- 1Department of Health Management, The Third Affiliated Hospital of Guangzhou Medical University, Guangzhou, China
- 2Department of Neurosurgery, Tianjin Medical University General Hospital, Tianjin, China
- 3Department of Obstetrics and Gynecology, Center for Reproductive Medicine, Guangdong Provincial Key Laboratory of Major Obstetric Diseases, The Third Affiliated Hospital of Guangzhou Medical University, Guangzhou, China
- 4Key Laboratory for Reproductive Medicine of Guangdong Province, The Third Affiliated Hospital of Guangzhou Medical University, Guangzhou, China
Stroke, a cerebrovascular accident, is prevalent and the second highest cause of death globally across patient populations; it is as a significant cause of morbidity and mortality. Mesenchymal stem cell (MSC) transplantation is emerging as a promising treatment for alleviating neurological deficits, as indicated by a great number of animal and clinical studies. The potential of regulating the immune system is currently being explored as a therapeutic target after ischemic stroke. This study will discuss recent evidence that MSCs can harness the immune system by interacting with immune cells to boost neurologic recovery effectively. Moreover, a notion will be given to MSCs participating in multiple pathological processes, such as increasing cell survival angiogenesis and suppressing cell apoptosis and autophagy in several phases of ischemic stroke, consequently promoting neurological function recovery. We will conclude the review by highlighting the clinical opportunities for MSCs by reviewing the safety, feasibility, and efficacy of MSCs therapy.
Introduction
Stroke is responsible for almost six million deaths, at least 10% of all mortalities yearly, and two-thirds of stroke survivors remain disabled (1). Worldwide, over 80 million people have survived a stroke; 70% of incident strokes are ischemic (1). Although recent evolutions of thrombectomy technology, as well as improvements in imaging devices, have achieved ground-breaking changes in ischemic stroke therapy (2), given its narrow therapeutic time window and the concern of hemorrhagic complications (3), thrombolysis is still not performed routinely (4). In this context, it is urgent to yield neurorestorative treatments for abrogating stroke-induced neurological deficits for both basic scientists and clinical researchers. Cell therapy is emerging as a promising novel modality for facilitating neurologic recovery after a stroke (5). Harnessing the immune system to function and effectively boost neurologic recovery has transitioned from a theoretical possibility to a viable therapeutic option for ischemic stroke. Mesenchymal stem cells (MSCs) transplantation is an attractive therapy method because they have the potential for proliferation, differentiation, and immunomodulatory properties (6, 7). While the MSCs can be derived from any type of tissue beyond the bone marrow, adipose, and placenta, these MSCs share the same core attributes of ability to cell migration patterns and behave as immunomodulatory cells (8–10). In addition to immunomodulation, growing evidence demonstrates that MSCs are involved in multiple pathological processes by targeting series downstream. Such downstream activities include the inhibition of apoptosis and autophagy and the promotion of angiogenesis, neurogenesis, and synaptic remodeling in several phases of ischemic stroke (11, 12). MSCs may also be an ideal candidate for cell transplantation therapy for ischemic stroke. Despite growing evidence indicating that MSCs may improve neurological function under pathological conditions, including stroke (13, 14), data on the interaction between MSCs and immunomodulation is limited. In this review, we summarize the therapeutic effects of MSCs both in preclinical studies and in clinical stroke trials. We also consider the mutual crosstalk between MSCs and immune cells under stroke conditions.
Mesenchymal stem cells
Rodent bone marrow cells were first ectopically transplanted into the kidney capsule by Friedenstein et al. in the 1960s and 1970s, showing an osteogenic effect (15). Given the potential to differentiate into various cell lineages, Caplan et al. suggested the “mesenchymal stem cells” term in 1991 (16, 17). MSCs are multipotent fibroblast-like cells that, interestingly, exist in various adult tissues, including adipose tissue, periosteum, liver, spleen, muscle connective tissue, placenta, umbilical cord blood, dental pulp, and aborted fetal tissues (18–20). Further, The Mesenchymal and Tissue Stem Cell Committee of the International Society for Cellular Therapy (ISCT) recommended specific minimum MSC criteria to distinguish them from other cell types by expression of many cell surface markers, including CD73, CD90, and CD105, and the absence of expression of CD45, CD34, CD14, CD19, CD11b, or Human Leukocyte Antigen–DR isotype (21–23). Recently, a significant number of novel cell surface markers associated with the stemness within MSCs, namely SSEA1/4, CD44, CD146, and CD271, have been revealed as well (23–26). A further two criteria are that isolated cells show adherence to plastic in culture and the capacity to differentiate into adipocytes, osteoblasts, and chondroblasts in vitro (21–23). To date, MSCs have become the most widely studied stem cell population and are studied in various preclinical models and clinical settings alike. And these studies have focused on the vital roles in coordinating tissue responses to ischemic stroke in acute and post-acute stroke settings, in which MSCs modulate cell survival, cell apoptosis, autophagy angiogenesis, and immunosuppression (23), consequently supporting neurological recovery.
Therapeutic application of MSCs in preclinical ischemic stroke study
MSCs promote post-stroke cell survival
Upon an ischemic stroke, the cerebral artery occlusion influences the survival of various brain cells, such as brain neurons, glial cells, and vascular cells. Among these cells, the neurons are the most vulnerable, and neuronal viability plays a crucial role in neurological recovery after ischemic stroke (27, 28). Studies in experimental models mimicking ischemic stroke imply that MSCs can abrogate ischemia-induced neuronal survival and neurological function recovery. As such, under such conditions, MSCs derived from bone marrow, adipose tissue, and umbilical cord can significantly reduce neuronal death (29–31). In addition, neurological recovery is also associated with the successful restitution of vascular and glial functions. During the ischemic lesion remodeling, neurons, glial cells, and vascular cells can strongly interact with each other, contributing to neurological recovery (27). Interestingly, it is demonstrated that MSCs are involved in promoting the survival of microglia, astrocyte, and endotheliocyte survival via regulating many pathways (32–35). Notably, white matter demyelination predates axonal injury in the early stage of ischemic stroke, indicating a time window for stroke intervention focusing on preventing or postponing axonal injury through myelin regeneration (36). Meanwhile, Bagdasarian et al. (37) applied therapeutic MSC to a rodent stroke model and demonstrated their efficacy in white matter by comparison of Diffusion tensor imaging and Neurite Orientation Dispersion and Density Imaging metrics. MSCs exert many unique biological effects, including self-recovery via promoting post-stroke cell survival, providing a promising cellular therapeutic approach for treating white matter injury (38).
MSCs suppress post-stroke cell apoptosis
Among the many cell death pathways (39), apoptosis accounts for a large proportion of cell death under such a condition (40), a rational and reactive performance made to sacrifice specific cells for the benefit of the tissue. Researchers have indicated that MSCs have vital roles in regulating cell apoptosis. For example, Kong et al. (41) demonstrated MSCs potentially protect the cortical neurons from OGD injury in vitro by rescuing neurons from apoptosis. Xiao et al. (42) indicated that bone marrow-derived MSC-exosomes repressed oligodendrocyte apoptosis via releasing exosomal miR-134, in turn negatively regulating the caspase-8-dependent apoptosis pathway. In addition to apoptosis, MSCs can promote cell survival by alleviating parthanatos and necroptosis. By co-culturing MSCs with hypoxic neurons, Kong et al. indicated that MSCs prevented neurons from parthanatos by suppressing the expression of nuclear translocation of apoptosis-inducing factors (41). The reduction of neuronal necrosis kinase RIP1 and RIP3 levels caused by MSCs, meanwhile, was tightly related to the suppression of neuronal necroptosis (41).
MSCs suppress post-stroke cell autophagy
Autophagy, another type of cell death, is an evolutionarily conserved cellular mechanism that balances cellular nerve homeostasis. It is a process that results from the injury in cells' internal conditions, including starvation, hypoxia, and infection (43). MSCs can inhibit autophagy and, in turn, promote cell survival. Kuang et al. (31) illustrated that the application of adipose-derived MSC-exosomes suppressed the autophagic response under both in vitro hypoxia and in vivo cerebral ischemia regarding cell survival through transferring of miR-25, as a consequence, supporting post-stroke neurological function recovery. Moreover, the knockdown of SNHG12 in MSCs boosted the effects of MSCs in suppressing hypoxia-induced autophagy in brain microvascular endothelial cells and MCAO rats by interacting with the PI3K/AKT/mTOR signaling pathway (44). By contrast, MSCs can reverse ischemic injury by enhancing autophagy as well (45, 46). Likewise, Zeng et al. indicated that PC12 cells were exposed to oxygen-glucose deprivation (OGD) and cocultured with MSCs secreted extracellular vesicles (EVs). Under such conditions, MSC-secreted EVs significantly attenuated pyroptosis mediated by NLRP3 inflammasome by promoting AMPK-dependent autophagy flux (47).
MSCs promote post-stroke angiogenesis
During post-stroke conditions, capillaries are dysfunctional, and blood-brain barrier permeability is increased, consequently aggravating the inflammatory reaction and neuronal necrosis. In addition to rescuing and restoring neuronal cells, increasing evidence has shown that increasing the survival of endothelial cells, ameliorating brain angiogenesis, and mediating the recanalization of brain collaterals are great therapeutic targets. MSCs transplantation has been revealed to migrate to the peri-infarct region and differentiate into neuronal, glial, and endothelial cells to enhance neuroplasticity (30). Moreover, MSCs act in an indirect paracrine way as well. MSCs have also been shown to induce regenerative processes by increasing the level of insulin-like growth factor 1 (IGF-1) and inducing vascular endothelial growth factor (VEGF), angiopoietin-1 (Ang-1), essential fibroblast growth factor (bFGF), and neurotrophic factors in the host brain (48–51). These bioactive factors of VEGF and Ang-1 are the most essential in promoting neurological recovery by boosting neurogenesis. Besides that, the hypoxia and 0.04 MHz ultrasound-modified MSCs and MSCs-derived exosomes have been illustrated to have the capacity to achieve angiogenic effects (14, 52–54). Significantly, implantation of MSCs promoted angiogenesis and increased neurogenesis by releasing these angiogenic and neurotrophic factors. By conducting a three-dimensional analysis of the neovascularization in the peri-infarct region, Toyama et al. (55) and Chen et al. (56) demonstrated that the capillary-like tube formation was significantly induced in stroke mice treated with MSCs, suggesting a direct effect of MSCs on facilitating angiogenesis.
MSCs support the post-stroke immunomodulatory effects
MSCs-microglia interactions
Microglia, which comprise a significant immune cell population in the central nervous system, appear as a ramified structure with a small soma in the resting form under physiological conditions (57, 58). When activated by ischemic stroke, microglia increase in number and transform to amoeboid forms characterized by the larger microglial cell body and shorter bumps. The activation of microglia activation is the first step in response to inflammation; further, the other immune cells, such as T cells, neutrophils, and natural killer cells, are activated (59, 60). While MSCs in microglial activation have been widely studied, there is not enough research on transplantation in ischemic stroke. Plenty of studies investigating various donor cell-derived MSCs identified a novel insight into crosstalk in ischemic stroke, and the role of MSCs in microglial activation has begun to be recognized (14, 61–63). For example, Yang et al. (64) indicated that bone marrow-MSCs can shift the microglia phenotype from M1 to M2, contributing to MSCs-induced brain repair. As a paracrine interaction between MSCs and microglia, the synergistic effect of MANF and PDGF-AA pathway governed M2 polarization. Furthermore, despite peripheral LPS treatment before the stroke, increased CD16/32-M1 microglia boosted the number of microglia surrounding the peri-infarct region and diminished CD206-M2 microglia on the post-stroke seventh day; they were rectified by the administration of human umbilical cord MSCs (65). Moreover, a series of researchers have accessed the effects of MSCs on microglial activation (14, 61–75); more details are shown in Table 1. To sum up, the application of MSCs appears to inhibit microglial activation and promote M2 polarization.
MSCs-neutrophils interactions
Neutrophils are the essential infiltrating cell type in the ischemic brain the first few days after stroke (76), tightly correlating with ischemic stroke-induced BBB disruption. The preclinical stroke studies have implied that MSCs' administration can reduce neutrophil accumulation in the brain. Vehicle or EVs (the equivalent of 2 × 106 MSCs) were intravenously administered to mice after transient intraluminal middle cerebral artery occlusion (77). MSC-EVs decreased specifically polymorphonuclear neutrophil infiltration in ischemic brains of aged mice. Moreover, MSCs can boost the beneficial effects of neutrophils on the brain. Bone marrow-MSCs can potentially induce interleukin-17 (IL-17) production in memory CD4+ T cells that, in turn, promote the enhanced phagocytic activity of neutrophils (78). Still, bone marrow-MSCs may also protect resting and interleukin-8-activated neutrophils from apoptosis, preserving their effector functions and suppressing the reactive oxygen species production (79).
MSCs-natural killer (NK) cells interactions
NK cells, one type of lymphocyte, belong to a part of the innate immune system that is well-known for the potential to mediate cytotoxicity and produce cytokines (80).
