- 1Department of Agricultural and Environmental Sciences, College of Agriculture, Tennessee State University, Nashville, Tenn, United States
- 2Department of Life and Physical Sciences, Fisk University, Nashville, Tenn, United States
Introducing foreign DNA into bacterial cells is essential in functional genomics and molecular research. Currently, heat shock and electroporation are the two major techniques of gene delivery in bacterial cells. However, both the techniques are time and resource consuming and are limited to a few species or strains of bacteria and there is a need to develop new transformation alternatives. Carbon dots with unique features such as facile synthesis, ease of functionalization, nontoxicity, and biocompatibility are considered novel biomolecule nanocarriers. In this study, we synthesized and evaluated DNA delivery potential of four carbon dots including: 1) amine-coated carbon dots (NH2-FCDs); 2) carboxylate carbon dots (COOH-FCDs); 3) L-arginine and glucose carbon dots (N-CDs), and 4) citric acid and polyethyleneimine (PEI) carbon dots into Escherichia. coli cells. We evaluated the minimum incubation time required for the plasmid DNA delivery and the maximum plasmid size that can be delivered into E. coli cells using these CDs. Bacteria were incubated with carbon dots solution for different lengths of time and plated on selection media. Transformed colonies were counted and data were analyzed to identify the optimum incubation time and measure DNA delivery of these CDs with plasmids of different sizes. Our study demonstrated that among all these CDs, only carboxylate carbon dots (COOH-FCDs) prepared from glucosamine and β-alanine were able to deliver plasmid DNA into E. coli cells and the best incubation time was between 30 and 60 min. The maximum plasmid size that could be delivered using these CDs was approximately 10 kb and transformation efficiency decreased with larger plasmids. This study shows the capacity of COOH-CDs to deliver plasmid DNA into bacteria with an immense potential to combine with modern genome-editing tools. However, further studies are needed to evaluate their potential in DNA delivery in other bacterial strains.
Introduction
Gene delivery and DNA manipulation are essential in most of the functional genomics research and molecular activities including cloning, mutant generation, and construction of DNA libraries. The most commonly used artificial transformation methods in bacteria are: 1) heat shock method that incorporates CaCl2 treatment of bacterial cells and 2) electroporation (Divya et al., 2011). However, both these methods have drawbacks such as lengthy procedures in preparing the competent cells or the need for sophisticated instruments to generate electric shock. Moreover, numerous factors such as electric field strength, pulse decay time, temperature, pulse shape, plasmid size, and type of growth medium affect the transformation of electrocompetent cells. Therefore, there is a need for alternative less complicated DNA delivery methods that are not limited to only a few species of bacteria.
Recently, nanomaterials have gained popularity as a gene delivery tool into the human, animal, and plant cells (Liu et al., 2012; Schwartz et al., 2019; Sharma and Das, 2019; Zhang et al., 2020). Carbon dots (CDs) are zero dimensional photoluminescent nanoparticles that are smaller than 20 nm in diameter (Sun et al., 2006; Ponomarenko et al., 2008; Fernando et al., 2015; Sagbas and Sahiner, 2018). Xu et al. (2004) reported the synthesis of CDs for the first time while working on the purification of single-walled carbon nanotubes (SWCNT). Soon after this discovery, many scientists were attracted to carbon dots, which have similar functions to metal-based nanomaterials but are less toxic to the mammalian cells and the environment. Carbon dots can be synthesized from numerous sources such as carbon nanotubes (Rao et al., 2018; Wang et al., 2019), fullerenes (Lin et al., 2018), graphene (Clancy et al., 2018), and nano diamonds (Georgakilas et al., 2015). The physicochemical properties of the carbon dots depend on their method of synthesis in addition to the chemical composition, structure, and surface-coating molecules in their carbon source. For example, dispersibility of CDs is the result of surface functional groups whereas their toxicity is mainly due to their core region (Huang et al., 2015; Kim et al., 2018; Yao et al., 2019).
One of the most important properties of CDs is their photoluminescence property. Most of the time, they emit blue light upon excitation by UV irradiation (Javed and O’Carroll, 2021). However, photoluminescence wavelength can be tuned anywhere from blue to red, which makes CDs suitable for a variety of applications (Lim et al., 2015). Apart from photoluminescence properties, other favorable attributes of CDs, such as their facile preparation, ease of functionalization, tunable fluorescence excitations and emissions, photostability, nontoxicity, and biocompatibility, make them attractive nano particles in comparison with semiconductor quantum dots and toxic organic dyes (Baker and Baker, 2010; Sagbas and Sahiner, 2018). The application of CDs continues to expand in different disciplines including cell imaging, biosensing, cancer therapy, gene therapy, drug/gene delivery, and antimicrobial treatments (Lu et al., 2015; Shen and Liu, 2016; Yuan et al., 2016; Namdari et al., 2017; Peng et al., 2017; Rai et al., 2017; Sun and Lei, 2017; Liu et al., 2018; Sagbas and Sahiner, 2018). The ultrafine size of carbon nanocarriers allows better penetration of these CDs into the cells and tissues, and enables their delivery into various genetic materials and biomolecules (DNA, RNA, proteins, and small molecules) with higher specificity (Demirer et al., 2019a).
In this prospect, CDs have been evaluated as candidates for gene delivery into the bacterial cells. Earlier reports indicate the use of CDs in gene delivery in animal and plant cells. For instance, Zuo et al. (2018) developed fluorine-doped cationic CDs and used them to deliver EGFP and luciferase gene into HepG2, HEK 293T, COS-7, and NIH 3T3 cells. These CDs possessed superior efficiency and biocompatibility compared to commercial reagents such as Lipofectamine 2000. Similarly, Hasanzadeh et al. (2019) developed CD-PEI as traceable multicolor CDs and used them to deliver the plasmid, pCRISPR into the HEK 293 cells lines. Wang et al. (2014) synthesized CDs from citric acid and tryptophan and used them for siRNAs delivery and gene silencing into human gastric cancer cell line MGC-803. CDs are also used for the delivery of small interfering RNA into the model plants Nicotiana benthamiana and tomato (Schwartz et al., 2020).
