- 1Department of Otology and Skull Base Surgery, Eye Ear Nose and Throat Hospital, Fudan University, Shanghai, China
- 2Key Laboratory of Hearing Medicine, Ministry of Health, Eye Ear Nose and Throat Hospital, Fudan University, Shanghai, China
- 3Department of Pharmacology, School of Basic Medical Sciences, State Key Laboratory of Medical Neurobiology and MOE Frontiers Center for Brain Science, and Institutes of Brain Science, Fudan University, Shanghai, China
Objective: MVN GABAergic neurons is involved in the rebalance of commissural system contributing to alleviating acute peripheral vestibular dysfunction syndrome. This study aims to depict monosynaptic inputs to MVN GABAergic neurons.
Methods: The modified rabies virus-based retrogradation method combined with the VGAT-IRES-Cre mice was used in this study. Moreover, the commissural connections with MVN GABAergic neurons were analyzed.
Results: We identified 60 nuclei projecting to MVN GABAergic neurons primarily distributed in the cerebellum and the medulla. The uvula-nodulus, gigantocellular reticular nucleus, prepositus nucleus, intermediate reticular nucleus, and three other nuclei sent dense inputs to MVN GABAergic neurons. The medial (fastigial) cerebellar nucleus, dorsal paragigantocellular nucleus, lateral paragigantocellular nucleus and 10 other nuclei sent moderate inputs to MVN GABAergic neurons. Sparse inputs to MVN GABAergic neurons originated from the nucleus of the solitary tract, lateral reticular nucleus, pedunculopontine tegmental nucleus and 37 other nuclei. The MVN GABAergic neurons were regulated by the contralateral MVN, lateral vestibular nucleus, superior vestibular nucleus, and inferior vestibular nucleus.
Conclusion: Our study contributes to further understanding of the vestibular dysfunction in terms of neural circuits and search for new strategies to facilitate vestibular compensation.
1 Introduction
The medial vestibular nuclei (MVN), is a crucial processor of vestibular inputs (1). These inputs primarily originate from crista ampullaries of two lateral semicircular canals (2). The MVN integrates information regarding the head movement in space. In addition, visual, and proprioceptive signals also converge in the MVN (3, 4). The MVN sends ascending axonal fibers to the oculomotor nuclei mediating the vestibuloocular reflex (VOR) and bilateral descending projections to the cervical ventral horn to control the vestibular-spinal reflex. Thus, it is essential in maintaining posture, clear vision and static and dynamic balance (5, 6). Furthermore, it is also involved in cognition, such as navigation, spatial memory and learning (7). Normal vestibular function is essential for daily life. When patients suffer from vestibular dysfunction, they complain acute vestibular syndrome (8). It is characterized by vertigo, gaze instability, vegetative disorders, and cognitive alterations which strongly limit daily activities (9, 10). Certain syndrome can alleviate over time is known as vestibular compensation (11). However, the mechanisms underlying vestibular compensation remain unclear.
In the rhombomeric perspective, the MVN in mouse extends at least from rhombomere r5 to r6. The MVN is comprised of two heterogeneous divisions: small dorsal neurons and larger ventral neurons. Cells in both divisions of the MVN express GAD67 mRNAs which labels cell bodies of GABAergic neurons (12, 13). Previous immunohistochemical studies demonstrated that dorsal neurons in the MVN synthesize gamma-aminobutyric acid (GABA) and are intensely stained by GABA-antibody, supporting a functional GABAergic system exists within the MVN (14–16). GABA is considered as a common inhibitory neurotransmitter within brain (17). Further studies had revealed that GABAergic neurons produced regular firing in electrophysiological recording technology (18–20).
GABAergic neurons within the MVN send axons to the cervical ventral horn and the oculomotor nuclei to mediate the inhibitory influence (21, 22). MVN GABAergic neurons project primarily to the caudal ventrolateral medulla (CVLM) to mediate vestibulosympathetic reflex, showing a target preference (23). Moreover, MVN GABAergic neurons are essential for vestibular compensation by involving in the commissural system between the bilateral MVN (17, 24–26).
Taken together, GABAergic neurons within the MVN are involved in controlling posture, balance, and gaze stabilization, particularly in vestibular compensation. Thus, investigating the afferent inputs to MVN GABAergic neurons will facilitate the search for optional circuits that manipulate GABAergic neurons. The connectivity of MVN neurons have been previously investigated using classic retrograde and anterograde tracers. These studies showed that projections to MVN originated from the dorsal raphe nucleus, inferior olivary, and parabrachial nucleus (27–31). However, specific inputs to MVN GABAergic neurons remain unelucidated. Unlike traditional tracers that cannot distinguish neuron types, the current modified rabies virus (RV) method and the Cre/LoxP system enable to identify specific neurons without affecting passing neural tracts. Accordingly, it allows us to explore neural connectivity of a well-defined neuron type rather than a specific brain region (32–35). In this study, we used modified RV and VGAT-IRES-Cre mice to map out monosynaptic inputs targeting MVN GABAergic neurons.
2 Materials and methods
2.1 Animals
Adult VGAT-IRES-Cre mice and their wild-type littermates were used in this study. All mice were housed under suitable environment (constant temperature: 22 ± 0.5°C and relative humidity: 60% ± 2%) and ensured an adequate supply of food and water. All animal experiments were approved by the Animal Experiments Ethics Committee at Shanghai Public Health Clinical Center, Fudan University.
2.2 Virus
All viruses used in the retrograde tracing study were acquired from BrainVTA (Wuhan, China). rAAV2/9-Ef1α-DIO-EGFP-TVA-WPRE (5 × 1012 genomic copies/mL) and rAAV2/9-Ef1α-DIO-RVG-WPRE (5 × 1012 genomic copies/mL) were combined in equal proportions as the helper virus. And the titer of the RV-ENVA-ΔRG-DsRed (RV) was 2 × 108 genomic copies/mL.
2.3 Virus injection and histological preparation
Virus injection and histological preparation were performed as previously described (32, 33). All mice undergone twice injections of virus injections, respectively. Brief description as following, anesthetized VGAT-IRES-Cre and wild-type mice (pentobarbital sodium, 50 mg/kg, intraperitoneal) were securely positioned on a stereotaxic instrument (RWD Life Science, China). And its skull was aligned to make it parallel to the reference plane. Firstly, 100 nL of the AAV-helper virus mixture were injected into the unilateral MVN (−6.0 mm AP, +0.8 mm ML, −3.2 mm DV). Three weeks afterward, double volume of RV was injected into the same position as before. An additional 10 min of holding the pipette was required to ensure full diffusion of virus particles into the target nuclei.
One week later, the anesthetized mice were perfused with 0.1 M phosphate-buffered saline, then with 4% paraformaldehyde. The brain samples were post-fixed in 4% paraformaldehyde overnight. Subsequently, they were dehydrated in various gradients (10, 20, 30%) of sucrose. Brain samples were coronally sectioned (30-μm thick). All samples were divided into three series.
2.4 Imaging and data analysis
All sections were imaged by virtual-slide microscope (Olympus, Tokyo, Japan). The Olympus analysis software (OlyVIA v.2.9, Tokyo, Japan) and ImageJ software (v.2.1.0, Bethesda, MD, United States) were utilized for detailed analyses. Starter cells were identified by co-expressing DsRed and GFP, whereas afferent neurons only expressed DsRed. Brain structures were recognized based on the standard atlas of mouse brain (36). The neurons labeled with DsRed were counted. To quantify ipsilateral afferent inputs, the input from each nucleus was quantified relative to the total number of input neurons. All data are presented in the form of mean ± standard error of the mean (SEM).
3 Results
3.1 Approaches for identifying monosynaptic inputs to MVN GABAergic neurons
The modified RV-based tracing system was utilized with VGAT-IRES-Cre mice in this study. The helper viruses were Cre-dependent, they can only infect the GABAergic neurons with Cre recombinase. Thus, the enhanced green fluorescent protein (EGFP), avian-specific retroviral receptor (TVA), and the rabies glycoprotein G (RG) were specifically expressed on GABAergic neurons. The modified RV with an avian virus envelope protein (EnvA) only infects neurons with TVA and spread retrogradely with the help of RG. Accordingly, the genetically modified RV retrograde tracing system combined with VGAT-IRES-Cre mice were used to map the afferent inputs to MVN GABAergic neurons (32–35).
On the first day, the helper virus (100 nL) was administered into the unilateral MVN of the wide-type and VGAT-IRES-Cre mice. These Cre-dependent viruses can exclusively infect GABA neurons where the Cre recombinase was present. Then GABA neurons infected with helper virus express TVA-GFP and RG proteins. After 3 weeks, double volume of RV was administered into the previous location. One week later, all mice were sacrificed and perfused (Figure 1).
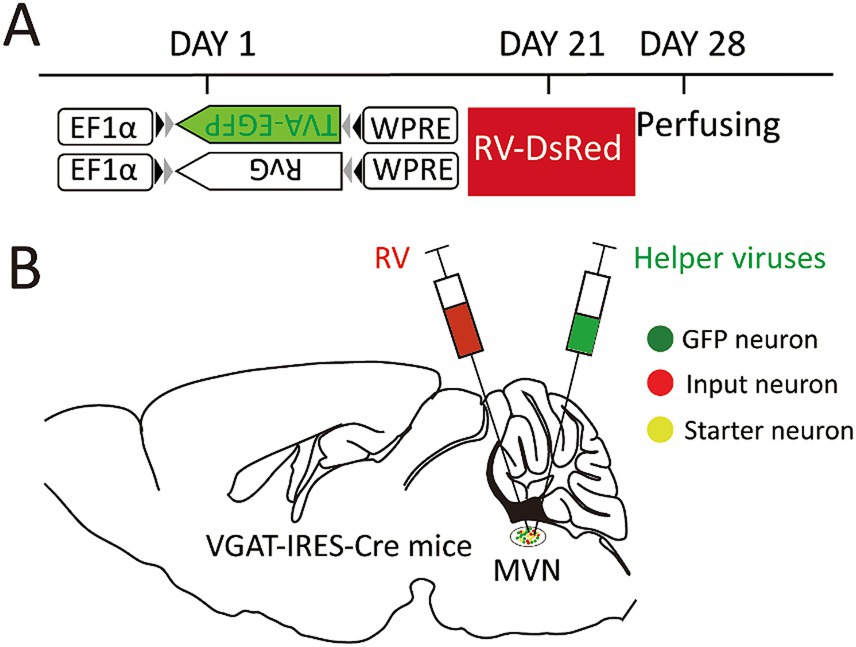
Figure 1. Experimental strategy for RV-based retrograde tracing in MVN GABAergic neurons. (A) A schematic diagram illustrating the viral vectors and injection steps for virus; (B) A schematic diagram showing the injection site into the MVN of VGAT-IRES-Cre mice.
