- 1College of Food Science, South China Agricultural University, Guangzhou, China
- 2Department of Laboratory Animal Science, College of Basic Medical Sciences, Third Military Medical University, Chongqing, China
There is an increasing interest in the effect of dietary polyphenols on the intestinal microbiota and the possible associations between this effect and the development of some cardiovascular diseases, such as atherosclerosis (AS). However, limited information is available on how these polyphenols affect the gut microbiota and AS development. This study was designed to evaluate the modulation of dietary tea polyphenols (TPs) on intestinal Bifidobacteria (IB) and its correlation with AS development in apolipoprotein E-deficient (ApoE−/−) mice. Fifty C57BL/6 ApoE−/− mice were randomized into one of the five treatment groups (n = 10/group): control group fed normal diet (CK); a group fed a high-fat diet (HFD); and the other three groups fed the same HFD supplemented with TPs in drinking water for 16 weeks. The total cholesterol and low-density lipoprotein cholesterol (LDL-C) were decreased significantly (P < 0.05) after TP interference. In addition, the TP diet also decreased the plaque area/lumen area (PA/LA) ratios (P < 0.01) in the TP diet group. Interestingly, copies of IB in the gut of ApoE−/− mice were notably increased with TP interference. This increase was dose dependent (P < 0.01) and negatively correlated with the PA/LA ratio (P < 0.05). We conclude that TPs could promote the proliferation of the IB, which is partially responsible for the reduction of AS plaque induced by HFD.
Introduction
Atherosclerosis (AS) is a leading cause of death in Western countries, which is a complex pathophenotype governed by genetic and environmental determinants. High-fat diet (HFD) is one of the environmental risk factors for the development of AS, which may cause changes in the intestinal microbial communities and may lead to obesity and low-grade intestinal inflammation (1). Moreover, the gut microbiota itself is an environmental risk factor for the development of AS (2).
Intestinal microbial communities may influence the efficiency of dietary energy uptake and consequently influence susceptibility to obesity. A novel pathway linking dietary lipid intake, intestinal microbiota, and AS was identified, and the regulation of surface expression levels of macrophage scavenger receptors is well known to participate in the atherosclerotic process (3). Gut microbiota is also believed to be responsible for the control of metabolic endotoxemia, low-grade inflammation, and obesity (4). Additionally, a strong correlation has been observed between microbiota composition and AS development (5, 6). Infection of Helicobacter cinaedi was found to alter expression of cholesterol receptors and transporters in cultured macrophages and caused foam cell formation, and enhanced AS in hyperlipidemic mice (7).
The principal component of AS plaques is lipids, which are derived from plasma lipids such as total cholesterol (TC), triglycerides (TG), and low-density lipoprotein cholesterol (LDL-C). High-density lipoprotein cholesterol (HDL-C) and LDL-C in serum are the major factors affecting the development of AS lesions (8). The concentration of serum LDL-C is positively correlated with the incidence of AS (9). Serum TG may also promote plaque formation (10, 11), which further promotes AS (12). Bifidobacterium is one of the major genera of Actinobacteria that make up the colon microflora in mammals. Phylum Actinobacteria was considered to suppress inflammation and obesity (13, 14). Moreover, Bifidobacteria may produce short-chain fatty acids to decrease gut pH (15), form a biological barrier, and secret antimicrobial compounds to attenuate harmful bacteria (16, 17). Populations of Bifidobacterium spp. are negatively correlated with serum TC and non-HDL-cholesterol level, autoantibodies against oxidized LDL, and AS lesion size (5). However, serum HDL-C level is positively correlated with Bifidobacterium spp. populations (5). Therefore, the diet pattern that modulates the intestinal Bifidobacteria (IB) may further influence the development of AS.
Tea polyphenols (TPs) contain catechins, flavonoids and flavonols, anthocyanins, and phenolic acids. They were identified to significantly reduce AS plaque area (18, 19) and have effects against coronary heart disease, high blood cholesterol concentrations, and high blood pressure (20). Loke et al. hypothesized that polyphenols in foods can slow down the inflammatory response, which reduces NO bioavailability and therefore help ApoE−/− mice fight against AS (1). TPs may adjust the composition of the gut microbiome to maintain intestinal micro-ecological balance (21, 22), and protect and promote the development of probiotics (23, 24). This phenomenon has also been observed in other food-derived polyphenols (25).
We hypothesize that TPs may modulate fat metabolism and AS development by adjusting the gut Bifidobacteria in some degree. The present study was designed to understand the role of TPs on gut Bifidobacteria, lipid metabolism, and AS by using different dosages of TPs fed to ApoE−/− mice. The relationship among dietary TPs, gut Bifidobacteria, and AS was also explored.
Materials and Methods
Animals, Diet, and Sample Collection
Fifty 8-week-old-specific pathogen-free (SPF) C57BL/6 ApoE−/− mice were randomized into one of the five treatment groups (n = 10/group, each half male and female): (1) high-dose TPs group (TPH), with 1.6 g/L of TP in the drinking water; (2) middle-dose TP group (TPM), with 0.8 g/L of TP in the drinking water; (3) low-dose TP group (TPL), with 0.4 g/L of TP in the drinking water; (4) high-fat control group (HFD); and (5) normal diet group (CK). In each group, 30 g/L sucrose was added to the drinking water to mask the bitterness of TP (19). The CK group was fed with regular feed (formulation is shown in Table S1 in Supplementary Material); the remaining four groups were fed a high-fat high-cholesterol feed (regular feed 83.25 g, fat 15 g, cholesterol 1.25 g, and cholic acid 0.5 g). The mice were housed in cages of the Third Military Medical University, Department of Experimental Animal Center of SPF animal house, at 23 ± 2°C with relative air humidity of 50 ± 5% on a 12-h light–dark cycle. All feeds were sterilized by 60Co gamma radiation (42.5 millirad, Radiation Centre of Third Military Medical University).
The study was approved and supervised by the Ethics Committee of the Third Military Medical University Chongqing, China. SPF C57BL/6 ApoE−/− mice (No. SCXK 2012-0003; SYXK 2012-0002) used in this study were provided by the Department of Laboratory Animal Science of the Third Military Medical University.
Drinking water was replaced daily according to the formulation, and food consumption and body weight changes were recorded weekly. Two to five feces pellets from each cage were weekly collected stored in micro-tubes at −80°C until use.
