- 1School of Physical Education and Sports Science, South China Normal University, Guangzhou, China
- 2College of Physical Education and Sports, Beijing Normal University, Beijing, China
Objective: This study investigates the causal relationship between moderate to vigorous physical activity and cognitive performance.
Methods: Genetic loci strongly related to moderate to vigorous physical activity from genome-wide association studies were used as instrumental variables. These were combined with genetic data on cognitive performance from different Genome-Wide Association Study (GWAS) to conduct a two-sample Mendelian randomization analysis. The primary analysis used inverse variance weighting within a random effects model, supplemented by weighted median estimation, MR-Egger regression and other methods, with results expressed as Beta coefficient.
Results: This study selected 19 SNPs closely related to physical activity as instrumental variables. The multiplicative random-effects Inverse-Variance Weighted (IVW) analysis revealed that moderate to vigorous physical activity was negatively associated with cognitive performance (Beta = −0.551; OR = 0.58; 95% CI: 0.46–0.72; p < 0.001). Consistent results were obtained using the fixed effects IVW model (Beta = −0.551; OR = 0.58; 95% CI: 0.52–0.63; p < 0.001), weighted median (Beta = −0.424; OR = 0.65; 95% CI: 0.55–0.78; p < 0.001), simple mode (Beta = −0.467; OR = 0.63; 95% CI: 0.44–0.90; p < 0.001), and weighted mode (Beta = −0.504; OR = 0.60; 95% CI: 0.44–0.83; p < 0.001). After adjusting for BMI, smoking, sleep duration, and alcohol intake frequency, the multivariate MR analysis also showed a significant association between genetically predicted MVPA and cognitive performance, with Beta of −0.599 and OR = 0.55 (95% CI: 0.44–0.69; p < 0.001).
Conclusion: The findings of this study indicate that genetically predicted moderate to vigorous physical activity may be associated with a decline in cognitive performance.
Introduction
A growing body of research suggests that physical activity has a significant impact on cognitive performance. However, establishing a causal relationship between physical activity and cognitive performance remains challenging due to confounding factors such as socioeconomic status, education, and access to healthcare. Cognitive performance refers to the brain’s ability to process and interpret information, encompassing essential functions such as reasoning, attention, thinking, reading, and learning (Gale et al., 2010). This critical aspect of brain function is linked to various health outcomes, including morbidity, mortality, mental disorders, coronary heart disease (CHD), and certain cancers (Gale et al., 2010; Hart et al., 2003; Jaycox et al., 2009; Lawlor et al., 2008a). In recent years, the prevalence of risk factors such as aging, obesity, and unhealthy lifestyles (e.g., smoking, excessive alcohol consumption, irregular sleep patterns, etc.) has been associated with a decline in cognitive performance (Albert et al., 2011; Colcombe and Kramer, 2003; Salthouse, 1996; Elias et al., 2003; Gunstad et al., 2007; Smith et al., 2011; Eckardt et al., 1998; Strine and Chapman, 2005). It is believed that individuals who engage in frequent physical activity in their early years are more likely to maintain a more active lifestyle in the middle and even old age. Additionally, research indicates that physical exercise and activity during early life can lead to beneficial changes in the brain, reducing the likelihood of cognitive deficits later in life (Sabia et al., 2017; Scarmeas and Stern, 2004). Therefore, identifying interventions or factors that can mitigate cognitive decline early is crucial for reducing the risk of cognitive-related diseases.
Physical activities encompass a range of daily activities such as walking, work exertion (da Silva et al., 2018), recreational activities, household chores, and more intense physical activities like running, dancing, and competitive sports. Work-related physical activity contributes most of overall physical activity, particularly for lower-income groups with household incomes below the median of $36,000 (Matsushita et al., 2015; Suminski et al., 2011). Numerous studies have consistently demonstrated the positive effects of moderate physical activity on cognitive performance (Cotman and Berchtold, 2002; Ngandu et al., 2015; Chen et al., 2016; Weaver and Jaeggi, 2021; Wollseiffen et al., 2016). For instance, a large cohort study from Brazil found that adolescents engaging in moderate to vigorous physical activity (MVPA) tend to exhibit higher cognitive performance (Esteban-Cornejo et al., 2015). Additionally, research indicates that older adults with higher levels of physical activity experience a slower decline in cognitive performance (Albert et al., 2011; Gauthier et al., 2011). However, a systematic review of studies found that the effects of physical activity on cognitive performance are inconsistent, with limited conclusive evidence supporting a positive impact of physical activity on cognition. It is inaccurate to generalize that physical activity universally improves cognitive performance, as the type, amount, frequency, and duration of physical activity can have varying effects (Barbosa et al., 2020; Donnelly et al., 2016; Latino and Tafuri, 2023). Some studies suggest that high-intensity physical activity may lead to a decline in cognitive performance (Samuel et al., 2017). Even the most rigorous studies on brain cognitive health, such as those employing population-based cohorts with longitudinal follow-up or natural experiments, may exhibit biases. These biases can arise from attrition (e.g., higher dropout rates among individuals with the outcome of interest), self-selection (e.g., lifestyle and exercise behavior changes following cognitive impairment in older adults), and residual confounding (Besser et al., 2021). In summary, the evidence from the aforementioned studies primarily relies on observational correlations, leaving the causal relationship between physical activity and cognitive performance uncertain.
While randomized controlled trials (RCTs) are the gold standard for establishing causal relationships, ethical and practical challenges often limit their feasibility in studying the effects of exercise on cognitive performance. Despite the established correlation between physical activity and cognitive performance, causality remains difficult to ascertain due to potential confounding factors. To overcome these limitations, this study employs Mendelian Randomization (MR) to investigate the causal relationship between physical activity and cognitive performance. MR leverages the principle of random gene allocation, as outlined by Mendel’s second law, to assess causal relationships between exposures and outcomes (Smith and Ebrahim, 2003). By using genetic variants associated with specific exposures as instrumental variables, MR minimizes confounding and reverse causality biases that often challenge traditional observational studies (Richmond and Smith, 2022).
In this study, a two-sample MR approach was utilized, drawing on data from Genome-Wide Association Studies (GWAS) to assess the independent role of moderate-to-vigorous physical activity (MVPA) in cognitive performance (Hu et al., 2023). Additionally, a multivariable Mendelian Randomization (MVMR) analysis was performed to evaluate the causal effect of MVPA on cognitive performance while adjusting for other risk factors, including BMI, smoking, alcohol consumption, and sleep patterns.
Methods
Study design
The study comprised five components: (1) Identifying genetic variants as instrumental SNPs for exposure data through screening; (2) Collecting instrumental SNPs for outcome data from a genome-wide association study database focused on cognitive performance; (3) Integrating instrumental SNPs from the exposure and outcome datasets; (4) Conducting two-sample Mendelian randomization analyses and multivariate Mendelian randomization analyses; and (5) Conducting sensitivity analyses to evaluate the robustness of the Mendelian randomization analysis. To ensure valid causal estimates from the Mendelian analyses, the selected single nucleotide polymorphisms (SNPs) as genetic instruments for physical activity must satisfy three assumptions: (A) strong association with physical activity, (B) no association with confounding factors of the exposure-outcome relationship, and (C) exclusive influence on cognitive performance risk through the exposure of physical activity (see Figure 1).
