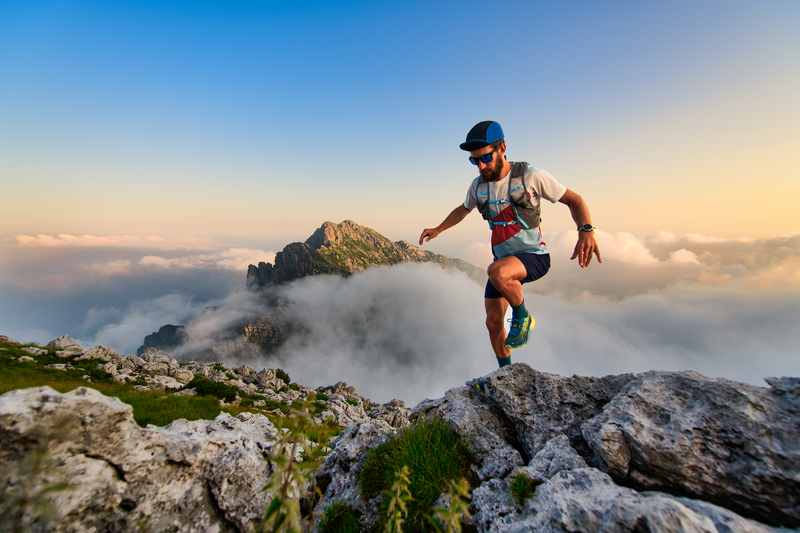
95% of researchers rate our articles as excellent or good
Learn more about the work of our research integrity team to safeguard the quality of each article we publish.
Find out more
REVIEW article
Front. Neurol. , 16 November 2021
Sec. Experimental Therapeutics
Volume 12 - 2021 | https://doi.org/10.3389/fneur.2021.768267
This article is part of the Research Topic Tissue Engineering Strategies for Nervous System Diseases: Mechanism, Diagnosis, and Treatment View all 4 articles
A peripheral nerve injury (PNI) has severe and profound effects on the life of a patient. The therapeutic approach remains one of the most challenging clinical problems. In recent years, many constructive nerve regeneration schemes are proposed at home and abroad. Nerve tissue engineering plays an important role. It develops an ideal nerve substitute called artificial nerve. Given the complexity of nerve regeneration, this review summarizes the pathophysiology and tissue-engineered repairing strategies of the PNI. Moreover, we discussed the scaffolds and seed cells for neural tissue engineering. Furthermore, we have emphasized the role of 3D printing in tissue engineering.
A peripheral nerve injury (PNI) is a medical problem mainly caused by external trauma after stretching, tearing, or extrusion of peripheral nerves. (1) It has attracted social attention because of its enormous social and economic pressure. In the United States, the economic loss caused by nerve injury exceeds $150 billion annually, and the treatment cost exceeds billions of dollars every year. People often underestimate the incidence of PNI, which seriously affects the quality of life of the patients (1–3).
The peripheral nervous system can self-regenerate and repair itself after injury. However, the repair is often slow and incomplete because axon extension depends on the synthesis and transportation of the intracellular substances, and the regeneration speed is similar to that of axon transportation, about 1–3 mm/day (4). In general, the stretch or crush injury results are better than those of transection. The recovery of the distal injury is better than that of the proximal injury, because axon growth only needs a short distance to reach the distal stump.
In the previous studies, substantial functional recovery of mild and moderate nerve injury can be achieved by surgical operation (such as, nerve suture and nerve transplantation) or non-surgical operation (such as, magnetic field, electric field, He-Ne Laser, and traditional Chinese Medicine) (3). The nerve suture and nerve grafts are extensively used for surgical nerve repair in the experiment and the clinic. An end-to-end nerve suture requires that the nerve stumps are aligned to achieve the best repair effect, and only the short nerve gap (<5 mm) can be used (5). When the damage gap is more significant (more than 5 mm), it is not possibly repaired by the meticulous microscopic surgery, and nerve autograft is regarded as the gold standard (3, 5). However, this damages the healthy nerves, and the number of donors is limited (6).
With the rapid development of cell biology and materials science, a new discipline, tissue engineering, is established to construct a different method of peripheral nerve repair. The core of nerve tissue engineering is to build a three-dimensional complex composed of cells and biomaterials and make the nerve guiding catheters (NGCs). The catheters are active scaffolds that can effectively guide axon regeneration. They contain the essential cells and neurotrophic factors that support axon regeneration. Implantation of the NGCs in the injured site can simulate the neural structure after Wallerian degeneration and avoid immune reactions. Even in some injury models, the clinical effect is similar to that of autologous nerve transplantation. The ideal NGCs should have excellent mechanical strength, good biocompatibility, biodegradability, and permeability (7).
In recent years, the research on neural tissue engineering has become more in-depth, and various NGCs have emerged one after another. To find a more suitable clinical treatment, the researchers need to pay attention to the regeneration effect of axons and focus on the transformation of basic research to clinical. Here, we aimed to review the pathophysiology and repairing strategies for PNI, focusing on the latest advances in nerve tissue engineering.
The periphery nerve system consists of all the nerves from the three main categories: the spine nerve, cranial nerve, visceral nerve, and their associated ganglia (8). The first two types of nerve fibers with myelin sheath are Schwann cells (SCs) wrapped in several layers, while the third nerve fibers are generally non-myelinated. The nerve bundles include the nerve fibers and connective tissue, which constitute the nerves as mentioned above. A connective tissue can be divided into three layers: epineurium, perineurium, and endoneurium. It contains the blood vessels in the epineurium, which provide the nerve fibers with trophic support. In the endoneurium, there are different types of cells except for connective tissue and nerve fibers (9, 10). For example, SCs produce neurotrophins and extracellular matrix (ECM), thus providing the nerve-growth microenvironment. In addition, it plays a crucial role in nerve regeneration. The macrophages are the essential cells in the inflammatory response, generally divided into two phenotypes and play different roles in nerve degeneration and regeneration (11).
Many factors contributed to the PNI (12). The basic injury types seen in the clinical practice are three main kinds (Table 1). The most common type is stretch-related injury. As we all know, after an external force pulls the spring, the spring will stretch in the direction of the power, and when the intensity exceeds a specific limit, plastic deformation will occur. Similarly, an injury occurs when traction forces exceed the stretch limit strength of the nerves, whose properties are similar to the properties of spring (15). For example, excessive traction caused by an overextension of the neck at birth leads to a particular type of birth paralysis limited to the fifth and sixth cervical nerves, which is also called Erb's palsy (13).
The laceration is another essential type of PNI, accounting for about 30% of the serious injuries (12). In this case, these can be complete transection of the nerve, and hence the process of nerve degeneration and regeneration can be observed clearly. Compression is the third injury type. There are two mechanisms of the damaged nerve, ischemia and extrusion.
In the clinical diagnosis and treatment of PNI, the first step is to clarify the degree of injury. The most classic injury classifications were proposed by Seddon and Sunderland and are still in use today (Figure 1) (15–17).
Figure 1. Graph illustrating the Sunderland and Seddon peripheral nerve injury (PNI) grading systems. The gradations in both the systems are associated with the injury's anatomical extent and the chance of a spontaneous (12).
The nerve injuries are divided into three categories by Seddon (16). In neurapraxia, the nerve fiber is slightly squeezed, but the axon is not broken, and there is no morphological change, only temporary functional changes. In axonotmesis, the nerve fibers are severely crushed, the axons are broken, and their functions temporarily disappear, accompanied by muscle atrophy, but the myelin and SCs still exist. In neurotmesis, the nerve bundle is broken due to severe trauma, which is difficult to recover. Sometimes it can be recovered from surgery, such as severe laceration. On this basis, Sunderland subdivided the nerve injury classification into five levels (15). The first-degree injury corresponds to Seddon's classification of neuropraxia. From first degree to fifth degree, the severity of the injury increases. The second, third, and fourth-degree injuries correspond to the endoneurium, perineurium, and epineurium lesions. The fifth degree of injury corresponds with the neurotmesis of Seddon, which is irrecoverable damage (3, 12).