Tea polyphenols were provided by Jiangxi Lvkang Natural Products Co., Ltd., Yichun, China (main components are shown in Table S2 in Supplementary Material).
DNA Extraction
Approximately 20 mg of mice fecal sample was enclosed in a pre-aseptic 2-mL screw-cap tube (Axygen, Union City, CA, USA) with 0.1-g zirconia beads (diameter of 0.1 mm, BioSpec, Bartlesville, OK, USA), 250 μL lysis buffer (0.5M NaCl, 50 mM EDTA, 50 mM Tris–HCl pH 8.0, and 4% SDS), and 100 μL phenol:chloroform:isoamyl alcohol (25:24:1) (v/v). Specimens were homogenized with a MiniBeadBeater-16 (BioSpec) for 2 min at maximum speed, and then 1–5 μL of DNA-free RNAase (10 mg/μL) was added to remove residual RNA. The DNA was purified with a phenol–chloroform extraction. The DNA concentration was measured with a NanoDrop ND-1000 spectrophotometer (Thermo, Wilmington, DE, USA) at 260 and 280 nm, and DNA integrity was examined by electrophoresis in 0.8% (w/v) agarose gels (26).
ApoE−/− Mice Abdominal Aorta Section and Atherosclerosis Plaque Analysis
After treatment for 16 weeks, ApoE−/− mice were anesthetized, their limbs were fixed on an anatomic plate, and their chest and abdomen were disinfected with 75% ethanol. After the thoracic cavity was opened to expose the heart, the left ventricle and right atrial appendage were cut. A perfusion needle was immediately inserted into the left ventricle after the cut to perfuse saline and 10% formaldehyde into heart for 40 min. About 10-mm specimens were cut from the abdominal aorta, fixed with 10% formalin, and embedded in paraffin after 48 h. Each aorta sample was stained with hematoxylin–eosin (HE) (27). The lesion area was measured by means of direct image capture from optical microscopy (Nikon digital system, Tokyo, Japan) and quantified using Image Pro Plus 6.0 (Media Cybernetics, Warrendale, PA, USA) software.
Serum Lipid and Lipoprotein Analyses
After 16 weeks, 3ApoE−/− mice were randomly selected from each group and anesthetized with 0.5% barbital sodium solution, and 0.2-mL whole blood samples from each mouse were drawn and stored in sterilized Eppendorf tubes. The blood was allowed to clot at room temperature before the serum was separated by centrifugation at 1000 g for 10 min. Serum was transferred to sterilized Eppendorf tubes and stored at −80°C until use. Serum parameters were determined by an Olympus Biochemical Auto-analyzer (Olympus AU 2700 Auto-analyzer, Olympus Corporation, Tokyo, Japan) using reagents from Olympus Diagnostics (28).
Quantitation of Bifidobacteria
A Bifidobacteria genus quantitative PCR standard curve was established by cloning a standard Bifidobacterium strain Bifidobacterium breve LMG11042 16S rRNA gene into a pMD19-T vector, as Bartosch et al. previously described (29). Plasmid standards and samples were assayed simultaneously in triplicate.
The qRT-PCR Analysis of Bifidobacterium
16S rRNA genes was extracted by using SYBR PCR reagents; the extracted genes were then analyzed by an iCycleriQ real-time detection system (Bio-Rad, Hercules, CA, USA) compatible with the iCycler Optical System Interface software program (Bio-Rad) and Bifidobacterium primers (Bifid-real F, 5′-GCGTGCTTAACACATGCAAGTC-3′; Bifid-real R, 5′-CACCCGTTTCCAGGAGCTATT-3′) (30). The 20-μL reaction mixture contained 2-μL DNA (diluted plasmid standards or samples), 0.2 μM of each primer, and appropriate amounts of other reagents as recommended by the manufacturer. The PCR thermocycling was programed to run the first cycle at 95°C for 3 min, followed by 35 cycles of denaturation at 95°C for 30 s, and annealing/elongation at 60°C for 1 min. After amplification, melting curve analysis of the PCR products was performed from 60 to 94°C, with increments of 0.5°C per 10 s. The copy number of the target sequence was calculated as previously described (31).
Statistical Analysis
Statistical analysis was performed using GraphPad Prism Software for Windows OS (version 6.05, Graphpad Software, La Jolla, CA, USA). Statistically significant differences between serum TC, TG, HDL-C, and LDL-C content and AS plaque area between the five treatment groups were determined by the non-parametric one-way ANOVA test with default parameters all the time. The Tukey’s multiple comparisons test was used for multiple pairwise comparisons and a dose–response relationship. A dose–response meta-analysis was performed to examine a potential non-linear relationship between TP dose, AS plaque area, and LgN (copies of Bifidobacteria), and a dose–response meta-analysis was conducted using generalized least squares regression test with EViews Software for Windows (version 8.0, IHS Global GNC, PA, USA). P < 0.05 was considered statistically significant.
Results
Body Weight and Feed Consumption of ApoE−/−Mice
ApoE−/− mice mean consumption of high-fat feed was less than that of the CK group, and the HFD and TPH groups had significantly lower food consumption (P < 0.05) in the first week (Figure 1A). However, the difference in feed consumption per week among TPH, TPM, TPL, HFD, and CK groups were not statistically different from the second week and over the whole study (P > 0.05) (Figure 1B). Figure 1C shows that the mean body weight of the TP group (TPH, TPM, and TPL) and HFD group were significantly lower than the CK group (P < 0.05) from the first week to the fourth week. The ApoE−/− mice in the HFD group gained weight faster than the other groups from 5th week and had the highest weight from the 11th week to the end of the study. However, this difference was not significant (P > 0.05).