Here, we report the synthesis of four different carbon dots including, 1) amine-coated carbon dots (NH2-FCDs); 2) carboxylate carbon dots (COOH-FCDs); 3) carbon dots from L-arginine and glucose (N-CDs); and 4) carbon dots from citric acid and PEI (Polyethyleneimine) and their gene delivery efficiency into E. coli cells. The concept of bacterial transformation using carbon dots is a novel approach and carries significant importance in recombinant DNA technology.
Materials and Methods
Synthesis of Carbon Dots
Four types of carbon dots (CDs) were synthesized from different precursors and their physical properties and gene delivery competencies for individual plasmid sizes were studied at distinct incubation times.
Materials: chemicals for carbon dots synthesis (Glucosamine HCl (GlcNH2·HCl, CAS# 66-84–2), 4,7,10-trioxa-1,13-tridecanediamine (TTDDA, CAS#4246-51–9), β-alanine (C3H7NO2, CAS# 107-95–9), glucose (C6H12O6, CAS# 50-99–7), L-arginine (C6H14N4O2, CAS# 74-79–3), citric acid (C6H8O7, CAS# 5,949-29–1), and polyethyleneimine (PEI) [(C37H24O6N2)n, CAS# 71-2,353] were purchased from VWR. Other components for the preparation of growth media such as bacteriological agar, yeast extract, tryptone, NaCl, proteose peptone, potassium phosphate dibasic, magnesium sulphate heptahydrate, glycerol, bactopeptone, and sterile water were ordered from VWR or Fisher Scientifics.
Synthesis of Amine-Coated Carbon Dots (NH2-FCDs)
Experimental setup: Amine-coated carbon dots were prepared according to Hill et al. (2016). Glucosamine hydrochloride (1.00 g, 4.63 mmol) was dissolved in distilled H2O (20 ml) and stirred to achieve complete dissolution in a 250 ml conical flask. 4,7,10-trioxa-1,13-tridecanediamine (TTDDA) (1.11 ml, 5.09 mmol) was then added to the sugar solution and agitated to ensure homogeneity. The conical flask was placed in a domestic microwave (Model#JES2251SJ02, GE Appliance, Canada), and heated for 3 min at 700 W power. The obtained viscous brown residue was dissolved in 10 ml distilled H2O and centrifuged for 3 min at 8,500 rpm. The solution was then purified by tube dialysis for 4 days using Spectra/Por® 7 Dialysis Membrane with 11.5 mm diameter. Dialysis water was refreshed every 2 h for the first 8 h, and once a day for the remaining 4 days. The final purified CDs were further sterilized by passing the solution through 0.22 µm filter.
Synthesis of Carboxylate Carbon Dots (COOH-FCDs)
Experimental setup: Carboxylated carbon dots were prepared according to Hill et al. (2016). Glucosamine hydrochloride (1.00 g, 4.63 mmol) was dissolved in distilled H2O (20 ml) and stirred to achieve complete dissolution in a 250 ml conical flask. β-alanine (0.45 g, 5.09 mmol) was then added to the sugar solution and agitated to ensure homogeneity. The conical flask was then placed in a domestic microwave and heated for 3 min at 700 W power. A viscous brown residue was obtained and dissolved in distilled H2O (10 ml) and centrifuged for 3 min at 8,500 rpm. The solution was purified by tube dialysis for 4 days using Spectra/Por® 7 Dialysis Membrane with 11.5 mm diameter. The dialysis water was refreshed every 2 h for the first 8 h, then once a day for the remaining 4 days. Then, the purified CDs were further sterilized by passing the solution through a filter with 0.22 µm pore size. Other carbon dots including NH2-FCDs (Glucosamine + TTDDA), N-CDs (Glucose + L-arginine), and PEI-CDs (Citric acid + PEI) were also synthesized according to Hill et al. (2016), Cao et al. (2018), and Wu et al. (2018), respectively
Characterization of CDs
FTIR analyses were used to confirm the presence of functional groups on the carbon dots. The attenuated Total Reflection-FTIR analysis of the freeze-dried CDs was carried out using a Perkin Elmer Frontier Infrared spectrometer equipped with a liquid N2-cooled MCT-A (mercury cadmium telluride) detector and an optics compartment purged with CO2- and H2O-free air delivered by a Balston-Parker air purger. The FTIR spectra of the CDs were recorded between 600 and 3,800 cm−1. Furthermore, photoluminescence properties such as absorbance, emission, and excitation wavelength of the CDs were recorded using Synergy H1 Hybrid Multi-Mode Microplate Reader (BioTek, Winooski, VT). Zetasizer nano zs (Malvern Panalytical Inc., Westborough, MA) was used to measure the electrostatic charges carried by CDs.
Preparation of Bacterial Culture and Growth Media
Bacterial Culture
E. coli DH5α was cultured in the LB medium at 37°C and harvested at OD600 of 0.5. To remove the bacteria from the culture media, 5 ml of culture was spun down and washed three times with sterile distilled water and the pellet eluted in 1 ml of 15% glycerol. Fifty μl of the cells were further aliquoted into 0.65 ml tubes and stored at −80 C freezer for future use.