The normal surrounding myelinated nerve is wrapped by a Schwann cell membrane and forms an onion skin-like myelin sheath. This remarkable structure ensures the transmission of specific electrical signals and maintains the normal neurophysiological functions. When an external force acts to stretch, compress, or tear the peripheral nerve, it will cause the distal nerve stump to degenerate, called Wallerian degeneration. At the site of nerve injury, the axon is broken and disintegrated by an external force, forming an inflammatory environment. The myelin sheath is also broken down and lost, and the macrophages are recruited to the injury site to swallow most myelin debris and lipid droplets.
Schwann cells are the most important cells for peripheral nerve regeneration. Due to the transection of the nerve fibers, they lose physical contact with the neuronal cells. Under the combined action of various cytokines (such as transcription factor c-Jun, and histone deacetylase 1 and 2), they reversely differentiate into repairing the phenotype cells. From the proximal stump to the distal stump, the Büngner band is formed, which plays a vital role in the peripheral nerve regeneration (18, 19). Furthermore, every Schwann cell has a set of genes. These genes can encode proteins or biologically active substances that promote nerve regeneration and prevent cell apoptosis, such as laminin, type V collagen, and nerve growth factor (NGF). Nerve regeneration is precise. Generally, the speed of nerve regeneration is 3–4 mm/day after compression and 2–3 mm/day after laceration. The regenerated nerve fibers can dominate the muscle preferentially (20).
With the development of medical technology and equipment, the treatment of peripheral nerve injury has made significant progress. Various strategies for peripheral nerve repair are summarized here. Briefly, there are two kinds of common repair strategies: surgical repair (such as neurorrhaphy, nerve transfer, nerve grafts, and tubes) and non-surgical repair (such as magnetic and electric field, He–Ne laser, electro photoluminescence, and traditional Chinese medicine) (Table 2) (3, 22).
A nerve suture is a standard method for the surgical treatment of nerve injury. The specific process is to remove the necrotic tissue and then suture the nerve end to end. The repair effect depends on the perfection of the repair technology and the distance from the injury site to the target tissue. If the space is too long, even if the nerve stumps are aligned, the time for axons to regenerate and connect to the corresponding part exceeds a time window, which will impair the effect of peripheral nerve repair (3). Nerve suture relies on the axon to produce collateral sprouting. During the operation, part of the axon of the nerve of the donor was artificially destroyed, causing its terminal to germinate, refilling the gap between the nerve stump (29).
A nerve transplantation is another surgical method, although autologous nerve transplantation is the gold standard for treating the long gap nerve injury. In large peripheral nerve lesions, a vascularized nerve transplant can provide blood to the nerve stump, prevent hypoxia, and provide nutrients (3). However, the neuronal process requires a complete nerve donor typically taken from the autologous sensory nerve, which will damage the original healthy tissue (21). With the development of decellularization technology, the allogeneic nerve transplantation can replace the autologous nerve transplantation, which reduces the damage to the autologous nerve and weakens the immune response to achieve the purpose of repair. Zhang et al. completely demyelinated and cellularized the heterogeneous nerves, leaving natural NGF and ECM, integrated them with the autologous nerve-differentiated adipose-derived mesenchymal stem cells (MSCs) to form a nerve conduit. The conduit was implanted in the rats to repair 10 mm sciatic nerve defects (30).
When there is no graft, another intervention may be used, i.e., nerve transfer. The proximal nerve strain is directly connected to the muscled abdomen (22). It can effectively restore the early damage caused by the loss of function, but it requires strict monitoring of the physiological state of the donor nerve by electromyography. The principle is that the healthy nerve bundles will be redirected to the distal part of the injured nerve (near the neuromuscular junction) to replace the role of the original nerve to achieve functional motor recovery. For the PNIs that cannot be regenerated entirely within a time window, nerve transfer helps protect and preserve the distal motor endplates until the natural axons are wholly regenerated (30). Different from the first three methods, the principle of “tubes” repair of nerve injury is to simulate the physiological structure of the healthy nerve, provide physical protection for peripheral nerve regeneration, and stimulate endogenous nerve regeneration potential. The preceding “tubes” were often natural products, such as muscle bundles, amniotic tubes, and venous tubes. The therapeutic effect is unsatisfying.
Over the past few years, the nerve-guided catheters are developed consisting of scaffolding and cells, which have opened a new world for peripheral nerve repair strategy. Of course, the role of non-surgical treatment in the repair of the peripheral nerves is not negligible. In a randomized controlled trial of the patients with incomplete long-term PNI, 780 nm laser irradiation treatment found that a specific wavelength of light can gradually improve the peripheral nerve function, leading to significant functional recovery (25). In addition, electro-photoluminescence has the potential to affect the peripheral nerve regeneration. A study found that after electroacupuncture stimulation of the injured site, mir-1b in the injured local nerve was regulated downward. In vitro, it was confirmed that the overexpression of mir-1b could inhibit the expression of brain-derived neurotrophic factor (BDNF) in the rat Schwann cell line. The research may indicate that the electroacupuncture might promote Schwann cell proliferation and recovery of sciatic nerve function by downregulating mir-1b (26).
A traditional Chinese medicine can also reduce neuronal mortality and promote the peripheral nerve regeneration. The treatment methods typically include internal and external application, acupuncture, massage, and bloodletting. The studies have demonstrated that the active principles of the traditional Chinese medicine have effects on anti-apoptosis and neurogenesis (27). Taking the example of Buyang Huanwu decoction, it contains four active ingredients, namely, alkaloid, glycoside, polysaccharide, and aglycone. It was found that they may reduce the production of inflammatory cytokines and reduce the neuronal apoptosis by inhibiting the expression of caspase-1 and caspase-3 (30). As well, Zhong et al. used a preparation of Astragalus (refined from the active ingredients of Astragalus extract) as an inducer and found that it may regulate the differentiation of MSCs into the neuron-like cells through the Wnt signaling pathways. This discovery provides an idea for using the preparation of Astragalus in the treatment of neurodegenerative diseases (28). Li et al. studied that Astragalus polysaccharides can protect mitochondria and anti-aging by inhibiting mitochondrial damage and recovery reactive oxygen species (ROS), meaning that they can be used to repair peripheral nerve damage (31).
After nerve suture, it took a long time to appreciate the importance of the nerve injury repair strategies and mechanisms. Until medical research entered the microcosmic domain, the neural microenvironment, the assessment of nerve injury, and the cellular and molecular mechanisms involved, and the clinical therapeutic principle for peripheral nerve injury were gradually understood. The methods of nerve tissue engineering for peripheral nerve injuries were also developed.
The core of nerve tissue engineering is to establish the composition of a three-dimensional complex with the cells and biological materials, and the key is manufactured scaffolds, selecting the seeding cells, and adding the neurotrophic factors (32). Compared with other treatment methods, it has taken another route, which develops nerve guidance conduits (NGCs) together with the nerve regeneration cells and small biological molecules to replace the traditional nerve transplantation. The applications of NGCs effectively avoid an immune response, and even in some of the injury scales, the clinical treatment effect is equivalent to the nerve autografts (33, 34).
Nerve repairing with the catheter is a part of the repair of PNI by tissue engineering, while recovering the function of the target organ is another part, especially rehabilitation treatment of muscle atrophy (35). That is because the nerve injury causes double damage. After PNI, muscle denervation causes atrophy and loss of original function.
The strategy of nerve tissue engineering to repair the PNI combines the advantages of the former two methods, changes the original repair ideas, and transforms the so-called “replace the damaged nerve” repair approach into “guide and promote the axon regeneration of the damaged part.” The purpose of nerve tissue engineering is to establish NGCs, which can accurately guide the reconnection of nerve stumps. In some long nerve gaps, it shows nerve recovery ability comparable with the autologous nerve transplantation (32, 35, 36). In the following chapters, the composition, development, and recent research results of NGCs will be described (Table 3). It can be seen from the table that excellent NGCs generally meet the following characteristics: absorbable/degradable, compound SCs/cytokine/cell affinity, topographic clues inside the scaffold conform to cell cord growth, and good elasticity/ductility. These NGCs can adapt to the repair of peripheral nerves with longer gaps (15 mm gap) and effectively promote nerve regeneration. Among them, the NGCs of the composite biomaterials have superior biocompatibility, biodegradability, excellent mechanical properties, good mechanical properties, and cell affinity. They are superior to the natural polymers and non-biosynthetic polymers in repairing the nerves (39, 44).
