCC cells. Enhanced miR-133b expression strongly decreased SIRT1 expression at both mRNA and protein levels. Overall, the anti-cancer effect of miR-133b in HCC cells appears to be achieved through inhibiting SIRT1 expression.
GPC3 stimulates HCC growth via Wnt signaling. It has been reported that SIRT1 inhibition decreases the expression of cancer markers AFP and GPC3. Decreased GPC3 and AFP expression indicate the development of an increasingly differentiated cell phenotype and may be beneficial (42). GPC3 is a membrane protein that is extremely overexpressed in HCC and is involved in hepatocarcinogenesis (161–163). GPC3 suppression in HCC cells inhibited proliferation and the expression of anti-apoptotic proteins (Mcl-1, Bcl-2, and Bcl-xL) and also increased TGF-β expression (47). GPC3 also promoted HCC cell EMT (164).
Malignant cells are characterized by inadequate intercellular adhesion and increased cellular motility. E-cadherin is a molecule vital for cell–cell adhesion in epithelial tissues (165). Dysregulation of E-cadherin-modulated intercellular adhesion is observed in human carcinomas and correlates with acquisition of metastatic potential (166). Downregulating SIRT1 decreased GPC3 mRNA and increased the mRNA expression of E-cadherin (42). miR-133b upregulation or GPC3 downregulation repressed GPC3, Mcl-1 Bcl-2, Bcl-xL, and SIRT1 expression and increased the expression of E-cadherin. Moreover, GPC3 downregulation canceled the SIRT1 overexpression-induced inhibition of apoptosis and accelerated invasion and proliferation (47). Additionally, in HCC cells, miR-133b overexpression inhibited GPC3 expression and cell proliferation.
GPC3 interacts with Wnt ligands, consequently stimulating cell migration and proliferation in HCC (167, 168). Activation of the Wnt signaling pathway induces cytoplasmic buildup and nuclear translocation of the transcription factor β-catenin. Intranuclear β-catenin induces the expression of genes that regulate cell differentiation, proliferation, migration, and apoptosis (168–170). SIRT1 upregulation increased the expression of GPC3, which consequently stimulated the Wnt/β-catenin pathway and induced cytosolic accumulation and nuclear translocation of β-catenin. Concluding, miR-133b suppresses cell proliferation and migration and activates cell apoptosis, by inhibiting the Sirt1-GPC3-Wnt/β-catenin signaling pathway (47).
However, SIRT1 was reported to suppress Wnt/β-catenin in multiple malignancies including mouse and human HCCs (171–175). Mechanistically, SIRT1 expression was shown to stimulate β-catenin phosphorylation, which promoted its degradation. SIRT1 regulation of β-catenin is contingent on protein kinase A (PKA). SIRT1 may stimulate PKAs auto-phosphorylation but may also influence PKA through transcriptional regulation of PGC1α–PKA's upstream regulator.
SIRT1 can activate the transcription of PGC1α in HCC cells. PGC1α was demonstrated to elevate cAMP, which, in turn, stimulates the activity of PKA. Thus, SIRT1 may collaterally promote the phosphorylation of PKA and β-catenin through a PGC1α-cAMP-dependent manner (175). SIRT1 also increases βTrCP gene expression. βTrCP is crucial for Sirt1-induced Wnt/β-catenin signaling inhibition. Namely, phosphorylated β-catenin is ubiquitinated by βTrCP and consequently degraded.
Overall, suppressing SIRT1 expression in HCC for therapeutic purposes may activate Wnt/β-catenin signaling and promote tumorigenesis. To short-circuit this potential side effect, the Wnt/β-catenin pathway should be simultaneously inhibited (175).
miR-449–SIRT1–SREBP-1c Signaling Pathway
Sterol regulatory element binding protein (SREBP)-1c is a transcription factor predominantly localized in adipocytes and hepatocytes, where it regulates lipid synthesis-related gene expression. Abnormal lipid metabolism has been connected to HCC development (65, 176) and SREBP-1c dysfunction is involved in oncogenesis (177–179). SIRT1 regulates lipid metabolism and its activation decreased the expression of hepatic SREBP-1c (180).
miR-449 is part of a miR family that regulates apoptosis and proliferation and may promote tumor suppression via downregulating histone deacetylases (181, 182). In Huh7 and HepG2 cell lines, miR-449 directly targets and inhibits SIRT1 mRNA expression, which consequently inhibits SREBP-1c and thus constrains cholesterol and fatty acid biosynthesis (151). Additionally, the SIRT1-SREBP-1c downstream metabolic oncogenes 3-hydroxy-3-methylglutaryl CoA reductase (HMGCR) and fatty acid synthase (FASN) (177, 183) are also downregulated. Overall, miR-449 expression decreases mice HCC xenograft development (151, 184).
Thus, miR-449 inhibited the SIRT1–SREBP pathway which decreased proliferation and DNA synthesis, reduced lipid anabolism, and suppressed tumorigenesis in Huh7 and HepG2 cell lines (151).
MALAT1–miR-204-5p–SIRT1 Signaling Pathway
The lncRNA metastasis associated lung adenocarcinoma transcript 1 (MALAT1) is decidedly expressed in HCC where it stimulates growth and invasion. MALAT1 activates mechanistic target of rapamycin (mTOR) signaling (185) and enhances the development of HCC CSC (186).
Oppositely to MALAT1, miR-204 promotes apoptosis by activating p53 and inhibiting anti-apoptotic protein Bcl-2 (187). miR-204 also inhibited cancer stemness and EMT and increases chemosensitivity (187, 188). However, MALAT1 expression was negatively correlated with miR-204 levels. MALAT1 directly attaches to miR-204 and negatively regulates its expression (189). SIRT1 appears be a vital intermediary in the interplay between MALAT1 and miR-204. It is known that SIRT1 is vital for HCC EMT, migration, and invasion. SIRT1 is directly targeted and silenced by miR-204 (189). However, SIRT1 and MALAT1 attach to the same miR-204 site; thus, MALAT1 might be in competition with SIRT1 for binding miR-204; this decreases miR-204-induced SIRT1 inhibition. Overall, MALAT1 negatively regulated miR-204 activity and consequently increased SIRT1, which, in turn, induced HCC migration and invasion (189). Inhibiting MALAT1 decreased the aggressive behavior of HCC, which makes it a potential therapeutic target (189).
Moreover, it was shown that miR-204-5p expression was decreased in multiple human HCC cell lines (189). Decreased miR-204-5p levels were associated with HCC metastasis and poor patient outcome. In HCC cell lines, miR-204-5p binds to the 3′UTR region of SIRT1 and consequently decreases its expression at both mRNA and protein levels. Likewise, in these same cell lines, miR-204-5p was inversely associated with SIRT1 expression. miR-204-5p decreased the invasion and survival of HCC cells by reducing SIRT1 expression and protein levels both in vivo and in vitro (190). Overall, miR-204-5p induced post-transcriptional inhibition of SIRT1 in multiple human HCC cell lines. Importantly, miR-204-5p overexpression strongly downregulated both SIRT1 mRNA and protein levels in HCC cells and thus attenuated tumoral growth. As such, miR-204-5p is a potential diagnostic marker and increasing its expression and activity in HCC cells is a therapeutic option that needs to be further explored (190).
miR-486
miR-486 downregulation may be a hallmark of HCC development and contributed to the differentiation of LCSCs into HCC cells (43). miR486 was shown to be strongly downregulated in HCC samples and in LCSCs (43).
On the contrary, SIRT1 expression was increased in LCSCs and maintained the tumorigenic and self-renewal proprieties of LCSCs in vivo, and was inversely correlated with miR-486 levels in LCSCs (43). miR-486 directly targets and strongly suppresses SIRT1 expression and decreased the tumorigenic and chemo-resistant properties of LCSCs and suppressed HCC invasion and tumorigenicity (43).
Overall, this further validates the role of SIRT1 as a promoter of HCC development, invasion, and recurrence, in part through maintaining the stemness of CSCs (43).
miR-29c
Decreased levels of the miR-29 family were associated with poor HCC survival. The miR-29 family have tumor-suppressive roles by targeting Mcl-1 and Bcl-2. Relevantly, miR-29c exerts tumor-suppressing functions by inhibiting hepatocytic SIRT1. Ectopic miR-29c expression decreased SIRT1 expression and consequently repressed cell proliferation. miR-29c directly targets and suppresses SIRT1 mRNA translation in hepatocytes (191).
miR-29c was shown to be strongly downregulated in HCC biopsies and correlated with poor patient prognosis. The 5-year survival rate of HCC patients with low miR-29c expression was pointedly inferior to that of HCC patients with high miR-29c expression. Future interventions that upregulate miR-29c in HCC models may provide insights into a more efficient management of HCC (191).
miR-29a
Decreased miR-29a expression is common in HCC, where it promoted metastasis and, as a predictor of early post-surgical recurrence, diminished overall survival and disease-free survival (192). miR-29a suppressed HCC proliferation. Tellingly, miR-29a was strongly downregulated in HCC tissue biopsies when compared with adjacent normal tissue. miR-29a upregulation suppressed HCC cell proliferation and colony formation. Mechanistically, miR-29a increased p21 expression and decreased CDK4 and CyclinD1 expression, consequently suppressing cell cycle progression; it also targeted the 3′UTR region of SIRT1 mRNA and decreased SIRT1 mRNA and protein expression consequently overturning HCC cell proliferation (192). Oppositely, SIRT1 overexpression decreased the protective effects exerted by miR-29a. Overall, data suggests that miR-29a may serve both as a prognostic marker and as a therapeutic target for HCC suppression.
miR-138
It has been confirmed that miRs have suppressive or promotive effects on tumor metastases and invasion and impact HCC progression (193).
miR-138 functions as a tumor suppressor and it was found to be downregulated in multiple cancers (194). In HCC cells, miR-138 suppresses cell invasion and proliferation. Interestingly, miR-138 expression levels were inversely correlated with SIRT1 mRNA levels in HCC tissues (193). Upregulation of miR-138 expression downregulated SIRT1 at the level of mRNA and protein levels. miR-138 binds to the 3′UTR unique complementary site of the SIRT1 gene and directly inhibits SIRT1 expression, which leads to hindered HCC proliferation, migration, and invasion (193). miR-138 was inversely correlated with SIRT1 mRNA in HCC tissues.
Multiple studies showed that miR-138 expression was downregulated in a majority of examined HCC samples compared with peritumoral non-cancerous tissue (193). SIRT1 is overexpressed while miR-138 levels are decreased in HepG2, SMMC7721, Bel7404, and HCCM3 compared to the normal hepatic cell line L02 (193).
Tellingly, increasing miR-138 expression in HepG2 and SMMC7721 cell lines inhibited their proliferation (193). This suggests that decreased miR-138 expression was associated with increased SIRT1 mRNA expression in HCC (193). This validates the role of miR-138 in HCC proliferation and metastasis (195).
miR-34a
miR-34a is a tumor suppressor in breast, colon (196), and a plethora of cancers. miR-34a expression was correlated with HCC metastasis (197). miR-34a downregulated c-Met expression and consequently inhibited HCC invasion and migration (198), which have oncogenic or tumor suppressor functions (199).
miR34a expression was decreased in Hep3B and Huh7 cells when compared to normal hepatocyte cell lines. Inducing miR-34a overexpression in Hep3B and Huh7 significantly decreased cellular invasion and migration (200). Moreover, miR-34a overexpression also decreased SIRT1 mRNA and protein levels and increased acetylated p53. Thus, miR-34a overexpression downregulated SIRT1 expression, which increased acetylated p53 levels and consequently suppressed HCC metastasis (200).
All in all, data suggest that the post-transcriptional overexpression of SIRT1 may be promoted by loss of suppressive mRNAs that normally target and inhibit its expression.
The Role of SIRT1 in the Metastasis of HCC
Metastases are a great contributor to HCC morbidity (12). SIRT1 overexpression in HCC samples correlated with advanced tumor stage and increased incidence of portal vein tumor thrombus. Moreover, SIRT1 overexpression in HCC facilitates invasion and proliferation and suppresses apoptosis (15, 126, 191). SIRT1 also increased the invasiveness and motility of human HCC cells and was necessary for HCC metastasis in vitro. Importantly, silencing SIRT1 reduced the aforementioned metastatic characteristics of human HCC cells (54). Accelerated cancer invasiveness is associated with increased mitochondrial activity, oxygen consumption, and ATP production (201).
One way in which SIRT1 promotes HCC metastasis is by its interaction with peroxisome proliferator–activated receptor γ coactivator 1α (PGC-1α). PGC-1α is a transcriptional co-activator that promotes mitochondrial biogenesis and respiration (19, 202). PGC-1α expression was demonstrated to enhance the invasion and migration of HCC cells (54). In HCC samples, SIRT1 overexpression was highly correlated with PGC-1α upregulation. Ectopic SIRT1 expression upregulated PGC-1α in HCC cells. Moreover, SIRT1 physically interacts with, deacetylates, and activates PGC-1α. SIRT1-induced PGC-1α increased mitochondrial copy numbers and mass, cellular ATP levels, DNA transcript levels, and mitochondrial biogenesis, which boosted the migration and invasion of HCC, thus promoting cancer dissemination (54, 203).