Gene Delivery
Four plasmids of different sizes were used for this experiment including pPSU1 (Addgene #89439, 10,000 bp), pPSU2 (Addgene #89566, 7,750 bp, gifts from Song Tan), CAS9-pMDC123 (Addgene #59184, 13,658 bp, gift from Robert Stupar), and pU6-pegRNA-GG-acceptor (Addgene # 132,777, 3,004 bp, gift from David Liu). One day old bacterial culture was thawed on ice and 2 μg of plasmid was added to each tube. Five μl of each CD (10 mg/ml) was added to each tube and the reaction mixture was incubated for different time intervals (5, 15, 30, 60 min, and 4 h) under white light in the fume hood. Then, the cells were plated on LB agar supplemented with ampicillin (100 mg/ml) in three replicates per treatment and grown overnight at 37°C. The following day, delivery efficiency was confirmed by counting the number of colonies in each plate (in case of pU6-pegRNA-GG-acceptor plasmid the colonies are in red color due to the presence of RFP reporter gene). Identity of colonies and positive confirmation was carried out using specific primers to each plasmid and PCR on five randomly selected colonies.
Confirmation of Transformed Colonies Using Colony PCR
Each single colony was diluted in 10 μL of double-distilled water and 1 μL of the diluted mixture was used for colony PCR, using M13 forward and M13 reverse universal primers. The DNA was amplified in a C1000 touch thermal cycler (Bio-Rad, Hercules, California, United States), programmed for one cycle of 95°C for 2 min, followed by 35 cycles of 95°C for 10 s, 55°C annealing temperature for 45 s, and 72°C of elongation for 30 s, followed by a final extension at 72°C for 10 min. 10 µL of PCR product was loaded on a 1% agarose gel, ran for 40 min at 100 V, and visualized and documented under ultraviolet light in Gel-Doc XR + system (Bio-Rad, Hercules, California, United States).
Statistical Analysis
The number of transformed colonies was counted using ImageJ software and analyzed in R software (V3.6.3). The statistical analysis was used to compare the variation in the number of colonies at different time intervals and plasmid sizes. Post hoc analysis was carried out using the TukeyHSD test to compare the mean difference between time intervals, plasmid sizes, and effect of light and temperature on gene delivery.
Results
FTIR Analysis of CDs
FTIR analyses were performed to confirm the functional groups of CDs. The spectral graph of FTIR is divided into four different regions: 1) the single-bond region (2,500–4,000 cm−1), 2) the triple-bond region (2000–2,500 cm−1), 3) the double-bond region (1,500–2000 cm−1), and 4) the fingerprint region (600–1,500 cm−1).
The FTIR results for NH2-FCDs showed peaks at 870.2 cm−1 (C=O) of double olefinic bonds in single vinyl (C=CH2), 1,461 cm−1 (C=N) of Nitrile group, 1,616 cm−1 (C=O) of ketone group, 2,867 cm−1 (C=OH) of Aldehyde group, and 3,255 cm−1 (-OH) of hydroxyl group (Nandiyanto et al., 2019) (Supplementary Figure S1). In the COOH-FCDs, distinct FTIR peaks were obtained at 3,340 cm−1 indicative of various amino and alcohol groups (Supplementary Figure S2). Similarly, peaks were observed around 1715 cm−1 of ketone (C=O) stretching vibrations, 1,627 cm−1 of amide groups and its surface passivation, 1,570 cm−1 showing strong N-H bending vibration peaks. The skeletal C-C vibrations of the residual carbohydrates were seen around 1,039 cm−1 to 1,231 cm−1. In L-Arginine and glucose (N-CDs), the peaks were observed at 3,903 cm−1 indicating the presence of hydroxyl ions, amino groups, and hydrate molecules (Supplementary Figure S3). Likewise, peaks were observed around 1,594 cm−1 indicating the presence of carbonyl group (C=C), amino group (C=N), and various aromatic rings. These aromatic rings are usually followed by the existence of weak-to-moderate absorption in the area 3,100 cm−1. In Citric acid and polyethyleneimine, the FTIR spectra showed an N-H bending peak at 1,500 cm−1, and O stretching vibration at 1700 cm−1 (C=O) indicating the ketone groups, characteristic absorption bands of O–H and N–H stretching vibrations at 3,367 indicating the characteristics of O-H and N-H stretching, which is a characteristic of citric acid and PEI. The other peak was observed at 2,889 indicating the presence of carbonyl C=C and nitrile C=N and various aromatic stretching. Similarly, peak band at 1,073 represents cyclohexane ring vibration (Supplementary Figure S4).
Photoluminescence Properties of Carbon Dots
Carbon-based quantum dots (CDs) are nanosized autofluorescent particles with a high quantum yield (QY). The photoluminescence properties for all the CDs used in this study showed an absorbance profile maximum of 280–285 nm, which fits in the range of 200-450 nm specific to the CDs synthesized using the bottom-up approach (Supplementary Figures S1–S4). The defined peak of 280 nm could be attributed to π–π* transition of aromatic/alkenyl C=C bonds or C=N bonds (Tan et al., 2016).
Zeta Potential of Synthesized CDs
Zeta potential was measured using Malvern Zetasizer Nano ZS instrument (Supplementary Figures S1–S4) which was similar to earlier reports for these CDs.
Carbon Dots for Gene Delivery Into Bacterial Cells
DNA delivery of the CDs was measured by incubating these CDs (Citric Acid + PEI, 100 µg; L-arginine and glucose, 66 µg; NH2-FCDs, 75 µg and COOH-FCDs, 50 µg) with E. coli cells in the presence of 2 μg plasmid DNA. Of all the CDs used in this study, only COOH-FCDs were able to deliver plasmid DNA into the E. coli cells (Figure 1).
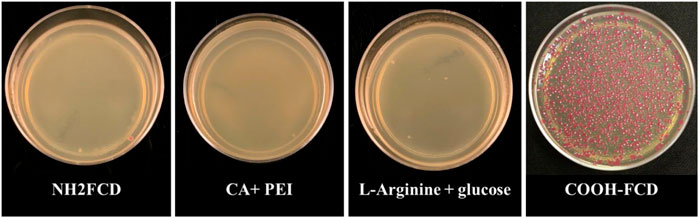
FIGURE 1. DNA delivery by different carbon dots (Citric Acid + PEI, 100 µg; L-arginine and glucose, 66 µg; NH2-FCDs, 75 µg and COOH-FCDs, 50 µg) into the E. coli cells. Only the COOH-FCDs were able to carry the plasmid into the E. coli cells. Optimum incubation time for DNA delivery by COOH-CDs.