Data sources for physical activity and cognitive performance
Genetic instruments for MVPA were obtained from a GWAS conducted by Klimentidis et al. (2018) on a sample of 377,234 individuals in Europe descent, comprising both males and females. Physical activity levels were assessed using self-reported data collected via a touchscreen questionnaire. Participants were asked: “In a typical week, how many days did you do 10 min or more of moderate activities like carrying light loads or cycling at a normal pace (excluding walking)?” For vigorous activities, they were asked: “In a typical week, how many days did you do 10 min or more of vigorous activities like fast cycling, aerobics, or heavy lifting?” Those who reported engaging in such activities on at least 1 day were then asked how many minutes they usually spent on these daily activities, including work, leisure, travel, and housework (Klimentidis et al., 2018). MVPA was quantified by multiplying the total number of minutes of moderate physical activity per week by four and the total number of minutes of vigorous physical activity per week by eight, corresponding to their metabolic equivalents, as previously described (Bassett, 2003; Ekelund et al., 2006). Data are available for download on the ieu website at https://gwas.mrcieu.ac.uk/datasets/ebi-a-GCST006097/.
Summary-level genetic data for cognitive performance was collected by the Cognitive Genomics Consortium (COGENT) (Lee et al., 2018). The COGENT consortium performed a GWAS for cognitive performance in 257,841 individuals, and these results were meta-analyzed with published results from the UK Biobank (Lee et al., 2018). The participants included in the study were individuals of European descent, ranging in age from 16 to 102 years. Cognitive performance was measured using the first unrotated component derived from at least three neuropsychological tests. These tests include digit span for working memory, logical memory for verbal declarative memory, and digit symbol coding for processing speed. Population-specific genotypic principal components were included as covariates (Trampush et al., 2017). Fluid intelligence was assessed using the verbal numerical reasoning test in the UK Biobank, which evaluates participants’ ability in numerical reasoning and verbal comprehension. Estimates are reported in standard deviation (SD) units per SNP.
Additionally, the UK Biobank provides data on three other cognitive tests: reaction time, pairs matching, and prospective memory (Lee et al., 2018). The cohorts participating in the GWAS mentioned above obtained ethics approval from the respective ethical review boards and informed written consent from all participants (Zonneveld et al., 2023). Data on Cognitive Performance can be downloaded from https://gwas.mrcieu.ac.uk/datasets/ebi-a-GCST006572/.
SNP selection
This study defined a genome-wide significance level of P < 5 × 10−8.To filter out weak instrumental variables, the potency of each SNP as an instrumental variable was assessed using the formula provided in a previous study to calculate the F statistic (Papadimitriou et al., 2020). An F > 10 was required for effective selection. SNPs with significant linkage disequilibrium needed to be removed to satisfy the independence assumption. An r2 value of 1 indicates complete linkage disequilibrium between the two SNPs, while an r2 value of 0 indicates complete linkage balance. In this study, the parameter r2 was set to 0.001, and kb was set to 10,000. This represents the removal of SNPs with an r2 value greater than 0.001 within a range of 10,000 kb. Consequently, instrumental variables with no linkage effect were screened from the different MVPA data.
Statistical analysis
The principal analysis was conducted with an inverse-variance weighted (IVW) multiplicative random-effects model. A series of sensitivity analyses were performed to calculate the error variance using a fixed IVW, an MR-Egger regression, a weighted median, a simple mode, and a weighted mode to account for potential invalid instrument bias or pleiotropy (Hu et al., 2023). Invalid instrument bias was assessed using the weighted median model, which provided consistent results even when up to 50% of the weight was derived from invalid SNPs (Burgess et al., 2017). MR-Egger regression analysis was used to detect and correct for directional pleiotropy, and the intercept from MR-Egger was assessed to determine whether horizontal pleiotropy existed (Bowden et al., 2015). Funnel plots were also used to identify potential horizontal pleiotropy by assessing asymmetry. A multivariable MR analysis was conducted to adjust for BMI and smoking as adjustment factors. Heterogeneity among the included SNPs was assessed using Cochrane’s Q value. SNPs individually were examined for their impact on the overall causal estimate by performing a leave-one-out sensitivity analysis. The “TwoSampleMR” and “MVMR” packages in R version 4.2.3 were used for all analyses.
Results
After confirming a strong correlation and resolving any potential chain imbalance, 1.5 million items associated with MVPA were extracted for further analysis. Table 1 presents the basic characteristics of the 19 SNPs linked to physical activity. In this table, “Chr” denotes chromosomal information, “NEA/EA” represents the non-effect and effect alleles, respectively, and “Beta” indicates the effect size of the MVPA-associated SNPs. The F statistics corresponding to individual SNPs in this study range from 30 to 52, with all F > 10. This suggests that the instrumental variables used are robust, thereby reducing the likelihood of weak instrument bias and reinforcing the reliability of the study’s findings.
As shown in Figure 2, genetically predicted MVPA had a significant effect on cognitive performance under the multiplicative random-effects inverse-variance weighted (IVW) model, with a Beta coefficient of −0.551 and odds ratio (OR) of 0.58 (95% confidence interval [CI]: 0.46–0.72; p = 0.000). Consistent results were obtained using the fixed effects IVW model (OR = 0.58; 95% CI: 0.52–0.63; p < 0.001), weighted median (Beta = −0.424; OR = 0.65; 95% CI: 0.55–0.78; p < 0.001), simple mode (Beta = −0.467; OR = 0.63; 95% CI: 0.44–0.90; p < 0.001), and weighted mode (Beta = −0.0.504; OR = 0.60; 95% CI: 0.44–0.83; p < 0.001). After adjusting for BMI, smoking, sleep duration, and alcohol intake frequency, the multivariate MR analysis also showed a significant association between genetically predicted MVPA and cognitive performance, with a Beta coefficient of −0.599 and OR = 0.55 (95% CI: 0.44–0.69; p < 0.001). However, only the MR-Egger model results indicated that MVPA did not significantly affect cognitive performance, with a Beta coefficient of −0.0.463 and OR of 0.63 (95% CI: 0.25–1.56; p = 0.330).
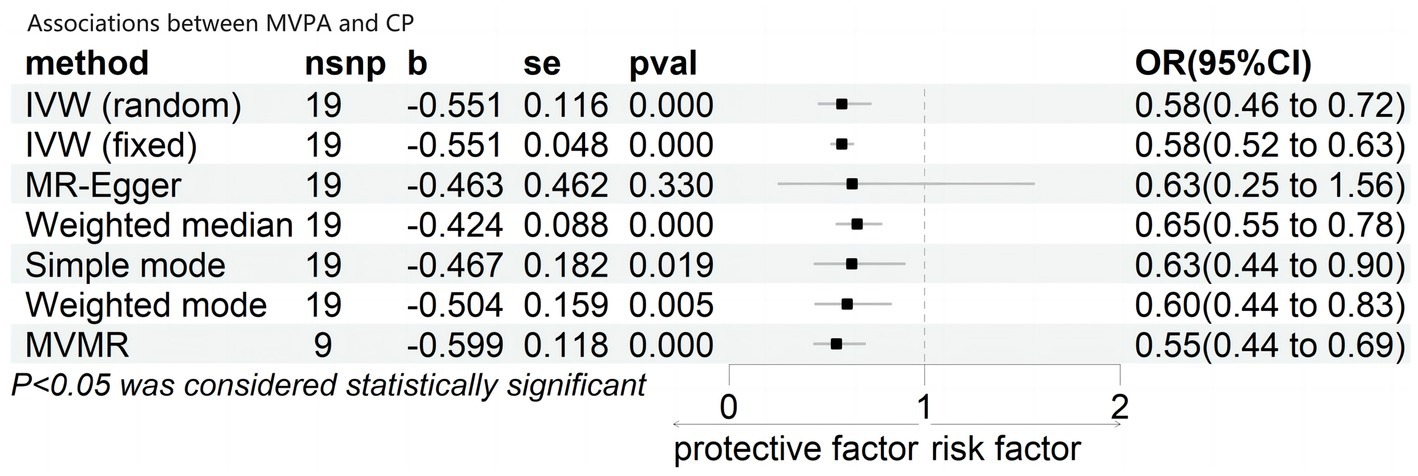
Figure 2. Associations between MVPA and cognitive performance. CI, confidence interval; OR, odd ratio; b, Beta; nsnp, number of SNP nsnp number.