PGC-1α-induced mitochondrial biogenesis and oxidative phosphorylation were crucial for HCC metastasis (204)SIRT1 knockdown in HCC cells reduced the expression of mitochondria biogenesis-related genes, diminished mitochondrial mass and copy number, intracellular ATP, and mitochondrial DNA transcript levels. These changes led to decreased intracellular ATP production and impaired HCC metastasis (54).
The epithelial-to-mesenchymal transition (EMT) was shown to promote HCC metastasis (205). Through EMT, epithelial cells gain mesenchymal-like characteristics such as reduced intercellular junctions, enhanced invasiveness and motility, chemoresistance, and decreased polarization (206). SIRT1 expression promoted migration and invasion of HCC cell lines and metastasis in an in vivo xenograft mice model by activating EMT (45).
However, other reports suggest that SIRT1 expression was not occasionally activated in human HCC cell lines, suggesting that SIRT1-arbitrated metastasis did not implicate EMT (54). The difference may be attributed to the different HCC models used in the experiments.
Combined, these results suggest that SIRT1 expression promotes progression, metastasis, and invasion of HCC. SIRT1 knockdown decreased migration and invasion of HCC cells in vitro, decreased HCC invasion and metastasis in vivo (45, 54), and impaired mitochondrial function and biogenesis, suggesting that the SIRT1/PGC-1α axis may be a viable therapeutic target for decreasing metastasis (54).
Implications of SIRT1 in the Treatment and Chemoresistance of HCC
HCC is a chemo-refractory cancer (207). Multidrug resistance (MDR) is partly incriminated for HCC metastasis and recurrence. The ATP binding cassette (ABC) transporters are involved in the cellular efflux of chemotherapeutics. ABC overexpression is partly incriminated for HCC MDR (208). The ABC transporters, P-glycoprotein, or multidrug resistance protein 1 (P-gp, MDR1) and multidrug resistance protein 3 (MRP3) are important for HCC chemotherapy (209–211).
SIRT1 expression was shown to stimulate oncogenesis and promote MDR in HCC (40, 212). SIRT1 overexpression upregulates MDR1 in HepG2 cells (213). Contrarywise, silencing FOXO1 or SIRT1 accentuates the cellular uptake of chemotherapeutics and reinstates HCC chemosensitivity. For instance, SIRT1 knockdown in human HCC cells enhanced doxorubicin-induced chemosensitivity and apoptosis (15).
Acetylated p53 promotes tumor suppression by interacting with its downstream targets. SIRT1 deacetylates both p53 and FOXO1, consequently suppressing their ability to induce apoptosis and cell growth arrest (214, 215).
FOXO1's activity is controlled by post-translational interventions, including acetylation, ubiquitination, and phosphorylation. SIRT1 deacetylates FOXO1 and thus promotes its nuclear localization and increases the FOXO-dependent transcription of stress-response genes (216, 217). Specifically, FOXO1 binds to the MDR1 gene promoter and increases MDR1 gene transcription.
Deleted in Liver Cancer-1 (DLC-1) is an established tumor suppressor that has important roles in cell motility and signal transduction pathways. Akt regulates DLC-1 activity by post-translational modifications. SIRT1, by inhibiting the PI3K/Akt pathway, could increase DLC-1 expression and thus promote HCC cell motility (218).
HULC/USP22/SIRT1/Autophagy Pathway
Autophagy is involved in the chemoresistance of cancer cells (219). Cisplatin, sorafenib, and 5-FU can induce autophagy in HCC cells, thus decreasing apoptosis and chemosensitivity (220). Long noncoding RNAs (lncRNAs) are transcripts involved in regulating gene expression. lncRNA HULC (highly upregulated in liver cancer) is involved in HCC chemoresistance and autophagy. In HCC biopsies and cell lines, SIRT1 and HULC are both aberrantly upregulated.
Oxaliplatin and 5-FU upregulated HULC expression in HCC cells. In turn, HULC strongly increased USP22 protein levels and promoted SIRT1 deubiquitylation, consequently decreasing the UPP-mediated degradation of SIRT1 and increased its protein stability. miR-6825-5p, miR-6886-3p, and miR-6845-5p bind to the 3′UTR region of USP22 mRNA and thus decrease its levels. HULC downregulates the abovementioned miRs and strongly upregulates USP22. HULC-induced SIRT1 upregulation enhanced the deacetylation of key autophagy components such as Atg7 and Atg5 and triggered autophagy, which decreased HCC chemosensitivity. In summary, the HULC/USP22/SIRT1 pathway induces protective autophagy and decreases HCC chemosensitivity. In vivo knockdown of HULC or SIRT1 sensitizes HCC to oxaliplatin. Thus, combining chemotherapy with HULC or SIRT1 inhibitors in MDR HCC is a therapeutic option that needs further exploration (see Table 2).
Cambinol and EX-527
EX-527 and cambinol are cytotoxic to and trigger apoptosis in both 2D and 3D HCC cultures (42). Cambinol and EX-527 are SIRT1 inhibitors with antitumor effects that decrease MDR1 mRNA expression in a p53 status- and dose-dependent manner. This suggests that their administration and compatibility with other chemotherapeutics should be adjusted according to the particular genotype of the HCC treated (221).
Cambinol or EX-527 decreased SIRT1 activity and protein levels and thus increased apoptosis, reduced cell migration, and decreased the growth and viability of HCC cell spheroids (60, 126). EX-527 increased the acetyl-p53/p53 ratio in HCC cells and promoted apoptosis. Surprisingly, cambinol decreased the acetyl-p53/p53 radio in Huh7 cells, and increased p53 protein levels. The reason for this discrepancy and the consequences of cambinol on p53 acetylation should be further explored in multiple HCC models. However, both Ex-527 and cambinol decreased FOXO1 expression and increased FOXO1 acetylation, which consequently decreases P-gp in 2D HCC cultures. Whether this reduces chemoresistance should be further explored in HCC spheroids or in vivo models.
SIRT1 downregulation suppresses MRP1 and increases intracellular concentration of Adriamycin in MDRHCC. Downregulating SIRT1 via shRNA decreases MRP3 and MRP1 protein levels. However, the effects of cambinol and Ex-527 on those proteins in HCC have not yet been explored.
Sorafenib
Sorafenib is used in the treatment of advanced HCC. Notably, HCC cells resistant to sorafenib showcased increased MRP3 and P-gp expression. Thus, combining cambinol or EX-527, which downregulates P-gp and MRP3 in HepG2, with conventional chemotherapeutics may offer new treatment options against MDRHCC. Surprisingly, cambinol and EX-527 induced MRP3 and P-gp expression in Huh7 cells. Since HepG2 are p53 WT while Huh7 are p53 mutant, it was suggested that this may at least partially account for the discrepancy.
Therapy response may depend on p53 status, which appears to influence the expression of ABC transporters in MDRHCC. Therefore, the p53 status of each HCC has to be considered when evaluating for susceptibility to chemotherapy. This undermines the importance of characterizing the chemosensitivity of multiple HCC types and personalizing treatment accordingly.
USP22/SIRT1/AKT/MRP1 Signaling Pathway
Ubiquitin-specific protease 22 (USP22) is part of a subfamily of deubiquitinating enzymes and a CSC marker. USP22 is exceedingly expressed in some multidrug resistant human HCC cell lines (MDRHCC) where it decreases sensitivity to 5-fluorouracil (5-FU), doxorubicin, and methotrexate; specifically, it reduced the intracellular concentration of doxorubicin by promoting efflux pump activity and inhibited 5-FU-induced apoptosis. Similarly, SIRT1 expression in MDRHCC decreased intracellular doxorubicin concentrations and promotes resistance to 5-FU.
Inhibiting USP22 in MDRHCC strongly decreased ABCC1 expression [encodes MRP1 (resistance-associated protein 1)] and thus increased intracellular doxorubicin concentrations, but only dimly influenced ABCB1 expression (encodes P-gp). Thus, UPP22 may predominantly induce MDR via MRP1.
USP22 deubiquitinated SIRT1 (222, 223) and thus increased SIRT1 protein levels, which deacetylated and thus activated the PI3K/AKT pathway (224) and consequently increased MRP1s expression (225), which promoted MDR in HCC. However, this mechanistic chain needs to be further validated by other experiments. In 168 HCC biopsies, the protein expressions of MRP1 and USP22 were strongly correlated. Inhibiting the PI3K/AKT pathway in MDRHCC suppressed MRP1's expression and promoted 5-FU-induced apoptosis (226). SIRT1 inhibition increased the sensitivity of MDRHCC to 5-FU and increased intracellular concentration of doxorubicin. Simply put, USP22 may activate the SIRT1–AKT–MRP1 pathway and consequently promote MDR in human HCC cells (226). Future studies should explore the relationship between USP22 and other proteins involved in MDRHCC such as MRP1 and cytochrome P450, which is involved in the hepatic metabolism of xenobiotics.
SIRT1–YAP Signaling Pathway
SIRT1 stimulated the transcription of MKK3 and Yes-associated protein (YAP), which in turn promoted the nuclear localization of p38. SIRT1 activated p38 and consequently promoted HCC development (44). In HCC cells, SIRT1 expression activated YAP2 transcriptional activity and also accentuated the interaction between YAP2 and the transcriptional cofactor TEAD4, and thus promoted the transcription of their downstream targets, which consequently accelerated HCC cell growth and survival. SIRT1 deacetylates YAP2 both in vitro and in vivo, which upregulated the YAP2/TEAD4 axis and promoted HCC cell proliferation (60).
Treatment with cisplatin accentuated the interaction between SIRT1 and YAP2 and promoted the expression of the YAP2 downstream genes. As a response to cisplatin, YAP2 translocates to the nucleus where it is deacetylated by SIRT1, which consequently protects HCC cells from cisplatin-induced apoptosis. Overall, both YAP2 and SIRT1 confer protection against cisplatin (60). Silencing SIRT1 inhibited the nuclear translocation of YAP2 and promoted sensitivity to cisplatin.
Since SIRT1 inhibition induced cytostatic effects in HCC (42), combining it with cytotoxic chemotherapeutics should be considered. Moreover, SIRT1 inhibiting compounds used for the purpose of treating HCC should selectively target tumoral SIRT1 and spare normal hepatocytes in order to preserve liver function.
AMPK–SIRT1–p53 Signaling Pathway
By targeting transcription factors such as p53 and FOXO, SIRT1 suppressed cellular differentiation and senescence and may promote HCC growth (227). Mutual regulation ensues among p53 and SIRT1. SIRT1 deacetylates and inactivates p53 while acetylated p53 downregulates the translation of SIRT1 via miR-34a (142). Silencing SIRT1 in HCC cell cultures increases acetylated p53 and promotes growth arrest (41). This relationship is further validated by nicotinamide (NAM), a SIRT1 inhibitor. In mice treated with NAM, p53 presented increased acetylation, which led to decreased HCC oncogenesis. Importantly, the protective effects of NAM were attributed to the inhibition of SIRT1, not to NAM's antioxidant effect.
In HCC cells, SIRT1 deacetylates p53, thus repressing cellular senescence and apoptosis, and promotes tumorigenesis (63, 228). SIRT1 knockdown in HCC cells increased acetylated p53, decreased proliferative activity activated senescence, and induced a more differentiated cellular state (26, 42). However, the response to silencing SIRT1 may depend on the p53 status of HCC cells. Silencing SIRT1 in p53 WT HepG2 cells increased AMPK phosphorylation, reduced phospho-mTOR, and promoted G1 phase arrest (41, 63). However, silencing SIRT1 in p53 mutant HCC decreased phospho-AMPK and increased mTOR phosphorylation, which stimulated HCC tumorigenesis. Moreover, some reports indicate that inhibiting SIRT1 prevented cell proliferation irrespective of the p53 status of the HCC cells (42). The dynamic between the p53 status of HCC cells and how it affects SIRT1 inhibition is not yet clear.
5′ AMP-activated protein kinase (AMPK) is an enzyme implicated in glucose and fatty acid uptake; its activation stimulates hepatic fatty acid oxidation, ketogenesis, and lipogenesis (229). AMPK is a downstream target of tumor suppressor LKB1. The LKB1–AMPK pathway is vital for the suppression of mTOR signaling. mTOR signaling is overactive in multiple solid tumors and modulates cell proliferation (230). The AMPK-α2 subunit was shown to be strongly downregulated in HCC compared with the corresponding normal hepatic tissue and was correlated with poor patient prognosis.
In HCC cells, AMPK-α2 and SIRT1 are co-localized in the nucleus where they directly interact. AMPK phosphorylates SIRT1 at Thr344 and thus inhibits its deacetylase activity and substrate binding capacity and consequently maintains acetylated p53 levels, which promote apoptosis. Thus, AMPK promotes p53 acetylation and exerts antioncogenic functions in HCC (231).
Metformin
Inducing senescence may be a viable strategy for the chronic management of HCC, since it has fewer negative consequences than therapies that activate apoptosis (232, 233).