The starter neurons were described as expressing both GFP and DsRed. Three types of neurons (GFP-labeled neurons, DsRed-labeled neurons, and start neurons labeled by both GAP and DsRed) were observed in the MVN of VGAT-Cre mice. Wild-type littermates without the Cre recombinase were used to verify virus specificity. In the MVN of wild-type mouse, neither GFP nor DsRed-positive cells were found (Figure 2).
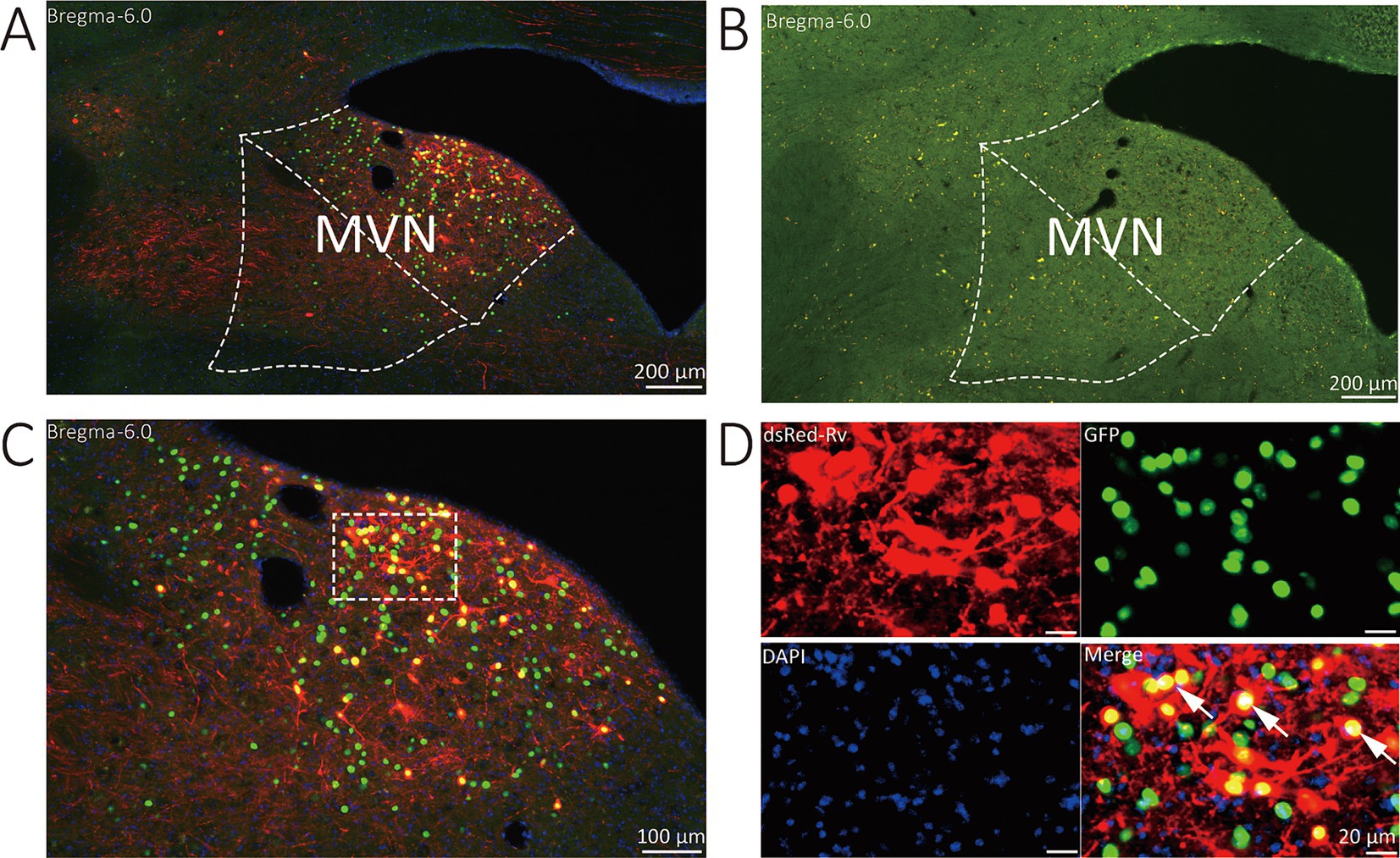
Figure 2. Representative images of MVN GABAergic neurons injected with tracing virus. (A) Injection site of the unilateral MVN of VGAT-IRES-Cre mice. (B) Injection site of the unilateral MVN of wide-type mice. (C,D) Representative images displaying starter neurons (yellow), helper viruses-labeled neurons (green) and input neurons (red). The white arrows show the starter neurons.
3.2 Overview of monosynaptic inputs to MVN GABAergic neurons
Serial coronal brain sections were imaged and brain structures were manually recognized by the atlas of mouse brain (36). We discovered that DsRed-labeled neurons were primarily located in the cerebellum and medulla. Only a few DsRed-labeled neurons were observed in the pons, midbrain, hypothalamus, thalamus, and cerebral cortex. Notably, DsRed-labeled neurons were primarily observed in the ipsilateral brain regions (Figure 3). To provide a detailed review of the presynaptic inputs, representative images were selected and enlarged, such as deep mesencephalic nucleus (DpMe), ventrolateral periaqueductal gray (VLPAG), parvicellular reticular nucleus (PCRt), dorsal raphe nucleus (DR), intermediate reticular nucleus (IRt), gigantocellular reticular nucleus (Gi), prepositus nucleus (Pr), locus coeruleus (LC), and dorsal paragigantocellular nucleus (DPGi) (Figure 4).
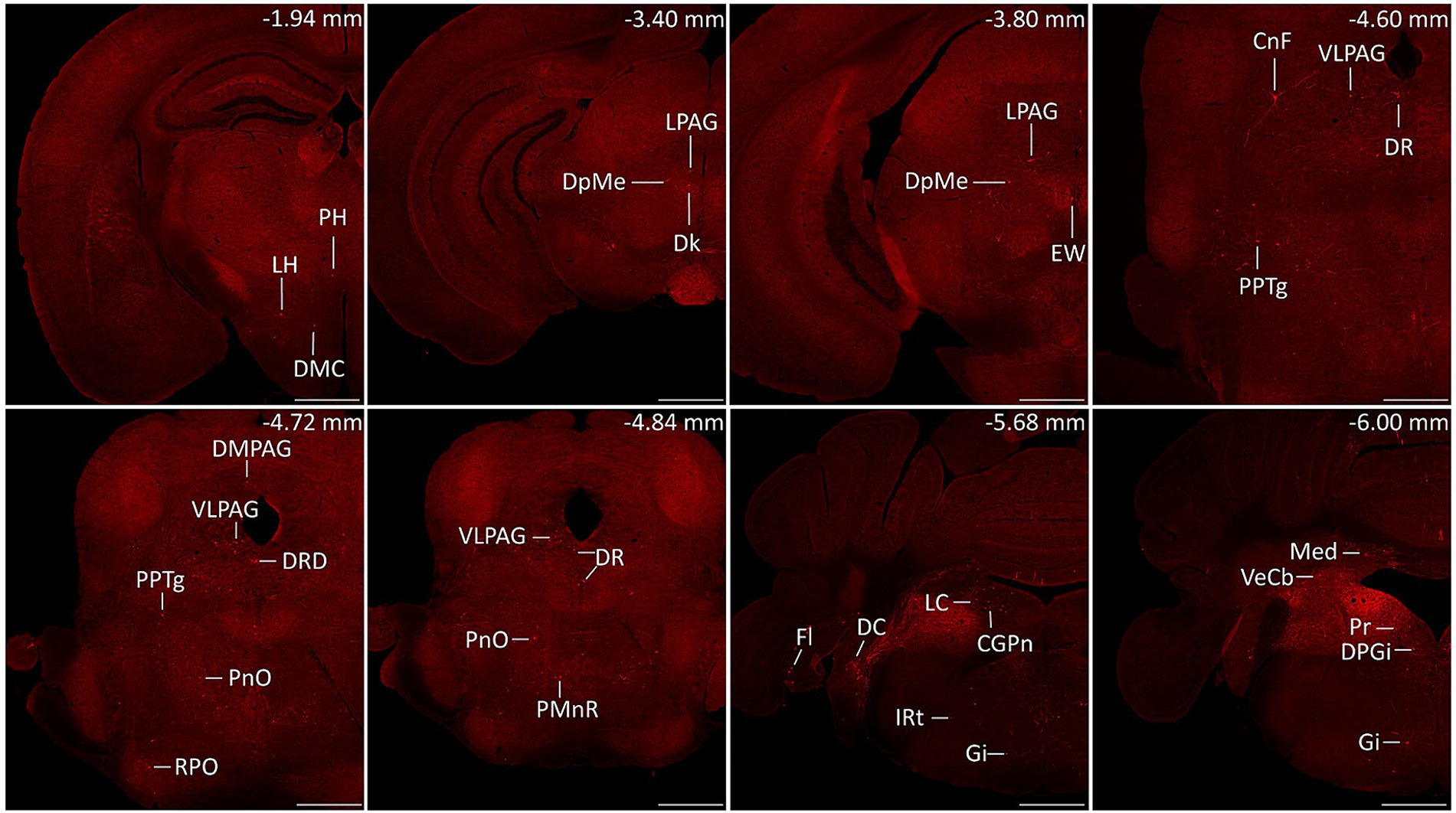
Figure 3. Representative images of monosynaptic inputs to MVN GABAergic neurons. Brain structures were determined according to the standard mouse atlas. Only the ipsilateral hemisphere was shown. Scale bar: 500 μm. CGPn, central gray of the pons; CnF, cuneiform nucleus; DC, dorsal cochlear nucleus; Dk, nucleus of Darkschewitsch; DMC dorsomedial hypothalamic nucleus, compact part; DMPAG, dorsomedial periaqueductal gray; DPGi, dorsal paragigantocellular nucleus; DpMe, deep mesencephalic nucleus; DR, dorsal raphe nucleus; DRD dorsal raphe nucleus, dorsal part; EW, Edinger-Westphal nucleus; Fl, flocculus; Gi, gigantocellular reticular nucleus; IRt, intermediate reticular nucleus; LC, locus coeruleus; LH, lateral hypothalamic area; LPAG, lateral periaqueductal gray; Med, medial (fastigial) cerebellar nucleus; PH, posterior hypothalamic area; PMnR, paramedian raphe nucleus; PnO, pontine reticular nucleus, oral part; PPTg, pedunculopontine tegmental nucleus; Pr, prepositus nucleus; RPO, rostral periolivary region; VeCb, vestibulocerebellar nucleus; VLPAG, ventrolateral periaqueductal gray.
