- 1Laboratory of Pharmacology and Toxicology of Natural Products, Biological Science Institute, Federal University of Para, Belém, Pará, Brazil
- 2Laboratory of Experimental Neuropathology, Joao de Barros Barreto University Hospital, Federal University of Para, Belem, Pará, Brazil
Introduction: Sleep disorders are common in the general population, necessitating the search for new strategies to address this public health challenge. The study aims to describe the electrocorticographic and behavioral changes in sleep deprived Wistar rats exposed to varying doses of camphor, to assess its effects on sleep and its potential as a sleep-inducing drug.
Materials and Methods: For the electrocorticographic evaluation, seventy-two rats were randomly assigned to distinct groups: a control group, a sleep-deprived group, three sleep-deprived groups receiving 10, 20, and 30 mg/kg i.p. of camphor respectively, and three groups that received these doses without sleep deprivation. For the behavioral analysis, twenty-seven rats were divided into three groups, each receiving the same doses as the previous test.
Results and Discussion: Our results showed that there was a decrease in the frequency of brain oscillatory patterns when camphor was administered at 10 mg/kg i.p. whereas there was a dose-dependent increase in the spectral power and distribution following the administration of 20 and 30 mg/kg i.p., with the emergence of Delta, Theta, Alpha, and Beta waves. As for the behavioral analysis, it was demonstrated that testicular relaxation, decreased motility, and light sleep induction also occurred in a dose-dependent manner. Thus, we conclude that camphor administration intensifies occipital electrocorticographic patterns in sleep-deprived rats, and its electrocorticographic and behavioral analysis could indicate a potential as a supporting agent in the insomnia treatment.
1 Introduction
Sleep disorders are highly prevalent in the general population (Gale et al., 2024; Qian et al., 2023; Rössler et al., 2017). It is well known the critical role of sleep quality in overall wellbeing, as it is associated with cortisol levels (Henry et al., 2021) and immunity (Ballestar-Tarín et al., 2023). Due to its significance, sleep restriction and circadian cycle disruption can impair systemic functioning of the organism and are correlated with the onset of numerous diseases (Touitou et al., 2017), including cancer (Straif et al., 2007; Stevens et al., 2011; Schernhammer et al., 2006; Papantoniou et al., 2015) and cardiovascular problems (Karlsson et al., 2001). Most importantly, these disturbances affect proper brain function, leading to cognitive decline (Marquié et al., 2015; Vallières et al., 2014; Fonken et al., 2012) and mood disorders (Lee et al., 2016; Bara and Arber, 2009; Kalmbach et al., 2015; Fonken et al., 2012).
Recent guidelines establish that cognitive-behavioral therapy (CBT) should be the first-line treatment for insomnia. When CBT proves insufficient, pharmacological interventions may be considered. For short-term insomnia, recommended medications include benzodiazepines (BZ), benzodiazepine receptor agonists (BZRAs), low-dose sedating antidepressants, and daridorexant (Riemann et al., 2023). Although BZ and BZRAs show positive outcomes, they should be prescribed with caution due to their association with tolerance, nocturnal confusion, falls, and cognitive impairment (Treves et al., 2018; Barker et al., 2004; Stranks and Crowe, 2014). Low-dose sedating antidepressants and daridorexant have proven useful for treating insomnia, but evidence supporting their effectiveness remains limited (Everitt et al., 2018). For chronic insomnia in adults, the latest American Academy of Sleep Medicine guidelines suggest that current pharmacological treatments — such as BZ, BZRAs, orexin receptor agonists, and melatonin agonists — have a weak strength of recommendation according to the GRADE methodology, which assesses the certainty of evidence in healthcare (Sateia et al., 2017; Morgenthaler et al., 2016). This does not imply that these drugs are ineffective, but rather that there is a lack of comprehensive understanding of their efficacy and potential risks. This underscores the ongoing need for research into these medications and the development of innovative approaches to address this public health issue.
Several essential oils have been described in the literature as influencing sleep induction (Dougnon and Ito, 2021; Lillehei and Halcon, 2014). Xiao et al. (2022) reported that borneol essential oil, a by-product of Cinnamomum camphora steam distillation, reduced locomotor activity, shortened sleep latency, and prolonged sleep duration in rats. Camphor is an aromatic terpene compound with a polyvalent structure that exhibits diverse biological properties, including antimicrobial, anti-inflammatory, analgesic, and antioxidative effects (Chen et al., 2013; Lee et al., 2022). However, its neurological actions remain poorly understood, with few studies examining camphor’s role in the induction and maintenance of sleep. Further research could potentially establish camphor as an alternative treatment for sleep disorders.
With this considerations in mind, the upcoming investigation aims to analyze and describe the electrocorticographic and behavioral changes observed in Wistar rats submitted to different doses of camphor with or without sleep deprivation, in order to verify the electrocorticographic changes generated in the medial visual cortex of these animals during sleep and assess the potential of camphor as a sleep inducer.
2 Materials and methods
2.1 Ethical considerations
The research was conducted in accordance with the rules of the National Legislation for the Use and Breeding of Experimental Animals, as well as in accordance with the ethical principles of the National Council for the Control of Experimental Animals (CONCEA), the ARRIVE 2.0 guidelines and the NIH Guide for the Care and Use of Laboratory Animals. The research project was submitted to and approved by the UFPA Research Ethics Committee file number 1380040822 [ID 002046].
2.2 Animals
The study used a total of ninety-nine adult male Wistar rats weighing 180–200 g. The animals were obtained from the Central Animal Facility of the Federal University of Pará (UFPA) and allocated individually to the Experimental Animal Facility of the Laboratory of Pharmacology and Toxicology of Natural Products. The animals received water and food ad libitum and were kept in a temperature-regulated environment (25°–28°C) with a 12-h light-dark cycle.
2.3 Chemical substances
The following chemical substances were used to carry out the research: ketamine hydrochloride anesthetic obtained from the Köing Laboratory (Santana de Parnaíba, SP, Brazil); xylazine hydrochloride obtained from the Vallée laboratory (Montes Claros, MG, Brazil); lidocaine obtained from the Hipolabor laboratory (Sabará, MG, Brazil) for electrode implantation; and camphor obtained from the Êxodo Científica laboratory (Sumaré, São Paulo, Brazil).
2.4 Experimental design
Seventy-two rats were randomly divided into the following groups: a) Control group (Control group without sleep deprivation); b) Control SLD (Control group with 24-h sleep deprivation); c) CPH 10 (Camphor-treated group 10 mg/kg i.p. without sleep deprivation); d) SLD- CPH 10 (Camphor-treated group 10 mg/kg i.p. with sleep deprivation); e) CPH 20 (Camphor-treated group 20 mg/kg i.p. without sleep deprivation); f) SLD- CPH 20 (Camphor-treated group 20 mg/kg i.p. with sleep deprivation); g) CPH 30 (Camphor-treated group 30 mg/kg i.p. without sleep deprivation); h) SLD- CPH 30 (Camphor-treated group 30 mg/kg i.p. with sleep deprivation). All ECoG recordings lasted 3 min. As for the behavioral analysis, twenty-seven rats were randomly separated into three groups: 10 mg/kg i.p. camphor-treated group; 20 mg/kg i.p. camphor-treated group; 30 mg/kg i.p. camphor-treated group. All groups were consisted of nine animals.
2.5 Electrocorticography (ECoG)
The entire experiment was conducted in a Faraday cage. The electrodes for ECoG acquisition were implanted in the bregma coordinate (−5.04 and 2 mm to the side of each hemisphere) represented by the medial visual cortex (Paxinos and Watson, 2013). On the fifth postoperative day, the electrodes were connected to a data acquisition system consisting of a high-impedance amplifier (Grass Technologies, P511), monitored by an oscilloscope (Protek, 6510). The data was continuously digitized at a rate of 1 KHz by a computer equipped with a data acquisition board (National Instruments, Austin, TX). The data was stored on a hard disk and processed using specialized software (LabVIEW express). The recording electrode was implanted in the right cerebral hemisphere, while the reference electrode was implanted in the left hemisphere. The electrophysiological recordings were analyzed up to 40 Hz. Brain oscillations were analyzed according to (Jalilifar and Yadollahpour, 2017; Hamoy et al., 2018), which correspond to: Delta (1–4 Hz), Theta (4–8 Hz), Alpha (8–12 Hz), Beta (12–28) and Gamma (28–40 Hz).