In addition, the sensitivity analysis results indicated the absence of pleiotropy in this study (p = 0.85). The MR-PRESSO test (p = 0.193) also yielded consistent results, as visualized in Figure 3D, where the funnel plot for the IVW method exhibited symmetry, indicating no presence of horizontal pleiotropy. Furthermore, scatter plots and forest plots based on all single nucleotide polymorphisms (SNPs) are presented in Figures 3A,B, respectively. In contrast, Figure 3C displays the sensitivity analysis results for the leave-one-out method.
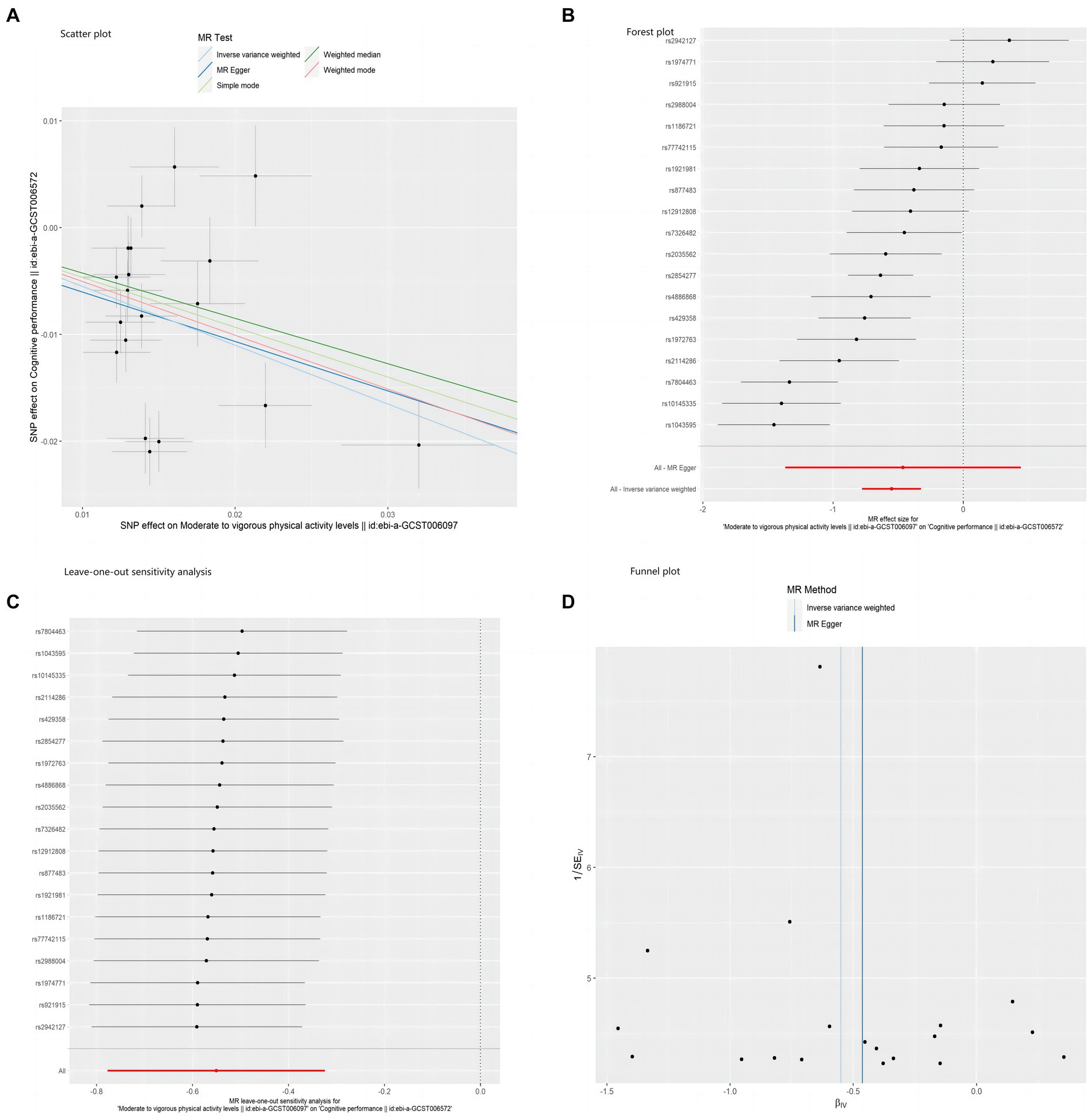
Figure 3. Scatter plot (A), forest plot (B), leave-one-out sensitivity analysis (C), and funnel plot (D) of the association of MVPA with cognitive performance.
Discussion
This study employed a two-sample MR analysis using genetic instruments derived from large-scale genome-wide association studies data to strengthen the causal inference. MR leverages gene variation associated with a target exposure to estimate causality. By relying on the random assignment of genetic variation from parents and offspring, MR helps overcome the limitations of observational studies, thereby reducing the potential for confounding (Lawlor et al., 2008b). Since genetic variation is determined at conception, the method minimizes the risk of reverse causation. If MVPA has a causal effect on cognitive performance, genetic variants associated with physical activity should also be linked to cognitive performance. The study investigates the relationship between MVPA and cognitive performance. The approach utilizes genetic information to evaluate the causal relationship between MVPA and cognitive performance, mitigating confounding factors. The findings suggest that MVPA may have a detrimental effect on cognitive performance. Despite being widely recognized for its benefits in improving cognitive performance and preventing cognitive impairment, this study indicates that there might not be a direct causal relationship between physical activity and cognitive performance enhancement. Therefore, recommendations to promote physical activity may have an uncertain impact on improving cognitive performance improvement.
