- 1Laboratory of Neurochemical Studies, Department of Physiology and Behavior, Bioscience Center, Federal University of Rio Grande do Norte, Natal, Brazil
- 2Laboratory of Neuroanatomy, Department of Morphology, Bioscience Center, Federal University of Rio Grande do Norte, Natal, Brazil
The mammalian retina captures a multitude of diverse features from the external environment and conveys them via the optic nerve to a myriad of retinorecipient nuclei. Understanding how retinal signals act in distinct brain functions is one of the most central and established goals of neuroscience. Using the common marmoset (Callithrix jacchus), a monkey from Northeastern Brazil, as an animal model for parsing how retinal innervation works in the brain, started decades ago due to their marmoset’s small bodies, rapid reproduction rate, and brain features. In the course of that research, a large amount of new and sophisticated neuroanatomical techniques was developed and employed to explain retinal connectivity. As a consequence, image and non-image-forming regions, functions, and pathways, as well as retinal cell types were described. Image-forming circuits give rise directly to vision, while the non-image-forming territories support circadian physiological processes, although part of their functional significance is uncertain. Here, we reviewed the current state of knowledge concerning retinal circuitry in marmosets from neuroanatomical investigations. We have also highlighted the aspects of marmoset retinal circuitry that remain obscure, in addition, to identify what further research is needed to better understand the connections and functions of retinorecipient structures.
1. Introduction
Afferents from the retina to the brain have been an important focus in connectional research for decades (Lane et al., 1971; Martin, 1986; Kaas and Huerta, 1988; Matteau et al., 2003). Although the geniculostriate circuitry has been the primary center of the research on retinal projection, it is long established that other retinorecipient nuclei and pathways exist, and over recent years a concerted effort to comprehend their functional significance has emerged (Kwan et al., 2018).
The body of work describing retinal innervation has relied on several animal models (Cassone et al., 1988; Murakami et al., 1989; Smale et al., 1991; Nemec et al., 2004; Scalia et al., 2014) to reveal the retinal terminal distribution (Guillery, 1970; Tigges and Tigges, 1981; Nascimento et al., 2010) and types of retinal fibers (Ling et al., 1997; de Góis Morais et al., 2014; Santana N. N. M. et al., 2018; Santana M. A. D. et al., 2018). Typical animal models for this research included non-human primates (Moore, 1973; Kaas et al., 1974; Tigges et al., 1977; Pinato et al., 2009), one of which is the common marmoset (Callithrix jacchus), which is a small Neotropical monkey, endemic to Northeastern of Brazil. Furthermore, progress in the knowledge of retinal circuitry has also been achieved by the refinement of the approaches for tracing (Salleeba et al., 2019). Pioneer studies used ablation combined with anterograde degeneration techniques (Campbell, 1969; Hendrickson et al., 1970; Scalia and Arango, 1979), while recent research has employed viral tracers (Martersteck et al., 2017; D’Souza et al., 2021). Even though these useful and sophisticated elements allow a connectional map of retinal inputs, the functional role of part of retinal projection remains unknown. Classically, image-forming (IF) and non-image forming (NIF) systems have been proposed to categorize a numerous of retinorecipient areas and retinal pathways (Cavalcante et al., 2005; Daneault et al., 2016). Here we described the retinorecipient areas with IF and NIF properties from neuroanatomical tracing techniques in common marmosets. This review is motivated by the recent emergence of this primate as a scientific model for studies of neural connections (Majka et al., 2016; Bakola et al., 2021), including research on retinal innervation. Marmosets are an already established animal model for brain research due to their unique neuroanatomy (Solomon and Rosa, 2014; Mitchell and Leopold, 2015; Fukushima et al., 2019; Morais et al., 2019; Ríos-Flórez et al., 2021), high reproductive efficiency, and small bodies (Okano et al., 2012; Ross, 2019). The renewed focus has been because, at least in part, of the successful generation of transgenic marmosets via lentiviral-mediated gene transfer (Sasaki et al., 2009) and by the development of gene-knockout marmosets via genome editing (Sato et al., 2016). Furthermore, to analyze and manipulate populations and networks in the marmoset brain, genetic approaches (MacDougall et al., 2016; Takaji et al., 2016; Mimura et al., 2021) and pipelines for the processing of neural anterograde tracer images (Abe et al., 2017; Lin et al., 2019; Skibbe et al., 2019) have become available. These genetic and anatomical techniques might will become new tools for neuroanatomical and functional investigations of retinal connections.
In the following sections, we will discuss the connectional criteria by which retinorecipient regions are categorized into distinct systems, as well as functional information that is still absent for the retinal innervation in multiple non-image-forming areas. Furthermore, to make this review more fluid, we will include references from other animal models to describe common morphological and functional characteristics of subcortical nuclei. In general, the comparative analysis can be found within those primary references. Several features of the subcortical nuclei of the marmoset are similar to those of other mammalian species, including rodents, macaques, and humans, and we will not emphasize them repeatedly.
2. Overall organization of the marmoset brain
Remarkably, the marmoset brain has several unique features that differentiated it from other primate species (Miller et al., 2016; Eliades and Miller, 2017; Hagan et al., 2017; Atapour et al., 2018), most notably the existence of an area 8C in premotor (Burman et al., 2015) and the lack of cortical area 44 (Roberts et al., 2007; Paxinos et al., 2012). At the same time, the marmoset brain shares common characteristics with other species within the primate order (Chaplin et al., 2013; Ghahremani et al., 2017), such as the dorsolateral prefrontal cortex, inferior temporal cortex, and dorsal pulvinar (Preuss, 2007). Another striking feature of this brain, similar to other primates, is the existence of the complex interconnected circuitry of subcortical areas that receive and process, both simultaneously and in parallel (Callaway, 2005; Nassi and Callaway, 2009). Obviously, the retina is the first step in this network, in which photic inputs are captured, transduced, and decomposed into multiple parallel pathways (Euler et al., 2014; Masland, 2017). These type of retinal signals are transmitted to the brain by diverse retinal ganglion cells (RGCs) (Martersteck et al., 2017). Each type of RGC is sensitive to distinct features of the external environment and conveys them via the optic nerve to retinorecipient areas (Masland, 2012), with IF and NIF functions (Sondereker et al., 2020). Bellow, we will briefly characterize IF and NIF circuits and describe the primary basis for the segregation between them.
3. Image forming and non-image forming circuits
Classically, the organization of retinal circuits has been divided into two functional branches, IF and NIF pathways (Seabrook et al., 2017; Sondereker et al., 2020). In this review, we have included the retinorecipient nuclei, that support vision indirectly in the IF circuitry. Our selection is based on the visuomotor features of these subcortical structures due to their involvement in the pupillary light reflex and involuntary eye movements to stabilize the image (see section 4.2).
The IF circuits give rise to vision directly. The high spatial and temporal resolution of IF pathways allows them to locate and perceive the shapes of objects, and their specific features, such as color, contrast, direction, and orientation (Sanes and Masland, 2015; Baden et al., 2016; Seabrook et al., 2017). The NIF circuits relay global luminance levels of the external environment to support photic-based modulation of core rhythmic physiological processes (Fu et al., 2005; Schmidt and Kofuji, 2009; Do and Yau, 2010; Daneault et al., 2016; Lazzerini Ospri et al., 2017; Seabrook et al., 2017), such as endogenous photoentrainment (Erkert, 1989; Wechselberger and Erkert, 1994; Glass et al., 2001; Silva et al., 2005), hormonal release (Sousa and Ziegler, 1998; Bertani et al., 2010), body temperature (Hetherington, 1978; Hoffmann et al., 2012), and sleep/awake cycle (Hoffmann et al., 2012), in addition to modulating the behavioral repertory for mating opportunities, foraging and predation (Vaze and Sharma, 2013).
Although several studies have been demonstrated interconnections across both IF and NIF circuits (Dacey et al., 2005; Estevez et al., 2012; Hannibal et al., 2014; Sonoda and Schmidt, 2016; Sondereker et al., 2020), the central element for the segregation between them is the partitioning of axonal projections from distinct classes of RGCs to different subcortical nuclei (Seabrook et al., 2017). It is known that RGCs project to (at least) 21 subcortical retinorecipient targets in the marmoset brain (Kaas et al., 1978; Costa et al., 1999; Cavalcante et al., 2005; Engelberth et al., 2008; Lima et al., 2012; de Sousa T. B. et al., 2013; Kwan et al., 2018), each of which exhibits a distinct functional role. The development of the connectomes pipeline for processing anterograde labeling data for the marmoset brain has opened the avenues for a rich understanding of retinal connectional patterns (Lin et al., 2019). Some of the retinorecipient structures, such as dorsal lateral geniculate nucleus (DLG) transmit retinal signals directly to the visual cortex, whereas others, such as superior colliculus (SC) indirectly connect to the cortex via intermediate nuclei, such as pulvinar or via feedforward pathways to the DLG. In addition, many (but not all) NIF structures receive cortical input. The functional significance of those cortical projections is uncertain. As far as we know, none of the hypothalamic retinorecipient centers, such as the suprachiasmatic nucleus (SCN), establish synaptic connections to the cortex.
Here, we do not characterize the organization, function, and homology of RGCs in marmoset retina. Previous publications (Ghosh et al., 1996; Goodchild et al., 1996; Wilder et al., 1996; Gomes et al., 2005; Jusuf et al., 2006; Eriköz et al., 2008; Szmajda et al., 2008; Masri et al., 2017) already provided an excellent in-depth description of the marmoset RGCs population. Our objective is to provide an overview of IF and NIF retinorecipient targets in the marmoset brain in order to demonstrate that there is a substantial body of knowledge regarding the retinal innervation pattern. This part of its functional characteristics still needs examination.
4. Image forming system
Anatomically, the IF circuitry is composed of a series of structures from the retina to the visual cortex, passing through several thalamic and midbrain nuclei (Daneault et al., 2016; Figure 1). In the first part of this review, we will focus on the subcortical nuclei of this system, which receive retinal afferents and exhibit predominantly, or exclusively IF functional properties, including visuomotor features.
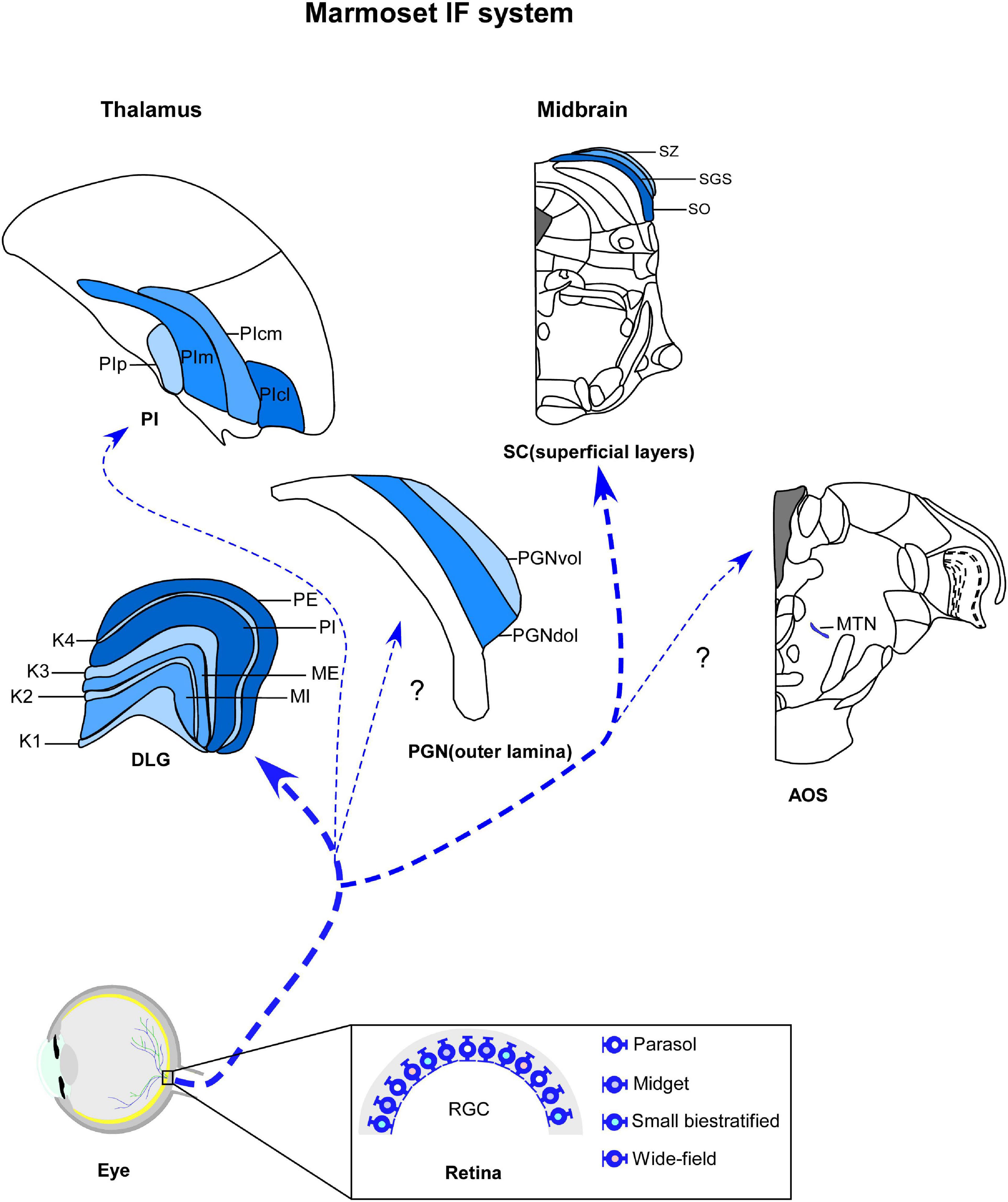
Figure 1. Schematic representation of marmoset image-foming system (blue). Distinct types of retinal ganglion cells (RGCs) bilaterally innervate visual thalamic and midbrain nuclei, such dorsal lateral geniculate nucleus (DLG), inferior pulvinar (PI), outer lamina of the pregeniculate nucleus (PGNol) and superficial layers of superior colliculus (SC). Parasol, midget and small bistratified cells send axonal projections to parvocellular, magnocellular and koniocellular layers of DLG, respectively. SC receives dominant projections from parasol cells. Additional wide-field cells, such as narrow thorny, broad thorny and recursive cell has been described as sending projections to K laminae, CS and PI. The RGC populations that innervates the PGNol, pretectal nuclei and accessory optic nuclei (AOS) still remain unclear. K1–K4, koniocellular layers 1–4; ME, external magnocellular layer; MI, internal magnocellular layer; PE, external parvocellular layer; PGNdol, pregeniculate nucleus dorsal outer lamina; PGNvol, Pregeniculate nucleus ventral outer lamina; PE, internal parvocellular layer; PIcl, central lateral nucleus of the inferior pulvinar; PIcm, central medial nucleus of the inferior pulvinar; PIm, medial nucleus of the inferior pulvinar; Pip, posterior nucleus of the inferior pulvinar; PM, medial pulvinar; PL, lateral pulvinar.
4.1. Thalamus
The IF thalamus is a collection of subcortical nuclei that receives, processes, and transmits information about the visual scene to the cortex (Kerschensteiner and Guido, 2017), in addition to supporting a visuomotor behaviors (Livingston and Fedder, 2003). The three main visual thalamic nuclei are the DLG, pulvinar complex (Sherman and Guillery, 2002; Saalmann and Kastner, 2011) and pregeniculate nucleus (PGN) (Moore, 1989, 1993). These structures are segregated based on their connectional, neurochemical, and functional patterns (Sherman, 2016). The DLG and pulvinar are retinorecipient components of the dorsal thalamus and comprise the two main, functionally distinct visual (geniculostriate and extrastriate) pathways, by which retinal information reaches multiple visual cortices (Harting et al., 1973; Casagrande and Khaytin, 2009). In contrast, the dorsolateral lamina of PGN, considered the non-primate homologous to the ventral lateral geniculate nucleus (VLG) (Moore, 1989, 1993; Lima et al., 2012), is the target of retinal afferents in the ventral thalamus and may modulate the gaze control (Livingston and Mustari, 2000; Livingston and Fedder, 2003). Here, we will highlight the retinorecipient thalamic nuclei involved with IF circuits in marmosets (Figure 1).
4.1.1. Dorsal lateral geniculate nucleus (DLG)
Traditionally, the DLG is a well-established retinorecipient thalamic station that relays image-forming visual input from the retina to primary visual cortex (V1) (Solomon and Rosa, 2014; Mitchell and Leopold, 2015), albeit subsequent studies have suggested a more complex functional role of this structure (see Dan et al., 1996; O’Connor et al., 2002; Belluccini et al., 2019; Dougherty et al., 2019; see Weyand, 2016, for a description of multifunctional nature of the DLG). Based on its relay function, which conveys peripheral (“driver”-type) information to the cortex along the retinocortical pathway, the DLG is considered a first-order thalamic nucleus (Sherman and Guillery, 1998; Bickford, 2016). In addition to retinal innervations that comprises only a minority of the synaptic input to the DLG (de Sousa A. A. et al., 2013), this nucleus receives projections from visual cortices, the thalamic reticular nucleus, and visuotopically organized subcortical structures (Hubel and Wiesel, 1961; Cleland et al., 1971; Usrey et al., 1999; Zeater et al., 2018). Therefore, the DLG represents the first stage of visual processing due to its modulatory influence on visual information before conveying it to the visual cortex (Kaas and Huerta, 1988; Litvina and Chen, 2017).
The DLG of marmosets has a laminar profile, similarly to other primates, with parvocellular (P), magnocellular (M), and koniocellular (K) neurons segregated in multiple and functionally distinct layers (Spatz, 1978; Fitzpatrick et al., 1983; de Sousa A. A. et al., 2013; Baldwin and Krubitzer, 2018; Figure 1). Each cell type differs dramatically in terms of their morphological, physiological, and connectional features, representing distinct parallel channels for visual processing (Mitchell and Leopold, 2015). The P pathways provide high-acuity vision and red-green color vision (Lennie and Movshon, 2005; Martin and Grünert, 2013), while the visual inputs conducted by the M channel provide for spatial and motion analysis (Lee et al., 2010; Percival et al., 2014). A third pathway involves cells located in the K layers, which form another chromatic channel that mediates blue/yellow opponency discrimination (Wässle, 2004; Szmajda et al., 2006, 2008; Roy et al., 2009). In the DLG of marmosets, through classical architectural procedures, such as Nissl and hematoxylin, it is possible to recognize two P layers (internal—PI and external—PE), two M layers (internal—MI and external—ME), and four K laminae (K1–K4) (Kaas et al., 1978; Spatz, 1978; Warner et al., 2010).
Connectional studies provided clear evidence of the retinorecipient nature of the marmoset DLG (Kaas et al., 1978; Lima et al., 2012; Kwan et al., 2018). In common with other mammalians, RGC axons project in an orderly anatomically manner to marmoset DLG (Kaas et al., 1978; Kaas and Huerta, 1988; White et al., 1998; Kwan et al., 2018). The patterning of retinogeniculate projections involves retinal topography, ocular map and lamination specificity of RGCs classes (Pfeiffenberger et al., 2005; Huberman et al., 2008).
Retinotopy. The RGCs send axonal projections to the DLG in an orderly fashion to preserve spatial information about the visual scene, forming retinotopic maps (Grubb and Thompson, 2003; Piscopo et al., 2013; Guido, 2018). Our comprehension of the retinotopic organization in the DLG of marmosets arises from an electrophysiological experiment performed by White et al. (1998). In this report, using extracellular recordings from the DLG neuronal responses, they showed that the contralateral hemifield is represented in this structure (White et al., 1998). Furthermore, these researchers demonstrated that, within each DLG layer, the dorsal visual field is represented laterally, and the ventral visual field is represented medially. The representation of the central foveal vision is located posterodorsally within the DLG, with the peripheral vision progressing anteroventrally (White et al., 1998). This visuotopic pattern is similar to those described for the DLG of other primates.
The mechanisms underlying the retinotopic order have been well studied, and much of our current knowledge may be attributed to the use of transgenic mouse models (Huberman et al., 2008; Kim et al., 2010; Cang and Feldheim, 2013). These reports reveal the cellular and molecular events and drive the refinement of the retinotopy of the DLG, including axon mapping, arbor pruning, neural activity, synapse elimination, and Eph/ephrin signaling (Hong and Chen, 2011; Ackman et al., 2012). However, the targeting mechanisms of retinogeniculate projections in marmosets remain unexplained.
Eye-specific and binocular projections Several electrophysiological (Warland et al., 2006; Zhang et al., 2012), molecular (Pfeiffenberger et al., 2005; Nakamoto et al., 2018), and anatomical (Godement et al., 1984; Muir-Robinson et al., 2002; Jaubert-Miazza et al., 2005) reports have strongly supported the canonical principle of eye-specific segregation of the RGCs axons in the DLG. According to this principle, retinal afferents are separated into distinct DLG laminae and occupy non-overlapping domains (Wallace et al., 2016). In carnivores and primates, each DLG layer receives visual monocular input from the ipsilateral or contralateral eye (Wiesel and Hubel, 1966; Casagrande and Norton, 1991). However, binocular responses or interactions were already has been reported in the DLG of mammalians (Erulkar and Fillenz, 1960; Xue et al., 1987). Binocular responses have been described in monkeys and cats in this structure (Sanderson et al., 1971; Rodieck and Dreher, 1979; Xue et al., 1987), albeit these interactions are naturally suppressive (Sanderson et al., 1971) and require conditions of specialized stimulus (Sanderson et al., 1969, 1971). In rodents, such as rats and mice, the DLG does not exhibit a discernible lamination, the retinal projections from both eyes are only partially segregated (Reese, 1988; Leamey et al., 2007) and many cells have binocular innervation (Grieve, 2005; Rompani et al., 2017). Similarly, it has been demonstrated that the all K layers of marmoset DLG receive a binocular input, although K1 and K3 layers showing columns of ocular segregation (Cheong et al., 2013; Zeater et al., 2015; Kwan et al., 2018).
In general, the organizational pattern of retinogeniculate projections in marmosets is similar to that seen in all primates studied as revealed by anterograde labeling techniques. The retinal inputs in marmoset DLG are topographically organized and delineated in a laminar pattern (White et al., 1998; Kwan et al., 2018). The P and M external laminae receive input from the contralateral nasal retina, whereas P and M internal layers are innervated by the ipsilateral temporal retina (Kaas et al., 1978; Kaas and Huerta, 1988; Kwan et al., 2018). In contrast to monocular excitatory responses of neurons in P and M layers, connectional and physiological studies demonstrated that the subset of K cells exhibits binocular responses (Cheong et al., 2013; Zeater et al., 2015; Kwan et al., 2018). Cheong et al. (2013) and Zeater et al. (2015), recorded single-cell activity in the DLG of anesthetized marmosets, and revealed that the subpopulation of K neurons showed vigorous excitatory response evoked through stimulation of either eye (Cheong et al., 2013 and Zeater et al., 2015). Although autoradiographic evidence has shown that K3 receives a retinal contralateral projection and K1 is innervated bilaterally by the retina (Spatz, 1978), anatomical studies, using bidirectional tracers, demonstrated that all K layers are a target of binocular input, with K1 and K3 laminae exhibiting alternating columns of ipsilateral and contralateral inputs (Kwan et al., 2018). It is speculated that connections from K layers with midbrain nuclei, that regulate spatial attention and orienting, such as CS and parabigeminal nucleus (Casagrande and Kaas, 1994; Hendry and Reid, 2000), potentially provide an indirect route to the V1 (Hendry and Yoshioka, 1994; Solomon et al., 2002; Casagrande et al., 2007) and visual association cortices (Sincich et al., 2004; Warner et al., 2010) used for higher-level form and motion analysis (Zeater et al., 2015). However, the functional and evolutionary role of binocular convergence in the K layers of the marmoset still needs to be extracted.
Numerous studies have shown that activity-mediated binocular competition (Lund et al., 1974; Chalupa and Williams, 1984; Guillery et al., 1985), remodeling of synaptic connections (Chen and Regehr, 2000; Guido, 2008; Hong and Chen, 2011), and retinal waves (Katz and Shatz, 1996; Cohen-Cory, 2002; Torborg and Feller, 2005; for review, see Thompson et al., 2017) play an instructional role in the formation of eye-specific retinogeniculate axons and retinotopic maps in several species of mammalians (Assali et al., 2014). In marmosets, these mechanisms of refinement of retinal circuits in the DLG remain unclear.
RGC class-specific projections. Morphological and connectional reports reveal diversity among RGCs, which comprise (at least) 17 distinct cell types in the retina of marmosets (Ghosh et al., 1996; Szmajda et al., 2005, 2008; Jusuf et al., 2006; Masri et al., 2017). These anatomical works demonstrate that subset of these RGCs types selectively project to the DLG, suggesting that parallel retinal signals enter it and remain segregated within this structure (Kaas and Huerta, 1988; Lee et al., 2010).
