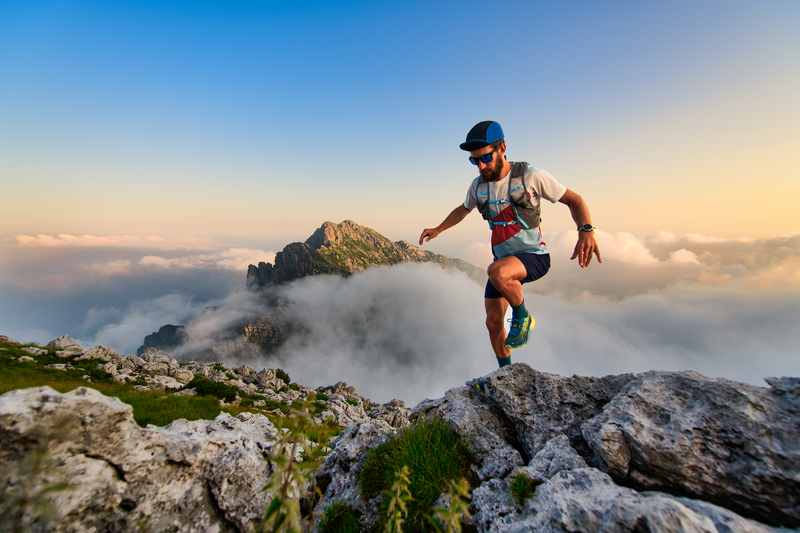
95% of researchers rate our articles as excellent or good
Learn more about the work of our research integrity team to safeguard the quality of each article we publish.
Find out more
PERSPECTIVE article
Front. Cell. Neurosci. , 14 November 2019
Sec. Non-Neuronal Cells
Volume 13 - 2019 | https://doi.org/10.3389/fncel.2019.00504
This article is part of the Research Topic Linking Neuroinflammation and Glial Phenotypic Changes in Neurological Diseases View all 15 articles
Microglia were previously regarded as a homogenous myeloid cell lineage in the mammalian central nervous system (CNS). However, accumulating evidences show that microglia in the brain and SC are quite different in development, cellular phenotypes and biological functions. Although this is a very interesting phenomenon, the underlying mechanisms and its significance for neurological diseases in association with behavioral and cognitive changes are still unclear. How microglia differ between these two regions and whether such diversity may contribute to CNS development and functions as well as neurological diseases will be discussed in this Perspective.
Microglia are important for building and defending the central nervous system (CNS) (Tay et al., 2017) are considered the most dynamic glial cell type in the CNS to sense and adapt to their surroundings, thereby giving them a complex feature of heterogeneity (Nayak et al., 2014). However, the implication of spatial heterogeneity of microglia in normal CNS functions and diseases is still evasive. Although the past years have witnessed a dramatic growth of attention on microglial functions in the CNS, evidence available for this Perspective is rather limited, because most microglial studies have investigated only the brain and have used disease models concerning the brain or the spinal microglial functions separately, and a direct comparison between the brain and the spinal cord (SC) under basal or diseased condition is rare. The aim of this paper is hence to provide an updated available knowledge on microglial differences between the brain and the SC and to discuss its potential application for treating neurological diseases. Besides region-specific features, microglia are also increasingly known to differ temporally and between genders, but these other heterogenous aspects will not be focused here and readers are referred to other recent reviews (Mapplebeck et al., 2016; Silvin and Ginhoux, 2018; Rahimian et al., 2019).