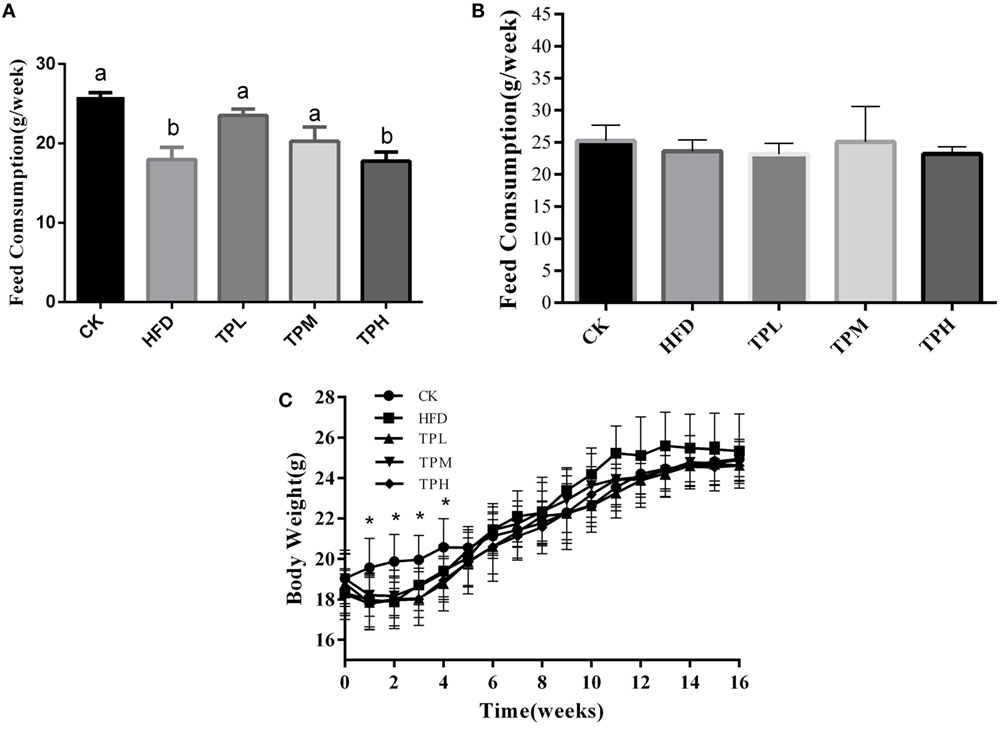
Figure 1. Effects of diet with tea polyphenol on feed consumption and body weight of C57BL/6 ApoE−/− mice. (A) Feed consumption in 1st week; (B) feed consumption in the whole period; (C) body weight per mouse. Bars represent means ± SEM, n = 10. (a,b) Different lowercase letters indicate significant differences (p < 0.05); the same lowercase letter indicates that the difference is not significant (p > 0.05); *means significant differences compared with CK group (p < 0.05).
TC, TG, HDL-C, and LDL-C of ApoE−/− Mice Serum
The effect of treatments on lipid parameters is demonstrated in Figure 2. After a HFD for 16 weeks, the mean serum TC (36.4 ± 12.2 mmol/L), LDL-C (28.4 ± 10.8 mmol/L), and HDL-C (4.1 ± 1.4 mmol/L) levels in the HFD group were significantly higher than those in the CK group (18.4 ± 0.7, 15.5 ± 0.6, and 2.1 ± 0.1 mmol/L, respectively) (P < 0.05).
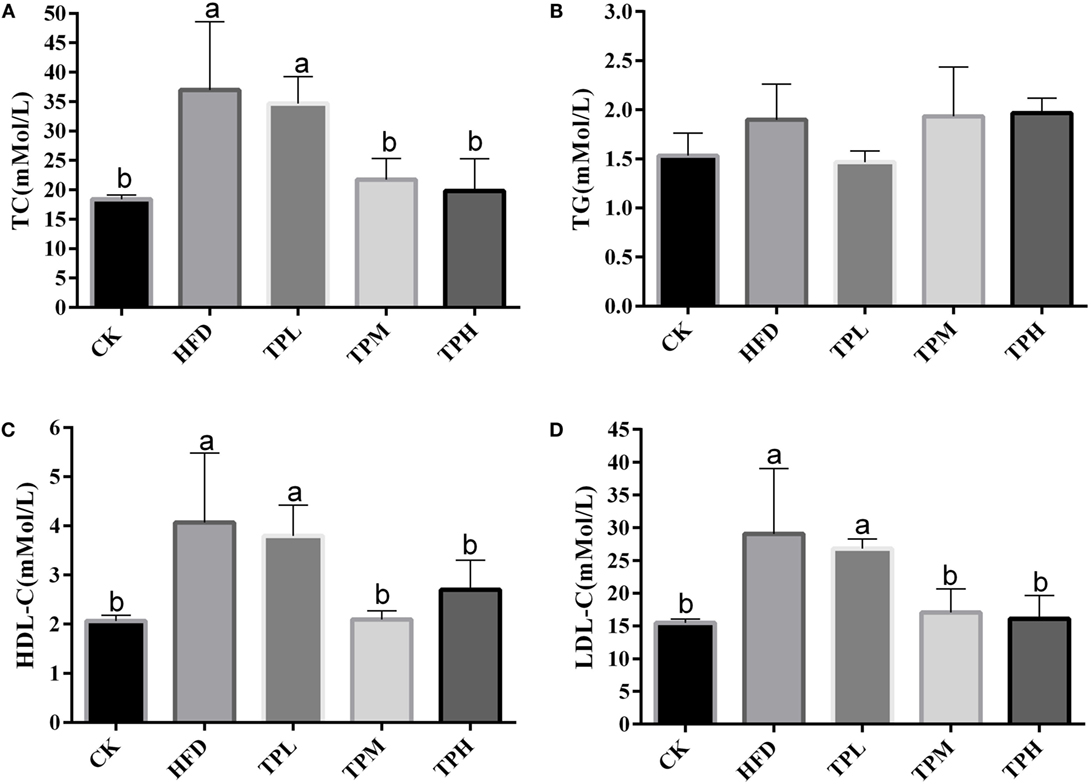
Figure 2. Effect on the serum levels in the C57BL/6 ApoE−/− mice after diet with tea polyphenol 16 weeks. (A) TC; (B) TG; (C) LDL-C; and (D) HDL-C. Bars represent means ± SEM, n = 3. (a,b) Different lowercase letters indicate significant differences (p < 0.05); the same lowercase letter indicates that the difference is not significant (p > 0.05).
No significant difference in serum TG level was found between the HFD and CK group. In the TPL group, no significant difference in lipid parameters was observed compared to the HFD group. The serum TC, LDL-C, and HDL-C levels were significantly lower among the TPH and TPM groups, when comparing to the HFD group (P < 0.05).