Consistent with previous findings in macaques, DLG layers of the marmoset receive input from specific classes of RGCs. Although melanopsinergic intrinsically photosensitive RGCs (ipRGCs), a heterogenous subpopulation that mediate NIF functions, send axonal projections to the DLG (Szmajda et al., 2008), the three best-understood retinogeniculate pathways originate from the parasol, midget, and small bistratified cells (Martin and Grünert, 2013). Most of the RGCs are of the midget type, and project to P layers of the DLG (Goodchild et al., 1996; Gomes et al., 2005; Jusuf et al., 2006). These cells are described as having sustained responses to photic stimuli (Ghosh and Grünert, 1999), selectivity to chromatic (red/green) signals (Martin and Grünert, 2013), and a small soma with a single primary dendrite that branches densely into small dendritic fields, as a main morphological feature (Szmajda et al., 2005; Masri et al., 2017). The second largest class of RGCs is that of parasol cells (Chan et al., 2001; Gomes et al., 2005; Eriköz et al., 2008), which are morphologically characterized by two-four dendrites emerging from a large soma, forming a large branched dendritic tree (Szmajda et al., 2005; Masri et al., 2017). They innervate the M laminae of the DLG (Szmajda et al., 2008), exhibit transient responses to photic input (Ghosh and Grünert, 1999), and contribute to motion perception and spatial vision at low image contrast (Szmajda et al., 2005). Small bistratified cells have synaptic connectivity with K layers of the DLG, particularly K3 (Szmajda et al., 2008), strong blue on/yellow off-color sensitivity (Martin et al., 1997), and relatively small dendritic field diameters (Szmajda et al., 2008; Masri et al., 2017; Paknahad et al., 2021).
Connectional works on the DLG of marmosets has demonstrated that other types of RGCs project to the K layers, albeit their physiological and functional characteristics are less well defined (Szmajda et al., 2008; Percival et al., 2013). Retrograde labeling techniques show that the K1 is a preferential target of narrow thorny cells (Percival et al., 2014). Based on the connectional pattern of K1 with the extrastriate regions, it is suggested that thorny-koniocellular circuitry takes part in residual visual capabilities (“blindsight”) following lesions of V1 in adult or early life (Rodman et al., 1989; Rosa and Tweedale, 2000; Percival et al., 2014). Furthermore, it has also been reported that broad thorny and recursive cells send sparse axons to the K3 lamina (Szmajda et al., 2008; Percival et al., 2011, 2013).
Despite the recent progress in the description of the marmoset retinogeniculate circuitry, the projection patterns of several classes of RGCs and their functional role are still unknown. The morphological diversity in the RGCs of marmosets (Szmajda et al., 2005, 2008; Jusuf et al., 2006; Moritoh et al., 2013; Masri et al., 2017) and their homology with RGCs of other species (Dacey, 2004; Berson et al., 2010; Sanes and Masland, 2015) have been scrutinized previously and, therefore, will not be addressed here. However, whether the connectional pattern of wide-field cells (non-midget, non-parasol, and non-small bistratified cells) in the retina of marmosets shows the same diversity in their retinorecipient nuclei as has recently been reported for the RGCs population in non-primates, especially for mice (Dhande and Huberman, 2014; Robles et al., 2014; Gauvain and Murphy, 2015; Ellis et al., 2016), will require further analysis.
4.1.2. Inferior pulvinar (PI)
The pulvinar complex, referred to as the lateral posterior nucleus in non-primates, is a higher-order thalamic nucleus with multimodal properties, which harbors visually responsive neurons (Kaas and Lyon, 2007; Kwan et al., 2018). Functionally, the pulvinar has been implicated in modulating of visual attention (Chalupa et al., 1976; Bender, 1982; Petersen et al., 1985; Robinson et al., 1986); integration of sensory and cognitive signals (Bridge et al., 2016); shaping of the functional organization of the extrastriate cortex, particularly during early development (Bridge et al., 2016); and regulating cortico-cortical communication (Jones, 2001; Sherman and Guillery, 2002; Shipp, 2003; Saalmann and Kastner, 2011).
Based on the descriptive analysis of chemoarchitectural (Cusick et al., 1993; Stepniewska and Kaas, 1997; Baldwin et al., 2011, 2013; Balaram et al., 2013) and anatomical studies (Warner et al., 2010; Kwan et al., 2018), the pulvinar complex is traditionally subdivided into anterior (oral) medial, lateral, and inferior nuclei (Olszewski, 1952; Kaas and Huerta, 1988; Kaas and Lyon, 2007; Homman-Ludiye and Bourne, 2019; Froesel et al., 2021). The two former nuclei exhibit multisensory (Barbas and Mesulam, 1981; Baleydier and Morel, 1992; de la Mothe et al., 2006, 2012) and somatosensory functions (Mesulam et al., 1977; Acuña et al., 1983), whereas the latter ones, collectively known as visual pulvinar, are dedicated to visual processing and contain a retinotopic map of the contralateral visual hemifield, as well as strong connections to the visual cortex and from the SC (Kaas and Huerta, 1988; Baleydier and Morel, 1992; Stepniewska, 2003; Kaas and Lyon, 2007; Kaas and Baldwin, 2019; Moore et al., 2019). However, only portions of the inferior pulvinar (PI) are also recipients of retinal projections (Benevento and Standage, 1983; Nakagawa and Tanaka, 1984).
The PI has functionally distinct areas, with differences in neuropeptidergic and connectional patterns (Lin and Kaas, 1980; Cusick et al., 1993; Gutierrez et al., 1995; Stepniewska and Kaas, 1997; Cola et al., 1999; Gray et al., 1999; Adams et al., 2000). Despite some divergence in the terminology used to categorize the PI subdivisions (Mathers, 1971; Spatz, 1975; Gutierrez et al., 1995), we have kept the terms adopted by Stepniewska and Kaas (1997), such as medial nucleus (PIm), posterior nucleus (PIp), central medial nucleus (PIcm) and central lateral nucleus (PIcl) of the inferior pulvinar (Figure 1; Stepniewska and Kaas, 1997; Kaas and Lyon, 2007; Kaas and Baldwin, 2019). In general, the PIm is the major target of retinal afferents in the primate pulvinar (Kaas and Lyon, 2007; Baldwin and Bourne, 2017). In combination with PIp and PIcm, it sends axonal projections to dorsal stream visual areas for visually guided actions, whereas the PIcl is mainly devoted to the ventral stream of cortical processing for visual perception (Kaas and Lyon, 2007; Kaas and Baldwin, 2019).
As in all primates studied so far, a retinopulvinar projections in marmosets has been documented (Warner et al., 2010; Kwan et al., 2018). Anatomical reports identified contralateral retinal terminations that are sparse and primarily restricted to PIm (Warner et al., 2010; Kwan et al., 2018), with a few scattered retinal inputs supplying the PIcm and PIcl (Kwan et al., 2018). These studies also show very sparse ipsilateral retinal projections in PIm, in addition to sparser terminals along the boundaries PIp, PIcm, and PIcl (Warner et al., 2010; Kwan et al., 2018). Furthermore, one of those works also reveals, through co-injections of bidirectional tracers, the RGCs subtypes that are the source of these retinal projections to PIm (Kwan et al., 2018). Contrary to a previous report in macaques (Cowey et al., 1994), the subpopulation of RGCs that innervates the PIm of marmosets is that of wide-field cells, mainly broad thorny cells, along with recursive bistratified, narrow thorny and large bistratified cells (Kwan et al., 2018; Grünert et al., 2021). Further studies are needed to discover if other classes of RGCs innervate different regions in the PI of marmosets.
Over the last four decades, considerable progress has been made in understanding the retinotopic organization of the primate pulvinar (Campos-Ortega and Hayhow, 1972; Gattass et al., 1978; Bender, 1981; Petersen et al., 1985; Baldwin et al., 2011; Li et al., 2013). Connectional and electrophysiological reports show it contains two retinotopic maps of the contralateral visual hemifield in its lateral and inferior subdivisions. Their positions and visual field representations exhibit some species-specific singularities (Gattass et al., 1978; Bender, 1981; Li et al., 2013). In marmosets, the visuotopic order of the pulvinar has not yet been investigated in any detail.
4.1.3. Outer lamina of the pregeniculate nucleus (PGNol)
The PGN is a retinorecipient structure of the ventral thalamus topographically dorsal and medial to the DLG (Livingston and Mustari, 2000; Livingston and Fedder, 2003). The prominent neurochemical content and anatomical connections of the PGN laminae with the retina (Babb, 1980; Moore, 1989, 1993; Costa et al., 1998; Pinato et al., 2009; Lima et al., 2012) and IF and NIF subcortical nuclei (Hendrickson et al., 1970; Mustari et al., 1994; Büttner-Ennever et al., 1996b; Chevassus-Au-Louis and Cooper, 1998; Kwan et al., 2018) suggest that it contributes significantly to visuomotor activities and circadian rhythmicity.
Traditionally, the PGN has been described as a laminar structure showed distinct regions with respect to retinal innervation patterns, functional role, and cytoarchitecture (Moore, 1989, 1993). These subsectors include (1) a large region located dorsomedially to the DLG, continuous with the zona incerta, which contains neuropeptide Y (NPY)-ergic neurons and dense retinal innervation, and (2) subdivision contain a scattered neuronal cluster located dorsal and lateral to the DLG (Moore, 1993; Costa et al., 1998; Pinato et al., 2009), that is contiguous with the reticular thalamic nucleus and sparsely receives retinal projections (Moore, 1989, 1993; Costa et al., 1998; Pinato et al., 2009). Despite the divergent nomenclatures of the PGN divisions (Hendrickson, 1973; Babb, 1980; Livingston and Mustari, 2000), its inner portion of PGN (PGNil) is considered equivalent to the intergeniculate leaflet (IGL) of non-primates, a modulating structure of the circadian timing system (CTS) (Moore, 1993; Costa et al., 1998; Pinato et al., 2009) that will be discussed in the next sections. The PGNol, by contrast, is likely the primate counterpart to the VLG (Moore, 1989, 1993; Costa et al., 1998; Lima et al., 2012).
Although more experimental approaches are needed to define the anatomical organization and functional role of the PGN of marmosets in the IF circuitry, its outer portion (PGNol) (Figure 1) has been proposed as a structure equivalent to the VLG (Lima et al., 2012), based on neuropeptidergic content, cell morphology, and connectional patterns with the retina (Costa et al., 1998; Lima et al., 2012) and pulvinar (Kwan et al., 2018). Here, we will follow this classification for a more complete characterization of the PGN of the marmoset.
As expected from reports on other species of primates, anterograde tract tracing has shown the bilateral retinal innervation in the PGN of marmosets (Costa et al., 1998; Lima et al., 2012). Cholera toxin B subunit (CTb)-labeled retinal fibers and terminals project sparsely to the PGNol, with contralateral predominance (Lima et al., 2012). In the ipsilateral side, the ventral portion of PGNol (PGNvol) exhibits a lower density of retinal terminal arbors compared to PGNil, whereas the dorsal part (PGNdol) is poorly innervated (Figure 1; Lima et al., 2012). These results suggest that PGNvol and PGNdol are equivalent to the external and internal portions of the VLG, respectively (Lima et al., 2012).
There has been limited investigation of the classes of RGCs that project to the PGN (Cowey et al., 2001; Hannibal et al., 2014). In macaques, neural tracer injections demonstrated that midget cells predominantly project to the PGN, although terminals from other RGCs subtypes were also identified (Cowey et al., 2001; Hannibal et al., 2014). In marmosets, no study has yet investigated the typology of RGCs that comprise the retina-PGN pathway.
Our knowledge about the visuomotor nature of the PGN comes mainly from ablation-behavioral evidence (Polyak, 1957) and electrode recordings of the PGN neuronal responses of macaques to visual stimuli (Büttner and Fuchs, 1973; Magnin and Fuchs, 1977; Livingston and Fedder, 2003). Although it was initially proposed that the PGN participates in the pupillary light reflex (Polyak, 1957), electrophysiological evidence revealed that it is involved in the modulation of gaze control; including saccadic movements, pursuit smooth eye movements, and visual motion or eye position (Büttner and Fuchs, 1973; Magnin and Fuchs, 1977; Livingston and Fedder, 2003); indicating its functional homology with the VLG. There are no equivalent reports for marmosets.
4.2. Midbrain
In different animal species, the IF midbrain (Figure 1) comprises several nuclear populations that mediate visuomotor reflexes (Gamlin, 2006; Giolli et al., 2006). Although functional and connectional similarities between some of these nuclei have been described (Simpson, 1984; Hoffmann et al., 1988; Simpson et al., 1988; Mustari et al., 1994), cytoarchitectonic evidence and other hodological connections have shown that there are distinctions in several mesencephalic nuclei (Gregory, 1985; Lui et al., 1995; Büttner-Ennever et al., 1996a,b), segregating them into different oculomotor subsystems. The most extensively studied midbrain nuclei are the SC, pretectal complex, and accessory optic system (AOS). The SC translates sensory inputs into motor outputs to guide innate behavior (Ito and Feldheim, 2018). The pretectal nuclei, such as the nucleus of the optic tract and the pretectal olivary nucleus, play a significant role in the optokinetic nystagmus (Hoffmann and Distler, 1989; Mustari and Fuchs, 1990) and pupillary light reflex (Pong and Fuchs, 2000; Szkudlarek et al., 2012). The accessory optic system has functional significance in the detection of retinal slip signals and relaying them to the oculomotor circuit for image stabilization (Fredericks et al., 1988; Masseck and Hoffmann, 2009; Lilley et al., 2018). As far as we know, systematic studies on the retinal projection to the pretectal complex in marmosets are needed. In addition, the typology of RGCs that comprise these retina-midbrain pathways has also not been completely elucidated. As we argued above, these limitations become particularly obvious when one considers the discussion of the retinal projection in the midbrain. Therefore, in this next section, we will explore the connectional pattern of the retina with the SC and AOS.
4.2.1. Superior colliculus (SC)
The SC, also known as the optic tectum in non-mammalians, is a multimodal integrative hub for mediating sensorimotor transformations (Sparks and Mays, 1990; Stein and Meredith, 1993; Hall and Moschovakis, 2004; Chong et al., 2022). Although higher cognitive functions are attributed to the SC (Basso and May, 2017), its two main functional roles are convey retinal signals to other subcortical visual nuclei (Kaas and Huerta, 1988; May, 2006; Basso and May, 2017) and integration of multimodal stimuli into motor commands for orienting movements, and to redirect attention (Gandhi and Sparks, 2003; Gandhi and Katnani, 2011; Basso and May, 2017; Villalobos et al., 2018; Farrow et al., 2019).
Residing on the roof (tectum) of the midbrain, the SC has a laminar profile with seven layers (Kaas and Huerta, 1988; Gandhi and Katnani, 2011; Timurkaan et al., 2013) grouped into two functional compartments (Gandhi and Sparks, 2003; Basso and May, 2017). The superficial one has been described as consisting of three superficial layers; stratum zonale (SZ), stratum griseum superficiale (SGS), and stratum opticum (SO); which are involved in the central processing of visual information and are the targets of retinal signals (Bourne and Rosa, 2003; Markus et al., 2009). In particular, the SGS is commonly subdivided into sublayers, an upper and lower lamina (uSGS and lSGS, respectively), although their distinction, size, and complexity exhibit species-specific differences (Kaas and Huerta, 1988; May, 2006; Basso and May, 2017). Neurons in the superficial layers are considered the visuosensory division of the SC (Basso and May, 2017). In contrast, those in the intermediate (stratum griesum intermedium, stratum album intermedium) and deep (stratum griseum profundum and stratum album profundum) strata, collectively referred to as the deep compartment, are more specifically devoted to multisensory and motor functions (Casagrande et al., 1972; Harting et al., 1973; Stein et al., 1976; McPeek and Keller, 2004), earning the epithet of motor division (Basso and May, 2017).
A ubiquitous aspect of the SC is its connectivity with the retina (for a review, see Kaas and Huerta, 1988; May, 2006; Basso and May, 2017) and so distinguishing the classes of RGCs that target the SC is of particular interest (Hoffmann, 1973; Marrocco and Li, 1977; Illing and Wässle, 1981; Leventhal, 1982). Congruent with studies in macaques, the SC of marmosets receives dominant projections from parasol cells and terminals from a variety of wide-field cells, such as broad thorny, narrow thorny, smooth mono stratified, recursive, large bistratified, and tufted cells, as evidenced by bidirectional tracer injections (Kwan et al., 2018; Grünert et al., 2021). Our knowledge of the functional properties of wide-field ganglion cells that innervate the SC in the marmoset is still scarce. As expected from studies in other species of primates, electrophysiological records show that broad thorny cells are a retinal source of ON/OFF type responses in the SC (Eiber et al., 2018), as well as parasol cells input can be clearly related to the high selectivity of collicular neurons for moving stimuli (Tailby et al., 2012).
Although intraspecific variations in the proportion (Wässle and Illing, 1980; Hofbauer and Dräger, 1985; Dhande and Huberman, 2014; Ellis et al., 2016) and sublaminar arrangement of retinotectal projections (Pollack and Hickey, 1979; Conley et al., 1985) have extensively been described, the spatial profiling and delineation of these pathways remain a matter of interest. As in all mammalians studied so far, the patterns of retinal afferents in the SC of marmosets has a characteristic distribution in superficial layers (Figure 1). Bilateral retinal afferents are distributed primarily to the SGS, with dense terminals in their sublayers, and weak label in the SO and SZ layers (Kwan et al., 2018).
One of the distinctive features of the SC is a well-organized retinotopy, which is evident in all studied mammalians (Lane et al., 1971, 1973; Cynader and Berman, 1972; Kaas et al., 1974; Updyke, 1974; Ito and Feldheim, 2018). For example, in primates, the SC contains a topographic map of the contralateral visual hemifield provided by both eyes. The dorsal visual field is represented medially and the ventral visual field projects laterally. The representation of the foveal vision is located rostrally within the SC, with peripheral representation progressing caudally (Kaas and Huerta, 1988). Consistent with previous reports in macaques (Pollack and Hickey, 1979), the central retinotopic representations in the SC of marmosets demonstrate a complex pattern of retinal projections. Some areas receive binocular inputs in both the uSGS and lSGS, or contralateral input in the lSGS and binocular input in the lSGS, and others receive exclusively contralateral input (Kwan et al., 2018). Moreover, the medial and lateral colliculus exhibit overt delineation of ipsilateral and contralateral inputs in the SGS (Kwan et al., 2018). Systematic studies are needed to describe whether the same ordered representation of visual space found in the surface layers of the SC of marmosets is also present in the deep compartment of this structure.
4.2.2. Accessory optic system (AOS)
In mammalians, the AOS comprises two sets of accessory fasciculi, the inferior and superior ones, and three paired terminal nuclei, the dorsal terminal, lateral terminal, and medial terminal nuclei (MTN), that receive retinal signals via the accessory optic tract (for a review, see Giolli et al., 2006; Brodsky, 2012). Different experimental approaches (Simpson et al., 1979, 1988; Clement and Magnin, 1984; Natal and Britto, 1988; Benassi et al., 1989; Lilley et al., 2018) support the functional significance for AOS in detecting signals of retinal slip and relaying them to the oculomotor circuits for image stabilization (Fredericks et al., 1988; Masseck and Hoffmann, 2009; Lilley et al., 2018). In particular, the terminal nuclei drive complementary directions of optokinetic nystagmus, albeit other oculomotor responses have been attributed to them (Simpson et al., 1979, 1988; Sun et al., 2015). The MTN and lateral terminal nuclei drive vertical optokinetic movements, while the dorsal terminal nucleus mediates the horizontal ones (Krause et al., 2014; Sun et al., 2015).
In marmosets, autoradiographic and histochemical anterograde labeling techniques revealed projections from the retina to the dorsal division of the MTN (Figure 1; Cooper and Magnin, 1986), which is congruent with anatomical studies in several species of primates (Itaya and Van Hoesen, 1983; Cooper, 1986; Weber and Giolli, 1986; Cooper and Magnin, 1987). Although retrograde tracer injections in different mammalian species showed that bistratified or gamma-like RGCs project to AOS (Farmer and Rodieck, 1982; Dann and Buhl, 1987), similar studies in marmosets are needed.
5. Non-image forming system
The NIF circuitry is formed by the diencephalic and midbrain nuclei, which detect environmental irradiance to modulate several physiological and behavioral processes (Daneault et al., 2016). Except for CTS, the functional significance of the NIF territories is unknown or merely speculative, a fact that contributes to its nebulous profile. In the next topic, we will discuss hodological evidence and the functional role of NIF domains in the brain of marmosets.
5.1. Circadian timing system
Although the anatomically-oriented discussion is necessary for a comprehensive understanding of NIF territories, in the next section we will assemble the neuroanatomical substrate of the CTS network (Figure 2). Given its pivotal role in generating and modulating circadian rhythmicity, as well as its adaptive aspect for living organisms, including marmosets. Below, we will review the central hypothalamic components of the CTS, since systematic studies of the retinal innervation in the dorsal (DRN) and median (MnR) raphe nuclei, a discrete cluster of serotonin-containing neurons implicated in different circadian functions are absent in marmosets.
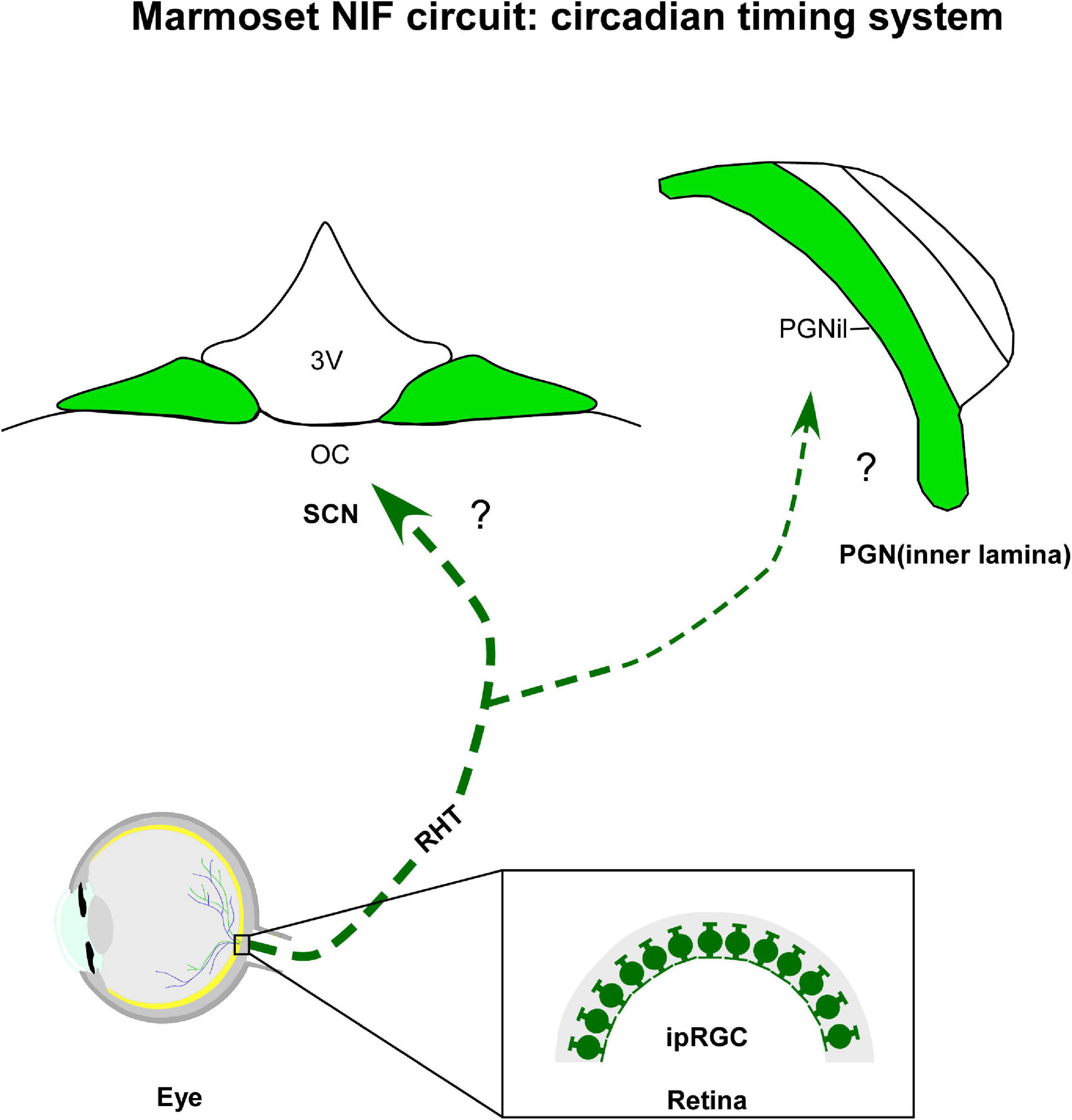
Figure 2. Diagrammatic representation of the marmoset circadian timing system (green). The suprachiasmatic nucleus (SCN) and inner laminar of pregeniculate nucleus (PGNil) are retinorecipient structures involved with the biological rhythms. Note that although melanopsin-containing intrinsically photosensitive retinal ganglion cells (ipRGCs) were evidenced in marmoset retina, the retinal cell type(s) providing the input to circadian centers have not yet been identified. 3v, third ventricle; oc, optic chiasma; RHT, retinohypothalamic tract.
