- 1Department of Microbiology and Systems Biology, Netherlands Organisation for Applied Scientific Research (TNO), Zeist, Netherlands
- 2Department of Risk Analysis for Products in Development, Netherlands Organisation for Applied Scientific Research (TNO), Zeist, Netherlands
- 3Department of Metabolic Health Research, Netherlands Organisation for Applied Scientific Research (TNO), Leiden, Netherlands
- 4Janssen Research & Development, LLC, Spring House, PA, United States
Obesity, type 2 diabetes, and other metabolic disorders have a large impact on global health, especially in Western countries. An important hallmark of metabolic disorders is chronic low-grade inflammation. A key player in chronic low-grade inflammation is dysmetabolism, which is defined as the inability to keep homeostasis resulting in loss of lipid control, oxidative stress, inflammation, and insulin resistance. Although often not yet detectable in the circulation, chronic low-grade inflammation can be present in one or multiple organs. The response to a metabolic challenge containing lipids may magnify dysfunctionalities at the tissue level, causing an overflow of inflammatory markers into the circulation and hence allow detection of early low-grade inflammation. Here, we summarize the evidence of successful application of metabolic challenge tests in type 2 diabetes, metabolic syndrome, obesity, and unhealthy aging. We also review how metabolic challenge tests have been successfully applied to evaluate nutritional intervention effects, including an “anti-inflammatory” mixture, dark chocolate, whole grain wheat and overfeeding. Additionally, we elaborate on future strategies to (re)gain inflammatory flexibility. Through epigenetic and metabolic regulation, the inflammatory response may be trained by regular mild and metabolic triggers, which can be understood from the perspective of trained immunity, hormesis and pro-resolution. New strategies to optimize dynamics of inflammation may become available.
Introduction
Obesity, type 2 diabetes, and other metabolic disorders have a large impact on the health of society in Western and developing countries. These disorders not only increase the risk of cardiovascular disease; they also represent a common preventable cause of cancer (1, 2). Currently, about 15% of the world population suffers from obesity, 5–10% has type 2 diabetes, and these numbers are increasing (3, 4). An important hallmark of metabolic disorders is chronic low-grade inflammation, marked by histological changes of tissues and a phenotypic shift in immune cell types (5–7). Eventually, such tissues release pro-inflammatory cytokines, acute phase proteins, pro-inflammatory lipids, and other biological inflammatory mediators into the circulation, converting tissue-based low-grade inflammation into a systemic inflammatory condition (5, 8, 9).
To be able to quantify low-grade inflammation without biopsies before it progresses to a systemic disease, one of the current challenges is to identify systemic biomarkers that reflect local tissue inflammation caused by metabolic dysfunction (8, 9). As a solution, dynamic time-resolved metabolic responses to metabolic challenge tests (e.g., the oral glucose challenge test (OGTT) or mixed meal test), have been used to evaluate inflammation in conditions of metabolic dysfunction (10).
While current anti-inflammation therapies often focus on inflammation only, new possibilities may exist for the creation of interventions in the interaction between metabolism and inflammation dynamics. Increased anti-inflammatory nutritional intake [as defined by an anti-inflammatory diet potential, see (2)], for example, is associated with a decreased risk in overall mortality, cardiovascular disease, and cancer (2). Inflammation is seen as a pathological state resulting from many disease processes that occur late in pathogenesis and normally is targeted at a late stage in disease pathogenesis. To intercept this process before irreversible changes occur, a refocus is needed onto the early stages of low-grade inflammation. Since metabolism and the innate immune system are closely connected [for review, see (5, 7)], interception could focus on metabolism as well as on inflammation dynamics to restore disbalances.
In this review, we give a short introduction to the role of chronic low-grade inflammation in metabolic disorders. Next, we describe the state-of-the-art of metabolic challenge tests for the diagnosis of chronic low-grade inflammation, and the evaluation of nutritional interventions to reverse it. Additionally, we give a future perspective on innovative strategies that focus on the training of inflammatory dynamics to prevent or reverse chronic low-grade inflammation in metabolic disorders.
Low-Grade Inflammation and Metabolic Control
Inflammation is a central part of the immune system as an attempt to heal the body after injury, defend itself against foreign invaders, and to repair damaged tissue. Healthy inflammation prevents wounds from persisting and infections from becoming lethal.
Inflammation may manifest in an acute, high-grade as well as in a chronic low-grade manner (Table 1). Acute inflammation is short-term and the effects subside after a few days once the damage/infection/irritation has been repaired or removed and mainly involves cells of the innate immune system. In contrast, chronic low-grade inflammation is long-term, and can constitute a damaging process with a mild sustained induction of inflammation. As a response to such an induction (e.g., metabolic stressor), tissue-resident cells and resident/patrolling leukocytes become activated, resulting in the secretion of pro-inflammatory mediators which leads to the recruitment/infiltration of pro-inflammatory leukocytes (11). Under healthy conditions, local immune cells such as macrophages and regulatory T cells produce anti-inflammatory cytokines that resolve inflammation and re-establish homeostasis (12, 13). However, without (or with insufficient) activation of anti-inflammatory or inflammation-resolving mediators, this results in the amplification of inflammation by autocrine and paracrine signals resulting in an imbalance of the immune regulatory network (14–16) (Figures 1A, 2).
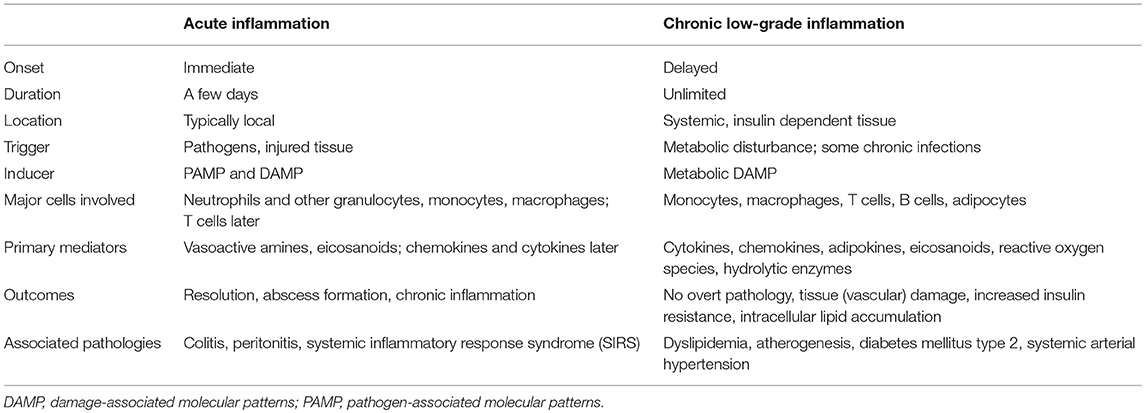
Table 1. Features of acute and chronic low-grade inflammation [adapted from Calder et al. (9)].
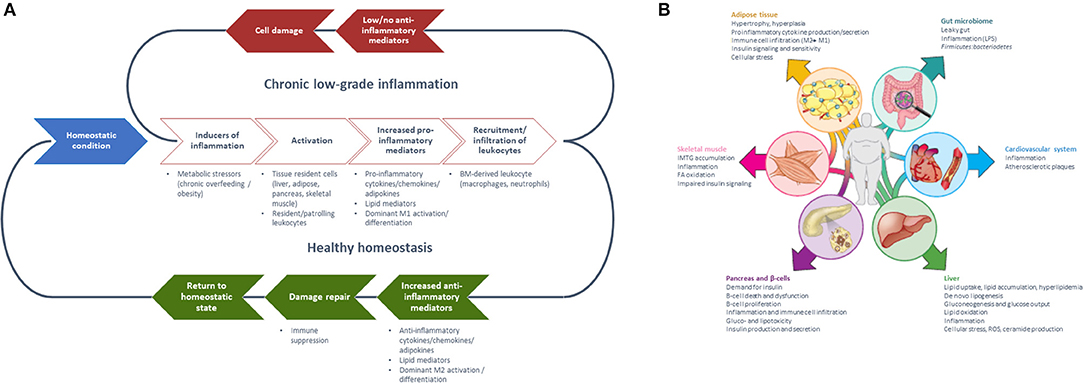
Figure 1. (A) Generalized model of sequential steps involved in the inflammatory response in metabolic tissues resulting in chronic low-grade inflammation or return to a healthy homeostatic condition [inspired by Villeneuve et al. (17)] and (B) Manifestation of chronic low-grade inflammation in the different metabolic tissues [Reprinted with permission from Ralston et al. (18)].
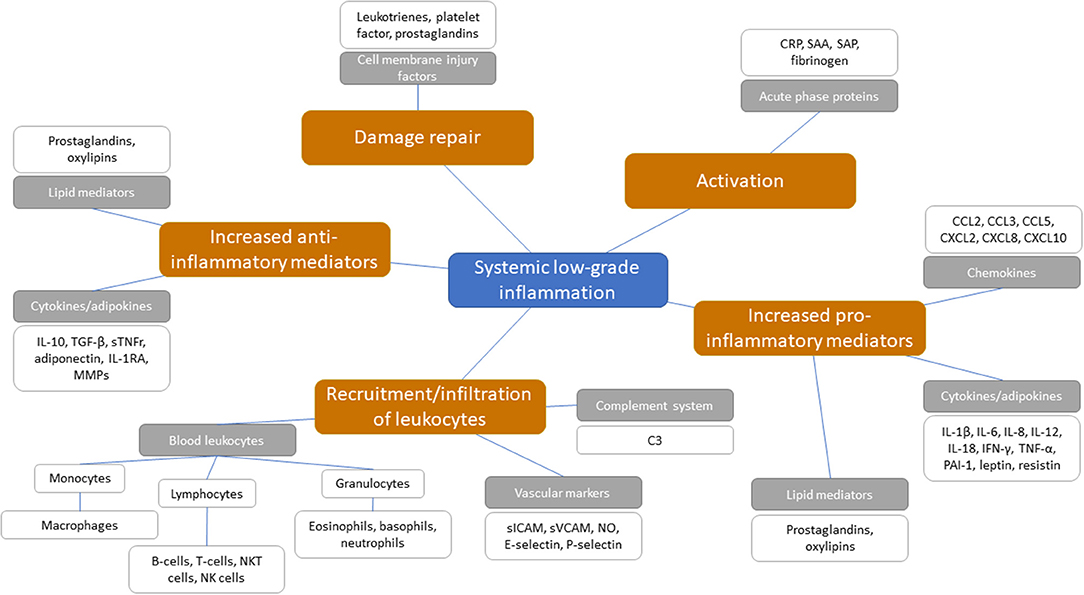
Figure 2. Potential systemic biomarkers categorized following the sequential steps involved in the inflammatory cascade with chronic low-grade inflammation. Orange boxes represent the sequential steps involved in the inflammatory response within chronic low-grade inflammation. Gray boxes represent inflammatory biomarker classes and are categorized under the sequential inflammatory steps. White boxes present the biomarkers as positioned under the inflammatory biomarker classes.