The mechanisms by which physical activity enhances cognitive performance can be explained on macro and cellular levels. On a macro level, individuals who engage in regular physical activity are often involved in cognitively stimulating activities such as gardening, hiking, or outdoor sports. These activities frequently include cognitive components, such as reading about outdoor sports, and increase social interaction, further stimulating cognitive processes and enhancing cognitive performance (Robitaille et al., 2014; Berchtold et al., 2005; Hall et al., 2000). Cross-sectional studies have consistently shown a positive correlation between physical activity and cognitive performance (Reas et al., 2019). Aerobic exercise and strength training have improved executive function, memory, and verbal fluency. Longitudinal studies also suggest that more excellent physical activity is associated with a slower decline in overall cognitive performance, memory, attention, and processing speed (Wang et al., 2023). On a cellular and physiological level, physical activity may exert its effects through several mechanisms. It stimulates the production of brain-derived neurotrophic factor (BDNF), crucial for cognitive performance and brain development (Khan and Hillman, 2014). BDNF supports neuronal cell survival, facilitates synaptic plasticity, and promotes neurogenesis and neuronal differentiation, all contributing to improved cognitive performance (Griffin et al., 2011). Physical activity leads to a rapid and sustained increase in mature BDNF protein and signaling in the brain (Hall et al., 2000; Berchtold et al., 2005), which in turn raises serum concentrations of these neurotrophins and enhances cognitive performance through regulatory mechanisms (Tyrrell and Pereira, 1980). In a study examining the effects of different interventions on BDNF levels in healthy older adults, 19 participants engaged in 35 min of physical exercise, cognitive training, and mindfulness exercises. The study compared the changes in serum BDNF levels among the three intervention groups. The results revealed a significantly higher increase in serum BDNF levels in the physical exercise group compared to the cognitive training and positive thinking group (Hakansson et al., 2017).
Research consistently highlights the positive impact of physical activity on the hippocampus, a brain region crucial for declarative memory consolidation, spatial orientation, and emotion regulation. Biologically, there is a positive correlation between hippocampal size and cognitive abilities, with a larger hippocampal volume generally being advantageous (Anblagan et al., 2018; Konishi et al., 2017; Reuben et al., 2011). Studies have shown that physical activity can increase hippocampal size in adults, thereby enhancing cognitive performance (Liu and Nusslock, 2018). The hippocampus, located deep within the brain’s medial temporal lobe, plays a critical role in cognitive performance (El-Falougy and Benuska, 2006; Geinisman et al., 1995). Impairment in the structure and function of the hippocampus can contribute to cognitive performance declines and cognitive deficit development (Geinisman et al., 1995). In a randomized controlled trial involving 120 older adults, aerobic exercise training increased the volume of the anterior hippocampus, leading to improvements in cognitive performance. The study observed a 2% increase in hippocampal volume due to exercise training, counteracting age-related volume loss and its associated adverse effects (Erickson et al., 2011). A study focusing on physically inactive older adults found that a physical activity intervention, including increased daily walking, increased hippocampal volume (Varma et al., 2015). Regarding the relationship between the BDNF gene and the hippocampus, the BDNF gene contains two main alleles that produce varying amounts of protein products. These products regulate neurons in the brain, including those in the hippocampus, influencing hippocampal volume and cognitive behavioral traits (Frodl et al., 2014; Marrocco et al., 2020; Palasz et al., 2020; Vilor-Tejedor et al., 2020).
However, there is ongoing debate regarding the effects of physical activity on cognitive performance. Research has shown that the relationship between the intensity, type, and amount of exercise and its impact on BDNF levels or cognitive performance is not always straightforward. While several physiological mechanisms suggest that physical activity may enhance cognition, others propose that it could negatively affect cognitive performance. Given that these mechanisms are complex and sometimes conflicting, current scientific understanding and technology have yet to describe the relationship between physical activity and cognition precisely.
Certain studies have indicated that intense and strenuous exercise can disrupt the body’s metabolism and physiological processes, negatively impacting cognitive performance (Aguiló et al., 2005). During physical activity, the rapid metabolism of oxygen produces reactive oxygen species (ROS) as a metabolic by-product. High levels of ROS accumulated during continuous intense exercise can lead to oxidative damage and increase neuronal mortality (Radak et al., 2016). While moderate physical activity enhances the body’s antioxidant defense system, excessive ROS generated from intense exercise may detrimentally affect cognitive performance if accumulated in excess (Mastaloudis et al., 2001). A cohort study on adolescents found that those engaged in high-intensity physical activity had lower cognitive scores, suggesting a negative impact of such exercise on cognitive performance during adolescence (Esteban-Cornejo et al., 2015). Additionally, a population-based study reported that extending exercise beyond 1 h could decrease cognitive performance. This effect might be linked to dehydration or hypoglycemia during acute exercise, as increased sweating and elevated body temperature can lead to water and electrolyte loss, impairing cognitive performance such as decision-making and perceptual tasks (Brisswalter et al., 2002). In a study involving older adults, 105 participants were randomly assigned to high-intensity interval training (n = 33), moderate-intensity continuous training (n = 24), or a control group (n = 48). The findings suggested that high-intensity interval exercise might negatively affect the hippocampus and overall cognitive performance (Pani et al., 2021). Furthermore, a large, nationally representative six-year longitudinal study on school sports participation revealed that increased participation had a negative effect on students’ performance in standardized tests (Marsh and Kleitman, 2003). Another study assessing an intensive physical education program found that intense intermittent aerobic exercise negatively impacted children’s numerical speed and accuracy in cognitive tasks such as simple addition problems (Travlos, 2010).
Given the inconsistent evidence regarding the causal relationship between physical activity and cognitive performance in previous studies, the present study conducted an MR analysis to explore this relationship at the genetic level. Additionally, external factors such as age, environment, lifestyle, diet, and comorbidities interact with the genome through epigenetic modifications. These modifications regulate gene expression across various tissues without altering the underlying DNA sequence (Barati et al., 2022). Research has shown that changes in the epigenome can contribute to cognitive disorders (Strafella et al., 2018). Thus, epigenetic changes might influence the relationship between physical activity-related SNPs and cognitive performance. To account for potential epigenetic influences, relevant risk factors such as sleep duration, alcohol consumption, BMI, and smoking were identified through a literature review and adjusted for in a multivariate MR analysis. The findings of this study indicate that genetically predicted MVPA may be associated with a decline in cognitive performance.
However, several potential positive biological adaptation pathways suggest that physical activity may help maintain cognitive performance throughout individual development. Aerobic exercise can modulate neurotransmission, promote angiogenesis, enhance neurotrophic factors and synaptic plasticity, and facilitate neurogenesis, thereby improving cerebrovascular function (Blomstrand et al., 1989; Isaacs et al., 1992; Farmer et al., 2004). The health benefits of physical activity are well-documented, including improved physical fitness, reduced disease risk, and enhanced quality of life. In both adults and adolescents, increased physical activity is strongly associated with positive subjective health outcomes (Mountjoy et al., 2011). Self-reported health status, which encompasses illness experience, physical ability, health behaviors, living situation, and self-esteem, has been shown to predict mortality (Idler and Benyamini, 1997). Regular physical activity can lower mortality risk in coronary heart disease patients and improve their functional and emotional wellbeing (Rodriguez et al., 1994; Haapanen et al., 1997; Lee et al., 2000). Studies on individuals with diabetes have shown moderate-intensity physical activity enhances metabolism (Lee et al., 2015), while high-intensity physical activity is particularly effective for cardiovascular health and blood sugar control (Jelleyman et al., 2015).
Furthermore, increasing physical activity is an effective strategy for improving the physical and mental health of the elderly (Scarmeas et al., 2001; Gilovich et al., 2002; Feldman et al., 2015). Physical activity benefits the elderly by enhancing physical and mental health, social adaptation, activity levels, and nutritional status. It can extend healthy life expectancy, improve quality of life, and boost overall wellbeing (Cui et al., 2021). For example, a meta-analysis of 36 studies found that elderly individuals participating in physical activity programs reported significantly higher levels of mental health, including emotional wellbeing, self-perception, and life satisfaction, compared to non-participants (Netz et al., 2005). Given the overall health benefits of physical activity, promoting it in the general population remains justified despite concerns about reduced cognitive performance. However, caution is advised with prolonged MVPA to mitigate potential negative impacts on cognitive performance. Future research should explore the effects of different types and intensities of exercise at the genetic level to identify the most beneficial forms for cognitive performance and overall health while minimizing the risk of cognitive decline.