To determine the optimum time for E. coli transformation, we incubated the mixture of COOH-CDs, plasmid, and E. coli cells for five different time periods including 5 min, 15 min, 30 min, 1 h, and 4 h. After the overnight culture, the number of colonies were counted using ImageJ software. As shown in Figure 2, the highest number of colonies was observed at 30 min of incubation throughout the replications; however, it was not significantly different from 60 min incubation (Figure 3). Shorter incubation times resulted in less colonies while no colonies were observed after 4 h incubation with COOH-CDs.
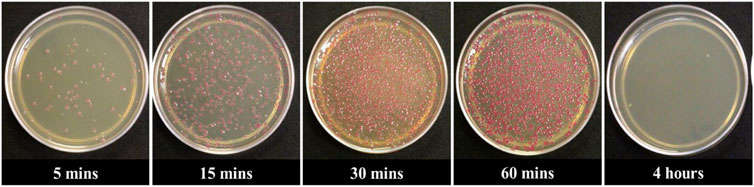
FIGURE 2. Gene delivery by COOH-FCDs at different time intervals using 50 µg of COOH-FCDs and plasmid (2 µg) in E. coli cells. 30 min incubation resulted in the highest number of colonies.
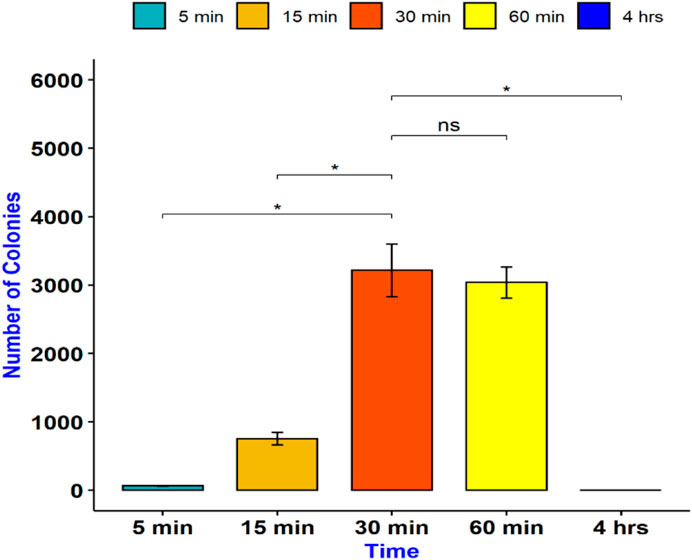
FIGURE 3. Gene delivery by COOH-FCDs at different time intervals. Maximum plasmid size that can be delivered into E. coli using COOH-CDs.
To determine the maximum plasmid size that can be delivered into E. coli cells, we incubated them with COOH-CDs and four plasmid DNA with sizes ranging from 3 to 13.5 kb. The plasmid sizes were as follows: pU6-pegRNA-GG-Acceptor (RFP): 3 kb, pPUS2: 7kb, pPSU1: 10 kb, Cas9-pMDC123: 13.5 kb. Bacterial colonies were counted using ImageJ software. Except for pU6-pegRNA-GG-Acceptor, which can be easily visualized with red color, colony PCR was performed for confirmation of positive colonies in other plasmids (Supplementary Figure S5).
Our results indicate that pU6-pegRNA-GG-Acceptor (RFP) and pPSU2 gave the highest number of colonies (Figures 4, 5). In contrast the number of obtained colonies dropped in pPSU1 with 10 kb plasmid size and no colonies were recovered from Cas9-pMDC123 plasmid that was 13.5 kb in size. This is an indication of plasmid size as a limiting factor in foreign DNA delivery into E. coli cells by COOH-CDs.
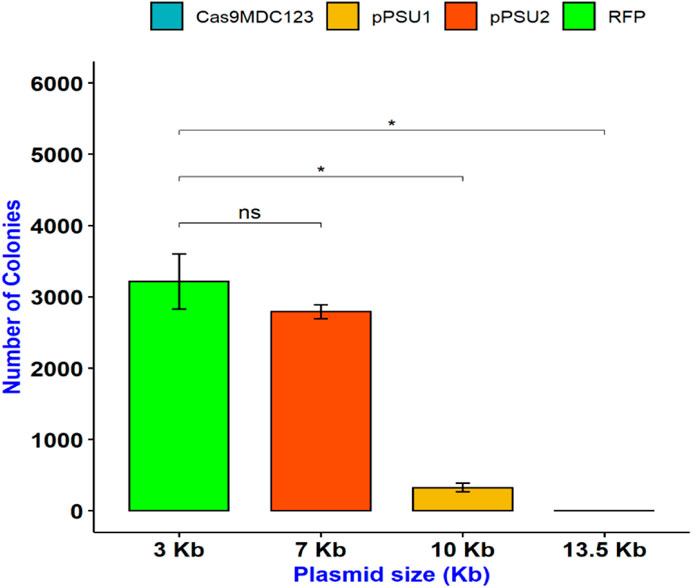
FIGURE 5. Delivery of plasmid DNAs with different sizes in E. coli cells using COOH-FCDs. Effect of temperature on DNA delivery by COOH-CDs in E. coli.
An experiment was conducted to study the effect of temperature and light conditions on DNA delivery using COOH-CDs by incubating the mixture of cells (E.coli, OD600 = 0.5), plasmid DNA (pU6-pegRNA-GG-Acceptor, 3 Kb), and COOH-CDs at three different temperatures (4°C, 25°C, and 37°C) under light and dark conditions. The mixture was incubated for 30 min under different temperature treatments and plated on LB + Amp100 plates and incubated overnight at 37°C. The number of colonies per plate was counted using ImageJ software and analyzed in R software. Highest number of transformed colonies were obtained when incubation was carried out at 4°C (Figures 6, 7) with no significant variation under light and dark conditions (Figures 6, 8).