The immunomodulatory effects of MSCs on NK cells have been extensively studied in the peripheral regions. MSCs are involved in inhibiting the differentiation, proliferation, cytotoxicity, and activation of the NK cells through a variety of cytokines (81). These cytokines may include prostaglandin E2 (PGE2), soluble human leukocyte antigen-G5 (sHLA-G5), and transforming growth factor-β (TGF-β), which is partly linked to glycoprotein A repetitions predominant on the surface of MSCs (82). Additionally, hypoxic MSCs can also repress NK cell cytotoxicity and reduce the accumulation of host-derived NK cells when transplanted in vivo, as a result, contributing to ameliorating limb ischemia in allogeneic recipients (83).
MSCs-dendritic cells (DCs) interactions
The immune response to ischemic stroke consists of inflammatory and regulatory processes. DC is one of the cell types involved in innate and adaptive immunity. Upon an ischemic condition, the brain DCs are increased at 24- and 72-h post-stroke and accumulated in the peri-infarct region near invading T cells (84). Peripheral DC appearing in the brain was apparent at 72-h post-stroke and was confined primarily to the lesion core (84). MSCs are revealed to have capacities to suppress DCs differentiation and maturation and even reverse mature DCs to immature states (85–88). Gao et al. (85) indicated that MSCs inhibited the differentiation of human monocyte-derived DCs through both releasing IL-10 and direct cell contact. Likewise, Zhao et al. (87) showed that MSCs can differentiate mature DCs into a distinct regulatory DC population characterized by a lower expression of CD1a, CD80, CD86, and CD40 and a higher expression of CD11b. Importantly, such an effect on inhibiting DCs differentiation and maturity is demonstrated to be linked to both maintaining homeostasis of regulatory T cells and lower levels of proinflammatory cytokines TNF-α and MHC II surface antigens (86, 87).
MSCs-T cells interactions
T cells, which are involved in both innate and adaptive immune responses, can be divided into the αβ subset and the unconventional γδ subset (89). The αβ subset includes CD4+ T helper cells (Th1, Th2, Th17) that mainly modulate the functions of phagocytes and granulocytes, CD8+ T cells that have a cytotoxic role, and regulatory T cells (Treg) that regulate immune responses (89). After the ischemia-onset, T cells are revealed at the border of the infarct, where they appear within days (90, 91). More specifically, CD8+ T cells are recruited as early as 3 h post-ischemia onset, with CD4+ T cells and NK T cells following within 24 h, and accumulation of these T cells peaks 3 to 4 days after ictus (76, 92, 93). There is solid evidence that MSCs are linked to direct immunosuppressive properties via suppressing the activation and proliferation of CD4+ and CD8+ T cells while promoting activation, differentiation, and proliferation of Tregs through direct cell-to-cell communication or releasing of various factors. Upon a hypoxic-ischemic encephalopathy condition, MSCs can induce persistent peripheral T-cell tolerance and inhibit the invasion of T-cells into the preterm brain (94). During a critical limb ischemia condition, MSCs showed effective prevention of Th1 priming, which was strongly related to an altered IL-12/IL-10 production (95). Likewise, in renal ischemia/reperfusion rats, by releasing TGF-β, MSCs can not only suppress CD8+ T cells but boost the development of Tregs, as a result, repressing T cell-related inflammation (96). As such, MSCs might therefore contribute to suppressing the activation and proliferation of CD4+ and CD8+ T cells and promoting the proliferation of Tregs during an ischemic condition. However, information regarding this aspect of the interaction between MSCs and T cells upon an ischemic stroke condition appears to be limited. It is scarce, so additional and reliable data is urgently needed.
MSCs-B cells interactions
B cells, one part of the adaptive immune response, have the capacity to present antigens, produce antibodies, and activate the immune system (97). These cells are detectable in insufficient quantities in the brain under normoxic conditions; however, they are trafficked in larger quantities to the brain tissues in response to injury (98, 99). B cell adoptive transfer to mice does not contribute to acute pathology but can support post-stroke recovery, independent of changing immune populations in recipient mice (100). Completed and ongoing clinical trials and preclinical studies on the therapeutic effects of MSCs transplantation against immune-mediated diseases have demonstrated an increased generation of B cells (101). The effectiveness of related MSCs-B cell interaction-based treatments dramatically depends on the functions of Bregs, as MSCs can increase the secretion of IL-10 by Bregs (101). On the contrary, several studies identified that MSCs are involved in suppressing the activation and proliferation of B cells. For instance, human adipose tissue-derived MSCs can inhibit the proliferation and chemotaxis of B cells by inducing cell cycle arrest in G0/G1 phase and regulating CXCR4 and CXCR5 expression, respectively (102). As such, in vitro, by secreting various factors, MSCs decreased the proliferation of B cells and the production of immunoglobulin (103). Taken together, the combined effects on the proliferation and activation of B cells are found in MSCs. However, the precise effect of inhibition/promotion on B cells modulated under ischemic stroke conditions is not fully clear yet. An overview of how MSCs interact with immune cells is shown in Figure 1.
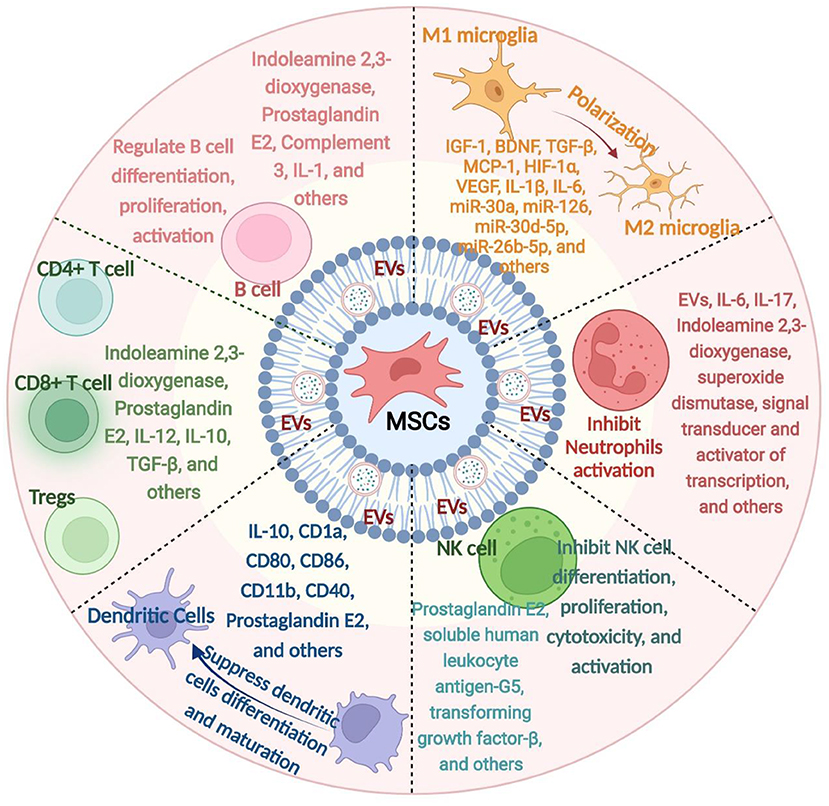
Figure 1. An overview of how mesenchymal stem cells (MSCs) interact with immune cells. MSCs derived from various tissue sources can release a series of mediators, which in turn interact with various immune cells, namely microglia, neutrophils, natural killer cells, dendritic cells, T cells, and B cells. IL, interleukin; EVs, extracellular vesicles; MSCs, mesenchymal stem cells; NK cell, Natural Killer cell; IGF-1, insulin-like growth factor 1; VEGF, vascular endothelial growth factor; bFGF, fibroblast growth factor; MCP-1, Monocyte chemoattractant protein-1; miR, microRNA; TGF-β, transforming growth factor-β.
MSCs improve the post-stroke neurological function recovery
The size of the infarct volume is tightly correlated with ischemic stroke severity. In vivo experiments on MCAO rats demonstrated that MSCs derived from bone marrow, adipose tissue, and the umbilical cord could reduce the post-stroke infarct volume (104). However, many conditions, such as the source of MSCs, species injected, and the timing and dose of MSC injection, can affect specific effects on decreasing infarct volume after stroke. Along with such a reduction of infarct volume, the behavioral test analyses illustrated better test scores of mice/rats transplanted with MSCs at either time. Of note, this better test performance in the corner turn test, the rotarod test, balance beam test, tightrope test, and paw slips recording was long-lasting and stable until the end of the observation period (31, 105–107). It is suggested that MSCs can potentially mitigate postischemic motor coordination impairment in preclinical stroke experiments. Significantly, post-stroke impairment of the blood-brain barrier and perifocal vasogenic edema are also alleviated by endovascular MSCs administration. Post-stroke edema, impairment of the blood-brain barrier, as well as upregulation of aquaporin 4 (AQP4) water transport channels, play an essential role in the progression of ischemia and deteriorating disease recovery. Datta et al. (108) presented preliminary evidence that 1 × 105 endovascular MSCs at 6 h post-stroke down-regulates AQP4 expression and alleviates vasogenic edema toward neuroprotection. Likewise, MSCs protected blood-brain barrier integrity by inhibiting the ischemia-induced astrocyte apoptosis, owing to the downregulation of AQP4 expression via the p38 signaling pathway (109).
The underlying patterns of how MSCs exhibit the therapeutic effects
MSC-EVs are critical players in treating ischemic stroke
EVs, the membrane-enclosed nanoscale particles secreted by all eukaryotes, always serve as a variety of molecular cargoes, such as peptides, lipids, proteins, and noncoding RNAs (43, 110). Based on the size of EVs, they can be divided into three subtypes: exosomes, microvesicles, and apoptotic bodies (111). Exosomes with a diameter of 30–150 nm form via the fusion of multivesicular bodies with membrane and are further released into the extracellular matrix (112, 113). Microvesicles with a diameter of 200–1,000 nm are produced owing to the outward budding of the plasma membrane (112, 113). Conversely, apoptotic bodies with a diameter of 1,000–5,000 nm are produced by dying cells and are even more abundant than the two other particles (111). Only exosomes and microvesicles are relevant to the therapeutic effects imparted by MSC-EVs. When these nanosized vesicles are released from donor cells into the extracellular matrix, they can be internalized by numerous recipient cells. In turn, they transfer the above bioactive cargos into recipient cells, including near and far from the secreting cell, further serving as messengers and performing biological functions. This cargo mix is revealed to mediate the biological properties of EVs and, indirectly, the treatment of MSCs under ischemic stroke conditions. The EVs derived from MSCs are emerging to be an appealing therapeutic tool for ischemic stroke, with the MSC-derived properties and the characteristics of effortless storage, lower immunogenicity, higher safety profile, and nature delivery vehicles. Previous research works indicated that EVs derived from MSCs promoted post-stroke recovery. They have the capacity to regulate the expression of recipient cell genes, alter cell properties involved in ischemic stroke, and mediate restorative effects, including cell survival, cell apoptosis, cell autophagy, angiogenesis, neurological function recovery, and immunomodulation, through a variety of molecular cargoes transfer (13, 31, 33, 34, 42, 53, 114–142). Moreover, by inhibiting the release of EVs, the beneficial effect on these aspects is also suppressed. For example, by establishing a coculture model that MSCs cocultured with hypoxic neurons and brain microvascular endothelial cells, the results showed that the MSCs treatment could inhibit the apoptosis of hypoxic neurons and restore the tube formation of brain microvascular endothelial cells (143). However, an inhibitor, GW4869, of EVs secretion can reverse these beneficial effects, indicating that these EVs are the key players that serve as the central mediator of the neuroprotective and angiogenic effects of MSCs (143). Currently, studies are paying attention to the function of the EVs isolated from bone marrow, adipose tissue, and, sometimes, umbilical cord-MSCs (144).