It is important to acknowledge certain limitations of this study. Firstly, the research focused exclusively on the unidirectional impact of physical activity on cognitive performance. However, literature suggests a potentially more complex bidirectional relationship between physical activity and cognitive performance. For instance, some studies have found that a decline in cognitive resources is associated with lower levels of moderate physical activity (Cheval et al., 2020) and that a bidirectional relationship exists between physical activity and executive function in older adults (Daly et al., 2015). Moreover, cognitive resources have been shown to moderate the negative impact of perceived poor neighborhood conditions on older adults’ self-reported physical activity (Cheval et al., 2019). Future research should employ bidirectional Mendelian randomization to address these complexities to investigate the reciprocal causal relationship between physical activity and cognitive ability. This approach will provide a more nuanced understanding of how physical activity and cognitive performance interact and offer valuable scientific evidence for developing more effective public health policies and interventions.
Data availability statement
The original contributions presented in the study are included in the article/supplementary material, further inquiries can be directed to the corresponding author.
Ethics statement
All study procedures were performed by the Declaration of Helsinki, and all study procedures were approved by the College of Physical Education and Sports Science, Beijing Normal University – Beijing, China. A relevant institutional review board had approved studies included in the GWAS and participants had provided informed consent.
Author contributions
YL: Conceptualization, Data curation, Formal analysis, Investigation, Methodology, Project administration, Resources, Supervision, Writing – original draft, Writing – review & editing. CF: Conceptualization, Data curation, Project administration, Writing – original draft, Writing – review & editing. HS: Conceptualization, Methodology, Writing – original draft, Writing – review & editing. ZZ: Conceptualization, Writing – original draft, Writing – review & editing. TL: Conceptualization, Supervision, Validation, Writing – original draft, Writing – review & editing.
Funding
The author(s) declare that no financial support was received for the research, authorship, and/or publication of this article.
Conflict of interest
The authors declare that the research was conducted in the absence of any commercial or financial relationships that could be construed as a potential conflict of interest.
Publisher’s note
All claims expressed in this article are solely those of the authors and do not necessarily represent those of their affiliated organizations, or those of the publisher, the editors and the reviewers. Any product that may be evaluated in this article, or claim that may be made by its manufacturer, is not guaranteed or endorsed by the publisher.
References
Aguiló, A., Tauler, P., Fuentespina, E., Tur, J. A., Córdova, A., and Pons, A. (2005). Antioxidant response to oxidative stress induced by exhaustive exercise. Physiol. Behav. 84, 1–7. doi: 10.1016/j.physbeh.2004.07.034
Albert, M. S., Dekosky, S. T., Dickson, D., Dubois, B., Feldman, H. H., Fox, N. C., et al. (2011). The diagnosis of mild cognitive impairment due to Alzheimer's disease: recommendations from the National Institute on Aging-Alzheimer's Association workgroups on diagnostic guidelines for Alzheimer's disease. Alzheimers Dement. 7, 270–279. doi: 10.1016/j.jalz.2011.03.008
Anblagan, D., Hernandez, M. C. V., Ritchie, S. J., Aribisala, B. S., Royle, N. A., Hamilton, I. F., et al. (2018). Coupled changes in hippocampal structure and cognitive ability in later life. Brain Behav. 8:e00838. doi: 10.1002/brb3.838
Barati, S., Fabrizio, C., Strafella, C., Cascella, R., Caputo, V., Megalizzi, D., et al. (2022). Relationship between nutrition, lifestyle, and neurodegenerative disease: lessons from ADH1B, CYP1A2 and MTHFR. Genes 13:1498. doi: 10.3390/genes13081498
Barbosa, A., Whiting, S., Simmonds, P., Moreno, R. S., Mendes, R., and Breda, J. (2020). Physical activity and academic achievement: an umbrella review. Int. J. Environ. Res. Public Health 17:5972. doi: 10.3390/ijerph17165972
Bassett, D. R. (2003). International physical activity questionnaire: 12-country reliability and validity. Med. Sci. Sports Exerc. 35:1396. doi: 10.1249/01.MSS.0000078923.96621.1D
Berchtold, N. C., Chinn, G., Chou, M., Kesslak, J. P., and Cotman, C. W. (2005). Exercise primes a molecular memory for brain-derived neurotrophic factor protein induction in the rat hippocampus. Neuroscience 133, 853–861. doi: 10.1016/j.neuroscience.2005.03.026
Besser, L. M., Brenowitz, W. D., Meyer, O. L., Hoermann, S., and Renne, J. (2021). Methods to address self-selection and reverse causation in studies of neighborhood environments and brain health. Int. J. Environ. Res. Public Health 18:6484. doi: 10.3390/ijerph18126484
Blomstrand, E., Perrett, D., Parry-Billings, M., and Newsholme, E. (1989). Effect of sustained exercise on plasma amino acid concentrations and on 5-hydroxytryptamine metabolism in six different brain regions in the rat. Acta Physiol. Scand. 136, 473–482. doi: 10.1111/j.1748-1716.1989.tb08689.x
Bowden, J., Smith, G. D., and Burgess, S. (2015). Mendelian randomization with invalid instruments: effect estimation and bias detection through egger regression. Int. J. Epidemiol. 44, 512–525. doi: 10.1093/ije/dyv080
Brisswalter, J., Collardeau, M., and Arcelin, R. (2002). Effects of acute physical exercise characteristics on cognitive performance. Sports Med. 32, 555–566. doi: 10.2165/00007256-200232090-00002
Burgess, S., Bowden, J., Fall, T., Ingelsson, E., and Thompson, S. G. (2017). Sensitivity analyses for robust causal inference from Mendelian randomization analyses with multiple genetic variants. Epidemiology 28, 30–42. doi: 10.1097/EDE.0000000000000559
Chen, L. J., Steptoe, A., Chung, M. S., and Ku, P. W. (2016). Association between actigraphy-derived physical activity and cognitive performance in patients with schizophrenia. Psychol. Med. 46, 2375–2384. doi: 10.1017/S0033291716000921
Cheval, B., Orsholits, D., Sieber, S., Courvoisier, D., Cullati, S., and Boisgontier, M. P. (2020). Relationship between decline in cognitive resources and physical activity. Health Psychol. 39, 519–528. doi: 10.1037/hea0000857
Cheval, B., Rebar, A. L., Miller, M. W., Sieber, S., Orsholits, D., Baranyi, G., et al. (2019). Cognitive resources moderate the adverse impact of poor perceived neighborhood conditions on self-reported physical activity of older adults. Prev. Med. 126:105741. doi: 10.1016/j.ypmed.2019.05.029
Colcombe, S., and Kramer, A. F. (2003). Fitness effects on the cognitive function of older adults: a meta-analytic study. Psychol. Sci. 14, 125–130. doi: 10.1111/1467-9280.t01-1-01430
Cotman, C. W., and Berchtold, N. C. (2002). Exercise: a behavioral intervention to enhance brain health and plasticity. Trends Neurosci. 25, 295–301. doi: 10.1016/S0166-2236(02)02143-4
Cui, D., Qiu, F., Qiu, F., Liu, J., Zhu, X., and Tang, X. (2021). Effect of participation physical activity on health, quality of life and well-being for elderly adults: a systematic review. Chin. J. Rehabil. Theory Pract. 27, 1176–1189.