The mechanistic basis for the interaction between metabolic and inflammatory systems is referred to as metaflammation [for review, see (5, 7)]. From an evolutionary perspective, the interaction between immune and metabolic pathways is highly conserved from invertebrates to mammals (5). The fat body of the fruit fly functions to sense and store nutrients, as well as to defend against pathogenic invaders (19). Throughout evolution, the fat body has developed into distinguishable metabolic and immune organs that exist in mammals, amongst which are adipose tissue and liver. Signaling pathways [e.g., NLRP3 inflammasome (18, 20), JNK-NFkB pathway (5)], however, are conserved within these tissues, so with either inflammation or metabolic dysfunction, the same pathways become activated (5). These mechanisms are ubiquitously present in all metabolic tissues and take part in the progression from a healthy to a metabolically compromised state (Figures 1B, 3). Nevertheless, tissue-specific characteristics of chronic low-grade inflammation also exist, which are described in more detail below (Figure 1B) (18).
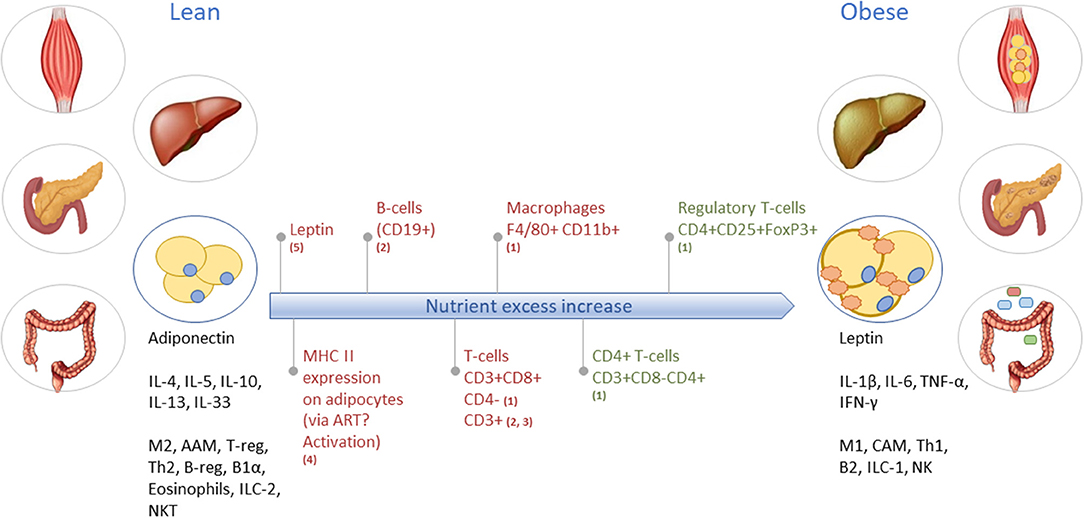
Figure 3. Obesity-induced development of tissue inflammation in various organs. As an example, the long-term inflammatory dynamics and characteristics of lean toward obese WAT are illustrated. In lean WAT, immune cells are generally in an overall Type2 state (left black text), while in obese WAT the immune cells operate in a Type1 state (right black text). Red text indicates increase/abundancy of the mentioned adipokine and immune cells. Green text represents a decrease in the mentioned immune cell. M2, macrophage type 2; AAM, alternatively activated macrophages; T-reg, regulatory T-cells; Th2, T-helper type 2 cells; B-reg, regulatory B-cells; B1α, B-cells type 1 alpha; ILC-2, innate lymphoid cells type 2; NKT, natural killer T-cells; M1, macrophage type 1; CAM, classically activated macrophages; Th1, T-helper type 1 cells; B2, B-cells type 2; ILC-1, innate lymphoid cells type 1; NK, natural killer cells. Based on (1) (21); (2) (22); (3) (23); (4) (24); and (5) (25).
Adipose tissue inflammation is characterized by hypoxia and mechanical stress following hypertrophy—and possibly also hyperplasia of fat tissue (26–29)—that causes adipocytes to change into a pro-inflammatory secretory profile of cytokines and adipokines (e.g., higher TNF-α and leptin, lower adiponectin, and resistin) (27). Furthermore, the metabolic balance within adipocytes shifts from glycolysis toward lipolysis, which leads to increased free fatty acid levels in the system that stimulate the cellular metaflammatory pathways (11, 28). Intestinal inflammation is associated with dysbiosis, i.e., the impaired ability of the microbiome-intestinal system to adapt to alterations (30). The breakdown of the human-microbiome partnership is able to negatively change the epithelial barrier function, as well as the production of specific metabolites and immune function (31, 32). Due to reduced barrier and adaptive and innate immune function, the translocation of bacterial endotoxins and dietary products from the gut into the systemic circulation is possible and can expand low-grade inflammation into other organs (33). The liver is exposed to these gut-derived products, which can lead to liver inflammation in metabolic syndrome (MetS) (34). Moreover, metabolic stressors (e.g., saturated fatty acids, glucose) activate hepatocytes to release alarmins (IL-33, HMGB1), extracellular vesicles that contain inflammatory effector proteins and microRNAs, hepatokines (fetuin-A, selenoprotein P), and other stress molecules such as ATP, ceramides and nitric oxide (18, 34–36). These stressors all activate a local inflammatory response via e.g., Kupffer cells (34). Investigations toward the initiation of skeletal muscle inflammation are ongoing, however, it appears that inflammation is mainly manifested in intermyocellular and perimuscular adipose tissue. Free fatty acids and inflammatory molecules from perimuscular adipose tissue and other tissues stimulate myocyte inflammation and dysregulated myocyte metabolism (20, 37, 38). The release of cytokines and myokines, in turn, contributes to insulin resistance and low-grade inflammation (37).
Given that such local tissue inflammation precedes the systemic manifestation of disease, it is a challenge to identify systemic biomarkers that represent local tissue inflammation related to metabolic dysfunction.
Dysfunctional Lipid Control Mechanisms as the Basis of Low-Grade Inflammation
Lipid control is a key player in local tissue inflammation. There is a tight connection between metabolic and inflammatory pathways; they overlap and use the same master regulators (5, 7). This may explain that metabolic overload requires adjustment of regulators of lipid metabolism that inherently also affects inflammatory processes (39, 40).
One or more of the following four impairments in lipid control mechanisms may cause metabolic inflammation and activation of inflammatory pathways:
- Inappropriate adjustment of lipid uptake and lipid handling processes via the enterohepatic cycle involving the gut, microbiota and the liver (41–43);
- Inappropriate storage of excess lipids as ectopic fat in organs not designed for lipid storage (44, 45). In contrast to adipose tissue depots that can respond with hyperplasia, these depots have limited storage capacity and respond mainly in terms of hypertrophy. For example, mesenteric adipose tissue that has close anatomical proximity to the liver (45, 46);
- Intracellular imbalance between pro-inflammatory vs. anti-inflammatory lipids;
- Unfavorable composition of circulating lipids and high content of fatty acids that are prone to oxidation (47). The intracellular presentation of lipids determines accessibility and utilization within a cell, e.g., as small or large lipid droplet, and their role in the circulation, e.g., as part of VLDL/LDL lipoprotein or HDL lipoprotein (47, 48). For example, it is assumed that larger droplets are more detrimental and associated with tissue inflammation. Indeed, large macro-vesicular lipid droplets are often found in pathological conditions (e.g., in non-alcoholic fatty liver disease) and are associated with inflammation and fibrosis (47, 49).
There is a series of blood-based biomarkers that may reflect the different steps in chronic low-grade inflammation (Figure 2). These include well-known biomarkers, e.g., acute phase proteins, cytokine and chemokine levels, as well as some more innovative biomarkers, e.g., oxylipins, an oxidation product of essential fatty acids and related to vascular function and inflammation associated with metabolic function (50, 51). While some of these biomarkers (e.g., acute phase proteins and cytokines) are appropriate for measuring acute inflammation, no good systemic blood-based biomarkers exist for the identification of chronic low-grade inflammation on an individual level (8, 9, 52, 53). Although chronic low-grade inflammation in multiple organs can be present, this may not yet be detectable in the circulation (54).
Measuring Phenotypic Flexibility for Early Detection of Metabolic Disorders
Phenotypic flexibility is referred to as the ability to maintain homeostasis by a highly energy-dependent, rapid and orchestrated adaptation to continuous changes of the environment, such as nutrient intake, physical exercise, and temperature changes. Phenotypic flexibility is the opposite of dysmetabolism, i.e., the inability to keep homeostasis, that eventually leads to e.g., oxidative stress, insulin resistance and dysbiosis (42, 55–59). Continued metabolic overload may eventually lead to chronic or permanent adaptation of the system, characterized by mitochondrial dysfunction and low-grade inflammation.
The measurement of one's health status via phenotypic flexibility is possible by analyzing the dynamical responses to a metabolic challenge test (60–62). Considering that lipid control plays a key role in chronic low-grade inflammation, the response to a metabolic challenge containing lipids may magnify dysfunctionalities on the tissue level causing an overflow of inflammatory markers into the circulation and hence allow early detection of metabolic and inflammatory dysfunction in an individual via dynamical response profiles (61–63) (Figure 4). Early signs of reduced flexibility (or dysmetabolism) manifest through processes that are regulatory and adaptive in order to keep core metabolic processes in balance (51) (Figure 5). An example of an adaptive process is the production of insulin, which is increased under insulin resistance conditions in order to maintain the core process of glucose dynamics. The regulatory, adaptive and core metabolic processes are closely connected, shown by the myriad of dynamical biomarker responses to a metabolic challenge, including incretins, satiety markers, bile acids, amino acids, fatty acids and inflammatory markers [see (10, 61) for review]. Inflammatory markers do respond to glucose (65) and lipid challenge tests (66), as well as a combination of both (67, 68), albeit that only subtle effects were observed for inflammatory mediators in the circulation. This is not surprising given that inflammatory control is one of the core metabolic processes (51). Interestingly, several oxylipins, acute phase proteins, and vascular markers showed stronger responses to metabolic challenge tests (51, 68), marking them as potential early dynamical markers for metabolic stress.