Scaffold fabrication is the cornerstone of NGCs. An ideal scaffold should be passive physical support connecting the proximal and distal stump and positively meeting the needs of multiple nerve regeneration. The appropriate scaffolds should provide a place for the growth factors to gather and SCs to grow, protect, and guide the proximal stump to extend to the distal stump, like a bridge to connect the distal nerve (45). At the same time, it can isolate the newborn axons from the external environment, avoid many inflammatory cells infiltrating into the injured area, help accumulate high concentration neurotrophic factors in it, and reduce scar formation and neuroma (46). In addition, the effects of topographic cues, electrical conductivity, and biodegradability on the nerve regeneration cannot be ignored (47). Although there is no ideal technology reported so far, there are many research reports on the design, materials, and fabrication methods of scaffolds (33, 36, 38).
In general, the scaffold should maintain a tubular or linear structure to adapt to the natural bundle structure of axons in biological design. Its primary characteristics include good mechanical properties, well-biocompatibility, biodegradability, and enough permeability (7). The collagen fiber, fibrin, poly (lactic-co-glycolic-acid) (PLGA), polylactic acid or polylactide (PLA), poly (propylene fumarate) (PPF), and agarose can be used as the scaffold materials, and there are pros and cons associated with each process (34).
In 1982, it was reported that the non-degradable silicone tube was used to wrap the severed sciatic nerve of the rats to repair the 6 mm gap in vivo system (37). The semipermeable collagen catheter could also repair the PNI in vitro (48). However, these scaffolds are unstable and inactive in vivo, so they are gradually eliminated. With the progress of materials science, more and more high-quality polymer materials are studied. The new scaffolds can simulate the physicochemical and mechanical properties of the biological tissues, have muscular mechanical strength, and their degradation pattern is based on simple hydrolysis (49).
There was a composite hydrogel catheter used for nerve regeneration, which was made from different volumes of polyacrylamide, graphene oxide, gelatin, and sodium alginate (PAM/GO/Gel/SA, and PGGS). The conduit had good elasticity and good mechanical properties. The catheter was found to have high mechanical stress, which adapted to the unexpected changes of nerve tissue during exercise. It promoted the regeneration of the sciatic nerve in the rats (36).
The agarose-based biomaterials have also achieved success in in vitro experiments. It is a polysaccharide extracted from agar found in red algae, which has biocompatibility and non-immunogenicity (50). The template agarose scaffolds made of the fiber bundles enhanced the structural stability and promoted the growth and extension of regenerated axon tissue in the gap of more than 10 mm (51). Chávez-Delgado et al. (52) used a chitosan prosthesis containing neurosteroids (progesterone [PROG] and pregnenolone [PREG]) to bridge the 10 mm gap in the facial nerve of a rabbit. The chitosan scaffold has good biocompatibility and slow degradation rate, and can be used as a guide channel for the axon growth. In addition, the chitosan scaffold can act as an in-situ neurosteroid delivery device, a long-term release neurosteroid carrier. After 45 days, the regenerated tissue showed myelinated nerve fibers of different sizes and shapes. The statistical methods showed that there was a significant difference between the experimental group and the control group, and the PROG-loaded chitosan prosthesis produced the best-guided nerve regeneration and recovery. The chitosan scaffolds containing PROG are used to bridge the 10 mm gap in the facial nerves of a rabbit, which can promote the regeneration of the injured peripheral nerves, indicating that the function of damaged nerves may be improved by PROG and other neurotrophic substances (53). A chitosan nerve conduit impregnated with neurosteroid PROG was used to induce regeneration of the sciatic nerve in the adult female dogs repairing a 15 mm defect. The histological analysis and electron microscopy studies showed that the damaged distal nerve segment showed a structure similar to that of a normal nerve (54).
Of course, it is too broad to rely solely on the scaffolds to promote peripheral nerve regeneration. With the development of research on the mechanism of nerve injury and repair, the role of nerve scaffolds in the nerve regeneration has become more diverse. Zhang et al. found that the effect of peripheral nerve regeneration with multiple factors (cells, growth factors, and scaffolds) is higher than that of a single element and can be compared with the effect of autologous nerve transplantation. The benefit of multiple factors is higher than that of a single factor and can be compared with the impact of nerve autografts. The bone mesenchymal stem cells (BMSCs) were introduced into the highly oriented poly (L-lactic acid) (PLLA)/soy protein isolate (SPI) nanofiber nerve conduits as the seed cells to repair the 10-mm sciatic nerve defects in the rats, and showed promising results and superior to the autografts group in some aspects (39).
The fabrication of scaffolds is not only simple stacking or cutting. The most common manufacturing methods include solvent casting (with or without salt leaching), gas foaming (with or without the leaching), phase separation, freeze-drying, and electrospinning (55). In addition, the topographic cues and fillers inside the scaffolds also increase the difficulty of production. Therefore, given these requirements, the traditional process is modified, and some unique manufacturing methods are adopted, such as micro-patterning, injection molding, unidirectional freezing, and electrospinning (9).
The process of nerve regeneration could be promoted by the cells. This is a rather complex and highly coordinated cell–cell interaction process in which the cellular, molecular pathways are not yet clarified (56). Therefore, the effect of the single-cell pathway on the nerve regeneration process is uncertain and may even be harmful. However, from the perspective of cells, the problem will become relatively simple.
In many studies, the scaffolds are combined with the peripheral nerve regeneration cells or cells that produce certain NGFs, and the nerve repair effect is enhanced (Table 4) (38, 40, 41). Berrocal et al. (38) used autologous SCs (200,000 cells/μl) combined with an absorbable collagen catheter, which was applied to the sciatic nerve defect in the rats. The results showed that the combined catheter enhanced the bridge PNIs with the extended segmental defects. Gonzalez-Perez et al. (40) placed MSCs and SCs in the chitosan tubes filled with collagen gel, respectively, which were used to repair a critical size defect of 15 mm in the rat sciatic nerve. And it was found that the SC-aligned scaffolds had the best repair effect. This means that cell technology can be applied to nerve tissue engineering, especially in the long nerve gap, and has a good prospect.
Schwann cells are glial cells in the peripheral nervous system and the most used seed cells in nerve tissue engineering. It can be made and repaired together with the scaffolds or used to enhance nerve regeneration separately. It is divided into a myelinated cell and a non-myelinated cell according to its structure and function. The former surrounds thicker axons, while the latter surrounds some slender axons. They can support and nourish nerves, have the ability to repair peripheral nerve damage and promote peripheral nerve regeneration. After the PNI, they reversely differentiate into the progenitor-like cells through genetic reprogramming and further transform into a repair phenotype to participate in the peripheral nerve regeneration (18, 64). The complex process involves the downregulation of the myelinating genes and high expression of regeneration genes, such as the upregulation of transcription factors (e.g., c-Jun) and histone deacetylase 1 and 2 (HDAC1/2) are involved (18, 19).
Schwann cells have two main functions for peripheral nerve repair: creating an environment to support the nerve regeneration and guiding the direction of axon growth. After nerve fiber transection, on the one hand, myelin sheath and axon fragments need to be removed timely and effectively. Otherwise, the efficiency and accuracy of reinnervation will be reduced if the time is too long, which is not conducive to the tissue regeneration (65). In the distal stump, the SCs and macrophages can help axons produce a pro-migration environment by removing the axons and myelin fragments.
On the other hand, if nerve stumps atrophy or nerve defect, the gap will be filled with the inflammatory cells and mediators, peripheral nerve cells, fibroblasts, and ECM, which is called the “bridge” of a new composite tissue reconnection. SCs need to cross the new bridge to guide axon regeneration. It cannot migrate directly into the matrix and depend on the macrophages, which secrete vascular endothelial growth factor A (VEGF-A) and other cytokines. Angiogenesis, remyelination, and axon regeneration occur sequentially in the process of nerve regeneration. A VEGF-A is a critical biological factor in angiogenesis, promoting the development and formation of blood vessels and protecting the motor and sensory neurons (66). Neuromodulin 1, endothelin, and Notch signaling molecules are also involved in this process. Neuromodulin 1 regulates the SC migration and proliferation by binding to ErBb2/3 on SCs. Notch signaling accelerates the SC transformation (67). When the SCs form cord-like structures along the new blood vessels and pass through the new bridge, that can guide the axons to grow in the right direction and promote peripheral nerve regeneration (18, 68). In addition, the effects of cytokines secreted by the SCs on the peripheral nerve regeneration cannot be ignored, such as neurotrophic factors (e.g., GDNF and artemin), adhesion molecules (e.g., N-cadherin and N-CAM). The studies have shown that the SCs can synthesize some neurosteroids (such as, PROG and PREG). These synthetic products can interact with the intracellular receptors to activate the synthesis of some myelin proteins (P0 and PMP22) or act on the gene encoding transcription factors Krox-20 (Egr-2) to induce myelination (52).