Using various immunohistochemical markers for microglia, including tomato lectin, CD45, CD68, major histocompatibility complex (MHC)-II, CD11b/c (OX-42), and ionized calcium-binding adaptor molecule-1 (Iba1), researchers have observed generally similar developmental aspects of microglia in their entrance and colonization in the brain and the SC, but minor differences exist (Rezaie and Male, 1999). In a study comparing cell populations in the lumbar locomotor region of the spinal cord and in the brainstem motor nucleus of new-born rats, Cifra et al. (2012) observed less than 10% of microglia in the neonate brainstem but very few microglia in the spinal gray matter (Table 1). Moreover, an earlier study discovered that microglial density in the adult colony-stimulating factor (CSF)1-deficient mice was specifically decreased by 86.4% in the white matter tract of the spinal dorsal column compared with that in age-matched wild-type controls, whereas the decrease was much less (24.0%) in the gray matter of cerebral cortex (Kondo and Duncan, 2009). This suggests that spinal microglia respond to CSF1-dependent cell proliferation more sensitively than cortical microglia, but whether this is due to the differential expression of CSF1 and/or its receptor in different CNS regions is unclear. A very recent study comparing cerebellar and cerebral microglia in CSF1-deficient mice also confirmed that CSF1 depletion did not affect microglia in the forebrain (Kana et al., 2019). Nevertheless, CSF1 is more enriched in the brain than the spinal cord. IL-34, an alternative ligand for CSF1R, is also highly expressed in the brain compared to the spinal cord and is critical for developing cerebral microglia, in contrast to CSF1 (Greter et al., 2012; Wang et al., 2012). However, whether it is dispensable for spinal microglia development or not is unclear. In another recent study using a diphtheria toxin receptor (DTR)-based genetic depletion approach for adult mice, Bruttger et al., 2015) further revealed that while microglia were rapidly ablated in all studied areas, e.g., the cortex, cerebellum and SC, within 3 days, residual microglia recovered more rapidly in the SC than in the cortex within 7 days, providing another evidence on the sensitivity of spinal microglia to changes in environmental cues.
Importantly, much of the knowledge on developmental features of microglia in the SC comes from previous studies on human post-mortem samples (Table 1). In the developing human CNS, both brain and SC microglial progenitors are suggested to enter their respective eminence from the meninges (Rezaie and Male, 1999; Monier et al., 2007). However, Rezaie and Male (1999) found that human amoeboid-like microglia-macrophages appeared in the SC at gestational weeks (GW)9 and peaked at GW16, which is later than entrance in the cerebrum at GW4-8 as described by Monier et al. (2007). Morphologically, Hutchins et al. found that during GW18-24, gray matter microglia were ramified while white matter microglia were amoeboid in the SC (Hutchins et al., 1992). Since morphological maturation of microglia- macrophage precursors stages a step-wise amoeboid, intermediate, and ramified transition (Verney et al., 2010), this indicates an inside-out migratory route of microglia-macrophage progenitors in the SC, which also resembles the early developmental cerebrum (Monier et al., 2007; Verney et al., 2010). McKay et al. found that morphologies of microglia even differed between the dorsal and ventral horns within the spinal gray matter in adult rats (McKay et al., 2007), with both microglial size (length between the tips of the two longest processes through the soma) and soma area smaller in the dorsal horn (DH) than in the ventral horn.
Other microglial phenotypes may also differ from those in the brain at adulthood (Table 1). Fundamental gene expression differences in microglia between the brain and SC have been particularly noticed. For instance, de Haas et al. made a pilot comparison of the protein expression of several microglial molecules among the mouse hippocampus, cerebral cortex, cerebellum and SC, and observed that levels of CD11b, CD45, CD86, and CCR9 were higher in the SC as compared to other regions (de Haas et al., 2008). A later study similarly found higher levels of several immune molecules on spinal microglia than their counterparts in the brain both at steady state and upon viral infection (Olson, 2010). We previously also confirmed that microglial expression of CD11b/c and MHCII were constitutively higher in the SC than in the cerebral cortex and thalamus, which conversely expressed more abundantly CD172a (SIRPα), an inhibitory immune receptor (Li et al., 2016).