The critical role of noncoding RNAs (NcRNAs) in treating ischemic stroke
Despite being well-established that most human RNA transcripts cannot encode proteins, the emerging evidence demonstrates that ncRNAs regulate cell physiology and shape cellular functions (145, 146). ncRNAs can be divided into long [namely long noncoding RNA (lncRNA) and circRNA] and small ncRNAs [including microRNAs (miRNAs), tRNAs, and piRNAs] by taking 200 nucleotides as the limit (147). miRNAs, ~18–24 nucleotides in size, are much earlier reported and the most discussed. lncRNAs are a large and heterogeneous kind of ncRNAs with more than 200 nucleotides and are involved in the modulation of transcription, translation, RNA metabolism, as well as homeostasis (148–150). CircRNAs are defined as circular covalently bonded structures associated with a higher tolerance to exonucleases (151), which serve as a scaffold for chromatin-modifying complexes, regulating the expression level of parental genes, modulating mRNA splicing, and acting as miRNA sponges (152, 153). Notably, the aberrant expression of many noncoding RNAs has been associated with aggressive pathologies. A variety of ncRNAs are reduced in the ischemic brain or blood after ischemic stroke, as previously reported for circSCMH1, miR-124-3p, miR-126, miR-221-3p, and miR-132 (114–119, 154), whereas other ncRNAs, namely miR-98 and miR-494, are increased at defined follow-up (155–157). MSC-based therapies offer an attractive approach because they promote cell survival, angiogenesis, and neurological function recovery, suppress cell apoptosis and autophagy, and regulate immunomodulation, where ncRNAs play an essential role. Interestingly, these ncRNAs were mainly derived from EVs, including lncRNA MALAT1, miR-1-3p, miR-17-92, miR-22-3p, miR-25, miR-26a, miR-26b-5p, miR-31, miR-124, miR-126, miR-132, miR-133b, miR-134, miR-138-5p, miR-146a-5p, miR-181b, miR-206, miR-210, miR-221-3p, miR-223-3p, miR-542-3p, and miR-1290 (31, 33, 34, 42, 114, 115, 117, 119, 121–125, 129–133, 135, 136, 138, 158). These EVs are isolated from bone marrow and adipose tissue, as well as umbilical cord-MSCs. Additionally, MSCs can regulate the expression of ncRNA directly, in turn, to support neuroprotection. For instance, Yang et al. indicated that MSCs-mediated mesencephalic astrocyte-derived neurotrophic factor paracrine signaling, the PDGF-AA/miR-30a*/XBP1/MANF pathway, synergistically mediates MSC-induced M2 polarization (64). Likewise, Huang et al. found that, with enhanced cell homing, MSCs can be applied to deliver miR-133b to boost the expression level of miR-133b in an ischemic lesion and further improve therapeutic effects (159). To sum up, MSCs can not only directly regulate the level of ncRNA but also indirectly regulate the level of ncRNA in the form of secreting exosomes, thus promoting the improvement of neurological function recovery. More details regarding preclinical studies that evaluate the effect of MSC-ncRNA on treating ischemic stroke are shown in Table 2 (31, 33, 34, 42, 64, 114, 115, 117, 119, 121–125, 129–133, 135, 136, 138, 158, 159).
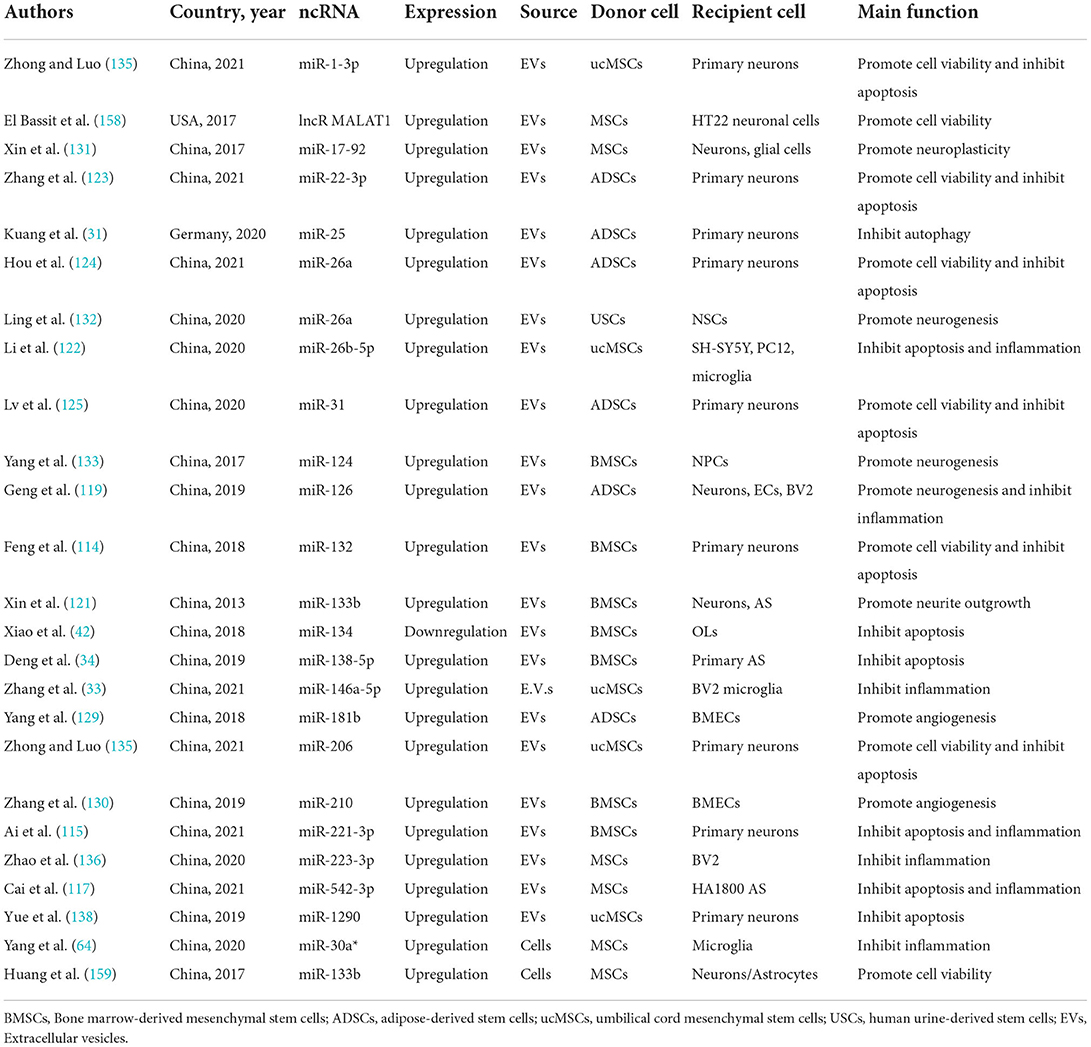
Table 2. Preclinical studies evaluating the effect of MSC-non-coding RNA on treating ischemic stroke.
The critical role of trophic factors and cytokines in treating ischemic stroke
Preclinical studies in rodent models of ischemic stroke have uncovered the potential effectiveness of the administration of trophic factors in ischemic brain injury recovery. The brain-derived neurotrophic factor (BDNF), glial cell line-derived neurotrophic factor (GDNF), and vascular endothelial growth factor (VEGF) are the most described (160). MSCs released or stimulated the release of three aforementioned neurotrophic factors associated with the contribution of ischemic stroke recovery. After administration, MSCs migrated from the vascular system outside the lesion to the area of the lesion core or peri-lesion to reduce the infarct volume by secreting BDNF, GDNF, and VEGF (161, 162). BDNF protein, highly expressed in the hippocampus, is known to affect the survival and proliferation of several neural cells, including cerebellar and cortical neurons (163). BDNF rapidly boosts in response to ischemic brain injury, contributing to reducing neuronal apoptosis and promoting neuronal survival (163). GDNF, produced by glial cells after brain injury, accelerates the survival and recovery of several types of mature neurons, including motor and dopaminergic neurons (164). VEGF, produced by neurons and astrocytes, is involved in various stages of neurodevelopment (proliferation, migration, differentiation, synaptogenesis, myelination (160). Additionally, VEGF stimulates angiogenesis by stimulating endothelial cell proliferation and migration and increases blood-brain barrier integrity (160). Notably, further growth and trophic factors, namely TGF-β, bFGF, IGF-1, HGF, and HGF, released or regulated by MSCs, are also involved in post-stroke neurological recovery. The types of cytokines released directly by MSCs or indirectly modulated in response to neuroinflammation due to stem cell transplantation are huge. They cannot be discussed in full detail here. Briefly, anti-inflammatory cytokines of IL-10 and IL-13, proinflammatory cytokines IL-8, IL-1α, and IL-12, and pleiotropic cytokines of IL-6, IL-11, IL-16, and IL-1β, correlated to immune function modulation after ischemic stroke, are revealed to be directly or indirectly produced by MSCs (165). In summary, MSCs played diverse therapeutic roles by secreting a series of trophic factors and cytokines. Hence, gene modification could be performed to enhance the therapeutic effects of MSCs by modulating the trophic factors and cytokines. However, attention should be given to the adverse effects of trophic factors and cytokines due to the adverse concentration. Some of the underlying therapeutic mechanisms associated with MSCs in ischemic stroke are summarized in Figure 2.
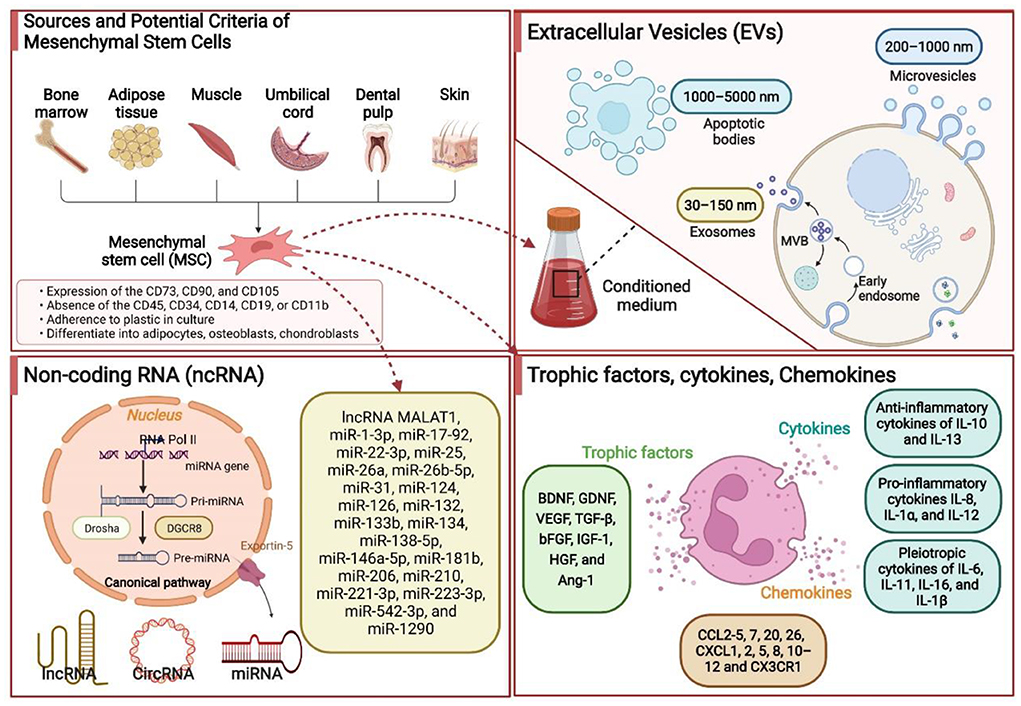
Figure 2. Some of the underlying therapeutic mechanisms associated with the mesenchymal stem cells (MSCs) in ischemic stroke. MSCs are isolated and identified from various tissue sources. These MSCs produce extracellular vesicles (EVs), noncoding RNAs (ncRNAs), trophic factors, chemokines, and cytokines by paracrine mechanisms to promote neurological recovery. Based on the EVs size, they can be divided into three subtypes, namely exosomes (30–150 nm), microvesicles (200–1,000 nm), and apoptotic bodies (1,000–5,000 nm). ncRNAs primarily include microRNA, long noncoding RNA, and circRNA. BDNF, brain-derived neurotrophic factor; GDNF, glial cell line-derived neurotrophic factor; VEGF, vascular endothelial growth factor; bFGF, basic fibroblast growth factor; IL, interleukin; TGF-β, transforming growth factor-β; HGF, hepatocyte growth factor; miR, microRNA.
Therapeutic application of stem cells in clinical ischemic stroke study
Meta-analysis: The clinical application of MSCs in treating ischemic stroke
A comprehensive literature search of several electronic databases, namely PubMed, Cochrane Library, EMBASE, and Web of Science, was performed by two researchers independently from the inception of these databases to 30 June 2022. We retrieved studies assessing stem cells in treating ischemic stroke by adopting the following keywords: “stem cell” together with “ischemia,” “stroke,” “middle cerebral artery occlusion,” or “MCAO.” References from the identified reports were manually searched to identify other potential qualifying studies. The specific screening process is shown in Supplementary Figure 1. A total of 16 reports were included in this section from South Korea, India, the UK, China, the United States, Egypt, and Spain, which were conducted varied from 2005 to 2019 (166–181). Table 3, Supplementary Table 1 described the characteristics and quality assessment of included studies, respectively. The Stata, version 12.0, was used for endpoint analyses. When I2 > 50%, the data were deemed to have apparent heterogeneity, and a random-effect model was adopted. Otherwise, a fixed-effects model was adopted. Among all outcomes, weighted mean differences (WMD) or rate differences (RDs) with 95% CIs were applied for the assessment.