2.6 Behavioral analysis
All the tests were conducted between 8 and 10 a.m. The animals were observed for 30 min after the administration of camphor to non-sleep-deprived animals. The behavioral parameters observed were according to the following sequence: a) testicular relaxation (maintenance of the testicle in the scrotum due to the relaxation of the Cremaster muscle); b) decrease in the animals’ motility; c) induction of superficial sleep (where the animal could still be awakened by stimulation).
2.7 Electrophysiological data analysis
For the analysis of acquired signals, a program was built using Python 2.7. The Numpy and Scipy libraries were employed for mathematical processing, while the Matlab library was utilized for graphical representation. The graphical interface was developed using the PyQt4 library. Amplitude graphs were employed to demonstrate the potential difference between the reference and recording electrodes. A sampling rate of 1,000 samples per second was observed for signal analysis. Spectrograms were computed using a Hamming window with 256 points (256/1,000 s). Each frame was generated with an overlap of 128 points per window. For each frame, the Spectral Power Density (SPD) was calculated using the Welch’s average periodogram method. The frequency histogram was generated by first computing the SPD of the signal using the 256-point Hamming window without overlap, resulting in a histogram constructed with 1 Hz bins.
To analyze the differences between experiments, a graph was constructed with the mean and standard deviation (SD) of the SPD from multiple experiments. Each wave in the graph was generated from a test set, where the SPD was generated, and the mean and SD calculated for each group of 256 points without overlap were used for the Hamming window PSD calculation.
2.8 Statistical analysis
The results are presented as the mean value ± SD. The normality of the variances was verified by the Kolmogorov-Smirnov test and the homogeneity by the Levene test. The groups were compared using a one-way Analysis of Variance (ANOVA), followed by Tukey’s post hoc test, whenever appropriate. All the analyses were run in GraphPad® Prism 6 and considered a p < 0.05 significance level.
3 Results
The ECoG recordings of the Control group showed amplitude below 0.05 mV (Figure 1A, left), as shown in the 1-s amplification with the background frequency in Alpha (Figure 1A, center) with spectrogram showing greater energy intensity below 10 Hz (Figure 1A, right). For the Control SLD group, the ECoG showed an increase in amplitude to 0.06 mV (Figure 1B, left), demonstrated in the 1-s amplification, with a preponderance of the background frequency in Alpha (Figure 1B, center). The frequency spectrogram showed a greater distribution of energy between the frequencies up to 40 Hz (Figure 1B, right).
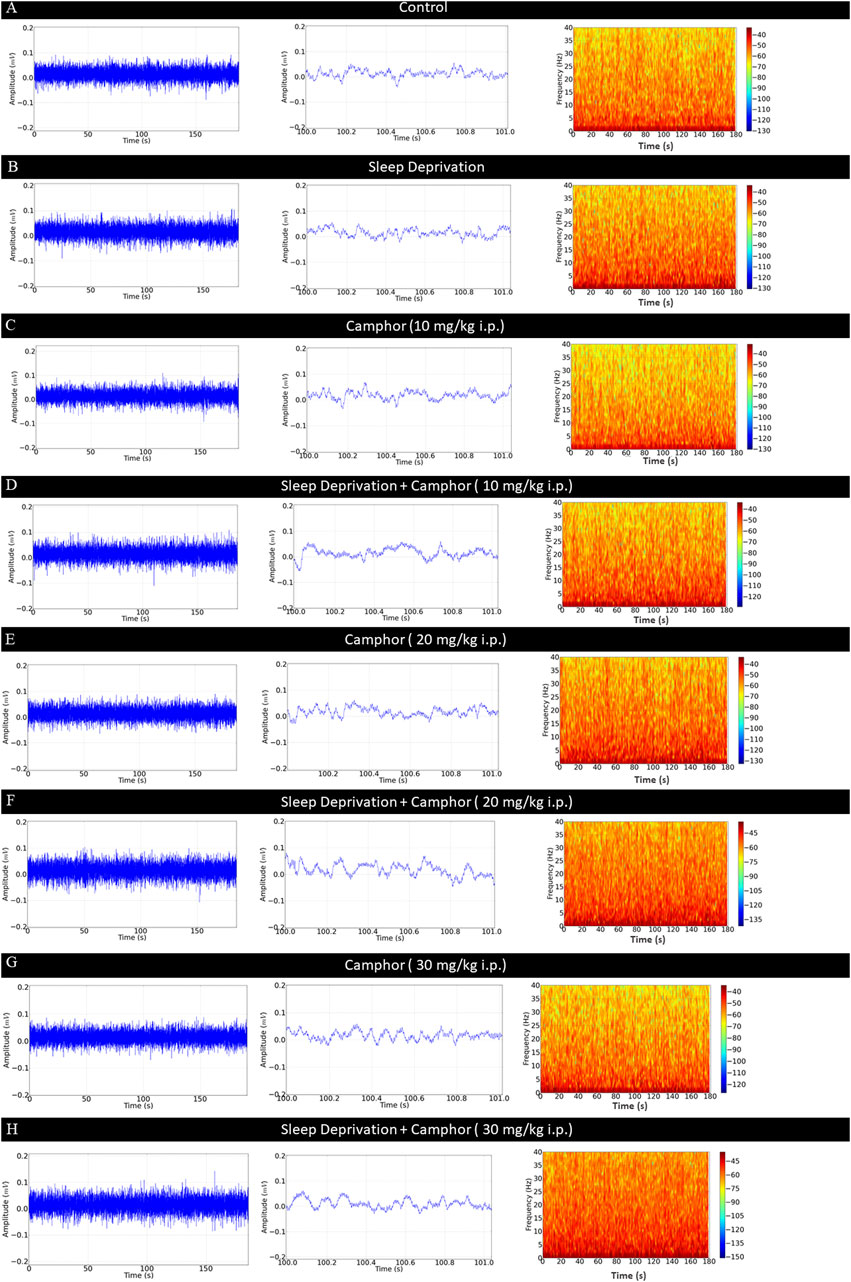
Figure 1. Demonstrations of 3-min ECoG recordings. ECoG tracing of the Control group (left), 1-s amplification of the tracing (center) and power distribution spectrogram (right) (A); ECoG tracing of the Control SLD group (left), 1-s amplification of the recording (center) and respective spectrogram with power distribution in frequencies up to 40 Hz (right) (B); ECoG tracing of the CPH 10 group (left), amplification of the recording in 1 s (center) and spectrogram (right) (C); ECoG tracing of the SLD- CPH 10 group (left), amplification of the recording in 1 s (center) and spectrogram (right) (D); ECoG tracing of the CPH 20 group (left), 1-s amplification (center) and spectrogram of energy distribution in frequencies up to 40 Hz (right) (E); ECoG tracing of the SLD - CPH 20 group (left), 1-s amplification (center) and spectrogram of energy distribution in frequencies up to 40 Hz (right) (F); ECoG recording of the CPH 30 group (left), amplification (center) and spectrogram (right) (G); ECoG recording of the SLD - CPH 30 group (left), amplification (center) and spectrogram (right) (H).
The electrocorticographic recording of the CPH 10 group showed amplitude with a variation of up to 0.05 mV (Figure 1C, left) as shown in the amplification of the recording (Figure 1C, center). The spectrogram shows energy intensity below 5 Hz with a lower distribution of energy in the frequencies up to 40 Hz (Figure 1C, right). The SLD-CPH 10 group showed an increase in the amplitude of the ECoG tracing by 0.07 mV with a preponderance of low frequency rhythms (Figure 1D, left), the amplification of the recording shows background Alpha oscillations (8–12 Hz) (Figure 1D, center), and the spectrogram shows a power distribution below 15 Hz (Figure 1D, right).