Using genome-wide chromatin gene expression profiling and/or single-cell RNA sequencing (scRNAseq) analysis, three recent pivotal studies included microglia in the SC (Matcovitch-Natan et al., 2016; Gosselin et al., 2017). Matcovitch-Natan et al. (2016) provided seminal evidence that microglia underwent a three-step developmental program - early, pre-, and adult microglia across the mouse brain. Gosselin et al. (2017) examined the transcriptomes and epigenetic landscapes of human microglia isolated from surgically resected brain tissue ex vivo and after transition to an in vitro environment, and studied transcriptomes of various mouse CNS regions. However, neither study specifically addressed regional aspects of spinal microglial transcriptomic features in development and adulthood. In the study of Gosselin et al., gene family members of Fos and Kif seemed to be more enriched in the SC than the brain as a function of development in mice (Gosselin et al., 2017). Very recently, Masuda et al. (2019) made a thorough scRNAseq analysis of mouse microglia across different CNS regions, including the SC, in embryonic, juvenile and adult stages and found that compared to juvenile microglia, adult microglial clusters showed a more homogenous distribution across regions. Although the authors did not focus on the SC in particular either, their results demonstrated that a minor cluster (C7, representing CST3–SPARC–IBA1+ microglia) was more prevalent in the juvenile SC and cerebellum as well as in the adult cerebellum and corpus callosum compared to the cortex and hippocampus (Masuda et al., 2019). These studies nevertheless provide some first clues on whether differentiation of microglia is orchestrated synchronically in different CNS regions across different developmental stages.
It is still unclear what underlies the fundamental difference between spinal and brain microglia and whether this is mainly caused by intrinsic or extrinsic factors (Figure 1). Guiding cues that drive the differences of microglia between the brain and the SC may come from the peripheral circulation, as blood-derived molecules penetrate the blood-brain barrier (BBB) and the blood-SC barrier (BSCB) in different manners (Abbott et al., 2010; Bartanusz et al., 2011). The BSCB is generally considered as the morphological extension of the BBB into the SC. Nevertheless, evidences suggest that structural and functional differences exist between them, so that the BSCB has higher permeability and lower expressions of tight junction proteins, such as ZO-1 and Occludin, and adherence junction proteins, such as VE-cadherin and β-catenin (reviewed in Bartanusz et al., 2011). It is noteworthy that glial cell types themselves may contribute to the morphological and functional differences between the BBB and BSCB.
Although unconfirmed yet, it is highly expected that basal differences of microglia between the SC and the brain may contribute to the onset, development and treatment response of respective CNS diseases. Such differences are parallel to the facts that the brain and the SC play non-redundant roles in high-order functions of vertebrates and hence require different neurotransmission signals and regional connections. Knowledge on spinal microglia-mediated neuroinflammation and in comparison to brain microglia, however, has been mostly derived from studies on neurological conditions so far, namely traumatic brain injury (TBI), spinal cord injury (SCI), as well as spinal nerve injury models (Details on the respective models are provided in these recent reviews (Zhang and Gensel, 2014; Gensel and Zhang, 2015; Hu et al., 2015; Martini and Willison, 2016; Jassam et al., 2017; Cox, 2018; Table 1). Subtle to dramatic pathological and neuroinflammatory differences exist among different models. For instance, TBI is regarded as a diffuse injury, whereas spinal cord injury and stroke are anatomically discrete (Cox, 2018). Whether microglia contribute to such differences is currently unknown. In a recent study using spared nerve injury (SNI) model in mice, Liu et al. showed opposite changes in dendritic lengths and spine densities between hippocampal CA1 pyramidal neurons and spinal neurons along with differential expression of hippocampal and spinal brain-derived neurotrophic factor (BDNF) after SNI, which was prevented by genetic deletion of tumor necrosis factor (TNF) receptor 1, a microglia-enriched cytokine receptor, and by inhibition or ablation of microglia (Liu et al., 2017). It is intriguing how the same TNF receptor can play opposite roles in regulation of neurons at different sites but differential microglial reaction after SNI would be expected. In line with this notion, using a similar SNI rat model, we earlier had observed that baseline differences of spinal and cortical microglia in CD172a+ and MHCII+ subpopulations as well as in expression of microglial activation genes underlined their differential responses to SNI as well as to the analgesic effect of minocycline, so that genes involved in M2 polarization and phagocytosis were upregulated in the spinal dorsal horn after SNI compared to Sham, but were downregulated in the cortex (Li et al., 2016). However, Liu et al. found that SNI increased TNF-α levels in both the hippocampus and the ipsilateral spinal dorsal horn compared with shams, which was also similarly dampened by minocycline treatment and by DTR-mediated genetic depletion of microglia (Liu et al., 2017), implying similar activation responses of spinal and hippocampal microglia to SNI. Causes for discrepancies in these studies need to be further examined.