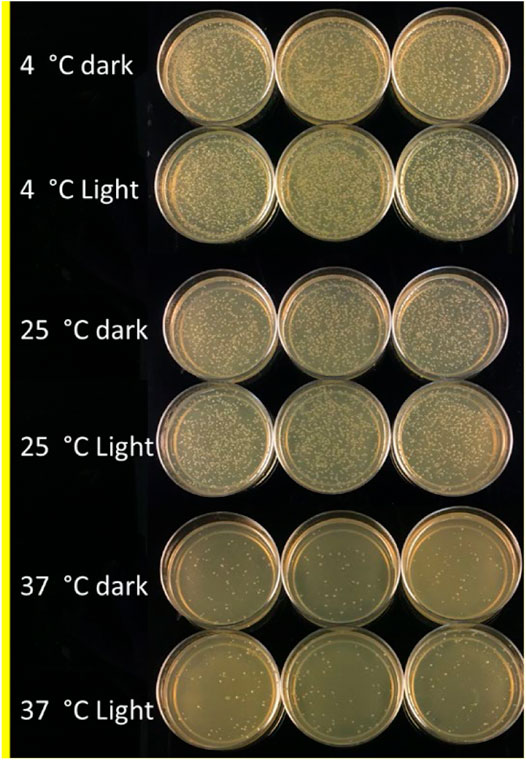
FIGURE 6. LB-plates with transformed colonies of E. coli after incubation at 4°C, 25°C, and 37°C in light and dark conditions using COOH-CDs.
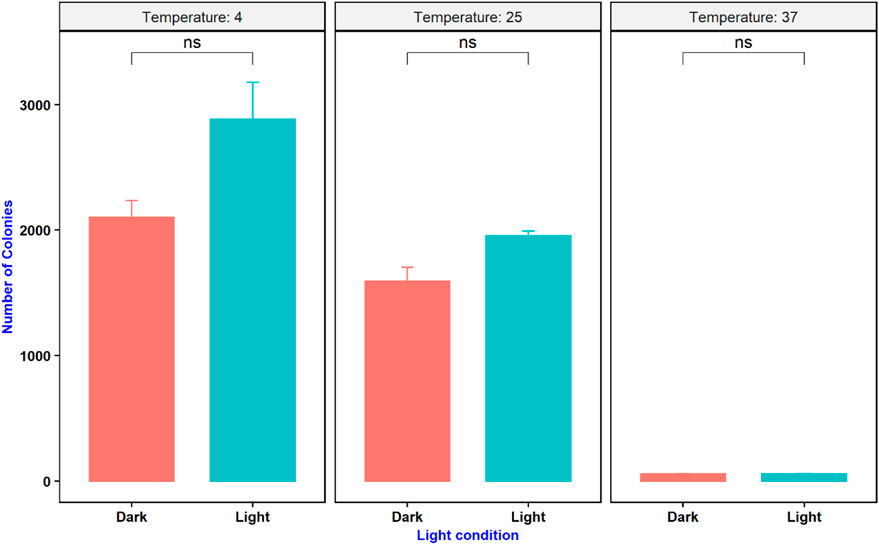
FIGURE 8. Effect of temperature and light condition on gene delivery ability of COOH-CDs on E. coli cells.
Discussion
In this research, we studied the gene delivery efficiency of four carbon dots in E. coli transformation. Successful transference of plasmid DNA into the microbial cells is fundamental in recombinant DNA technology. Natural transformation in bacteria is limited only to certain species due to many reasons including the repulsive forces between negatively charged DNA and bacterial membrane (Lederberg, 1994). Most common DNA delivery systems in bacteria include artificial transformation through heat shock or electroporation. These methods rely either on the time-consuming competent cell preparation protocols or on sophisticated instruments. Electroporation uses electric field pulses to cause disruption of cell membrane that leads to the formation of small pores that facilitate foreign DNA entry into the bacterial cells (Azencott et al., 2007). Although electroporation is easier, it is quite expensive and resource-intensive, which might be an economic burden to many researchers in developing countries. To find an alternative approach in DNA delivery that is less complicated and time consuming, we used carbon dots as a nonviral gene delivery approach in this study.
Carbon dots are excellent candidates for gene delivery due to their unique properties including ease of synthesis and functionalization, nano size particles, minimal toxicity, biocompatibility, and ability to penetrate the cells. In addition, their autofluorescence properties make it possible to track their interaction, penetrance, and accumulation inside the cells without staining or fluorescent tagging. We tested four different CDs for their plasmid delivery into E. coli cells. Among the CDs we tested, only carboxylate CDs (COOH-FCDs) synthesized from Glucosamine and β-alanine could deliver plasmid DNA in E. coli. The carboxylate carbon dot (CCOH-FCDs) is a multifunctional zwitterionic CD with an equal number of positive and negative charges on its surface. Even though the COOH-FCDs synthesized in this experiment possessed a net negative charge, the positive charges present on the COOH-FCDs interacted with the negatively charged plasmids and were preferentially internalized by the cells. From this observation, it is believed that preparation of CDs from zwitterions such as β-Alanine was advantageous due to the presence of both positive and negative charges which facilitates cytoplasmic uptake of the CDs loaded with plasmids (Jung et al., 2015). We also determined the optimum time required for transforming E. coli cells using COOH-FCDs. Highest number of transformed colonies were obtained at 30 min of incubation, while no colonies were obtained after 4 h of incubation indicating toxicity of these carbon dots on E. coli cells with longer incubation. In addition, we investigated the maximum plasmid size that the COOH-FCDs could deliver into the E. coli cells using four separate plasmids with different sizes ranging from 3 to 13.5 kb. COOH-FCDs were able to deliver plasmid sizes of less than 10 kb successfully into the E. coli cells; however, the highest number of positive colonies was obtained for plasmids that were smaller than 7 kb (pU6-pegRNA-GG-Acceptor and pPSU2). This finding indicates that plasmid size is the limiting factor in DNA delivery into the cells. COOH-FCD synthesized using this protocol have an average particle size of 3.85 nm (Hill et al., 2016) and fabrication of larger carbon dots through adjustment of temperature and precursor ratios (KhairolAnuar et al., 2021) or the use of single-walled carbon dots (Demirer et al., 2019b) could be used as alternatives for delivery of larger DNA plasmids into the cells. Our findings also indicate that the highest number of colonies can be obtained when the incubation of the plasmid DNA and CDs is carried out at 4 C as compared with 25 C and 37 C which could be due to increased concentration of ROS at higher temperatures (Li et al., 2018). Use of other techniques such as TEM and XPS spectra could reveal the interaction of plasmid DNA with these CDs and mechanism they use for the delivery of foreign DNA into the cells.