Da Silva, I. C. M., Mielke, G. I., Bertoldi, A. D., Arrais, P. S. D., Luiza, V. L., Mengue, S. S., et al. (2018). Overall and leisure-time physical activity among Brazilian adults: National Survey Based on the global physical activity questionnaire. J. Phys. Act. Health 15, 212–218. doi: 10.1123/jpah.2017-0262
Daly, M., Mcminn, D., and Allan, J. L. (2015). A bidirectional relationship between physical activity and executive function in older adults. Front. Hum. Neurosci. 8:1044. doi: 10.3389/fnhum.2014.01044
Donnelly, J. E., Hillman, C. H., Castelli, D., Etnier, J. L., Lee, S., Tomporowski, P., et al. (2016). Physical activity, fitness, cognitive function, and academic achievement in children: a systematic review. Med. Sci. Sports Exerc. 48, 1223–1224. doi: 10.1249/MSS.0000000000000966
Eckardt, M. J., File, S. E., Gessa, G. L., Grant, K. A., Guerri, C., Hoffman, P. L., et al. (1998). Effects of moderate alcohol consumption on the central nervous system. Alcohol. Clin. Exp. Res. 22, 998–1040. doi: 10.1111/j.1530-0277.1998.tb03695.x
Ekelund, U., Sepp, H., Brage, S., Becker, W., Jakes, R., Hennings, M., et al. (2006). Criterion-related validity of the last 7-day, short form of the international physical activity questionnaire in Swedish adults. Public Health Nutr. 9, 258–265. doi: 10.1079/PHN2005840
El-Falougy, H., and Benuska, J. (2006). History, anatomical nomenclature, comparative anatomy and functions of the hippocampal formation. Bratislavske Lekarske Listy 107, 103–106
Elias, M. F., Elias, P. K., Sullivan, L. M., Wolf, P. A., and D'agostino, R. B. (2003). Lower cognitive function in the presence of obesity and hypertension: the Framingham heart study. Int. J. Obes. 27, 260–268. doi: 10.1038/sj.ijo.802225
Erickson, K. I., Voss, M. W., Prakash, R. S., Basak, C., Szabo, A., Chaddock, L., et al. (2011). Exercise training increases size of hippocampus and improves memory. Proc. Natl. Acad. Sci. USA 108, 3017–3022. doi: 10.1073/pnas.1015950108
Esteban-Cornejo, I., Hallal, P. C., Mielke, G. I., Menezes, A. M. B., Goncalves, H., Wehrmeister, F., et al. (2015). Physical activity throughout adolescence and cognitive performance at 18 years of age. Med. Sci. Sports Exerc. 47, 2552–2557. doi: 10.1249/MSS.0000000000000706
Farmer, J., Zhao, X., Van Praag, H., Wodtke, K., Gage, F., and Christie, B. (2004). Effects of voluntary exercise on synaptic plasticity and gene expression in the dentate gyrus of adult male Sprague–dawley rats in vivo. Neuroscience 124, 71–79. doi: 10.1016/j.neuroscience.2003.09.029
Feldman, D. I., Al-Mallah, M. H., Keteyian, S. J., Brawner, C. A., Feldman, T., Blumenthal, R. S., et al. (2015). No evidence of an upper threshold for mortality benefit at high levels of cardiorespiratory fitness. J. Am. Coll. Cardiol. 65, 629–630. doi: 10.1016/j.jacc.2014.11.030
Frodl, T., Skokauskas, N., Frey, E.-M., Morris, D., Gill, M., and Carballedo, A. (2014). Bdnf Val66Met genotype interacts with childhood adversity and influences the formation of hippocampal subfields. Hum. Brain Mapp. 35, 5776–5783. doi: 10.1002/hbm.22584
Gale, C. R., Batty, G. D., Tynelius, P., Deary, I. J., and Rasmussen, F. (2010). Intelligence in early adulthood and subsequent hospitalization for mental disorders. Epidemiology 21, 70–77. doi: 10.1097/EDE.0b013e3181c17da8
Gauthier, S., Patterson, C., Gordon, M., Soucy, J.-P., Schubert, F., and Leuzy, A. (2011). Commentary on "recommendations from the National Institute on Aging-Alzheimer's Association workgroups on diagnostic guidelines for Alzheimer's disease." a Canadian perspective. Alzheimers Dement. 7, 330–332. doi: 10.1016/j.jalz.2011.03.006
Geinisman, Y., Detoledomorrell, L., Morrell, F., and Heller, R. E. (1995). Hippocampal markers of age-related memory dysfunction-behavioral, electrophysiological and morphological perspectives. Prog. Neurobiol. 45, 223–252. doi: 10.1016/0301-0082(94)00047-L
Gilovich, T., Griffin, D., and Kahneman, D. (2002). Heuristics and biases: the psychology of intuitive judgment. Cambridge, UK: Cambridge University Press.