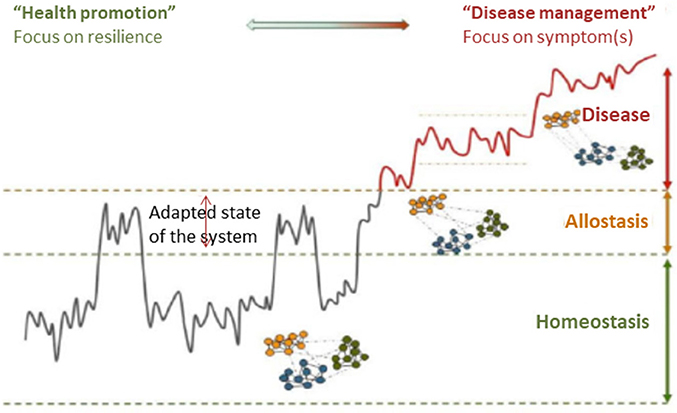
Figure 4. A framework for understanding the progression of healthy homeostasis to disease. For chronic diseases, the pathological development toward a disease state typically takes years. While the medical world has made tremendous progress toward the measurement of disease, the ability to measure the progress toward the disease is lacking. Resilience as measure of health status provides a promising solution to this problem. In contrast to the classical symptom/biomarker approach, challenge tests are used to measure resilience as an early biomarker of disease or (nutritional) effect. Reprinted with permission from van der Greef et al. (64).
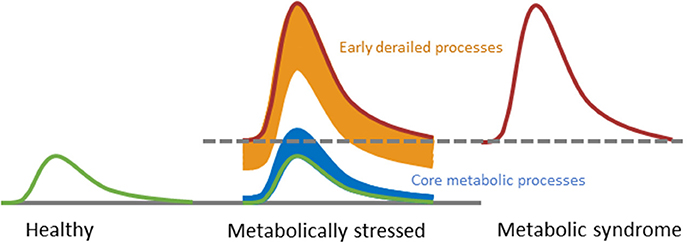
Figure 5. Conceptual representation of challenge tests may evaluate the progression toward metabolic disorder. During progression from a healthy state toward a state of metabolic syndrome, only the early derailed processes respond differently to a challenge test in order to keep the core processes in balance. Eventually, the balance of the core processes is also disrupted. Modified with permission from Kardinaal et al. (51).
With the aim to measure phenotypic flexibility by one standardized oral challenge, a systematic literature evaluation was previously performed to identify the optimal composition of a metabolic challenge test (10). A challenge test that combined glucose, proteins, and lipids in one drink was superior to those with fewer macronutrients. The systemic responses involved multiple local and systemic processes, including those from the gut, adipose tissue, muscle, liver, kidney, vasculature, pancreas, brain (10, 69). The work resulted in the standardized so-called “PhenFlex” oral challenge test, composed of 75 g glucose, 60 g palm olein (36.6% mono-unsaturated fatty acids, 48.8% saturated fatty acids, 9.1% poly-unsaturated fatty acids) and 20 g protein, enabling the comparison of phenotypic flexibility among studies and different stages of health (10, 54, 62).
Several studies have evaluated the potential of metabolic challenge tests for early diagnosis of chronic low-grade inflammation in the context of metabolic diseases (Table 2).
- In type 2 diabetes, both fasting and postprandial concentrations of inflammatory and vascular markers are higher as compared to healthy individuals (65, 74, 75). Furthermore, the post-prandial increase of pro-inflammatory mediators and decrease of anti-inflammatory mediators was stronger in type 2 diabetics (74), and it took longer for cytokines and vascular markers to return to baseline (75).
- In MetS vs. healthy subjects acute phase proteins were increased at fasting, while a challenge test was needed to reveal increased responses of cytokines and vascular markers and reduced responses of oxylipins (51).
- In obese subjects, fasting levels and challenge responses of acute phase proteins were increased as compared to healthy individuals (66, 72). In contrast to MetS, however, of the cytokines only TNF-α showed an increased response to a lipid challenge test (73). Other cytokines, vascular markers, and oxylipins showed no difference between obese and healthy persons (50, 66, 72, 73). Additionally, gene expression of platelet activation and human leukocyte antigen class II was increased, as well as the challenge test response of lymphocytes and neutrophils (71, 72).
- Having a high percentage of bodyfat in combination with aging leads to higher levels of pro-inflammatory cytokines, and lower levels of anti-inflammatory cytokines (70, 76).
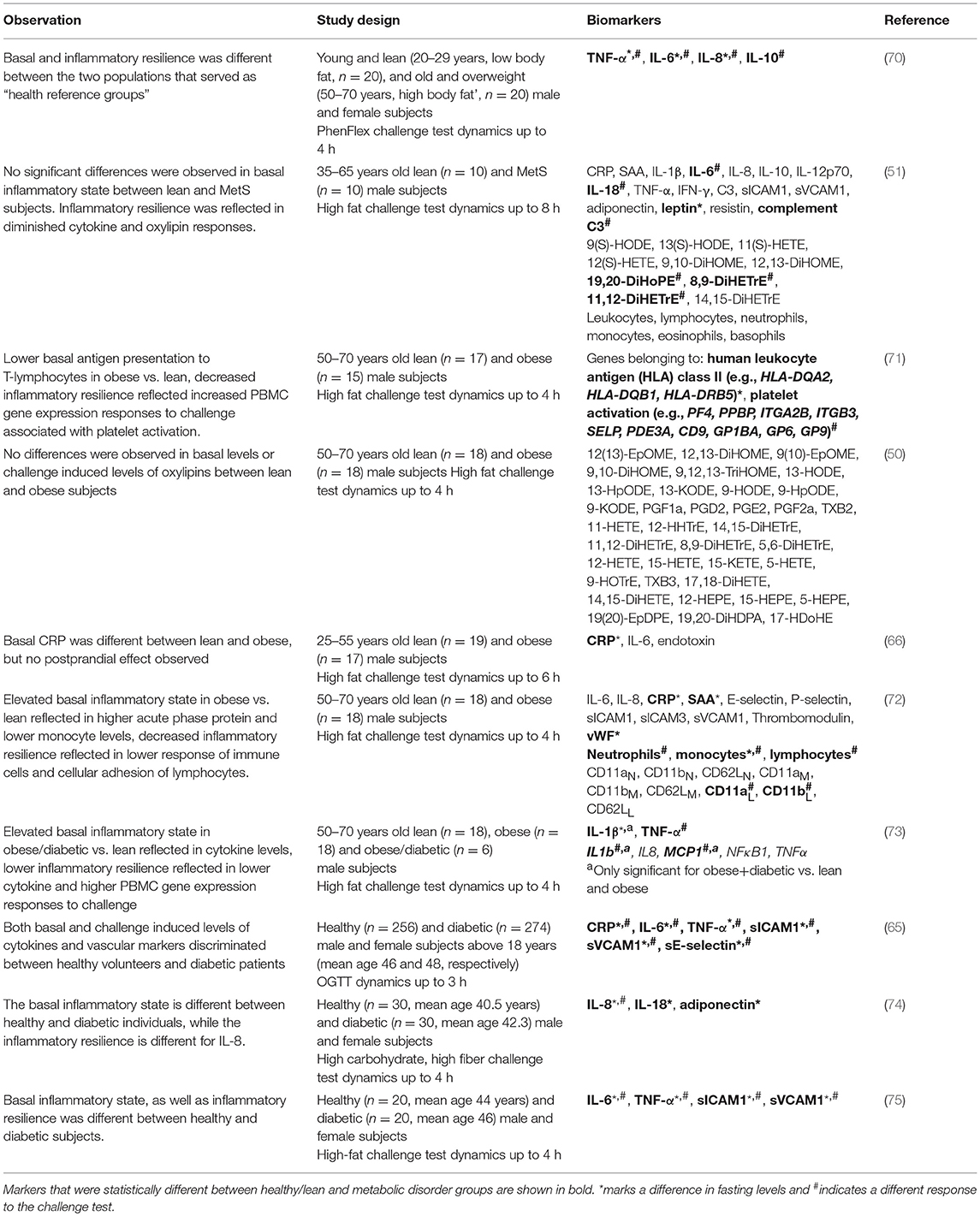
Table 2. Metabolic challenge tests to diagnose chronic low-grade inflammation in metabolic disorders.
Altogether, the application of a challenge test provides richer results contributing to a more comprehensive diagnosis of chronic low-grade inflammation in type 2 diabetes, MetS, and obesity.
Measuring Phenotypic Flexibility to Demonstrate the Beneficial Effects of Interventions on Low-Grade Chronic Inflammation
Ultimately, it is desirable to develop nutritional intervention strategies to impact an individual's health status toward a healthier phenotype (59). Epidemiological studies have shown associations of improved nutritional habits with reduced inflammation, including a high unsaturated/saturated dietary fat balance, low dietary carbohydrates, dietary fibers, probiotics, vitamins, as well as flavonoids (8, 28, 52, 77–80). Other interventions, such as dietary supplements and weight loss can also significantly reduce levels of inflammatory markers (79, 81–83). Several studies have identified novel biomarkers that represent nutrient-induced modulation of inflammation indicating an anti-inflammatory effect (9, 53, 79, 84, 85). However, the subtle and presumably chronic effects of nutrition limit the substantiation of positive health effects in randomized clinical trials, regardless of the epidemiological and mechanistic evidence (86). Here, we summarize the current findings from applying metabolic challenge tests for evaluation of nutritional effects focusing on dynamics of inflammation (Table 3).
- An anti-inflammatory dietary mixture (AIDM) containing resveratrol, green tea extract, α-tocopherol, Vitamin C, n3-poly-unsaturated fatty acids and tomato extract showed improvement of inflammatory resilience in a 5-week randomized, double-blind crossover trial. Although fasting levels of sVCAM-1 and IL-18 decreased with the intervention, other cytokines, vascular markers, blood-clotting factors, and immune markers were found reduced post-prandially only (67, 79).
- Dark chocolate consumption was found having anti-inflammatory effects in a 4-week randomized clinical trial, which was especially visible in the reduced post-challenge responses of cytokines, vascular markers, white blood-cells and leukocyte-activation markers (87).