Many studies have shown that there are many difficulties in using the NGCs to repair the peripheral nerve (3, 32, 57). For example, the effect of repair in vivo is poor, and some severe injuries cannot be fully repaired. This may be since nerve conduits can only provide physical support but cannot give endogenous or exogenous regenerative power. Therefore, simple nerve conduits are only suitable for supporting the growth of the nerve cells and transporting nutrients, and cell therapy is an essential basis for repairing the PNI. The first crucial step to introduce the SCs based therapy into the PNI is to add SCs to the guiding catheter. It is proved that the NGCs filled with SCs can repair the long segmental spaces faster and more effectively than the tubes alone (38). In addition, in some studies, SCs were programmed to overexpress neurogenic factors, such as VEGF-A, and then loaded onto the inner wall of hydroxyethyl cellulose/soy protein isolate/polyaniline sponge (HSPS) conduits and transplanted to the damaged site. Three months later, immunofluorescence co-staining showed that MBP + Schwann cells in the HSPS-SC (VEGF) group were far superior to the HSPS-SC group (MBP protein is a biomarker of Schwann cell myelin differentiation). The final results also showed that the NGCs have good functional and morphological repairs to peripheral nerve injuries (66, 69).
Because of the critical role of endogenous regeneration in the PNI, the SCs are often used as transplantable cells for nerve repair, which has been proved by in vivo and in vitro experiments. Moreover, due to the limited proliferation ability of SCs, their application is minimal. The self-renewing ability and pluripotency of stem cells are considered the ideal source of seed cells in nerve tissue engineering. Therefore, the scientists hope to repair nerve injury by replacing the cells (35).
Unlike SCs, the stem cells are abundant in variety and source. Nerve tissue engineering needs to be screened from many stem cells. The cells need to include the following characteristics: easy to obtain, rapid expansion in culture, survival in the damaged site, stable transfection and expression of foreign genes, and binding with NGCs (70). The embryonic stem cells (ESCs), neural stem cells (NSCs), induced pluripotent stem cells (IPSCs), mesenchymal stem cells (MSCs), adipose-derived stem cells (ASCs), neural crest stem cells, dental pulp stem cells, skin, and umbilical cord-derived those can be used to repair the PNI (35, 70).
The ESCs are highly undifferentiated cells with totipotency, differentiating into all the tissues and organs in the adult animals. The ESCs can be induced into a neural phenotype before transplantation and can differentiate into the SCs and play their physiological functions after transplantation, promoting angiogenesis, nerve growth, and myelination. Compared with the SCs, the proliferation of ESCs is more active. Moreover, the SCs differentiated by the ESCs can express relevant markers, glial fibrillary acidic protein, S100, and p75, and induce neuronal myelination (71). In the rat sciatic nerve transection model, using a peripheral nerve adventitia as a natural conduit, implantation of the neural progenitor cells derived from the ESCs can promote the repair of severely injured peripheral nerve (41).
The NSCs are the primitive cells in the nervous system and are also the essential cellular sources of the neurons and glial cells. They can be used as an essential cell source for the nerve regeneration. A study showed that after implantation of the NSCs, the abundance of IL12p80 will increase, which directly stimulates SC differentiation and promotes the peripheral nerve recovery (72). The NSCs combined with the NGCs can differentiate into the neurons and Schwann-like cells and secrete many important neurotrophic factors, such as brain-derived neurotrophic factor, fibroblast growth factor, NGF, insulin-like growth factor, and hepatocyte growth factor, after transplanting to the injured area, to promote nerve regeneration (58).
In addition, intravenous injection of the NSCs can cause physiological nerve repair, thus reducing the neuropathic pain symptoms (59). IPSC has always been a research hotspot, which refers to the recovery of terminally differentiated cells to totipotent state or the formation of ESC lines under the specific conditions. In relevant studies, the implantation of polycaprolactone (PCL) scaffolds loaded with active Schwann cells (ASCs) and IPSC -derived NSCs at the injured site can improve the motor recovery of the rats (60). Bone marrow MSCs are pluripotent adult stem cells obtained by bone marrow aspiration or artificial culture. The rat bone marrow MSCs are used as the supporting cells introduced into the silk fibroin (SF)-based scaffolds. After catheter transplantation, it was found that the gene expression of S100 and several growth factors (brain-derived neurotrophic factor, ciliary neurotrophic factor, and fibroblast growth factor) were upregulated, and many ECM components were secreted, such as collagen, fibronectin, and laminin promote the histological and functional recovery of the damaged sciatic nerve in the rats (73). The chitosan/ PLGA nerve scaffolds containing autologous MSCs have been used to repair the long nerve gaps in the large animals (such as bridging the 50-mm-long gap in the sciatic nerve of a dog) (74).
At present, the ASCs are the most valuable source of the transplanted cells (57). The ASCs can be separated from human subcutaneous fat by conventional liposuction under anesthesia and cannot be used directly to repair the nerve damage. They need to be further separated from the vascular matrix component. Otherwise, it will block the nerve conduit and hinder regeneration (59). They are easy to harvest and have strong differentiation potential. They can differentiate into various phenotypes along the mesoderm lineage, such as osteoblasts, chondrocytes, and muscle cells. The neural phenotypic differentiation of ASCs involves a combination of multiple growth factors. The ECM molecules affect the cell viability, adhesion, and neurotrophic behavior of differentiated ASCs, and coating them with the nerve ducts can increase the regeneration rate. Recent studies have shown that human platelet lysates have neurotrophic properties (such as the release of BDNF). The synergistic effect with laminin can enhance the neurotrophic effect of ASCs on the primary neurons in vitro (75). The acquisition site of ASCs (the best subcutaneous and perennial) will also affect the differentiation results (76). The neural phenotype ASCs have neurotrophic characteristics and express SC markers (such as glial fibrillary acidic protein (GFAP), S100, and the low-affinity receptor for NGF p. 75), which can differentiate into the SC -like cells and secrete a variety of neurotrophic factors. Several studies have shown that the ADSCs can improve the neuronal differentiation and neural repair (58, 59). Recent studies have found that the exogenous neurotrophic factors can be used to enhance the neurotrophic capacity of the human adipose derived stem cells in vitro (66).
In neural tissue engineering, the use of stem cells requires selecting the appropriate type, optimizing the number and methods of transplantation, and using the exogenous factors to ensure cell survival, reduce tumorigenicity, ensure safety, and maximize therapeutic efficacy (70). The human embryonic stem cells (HESCs) overexpressing the fibroblast growth factor 2 (FGF2) combined with heterogenous fibrin sealant scaffold can support the survival and regeneration of neurons in a mouse model of sciatic nerve injury (42). Beyond that, incorporating the ESCs, NSCs, bone marrow MSCs, adipose-derived cells, skin-derived precursor stem cells, and IPSCs enhances the therapeutic effect of tissue-engineered nerve graft (58).
The macrophages are mononuclear phagocytes, which show significant plasticity in vivo and in vitro. As the prominent role of inflammatory cells, there are apparent morphological and functional differences in the different micro-environments. At present, it can be divided into two phenotypes, M1 and M2 macrophages. The M1 macrophages are effectors and induce cells in inflammation and participate in a positive immune response by secreting inflammatory cytokines and chemokines, which activation is related to the lipopolysaccharide (LPS) and interferon-γ (IFN-γ) and so on. The M2 macrophages have a weak ability to antigen presentation, secrete anti-inflammatory cytokines, participate in anti-inflammatory response, and are activated in the specific micro-environments to play the role of immune regulation and participate in tissue repair.