However, TG levels in the TPH, TPM, and TPL groups were 2.0 ± 0.5, 1.9 ± 0.2, and 1.5 ± 0.1 mmol/L, respectively, with no significant differences among the TPH, TPM, TPL, and HFD groups (P > 0.05) (Figure 2B).
If the mice consume 0.25-mg TPs per gram body weight per day, it will get better protection on AS. These results are calculated from the following fact: each mouse consumes about 4-mL (contain 1.6 g/L TPs) water; each mouse’s average body weight is 25 g.
Atherosclerosis Plaques in ApoE−/−Mice Abdominal Aortas
ApoE−/− mice abdominal aorta AS lesion progression was assessed by AS plaque size, intimal and medial integrity, and foam cell morphology observed by optical microscopy. The results indicated an association between the fat content in the feed and development of AS lesions: the PA/LA ratio in the HFD group was 0.69 ± 0.18, whereas no AS plaques were observed in the CK group. The ruptured foam cells formed AS plaques which accumulated in the intimal membrane, thus causing narrower blood vessels in mice of the HFD group. Therefore, the AS model in ApoE−/− mice was successfully constructed with the HFD. After 16 weeks of treatment with TPs, microscopic analyses of the abdominal aorta lesions showed that atherosclerotic lesion development was retarded by dietary TP. AS plaque area in the abdominal aorta among TP fed groups were smaller than those in the HFD group (Figure 3). The PA/LA ratios in the TPL, TPM, and TPH groups were 0.36 ± 0.07, 0.10 ± 0.01, and 0.06 ± 0.02, respectively, and were significantly lower than those of the HFD group (0.69 ± 0.18) (P < 0.01) (Figure 4). No significant difference of AS lesion between the TPH and TPM groups (P > 0.05) was shown in this study; however, the AS lesion in TPH and TPM groups were significantly lower than that of the TPL group (P < 0.05).
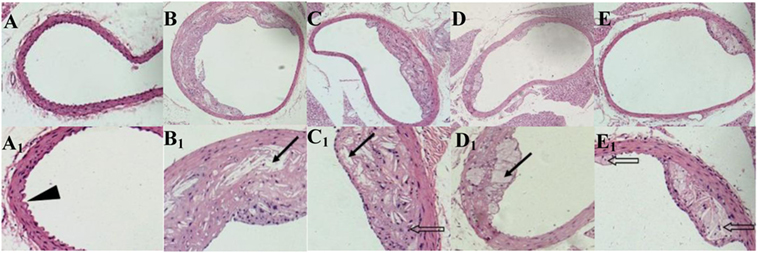
Figure 3. Cross-sections of abdominal aorta stained by HE staining from C57BL/6 ApoE−/− mice fed a normal diet (A), a high-fat diet (B) and tea polyphenols diet (C–E) for 16 weeks. Representative photographs are also shown for abdominal aorta AS plaque area in C57BL/6 ApoE−/− mice of CK group (A,A1), HFD group (B,B1), TPL group (C,C1), TPM group (D,D1), TPH group (E,E1). Note: black triangle (►) is shown for intimal membrane protuberance; solid black arrows () indicate AS material; and feint arrows (
) indicate foam cells.
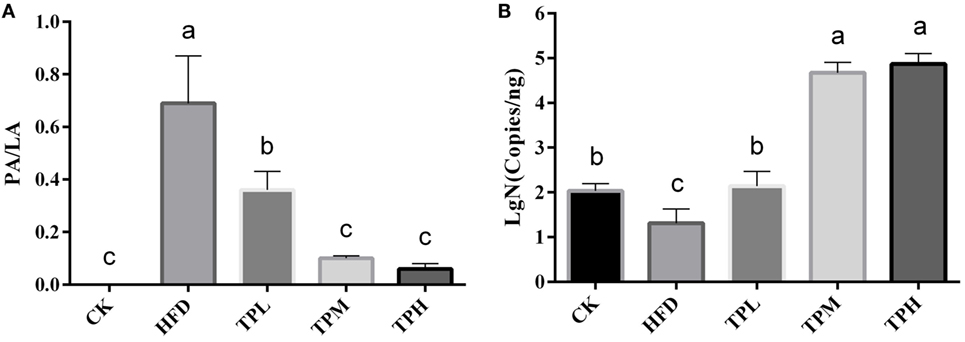
Figure 4. Abdominal aorta AS plaque area and lumen area ratio (PA/LA, P < 0.01) in the C57BL/6 ApoE−/− mice diet with tea polyphenol for 16 weeks (A). After a 16-week TP diet, Bifidobacteria copies in the TPL (P < 0.01), TPM (P < 0.001), and TPH (P < 0.001) groups were all significantly higher than that in the HFD (P < 0.01) group (B). Bars represent means ± SEM, n = 10. (a,b,c) Different lowercase letters indicate significant differences (p < 0.05); the same lowercase letter indicates that the difference is not significant (p > 0.05).
Copies of Bifidobacteria in the ApoE−/−Mouse Gut
The number of copies of Bifidobacteria was 1.31 ± 0.32 log copies/ng in feces of the HFD group, which was significantly lower than that in the CK group (2.03 ± 0.17 log copies/ng, P < 0.01). Therefore, a HFD may lower the amount of Bifidobacteria in the ApoE−/− mouse gut. After a 16-week TP diet, Bifidobacteria copies in the TPL (2.46 ± 1.80 log copies/ng, P < 0.01), TPM (4.67 ± 0.23 log copies/ng, P < 0.001), and TPH (4.87 ± 0.23 log copies/ng, P < 0.001) groups were all significantly higher than that in the HFD group. Furthermore, copies in the TPM and TPH groups were markedly higher than those in the TPL group (P < 0.01), but there was no significant different between copy numbers in the TPH and TPM groups (P > 0.05). This illustrates that TP could increase copy numbers of Bifidobacteria in the ApoE−/− mouse gut dose-dependently (Figure 4B).
Non-Linear Dose–Response Analyses of TP, IB Copies, and AS Plaque
There was a significant non-linear dose–response association observed between AS plaque PA/PL value and the dose of TP (R2 = 0.9033, P = 0.0496) (Figure 5A), and it is also significant between PA/PL value and Bifidobacteria copy number (R2 = 0.9773, P = 0.0114) (Figure 5C); however, there no statistically significance between TP dose and the Bifidobacteria copy number (R2 = 0.9059, P = 0.4839) (Figure 5B).