- Overfeeding of healthy males in a 4-week double-blind crossover study led to disturbed post-challenge dynamics of cytokine markers, white blood cells, vascular markers, and oxylipin markers (51). Interestingly, the inflammatory resilience phenotype of the overfed healthy males moved toward that of males diagnosed with MetS (51).
- Wholegrain vs. refined wheat intervention in a 13-week randomized, double-blind trial showed a beneficial effect on the post-prandial responses of acute phase proteins and cytokines, but not on their fasting levels (70).
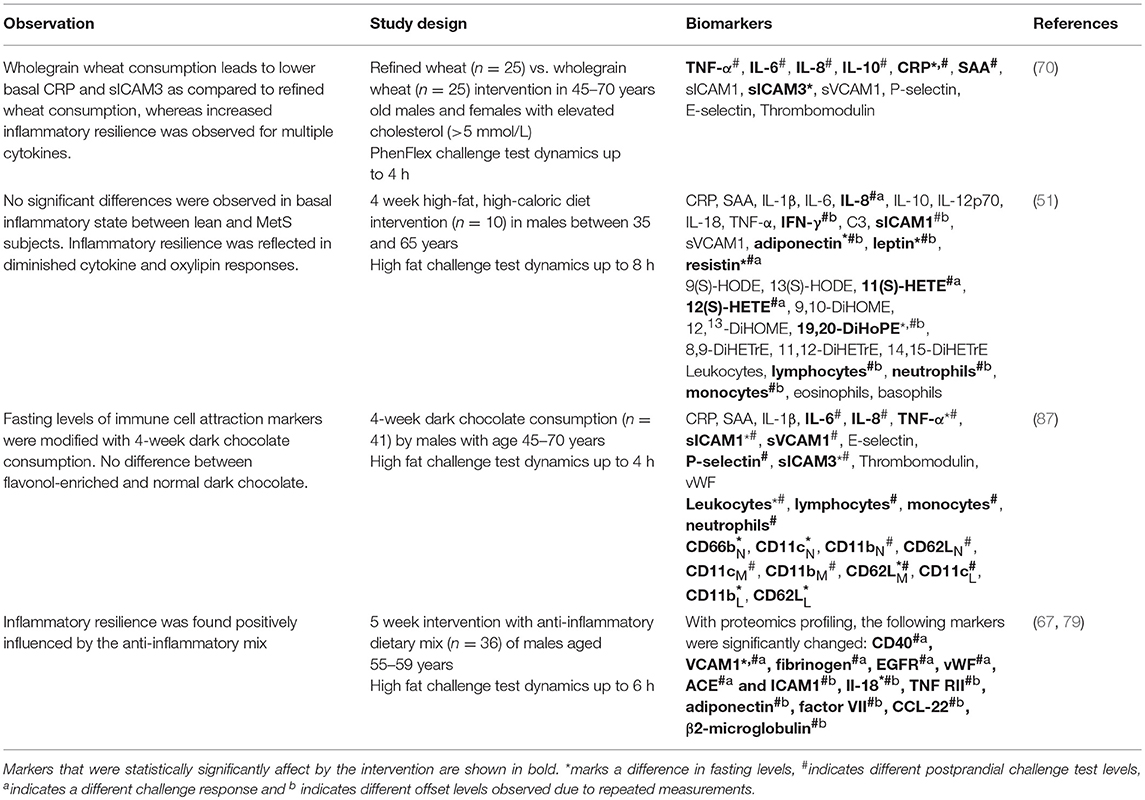
Table 3. Metabolic challenge test to evaluate nutritional effects on chronic low-grade inflammation.
Altogether, only a few studies have employed a metabolic challenge test to evaluate the effects of nutritional interventions on inflammatory status. They indicate that post-challenge effects may reveal intervention effects on the adaptive and regulatory processes that derail early in the progression toward an unhealthy phenotype. Future applications of metabolic challenges are envisioned to increase insight into dynamics of inflammation to reveal intervention effects on a state of low-grade chronic inflammation.
Strategies to Improve Inflammatory Dynamics
As mentioned previously, a metabolic challenge test quantifies low-grade chronic inflammation and nutritional intervention effects more precisely than a classical overnight fasting assay. Ideally, an inflammatory challenge test, e.g., by providing volunteers a low dose of bacterial liposaccharides (LPS) or an attenuated infection such as diarrheagenic E. coli, may be even better, but practical and ethical objections limit its use in human studies. However, such an inflammatory challenge test in model animals is feasible, and for example, IL1-β injection as an inflammatory challenge test has been used in mouse studies to study nutritional intervention effects on inflammation. Concomitant to the human intervention study, a 6-weeks high fat intervention study using humanCRP transgenic mice, where the AIDM was responsible for a strong decrease of the Il-1β induced humanCRP and fibrinogen as compared to control (Figure 6) (88). A 16-week exposure of female ApoE3Leiden mice on a high-fat diet with AIDM resulted in an almost complete (96%) reduction of atherosclerotic plaques as compared to the control mice on a high-fat diet (88).
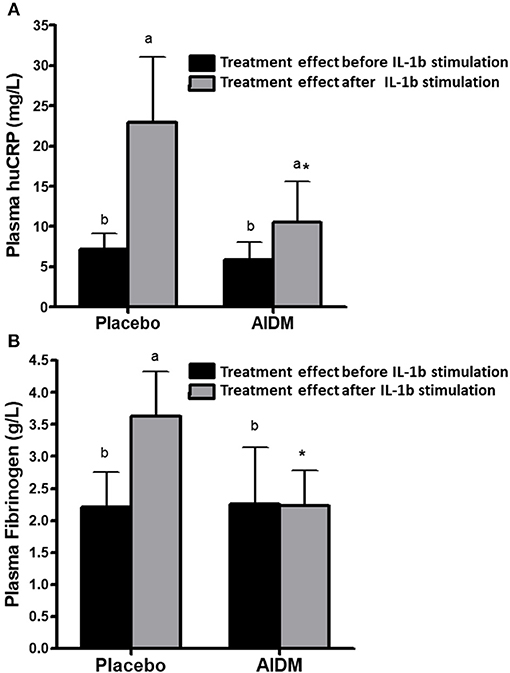
Figure 6. The effect of an “anti-inflammation dietary mixture” (AIDM) vs. placebo quantified at fasting and after Il-1B stimulation on humanCRP (A) and fibrinogen (B) in transgenic mice. Mice were fed the AIDM and the inflammation markers were quantified after 6 weeks of treatment. The effects of AIDM vs. placebo were only visible after IL1B stimulation and not at fasting, showing the added value of applying a perturbation test to show treatment effects on inflammation. Adapted with permission from Verschuren et al. (88).
There may also be strategies other than static nutritional interventions focusing on optimizing inflammatory dynamics to reverse or prevent low-grade inflammation. Training of the immune system is a widely accepted biological phenomenon (89–91). The intimate linkage of metabolic pathways and immunity together with the recent progress in our understanding of stress responses as training suggest that metabolism must be trained too to function optimally and flexible. Biological systems which depend on adaptation for their defense, seem to profit from alternating exposure such as low/high dose, intermittent fasting, glucose/fat cycling and pulse exposure (92, 93). For instance, an alternating high-fat dietary regimen reduced systemic insulin resistance, hepatic and renal inflammation, atherosclerosis and improved renal function (92, 94), as well as increased protein content and mitochondrial function in skeletal muscle (95). Although these studies were performed in mice and rats, there is potential for translation to humans given that the processes involved overlap across these mammalian species. An overview of potential ingredients and strategies for training of metaflammation is shown in Figure 7. An effective immune response is characterized by a sequence of counteracting pro- and anti-inflammatory signals that are properly orchestrated to have adequate onset, optimal response, and decay. Each time these mechanisms are challenged by an external trigger (e.g., infections, LPS, extreme physical activity, cellular oxidative stress, inflammatory lipids, …), the immune system is trained to deal with future challenges. This implicates a memory function that is not only observed in the adaptive immune system (which generates memory for specific triggers), but also in the innate immune system, generating a less specific variant of memory referred to as “trained immunity” or “innate immune memory” (89). There are recent indications that the innate immune cells such as monocytes, macrophages, or natural killer cells may be trained. Trained immune cells increase responsiveness upon secondary stimulation by microbial pathogens by expression of so-called pattern recognition receptors (PRRs) that recognize so-called pathogen-associated molecular pattern (PAMPs), such as LPS, flagellin, β-glucan, and lipoteichoic acid (LTA). Initial stimulation with these PAMPs influence long-term production of inflammatory mediators inducing a shift from oxidative phosphorylation toward aerobic glycolysis (the Warburg effect) (89, 96–98). Trained immunity (not to be confused with acute and short-term adaptation mechanisms, such as tachyphylaxis) seems to be a main immunological process leading to non-specific protective effects against infections that persist for weeks to months, however when not properly activated, trained immunity can cause a dysbalanced immune system as in immune paralysis in sepsis, hyperinflammation in tissues, or atherosclerosis (99).
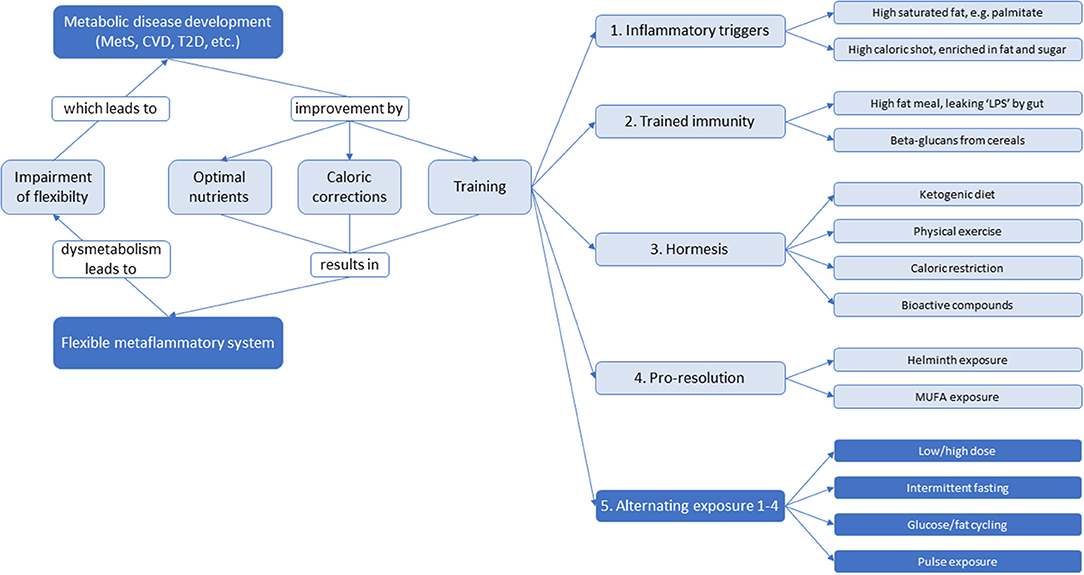
Figure 7. An overview of potential ingredients and strategies for systemic low-grade inflammation therapy to optimize dynamics of inflammation and to maintain a flexible metabolic-inflammatory system. With impairment of flexibility, the system will develop toward metabolic disease development. This can be returned via static nutritional strategies by optimizing nutrients and calories, as well as training strategies through inflammatory triggers, trained immunity, hormesis, pro-resolution, or an alternating combination thereof.