After PNI, it will lead to a local inflammatory reaction, a large number of inflammatory factors are released. The macrophages are recruited by chemokines (CCL2) and gather in the area with other inflammatory cells, participate in Wallerian degeneration of the nerve cells, polarize into anti-inflammatory phenotype (M2) under the effect of IL-4, and participate in axonal regeneration. The results show that the SCs and fibroblasts control and modify the macrophage reactions. And the cytokines secreted by them release into the blood, chemotactic monocytes, and change their behavior (56). In addition, the macrophages can facilitate axon regeneration by removing the axon and myelin fragments in distal stumps (10).
The regulation of macrophage function is an effective strategy to improve the peripheral nerve regeneration and repair injury. Currently, it is also included in the nerve tissue engineering to repair the nerve injuries. Jason R Potas et al. used electrospinning combined IL-10 with the PCL nanofibrous scaffolds to successfully induce the macrophages to polarize to the M2 activated state in the scaffolds and adjacent tissues around the nerve, which confirmed that macrophage function could be regulated by manipulating the cell micro-environment, thus affecting the process of nerve regeneration (43). Recent studies have shown that the macrophages polarize into the M2 type in the micro-environment after peripheral nerve injury, which may involve the IL-10 and collagen VI upregulated. Therefore, IL-4 and other factors can be embedded in the nerve guide catheter to promote the transformation of the macrophage M2 phenotype (11). However, the factors that promote the M2 polarization of macrophages may have the property of promoting tumor growth. Therefore, the use of these factors needs fine control to produce positive therapeutic value for the PNI (43). For example, through cell genetic engineering, some cells overexpressing the above factors are loaded on the nerve scaffolds, which can effectively regulate their release. Or changing the physical or chemical properties of scaffolds, and using additive manufacturing technology to construct the biological components of scaffolds layer by layer, so that the factors can be degraded and released at an appropriate time to achieve the purpose of peripheral nerve repair (3).
A new technique, 3D printing, is also called additive manufacturing, which means adding materials to make things. 3D printing technology was used in the industrial production before, but now bioprinting is common, such as bioprinting of NGCs (77). Micropatterning, injection molding, and unidirectional freezing are the unique manufacturing methods of some NGCs (9). In recent years, 3D printing has become a matured technique, and its most commonly used technologies include extrusion (fuse manufacturing and direct ink writing), powder, and photoinduced polymerization (77). 3D bioprinting is used to make the biomaterials containing cells, that is, to build the complex 3D functional living tissues or artificial organs (49).
Among them, the droplet-based bioprinting (DBB) can print tissue or organs embedded in the cells based on or without a scaffold. It has been reported that in the rat model, the effect of a 3D printed human fibroblast catheter in repairing a 5 mm nerve gap is not significantly different from that in autologous nerve transplantation (33). Because of the personalized manufacturing characteristics of 3D printing, it is possible to customize the size, shape, and structure required. The scaffolds printed combine with biological factors (such as nanoparticles and cells) to achieve exact control (77). Therefore, the NGCs made of various biomaterials are produced through research. Recently, a catheter is developed, consisting of nanoparticles in hydrogel and hydrogel matrix. The hydrogel provided a physical microenvironment for axonal elongation, and the nanoparticles continuously released drugs to promote the nerve regeneration. The drug is an inhibitor of the Hippo pathway. The previous studies have found that the Hippo pathway affects the peripheral nerve regeneration (44). Therefore, Jie Tao et al. upregulated the downstream gene (yes-associated-protein) by targeting the inhibition of this pathway in the SCs to promote the regeneration of PNI. The experimental results showed that the functional nerve conduit could effectively induce the recovery of sciatic nerve injury (78).
The target organs of peripheral innervation include the sensory and motor organs (muscles). After PNI, the sensation will remain for several years, even for a long time to repair, and the function can be restored. In contrast, the denervated muscle progressively loses its ability to become reinnervated (35). In the process of muscle atrophy, the proteasome pathway and the autophagy-lysosomal pathway are activated, leading to different amount of loss of muscle mass and loss of its original function (79).
In neurogenic atrophy, it is proved that the regulation of myogenin is a regulator of muscle development and an inducer of neurogenic atrophy. After denervation, myogenin is upregulated, the expression of E3 ubiquitin ligases is decreased, and the muscle proteolysis and atrophy are promoted (80). A study reported that when the PNI was treated early, the atrophy of the distal muscle would stop, and the function would recover (35).
At present, in addition to exercise, there is no effective treatment for reducing the muscle atrophy. Jin Li et al. demonstrated for the first time that CRISPR-based genome editing can specifically target mir-29b in the mouse denervation model, thus preventing angiotensin-II (Ang-II) and myocyte apoptosis induced by Ang-II (81). Beyond that, intramuscular injection of various growth factors (such as IGF-1) and stem cells (such as muscle satellite cells) can also alleviate the muscle atrophy, but its mechanism is still unknown (35).
Additionally, laser phototherapy has potential therapeutic value. The 780 nm laser phototherapy could temporarily retain the function of denervating muscle and accelerate and enhance the axon growth and regeneration after the PNI or reconstruction surgery (25). However, tissue engineering may be a good choice when needed to reconstruct the muscle, and the regenerated axons cannot be accepted after extensive denervation.
The peripheral nerve regeneration strategy includes the treatment of muscle reinnervation. After the PNI, the innervated muscles will atrophy, and muscle regeneration depends on its endogenous regeneration ability (35). The satellite cells, a group of undifferentiated muscle cells located between the basal layer and the plasma membrane of the muscle fibers, are the main participants in muscle regeneration (79). But when the ability is exhausted, the muscle tissue engineering therapy is essential. However, up to now, muscle tissue engineering has not been able to restore all the functions of the replaced muscle (82). The reason might be that the mechanical stress stimulation of the human muscle bundle is challenging to simulate fully. Therefore, the bioreactor can be used to stimulate the cells to achieve muscle tissue reconstruction in vivo.
However, the above conditions need to be met in nerve tissue engineering because the loose cells cannot regenerate directly, and scaffold support is also required. Perez-Puyana et al. used electrospinning technology to add elastin to the PCL-based scaffolds for skeletal muscle. The scaffolds had more petite size and better hydrophilicity, and the biocompatibility was also improved (83).
This review describes the pathophysiology of PNI and its repair strategies. The repair strategies involve the intersection of biology, medicine, materials science, and engineering. Neural tissue engineering has proposed many new approaches to manufacture the highly adjustable and functional scaffolds, which have great potential for repairing the PNIs. However, so far, a large number of NGCs developed, whether in vivo or in vitro experiments, still have a particular gap with autologous nerve transplantation. In the follow-up research, the physical, chemical. and mechanical properties of scaffolds need to be adjusted to optimize the interaction between the cells in the scaffold and the implanted tissue to realize the large-scale promotion and application of NGCs. The biodegradation rate of the scaffold also needs to be controlled to maintain its integrity until the regenerated tissue matures. In addition, the production technology of NGCs is also a hot spot of current research. The 3D bioprinted NGCs support the migration and proliferation of the seed cells. 3D printing based on digital light processing (DLP) technology can develop a more precise internal structure of the scaffold and promote more efficient nerve regeneration.
Given the severity and complexity of the PNI, the current tissue engineering repair strategy still has some problems to be solved. For example, what is the optimal time window for nerve guide catheter implantation? Is it possible to use clinical interventional therapy to reduce the secondary damage to the injured site by implant surgery? In the above, the delivery of the nerve cells, NGF, and drugs can effectively promote axon regeneration, but the interaction of too many cytokines may cause adverse solid immune reactions. Therefore, the study of single-factor NGCs can become a future research hotspot. Vascularization is an essential factor in the survival and function of nerve grafts. The fascial flaps or vascularized nerve grafts have limited clinical applicability and therapeutic effects. The NGCs carrying angiogenic active substances (such as VEGF) is one of the focuses of current research and may become the development direction of NGCs in the future. In addition, a variety of nerve guiding catheters should be designed, such as multicellular components, pro-angiogenesis, and Büngner-like structures to improve the long-distance growth of axons. In the future, the functionalized NGCs produced by 4D printing will be one of the hot spots in the future research on the peripheral nerve regeneration.