Studies on TBI and SCI have reported that acute inflammatory response to traumatic injury is significantly greater in the SC than in the cerebral cortex. A more careful dissection on these differences was thoroughly provided in an earlier review (Zhang and Gensel, 2014) and therefore we will not reiterate here but only give a couple of exemplar evidences briefly. For instance, Schnell et al. originally found that myeloid recruitment to the lesion site and the surrounding parenchyma was significantly higher in the SC than in the brain. The area of BBB breakdown was substantially larger and vascular damage persisted longer in the SC (Schnell et al., 1999). A later study consistently found that, one week after mechanical injuries to both the gray and white matters, microglial response was significantly greater in the SC compared to the brain (Batchelor et al., 2008). In addition, a greater inflammatory response in the white matter compared to the gray matter within both the brain and SC was observed (McKay et al., 2007; Batchelor et al., 2008). In an in vitro cell culture study on morphological properties and secretion of inflammatory and trophic effectors by microglia derived from the brain or spinal cord of neonatal rats, Baskar Jesudasan et al. (2014) demonstrated that spinal microglia assumed a less inflammatory phenotype after lipopolysaccharide-induced activation, with reduced release of proinflammatory cytokines and nitric oxide, a less amoeboid morphology, and reduced phagocytosis relative to brain-derived microglia. These results suggest that local instead of global microglia-modulatory and/or anti-inflammatory strategies targeting microglia more specifically may be more valuable to reduce damages caused by local microglial activation in treating SC-related neurological diseases.
Brain microglia may be more important for regulating neuron-mediated cognition and emotion, whereas spinal microglia more relevant for controlling neuron-mediated sensory-motor functions. This may commit brain and spinal microglia to different CNS conditions, e.g., psychiatric and mental disorders versus sensory-motor neurological diseases. It is doubtless that more and more researchers are recognizing the existence of microglial regional specificity, but more vigorous investigations are still required for us to more deeply understand this interesting phenomenon.
All authors listed have made a substantial, direct and intellectual contribution to the work, and approved it for publication.
LT acknowledges the Beijing Municipal Science and Technology Commission No. Z171100001017021, and the Estonian Research Council-European Union Regional Developmental Fund Mobilitas Pluss Program No. MOBTT77.
The authors declare that the research was conducted in the absence of any commercial or financial relationships that could be construed as a potential conflict of interest.
Abbott, N. J., Patabendige, A. A., Dolman, D. E., Yusof, S. R., and Begley, D. J. (2010). Structure and function of the blood-brain barrier. Neurobiol. Dis. 37, 13–25.
Bartanusz, V., Jezova, D., Alajajian, B., and Digicaylioglu, M. (2011). The blood-spinal cord barrier: morphology and clinical implications. Ann. Neurol. 70, 194–206. doi: 10.1002/ana.22421
Baskar Jesudasan, S. J., Todd, K. G., and Winship, I. R. (2014). Reduced inflammatory phenotype in microglia derived from neonatal rat spinal cord versus brain. PLoS One 9:e99443. doi: 10.1371/journal.pone.0099443
Batchelor, P. E., Tan, S., Wills, T. E., Porritt, M. J., and Howells, D. W. (2008). Comparison of inflammation in the brain and spinal cord following mechanical injury. J. Neurotrauma 25, 1217–1225. doi: 10.1089/neu.2007.0308
Bruttger, J., Karram, K., Wortge, S., Regen, T., Marini, F., Hoppmann, N., et al. (2015). Genetic cell ablation reveals clusters of local self-renewing microglia in the mammalian central nervous system. Immunity 43, 92–106. doi: 10.1016/j.immuni.2015.06.012