A molecular basis for trained immunity is believed to be an epigenetic-metabolic interplay (89, 98). Epigenetic mechanisms control gene expression via DNA methylation, chromatin remodeling, and microRNA-regulated transcriptional silencing. Cellular metabolites, such as acetyl-CoA, α-ketoglutarate, and succinate act as a cofactor on epigenetic enzymes influencing the functionality of epigenetic mechanisms (89, 96). Indeed, trained immunity seems dependent on the metabolic status of an individual. For example, mevalonate, a precursor of the cholesterol synthesis pathway, was demonstrated to induce trained immunity by epigenetic reprogramming and increased mevalonate levels were associated with higher cytokine levels, while reduction of mevalonate by statins resulted in lower cytokine levels (100).
Although the field of “immunometabolism” has emerged where the relationship between metabolism and trained innate immunity are becoming more mechanistically clear (89), at this stage it is not known for certain whether this training aspect is valid only for external inflammatory triggers (infections, etc.) or for metabolism triggered inflammation and metabolic health/flexibility as well. One could speculate that metabolic triggers known to cause a mild acute inflammatory response improve inflammation dynamics via such mechanism. It is known that saturated fat, especially palmitate (10, 101), high-fat meals (74), calorie-dense meals (66) and meals enriched in fat and glucose (68) all cause an acute inflammatory trigger in healthy individuals. On the other hand, it is also known that diets enriched in mono-unsaturated fat result in reduced postprandial activity of transcription factor NF-kB, protein levels of nuclear p65 and TNF-α (77). It will be interesting to see whether well-scheduled consumption of these type of meals as an inflammatory trigger may improve long-term inflammatory status.
Training aspects have also been described for a process referred to as hormesis. Hormesis is the adaptive reaction of a biological system to external stresses to improve its resilience and tolerance to stronger stresses in the future (90, 102). This involves the observations that low doses of chemicals that plants make as a defense to ward off insects, consumed by humans in low levels via fruit and vegetables, are associated with health benefits. A well-known example is represented by the phytochemical resveratrol. At low doses (2.5 and 5 mg/kg) a reduction of myocardial infarction was seen in rats upon 14 days of consecutive administration, while at high doses (25 and 50 mg/kg), an increase was observed (103). In vitro and ex vivo studies highlight multiple pathways for which hormesis has been observed in case of resveratrol, including inflammatory pathways (103, 104). Indeed, the secretion of IFN-γ, IL-2, and IL-4 from stimulated human peripheral blood mononuclear cells (PBMCs) demonstrated a clear bi-phasic pattern with increasing doses of resveratrol (104). These apparently strange findings illustrate a highly interesting principle. Relatively low concentrations of anti-inflammatory compounds might actually induce the inflammatory capacity by their pro-inflammatory action, by creating alertness toward these foreign compounds. Thus, instead of a continuous relaxation and “high-dose drug-like” anti-inflammatory condition, these low dose (and often intermittent concentrations) of foreign compounds create a “first line of defense” barrier of inflammation dynamics.
Although this line of research has not yet been thoroughly investigated, numerous anecdotal reports describe the pro- and anti-inflammatory (= hormesis) action of natural compounds (90). For example, livestock in Europe greatly benefitted from inflammatory hormesis by providing multiple mixtures of natural compounds upon the recent ban of antibiotics (105).
These mild stresses from natural compounds induce a memory or training by adapting mammalian physiology to increase their capacity to deal with higher amounts of stress. Other well-known examples of hormesis include calorie-restriction, exercise, and glucose restriction all resulting in longevity in model systems, suggesting that mild induction of oxidative stress within the mitochondria causes an adaptive response that results in increased stress resistance and long-term reduction of oxidative stress (106). Hormesis seems to be induced via epigenetic regulation, which can occur not only during early developmental stages but also through adulthood (107). From a molecular point of view, trained immunity and hormesis may thus be similar stimuli to the immune system. In this hormesis context, the so-called epigenetic diet has been proposed, which introduces bioactive compounds such as genistein, sulforaphane, tea polyphenols, curcumin, resveratrol, vitamins, and minerals that have been demonstrated to act through an epigenetic mechanism resulting in restoration of immune homeostasis thereby supporting healthy aging (108, 109).
A final aspect to consider in the promotion of metaflammation is to stimulate a pro-resolution (Th2 and/or T regulatory) response by changing the environmental hygiene by exposure to pathogens or their products. There is evidence that exposure to pathogens (bacteria, viruses, and helminths) is critical to promote normal immune development, which is reflected in the so-called (revised) hygiene hypothesis (91, 110). Parasitic helminths have been shown to modulate the host immune system and induce responses of Th2 and regulatory immune cells (111, 112). Since pro-inflammatory immune responses play a key role in the development of lifestyle-related diseases such as type 2 diabetes, helminth-induced immunomodulation through controlled infections with helminths or helminth-derived molecules has been investigated and shown to be promising to improve metabolic outcomes in mice (111–115), although at this stage there is very little human data available. Studies indicate that helminths may improve dynamics of inflammation, at least partly, by increasing the number of anti-inflammatory immune cells (Th2 cells, eosinophils, M2 macrophages, Tregs, and ILC2s) in adipose tissue, thereby reducing inflammation and improving glucose tolerance [reviewed by de Ruiter et al. (112)]. Besides changes in adipose tissue, the cellular hepatic composition appeared to be affected by helminth products (113). Furthermore, the helminth-induced increase in ILC2, eosinophils and M2 macrophages may result in browning of white adipose tissue, generating “beige” cells that increase energy expenditure, thereby decreasing adiposity, which may prevent the development of insulin resistance (111, 114, 115). Besides stimulating an anti-inflammatory immune response, helminth infections have been shown in an experimental setting to induce weight loss in mice with diet-induced obesity by impairing glucose absorption (116) or to suppress adipogenesis, thereby preventing the onset of MetS including diabetes (111, 116). In humans, epidemiological cross-sectional studies suggest that chronic helminth infections may prevent development of metabolic diseases and that these effects can be long-lasting [reviewed by de Ruiter et al. (112)]. Longitudinal studies are now required to provide information on the causal relationship between helminths and prevention of metabolic diseases.
Conclusion
In conclusion, metabolic inflammation should be quantified as a systemic dynamic process. We have presented a state-of-the-art overview of methods to evaluate the inflammatory dynamics of individuals by applying metabolic challenge tests. These dynamics can be optimized by dietary and other interventions, i.e., by exposure to nutrients that improve inflammatory resilience. The first nutritional intervention studies have been published that showed that inflammatory dynamics can be improved (see Table 3), and we envision the next step that focusses on strategies that (re)gain inflammatory flexibility. Literature is providing more and more indications that the inflammatory response may be trained by regular mild inflammatory, as well as metabolic triggers, regulated through epigenetic and metabolic modifications. By combining aspects of metabolic and inflammatory triggers that introduce mild inflammation with knowledge on trained immunity, hormesis and pro-resolution, new strategies to optimize dynamics of inflammation may become available.
Disclosure
The work was sponsored by Johnson & Johnson Family of Consumer Companies. The sponsor had no input on the design, data, or conclusions of the study.
Author Contributions
SW, BvO, and WvdB contributed conception of the review. WvdB, JvB, KS, FH, LV, RK, BvO, and SW wrote sections of the manuscript. All authors contributed to manuscript revision, read and approved the submitted version.
Conflict of Interest Statement
GR, an employee of Janssen Research and Development, LLC, contributed to the revision, read and approved the manuscript, but had no role in the conceptualization and writing of the manuscript.
The remaining authors declare that the research was conducted in the absence of any commercial or financial relationships that could be construed as a potential conflict of interest.
Acknowledgments
We would like to acknowledge Carina de Jong-Rubingh and Nynke van Berkum for coordinating the project, and Frank Schuren, Valeria Agamennone, Anita van den Hoek for the fruitful discussions on the content of this review, and Andrew Bedard for the critical textual revision of the manuscript.
Abbreviations
OGTT, oral glucose tolerance test; MetS, metabolic syndrome; AIDM, anti-inflammatory dietary mixture; LPS, lipopolysaccharide; PBMC, peripheral blood mononuclear cell.
References
1. Pischon T, Nimptsch K. Obesity and Cancer. Schlag P, Senn Cham H, editors. Berlin: Springer International Publishing (2016).