The CPH 20 group displayed an amplitude of 0.05 mV (Figure 1E, left). This group showed a preponderance of low-frequency oscillations (Figure 1E, center), as the spectrogram showed power distribution up to 10 Hz (Figure 1E, right). The SLD-CPH 20 group displayed trace slowdown with a preponderance of frequencies between Delta, Theta, and Alpha. There was also a considerable gain in the power at the frequencies from 1 to 12 Hz (Figure 1F, left), which is a characteristic of electroencephalogram (EEG) and ECoG slowing (Figure 1F, center). The spectrogram demonstrated an energy intensity above 15 Hz (Figure 1F, right), but greater intensity up to 40 Hz.
The electrocorticographic recordings for the CPH 30 group displayed an amplitude up to 0.05 mV (Figure 1G, right) with a decrease in frequency that can be observed in the amplification of the recording (Figure 1G, center). The spectrogram showed energy intensity below 15 Hz (Figure 1G, right). For the SLD-CPH 30 group, the animals’ electrocorticographic tracings showed a preponderance of low frequencies such as Delta, Theta, Alpha, and Beta (below 30 Hz). The amplification of the recordings demonstrates a trace slowdown (Figure 1H, center). The spectrogram shows a greater distribution of power up to 40 Hz than the other groups (Figure 1H, right).
The mean power in the Control group (0.4588 ± 0.034 mV2/Hz × 10−³) was lower than the Control SLD group (0.587 ± 0.0668 mV2/Hz × 10−³) but was similar to the groups: CPH 10 (0.536 ± 0.0668 mV2/Hz × 10−³) (p= 0.1720), SLD-CPH 10 (0.546 ± 0.0699 mV2/Hz × 10−³) (p= 0.0804), CPH 20 (0.482 ± 0.0861 mV2/Hz × 10−³) (p= 0.993), and CPH 30 (0.505 ± 0.0670 mV2/Hz × 10−³) (p= 0.769). The Control group had lower potency than the SLD-CPH 20 (0.689 ± 0.0639 mV2/Hz × 10−³) and SLD-CPH 30 (0.710 ± 0.0516 mV2/Hz × 10−³) groups. The Control SLD group had a mean power similar to the CPH 10 (p= 0.685), SLD-CPH 10 (p= 0.8686) and the CPH 30 groups (p= 0.1275). The mean linear potency was higher than that of the CPH 20 group, but it was lower than the mean potency of the SLD-CPH 20 and SLD-CPH 30 groups. The mean potency of the CPH 10 group was similar to the SLD-CPH 10 group (p = 0.999). The mean potency of the CPH 20 and CPH 30 groups was lower when compared to the SLD-CPH 20 and SLD-CPH 30 groups (Figure 2A).
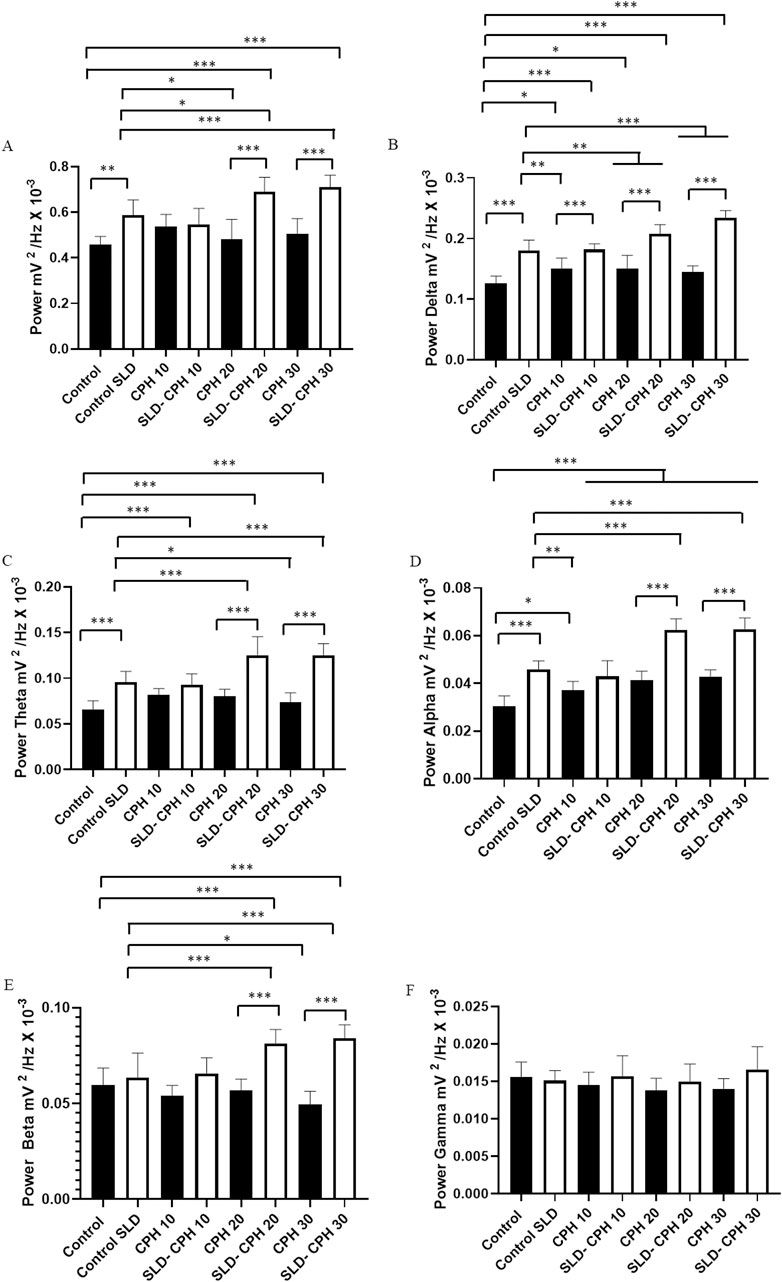
Figure 2. Graph of linear power distribution in the frequencies up to 40 Hz in the ECoG recordings between the groups with different camphor treatments (A); Average linear power between the groups with frequency in delta oscillations (1–4 Hz) (B); average power distribution in theta frequency (4–8 Hz) for the groups (C); Graph of linear power distribution in the recordings between the groups in the oscillations in Alpha (8–12 Hz) (D); Average power distribution of the groups in the frequency in beta (12–28 Hz) (E); Graph of linear power distribution between the groups in the frequency in gamma (28–40 Hz) (F). (After ANOVA followed by Tukey, *P< 0.05 **P< 0.01 ***P< 0.001, n = 9).
For Delta oscillations (1–4 Hz), the Control group (0.1258 ± 0.0122 mV2/Hz × 10−³) had lower mean Delta power than the groups: Control SLD (0.180 ± 0.0173 mV2/Hz × 10−³), CPH 10 (0.150 ± 0.0173 mV2/Hz × 10−³), SLD- CPH 10 (0.182 ± 0.009 mV2/Hz × 10−³), CPH 20 (0.150 ± 0.022 mV2/Hz × 10−³), SLD-CPH 20 (0.207 ± 0.0153 mV2/Hz × 10−³) and SLD-CPH 30 (0.234 ± 0.0116 mV2/Hz × 10−³), but was similar to the CPH 30 group (0.144 ± 0.0105 mV2/Hz × 10−³) (p = 0.160). The Control SLD group had a higher value than the groups treated with camphor alone, however, it was lower than the SLD-CPH 20 and SLD-CPH 30 groups. All the camphor-treated groups showed lower Delta power when compared to the SLD-CPH 10, SLD-CPH 20, and SLD-CPH 30 groups (Figure 2B).
