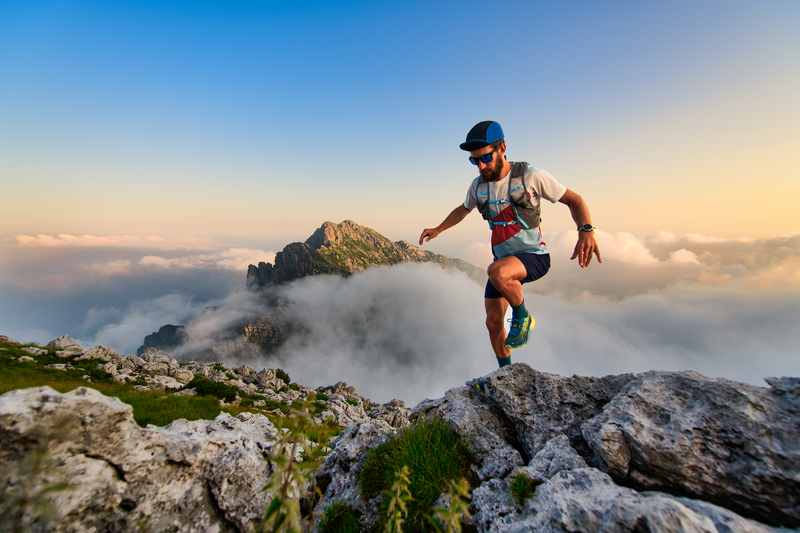
95% of researchers rate our articles as excellent or good
Learn more about the work of our research integrity team to safeguard the quality of each article we publish.
Find out more
ORIGINAL RESEARCH article
Front. Aging Neurosci. , 26 February 2024
Sec. Neurocognitive Aging and Behavior
Volume 16 - 2024 | https://doi.org/10.3389/fnagi.2024.1357347
Introduction: Deterioration of cognitive functions is commonly associated with aging, although there is wide variation in the onset and manifestation. Albeit heterogeneity in age-related cognitive decline has been studied at the cellular and molecular level, there is poor evidence for electrophysiological correlates. The aim of the current study was to address the electrophysiological basis of heterogeneity of cognitive functions in cognitively Inferior and Superior old (19-20 months) rats in the ventral tegmental area (VTA) and the hippocampus, having Young (12 weeks) rats as a control. The midbrain VTA operates as a hub amidst affective and cognitive facets, processing sensory inputs related to motivated behaviours and hippocampal memory. Increasing evidence shows direct dopaminergic and non-dopaminergic input from the VTA to the hippocampus.
Methods: Aged Superior and Inferior male rats were selected from a cohort of 88 animals based on their performance in a spatial learning and memory task. Using in vivo single-cell recording in the VTA, we examined the electrical activity of different neuronal populations (putative dopaminergic, glutamatergic and GABAergic neurons). In the same animals, basal synaptic transmission and synaptic plasticity were examined in hippocampal slices.
Results: Electrophysiological recordings from the VTA and hippocampus showed alterations associated with aging per se, together with differences specifically linked to the cognitive status of aged animals. In particular, the bursting activity of dopamine neurons was lower, while the firing frequency of glutamatergic neurons was higher in VTA of Inferior old rats. The response to high-frequency stimulation in hippocampal slices also discriminated between Superior and Inferior aged animals.
Discussion: This study provides new insight into electrophysiological information underlying compromised cerebral ageing. Further understanding of brain senescence, possibly related to neurocognitive decline, will help develop new strategies towards the preservation of a high quality of life.
In the last decades, improved medical care and lifestyle contributed to extending life expectancy (Friedman, 2020). The process of aging is highly variable and not easily predictable, being influenced by several factors, ranging from genetics to lifestyle and dietary habits and incidental events. Preservation of well-being, independence, and high quality of life relies on the maintenance of molecular and functional processes underlying affective and cognitive brain activity (Cabeza et al., 2018; Aron et al., 2022).
Among other brain regions, the limbic system has been shown to be compromised throughout the lifespan (Penner and Mizumori, 2012; Bettio et al., 2017). The limbic system operates as a hub amidst affective and cognitive facets by processing sensory inputs related to motivated behaviors and memory (McLachlan, 2009). In this regard, the midbrain ventral tegmental area (VTA) is a major source of dopamine projections, either by unique-or by GABA/Glutamate co-release (Sulzer et al., 1998; Tritsch et al., 2012; Cai and Ford, 2018) to subcortical limbic structures, such as the nucleus accumbens and the hippocampus, which are implicated in positive/negative reinforcement and memory, respectively (Scatton et al., 1980; Swanson, 1982; Itoh et al., 1984; Verney et al., 1985; Gasbarri et al., 1994; Lammel et al., 2014; Tsetsenis et al., 2022). Additionally, a subset of non-dopaminergic neurons has been shown to send projections from the VTA to the hippocampus (Ntamati and Luscher, 2016), although their functional relevance remains to be fully elucidated.