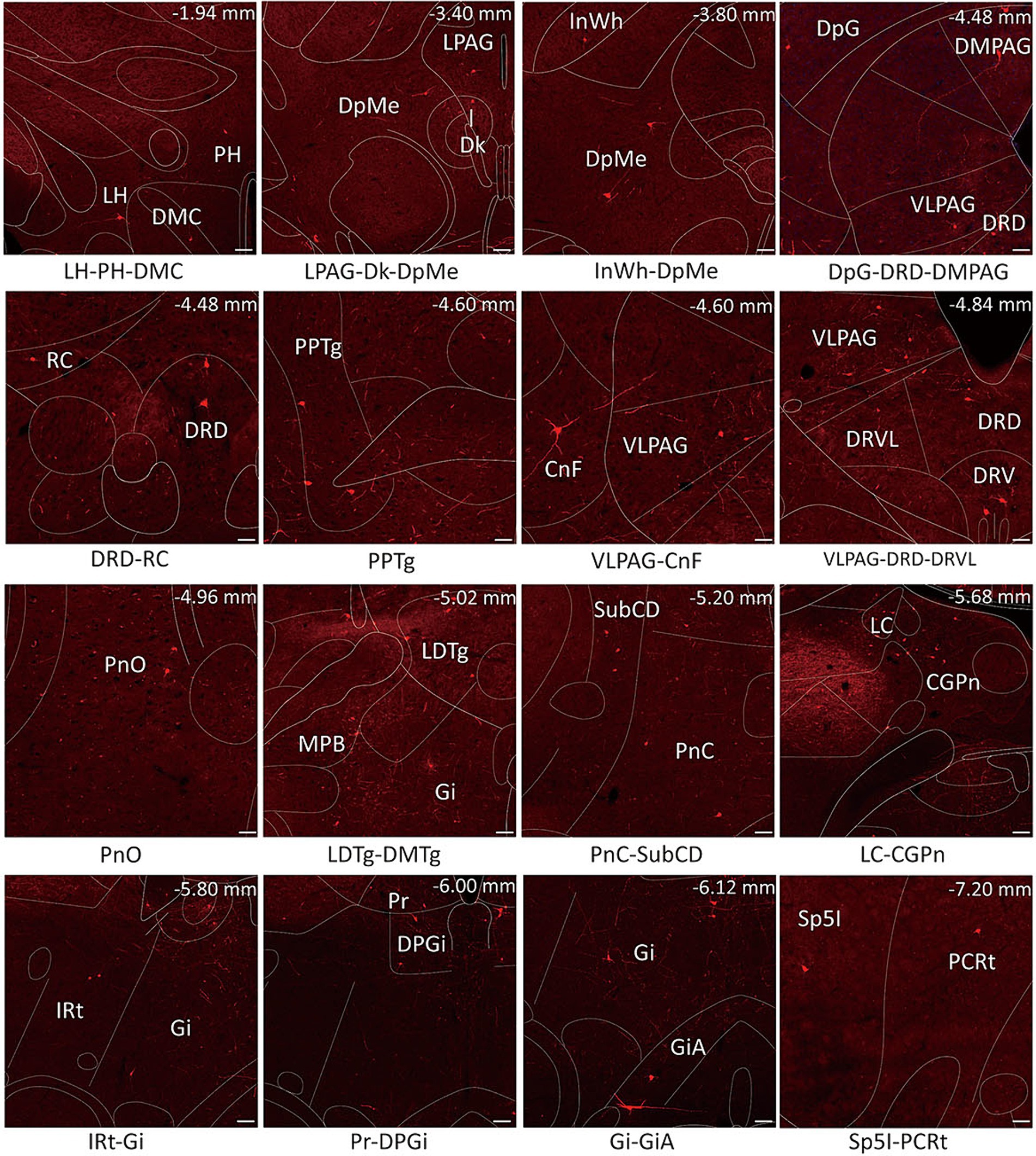
Figure 4. Schematic images of the functional regions with monosynaptic inputs to MVN GABAergic neurons. Primary inputs to MVN GABAergic neurons originated from brain regions associated with oculomotor controlling [e.g., flocculus, medial (fastigial) cerebellar nucleus and prepositus nucleus], sleep–wake regulation (e.g., dorsal paragigantocellular nucleus, lateral paragigantocellular nucleus and ventrolateral periaqueductal gray) and sympathetic response (e.g., gigantocellular reticular nucleus and intermediate reticular nucleus). Scale bar: 100 μm. CGPn, central gray of the pons; CnF, cuneiform nucleus; Dk, nucleus of Darkschewitsch; DMC,dorsomedial hypothalamic nucleus, compact part; DpG, deep gray layer of the superior colliculus; DPGi, dorsal paragigantocellular nucleus; DpMe, deep mesencephalic nucleus; DRD, dorsal raphe nucleus, dorsal part; DRV, dorsal raphe nucleus, ventral part; DRVL, dorsal raphe nucleus, ventrolateral part; Gi, gigantocellular reticular nucleus; GiA, gigantocellular reticular nucleus, alpha part; InWh, intermediate white layer of the superior colliculus; IRt, intermediate reticular nucleus; LC, locus coeruleus; LDTg, laterodorsal tegmental nucleus; LH, lateral hypothalamic area; LPAG, lateral periaqueductal gray; MPB, medial parabrachial nucleus; PCRt, parvicellular reticular nucleus; PH, posterior hypothalamic area; PnC, pontine reticular nucleus, caudal part; PnO, pontine reticular nucleus, oral part; PPTg, pedunculopontine tegmental nucleus; Pr, prepositus nucleus; RC, raphe cap; Sp5I spinal trigeminal nucleus, interpolar part; VLPAG, ventrolateral periaqueductal gray.
3.3 Commissural connections of GABAergic neurons in the MVN
The contralateral vestibular nuclei complex (VNC), which includes the MVN, superior vestibular nucleus (SVN), lateral vestibular nuclei (LVN) and descending vestibular nucleus (DVN) was observed to reveal the commissural connection (37). The proportion of inputs from subnucleus was calculated as the count of DsRed-labeled cells in each subnucleus divided by the total count of DsRed-labeled cells in VNC. The MVN GABAergic neurons received most inputs from the contralateral MVN (68.54% ± 3.58%), as well as the contralateral DVN (13.68% ± 4.23%), SVN (10.87% ± 0.28%) and LVN (6.90% ± 1.79%) (Figure 5).
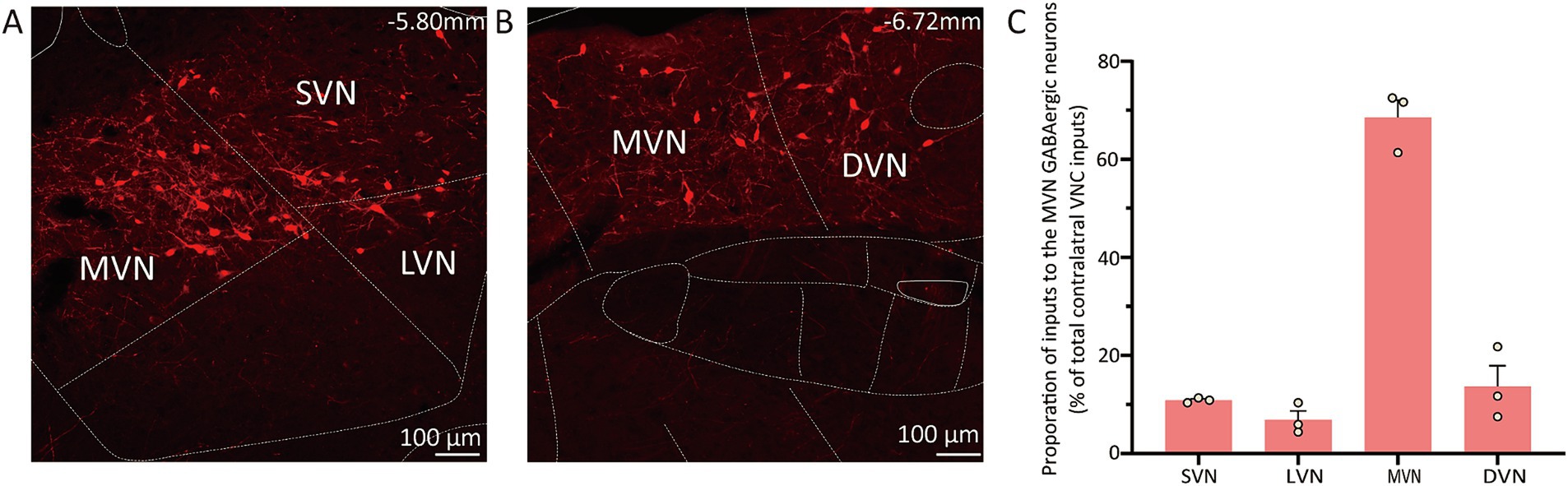
Figure 5. Connectivity between MVN GABAergic neurons and the contralateral VNC. (A) (B) Images showing dsRed-labeled neurons in contralateral MVN, LVN, SVN and DVN; (C) Statistical analysis of commissure connection (n = 3). VNC, vestibular nuclei complex; MVN, medial vestibular nucleus; LVN, lateral vestibular nucleus; SVN, superior vestibular nucleus; DVN, descending vestibular nucleus.