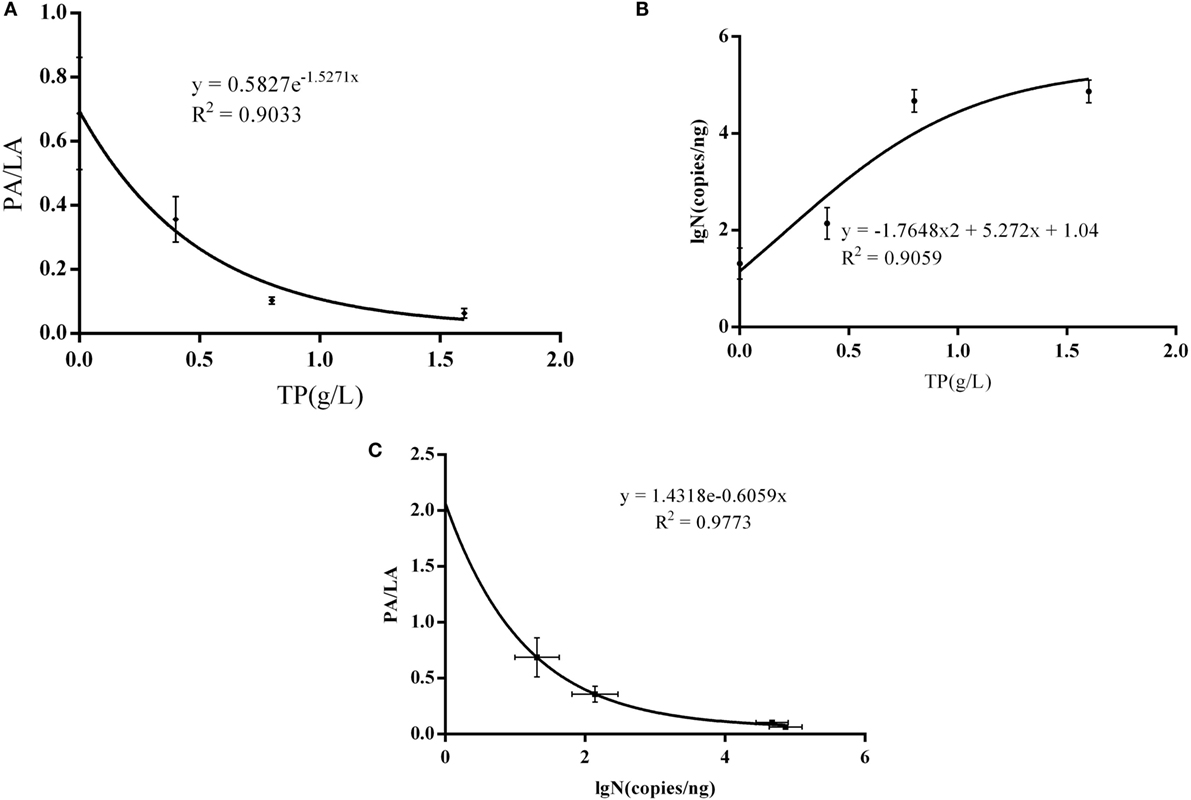
Figure 5. Interaction between AS, IB, and TP. (A) Correlations between TP doses and PA/PL ratio; (B) correlation between Bifidobacteria copies and PA/PL ratio; (C) correlation between TP doses and Bifidobacteria copies; the C57BL/6 ApoE−/− mice fed a high-fat and tea polyphenols diet for 16 weeks. Bars represent means ± SEM, n = 3, (A) P = 0.0496, (B) P = 0.4839, and (C) P = 0.0114.
Discussion
Tea polyphenols, the most important bioactive ingredients present in tea, are believed to exert a diversity of beneficial effects in preventing the development of AS (32, 33). LDL-C is the most significant risk factor for AS development: LDL-C can be oxidized into ox-LDL, which may then interact with the scavenger receptors of macrophages and vascular smooth muscle membranes in the arterial wall. This interaction leads to the formation of fat-derived and muscle-derived foam cells; an AS plaque will develop due to the accumulation of the foam cell (34). It is believed that TPs may alleviate lipid metabolic abnormalities by reducing LDL-C levels (35) and inhibiting LDL-C oxidation (32, 33). The results of this study showed that TP could significantly retard AS lesion formation in ApoE−/− mouse abdominal aorta. Moreover, the dose of TP was negatively correlated with the area of AS lesions.
Atherosclerosis is also considered as a type of chronic inflammation (36), which is closely related to metabolic diseases such as obesity and diabetes (36–38). In recent years, increasing evidence has found an association between metabolic disease and intestinal flora (39, 40). However, no study has fully demonstrated the relationship among the dietary polyphenols, the gut microbiota, and the development of AS. The further possible mechanism of TPs can inhabit AS development maybe the anti-inflammatory ability of itself.
Diet is the main environmental factor impacting the gut microbiome composition. We investigated the effects of a TP diet on ApoE−/− mice fed a HFD, and our results showed that TP can dramatically increase the amount of gut Bifidobacteria. Lee (23) reported that growth of certain pathogenic bacteria, such as Clostridium perfringens, Clostridium difficile, and Bacteroides spp., was significantly repressed by TPs, while Bifidobacterium spp. and Lactobacillus spp. were less severely affected. According to the result that different intestinal bacteria had varying degrees of sensitivity to TPs, we concluded that TPs can increase the number of Bifidobacteria. It has been shown that Bifidobacteria species have beneficial effects on cardiovascular health. B. breve may significantly reduce the serum TC level when given to obese mice induced by HFD (14). In this study, we found that TP diet significantly reduced serum TC and LDL-C levels of ApoE−/− mice (P < 0.05). Meanwhile, a recent metabolomic study indicated that product(s) yielded from microbial metabolism of lipids in the gut may promote AS (3).
It was believed that the serum HDL-C level is positively correlated with the existence of Bifidobacteria populations in the gut (5). However, this study found that TP diet significantly decreased (P < 0.05) the serum HDL-C levels in mice. A possible explanation is that TP diet decreases the serum cholesterol level, thus lowering the serum HDL-C level. HDL-C plays a key role in removing cholesterol from cholesterol-loaded macrophages, thus preventing the accumulation of arterial plaque (41, 42). When ApoE−/− mice were fed with a HFD, the serum cholesterol level was elevated. This elevation induced the production of the HDL particles. Other studies have also found that a HFD may induce an elevation in serum HDL-C level (43), while a low-fat diet may decrease the serum HDL-C level (44).