YL: methodology, writing an original draft, and visualization. ZM: conceptualization, methodology, validation, investigation, writing-review and editing, and funding acquisition. YR and DL: writing an original draft and writing-review and editing. WL: writing original draft. TL: investigation and writing-review and editing. JW: conceptualization, validation, and investigation. HM: conceptualization, validation, investigation, writing-review and editing, and supervision. JZ: validation, investigation, supervision, project administration, and funding acquisition. All authors contributed to the article and approved the submitted version.
This work was financially supported by the Shanghai Leading Talents Program in 2020 (No. 110), the National Key R&D Program of China (2020YFC2008700/2018YFA0703000), and the Project of Shanghai Jiading National Health and Family Planning Commission (KYXM 2018-KY-03).
The authors declare that the research was conducted in the absence of any commercial or financial relationships that could be construed as a potential conflict of interest.
All claims expressed in this article are solely those of the authors and do not necessarily represent those of their affiliated organizations, or those of the publisher, the editors and the reviewers. Any product that may be evaluated in this article, or claim that may be made by its manufacturer, is not guaranteed or endorsed by the publisher.
1. Kouyoumdjian JA. Peripheral nerve injuries: a retrospective survey of 456 cases. Muscle Nerve. (2006) 34:785–8. doi: 10.1002/mus.20624
2. Tian L, Prabhakaran MP, Ramakrishna S. Strategies for regeneration of components of nervous system: scaffolds, cells and biomolecules. Regen Biomater. (2015) 2:31–45. doi: 10.1093/rb/rbu017
3. Raza C, Riaz HA, Anjum R, Shakeel NUA. Repair strategies for injured peripheral nerve: review. Life Sci. (2020) 243:117308. doi: 10.1016/j.lfs.2020.117308
4. Scheib J, Höke A. Advances in peripheral nerve regeneration. Nat Rev Neurol. (2013) 9:668–76. doi: 10.1038/nrneurol.2013.227
5. Daly W, Yao L, Zeugolis D, Windebank A, Pandit A. A biomaterials approach to peripheral nerve regeneration: bridging the peripheral nerve gap and enhancing functional recovery. J R Soc Interface. (2012) 9:202–21. doi: 10.1098/rsif.2011.0438
6. Meyer C, Stenberg L, Gonzalez-Perez F, Wrobel S, Ronchi G, Udina E, et al. Chitosan-film enhanced chitosan nerve guides for long-distance regeneration of peripheral nerves. Biomaterials. (2016) 76:33–51. doi: 10.1016/j.biomaterials.2015.10.040
7. Amani H, Kazerooni H, Hassanpoor H, Akbarzadeh A, Pazoki-Toroudi H. Tailoring synthetic polymeric biomaterials towards nerve tissue engineering: a review. Artif Cells Nanomed Biotechnol. (2019) 47:3524–39. doi: 10.1080/21691401.2019.1639723
8. Schmidt CE, Leach JB. Neural tissue engineering: strategies for repair and regeneration. Annu Rev Biomed Eng. (2003) 5:293–347. doi: 10.1146/annurev.bioeng.5.011303.120731
9. Vijayavenkataraman S. Nerve guide conduits for peripheral nerve injury repair: a review on design, materials and fabrication methods. Acta Biomater. (2020) 106:54–69. doi: 10.1016/j.actbio.2020.02.003
10. Hall S. The response to injury in the peripheral nervous system. J Bone Joint Surg Br. (2005) 87:1309–19. doi: 10.1302/0301-620X.87B10.16700
11. Chen P, Piao X, Bonaldo P. Role of macrophages in wallerian degeneration and axonal regeneration after peripheral nerve injury. Acta Neuropathol. (2015) 130:605–18. doi: 10.1007/s00401-015-1482-4
12. Burnett MG, Zager EL. Pathophysiology of peripheral nerve injury: a brief review. Neurosurg Focus. (2004) 16:E1. doi: 10.3171/foc.2004.16.5.2
14. Doughty CT, Bowley MP. Entrapment neuropathies of the upper extremity. Med Clin North Am. (2019) 103:357–70. doi: 10.1016/j.mcna.2018.10.012
15. Sunderland S. The anatomy and physiology of nerve injury. Muscle Nerve. (1990) 13:771–84. doi: 10.1002/mus.880130903
16. Seddon HJ. A classification of nerve injuries. Br Med J. (1942) 2:237–9. doi: 10.1136/bmj.2.4260.237
17. Kamble N, Shukla D, Bhat D. Peripheral nerve injuries: electrophysiology for the neurosurgeon. Neurol India. (2019) 67:1419–22. doi: 10.4103/0028-3886.273626
18. Nocera G, Jacob C. Mechanisms of schwann cell plasticity involved in peripheral nerve repair after injury. Cell Mol Life Sci. (2020) 77:3977–89. doi: 10.1007/s00018-020-03516-9
19. Gomez-Sanchez JA, Pilch KS, van der Lans M, Fazal SV, Benito C, Wagstaff LJ, et al. After nerve injury, lineage tracing shows that myelin and remak schwann cells elongate extensively and branch to form repair schwann cells, which shorten radically on remyelination. J Neurosci. (2017) 37:9086–99. doi: 10.1523/JNEUROSCI.1453-17.2017
20. Stoll G, Muller HW. Nerve injury, axonal degeneration and neural regeneration: basic insights. Brain Pathol. (1999) 9:313–25. doi: 10.1111/j.1750-3639.1999.tb00229.x
21. M FG, M M, S H, Khan WS. Peripheral nerve injury: principles for repair and regeneration. Open Orthop J. (2014) 8:199–203. doi: 10.2174/1874325001408010199
22. Kehoe S, Zhang XF, Boyd D. FDA Approved guidance conduits and wraps for peripheral nerve injury: a review of materials and efficacy. Injury. (2012) 43:553–72. doi: 10.1016/j.injury.2010.12.030
23. Belanger K, Dinis TM, Taourirt S, Vidal G, Kaplan DL, Egles C. Recent strategies in tissue engineering for guided peripheral nerve regeneration. Macromol Biosci. (2016) 16:472–81. doi: 10.1002/mabi.201500367
24. Page MJ, Green S, Mrocki MA, Surace SJ, Deitch J, McBain B, et al. Electrotherapy modalities for rotator cuff disease. Cochrane Database Syst Rev. (2016) 16:CD012225. doi: 10.1002/14651858.CD012225
25. Rochkind S, Geuna S, Shainberg A. Chapter 25: phototherapy in peripheral nerve injury: effects on muscle preservation and nerve regeneration. Int Rev Neurobiol. (2009) 87:445–64. doi: 10.1016/S0074-7742(09)87025-6
26. Liu Y-P, Luo Z-R, Wang C, Cai H, Zhao T-T, Li H, et al. Electroacupuncture promoted nerve repair after peripheral nerve injury by regulating mir-1b and its target brain-derived neurotrophic factor. Front Neurosci. (2020) 14:525144. doi: 10.3389/fnins.2020.525144
27. Sun K, Fan J, Han J. Ameliorating effects of traditional chinese medicine preparation, chinese materia medica and active compounds on ischemia/reperfusion-induced cerebral microcirculatory disturbances and neuron damage. Acta Pharm Sin B. (2015) 5:2. doi: 10.1016/j.apsb.2014.11.002
28. Zhong J, Cao H, Chen Z, Zhou F, Tan X. Wnt signaling pathways participate in astragalus injection-induced differentiation of bone marrow mesenchymal stem cells. Neurosci Lett. (2013) 553:29–34. doi: 10.1016/j.neulet.2013.08.007
29. Geuna S, Papalia I, Tos P. End-to-Side (Terminolateral) nerve regeneration: a challenge for neuroscientists coming from an intriguing nerve repair concept. Brain Res Rev. (2006) 52:381–8. doi: 10.1016/j.brainresrev.2006.05.002