3.4 Analysis of afferent neurons providing input to MVN GABAergic neurons
We calculated the radio for each nucleus by dividing the count of DsRed-labeled neurons in a region by the total count of DsRed-labeled neurons ipsilaterally. We identified 60 nuclei projecting to MVN GABAergic neurons, each contributing over 0.1% of the total labeled neurons on the ipsilateral side. And proportions above 3% were defined as dense inputs, between 1 and 3% were defined as moderate inputs, and below 1% were defined as sparse inputs (35).
Dense inputs (>3% of total DsRed-labeled neurons) to MVN GABAergic neurons originated from following nucleus: uvula-nodulus (40.675 ± 6.76%), Gi (6.48% ± 1.31%), Pr, (4.39 ± 1.27%), IRt (3.28% ± 0.93%), pontine reticular nucleus, caudal part (4.10% ± 0.73%), pontine reticular nucleus, oral part (3.28 ± 0.66%), central gray of the pons (3.25% ± 1.49%). Besides, MVN GABAergic neurons also received moderate monosynaptic inputs (more than 1% of total DsRed-labeled neurons) from several nuclei, such as: vestibulocerebellar nucleus, medial (fastigial) cerebellar nucleus (Med), dorsal cochlear nucleus, DPGi, raphe magnus nucleus, spinal trigeminal nucleus, lateral paragigantocellular nucleus (LPGi), PCRt, laterodorsal tegmental nucleus (LDTg), LC, DpMe, VLPAG, DR, lateral periaqueductal gray (LPAG) (Figure 6). A schematic diagram displaying the monosynaptic inputs to the MVN GABAergic neurons is shown in Figure 7.
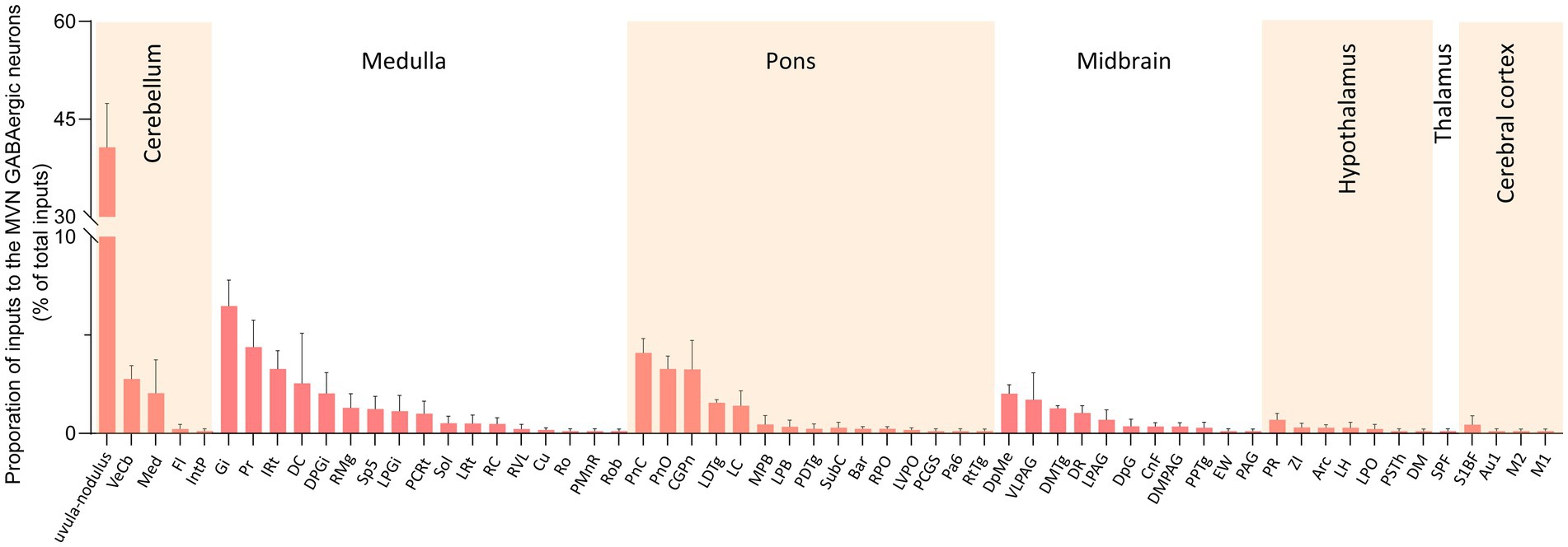
Figure 6. Statistical analysis of ipsilateral monosynaptic inputs to MVN GABAergic neurons. The average proportion of monosynaptic inputs from brain regions contributing more than 0.1% of the total inputs to MVN GABAergic neurons was analyzed and listed. Brain regions are categorized into seven general structures and presented at the top, Sample size: n = 3. Arc, arcuate hypothalamic nucleus; Bar, Barrington’s nucleus; Au1, primary auditory cortex; CGPn, central gray of the pons; CnF, cuneiform nucleus; Cu, cuneate nucleus; DC, dorsal cochlear nucleus; DM, dorsomedial hypothalamic nucleus; DMPAG, dorsomedial periaqueductal gray; DMTg, dorsomedial tegmental area; DpG, deep gray layer of the superior colliculus; DPGi, dorsal paragigantocellular nucleus; DpMe, deep mesencephalic nucleus; DR, dorsal raphe nucleus; EW, Edinger-Westphal nucleus; Fl, flocculus; Gi, gigantocellular reticular nucleus; IntP, interposed cerebellar nucleus, posterior part; IRt, intermediate reticular nucleus; LC, locus coeruleus; LDTg, laterodorsal tegmental nucleus; LH, lateral hypothalamic area; LPAG, lateral periaqueductal gray; MPB, medial parabrachial nucleus; LPGi, lateral paragigantocellular nucleus; LPO, lateral preoptic area; LRt, lateral reticular nucleus; LVPO, lateroventral periolivary nucleus; M1, primary motor cortex; M2, secondary motor cortex; Med, medial (fastigial) cerebellar nucleus; MPB, medial parabrachial nucleus; Pa6, paraabducens nucleus; PAG, periaqueductal gray; PCGS, paracochlear glial substance; PCRt, parvicellular reticular nucleus; PDTg posterodorsal tegmental nucleus; PMnR, paramedian raphe nucleus; PnC, pontine reticular nucleus, caudal part; PnO, pontine reticular nucleus, oral part; PPTg, pedunculopontine tegmental nucleus; Pr, prepositus nucleus; PR, prerubral field; PSTh, parasubthalamic nucleus; RC, raphe cap; RMg, raphe magnus nucleus; Ro, nucleus of Roller; Rob, raphe obscurus nucleus; RPO, rostral periolivary region; RtTg, reticulotegmental nucleus of the pons; RVL, rostroventrolateral reticular nucleus; S1BF, primary somatosensory cortex, barrel field; Sol, nucleus of the solitary tract; Sp5, spinal trigeminal tract; SPF, subparafascicular thalamic nucleus; SubC, subcoeruleus nucleus; VeCb, vestibulocerebellar nucleus; VLPAG, ventrolateral periaqueductal gray; ZI, zona incerta.