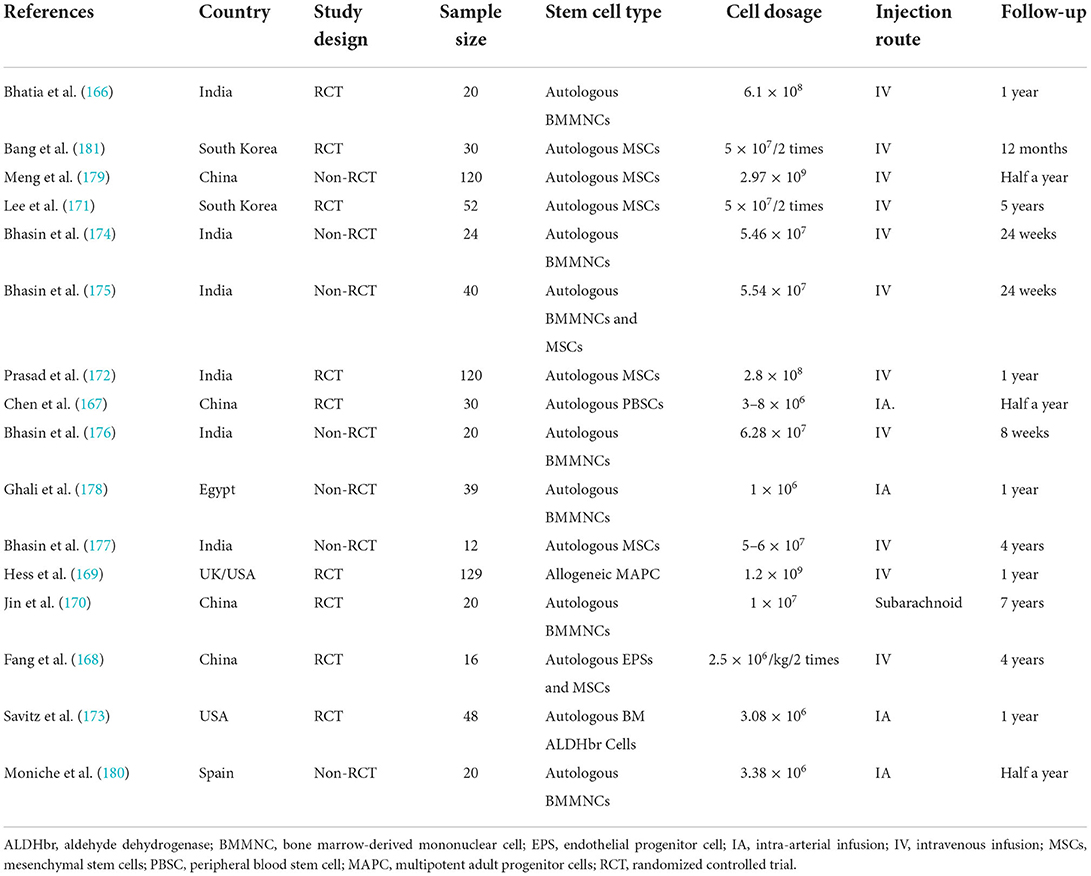
Table 3. Main characteristics of the clinical study assessing stem cells in treating ischemic stroke.
First, this study analyzed the efficacy of MSCs on patients with ischemic stroke through the modified Rankin Scale (mRS), National Institutes of Health Stroke Scale (NIHSS), and Barthel index (BI). Data on mRS were provided by night studies. There are 219 and 227 participants in the MSCs and control groups. The patients treated with MSCs were associated with a statistically significant lower mRS value (WMD, −0.354; 95% CI, −0.681 to −0.027; P = 0.034, Figure 3A). Similarly, seven and nine studies reported the data of NIHSS and BI, respectively. The cross-sectional data from various studies were plotted and demonstrated that the NIHSS was statistically lower (WMD, −1.538; 95% CI, −2.506 to −0.571; P = 0.002, Figure 3B) and BI was statistically higher (WMD, 7.444; 95% CI, −4.488 to 10.401; P < 0.001, Figure 3C) in the MSCs group than that of the control group. Second, we also evaluated the safety of MSCs on patients with ischemic stroke; 15 studies (356 and 354 patients in the MSCs and control group, respectively) reported the death rate. No significant heterogeneity was observed, and a fix-effect model was used (I2 = 40%, P = 0.055). The death rate between the experimental and control groups was statistically significant (RD, −0.046; 95% CI, −0.086 to −0.005; P = 0.026, Figure 3D). More details regarding the results are described in Supplementary Table 2. Altogether, stem cell-based therapies have the capacity to improve neurological deficits and activities of daily living in patients with ischemic stroke. However, several common limitations exist for current studies, such as small sample size, long-term waiting for MSC culture, age of participants, heterogeneity of ischemic brain injury site, and severity (155, 156).
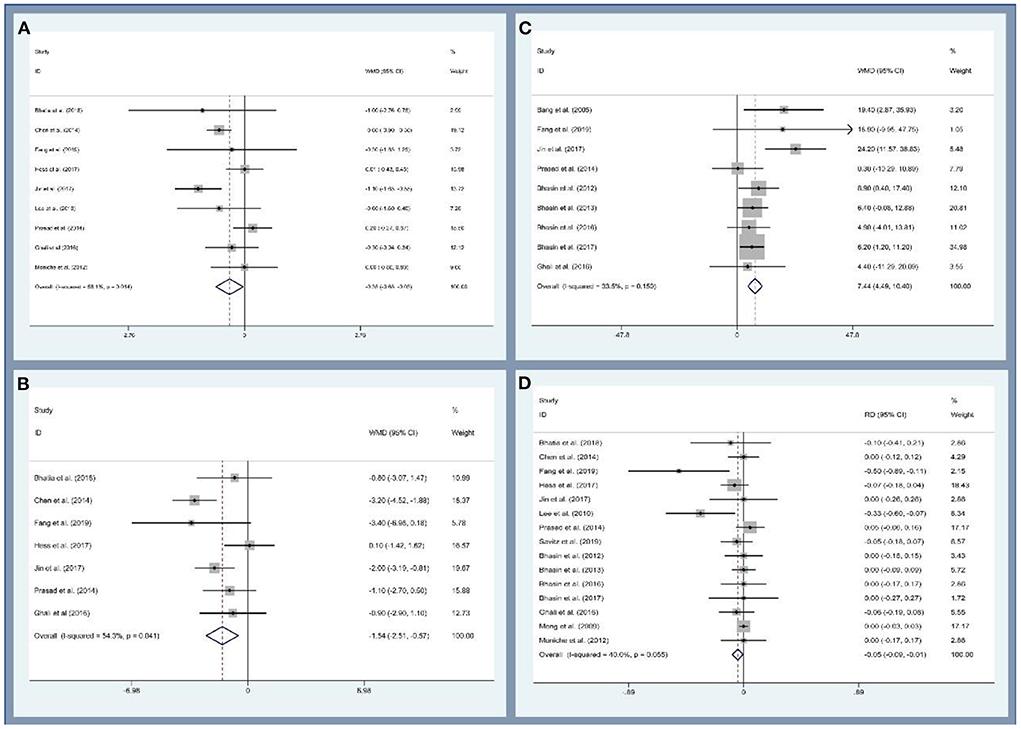
Figure 3. Forest plot for meta-analysis of the modified Rankin Scale (mRS) (A), National Institute of Health Stroke Scale (NIHSC) (B), Barthel index (BI) (C), and death rate (D).
The clinical translation of MSC-based therapy for ischemic stroke is booming, and MSCs are expected to improve the sequence of ischemic stroke in patients. Nevertheless, this treatment has led to some controversy as well. (I) Stem cell translation has the potential to result in tumor formation (157). For example, stem cells derived from embryonic stem cells may have the potential for tumorigenicity. Moreover, a reduction of genetic modification of stem cells will be associated with a lower risk of tumor formation. (II) the controlled treatment of transplanted exogenous stem cells to regulate differentiation and achieve the desired therapeutic effect has yet to be studied (157, 182). (III) the insufficient brain delivery and retention and the invasiveness of current administration routes prevent MSCs from fully exerting their clinical therapeutic potential (183). (IV) the issue of immune rejection is also necessary to be addressed. Although MSCs rarely express the major histocompatibility complex, they can still cause some immunological issues (182).
Conclusion
The application of MSCs in treating ischemic stroke is vast. In preclinical settings, the transplantation of MSCs offers an excellent opportunity for adjuvant ischemic stroke treatment, participating in multiple pathological processes, such as increasing cell survival angiogenesis and suppressing cell apoptosis and autophagy. Importantly, immunomodulation is another excellent target of MSCs by interacting with a variety of immune cells, namely microglia, neutrophils, NK cells, DCs, T cells, and B cells. However, no large-scale randomized, double-blind, multicenter clinical study exists to prove their effectiveness. In clinic, MSCs have many advantages: they are easy to harvest, expand, and store for a long time and are convenient to manage in many ways. Additionally, their clinical use does not raise many ethical issues. Increasing evidence supports the potential of MSCs to treat stroke, and autologous stem cell-based therapies can improve post-stroke neurological deficits and daily living activities in patients with minimal clinical adverse events. Nevertheless, the heterogeneity of MSCs is the primary barrier to their clinical application and therapeutic effect. Nonetheless, despite these issues, the application of MSCs appears to achieve neuroprotective effects, which result from the release of EVs and modification of various signaling pathways, such as ncRNAs, trophic factors, and cytokines.
Author contributions
NT, MH, and YM designed and conceptualized the article. NT prepared the figures and tables. All authors significantly contributed to writing the paper and provided important intellectual content.
Conflict of interest
The authors declare that the research was conducted in the absence of any commercial or financial relationships that could be construed as a potential conflict of interest.
Publisher's note
All claims expressed in this article are solely those of the authors and do not necessarily represent those of their affiliated organizations, or those of the publisher, the editors and the reviewers. Any product that may be evaluated in this article, or claim that may be made by its manufacturer, is not guaranteed or endorsed by the publisher.
Supplementary material
The Supplementary Material for this article can be found online at: https://www.frontiersin.org/articles/10.3389/fneur.2022.1048113/full#supplementary-material
References
1. Phipps MS, Cronin CA. Management of acute ischemic stroke. BMJ. (2020) 368:l6983. doi: 10.1136/bmj.l6983
2. Khandelwal P, Yavagal DR, Sacco RL. Acute ischemic stroke intervention. J Am Coll Cardiol. (2016) 67:2631–44. doi: 10.1016/j.jacc.2016.03.555
3. Del Zoppo GJ, Saver JL, Jauch EC, Adams HP Jr. Expansion of the time window for treatment of acute ischemic stroke with intravenous tissue plasminogen activator: a science advisory from the American Heart Association/American Stroke Association. Stroke. (2009) 40:2945–8. doi: 10.1161/STROKEAHA.109.192535
4. Liu X. Beyond the time window of intravenous thrombolysis: standing by or by stenting? Interv Neurol. (2012) 1:3–15. doi: 10.1159/000338389
5. Savitz SI, Chopp M, Deans R, Carmichael ST, Phinney D, Wechsler L. Stem cell therapy as an emerging paradigm for stroke (STEPS) II. Stroke. (2011) 42:825–9. doi: 10.1161/STROKEAHA.110.601914
6. Sui BD, Zheng CX, Li M, Jin Y, Hu CH. Epigenetic regulation of mesenchymal stem cell homeostasis. Trends Cell Biol. (2020) 30:97–116. doi: 10.1016/j.tcb.2019.11.006
7. Fu X, Liu G, Halim A, Ju Y, Luo Q, Song AG. Mesenchymal stem cell migration and tissue repair. Cells. (2019) 8:784. doi: 10.3390/cells8080784
8. Chamberlain G, Fox J, Ashton B, Middleton J. Concise review: mesenchymal stem cells: their phenotype, differentiation capacity, immunological features, and potential for homing. Stem Cells. (2007) 25:2739–49. doi: 10.1634/stemcells.2007-0197
9. Djouad F, Plence P, Bony C, Tropel P, Apparailly F, Sany J, et al. Immunosuppressive effect of mesenchymal stem cells favors tumor growth in allogeneic animals. Blood. (2003) 102:3837–44. doi: 10.1182/blood-2003-04-1193
10. Song N, Scholtemeijer M, Shah K. Mesenchymal stem cell immunomodulation: mechanisms and therapeutic potential. Trends Pharmacol Sci. (2020) 41:653–64. doi: 10.1016/j.tips.2020.06.009
11. Kim HY, Kim TJ, Kang L, Kim YJ, Kang MK, Kim J, et al. Mesenchymal stem cell-derived magnetic extracellular nanovesicles for targeting and treatment of ischemic stroke. Biomaterials. (2020) 243:119942. doi: 10.1016/j.biomaterials.2020.119942
12. Wang F, Tang H, Zhu J, Zhang JH. Transplanting mesenchymal stem cells for treatment of ischemic stroke. Cell Transpl. (2018) 27:1825–34. doi: 10.1177/0963689718795424
13. Zhao Y, Gan Y, Xu G, Yin G, Liu D. MSCs-derived exosomes attenuate acute brain injury and inhibit microglial inflammation by reversing CysLT2R-ERK1/2 mediated microglia M1 polarization. Neurochem Res. (2020) 45:1180–90. doi: 10.1007/s11064-020-02998-0
14. Wei L, Fraser JL, Lu ZY, Hu X, Yu SP. Transplantation of hypoxia preconditioned bone marrow mesenchymal stem cells enhances angiogenesis and neurogenesis after cerebral ischemia in rats. Neurobiol Dis. (2012) 46:635–45. doi: 10.1016/j.nbd.2012.03.002
15. Friedenstein AJ, Petrakova KV, Kurolesova AI, Frolova GP. Heterotopic of bone marrow. Analysis of precursor cells for osteogenic and hematopoietic tissues. Transplantation. (1968) 6:230–47. doi: 10.1097/00007890-196803000-00009
16. Andrzejewska A, Lukomska B, Janowski M. Concise review: mesenchymal stem cells: from roots to boost. Stem Cells. (2019) 37:855–64. doi: 10.1002/stem.3016
17. Xin WQ, Wei W, Pan YL, Cui BL, Yang XY, Bähr M, et al. Modulating poststroke inflammatory mechanisms: novel aspects of mesenchymal stem cells, extracellular vesicles and microglia. World J Stem Cells. (2021) 13:1030–48. doi: 10.4252/wjsc.v13.i8.1030
18. Kern S, Eichler H, Stoeve J, Klüter H, Bieback K. Comparative analysis of mesenchymal stem cells from bone marrow, umbilical cord blood, or adipose tissue. Stem Cells. (2006) 24:1294–301. doi: 10.1634/stemcells.2005-0342
19. In 'T Anker PS, Noort WA, Scherjon SA, Kleijburg-Van Der Keur C, Kruisselbrink AB, Van Bezooijen RL, et al. Mesenchymal stem cells in human second-trimester bone marrow, liver, lung, and spleen exhibit a similar immunophenotype but a heterogeneous multilineage differentiation potential. Haematologica. (2003) 88:845–52.