2. Kaluza J, Håkansson N, Harris HR, Orsini N, Michaëlsson K, Wolk A. Influence of anti-inflammatory diet and smoking on mortality and survival in men and women: two prospective cohort studies. J Intern Med. (2019) 285:75–91. doi: 10.1111/joim.12823
3. Guariguata L, Whiting DR, Hambleton I, Beagley J, Linnenkamp U, Shaw JE. Global estimates of diabetes prevalence for 2013 and projections for 2035. Diabetes Res Clin Pract. (2014) 103:137–49. doi: 10.1016/j.diabres.2013.11.002
4. Oggioni C, Lara J, Wells JCK, Soroka K, Siervo M. Shifts in population dietary patterns and physical inactivity as determinants of global trends in the prevalence of diabetes: An ecological analysis. Nutr Metab Cardiovasc Dis. (2014) 24:1105–11. doi: 10.1016/j.numecd.2014.05.005
5. Hotamisligil GS. Inflammation, metaflammation and immunometabolic disorders. Nature. (2017) 542:177–85. doi: 10.1038/nature21363
6. Gregor MF, Hotamisligil GS. Inflammatory mechanisms in obesity. Annu Rev Immunol. (2011) 29:415–45. doi: 10.1146/annurev-immunol-031210-101322
7. Hotamisligil GS. Inflammation and metabolic disorders. Nature. (2006) 444:860–7. doi: 10.1038/nature05485
8. Minihane AM, Vinoy S, Russell WR, Baka A, Roche HM, Tuohy KM, et al. Low-grade inflammation, diet composition and health: current research evidence and its translation. Br J Nutr. (2015) 114:999–1012. doi: 10.1017/S0007114515002093
9. Calder PC, Ahluwalia N, Albers R, Bosco N, Haller D, Holgate ST, et al. A consideration of biomarkers to be used for evaluation of inflammation in human nutritional studies. Br J Nutr. (2013) 109:S1–34. doi: 10.1017/S0007114512005119
10. Stroeve JHM, van Wietmarschen H, Kremer BHA, van Ommen B, Wopereis S. Phenotypic flexibility as a measure of health: the optimal nutritional stress response test. Genes Nutr. (2015) 10:13. doi: 10.1007/s12263-015-0459-1
11. Chawla A, Nguyen DK, Goh YPS. Macrophage-mediated inflammation in metabolic disease. Nat Rev Immunol. (2012) 11:738–49. doi: 10.1038/nri3071
12. Cipolletta D, Feuerer M, Li A, Kamei N, Lee J, Shoelson E, et al. PPARγ is a major driver of the accumulation and phenotype of adipose-tissue Treg cells. Nature. (2012) 486:549–53. doi: 10.1038/nature11132
13. Ortega-Gómez A, Perretti M, Soehnlein O. Resolution of inflammation: an integrated view. EMBO Mol Med. (2013) 5:661–74. doi: 10.1002/emmm.201202382
14. Maitra U, Deng H, Glaros T, Baker B, Capelluto DGS, Li Z, et al. Molecular mechanisms responsible for the selective and low-grade induction of proinflammatory mediators in murine macrophages by lipopolysaccharide. J Immunol. (2012) 189:1014–23. doi: 10.4049/jimmunol.1200857
15. Nathan C, Ding A. Nonresolving inflammation. Cell. (2010) 140:871–82. doi: 10.1016/j.cell.2010.02.029
16. Macdougall CE, Wood EG, Loschko J, Scagliotti V, Cassidy FC, Robinson ME, et al. Visceral adipose tissue immune homeostasis is regulated by the crosstalk between adipocytes and dendritic cell subsets. Cell Metab. (2018) 27:588–601.e4. doi: 10.1016/j.cmet.2018.02.007
17. Villeneuve DL, Landesmann B, Allavena P, Ashley N, Bal-Price A, Corsini E, et al. Representing the process of inflammation as key events in adverse outcome pathways. Toxicol Sci. (2018) 163:1–7. doi: 10.1093/toxsci/kfy047
18. Ralston JC, Lyons CL, Kennedy EB, Kirwan AM, Roche HM. Fatty acids and NLRP3 inflammasome–mediated inflammation in metabolic tissues. Annu Rev Nutr. (2017) 37:77–102. doi: 10.1146/annurev-nutr-071816-064836
19. Agrawal N, Delanoue R, Mauri A, Basco D, Pasco M, Thorens B, et al. The drosophila TNF eiger is an adipokine that acts on insulin-producing cells to mediate nutrient response. Cell Metab. (2016) 23:675–84. doi: 10.1016/j.cmet.2016.03.003
20. Benetti E, Chiazza F, Patel NSA, Collino M. The NLRP3 inflammasome as a novel player of the intercellular crosstalk in metabolic disorders. Mediators Inflamm. (2013) 2013:678627. doi: 10.1155/2013/678627
21. Nishimura S, Manabe I, Nagasaki M, Eto K, Yamashita H, Ohsugi M, et al. CD8+ effector T cells contribute to macrophage recruitment and adipose tissue inflammation in obesity. Nat Med. (2009) 15:914–21. doi: 10.1038/nm.1964
22. Duffaut C, Galitzky J, Lafontan M, Bouloumié A. Unexpected trafficking of immune cells within the adipose tissue during the onset of obesity. Biochem Biophys Res Commun. (2009) 384:482–5. doi: 10.1016/j.bbrc.2009.05.002
23. Kintscher U, Hartge M, Hess K, Foryst-Ludwig A, Clemenz M, Wabitsch M, et al. T-lymphocyte infiltration in visceral adipose tissue: a primary event in adipose tissue inflammation and the development of obesity-mediated insulin resistance. Arterioscler Thromb Vasc Biol. (2008) 28:1304–10. doi: 10.1161/ATVBAHA.108.165100
24. Deng T, Lyon CJ, Minze LJ, Lin J, Zou J, Liu JZ, et al. Class II major histocompatibility complex plays an essential role in obesity-induced adipose inflammation. Cell Metab. (2013) 17:411–22. doi: 10.1016/j.cmet.2013.02.009
25. Lord GM, Matarese G, Howard JK, Baker RJ, Bloom SR, Lechler RI. Leptin modulates the T-cell immune response and reverses starvation-induced immunosuppression. Nature. (1998) 394:897–901. doi: 10.1038/29795
26. Saltiel, Alan R, Olefsky JM. Inflammatory mechanisms linking obesity and metabolic disease. J Clin Invest. (2017) 127:1–4. doi: 10.1172/JCI92035
27. Pereira SS, Alvarez-Leite JI. Low-grade inflammation, obesity, and diabetes. Curr Obes Rep. (2014) 3:422–31. doi: 10.1007/s13679-014-0124-9
28. Rogero MM, Calder PC. Obesity, inflammation, toll-like receptor 4 and fatty acids. Nutrients. (2018) 10:1–19. doi: 10.3390/nu10040432
29. Nguyen MTA, Favelyukis S, Nguyen AK, Reichart D, Scott PA, Jenn A, et al. A subpopulation of macrophages infiltrates hypertrophic adipose tissue and is activated by free fatty acids via toll-like receptors 2 and 4 and JNK-dependent pathways. J Biol Chem. (2007) 282:35279–92. doi: 10.1074/jbc.M706762200
30. Kamada N, Seo S-U, Chen GY, Núñez G. Role of the gut microbiota in immunity and inflammatory disease. Nat Rev Immunol. (2013) 13:321. doi: 10.1038/nri3430
31. Rivas M, Sosa-Estani S, Rangel J, Caletti MG, Vallés P, Roldán CD, et al. Risk factors for sporadic shiga toxin–producing Escherichia coli infections in children, Argentina. Emerg Infect Dis. (2008) 14:763–71. doi: 10.3201/eid1405.071050
32. Zeng MY, Inohara N, Nuñez G. Mechanisms of inflammation-driven bacterial dysbiosis in the gut. Mucosal Immunol. (2017) 10:18–26. doi: 10.1038/mi.2016.75
33. Burcelin R. Gut microbiota and immune crosstalk in metabolic disease. Mol Metab. (2016) 5:771–81. doi: 10.1016/j.molmet.2016.05.016
34. Koyama Y, Brenner DA. Liver inflammation and fibrosis. J Cinical Investig. (2017) 127:55–64. doi: 10.1172/JCI88881
35. Yoo HJ, Choi KM. Hepatokines as a link between obesity and cardiovascular diseases. Diabetes Metab J. (2015) 39:10–15. doi: 10.4093/dmj.2015.39.1.10
36. van Herpen NA, Schrauwen-Hinderling VB. Lipid accumulation in non-adipose tissue and lipotoxicity. Physiol Behav. (2008) 94:231–41. doi: 10.1016/j.physbeh.2007.11.049
37. Wu H, Ballantyne CM. Skeletal muscle inflammation and insulin resistance in obesity. J Clin Invest. (2017) 127:43–54. doi: 10.1172/JCI88880
38. Hoffmann C, Weigert C. Skeletal muscle as an endocrine organ: the role of myokines in exercise adaptations. Cold Spring Harb Perspect Med. (2017) 7:a029793. doi: 10.1101/cshperspect.a029793
39. Kleemann R, Verschuren L, van Erk MJ, Nikolsky Y, Cnubben NHP, Verheij ER, et al. Atherosclerosis and liver inflammation induced by increased dietary cholesterol intake: a combined transcriptomics and metabolomics analysis. Genome Biol. (2007) 8:R200. doi: 10.1186/gb-2007-8-9-r200
40. Liang W, Lindeman JH, Menke AL, Koonen DP, Morrison M, Havekes LM, et al. Metabolically induced liver inflammation leads to NASH and differs from LPS- or IL-1β-induced chronic inflammation. Lab Investig. (2014) 94:491. doi: 10.1038/labinvest.2014.11
41. Chávez-Talavera O, Tailleux A, Lefebvre P, Staels B. Bile acid control of metabolism and inflammation in obesity, type 2 diabetes, dyslipidemia, and nonalcoholic fatty liver disease. Gastroenterology. (2017) 152:1679–94.e3. doi: 10.1053/j.gastro.2017.01.055
42. Janssen AWF, Houben T, Katiraei S, Dijk W, Boutens L, van der Bolt N, et al. Modulation of the gut microbiota impacts nonalcoholic fatty liver disease: a potential role for bile acids. J Lipid Res. (2017) 58:1399–416. doi: 10.1194/jlr.M075713
43. Macpherson AJ, Heikenwalder M, Ganal-Vonarburg SC. The liver at the nexus of host-microbial interactions. Cell Host Microbe. (2016) 20:561–71. doi: 10.1016/j.chom.2016.10.016
44. Mulder P, Morrison MC, Wielinga PY, van Duyvenvoorde W, Kooistra T, Kleemann R. Surgical removal of inflamed epididymal white adipose tissue attenuates the development of non-alcoholic steatohepatitis in obesity. Int J Obes. (2016) 40:675–84. doi: 10.1038/ijo.2015.226
45. Item F, Konrad D. Visceral fat and metabolic inflammation: the portal theory revisited. Obes Rev. (2012) 13:30–9. doi: 10.1111/j.1467-789X.2012.01035.x