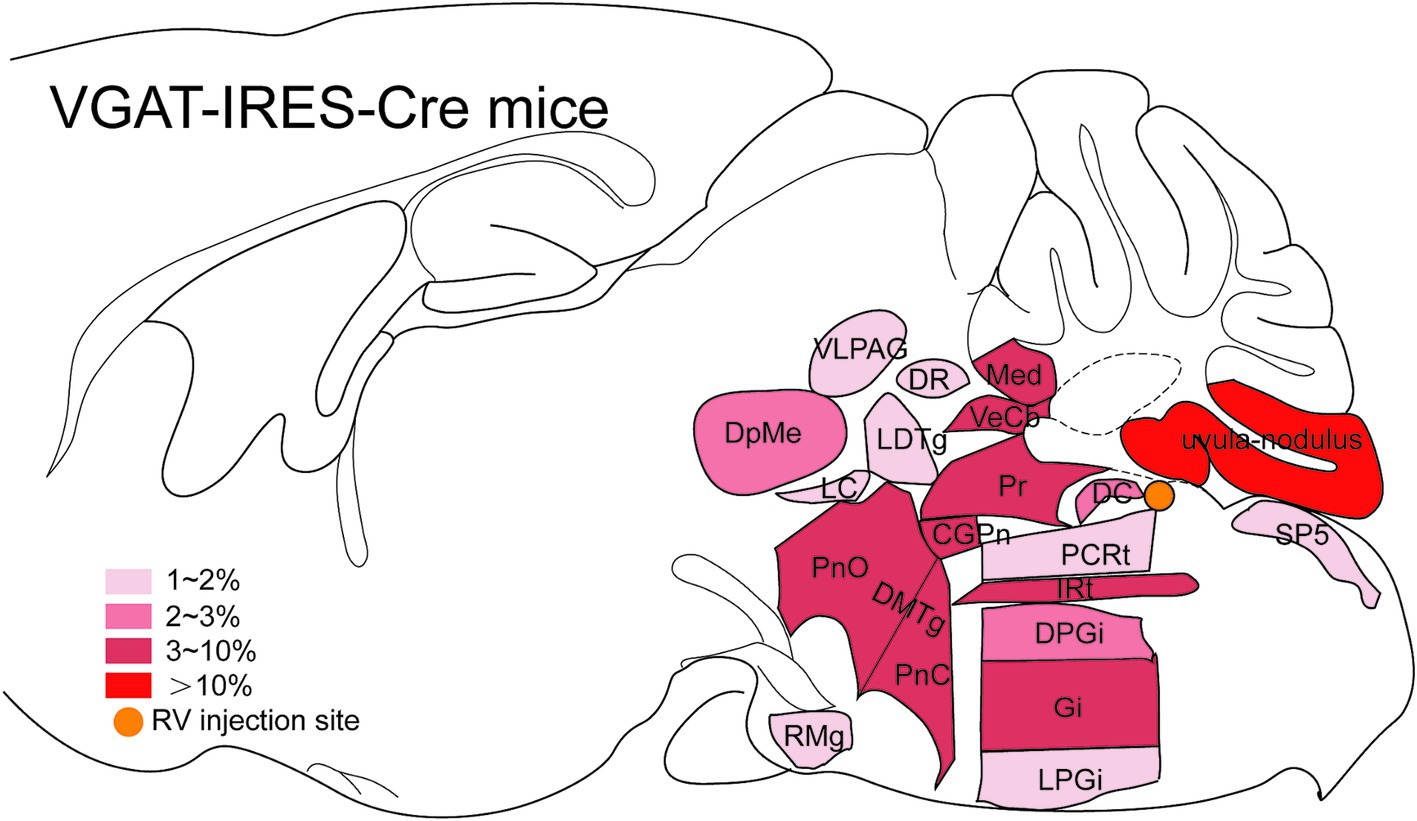
Figure 7. Schematic illustration showing the distribution of monosynaptic inputs to MVN GABAergic neurons. This figure provides a schematic illustration of the distribution patterns of monosynaptic inputs to MVN GABAergic neurons. The color density represents the amount of input neurons. CGPn, central gray of the pons; DC, dorsal cochlear nucleus; DMTg, dorsomedial tegmental area; DPGi, dorsal paragigantocellular nucleus; DpMe, deep mesencephalic nucleus; DR, dorsal raphe nucleus; Gi, gigantocellular reticular nucleus; IRt, intermediate reticular nucleus; LC, locus coeruleus; LDTg, laterodorsal tegmental nucleus; LPGi, lateral paragigantocellular nucleus; Med, medial (fastigial) cerebellar nucleus; PCRt, parvicellular reticular nucleus; PnC, pontine reticular nucleus, caudal part; PnO, pontine reticular nucleus, oral part; Pr, prepositus nucleus; RMg, raphe magnus nucleus; Sp5, spinal trigeminal tract; VeCb, vestibulocerebellar nucleus; VLPAG, ventrolateral periaqueductal gray.
4 Discussion
To gain a deeper insight of how MVN GABAergic neurons mediate physiological behaviors, it is necessary to explore the monosynaptic inputs to them which modulate their activity. In this study, a modified RV-based tracing system and VGAT-Cre mice were utilized. Our results revealed the presynaptic inputs to MVN GABAergic neurons, providing insight into the mechanisms mediating their activity. Additionally, we explored the commissural system and found that MVN GABAergic neurons are influenced by inputs from the contralateral MVN, LVN, SVN and DVN. These findings contribute to understanding commissure system and providing strategies to facilitate vestibular compensation.
4.1 Comparison with earlier tracing studies
Previous research in rats has revealed connectivity between the MVN and DR by using both the anterograde transport of biotinylated dextran amine and retrograde transport of Fluoro-Gold (27). This pathway was also confirmed on mice in our study. The traditional retrograde method using horseradish peroxidase showed the inferior olive (IO) projects to the MVN in rabbits (28, 30). However, we did not find specific inputs from the IO to the MVN GABAergic neurons, suggesting the IO may project to other neuron types of the MVN. This highlighted a limitation of traditional tracer methods which cannot identify cell type-specific neurons in the target nucleus. Genetically modified RV has been extensively used in anatomical studies, particularly in neurosciences, due to its effectiveness in labeling presynaptic inputs of defined neuronal cell-types in transgenic mice (38, 39).
To address this limitation, our group previously used the RV retrograde tracing system to investigate the monosynaptic inputs to GABAergic neurons in the VNC (32). However, heterogeneous subnuclei which performed distinct functions and commissure connections which attributed to vestibular compensation were not considered. In this study, we focused on the MVN, the largest subnucleus of the VNC. The RV-based retrograde system combined with VGAT-IRES-Cre mice was utilized to investigate the presynaptic inputs to GABAergic neurons of the MVN in this study. We discovered 60 upstream nuclei that innervated MVN GABAergic neurons, as well as inputs from the contralateral VNC that formed the commissural system. In conclusion, our study offered a more detailed and systematic mapping of inputs to MVN GABAergic neurons.
4.2 Implications for MVN GABAergic neurons in physiological behavior
The MVN neurons bilaterally travel through the medial longitudinal fasciculus to the medial ventral horn of the cervical cord. These neurons control the contraction of neck muscles to adjust the head and neck movements to maintain balance forming the vestibulospinal reflex. The MVN send ascending fibers to the ipsilateral oculomotor nucleus (CN 3) and contralateral abducens nucleus (CN 6) along with the SVN mediating the vestibuloocular reflex. This coordinate horizontal eye movements (40, 41).
Increased evidences have shown that the neurons connecting the MVN and the oculomotor nucleus was GABAergic, and these GABAergic neurons were also regulated by brain regions associated with oculomotor control (42, 43).
Results of this study confirmed this finding. The cerebellum gains direct projections from the vestibular end-organs and project to the MVN, acting as an adaptive processor (44–46). Direct inputs from the flocculus (Fl) and uvula-nodulus to the MVN have been revealed in cats and rabbits (1, 47–50). The cerebellum regulated the MVN through inhibitory inputs. Different regions projecting to MVN GABAergic neurons played distinct roles in regulating VOR. The unipolar brush cells within the uvula-nodulus receive vestibular inputs via mossy fibers from the vestibular end-organs and the vestibular nuclei. As feedback, these cells mediate the activity of the mossy fibers to control the vestibular inputs (51–55). Previous studies have shown damage of the uvula-nodulus affected the speed of the slow phase of eye movements relative to the head position, rather than the spatial orientation of the nystagmus (56, 57). Unlike the uvula-nodulus, the flocculus participated in the gain of the VOR (58). The Pr integrated the velocity and position signals of horizontal eye movements to maintain stable gaze (59). Researches in monkeys and humans have revealed the lesions of Pr results in defects in maintaining stable gaze (60–62). These indicated the uvula-nodulus, flocculus, and Pr are crucial components of the VOR circuits.
Additionally, sleep–wake system and vestibular system also interact. Clinically, patients with vestibular dysfunction often exhibit sleep disturbances, however, activation by electricity or rocking movements of the vestibular system can facilitate non-rapid eye movement (NREM) sleep (63–66). Franken and his colleagues found NREM sleep was increased and wakefulness episodes were shortened through stimulating the vestibular system by rocking movements at 1.0 Hz (67). Further studies revealed that neurotensinergic neurons in the MVN promoted NREM sleep, and these neurons were primarily GABAergic (68). By contrast, Yanagisawa et al. found that GABAergic neurons in the lateral MVN contributed to stabilizing wakefulness and regulating the transition into rapid eye movement (REM) sleep based on vestibular information (42). This may be reasonable because MVN GABAergic neurons were linked to various brain regions involved in not only improving sleep but also developing wakefulness. Likewise, MVN GABAergic neurons received direct projections from brain area related to sleep/wake cycle control. The LC and DR have been demonstrated to facilitate arousal (69, 70). Previous experiments showed there are projections from LC and DR to the vestibular nuclei (71, 72). In the present study, we further revealed the LC and DR send moderate projections to MVN GABAergic neurons. Inputs from the LC and DR can influence the gain of the vestibular reflexes and cerebellar-vestibular pathway, respectively (27, 73–75). In addition, afferent inputs to MVN GABAergic neurons also arose from NREM sleep-developing brain structures, such as the VLPAG and DpMe. The excitation of VLPAG GABAergic neurons increased NREM sleep and decreased REM sleep (76, 77). Chen et al. revealed that exciting GABAergic neurons in the dorsal part of DpMe promoted NREM sleep via the sublaterodorsal nucleus pathway (78). Brain nuclei that enhance REM sleep, such as the DPGi, LPGi, and LDTG, were found to send moderate inputs to the MVN GABAergic neurons in this study. DPGi GABAergic neurons enhanced REM sleep through the suppression of the LC and DR (79–81). Similarly, LPGi may hyperpolarize REM-off neurons in the LC to generate REM sleep (82). Electrical stimulation of LDTG also increased REM sleep (83).
GABAergic neurons in the MVN also participate in the vestibulosympathetic reflex to moderate blood distribution during postural change and movement. The MVN GABAergic neurons projected primarily to the caudal ventrolateral medulla (CVLM) which influenced sympathetic nerve activity by influencing the rostral ventrolateral medulla (23, 84). MVN GABAergic neurons receive feedback signals from sympathetic-related brain structures, such as the Gi and IRt. Kuo et al. found that activation of certain regions of the Gi induced a decrease in heart rate and caused hypotension in cats (85). The IRt served as a hub transmitting post-inspiratory activity to sympathetic and motor outputs (86, 87).
Our results revealed that MVN GABAergic neurons integrated multisensory signals related to oculomotor control, sleep/wakefulness regulation, and sympathetic responses. These findings established a basis for deeper investigation into the neural pathways mediating the physiological functions of MVN GABA neurons.