20. Yeo RW, Lai RC, Zhang B, Tan SS, Yin Y, Teh BJ, et al. Mesenchymal stem cell: an efficient mass producer of exosomes for drug delivery. Adv Drug Deliv Rev. (2013) 65:336–41. doi: 10.1016/j.addr.2012.07.001
21. Horwitz EM, Le Blanc K, Dominici M, Mueller I, Slaper-Cortenbach I, Marini FC, et al. Clarification of the nomenclature for MSC: the International Society for Cellular Therapy position statement. Cytotherapy. (2005) 7:393–5. doi: 10.1080/14653240500319234
22. Dominici M, Le Blanc K, Mueller I, Slaper-Cortenbach I, Marini F, Krause D, et al. Minimal criteria for defining multipotent mesenchymal stromal cells. The International Society for Cellular Therapy position statement. Cytotherapy. (2006) 8:315–17. doi: 10.1080/14653240600855905
23. Li J, Zhang Q, Wang W, Lin F, Wang S, Zhao J. Mesenchymal stem cell therapy for ischemic stroke: a look into treatment mechanism and therapeutic potential. J Neurol. (2021) 268:4095–107. doi: 10.1007/s00415-020-10138-5
24. Lv FJ, Tuan RS, Cheung KM, Leung VY. Concise review: the surface markers and identity of human mesenchymal stem cells. Stem Cells. (2014) 32:1408–19. doi: 10.1002/stem.1681
25. Camilleri ET, Gustafson MP, Dudakovic A, Riester SM, Garces CG, Paradise CR, et al. Identification and validation of multiple cell surface markers of clinical-grade adipose-derived mesenchymal stromal cells as novel release criteria for good manufacturing practice-compliant production. Stem Cell Res Ther. (2016) 7:107. doi: 10.1186/s13287-016-0370-8
26. Pachón-Peña G, Donnelly C, Ruiz-Cañada C, Katz A, Fernández-Veledo S, Vendrell J, et al. A glycovariant of human CD44 is characteristically expressed on human mesenchymal stem cells. Stem Cells. (2017) 35:1080–92. doi: 10.1002/stem.2549
27. Hermann DM, Chopp M. Promoting brain remodelling and plasticity for stroke recovery: therapeutic promise and potential pitfalls of clinical translation. Lancet Neurol. (2012) 11:369–80. doi: 10.1016/S1474-4422(12)70039-X
28. Reitmeir R, Kilic E, Kilic U, Bacigaluppi M, Elali A, Salani G, et al. Post-acute delivery of erythropoietin induces stroke recovery by promoting perilesional tissue remodelling and contralesional pyramidal tract plasticity. Brain. (2011) 134(Pt. 1):84–99. doi: 10.1093/brain/awq344
29. Xiao X, Li W, Xu Z, Sun Z, Ye H, Wu Y, et al. Extracellular vesicles from human umbilical cord mesenchymal stem cells reduce lipopolysaccharide-induced spinal cord injury neuronal apoptosis by mediating miR-29b-3p/PTEN. Connect Tissue Res. (2022) 63:634–49. doi: 10.1080/03008207.2022.2060826
30. De Simone U, Spinillo A, Caloni F, Gribaldo L, Coccini T. Neuron-like cells generated from human umbilical cord lining-derived mesenchymal stem cells as a new in vitro model for neuronal toxicity screening: using magnetite nanoparticles as an example. Int J Mol Sci. (2019) 21:271. doi: 10.3390/ijms21010271
31. Kuang Y, Zheng X, Zhang L, Ai X, Venkataramani V, Kilic E, et al. Adipose-derived mesenchymal stem cells reduce autophagy in stroke mice by extracellular vesicle transfer of miR-25. J Extracell Vesicles. (2020) 10:e12024. doi: 10.1002/jev2.12024
32. Liu X, Zhang M, Liu H, Zhu R, He H, Zhou Y, et al. Bone marrow mesenchymal stem cell-derived exosomes attenuate cerebral ischemia-reperfusion injury-induced neuroinflammation and pyroptosis by modulating microglia M1/M2 phenotypes. Exp Neurol. (2021) 341:113700. doi: 10.1016/j.expneurol.2021.113700
33. Zhang Z, Zou X, Zhang R, Xie Y, Feng Z, Li F, et al. Human umbilical cord mesenchymal stem cell-derived exosomal miR-146a-5p reduces microglial-mediated neuroinflammation via suppression of the IRAK1/TRAF6 signaling pathway after ischemic stroke. Aging. (2021) 13:3060–79. doi: 10.18632/aging.202466
34. Deng Y, Chen D, Gao F, Lv H, Zhang G, Sun X, et al. Exosomes derived from microRNA-138–5p-overexpressing bone marrow-derived mesenchymal stem cells confer neuroprotection to astrocytes following ischemic stroke via inhibition of LCN2. J Biol Eng. (2019) 13:71. doi: 10.1186/s13036-019-0193-0
35. Liu K, Guo L, Zhou Z, Pan M, Yan C. Mesenchymal stem cells transfer mitochondria into cerebral microvasculature and promote recovery from ischemic stroke. Microvasc Res. (2019) 123:74–80. doi: 10.1016/j.mvr.2019.01.001
36. Li S, Rao JH, Lan XY, Li X, Chu CY, Liang Y, et al. White matter demyelination predates axonal injury after ischemic stroke in cynomolgus monkeys. Exp Neurol. (2021) 340:113655. doi: 10.1016/j.expneurol.2021.113655
37. Bagdasarian FA, Yuan X, Athey J, Bunnell BA, Grant SC. NODDI highlights recovery mechanisms in white and gray matter in ischemic stroke following human stem cell treatment. Magn Reson Med. (2021) 86:3211–23. doi: 10.1002/mrm.28929
38. Li J, Xiao L, He D, Luo Y, Sun H. Mechanism of white matter injury and promising therapeutic strategies of MSCs after intracerebral hemorrhage. Front Aging Neurosci. (2021) 13:632054. doi: 10.3389/fnagi.2021.632054
39. Datta A, Sarmah D, Mounica L, Kaur H, Kesharwani R, Verma G, et al. Cell death pathways in ischemic stroke and targeted pharmacotherapy. Transl Stroke Res. (2020) 11:1185–202. doi: 10.1007/s12975-020-00806-z
40. Radak D, Katsiki N, Resanovic I, Jovanovic A, Sudar-Milovanovic E, Zafirovic S, et al. Apoptosis and acute brain ischemia in ischemic stroke. Curr Vasc Pharmacol. (2017) 15:115–22. doi: 10.2174/1570161115666161104095522
41. Kong D, Zhu J, Liu Q, Jiang Y, Xu L, Luo N, et al. Mesenchymal stem cells protect neurons against hypoxic-ischemic injury via inhibiting parthanatos, necroptosis, and apoptosis, but not autophagy. Cell Mol Neurobiol. (2017) 37:303–13. doi: 10.1007/s10571-016-0370-3
42. Xiao Y, Geng F, Wang G, Li X, Zhu J, Zhu W. Bone marrow-derived mesenchymal stem cells-derived exosomes prevent oligodendrocyte apoptosis through exosomal miR-134 by targeting caspase-8. J Cell Biochem. (2018) 120:2109–18. doi: 10.1002/jcb.27519
43. Wei W, Pan Y, Yang X, Chen Z, Heng Y, Yang B, et al. The emerging role of the interaction of extracellular vesicle and autophagy-novel insights into neurological disorders. J Inflamm Res. (2022) 15:3395–407. doi: 10.2147/JIR.S362865
44. Li Y, Guo S, Liu W, Jin T, Li X, He X, et al. Silencing of SNHG12 enhanced the effectiveness of MSCs in alleviating ischemia/reperfusion injuries via the PI3K/AKT/mTOR signaling pathway. Front Neurosci. (2019) 13:645. doi: 10.3389/fnins.2019.00645
45. Huang X, Ding J, Li Y, Liu W, Ji J, Wang H, et al. Exosomes derived from PEDF modified adipose-derived mesenchymal stem cells ameliorate cerebral ischemia-reperfusion injury by regulation of autophagy and apoptosis. Exp Cell Res. (2018) 371:269–77. doi: 10.1016/j.yexcr.2018.08.021
46. Yang BO, Duan WU, Wei L, Zhao Y, Han Z, Wang J, et al. Bone marrow mesenchymal stem cell-derived hepatocyte-like cell exosomes reduce hepatic ischemia/reperfusion injury by enhancing autophagy. Stem Cells Dev. (2020) 29:372–9. doi: 10.1089/scd.2019.0194
47. Zeng Q, Zhou Y, Liang D, He H, Liu X, Zhu R, et al. Exosomes secreted from bone marrow mesenchymal stem cells attenuate oxygen-glucose deprivation/reoxygenation-induced pyroptosis in PC12 cells by promoting AMPK-dependent autophagic flux. Front Cell Neurosci. (2020) 14:182. doi: 10.3389/fncel.2020.00182
48. Wakabayashi K, Nagai A, Sheikh Am Shiota Y, Narantuya D, Watanabe T, Masuda J, et al. Transplantation of human mesenchymal stem cells promotes functional improvement and increased expression of neurotrophic factors in a rat focal cerebral ischemia model. J Neurosci Res. (2010) 88:1017–25. doi: 10.1002/jnr.22279
49. Kurozumi K, Nakamura K, Tamiya T, Kawano Y, Kobune M, Hirai S, et al. BDNF gene-modified mesenchymal stem cells promote functional recovery and reduce infarct size in the rat middle cerebral artery occlusion model. Mol Ther. (2004) 9:189–97. doi: 10.1016/j.ymthe.2003.10.012
50. Nomura T, Honmou O, Harada K, Houkin K, Hamada H, Kocsis JD. IV. Infusion of brain-derived neurotrophic factor gene-modified human mesenchymal stem cells protects against injury in a cerebral ischemia model in adult rat. Neuroscience. (2005) 136:161–9. doi: 10.1016/j.neuroscience.2005.06.062
51. Onda T, Honmou O, Harada K, Houkin K, Hamada H, Kocsis JD. Therapeutic benefits by human mesenchymal stem cells (hMSCs) and Ang-1 gene-modified hMSCs after cerebral ischemia. J Cereb Blood Flow Metab. (2008) 28:329–40. doi: 10.1038/sj.jcbfm.9600527
52. Jeong CH, Kim SM, Lim JY, Ryu CH, Jun JA, Jeun SS. Mesenchymal stem cells expressing brain-derived neurotrophic factor enhance endogenous neurogenesis in an ischemic stroke model. BioMed Res Int. (2014) 2014:129145. doi: 10.1155/2014/129145
53. Xin H, Li Y, Cui Y, Yang JJ, Zhang ZG, Chopp M. Systemic administration of exosomes released from mesenchymal stromal cells promote functional recovery and neurovascular plasticity after stroke in rats. J Cereb Blood Flow Metab. (2013) 33:1711–15. doi: 10.1038/jcbfm.2013.152
54. Cho SE, Kim YM, Jeong JS, Seo YK. The effect of ultrasound for increasing neural differentiation in hBM-MSCs and inducing neurogenesis in ischemic stroke model. Life Sci. (2016) 165:35–42. doi: 10.1016/j.lfs.2016.08.029
55. Toyama K, Honmou O, Harada K, Suzuki J, Houkin K, Hamada H, et al. Therapeutic benefits of angiogenetic gene-modified human mesenchymal stem cells after cerebral ischemia. Exp Neurol. (2009) 216:47–55. doi: 10.1016/j.expneurol.2008.11.010
56. Chen J, Zhang ZG, Li Y, Wang L, Xu YX, Gautam SC, et al. Intravenous administration of human bone marrow stromal cells induces angiogenesis in the ischemic boundary zone after stroke in rats. Circ Res. (2003) 92:692–9. doi: 10.1161/01.RES.0000063425.51108.8D
57. Guruswamy R, Elali A. Complex roles of microglial cells in ischemic stroke pathobiology: new insights and future directions. Int J Mol Sci. (2017) 18:496. doi: 10.3390/ijms18030496