Conclusion
In this study, we synthesized four different carbon dots including amine-coated carbon dots (NH2-FCDs), carboxylate carbon dots (COOH-FCDs), carbon dots from L-arginine and glucose (N-CDs), and carbon dots from citric acid and PEI (Polyethyleneimine) and demonstrated that COOH-FCDs could deliver DNA into bacterial cells, which is affected by the incubation time, plasmid size, incubation temperature, and light condition. The use of CDs as a plasmid delivery tool into bacteria is a novel approach and is promising in terms of cost and sustainability. However, further studies and optimization of carbon dots are necessary for their widespread use in gene/plasmid delivery, especially in other bacterial species that are resistant to transformation with other common techniques. Understanding the chemical and physical properties of CDs and the quality of their interaction with living cells are essential to understand their antimicrobial properties as well as gene delivery capabilities.
Data Availability Statement
The original contributions presented in the study are included in the article/Supplementary Material, further inquiries can be directed to the corresponding author.
Author Contributions
AD, AP, and AT all conducted the nanomaterials investigation; AD prepared the draft version, AT, ZY and KD prepared the resources, supervised the project progress and edited the manuscript.
Funding
The authors wish to thank USDA for an Evans Allen funding (1005722), Tennessee Soybean Promotion Board (16-123-P) and USDA NIFA EGP (2020-70410-32909).
Conflict of Interest
The authors declare that the research was conducted in the absence of any commercial or financial relationships that could be construed as a potential conflict of interest.
Publisher’s Note
All claims expressed in this article are solely those of the authors and do not necessarily represent those of their affiliated organizations, or those of the publisher, the editors and the reviewers. Any product that may be evaluated in this article, or claim that may be made by its manufacturer, is not guaranteed or endorsed by the publisher.
Acknowledgments
We are also thankfull to Sudipta Rakshit and Ying Wu for giving us access to their labs, training and the use of FTIR, freeze dryer and Zetasizer in their labs.
Supplementary Material
The Supplementary Material for this article can be found online at: https://www.frontiersin.org/articles/10.3389/fnano.2021.777810/full#supplementary-material
References
Azencott, H. R., Peter, G. F., and Prausnitz, M. R. (2007). Influence of the Cell Wall on Intracellular Delivery to Algal Cells by Electroporation and Sonication. Ultrasound Med. Biol. 33 (11), 1805–1817. doi:10.1016/j.ultrasmedbio.2007.05.008
Baker, S. N., and Baker, G. A. (2010). Luminescent Carbon Nanodots: Emergent Nanolights. Angew. Chem. Int. Edition 49 (38), 6726–6744. doi:10.1002/anie.200906623
Cao, X., Wang, J., Deng, W., Chen, J., Wang, Y., Zhou, J., et al. (2018). Photoluminescent Cationic Carbon Dots as Efficient Non-viral Delivery of Plasmid SOX9 and Chondrogenesis of Fibroblasts. Sci. Rep. 8 (1), 7057–7111. doi:10.1038/s41598-018-25330-x
Clancy, A. J., Bayazit, M. K., Hodge, S. A., Skipper, N. T., Howard, C. A., and Shaffer, M. S. P. (2018). Charged Carbon Nanomaterials: Redox Chemistries of Fullerenes, Carbon Nanotubes, and Graphenes. Chem. Rev. 118 (16), 7363–7408. doi:10.1021/acs.chemrev.8b00128
Demirer, G. S., Zhang, H., Goh, N. S., González-Grandío, E., and Landry, M. P. (2019). Carbon Nanotube-Mediated DNA Delivery without Transgene Integration in Intact Plants. Nat. Protoc. 14 (10), 2954–2971. doi:10.1038/s41596-019-0208-9
Demirer, G. S., Zhang, H., Matos, J. L., Goh, N. S., Cunningham, F. J., Sung, Y., et al. (2019). High Aspect Ratio Nanomaterials Enable Delivery of Functional Genetic Material without DNA Integration in Mature Plants. Nat. Nanotechnol. 14 (5), 456–464. doi:10.1038/s41565-019-0382-5
Divya, P. G., Anish, R. V., Jagadeesh, G., and Chakravortty, D. (2011). Bacterial Transformation Using Micro-shock Waves. Anal. Biochem. 419 (2), 292–301. doi:10.1016/j.ab.2011.08.038
Fernando, K. A. S., Sahu, S., Liu, Y., Lewis, W. K., Guliants, E. A., Jafariyan, A., et al. (2015). Carbon Quantum Dots and Applications in Photocatalytic Energy Conversion. ACS Appl. Mater. Inter. 7 (16), 8363–8376. doi:10.1021/acsami.5b00448
Georgakilas, V., Perman, J. A., Tucek, J., and Zboril, R. (2015). Broad Family of Carbon Nanoallotropes: Classification, Chemistry, and Applications of Fullerenes, Carbon Dots, Nanotubes, Graphene, Nanodiamonds, and Combined Superstructures. Chem. Rev. 115 (11), 4744–4822. doi:10.1021/cr500304f