Metformin is a drug primarily used for the treatment of type 2 diabetes; it also has antitumoral effects in HCC (127, 234). A low dose of metformin strongly suppressed HCC growth in vivo and in vitro by inducing senescence, inhibiting proliferation, and activating apoptosis (127, 233). Metformin promoted phosphorylation and activated AMPK, which in turn phosphorylated SIRT1 and disabled its enzymatic activity. Consequently, levels of acetylated p53 and p21 were increased ensuing HCC senescence (127). Additionally, AMPK phosphorylation induced by metformin inactivated mTOR in p53 mutant HCC and negatively regulated oncogenesis (63). These results need to be replicated with a larger variety of HCC cell lines (233).
However, both metformin and AMPK modulate NAD+ metabolism and can induce SIRT1 activity (127, 235, 236). Notably, SIRT1 can be phosphorylated at multiple sites that promote different phenotypes. Nevertheless, in HepG2 cells cultured in a high glucose medium, metformin activated both AMPK and SIRT1 and amplified p53 deacetylation contributing to p53 degradation (237). Specifically, metformin primarily targeted AMPK, which activated SIRT1. In this model, both AMPK and SIRT1 were required for metformin-induced p53 degradation (237).
Gallotannin
Gallotannin is a plant-derived compound with anticancer effects (125). Treating HCC cells with gallotannin in vitro resulted in reduced colony formation, amplified cytotoxicity, increased senescence, impaired autophagy, upregulated p21, and promoted cell death. In a mouse xenograft model, gallotannin decreased tumor growth (128). Mechanistically, gallotannin activated AMPK phosphorylation and decreased SIRT1 expression in both in vitro and in vivo HCC models (128).
Everolimus and Ku0063794
The PI3K/AKT/mTOR signaling pathway is involved in the development of multiple malignancies, including HCC (129).
Abnormal mTOR signaling is present in up to 48% of HCC patients and is associated with a meager prognosis. Everolimus is an mTOR complex 1 (mTORC1) inhibitor. However, targeting both mTORC1 and 2 is pivotal for evading drug resistance (129). Administering everolimus with Ku0063794, an mTORC2 inhibitor, produced a potent antioncogenic effect in HCC cells (130). mTOR and SIRT1 both regulate autophagy (130). SIRT1 may promote autophagy via deacetylating transcription factors such as E2F1, FOXO1, histone H4, and p53, which subsequently induce autophagy-related genes (238). Moreover, inhibiting mTOR can also activate autophagy (130, 239).
In HepG2 cells, combining Ku0063794 with everolimus decreased autophagy and inhibited SIRT1 expression, whereas individual monotherapy with either of the compounds did not inhibit SIRT1 and promoted autophagy (130, 240). Blocking autophagy stimulated apoptosis, decreased proliferation, and inhibited SIRT1 expression. This suggests that autophagy may promote survival in HCC cells (130).
Overall, combined use of Ku0063794 and everolimus downregulated autophagy by decreasing SIRT1 and consequently promoted antioncogenic effects in HepG2 cells (130). This experiment should be further validated with other HCC cell lines and by using spheroids or in vivo models. Moreover, testing whether mTORC1/2 inhibitor AZD8055 also inhibits autophagy would further validate the result obtained with Ku0063794 and everolimus in HepG2 cells (130).
In summary, in vivo, ex vivo, and in vitro results with HCC cells confirmed that combined Ku0063794 and everolimus therapy was superior to administering either compound alone as indicated by their increased reduction of cell invasion, migration, proliferation, and increased EMT inhibition (240). EMT inhibition was partly produced by decreased SIRT1 levels. Thus, combining everolimus with the mTORC1/2 inhibitor Ku0063794 provides potent anticancer effects (240).
tNOX-SIRT1-p53
First-line HCC treatment compounds such as doxorubicin are associated with systemic toxicity, inefficacy, and chemoresistance (10, 11). Recently, new anti-cancer compounds with high antiproliferative activity against chemoresistant cells have been developed (241, 242). The human tNOX gene encodes for a protein that is expressed in multiple solid malignancies where it is crucial for cellular migration and proliferation. tNOX converts reduced NADH to oxidized NAD. Importantly, tNOX inhibition reduces intracellular NAD concentration, which influences SIRT1 function (241, 243, 244). Suppression of tNOX by a multitude of agents activated apoptosis and diminished malignant cell growth (244, 245).
Two 2-unsubstituted 4,11-diaminoanthra[2,3-b]furan-5,10-dione derivatives promoted apoptosis in human HCC cells in a tNOX-dependent manner. In p53 WT HCC cells, these anti-cancer compounds bound and downregulated tNOX, which decreased intracellular NAD, and consequently suppressed SIRT1 activity. Decreased SIRT1 activity led to increased p53 acetylation and activation, which upregulated its downstream target, pro-apoptotic Bak and thus increased apoptosis (246). Augmented p53 acetylation promoted by SIRT1 inhibition was also associated with activation of PUMA, which upregulates Bak and prompts apoptosis (246, 247).
tNOX reduction reestablished non-cancer phenotypes, such as decreased migration, and amplified sensitivity to stress-induced apoptosis. Accumulating evidence suggests that suppressing tNOX may improve patient prognosis (246, 248–250).
c-Myc was demonstrated to be an HCC initiating oncogene (251). C-Myc expression is associated with HCC progression and poor patient prognosis (26, 252). In HCC cells, SIRT1 induced the expression of oncogenic c-Myc, which in turn increased β-catenin mRNA and protein expression and amplified the transcription and expression of the β-catenin target genes survinin and cyclin D1. Therefore, SIRT1 overexpression promoted oncogenesis via c-Myc activation (39, 246) and protected against p53 induced apoptosis (26).
c-Myc was shown to increase SIRT1 through transcriptional and post-transcriptional regulation. The SIRT1-c-Myc axis impacts cellular growth; however, the result of this interaction is still controversial (253–255).
Concluding Remarks
HCC accounts for immense mortality rates worldwide and poses difficult therapeutic problems. The therapies used today are inefficient at managing late-stage disease or metastasis. A better understanding of the underlying mechanisms that promote HCC development, metastasis, and chemoresistance may enable the development of more efficient therapeutic protocols. SIRT1 mediates LCSCs stemness, HCC metastasis, and chemoresistance. Targeting SIRT1 either to hinder the progression and metastasis of HCC or to decrease LCSCs stemness may be a viable therapeutic option. Directly inhibiting SIRT1 via miRs, exogenous compounds, or combining conventional chemotherapeutics with tumor-selective SIRT1 inhibitors may improve treatment outcomes. However, a better understanding of the biology of SIRT1 in HCC is needed in order to efficiently inhibit related pathways and constrain HCC development.
Author Contributions
IB-N and CC designed the study and approved the final version of the manuscript. MF, A-AG, and DG wrote the article, while CI and AI selected the most relevant articles to be included in the paper. The figures were created by MF and DG and tables were made by A-AG.
Funding
This work was supported by Project PNCDI III 2015-2020 entitled Increasing the performance of scientific research and technology transfer in translational medicine through the formation of a new generation of young researchers—ECHITAS, no. 29PFE/18.10.2018, Competitivity Operational Program, 2014–2020, entitled Clinical and economic impact of personalized targeted anti-miR therapies in reconverting lung cancer chemoresistance—CANTEMIR, and project PN-III-P1-1.2-PCCDI-2017-0737, contract number 35PCCDI/2018—Genomic mapping of population from polluted area with radioactivity and heavy metals to increase national security.
Conflict of Interest
The authors declare that the research was conducted in the absence of any commercial or financial relationships that could be construed as a potential conflict of interest.
References
1. Altekruse SF, McGlynn KA, Reichman ME. Hepatocellular carcinoma incidence, mortality, and survival trends in the United States from 1975 to 2005. J Clin Oncol. (2009) 27:1485–91. doi: 10.1200/JCO.2008.20.7753
2. El-Serag HB. Epidemiology of viral hepatitis and hepatocellular carcinoma. Gastroenterology. (2012) 142:1264–73.e1. doi: 10.1053/j.gastro.2011.12.061
3. Gomaa A-I, Khan S-A, Toledano M-B, Waked I, Taylor-Robinson S-D. Hepatocellular carcinoma: epidemiology, risk factors and pathogenesis. World J Gastroenterol. (2008) 14:4300–8. doi: 10.3748/wjg.14.4300
4. White DL, Kanwal F, El-Serag HB. Association between nonalcoholic fatty liver disease and risk for hepatocellular cancer, based on systematic review. Clin Gastroenterol Hepatol. (2012) 10:1342–59.e2. doi: 10.1016/j.cgh.2012.10.001
5. Regimbeau JM, Colombat M, Mognol P, Durand F, Abdalla E, Degott C, et al. Obesity and diabetes as a risk factor for hepatocellular carcinoma. Liver Transplant. (2004) 10(2 Suppl. 1):S69–73. doi: 10.1002/lt.20033
6. Cohen JC, Horton JD, Hobbs HH. Human fatty liver disease: old questions and new insights. Science. (2011) 332:1519–23. doi: 10.1126/science.1204265
7. Baffy G, Brunt EM, Caldwell SH. Hepatocellular carcinoma in non-alcoholic fatty liver disease: an emerging menace. J Hepatol. (2012) 56:1384–91. doi: 10.1016/j.jhep.2011.10.027
8. Varela M, Sala M, Llovet JM, Bruix J. Treatment of hepatocellular carcinoma: is there an optimal strategy? Cancer Treat Rev. (2003) 29:99–104. doi: 10.1016/S0305-7372(02)00123-8
9. Yang S-F, Chang C-W, Wei R-J, Shiue Y-L, Wang S-N, Yeh Y-T. Involvement of DNA damage response pathways in hepatocellular carcinoma. BioMed Res Int. (2014) 2014:153867. doi: 10.1155/2014/153867
10. Ganne-Carrié N, Trinchet J-C. Systemic treatment of hepatocellular carcinoma. Eur J Gastroenterol Hepatol. (2004) 16:275–81. doi: 10.1097/00042737-200403000-00005
11. Cox J, Weinman S. Mechanisms of doxorubicin resistance in hepatocellular carcinoma. Hepatic Oncol. (2016) 3:57–9. doi: 10.2217/hep.15.41
12. El-Serag HB, Marrero JA, Rudolph L, Reddy KR. Diagnosis and treatment of hepatocellular carcinoma. Gastroenterology. (2008) 134:1752–63. doi: 10.1053/j.gastro.2008.02.090
13. Longo VD, Kennedy BK. Sirtuins in aging and age-related disease. Cell. (2006) 126:257–68. doi: 10.1016/j.cell.2006.07.002