Mammalian species possess an endogenous system that synchronizes time cues, most importantly the environmental light-dark cycle, to orchestrate rhythmic biological functions, as well as ethological outputs (Xie et al., 2019; Finger and Kramer, 2021). This temporal coordination is traditionally driven by four main elements: (a) synchronizing pathways responsible for phototransduction and transmission of bioelectrical signals to a central oscillator; (b) a central oscillator, also known as the central pacemaker or master clock, a neural structure that governs circadian rhythms; (c) modulating nuclei which modify the function of the master clock and provide an indirect source of photic signaling; and (d) efferent pathways which relay timing signals to different body systems (Morin and Allen, 2006; Rosenwasser and Turek, 2015; Hastings et al., 2018). Despite this configuration being a simplified model of the CTS, a range of evidence has demonstrated that this circuitry is more complex (Zehring et al., 1984; Vosshall et al., 1994; Brancaccio et al., 2013; Mei et al., 2018). It is now clear that the CTS is a hierarchically organized network, comprising a body-wide multiplicity of circadian oscillators (extra-SCN brain clocks and peripheral clocks), in addition to cell-autonomous oscillators within virtually every cell class (Mure et al., 2018). The complexity of the circadian clock networks surpasses the purpose of this review. Recent publications (Astiz et al., 2019; Hastings et al., 2019; Finger and Kramer, 2021) approached molecular machinery, communication, and anatomy of the CTS for in-depth comprehension.
5.1.1. Suprachiasmatic nucleus (SCN)
As the primary oscillator of the CTS, the SCN (Figure 2) of the anterior hypothalamus conveys temporal information, synchronizing the other clocks in the brain and body to produce coherent circadian rhythms at physiological and behavioral levels (Astiz et al., 2019). Immediately dorsal to the optic chiasm, and flanking the third ventricle (Moore and Lenn, 1972; Van den Pol, 1991), the SCN is conventionally divided into two functionally distinct domains, a ventrolateral/core and dorsomedial/shell subnuclei, distinguished by neuronal cytoarchitecture (Van den Pol, 1980; Mammen and Jagota, 2011), neurochemical phenotype (Moore et al., 2002; Morin, 2013; Allali et al., 2017), organization of afferent innervation (Moga and Moore, 1997), distribution of efferent projections (Leak and Moore, 2001), pattern of gene expression (Dardente et al., 2002), and electrical activity (Schaap et al., 2003). The functional significance of SCN compartments remains to be explored in detail, however, it is hypothesized that the prominent role of the core subregion is to maintain cellular coupling within the SCN and integrate relevant afferents for the entrainment of the master clock, while its shell subregion may have primary responsibility for coordinating the phase configuration of oscillators present in peripheral tissues and brain regions other than the SCN (Dibner et al., 2010; Welsh et al., 2010; Evans et al., 2015).
The interneuronal network of the SCN has been examined over the years. Tracing techniques have revealed the multiple neuronal connections linking the central clock with other brain territories. The foremost afferent systems of the SCN arise from the retina, IGL, pretectal complex, and the MnR (Hendrickson et al., 1972; Card and Moore, 1984; Meyern-Berstein and Morin, 1996; for a review, see Rosenwasser and Turek, 2015). At the same time, the SCN forms afferent connections with hypothalamic and extra-hypothalamic domains, allowing the adjustment of outputs from this nucleus. In addition to receiving these projections, the SCN produces diffusible signals targeting thalamic, hypothalamic, and forebrain territories (Buijs et al., 1993, 2017; Kalsbeek et al., 1993; Leak and Moore, 2001; for review see Hastings et al., 2019).
In all mammalians studied so far, the SCN receives direct photic inputs from ipRGC via the retinohypothalamic tract (RHT), a monosynaptic pathway that also innervates other NIF centers (LeGates et al., 2014). The RHT is both necessary and sufficient for photic entrainment of the SCN, as revealed by ablation, lesion, and genetic studies (Klein and Moore, 1979; Johnson et al., 1989; Panda et al., 2002). In marmosets, a dense bilateral retinal projections to the SCN have its core sub-domain as the main target, with a contralateral predominance. Sparse terminals and fibers were observed in the shell portion, specifically at intermediate and caudal levels of the SCN (Costa and Britto, 1997; Costa et al., 1998, 1999). Although the ipRGCs and their subclasses have been identified in the retina of marmosets (Ghosh et al., 1996; Jusuf et al., 2006; Szmajda et al., 2008; Masri et al., 2017), the subtypes that form the RHT remain uncertain. Further research is needed to verify this issue.
5.1.2. Inner lamina of pregeniculate nucleus (PGNil)
The PGNil of marmosets (Figure 2), which lies dorsomedial to the DLG, is the probable homologous of the IGL found in the brain of non-primates. This hypothesis is classically based on the presence of the NPY+ cells, as well as a dense plexus of serotonergic and retinal fibers (Moore, 1989, 1993; Costa and Britto, 1997; Costa et al., 1998; Pinato et al., 2009; Lima et al., 2012) in the ventral area of the PGN. This is supported by other cytoarchitectonic (Polyak, 1957; Niimi et al., 1963; Hendrickson, 1973; Babb, 1980; Livingston and Mustari, 2000) and neurochemical evidences (Moore, 1993; Costa et al., 1998; Lima et al., 2012).
The anterograde tracer labeling shows that the PGNil receives a bilateral retinal innervation, with a contralateral predominance (Costa and Britto, 1997; Costa et al., 1998). Particularly on the ipsilateral side, a dense fiber plexus was evident when compared to the PGNol (see section 4.1.3.). Consistent with other species of primates (Moore, 1993; Théoret et al., 2000; Pinato et al., 2009), the bilateral retinal projections are concentrated in the ventral portion of the PGN of marmosets, near the P layers of the DLG, and they are sparsely distributed in the dorsal area, closer to the reticular thalamic nucleus (Costa et al., 1998; Lima et al., 2012). A comparative analysis between monkeys and humans proposes that this ventral domain would be equivalent to the IGL of rodents (Moore, 1993), a modulating nucleus, which integrates a variety of stimuli, both photic and non-photic, and transmits this consolidated information to the SCN (Sanetra et al., 2021). However, whether the PGNil neurons of marmosets show the same functional properties reported for their rodent counterpart (Dark and Asdourian, 1975; Harrington and Rusak, 1986; Cipolla-Neto et al., 1995; Goel et al., 2000; Gall et al., 2013; Sanetra et al., 2021) also needs further examination.
One open question about the retina-PGN pathway is the RGCs subtypes that innervate the PGNil. Although double immunohistochemistry for pituitary adenylate cyclase-activating polypeptide (PACAP) and CTb showed that the most ventral part of the PGN in macaques receives projections from the ipRGC (Hannibal et al., 2014), information about the typology of this retinal population, as well as hodological evidence of this connection, is still absent for marmosets.
5.2. Thalamus
Different non-image forming processes involve distinct thalamic nuclei, which form miscellaneous thalamocortical circuitry that helps to maintain homeostasis, with nociception, visceral activity, cognition, arousal, and sensorimotor activity being the most crucial functions. The mediodorsal nucleus (MD), as well as the midline and intralaminar nuclei (MIN) are key structures implicated in this functional repertory. In the next section, we will describe the retinorecipient targets in the thalamus of marmosets, with NIF properties (Figure 3A).
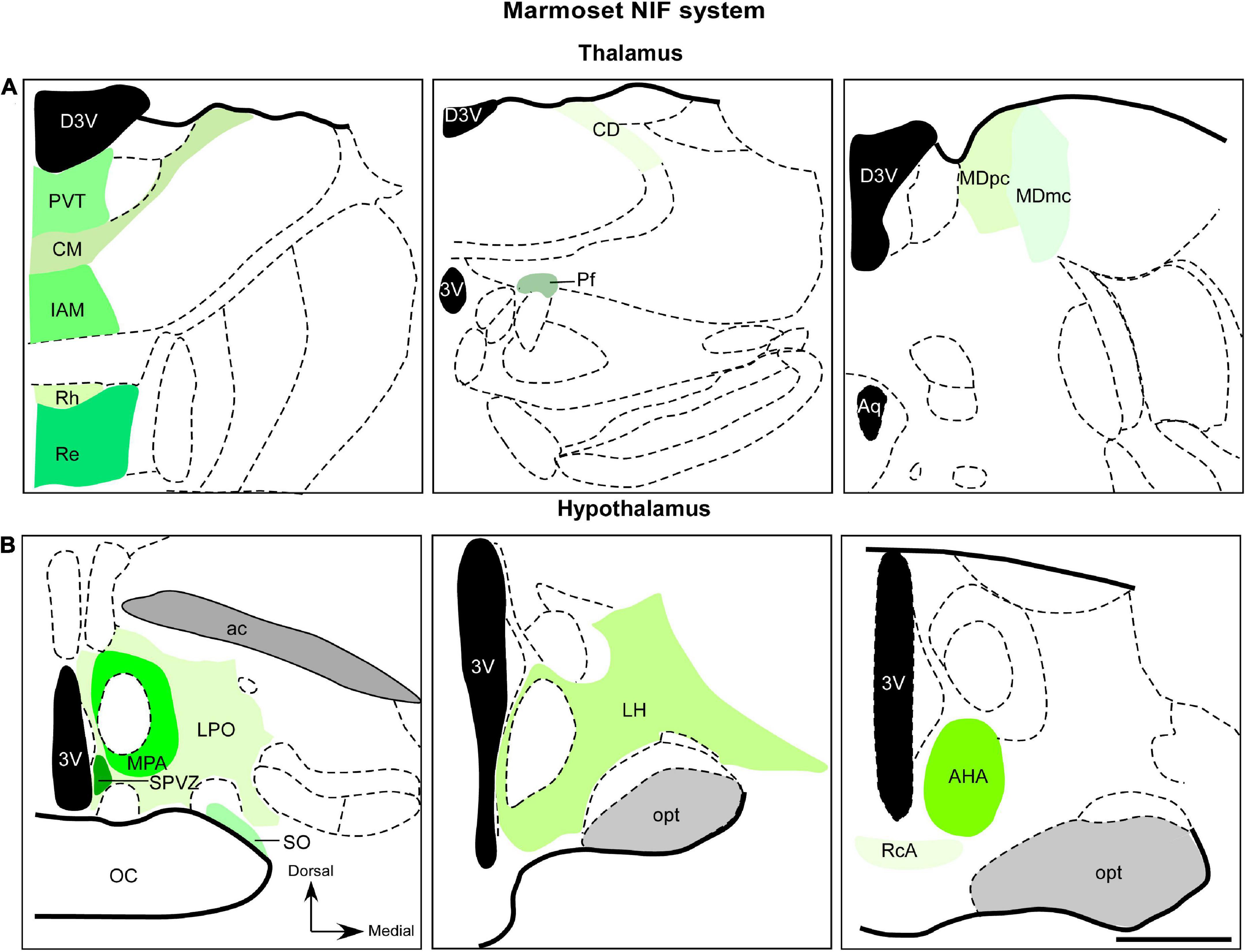
Figure 3. Diagrammatic representation of diencephalic marmoset non-image-foming nuclei (green). Retinal projections were described in mediodorsal nucleus, midline and intralaminar thalamic structures (A) and hypothalamic domains (B). The RGCs subtypes that project to these regions have not yet been elucidated. 3v, third ventricle; ac, anterior commissure; AHA, anterior hypothalamic area; aq, cerebral aqueduct; CD, central dorsal nucleus; CM, central medial nucleus; D3v, dorsal 3v; Iam, inter-antero medial nucleus; LH, lateral hypothalamic area; LPO, lateral preoptic area; MDmc, magnocellular division of mediodorsal nucleus; MDpc, parvocellular division of mediodorsal nucleus; MPA, medial preoptic area; opt, optic tract; Pf, parafascicular nucleus; PvT, paraventricular thalamic nucleus; RcA, retrochiasmatic area; Re, reuniens nucleus; Rh, rhomboid nucleus; SO, supraoptic nucleus; SPVZ; sub paraventricular zone. Scale bar: 500 μm. Adapted from Paxinos et al. (2012).
5.2.1. Mediodorsal nucleus (MD)
The MD, also referred to as medial dorsal thalamic nuclei, nucleus medialis dorsalis, and the dorsomedial thalamus (Mitchell and Chakraborty, 2013), is a high-order thalamic relay nucleus (Guillery, 2005; Sherman, 2016) that participates in several corticosubcortical circuits, mainly those involving the prefrontal cortex (Mitchell, 2015; Golden et al., 2016). Topographically lateral to the midline nuclei and medial to the intralaminar thalamic complex, the MD is primarily involved in cognitive functions, such as learning (Gaffan and Parker, 2000; Parnaudeau et al., 2013, 2015; Ouhaz et al., 2015, 2017, 2018), odor perception (Courtiol and Wilson, 2014, 2015; Wilson et al., 2014), emotion (Timbie and Barbas, 2015), and memory processing (Funahashi, 2013), although other additional functions are suggested (Blanchard and Blanchard, 1972; Gillett and Webster, 1975; Li et al., 2004).
In primates, the MD is considered one of the largest thalamic nuclei and is cytoarchitectonically divided into at least four distinct subnuclei (Bentivoglio et al., 1993; Bachevalier et al., 1997). Despite the existence of further subdivisions, the MD domains are typically distinguished into magnocellular (MDmc), parvocellular (MDpc), caudodorsal, and lateral (Ouhaz et al., 2018). An exception to this neuroanatomical organization is described in the MD of marmosets, which is characterized by two different subregions based on cell morphology (Roberts et al., 2007), a rostromedially MDmc division, and a caudolateral MDpc one (Ray and Price, 1992; de Sousa T. B. et al., 2013). The major neural connections of the primate compartments of the MD are unique to each subregion and have been extensively summarized (Mitchell and Chakraborty, 2013; Mitchell, 2015). In addition to receiving driving inputs mainly from the prefrontal cortex (Krettek and Price, 1977; Goldman-Rakic and Porrino, 1985; Groenewegen, 1988; Ray and Price, 1993; McFarland and Haber, 2002; Xiao et al., 2009), the distinct portions of the MD have differential connectional patterns with areas of the medial temporal lobes (perirhinal and entorhinal cortex and the amygdala (Krettek and Price, 1977; Aggleton and Mishkin, 1984; Aggleton et al., 1986; Russchen et al., 1987; Groenewegen et al., 1990; Saunders et al., 2005), as well as the cingulate cortex, insular cortex, and supplementary motor cortex (for a review, see Mitchell and Chakraborty, 2013; Mitchell, 2015; Ouhaz et al., 2018). Furthermore, the MD is a target of modulatory inputs from the pallidum, the reticular thalamus, midbrain, and brainstem regions (Kuroda and Price, 1991a,b; Sherman and Guillery, 1996), structures particularly related to ocular movements, such as the substantia nigra pars reticulata (Wurtz and Goldberg, 1972; Harting et al., 1980; Ilinsky et al., 1985; Russchen et al., 1987) and the motor layers of the SC (Erickson et al., 2004).
The retinal afferents in the MD of marmosets was revealed by anterograde tracer histochemistry (de Sousa T. B. et al., 2013). This work showed an exclusive retinal contralateral innervation, with sparse retinal arbors and terminals into MDmc and MDpc subnuclei (Figure 3A) in the caudal aspect of the MD. Furthermore, retinal fibers oriented dorsoventrally, and detailed morphology of the retinal axons were described, including simple endings, large caliber axons with numerous varicosities, and rosette-like clusters (de Sousa T. B. et al., 2013). The retina-MD pathway has also been described in rock cavy (Kerodon rupestris), as revealed by an anatomical study, although this innervation is restricted to the medial parts in the mid and caudal levels of this nucleus (Nascimento et al., 2010).
Although electrophysiological (Schlag and Schlag-Rey, 1986; Tanibuchi and Goldman-Rakic, 2003; Wyder et al., 2003; Tanaka, 2007) and anatomical studies (Wurtz and Goldberg, 1972; Kievit and Kuypers, 1977; Goldman-Rakic and Porrino, 1985; Russchen et al., 1987) indicate the MD participates in visuomotor integration in primates (Wurtz and Albano, 1980; Tanaka, 2007), the functional role of the retinal-MD circuit remains unexplored. It is speculated that the retina-MD projection potentially provides an indirect route from the retina to the prefrontal cortex, whose photic input might exert a specific influence on prefrontal cortical functioning (de Sousa T. B. et al., 2013). Furthermore, substantial research is needed on the issue of the RGCs subtypes that innervate the MD.
5.2.2. Midline and intralaminar nuclei (MIN)
The MIN are a higher-order nuclear complex, which was initially thought to be a non-specific arousing circuit in the brain due, among other features, to their widespread connectional pattern with the cortex (Bentivoglio et al., 1991; Saalman, 2014; Zhou and Zhu, 2019). Anatomical and functional data have demonstrated that the MIN are involved in specific brain functions, from cognitive to sensorimotor properties (Bentivoglio et al., 1991; Groenewegen and Berendse, 1994; Van der Werf et al., 2002; Vertes et al., 2022). Furthermore, the growing electrophysiological evidence supports the functional role of the MIN in the control of the transmission of cortical information (for a review, see Saalman, 2014). Due to space limitations, we will not discuss the architecture, connectivity and functions of the marmoset MIN, and will confine this review to retinothalamic projections. Previous studies (Bentivoglio et al., 1991; Groenewegen and Berendse, 1994; Van der Werf et al., 2002) provide a well-documented characterization of the MIN.
Anterograde labeling from the marmoset retina has revealed a moderate plexus of retinal fibers, forming a “continuum” in the dorsoventral direction (Figure 3B). This innervation starts from the paraventricular nucleus (PVT), reaching the inter-antero-medial reuniens and rhomboid nuclei. In the intralaminar complex, a sparse terminal plexus was found contralaterally, in the central dorsal nucleus. The central medial and parafascicular nuclei also exhibited scattered terminal fibers (Cavalcante et al., 2005).
As far as we know, except for the rock cavy, the retinal afferences to the MIN have not been reported in any vertebrate species. Only the PVT receives a direct retinal projection in the rock cavy (Nascimento et al., 2008). Under these circumstances, it is easy to suppose that retinal innervations in the MIN of marmosets, except for the PVT, are a species-specific characteristic. However, anatomical and evolutionary studies in other species are needed to verify the possible universality of these afferences and elucidate their functional significance. Moreover, it is important to stress that both were studies performed the anterograde transport of CTb, a tracer extensively used for monosynaptic mapping (Lai et al., 2015). Therefore, it is unlikely that the labeled CTb-fibers/terminals described in those works could be due to the transsynaptic transport from other retinorecipient domains (see comments in Costa et al., 1999). Furthermore, the CTb immunoreactive elements were not observed in well-stablished secondary visual areas, such as the visual cortex (Cavalcante et al., 2005; Nascimento et al., 2008), which corroborates the evidence. Surely, specific functional and evolutionary work is needed regarding the participation of retinal projections in the functional and phylogenetic aspects of the MIN. Further studies on the class of RGCs that innervates the MIN of marmosets are also required since this specific cell population was not yet characterized.
One interesting aspect to be considered is the retina-PVT pathway identified in the marmoset brain (Figure 3B). PVT is the main component of the midline thalamic nuclei, which extends rostrocaudally and ventral to the third ventricle (Kirouac, 2015). This nucleus is considered a hub of neural circuits underlying drug addiction, anxiety, emotional processing, and defensive responses (Zhou and Zhu, 2019; Barson et al., 2020; Kirouac, 2021). However, it is suggested that the PVT also takes part in the circadian regulation, based on lesion studies (Bhatnagar and Dallman, 1999; Moga and Moore, 2000; Salazar-Juárez et al., 2002). The fact that the PVT receives input from CTS structures, including IGL, DRN, and MnR nuclei (Cornwall and Phillipson, 1988; Moga and Moore, 2000; Leak and Moore, 2001; Li and Kirouac, 2012), as well as reciprocal connections with the SCN (Moga et al., 1995; Vertes and Hoover, 2008) also indicates that it may be involved in functions related to the modulation of circadian rhythms. This is in line with the view that the neural activity of PVT is enhanced during the active phase of the light cycle (Peng et al., 1995; Novak and Nunez, 1998; Kolaj et al., 2012). Since this structure has been hypothesized to be involved in circadian modulation, PVT neurons may be the centers of regulatory circuits of the sleep-wake cycle and circadian system (see comments in Colavito et al., 2015). Nevertheless, functional properties and phylogenetic evidence of the retina-PVT pathway require further research.
5.3. Hypothalamus
The NIF hypothalamic network in the marmoset brain comprises extra-SCN nuclei involved in control of many fundamental processes, from circadian rhythms to reproductive behaviors. In this section, we will highlight evidence from retinal innervation, as well as discuss the possible functional aspect of this NIF circuitry.
5.3.1. Extra-SCN regions
Although the SCN is a well-known hypothalamic target of the retina, hodological studies demonstrated retinal projections to other domains of this structure in several mammalian species (Pickard and Silverman, 1981; Johnson et al., 1988; Murakami et al., 1989; Levine et al., 1991; Youngstrom et al., 1991; Tessoneaud et al., 1994; Abizaid et al., 2004; Hattar et al., 2006). These nuclei play different NIF functional roles, from circadian rhythmicity to reproductive behavior (Saper and Lowell, 2014). In marmosets, diffuse retinohypothalamic projections were described in lateral and medial preoptic, anterior hypothalamic, lateral hypothalamic, and retro chiasmatic areas, besides the supraoptic nucleus, and subparaventricular zone, as revealed by tract-tracing procedures (Costa et al., 1999; Figure 3B). This latter structure is known as a critical hypothalamic hub for driving rhythmic output from SCN and ultimately modulate circadian rhythms of a several physiological process (Saper, 2013; Vujovic et al., 2015; Wu et al., 2018). A comprehensive overview of these hypothalamic regions in mammalians is beyond the scope of this review and a detailed discussion of these nuclei can be found within classical (Moore and Lenn, 1972; Moore, 1973) and recent works (Canteras et al., 2011; Morin and Studholme, 2014). Here, we will focus on retinal innervation and the functional significance of these afferences.
Costa et al. (1999), via the analysis of anterograde tracing, showed that several hypothalamic areas receive retinal projections, particularly those involved in many distinct light-mediated behaviors, such as sleep, body temperature, circadian rhythm phase control, and neuroendocrine processes related to reproductive functions (Costa et al., 1999). However, there are no functional studies of these retinohypothalamic projections. The organization of RGCs that innervate the hypothalamic extra-SCN regions has also not been researched in marmosets.
5.4. Midbrain
Retinal afferents innervate a restricted cell grouping in the midbrain, which exhibits NIF functional characteristics, such as pain responses (Tracey et al., 2002; Loyd and Murphy, 2009), defensive and aversive behaviors (De Oca et al., 1998; Benarroch, 2012), central autonomic control (Saper and Stornetta, 2015), and modulation of circadian rhythms (Ciarleglio et al., 2011; Whitney et al., 2016). In a variety of species, retinal inputs have been described in the periaqueductal gray (Fite et al., 1999), DRN (Foote et al., 1978; Kawano et al., 1996; Reuss and Fuchs, 2000), and parabrachial complex (PBN) (Fite and Janusonis, 2002). It is important to explain that, although the periaqueductal gray plays a critical role in neurovegetative functions and behavioral responses to threatening stimuli (Faull et al., 2019), its retinal innervation is yet to be determined in marmosets. Furthermore, as previously mentioned, there is no hodological evidence in marmosets demonstrating the retina-DRN pathway. Thus, these factors restrict the explanation of the retinal input to the PBN, a hub for autonomic functions, and interoceptive and exteroceptive inputs relevant to sensory processing (Chiang et al., 2019).
5.4.1. Parabrachial complex (PBN)
In most mammals studied to date, the PBN has been described as a cell cluster in the dorsolateral pons, which is dissected by the superior cerebellar peduncle into two distinct subnuclei, the medial parabrachial (mPBN) and lateral parabrachial (lPBN) nuclei (Fulwiler and Saper, 1984; Hansell and Frank, 1991; Chiang et al., 2019; Figure 4). However, anatomical studies in mice, cats, and monkeys demonstrated a third subdivision of the PBN, the Kölliker–Fuse nucleus, a collection of neurons located in the ventrolateral region of the superior cerebellar peduncle (Saper and Loewy, 1980; Fulwiler and Saper, 1984). In marmosets, the PBN is formed by mPBN and lPBN, based on cytoarchitectonic data (Engelberth et al., 2008).