30. Barbour J, Yee A, Kahn LC, Mackinnon SE. Supercharged end-to-side anterior interosseous to ulnar motor nerve transfer for intrinsic musculature reinnervation. J Hand Surg Am. (2012) 37:2150–9. doi: 10.1016/j.jhsa.2012.07.022
31. Li X-T, Zhang Y-K, Kuang H-X, Jin F-X, Liu D-W, Gao M-B, et al. Mitochondrial protection and anti-aging activity of astragalus polysaccharides and their potential mechanism. Int J Mol Sci. (2012) 13:1747–61. doi: 10.3390/ijms13021747
32. Gu X, Ding F, Williams DF. Neural tissue engineering options for peripheral nerve regeneration. Biomaterials. (2014) 35:6143–56. doi: 10.1016/j.biomaterials.2014.04.064
33. Ando M, Ikeguchi R, Aoyama T, Tanaka M, Noguchi T, Miyazaki Y, et al. Long-term outcome of sciatic nerve regeneration using bio3d conduit fabricated from human fibroblasts in a rat sciatic nerve model. Cell Transplant. (2021) 30:9636897211021357. doi: 10.1177/09636897211021357
34. Jahromi M, Razavi S, Bakhtiari A. The advances in nerve tissue engineering: from fabrication of nerve conduit to in vivo nerve regeneration assays. J Tissue Eng Regen Med. (2019) 13:2077–100. doi: 10.1002/term.2945
35. Faroni A, Mobasseri SA, Kingham PJ, Reid AJ. Peripheral nerve regeneration: experimental strategies and future perspectives. Adv Drug Deliv Rev. (2015) 82-83:160–7. doi: 10.1016/j.addr.2014.11.010
36. Chen S, Zhao Y, Yan X, Zhang L, Li G, Yang Y. PAM/GO/gel/SA Composite hydrogel conduit with bioactivity for repairing peripheral nerve injury. J Biomed Mater Res A. (2019) 107:1273–83. doi: 10.1002/jbm.a.36637
37. Lundborg G, Gelberman RH, Longo FM, Powell HC, Varon S. In Vivo regeneration of cut nerves encased in silicone tubes: growth across a six-millimeter gap. J Neuropathol Exp Neurol. (1982) 41:412–22. doi: 10.1097/00005072-198207000-00004
38. Berrocal YA, Almeida VW, Gupta R, Levi AD. Transplantation of schwann cells in a collagen tube for the repair of large, segmental peripheral nerve defects in rats. J Neurosurg. (2013) 119:720–32. doi: 10.3171/2013.4.JNS121189
39. Zhang Q, Wu P, Chen F, Zhao Y, Li Y, He X, et al. Brain Derived Neurotrophic Factor And Glial Cell Line-Derived Neurotrophic Factor-Transfected Bone Mesenchymal Stem Cells For The Repair Of Periphery Nerve Injury. Front Bioeng Biotechnol. (2020) 8:874. doi: 10.3389/fbioe.2020.00874
40. Gonzalez-Perez F, Hernández J, Heimann C, Phillips JB, Udina E, Navarro X. Schwann cells and mesenchymal stem cells in laminin- or fibronectin-aligned matrices and regeneration across a critical size defect of 15 mm in the rat sciatic nerve. J Neurosurg Spine. (2018) 28:109–18. doi: 10.3171/2017.5.SPINE161100
41. Cui L, Jiang J, Wei L, Zhou X, Fraser JL, Snider BJ, et al. Transplantation of embryonic stem cells improves nerve repair and functional recovery after severe sciatic nerve axotomy in rats. Stem Cells. (2008) 26:1356–65. doi: 10.1634/stemcells.2007-0333
42. Mozafari R, Kyrylenko S, Castro MV, Ferreira RS, Barraviera B, Oliveira ALR. Combination of heterologous fibrin sealant and bioengineered human embryonic stem cells to improve regeneration following autogenous sciatic nerve grafting repair. J Venom Anim Toxins Incl Trop Dis. (2018) 24:11. doi: 10.1186/s40409-018-0147-x
43. Potas JR, Haque F, Maclean FL, Nisbet DR. Interleukin-10 conjugated electrospun polycaprolactone (PCL) nanofibre scaffolds for promoting alternatively activated (M2) macrophages around the peripheral nerve in vivo. J Immunol Method. (2015) 420:38–49. doi: 10.1016/j.jim.2015.03.013
44. Fernando RN, Cotter L, Perrin-Tricaud C, Berthelot J, Bartolami S, Pereira JA, et al. Optimal myelin elongation relies on YAP activation by axonal growth and inhibition by Crb3/Hippo Pathway. Nat Commun. (2016) 7:12186. doi: 10.1038/ncomms12186
45. Cattin A-L, Burden JJ, Van Emmenis L, Mackenzie FE, Hoving JJA, Garcia Calavia N, et al. Macrophage-induced blood vessels guide schwann cell-mediated regeneration of peripheral nerves. Cell. (2015) 162:1127–39. doi: 10.1016/j.cell.2015.07.021
46. Wang ML, Rivlin M, Graham JG, Beredjiklian PK. Peripheral nerve injury, scarring, and recovery. Connect Tissue Res. (2019) 60:3–9. doi: 10.1080/03008207.2018.1489381
47. Li R, Liu Z, Pan Y, Chen L, Zhang Z, Lu L. Peripheral Nerve Injuries Treatment: A Systematic Review. Cell Biochem Biophys. (2014) 68:449–54. doi: 10.1007/s12013-013-9742-1
48. Li ST, Archibald SJ, Krarup C, Madison RD. Peripheral Nerve repair with collagen conduits. Clin Mater. (1992) 9:195–200. doi: 10.1016/0267-6605(92)90100-8
49. Ratheesh G, Venugopal JR, Chinappan A, Ezhilarasu H, Sadiq A, Ramakrishna S. 3D fabrication of polymeric scaffolds for regenerative therapy. ACS Biomater Sci Eng. (2017) 3:1175–94. doi: 10.1021/acsbiomaterials.6b00370
50. Khaing ZZ, Schmidt CE. Advances in natural biomaterials for nerve tissue repair. Neurosci Lett. (2012) 519:103–14. doi: 10.1016/j.neulet.2012.02.027
51. Gros T, Sakamoto JS, Blesch A, Havton LA, Tuszynski MH. Regeneration of long-tract axons through sites of spinal cord injury using templated agarose scaffolds. Biomaterials. (2010) 31:6719–29. doi: 10.1016/j.biomaterials.2010.04.035
52. Chávez-Delgado ME, Gomez-Pinedo U, Feria-Velasco A, Huerta-Viera M, Castañeda SC, Toral FAL-D, et al. Ultrastructural analysis of guided nerve regeneration using progesterone- and pregnenolone-loaded chitosan prostheses. J Biomed Mater Res B Appl Biomater. (2005) 74:589–600. doi: 10.1002/jbm.b.30243
53. Chávez-Delgado ME, Mora-Galindo J, Gómez-Pinedo U, Feria-Velasco A, Castro-Castañeda S, López-Dellamary Toral FA, et al. Facial nerve regeneration through progesterone-loaded chitosan prosthesis. a preliminary report. J Biomed Mater Res B Appl Biomater. (2003) 67:702–11. doi: 10.1002/jbm.b.10059
54. Rosales-Cortes M, Peregrina-Sandoval J, Bañuelos-Pineda J, Castellanos-Martínez EE, Gómez-Pinedo UA, Albarrán-Rodríguez E. Regeneration of the axotomised sciatic nerve in dogs using the tubulisation technique with chitosan biomaterial preloaded with progesterone. Rev Neurol. (2003) 36:1137–41. doi: 10.33588/rn.3612.2002394
55. Subia B, Kundu J, C S. Biomaterial scaffold fabrication techniques for potential tissue engineering applications. Tissue Eng. (2010). 524:142–157. doi: 10.5772/8581
56. Cattin A-L, Lloyd AC. The multicellular complexity of peripheral nerve regeneration. Curr Opin Neurobiol. (2016) 39:38–46. doi: 10.1016/j.conb.2016.04.005
57. Pabari A, Lloyd-Hughes H, Seifalian AM, Mosahebi A. Nerve conduits for peripheral nerve surgery. Plast Reconstr Surg. (2014) 133:1420–30. doi: 10.1097/PRS.0000000000000226
58. Yi S, Zhang Y, Gu X, Huang L, Zhang K, Qian T, et al. Application of stem cells in peripheral nerve regeneration. Burns Trauma. (2020) 8:Tkaa002. doi: 10.1093/burnst/tkaa002
59. Franchi S, Valsecchi AE, Borsani E, Procacci P, Ferrari D, Zaffa C, et al. Intravenous neural stem cells abolish nociceptive hypersensitivity and trigger nerve regeneration in experimental neuropathy. Pain. (2012) 153:850–61. doi: 10.1016/j.pain.2012.01.008