Cifra, A., Mazzone, G. L., Nani, F., Nistri, A., and Mladinic, M. (2012). Postnatal developmental profile of neurons and glia in motor nuclei of the brainstem and spinal cord, and its comparison with organotypic slice cultures. Dev. Neurobiol. 72, 1140–1160. doi: 10.1002/dneu.20991
Cox, C. S. Jr. (2018). Cellular therapy for traumatic neurological injury. Pediatr. Res. 83, 325–332. doi: 10.1038/pr.2017.253
de Haas, A. H., Boddeke, H. W., and Biber, K. (2008). Region-specific expression of immunoregulatory proteins on microglia in the healthy CNS. Glia 56, 888–894. doi: 10.1002/glia.20663
Gensel, J. C., and Zhang, B. (2015). Macrophage activation and its role in repair and pathology after spinal cord injury. Brain Res. 1619, 1–11. doi: 10.1016/j.brainres.2014.12.045
Gosselin, D., Skola, D., Coufal, N. G., Holtman, I. R., Schlachetzki, J. C. M., Sajti, E., et al. (2017). An environment-dependent transcriptional network specifies human microglia identity. Science 356:eaal3222. doi: 10.1126/science.aal3222
Greter, M., Lelios, I., Pelczar, P., Hoeffel, G., Price, J., Leboeuf, M., et al. (2012). Stroma-derived interleukin-34 controls the development and maintenance of langerhans cells and the maintenance of microglia. Immunity 37, 1050–1060. doi: 10.1016/j.immuni.2012.11.001
Hu, X., Leak, R. K., Shi, Y., Suenaga, J., Gao, Y., Zheng, P., et al. (2015). Microglial and macrophage polarization-new prospects for brain repair. Nat. Rev. Neurol. 11, 56–64. doi: 10.1038/nrneurol.2014.207
Hutchins, K. D., Dickson, D. W., Rashbaum, W. K., and Lyman, W. D. (1992). Localization of microglia in the human fetal cervical spinal cord. Brain Res. Dev. Brain Res. 66, 270–273. doi: 10.1016/0165-3806(92)90091-a
Jassam, Y. N., Izzy, S., Whalen, M., McGavern, D. B., and El Khoury, J. (2017). Neuroimmunology of traumatic brain injury: time for a paradigm shift. Neuron 95, 1246–1265. doi: 10.1016/j.neuron.2017.07.010
Kana, V., Desland, F. A., Casanova-Acebes, M., Ayata, P., Badimon, A., Nabel, E., et al. (2019). CSF-1 controls cerebellar microglia and is required for motor function and social interaction. J. Exp. Med. 216, 2265–2281. doi: 10.1084/jem.20182037
Kondo, Y., and Duncan, I. D. (2009). Selective reduction in microglia density and function in the white matter of colony-stimulating factor-1-deficient mice. J. Neurosci. Res. 87, 2686–2695. doi: 10.1002/jnr.22096
Li, Z., Wei, H., Piirainen, S., Chen, Z., Kalso, E., Pertovaara, A., et al. (2016). Spinal versus brain microglial and macrophage activation traits determine the differential neuroinflammatory responses and analgesic effect of minocycline in chronic neuropathic pain. Brain Behav. Immun. 58, 107–117. doi: 10.1016/j.bbi.2016.05.021
Liu, Y., Zhou, L. J., Wang, J., Li, D., Ren, W. J., Peng, J., et al. (2017). TNF-alpha differentially regulates synaptic plasticity in the hippocampus and spinal cord by microglia-dependent mechanisms after peripheral nerve injury. J. Neurosci. 37, 871–881. doi: 10.1523/JNEUROSCI.2235-16.2016
Mapplebeck, J. C., Beggs, S., and Salter, M. W. (2016). Sex differences in pain: a tale of two immune cells. Pain 157(Suppl. 1), S2–S6. doi: 10.1097/j.pain.0000000000000389
Martini, R., and Willison, H. (2016). Neuroinflammation in the peripheral nerve: cause, modulator, or bystander in peripheral neuropathies? Glia 64, 475–486. doi: 10.1002/glia.22899
Masuda, T., Sankowski, R., Staszewski, O., Bottcher, C., Amann, L., Sagar, C., et al. (2019). Spatial and temporal heterogeneity of mouse and human microglia at single-cell resolution. Nature 566, 388–392. doi: 10.1038/s41586-019-0924-x
Matcovitch-Natan, O., Winter, D. R., Giladi, A., Vargas Aguilar, S., Spinrad, A., Sarrazin, S., et al. (2016). Microglia development follows a stepwise program to regulate brain homeostasis. Science 353:aad8670. doi: 10.1126/science.aad8670