Hasanzadeh, A., Radmanesh, F., Kiani, J., Bayandori, M., Fatahi, Y., Aref, A. R., et al. (2019). Photoluminescent Functionalized Carbon Dots for CRISPR Delivery Synthesis, Optimization and Cellular Investigation. Mater. Res. Express, 30, 12. doi:10.1088/1361-6528/aafbf9
Hill, S. A., Benito-Alifonso, D., Morgan, D. J., Davis, S. A., Berry, M., and Galan, M. C. (2016). Three-minute Synthesis of Sp3nanocrystalline Carbon Dots as Non-toxic Fluorescent Platforms for Intracellular Delivery. Nanoscale 8 (44), 18630–18634. doi:10.1039/c6nr07336k
Huang, H., Hu, H., Qiao, S., Bai, L., Han, M., Liu, Y., et al. (2015). Carbon Quantum dot/CuSxnanocomposites towards Highly Efficient Lubrication and Metal Wear Repair. Nanoscale 7 (26), 11321–11327. doi:10.1039/c5nr01923k
Javed, N., and O’Carroll, D. M. (2021). Carbon Dots and Stability of Their Optical Properties. Part. Part. Syst. Charact. 2000271, 1–12. doi:10.1002/ppsc.202000271
Jung, Y. K., Shin, E., and Kim, B. S. (2015). Cell Nucleus-Targeting Zwitterionic Carbon Dots. Sci. Rep. 5 (1), 18807–18809. doi:10.1038/srep18807
KhairolAnuar, N. K., Tan, H. L., Lim, Y. P., So’aib, M. S., and Abu Bakar, N. F. (2021). A Review on Multifunctional Carbon-Dots Synthesized from Biomass Waste: Design/Fabrication, Characterization and Applications. Front. Energ. Res. 9, 67. doi:10.3389/fenrg.2021.626549
Kim, T. H., Sirdaarta, J. P., Zhang, Q., Eftekhari, E., St. John, J., Kennedy, D., et al. (2018). Selective Toxicity of Hydroxyl-Rich Carbon Nanodots for Cancer Research. Nano Res. 11 (4), 2204–2216. doi:10.1007/s12274-017-1838-2
Lederberg, J. (1994). The Transformation of Genetics by DNA: an Anniversary Celebration of Avery, MacLeod and McCarty (1944). Genetics 136 (2), 423–426. doi:10.1093/genetics/136.2.423
Li, H., Huang, J., Song, Y., Zhang, M., Wang, H., Lu, F., et al. (2018). Degradable Carbon Dots with Broad-Spectrum Antibacterial Activity. ACS Appl. Mater. Inter. 10 (32), 26936–26946. doi:10.1021/acsami.8b08832
Lim, S. Y., Shen, W., and Gao, Z. (2015). Carbon Quantum Dots and Their Applications. Chem. Soc. Rev. 44 (1), 362–381. doi:10.1039/c4cs00269e
Lin, H.-S., Jeon, I., Xiang, R., Seo, S., Lee, J.-W., Li, C., et al. (2018). Achieving High Efficiency in Solution-Processed Perovskite Solar Cells Using C60/C70 Mixed Fullerenes. ACS Appl. Mater. Inter. 10 (46), 39590–39598. doi:10.1021/acsami.8b11049
Liu, C., Zhang, P., Zhai, X., Tian, F., Li, W., Yang, J., et al. (2012). Nano-carrier for Gene Delivery and Bioimaging Based on Carbon Dots with PEI-Passivation Enhanced Fluorescence. Biomaterials 33 (13), 3604–3613. doi:10.1016/j.biomaterials.2012.01.052
Liu, X., Yang, C., Zheng, B., Dai, J., Yan, L., Zhuang, Z., et al. (2018). Green Anhydrous Synthesis of Hydrophilic Carbon Dots on Large-Scale and Their Application for Broad Fluorescent pH Sensing. Sensors Actuators B: Chem. 255, 572–579. doi:10.1016/j.snb.2017.08.101
Lu, W., Gong, X., Nan, M., Liu, Y., Shuang, S., and Dong, C. (2015). Comparative Study for N and S Doped Carbon Dots: Synthesis, Characterization and Applications for Fe3+ Probe and Cellular Imaging. Analytica Chim. Acta 898, 116–127. doi:10.1016/j.aca.2015.09.050
Namdari, P., Negahdari, B., and Eatemadi, A. (2017). Synthesis, Properties and Biomedical Applications of Carbon-Based Quantum Dots: An Updated Review. Biomed. Pharmacother. 87 (88), 209–222. doi:10.1016/j.biopha.2016.12.108
Nandiyanto, A. B. D., Oktiani, R., and Ragadhita, R. (2019). How to Read and Interpret Ftir Spectroscope of Organic Material. Indonesian J. Sci. Technol. 4 (1), 97–118. doi:10.17509/ijost.v4i1.15806
Peng, Z., Han, X., Li, S., Al-Youbi, A. O., Bashammakh, A. S., El-Shahawi, M. S., et al. (2017). Carbon Dots: Biomacromolecule Interaction, Bioimaging and Nanomedicine. Coord. Chem. Rev. 343, 256–277. doi:10.1016/j.ccr.2017.06.001
Ponomarenko, L. A., Schedin, F., Katsnelson, M. I., Yang, R., Hill, E. W., Novoselov, K. S., et al. (2008). Chaotic Dirac Billiard in Graphene Quantum Dots. Science 320 (5874), 356–358. doi:10.1126/science.1154663
Rai, S., Singh, B. K., Bhartiya, P., Singh, A., Kumar, H., Dutta, P. K., et al. (2017). Lignin Derived Reduced Fluorescence Carbon Dots with Theranostic Approaches: Nano-Drug-Carrier and Bioimaging. J. Lumin. 190 (June), 492–503. doi:10.1016/j.jlumin.2017.06.008
Rao, R., Pint, C. L., Islam, A. E., Weatherup, R. S., Hofmann, S., Meshot, E. R., et al. (2018). Carbon Nanotubes and Related Nanomaterials: Critical Advances and Challenges for Synthesis toward Mainstream Commercial Applications. ACS Nano 12 (12), 11756–11784. doi:10.1021/acsnano.8b06511
Sagbas, S., and Sahiner, N. (2018). Carbon Dots: Preparation, Properties, and Application. Amsterdam, Elsevier.