4.3 Implications for MVN GABAergic neurons in vestibular compensation
Normal vestibular system is essential for visual stabilization, postural maintenance, and equilibrium control, by relying on symmetrical afferent inputs to the vestibular nuclei (88). Several researches have shown that there are inter-nuclear connections between the bilateral vestibular nuclei (20, 89, 90). The inhibitory commissural system linking the MVN and its contralateral counterpart is fundamental to complete vestibular reflexes (91). Partial or total interruption of unilateral inputs, such as unilateral vestibular deafferentation (UVD) and unilateral labyrinthectomy led to postural and oculomotor deficits (92–94). These deficits were induced by the imbalance in activity between bilateral MVNs (95). The resting discharges of neurons in the ipsilesional MVN were almost silenced, whereas the contralesional MVN neurons became hyperactive (92, 96, 97). Another study demonstrated the resting potential of MVN neurons only decreased by 50% compared to normal situation after bilateral labyrinthectomy (98). These findings indicated that the silence of ipsilesional MVN neurons was primarily caused by enhanced suppression from contralesional MVN neurons (92). The vestibular dysfunction was characterized by static (without movement) and dynamic symptoms (with movement) (96). Static symptoms gradually disappeared within days known as vestibular compensation (91, 96, 99). Inhibitory commissural connections were crucial for the recovery of spontaneous resting potential of the lesioned side and rebalancing neural discharge between the bilateral MVN during vestibular compensation (91, 96).
Our findings showed that GABAergic neurons in the MVN were heavily innervated by projections from the contralateral MVN as well as the contralateral LVN, SVN, and DVN. These patterns were similar to the connections observed in hamsters, in contrast to the commissural connections in cats and monkeys showing afferents to the MVN arising from all parts of contralateral MVN, parts of contralateral SVN and DVN (71, 100). These discrepancies may be due to the differences between species. The commissural system to MVN GABAergic neurons revealed in the present study suggests that these neurons may be regulated by contralateral VNC to achieve bilateral balance, which was crucial for normal vestibular function.
In conclusion, we illustrated monosynaptic inputs to MVN GABAergic neurons. It suggested that MVN GABAergic neurons received information from various brain regions. This finding underscores the crucial role of MVN GABAergic neurons in integrating multiple signals. In addition, the confirmation of the commissure system provides provided evidences that MVN GABAergic neurons were involved in facilitating vestibular compensation.
Data availability statement
The raw data supporting the conclusions of this article will be provided by corresponding author without reservation.
Ethics statement
The animal study was approved by Animal Experiments Ethics Committee at Shanghai Public Health Clinical Center, Fudan University. The study was conducted in accordance with the local legislation and institutional requirements.
Author contributions
DK: Investigation, Methodology, Software, Writing – original draft. LK: Data curation, Investigation, Writing – original draft. CL: Investigation, Writing – original draft. QW: Methodology, Writing – original draft. JW: Methodology, Supervision, Validation, Writing – original draft. CD: Supervision, Writing – review & editing.
Funding
The author(s) declare that financial support was received for the research, authorship, and/or publication of this article. This work was supported by National Natural Science Foundation of China (Nos. 82371143 and 82171142 CFD) and Youth Program of National Natural Science Foundation of China (82201282).
Acknowledgments
We thank all members of Zhili Huang’s lab at Fudan University, Shanghai.
Conflict of interest
The authors declare that the research was conducted in the absence of any commercial or financial relationships that could be construed as a potential conflict of interest.
Publisher’s note
All claims expressed in this article are solely those of the authors and do not necessarily represent those of their affiliated organizations, or those of the publisher, the editors and the reviewers. Any product that may be evaluated in this article, or claim that may be made by its manufacturer, is not guaranteed or endorsed by the publisher.
References
1. Barmack, NH. Central vestibular system: vestibular nuclei and posterior cerebellum. Brain Res Bull. (2003) 60:511–41. doi: 10.1016/S0361-9230(03)00055-8
2. Maklad, A, and Fritzsch, B. The developmental segregation of posterior crista and saccular vestibular fibers in mice: a carbocyanine tracer study using confocal microscopy. Brain Res Dev Brain Res. (2002) 135:1–17. doi: 10.1016/S0165-3806(01)00327-3
3. Cullen, KE, and Zobeiri, OA. Proprioception and the predictive sensing of active self-motion. Curr Opin Physio. (2021) 20:29–38. doi: 10.1016/j.cophys.2020.12.001
4. Mildren, RL, and Cullen, KE. Vestibular contributions to primate neck postural muscle activity during natural motion. J Neurosci. (2023) 43:2326–37. doi: 10.1523/JNEUROSCI.1831-22.2023
5. Cullen, KE, and Wang, L. Predictive coding in early vestibular pathways: implications for vestibular cognition. Cogn Neuropsychol. (2020) 37:423–6. doi: 10.1080/02643294.2020.1783222
6. Horn, AKE, and Straka, H. Functional Organization of Extraocular Motoneurons and eye Muscles. Annu Rev Vis Sci. (2021) 7:793–825. doi: 10.1146/annurev-vision-100119-125043
7. Hitier, M, Besnard, S, and Smith, PF. Vestibular pathways involved in cognition. Front Integr Neurosci. (2014) 8:59. doi: 10.3389/fnint.2014.00059
8. Casani, AP, Gufoni, M, and Capobianco, S. Current insights into treating Vertigo in older adults. Drugs Aging. (2021) 38:655–70. doi: 10.1007/s40266-021-00877-z
9. Tighilet, B, and Chabbert, C. Adult neurogenesis promotes balance recovery after vestibular loss. Prog Neurobiol. (2019) 174:28–35. doi: 10.1016/j.pneurobio.2019.01.001
10. Lacour, M, and Tighilet, B. Plastic events in the vestibular nuclei during vestibular compensation: the brain orchestration of a "deafferentation" code. Restor Neurol Neurosci. (2010) 28:19–35. doi: 10.3233/RNN-2010-0509
11. Lacour, M, Helmchen, C, and Vidal, PP. Vestibular compensation: the neuro-otologist's best friend. J Neurol. (2016) 263:54–S64. doi: 10.1007/s00415-015-7903-4
12. Diaz, C, and Glover, JC. The vestibular column in the mouse: a Rhombomeric perspective. Front Neuroanat. (2022) 15:806815. doi: 10.3389/fnana.2021.806815
13. Tanaka, I, and Ezure, K. Overall distribution of GLYT2 mRNA-containing versus GAD67 mRNA-containing neurons and colocalization of both mRNAs in midbrain, pons, and cerebellum in rats. Neurosci Res. (2004) 49:165–78. doi: 10.1016/j.neures.2004.02.007
14. Walberg, F, Ottersen, OP, and Rinvik, E. GABA, glycine, aspartate, glutamate and taurine in the vestibular nuclei: an immunocytochemical investigation in the cat. Exp Brain Res. (1990) 79:547–63. doi: 10.1007/BF00229324
15. Kumoi, K, Saito, N, and Tanaka, C. Immunohistochemical localization of gamma-aminobutyric acid-and aspartate-containing neurons in the guinea pig vestibular nuclei. Brain Res. (1987) 416:22–33. doi: 10.1016/0006-8993(87)91492-2
16. Rácz, E, Gaál, B, Kecskes, S, and Matesz, C. Molecular composition of extracellular matrix in the vestibular nuclei of the rat. Brain Struct Funct. (2014) 219:1385–403. doi: 10.1007/s00429-013-0575-x
17. Gliddon, CM, Darlington, CL, and Smith, PF. GABAergic systems in the vestibular nucleus and their contribution to vestibular compensation. Prog Neurobiol. (2005) 75:53–81. doi: 10.1016/j.pneurobio.2004.11.001
18. Takazawa, T, Saito, Y, Tsuzuki, K, and Ozawa, S. Membrane and firing properties of glutamatergic and GABAergic neurons in the rat medial vestibular nucleus. J Neurophysiol. (2004) 92:3106–20. doi: 10.1152/jn.00494.2004
19. Saito, Y, Takazawa, T, and Ozawa, S. Relationship between afterhyperpolarization profiles and the regularity of spontaneous firings in rat medial vestibular nucleus neurons. Eur J Neurosci. (2008) 28:288–98. doi: 10.1111/j.1460-9568.2008.06338.x
20. Babalian, A, Vibert, N, Assie, G, Serafin, M, Mühlethaler, M, and Vidal, PP. Central vestibular networks in the guinea-pig: functional characterization in the isolated whole brain in vitro. Neuroscience. (1997) 81:405–26. doi: 10.1016/S0306-4522(97)00069-9
21. Blessing, WW, Hedger, SC, and Oertel, WH. Vestibulospinal pathway in rabbit includes GABA-synthesizing neurons. Neurosci Lett. (1987) 80:158–62. doi: 10.1016/0304-3940(87)90646-X
22. Precht, W, Baker, R, and Okada, Y. Evidence for GABA as the synaptic transmitter of the inhibitory vestibulo-ocular pathway. Exp Brain Res. (1973) 18:415–28. doi: 10.1007/BF00239109
23. Holstein, GR, Friedrich, VL Jr, and Martinelli, GP. Glutamate and GABA in Vestibulo-sympathetic pathway neurons. Front Neuroanat. (2016) 10:7. doi: 10.3389/fnana.2016.00007
24. Malinvaud, D, Vassias, I, Reichenberger, I, Rössert, C, and Straka, H. Functional organization of vestibular commissural connections in frog. J Neurosci. (2010) 30:3310–25. doi: 10.1523/JNEUROSCI.5318-09.2010
25. Precht, W, Schwindt, PC, and Baker, R. Removal of vestibular commissural inhibition by antagonists of GABA and glycine. Brain Res. (1973) 62:222–6. doi: 10.1016/0006-8993(73)90631-8
26. Furuya, N, Yabe, T, and Koizumi, T. Neurotransmitters regulating vestibular commissural inhibition in the cat. Acta Otolaryngol Suppl. (1991) 481:205–8. doi: 10.3109/00016489109131381
27. Halberstadt, AL, and Balaban, CD. Organization of projections from the raphe nuclei to the vestibular nuclei in rats. Neuroscience. (2003) 120:573–94. doi: 10.1016/S0306-4522(02)00952-1
28. Balaban, CD. Distribution of inferior olivary projections to the vestibular nuclei of albino rabbits. Neuroscience. (1988) 24:119–34. doi: 10.1016/0306-4522(88)90317-X
29. Balaban, CD. Olivo-vestibular and cerebello-vestibular connections in albino rabbits. Neuroscience. (1984) 12:129–49. doi: 10.1016/0306-4522(84)90143-X
30. Balaban, CD, Kawaguchi, Y, and Watanabe, E. Evidence of a collateralized climbing fiber projection from the inferior olive to the flocculus and vestibular nuclei in rabbits. Neurosci Lett. (1981) 22:23–9. doi: 10.1016/0304-3940(81)90279-2
31. Balaban, CD. Projections from the parabrachial nucleus to the vestibular nuclei: potential substrates for autonomic and limbic influences on vestibular responses. Brain Res. (2004) 996:126–37. doi: 10.1016/j.brainres.2003.10.026
32. Shi, XB, Wang, J, Li, FT, Zhang, YB, Qu, WM, Dai, CF, et al. Whole-brain monosynaptic outputs and presynaptic inputs of GABAergic neurons in the vestibular nuclei complex of mice. Front Neurosci. (2022) 16:982596. doi: 10.3389/fnins.2022.982596
33. Ma, WX, Li, L, Kong, LX, Zhang, H, Yuan, PC, Huang, ZL, et al. Whole-brain monosynaptic inputs to lateral periaqueductal gray glutamatergic neurons in mice. CNS Neurosci Ther. (2023) 29:4147–59. doi: 10.1111/cns.14338
34. Wickersham, IR, Lyon, DC, Barnard, RJ, Mori, T, Finke, S, Conzelmann, KK, et al. Monosynaptic restriction of transsynaptic tracing from single, genetically targeted neurons. Neuron. (2007) 53:639–47. doi: 10.1016/j.neuron.2007.01.033
35. Shi, X, Wei, H, Chen, Z, Wang, J, Qu, W, Huang, Z, et al. Whole-brain monosynaptic inputs and outputs of glutamatergic neurons of the vestibular nuclei complex in mice. Hear Res. (2021) 401:108159. doi: 10.1016/j.heares.2020.108159