Epidemiological studies have also showed that serum HDL-C level is inversely correlated with risk of cardiovascular events (41). However, our investigation demonstrated that the elevated serum HDL-C level in the HFD group did not play a protective role in preventing the formation of abdominal aorta AS plaques in ApoE−/− mice (Figure 4A). It is important to note that HDL-C particles in ApoE−/− mice are abnormal and disrupted (45) due to the lack of ApoE. The lack of ApoE in the HDL particles leads to a weaker cholesterol efflux capacity (the ability to remove cholesterol from cholesterol-loaded macrophages) (46–48). Hence, the formation of abdominal aorta AS plaques is expected. Furthermore, it is well known that AS is strongly associated with obesity. Zhao and his colleagues reported that Bifidobacteria may reduce fat accumulation (49). It was observed that the abundance of Bifidobacteria in the mouse gut declined significantly due to HFD feeding (50, 51). This phenomenon was also observed in our study (Figure 4B). A dose-dependent effect was found between the dietary TP and the number of Bifidobacteria in this study. The AS plaque PA/PL value was significantly and negatively correlated with the Bifidobacteria count. The dominant Bifidobacteria species in the ApoE−/− mouse gut is Bifidobacterium pseudolongum (52). We make a putative opinion that TP could induce a Bifidobacteria boom, which could prevent AS development by modulating the fat metabolism. This process could be a part of the mechanism of the effect of TP on cardiovascular health.
Author Contributions
The manuscript was written through contributions of all authors. All authors have given approval to the final version of the manuscript.
Conflict of Interest Statement
The authors declare that the research was conducted in the absence of any commercial or financial relationships that could be construed as a potential conflict of interest.
Funding
This work was financially supported by the National Natural Science Foundation of China (NSFC 31071528 and NSFC 31171673), special fund from Modern Agricultural Industry (CARS-23), National High Technology Research and Development Program of China (2014AA022204, 2014AA022209), and National Basic Research Program of China (2013CB531406).
Supplementary Material
The Supplementary Material for this article can be found online at http://journal.frontiersin.org/article/10.3389/fnut.2016.00042/full#Supplementary-Material.
Abbreviations
AS, atherosclerosis; CK, normal diet group; HDL-C, high-density lipoprotein cholesterol; HFD, high-fat diet group; IB, intestinal Bifidobacteria; LDL-C, low-density lipoprotein-cholesterol; PA/LA, plaque area/lumen area; TC, total cholesterol; TG, triglycerides; TPH, tea polyphenols high-dose group; TPL, tea polyphenols low-dose group; TPM, tea polyphenols medium-dose group; TPs, tea polyphenols.
References
1. Loke WM, Proudfoot JM, Hodgson JM, McKinley AJ, Hime N, Magat M, et al. Specific dietary polyphenols attenuate atherosclerosis in apolipoprotein E-knockout mice by alleviating inflammation and endothelial dysfunction. Arterioscler Thromb Vasc Biol (2010) 30:749–U243. doi:10.1161/ATVBAHA.109.199687
2. Stock J. Gut microbiota: an environmental risk factor for cardiovascular disease. Atherosclerosis (2013) 229:440–2. doi:10.1016/j.atherosclerosis.2013.05.019
3. Wang ZN, Klipfell E, Bennett BJ, Koeth R, Levison BS, Dugar B, et al. Gut flora metabolism of phosphatidylcholine promotes cardiovascular disease. Nature (2011) 472:57–U82. doi:10.1038/nature09922
4. Cani PD, Bibiloni R, Knauf C, Neyrinck AM, Neyrinck AM, Delzenne NM, et al. Changes in gut microbiota control metabolic endotoxemia-induced inflammation in high-fat diet-induced obesity and diabetes in mice. Diabetes (2008) 57:1470–81. doi:10.2337/db07-1403
5. Cavallini DU, Suzuki JY, Abdalla DP, Vendramini RC, Pauly-Silveira ND, Roselino MN, et al. Influence of a probiotic soy product on fecal microbiota and its association with cardiovascular risk factors in an animal model. Lipids Health Dis (2011) 10:126. doi:10.1186/1476-511X-10-126
6. Koren O, Spor A, Felin J, Fak F, Stombaugh J, Tremaroli V, et al. Human oral, gut, and plaque microbiota in patients with atherosclerosis. Proc Natl Acad Sci U S A (2011) 108:4592–8. doi:10.1073/pnas.1011383107
7. Khan S, Rahman HA, Okamoto T, Matsunaga T, Fujiwara Y, Sawa T, et al. Promotion of atherosclerosis by Helicobacter cinaedi infection that involves macrophage-driven proinflammatory responses. Sci Rep (2014) 4:4680. doi:10.1038/srep04680
8. Kushwaha RS, McGill HC. Diet, plasma lipoproteins and experimental atherosclerosis in baboons (Papio sp.). Hum Reprod Update (1998) 4(4):420–9. doi:10.1093/humupd/4.4.420
9. Takeichi S, Yukawa N, Nakajima Y, Osawa M, Saito T, Seto Y, et al. Association of plasma triglyceride-rich lipoprotein remnants with coronary atherosclerosis in cases of sudden cardiac death. Atherosclerosis (1999) 142(2):309–15.
10. Labreuche J, Deplanque D, Touboul PJ, Bruckert E, Amarenco P. Association between change in plasma triglyceride levels and risk of stroke and carotid atherosclerosis – systematic review and meta-regression analysis. Atherosclerosis (2010) 212(1):9–15. doi:10.1016/j.atherosclerosis.2010.02.011
11. Norata GD, Raselli S, Grigore L, Maggi FM, Catapano AL. Triglycerides rich lipoproteins and atherosclerosis: in vitro and in vivo studies. Int Congr Ser (2003) 1262:507–10. doi:10.1016/j.ics.2003.12.040
12. Khurana R, Moons L, Shafi S, Luttun A, Collen D, Martin JF, et al. Placental growth factor promotes atherosclerotic intimal thickening and macrophage accumulation. Circulation (2005) 111(21):2828–36. doi:10.1161/CIRCULATIONAHA.104.495887
13. Kalliomaki M, Collado MC, Salminen S, Isolauri E. Early differences in fecal microbiota composition in children may predict overweight. Am J Clin Nutr (2008) 87(3):534–8.