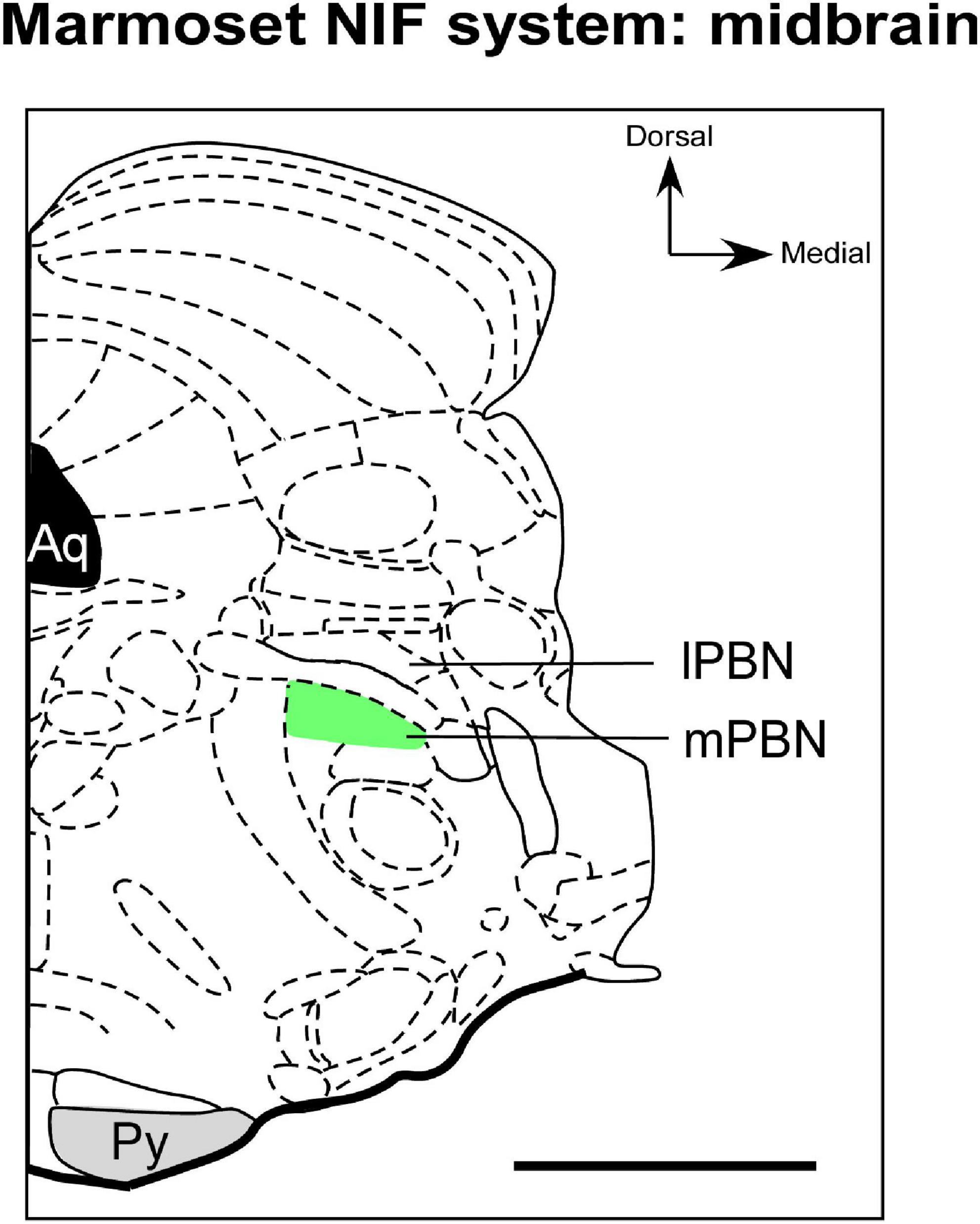
Figure 4. Schematic representation of the parabrachial complex in coronal section of the marmoset brain. The types of RGCs that send afferents to mPBN (green), a NIF site in midbrain, have not yet been documented in marmoset. aq, cerebral aqueduct; lPBN, lateral parabrachial nucleus; mPBN, medial parabrachial nucleus; Py, pyramidal tract. Scale bar: 500 μm. Adapted from Paxinos et al. (2012).
As the interface between the medullary reflex control and the behavioral and integrative regulation of the central autonomic network, the PBN has long been recognized as a pivotal structure in autonomic control (Saper and Stornetta, 2015). Traditionally, sensory input relevant to taste is processed by the mPBN, and viscerosensory information (visceral malaise, itch, blood pressure, hydric ingestion, and sodium appetite) has been consistently correlated with the lPBN activity (Hugelin and Vibert, 1974; Hansell and Frank, 1991; Reilly, 1999; Davern, 2014; Menani et al., 2014). Furthermore, its functional role in processing nociceptive and thermosensory stimuli has been revealed in electrophysiological, optogenetic, and behavioral approaches (Yahiro et al., 2017; Barik et al., 2018; Xu et al., 2019; Sun et al., 2020).
This functional complexity is based on the hodological pattern of the PBN (Gioia et al., 2000). Retrograde and anterograde tract tracing both revealed that the PBN is the target of axonal inputs primarily from the nucleus tractus solitarii (Ricardo and Koh, 1978; Tokita et al., 2009) as well as trigeminal and spinal dorsal horns projections (Cechetto et al., 1985; Hylden et al., 1985). Other connectional studies demonstrated that the PBN innervated by several areas of the brain, such as the ventral thalamus, insular cortex, limbic cortex, central nucleus of the amygdala, bed nucleus of the stria terminalis, and hypothalamus (Saper and Loewy, 1980; Fulwiler and Saper, 1984; Bernard et al., 1993; Bester et al., 1997; Krout and Loewy, 2000; Grady et al., 2020).
Regarding retinal innervation, retrograde labeling techniques show that there is discrete and exclusive retinal input in the mPBN of marmosets (Figure 4; Engelberth et al., 2008). This pattern is different from all mammalians studied so far, in which their projection appears to involve the lPBN (Fite and Janusonis, 2002). It is speculated that this hodological variation in retinal innervation of the PBN of marmosets could have a functional partition, a characteristic that rodents apparently do not exhibit (Engelberth et al., 2008). To our knowledge, no comparative or phylogenetic study evidenced whether the retina-mPBN circuitry is a general primate attribute or just a species-specific feature. Furthermore, the functional role of the retina-PBN pathway has also not been clarified in marmosets. Engelberth et al. (2008) suggest that this connectional pattern may represent a photic integration node and viscerosensory stimuli to modulate visual processing.
6. Conclusion
We aimed to demonstrate the current state of knowledge on the IF and NIF circuitry of marmosets. The most studied structures of IF processing, in marmosets and other primates, are the DLG, PI, and SC. One the other hand, the SCN is a well-characterized NIF domain in all animals studied so far. The evidence, considered here for these nuclei, supports considerable progress made in understanding the retinal connectivity of marmosets in the past decades. Consequently, it can be considered an excellent non-human primate model to investigate the anatomy and function of the IF and NIF systems.
Besides the regions of intense research interest mentioned above, our knowledge regarding the IF and NIF networks in marmosets remains incomplete. In the case of IF midbrain structures; such as the pretectal complex; our limited knowledge reflects, in part, the difficulty in delineating the cytoarchitectonic boundaries of these nuclei and the fact that few publications describe the presence of retina-PTC pathways. Regarding NIF territories, the functional properties and phylogenetic significance of the retinal innervation in the mPBN and MIN of the marmoset are uncertain. Further comparative work is needed to solidify knowledge regarding the function and universality of these pathways.
It is important to note that little is known about the functionality and RGC types that innervate the retinorecipient nuclei of marmosets. Although the wide-field RGC classes have been reported by their projections to DLG, PI, and SC, their functional aspect remains opaque. In the case of all NIF territories, the retinal population is uncertain or merely hypothetical, particularly related to the function of the involved system, such as the CTS. Substantial morphological retinal studies combined with hodological techniques are needed to draw conclusions regarding the origin of retinal fibers in the NIF domains.
The two last decades have seen a rapid advancement in the establishment of robust protocols for viral tracers, computational pipelines, structural MRI, functional MRI, and genetic modifications among other important developments. We believe that these approaches could reveal the precise functional and connectional organization of retinorecipient areas in all species of vertebrates, including marmosets, with three-dimensional reconstruction of their retinal axonal projections and targets, from fetal to all aging levels.
Finally, we have noted that, although retinal connectivity has been a prominent focus for hodological research for years and impressive progress has been made in understanding its functionality, pivotal information is still absent, as mentioned throughout this review. These issues represent the next set of challenges for keeping this field relevant and for building essential tools to comprehend IF and NIF functions.
Author contributions
NS, ES, and RE conceived and wrote the manuscript with support from EN, MC, and SS. JC prepared and revised the manuscript critically. All authors contributed to the article and approved the submitted version.
Funding
This study was supported by funding from the National Council of Technological and Scientific Development (CNPq), Coordination for Improvement of High-Level Staff (CAPES), and Foundation for Scientific Development of Rio Grande do Norte (FAPERN).
Acknowledgments
We thank all former and current members of our laboratory and the Laboratory of Neuroanatomy from Federal University of Rio Grande do Norte, who contributed to the published work discussed in this review. Furthermore, this manuscript is dedicated to our colleague and friend, MC, for her research of retinal connectivity in mammalian brains, continued mentorship, and dedication to morphological neuroscience.
Conflict of interest
The authors declare that the research was conducted in the absence of any commercial or financial relationships that could be construed as a potential conflict of interest.
Publisher’s note
All claims expressed in this article are solely those of the authors and do not necessarily represent those of their affiliated organizations, or those of the publisher, the editors and the reviewers. Any product that may be evaluated in this article, or claim that may be made by its manufacturer, is not guaranteed or endorsed by the publisher.
Abbreviations
AOS, acessory optic system; CTb, cholera toxin subunit B; CTS, circadian timing system; DRN, dorsal raphe nucleus; DLG, dorsal lateral geniculate nucleus; IF, image-forming; IGL, intergeniculate leaflet; ipRGCs, intrinsically photosensitive retinal ganglion cells; K, koniocellular; K1, koniocellular layer 1; lPBN, lateral parabrachial nucleus; lSGS, lower stratum griseum superficiale; mPBN, medial parabrachial nucleus; M, magnocellular; MD, mediodorsal nucleus; MDmc, magnocellular mediodorsal nucleus; MDpc, parvocellular mediodorsal nucleus; MIN, midline and intralaminar nuclei; MnR, median raphe nucleus; MTN, medial terminal nucleus; NIF, non-image forming; NPY, neuropeptide Y; P, parvocellular; PACAP, pituitary adenylate cyclase-activating polypeptide; PBN, parabrachial nucleus; PGN, pregeniculate nucleus; PGNdol, pregeniculate nucleus dorsal outer lamina; PGNil, inner lamina of pregeniculate nucleus; PGNol, outer lamina of pregeniculate nucleus; PGNvol, pregeniculate nucleus ventral outer lamina; PI, pulvinar inferior; PIcl, central lateral nucleus of the inferior pulvinar; PIcm, central medial nucleus of the inferior pulvinar; PIm, medial nucleus of the inferior pulvinar; Pip, posterior nucleus of the inferior pulvinar; PVT, paraventricular nucleus of thalamus; RGCs, retinal ganglion cells; SC, superior colliculus; SCN, suprachiasmatic nucleus; SGS, stratum griseum superficiale; SO, stratum opticum; SZ, stratum zonale; uSGS, upper stratum griseum superficiale; V1, primary visual cortex; VLG, ventral lateral geniculate nucleus.
References
Abe, H., Tani, T., Mashiko, H., Kitamura, N., Miyakawa, N., Mimura, K., et al. (2017). 3rd reconstruction of brain section images for creating axonal projection maps in marmosets. J. Neurosci. Methods 286, 102–113. doi: 10.1016/j.jneumeth.2017.04.016
Abizaid, A., Horvath, B., Keefe, D. L., Leranth, C., and Horvath, T. L. (2004). Direct visual and circadian pathways target neuroendocrine cells in primates. Eur. J. Neurosci. 20, 2767–2776. doi: 10.1111/j.1460-9568.2004.03737.x
Ackman, J. B., Burbridge, T. J., and Crair, M. C. (2012). Retinal waves coordinate patterned activity throughout the developing visual system. Nature 490, 219–225. doi: 10.1038/nature11529
Acuña, C., Gonzalez, F., and Dominguez, R. (1983). Sensorimotor unit activity related to intention in the pulvinar of behaving Cebus apella monkeys. Exp. Brain Res. 52, 411–422. doi: 10.1007/BF00238034
Adams, M. M., Hof, P. R., Gattass, R., Webster, M. J., and Ungerleider, L. G. (2000). Visual cortical projections and chemoarchitecture of macaque monkey pulvinar. J. Comp. Neurol. 419, 377–393.
Aggleton, J. P., Desimone, R., and Mishkin, M. (1986). The origin, course, and termination of the hippocampothalamic projections in the macaque. J. Comp. Neurol. 243, 409–421. doi: 10.1002/cne.902430310
Aggleton, J. P., and Mishkin, M. (1984). Projections of the amygdala to the thalamus in the cynomolgus monkey. J. Comp. Neurol. 222, 56–68. doi: 10.1002/cne.902220106
Allali, K. E., Achaâban, M. R., Piro, M., Ouassat, M., Challet, E., and Errami, M. (2017). The suprachiasmatic nucleus of the dromedary camel (Camelus dromedarius): Cytoarchitecture and neurochemical anatomy. Front. Neuroat. 11:103. doi: 10.3389/fnana.2017.00103
Assali, A., Gaspar, P., and Rebsam, A. (2014). Activity dependent mechanisms of visual map formation - from retinal waves to molecular regulators. Semin. Cell Dev. Biol. 35, 136–146. doi: 10.1016/j.semcdb.2014.08.008
Astiz, M., Heyde, I., and Oster, H. (2019). Mechanisms of communication in the mammalian circadian timing system. Int. J. Mol. Sci. 20:343. doi: 10.3390/ijms20020343
Atapour, N., Majka, P., Wolkowicz, I. H., Malamanova, D., Worthy, K. H., and Rosa, M. G. P. (2018). Neuronal distribution across the cerebral cortex of the marmoset monkey (Callithrix jacchus). Cereb. Cortex 29, 3836–3863. doi: 10.1093/cercor/bhy263
Babb, R. S. (1980). The pregeniculate nucleus of the monkey (Macaca mulatta). IA study at the light microscopy level. J. Comp. Neurol. 190, 651–672. doi: 10.1002/cne.901900404
Bachevalier, J., Meunier, M., Lu, M. X., and Ungerleider, L. G. (1997). Thalamic and temporal cortex input to medial prefrontal cortex in rhesus monkeys. Exp. Brain Res. 115, 430–444. doi: 10.1007/pl00005713
Baden, T., Berens, P., Franke, K., Roman Roson, M., Bethge, M., and Euler, T. (2016). The functional diversity of mouse retinal ganglion cells. Nature 529, 345–350. doi: 10.1038/nature16468
Bakola, S., Burman, K. J., Bednarek, S., Chan, J. M., Jermakow, N., Worthy, K., et al. (2021). Afferent connections of cytoarchitectural area 6m and surrounding cortex in the marmoset: Putative homologues of the supplementary and pre-supplementary motor areas. Cereb. Cortex 193, 41–62. doi: 10.1093/cercor/bhab193
Balaram, P., Hackett, T. A., and Kaas, J. H. (2013). Differential expression of vesicular glutamate transporters 1 and 2 may identify distinct modes of glutamatergic transmission in the macaque visual system. J. Chem. Neuroanat. 50, 21–38. doi: 10.1016/j.jchemneu.2013.02.007
Baldwin, M. K., and Bourne, J. A. (2017). “The evolution of subcortical pathways to the extrastriate cortex,” in The evolution of nervous systems, ed. J. H. Kaas (Oxford: Academic Press), 165–185.
Baldwin, M. K. L., Balaram, P., and Kaas, J. H. (2013). Projections of the superior colliculus to the pulvinar in prosimian galagos (Otolemur garnettii) and VGLUT2 staining of the visual pulvinar. J. Comp. Neurol. 521, 1664–1682. doi: 10.1002/cne.23252
Baldwin, M. K. L., and Krubitzer, L. (2018). Architectonic characteristics of the visual thalamus and superior colliculus in titi monkeys. J. Comp. Neurol. 526, 1760–1776. doi: 10.1002/cne.24445
Baldwin, M. K. L., Wong, P., Reed, J. L., and Kaas, J. H. (2011). Superior colliculus connections with visual thalamus in gray squirrels (Sciurus carolinensis): Evidence for four subdivisions within the pulvinar complex. J. Comp. Neurol. 519, 1071–1094. doi: 10.1002/cne.22552
Baleydier, C., and Morel, A. N. N. E. (1992). Segregated thalamocortical pathways to inferior parietal and inferotemporal cortex in macaque monkey. Vis. Neurosci. 8, 391–405. doi: 10.1017/s0952523800004922
Barbas, H., and Mesulam, M. M. (1981). Organization of afferent input to subdivisions of area 8 in the rhesus monkey. J. Comp. Neurol. 200, 407–431. doi: 10.1002/cne.902000309
Barik, A., Thompson, J. H., Seltzer, M., Ghitani, N., and Chesler, A. T. (2018). A brainstem-spinal circuit controlling nocifensive behavior. Neuron 100, 1491–1503. doi: 10.1016/j.neuron.2018.10.037
Barson, J. R., Mack, N. R., and Gao, W. J. (2020). The paraventricular nucleus of the thalamus is an important node in the emotional processing network. Front. Behav. Neurosci. 14:598469. doi: 10.3389/fnbeh.2020.598469
Basso, M. A., and May, P. J. (2017). Circuits for action and cognition: A view from the superior colliculus. Annu. Rev. Vis. Sci. 3, 197–226. doi: 10.1146/annurev-vision-102016-061234
Belluccini, E., Zeater, N., Pietersen, A. N. J., Eiber, C. D., and Martin, P. R. (2019). Binocular summation in marmoset lateral geniculate nucleus. Vis. Neurosci. 36:E012. doi: 10.1017/s0952523819000099
Benarroch, E. E. (2012). Periaqueductal gray: An interface for behavioral control. Neurology 78, 210–217. doi: 10.1212/WNL.0b013e31823fcdee
Benassi, C., Biral, G. P., Lui, F., Porro, C. A., and Corazza, R. (1989). The interstitial nucleus of the superior fasciculus, posterior bundle (INSFp) in the guinea pig: Another nucleus of the accessory optic system processing the vertical retinal slip signal. Vis. Neurosci. 2, 377–382. doi: 10.1017/s0952523800002182
Bender, D. B. (1981). Retinotopic organization of macaque pulvinar. J. Neurophysiol. 46, 682–693. doi: 10.1152/jn.1981.46.3.672
Bender, D. B. (1982). Receptive-field properties of neurons in the macaque inferior pulvinar. J. Neurophysiol. 48, 1–17. doi: 10.1152/jn.1982.48.1.1
Benevento, L. A., and Standage, G. P. (1983). The organization of projections of the retinorecipient and nonretinorecipient nuclei of the pretectal complex and layers of the superior colliculus to the lateral pulvinar and medial pulvinar in the macaque monkey. J. Comp. Neurol. 217, 307–336. doi: 10.1002/cne.902170307
Bentivoglio, M., Balercia, G., and Kruger, L. (1991). The specificity of the nonspecific thalamus: The midline nuclei. Prog. Brain Res. 87, 53–80. doi: 10.1016/s0079-6123(08)63047-2
Bentivoglio, M., Kultas-Ilinsky, K., and Ilinsky, I. (1993). “Limbic thalamus: Structure, intrinsic organization, and connections,” in Neurobiology of cingulate cortex and limbic thalamus, eds B. A. Vogt and M. Gabriel (Boston, MA: Birkhäuser), 71–122.
Bernard, J. F., Alden, M., and Besson, J. M. (1993). The organization of the efferent projections from the pontine parabrachial area to the amygdaloid complex: A Phaseolus vulgaris leucoagglutinin (PHA-L) study in the rat. J. Comp. Neurol. 329, 201–229. doi: 10.1002/cne.903290205
Berson, D. M., Castrucci, A. M., and Provencio, I. (2010). Morphology and mosaics of melanopsin-expressing retinal ganglion cell types in mice. J. Comp. Neurol. 518, 2405–2422. doi: 10.1002/cne.22381
Bertani, S., Carboni, L., Criado, A., Michielin, F., Mangiarini, L., and Vicentini, E. (2010). Circadian profile of peripheral hormone levels in Sprague-Dawley rats and in common marmosets (Callithrix jacchus). In Vivo 24, 827–836.
Bester, H., Besson, J. M., and Bernard, J. F. (1997). Organization of efferent projections from the parabrachial area to the hypothalamus: A Phaseolus vulgaris-leucoagglutinin study in the rat. J. Comp. Neurol. 383, 245–281. doi: 10.1002/(sici)1096-9861(19970707)383:3<245::aid-cne1>3.0.co;2-3
Bhatnagar, S., and Dallman, M. F. (1999). The paraventricular nucleus of the thalamus alters rhythms in core temperature and energy balance in a state-dependent manner. Brain Res. 851, 66–75. doi: 10.1016/s0006-8993(99)02108-3
Bickford, M. E. (2016). Thalamic circuit diversity: Modulation of the driver/modulator framework. Front. Neural Circuits 9:86. doi: 10.3389/fncir.2015.00086
Blanchard, D. C., and Blanchard, R. J. (1972). Innate and conditioned reactions to threat in rats with amygdaloid lesions. J. Comp. Physiol. Psychol. 81, 281–290. doi: 10.1037/h0033521
Bourne, J. A., and Rosa, M. G. P. (2003). Laminar expression of neurofilament protein in the superior colliculus of the marmoset monkey (Callithrix jacchus). Brain Res. 973, 142–145. doi: 10.1016/s0006-8993(03)02527-7
Brancaccio, M., Maywood, E. S., Chesham, J. E., Loudon, A. S., and Hastings, M. H. (2013). A Gq-Ca2+ axis controls circuit-level encoding of circadian time in the suprachiasmatic nucleus. Neuron 78, 714–728. doi: 10.1016/j.neuron.2013.03.011
Bridge, H., Leopold, D. A., and Bourne, J. A. (2016). Adaptive pulvinar circuitry supports visual cognition. Trends Cogn. Sci. 20, 146–157. doi: 10.1016/j.tics.2015.10.003
Brodsky, M. C. (2012). The accessory optic system: The fugitive visual control system in infantile strabismus. Arch. Ophthalmol. 130, 1055–1058. doi: 10.1001/archophthalmol.2011.2888
Buijs, F. N., Guzmán-Ruiz, M., León-Mercado, L., Basualdo, M. C., Escobar, C., Kalsbeek, A., et al. (2017). Suprachiasmatic nucleus interaction with the arcuate nucleus; essential for organizing physiological rhythms. Eneuro 4:ENEURO.0028-17.2017. doi: 10.1523/ENEURO.0028-17.201
Buijs, R. M., Kalsbeek, A., van der Woude, T. P., van Heerikhuize, J. J., and Shinn, S. (1993). Suprachiasmatic nucleus lesion increases corticosterone secretion. Am. J. Physiol. 264, R1186–R1192. doi: 10.1152/ajpregu.1993.264.6.r1186
Burman, K. J., Bakola, S., Richardson, K. E., Yu, H., Reser, D. H., and Rosa, M. G. P. (2015). Cortical and thalamic projections to cytoarchitectural areas 6Va and 8C of the marmoset monkey: Connectionally distinct subdivisions of the lateral premotor cortex. J. Comp. Neurol. 523, 1222–1247. doi: 10.1002/cne.23734
Büttner, U., and Fuchs, A. F. (1973). Influence of saccadic eye movements on unit activity in simian lateral geniculate and pregeniculate nuclei. J. Neurophysiol. 36, 127–141. doi: 10.1152/jn.1973.36.1.127
Büttner-Ennever, J. A., Cohen, B., Horn, A. K. E., and Reisine, H. (1996b). Pretectal projections to the oculomotor complex of the monkey and their role in eye movements. J. Comp. Neurol. 366, 348–359. doi: 10.1002/(SICI)1096-9861(19960304)366:2<348::AID-CNE12>3.0.CO;2-L
Büttner-Ennever, J. A., Cohen, B., Horn, A. K. E., and Reisine, H. (1996a). Efferent pathways of the nucleus of the optic tract in monkey and their role in eye movements. J. Comp. Neurol. 373, 90–107.
Callaway, E. M. (2005). Structure and function of parallel pathways in the primate early visual system. J. Physiol. 566, 13–19. doi: 10.1113/jphysiol.2005.088047
Campbell, C. B. G. (1969). The visual system of insectivores and primates. Ann. N. Y. Acad. Sci. 167, 388–403. doi: 10.1111/j.1749-6632.1969.tb20458.x
Campos-Ortega, J. A., and Hayhow, W. R. (1972). On the organization of the visual cortical projection to the pulvinar in Macaca mulatta. Brain Behav. Evol. 6, 394–423. doi: 10.1159/000123725
Cang, J., and Feldheim, D. A. (2013). Developmental mechanisms of topographic map formation and alignment. Annu. Rev. Neurosci. 36, 51–77. doi: 10.1146/annurev-neuro-062012-170341
Canteras, N. S., Ribeiro-Barbosa, E. R., Goto, M., Cipolla-Neto, J., Larry, W., and Swanson, L. W. (2011). The retinohypothalamic tract: Comparison of axonal projection patterns from four major targets. Brain Res. Rev. 65, 150–183. doi: 10.1016/j.brainresrev.2010.09.006
Card, J. P., and Moore, R. Y. (1984). The suprachiasmatic nucleus of the golden hamster: Immunohistochemical analysis of cell and fiber distribution. Neuroscience 13, 415–431. doi: 10.1016/0306-4522(84)90240-9
Casagrande, V. A., Harting, J. K., Hall, W. C., Diamond, I. T., and Martin, G. F. (1972). Superior colliculus of the tree shrew: A structural and functional subdivision into superficial and deep layers. Science 177, 444–447. doi: 10.1126/science.177.4047.444
Casagrande, V. A., and Kaas, J. H. (1994). “The afferent, intrinsic and efferent connections of primary visual cortex in primates,” in Primary visual cortex, eds A. Peters and K. S. Rockland (New York, NY: Plenum), 201–259. doi: 10.1523/JNEUROSCI.2069-15.2015
Casagrande, V. A., and Khaytin, I. (2009). “The evolution of parallel pathways in the brains of primates,” in Evolution of nervous systems, ed. J. H. Kaas (Oxford: Academic Press), 871–892.