36. Paxinos, G, and Franklin, KBJ. The mouse brain in stereotaxic coordinates. 2nd ed. San Diego: Academic Press (2001).
37. Straka, H, and Dieringer, N. Basic organization principles of the VOR: lessons from frogs. Prog Neurobiol. (2004) 73:259–309. doi: 10.1016/j.pneurobio.2004.05.003
38. Watabe-Uchida, M, Zhu, L, Ogawa, SK, Vamanrao, A, and Uchida, N. Whole-brain mapping of direct inputs to midbrain dopamine neurons. Neuron. (2012) 74:858–73. doi: 10.1016/j.neuron.2012.03.017
39. Pollak Dorocic, I, Fürth, D, Xuan, Y, Johansson, Y, Pozzi, L, Silberberg, G, et al. A whole-brain atlas of inputs to serotonergic neurons of the dorsal and median raphe nuclei. Neuron. (2014) 83:663–78. doi: 10.1016/j.neuron.2014.07.002
40. Khan, S, and Chang, R. Anatomy of the vestibular system: a review. Neuro Rehabil. (2013) 32:437–43. doi: 10.3233/NRE-130866
41. McCall, AA, Miller, DM, and Yates, BJ. Descending influences on Vestibulospinal and Vestibulosympathetic reflexes. Front Neurol. (2017) 8:112. doi: 10.3389/fneur.2017.00112
42. Nakatsuka, D, Kanda, T, Sato, M, Ishikawa, Y, Cherasse, Y, and Yanagisawa, M. A novel GABAergic population in the medial vestibular nucleus maintains wakefulness and gates rapid eye movement sleep. iScience. (2024) 27:109289. doi: 10.1016/j.isci.2024.109289
43. Wentzel, PR, De Zeeuw, CI, Holstege, JC, and Gerrits, NM. Inhibitory synaptic inputs to the oculomotor nucleus from vestibulo-ocular-reflex-related nuclei in the rabbit. Neuroscience. (1995) 65:161–74. doi: 10.1016/0306-4522(94)00471-G
44. Cullen, KE. Internal models of self-motion: neural computations by the vestibular cerebellum. Trends Neurosci. (2023) 46:986–1002. doi: 10.1016/j.tins.2023.08.009
45. Zobeiri, OA, and Cullen, KE. Distinct representations of body and head motion are dynamically encoded by Purkinje cell populations in the macaque cerebellum. eLife. (2022) 11:e75018. doi: 10.7554/eLife.75018
46. Zobeiri, OA, and Cullen, KE. Cerebellar Purkinje cells in male macaques combine sensory and motor information to predict the sensory consequences of active self-motion. Nat Commun. (2024) 15:4003. doi: 10.1038/s41467-024-48376-0
47. Barmack, NH, Qian, Z, and Yoshimura, J. Regional and cellular distribution of protein kinase C in rat cerebellar Purkinje cells. J Comp Neurol. (2000) 427:235–54. doi: 10.1002/1096-9861(20001113)427:2<235::AID-CNE6>3.0.CO;2-6
48. Shojaku, H, Sato, Y, Ikarashi, K, and Kawasaki, T. Topographical distribution of Purkinje cells in the uvula and the nodulus projecting to the vestibular nuclei in cats. Brain Res. (1987) 416:100–12. doi: 10.1016/0006-8993(87)91501-0
49. Walberg, F, and Dietrichs, E. The interconnection between the vestibular nuclei and the nodulus: a study of reciprocity. Brain Res. (1988) 449:47–53. doi: 10.1016/0006-8993(88)91022-0
50. Fujita, H, Kodama, T, and du Lac, S. Modular output circuits of the fastigial nucleus for diverse motor and nonmotor functions of the cerebellar vermis. eLife. (2020) 9:e58613. doi: 10.7554/eLife.58613
51. Elliott, KL, Kersigo, J, Lee, JH, Yamoah, EN, and Fritzsch, B. Sustained loss of Bdnf affects peripheral but not central vestibular targets. Front Neurol. (2021) 12:768456. doi: 10.3389/fneur.2021.768456
52. Pan, N, Jahan, I, Lee, JE, and Fritzsch, B. Defects in the cerebella of conditional Neurod 1 null mice correlate with effective Tg (Atoh 1-cre) recombination and granule cell requirements for Neurod 1 for differentiation. Cell Tissue Res. (2009) 337:407–28. doi: 10.1007/s00441-009-0826-6
53. Maklad, A, and Fritzsch, B. Partial segregation of posterior crista and saccular fibers to the nodulus and uvula of the cerebellum in mice, and its development. Brain Res Dev Brain Res. (2003) 140:223–36. doi: 10.1016/S0165-3806(02)00609-0
54. Balmer, TS, and Trussell, LO. Selective targeting of unipolar brush cell subtypes by cerebellar mossy fibers. eLife. (2019) 8:e44964. doi: 10.7554/eLife.44964
55. Diño, MR, Perachio, AA, and Mugnaini, E. Cerebellar unipolar brush cells are targets of primary vestibular afferents: an experimental study in the gerbil. Exp Brain Res. (2001) 140:162–70. doi: 10.1007/s002210100790
56. Errico, P, Ferraresi, AA, Barmack, NH, and Pettorossi, VE. Role of cerebellar uvula-nodulus in the control of head orientation-specific eye velocity in the rabbit. Ann N Y Acad Sci. (1996) 781:614–8. doi: 10.1111/j.1749-6632.1996.tb15738.x
57. Voogd, J, and Barmack, NH. Oculomotor cerebellum. Prog Brain Res. (2006) 151:231–68. doi: 10.1016/S0079-6123(05)51008-2
58. Chang, HHV, Cook, AA, Watt, AJ, and Cullen, KE. Loss of Flocculus Purkinje cell firing precision leads to impaired gaze stabilization in a mouse model of spinocerebellar Ataxia type 6 (SCA6). Cells. (2022) 11:2739. doi: 10.3390/cells11172739
59. Kim, SH, Zee, DS, du Lac, S, Kim, HJ, and Kim, JS. Nucleus prepositus hypoglossi lesions produce a unique ocular motor syndrome. Neurology. (2016) 87:2026–33. doi: 10.1212/WNL.0000000000003316
60. Kaneko, CR. Eye movement deficits after ibotenic acid lesions of the nucleus prepositus hypoglossi in monkeys. I Saccades and fixation. J Neurophysiol. (1997) 78:1753–68. doi: 10.1152/jn.1997.78.4.1753
61. Kaneko, CR. Eye movement deficits following ibotenic acid lesions of the nucleus prepositus hypoglossi in monkeys II. Pursuit, vestibular, and optokinetic responses. J Neurophysiol. (1999) 81:668–81. doi: 10.1152/jn.1999.81.2.668
62. Cho, HJ, Choi, HY, Kim, YD, Seo, SW, and Heo, JH. The clinical syndrome and etiological mechanism of infarction involving the nucleus prepositus hypoglossi. Cerebrovasc Dis. (2008) 26:178–83. doi: 10.1159/000145325
63. Kim, SK, Kim, JH, Jeon, SS, and Hong, SM. Relationship between sleep quality and dizziness. PLoS One. (2018) 13:e0192705. doi: 10.1371/journal.pone.0192705
64. Nakayama, M, Suzuki, M, Inagaki, A, Takemura, K, Watanabe, N, Tanigawa, T, et al. Impaired quality of sleep in Ménière's disease patients. J Clin Sleep Med. (2010) 6:445–9. doi: 10.5664/jcsm.27933
65. van Sluijs, RM, Rondei, QJ, Schluep, D, Jäger, L, Riener, R, Achermann, P, et al. Effect of rocking movements on afternoon sleep. Front Neurosci. (2020) 13:1446. doi: 10.3389/fnins.2019.01446
66. Goothy, SSK, and McKeown, J. Modulation of sleep using electrical vestibular nerve stimulation prior to sleep onset: a pilot study. J Basic Clin Physiol Pharmacol. (2020) 32:19–23. doi: 10.1515/jbcpp-2020-0019
67. Kompotis, K, Hubbard, J, Emmenegger, Y, Perrault, A, Mühlethaler, M, Schwartz, S, et al. Rocking promotes sleep in mice through rhythmic stimulation of the vestibular system. Curr Biol. (2019) 29:392–401.e4. doi: 10.1016/j.cub.2018.12.007
68. Kashiwagi, M, Kanuka, M, Tatsuzawa, C, Suzuki, H, Morita, M, Tanaka, K, et al. Widely distributed Neurotensinergic neurons in the brainstem regulate NREM sleep in mice. Curr Biol. (2020) 30:1002–1010.e4. doi: 10.1016/j.cub.2020.01.047
69. Carter, ME, Yizhar, O, Chikahisa, S, Nguyen, H, Adamantidis, A, Nishino, S, et al. Tuning arousal with optogenetic modulation of locus coeruleus neurons. Nat Neurosci. (2010) 13:1526–33. doi: 10.1038/nn.2682
70. Ito, H, Yanase, M, Yamashita, A, Kitabatake, C, Hamada, A, Suhara, Y, et al. Analysis of sleep disorders under pain using an optogenetic tool: possible involvement of the activation of dorsal raphe nucleus-serotonergic neurons. Mol Brain. (2013) 6:59. doi: 10.1186/1756-6606-6-59
71. Horowitz, SS, Blanchard, J, and Morin, LP. Medial vestibular connections with the hypocretin (orexin) system. J Comp Neurol. (2005) 487:127–46. doi: 10.1002/cne.20521
72. Schuerger, RJ, and Balaban, CD. Immunohistochemical demonstration of regionally selective projections from locus coeruleus to the vestibular nuclei in rats. Exp Brain Res. (1993) 92:351–9.