McKay, S. M., Brooks, D. J., Hu, P., and McLachlan, E. M. (2007). Distinct types of microglial activation in white and grey matter of rat lumbosacral cord after mid-thoracic spinal transection. J. Neuropathol. Exp. Neurol. 66, 698–710. doi: 10.1097/nen.0b013e3181256b32
Monier, A., Adle-Biassette, H., Delezoide, A. L., Evrard, P., Gressens, P., and Verney, C. (2007). Entry and distribution of microglial cells in human embryonic and fetal cerebral cortex. J. Neuropathol. Exp. Neurol. 66, 372–382. doi: 10.1097/nen.0b013e3180517b46
Nayak, D., Roth, T. L., and McGavern, D. B. (2014). Microglia development and function. Annu. Rev. Immunol. 32, 367–402. doi: 10.1146/annurev-immunol-032713-120240
Olson, J. K. (2010). Immune response by microglia in the spinal cord. Ann. N. Y. Acad. Sci. 1198, 271–278. doi: 10.1111/j.1749-6632.2010.05536.x
Rahimian, R., Cordeau, P. Jr., and Kriz, J. (2019). Brain response to injuries: when microglia go sexist. Neuroscience 405, 14–23. doi: 10.1016/j.neuroscience.2018.02.048
Rezaie, P., and Male, D. (1999). Colonisation of the developing human brain and spinal cord by microglia: a review. Microsc. Res. Tech. 45, 359–382. doi: 10.1002/(sici)1097-0029(19990615)45:6<359::aid-jemt4>3.3.co;2-4
Schnell, L., Fearn, S., Klassen, H., Schwab, M. E., and Perry, V. H. (1999). Acute inflammatory responses to mechanical lesions in the CNS: differences between brain and spinal cord. Eur. J. Neurosci. 11, 3648–3658. doi: 10.1046/j.1460-9568.1999.00792.x
Silvin, A., and Ginhoux, F. (2018). Microglia heterogeneity along a spatio-temporal axis: more questions than answers. Glia 66, 2045–2057. doi: 10.1002/glia.23458
Tay, T. L., Savage, J. C., Hui, C. W., Bisht, K., and Tremblay, M. E. (2017). Microglia across the lifespan: from origin to function in brain development, plasticity and cognition. J. Physiol. 595, 1929–1945. doi: 10.1113/JP272134
Verney, C., Monier, A., Fallet-Bianco, C., and Gressens, P. (2010). Early microglial colonization of the human forebrain and possible involvement in periventricular white-matter injury of preterm infants. J. Anat. 217, 436–448. doi: 10.1111/j.1469-7580.2010.01245.x
Wang, Y., Szretter, K. J., Vermi, W., Gilfillan, S., Rossini, C., Cella, M., et al. (2012). IL-34 is a tissue-restricted ligand of CSF1R required for the development of Langerhans cells and microglia. Nat. Immunol. 13, 753–760. doi: 10.1038/ni.2360
Keywords: microglia, spinal cord, brain, neuroinflammation, neurological diseases
Citation: Xuan F-L, Chithanathan K, Lilleväli K, Yuan X and Tian L (2019) Differences of Microglia in the Brain and the Spinal Cord. Front. Cell. Neurosci. 13:504. doi: 10.3389/fncel.2019.00504
Received: 15 June 2019; Accepted: 25 October 2019;
Published: 14 November 2019.
Edited by:
Yu Tang, Central South University, ChinaReviewed by:
Hidetoshi Saitoh, Kyushu University, JapanCopyright © 2019 Xuan, Chithanathan, Lilleväli, Yuan and Tian. This is an open-access article distributed under the terms of the Creative Commons Attribution License (CC BY). The use, distribution or reproduction in other forums is permitted, provided the original author(s) and the copyright owner(s) are credited and that the original publication in this journal is cited, in accordance with accepted academic practice. No use, distribution or reproduction is permitted which does not comply with these terms.
*Correspondence: Li Tian, bGkudGlhbkB1dC5lZQ==
Disclaimer: All claims expressed in this article are solely those of the authors and do not necessarily represent those of their affiliated organizations, or those of the publisher, the editors and the reviewers. Any product that may be evaluated in this article or claim that may be made by its manufacturer is not guaranteed or endorsed by the publisher.
Research integrity at Frontiers
Learn more about the work of our research integrity team to safeguard the quality of each article we publish.