14. Kondo S, Xiao JZ, Satoh T, Odamaki T, Takahashi S, Sugahara H, et al. Antiobesity effects of Bifidobacterium breve strain B-3 supplementation in a mouse model with high-fat diet-induced obesity. Biosci Biotechnol Biochem (2010) 74(8):1656–61. doi:10.1271/bbb.100267
15. Mitsuoka T, Hidaka H, Eida T. Effect of fructo-oligosaccharides on intestinal microflora. Nahrung (1987) 31(5–6):427–36.
16. Gibson GR, Wang X. Regulatory effects of Bifidobacteria on the growth of other colonic bacteria. J Appl Bacteriol (1994) 77(4):412–20. doi:10.1111/j.1365-2672.1994.tb03443.x
17. Wang X, Gibson GR. Effects of the in-vitro fermentation of oligofructose and inulin by bacteria growing in the human large-intestine. J Appl Bacteriol (1993) 75(4):373–80. doi:10.1111/j.1365-2672.1993.tb02790.x
18. Vinson JA, Teufel K, Wu N. Green and black teas inhibit atherosclerosis by lipid, antioxidant, and fibrinolytic mechanisms. J Agric Food Chem (2004) 52(11):3661–5. doi:10.1021/jf035255l
19. Miura Y, Chiba T, Tomita I, Koizumi H, Miura S, Umegaki K, et al. Tea catechins prevent the development of atherosclerosis in apoprotein E-deficient mice. J Nutr (2001) 131(1):27–32.
20. Mukhtar H, Ahmad N. Tea polyphenols: prevention of cancer and optimizing health. Am J Clin Nutr (2000) 1(6):1698–702.
21. Cardona F, Andres-Lacueva C, Tulipani S, Tinahones FJ, Queipo-Ortuno MI. Benefits of polyphenols on gut microbiota and implications in human health. J Nutr Biochem (2013) 24(8):1415–22. doi:10.1016/j.jnutbio.2013.05.001
22. van Duynhoven J, Vaughan EE, van Dorsten F, Gomez-Roldan V, de Vos R, Vervoort J, et al. Interactions of black tea polyphenols with human gut microbiota: implications for gut and cardiovascular health. Am J Clin Nutr (2013) 98(6):1631–41. doi:10.3945/ajcn.113.058263
23. Lee HC, Jenner AM, Low CS, Lee YK. Effect of tea phenolics and their aromatic fecal bacterial metabolites on intestinal microbiota. Res Microbiol (2006) 157(9):876–84. doi:10.1016/j.resmic.2006.07.004
24. Okubo T, Ishihara N, Oura A, Serit M, Kim M, Yamamoto T, et al. In vivo effects of tea polyphenol intake on human intestinal microflora and metabolism. Biosci Biotechnol Biochem (1992) 56(4):4. doi:10.1271/bbb.56.588
25. Espley RV, Butts CA, Laing WA, Martell S, Smith H, McGhie TK, et al. Dietary flavonoids from modified apple reduce inflammation markers and modulate gut microbiota in mice. J Nutr (2014) 144(2):146–54. doi:10.3945/jn.113.182659
26. Zeng BH, Li GQ, Yuan J, Li WX, Tang H, Wei H. Effects of age and strain on the microbiota colonization in an infant human flora-associated mouse model. Curr Microbiol (2013) 67(3):313–21. doi:10.1007/s00284-013-0360-3
27. Paigen B, Morrow A, Holmes PA, Mitchell D, Williams RA. Quantitative assessment of atherosclerotic lesions in mice. Atherosclerosis (1987) 68(3):231–40.
28. Atalay G, Dirix L, Biganzoli L, Beex L, Nooij M, Cameron D, et al. The effect of exemestane on serum lipid profile in postmenopausal women with metastatic breast cancer: a companion study to EORTC Trial 10951, ‘randomized phase II study in first line hormonal treatment for metastatic breast cancer with exemestane or tamoxifen in postmenopausal patients’. Ann Oncol (2004) 15(2):211–7. doi:10.1093/annonc/mdh064
29. Bartosch S, Fite A, Macfarlane GT, McMurdo M. Characterization of bacterial communities in feces from healthy elderly volunteers and hospitalized elderly patients by using real-time PCR and effects of antibiotic treatment on the fecal microbiota. Appl Environ Microbiol (2004) 70(6):3575–81. doi:10.1128/AEM.70.6.3575-3581.2004
30. Penders J, Vink C, Driessen C, London N, Thijs C, Stobberingh EE. Quantification of Bifidobacterium spp., Escherichia coli and Clostridium difficile in faecal samples of breast-fed and formula-fed infants by real-time PCR. FEMS Microbiol Lett (2005) 243(1):141–7. doi:10.1016/j.femsle.2004.11.052
31. Monnet C, Correia K, Sarthou AS, Irlinger F. Quantitative detection of Corynebacterium casei in cheese by real-time PCR. Appl Environ Microbiol (2006) 72(11):6972–9. doi:10.1128/AEM.01303-06
32. Tijburg LB, Wiseman SA, Meijer GW, Weststrate JA. Effects of green tea, black tea and dietary lipophilic antioxidants on LDL oxidizability and atherosclerosis in hypercholesterolaemic rabbits. Atherosclerosis (1997) 135:37–47. doi:10.1016/S0021-9150(97)00139-1
33. Wang BS, Yu HM, Chang LW, Yen WJ, Duh PD. Protective effects of pu-erh tea on LDL oxidation and nitric oxide generation in macrophage cells. LWT Food Sci Technol (2008) 41:1122–32. doi:10.1016/j.lwt.2007.07.002
34. Funk CD, Cyrus T. 12/15-lipoxygenase, oxidative modification of LDL and atherogenesis. Trends Cardiovasc Med (2001) 11(3–4):116–24. doi:10.1016/S1050-1738(01)00096-2
35. Imai K, Nakachi K. Cross sectional study of effects of drinking green tea on cardiovascular and liver diseases. BMJ (1995) 310:693–6. doi:10.1136/bmj.310.6981.693
36. Caesar R, Fak F, Backhed F. Effects of gut microbiota on obesity and atherosclerosis via modulation of inflammation and lipid metabolism. J Intern Med (2010) 268:320–8. doi:10.1111/j.1365-2796.2010.02270.x