46. Dam V, Sikder T, Santosa S. From neutrophils to macrophages: differences in regional adipose tissue depots. Obes Rev. (2016) 17:1–17. doi: 10.1111/obr.12335
47. Gluchowski NL, Becuwe M, Walther TC, Farese RV. Lipid droplets and liver disease: from basic biology to clinical implications. Nat Rev Gastroenterol Hepatol. (2017) 14:343–55. doi: 10.1038/nrgastro.2017.32
48. Ramsden CE, Zamora D, Majchrzak-Hong S, Faurot KR, Broste SK, Frantz RP, et al. Re-evaluation of the traditional diet-heart hypothesis: Analysis of Recovered data from Minnesota Coronary Experiment (1968-73). BMJ. (2016) 353:i1246. doi: 10.1136/bmj.i1246
49. Mulder P, Liang W, Wielinga PY, Verschuren L, Toet K, Havekes LM, et al. Macrovesicular steatosis is associated with development of lobular inflammation and fibrosis in diet-induced non-alcoholic steatohepatitis (NASH). Inflamm Cell Signal. (2015) 2:e804. doi: 10.14800/ics.804
50. Strassburg K, Esser D, Vreeken RJ, Hankemeier T, Müller M, van Duynhoven J, et al. Postprandial fatty acid specific changes in circulating oxylipins in lean and obese men after high-fat challenge tests. Mol Nutr Food Res. (2014) 58:591–600. doi: 10.1002/mnfr.201300321
51. Kardinaal AFM, Van Erk MJ, Dutman AE, Stroeve JHM, Van De Steeg E, Bijlsma S, et al. Quantifying phenotypic flexibility as the response to a high-fat challenge test in different states of metabolic health. FASEB J. (2015) 29:4600–13. doi: 10.1096/fj.14-269852
52. Calder PC, Ahluwalia N, Brouns F, Buetler T, Clement K, Cunningham K, et al. Dietary factors and low-grade inflammation in relation to overweight and obesity. Br J Nutr. (2011) 106:S5–78. doi: 10.1017/S0007114511005460
53. Albers R, Bourdet-Sicard R, Braun D, Calder PC, Herz U, Lambert C, et al. Monitoring immune modulation by nutrition in the general population: identifying and substantiating effects on human health. Br J Nutr. (2013) 110:1–30. doi: 10.1017/S0007114513001505
54. Morrison M, van der Heijden R, Heeringa P, Kaijzel E, Verschuren L, Blomhoff R, et al. Epicatechin attenuates atherosclerosis and exerts anti-inflammatory effects on diet-induced human-CRP and NFκB in vivo. Atherosclerosis. (2014) 233:149–56. doi: 10.1016/j.atherosclerosis.2013.12.027
55. Netzer N, Gatterer H, Faulhaber M, Burtscher M, Pramsohler S, Pesta D. Hypoxia, oxidative stress and fat. Biomolecules. (2015) 5:1143–50. doi: 10.3390/biom5021143
56. Marseglia L, Manti S, D'Angelo G, Nicotera A, Parisi E, Di Rosa G, et al. Oxidative stress in obesity: a critical component in human diseases. Int J Mol Sci. (2015) 16:378–400. doi: 10.3390/ijms16010378
57. Montgomery MK, Turner N. Mitochondrial dysfunction and insulin resistance: an update. Endocr Connect. (2014) 4:R1–15. doi: 10.1530/EC-14-0092
58. Salminen A, Ojala J, Kaarniranta K, Kauppinen A. Mitochondrial dysfunction and oxidative stress activate inflammasomes: impact on the aging process and age-related diseases. Cell Mol Life Sci. (2012) 69:2999–3013. doi: 10.1007/s00018-012-0962-0
59. van Ommen B, van den Broek T, de Hoogh I, van Erk M, van Someren E, Rouhani-Rankouhi T, et al. Systems biology of personalized nutrition. Nutr Rev. (2017) 75:579–99. doi: 10.1093/nutrit/nux029
60. van den Broek TJ, Bakker GCM, Rubingh CM, Bijlsma S, Stroeve JHM, van Ommen B, et al. Ranges of phenotypic flexibility in healthy subjects. Genes Nutr. (2017) 12:1–14. doi: 10.1186/s12263-017-0589-8
61. van Ommen B, van der Greef J, Ordovas JM, Daniel H. Phenotypic flexibility as key factor in the human nutrition and health relationship. Genes Nutr. (2014) 9:423. doi: 10.1007/s12263-014-0423-5
62. Huber M, André Knottnerus J, Green L, Van Der Horst H, Jadad AR, Kromhout D, et al. How should we define health? BMJ. (2011) 343:1–3. doi: 10.1136/bmj.d4163
63. van Ommen B, Keijer J, Heil SG, Kaput J. Challenging homeostasis to define biomarkers for nutrition related health. Mol Nutr Food Res. (2009) 53:795–804. doi: 10.1002/mnfr.200800390
64. van der Greef J, van Wietmarschen H, van Ommen B, Verheij E. Looking back into the future: 30 years of metabolomics at TNO. Mass Spectrom Rev. (2013) 32:399–415. doi: 10.1002/mas.21370
65. Derosa G, D'Angelo A, Salvadeo SAT, Ferrari I, Fogari E, Gravina A, et al. Oral glucose tolerance test effects on endothelial inflammation markers in healthy subjects and diabetic patients. Horm Metab Res. (2010) 42:8–13. doi: 10.1055/s-0029-1237728
66. Schwander F, Kopf-Bolanz KA, Buri C, Portmann R, Egger L, Chollet M, et al. A dose-response strategy reveals differences between normal-weight and obese men in their metabolic and inflammatory responses to a high-fat meal. J Nutr. (2014) 144:1517–23. doi: 10.3945/jn.114.193565
67. Pellis L, van Erk MJ, van Ommen B, Bakker GCM, Hendriks HFJ, Cnubben NHP, et al. Plasma metabolomics and proteomics profiling after a postprandial challenge reveal subtle diet effects on human metabolic status. Metabolomics. (2012) 8:347–59. doi: 10.1007/s11306-011-0320-5
68. Wopereis S, Wolvers D, Van Erk M, Gribnau M, Kremer B, Van Dorsten FA, et al. Assessment of inflammatory resilience in healthy subjects using dietary lipid and glucose challenges. BMC Med Genomics. (2013) 6:44. doi: 10.1186/1755-8794-6-44
69. Wopereis S, Stroeve JHM, Stafleu A, Bakker GCM, Burggraaf J, van Erk MJ, et al. Multi-parameter comparison of a standardized mixed meal tolerance test in healthy and type 2 diabetic subjects: the PhenFlex challenge. Genes Nutr. (2017) 12:1–14. doi: 10.1186/s12263-017-0570-6
70. Hoevenaars FPM, Esser D, Schutte S, Priebe MG, Vonk RJ, van den Brink WJ, et al. Whole grain wheat consumption affects post-prandial inflammatory response in a randomized controlled trial in overweight and obese adults with mild hypercholesterolemia in the Graandioos study. (accepted). doi: 10.1093/jn/nxz177
71. Esser D, van Dijk SJ, Oosterink E, Lopez S, Müller M, Afman LA. High fat challenges with different fatty acids affect distinct atherogenic gene expression pathways in immune cells from lean and obese subjects. Mol Nutr Food Res. (2015) 59:1563–72. doi: 10.1002/mnfr.201400853
72. Esser D, van Dijk SJ, Oosterink E, Muller M, Afman LA. A high-fat SFA, MUFA, or n3 PUFA challenge affects the vascular response and initiates an activated state of cellular adherence in lean and obese middle-aged men. J Nutr. (2013) 143:843–51. doi: 10.3945/jn.113.174540
73. van Dijk SJ, Mensink M, Esser D, Feskens EJM, Müller M, Afman LA. Responses to high-fat challenges varying in fat type in subjects with different metabolic risk phenotypes: a randomized trial. PLoS ONE. (2012) 7:e41388. doi: 10.1371/journal.pone.0041388
74. Esposito K, Nappo F, Giugliano F, Di Palo C, Ciotola M, Barbieri M, et al. Meal modulation of circulating interleukin 18 and adiponectin concentrations in healthy subjects and in patients with type 2 diabetes mellitus. Am J Clin Nutr. (2003) 78:1135–40. doi: 10.1093/ajcn/78.6.1135
75. Nappo F, Esposito K, Cioffi M, Giugliano G, Molinari AM, Paolisso G, et al. Postprandial endothelial activation in healthy subjects and in type 2 diabetic patients: role of fat and carbohydrate meals. J Am Coll Cardiol. (2002) 39:1145–50. doi: 10.1016/S0735-1097(02)01741-2
76. Calder PC, Bosco N, Bourdet-Sicard R, Capuron L, Delzenne N, Doré J, et al. Health relevance of the modification of low grade inflammation in ageing (inflammageing) and the role of nutrition. Ageing Res Rev. (2017) 40:95–119. doi: 10.1016/j.arr.2017.09.001
77. Cruz-Teno C, Pérez-Martínez P, Delgado-Lista J, Yubero-Serrano EM, García-Ríos A, Marín C, et al. Dietary fat modifies the postprandial inflammatory state in subjects with metabolic syndrome: the LIPGENE study. Mol Nutr Food Res. (2012) 56:854–65. doi: 10.1002/mnfr.201200096
78. Custodero C, Mankowski RT, Lee SA, Chen Z, Wu S, Manini TM, et al. Evidence-based nutritional and pharmacological interventions targeting chronic low-grade inflammation in middle-age and older adults: a systematic review and meta-analysis. Ageing Res Rev. (2018) 46:42–59. doi: 10.1016/j.arr.2018.05.004
79. Bakker GC, van Erk MJ, Pellis L, Wopereis S, Rubingh CM, Cnubben NH, et al. An antiinflammatory dietary mix modulates inflammation and oxidative and metabolic stress in overweight men: a nutrigenomics approach. Am J Clin Nutr. (2010) 91:1044–59. doi: 10.3945/ajcn.2009.28822
80. van den Broek TJ, Kremer BHA, Marcondes Rezende M, Hoevenaars FPM, Weber P, Hoeller U, et al. The impact of micronutrient status on health: correlation network analysis to understand the role of micronutrients in metabolic-inflammatory processes regulating homeostasis and phenotypic flexibility. Genes Nutr. (2017) 12:1–14. doi: 10.1186/s12263-017-0553-7
81. Ahmadi N, Eshaghian S, Huizenga R, Sosnin K, Ebrahimi R, Siegel R. Effects of intense exercise and moderate caloric restriction on cardiovascular risk factors and inflammation. Am J Med. (2011) 124:978–82. doi: 10.1016/j.amjmed.2011.02.032