14. Michan S, Sinclair D. Sirtuins in mammals: insights into their biological function. Biochem J. (2007) 404:1–13. doi: 10.1042/BJ20070140
15. Chen J, Zhang B, Wong N, Lo AWI, To K-F, Chan AWH, et al. Sirtuin 1 is upregulated in a subset of hepatocellular carcinomas where it is essential for telomere maintenance and tumor cell growth. Cancer Res. (2011) 71:4138–49. doi: 10.1158/0008-5472.CAN-10-4274
16. Haigis MC, Guarente LP. Mammalian sirtuins–emerging roles in physiology, aging, and calorie restriction. Genes Dev. (2006) 20:2913–21. doi: 10.1101/gad.1467506
17. Ji Yu E, Kim S-H, Heo K, Ou C-Y, Stallcup MR, Kim JH. Reciprocal roles of DBC1 and SIRT1 in regulating estrogen receptor α activity and co-activator synergy. Nucleic Acids Res. (2011) 39:6932–43. doi: 10.1093/nar/gkr347
18. Han L, Zhou R, Niu J, McNutt MA, Wang P, Tong T. SIRT1 is regulated by a PPARγ-SIRT1 negative feedback loop associated with senescence. Nucleic Acids Res. (2010) 38:7458–71. doi: 10.1093/nar/gkq609
19. Rodgers JT, Lerin C, Haas W, Gygi SP, Spiegelman BM, Puigserver P. Nutrient control of glucose homeostasis through a complex of PGC-1alpha and SIRT1. Nature. (2005) 434:113–8. doi: 10.1038/nature03354
20. Fu M, Liu M, Sauve AA, Jiao X, Zhang X, Wu X, et al. Hormonal control of androgen receptor function through SIRT1. Mol Cell Biol. (2006) 26:8122–35. doi: 10.1128/MCB.00289-06
21. Motta MC, Divecha N, Lemieux M, Kamel C, Chen D, Gu W, et al. Mammalian SIRT1 represses forkhead transcription factors. Cell. (2004) 116:551–63. doi: 10.1016/S0092-8674(04)00126-6
22. Vaziri H, Dessain SK, Eaton EN, Imai SI, Frye RA, Pandita TK, et al. hSIR2SIRT1functions as an NAD-dependent p53 deacetylase. Cell. (2001) 107:149–59. doi: 10.1016/S0092-8674(01)00527-X
23. Yeung F, Hoberg JE, Ramsey CS, Keller MD, Jones DR, Frye RA, et al. Modulation of NF-kappaB-dependent transcription and cell survival by the SIRT1 deacetylase. EMBO J. (2004) 23:2369–80. doi: 10.1038/sj.emboj.7600244
24. Wang R-H, Zheng Y, Kim H-S, Xu X, Cao L, Luhasen T, et al. Interplay among BRCA1, SIRT1, and Survivin during BRCA1-associated tumorigenesis. Mol Cell. (2008) 32:11–20. doi: 10.1016/j.molcel.2008.09.011
25. Firestein R, Blander G, Michan S, Oberdoerffer P, Ogino S, Campbell J, et al. The SIRT1 deacetylase suppresses intestinal tumorigenesis and colon cancer growth. PLoS ONE. (2008) 3:e2020. doi: 10.1371/journal.pone.0002020
26. Jang KY, Noh SJ, Lehwald N, Tao G-Z, Bellovin DI, Park HS, et al. SIRT1 and c-Myc promote liver tumor cell survival and predict poor survival of human hepatocellular carcinomas. PLoS ONE. (2012) 7:e45119. doi: 10.1371/journal.pone.0045119
27. Laemmle A, Lechleiter A, Roh V, Schwarz C, Portmann S, Furer C, et al. Inhibition of SIRT1 impairs the accumulation and transcriptional activity of HIF-1α protein under hypoxic conditions. PLoS ONE. (2012) 7:e33433. doi: 10.1371/journal.pone.0033433
28. Boily G, Seifert EL, Bevilacqua L, He XH, Sabourin G, Estey C, et al. SirT1 regulates energy metabolism and response to caloric restriction in mice. PLoS ONE. (2008) 3:e1759. doi: 10.1371/journal.pone.0001759
29. Purushotham A, Schug TT, Xu Q, Surapureddi S, Guo X, Li X. Hepatocyte-specific Deletion of SIRT1 alters fatty acid metabolism and results in hepatic steatosis and inflammation. Cell Metab. (2009) 9:327–38. doi: 10.1016/j.cmet.2009.02.006
30. Li K, Lv G, Pan L. Sirt1 alleviates LPS induced inflammation of periodontal ligament fibroblasts via downregulation of TLR4. Int J Biol Macromol. (2018) 119:249–54. doi: 10.1016/j.ijbiomac.2018.07.099
31. Yang S-R, Wright J, Bauter M, Seweryniak K, Kode A, Rahman I. Sirtuin regulates cigarette smoke-induced proinflammatory mediator release via RelA/p65 NF-kappaB in macrophages in vitro and in rat lungs in vivo: implications for chronic inflammation and aging. Am J Physiol Lung Cell Mol Physiol. (2007) 292:L567–76. doi: 10.1152/ajplung.00308.2006
32. Li X. SIRT1 and energy metabolism. Acta Biochim Biophys Sin. (2013) 45:51–60. doi: 10.1093/abbs/gms108
33. Schug TT, Li X. Sirtuin 1 in lipid metabolism and obesity. Ann Med. (2011) 43:198–211. doi: 10.3109/07853890.2010.547211
34. Bradbury CA, Khanim FL, Hayden R, Bunce CM, White DA, Drayson MT, et al. Histone deacetylases in acute myeloid leukaemia show a distinctive pattern of expression that changes selectively in response to deacetylase inhibitors. Leukemia. (2005) 19:1751–9. doi: 10.1038/sj.leu.2403910
35. Stünkel W, Peh BK, Tan YC, Nayagam VM, Wang X, Salto-Tellez M, et al. Function of the SIRTI protein deacetylase in cancer. Biotechnol J. (2007) 2:1360–8. doi: 10.1002/biot.200700087
36. Huffman DM, Grizzle WE, Bamman MM, Kim JS, Eltoum IA, Elgavish A, et al. SIRT1 is significantly elevated in mouse and human prostate cancer. Cancer Res. (2007) 67:6612–8. doi: 10.1158/0008-5472.CAN-07-0085
37. Hida Y, Kubo Y, Murao K, Arase S. Strong expression of a longevity-related protein, SIRT1, in Bowen's disease. Arch Dermatol Res. (2007) 299:103–6. doi: 10.1007/s00403-006-0725-6
38. Wang R, Sengupta K, Li C, Kim H, Cao L, Kim S, et al. Impaired DNA damage response, genome instability, and tumorigenesis in SIRT1 mutant mice. Cancer Cell. (2008) 14:312–23. doi: 10.1016/j.ccr.2008.09.001
39. Liu T, Liu PY, Marshall GM. The critical role of the class III histone deacetylase SIRT1 in cancer. Cancer Res. (2009) 69:1702–5. doi: 10.1158/0008-5472.CAN-08-3365
40. Chen H-C, Jeng Y-M, Yuan R-H, Hsu H-C, Chen Y-L. SIRT1 promotes tumorigenesis and resistance to chemotherapy in hepatocellular carcinoma and its expression predicts poor prognosis. Ann Surg Oncol. (2012) 19:2011–9. doi: 10.1245/s10434-011-2159-4
41. Choi HN, Bae JS, Jamiyandorj U, Noh SJ, Park HS, Jang KY, et al. Expression and role of SIRT1 in hepatocellular carcinoma. Oncol Rep. (2011) 26:503–10. doi: 10.3892/or.2011.1301
42. Portmann S, Fahrner R, Lechleiter A, Keogh A, Overney S, Laemmle A, et al. Antitumor effect of SIRT1 inhibition in human HCC tumor models in vitro and in vivo. Mol Cancer Ther. (2013) 12:499–508. doi: 10.1158/1535-7163.MCT-12-0700
43. Yan X, Yan X, Liu X, Liu X, Wang Z, Wang Z, et al. MicroRNA-486-5p functions as a tumor suppressor of proliferation and cancer stem-like cell properties by targeting Sirt1 in liver cancer. Oncol Rep. (2019) 41:1938–48. doi: 10.3892/or.2018.6930
44. Wang Y, Cui R, Zhang X, Qiao Y, Liu X, Chang Y, et al. SIRT1 increases YAP- and MKK3-dependent p38 phosphorylation in mouse liver and human hepatocellular carcinoma. Oncotarget. (2016) 7:11284–98. doi: 10.18632/oncotarget.7022
45. Hao C, Zhu P-X, Yang X, Han Z-P, Jiang J-H, Zong C, et al. Overexpression of SIRT1 promotes metastasis through epithelial-mesenchymal transition in hepatocellular carcinoma. BMC Cancer. (2014) 14:978. doi: 10.1186/1471-2407-14-978
46. Cheng J, Liu C, Liu L, Chen X, Shan J, Shen J, et al. MEK1 signaling promotes self-renewal and tumorigenicity of liver cancer stem cells via maintaining SIRT1 protein stabilization. Oncotarget. (2016) 7:20597–611. doi: 10.18632/oncotarget.7972
47. Tian Z, Jiang H, Liu Y, Huang Y, Xiong X, Wu H, et al. MicroRNA-133b inhibits hepatocellular carcinoma cell progression by targeting Sirt1. Exp Cell Res. (2016) 343:135–47. doi: 10.1016/j.yexcr.2016.03.027
48. Bordone L, Cohen D, Robinson A, Motta MC, van Veen E, Czopik A, et al. SIRT1 transgenic mice show phenotypes resembling calorie restriction. Aging Cell. (2007) 6:759–67. doi: 10.1111/j.1474-9726.2007.00335.x
49. Fang Y, Nicholl MB. Sirtuin 1 in malignant transformation: friend or foe? Cancer Lett. (2011) 306:10–4. doi: 10.1016/j.canlet.2011.02.019
50. Song S, Luo M, Song Y, Liu T, Zhang H, Xie Z. Prognostic role of SIRT1 in hepatocellular carcinoma. J Coll Phys Surg Pak. (2014) 24:849–54.
51. Herranz D, Muñoz-Martin M, Cañamero M, Mulero F, Martinez-Pastor B, Fernandez-Capetillo O, et al. Sirt1 improves healthy ageing and protects from metabolic syndrome-associated cancer. Nat Commun. (2010) 1:3. doi: 10.1038/ncomms1001
52. Tanos B, Rodriguez-Boulan E. The epithelial polarity program: machineries involved and their hijacking by cancer. Oncogene. (2008) 27:6939–57. doi: 10.1038/onc.2008.345
53. Huang J, Chen K, Gong W, Zhou Y, Le Y, Bian X, et al. Receptor “hijacking” by malignant glioma cells: a tactic for tumor progression. Cancer Lett. (2008) 267:254–61. doi: 10.1016/j.canlet.2008.03.014
54. Li Y, Xu S, Li J, Zheng L, Feng M, Wang X, et al. SIRT1 facilitates hepatocellular carcinoma metastasis by promoting PGC-1α-mediated mitochondrial biogenesis. Oncotarget. (2016) 7:29255–74. doi: 10.18632/oncotarget.8711
55. Jiang H, Zhang X, Tao Y, Shan L, Jiang Q, Yu Y, et al. Prognostic and clinicopathologic significance of SIRT1 expression in hepatocellular carcinoma. Oncotarget. (2016) 8:52357–65. doi: 10.18632/oncotarget.14096
56. Yang H, Bi Y, Xue L, Wang J, Lu Y, Zhang Z, et al. Multifaceted Modulation of SIRT1 in Cancer and Inflammation. Crit Rev Oncog. (2015) 20:49–64. doi: 10.1615/CritRevOncog.2014012374
57. Kume S, Haneda M, Kanasaki K, Sugimoto T, Araki S, Isshiki K, et al. SIRT1 inhibits transforming growth factor beta-induced apoptosis in glomerular mesangial cells via Smad7 deacetylation. J Biol Chem. (2007) 282:151–8. doi: 10.1074/jbc.M605904200
58. Song YM, Lee Y, Kim J-W, Ham D-S, Kang E-S, Cha BS, et al. Metformin alleviates hepatosteatosis by restoring SIRT1-mediated autophagy induction via an AMP-activated protein kinase-independent pathway. Autophagy. (2015) 11:46–59. doi: 10.4161/15548627.2014.984271
59. Wang Y, Jiang Y, Fan X, Tan H, Zeng H, Wang Y, et al. Hepato-protective effect of resveratrol against acetaminophen-induced liver injury is associated with inhibition of CYP-mediated bioactivation and regulation of SIRT1-p53 signaling pathways. Toxicol Lett. (2015) 236:82–9. doi: 10.1016/j.toxlet.2015.05.001
60. Mao B, Hu F, Cheng J, Wang P, Xu M, Yuan F, et al. SIRT1 regulates YAP2-mediated cell proliferation and chemoresistance in hepatocellular carcinoma. Oncogene. (2014) 33:1468–74. doi: 10.1038/onc.2013.88
61. Psyrri A, Arkadopoulos N, Vassilakopoulou M, Smyrniotis V, Dimitriadis G. Pathways and targets in hepatocellular carcinoma. Expert Rev Anticancer Ther. (2012) 12:1347–57. doi: 10.1586/era.12.113
62. Wysocki PJ. Targeted therapy of hepatocellular cancer. Expert Opin Investig Drugs. (2010) 19:265–74. doi: 10.1517/13543780903514110
63. Zhang ZY, Hong D, Nam SH, Kim JM, Paik YH, Joh JW, et al. SIRT1 regulates oncogenesis via a mutant p53-dependent pathway in hepatocellular carcinoma. J Hepatol. (2015) 62:121–30. doi: 10.1016/j.jhep.2014.08.007