60. Zhou X, Shi G, Fan B, Cheng X, Zhang X, Wang X, et al. Polycaprolactone electrospun fiber scaffold loaded with IPSCs-NSCs and ASCs as a novel tissue engineering scaffold for the treatment of spinal cord injury. Int J Nanomed. (2018) 13:6265–77. doi: 10.2147/IJN.S175914
61. Fairbairn NG, Meppelink AM, Ng-Glazier J, Randolph MA, Winograd JM. Augmenting peripheral nerve regeneration using stem cells: a review of current opinion. World J Stem Cells. (2015) 7:11–26. doi: 10.4252/wjsc.v7.i1.11
62. Bucan V, Vaslaitis D, Peck C-T, Strauß S, Vogt PM, Radtke C. Effect of exosomes from rat adipose-derived mesenchymal stem cells on neurite outgrowth and sciatic nerve regeneration after crush injury. Mol Neurobiol. (2019) 56:1812–24. doi: 10.1007/s12035-018-1172-z
63. Faroni A, Terenghi G, Reid AJ. Adipose-derived stem cells and nerve regeneration: promises and pitfalls. Int Rev Neurobiol. (2013) 108:121–36. doi: 10.1016/B978-0-12-410499-0.00005-8
64. Gordon T. Peripheral nerve regeneration and muscle reinnervation. Int J Mol Sci. (2020) 21–8652. doi: 10.3390/ijms21228652
65. Kang H, Lichtman JW. Motor axon regeneration and muscle reinnervation in young adult and aged animals. J Neurosci. (2013) 33:19480–91. doi: 10.1523/JNEUROSCI.4067-13.2013
66. Wu P, Tong Z, Luo L, Zhao Y, Chen F, Li Y, et al. Comprehensive strategy of conduit guidance combined with VEGF producing schwann cells accelerates peripheral nerve repair. Bioactive Mater. (2021) 6:3515–27. doi: 10.1016/j.bioactmat.2021.03.020
67. Han G-H, Peng J, Liu P, Ding X, Wei S, Lu S, et al. Therapeutic strategies for peripheral nerve injury: decellularized nerve conduits and schwann cell transplantation. Neural Regen Res. (2019) 14:1343–51. doi: 10.4103/1673-5374.253511
68. Martini R, Fischer S, López-Vales R, David S. Interactions between schwann cells and macrophages in injury and inherited demyelinating disease. Glia. (2008) 56:1566–77. doi: 10.1002/glia.20766
69. Xu H, Yan Y, Li S. PDLLA/Chondroitin Sulfate/Chitosan/NGF conduits for peripheral nerve regeneration. Biomaterials. (2011) 32:4506–16. doi: 10.1016/j.biomaterials.2011.02.023
70. Walsh S, Midha R. Practical considerations concerning the use of stem cells for peripheral nerve repair. Neurosurg Focus. (2009) 26:E2. doi: 10.3171/FOC.2009.26.2.E2
71. Liu Q, Spusta SC, Mi R, Lassiter RNT, Stark MR, Höke A, et al. Human neural crest stem cells derived from human escs and induced pluripotent stem cells: induction, maintenance, and differentiation into functional schwann cells. Stem Cells Transl Med. (2012) 1:266–78. doi: 10.5966/sctm.2011-0042
72. Lee D-C, Chen J-H, Hsu T-Y, Chang L-H, Chang H, Chi Y-H, et al. Neural stem cells promote nerve regeneration through IL12-induced schwann cell differentiation. Mol Cell Neurosci. (2017) 79:07. doi: 10.1016/j.mcn.2016.11.007
73. Yang Y, Yuan X, Ding F, Yao D, Gu Y, Liu J, et al. Repair of rat sciatic nerve gap by a silk fibroin-based scaffold added with bone marrow mesenchymal stem cells. Tissue Eng Part A. (2011) 17:2231–44. doi: 10.1089/ten.tea.2010.0633
74. Ding F, Wu J, Yang Y, Hu W, Zhu Q, Tang X, et al. Use of Tissue-engineered nerve grafts consisting of a chitosan/poly(Lactic-Co-Glycolic Acid)-based scaffold included with bone marrow mesenchymal cells for bridging 50-mm dog sciatic nerve gaps. Tissue Eng Part A. (2010) 16:3779–90. doi: 10.1089/ten.tea.2010.0299
75. Guiotto M, Raffoul W, Hart AM, Riehle MO, di Summa PG. Human platelet lysate acts synergistically with laminin to improve the neurotrophic effect of human adipose-derived stem cells on primary neurons. Front Bioeng Biotechnol. (2021) 9:658176. doi: 10.3389/fbioe.2021.658176
76. Kaewkhaw R, Scutt AM, Haycock JW. Anatomical site influences the differentiation of adipose-derived stem cells for schwann-cell phenotype and function. Glia. (2011) 59:734–49. doi: 10.1002/glia.21145
77. Palmara G, Frascella F, Roppolo I, Chiappone A, Chiadò A. functional 3d printing: approaches and bioapplications. Biosens Bioelectron. (2021) 175:112849. doi: 10.1016/j.bios.2020.112849
78. Tao J, Zhang J, Du T, Xu X, Deng X, Chen S, et al. Rapid 3D printing of functional nanoparticle-enhanced conduits for effective nerve repair. Acta Biomater. (2019) 90:49–59. doi: 10.1016/j.actbio.2019.03.047
79. Bonaldo P, Sandri M. Cellular and molecular mechanisms of muscle atrophy. Dis Model Mech. (2013) 6:25–39. doi: 10.1242/dmm.010389
80. Moresi V, Williams AH, Meadows E, Flynn JM, Potthoff MJ, McAnally J, et al. Myogenin and class II HDACs control neurogenic muscle atrophy by inducing E3 ubiquitin ligases. Cell. (2010) 143:35–45. doi: 10.1016/j.cell.2010.09.004
81. Li J, Wang L, Hua X, Tang H, Chen R, Yang T, et al. CRISPR/Cas9-Mediated miR-29b editing as a treatment of different types of muscle atrophy in mice. Mol Ther. (2020) 28:1359–72. doi: 10.1016/j.ymthe.2020.03.005
82. Koning M, Harmsen MC, van Luyn MJA, Werker PMN. Current opportunities and challenges in skeletal muscle tissue engineering. J Tissue Eng Regen Med. (2009) 3:407–15. doi: 10.1002/term.190
Keywords: peripheral nerve regeneration, nerve tissue engineering, pathophysiology, scaffolds, cells, 3D printing
Citation: Li Y, Ma Z, Ren Y, Lu D, Li T, Li W, Wang J, Ma H and Zhao J (2021) Tissue Engineering Strategies for Peripheral Nerve Regeneration. Front. Neurol. 12:768267. doi: 10.3389/fneur.2021.768267
Received: 31 August 2021; Accepted: 15 October 2021;
Published: 16 November 2021.
Edited by:
Ulises Gomez-Pinedo, Instituto de Investigación Sanitaria del Hospital Clínico San Carlos, SpainReviewed by:
Francisco Javier Sancho-Bielsa, University of Castilla-La Mancha, SpainCopyright © 2021 Li, Ma, Ren, Lu, Li, Li, Wang, Ma and Zhao. This is an open-access article distributed under the terms of the Creative Commons Attribution License (CC BY). The use, distribution or reproduction in other forums is permitted, provided the original author(s) and the copyright owner(s) are credited and that the original publication in this journal is cited, in accordance with accepted academic practice. No use, distribution or reproduction is permitted which does not comply with these terms.
*Correspondence: Hui Ma, b3J0aG9wYWluQDE2My5jb20=; Zhenjiang Ma, ZHJfemptYUAxNjMuY29t; Jie Zhao, cHJvZnpoYW9qaWVAMTI2LmNvbQ==
†These authors have contributed equally to this work
Disclaimer: All claims expressed in this article are solely those of the authors and do not necessarily represent those of their affiliated organizations, or those of the publisher, the editors and the reviewers. Any product that may be evaluated in this article or claim that may be made by its manufacturer is not guaranteed or endorsed by the publisher.
Research integrity at Frontiers
Learn more about the work of our research integrity team to safeguard the quality of each article we publish.