82. Fazelzadeh P, Hangelbroek RWJ, Joris PJ, Schalkwijk CG, Esser D, Afman L, et al. Weight loss moderately affects the mixed meal challenge response of the plasma metabolome and transcriptome of peripheral blood mononuclear cells in abdominally obese subjects. Metabolomics. (2018) 14:1–13. doi: 10.1007/s11306-018-1328-x
83. Fiamoncini J, Rundle M, Gibbons H, Thomas L, Geillinger-Kästle K, Bunzel D, et al. Plasma metabolome analysis identifies distinct human metabotypes in the postprandial state with different susceptibility to weight loss–mediated metabolic improvements. FASEB J. (2018) 32:5447–58. doi: 10.1096/fj.201800330R
84. Van Erk MJ, Wopereis S, Rubingh C, Van Vliet T, Verheij E, Cnubben NH, et al. Insight in modulation of inflammation in response to diclofenac intervention: a human intervention study. BMC Med Genomics. (2010) 3:5. doi: 10.1186/1755-8794-3-5
85. Rudkowska I, Paradis AM, Thifault E, Julien P, Tchernof A, Couture P, et al. Transcriptomic and metabolomic signatures of an n-3 polyunsaturated fatty acids supplementation in a normolipidemic/normocholesterolemic Caucasian population. J Nutr Biochem. (2013) 24:54–61. doi: 10.1016/j.jnutbio.2012.01.016
86. Grimaldi KA, van Ommen B, Ordovas JM, Parnell LD, Mathers JC, Bendik I, et al. Proposed guidelines to evaluate scientific validity and evidence for genotype-based dietary advice. Genes Nutr. (2017) 12:1–12. doi: 10.1186/s12263-017-0584-0
87. Esser D, Mars M, Oosterink E, Stalmach A, Müller M, Afman LA. Dark chocolate consumption improves leukocyte adhesion factors and vascular function in overweight men. FASEB J. (2014) 28:1464–73. doi: 10.1096/fj.13-239384
88. Verschuren L, Wielinga PY, van Duyvenvoorde W, Tijani S, Toet K, van Ommen B, et al. A Dietary mixture containing fish oil, resveratrol, lycopene, catechins, and vitamins E and C reduces atherosclerosis in transgenic mice. J Nutr. (2011) 141:863–9. doi: 10.3945/jn.110.133751
89. Netea MG, Joosten LAB, Latz E, Mills KHG, Natoli G, Stunnenberg HG, et al. Trained immunity: a program of innate immune memory in health and disease. Sci Transl Med. (2016) 352:aaf1098. doi: 10.1126/science.aaf1098
90. Calabrese EJ. Hormetic dose-response relationships in immunology: Occurrence, quantitative features of the dose response, mechanistic foundations, and clinical implications. Crit Rev Toxicol. (2005) 35:89–295. doi: 10.1080/10408440590917044
91. Stiemsma LT, Reynolds LA, Turvey SE, Finlay BB. The hygiene hypothesis: current perspectives and future therapies. Immunotargets Ther. (2015) 4:143–57. doi: 10.2147/ITT.S61528
92. Wielinga PY, Yakala GK, Heeringa P, Kleemann R, Kooistra T. Beneficial effects of alternate dietary regimen on liver inflammation, atherosclerosis and renal activation. PLoS ONE. (2011) 6:e18432. doi: 10.1371/journal.pone.0018432
93. Varady KA, Bhutani S, Church EC, Klempel MC. Short-term modified alternate-day fasting: a novel dietary strategy for weight loss and cardioprotection in obese adults. Am J Clin Nutr. (2009) 90:1138–43. doi: 10.3945/ajcn.2009.28380
94. Yakala GK, van der Heijden R, Molema G, Schipper M, Wielinga PY, Kleemann R, et al. Beneficial effects of an alternating high- fat dietary regimen on systemic insulin resistance, hepatic and renal inflammation and renal function. PLoS ONE. (2012) 7:e45866. doi: 10.1371/journal.pone.0045866
95. Li X, Higashida K, Kawamura T, Higuchi M. Alternate-day high-fat diet induces an increase in mitochondrial enzyme activities and protein content in rat skeletal muscle. Nutrients. (2016) 8:203. doi: 10.3390/nu8040203
96. Baardman J, Licht I, de Winther MP, Van den Bossche J. Metabolic – epigenetic crosstalk in macrophage activation. Epigenomics. (2015) 7:1155–64. doi: 10.2217/epi.15.71
97. Pearce EL, Pearce EJ. Metabolic pathways in immune cell activation and quiescence. Immunity. (2013) 38:633–43. doi: 10.1016/j.immuni.2013.04.005
98. Saeed S, Quintin J, Kerstens HHD, Rao NA, Aghajanirefah A, Matarese F, et al. Epigenetic programming of monocyte-to-macrophage differentiation and trained innate immunity. Science. (2014) 345:1251086. doi: 10.1126/science.1251086
99. Leentjens J, Bekkering S, Joosten LA, Netea MG, Burgner DP, Riksen NP. Trained innate immunity as a novel mechanism linking infection and the development of atherosclerosis. Circ Res. (2018) 122:664–9. doi: 10.1161/CIRCRESAHA.117.312465
100. Bekkering S, Arts RJW, Novakovic B, Kourtzelis I, van der Heijden CDCC, Li Y, et al. Metabolic induction of trained immunity through the mevalonate pathway. Cell. (2018) 172:135–46.e9. doi: 10.1016/j.cell.2017.11.025
101. Newens KJ, Thompson AK, Jackson KG, Wright J, Williams CM. Acute effects of elevated NEFA on vascular function: a comparison of SFA and MUFA. Br J Nutr. (2011) 105:1343–51. doi: 10.1017/S0007114510004976
102. Calabrese EJ, Mattson MP. How does hormesis impact biology, toxicology, and medicine? NPJ Aging Mech Dis. (2017) 3:13. doi: 10.1038/s41514-017-0013-z
103. Calabrese EJ, Mattson MP, Calabrese V. Resveratrol commonly displays hormesis: occurrence and biomedical significance. Hum Exp Toxicol. (2010) 29:980–1015. doi: 10.1177/0960327110383625
104. Falchetti R, Fuggetta MP, Lanzilli G, Tricarico M, Ravagnan G. Effects of resveratrol on human immune cell function. Life Sci. (2001) 70:81–96. doi: 10.1016/S0024-3205(01)01367-4
105. Amouri S El. Progressive ban has led to more antibiotic alternatives. Poultry World. (2015) Available online at: https://www.poultryworld.net/Home/General/2015/3/Progressive-ban-has-led-to-more-antibiotic-alternatives-1719102W/ (accessed December 11, 2018).
106. Ristow M, Zarse K. How increased oxidative stress promotes longevity and metabolic health: the concept of mitochondrial hormesis (mitohormesis). Exp Gerontol. (2010) 45:410–8. doi: 10.1016/j.exger.2010.03.014
107. Vaiserman AM. Hormesis and epigenetics: is there a link? Ageing Res Rev. (2011) 10:413–21. doi: 10.1016/j.arr.2011.01.004
108. Hanjani NA, Vafa M. Protein restriction, epigenetic diet, intermittent fasting as new approaches for preventing age-associated diseases. Int J Prev Med. (2018) 9:58. doi: 10.4103/ijpvm.IJPVM_397_16
109. Szarc vel Szic K, Declerck K, Vidaković M, Vanden Berghe W. From inflammaging to healthy aging by dietary lifestyle choices: is epigenetics the key to personalized nutrition? Clin Epigenetics. (2015) 7:33. doi: 10.1186/s13148-015-0068-2
110. Strachan DP. Hay fever, hygiene, and household size. BMJ Br Med J. (1989) 299:1259–60. doi: 10.1136/bmj.299.6710.1259
111. Berbudi A, Ajendra J, Wardani APF, Hoerauf A, Hübner MP. Parasitic helminths and their beneficial impact on type 1 and type 2 diabetes. Diabetes Metab Res Rev. (2016) 32:238–50. doi: 10.1002/dmrr.2673
112. de Ruiter K, Tahapary DL, Sartono E, Soewondo P, Supali T, Smit JWA, et al. Helminths, hygiene hypothesis and type 2 diabetes. Parasite Immunol. (2017) 39:12404. doi: 10.1111/pim.12404
113. Hussaarts L, García-Tardón N, Van Beek L, Heemskerk MM, Haeberlein S, Van Der Zon GC, et al. Chronic helminth infection and helminth-derived egg antigens promote adipose tissue M2 macrophages and improve insulin sensitivity in obese mice. FASEB J. (2015) 29:3027–39. doi: 10.1096/fj.14-266239
114. Brestoff JR, Kim BS, Saenz SA, Stine RR, Monticelli LA, Sonnenberg GF, et al. Group 2 innate lymphoid cells promote beiging of adipose and limit obesity. Nature. (2016) 519:242–6. doi: 10.1038/nature14115
115. Lee M, Odegaard JI, Mukundan L, Qiu Y, Molofsky AB, Nussbaum C, et al. Activated type 2 innate lymphoid cells regulate beige fat biogenesis. Cell. (2015) 160:74–87. doi: 10.1016/j.cell.2014.12.011
Keywords: chronic low-grade inflammation, lifestyle, metabolism, phenotypic flexibility, resilience, nutrition
Citation: van den Brink W, van Bilsen J, Salic K, Hoevenaars FPM, Verschuren L, Kleemann R, Bouwman J, Ronnett GV, van Ommen B and Wopereis S (2019) Current and Future Nutritional Strategies to Modulate Inflammatory Dynamics in Metabolic Disorders. Front. Nutr. 6:129. doi: 10.3389/fnut.2019.00129
Received: 15 May 2019; Accepted: 30 July 2019;
Published: 26 August 2019.
Edited by:
Vida Abedi, Geisinger Health System, United StatesReviewed by:
Brandt D. Pence, University of Memphis, United StatesJulio Villena, CONICET Centro de Referencia para Lactobacilos (CERELA), Argentina
Copyright © 2019 van den Brink, van Bilsen, Salic, Hoevenaars, Verschuren, Kleemann, Bouwman, Ronnett, van Ommen and Wopereis. This is an open-access article distributed under the terms of the Creative Commons Attribution License (CC BY). The use, distribution or reproduction in other forums is permitted, provided the original author(s) and the copyright owner(s) are credited and that the original publication in this journal is cited, in accordance with accepted academic practice. No use, distribution or reproduction is permitted which does not comply with these terms.
*Correspondence: Suzan Wopereis, c3V6YW4ud29wZXJlaXNAdG5vLm5s