Griffin, E. W., Mullally, S., Foley, C., Warmington, S. A., O’mara, S. M., and Kelly, A. M. (2011). Aerobic exercise improves hippocampal function and increases Bdnf in the serum of young adult males. Physiol. Behav. 104, 934–941. doi: 10.1016/j.physbeh.2011.06.005
Gunstad, J., Paul, R. H., Cohen, R. A., Tate, D. F., Spitznagel, M. B., and Gordon, E. (2007). Elevated body mass index is associated with executive dysfunction in otherwise healthy adults. Compr. Psychiatry 48, 57–61. doi: 10.1016/j.comppsych.2006.05.001
Haapanen, N., Miilunpalo, S., Vuori, I., Oja, P., and Pasanen, M. (1997). Association of leisure time physical activity with the risk of coronary heart disease, hypertension and diabetes in middle-aged men and women. Int. J. Epidemiol. 26, 739–747. doi: 10.1093/ije/26.4.739
Hakansson, K., Ledreux, A., Daffner, K., Terjestam, Y., Bergman, P., Carlsson, R., et al. (2017). Bdnf responses in healthy older persons to 35 minutes of physical exercise, cognitive training, and mindfulness: associations with working memory function. J. Alzheimer Dis. 55, 645–657. doi: 10.3233/JAD-160593
Hall, J., Thomas, K. L., and Everitt, B. J. (2000). Rapid and selective induction of Bdnf expression in the hippocampus during contextual learning. Nat. Neurosci. 3, 533–535. doi: 10.1038/75698
Hart, C. L., Taylor, M. D., Smith, G. D., Whalley, L. J., Starr, J. M., Hole, D. J., et al. (2003). Childhood Iq, social class, deprivation, and their relationships with mortality and morbidity risk in later life: prospective observational study linking the Scottish mental survey 1932 and the Midspan studies. Psychosom. Med. 65, 877–883. doi: 10.1097/01.PSY.0000088584.82822.86
Hu, M., Wang, X., and Yang, Y. (2023). Causal relationship between moderate to vigorous physical activity and venous thromboembolism. J. Thromb. Thrombolysis 55, 576–583. doi: 10.1007/s11239-022-02754-x
Idler, E. L., and Benyamini, Y. (1997). Self-rated health and mortality: a review of twenty-seven community studies. J. Health Soc. Behav. 38, 21–37. doi: 10.2307/2955359
Isaacs, K. R., Anderson, B. J., Alcantara, A. A., Black, J. E., and Greenough, W. T. (1992). Exercise and the brain: angiogenesis in the adult rat cerebellum after vigorous physical activity and motor skill learning. J. Cereb. Blood Flow Metab. 12, 110–119. doi: 10.1038/jcbfm.1992.14
Jaycox, L. H., Stein, B. D., Paddock, S., Miles, J. N. V., Chandra, A., Meredith, L. S., et al. (2009). Impact of teen depression on academic, social, and physical functioning. Pediatrics 124, E596–E605. doi: 10.1542/peds.2008-3348
Jelleyman, C., Yates, T., O’donovan, G., Gray, L. J., King, J. A., Khunti, K., et al. (2015). The effects of high-intensity interval training on glucose regulation and insulin resistance: a meta-analysis. Obes. Rev. 16, 942–961. doi: 10.1111/obr.12317
Khan, N. A., and Hillman, C. H. (2014). The relation of childhood physical activity and aerobic fitness to brain function and cognition: a review. Pediatr. Exerc. Sci. 26, 138–146. doi: 10.1123/pes.2013-0125
Klimentidis, Y. C., Raichlen, D. A., Bea, J., Garcia, D. O., Wineinger, N. E., Mandarino, L. J., et al. (2018). Genome-wide association study of habitual physical activity in over 377, 000 Uk biobank participants identifies multiple variants including Cadm2 and Apoe. Int. J. Obes. 42, 1161–1176. doi: 10.1038/s41366-018-0120-3
Konishi, K., Mckenzie, S., Etcharnendy, N., Roy, S., and Bohbot, V. D. (2017). Hippocampus-dependent spatial learning is associated with higher global cognition among healthy older adults. Neuropsychologia 106, 310–321. doi: 10.1016/j.neuropsychologia.2017.09.025
Latino, F., and Tafuri, F. (2023). Physical activity and academic performance in school-age children: a systematic review. Sustain. For. 15:6616. doi: 10.3390/su15086616
Lawlor, D. A., Batty, G. D., Clark, H., Mcintyre, S., and Leon, D. A. (2008a). Association of childhood intelligence with risk of coronary heart disease and stroke: findings from the Aberdeen children of the 1950s cohort study. Eur. J. Epidemiol. 23, 695–706. doi: 10.1007/s10654-008-9281-z
Lawlor, D. A., Harbord, R. M., Sterne, J. A. C., Timpson, N., and Smith, G. D. (2008b). Mendelian randomization: using genes as instruments for making causal inferences in epidemiology. Stat. Med. 27, 1133–1163. doi: 10.1002/sim.3034
Lee, M. S., Jun, J. H., Lim, H.-J., and Lim, H.-S. (2015). A systematic review and meta-analysis of tai chi for treating type 2 diabetes. Maturitas 80, 14–23. doi: 10.1016/j.maturitas.2014.09.008
Lee, I.-M., Sesso, H. D., and Paffenbarger, R. S. Jr. (2000). Physical activity and coronary heart disease risk in men: does the duration of exercise episodes predict risk? Circulation 102, 981–986. doi: 10.1161/01.CIR.102.9.981
Lee, J. J., Wedow, R., Okbay, A., Kong, E., Maghzian, O., Zacher, M., et al. (2018). Gene discovery and polygenic prediction from a genome-wide association study of educational attainment in 1.1 million individuals. Nat. Genet. 50:1112. -+. doi: 10.1038/s41588-018-0147-3
Liu, P. Z., and Nusslock, R. (2018). Exercise-mediated neurogenesis in the Hippocampus via Bdnf. Front. Neurosci. 12:52. doi: 10.3389/fnins.2018.00052
Marrocco, J., Einhorn, N. R., Petty, G. H., Li, H., Dubey, N., Hoffman, J., et al. (2020). Epigenetic intersection of Bdnf Val66Met genotype with premenstrual dysphoric disorder transcriptome in a cross-species model of estradiol add-back. Mol. Psychiatry 25, 572–583. doi: 10.1038/s41380-018-0274-3
Marsh, H. W., and Kleitman, S. (2003). School athletic participation: mostly gain with little pain. J. Sport Exerc. Psychol. 25, 205–228. doi: 10.1123/jsep.25.2.205
Mastaloudis, A., Leonard, S. W., and Traber, M. G. (2001). Oxidative stress in athletes during extreme endurance exercise. Free Radic. Biol. Med. 31, 911–922. doi: 10.1016/S0891-5849(01)00667-0
Matsushita, M., Harada, K., and Arao, T. (2015). Socioeconomic position and work, travel, and recreation-related physical activity in Japanese adults: a cross-sectional study. BMC Public Health 15:916. doi: 10.1186/s12889-015-2226-z
Mountjoy, M., Andersen, L. B., Armstrong, N., Biddle, S., Boreham, C., Bedenbeck, H.-P. B., et al. (2011). International Olympic Committee consensus statement on the health and fitness of young people through physical activity and sport. Br. J. Sports Med. 45, 839–848. doi: 10.1136/bjsports-2011-090228
Netz, Y., Wu, M.-J., Becker, B. J., and Tenenbaum, G. (2005). Physical activity and psychological well-being in advanced age: a meta-analysis of intervention studies. Psychol. Aging 20, 272–284. doi: 10.1037/0882-7974.20.2.272
Ngandu, T., Lehtisalo, J., Solomon, A., Levalahti, E., Ahtiluoto, S., Antikainen, R., et al. (2015). A 2 year multidomain intervention of diet, exercise, cognitive training, and vascular risk monitoring versus control to prevent cognitive decline in at-risk elderly people (finger): a randomised controlled trial. Lancet 385, 2255–2263. doi: 10.1016/S0140-6736(15)60461-5
Palasz, E., Wysocka, A., Gasiorowska, A., Chalimoniuk, M., Niewiadomski, W., and Niewiadomska, G. (2020). BDNF as a promising therapeutic agent in Parkinson's disease. Int. J. Mol. Sci. 21:1170. doi: 10.3390/ijms21031170
Pani, J., Reitlo, L. S., Evensmoen, H. R., Lydersen, S., Wisloff, U., Stensvold, D., et al. (2021). Effect of 5 years of exercise intervention at different intensities on brain structure in older adults from the general population: a generation 100 substudy. Clin. Interv. Aging 16, 1485–1501. doi: 10.2147/CIA.S318679
Papadimitriou, N., Dimou, N., Tsilidis, K. K., Banbury, B., Martin, R. M., Lewis, S. J., et al. (2020). Physical activity and risks of breast and colorectal cancer: a Mendelian randomisation analysis. Nat. Commun. 11:597. doi: 10.1038/s41467-020-14389-8
Radak, Z., Suzuki, K., Higuchi, M., Balogh, L., Boldogh, I., and Koltai, E. (2016). Physical exercise, reactive oxygen species and neuroprotection. Free Radic. Biol. Med. 98, 187–196. doi: 10.1016/j.freeradbiomed.2016.01.024
Reas, E. T., Laughlin, G. A., Bergstrom, J., Kritz-Silverstein, D., and Mcevoy, L. K. (2019). Physical activity and trajectories of cognitive change in community-dwelling older adults: the rancho Bernardo Study. J. Alzheimer Dis. 71, 109–118. doi: 10.3233/JAD-190491
Reuben, A., Brickman, A. M., Muraskin, J., Steffener, J., and Stern, Y. (2011). Hippocampal atrophy relates to fluid intelligence decline in the elderly. J. Int. Neuropsychol. Soc. 17, 56–61. doi: 10.1017/S135561771000127X
Richmond, R. C., and Smith, G. D. (2022). Mendelian randomization: concepts and scope. Cold spring Harb. Perspect. Med. 12:a040501. doi: 10.1101/cshperspect.a040501
Robitaille, A., Muniz, G., Lindwall, M., Piccinin, A. M., Hoffman, L., Johansson, B., et al. (2014). Physical activity and cognitive functioning in the oldest old: within-and between-person cognitive activity and psychosocial mediators. Eur. J. Ageing 11, 333–347. doi: 10.1007/s10433-014-0314-z
Rodriguez, B. L., Curb, J. D., Burchfiel, C. M., Abbott, R. D., Petrovitch, H., Masaki, K., et al. (1994). Physical activity and 23-year incidence of coronary heart disease morbidity and mortality among middle-aged men the Honolulu Heart Program. Circulation 89, 2540–2544.