Casagrande, V. A., and Norton, T. T. (1991). “Lateral geniculate nucleus: A review of its physiology and function,” in The neural basis of visual function: Vision and visual dysfunction, ed. A. Leventhal (London: Macmillan Press), 41–84.
Casagrande, V. A., Yazar, F., Jones, K. D., and Ding, Y. (2007). The morphology of the koniocellular axon pathway in the macaque monkey. Cereb. Cortex 17, 2334–2345. doi: 10.1093/cercor/bhl142
Cassone, V. M., Speh, J. C., Card, J. P., and Moore, R. Y. (1988). Comparative anatomy of the mammalian hypothalamic suprachiasmatic nucleus. J. Biol. Rhythms 3, 71–91. doi: 10.1177/074873048800300106
Cavalcante, J. S., Costa, S. M. M. O., Santee, U. R., and Britto, L. G. R. (2005). Retinal projections to the midline and intralaminar thalamic nuclei in the common marmoset (Callithrix jacchus). Brain Res. 1043, 42–47. doi: 10.1016/j.brainres.2005.02.035
Cechetto, D. F., Standaert, D. G., and Saper, C. B. (1985). Spinal and trigeminal dorsal horn projections to the parabrachial nucleus in the rat. J. Comp. Neurol. 240, 153–160. doi: 10.1002/cne.902400205
Chalupa, L. M., Coyle, R. S., and Lindsley, D. B. (1976). Effect of pulvinar lesions on visual pattern discrimination in monkeys. J. Neurophysiol. 39, 354–369. doi: 10.1152/jn.1976.39.2.354
Chalupa, L. M., and Williams, R. W. (1984). Organization of the cat’s lateral geniculate nucleus following interruption of prenatal binocular competition. Hum. Neurobiol. 3, 103–107.
Chan, T. L., Martin, P. R., Clunas, N., and Grünert, U. (2001). Bipolar cell diversity in the primate retina: Morphologic and immunocytochemical analysis of a new world monkey, the marmoset Callithrix jacchus. J. Comp. Neurol. 437, 219–239. doi: 10.1002/cne.1280
Chaplin, T. A., Yu, H. H., Soares, J. G., Gattass, R., and Rosa, M. G. P. (2013). A conserved pattern of differential expansion of cortical areas in simian primates. J. Neurosci. 33, 15120–15125. doi: 10.1523/JNEUROSCI.2909-13.2013
Chen, C., and Regehr, W. G. (2000). Developmental remodeling of the retinogeniculate synapse. Neuron 28, 955–966. doi: 10.1016/S0896-6273(00)00166-5
Cheong, S. K., Tailby, C., Solomon, S. G., and Martin, P. R. (2013). Cortical-like receptive fields in the lateral geniculate nucleus of marmoset monkeys. J. Neurosci. 33, 6864–6876. doi: 10.1523/JNEUROSCI.5208-12.2013
Chevassus-Au-Louis, N., and Cooper, H. M. (1998). Is there a geniculohypothalamic tract in primates? A comparative immunohistochemical study in the circadian system of strepsirhine and haplorhine species. Brain Res. 805, 213–219. doi: 10.1016/s0006-8993(98)00741-0
Chiang, M. C., Bowen, A., Schier, L. A., Tupone, D., Uddin, O., and Heinricher, M. M. (2019). Parabrachial complex: A hub for pain and aversion. J. Neurosci. 39, 8225–8230. doi: 10.1523/JNEUROSCI.1162-19.2019
Chong, M. H. Y., Worthy, K. H., Rosa, M. G. P., and Atapour, N. (2022). Neuronal density and expression of calcium-binding proteins across the layers of the superior colliculus in the common marmoset (Callithrix jacchus). J. Comp. Neurol. 530, 2966–2976. doi: 10.1002/cne.25388
Ciarleglio, C. M., Resuehr, H. E. S., and McMahon, D. G. (2011). Interactions of the serotonin and circadian systems: Nature and nurture in rhythms and blues. Neuroscience 197, 8–16. doi: 10.1016/j.neuroscience.2011.09.036
Cipolla-Neto, J., Bartol, I., Seraphim, P. M., Afeche, S. C., Scialfa, J. H., and Peraçoli, A. M. (1995). The effects of lesions of the thalamic intergeniculate leaflet on the pineal metabolism. Brain Res. 691, 133–141. doi: 10.1016/0006-8993(95)00654-9
Cleland, B. G., Dubin, M. W., and Levick, W. R. (1971). Sustained and transient neurons in the cat’s retina and lateral geniculate nucleus. J. Physiol. 217, 473–496. doi: 10.1113/jphysiol.1971.sp009581
Clement, G., and Magnin, M. (1984). Effects of accessory optic system lesions on vestibule-ocular and optokinetic reflexes in the cat. Exp. Brain Res. 55, 49–59. doi: 10.1007/BF00240497
Cohen-Cory, S. (2002). The developing synapse: Construction and modulation of synaptic structures and circuits. Science 298, 770–776. doi: 10.1126/science.1075510
Cola, M. G., Gray, D. N., Seltzer, B., and Cusick, C. G. (1999). Human thalamus: Neurochemical mapping of inferior pulvinar complex. Neuroreport 10, 3733–3738. doi: 10.1097/00001756-199912160-00002
Colavito, V., Tesoriero, C., Wirtu, A. T., Grassi-Zucconi, G., and Bentivoglio, M. (2015). Limbic thalamus and state-dependent behavior: The paraventricular nucleus of the thalamic midline as a node in circadian timing and sleep/wake-regulatory networks. Neurosci. Biobehav. Rev. 54, 3–17. doi: 10.1016/j.neubiorev.2014.11.021
Conley, M., Lachica, E. A., and Casagrande, V. A. (1985). Demonstration of ipsilateral retinocollicular projections in the tree shrew (Tupaia glis). Brain Res. 346, 181–185. doi: 10.1016/0006-8993(85)91113-8
Cooper, H. M. (1986). The accessory optic system in a prosimian primate (Microcebus murinus): Evidence for a direct retinal projection to the medial terminal nucleus. J. Comp. Neurol. 249, 28–47. doi: 10.1002/cne.902490104
Cooper, H. M., and Magnin, M. (1986). A common mammalian plan of accessory optic system organization revealed in all primates. Nature 324, 457–459. doi: 10.1038/324457a0
Cooper, H. M., and Magnin, M. (1987). Accessory optic system of an anthropoid primate, the gibbon (Hylobates concolor): Evidence of a direct retinal input to the medial terminal nucleus. J. Comp. Neurol. 259, 467–482. doi: 10.1002/cne.902590402
Cornwall, J., and Phillipson, O. T. (1988). Afferent projections to the dorsal thalamus of the rat as shown by retrograde lectin transport. II. The midline nuclei. Brain Res. Bull. 21, 147–161. doi: 10.1016/0361-9230(88)90227-4
Costa, M. S. M. O., and Britto, L. R. (1997). Calbindin immunoreactivity delineates the circadian visual centers of the brain of the common marmoset (Callithrix jacchus). Brain Res. Bull. 43, 369–373. doi: 10.1016/s0361-9230(96)00378-4
Costa, M. S. M. O., Moreira, L. F., Alones, V., Lu, J., Santee, U. R., Cavalcante, J. S., et al. (1998). Characterization of the circadian system in a Brazilian species of monkey (Callithrix jacchus): Immunohistochemical analysis and retinal projections. Biol. Rhythm Res. 29, 510–520. doi: 10.1076/brhm.29.5.510.4829
Costa, M. S. M. O., Santee, U. S., Cavalcante, J. S., Moraes, P. R. A., Santos, N. P., and Britto, L. R. G. (1999). Retinohypothalamic projections in the common marmoset (Callithrix jacchus): A study using cholera toxin subunit B. J. Comp. Neurol. 415, 393–403. doi: 10.1002/(sici)1096-9861(19991220)415:3<393::aid-cne5>3.0.co;2-r
Courtiol, E., and Wilson, D. A. (2014). Thalamic olfaction: Characterizing odor processing in the mediodorsal thalamus of the rat. J. Neurophysiol. 111, 1274–1285. doi: 10.1152/jn.00741.2013
Courtiol, E., and Wilson, D. A. (2015). The olfactory thalamus: Unanswered questions about the role of the mediodorsal thalamic nucleus in olfaction. Front. Neural Circuits 9:49. doi: 10.3389/fncir.2015.00049
Cowey, A., Johnson, H., and Stoerig, P. (2001). The retinal projection to the pregeniculate nucleus in normal and destriate monkeys. Eur. J. Neurosci. 13, 279–290. doi: 10.1046/j.0953-816x.2000.01389.x
Cowey, A., Stoerig, P., and Bannister, M. (1994). Retinal ganglion cells labelled from the pulvinar nucleus in macaque monkeys. Neuroscience 61, 691–705. doi: 10.1016/0306-4522(94)90445-6
Cusick, C. G., Scripter, J. L., Darensbourg, J. G., and Weber, J. T. (1993). Chemoarchitectonic subdivisions of the visual pulvinar in monkeys and their connectional relations with the middle temporal and rostral dorsolateral visual areas, MT and DLr. J. Comp. Neurol. 336, 1–30. doi: 10.1002/cne.903360102
Cynader, M., and Berman, N. (1972). Receptive-field organization of monkey superior colliculus. J. Neurophysiol. 35, 187–201. doi: 10.1152/jn.1972.35.2.187
Dacey, D. M. (2004). “Origins of perception: Retinal ganglion cell diversity and the creation of parallel visual pathways,” in The cognitive neurosciences, ed. M. S. Gazzaniga (Cambridge, MA: MIT Press), 281–301.
Dacey, D. M., Liao, H. W., Peterson, B. B., Robinson, F. R., Smith, V. C., Pokorny, J., et al. (2005). Melanopsin-expressing ganglion cells in primate retina signal colour and irradiance and project to the LGN. Nature 433, 749–754. doi: 10.1038/nature03387
Dan, Y., Atick, J. J., and Reid, R. C. (1996). Efficient coding of natural scenes in the lateral geniculate nucleus: Experimental test of a computational theory. J. Neurosci. 16, 3351–3362. doi: 10.1523/JNEUROSCI.16-10-03351
Daneault, V., Dumont, M., Massé, É, Vandewalle, G., and Carrier, J. (2016). Light-sensitive brain pathways and aging. J. Physiol. Anthropol. 35:9. doi: 10.1186/s40101-016-0091-9
Dann, J. F., and Buhl, E. H. (1987). Retinal ganglion cells projecting to the accessory optic system in the rat. J. Comp. Neurol. 262, 141–158. doi: 10.1002/cne.902620111
Dardente, H., Poirel, V. J., Klosen, P., Pévet, P., and Masson-Pévet, M. (2002). Per and neuropeptide expression in the rat suprachiasmatic nuclei: Compartmentalization and differential cellular induction by light. Brain Res. 958, 261–271. doi: 10.1016/s0006-8993(02)03563-1
Dark, J. G., and Asdourian, D. (1975). Entrainment of the rat’s activity rhythm by cyclic light following lateral geniculate nucleus lesions. Physiol. Behav. 15, 295–301. doi: 10.1016/0031-9384(75)90097-9
Davern, P. J. (2014). A role for the lateral parabrachial nucleus in cardiovascular function and fluid homeostasis. Front. Physiol. 5:436. doi: 10.3389/fphys.2014.00436
de Góis Morais, P. L. A., Santana, M. A. D., Cavalcante, J. C., Costa, M. S. M. O., Cavalcante, J. S., and Nascimento, E. S. Jr. (2014). Retinal projections into the Zona Incerta of the rock cavy (Kerodon rupestris): A CTb study. Neurosci. Res. 89, 75–80. doi: 10.1016/j.neures.2014.08.016
de la Mothe, L. A., Blumell, S., Kajikawa, Y., and Hackett, T. A. (2006). Thalamic connections of auditory cortex in marmoset monkeys: Core and medial belt regions. J. Comp. Neurol. 496, 27–71. doi: 10.1002/cne.20924
de la Mothe, L. A., Blumell, S., Kajikawa, Y., and Hackett, T. A. (2012). Thalamic connections of auditory cortex in marmoset monkeys: Lateral belt and parabelt regions. Anat. Rec. 295, 822–836. doi: 10.1002/ar.22454
De Oca, B. M., DeCola, J. P., Maren, S., and Fanselow, M. S. (1998). Distinct regions of the periaqueductal gray are involved in the acquisition and expression of defensive responses. J. Neurosci. 18, 3426–3432. doi: 10.1523/JNEUROSCI.18-09-03426.1998
de Sousa, A. A., Sherwood, C. C., Hof, P. R., and Zilles, K. (2013). Lamination of the lateral geniculate nucleus of catarrhine primates. Brain Behav. Evol. 81, 93–108. doi: 10.1159/000346495
de Sousa, T. B., de Santana, M. A. D., de Medeiros Silva, A., Guzen, F. P., Oliveira, F. G., Cavalcante, J. C., et al. (2013). Mediodorsal thalamic nucleus receives a direct retinal input in marmoset monkey (Callithrix jacchus): A subunit B cholera toxin study. Ann. Anat. 195, 32–38. doi: 10.1016/j.aanat.2012.04.005
Dhande, O. S., and Huberman, A. D. (2014). Retinal ganglion cell maps in the brain: Implications for visual processing. Curr. Opin. Neurobiol. 24, 133–142. doi: 10.1016/j.conb.2013.08.006
Dibner, C., Schibler, U., and Albrecht, U. (2010). The mammalian circadian timing system: Organization and coordination of central and peripheral clocks. Annu. Rev. Physiol. 72, 517–549. doi: 10.1146/annurev-physiol-021909-135821
Do, M. T., and Yau, K. W. (2010). Intrinsically photosensitive retinal ganglion cells. Physiol. Rev. 90, 1547–1581. doi: 10.1152/physrev.00013.2010
Dougherty, K., Schmid, M. C., and Maier, A. (2019). Binocular response modulation in the lateral geniculate nucleus. J. Comp. Neurol. 527, 522–534. doi: 10.1002/cne.24417
D’Souza, S. P., Swygart, D. I., Wienbar, S. R., Upton, B. A., Zhang, K. X., Mackin, R. D., et al. (2021). Retinal patterns and the cellular repertoire of neuropsin (Opn5) retinal ganglion cells. J. Comp. Neurol. 530, 1247–1262. doi: 10.1002/cne.25272
Eiber, C. D., Rahman, A. S., Pietersen, A. N., Zeater, N., Dreher, B., Solomon, S. G., et al. (2018). Receptive field properties of koniocellular on/off neurons in the lateral geniculate nucleus of marmoset monkeys. J. Neurosci. 38, 10384–10398. doi: 10.1523/JNEUROSCI.1679-18.2018
Eliades, E. J., and Miller, C. T. (2017). Marmoset vocal communication: Behavior and neurobiology. Dev. Neurobiol. 77, 286–299. doi: 10.1002/dneu.22464
Ellis, E. M., Gauvain, G., Sivyer, B., and Murphy, G. J. (2016). Shared and distinct retinal input to the mouse superior colliculus and dorsal lateral geniculate nucleus. J. Neurophysiol. 116, 602–610. doi: 10.1152/jn.00227.2016
Engelberth, R. C. G. J., de Pontes, A. L. B., do Nascimento, R. B. S., de Lima, R. R. M., de Lima, R. R., de Toledo, C. A. B., et al. (2008). Discrete retinal input to the parabrachial complex of a new-world primate, the common marmoset (Callithrix jacchus). Neurosci. Lett. 443, 99–103. doi: 10.1016/j.neulet.2008.07.065
Erickson, S. L., Melchitzky, D. S., and Lewis, D. A. (2004). Subcortical afferents to the lateral mediodorsal thalamus in cynomolgus monkeys. Neuroscience 129, 675–690. doi: 10.1016/j.neuroscience.2004.08.016
Eriköz, B., Jusuf, P. R., Percival, K. A., and Grünert, U. (2008). Distribution of bipolar input to midget and parasol ganglion cells in marmoset retina. Vis. Neurosci. 25, 67–76. doi: 10.1017/S0952523808080073
Erkert, H. G. (1989). Characteristics of the circadian activity rhythm in common marmoset (Callithrix j. jacchus). Am. J. Primatol. 17, 271–286. doi: 10.1002/ajp.1350170403
Erulkar, S. D., and Fillenz, M. (1960). Single-unit activity in the lateral geniculate body of the cat. J. Physiol. 154, 206–218. doi: 10.1113/jphysiol.1960.sp006574
Estevez, M. E., Fogerson, P. M., Ilardi, M. C., Borghuis, B. G., Chan, E., Weng, S., et al. (2012). Form and function of the M4 cell, an intrinsically photosensitive retinal ganglion cell type contributing to geniculocortical vision. J. Neurosci. 32, 13608–13620. doi: 10.1523/JNEUROSCI.1422-12.2012
Euler, T., Haverkamp, S., Schubert, T., and Baden, T. (2014). Retinal bipolar cells: Elementary building blocks of vision. Nat. Rev. Neurosci. 15, 507–519. doi: 10.1038/nrn3783
Evans, J. A., Suen, T. C., Callif, B. L., Mitchell, A. S., Castanon-Cervantes, O., and Baker, K. M. (2015). Shell neurons of the master circadian clock coordinate the phase of tissue clocks throughout the brain and body. BMC Biol. 13:43. doi: 10.1186/s12915-015-0157-x
Farmer, S. G., and Rodieck, R. W. (1982). Ganglion cells of the cat accessory optic system: Morphology and retinal topography. J. Comp. Neurol. 205, 190–198. doi: 10.1002/cne.902050210
Farrow, K., Isa, T., Luksch, H., and Yonehara, K. (2019). The superior colliculus/tectum: Cell types, circuits, computations, behaviors. Front. Neural Circuits 13:39. doi: 10.3389/fncir.2019.00039
Faull, O. K., Subramanian, H. H., Ezra, M., and Pattinson, K. T. S. (2019). The midbrain periaqueductal gray as an integrative and interoceptive neural structure for breathing. Neurosci. Biobehav. Rev. 98, 135–144. doi: 10.1016/j.neubiorev.2018.12.020
Finger, A. M., and Kramer, A. (2021). Mammalian circadian systems: Organization and modern life challenges. Acta Physiol. 231:e13548. doi: 10.1111/apha.13548
Fite, K. V., and Janusonis, S. (2002). Optic afferents to the parabrachial nucleus. Brain Res. 943, 9–14. doi: 10.1016/s0006-8993(02)02421-6
Fite, K. V., Janusonis, S., Foote, W. E., and Bengston, L. (1999). Retinal afferents to the dorsal raphe nucleus in rats and Mongolian gerbils. J. Comp. Neurol. 414, 469–484. doi: 10.1002/(sici)1096-9861(19991129)414:4<469::aid-cne4>3.0.co;2-p
Fitzpatrick, D., Itoh, K. A. Z. U. O., and Diamond, I. T. (1983). The laminar organization of the lateral geniculate body and the striate cortex in the squirrel monkey (Saimiri sciureus). J. Neurosci. 3, 673–702. doi: 10.1523/JNEUROSCI.03-04-00673.1983
Foote, W. E., Taber-Pierce, E., and Edwards, L. (1978). Evidence for a retinal projection to the midline raphe of cat. Brain Res. 156, 135–140. doi: 10.1016/0006-8993(78)90089-6
Fredericks, C. A., Giolli, R. A., Blanks, R. H., and Sadun, A. A. (1988). The human accessory optic system. Brain Res. 454, 116–122. doi: 10.1016/0006-8993(88)90809-8
Froesel, M., Cappe, C., and Ben Hamed, S. (2021). A multisensory perspective onto primate pulvinar functions. Neurosci. Biobehav. Rev. 125, 231–243. doi: 10.1016/j.neubiorev.2021.02.043
Fu, Y., Liao, H. W., Do, M. T., and Yau, K. W. (2005). Non-image forming ocular photoreception in vertebrates. Curr. Opin. Neurobiol. 15, 415–422. doi: 10.1016/j.conb.2005.06.011
Fukushima, M., Ichinohe, N., and Okano, H. (2019). “Neuroanatomy of the marmoset,” in The common marmoset in captivity and biomedical research, eds R. Marini, L. Watchman, S. Tardiff, D. Mansfield, and J. Fox (Cambridge, MA: Academic Press), 43–62. doi: 10.1016/B978-0-12-811829-0.00003-0
Fulwiler, C. E., and Saper, C. B. (1984). Subnuclear organization of the efferent connections of the parabrachial nucleus in the rat. Brain Res. Rev. 7, 229–259. doi: 10.1016/0165-0173(84)90012-2
Funahashi, S. (2013). Thalamic mediodorsal nucleus and its participation in spatial working memory processes: Comparison with the prefrontal cortex. Front. Syst. Neurosci. 7:36. doi: 10.3389/fnsys.2013.00036
Gaffan, D., and Parker, A. (2000). Mediodorsal thalamic function in scene memory in rhesus monkeys. Brain 123, 816–827. doi: 10.1093/brain/123.4.816
Gall, A. J., Smale, L., Yan, L., and Nun, A. A. (2013). Lesions of the intergeniculate leaflet lead to a reorganization in circadian regulation and a reversal in masking responses to photic stimuli in the Nile Grass Rat. PLoS One 8:e67387. doi: 10.1371/journal.pone.0067387
Gamlin, P. D. (2006). The pretectum: Connections and oculomotor-related roles. Prog. Brain Res. 151, 379–405. doi: 10.1016/S0079-6123(05)51012-4
Gandhi, N. J., and Katnani, H. A. (2011). Motor functions of the superior colliculus. Ann. Rev. Neurosci. 34, 205–231. doi: 10.1146/annurev-neuro-061010-113728
Gandhi, N. J., and Sparks, D. L. (2003). “Changing views of the role of superior colliculus in the control of gaze,” in The visual neurosciences, eds L. M. Chalupa and J. S. Werner (Cambridge, MA: MIT Press), 1449–1465.
Gattass, R., Oswaldo-Cruz, E., and Sousa, A. P. (1978). Visuotopic organization of the cebus pulvinar: A double representation the contralateral hemifield. Brain Res. 152, 1–16. doi: 10.1016/0006-8993(78)90130-0
Gauvain, G., and Murphy, G. J. (2015). Projection-specific characteristics of retinal input to the brain. J. Neurosci. 35, 6575–6583. doi: 10.1523/JNEUROSCI.4298
Ghahremani, M., Hutchison, R. M., Menon, R. S., and Everling, S. (2017). Frontoparietal functional connectivity in the common marmoset. Cereb. Cortex 27, 3890–3905. doi: 10.1093/cercor/bhw198
Ghosh, K. K., Goodchild, A. K., Sefton, A. E., and Martin, P. R. (1996). Morphology of retinal ganglion cells in a new world monkey, the marmoset Callithrix jacchus. J. Comp. Neurol. 366, 76–92. doi: 10.1002/(SICI)1096-9861(19960226)366:1<76::AID-CNE6>3.0.CO;2-H
Ghosh, K. K., and Grünert, U. (1999). Synaptic input to small bistratified (blue-ON) ganglion cells in the retina of a new world monkey, the marmoset Callithrix jacchus. J. Comp. Neurol. 413, 417–428. doi: 10.1002/(sici)1096-9861(19991025)413:3<417::aid-cne5>3.0.co;2-h
Gillett, G. R., and Webster, D. M. (1975). Mediodorsal nucleus and behavior regulation in the rat. Physiol. Behav. 14, 883–885. doi: 10.1016/0031-9384(75)90087-6
Gioia, M., Rodella, L., Petruccioli, M. G., and Bianchi, R. (2000). The cytoarchitecture of the adult human parabrachial nucleus: A Nissl and Golgi study. Arch. Histol. Cytol. 63, 411–424. doi: 10.1679/aohc.63.411
Giolli, R. A., Blanhs, R. H. I., and Lui, F. (2006). The accessory optic system: Basic organization with an update on connectivity, neurochemistry, and function. Prog. Brain Res. 151, 407–440. doi: 10.1016/S0079-6123(05)51013-6
Glass, J. D., Tardif, S. D., Clements, R., and Mrosovsky, N. (2001). Photic and non-photic circadian phase resenting in a diurnal primate, the common marmoset. Am. J. Physiol. 280, R191–R197. doi: 10.1186/1740-3391-3-10
Godement, P., Salaün, J., and Imbert, M. (1984). Prenatal and postnatal development of retinogeniculate and retinocollicular projections in the mouse. J. Comp. Neurol. 230, 552–575. doi: 10.1002/cne.902300406
Goel, N., Governale, M. M., Jechurab, T. J., and Leeb, T. M. (2000). Effects of intergeniculate leaflet lesions on circadian rhythms in Octodon degus. Brain Res. 877, 306–313. doi: 10.1016/s0166-4328(01)00264-9
Golden, E. C., Graff-Radford, J., Jones, D. T., and Benarroch, E. E. (2016). Mediodorsal nucleus and its multiple cognitive functions. Neurology 87, 2161–2168. doi: 10.1212/WNL.0000000000003344
Goldman-Rakic, P. S., and Porrino, L. J. (1985). The primate mediodorsal (MD) nucleus and its projection to the frontal lobe. J. Comp. Neurol. 242, 535–560.