The mean Theta power in the Control group (0.0654 ± 0.0099 mV2/Hz × 10−³) was lower than the Control SLD (0.0955 ± 0.0120 mV2/Hz × 10−³), SLD-CPH 10 (0.0928 ± 0.0119 mV2/Hz × 10−³), SLD-CPH 20 (0.125 ± 0.0202 mV2/Hz × 10−³) and SLD-CPH 30 groups (0.124 ± 0.0130 mV2/Hz × 10−³), but it was similar to the CPH 10 (0.0821 ± 0.0066 mV2/Hz × 10−³) (p = 0.0829), CPH 20 (0.080 ± 0.0078 mV2/Hz × 10−³) (p = 0187) and CPH 30 groups (0.073 ± 0.010 mV2/Hz × 10−³) (p= 0.8023). The Control SLD group had lower mean Theta power than the SLD-CPH 20 and SLD-CPH 30 groups and was higher than the CPH 30 group. The Control SLD group was similar to the following groups: CPH 10 (p = 0.2818), SLD-CPH 10 (p = 0.999) and CPH 20 (p= 0.1355). The CPH 10 group was similar to the SLD-CPH 10 group (p = 0.5563). The power of the CPH 20 and CPH 30 groups was weaker than the SLD-CPH 10, SLD-CPH 20 and SLD-CPH 30 groups (Figure 2C).
The mean power of Alpha oscillations for the Control group (0.0304 ± 0.0043 mV2/Hz × 10−³) was lower than the following groups: Control SLD (0.0458 ± 0.0035 mV2/Hz × 10−³), CPH 10 (0.0371 ± 0.0036 mV2/Hz × 10−³), SLD-CPH 10 (0.042 ± 0.0065 mV2/Hz × 10−³), CPH 20 (0.041 ± 0.0037 mV2/Hz × 10−³), SLD-CPH 20 (0.062 ± 0.0046 mV2/Hz × 10−³), CPH 30 (0.042 ± 0.0029 mV2/Hz × 10−³) and SLD-CPH 30 (0.062 ± 0.0046 mV2/Hz × 10−³). The Control SLD group had greater power than the CPH 10 group and lower than the SLD- CPH 20 and SLD- CPH 30 groups but was similar to the following groups: SLD-CPH 10 (p = 0.859), CPH 20 (p = 0.403) and CPH 30 (p= 0.818). The SLD-CPH 20 and SLD-CPH 30 groups had higher power means than the groups without sleep deprivation (Figure 2D).
The mean power in Beta oscillations for the Control group (0.059 ± 0.0086 mV2/Hz × 10−³) was similar to the Control SLD (0.063 ± 0.012 mV2/Hz × 10−³) (p= 0.977), CPH 10 (0.054 ± 0.0054 mV2/Hz × 10−³) (p = 0.790), SLD- CPH 10 (0.0657 ± 0.0060 mV2/Hz × 10−³) (p= 0.773), CPH 20 (0.0566 ± 0.0060 mV2/Hz × 10−³) (p= 0.999), and CPH 30 groups (0.049 ± 0.0067 mV2/Hz × 10−³) (p= 0.141). The Control group Beta power was weaker than the SLD-CPH 20 (0.0813 ± 0.0072 mV2/Hz × 10−³) and SLD-CPH 30 groups (0.084 ± 0.0068 mV2/Hz × 10−³). The groups of animals deprived of sleep showed similar power in Beta oscillations to the CPH 10 (p= 0.216), SLD-CPH 10 (p= 0.998) and SLD-CPH 20 groups (p= 0.626). However, the Control SLD group had a power mean power than the SLD- CPH 20 and SLD-CPH 30 groups (Figure 2E).
The average power in Gamma oscillations for the Control group (0.0155 ± 0.0020 mV2/Hz × 10−³) was similar to the following groups: Control SLD (0.0150 ± 0.0013 mV2/Hz × 10−³) (p = 0.999), CPH 10 (0.0145 ± 0.0017 mV2/Hz × 10−³) (p= 0.969), SLD-CPH 10 (0.0157 ± 0.0027 mV2/Hz × 10−³) (p= 0.999), CPH 20 (0.0137 ± 0.0016 mV2/Hz × 10−³) (p= 0.643), SLD-CPH 20 (0.0149 ± 0.0023 mV2/Hz × 10−³), CPH 30 (0.0140 ± 0.0135 mV2/Hz × 10−³) (p= 0.786), and SLD-CPH 30 (0.016 ± 0.0030 mV2/Hz × 10−³) (p= 0.961) (Figure 2F).
By individualizing the frequencies in the Alpha spectrum, we can assess camphor’s contribution to the increase of Alpha waves. This way, the mean power evaluated between 8 and 9 Hz for the Control group (0.00786 ± 0.00083 mV2/Hz × 10−³) was lower than the following groups: Control SLD, (0.010 ± 0.00043 mV2/Hz × 10−³), CPH 10 (0.011 ± 0.00075 mV2/Hz × 10−³), SLD- CPH 10 (0.012 ± 0.00077 mV2/Hz × 10−³), CPH 20 (0.0112 ± 0.00083 mV2/Hz × 10−³), SLD-CPH 20 (0.0153 ± 0.00190 mV2/Hz × 10−³), CPH 30 (0.0120 ± 0.0016 mV2/Hz × 10−³), and SLD-CPH 30 (0.0166 ± 0.0010 mV2/Hz × 10−³). The Control SLD group power was lower than the SLD-CPH 10, SLD-CPH 20, SLD-CPH 30 groups. And it was similar to the CPH 10 (p= 0.964), CPH 20 (p= 0.7716), CPH 30 (p = 0.077) groups. The CPH 10 group was similar to the SLD-CPH 10 group (p= 0.1907). The SLD-CPH 20, SLD-CPH 30 groups had higher powers than the groups treated with camphor alone (Figure 3A).
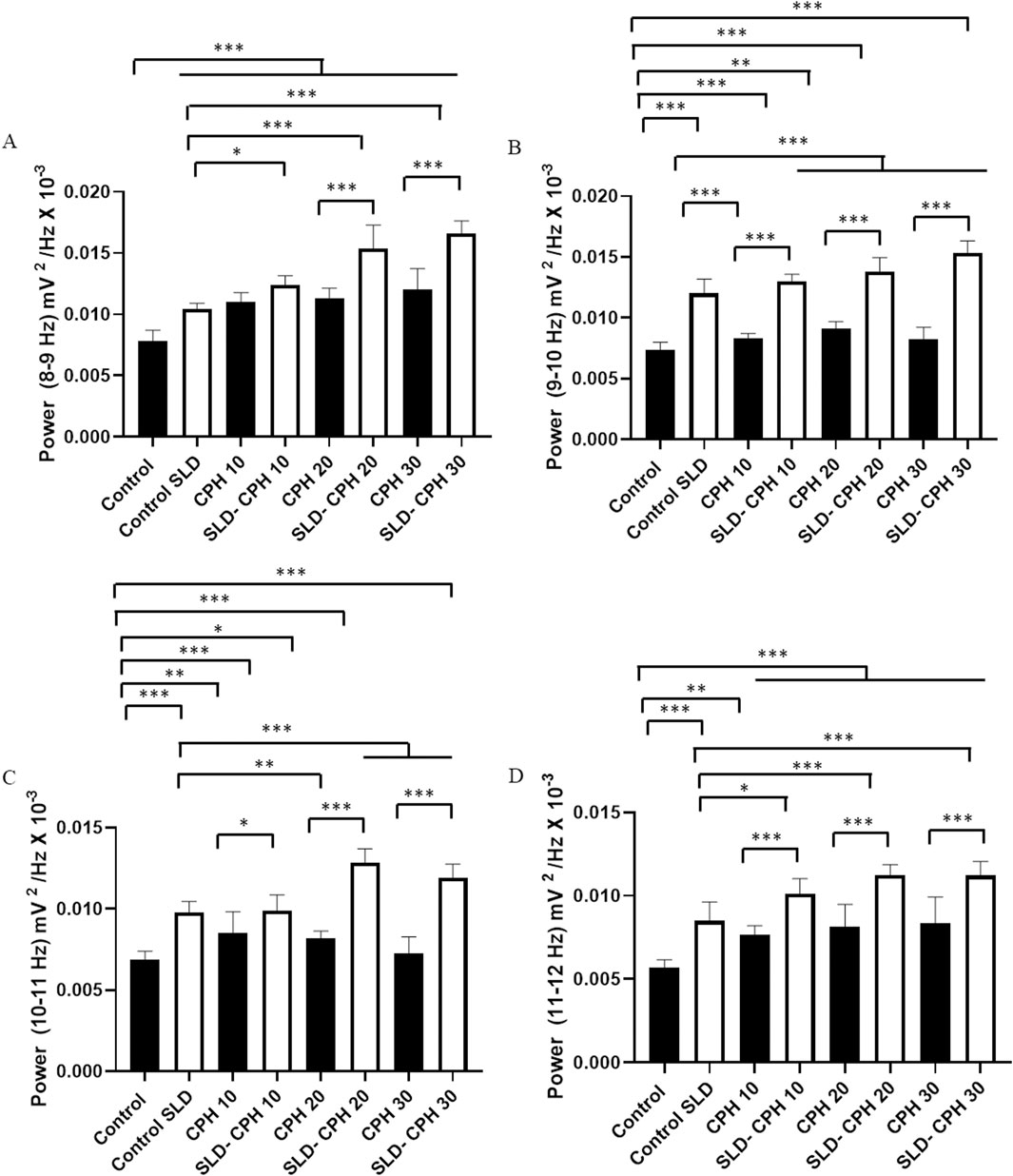
Figure 3. Linear power distribution graph for each frequency that makes up the alpha oscillations (8–12 Hz): Average power at frequencies between (8–9 Hz) (A); Average power at frequencies between 9–10 Hz (B); Average power between 10–11 Hz (C); Average power between 11–12 Hz (D). (After ANOVA followed by Tukey, *P< 0.05 **P< 0.01 ***P< 0.001, n = 9).