Schwartz, S. H., Hendrix, B., Hoffer, P., Sanders, R. A., and Zheng, W. (2019). Carbon Dots for Efficient siRNA Delivery and Gene Silencing in Plants. Plant Physiology 184(2):647-657. doi:10.1104/pp.20.00733
Schwartz, S. H., Hendrix, B., Hoffer, P., Sanders, R. A., and Zheng, W. (2020). Carbon Dots for Efficient Small Interfering RNA Delivery and Gene Silencing in Plants. Plant Physiol. 184 (2), 647–657. doi:10.1104/pp.20.00733
Sharma, A., and Das, J. (2019). Small Molecules Derived Carbon Dots: Synthesis and Applications in Sensing, Catalysis, Imaging, and Biomedicine. J. Nanobiotechnology 17(1), 92. doi:10.1186/s12951-019-0525-8
Shen, L.-M., and Liu, J. (2016). New Development in Carbon Quantum Dots Technical Applications. Talanta 156-157, 245–256. doi:10.1016/j.talanta.2016.05.028
Sun, X., and Lei, Y. (2017). Fluorescent Carbon Dots and Their Sensing Applications. Trac Trends Anal. Chem. 89, 163–180. doi:10.1016/j.trac.2017.02.001
Sun, Y.-P., Zhou, B., Lin, Y., Wang, W., Fernando, K. A. S., Pathak, P., et al. (2006). Quantum-sized Carbon Dots for Bright and Colorful Photoluminescence. J. Am. Chem. Soc. 128 (24), 7756–7757. doi:10.1021/ja062677d
Tan, J., Zou, R., Zhang, J., Li, W., Zhang, L., and Yue, D. (2016). Large-scale Synthesis of N-Doped Carbon Quantum Dots and Their Phosphorescence Properties in a Polyurethane Matrix. Nanoscale 8 (8), 4742–4747. doi:10.1039/c5nr08516k
Wang, Q., Zhang, C., Shen, G., Liu, H., Fu, H., and Cui, D. (2014). Fluorescent Carbon Dots as an Efficient siRNA Nanocarrier for its Interference Therapy in Gastric Cancer Cells. J. Nanobiotechnology 12(1). doi:10.1186/s12951-014-0058-0
Wang, X., Feng, Y., Dong, P., and Huang, J. (2019). A Mini Review on Carbon Quantum Dots: Preparation, Properties, and Electrocatalytic Application. Front. Chem. 7 (October), 671–679. doi:10.3389/fchem.2019.00671
Wu, X., Wu, L., Cao, X., Li, Y., Liu, A., and Liu, S. (2018). Nitrogen-doped Carbon Quantum Dots for Fluorescence Detection of Cu2+ and Electrochemical Monitoring of Bisphenol A. RSC Adv. 8 (36), 20000–20006. doi:10.1039/c8ra03180k
Xu, X., Ray, R., Gu, Y., Ploehn, H. J., Gearheart, L., Raker, K., et al. (2004). Electrophoretic Analysis and Purification of Fluorescent Single-Walled Carbon Nanotube Fragments. J. Am. Chem. Soc. 126 (40), 12736–12737. doi:10.1021/ja040082h
Yao, B., Huang, H., Liu, Y., and Kang, Z. (2019). Carbon Dots: A Small Conundrum. Trends Chem. 1 (2), 235–246. doi:10.1016/j.trechm.2019.02.003
Yuan, F., Li, S., Fan, Z., Meng, X., Fan, L., and Yang, S. (2016). Shining Carbon Dots: Synthesis and Biomedical and Optoelectronic Applications. Nano Today 11 (5), 565–586. doi:10.1016/j.nantod.2016.08.006
Zhang, H., Zhang, H., Demirer, G. S., González-Grandío, E., Fan, C., and Landry, M. P. (2020). Engineering DNA Nanostructures for siRNA Delivery in Plants. Nat. Protoc. 15 (9), 3064–3087. doi:10.1038/s41596-020-0370-0
Keywords: gene delivery, carbon dots, bacteria, transformation, E. coli
Citation: Devkota A, Pandey A, Yadegari Z, Dumenyo K and Taheri A (2021) Glucosamine/β-Alanine Carbon Dots Use as DNA Carriers Into E. coli Cells. Front. Nanotechnol. 3:777810. doi: 10.3389/fnano.2021.777810
Received: 15 September 2021; Accepted: 18 October 2021;
Published: 16 November 2021.
Edited by:
Vinay Sharma, Indian Institute of Technology Jammu, IndiaReviewed by:
Pranav Tiwari, University of Notre Dame, United StatesMoon Il Kim, Gachon University, South Korea
Copyright © 2021 Devkota, Pandey, Yadegari, Dumenyo and Taheri. This is an open-access article distributed under the terms of the Creative Commons Attribution License (CC BY). The use, distribution or reproduction in other forums is permitted, provided the original author(s) and the copyright owner(s) are credited and that the original publication in this journal is cited, in accordance with accepted academic practice. No use, distribution or reproduction is permitted which does not comply with these terms.
*Correspondence: Ali Taheri, YXRhaGVyaTFAdG5zdGF0ZS5lZHU=