64. Saklayen MG. The global epidemic of the metabolic syndrome. Curr Hypertens Rep. (2018) 20:12. doi: 10.1007/s11906-018-0812-z
65. Karagozian R, Derdák Z, Baffy G. Obesity-associated mechanisms of hepatocarcinogenesis. Metabolism. (2014) 63:607–17. doi: 10.1016/j.metabol.2014.01.011
66. Powell EE, Jonsson JR, Clouston AD. Steatosis: co-factor in other liver diseases. Hepatology. (2005) 42:5–13. doi: 10.1002/hep.20750
67. Watanabe S, Yaginuma R, Ikejima K, Miyazaki A. Liver diseases and metabolic syndrome. J Gastroenterol. (2008) 43:509–18. doi: 10.1007/s00535-008-2193-6
68. Cholankeril G, Patel R, Khurana S, Satapathy SK. Hepatocellular carcinoma in non-alcoholic steatohepatitis: current knowledge and implications for management. World J Hepatol. (2017) 9:533–43. doi: 10.4254/wjh.v9.i11.533
69. Pfluger PT, Herranz D, Velasco-Miguel S, Serrano M, Tschop MH. Sirt1 protects against high-fat diet-induced metabolic damage. Proc Natl Acad Sci USA. (2008) 105:9793–8. doi: 10.1073/pnas.0802917105
70. Escande C, Chini CCS, Nin V, Dykhouse KM, Novak CM, Levine J, et al. Deleted in breast cancer – 1 regulates SIRT1 activity and contributes to high-fat diet – induced liver steatosis in mice. J Clin Invest. (2010) 120:545–558. doi: 10.1172/JCI39319
71. Gilbert RE, Thai K, Advani SL, Cummins CL, Kepecs DM, Schroer SA, et al. SIRT1 activation ameliorates hyperglycaemia by inducing a torpor-like state in an obese mouse model of type 2 diabetes. Diabetologia. (2015) 58:819–27. doi: 10.1007/s00125-014-3485-4
72. Kitada M, Kume S, Kanasaki K, Takeda-Watanabe A, Koya D. Sirtuins as possible drug targets in type 2 diabetes. Curr Drug Targets. (2013) 14:622–36. doi: 10.2174/1389450111314060002
73. Banks AS, Kon N, Knight C, Matsumoto M, Gutiérrez-Juárez R, Rossetti L, et al. SirT1 gain of function increases energy efficiency and prevents diabetes in mice. Cell Metab. (2008) 8:333–41. doi: 10.1016/j.cmet.2008.08.014
74. Polakis P. The many ways of Wnt in cancer. Curr Opin Genet Dev. (2007) 17:45–51. doi: 10.1016/j.gde.2006.12.007
75. Reya T, Morrison SJ, Clarke MF, Weissman IL. Stem cells, cancer, and cancer stem cells. Nature. (2001) 414:105–11. doi: 10.1038/35102167
76. Visvader JE, Lindeman GJ. Cancer stem cells: current status and evolving complexities. Cell Stem Cell. (2012) 10:717–28. doi: 10.1016/j.stem.2012.05.007
77. Muthukrishnan SD, Alvarado AG, Kornblum HI. Building bonds: cancer stem cells depend on their progeny to drive tumor progression. Cell Stem Cell. (2018) 22:473–4. doi: 10.1016/j.stem.2018.03.008
79. Easwaran H, Tsai H-C, Baylin SB. Cancer epigenetics: tumor heterogeneity, plasticity of stem-like states, and drug resistance. Mol Cell. (2014) 54:716–27. doi: 10.1016/j.molcel.2014.05.015
80. Zheng H, Pomyen Y, Hernandez MO, Li C, Livak F, Tang W, et al. Single-cell analysis reveals cancer stem cell heterogeneity in hepatocellular carcinoma. Hepatology. (2018) 68:127–40. doi: 10.1002/hep.29778
81. Zhang W, Mu D, Feng K. Hierarchical potential differentiation of liver cancer stem cells. Adv Clin Exp Med. (2017) 26:1137–41. doi: 10.1007/978-981-10-5562-1
82. Yagci T, Cetin M, Ercin PB. Cancer stem cells in hepatocellular carcinoma. J Gastrointest Cancer. (2017) 48:241–5. doi: 10.1007/s12029-017-9960-7
83. Magee JA, Piskounova E, Morrison SJ. Cancer stem cells: impact, heterogeneity, and uncertainty. Cancer Cell. (2012) 21:283–96. doi: 10.1016/j.ccr.2012.03.003
84. Nio K, Yamashita T, Kaneko S. The evolving concept of liver cancer stem cells. Mol Cancer. (2017) 16:4. doi: 10.1186/s12943-016-0572-9
85. Lv H, Lv G, Han Q, Yang W, Wang H. Noncoding RNAs in liver cancer stem cells: the big impact of little things. Cancer Lett. (2018) 418:51–63. doi: 10.1016/j.canlet.2018.01.001
86. Flores-Téllez TN, Villa-Treviño S, Piña-Vázquez C. Road to stemness in hepatocellular carcinoma. World J Gastroenterol. (2017) 23:6750–76. doi: 10.3748/wjg.v23.i37.6750
87. Spelt L, Sasor A, Ansari D, Hilmersson KS, Andersson R. The prognostic role of cancer stem cell markers for long-term outcome after resection of colonic liver metastases. Anticancer Res. (2018) 38:313–20. doi: 10.21873/anticanres.12224
88. Liu C, Liu L, Chen X, Cheng J, Zhang H, Shen J, et al. Sox9 regulates self-renewal and tumorigenicity by promoting symmetrical cell division of cancer stem cells in hepatocellular carcinoma. Hepatol Baltim Md. (2016) 64:117–29. doi: 10.1002/hep.28509
89. Yang ZF, Ho DW, Ng MN, Lau CK, Yu WC, Ngai P, et al. Significance of CD90+ cancer stem cells in human liver cancer. Cancer Cell. (2008) 13:153–66. doi: 10.1016/j.ccr.2008.01.013
90. Zhang L, Theise N, Chua M, Reid LM. The stem cell niche of human livers: symmetry between development and regeneration. Hepatol Baltim Md. (2008) 48:1598–607. doi: 10.1002/hep.22516
91. Tang Y, Kitisin K, Jogunoori W, Li C, Deng C-X, Mueller SC, et al. Progenitor/stem cells give rise to liver cancer due to aberrant TGF-beta and IL-6 signaling. Proc Natl Acad Sci USA. (2008) 105:2445–50. doi: 10.1073/pnas.0705395105
92. Chen Y, Wong PP, Sjeklocha L, Steer CJ, Sahin MB. Mature hepatocytes exhibit unexpected plasticity by direct dedifferentiation into liver progenitor cells in culture. Hepatol Baltim Md. (2012) 55:563–74. doi: 10.1002/hep.24712
93. Zhang X-Y, Varthi M, Sykes SM, Phillips C, Warzecha C, Zhu W, et al. The putative cancer stem cell marker USP22 is a subunit of the human SAGA complex required for activated transcription and cell-cycle progression. Mol Cell. (2008) 29:102–11. doi: 10.1016/j.molcel.2007.12.015
94. Han M-K, Song E-K, Guo Y, Ou X, Mantel C, Broxmeyer HE. SIRT1 regulates apoptosis and Nanog expression in mouse embryonic stem cells by controlling p53 subcellular localization. Cell Stem Cell. (2008) 2:241–51. doi: 10.1016/j.stem.2008.01.002
95. Ou X, Chae H-D, Wang R-H, Shelley WC, Cooper S, Taylor T, et al. SIRT1 deficiency compromises mouse embryonic stem cell hematopoietic differentiation, and embryonic and adult hematopoiesis in the mouse. Blood. (2011) 117:440–50. doi: 10.1182/blood-2010-03-273011
96. DeBerardinis RJ, Chandel NS. Fundamentals of cancer metabolism. Sci Adv. (2016) 2:e1600200. doi: 10.1126/sciadv.1600200
97. Houtkooper RH, Mouchiroud L, Ryu D, Moullan N, Katsyuba E, Knott G, et al. Mitonuclear protein imbalance as a conserved longevity mechanism. Nature. (2013) 497:451–7. doi: 10.1038/nature12188
98. Kim H-J, Maiti P, Barrientos A. Mitochondrial ribosomes in cancer. Semin Cancer Biol. (2017) 47:67–81. doi: 10.1016/j.semcancer.2017.04.004
99. Cao X, Li C, Xiao S, Tang Y, Huang J, Zhao S, et al. Acetylation promotes TyrRS nuclear translocation to prevent oxidative damage. Proc Natl Acad Sci USA. (2017) 114:687–92. doi: 10.1073/pnas.1608488114
100. Liu L, Liu C, Zhang Q, Shen J, Zhang H, Shan J, et al. SIRT1-mediated transcriptional regulation of SOX2 is important for self-renewal of liver cancer stem cells. Hepatol Baltim Md. (2016) 64:814–27. doi: 10.1002/hep.28690
101. Wei Z, Jia J, Heng G, Xu H, Shan J, Wang G, et al. SIRT1/MRPS5 axis enhances the metabolic flexibility of liver cancer stem cells. Hepatology. (2019). doi: 10.1002/hep.30622. [Epub ahead of print].
102. Choudhary C, Weinert BT, Nishida Y, Verdin E, Mann M. The growing landscape of lysine acetylation links metabolism and cell signalling. Nat Rev Mol Cell Biol. (2014) 15:536–50. doi: 10.1038/nrm3841
103. Mouchiroud L, Houtkooper RH, Moullan N, Katsyuba E, Ryu D, Cantó C, et al. The NAD(+)/Sirtuin pathway modulates longevity through activation of mitochondrial UPR and FOXO signaling. Cell. (2013) 154:430–41. doi: 10.1016/j.cell.2013.06.016
104. Berdichevsky A, Viswanathan M, Horvitz HR, Guarente L. C. elegans SIR-2.1 interacts with 14-3-3 proteins to activate DAF-16 and extend life span. Cell. (2006) 125:1165–77. doi: 10.1016/j.cell.2006.04.036
105. Li L, Wang L, Li L, Wang Z, Ho Y, McDonald T, et al. Activation of p53 by SIRT1 inhibition enhances elimination of CML leukemia stem cells in combination with imatinib. Cancer Cell. (2012) 21:266–81. doi: 10.1016/j.ccr.2011.12.020
106. Liu C, Liu L, Shan J, Shen J, Xu Y, Zhang Q, et al. Histone deacetylase 3 participates in self-renewal of liver cancer stem cells through histone modification. Cancer Lett. (2013) 339:60–9. doi: 10.1016/j.canlet.2013.07.022
107. O'Hagan HM, Wang W, Sen S, Destefano Shields C, Lee SS, Zhang YW, et al. Oxidative damage targets complexes containing DNA methyltransferases, SIRT1, and polycomb members to promoter CpG Islands. Cancer Cell. (2011) 20:606–19. doi: 10.1016/j.ccr.2011.09.012
108. Ford J, Ahmed S, Allison S, Jiang M, Milner J. JNK2-dependent regulation of SIRT1 protein stability. Cell Cycle Georget Tex. (2008) 7:3091–7. doi: 10.4161/cc.7.19.6799
109. Gao Z, Zhang J, Kheterpal I, Kennedy N, Davis RJ, Ye J. Sirtuin 1 (SIRT1) protein degradation in response to persistent c-Jun N-terminal Kinase 1 (JNK1) activation contributes to hepatic steatosis in obesity. J Biol Chem. (2011) 286:22227–34. doi: 10.1074/jbc.M111.228874
110. Mu X, Español-Suñer R, Mederacke I, Affò S, Manco R, Sempoux C, et al. Hepatocellular carcinoma originates from hepatocytes and not from the progenitor/biliary compartment. J Clin Invest. (2015) 125:3891–903. doi: 10.1172/JCI77995
111. Guo Z, Jiang J-H, Zhang J, Yang H-J, Zhong Y-P, Su J, et al. Side population in hepatocellular carcinoma HCCLM3 cells is enriched with stem-like cancer cells. Oncol Lett. (2016) 11:3145–51. doi: 10.3892/ol.2016.4343
112. Xia H, Cao J, Li Q, Lv Y, Jia W, Ren W, et al. Hepatocellular carcinoma-propagating cells are detectable by side population analysis and possess an expression profile reflective of a primitive origin. Sci Rep. (2016) 6:34856. doi: 10.1038/srep34856
113. Chen B, Hui Y, Zhang H, Fan L, Si M, Yang G. [Expression of CD90/EpCAM/CD24 in hepatocellular carcinoma cell lines at various stages of differentiation]. Zhonghua Gan Zang Bing Za Zhi Zhonghua Ganzangbing Zazhi Chin J Hepatol. (2013) 21:688–91. doi: 10.3760/cma.j.issn.1007-3418.2013.09.010
114. Hadjimichael C, Chanoumidou K, Papadopoulou N, Arampatzi P, Papamatheakis J, Kretsovali A. Common stemness regulators of embryonic and cancer stem cells. World J Stem Cells. (2015) 7:1150–84. doi: 10.4252/wjsc.v7.i9.1150
115. Tomuleasa C, Soritau O, Rus-Ciuca D, Pop T, Todea D, Mosteanu O, et al. Isolation and characterization of hepatic cancer cells with stem-like properties from hepatocellular carcinoma. J Gastrointest Liver Dis. (2010) 19:61–7.