The mean power evaluated between 9 and 10 Hz for the Control group (0.00737 ± 0.00061 mV2/Hz × 10−³) was lower than all the sleep deprived groups: Control SLD (0.0120 ± 0.00116 mV2/Hz × 10−³), SLD-CPH 10 (0.0129 ± 0.00059 mV2/Hz × 10-³), SLD-CPH 20 (0.0138 ± 0.0011 mV2/Hz × 10−³), SLD-CPH 30 (0.0152 ± 0.00102 mV2/Hz × 10−³). The camphor-treated groups were similar to the Control group: CPH 10 (0.0083 ± 0.00039 mV2/Hz × 10−³) (p= 0.3080), and the CPH 30 (0.0082 ± 0.00103 mV2/Hz × 10−³) (p= 0.470). The CPH 20 group (0.00911 ± 0.00058 mV2/Hz × 10−³) was lower than the Control group. The sleep-deprived group showed higher mean power than the camphor-treated groups. However, it was lower than the SLD-CPH 20 and SLD-CPH 30 groups (Figure 3B).
The mean power evaluated between 10 and 11 Hz for the Control group (0.00689 ± 0.00049 mV2/Hz × 10−³) was lower than the groups: Control SLD (0.0097 ± 0.00067 mV2/Hz × 10−³), CPH 10 (0.00852 ± 0.001304 mV2/Hz × 10−³), SLD-CPH 10 (0.0098 ± 0.00098 mV2/Hz × 10−³), CPH 20 (0.0081 ± 0.00044 mV2/Hz × 10−³), SLD-CPH 20 (0.0128 ± 0.00086 mV2/Hz × 10−³), and SLD- CPH 30 (0.0119 ± 0.00084 mV2/Hz × 10−³). However, it was similar to the CPH 30 group (0.0072 ± 0.000998 mV2/Hz × 10−³) (p= 0.9821). The Control SLD group had a lower mean power than the SLD-CPH 20 and SLD-CPH 30 groups. The CPH 20 and CPH 30 groups had lower mean power. The CPH 10 group (p= 0.060) and the SLD-CPH 10 group (p= 0.999) were similar. The SLD-CPH 10, SLD-CPH 20, SLD-CPH 30 groups had higher means than the groups treated with camphor alone (Figure 3C).
The mean power evaluated between 11 and 12 Hz for the Control group (0.0056 ± 0.00049 mV2/Hz × 10−³) was lower than the following groups: Control SLD (0.0084 ± 0.0011 mV2/Hz × 10−³), CPH 10 (0.0076 ± 0.00052 mV2/Hz × 10−³), SLD-CPH 10 (0.010 ± 0.00090 mV2/Hz × 10−³), CPH 20 (0.0081 ± 0.0013 mV2/Hz × 10−³), SLD CPH 20 (0.011 ± 0.00061 mV2/Hz × 10−³), CPH 30 (0.0083 ± 0.0015 mV2/Hz × 10−³) and SLD-CPH 30 (0.011 ± 0.00084 mV2/Hz × 10−³). The Control SLD group had a lower mean than the SLD-CPH 10, SLD-CPH 20 and SLD-CPH 30 groups. And it was similar to the CPH 10 (p= 0.6614), CPH 20 (p= 0.996) and CPH 30 (p = 0.999). The CPH 10, CPH 20, and CPH 30 groups had lower values than the SLD-CPH 10, SLD-CPH 20, and SLD-CPH 30 groups (Figure 3D).
As for the behavioral analyses, the first observed behavior in the animals was the total relaxation of the testicles while they remained in the scrotum, and the latency time for this behavior was shorter with higher doses of camphor. Shortly afterward, the animals showed decreased motility, remaining still for longer periods, although stimuli could provoke movement. This pattern exhibited similar characteristics in terms of latency of occurrence and administered dose to the previous test. Regarding the induction of light sleep, the only dose that induced sleep in the animals was 30 mg/kg i.p., with a latency of 553.8 ± 46.33 s. The induced sleep was superficial, and the animals could be awakened by sound and tactile stimuli (Table 1).
4 Discussion
Camphor, a crystalline white substance with a strong odor, is one of the byproducts extracted from this plant and has various known pharmacological applications (Hamidpour et al., 2013). Not much is known about the mechanism of action of camphor on the central nervous system. Vatanparast and Andalib-Lari., 2017 proposed, through experiments on snail neurons, that camphor can inhibit K+ channels, resulting in increased Ca2+ influx into neurons. This inhibition reduces the neuron’s hyperpolarization time and facilitates the generation of action potentials. At higher doses of the substance, this may lead to epileptiform patterns on the electroencephalogram, marked by waves with multiple peaks (polyspike waves) indicative of ictal activity (Ferreira et al., 2020). The hyperpolarization phase typically serves as a negative feedback mechanism to regulate neuronal excitability (Janahmadi et al., 2008; Vatanparast and Andalib-Lari., 2017 proposed mechanism of action justifies well how camphor is capable of inducing seizures, but it is still unclear how low doses of camphor induce light sleep as observed in our results.
Studies have shown that zolpidem, a widely used hypnotic drug that acts through interaction with γ-aminobutyric acid receptor subunit alpha-1, tends to intensify Delta waves and attenuate the power of waves in the 4–12 Hz range, particularly Theta waves, while also intensifying Beta waves in the 25–30 Hz range. Consequently, this drug is effective in prolonging total sleep time and slow-wave sleep (SWS). In comparison, camphor also increases the power of Delta and Beta waves; however, it additionally tends to potentiate Theta and Alpha waves (Crestani et al., 2000; Landolt et al., 2000; Kopp et al., 2004; Bettica et al., 2012; Fox et al., 2013).
Baclofen, in the other hand, is a γ-aminobutyric acid type B (GABAb) receptor agonist that has similar effects to camphor in the CNS. It induces a hypersynchronous slow wave EEG pattern characterized by high amplitude Delta frequencies, enhancing the power density in non-rapid eye movement (NREM) sleep significantly in the 2.25–5.5 and 10.75–14.75 Hz, which are correspondent to Delta, Theta, and Alpha frequencies. It also generates behavioral alterations consistent with relaxation that depended on other variables such as light exposure, and was able to increase sleep intensity (Pressman, 2004; Hodor et al., 2015).
Gamma-hydroxybutyrate, another GABAb receptor agonist, induces a hypersynchronous EEG pattern similar to that of Baclofen. It increases EEG power density in NREM sleep within the frequency range associated with slow-wave activity. However, a significant effect was observed only under low light exposure (Hodor et al., 2015). Based on these electroencephalographic similarities, we hypothesize that low doses of camphor may have agonistic effects on GABAb receptors, which could explain its effects on the CNS.