Gomes, F. L., Silveira, L. C. L., Saito, C. A., and Yamada, E. S. (2005). Density, proportion, and dendritic coverage of retinal ganglion cells of the common marmoset (Callithrix jacchus jacchus). Braz. J. Med. Biol. Res. 38, 915–924. doi: 10.1590/S0100-879X2005000600014
Goodchild, A. K., Ghosh, K. K., and Martin, P. R. (1996). Comparison of photoreceptor spatial density and ganglion cell morphology in the retina of human, macaque monkey, cat, and the marmoset Callithrix jacchus. J. Comp. Neurol. 366, 55–75. doi: 10.1002/(SICI)1096-9861(19960226)366:1<55::AID-CNE5>3.0.CO;2-J
Grady, F., Peltekian, L., Iverson, G., and Geerling, J. C. (2020). Direct parabrachial-cortical connectivity. Cereb. Cortex 30, 4811–4833. doi: 10.1093/cercor/bhaa072
Gray, D., Gutierrez, C., and Cusick, C. G. (1999). Neurochemical organization of inferior pulvinar complex in squirrel monkeys and macaques revealed by acetylcholinesterase histochemistry, calbindin and Cat-301 immunostaining, and Wisteria floribunda agglutinin binding. J. Comp. Neurol. 409, 452–468. doi: 10.1002/(sici)1096-9861(19990705)409:3<452::aid-cne9>3.0.co;2-i
Gregory, K. M. (1985). The dendritic architecture of the visual pretectal nuclei of the rat: A study with the Golgi-Cox method. J. Comp. Neurol. 234, 122–135. doi: 10.1002/cne.90234011
Grieve, K. L. (2005). Binocular visual responses in cells of the rat dLGN. J. Physiol. 566, 119–124. doi: 10.1113/jphysiol.2005.090878
Groenewegen, H. J. (1988). Organization of the afferent connections of the mediodorsal thalamic nucleus in the rat, related to the mediodorsal-prefrontal topography. Neuroscience 24, 379–431. doi: 10.1016/0306-4522(88)90339-9
Groenewegen, H. J., and Berendse, H. W. (1994). The specificity of the ‘nonspecific’midline and intralaminar thalamic nuclei. Trends Neurosci. 17, 52–57. doi: 10.1016/0166-2236(94)90074-4
Groenewegen, H. J., Berendse, H. W., Wolters, J. G., and Lohman, A. H. (1990). The anatomical relationship of the prefrontal cortex with the striatopallidal system, the thalamus and the amygdala: Evidence for a parallel organization. Prog. Brain Res. 85, 95–116. doi: 10.1016/s0079-6123(08)62677-1
Grubb, M. S., and Thompson, I. D. (2003). Quantitative characterization of visual response properties in the mouse dorsal lateral geniculate nucleus. J. Neurophysiol. 90, 3594–3607. doi: 10.1152/jn.00699.2003
Grünert, U., Lee, S., Kwan, W. C., Mundinano, I. C., Bourne, J. A., and Martin, P. R. (2021). Retinal ganglion cells projecting to superior colliculus and pulvinar in marmoset. Brain Struct. Funct. 226, 2745–2762. doi: 10.1007/s00429-021-02295-8
Guido, W. (2008). Refinement of the retinogeniculate pathway. J. Physiol. 586, 4357–4362. doi: 10.1113/jphysiol.2008.157115
Guido, W. (2018). Development, form, and function of the mouse visual thalamus. J. Neurophysiol. 120, 211–225. doi: 10.1152/jn.00651.2017
Guillery, R. W. (1970). The laminar distribution of retinal fibers in the dorsal lateral geniculate nucleus of the cat: A new interpretation. J. Comp. Neurol. 138, 339–367. doi: 10.1002/cne.901380307
Guillery, R. W. (2005). Anatomical pathways that link perception and action. Prog. Brain Res. 149, 235–256. doi: 10.1016/S0079-6123(05)49017-2
Guillery, R. W., LaMantia, A. S., Robson, J. A., and Huang, K. (1985). The influence of retinal afferents upon the development of layers in the dorsal lateral geniculate nucleus of mustelids. J. Neurosci. 5, 1370–1379. doi: 10.1523/JNEUROSCI.05-05-01370.1985
Gutierrez, C., Yaun, A., and Cusick, C. G. (1995). Neurochemical subdivisions of the inferior pulvinar in macaque monkeys. J. Comp. Neurol. 363, 545–562. doi: 10.1002/cne.903630404
Hagan, M. A., Rosa, M. G., and Lui, L. L. (2017). Neural plasticity following lesions of the primate occipital lobe: The marmoset as an animal model for studies of blindsight. Dev. Neurobiol. 77, 314–327. doi: 10.1002/dneu.22426
Hall, W. C., and Moschovakis, A. (2004). The superior colliculus: New approaches for studying sensorimotor integration. Boca Raton, FL: CRC, 324.
Hannibal, J., Kankipati, L., Strang, C. E., Peterson, B. B., Dacey, D., and Gamlin, P. D. (2014). Central projections of intrinsically photosensitive retinal ganglion cells in the macaque monkey. J. Comp. Neurol. 522, 2231–2248. doi: 10.1002/cne.23555
Hansell, C. B., and Frank, M. F. (1991). Mapping study of the parabrachial taste-responsive area for the anterior tongue in the golden hamster. J. Comp. Neurol. 306, 708–722. doi: 10.1002/cne.903060412
Harrington, M. E., and Rusak, B. (1986). Lesions of the thalamic intergeniculate leaflet alter hamster circadian rhythms. J. Biol. Rhythms 1, 309–325. doi: 10.1177/074873048600100405
Harting, J. K., Hall, W. C., Diamond, I. T., and Martin, G. F. (1973). Anterograde degeneration study of the superior colliculus in Tupaia glis: Evidence for a subdivision between superficial and deep layers. J. Comp. Neurol. 148, 361–386. doi: 10.1002/cne.901480305
Harting, J. K., Huerta, M. F., Frankfurter, A. J., Strominger, N. L., and Royce, G. J. (1980). Ascending pathways from the monkey superior colliculus: An autoradiographic analysis. J. Comp. Neurol. 192, 853–882. doi: 10.1002/cne.901920414
Hastings, M. H., Maywood, E. S., and Brancaccio, M. (2018). Generation of circadian rhythms in the suprachiasmatic nucleus. Nat. Rev. Neurosci. 19, 453–469. doi: 10.1038/s41583-018-0026-z
Hastings, M. H., Maywood, E. S., and Brancaccio, M. (2019). The mammalian circadian timing system and the suprachiasmatic nucleus as its pacemaker. Biology 8:13. doi: 10.3390/biology8010013
Hattar, S., Kumar, M., Park, A., Tong, P., Tung, J., Yau, K., et al. (2006). Central projections of melanopsin-expressing retinal ganglion cells in the mouse. J. Comp. Neurol. 497, 326–349. doi: 10.1002/cne.20970
Hendrickson, A., Wilson, M. E., and Toyne, M. J. (1970). The distribution of optic nerve fibers in Macaca mulatta. Brain Res. 23, 425–427. doi: 10.1016/0006-8993(70)90068-5
Hendrickson, A. E., Wagoner, N., and Cowan, W. M. (1972). An autoradiographic and electron microscopic study of retino-hypothalamic connections. Cell Tissue Res. 135, 1–26. doi: 10.1007/BF00307084
Hendry, S. H., and Reid, R. C. (2000). The koniocellular pathway in primate vision. Annu. Rev. Neurosci. 23, 127–153. doi: 10.1146/annurev.neuro.23.1.127
Hendry, S. H. C., and Yoshioka, T. A. (1994). Neurochemically distinct third channel in the macaque dorsal lateral geniculate nucleus. Science 264, 575–577. doi: 10.1126/science.8160015
Hetherington, C. M. (1978). Circadian oscillations of body temperature in the marmoset Callithrix jacchus. Lab. Anim. 12, 107–108. doi: 10.1258/002367778780953215
Hofbauer, A., and Dräger, U. C. (1985). Depth segregation of retinal ganglion cells projecting to mouse superior colliculus. J. Comp. Neurol. 234, 465–474.
Hoffmann, K., Coolen, A., Schlumbohm, C., Meerlo, P., and Fuchs, E. (2012). Remote long-term registrations of sleep-wake rhythms, core body temperature and activity in marmoset monkeys. Behav. Brain Res. 235, 113–123. doi: 10.1016/j.bbr.2012.07.033
Hoffmann, K. P. (1973). Conduction velocity in pathways from retina to superior colliculus in the cat: A correlation with receptive-field properties. J. Neurophysiol. 36, 409–424. doi: 10.1152/jn.1973.36.3.409
Hoffmann, K. P., and Distler, C. (1989). Quantitative analysis of visual receptive fields of neurons in nucleus of the optic tract and dorsal terminal nucleus of the accessory optic tract in macaque monkey. J. Neurophysiol. 62, 416–428. doi: 10.1152/jn.1989.62.2.416
Hoffmann, K. P., Distler, C., Erickson, R. G., and Mader, W. (1988). Physiological and anatomical identification of the nucleus of the optic tract and dorsal terminal nucleus of the accessory optic tract in monkeys. Exp. Brain Res. 69, 635–644. doi: 10.1007/BF00247315
Homman-Ludiye, J., and Bourne, J. A. (2019). The medial pulvinar: Function, origin and association with neurodevelopmental disorders. J. Anat. 235, 507–520. doi: 10.1111/joa.12932
Hong, Y. K., and Chen, C. (2011). Wiring and rewiring of the retinogeniculate synapse. Curr. Opin. Neurobiol. 21, 228–237. doi: 10.1016/j.conb.2011.02.007
Hubel, D. H., and Wiesel, T. N. (1961). Integrative action in the cat’s lateral geniculate body. J. Physiol. 155, 385–398.
Huberman, A. D., Manu, M., Koch, S. M., Susman, M. W., Lutz, A. B., Ullian, E. M., et al. (2008). Architecture and activity-mediated refinement of axonal projections from a mosaic of genetically identified retinal ganglion cells. Neuron 59, 425–438. doi: 10.1016/j.neuron.2008.07.018
Hugelin, B. A., and Vibert, J. F. (1974). A stereotaxic model of pneumotaxic oscillator based on spatial and temporal distributions of neuronal bursts. J. Neurophysiol. 37, 91–107. doi: 10.1152/jn.1974.37.1.91
Hylden, J. L., Hayashi, H., Bennett, G. J., and Dubner, R. (1985). Spinal lamina I neurons projecting to the parabrachial area of the cat midbrain. Brain Res. 336, 195–198. doi: 10.1016/0006-8993(85)90436-6
Ilinsky, I. A., Jouandet, M. L., and Goldman-Rakic, P. S. (1985). Organization of the nigrothalamocortical system in the rhesus monkey. J. Comp. Neurol. 236, 315–330. doi: 10.1002/cne.902360304
Illing, R. B., and Wässle, H. (1981). The retinal projection to the thalamus in the cat: A quantitative investigation and a comparison with the retinotectal pathway. J. Comp. Neurol. 202, 265–285. doi: 10.1002/cne.902020211
Itaya, S. K., and Van Hoesen, G. W. (1983). Retinal axons to the medial terminal nucleus of the accessory optic system in old world monkeys. Brain Res. 269, 361–364. doi: 10.1016/0006-8993(83)90147-6
Ito, S., and Feldheim, D. A. (2018). The mouse superior colliculus: An emerging model for studying circuit formation and function. Front. Neural Circuits 12:10. doi: 10.3389/fncir.2018.00010
Jaubert-Miazza, L., Green, E., Lo, F. S., Bui, K., Mills, J., and Guido, W. (2005). Structural and functional composition of the developing retinogeniculate pathway in the mouse. Vis. Neurosci. 22, 661–676. doi: 10.1017/S0952523805225154
Johnson, R. F., Moore, R. Y., and Morin, L. P. (1989). Lateral geniculate lesions alter circadian activity rhythms in the hamster. Brain Res. Bull. 22, 411–422.
Johnson, R. F., Morin, L. P., and Moore, R. Y. (1988). Retinohypothalamic projections in the rat and hamster demonstrated using cholera toxin. Brain Res. 462, 301–312. doi: 10.1016/0006-8993(88)90558-6
Jones, E. G. (2001). The thalamic matrix and thalamocortical synchrony. Trends Neurosci. 24, 595–601. doi: 10.1016/s0166-2236(00)01922-6
Jusuf, P. R., Martin, P. R., and Grünert, U. (2006). Synaptic connectivity in the midget-parvocellular pathway of primate central retina. J. Comp. Neurol. 494, 260–274. doi: 10.1002/cne.20804
Kaas, J. H., and Baldwin, M. K. L. (2019). The evolution of the pulvinar complex in primates and its role in the dorsal and ventral streams of cortical processing. Vision 4:3. doi: 10.3390/vision4010003
Kaas, J. H., Harting, J. K., and Guillery, R. W. (1974). Representations of the complete retina in the contralateral superior colliculus in some mammals. Brain Res. 65, 343–346. doi: 10.1016/0006-8993(74)90047-x
Kaas, J. H., and Huerta, M. F. (1988). “The subcortical visual system of primates,” in Comparative primate biology, ed. H. P. Steklis (New York, NY: Liss), 327–391.
Kaas, J. H., Huerta, M. F., Weber, J. T., and Harting, J. K. (1978). Patterns of retinal terminations and laminar organization of the lateral geniculate nucleus of primates. J. Comp. Neurol. 182, 517–553. doi: 10.1002/cne.901820308
Kaas, J. H., and Lyon, D. C. (2007). Pulvinar contributions to the dorsal and ventral streams of visual processing in primates. Brain Res. Rev. 55, 285–296. doi: 10.1016/j.brainresrev.2007.02.008
Kalsbeek, A., Teclemariam-Mesbah, R., and Péavet, P. (1993). Efferent projections of the suprachiasmatic nucleus in the golden hamster (Mesocricetus auratus). J. Comp. Neurol. 332, 293–314. doi: 10.1002/cne.903320304
Katz, L. C., and Shatz, C. J. (1996). Synaptic activity and the construction of cortical circuit. Science 274, 1133–1138. doi: 10.1126/science.274.5290.1133
Kawano, H., Decker, K., and Reuss, S. (1996). Is there a direct retina-raphe-suprachiasmatic nucleus pathway in the rat? Neurosci. Lett. 212, 143–146. doi: 10.1016/0304-3940(96)12795-6
Kerschensteiner, D., and Guido, W. (2017). Organization of the dorsal lateral geniculate nucleus in the mouse. Vis. Neurosci. 34:E008. doi: 10.1017/S0952523817000062
Kievit, J., and Kuypers, H. G. J. M. (1977). Organization of the thalamo-cortical connections to the frontal lobe in the rhesus monkey. Exp. Brain Res. 29, 299–322. doi: 10.1007/BF00236173
Kim, I. J., Zhang, Y., Meister, M., and Sanes, J. R. (2010). Laminar restriction of retinal ganglion cell dendrites and axons: Subtype-specific developmental patterns revealed with transgenic markers. J. Neurosci. 30, 1452–1462. doi: 10.1523/JNEUROSCI.4779-09.2010
Kirouac, G. J. (2015). Placing the paraventricular nucleus of the thalamus within the brain circuits that control behavior. Neurosci. Biobehav. Rev. 56, 315–329. doi: 10.1016/j.neubiorev.2015.08.005
Kirouac, G. J. (2021). The paraventricular nucleus of the thalamus as an integrating and relay node in the brain anxiety network. Front. Behav. Neurosci. 15:627633. doi: 10.3389/fnbeh.2021.627633
Klein, D. C., and Moore, R. Y. (1979). Pineal N-acetyltransferase and hydroxyindole-O-methyltransferase: Control by retinothalamic tract and suprachiasmatic nucleus. Brain Res. 174, 254–262. doi: 10.1016/0006-8993(79)90848-5
Kolaj, M., Zhang, L., Rønnekleiv, O. K., and Renaud, L. P. (2012). Midline thalamic paraventricular nucleus neurons display diurnal variation in resting membrane potentials, conductances, and firing patterns in vitro. J. Neurophysiol. 107, 1835–1844. doi: 10.1152/jn.00974.2011
Krause, M., Distler, C., and Hoffman, K. (2014). Retinal ganglion cells projecting to the accessory optic system in optokinetic blind albinotic rats are direction-selective. Eur. J. Neurosci. 40, 2274–2282. doi: 10.1111/ejn.12572
Krettek, J. E., and Price, J. L. (1977). The cortical projections of the mediodorsal nucleus and adjacent thalamic nuclei in the rat. J. Comp. Neurol. 171, 157–191. doi: 10.1002/cne.901710204
Krout, K. E., and Loewy, A. D. (2000). Parabrachial nucleus projections to midline and 496 intralaminar thalamic nuclei of the rat. J. Comp. Neurol. 428, 475–494. doi: 10.1002/1096-9861(20001218)428:3<475::aid-cne6>3.0.co;2-9
Kuroda, M., and Price, J. L. (1991a). Synaptic organization of projections from basal forebrain structures to the mediodorsal thalamic nucleus of the rat. J. Comp. Neurol. 303, 513–533. doi: 10.1002/cne.903030402
Kuroda, M., and Price, J. L. (1991b). Ultrastructure and synaptic organization of axon terminals from brainstem structures to the mediodorsal thalamic nucleus of the rat. J. Comp. Neurol. 313, 539–552. doi: 10.1002/cne.903130313
Kwan, W. C., Mundinano, I., de Souza, M. J., Lee, S. C. S., Martin, P. R., Grünert, U., et al. (2018). Unravelling the subcortical and retinal circuitry of the primate inferior pulvinar. J. Comp. Neurol. 527, 558–576. doi: 10.1002/cne.24387
Lai, B. Q., Qiu, X. C., Zhang, K., Zhang, R. Y., Jin, H., Li, G., et al. (2015). Cholera toxin B subunit shows transneuronal tracing after injection in an injured sciatic nerve. PLoS One 10:e0144030. doi: 10.1371/journal.pone.0144030
Lane, R. H., Allman, J. M., and Kaas, J. H. (1971). Representation of the visual field in the superior colliculus of gray squirrel (Sciurus carolinensis) and tree shrew (Tupaia glis). Brain Res. 26, 277–292. doi: 10.1016/S0006-8993(71)80005-7
Lane, R. H., Allman, J. M., and Kaas, J. H. (1973). The visuotopic organization of the superior colliculus of the owl monkey (Aotus trivirgatus) and the bush baby (Galago senegalensis). Brain Res. 26, 277–292. doi: 10.1016/0006-8993(73)90794-4
Lazzerini Ospri, L., Prusky, G., and Hattar, S. (2017). Mood, the circadian system, and melanopsin retinal ganglion cells. Annu. Rev. Neurosci. 40, 539–556. doi: 10.1146/annurev-neuro-072116-031324
Leak, R. K., and Moore, R. Y. (2001). Topographic organization of suprachiasmatic nucleus projections neurons. J. Comp. Neurol. 433, 312–334. doi: 10.1002/cne.1142
Leamey, C. A., Merlin, S., Lattouf, P., Sawatari, A., Zhou, X., Demel, N., et al. (2007). Ten_m3 regulates eye-specific patterning in the mammalian visual pathway and is required for binocular vision. PLoS Biol. 5:e241. doi: 10.1371/journal.pbio.0050241
Lee, B. B., Martin, P. R., and Grünert, U. (2010). Retinal connectivity and primate vision. Prog. Retin. Eye Res. 29, 622–639. doi: 10.1016/j.preteyeres.2010.08.004
LeGates, T. A., Fernandez, D. C., and Hattar, S. (2014). Light as a central modulator of circadian rhythms, sleep and affect. Nat. Rev. Neurosci. 15, 443–454. doi: 10.1038/nrn3743
Lennie, P., and Movshon, J. A. (2005). Coding of color and form in the geniculostriate visual pathway (invited review). J. Opt. Soc. Am. A Opt. Image Sci. Vis. 22, 2013–2033. doi: 10.1364/josaa.22.002013
Leventhal, A. G. (1982). Morphology and distribution of retinal ganglion cells projecting to different layers of the dorsal lateral geniculate nucleus in normal and siamese cats. J. Neurosci. 2, 1024–1042. doi: 10.1523/JNEUROSCI.02-08-01024.1982
Levine, J. D., Weiss, M. L., Rosenwasser, A. M., and Miselis, R. R. (1991). Retinohypothalamic tract in the female albino rat: A study using horseradish peroxidase conjugated to cholera toxin. J. Comp. Neurol. 306, 344–360. doi: 10.1002/cne.903060210
Li, K., Patel, J., Purushothaman, G., Marion, R. T., and Casagrande, V. A. (2013). Retinotopic maps in the pulvinar of bush baby (Otolemur garnettii). J. Comp. Neurol. 521, 3432–3450. doi: 10.1002/cne.23358
Li, S., and Kirouac, G. J. (2012). Sources of inputs to the anterior and posterior aspects of the paraventricular nucleus of the thalamus. Brain Struct. Funct. 217, 257–273. doi: 10.1007/s00429-011-0360-7
Li, X. B., Inoue, T., Nakagawa, S., and Koyama, T. (2004). Effect of mediodorsal thalamic nucleus lesion on contextual fear conditioning in rats. Brain Res. 1008, 261–272. doi: 10.1016/j.brainres.2004.02.038
Lilley, B. N., Sabbah, S., Hunyara, J. L., Gribble, K. D., Al-Khindi, T., Xiong, J., et al. (2018). Genetic access to neurons in the accessory optic system reveals a role for Sema6A in midbrain circuitry mediating motion perception. J. Comp. Neurol. 527, 282–296. doi: 10.1002/cne.24507
Lima, R. R., Pinato, L., Nascimento, R. B., Engelberth, R. C. G., Junior, E. S. N., Cavalcante, J. C., et al. (2012). Retinal projections and neurochemical characterization of the pregeniculate nucleus of the common marmoset (Callithrix jacchus). J. Chem. Neuroanat. 44, 34–44. doi: 10.1016/j.jchemneu.2012.04.001
Lin, C. S., and Kaas, J. H. (1980). Projections from the medial nucleus of the interior pulvinar complex to the middle temporal area of visual cortex. Neuroscience 5, 2219–2228. doi: 10.1016/j.neuroscience.2020.08.031
Lin, M. K., Takahashi, Y. S., Huo, B. X., Hanada, M., Nagashima, J., Hata, J., et al. (2019). A high-throughput neurohistological pipeline for brain-wide mesoscale connectivity mapping of the common marmoset. eLife 8:e40042. doi: 10.7554/eLife.40042
Ling, C., Schneider, G. E., Northmore, D., and Jhaveri, S. (1997). Afferents from the colliculus, cortex, and retina have distinct terminal morphologies in the lateral posterior thalamic nucleus. J. Comp. Neurol. 388, 467–483.