116. Adamo A, Barrero MJ, Izpisua Belmonte JC. LSD1 and pluripotency: a new player in the network. Cell Cycle Georget Tex. (2011) 10:3215–6. doi: 10.4161/cc.10.19.17052
117. Whyte WA, Bilodeau S, Orlando DA, Hoke HA, Frampton GM, Foster CT, et al. Enhancer decommissioning by LSD1 during embryonic stem cell differentiation. Nature. (2012) 482:221–5. doi: 10.1038/nature10805
118. Thambyrajah R, Mazan M, Patel R, Moignard V, Stefanska M, Marinopoulou E, et al. GFI1 proteins orchestrate the emergence of haematopoietic stem cells through recruitment of LSD1. Nat Cell Biol. (2016) 18:21–32. doi: 10.1038/ncb3276
119. Liu C, Liu L, Chen X, Cheng J, Zhang H, Zhang C, et al. LSD1 Stimulates Cancer-associated fibroblasts to drive Notch3-dependent self-renewal of liver cancer stem–like cells. Cancer Res. (2018) 78:938–49. doi: 10.1158/0008-5472.CAN-17-1236
120. Bouras T, Pal B, Vaillant F, Harburg G, Asselin-Labat M-L, Oakes SR, et al. Notch signaling regulates mammary stem cell function and luminal cell-fate commitment. Cell Stem Cell. (2008) 3:429–41. doi: 10.1016/j.stem.2008.08.001
121. Tschaharganeh DF, Chen X, Latzko P, Malz M, Gaida MM, Felix K, et al. Yes-associated protein up-regulates Jagged-1 and activates the Notch pathway in human hepatocellular carcinoma. Gastroenterology. (2013) 144:1530–42.e12. doi: 10.1053/j.gastro.2013.02.009
122. Wang R, Sun Q, Wang P, Liu M, Xiong S, Luo J, et al. Notch and Wnt/β-catenin signaling pathway play important roles in activating liver cancer stem cells. Oncotarget. (2016) 7:5754. doi: 10.18632/oncotarget.6805
123. Wu CX, Xu A, Zhang CC, Olson P, Chen L, Lee TK, et al. Notch inhibitor PF-03084014 inhibits hepatocellular carcinoma growth and metastasis via suppression of cancer stemness due to reduced activation of Notch1–Stat3. Mol Cancer Ther. (2017) 16:1531–43. doi: 10.1158/1535-7163.MCT-17-0001
124. Xu M, Fang S, Song J, Chen M, Zhang Q, Weng Q, et al. CPEB1 mediates hepatocellular carcinoma cancer stemness and chemoresistance. Cell Death Dis. (2018) 9:957. doi: 10.1038/s41419-018-0974-2
125. Park E, Kwon HY, Jung JH, Jung D-B, Jeong A, Cheon J, et al. Inhibition of myeloid cell leukemia 1 and activation of caspases are critically involved in gallotannin-induced apoptosis in prostate cancer cells. Phytother Res. (2015) 29:1225–36. doi: 10.1002/ptr.5371
126. Wang H, Wang H, Liu H, Liu H, Chen K, Chen K, et al. SIRT1 promotes tumorigenesis of hepatocellular carcinoma through PI3K/PTEN/AKT signaling. Oncol Rep. (2012) 28:311–8. doi: 10.3892/or.2012.1788
127. Zhou X, Chen J, Chen L, Feng X, Liu Z, Hu L, et al. Negative regulation of Sirtuin 1 by AMP-activated protein kinase promotes metformin-induced senescence in hepatocellular carcinoma xenografts. Cancer Lett. (2017) 411:1–11. doi: 10.1016/j.canlet.2017.09.027
128. Kwon HY, Kim J-H, Kim B, Srivastava SK, Kim S-H. Regulation of SIRT1/AMPK axis is critically involved in gallotannin-induced senescence and impaired autophagy leading to cell death in hepatocellular carcinoma cells. Arch Toxicol. (2018) 92:241–57. doi: 10.1007/s00204-017-2021-y
129. Sahin F, Kannangai R, Adegbola O, Wang J, Su G, Torbenson M. mTOR and P70 S6 kinase expression in primary liver neoplasms. Clin Cancer Res. (2004) 10:8421–5. doi: 10.1158/1078-0432.CCR-04-0941
130. Lee SC, Kim K-H, Kim O-H, Lee SK, Hong H-E, Choi BJ, et al. Everolimus plus Ku0063794 regimen promotes anticancer effects against hepatocellular carcinoma cells through the paradoxical inhibition of autophagy. Cancer Res Treat. (2018) 50:1023–38. doi: 10.4143/crt.2017.085
131. Zempleni J, Baier SR, Howard KM, Cui J. Gene regulation by dietary microRNAs. Can J Physiol Pharmacol. (2015) 93:1097–102. doi: 10.1139/cjpp-2014-0392
132. Filipowicz W. RNAi: the nuts and bolts of the RISC machine. Cell. (2005) 122:17–20. doi: 10.1016/j.cell.2005.06.023
133. Calin GA, Croce CM. MicroRNA signatures in human cancers. Nat Rev Cancer. (2006) 6:857–66. doi: 10.1038/nrc1997
134. Lu J, Getz G, Miska EA, Alvarez-Saavedra E, Lamb J, Peck D, et al. MicroRNA expression profiles classify human cancers. Nature. (2005) 435:834. doi: 10.1038/nature03702
135. Ranganathan P, Weaver KL, Capobianco AJ. Notch signalling in solid tumours: a little bit of everything but not all the time. Nat Rev Cancer. (2011) 11:338–51. doi: 10.1038/nrc3035
136. Takebe N, Warren RQ, Ivy SP. Breast cancer growth and metastasis: interplay between cancer stem cells, embryonic signaling pathways and epithelial-to-mesenchymal transition. Breast Cancer Res. (2011) 13:211. doi: 10.1186/bcr2876
137. Xia C, Shui L, Lou G, Ye B, Zhu W, Wang J, et al. 0404 inhibits hepatocellular carcinoma through a p53/miR-34a/SIRT1 positive feedback loop. Sci Rep. (2017) 7:4396. doi: 10.1038/s41598-017-04487-x
138. Zhang Y, Dai J, Deng H, Wan H, Liu M, Wang J, et al. miR-1228 promotes the proliferation and metastasis of hepatoma cells through a p53 forward feedback loop. Br J Cancer. (2015) 112:365–74. doi: 10.1038/bjc.2014.593
139. Xiao Z, Li CH, Chan SL, Xu F, Feng L, Wang Y, et al. A small-molecule modulator of the tumor-suppressor miR34a inhibits the growth of hepatocellular carcinoma. Cancer Res. (2014) 74:6236–47. doi: 10.1158/0008-5472.CAN-14-0855
140. Lou G, Liu Y, Wu S, Xue J, Yang F, Fu H, et al. The p53/miR-34a/SIRT1 positive feedback loop in Quercetin-induced apoptosis. Cell Physiol Biochem Int J Exp Cell Physiol Biochem Pharmacol. (2015) 35:2192–202. doi: 10.1159/000374024
141. Hünten S, Siemens H, Kaller M, Hermeking H. The p53/microRNA network in cancer: experimental and bioinformatics approaches. Adv Exp Med Biol. (2013) 774:77–101. doi: 10.1007/978-94-007-5590-1_5
142. Yamakuchi M, Ferlito M, Lowenstein CJ. miR-34a repression of SIRT1 regulates apoptosis. Proc Natl Acad Sci USA. (2008) 105:13421–6. doi: 10.1073/pnas.0801613105
143. Rottiers V, Näär AM. MicroRNAs in metabolism and metabolic disorders. Nat Rev Mol Cell Biol. (2012) 13:239–50. doi: 10.1038/nrm3313
144. Lou W, Chen Q, Ma L, Liu J, Yang Z, Shen J, et al. Oncolytic adenovirus co-expressing miRNA-34a and IL-24 induces superior antitumor activity in experimental tumor model. J Mol Med Berl Ger. (2013) 91:715–25. doi: 10.1007/s00109-012-0985-x
145. Besten G den, Eunen K van, Groen AK, Venema K, Reijngoud D-J, Bakker BM. The role of short-chain fatty acids in the interplay between diet, gut microbiota, and host energy metabolism. J Lipid Res. (2013) 54:2325–40. doi: 10.1194/jlr.R036012
146. Tailor D, Hahm E-R, Kale RK, Singh SV, Singh RP. Sodium butyrate induces DRP1-mediated mitochondrial fusion and apoptosis in human colorectal cancer cells. Mitochondrion. (2014) 16:55–64. doi: 10.1016/j.mito.2013.10.004
147. Rahmani M, Dai Y, Grant S. The Histone Deacetylase inhibitor sodium butyrate interacts synergistically with Phorbol Myristate Acetate (PMA) to induce mitochondrial damage and apoptosis in human myeloid leukemia cells through a tumor necrosis factor-α-mediated process. Exp Cell Res. (2002) 277:31–47. doi: 10.1006/excr.2002.5548
148. Rada-Iglesias A, Enroth S, Ameur A, Koch CM, Clelland GK, Respuela-Alonso P, et al. Butyrate mediates decrease of histone acetylation centered on transcription start sites and down-regulation of associated genes. Genome Res. (2007) 17:708–19. doi: 10.1101/gr.5540007
149. Zhang J, Yang Y, Yang T, Liu Y, Li A, Fu S, et al. microRNA-22, downregulated in hepatocellular carcinoma and correlated with prognosis, suppresses cell proliferation and tumourigenicity. Br J Cancer. (2010) 103:1215–20. doi: 10.1038/sj.bjc.6605895
150. Pant K, Yadav AK, Gupta P, Islam R, Saraya A, Venugopal SK. Butyrate induces ROS-mediated apoptosis by modulating miR-22/SIRT-1 pathway in hepatic cancer cells. Redox Biol. (2017) 12:340–9. doi: 10.1016/j.redox.2017.03.006
151. Zhang H, Feng Z, Huang R, Xia Z, Xiang G, Zhang J. MicroRNA-449 suppresses proliferation of hepatoma cell lines through blockade lipid metabolic pathway related to SIRT1. Int J Oncol. (2014) 45:2143–52. doi: 10.3892/ijo.2014.2596
152. Pampaloni F, Reynaud EG, Stelzer EHK. The third dimension bridges the gap between cell culture and live tissue. Nat Rev Mol Cell Biol. (2007) 8:839–45. doi: 10.1038/nrm2236
153. Friedrich J, Seidel C, Ebner R, Kunz-Schughart LA. Spheroid-based drug screen: considerations and practical approach. Nat Protoc. (2009) 4:309–24. doi: 10.1038/nprot.2008.226
154. Mehta G, Hsiao AY, Ingram M, Luker GD, Takayama S. Opportunities and challenges for use of tumor spheroids as models to test drug delivery and efficacy. J Controlled Release. (2012) 164:192–204. doi: 10.1016/j.jconrel.2012.04.045
155. Vinci M, Gowan S, Boxall F, Patterson L, Zimmermann M, Court W, et al. Advances in establishment and analysis of three-dimensional tumor spheroid-based functional assays for target validation and drug evaluation. BMC Biol. (2012) 10:29. doi: 10.1186/1741-7007-10-29
156. Nejak-Bowen KN, Monga SPS. Beta-catenin signaling, liver regeneration and hepatocellular cancer: sorting the good from the bad. Semin Cancer Biol. (2011) 21:44–58. doi: 10.1016/j.semcancer.2010.12.010
157. Chen W, Zhang Y-W, Li Y, Zhang J-W, Zhang T, Fu B-S, et al. Constitutive expression of Wnt/β-catenin target genes promotes proliferation and invasion of liver cancer stem cells. Mol Med Rep. (2016) 13:3466–74. doi: 10.3892/mmr.2016.4986
158. de La Coste A, Romagnolo B, Billuart P, Renard CA, Buendia MA, Soubrane O, et al. Somatic mutations of the beta-catenin gene are frequent in mouse and human hepatocellular carcinomas. Proc Natl Acad Sci USA. (1998) 95:8847–51. doi: 10.1073/pnas.95.15.8847
159. Hu G, Chen D, Li X, Yang K, wang H, Wu W. miR-133b regulates the MET proto-oncogene and inhibits the growth of colorectal cancer cells in vitro and in vivo. Cancer Biol Ther. (2010) 10:190–7. doi: 10.4161/cbt.10.2.12186
160. El-Halawany MS, Ismail HM, Zeeneldin AA, Elfiky A, Tantawy M, Kobaisi MH, et al. Investigating the pretreatment miRNA expression patterns of advanced hepatocellular carcinoma patients in association with response to TACE Treatment. BioMed Res Int. (2015) 2015:649750. doi: 10.1155/2015/649750
161. Patil MA, Chua M-S, Pan K-H, Lin R, Lih C-J, Cheung S-T, et al. An integrated data analysis approach to characterize genes highly expressed in hepatocellular carcinoma. Oncogene. (2005) 24:3737. doi: 10.1038/sj.onc.1208479
162. Filmus J, Capurro M. The role of glypican-3 in the regulation of body size and cancer. Cell Cycle. (2008) 7:2787–90. doi: 10.4161/cc.7.18.6672
163. Midorikawa Y, Ishikawa S, Iwanari H, Imamura T, Sakamoto H, Miyazono K, et al. Glypican-3, overexpressed in hepatocellular carcinoma, modulates FGF2 and BMP-7 signaling. Int J Cancer. (2003) 103:455–65. doi: 10.1002/ijc.10856
164. Wu Y, Liu H, Weng H, Zhang X, Li P, Fan C-L, et al. Glypican-3 promotes epithelial-mesenchymal transition of hepatocellular carcinoma cells through ERK signaling pathway. Int J Oncol. (2015) 46:1275–85. doi: 10.3892/ijo.2015.2827
165. Pećina-Šlaus N. Tumor suppressor gene E-cadherin and its role in normal and malignant cells. Cancer Cell Int. (2003) 3:17. doi: 10.1186/1475-2867-3-17
166. Riethmacher D, Brinkmann V, Birchmeier C. A targeted mutation in the mouse E-cadherin gene results in defective preimplantation development. Proc Natl Acad Sci USA. (1995) 92:855–9. doi: 10.1073/pnas.92.3.855