Despite the current limited understanding of the neural mechanisms underlying cognitive impairments and electrocortical alterations caused by sleep restriction, Vyazovskiy et al. (2013) suggest that prolonged periods of wakefulness affect the cortex’s responsiveness to stimuli by altering cortical bistability, in other words, the ability of neurons to stabilize themselves in a state of both depolarization and hyperpolarization (Wilson, 2008). According to the author, heightened excitability, functional connectivity, and bistability increase the likelihood of bursts of activity, as cortical responsiveness is enhanced, with stimuli often coming from sources far from the neuronal network. This finding could explain why rats exposed to both sleep deprivation and camphor show a significant increase in Delta, Theta, Alpha, and Beta wave power compared to those not subjected to sleep deprivation. The altered responsiveness of the neurons may synergize with camphor’s action, thereby enhancing brain wave power, especially at lower frequencies.
Furthermore, it is known that sleep deprivation can promote the detachment of pericytes from the endothelium of cerebral vessels by decreasing the expression of proteins important for maintaining the integrity of the blood-brain barrier (Medina-Flores et al., 2020), thus increasing the permeability of the blood-brain barrier by multiple factors and possibly allowing greater passage of camphor into brain tissue. Therefore, it is possible that sleep deprivation allows camphor to reach neurons more easily, increasing its concentration in the tissue and potentiating its effects, especially considering camphor’s highly lipophilic structure, which would have a high affinity for brain tissue, rich in lipids (Vatanparast and Andalib-Lari, 2017).
Our results also showed that there was a decrease in the predominant frequency of brain oscillatory patterns in the group exposed to 10 mg/kg i.p. of camphor, shifting the oscillatory patterns toward the Alpha frequency range. When administered 20 and 30 mg/kg i.p., there was a preponderance of low-frequency Delta, Theta-Theta, and Alpha bands, indicating an oscillatory pattern with deceleration characteristics, with a considerable gain in power in the 1–12 Hz range. When observed at a dose of 30 mg/kg i.p., despite the pattern of trace slowdown, there was also an intensification in the high frequency bands, especially Beta, which must be correlated with the excitatory effect of camphor on neurons. It was observed that the power of the Delta, Theta, Alpha, and Beta waves changed in a dose-dependent manner and the Alpha waves showed an increase in distribution between the 8–10 Hz and 11–12 Hz frequency bands, also in a dose-dependent manner. All the changes described were potentiated by sleep deprivation.
These findings indicate camphor exhibits properties suggestive of a sleep-inducing agent when administered within therapeutic concentrations. Specifically, camphor induces electrocorticographic patterns of trace slowdown, which is characterized by a preponderance of low-frequency oscillations akin to those observed during physiological sleep. Moreover, our behavioral evaluation demonstrated light sleep induction within non-sleep-deprived rats that received 30 mg/kg i.p. of camphor, preceded by decreased mobility and testicular relaxation, further supporting this hypothesis.
According to the most common classification, sleep is divided into 5 phases: Wake, characterized by low-amplitude Beta waves in alert states, and by a predominance of Alpha waves in states of relaxation, similar to those obtained in our study among groups that received doses of 10 mg/kg i.p. of camphor; N1 - Light Sleep, characterized by low voltage Theta waves; N2 - Heavy Sleep, characterized by sleep spindles and K complexes; N3 - SWS, characterized by higher amplitude Delta waves; and rapid eye movement (REM) sleep, characterized by the presence of Beta waves similar to the alert state. Our findings show similarities in the tracings of the sleep deprived groups who received doses of 20 and 30 mg/kg i.p. with brainwave oscillatory sleep patterns. The sleep deprived group that received 20 mg/kg showed patterns that were similar to SWS due to the trace slowdown and potentiation of Delta waves, while the 30 mg/kg group resembled REM sleep patterns, due to the trace slowdown plus the appearance of Beta waves. These findings could indicate that camphor can facilitate sleep induction through changes in neuronal activity, which is potentiated by sleep deprivation. However, due to the neuro-excitatory nature of camphor, higher doses could induce the appearance of increasingly higher frequencies, up to the appearance of potentially harmful epileptiform patterns.
It is important to note that camphor can induce toxicity when administered in high doses. Gastrointestinal symptoms frequently arise due to direct irritation of the mucous membranes, oxidative stress, and cardiotoxicity (Eldridge et al., 2007; Love et al., 2004; Du et al., 2021). Additionally, camphor toxicity can precipitate neurological effects, including agitation, anxiety, spasms, convulsions, hallucinations, hyperreflexia, and, in severe cases, coma and respiratory arrest, with these severe outcomes more commonly observed in pediatric patients (Rahimi et al., 2017; Eldridge et al., 2007; Love et al., 2004; Phelan, 1976; Bahr et al., 2019). The International Programme on Chemical Safety (1989) estimates that the probable lethal oral dose of pure camphor is approximately 4 g for adults, while in children, the lethal dose is estimated to be between 0.5 and 1 g (70 mg/kg). In rats, the intraperitoneal lethal dose is reported to be 900 mg/kg.
In conclusion, applying camphor to sleep-deprived Wistar rats alters occipital electrocorticographic patterns by increasing the total mean power of spikes, especially in the frequency bands of Delta, Theta, Alpha, and Beta, and inducing sleep-like behavior in Wistar rats. This activity is likely due to multiple factors, including the synergistic effect of sleep deprivation and camphor and the increased blood-brain barrier permeability caused by sleep deprivation. While camphor may be potentially harmful at high doses due to its toxicity, it could be a useful adjunctive medication in the treatment of insomnia. However, further studies are needed to confirm its efficacy.
Data availability statement
The raw data supporting the conclusions of this article will be made available by the authors, without undue reservation.
Ethics statement
The animal study was approved by the animal study was approved by the Animal Ethics Committee (CEUA—UFPA) number 1380040822 [002046]. The study was conducted in accordance with the local legislation and institutional requirements. The study was conducted in accordance with the local legislation and institutional requirements.
Author contributions
ALGA: Methodology, Investigation, Writing–original draft, Writing–review and editing. GBB: Methodology, Investigation, Writing–original draft, Writing–review and editing. MFS: Writing–review and editing. LVS: Writing–review and editing. RGS: Methodology, Investigation, Writing–original draft. TMC: Methodology, Writing–review and editing. TSR: Writing–review and editing. PFPH: Writing–review and editing. LEQ: Writing–review and editing. CAP: Writing–review and editing. DBA: Writing–review and editing. DCFL: Writing–review and editing. MTS: Writing–review and editing. MH: Data curation, Methodology, Supervision, Conceptualization, Formal analysis, Project administration, Validation, Investigation, Funding acquisition, Resources, Visualization, Software, Writing–original draft, Writing–review and editing.
Funding
The author(s) declare financial support was received for the research, authorship, and/or publication of this article. This work was supported by the Fundação Amazônia de Amparo a Estudos e Pesquisas do Estado do Pará (FAPESPA); the Coordenação de Aperfeiçoamento de Pessoal de Nível Superior (CAPES); the Conselho Nacional de Desenvolvimento Científico e Tecnológico (CNPQ); the Pró-Reitoria de Pesquisa e Pós-Graduação - PROPESP/UFPA and Federal University of Pará (UFPA).
Acknowledgments
The authors would like to acknowledge Programa Institucional de Bolsas de Iniciação Científica (PIBIC)/UFPA for their financial support provided of this research and Programa de Pós graduação em Farmacologia e Bioquímica (FARMABIO).
Conflict of interest
The authors declare that the research was conducted in the absence of any commercial or financial relationships that could be construed as a potential conflict of interest.
Publisher’s note
All claims expressed in this article are solely those of the authors and do not necessarily represent those of their affiliated organizations, or those of the publisher, the editors and the reviewers. Any product that may be evaluated in this article, or claim that may be made by its manufacturer, is not guaranteed or endorsed by the publisher.