58. Qin C, Zhou LQ, Ma XT, Hu ZW, Yang S, Chen M, et al. Dual functions of microglia in ischemic stroke. Neurosci Bull. (2019) 35:921–33. doi: 10.1007/s12264-019-00388-3
59. Iadecola C, Anrather J. The immunology of stroke: from mechanisms to translation. Nat Med. (2011) 17:796–808. doi: 10.1038/nm.2399
60. Jin R, Yang G, Li G. Inflammatory mechanisms in ischemic stroke: role of inflammatory cells. J Leukoc Biol. (2010) 87:779–89. doi: 10.1189/jlb.1109766
61. Cunningham CJ, Wong R, Barrington J, Tamburrano S, Pinteaux E, Allan SM. Systemic conditioned medium treatment from interleukin-1 primed mesenchymal stem cells promotes recovery after stroke. Stem Cell Res Ther. (2020) 11:1–12. doi: 10.1186/s13287-020-1560-y
62. Yamaguchi S, Horie N, Satoh K, Ishikawa T, Mori T, Maeda H, et al. Age of donor of human mesenchymal stem cells affects structural and functional recovery after cell therapy following ischaemic stroke. J Cereb Blood Flow Metab. (2018) 38:1199–212. doi: 10.1177/0271678X17731964
63. McGuckin CP, Jurga M, Miller AM, Sarnowska A, Wiedner M, Boyle NT, et al. Ischemic brain injury: a consortium analysis of key factors involved in mesenchymal stem cell-mediated inflammatory reduction. Arch Biochem Biophys. (2013) 534:88–97. doi: 10.1016/j.abb.2013.02.005
64. Yang F, Li WB, Qu YW, Gao JX, Tang YS, Wang DJ, et al. Bone marrow mesenchymal stem cells induce M2 microglia polarization through PDGF-AA/MANF signaling. World J Stem Cells. (2020) 12:633–58. doi: 10.4252/wjsc.v12.i7.633
65. Feng YW, Wu C, Liang FY, Lin T, Li WQ, Jing YH, et al. hUCMSCs mitigate LPS-induced trained immunity in ischemic stroke. Front Immunol. (2020) 11:1746. doi: 10.3389/fimmu.2020.01746
66. Narantuya D, Nagai A, Sheikh AM, Wakabayashi K, Shiota Y, Watanabe T, et al. Microglia transplantation attenuates white matter injury in rat chronic ischemia model via matrix metalloproteinase-2 inhibition. Brain Res. (2010) 1316:145–52. doi: 10.1016/j.brainres.2009.12.043
67. Ishizaka S, Horie N, Satoh K, Fukuda Y, Nishida N, Nagata I. Intra-arterial cell transplantation provides timing-dependent cell distribution and functional recovery after stroke. Stroke. (2013) 44:720–26. doi: 10.1161/STROKEAHA.112.677328
68. Wang LQ, Lin ZZ, Zhang HX, Shao B, Xiao L, Jiang HG, et al. Timing and dose regimens of marrow mesenchymal stem cell transplantation affect the outcomes and neuroinflammatory response after ischemic stroke. CNS Neurosci Ther. (2014) 20:317–26. doi: 10.1111/cns.12216
69. Nakajima M, Nito C, Sowa K, Suda S, Nishiyama Y, Nakamura-Takahashi A, et al. Mesenchymal stem cells overexpressing interleukin-10 promote neuroprotection in experimental acute ischemic stroke. Mol Ther Methods Clin Dev. (2017) 6:102–111. doi: 10.1016/j.omtm.2017.06.005
70. Li X, Huang M, Zhao R, Zhao C, Liu Y, Zou H, et al. Intravenously delivered allogeneic mesenchymal stem cells bidirectionally regulate inflammation and induce neurotrophic effects in distal middle cerebral artery occlusion rats within the first 7 days after stroke. Cell Physiol Biochem. (2018) 46:1951–70. doi: 10.1159/000489384
71. Lv B, Li F, Fang J, Xu L, Sun C, Han J, et al. Activated microglia induce bone marrow mesenchymal stem cells to produce glial cell-derived neurotrophic factor and protect neurons against oxygen-glucose deprivation injury. Front Cell Neurosci. (2016) 10:283. doi: 10.3389/fncel.2016.00283
72. Sheikh AM, Yano S, Mitaki S, Haque MA, Yamaguchi S, Nagai A. A mesenchymal stem cell line (B10) increases angiogenesis in a rat MCAO model. Exp Neurol. (2019) 311:182–93. doi: 10.1016/j.expneurol.2018.10.001
73. Wang H, Nagai A, Sheikh AM, Liang XY, Yano S, Mitaki S, et al. Human mesenchymal stem cell transplantation changes proinflammatory gene expression through a nuclear factor-κB-dependent pathway in a rat focal cerebral ischemic model. J Neurosci Res. (2013) 91:1440–49. doi: 10.1002/jnr.23267
74. Yoo SW, Chang DY, Lee HS, Kim GH, Park JS, Ryu BY, et al. Immune following suppression mesenchymal stem cell transplantation in the ischemic brain is mediated by TGF-β. Neurobiol Dis. (2013) 58:249–57. doi: 10.1016/j.nbd.2013.06.001
75. Sheikh AM, Nagai A, Wakabayashi K, Narantuya D, Kobayashi S, Yamaguchi S, et al. Mesenchymal stem cell transplantation modulates neuroinflammation in focal cerebral ischemia: contribution of fractalkine and IL-5. Neurobiol Dis. (2011) 41:717–24. doi: 10.1016/j.nbd.2010.12.009
76. Gelderblom M, Leypoldt F, Steinbach K, Behrens D, Choe CU, Siler DA, et al. Temporal and spatial dynamics of cerebral immune cell accumulation in stroke. Stroke. (2009) 40:1849–57. doi: 10.1161/STROKEAHA.108.534503
77. Wang C, Börger V, Mohamud Yusuf A, Tertel T, Stambouli O, Murke F, et al. Postischemic neuroprotection associated with anti-inflammatory effects by mesenchymal stromal cell-derived small extracellular vesicles in aged mice. Stroke. (2022) 53:e14–18. doi: 10.1161/STROKEAHA.121.035821
78. Hsu SC, Wang LT, Yao CL, Lai HY, Chan KY, Liu BS, et al. Mesenchymal stem cells promote neutrophil activation by inducing IL-17 production in CD4+ CD45RO+ T cells. Immunobiology. (2013) 218:90–95. doi: 10.1016/j.imbio.2012.02.007
79. Raffaghello L, Bianchi G, Bertolotto M, Montecucco F, Busca A, Dallegri F, et al. Human mesenchymal stem cells inhibit neutrophil apoptosis: a model for neutrophil preservation in the bone marrow niche. Stem Cells. (2008) 26:151–62. doi: 10.1634/stemcells.2007-0416
80. Vivier E, Tomasello E, Baratin M, Walzer T, Ugolini S. Functions of natural killer cells. Nat Immunol. (2008) 9:503–10. doi: 10.1038/ni1582
81. Wang Y, Chen X, Cao W, Shi Y. Plasticity of mesenchymal stem cells in immunomodulation: pathological and therapeutic implications. Nat Immunol. (2014) 15:1009–16. doi: 10.1038/ni.3002
82. Carrillo-Galvez Ab Cobo M, Cuevas-Ocaña S, Gutiérrez-Guerrero A, Sánchez-Gilabert A, Bongarzone P, García-Pérez A, et al. Mesenchymal stromal cells express GARP/LRRC32 on their surface: effects on their biology and immunomodulatory capacity. Stem Cells. (2015) 33:183–95. doi: 10.1002/stem.1821
83. Huang WH, Chen HL, Huang PH, Yew TL, Lin MW, Lin SJ, et al. Hypoxic mesenchymal stem cells engraft and ameliorate limb ischaemia in allogeneic recipients. Cardiovasc Res. (2014) 101:266–76. doi: 10.1093/cvr/cvt250
84. Felger JC, Abe T, Kaunzner UW, Gottfried-Blackmore A, Gal-Toth J, Mcewen BS, et al. Brain dendritic cells in ischemic stroke: time course, activation state, and origin. Brain Behav Immunity. (2010) 24:724–37. doi: 10.1016/j.bbi.2009.11.002
85. Gao WX, Sun YQ, Shi J, Li CL, Fang SB, Wang D, et al. Effects of mesenchymal stem cells from human induced pluripotent stem cells on differentiation, maturation, and function of dendritic cells. Stem Cell Res Ther. (2017) 8:48. doi: 10.1186/s13287-017-0499-0
86. Jo H, Eom YW, Kim HS, Park HJ, Kim HM, Cho MY. Regulatory dendritic cells induced by mesenchymal stem cells ameliorate dextran sodium sulfate-induced chronic colitis in mice. Gut Liver. (2018) 12:664–73. doi: 10.5009/gnl18072
87. Zhao Zg Xu W, Sun L, You Y, Li F, Li Qb Zou P. Immunomodulatory function of regulatory dendritic cells induced by mesenchymal stem cells. Immunol Investig. (2012) 41:183–98. doi: 10.3109/08820139.2011.607877
88. Zhang B, Liu R, Shi D, Liu X, Chen Y, Dou X, et al. Mesenchymal stem cells induce mature dendritic cells into a novel Jagged-2-dependent regulatory dendritic cell population. Blood. (2009) 113:46–57. doi: 10.1182/blood-2008-04-154138
89. Rich Robert R, Fleisher Thomas A, Shearer William T, Schroeder Jr Harry W, Frew Anthony J, Weyand Cornelia M. Clinical Immunology E-Book: Principles and Practice. Elsevier Health Sciences (2012).
90. Selvaraj UM, Stowe AM. Long-term T cell responses in the brain after an ischemic stroke. Discov Med. (2017) 24:323–33.
91. Zhang D, Ren J, Luo Y, He Q, Zhao R, Chang J, et al. T Cell response in ischemic stroke: from mechanisms to translational insights. Front Immunol. (2021) 12:707972. doi: 10.3389/fimmu.2021.707972
92. Chu HX, Kim HA, Lee S, Moore JP, Chan CT, Vinh A, et al. Immune cell infiltration in malignant middle cerebral artery infarction: comparison with transient cerebral ischemia. J Cereb Blood Flow Metab. (2014) 34:450–59. doi: 10.1038/jcbfm.2013.217
93. Liesz A, Suri-Payer E, Veltkamp C, Doerr H, Sommer C, Rivest S, et al. Regulatory T cells are key cerebroprotective immunomodulators in acute experimental stroke. Nat Med. (2009) 15:192–9. doi: 10.1038/nm.1927
94. Jellema RK, Wolfs TG, Lima Passos V, Zwanenburg A, Ophelders DR, Kuypers E, et al. Mesenchymal stem cells induce T-cell tolerance and protect the preterm brain after global hypoxia-ischemia. PLoS ONE. (2013) 8:e73031. doi: 10.1371/journal.pone.0073031
95. Consentius C, Akyüz L, Schmidt-Lucke Ja Tschöpe C, Pinzur L, Ofir R, Reinke P, et al. Mesenchymal stromal cells prevent allostimulation in vivo and control checkpoints of Th1 priming: migration of human DC to lymph nodes and NK cell activation. Stem Cells. (2015) 33:3087–99. doi: 10.1002/stem.2104
96. Cai J, Jiao X, Zhao S, Liang Y, Ning Y, Shi Y, et al. Transforming growth factor-β1-overexpressing mesenchymal stromal cells induced local tolerance in rat renal ischemia/reperfusion injury. Cytotherapy. (2019) 21:535–45. doi: 10.1016/j.jcyt.2018.12.003
97. Boothby M, Rickert RC. Metabolic regulation of the immune humoral response. Immunity. (2017) 46:743–55. doi: 10.1016/j.immuni.2017.04.009
98. Anthony IC, Crawford DH, Bell JE. B lymphocytes in the normal brain: contrasts with HIV-associated lymphoid infiltrates and lymphomas. Brain. (2003) 126:1058–67. doi: 10.1093/brain/awg118
99. Funaro MG, Messina M, Shabbir M, Wright P, Najjar S, Tabansky I, et al. The role of B cells in multiple sclerosis: more than antibodies. Discov Med. (2016) 22:251–5.