167. Capurro MI, Xiang Y-Y, Lobe C, Filmus J. Glypican-3 Promotes the growth of hepatocellular carcinoma by stimulating canonical Wnt signaling. Cancer Res. (2005) 65:6245–54. doi: 10.1158/0008-5472.CAN-04-4244
168. Filmus J, Capurro M. Glypican-3: a marker and a therapeutic target in hepatocellular carcinoma. FEBS J. (2013) 280:2471–6. doi: 10.1111/febs.12126
169. Stigliano I, Puricelli L, Filmus J, Sogayar MC, Joffé EB de K, Peters MG. Glypican-3 regulates migration, adhesion and actin cytoskeleton organization in mammary tumor cells through Wnt signaling modulation. Breast Cancer Res Treat. (2009) 114:251–62. doi: 10.1007/s10549-008-0009-2
170. Kahn M. Can we safely target the WNT pathway? Nat Rev Drug Discov. (2014) 13:513. doi: 10.1038/nrd4233
171. -Acetyl goniothalamin suppresses proliferation of breast cancer cells via Wnt/β-catenin signaling. Eur J Pharmacol. (2016) 791:455–64. doi: 10.1016/j.ejphar.2016.09.024
172. Slominski A, Pruski D. Melatonin inhibits proliferation and melanogenesis in rodent melanoma cells. Exp Cell Res. (1993) 206:189–94. doi: 10.1006/excr.1993.1137
173. Rhee Y-H, Ahn J-C. Melatonin attenuated adipogenesis through reduction of the CCAAT/enhancer binding protein beta by regulating the glycogen synthase 3 beta in human mesenchymal stem cells. J Physiol Biochem. (2016) 72:145–55. doi: 10.1007/s13105-015-0463-3
174. Dong X, Yang J, Nie X, Xiao J, Jiang S. Perfluorooctane sulfonate (PFOS) impairs the proliferation of C17.2 neural stem cells via the downregulation of GSK-3β/β-catenin signaling. J Appl Toxicol. (2016) 36:1591–8. doi: 10.1002/jat.3320
175. Wu Q, Wang Y, Qian M, Qiao Y, Zou S, Chen C, et al. Sirt1 suppresses Wnt/βCatenin signaling in liver cancer cells by targeting βCatenin in a PKAα-dependent manner. Cell Signal. (2017) 37:62–73. doi: 10.1016/j.cellsig.2017.06.001
176. Pralhada Rao R, Vaidyanathan N, Rengasamy M, Oommen A, Somaiya N, R Jagannath M. Sphingolipid metabolic pathway: an overview of major roles played in human diseases. J Lipids. (2013) 2013:178910. doi: 10.1155/2013/178910
177. Giandomenico V, Simonsson M, Grönroos E, Ericsson J. Coactivator-dependent acetylation stabilizes members of the SREBP family of transcription factors. Mol Cell Biol. (2003) 23:2587–99. doi: 10.1128/MCB.23.7.2587-2599.2003
178. Swinnen JV, Heemers H, van de Sande T, de Schrijver E, Brusselmans K, Heyns W, et al. Androgens, lipogenesis and prostate cancer. J Steroid Biochem Mol Biol. (2004) 92:273–9. doi: 10.1016/j.jsbmb.2004.10.013
179. Repa JJ, Liang G, Ou J, Bashmakov Y, Lobaccaro J-MA, Shimomura I, et al. Regulation of mouse sterol regulatory element-binding protein-1c gene (SREBP-1c) by oxysterol receptors, LXRα and LXRβ. Genes Dev. (2000) 14:2819–30. doi: 10.1101/gad.844900
180. Ponugoti B, Kim D-H, Xiao Z, Smith Z, Miao J, Zang M, et al. SIRT1 deacetylates and inhibits SREBP-1C activity in regulation of hepatic lipid metabolism. J Biol Chem. (2010) 285:33959–70. doi: 10.1074/jbc.M110.122978
181. Lizé M, Klimke A, Dobbelstein M. MicroRNA-449 in cell fate determination. Cell Cycle Georget Tex. (2011) 10:2874–82. doi: 10.4161/cc.10.17.17181
182. Lizé M, Pilarski S, Dobbelstein M. E2F1-inducible microRNA 449a/b suppresses cell proliferation and promotes apoptosis. Cell Death Differ. (2010) 17:452–8. doi: 10.1038/cdd.2009.188
183. Calkin AC, Tontonoz P. Transcriptional integration of metabolism by the nuclear sterol-activated receptors LXR and FXR. Nat Rev Mol Cell Biol. (2012) 13:213–24. doi: 10.1038/nrm3312
184. Buurman R, Gürlevik E, Schäffer V, Eilers M, Sandbothe M, Kreipe H, et al. Histone deacetylases activate hepatocyte growth factor signaling by repressing microRNA-449 in hepatocellular carcinoma cells. Gastroenterology. (2012) 143:811–20.e15. doi: 10.1053/j.gastro.2012.05.033
185. Malakar P, Shilo A, Mogilevsky A, Stein I, Pikarsky E, Nevo Y, et al. Long noncoding RNA MALAT1 promotes hepatocellular carcinoma development by SRSF1 upregulation and mTOR activation. Cancer Res. (2017) 77:1155–67. doi: 10.1158/0008-5472.CAN-16-1508
186. Yuan P, Cao W, Zang Q, Li G, Guo X, Fan J. The HIF-2α-MALAT1-miR-216b axis regulates multi-drug resistance of hepatocellular carcinoma cells via modulating autophagy. Biochem Biophys Res Commun. (2016) 478:1067–73. doi: 10.1016/j.bbrc.2016.08.065
187. Ryan J, Tivnan A, Fay J, Bryan K, Meehan M, Creevey L, et al. MicroRNA-204 increases sensitivity of neuroblastoma cells to cisplatin and is associated with a favourable clinical outcome. Br J Cancer. (2012) 107:967–76. doi: 10.1038/bjc.2012.356
188. Sacconi A, Biagioni F, Canu V, Mori F, Di Benedetto A, Lorenzon L, et al. miR-204 targets Bcl-2 expression and enhances responsiveness of gastric cancer. Cell Death Dis. (2012) 3:e423. doi: 10.1038/cddis.2012.160
189. Hou Z, Xu X, Zhou L, Fu X, Tao S, Zhou J, et al. The long non-coding RNA MALAT1 promotes the migration and invasion of hepatocellular carcinoma by sponging miR-204 and releasing SIRT1. Tumour Biol J Int Soc Oncodevelopmental Biol Med. (2017) 39:1010428317718135. doi: 10.1177/1010428317718135
190. Jiang G, Wen L, Zheng H, Jian Z, Deng W. miR-204-5p targeting SIRT1 regulates hepatocellular carcinoma progression. Cell Biochem Funct. (2016) 34:505–10. doi: 10.1002/cbf.3223
191. Bae HJ, Noh JH, Kim JK, Eun JW, Jung KH, Kim MG, et al. MicroRNA-29c functions as a tumor suppressor by direct targeting oncogenic SIRT1 in hepatocellular carcinoma. Oncogene. (2014) 33:2557–67. doi: 10.1038/onc.2013.216
192. Zhang Y, Yang L, Wang S, Liu Z, Xiu M. MiR-29a suppresses cell proliferation by targeting SIRT1 in hepatocellular carcinoma. Cancer Biomark Sect Dis Markers. (2018) 22:151–9. doi: 10.3233/CBM-171120
193. Luo J, Chen P, Xie W, Wu F. MicroRNA-138 inhibits cell proliferation in hepatocellular carcinoma by targeting Sirt1. Oncol Rep. (2017) 38:1067–74. doi: 10.3892/or.2017.5782
194. Xu R, Zeng G, Gao J, Ren Y, Zhang Z, Zhang Q, et al. miR-138 suppresses the proliferation of oral squamous cell carcinoma cells by targeting Yes-associated protein 1. Oncol Rep. (2015) 34:2171–8. doi: 10.3892/or.2015.4144
195. Wang W, Zhao L-J, Tan Y-X, Ren H, Qi Z-T. MiR-138 induces cell cycle arrest by targeting cyclin D3 in hepatocellular carcinoma. Carcinogenesis. (2012) 33:1113–20. doi: 10.1093/carcin/bgs113
196. Lodygin D, Tarasov V, Epanchintsev A, Berking C, Knyazeva T, Körner H, et al. Inactivation of miR-34a by aberrant CpG methylation in multiple types of cancer. Cell Cycle Georget Tex. (2008) 7:2591–600. doi: 10.4161/cc.7.16.6533
197. Liu C, Kelnar K, Liu B, Chen X, Calhoun-Davis T, Li H, et al. The microRNA miR-34a inhibits prostate cancer stem cells and metastasis by directly repressing CD44. Nat Med. (2011) 17:211–5. doi: 10.1038/nm.2284
198. Li N, Fu H, Tie Y, Hu Z, Kong W, Wu Y, et al. miR-34a inhibits migration and invasion by down-regulation of c-Met expression in human hepatocellular carcinoma cells. Cancer Lett. (2009) 275:44–53. doi: 10.1016/j.canlet.2008.09.035
199. Iorio MV, Croce CM. MicroRNAs in cancer: small molecules with a huge impact. J Clin Oncol. (2009) 27:5848–56. doi: 10.1200/JCO.2009.24.0317
200. Zhou J, Zhou W, Kong F, Xiao X, Kuang H, Zhu Y. microRNA-34a overexpression inhibits cell migration and invasion via regulating SIRT1 in hepatocellular carcinoma. Oncol Lett. (2017) 14:6950–4. doi: 10.3892/ol.2017.7090
201. Caneba CA, Bellance N, Yang L, Pabst L, Nagrath D. Pyruvate uptake is increased in highly invasive ovarian cancer cells under anoikis conditions for anaplerosis, mitochondrial function, and migration. Am J Physiol Endocrinol Metab. (2012) 303:E1036–52. doi: 10.1152/ajpendo.00151.2012
202. Puigserver P, Spiegelman BM. Peroxisome proliferator-activated receptor-gamma coactivator 1 alpha (PGC-1 alpha): transcriptional coactivator and metabolic regulator. Endocr Rev. (2003) 24:78–90. doi: 10.1210/er.2002-0012
203. Nemoto S, Fergusson MM, Finkel T. SIRT1 functionally interacts with the metabolic regulator and transcriptional coactivator PGC-1{alpha}. J Biol Chem. (2005) 280:16456–60. doi: 10.1074/jbc.M501485200
204. LeBleu VS, O'Connell JT, Gonzalez Herrera KN, Wikman H, Pantel K, Haigis MC, et al. PGC-1α mediates mitochondrial biogenesis and oxidative phosphorylation in cancer cells to promote metastasis. Nat Cell Biol. (2014) 16:992–1003. doi: 10.1038/ncb3039
205. Ding W, You H, Dang H, LeBlanc F, Galicia V, Lu SC, et al. Epithelial-to-mesenchymal transition of murine liver tumor cells promotes invasion. Hepatol Baltim Md. (2010) 52:945–53. doi: 10.1002/hep.23748
206. Thiery JP, Acloque H, Huang RYJ, Nieto MA. Epithelial-mesenchymal transitions in development and disease. Cell. (2009) 139:871–90. doi: 10.1016/j.cell.2009.11.007
207. Avila MA, Berasain C, Sangro B, Prieto J. New therapies for hepatocellular carcinoma. Oncogene. (2006) 25:3866. doi: 10.1038/sj.onc.1209550
208. Chen Z, Shi T, Zhang L, Zhu P, Deng M, Huang C, et al. Mammalian drug efflux transporters of the ATP binding cassette (ABC) family in multidrug resistance: a review of the past decade. Cancer Lett. (2016) 370:153–64. doi: 10.1016/j.canlet.2015.10.010
209. Tomonari T, Takeishi S, Taniguchi T, Tanaka T, Tanaka H, Fujimoto S, et al. MRP3 as a novel resistance factor for sorafenib in hepatocellular carcinoma. Oncotarget. (2016) 7:7207–15. doi: 10.18632/oncotarget.6889
210. Sun L, Chen W, Qu L, Wu J, Si J. Icaritin reverses multidrug resistance of HepG2/ADR human hepatoma cells via downregulation of MDR1 and P-glycoprotein expression. Mol Med Rep. (2013) 8:1883–7. doi: 10.3892/mmr.2013.1742
211. Yang T, Zheng Z, Li X, Li Z, Wang Y, Geng Y, et al. MiR-223 modulates multidrug resistance via downregulation of ABCB1 in hepatocellular carcinoma cells: Exp Biol Med. (2013) 238:1024–32. doi: 10.1177/1535370213497321
212. Liang X-J, Finkel T, Shen D-W, Yin J-J, Aszalos A, Gottesman MM. SIRT1 Contributes in part to cisplatin resistance in cancer cells by altering mitochondrial metabolism. Mol Cancer Res. (2008) 6:1499–506. doi: 10.1158/1541-7786.MCR-07-2130
213. Jin Y-D, Ren Y, Wu M-W, Chen P, Lu J. Effect of shikonin on multidrug resistance in HepG2: The role of SIRT1. Pharm Biol. (2015) 53:1016–21. doi: 10.3109/13880209.2014.952836
214. Daitoku H, Sakamaki J, Fukamizu A. Regulation of FoxO transcription factors by acetylation and protein–protein interactions. Biochim Biophys Acta. (2011) 1813:1954–60. doi: 10.1016/j.bbamcr.2011.03.001
215. Yang Y, Hou H, Haller EM, Nicosia SV, Bai W. Suppression of FOXO1 activity by FHL2 through SIRT1-mediated deacetylation. EMBO J. (2005) 24:1021–32. doi: 10.1038/sj.emboj.7600570
216. Han C-Y, Cho K-B, Choi H-S, Han H-K, Kang K-W. Role of FoxO1 activation in MDR1 expression in adriamycin-resistant breast cancer cells. Carcinogenesis. (2008) 29:1837–44. doi: 10.1093/carcin/bgn092
217. Oh WK, Cho KB, Hien TT, Kim TH, Kim HS, Dao TT, et al. Amurensin G, a Potent natural SIRT1 inhibitor, rescues doxorubicin responsiveness via down-regulation of multidrug resistance 1. Mol Pharmacol. (2010) 78:855–64. doi: 10.1124/mol.110.065961
218. Ng IO, Liang ZD, Cao L, Lee TK. DLC-1 is deleted in primary hepatocellular carcinoma and exerts inhibitory effects on the proliferation of hepatoma cell lines with deleted DLC-1. Cancer Res. (2000) 60:6581–4.