37. Sanz Y, Santacruz A, Gauffin P. Gut microbiota in obesity and metabolic disorders. Proc Nutr Soc (2010) 69:434–41. doi:10.1017/S0029665110001813
38. Cani PD, Delzenne NM. The role of the gut microbiota in energy metabolism and metabolic disease. Curr Pharm Des (2009) 15:1546–58. doi:10.2174/138161209788168164
39. Turnbaugh PJ, Hamady M, Yatsunenko T, Cantarel BL, Duncan A, Ley RE, et al. A core gut microbiome in obese and lean twins. Nature (2009) 457:480–4. doi:10.1038/nature07540
40. Vijay-Kumar M, Aitken JD, Carvalho FA, Cullender TC, Mwangi S, Srinivasan S, et al. Metabolic syndrome and altered gut microbiota in mice lacking Toll-like receptor 5. Science (2010) 328:228–31. doi:10.1126/science.1179721
41. Khera AV, Cuchel M, Llera-Moya M, Rodrigues A, Burke MF, Jafri K, et al. Cholesterol efflux capacity, high-density lipoprotein function, and atherosclerosis. N Engl J Med (2011) 364(2):127–35. doi:10.1056/NEJMoa1001689
42. Chapman MJ. Therapeutic elevation of HDL-cholesterol to prevent atherosclerosis and coronary heart disease. Pharmacol Ther (2006) 111(3):893–908. doi:10.1016/j.pharmthera.2006.02.003
43. Hayek T, Ito Y, Azrolan N, Verdery RB, Aaltosetala K, Walsh A, et al. Dietary-fat increases high-density-lipoprotein (HDL) levels both by increasing the transport rates and decreasing the fractional catabolic rates of HDL cholesterol ester and apolipoprotein (Apo) A-I – presentation of a new animal-model and mechanistic studies in human Apo A-I transgenic and control mice. J Clin Invest (1993) 91(4):1665–71.
44. Brinton EA, Eisenberg S, Breslow JL. A low-fat diet decreases high-density lipoprotein (HDL) cholesterol levels by decreasing HDL apolipoprotein transport rates. J Clin Invest (1990) 85(1):144–51. doi:10.1172/JCI114405
45. Tani M, Matera R, Horvath KV, Hasan TS, Schaefer EJ, Asztalos BF. The influence of apoE-deficiency and LDL-receptor-deficiency on the HDL subpopulation profile in mice and in humans. Atherosclerosis (2014) 233(1):39–44. doi:10.1016/j.atherosclerosis.2013.11.080
46. De Geest B, Stengel D, Landeloos M, Lox M, Le Gat L, Collen D, et al. Effect of overexpression of human apo A-I in C57BL/6 and C57BL/6 apo E-deficient mice on 2 lipoprotein-associated enzymes, platelet-activating factor acetylhydrolase and paraoxonase – comparison of adenovirus-mediated human apo A-I gene transfer and human apo A-I transgenesis. Arterioscler Thromb Vasc (2000) 20(10):E68–75. doi:10.1161/01.ATV.20.10.e68
47. Hayek T, Oiknine J, Brook JG, Aviram M. Increased plasma and lipoprotein lipid-peroxidation in Apo E-deficient mice. Biochem Biophys Res Commun (1994) 201(3):1567–74. doi:10.1006/bbrc.1994.1883
48. Hayek T, Oiknine J, Brook JG, Aviram M. Role of HDL apolipoprotein E in cellular cholesterol efflux – studies in Apo-E knockout transgenic mice. Biochem Biophys Res Commun (1994) 205(2):1072–8. doi:10.1006/bbrc.1994.2775
49. Wang J, Tang H, Zhang C, Zhao Y, Derrien M, Rocher E, et al. Modulation of gut microbiota during probiotic-mediated attenuation of metabolic syndrome in high fat diet-fed mice. ISME J (2015) 9:1–15. doi:10.1038/ismej.2014.99
50. Murphy EF, Cotter PD, Healy S, Marques TM, O’Sullivan O, Fouhy F, et al. Composition and energy harvesting capacity of the gut microbiota: relationship to diet, obesity and time in mouse models. Gut (2010) 59:1635–42. doi:10.1136/gut.2010.215665
51. Cani PD, Neyrinck AM, Fava F, Knauf C, Burcelin RG, Tuohy KM, et al. Selective increases of Bifidobacteria in gut microflora improve high-fat-diet-induced diabetes in mice through a mechanism associated with endotoxaemia. Diabetologia (2007) 50:2374–83. doi:10.1007/s00125-007-0791-0
Keywords: atherosclerosis, Bifidobacteria, gut microbiome, tea polyphenols, C57BL/6 ApoE−/− mice
Citation: Liao Z-L, Zeng B-H, Wang W, Li G-H, Wu F, Wang L, Zhong Q-P, Wei H and Fang X (2016) Impact of the Consumption of Tea Polyphenols on Early Atherosclerotic Lesion Formation and Intestinal Bifidobacteria in High-Fat-Fed ApoE−/− Mice. Front. Nutr. 3:42. doi: 10.3389/fnut.2016.00042
Received: 04 January 2016; Accepted: 20 September 2016;
Published: 21 December 2016
Edited by:
Dongsheng Zhou, Beijing Institute of Microbiology and Epidemiology, ChinaReviewed by:
Liang Xiao, Beijing Genomics Institute, ChinaDongliang Wang, Sun Yat-sen University, China
Copyright: © 2016 Liao, Zeng, Wang, Li, Wu, Wang, Zhong, Wei and Fang. This is an open-access article distributed under the terms of the Creative Commons Attribution License (CC BY). The use, distribution or reproduction in other forums is permitted, provided the original author(s) or licensor are credited and that the original publication in this journal is cited, in accordance with accepted academic practice. No use, distribution or reproduction is permitted which does not comply with these terms.
*Correspondence: Hong Wei, d2VpaG9uZzYzNTI4JiN4MDAwNDA7MTYzLmNvbQ==;
Xiang Fang, ZnhpYW5nMjAwNCYjeDAwMDQwO2hvdG1haWwuY29t
†Zhen-Lin Liao, Ben-Hua Zeng, and Wei Wang contributed equally.