100. Ortega SB, Torres VO, Latchney SE, Whoolery CW, Noorbhai IZ, Poinsatte K, et al. B cells migrate into remote brain areas and support neurogenesis and functional recovery after focal stroke in mice. Proc Natl Acad Sci USA. (2020) 117:4983–93. doi: 10.1073/pnas.1913292117
101. Liu J, Liu Q, Chen X. The immunomodulatory effects of mesenchymal stem cells on regulatory B cells. Front Immunol. (2020) 11:1843. doi: 10.3389/fimmu.2020.01843
102. Shin TH, Lee BC, Choi SW, Shin JH, Kang I, Lee JY, et al. Human adipose tissue-derived mesenchymal stem cells alleviate atopic dermatitis via regulation of B lymphocyte maturation. Oncotarget. (2017) 8:512–22. doi: 10.18632/oncotarget.13473
103. Rosado MM, Bernardo ME, Scarsella M, Conforti A, Giorda E, Biagini S, et al. Inhibition of B-cell proliferation and antibody production by mesenchymal stromal cells is mediated by T cells. Stem Cells Dev. (2015) 24:93–103. doi: 10.1089/scd.2014.0155
104. Noh JE, Oh SH, Park IH, Song J. Intracerebral transplants of GMP-grade human umbilical cord-derived mesenchymal stromal cells effectively treat subacute-phase ischemic stroke in a rodent model. Front Cell Neurosci. (2020) 14:546659. doi: 10.3389/fncel.2020.546659
105. Kurozumi K, Nakamura K, Tamiya T, Kawano Y, Ishii K, Kobune M, et al. Mesenchymal stem cells that produce neurotrophic factors reduce ischemic damage in the rat middle cerebral artery occlusion model. Mol Ther. (2005) 11:96–104. doi: 10.1016/j.ymthe.2004.09.020
106. Vu Q, Xie K, Eckert M, Zhao W, Cramer SC. Meta-analysis of preclinical studies of mesenchymal stromal cells for ischemic stroke. Neurology. (2014) 82:1277–86. doi: 10.1212/WNL.0000000000000278
107. Haupt M, Zheng X, Kuang Y, Lieschke S, Janssen L, Bosche B, et al. Lithium modulates miR-1906 levels of mesenchymal stem cell-derived extracellular vesicles contributing to poststroke neuroprotection by toll-like receptor 4 regulation. Stem Cells Transl Med. (2021) 10:357–73. doi: 10.1002/sctm.20-0086
108. Datta A, Sarmah D, Kaur H, Chaudhary A, Mounica Kl Kalia K, Borah A, et al. Post-stroke impairment of the blood-brain barrier and perifocal vasogenic edema is alleviated by endovascular mesenchymal stem cell administration: modulation of the PKCδ/MMP9/AQP4-mediated pathway. Mol Neurobiol. (2022) 59:2758–75. doi: 10.1007/s12035-022-02761-2
109. Tang G, Liu Y, Zhang Z, Lu Y, Wang Y, Huang J, et al. Mesenchymal stem cells maintain blood-brain barrier integrity by inhibiting aquaporin-4 upregulation after cerebral ischemia. Stem Cells. (2014) 32:3150–62. doi: 10.1002/stem.1808
110. Akbar N, Azzimato V, Choudhury RP, Aouadi M. Extracellular vesicles in metabolic disease. Diabetologia. (2019) 62:2179–87. doi: 10.1007/s00125-019-05014-5
111. Li F, Kang X, Xin W, Li X. The emerging role of extracellular vesicle derived from neurons/neurogliocytes in central nervous system diseases: novel insights into ischemic stroke. Front Pharmacol. (2022) 13:890698. doi: 10.3389/fphar.2022.890698
112. Théry C, Ostrowski M, Segura E. Membrane vesicles as conveyors of immune responses. Nat Rev Immunol. (2009) 9:581–93. doi: 10.1038/nri2567
113. Saltarella I, Lamanuzzi A, Apollonio B, Desantis V, Bartoli G, Vacca A, et al. Role of extracellular vesicle-based cell-to-cell communication in multiple myeloma progression. Cells. (2021) 10:3185. doi: 10.3390/cells10113185
114. Feng B, Meng L, Luan L, Fang Z, Zhao P, Zhao G. viaUpregulation of extracellular vesicles-encapsulated mir-132 released from mesenchymal stem cells attenuates ischemic neuronal injury by inhibiting Smad2/c-jun pathway Acvr2b suppression. Front Cell Dev Biol. (2020) 8:568304. doi: 10.3389/fcell.2020.568304
115. Ai Z, Cheng C, Zhou L, Yin S, Wang L, Liu Y. Bone marrow mesenchymal stem cells-derived extracellular vesicles carrying microRNA-221–3p protect against ischemic stroke via ATF3. Brain Res Bull. (2021) 172:220–28. doi: 10.1016/j.brainresbull.2021.04.022
116. Venkat P, Cui C, Chopp M, Zacharek A, Wang F, Landschoot-Ward J, et al. MiR-126 mediates brain endothelial cell exosome treatment-induced neurorestorative effects after stroke in type 2 diabetes mellitus mice. Stroke. (2019) 50:2865–74. doi: 10.1161/STROKEAHA.119.025371
117. Cai G, Cai G, Zhou H, Zhuang Z, Liu K, Pei S, et al. Mesenchymal stem cell-derived exosome miR-542–3p suppresses inflammation and prevents cerebral infarction. Stem Cell Res Ther. (2021) 12:2. doi: 10.1186/s13287-020-02030-w
118. Qi Z, Zhao Y, Su Y, Cao B, Yang Jj Xing Q. Serum extracellular vesicle-derived mir-124–3p as a diagnostic and predictive marker for early-stage acute ischemic stroke. Front Mol Biosci. (2021) 8:685088. doi: 10.3389/fmolb.2021.685088
119. Geng W, Tang H, Luo S, Lv Y, Liang D, Kang X, et al. Exosomes from miRNA-126-modified ADSCs promotes functional recovery after stroke in rats by improving neurogenesis and suppressing microglia activation. Am J Transl Res. (2019) 11:780–92.
120. Yang J, Cao LL, Wang XP, Guo W, Guo RB, Sun YQ, et al. Neuronal extracellular vesicle derived miR-98 prevents salvageable neurons from microglial phagocytosis in acute ischemic stroke. Cell Death Dis. (2021) 12:23. doi: 10.1038/s41419-020-03310-2
121. Xin H, Li Y, Liu Z, Wang X, Shang X, Cui Y, et al. MiR-133b promotes neural plasticity and functional recovery after treatment of stroke with multipotent mesenchymal stromal cells in rats via transfer of exosome-enriched extracellular particles. Stem Cells. (2013) 31:2737–746. doi: 10.1002/stem.1409
122. Li G, Xiao L, Qin H, Zhuang Q, Zhang W, Liu L, et al. Exosomes-carried microRNA-26b-5p regulates microglia M1 polarization after cerebral ischemia/reperfusion. Cell Cycle. (2020) 19:1022–35. doi: 10.1080/15384101.2020.1743912
123. Zhang Y, Liu J, Su M, Wang X, Xie C. Exosomal microRNA-22–3p alleviates cerebral ischemic injury by modulating KDM6B/BMP2/BMF axis. Stem Cell Res Ther. (2021) 12:111. doi: 10.1186/s13287-020-02091-x
124. Hou Z, Chen J, Yang H, Hu X, Yang F. microRNA-26a shuttled by extracellular vesicles secreted from adipose-derived mesenchymal stem cells reduce neuronal damage through KLF9-mediated regulation of TRAF2/KLF2 axis. Adipocyte. (2021) 10:378–93. doi: 10.1080/21623945.2021.1938829
125. Lv H, Li J, Che Y. miR-31 from adipose stem cell-derived extracellular vesicles promotes recovery of neurological function after ischemic stroke by inhibiting TRAF6 and IRF5. Exp Neurol. (2021) 342:113611. doi: 10.1016/j.expneurol.2021.113611
126. Wu W, Liu J, Yang C, Xu Z, Huang J, Lin J. Astrocyte-derived exosome-transported microRNA-34c is neuroprotective against cerebral ischemia/reperfusion injury via TLR7 and the NF-κB/MAPK pathways. Brain Res Bull. (2020) 163:84–94. doi: 10.1016/j.brainresbull.2020.07.013
127. Xu L, Cao H, Xie Y, Zhang Y, Du M, Xu X, et al. Exosome-shuttled miR-92b-3p from ischemic preconditioned astrocytes protects neurons against oxygen and glucose deprivation. Brain Res. (2019) 1717:66–73. doi: 10.1016/j.brainres.2019.04.009
128. Li Z, Song Y, He T, Wen R, Li Y, Chen T, et al. viaM2 microglial small extracellular vesicles reduce glial scar formation the miR-124/STAT3 pathway after ischemic stroke in mice. Theranostics. (2021) 11:1232–48. doi: 10.7150/thno.48761
129. Yang Y, Cai Y, Zhang Y, Liu J, Xu Z. Exosomes secreted by adipose-derived stem cells contribute to angiogenesis of brain microvascular endothelial cells following oxygen-glucose deprivation in vitro through MicroRNA-181b/TRPM7 Axis. J Mol Neurosci. (2018) 65:74–83. doi: 10.1007/s12031-018-1071-9
130. Zhang H, Wu J, Wu J, Fan Q, Zhou J, Wu J, et al. Exosome-mediated targeted delivery of miR-210 for angiogenic therapy after cerebral ischemia in mice. J Nanobiotechnol. (2019) 17:29. doi: 10.1186/s12951-019-0461-7
131. Xin H, Katakowski M, Wang F, Qian Jy Liu Xs Ali Mm Buller B, Zhang Zg Chopp M. MicroRNA cluster miR-17–92 cluster in exosomes enhance neuroplasticity and functional recovery after stroke in rats. Stroke. (2017) 48:747–53. doi: 10.1161/STROKEAHA.116.015204
132. Ling X, Zhang G, Xia Y, Zhu Q, Zhang J, Li Q, et al. Exosomes from human urine-derived stem cells enhanced neurogenesis via miR-26a/HDAC6 axis after ischaemic stroke. J Cell Mol Med. (2020) 24:640–54. doi: 10.1111/jcmm.14774
133. Yang J, Zhang X, Chen X, Wang L, Yang G. Exosome mediated delivery of miR-124 promotes neurogenesis after ischemia. Mol Ther Nucleic Acids. (2017) 7:278–87. doi: 10.1016/j.omtn.2017.04.010
134. Song H, Zhang X, Chen R, Miao J, Wang L, Cui L, et al. Cortical neuron-derived exosomal MicroRNA-181c-3p inhibits neuroinflammation by downregulating CXCL1 in astrocytes of a rat model with ischemic brain injury. Neuroimmunomodulation. (2019) 26:217–33. doi: 10.1159/000502694
135. Zhong Y, Luo L. Exosomes from human umbilical vein endothelial cells ameliorate ischemic injuries by suppressing the RNA component of mitochondrial RNA-processing endoribonuclease via the induction of miR-206/miR-1–3p levels. Neuroscience. (2021) 476:34–44. doi: 10.1016/j.neuroscience.2021.08.026
136. Zhao Y, Gan Y, Xu G, Hua K, Liu D. Exosomes from MSCs overexpressing microRNA-223–3p attenuate cerebral ischemia through inhibiting microglial M1 polarization mediated inflammation. Life Sci. (2020) 260:118403. doi: 10.1016/j.lfs.2020.118403
137. Bu X, Li D, Wang F, Sun Q, Zhang Z. microRNA-361 Protective role of astrocyte-derived exosomal in cerebral ischemic-reperfusion injury by regulating the signaling pathway and targeting. Neuropsychiatr Dis Treat. (2020) 16:1863–77. doi: 10.2147/NDT.S260748
138. Yue KY, Zhang PR, Zheng MH, Cao XL, Cao Y, Zhang YZ, et al. Neurons can upregulate Cav-1 to increase intake of endothelial cells-derived extracellular vesicles that attenuate apoptosis via miR-1290. Cell Death Dis. (2019) 10:869. doi: 10.1038/s41419-019-2100-5
139. Ye Z, Hu J, Xu H, Sun B, Jin Y, Zhang Y, et al. Serum exosomal microRNA-27–3p aggravates cerebral injury and inflammation in patients with acute cerebral infarction by targeting PPARγ. Inflammation. (2021) 44:1035–48. doi: 10.1007/s10753-020-01399-3
140. Cui J, Liu N, Chang Z, Gao Y, Bao M, Xie Y, et al. Exosomal microRNA-126 from RIPC serum is involved in hypoxia tolerance in SH-SY5Y cells by downregulating DNMT3B. Mol Ther Nucleic Acids. (2020) 20:649–60. doi: 10.1016/j.omtn.2020.04.008