73. Schuerger, RJ, and Balaban, CD. Organization of the coeruleo-vestibular pathway in rats, rabbits, and monkeys. Brain Res Brain Res Rev. (1999) 30:189–217. doi: 10.1016/S0165-0173(99)00015-6
74. Foote, SL, Bloom, FE, and Aston-Jones, G. Nucleus locus ceruleus: new evidence of anatomical and physiological specificity. Physiol Rev. (1983) 63:844–914. doi: 10.1152/physrev.1983.63.3.844
75. Kishimoto, T, Sasa, M, and Takaori, S. Inhibition of lateral vestibular nucleus neurons by 5-hydroxytryptamine derived from the dorsal raphe nucleus. Brain Res. (1991) 553:229–37. doi: 10.1016/0006-8993(91)90830-O
76. Weber, F, Hoang Do, JP, Chung, S, Beier, KT, Bikov, M, Doost, MS, et al. Regulation of REM and non-REM sleep by periaqueductal GABAergic neurons. Nat Commun. (2018) 9:354. doi: 10.1038/s41467-017-02765-w
77. Weber, F, Chung, S, Beier, KT, Xu, M, Luo, L, and Dan, Y. Control of REM sleep by ventral medulla GABAergic neurons. Nature. (2015) 526:435–8. doi: 10.1038/nature14979
78. Chen, ZK, Dong, H, Liu, CW, Liu, WY, Zhao, YN, Xu, W, et al. A cluster of mesopontine GABAergic neurons suppresses REM sleep and curbs cataplexy. Cell Discov. (2022) 8:115. doi: 10.1038/s41421-022-00456-5
79. Fraigne, JJ, Torontali, ZA, Snow, MB, and Peever, JH. REM sleep at its Core-circuits, neurotransmitters, and pathophysiology. Front Neurol. (2015) 6:123. doi: 10.3389/fneur.2015.00123
80. Gervasoni, D, Peyron, C, Rampon, C, Barbagli, B, Chouvet, G, Urbain, N, et al. Role and origin of the GABAergic innervation of dorsal raphe serotonergic neurons. J Neurosci. (2000) 20:4217–25. doi: 10.1523/JNEUROSCI.20-11-04217.2000
81. Ennis, M, and Aston-Jones, G. GABA-mediated inhibition of locus coeruleus from the dorsomedial rostral medulla. J Neurosci. (1989) 9:2973–81. doi: 10.1523/JNEUROSCI.09-08-02973.1989
82. Sirieix, C, Gervasoni, D, Luppi, PH, and Léger, L. Role of the lateral paragigantocellular nucleus in the network of paradoxical (REM) sleep: an electrophysiological and anatomical study in the rat. PLoS One. (2012) 7:e28724. doi: 10.1371/journal.pone.0028724
83. Thakkar, M, Portas, C, and McCarley, RW. Chronic low-amplitude electrical stimulation of the laterodorsal tegmental nucleus of freely moving cats increases REM sleep. Brain Res. (1996) 723:223–7. doi: 10.1016/0006-8993(96)00256-9
84. Holstein, GR, Friedrich, VL Jr, and Martinelli, GP. Projection neurons of the vestibulo-sympathetic reflex pathway. J Comp Neurol. (2014) 522:2053–74. doi: 10.1002/cne.23517
85. Kuo, JS, Hwa, Y, and Chai, CY. Cardio-inhibitory mechanism in the gigantocellular reticular nucleus of the medulla oblongata. Brain Res. (1979) 178:221–32. doi: 10.1016/0006-8993(79)90691-7
86. Toor, RUA, Sun, QJ, Kumar, NN, Le, S, Hildreth, CM, Phillips, JK, et al. Neurons in the intermediate reticular nucleus coordinate Postinspiratory activity, swallowing, and respiratory-sympathetic coupling in the rat. J Neurosci. (2021) 41:1617. doi: 10.1523/JNEUROSCI.0502-19.2019
87. Huff, A, Karlen-Amarante, M, Oliveira, LM, and Ramirez, JM. Role of the postinspiratory complex in regulating swallow-breathing coordination and other laryngeal behaviors. eLife. (2023) 12:e86103. doi: 10.7554/eLife.86103
88. Swamy Suman, N, Kumar Rajasekaran, A, Yuvaraj, P, Pruthi, N, and Thennarasu, K. Measure of central vestibular compensation: a review. J Int Adv Otol. (2022) 18:441–6. doi: 10.5152/iao.2022.21207
89. Newlands, SD, Kevetter, GA, and Perachio, AA. A quantitative study of the vestibular commissures in the gerbil. Brain Res. (1989) 487:152–7. doi: 10.1016/0006-8993(89)90951-7
90. Epema, AH, Gerrits, NM, and Voogd, J. Commissural and intrinsic connections of the vestibular nuclei in the rabbit: a retrograde labeling study. Exp Brain Res. (1988) 71:129–46. doi: 10.1007/BF00247528
91. Graham, BP, and Dutia, MB. Cellular basis of vestibular compensation: analysis and modelling of the role of the commissural inhibitory system. Exp Brain Res. (2001) 137:387–96. doi: 10.1007/s002210100677
92. Paterson, JM, Menzies, JRW, Bergquist, F, and Dutia, MB. Cellular mechanisms of vestibular compensation. Neuroembryol Aging. (2006) 3:183–93. doi: 10.1159/000096796
93. Curthoys, IS. Vestibular compensation and substitution. Curr Opin Neurol. (2000) 13:27–30. doi: 10.1097/00019052-200002000-00006
94. Dieringer, N. Activity-related postlesional vestibular reorganization. Ann N Y Acad Sci. (2003) 1004:50–60. doi: 10.1196/annals.1303.006
95. Fisch, U. The vestibular response following unilateral vestibular neurectomy. Acta Otolaryngol. (1973) 76:229–38. doi: 10.3109/00016487309121503
96. Jones, SM, Jones, TA, Mills, KN, and Gaines, GC. Anatomical and physiological considerations in vestibular dysfunction and compensation. Semin Hear. (2009) 30:231–41. doi: 10.1055/s-0029-1241124
97. Smith, PF, and Curthoys, IS. Mechanisms of recovery following unilateral labyrinthectomy: a review. Brain Res Brain Res Rev. (1989) 14:155–80. doi: 10.1016/0165-0173(89)90013-1
98. Ris, L, and Godaux, E. Neuronal activity in the vestibular nuclei after contralateral or bilateral labyrinthectomy in the alert guinea pig. J Neurophysiol. (1998) 80:2352–67. doi: 10.1152/jn.1998.80.5.2352
99. Tighilet, B, and Lacour, M. Gamma amino butyric acid (GABA) immunoreactivity in the vestibular nuclei of normal and unilateral vestibular neurectomized cats. Eur J Neurosci. (2001) 13:2255–67. doi: 10.1046/j.0953-816x.2001.01622.x
Keywords: vestibular function, vestibular disorders, vestibular compensation, medial vestibular nucleus, GABAergic neurons
Citation: Kong D, Kong L, Liu C, Wu Q, Wang J and Dai C (2024) Commissural and monosynaptic inputs to medial vestibular nucleus GABAergic neurons in mice. Front. Neurol. 15:1484488. doi: 10.3389/fneur.2024.1484488
Edited by:
Sulin Zhang, Huazhong University of Science and Technology, ChinaReviewed by:
Viviana Mucci, Western Sydney University, AustraliaBernd Fritzsch, University of Nebraska Medical Center, United States
Qing Zhang, Shanghai Jiaotong University School of Medicine, China
Jun Wang, The First Affiliated Hospital of Nanchang University, China
Copyright © 2024 Kong, Kong, Liu, Wu, Wang and Dai. This is an open-access article distributed under the terms of the Creative Commons Attribution License (CC BY). The use, distribution or reproduction in other forums is permitted, provided the original author(s) and the copyright owner(s) are credited and that the original publication in this journal is cited, in accordance with accepted academic practice. No use, distribution or reproduction is permitted which does not comply with these terms.
*Correspondence: Jing Wang, d2FuZ2ppbmc4NTk3QDEyNi5jb20=; Chunfu Dai, Y2ZkYWk2NkAxMjYuY29t
†These authors have contributed equally to this work