Litvina, E., and Chen, C. (2017). Functional convergence at the retinogeniculate synapse. Neuron 96, 330–338. doi: 10.1016/j.neuron.2017.09.037
Livingston, C. A., and Fedder, S. R. (2003). Visual-ocular motor activity in the macaque pregeniculate complex. J. Neurophysiol. 90, 226–244. doi: 10.1152/jn.00033.2003
Livingston, C. A., and Mustari, M. J. (2000). The anatomical organization of the macaque pregeniculate complex. Brain Res. 876, 166–179. doi: 10.1016/s0006-8993(00)02647-0
Loyd, D. R., and Murphy, A. Z. (2009). The role of the periaqueductal gray in the modulation of pain in males and females: Are the anatomy and physiology really that different? Neural Plast. 2009:462879. doi: 10.1155/2009/462879
Lui, F., Gregory, K. M., Blanks, R. H. I., and Giolli, R. A. (1995). Projections from visual areas of the cerebral cortex to pretectal nuclear complex, terminal accessory optic nuclei, and superior colliculus in macaque monkey. J. Comp. Neurol. 363, 439–460. doi: 10.1002/cne.903630308
Lund, R. D., Lund, J. S., and Wise, R. P. (1974). The organization of the retinal projection to the dorsal lateral geniculate nucleus in pigmented and albino rats. J. Comp. Neurol. 158, 383–403. doi: 10.1002/cne.901580403
MacDougall, M., Nummela, S. U., Coop, S., Disney, A., Mitchell, J. F., and Miller, C. T. (2016). Optogenetic manipulation of neural circuits in awake marmosets. J. Neurophysiol. 116, 1286–1294. doi: 10.1152/jn.00197.2016
Magnin, M., and Fuchs, A. F. (1977). Discharge properties of neurons in the monkey thalamus tested with angular acceleration, eye movement and visual stimuli. Exp. Brain Res. 28, 293–299. doi: 10.1007/BF00235710
Majka, P., Chaplin, T. A., Yu, H., Tolpygo, A., Mitra, P., Wójcik, D. K., et al. (2016). Towards a comprehensive atlas of cortical connections in a primate brain: Mapping tracer injection studies of the common marmoset into a reference digital template. J. Comp. Neurol. 11, 2161–2181. doi: 10.1002/cne.24023
Mammen, A. P., and Jagota, A. (2011). Immunocytochemical evidence for different patterns in daily rhythms of VIP and AVP peptides in the suprachiasmatic nucleus of diurnal Funambulus palmarum. Brain Res. 1373, 39–47. doi: 10.1016/j.brainres.2010.12.018
Markus, Z., Berenyi, A., Paroczy, Z., Wypych, M., Waleszczyk, W. J., Benedek, J., et al. (2009). Spatial and temporal visual properties of the neurons in the intermediate layers of the superior colliculus. Neurosci. Lett. 454, 76–80. doi: 10.1016/j.neulet.2009.02.063
Marrocco, R. T., and Li, R. H. (1977). Monkey superior colliculus: Properties of single cells and their afferent inputs. J. Neurophysiol. 40, 844–860. doi: 10.1152/jn.1977.40.4.844
Martersteck, E., Hirokawa, K. E., Evarts, M., Bernard, A., Duan, X., Li, Y., et al. (2017). Diverse central projection patterns of retinal ganglion cells. Cell Rep. 18, 2058–2072. doi: 10.1016/j.celrep.2017.01.075
Martin, P. R. (1986). The projection of different retinal ganglion cell classes to the dorsal lateral geniculate nucleus in the hooded rat. Exp. Brain Res. 162, 77–88. doi: 10.1007/bf00237404
Martin, P. R., and Grünert, U. (2013). Color signals in retina and lateral geniculate nucleus of marmoset monkeys. Psychol. Neurosci. 6, 151–163. doi: 10.3922/j.psns.2013.2.04
Martin, P. R., White, A. J., Goodchild, A. K., Wilder, H. D., and Sefton, A. E. (1997). Evidence that blue-on cells are part of the third geniculocortical pathway in primates. Eur. J. Neurosci. 9, 1536–1541. doi: 10.1111/j.1460-9568.1997.tb01509.x
Masland, R. H. (2012). The neuronal organization of the retina. Neuron 76, 266–280. doi: 10.1016/j.neuron.2012.10.002
Masland, R. H. (2017). Vision: Two speeds in the retina. Curr. Biol. 27, R303–R305. doi: 10.1016/j.cub.2017.02.056
Masri, R. A., Percival, K. A., Koizumi, A., Martin, P. R., and Grünert, U. (2017). Survey of retinal ganglion cell morphology in marmoset. J. Comp. Neurol. 527, 236–258. doi: 10.1002/cne.24157
Masseck, O. A., and Hoffmann, K. (2009). Question of reference frames: Visual direction-selective neurons in the accessory optic system of goldfish. J. Neurophysiol. 102, 2781–2789. doi: 10.1152/jn.00415.2009
Mathers, L. H. (1971). Tectal projection to the posterior thalamus of the squirrel monkey. Brain Res. 35, 295–298. doi: 10.1016/0006-8993(71)90622-6
Matteau, L., Boire, D., and Ptito, M. (2003). Retinal projections in the cat: A cholera toxin B subunit study. Vis. Neurosci. 20, 481–493. doi: 10.1017/s0952523803205022
May, P. J. (2006). The mammalian superior colliculus: Laminar structure and connections. Prog. Brain Res. 151, 321–378. doi: 10.1016/S0079-6123(05)51011-2
McFarland, N. R., and Haber, S. N. (2002). Thalamic relay nuclei of the basal ganglia form both reciprocal and nonreciprocal cortical connections, linking multiple frontal cortical areas. J. Neurosci. 22, 8117–8132. doi: 10.1523/JNEUROSCI.22-18-08117.2002
McPeek, R. M., and Keller, E. L. (2004). Deficits in saccade target selections after inactivation of superior colliculus. Nat. Neurosci. 7, 757–763. doi: 10.1038/nn1269
Mei, L., Fan, Y., Lv, X., Welsh, D. K., Zhan, C., and Zhang, E. E. (2018). Long-term in vivo recording of circadian rhythms in brains of freely moving mice. Proc. Nat. Acad. Sci. U.S.A. 115, 4276–4281. doi: 10.1073/pnas.171773511
Menani, J. V., De Luca, L. A. Jr., and Johnson, A. K. (2014). Role of the lateral parabrachial nucleus in the control of sodium appetite. Am. J. Physiol. Regul. Integr. Comp. Physiol. 306, R201–R210. doi: 10.1152/ajpregu.00251.2012
Mesulam, M. M., Van Hoesen, G. W., Pandya, D. N., and Geschwind, N. (1977). Limbic and sensory connections of the inferior parietal lobule (area PG) in the rhesus monkey: A study with a new method for horseradish peroxidase histochemistry. Brain Res. 136, 393–414. doi: 10.1016/0006-8993(77)90066-x
Meyern-Berstein, E. L., and Morin, L. P. (1996). Differential serotonergic innervation of the suprachiasmatic nucleus and the intergeniculate leaflet and its role in circadian rhythm modulation. J. Neurosci. 16, 2097–2111. doi: 10.1523/JNEUROSCI.16-06-02097
Miller, C. T., Freiwald, W. A., Leopold, D. A., Mitchell, J. F., Silva, A. C., and Wang, X. (2016). Marmosets: A neuroscientific model of human social behavior. Neuron 90, 219–233.
Mimura, K., Nagai, Y., Inoue, K., Matsumoto, J., Hori, Y., Sato, C., et al. (2021). Chemogenetic activation of nigrostriatal dopamine neurons in freely moving common marmosets. Iscience 24:103066. doi: 10.1016/j.isci.2021.103066
Mitchell, A. S. (2015). The mediodorsal thalamus as a higher order thalamic relay nucleus important for learning and decision-making. Neurosci. Biobehav. Rev. 54, 76–88. doi: 10.1016/j.neubiorev.2015.03.001
Mitchell, A. S., and Chakraborty, S. (2013). What does the mediodorsal thalamus do? Front. Syst. Neurosci. 7:37. doi: 10.3389/fnsys.2013.00037
Mitchell, J. F., and Leopold, D. A. (2015). The marmoset monkey as a model for visual neuroscience. Neurosci. Res. 93, 20–46. doi: 10.1016/j.neures.2015.01.008
Moga, M. M., and Moore, R. Y. (1997). Organization of neural inputs to the suprachiasmatic nucleus in the rat. J. Comp. Neurol. 389, 508–534. doi: 10.1002/(sici)1096-9861(19971222)389:3<508::aid-cne11>3.0.co;2-h
Moga, M. M., and Moore, R. Y. (2000). Paraventricular thalamus lesions alter circadian period and activity distribution in the blinded rat. Biol. Rhythm Res. 31, 212–219.
Moga, M. M., Weis, R. P., and Moore, R. Y. (1995). Efferent projections of the paraventricular thalamic nucleus in the rat. J. Comp. Neurol. 359, 221–238. doi: 10.1002/cne.903590204
Moore, B., Li, K., Kaas, J. H., Liao, C. C., Boal, A. M., Mavity-Hudson, J., et al. (2019). Cortical projections to the two retinotopic maps of primate pulvinar are distinct. J. Comp. Neurol. 527, 577–588. doi: 10.1002/cne.24515
Moore, R. Y. (1973). Retinohypothalamic projection in mammals: A comparative study. Brain Res. 49, 403–409. doi: 10.1016/0006-8993(73)90431-9
Moore, R. Y. (1989). The geniculohypothalamic tract in monkey and man. Brain Res. 486, 190–194. doi: 10.1016/0006-8993(89)91294-8
Moore, R. Y., and Lenn, N. J. (1972). A retinohypothalamic projection in the rat. J. Comp. Neurol. 180, 1–14. doi: 10.1002/cne.901460102
Moore, R. Y., Speh, J. C., and Leak, R. K. (2002). Suprachiasmatic nucleus organization. Cell Tissue Res. 309, 89–98.
Morais, P. L. A. G., García-Amado, M., Lima, R. R. M., Córdoba-Claros, A., Cavalcante, J. S., Clascá, F., et al. (2019). Cyto- and myelo-architecture of the amygdaloid complex of the common marmoset monkey (Callithrix jacchus). Front. Neuroanat. 13:36. doi: 10.3389/fnana.2019.00036
Morin, L. P. (2013). Neuroanatomy of the extended circadian rhythm system. Exp. Neurol. 243, 4–20. doi: 10.1016/j.expneurol.2012.06.026
Morin, L. P., and Allen, C. N. (2006). The circadian visual system. Brain Res. Rev. 51, 1–60. doi: 10.1016/j.brainresrev.2005.08.003
Morin, L. P., and Studholme, K. M. (2014). Retinofugal projections in the mouse. J. Comp. Neurol. 522, 3733–3753. doi: 10.1002/cne.23635
Moritoh, S., Komatsu, Y., Yamamori, T., and Koizumi, A. (2013). Diversity of retinal ganglion cells identified by transient GFP transfection in organotypic tissue culture of adult marmoset monkey retina. PLoS One 8:e54667. doi: 10.1371/journal.pone.0054667
Muir-Robinson, G., Hwang, B. J., and Feller, M. B. (2002). Retinogeniculate axons undergo eye-specific segregation in the absence of eye-specific layers. J. Neurosci. 22, 5259–5264. doi: 10.1523/JNEUROSCI.22-13-05259.2002
Murakami, D. M., Miller, J. D., and Fuller, C. A. (1989). The retinohypothalamic tract in the cat: Retinal ganglion cell morphology and pattern of projection. Brain Res. 482, 283–296. doi: 10.1016/0006-8993(89)91191-8
Mure, L. S., Le, H. D., Benegiamo, G., Chang, M. W., Rios, L., and Jillani, N. (2018). Diurnal transcriptome atlas of a primate across major neural and peripheral tissues. Science 359:eaao0318. doi: 10.1126/science.aao0318
Mustari, M. J., and Fuchs, A. F. (1990). Discharge patterns of neurons in the pretectal nucleus of the optic tract (NOT) in the behaving primate. J. Neurophysiol. 64, 77–90. doi: 10.1152/jn.1990.64.1.77
Mustari, M. J., Fuchs, A. F., Kaneko, C. R., and Robinson, F. R. (1994). Anatomical connections of the primate pretectal nucleus of the optic tract. J. Comp. Neurol. 349, 111–128. doi: 10.1002/cne.903490108
Nakagawa, S., and Tanaka, S. (1984). Retinal projections to the pulvinar nucleus of the macaque monkey: A re-investigation using autoradiography. Exp. Brain Res. 57, 151–157. doi: 10.1007/BF00231141
Nakamoto, C., Durward, E., Horie, M., and Nakamoto, M. (2018). Nell2 regulates the contralateral-versus-ipsilateral visual projection as a layer-specific positional cue. Development 146:dev170704. doi: 10.1242/dev.170704
Nascimento, E. S. Jr., Duarte, R. B., Silva, S. F., Engelberth, R. C. G. J., Toledo, C. A. B., Cavalcante, J. S., et al. (2008). Retinal projections to the thalamic paraventricular nucleus in the rock cavy (Kerodon rupestris). Brain Res. 241, 56–61. doi: 10.1016/j.brainres.2008.09.017
Nascimento, E. S. Jr., Souza, A. P. M., Duarte, R. B., Magalhães, M. A. F., Silva, S. F., Cavalcante, J. S., et al. (2010). The suprachiasmatic nucleus and the intergeniculate leaflet in the rock cavy (Kerodon rupestris): Retinal projections and immunohistochemical characterization. Brain Res. 1320, 34–46. doi: 10.1016/j.brainres.2010.01.034
Nassi, J. J., and Callaway, E. M. (2009). Parallel processing strategies of the primate visual system. Nat. Rev. Neurosci. 10, 360–372. doi: 10.1038/nrn2619
Natal, C. L., and Britto, L. R. G. (1988). The rat accessory optic system: Effects of cortical lesions on the directional selectivity of units within the medial terminal nucleus. Neurosci. Lett. 91, 154–159. doi: 10.1016/0304-3940(88)90760-4
Nemec, P., Burda, H. H., and Peichl, L. (2004). Subcortical visual system of the African mole-rat Cryptomys anselli: To see or not to see? Eur. J. Neurosci. 20, 757–768. doi: 10.1111/j.1460-9568.2004.03510.x
Niimi, K., Kanaseki, T., and Takimoto, T. (1963). The comparative anatomy of the ventral nucleus of the lateral geniculate body in mammals. J. Comp. Neurol. 121, 313–324. doi: 10.1002/cne.901210303
Novak, C. M., and Nunez, A. A. (1998). Daily rhythms in Fos activity in the rat ventrolateral preoptic area and midline thalamic nuclei. Am. J. Physiol. 275, R1620–R1626. doi: 10.1152/ajpregu.1998.275.5.R1620
O’Connor, D. H., Fukui, M. M., Pinsk, M. A., and Kastner, S. (2002). Attention modulates responses in the human lateral geniculate nucleus. Nat. Neurosci. 5, 1203–1209. doi: 10.1038/nn957
Okano, H., Hikishima, K., Iriki, A., and Sasaki, E. (2012). The common marmoset as a novel animal model system for biomedical and neuroscience research application. Semin. Fetal Neonatal Med. 17, 336–340. doi: 10.1016/j.siny.2012.07.002
Olszewski, J. (1952). The thalamus of the Macaca mulatta: An atlas for the use with the stereotaxic instrument. Basel, CH: Karger Press, 43.
Ouhaz, Z., Ba-M’hamed, S., and Bennis, M. (2017). Morphological, structural, and functional alterations of the prefrontal cortex and the basolateral amygdala after early lesion of the rat mediodorsal thalamus. Brain Struct. Funct. 222, 2527–2545. doi: 10.1007/s00429-016-1354-2
Ouhaz, Z., Ba-M’hamed, S., Mitchell, A. S., Elidrissi, A., and Bennis, M. (2015). Behavioral and cognitive changes after early postnatal lesions of the rat mediodorsal thalamus. Behav. Brain Res. 292, 219–232. doi: 10.1016/j.bbr.2015.06.017
Ouhaz, Z., Fleming, H., and Mitchell, A. S. (2018). Cognitive functions and neurodevelopmental disorders involving the prefrontal cortex and mediodorsal thalamus. Front. Neurosci. 12:33. doi: 10.3389/fnins.2018.00033
Paknahad, J., Loizos, K., Yue, L., Humanyum, M. S., and Lazzi, G. (2021). Color and cellular selectivity of retinal ganglion cell subtypes through frequency modulation of electrical stimulation. Sci. Rep. 11:5177. doi: 10.1038/s41598-021-84437-w
Panda, S., Hogenesch, J. B., and Kay, S. A. (2002). Circadian rhythms from flies to human. Nature 417, 329–335. doi: 10.1038/417329a
Parnaudeau, S., O’neill, P. K., Bolkan, S. S., Ward, R. D., Abbas, A. I., Roth, B. L., et al. (2013). Inhibition of mediodorsal thalamus disrupts thalamofrontal connectivity and cognition. Neuron 77, 1151–1162. doi: 10.1016/j.neuron.2013.01.038
Parnaudeau, S., Taylor, K., Bolkan, S. S., Ward, R. D., Balsam, P. D., and Kellendonk, C. (2015). Mediodorsal thalamus hypofunction impairs flexible goal-directed behavior. Biol. Psychiatry 77, 445–453. doi: 10.1016/j.biopsych.2014.03.020
Paxinos, G., Watson, C., Petrides, M., Rosa, M., and Tokuno, H. (2012). The marmoset brain in stereotaxic coordinates. San Diego, LA: Academic Press, 13.
Peng, Z.-C., Grassi-Zucconi, G., and Bentivoglio, M. (1995). Fos-related protein expression in the midline paraventricular nucleus of the rat thalamus: Basal oscillation and relationship with limbic efferents. Exp. Brain Res. 104, 21–29. doi: 10.1007/BF00229852
Percival, K. A., Koizumi, A., Masri, R. A., Buzas, P., Martin, P. R., and Grünert, U. (2014). Identification of a pathway from the retina to koniocellular layer K1 in the lateral geniculate nucleus of marmoset. J. Neurosci. 34, 3821–3825. doi: 10.1523/JNEUROSCI.4491-13.2014
Percival, K. A., Martin, P. R., and Grünert, U. (2011). Synaptic inputs to two types of koniocellular pathway ganglion cells in marmoset retina. J. Comp. Neurol. 519, 2135–2153. doi: 10.1002/cne.22586
Percival, K. A., Martin, P. R., and Grünert, U. (2013). Organization of koniocellular-projecting ganglion cells and diffuse bipolar cells in the primate fovea. Eur. J. Neurosci. 37, 1072–1089. doi: 10.1111/ejn.12117
Petersen, S. E., Robinson, D. L., and Keys, W. (1985). Pulvinar nuclei of the behaving Rhesus monkey: Visual responses and their modulation. J. Neurophysiol. 54, 867–886. doi: 10.1152/jn.1985.54.4.867
Pfeiffenberger, C., Cutforth, T., Woods, G., Yamada, J., Renteria, R. C., Copenhagen, D. R., et al. (2005). Ephrin-As and neural activity are required for eye-specific patterning during retinogeniculate mapping. Nat. Neurosci. 8, 1022–1027. doi: 10.1038/nn1508
Pickard, G. E., and Silverman, A. J. (1981). Direct retinal projection to the hypothalamus, piriform cortex and accessory optic nuclei in the Golden hamster as demonstrated by a sensitive anterograde horseradish peroxidase technique. J. Comp. Neurol. 196, 155–172. doi: 10.1002/cne.901960111
Pinato, L., Frazão, R., Cruz-Rizzolo, R. J., Cavalcante, J. S., and Nogueira, M. I. (2009). Immunocytochemical characterization of the pregeniculate nucleus and distribution of retinal and neuropeptide Y terminals in the suprachiasmatic nucleus of the Cebus monkey. J. Chem. Neuroanat. 37, 207–213. doi: 10.1016/j.jchemneu.2009.01.005
Piscopo, D. M., El-Danaf, R. N., Huberman, A. D., and Niell, C. M. (2013). Diverse visual features encoded in mouse lateral geniculate nucleus. J. Neurosci. 33, 4642–4656. doi: 10.1523/JNEUROSCI.5187-12.2013
Pollack, J. G., and Hickey, T. L. (1979). The distribution of retino-collicular axon terminals in rhesus monkey. J. Comp. Neurol. 185, 587–602. doi: 10.1002/cne.901850402
Polyak, S. (1957). “The pregeniculate gray nucleus and the pupillary reflex pathway,” in The vertebrate visual system, ed. H. Klüver (Chicago, IL: University of Chicago Press), 376–385.
Pong, M., and Fuchs, A. F. (2000). Characteristics of the pupillary light reflex in the macaque monkey: Discharge patterns of pretectal neurons. J. Neurophysiol. 84, 964–974. doi: 10.1152/jn.2000.84.2.964
Preuss, T. M. (2007). “Evolutionary specializations of primate brain systems,” in Primate origins: Adaptations and evolution, eds M. J. Ravosa and M. Dagasto (Boston, MA: Springer), 625–675.
Ray, J. P., and Price, J. L. (1992). The organization of the thalamocortical connections of the mediodorsal thalamic nucleus in the rat, related to the ventral forebrain–prefrontal cortex topography. J. Comp. Neurol. 323, 167–197. doi: 10.1002/cne.903230204
Ray, J. P., and Price, J. L. (1993). The organization of projections from the mediodorsal nucleus of the thalamus to orbital and medial prefrontal cortex in macaque monkeys. J. Comp. Neurol. 337, 1–31. doi: 10.1002/cne.903370102
Reese, B. E. (1988). ‘Hidden lamination’ in the dorsal lateral geniculate nucleus: The functional organization of this thalamic region in the rat. Brain Res. 13, 119–137. doi: 10.1016/0165-0173(88)90017-3
Reilly, S. (1999). The parabrachial nucleus and conditioned taste aversion. Brain Res. Bull. 48, 239–254. doi: 10.1016/s0361-9230(98)00173-7
Reuss, S., and Fuchs, E. (2000). Anterograde tracing of retinal afferents to the tree shrew hypothalamus and raphe. Brain Res. 874, 66–74. doi: 10.1016/s0006-8993(00)02578-6
Ricardo, J. A., and Koh, E. T. (1978). Anatomical evidence of direct projections from the nucleus of the nucleus tractus solitarius to the hypothalamus, amygdala, and other forebrain structures in the rat. Brain Res. 153, 1–26. doi: 10.1016/0006-8993(78)91125-3
Ríos-Flórez, J. A., Lima, R. R. M., Morais, P. L. A. G., de Medeiros, H. H. A., Cavalcante, J. S., and Junior, E. S. N. (2021). Medial prefrontal cortex (A32 and A25) projections in the common marmoset: A subcortical anterograde study. Sci. Rep. 11:14565. doi: 10.1038/s41598-021-93819-z
Roberts, A. C., Tomic, D. L., Parkinson, C. H., Roeling, T. A., Cutter, D. J., Robbins, T. W., et al. (2007). Forebrain connectivity of the prefrontal cortex in the marmoset monkey (Callithrix jacchus): An anterograde and retrograde tract-tracing study. J. Comp. Neurol. 502, 86–112. doi: 10.1002/cne.21300
Robinson, D. L., Petersen, S. E., and Keys, W. (1986). Saccade-related and visual activities in the pulvinar nuclei of the behaving rhesus monkey. Exp. Brain Res. 62, 625–634. doi: 10.1007/bf00236042
Robles, E., Laurell, E., and Baier, H. (2014). The retinal projectome reveals brain-area-specific visual representations generated by ganglion cell diversity. Curr. Biol. 24, 2085–2096. doi: 10.1016/j.cub.2014.07.080
Rodieck, R. W., and Dreher, B. (1979). Visual suppression from nondominant eye in the lateral geniculate nucleus: A comparison of cat and monkey. Exp. Brain Res. 35, 465–477. doi: 10.1007/BF00236765
Rodman, H. R., Gross, C. G., and Albright, T. D. (1989). Afferent basis of visual response properties in area MT of the macaque. I. Effects of striate cortex removal. J. Neurosci. 9, 2033–2050. doi: 10.1523/JNEUROSCI.09-06-02033.1989
Rompani, S. B., Müllner, F. E., Wanner, A., Zhang, C., Roth, C. N., Yonehara, K., et al. (2017). Different modes of visual integration in the lateral geniculate nucleus revealed by single-cell-initiated transsynaptic tracing. Neuron 93, 767–776.e6. doi: 10.1016/j.neuron.2017.01.028
Rosa, M. G., and Tweedale, R. (2000). Visual areas in lateral and ventral extrastriate cortices of the marmoset monkey. J. Comp. Neurol. 422, 621–651.
Rosenwasser, A. M., and Turek, F. W. (2015). Neurobiology of circadian rhythm regulation. Sleep Med. Clin. 10, 403–412. doi: 10.1016/j.jsmc.2015.08.003
Ross, C. N. (2019). “Marmosets in aging research,” in The common marmoset in captivity and biomedical research, eds R. Marini, L. Watchman, S. Tardiff, D. Mansfield, and J. Fox (Cambridge, MA: Academic Press), 355–376.