References
Bahr, T. A., Rodriguez, D., Beaumont, C., and Allred, K. (2019). The effects of various essential oils on epilepsy and acute seizure: a systematic review. Evid. Based Complement. Altern. Med. 6216745 2019, 1–14. doi:10.1155/2019/6216745
Ballestar-Tarín, M. L., Ibáñez-Del Valle, V., Mafla-España, M. A., Cauli, O., and Navarro-Martínez, R. (2023). Increased salivary IL-1 beta level is associated with poor sleep quality in university students. Diseases 11 (4), 136. doi:10.3390/diseases11040136
Bara, A. C., and Arber, S. (2009). Working shifts and mental health--findings from the British household panel survey (1995-2005). Scand. J. Work Environ. Health 35 (5), 361–367. doi:10.5271/sjweh.1344
Barker, M. J., Greenwood, K. M., Jackson, M., and Crowe, S. F. (2004). Persistence of cognitive effects after withdrawal from long-term benzodiazepine use: a meta-analysis. Archives Clin. Neuropsychology 19, 437–454. doi:10.1016/s0887-6177(03)00096-9
Bettica, P., Squassante, L., Groeger, J. A., Gennery, B., Winsky-Sommerer, R., and Dijk, D. J. (2012). Differential effects of a dual orexin receptor antagonist (SB-649868) and zolpidem on sleep initiation and consolidation, SWS, REM sleep, and EEG power spectra in a model of situational insomnia. Neuropsychopharmacology 37 (5), 1224–1233. doi:10.1038/npp.2011.310
Chen, W., Vermaak, I., and Viljoen, Á. (2013). Camphor—a fumigant during the black death 424 and a coveted fragrant wood in ancient Egypt and babylon—a review. Molecules 18 (5), 5434–5454. doi:10.3390/molecules18055434
Crestani, F., Martin, J. R., Möhler, H., and Rudolph, U. (2000). Mechanism of action of the hypnotic zolpidem in vivo. Br. J. Pharmacol. 131 (7), 1251–1254. doi:10.1038/sj.bjp.0703717
Dougnon, G., and Ito, M. (2021). Role of ascaridole and p-cymene in the sleep-promoting effects of Dysphania ambrosioides essential oil via the GABAergic system in a ddY mouse inhalation model. J. Nat. Prod. 84 (1), 91–100. doi:10.1021/acs.jnatprod.0c01137
Du, Z. C., Xia, Z. S., Zhang, M. Z., Wei, Y. T., Malhotra, N., Saputra, F., et al. (2021). Sub-lethal camphor exposure triggers oxidative stress, cardiotoxicity, and cardiac physiology alterations in zebrafish embryos. Cardiovasc. Toxicol. 21 (11), 901–913. doi:10.1007/s12012-021-09682-x
Eldridge, D. L., Eyk, J. V., and Kornegay, C. (2007). Pediatric Toxicology. Emerg. Med. Clin. North Am. 25 (2), 283–308. doi:10.1016/j.emc.2007.02.011
Everitt, H., Baldwin, D. S., Stuart, B., Lipinska, G., Mayers, A., Malizia, A. L., et al. (2018). Antidepressants for insomnia in adults. Cochrane Database Syst. Rev. 5, D010753. doi:10.1002/14651858.cd010753.pub2
Ferreira, L. O., de Souza, R. D., Silva, F. A., Costa, F. F. M., Farias, R. A. F., Hamoy, A. O., et al. (2020). Electrocorticographic patterns dominated by low-frequency waves in camphor-induced seizures. Sci. Rep. 10, 18222. doi:10.1038/s41598-020-75309-w
Fonken, L. K., Kitsmiller, E., Smale, L., and Nelson, R. J. (2012). Dim nighttime light impairs cognition and provokes depressive-like responses in a diurnal rodent. J. Biol. Rhythms 27 (4), 319–327. doi:10.1177/0748730412448324
Fox, S. V., Gotter, A. L., Tye, S. J., Garson, S. L., Savitz, A. T., Uslaner, J. M., et al. (2013). Quantitative electroencephalography within sleep/wake states differentiates GABAA modulators eszopiclone and zolpidem from dual orexin receptor antagonists in rats. Neuropsychopharmacology 38 (12), 2401–2408. doi:10.1038/npp.2013.139
Gale, E. L., Williams, A. J., and Cecil, J. E. (2024). The relationship between multiple sleep dimensions and obesity in adolescents: a systematic review. Sleep. Med. Rev. 73, 101875. doi:10.1016/j.smrv.2023.101875
Hamidpour, R., Hamidpour, S., Hamidpour, M., and Shahlari, M. (2013). Camphor (Cinnamomum camphora), a traditional remedy with the history of treating several diseases. Int. J. Case Rep. Imag. 4 (2), 86. doi:10.5348/ijcri-2013-02-267-RA-1
Hamoy, M., Batista, L. D. S., de Mello, V. J., Gomes-Leal, W., Farias, R. A. F., Batista, P. D. S., et al. (2018). Cunaniol-elicited seizures: behavior characterization and electroencephalographic analyses. Toxicol. Appl. Pharmacol. 360, 193–200. doi:10.1016/j.taap.2018.10.008
Henry, M., Thomas, K. G. F., and Ross, I. L. (2021). Sleep, cognition and cortisol in addison's disease: a mechanistic relationship. Front. Endocrinol. (Lausanne) 12, 694046. doi:10.3389/fendo.2021.694046
Hodor, A., Palchykova, S., Gao, B., and Bassetti, C. L. (2015). Baclofen and gamma-hydroxybutyrate differentially altered behavior, EEG activity and sleep in rats. Neuroscience 28, 18–28. doi:10.1016/j.neuroscience.2014.08.061
International Programme on Chemical Safety (1989). Camphor. Available at: http://www.inchem.org/documents/pims/pharm/camphor.htm (Accessed September 1, 2024).
Jalilifar, M., and Yadollahpour, A. (2017). Dataset of quantitative spectral EEG of different stages of kindling acquisition in rats. Data Brief 16, 239–243. doi:10.1016/j.dib.2017.11.045
Janahmadi, M., Farajnia, S., Vatanparast, J., Abbasipour, H., and Kamalinejad, M. (2008). The fruit essential oil of Pimpinella anisum L. (Umblliferae) induces neuronal hyperexcitability in snail partly through attenuation of after-hyperpolarization. J. Ethnopharmacol. 120 (3), 360–365. doi:10.1016/j.jep.2008.09.008
Kalmbach, D. A., Pillai, V., Cheng, P., Arnedt, J. T., and Drake, C. L. (2015). Shift work disorder, depression, and anxiety in the transition to rotating shifts: the role of sleep reactivity. Sleep. Med. 16 (12), 1532–1538. doi:10.1016/j.sleep.2015.09.007
Karlsson, B., Knutsson, A., and Lindahl, B. (2001). Is there an association between shift work and having a metabolic syndrome? Results from a population based study of 27,485 people. Occup. Environ. Med. 58, 747–752. doi:10.1136/oem.58.11.747
Kopp, C., Rudolph, U., and Tobler, I. (2004). Sleep EEG changes after zolpidem in mice. Neuroreport 15 (14), 2299–2302. doi:10.1097/00001756-200410050-00031
Landolt, H. P., Finelli, L. A., Roth, C., Buck, A., Achermann, P., and Borbély, A. A. (2000). Zolpidem and sleep deprivation: different effect on EEG power spectra. J. Sleep. Res. 9 (2), 175–183. doi:10.1046/j.1365-2869.2000.00192.x
Lee, H. Y., Kim, M. S., Kim, O., Lee, I. H., and Kim, H. K. (2016). Association between shift work and severity of depressive symptoms among female nurses: the Korea Nurses' Health Study. Health Study. J. Nurs. Manag. 24, 192–200. doi:10.1111/jonm.12298
Lee, S. H., Kim, D. S., Park, S. H., and Park, H. (2022). Phytochemistry and applications of Cinnamomum camphora essential oils. Molecules 27 (9), 2695. doi:10.3390/molecules27092695
Lillehei, A. S., and Halcon, L. L. (2014). A systematic review of the effect of inhaled essential oils on sleep. J. Altern. Complement. Med. 20 (6), 441–451. doi:10.1089/acm.2013.0311
Love, J. N., Sammon, M., and Smereck, J. (2004). Are one or two dangerous? Camphor exposure in toddlers. J. Emerg. Med. 27 (1), 49–54. doi:10.1016/j.jemermed.2004.02.010
Marquié, J. C., Tucker, P., Folkard, S., Gentil, C., and Ansiau, D. (2015). Chronic effects of shift work on cognition: findings from the VISAT longitudinal study. Occup. Environ. Med. 72 (4), 258–264. doi:10.1136/oemed-2013-101993
Medina-Flores, F., Hurtado-Alvarado, G., de Oca, A. C. M., López-Cervantes, S. P., Konigsberg, M., Deli, M. A., et al. (2020). Sleep loss disrupts pericyte-brain endothelial cell interactions impairing blood-brain barrier function. Brain Behav. Immun. 89, 118–132. doi:10.1016/j.bbi.2020.05.077
Morgenthaler, T. I., Deriy, L., Heald, J. L., and Thomas, S. M. (2016). The evolution of the AASM clinical practice guidelines: another step forward. J. Clin. Sleep. Med. 12 (1), 129–135. doi:10.5664/jcsm.5412
Papantoniou, K., Castaño-Vinyals, G., Espinosa, A., Aragonés, N., Pérez-Gómez, B., Burgos, J., et al. (2015). Night shift work, chronotype and prostate cancer risk in the MCC-Spain case-control study. Int. J. Cancer 137, 1147–1157. doi:10.1002/ijc.29400
Paxinos, G., and Watson, C. (2013). The rat brain in stereotaxic coordinates. 7th edition. Academic Press.