219. Song J, Qu Z, Guo X, Zhao Q, Zhao X, Gao L, et al. Hypoxia-induced autophagy contributes to the chemoresistance of hepatocellular carcinoma cells. Autophagy. (2009) 5:1131–44. doi: 10.4161/auto.5.8.9996
220. Guo X, Hu F, Zhang S, Zhao Q, Zong C, Ye F, et al. Inhibition of p53 increases chemosensitivity to 5-FU in nutrient-deprived hepatocarcinoma cells by suppressing autophagy. Cancer Lett. (2014) 346:278–84. doi: 10.1016/j.canlet.2014.01.011
221. Cea M, Soncini D, Fruscione F, Raffaghello L, Garuti A, Emionite L, et al. Synergistic interactions between HDAC and sirtuin inhibitors in human leukemia cells. PLoS ONE. (2011) 6:e22739. doi: 10.1371/journal.pone.0022739
222. Armour SM, Bennett EJ, Braun CR, Zhang X-Y, McMahon SB, Gygi SP, et al. A high-confidence interaction map identifies SIRT1 as a mediator of acetylation of USP22 and the SAGA coactivator complex. Mol Cell Biol. (2013) 33:1487–502. doi: 10.1128/MCB.00971-12
223. Lin Z, Yang H, Kong Q, Li J, Lee S-M, Gao B, et al. USP22 antagonizes p53 transcriptional activation by deubiquitinating Sirt1 to suppress cell apoptosis and is required for mouse embryonic development. Mol Cell. (2012) 46:484–94. doi: 10.1016/j.molcel.2012.03.024
224. Wang B, Wang D, Yan T, Yuan H. MiR-138-5p promotes TNF-α-induced apoptosis in human intervertebral disc degeneration by targeting SIRT1 through PTEN/PI3K/Akt signaling. Exp Cell Res. (2016) 345:199–205. doi: 10.1016/j.yexcr.2016.05.011
225. Wang H, Jia XH, Chen JR, Yi YJ, Wang JY, Li YJ, et al. HOXB4 knockdown reverses multidrug resistance of human myelogenous leukemia K562/ADM cells by downregulating P-gp, MRP1 and BCRP expression via PI3K/Akt signaling pathway. Int J Oncol. (2016) 49:2529–37. doi: 10.3892/ijo.2016.3738
226. Ling S, Li J, Shan Q, Dai H, Lu D, Wen X, et al. USP22 mediates the multidrug resistance of hepatocellular carcinoma via the SIRT1/AKT/MRP1 signaling pathway. Mol Oncol. (2017) 11:682–95. doi: 10.1002/1878-0261.12067
227. Ota H, Tokunaga E, Chang K, Hikasa M, Iijima K, Eto M, et al. Sirt1 inhibitor, Sirtinol, induces senescence-like growth arrest with attenuated Ras-MAPK signaling in human cancer cells. Oncogene. (2006) 25:176–85. doi: 10.1038/sj.onc.1209049
228. Wu Y, Meng X, Huang C, Li J. Emerging role of silent information regulator 1 (SIRT1) in hepatocellular carcinoma: a potential therapeutic target. Tumour Biol J Int Soc Oncodevelopmental Biol Med. (2015) 36:4063–74. doi: 10.1007/s13277-015-3488-x
229. Winder WW, Hardie DG. AMP-activated protein kinase, a metabolic master switch: possible roles in type 2 diabetes. Am J Physiol. (1999) 277:E1–10. doi: 10.1152/ajpendo.1999.277.1.E1
230. Shaw RJ. LKB1 and AMP-activated protein kinase control of mTOR signalling and growth. Acta Physiol Oxf Engl. (2009) 196:65–80. doi: 10.1111/j.1748-1716.2009.01972.x
231. Lee C-W, Wong LL-Y, Tse EY-T, Liu H-F, Leong VY-L, Man-Fong J, et al. AMPK promotes p53 acetylation via phosphorylation and inactivation of SIRT1 in liver cancer cells. Cancer Res. (2012) 72:4394–404. doi: 10.1158/0008-5472.CAN-12-0429
232. Ewald JA, Desotelle JA, Wilding G, Jarrard DF. Therapy-induced senescence in cancer. J Natl Cancer Inst. (2010) 102:1536–46. doi: 10.1093/jnci/djq364
233. Yi G, He Z, Zhou X, Xian L, Yuan T, Jia X, et al. Low concentration of metformin induces a p53-dependent senescence in hepatoma cells via activation of the AMPK pathway. Int J Oncol. (2013) 43:1503–10. doi: 10.3892/ijo.2013.2077
234. Chen H-P, Shieh J-J, Chang C-C, Chen T-T, Lin J-T, Wu M-S, et al. Metformin decreases hepatocellular carcinoma risk in a dose-dependent manner: population-based and in vitro studies. Gut. (2013) 62:606–15. doi: 10.1136/gutjnl-2011-301708
235. Caton PW, Nayuni NK, Kieswich J, Khan NQ, Yaqoob MM, Corder R. Metformin suppresses hepatic gluconeogenesis through induction of SIRT1 and GCN5. J Endocrinol. (2010) 205:97–106. doi: 10.1677/JOE-09-0345
236. Cantó C, Gerhart-Hines Z, Feige JN, Lagouge M, Noriega L, Milne JC, et al. AMPK regulates energy expenditure by modulating NAD+ metabolism and SIRT1 activity. Nature. (2009) 458:1056–60. doi: 10.1038/nature07813
237. Nelson LE, Valentine RJ, Cacicedo JM, Gauthier M-S, Ido Y, Ruderman NB. A novel inverse relationship between metformin-triggered AMPK-SIRT1 signaling and p53 protein abundance in high glucose-exposed HepG2 cells. Am J Physiol Cell Physiol. (2012) 303:C4–13. doi: 10.1152/ajpcell.00296.2011
238. Füllgrabe J, Klionsky DJ, Joseph B. Histone post-translational modifications regulate autophagy flux and outcome. Autophagy. (2013) 9:1621–3. doi: 10.4161/auto.25803
239. Ravikumar B, Sarkar S, Davies JE, Futter M, Garcia-Arencibia M, Green-Thompson ZW, et al. Regulation of mammalian autophagy in physiology and pathophysiology. Physiol Rev. (2010) 90:1383–435. doi: 10.1152/physrev.00030.2009
240. Kim J-O, Kim K-H, Song IS, Cheon K-S, Kim O-H, Lee SC, et al. Potentiation of the anticancer effects of everolimus using a dual mTORC1/2 inhibitor in hepatocellular carcinoma cells. Oncotarget. (2016) 8:2936–48. doi: 10.18632/oncotarget.13808
241. Tikhomirov AS, Shchekotikhin AE, Lee Y-H, Chen Y-A, Yeh C-A, Tatarskiy VV, et al. Synthesis and Characterization of 4,11-Diaminoanthra[2,3-b]furan-5,10-diones: tumor cell apoptosis through tNOX-Modulated NAD(+)/NADH Ratio and SIRT1. J Med Chem. (2015) 58:9522–34. doi: 10.1021/acs.jmedchem.5b00859
242. Tikhomirov AS, Lin CY, Volodina YL, Dezhenkova LG, Tatarskiy VV, Schols D, et al. New antitumor anthra[2,3-b]furan-3-carboxamides: Synthesis and structure-activity relationship. Eur J Med Chem. (2018) 148:128–39. doi: 10.1016/j.ejmech.2018.02.027
243. Lee YH, Chen HY, Su LJ, Chueh PJ. Sirtuin 1 (SIRT1) deacetylase activity and NAD?/NADH Ratio are imperative for capsaicin-mediated programmed cell death. J Agric Food Chem. (2015) 63:7361–70. doi: 10.1021/acs.jafc.5b02876
244. Chen HY, Cheng HL, Lee YH, Yuan TM, Chen SW, Lin YY, et al. Tumor-associated NADH oxidase (tNOX)-NAD+-sirtuin 1 axis contributes to oxaliplatin-induced apoptosis of gastric cancer cells. Oncotarget. (2017) 8:15338–48. doi: 10.18632/oncotarget.14787.
245. Shchekotikhin AE, Dezhenkova LG, Tsvetkov VB, Luzikov YN, Volodina YL, Tatarskiy VV, et al. Discovery of antitumor anthra[2,3-b]furan-3-carboxamides: Optimization of synthesis and evaluation of antitumor properties. Eur J Med Chem. (2016) 112:114–29. doi: 10.1016/j.ejmech.2016.01.050
246. Lin C-Y, Islam A, Su CJ, Tikhomirov AS, Shchekotikhin AE, Chuang S-M, et al. Engagement with tNOX (ENOX2) to Inhibit SIRT1 and Activate p53-Dependent and -Independent apoptotic pathways by novel 4,11-Diaminoanthra[2,3-b]furan-5,10-diones in hepatocellular carcinoma cells. Cancers. (2019) 11:420. doi: 10.3390/cancers11030420
247. An X, Sarmiento C, Tan T, Zhu H. Regulation of multidrug resistance by microRNAs in anti-cancer therapy. Acta Pharm Sin B. (2017) 7:38. doi: 10.1016/j.apsb.2016.09.002
248. Chueh P-J, Kim C, Cho N, Morré DM, Morré DJ. Molecular cloning and characterization of a tumor-associated, growth-related, and time-keeping hydroquinone (NADH) Oxidase (tNOX) of the HeLa cell surface. Biochemistry. (2002) 41:3732–41. doi: 10.1021/bi012041
249. Lin MH, Lee YH, Cheng HL, Chen HY, Jhuang FH, Chueh PJ. Capsaicin Inhibits Multiple Bladder Cancer Cell Phenotypes by Inhibiting Tumor-Associated NADH Oxidase (tNOX) and Sirtuin1 (SIRT1). Molecules. (2016) 21:E849.
250. Chueh P-J, Wu L-Y, Morré DM, Morré DJ. tNOX is both necessary and sufficient as a cellular target for the anticancer actions of capsaicin and the green tea catechin (-)-epigallocatechin-3-gallate. Biofactors. (2004) 20:235–49.
251. Shachaf CM, Kopelman AM, Arvanitis C, Karlsson A, Beer S, Mandl S, et al. MYC inactivation uncovers pluripotent differentiation and tumour dormancy in hepatocellular cancer. Nature. (2004) 431:1112–7. doi: 10.1038/nature03043
252. Wang Y, Wu M-C, Sham JST, Zhang W, Wu W-Q, Guan X-Y. Prognostic significance of c-myc and AIB1 amplification in hepatocellular carcinoma. A broad survey using high-throughput tissue microarray. Cancer. (2002) 95:2346–52. doi: 10.1002/cncr.10963
253. Menssen A, Hydbring P, Kapelle K, Vervoorts J, Diebold J, Lüscher B, et al. The c-MYC oncoprotein, the NAMPT enzyme, the SIRT1-inhibitor DBC1, and the SIRT1 deacetylase form a positive feedback loop. Proc Natl Acad Sci USA. (2012) 109:E187–96. doi: 10.1073/pnas.1105304109
254. Hong K-S, Park J-I, Kim M-J, Kim H-B, Lee J-W, Dao TT, et al. Involvement of SIRT1 in hypoxic down-regulation of c-Myc and β-catenin and hypoxic preconditioning effect of polyphenols. Toxicol Appl Pharmacol. (2012) 259:210–8. doi: 10.1016/j.taap.2011.12.025
Keywords: miRNA, cancer, HCC, sirtuin 1, cancer stem cells
Citation: Farcas M, Gavrea A-A, Gulei D, Ionescu C, Irimie A, Catana CS and Berindan-Neagoe I (2019) SIRT1 in the Development and Treatment of Hepatocellular Carcinoma. Front. Nutr. 6:148. doi: 10.3389/fnut.2019.00148
Received: 15 February 2019; Accepted: 27 August 2019;
Published: 25 September 2019.
Edited by:
Anna Alisi, Bambino Gesù Children Hospital (IRCCS), ItalyReviewed by:
Daniele Lettieri Barbato, University of Rome Tor Vergata, ItalyOndrej Slaby, Brno University of Technology, Czechia
Copyright © 2019 Farcas, Gavrea, Gulei, Ionescu, Irimie, Catana and Berindan-Neagoe. This is an open-access article distributed under the terms of the Creative Commons Attribution License (CC BY). The use, distribution or reproduction in other forums is permitted, provided the original author(s) and the copyright owner(s) are credited and that the original publication in this journal is cited, in accordance with accepted academic practice. No use, distribution or reproduction is permitted which does not comply with these terms.
*Correspondence: Cristina S. Catana, Y2NhdGFuYTAxMjgmI3gwMDA0MDtnbWFpbC5jb20=; Ioana Berindan-Neagoe, aW9hbmFuZWFnb2UyOSYjeDAwMDQwO2dtYWlsLmNvbQ==