Sabia, S., Dugravot, A., Dartigues, J.-F., Abell, J., Elbaz, A., Kivimaki, M., et al. (2017). Physical activity, cognitive decline, and risk of dementia: 28 year follow-up of Whitehall ii cohort study. BMJ 357:j2709. doi: 10.1136/bmj.j2709
Salthouse, T. A. (1996). The processing-speed theory of adult age differences in cognition. Psychol. Rev. 103, 403–428. doi: 10.1037/0033-295X.103.3.403
Samuel, R. D., Zavdy, O., Levav, M., Reuveny, R., Katz, U., and Dubnov-Raz, G. (2017). The effects of maximal intensity exercise on cognitive performance in children. J. Hum. Kinet. 57, 85–96. doi: 10.1515/hukin-2017-0050
Scarmeas, N., Levy, G., Tang, M.-X., Manly, J., and Stern, Y. (2001). Influence of leisure activity on the incidence of Alzheimer’s disease. Neurology 57, 2236–2242. doi: 10.1212/WNL.57.12.2236
Scarmeas, N., and Stern, Y. (2004). Cognitive reserve: implications for diagnosis and prevention of Alzheimer's disease. Curr. Neurol. Neurosci. Rep. 4, 374–380. doi: 10.1007/s11910-004-0084-7
Smith, G. D., and Ebrahim, S. (2003). 'Mendelian randomization': can genetic epidemiology contribute to understanding environmental determinants of disease? Int. J. Epidemiol. 32, 1–22. doi: 10.1093/ije/dyg070
Smith, E., Hay, P., Campbell, L., and Trollor, J. N. (2011). A review of the association between obesity and cognitive function across the lifespan: implications for novel approaches to prevention and treatment. Obes. Rev. 12, 740–755. doi: 10.1111/j.1467-789X.2011.00920.x
Strafella, C., Caputo, V., Galota, M. R., Zampatti, S., Marella, G., Mauriello, S., et al. (2018). Application of precision medicine in neurodegenerative diseases. Front. Neurol. 9:701. doi: 10.3389/fneur.2018.00701
Strine, T. W., and Chapman, D. P. (2005). Associations of frequent sleep insufficiency with health-related quality of life and health behaviors. Sleep Med. 6, 23–27. doi: 10.1016/j.sleep.2004.06.003
Suminski, R. R., Ding, D., Lee, R., May, L., Tota, T., and Dinius, D. (2011). Youth physical activity opportunities in lower and higher income neighborhoods. J. Urban Health 88, 599–615. doi: 10.1007/s11524-011-9577-2
Trampush, J. W., Yang, M. L. Z., Yu, J., Knowles, E., Davies, G., Liewald, D. C., et al. (2017). Gwas meta-analysis reveals novel loci and genetic correlates for general cognitive function: a report from the Cogent consortium (vol 22, pg 336, 2017). Mol. Psychiatry 22, 1651–1652. doi: 10.1038/mp.2017.197
Travlos, A. K. (2010). High intensity physical education classes and cognitive performance in eighth-grade students: an applied study. Int. J. Sport Exerc. Psychol. 8, 302–311. doi: 10.1080/1612197X.2010.9671955
Tyrrell, D. A. J., and Pereira, H. G. (1980). Philosophical transactions of the Royal Society of London - B biological sciences-influenza-preface. Philos. Trans. R. Soc. Lond. B Biol. Sci. 288:289.
Varma, V. R., Chuang, Y.-F., Harris, G. C., Tan, E. J., and Carlson, M. C. (2015). Low-intensity daily walking activity is associated with hippocampal volume in older adults. Hippocampus 25, 605–615. doi: 10.1002/hipo.22397
Vilor-Tejedor, N., Operto, G., Evans, T. E., Falcon, C., Crous-Bou, M., Minguillon, C., et al. (2020). Effect of Bdnf Val66Met on hippocampal subfields volumes and compensatory interaction with Apoe−ε4 in middle-age cognitively unimpaired individuals from the Alfa study. Brain Struct. Funct. 225, 2331–2345. doi: 10.1007/s00429-020-02125-3
Wang, X., Zhang, J., Chen, C., Lu, Z., Zhang, D., and Li, S. (2023). The association between physical activity and cognitive function in the elderly in rural areas of northern China. Front. Aging Neurosci. 15:1168892. doi: 10.3389/fnagi.2023.1168892
Weaver, A. N., and Jaeggi, S. M. (2021). Activity engagement and cognitive performance amongst older adults. Front. Psychol. 12:620867. doi: 10.3389/fpsyg.2021.620867
Wollseiffen, P., Ghadiri, A., Scholz, A., Strueder, H. K., Herpers, R., Peters, T., et al. (2016). Short bouts of intensive exercise during the workday have a positive effect on neuro-cognitive performance. Stress. Health 32, 514–523. doi: 10.1002/smi.2654
Keywords: moderate to vigorous physical activity, cognitive performance, Mendelian randomization, multivariate MR analysis, epigenetics
Citation: Li Y, Fu C, Song H, Zhang Z and Liu T (2024) Prolonged moderate to vigorous physical activity may lead to a decline in cognitive performance: a Mendelian randomization study. Front. Aging Neurosci. 16:1403464. doi: 10.3389/fnagi.2024.1403464
Edited by:
Kristin Rene Krueger, Rush University Medical Center, United StatesReviewed by:
Roy James Hardman, Swinburne University of Technology, AustraliaTeresa Dianne Hawkes, Applied Research Solutions, United States
Copyright © 2024 Li, Fu, Song, Zhang and Liu. This is an open-access article distributed under the terms of the Creative Commons Attribution License (CC BY). The use, distribution or reproduction in other forums is permitted, provided the original author(s) and the copyright owner(s) are credited and that the original publication in this journal is cited, in accordance with accepted academic practice. No use, distribution or reproduction is permitted which does not comply with these terms.
*Correspondence: Tianbiao Liu, bHRiQGJudS5lZHUuY24=
†These authors have contributed equally to this work