Phelan, W. J. 3rd. (1976). Camphor poisoning: over-the-counter dangers. Pediatrics 57 (3), 428–431. doi:10.1542/peds.57.3.428
Pressman, M. R. (2004). Hypersynchronous delta sleep EEG activity and sudden arousals from slow-wave sleep in adults without a history of parasomnias: clinical and forensic implications. Sleep 27 (4), 706–710. doi:10.1093/sleep/27.4.706
Qian, Y., Dharmage, S. C., Hamilton, G. S., Lodge, C. J., Lowe, A. J., Zhang, J., et al. (2023). Longitudinal risk factors for obstructive sleep apnea: a systematic review. Sleep. Med. Rev. 71, 101838. doi:10.1016/j.smrv.2023.101838
Rahimi, M., Shokri, F., Hassanian-Moghaddam, H., Zamani, N., Pajoumand, A., and Shadnia, S. (2017). Severe camphor poisoning, a seven-year observational study. Environ. Toxicol. Pharmacol. 52, 8–13. doi:10.1016/j.etap.2017.03.009
Riemann, D., Espie, C. A., Altena, E., Arnardottir, E. S., Baglioni, C., Bassetti, C. L. A., et al. (2023). The European Insomnia Guideline: an update on the diagnosis and treatment of insomnia 2023. J. Sleep Res. 32 (6), e14035. doi:10.1111/jsr.14035
Rössler, W., AjdacicGross, V., Glozier, N., Rodgers, S., Haker, H., and Müller, M. (2017). Sleep disturbances in young and middle-aged adults - empirical patterns and related factors from an epidemiological survey. Compr. Psychiatry 78, 83–90. doi:10.1016/j.comppsych.2017.07.009
Sateia, M. J., Buysse, D. J., Krystal, A. D., Neubauer, D. N., and Heald, J. L. (2017). Clinical practice guideline for the pharmacologic treatment of chronic insomnia in adults: an American Academy of sleep medicine clinical practice guideline. J. Clin. Sleep. Med. 13 (2), 307–349. doi:10.5664/jcsm.6470
Schernhammer, E. S., Kroenke, C. H., Laden, F., and Hankinson, S. E. (2006). Night work and risk of breast cancer. Epidemiology 17 (1), 108–111. doi:10.1097/01.ede.0000190539.03500.c1
Stevens, R. G., Hansen, J., Costa, G., Haus, E., Kauppinen, T., Aronson, K. J., et al. (2011). Considerations of circadian impact for defining 'shift work' in cancer studies: IARC Working Group Report. Occup. Environ. Med. 68 (2), 154–162. doi:10.1136/oem.2009.053512
Straif, K., Baan, R., Grosse, Y., Secretan, B., El Ghissassi, F., Bouvard, V., et al. (2007). Carcinogenicity of shift-work, painting, and fire-fighting. Lancet Oncol. 8 (12), 1065–1066. doi:10.1016/S1470-2045(07)70373-X
Stranks, E. K., and Crowe, S. F. (2014). The acute cognitive effects of zopiclone, zolpidem, zaleplon, and eszopiclone: a systematic review and meta-analysis. J. Clin. Exp. Neuropsyvchology 36, 691–700. doi:10.1080/13803395.2014.928268
Touitou, Y., Reinberg, A., and Touitou, D. (2017). Association between light at night, melatonin secretion, sleep deprivation, and the internal clock: health impacts and mechanisms of circadian disruption. Life Sci. 173, 94–106. doi:10.1016/j.lfs.2017.02.008
Treves, N., Perlman, A., Kolenberg, G. L., Asaly, A., and Matok, I. (2018). Z-drugs and risk for falls and fractures in older adults – a systematic review and meta-analysis. Age Ageing 47, 201–208. doi:10.1093/ageing/afx167
Vallières, A., Azaiez, A., Moreau, V., LeBlanc, M., and Morin, C. M. (2014). Insomnia in shift work. Sleep. Med. 15 (12), 1440–1448. doi:10.1016/j.sleep.2014.06.021
Vatanparast, J., and Andalib-Lari, F. (2017). Camphor elicits epileptiform discharges in snail neurons: the role of ion channels modulation. Neurotoxicology 60, 299–307. doi:10.1016/j.neuro.2015.12.012
Vyazovskiy, V. V., Olcese, U., Cirelli, C., and Tononi, G. (2013). Prolonged wakefulness alters neuronal responsiveness to local electrical stimulation of the neocortex in awake rats. J. Sleep. Res. 22 (3), 239–250. doi:10.1111/jsr.12009
Xiao, S., Liu, S., Yu, H., Xie, Y., Guo, Y., Fan, J., et al. (2022). A study on the mechanism of the sedative-hypnotic effect of Cinnamomum camphora chvar. Borneol essential oil based on network Pharmacology. J. Oleo Sci. 71 (7), 1063–1073. doi:10.5650/jos.ess21278
Nomenclature
Keywords: camphor, electrocorticography, sleep deprivation, insomnia, neuropharmacology, sleep disorders
Citation: Gurgel do Amaral AL, Barbosa GB, Farias dos Santos M, Vasconcelos de Souza L, Gonçalves dos Santos R, Câmara TM, de Sousa Reis T, Pacheco Hartcopff PF, Eiró-Quirino L, Araújo da Paz C, Bastos de Araújo D, Favacho Lopes DC, Saito MT and Hamoy M (2024) Camphor alters occipital electrocorticographic patterns during sleep deprivation in Wistar rats. Front. Nat. Produc. 3:1449362. doi: 10.3389/fntpr.2024.1449362
Received: 14 June 2024; Accepted: 16 September 2024;
Published: 11 October 2024.
Edited by:
Louis Pergaud Sandjo, Federal University of Santa Catarina, BrazilReviewed by:
Tiago Tizziani, Federal University of Santa Catarina, BrazilAnkanahalli N. Nanjaraj Urs, Washington University in St. Louis, United States
Copyright © 2024 Gurgel do Amaral, Barbosa, Farias dos Santos, Vasconcelos de Souza, Gonçalves dos Santos, Câmara, de Sousa Reis, Pacheco Hartcopff, Eiró-Quirino, Araújo da Paz, Bastos de Araújo, Favacho Lopes, Saito and Hamoy. This is an open-access article distributed under the terms of the Creative Commons Attribution License (CC BY). The use, distribution or reproduction in other forums is permitted, provided the original author(s) and the copyright owner(s) are credited and that the original publication in this journal is cited, in accordance with accepted academic practice. No use, distribution or reproduction is permitted which does not comply with these terms.
*Correspondence: Daniella Bastos de Araújo, ZGFuaXZpdmFmQGdtYWlsLmNvbQ==
†These authors have contributed equally to this work and share first authorship