141. Liu Y, Li YP, Xiao LM, Chen LK, Zheng SY, Zeng EM, et al. Extracellular vesicles derived from M2 microglia reduce ischemic brain injury through microRNA-135a-5p/TXNIP/NLRP3 axis. Lab Investig. (2021) 101:837–50. doi: 10.1038/s41374-021-00545-1
142. Zhang D, Cai G, Liu K, Zhuang Z, Jia K, Pei S, et al. Microglia exosomal miRNA-137 attenuates ischemic brain injury through targeting Notch1. Aging. (2021) 13:4079–95. doi: 10.18632/aging.202373
143. Guo Y, Peng Y, Zeng H, Chen G. Progress in mesenchymal stem cell therapy for ischemic stroke. Stem Cells Int. (2021) 2021:9923566. doi: 10.1155/2021/9923566
144. Xiang E, Han B, Zhang Q, Rao W, Wang Z, Chang C, et al. Human umbilical cord-derived mesenchymal stem cells prevent the progression of early diabetic nephropathy through inhibiting inflammation and fibrosis. Stem Cell Res Ther. (2020) 11:336. doi: 10.1186/s13287-020-01852-y
145. Mohapatra S, Pioppini C, Ozpolat B, Calin GA. Non-coding RNAs regulation of macrophage polarization in cancer. Mol Cancer. (2021) 20:24. doi: 10.1186/s12943-021-01313-x
146. Panni S, Lovering RC, Porras P, Orchard S. Non-coding RNA regulatory networks. Biochim Biophys Acta Gene Regul Mech. (2020) 1863:194417. doi: 10.1016/j.bbagrm.2019.194417
147. Smolle Ma Prinz F, Calin Ga Pichler M. Current concepts of non-coding RNA regulation of immune checkpoints in cancer. Mol Aspects Med. (2019) 70:117–126. doi: 10.1016/j.mam.2019.09.007
148. Mercer TR, Dinger ME, Mattick JS. Long non-coding RNAs: insights into functions. Nat Rev Genet. (2009) 10:155–9. doi: 10.1038/nrg2521
149. Rinn JL, Chang HY. Genome regulation by long noncoding RNAs. Ann Rev Biochem. (2012) 81:145–66. doi: 10.1146/annurev-biochem-051410-092902
150. Chen LL. Linking long noncoding RNA localization and function. Trends Biochem Sci. (2016) 41:761–72. doi: 10.1016/j.tibs.2016.07.003
151. Wu P, Mo Y, Peng M, Tang T, Zhong Y, Deng X, et al. Emerging role of tumor-related functional peptides encoded by lncRNA and circRNA. Mol Cancer. (2020) 19:22. doi: 10.1186/s12943-020-1147-3
152. Ashwal-Fluss R, Meyer M, Pamudurti NR, Ivanov A, Bartok O, Hanan M, et al. circRNA biogenesis competes with pre-mRNA splicing. Mol Cell. (2014) 56:55–66. doi: 10.1016/j.molcel.2014.08.019
153. Meng S, Zhou H, Feng Z, Xu Z, Tang Y, Li P, et al. CircRNA: functions and properties of a novel potential biomarker for cancer. Mol Cancer. (2017) 16:94. doi: 10.1186/s12943-017-0663-2
154. Yang L, Han B, Zhang Z, Wang S, Bai Y, Zhang Y, et al. Extracellular vesicle-mediated delivery of circular RNA SCMH1 promotes functional recovery in rodent and nonhuman primate ischemic stroke models. Circulation. (2020) 142:556–74. doi: 10.1161/CIRCULATIONAHA.120.045765
155. Law ZK, Tan HJ, Chin SP, Wong CY, Yahya WN, Muda AS, et al. The effects of intravenous infusion of autologous mesenchymal stromal cells in patients with subacute middle cerebral artery infarct: a phase 2 randomized controlled trial on safety, tolerability and efficacy. Cytotherapy. (2021) 23:833–40. doi: 10.1016/j.jcyt.2021.03.005
156. Chung JW, Chang WH, Bang OY, Moon GJ, Kim SJ, Kim SK, et al. Efficacy and safety of intravenous mesenchymal stem cells for ischemic stroke. Neurology. (2021) 96:e1012–23. doi: 10.1212/WNL.0000000000011440
157. Ottoboni L, Von Wunster B, Martino G. Therapeutic plasticity of neural stem cells. Front Neurol. (2020) 11:148. doi: 10.3389/fneur.2020.00148
158. El Bassit G, Patel RS, Carter G, Shibu V, Patel AA, Song S, et al. MALAT1 in human adipose stem cells modulates survival and alternative splicing of PKCδII in HT22 cells. Endocrinology. (2017) 158:183–95. doi: 10.1210/en.2016-1819
159. Huang B, Jiang XC, Zhang TY, Hu YL, Tabata Y, Chen Z, et al. Peptide modified mesenchymal stem cells as targeting delivery system transfected with miR-133b for the treatment of cerebral ischemia. Int J Pharm. (2017) 531:90–100. doi: 10.1016/j.ijpharm.2017.08.073
160. Wagenaar N, De Theije CGM, De Vries LS, Groenendaal F, Benders Mjnl Nijboer CHA. Promoting neuroregeneration after perinatal arterial ischemic stroke: neurotrophic factors and mesenchymal stem cells. Pediatr Res. (2018) 83:372–84. doi: 10.1038/pr.2017.243
161. Tanaka E, Ogawa Y, Mukai T, Sato Y, Hamazaki T, Nagamura-Inoue T, et al. Dose-dependent effect of intravenous administration of human umbilical cord-derived mesenchymal stem cells in neonatal stroke mice. Front Neurol. (2018) 9:133. doi: 10.3389/fneur.2018.00133
162. Zhang Y, Dong N, Hong H, Qi J, Zhang S, Wang J. Mesenchymal stem cells: therapeutic mechanisms for stroke. Int J Mol Sci. (2022) 23:2550. doi: 10.3390/ijms23052550
163. Binder DK, Scharfman HE. Brain-derived neurotrophic factor. Growth Fact. (2004) 22:123–31. doi: 10.1080/08977190410001723308
164. Li L, Wu W, Lin LF, Lei M, Oppenheim RW, Houenou LJ. Rescue of adult mouse motoneurons from injury-induced cell death by glial cell line-derived neurotrophic factor. Proc Natl Acad Sci USA. (1995) 92:9771–5. doi: 10.1073/pnas.92.21.9771
165. Zhou L, Zhu H, Bai X, Huang J, Chen Y, Wen J, et al. Potential mechanisms and therapeutic targets of mesenchymal stem cell transplantation for ischemic stroke. Stem Cell Res Ther. (2022) 13:195. doi: 10.1186/s13287-022-02876-2
166. Bhatia V, Gupta V, Khurana D, Sharma Rr Khandelwal N. Randomized assessment of the safety and efficacy of intra-arterial infusion of autologous stem cells in subacute ischemic stroke. Am J Neuroradiol. (2018) 39:899–904. doi: 10.3174/ajnr.A5586
167. Chen DC, Lin SZ, Fan JR, Lin CH, Lee W, Lin CC, et al. Intracerebral implantation of autologous peripheral blood stem cells in stroke patients: a randomized phase II study. Cell Transpl. (2014) 23:1599–612. doi: 10.3727/096368914X678562
168. Fang J, Guo Y, Tan S, Li Z, Xie H, Chen P, et al. Autologous endothelial progenitor cells transplantation for acute ischemic stroke: a 4-year follow-up study. Stem Cells Transl Med. (2019) 8:14–21. doi: 10.1002/sctm.18-0012
169. Hess DC, Wechsler LR, Clark WM, Savitz SI, Ford GA, Chiu D, et al. Safety and efficacy of multipotent adult progenitor cells in acute ischaemic stroke (MASTERS): a randomised, double-blind, placebo-controlled, phase 2 trial. Lancet Neurol. (2017) 16:360–8. doi: 10.1016/S1474-4422(17)30046-7
170. Jin Y, Ying L, Yu G, Nan G. Analysis of the long-term effect of bone marrow mononuclear cell transplantation for the treatment of cerebral infarction. Int J Clin Exp Med. (2017) 10:3059–68.
171. Lee JS, Hong JM, Moon GJ, Lee PH, Ahn YH, et al. A long-term follow-up study of intravenous autologous mesenchymal stem cell transplantation in patients with ischemic stroke. Stem Cells. (2010) 28:1099–106. doi: 10.1002/stem.430
172. Prasad K, Sharma A, Garg A, Mohanty S, Bhatnagar S, Johri S, et al. Intravenous autologous bone marrow mononuclear stem cell therapy for ischemic stroke: a multicentric, randomized trial. Stroke. (2014) 45:3618–624. doi: 10.1161/STROKEAHA.114.007028
173. Savitz SI, Yavagal D, Rappard G, Likosky W, Rutledge N, Graffagnino C, et al. A phase 2 randomized, sham-controlled trial of internal carotid artery infusion of autologous bone marrow–derived ALD-401 cells in patients with recent stable ischemic stroke (RECOVER-Stroke). Circulation. (2019) 139:192–205. doi: 10.1161/CIRCULATIONAHA.117.030659
174. Bhasin A, Srivastava MV, Bhatia R, Mohanty S, Kumaran SS, Bose S. Autologous intravenous mononuclear stem cell therapy in chronic ischemic stroke. J Stem Cells Regen Med. (2012) 8:181. doi: 10.46582/jsrm.0803011
175. Bhasin Ashu, Srivastava Mv Padma, Mohanty Sujata, Bhatia Rohit, Kumaran Senthil S, Bose Sushmita. Stem cell therapy: a clinical trial of stroke. Clin Neurol Neurosurg. (2013) 115:1003–8. doi: 10.1016/j.clineuro.2012.10.015
176. Bhasin A, Srivastava MP, Mohanty S, Bhatia R, Kumaran SS, Bose S. Paracrine mechanisms of intravenous bone marrow-derived mononuclear stem cells in chronic ischemic stroke. Cerebrovasc Dis Extra. (2016) 6:107–19. doi: 10.1159/000446404
177. Bhasin A, Kumaran SS, Bhatia R, Mohanty S, Srivastava MP. Safety and feasibility of autologous mesenchymal stem cell transplantation in chronic stroke in Indian patients. A four-year follow up. J Stem Cells Regen Med. (2017) 13:14. doi: 10.46582/jsrm.1301003
178. Ghali AA, Yousef MK, Ragab OA, ElZamarany EA. Intra-arterial infusion of autologous bone marrow mononuclear stem cells in subacute ischemic stroke patients. Front Neurol. (2016) 7:228. doi: 10.3389/fneur.2016.00228
179. Meng XG, Zhu SW, Gao H, Li YZ, Shi Q, Hou HS, et al. Treatment of cerebral infarction using autologous marrow mesenchymal stem cells transplantation: a six-month follow-up. J Clin Rehabil Tissue Eng Res. (2009) 13:6374–8. doi: 10.3969/j.issn.1673-8225.2009.32.036
180. Moniche F, Gonzalez A, Gonzalez-Marcos JR, Carmona M, Pinero P, Espigado I, et al. Intra-arterial bone marrow mononuclear cells in ischemic stroke: a pilot clinical trial. Stroke. (2012) 43:2242–4. doi: 10.1161/STROKEAHA.112.659409
181. Bang OY, Lee JS, Lee PH, Lee G. Autologous mesenchymal stem cell transplantation in stroke patients. Ann Neurol. (2005) 57:874–82. doi: 10.1002/ana.20501
182. Zhao T, Zhu T, Xie L, Li Y, Xie R, Xu F, et al. Neural stem cells therapy for ischemic stroke: progress and challenges. Transl Stroke Res. (2022) 13:665–75. doi: 10.1007/s12975-022-00984-y
Keywords: ischemic stroke, mesenchymal stem cells, immunomodulation, preclinical study, clinical trials
Citation: Tan N, Xin W, Huang M and Mao Y (2022) Mesenchymal stem cell therapy for ischemic stroke: Novel insight into the crosstalk with immune cells. Front. Neurol. 13:1048113. doi: 10.3389/fneur.2022.1048113
Received: 19 September 2022; Accepted: 17 October 2022;
Published: 08 November 2022.
Edited by:
Yanlin Zhang, Second Affiliated Hospital of Soochow University, ChinaReviewed by:
Chengyan Chu, Dalian Municipal Central Hospital, ChinaYimei Hong, Guangdong Provincial People's Hospital, China
Copyright © 2022 Tan, Xin, Huang and Mao. This is an open-access article distributed under the terms of the Creative Commons Attribution License (CC BY). The use, distribution or reproduction in other forums is permitted, provided the original author(s) and the copyright owner(s) are credited and that the original publication in this journal is cited, in accordance with accepted academic practice. No use, distribution or reproduction is permitted which does not comply with these terms.
*Correspondence: Min Huang, aG13YWxrbWFuJiN4MDAwNDA7MTYzLmNvbQ==; Yuling Mao, bWFvdnVsaW5nMDEyMSYjeDAwMDQwOzEyNi5jb20=