Roy, S., Jayakumar, J., Martin, P. R., Dreher, B., Saalmann, Y. B., Hu, D., et al. (2009). Segregation of short-wavelength-sensitive (S) cone signals in the macaque dorsal lateral geniculate nucleus. Eur. J. Neurosci. 30, 1517–1526. doi: 10.1111/j.1460-9568.2009.06939.x
Russchen, F. T., Amaral, D. G., and Price, J. L. (1987). The afferent input to the magnocellular division of the mediodorsal thalamic nucleus in the monkey, Macaca fascicularis. J. Comp. Neurol. 256, 175–210. doi: 10.1002/cne.902560202
Saalman, Y. B. (2014). Intralaminar ad medial thalamic influence on cortical synchrony, information transmission and cognition. Front. Syst. Neurosci. 8:83. doi: 10.3389/fnsys.2014.00083
Saalmann, Y. B., and Kastner, S. (2011). Cognitive and perceptual functions of the visual thalamus. Neuron 71, 209–223. doi: 10.1016/j.neuron.2011.06.027
Salazar-Juárez, A., Escobar, C., and Aguilar-Roblero, R. (2002). Anterior paraventricular thalamus modulates light-induced phase shifts in circadian rhythmicity in rats. Am. J. Physiol. Regul. Integr. Comp. Physiol. 283, R897–R904. doi: 10.1152/ajpregu.00259.2002
Salleeba, C., Dempsey, B., Le, S., Goodchild, A., and McMullan, S. (2019). A student’s guide to neural circuit tracing. Front. Neurosci. 13:897. doi: 10.3389/fnins.2019.00897
Sanderson, K. J., Bishop, P. O., and Darian-Smith, I. (1971). The properties of the binocular receptive fields of lateral geniculate neurons. Exp. Brain Res. 13, 178–207. doi: 10.1007/BF00234085
Sanderson, K. J., Darian-Smith, I., and Bishop, P. O. (1969). Binocular corresponding receptive fields of single units in the cat dorsal lateral geniculate nucleus. Vision Res. 9, 1297–1303. doi: 10.1016/0042-6989(69)90117-5
Sanes, J. R., and Masland, R. H. (2015). The types of retinal ganglion cells: Current status and implications for neuronal classification. Annu. Rev. Neurosci. 38, 221–246. doi: 10.1146/annurev-neuro-071714-034120
Sanetra, A. M., Palus-Chramiec, K., and Lewandowski, M. H. (2021). Modulation of the rat intergeniculate leaflet of the thalamus network by norepinephrine. Neuroscience 469, 1–16. doi: 10.1016/j.neuroscience.2021.06.027
Santana, M. A. D., Medeiros, H. H. A., Leite, M. D., Barros, M. A. S., de Góis Morais, P. L. A., Soares, J. G., et al. (2018). Retinofugal projections into visual brain structures in the bat Artibeus planirostris: A CTb study. Front. Neuroanat. 12:66. doi: 10.3389/fnana.2018.00066
Santana, N. N. M., Barros, M. A. S., Medeiros, H. H. A., Santana, M. A. D., Silva, L. L., Morais, P. L. A. G., et al. (2018). The suprachiasmatic nucleus and the intergeniculate leaflet of the flat-faced fruit-eating bat (Artibeus planirostris): Retinal projections and neurochemical anatomy. Front. Neuroanat. 12:36. doi: 10.3389/fnana.2018.00036
Saper, C. B. (2013). The central circadian timing system. Curr. Opin. Neurobiol. 23, 747–751. doi: 10.1016/j.conb.2013.04.004
Saper, C. B., and Loewy, A. D. (1980). Efferent connections of the parabrachial nucleus in the rat. Brain Res. 197, 291–317. doi: 10.1016/0006-8993(80)91117-8
Saper, C. B., and Lowell, B. B. (2014). The hypothalamus. Curr. Biol. 24, R1111–R1116. doi: 10.1016/j.cub.2014.10.02
Saper, C. B., and Stornetta, R. L. (2015). “Central autonomic system,” in The rat nervous system, ed. G. Paxinos (Oxford: Academic Press), 629–673. doi: 10.1016/b978-0-12-374245-2.00023-1
Sasaki, E., Suemizu, H., Shimada, A., Hanazawa, K., Oiwa, R., Kamioka, M., et al. (2009). Generation of transgenic non-human primates with germline transmission. Nature 459, 523–527. doi: 10.1038/nature08090
Sato, K., Oiwa, R., Kumita, W., Henry, R., Sakuma, T., Ito, R., et al. (2016). Generation of a nonhuman primate model of severe combined immunodeficiency using highly efficient genome editing. Cell Stem Cell 19, 127–138. doi: 10.1016/j.stem.2016.06.003
Saunders, R. C., Mishkin, M., and Aggleton, J. P. (2005). Projections from the entorhinal cortex, perirhinal cortex, presubiculum, and parasubiculum to the medial thalamus in macaque monkeys: Identifying different pathways using disconnection techniques. Exp. Brain Res. 167, 1–16. doi: 10.1007/s00221-005-2361-3
Scalia, F., and Arango, V. (1979). Topographic organization of the projections of the retina to the pretectal region in the rat. J. Comp. Neurol. 186, 271–292. doi: 10.1002/cne.901860210
Scalia, F., Rasweiller, J. J. I, and Danias, J. (2014). Retinal projections in the short-tailed fruit bat, Carollia perspicillata, as studied using the axonal transport of cholera toxin b subunit: Comparison with mouse. J. Comp. Neurol. 523, 1756–1791. doi: 10.1002/cne.23723
Schaap, J., Albus, H., VanderLeest, H. T., Eilers, P. H., Détári, L., and Meijer, J. H. (2003). Heterogeneity of rhythmic suprachiasmatic nucleus neurons: Implications for circadian waveform and photoperiodic encoding. Proc. Natl. Acad. Sci. U.S.A. 100, 15994–15999. doi: 10.1073/pnas.2436298100
Schlag, J., and Schlag-Rey, M. (1986). Role of the central thalamus in gaze control. Prog. Brain Res. 64, 191–201. doi: 10.1016/S0079-6123(08)63413-5
Schmidt, T. M., and Kofuji, P. (2009). Functional and morphological differences among intrinsically photosensitive retinal ganglion cells. J. Neurosci. 29, 476–482. doi: 10.1523/JNEUROSCI.4117-08.2009
Seabrook, T. A., Burbridge, T. J., Crair, M. C., and Huberman, A. D. (2017). Architecture, function, and assembly of the mouse visual system. Annu. Rev. Neurosci. 40, 499–538. doi: 10.1146/annurev-neuro-071714-033842
Sherman, S. M. (2016). Thalamus plays a central role in ongoing cortical functioning. Nat. Neurosci. 19, 533–541. doi: 10.1038/nn.4269
Sherman, S. M., and Guillery, R. W. (1996). The functional organization of thalamocortical relays. J. Neurophysiol. 76, 1367–1395. doi: 10.1152/jn.1996.76.3.1367
Sherman, S. M., and Guillery, R. W. (1998). On the actions that one nerve cell can have on another: Distinguishing ‘drivers’ from ‘modulators’. Proc. Natl. Acad. Sci. U.S.A. 95, 7121–7126. doi: 10.1073/pnas.95.12.7121
Sherman, S. M., and Guillery, R. W. (2002). The role of the thalamus in the flow of information to the cortex. Philos. Trans. R. Soc. Lond. B Biol. Sci. 357, 1695–1708. doi: 10.1098/rstb.2002.1161
Shipp, S. (2003). The functional logic of cortico–pulvinar connections. Trans. R. Soc. Lond. B Biol. Sci. 358, 1605–1624. doi: 10.1098/rstb.2002.1213
Silva, M. M. A., Albuquerque, A. M., and Araujo, J. F. (2005). Light-dark cycle synchronization of circadian rhythm in blind primates. J. Circadian Rhythms 3:10.
Simpson, J. I. (1984). The accessory optic system. Annu. Rev. Neurosci. 7, 13–41. doi: 10.1146/annurev.ne.07.030184.000305
Simpson, J. I., Leonard, C. S., and Soodak, R. E. (1988). The accessory optic system of rabbit. II. Spatial organization of direction selectivity. J. Neurophysiol. 60, 2055–2072. doi: 10.1152/jn.1988.60.6.2055
Simpson, J. I., Soodak, R. E., and Hess, R. (1979). The accessory optic system and its relation to the vestibulocerebellum. Prog. Brain Res. 50, 715–724. doi: 10.1016/S0079-6123(08)60868-7
Sincich, L. C., Park, K. F., Wohlgemuth, M. J., and Horton, J. C. (2004). Bypassing V1: A direct geniculate input to area MT. Nat. Neurosci. 7, 1123–1128. doi: 10.1038/nn1318
Skibbe, H., Watakabe, A., Nakae, K., Gutierrez, C. E., Tsukada, H., Hata, J., et al. (2019). Marmo Net: A pipeline for automated projection mapping of the common marmoset brain from whole-brain serial two-photon tomography. Tech. Rep. 1, 1–15. doi: 10.13140/RG.2.2.29979.21287
Smale, L., Blanchard, J., Moore, R. Y., and Morin, L. P. (1991). Immunocytochemical characterization of the suprachiasmatic nucleus and the intergeniculate leaflet in the diurnal ground squirrel, Spermophilus lateralis. Brain Res. 563, 77–86. doi: 10.1016/0006-8993(91)91517-5
Solomon, S. G., and Rosa, M. G. (2014). A simpler primate brain: The visual system of the marmoset monkey. Front. Neural Circuits 8:96. doi: 10.3389/fncir.2014.00096
Solomon, S. G., White, A. J., and Martin, P. R. (2002). Extraclassical receptive field properties of parvocellular, magnocellular, and koniocellular cells in the primate lateral geniculate nucleus. J. Neurosci. 22, 338–349. doi: 10.1523/JNEUROSCI.22-01-00338.2002
Sondereker, K. B., Stabio, M. E., and Renna, J. M. (2020). Crosstalk: The diversity of melanopsin ganglion cell types has begun to challenge the canonical divide between image-forming and non-image-forming vision. J. Comp. Neurol. 528, 2044–2067. doi: 10.1002/cne.24873
Sonoda, T., and Schmidt, T. M. (2016). Re-evaluating the role of intrinsically photosensitive retinal ganglion cells: New roles in image-forming functions. Integr. Comp. Biol. 56, 834–841. doi: 10.1093/icb/icw066
Sousa, M. B. C., and Ziegler, T. E. (1998). Diurnal variation on the excretion patterns of fecal steroids in common marmoset (Callithrix jacchus) females. Am. J. Primatol. 46, 105–117. doi: 10.1002/(SICI)1098-2345(1998)46:2<105::AID-AJP1>3.0.CO;2-#
Sparks, D. L., and Mays, L. E. (1990). Signal transformations required for the generation of saccadic eye movements. Ann. Rev. Neurosci. 13, 309–336. doi: 10.1146/annurev.ne.13.030190.001521
Spatz, W. B. (1975). Thalamic and other subcortical projections to area MT (visual area of superior temporal sulcus) in the marmoset Callithrix jacchus. Brain Res. 99, 129–134. doi: 10.1016/0006-8993(75)90614-9
Spatz, W. B. (1978). The retino-geniculo-cortical pathway in Callithrix. I. Intraspecific variations in the lamination pattern of the lateral geniculate nucleus. Exp. Brain Res. 33, 551–563. doi: 10.1007/BF00235574
Stein, B. E., Magalhaes-Castro, B., and Kruger, L. (1976). Relationship between visual and tactile representations in cat superior colliculus. J. Neurophysiol. 39, 410–419. doi: 10.1152/jn.1976.39.2.401
Stepniewska, I. (2003). “The pulvinar complex,” in The primate visual system, eds J. Kaas and C. Collins (Boca Raton, FL: CRC Press), 53–80.
Stepniewska, I., and Kaas, J. H. (1997). Architectonic subdivisions of the inferior pulvinar in new world and old world monkeys. Vis. Neurosci. 14, 1043–1060. doi: 10.1017/s0952523800011767
Sun, L., Liu, R., Guo, F., Wen, M. Q., Ma, X. L., Li, K. Y., et al. (2020). Parabrachial nucleus circuit governs neuropathic pain-like behavior. Nat. Commun. 11:5974. doi: 10.1038/s41467-020-19767-w
Sun, L. O., Brady, C. M., Cahill, H., Al-Khindi, T., Sakuta, H., Dhande, O. S., et al. (2015). Functional assembly of accessory optic system circuitry critical for compensatory eye movements. Neuron 86, 971–984. doi: 10.1016/j.neuron.2015.03.064
Szkudlarek, H. J., Orlowska, P., and Lewandowski, M. H. (2012). Light-induced responses of slow oscillatory neurons of the rat olivary pretectal nucleus. PLoS One 7:e33083. doi: 10.1371/journal.pone.0033083
Szmajda, B. A., Buzás, P., Fitzgibbon, T., and Martin, P. R. (2006). Geniculocortical relay of blue-off signals in the primate visual system. Proc. Natl. Acad. Sci. U.S.A. 103, 19512–19517. doi: 10.1073/pnas.0606970103
Szmajda, B. A., Grünert, U., and Martin, P. R. (2005). Mosaic properties of midget and parasol ganglion cells in the marmoset retina. Vis. Neurosci. 22, 395–404. doi: 10.1017/S0952523805224021
Szmajda, B. A., Grünert, U., and Martin, P. R. (2008). Retinal ganglion cell inputs to the koniocellular pathway. J. Comp. Neurol. 510, 251–268. doi: 10.1002/cne.21783
Tailby, C., Cheong, S. K., Pietersen, A. N., Solomon, S. G., and Martin, P. R. (2012). Colour and pattern selectivity of receptive fields in superior colliculus of marmoset monkeys. J. Physiol. 590, 4061–4077. doi: 10.1113/jphysiol.2012.230409
Takaji, M., Takemoto, A., Yokoyama, C., Watakabe, A., Mizukami, H., Ozawa, K., et al. (2016). Distinct roles for primate caudate dopamine D1 and D2 receptors in visual discrimination learning revealed using shRNA knockdown. Sci. Rep. 6:35809. doi: 10.1038/srep35809
Tanaka, M. (2007). Spatiotemporal properties of eye position signals in the primate central thalamus. Cereb. Cortex 17, 1504–1515. doi: 10.1093/cercor/bhl061
Tanibuchi, I., and Goldman-Rakic, P. S. (2003). Dissociation of spatial-, object-, and sound-coding neurons in the mediodorsal nucleus of the primate thalamus. J. Neurophysiol. 89, 1067–1077. doi: 10.1152/jn.00207.2002
Tessoneaud, A., Cooper, H. M., Caldani, M., Locatelli, A., and Viguier-Martinez, M. C. (1994). The suprachiasmatic nucleus in the sheep: Retinal projections and cytoarchitectural organization. Cell Tissue Res. 278, 65–84. doi: 10.1007/BF00305779
Théoret, H., Boire, D., and Ptito, M. (2000). Retinal projections to the pregeniculate nucleus in the hemispherectomized monkey. Brain Res. Bull. 53, 239–243. doi: 10.1016/s0361-9230(00)00345-2
Thompson, A., Gribizis, A., Chen, C., and Crair, M. C. (2017). Activity-dependent development of visual receptive fields. Curr. Opin. Neurobiol. 42, 136–143. doi: 10.1016/j.conb.2016.12.007
Tigges, J., Bos, J., and Tigges, M. (1977). An autoradiographic investigation of the subcortical visual system in chimpanzee. J. Comp. Neurol. 172, 367–380. doi: 10.1002/cne.901720211
Tigges, J., and Tigges, M. (1981). Distribution of retinofugal and corticofugal axon terminals in the superior colliculus of squirrel monkey. Invest. Ophthalmol. Vis. Sci. 20, 149–158.
Timbie, C., and Barbas, H. (2015). Pathways for emotions: Specializations in the amygdalar, mediodorsal thalamic, and posterior orbitofrontal network. J. Neurosci. 35, 11976–11987. doi: 10.1523/JNEUROSCI.2157-15.2015
Timurkaan, S., Karan, M., Aydın, A., and Gür, F. M. (2013). Immunohistochemical localization of serotonin in the superior colliculus of porcupine (Hystrix cristata). Qscience Connect 2013:5. doi: 10.5339/connect.2013.5
Tokita, K., Inoue, T., and Boughter, J. D. Jr. (2009). Afferent connections of the parabrachial nucleus in C57BL/6J mice. Neuroscience 161, 475–488. doi: 10.1016/j.neuroscience.2009.03.046
Torborg, C. L., and Feller, M. B. (2005). Spontaneous patterned retinal activity and the refinement of retinal projections. Prog. Neurobiol. 76, 213–235. doi: 10.1016/j.pneurobio.2005.09.00
Tracey, I., Ploghaus, A., Gati, J. S., Clare, S., Smith, S., Menon, R. S., et al. (2002). Imaging attentional modulation of pain in the periaqueductal gray in humans. J. Neurosci. 22, 2748–2752. doi: 10.1523/JNEUROSCI.22-07-02748.2002
Updyke, B. V. (1974). Characteristics of unit responses in superior colliculus of the Cebus monkey. J. Neurophysiol. 37, 896–909. doi: 10.1152/jn.1974.37.5.896
Usrey, W. M., Reppas, J. B., and Reid, R. C. (1999). Specificity and strength of retinogeniculate connections. J. Neurophysiol. 82, 3527–3540. doi: 10.1152/jn.1999.82.6.3527
Van den Pol, A. N. (1980). The hypothalamic suprachiasmatic nucleus of rat: Intrinsic anatomy. J. Comp. Neurol. 191, 661–702. doi: 10.1002/cne.901910410
Van den Pol, A. N. (1991). Glutamate and aspartate immunoreactivity in hypothalamic presynaptic axons. J. Neurosci. 11, 2087–2101. doi: 10.1523/JNEUROSCI.11-07-02087.1991
Van der Werf, Y. D., Witter, M. P., and Groenewegen, H. J. (2002). The intralaminar and midline nuclei of the thalamus. Anatomical and functional evidence for participation in processes of arousal and awareness. Brain Res. Rev. 39, 107–140. doi: 10.1016/s0165-0173(02)00181-9
Vaze, K. M., and Sharma, V. K. (2013). On the adaptive significance of circadian clocks for their owners. Chronobiol. Int. 30, 413–433. doi: 10.3109/07420528.2012.754457
Vertes, R. P., and Hoover, W. B. (2008). Projections of the paraventricular and paratenial nuclei of the dorsal midline thalamus in the rat. J. Comp. Neurol. 508, 212–237. doi: 10.1002/cne.21679
Vertes, R. P., Linley, S. B., and Rojas, A. K. P. (2022). Structural and functional organization of the midline and intralaminar nuclei of the thalamus. Front. Behav. Neurosci. 16:964644. doi: 10.3389/fnbeh.2022.964644
Villalobos, C. A., Wu, Q., Lee, P. H., May, P. J., and Basso, M. A. (2018). Parvalbumin and GABA microcircuits in the mouse superior colliculus. Front. Neural Circuits 12:35. doi: 10.3389/fncir.2018.00035
Vosshall, L. B., Price, J. L., Sehgal, A., Saez, L., and Young, M. W. (1994). Block in nuclear localization of period protein by a second clock mutation, timeless. Science 263, 1606–1609. doi: 10.1126/science.8128247
Vujovic, N., Gooley, J. J., Jhou, T. C., and Saper, C. B. (2015). Projections from the subparaventricular zone define four channels of output from the circadian timing system. J. Comp. Neurol. 523, 2714–2737. doi: 10.1002/cne.23812
Wallace, D. J., Fitzpatrick, D., and Kerr, J. N. (2016). Primate thalamus: More than meets an eye. Curr. Biol. 26, R60–R61. doi: 10.1016/j.cub.2015.11.025
Warland, D. K., Huberman, A. D., and Chalupa, L. M. (2006). Dynamics of spontaneous activity in the fetal macaque retina during development of retinogeniculate pathways. J. Neurosci. 26, 5190–5197. doi: 10.1523/JNEUROSCI.0328-06.2006
Warner, C. E., Goldshmit, Y., and Bourne, J. A. (2010). Retinal afferents synapse with relay cells targeting the middle temporal area in the pulvinar and lateral geniculate nuclei. Front. Neuroanat. 4:8. doi: 10.3389/neuro.05.008.2010
Wässle, H. (2004). Parallel processing in the mammalian retina. Nat. Rev. Neurosci. 5, 747–757. doi: 10.1038/nrn1497
Wässle, H., and Illing, R. B. (1980). The retinal projection to the superior colliculus in the cat: A quantitative study with HRP. J. Comp. Neurol. 190, 333–356. doi: 10.1002/cne.901900208
Weber, J. T., and Giolli, R. A. (1986). The medial terminal nucleus of the monkey: Evidence for a “complete” accessory optic system. Brain Res. 365, 164–168.
Wechselberger, E., and Erkert, H. G. (1994). Characteristics of the light induced phase response of circadian activity rhythms in common marmoset, Callithrix j. jacchus (Primates Cebidae). Chronobiol. Int. 11, 275–284. doi: 10.3109/07420529409057243
Welsh, D. K., Takahashi, J. S., and Kay, S. A. (2010). Suprachiasmatic nucleus: Cell autonomy and network properties. Annu. Rev. Physiol. 72, 552–577. doi: 10.1146/annurev-physiol-021909-135919
Weyand, T. G. (2016). The multifunctional lateral geniculate nucleus. Rev. Neurosci. 27, 135–157. doi: 10.1515/revneuro-2015-0018
White, A. J., Wilder, H. D., Goodchild, A. K., Sefton, A. J., and Martin, P. R. (1998). Segregation of receptive field properties in the lateral geniculate nucleus of a new-world monkey, the marmoset Callithrix jacchus. J. Neurophysiol. 80, 2063–2076. doi: 10.1152/jn.1998.80.4.2063
Whitney, M. S., Shemery, A. M., Yaw, A. M., Donovan, L. J., Glass, J. D., and Deneris, E. S. (2016). Adult brain serotonin deficiency causes hyperactivity, circadian disruption, and elimination of siestas. J. Neurosci. 36, 9828–9842. doi: 10.1523/JNEUROSCI.1469-16.2016
Wiesel, T. N., and Hubel, D. H. (1966). Spatial and chromatic interactions in the lateral geniculate body of the rhesus monkey. J. Neurophysiol. 29, 1115–1156. doi: 10.1152/jn.1966.29.6.1115
Wilder, H. D., Grünert, U., Lee, B. B., and Martin, P. R. (1996). Topography of ganglion cells and photoreceptors in the retina of a new world monkey: The marmoset Callithrix jacchus. Vis. Neurosci. 13, 335–352. doi: 10.1017/S0952523800007586
Wilson, D. A., Xu, W., Sadrian, B., Courtiol, E., Cohen, Y., and Barnes, D. C. (2014). Cortical odor processing in health and disease. Prog. Brain Res. 208, 275–305. doi: 10.1016/B978-0-444-63350-7.00011-5
Wu, Y., Enoki, R., Oda, Y., Huang, Z., Homna, K., and Homna, S. (2018). Ultradian calcium rhythms in the paraventricular nucleus and subparaventricular zone in the hypothalamus. Proc. Natl. Acad. Sci. U.S.A. 115, E9469–E9478. doi: 10.1073/pnas.1804300115
Wurtz, R. H., and Albano, J. E. (1980). Visual-motor function of the primate superior colliculus. Annu. Rev. Neurosci. 3, 189–226. doi: 10.1146/annurev.ne.03.030180.001201
Wurtz, R. H., and Goldberg, M. E. (1972). Activity of superior colliculus in behaving monkey. III. Cells discharging before eye movements. J. Neurophysiol. 135, 575–586. doi: 10.1152/jn.1972.35.4.575
Wyder, M. T., Massoglia, D. P., and Stanford, T. R. (2003). Quantitative assessment of the timing and tuning of visual-related, saccade-related, and delay period activity in primate central thalamus. J. Neurophysiol. 90, 2029–2052. doi: 10.1152/jn.00064.2003
Xiao, D., Zikopoulos, B., and Barbas, H. (2009). Laminar and modular organization of prefrontal projections to multiple thalamic nuclei. Neuroscience 161, 1067–1081. doi: 10.1016/j.neuroscience.2009.04.034
Xie, Y., Tang, Q., Chen, G., Xie, M., Yu, S., Zhao, J., et al. (2019). New insights into the circadian rhythm and its related diseases. Front. Physiol. 10:682. doi: 10.3389/fphys.2019.00682
Xu, J., Tang, Y., Qi, H., Yu, X., Liu, M., Wang, N., et al. (2019). Electrophysiological properties of thermosensitive neurons in slices of rat lateral parabrachial nucleus. J. Therm. Biol. 83, 87–94. doi: 10.1016/j.jtherbio.2019.05.020
Xue, J. T., Ramoa, A. S., Carney, T., and Freeman, R. D. (1987). Binocular interaction in the dorsal lateral geniculate nucleus of the cat. Exp. Brain Res. 68, 305–310. doi: 10.1007/BF00248796
Yahiro, T., Kataoka, N., Nakamura, Y., and Nakamura, K. (2017). The lateral parabrachial nucleus, but not the thalamus, mediates thermosensory pathways for behavioural thermoregulation. Sci. Rep. 7:5031. doi: 10.1038/s41598-017-05327-8
Youngstrom, T. G., Weiss, M. L., and Nunez, A. A. (1991). Retinofugal projections to the hypothalamus anterior hypothalamus and basal forebrain in hamsters. Brain Res. Bull. 5, 486–500. doi: 10.1016/0361-9230(91)90014-b
Zeater, N., Buzás, P., Dreher, B., Grünert, U., and Martin, P. R. (2018). Projections of three subcortical visual centers to marmoset lateral geniculate nucleus. J. Comp. Neurol. 527, 535–545. doi: 10.1002/cne.24390
Zeater, N., Cheong, S. K., Solomon, S. G., Dreher, B., and Martin, P. R. (2015). Binocular visual responses in the primate lateral geniculate nucleus. Curr. Biol. 25, 3190–3195. doi: 10.016/j.cub.2015.10.033
Zehring, W. A., Wheeler, D. A., Reddy, P., Konopka, R. J., Kyriacou, C. P., Rosbash, M., et al. (1984). P-element transformation with period locus DNA restores rhythmicity to mutant, arrhythmic Drosophila melanogaster. Cell 39, 369–376.
Zhang, P., Jiang, Y., and He, S. (2012). Voluntary attention modulates processing of eye-specific visual information. Psychol. Sci. 23, 254–260. doi: 10.1177/0956797611424289
Keywords: retinal projection, marmoset (Callithrix jacchus), image forming system, non-image forming system, retinorecipient areas
Citation: Santana NNM, Silva EHA, dos Santos SF, Costa MSMO, Nascimento Junior ES, Engelberth RCJG and Cavalcante JS (2023) Retinorecipient areas in the common marmoset (Callithrix jacchus): An image-forming and non-image forming circuitry. Front. Neural Circuits 17:1088686. doi: 10.3389/fncir.2023.1088686
Received: 03 November 2022; Accepted: 10 January 2023;
Published: 02 February 2023.
Edited by:
Sarah L. Pallas, University of Massachusetts Amherst, United StatesReviewed by:
William David Todd, University of Wyoming, United StatesNewton Sabino Canteras, University of São Paulo, Brazil
Copyright © 2023 Santana, Silva, dos Santos, Costa, Nascimento Junior, Engelberth and Cavalcante. This is an open-access article distributed under the terms of the Creative Commons Attribution License (CC BY). The use, distribution or reproduction in other forums is permitted, provided the original author(s) and the copyright owner(s) are credited and that the original publication in this journal is cited, in accordance with accepted academic practice. No use, distribution or reproduction is permitted which does not comply with these terms.
*Correspondence: Jeferson S. Cavalcante, amVmc2NAdW9sLmNvbS5icg==