Impairment of these brain areas has been associated with age-related pathological conditions, such as dementia and Alzheimer’s disease (AD). Besides the well-known degeneration of the hippocampus (Jaroudi et al., 2017; Josephs et al., 2017; Rao et al., 2022), impairments of the VTA and the dopaminergic system in AD have been proposed (Nobili et al., 2017; Cordella et al., 2018; Bozzali et al., 2019; Krashia et al., 2019; Gloria et al., 2021; La Barbera et al., 2022; Spoleti et al., 2024). Indeed, understanding age-related mechanisms in the VTA and limbic projections could provide novel preventive and/or therapeutic approaches (Cordella et al., 2018; La Barbera et al., 2021; Spoleti et al., 2022), a major goal to counteract age-related cognitive decline as well as dementia and AD (Scheltens et al., 2021). Accordingly, our previous findings indicate that modulation of the dopamine system by the selective dopamine transporter (DAT) inhibition could prove beneficial to restoring several brain alterations associated with aging and age-related cognitive decline (Sagheddu et al., 2020; Lubec et al., 2021; Kouhnavardi et al., 2022; Lubec et al., 2023; Sagheddu et al., 2023).
In this scenario, we took advantage of our validated method to separate superior-and inferior-performing rats (Lubec et al., 2019, 2021) as a model of healthy versus compromised aging. By combining behavioral and in vivo as well as ex vivo electrophysiological procedures, we investigated the functional outcomes of different cell populations in the VTA and hippocampal plasticity.
Male Sprague–Dawley rats (88 rats with age of 19–20 months and 10 rats 12 weeks old at the beginning of the hole-board experiment) were bred and maintained in the Core Unit of Biomedical Research, Division of Laboratory Animal Science and Genetics, Medical University of Vienna. Rats were housed in groups of two in standard Makrolon cages filled with autoclaved woodchips (temperature: 22 ± 2°C; humidity: 55 ± 5%; 12 h artificial light/12 h dark cycle: light on at 7:00 am). Aged rats were on a low-calorie diet (ssniff, R/M-H Ered II, Soest, Germany) from fourth month of age. Tap water and food was provided ad libitum. All behavioral experiments were performed during the light phase of the light–dark cycle. The study was carried out in accordance with the 2010/63/EU guidelines, evaluated by the ethics committee of the Medical University of Vienna, Austria and approved by the Federal Ministry of Education, Science and Culture, Austria (66.009/0018-V/3b/2019).
Animals were habituated to the experimental room at least 24 h prior to the start of the experiment. Food intake was restricted to reduce the weight of the rats to 85% of their initial body weight. A hole-board test was performed as previously described (Lubec et al., 2019) with minor modifications. The hole-board with the dimension of 1 m × 1 m was manufactured of a black plastic board bounded by a translucent plexiglass wall, with proximal spatial cues (black/white symbols) and surrounded by room structures that served as distal cues. A second board below the testing arena was scattered with food pellets to avoid olfactory orientation. Light was adjusted to 15 lux.
The test started with handling (15 min per day for four consecutive days) to acclimatize animals to human interaction. During the following 2 days, animals were habituated to the hole-board apparatus [free exploration of the maze for 15 min each day with access to food pellets (dustless precision pellets, 45 mg, Bioserv®, Flemington, NJ, USA)]. The training was conducted over four consecutive days (five trials on day 1, four trials on day 2, three trials on day 3 and one trial on day 4) with an inter-trial interval of 20 min. Four out of 16 regularly arranged holes (diameter and depth 7 cm) were baited. The pattern of baited holes remained the same during the entire experiment. Each trial lasted 2 min or until all four pellets were found; exception was the first trial on day 1 (lasting 4 min). The board was cleaned with 1% Incidin® after each trial in order to remove any olfactory cues. All trials were videotaped and the number of hole-visits was manually counted. Reference memory index (RMI) was calculated using the formula (first + revisits of baited holes)/total visits of all holes. Animals’ tracks were acquired using the AnyMaze video-tracking system (Stoelting Co., IL, USA).
The open field consisted of a black wooden board (1.20 m × 1.20 m) surrounded by white wooden walls (50 cm in height); light was adjusted to 15 lux. Each animal was placed in the center of the open field and behavior was recorded for 10 min. Data analysis was performed using the AnyMaze software (Stoelting Co., IL, USA); total distance traveled, immobility time and numbers of inner zone crossing were compared between the groups.
The voluntary sucrose consumption was determined 1 day after conducting the open field. Rats were given a free choice between two bottles for 24 h, one containing tap water and another a 2% sucrose solution. Fluid (sucrose solution or tap water) consumption was calculated by weighing the bottles before and after exposure to the animals. The preference for sucrose was calculated as a percentage of the consumed sucrose solution relative to the total amount of liquid drunk.
Electrophysiological recordings were performed 1–3 months after finishing behavioral tests. Rats were anesthetized with chloral hydrate (300 mg/kg, i.p. and additional doses when necessary) and placed in the stereotaxic apparatus (Kopf, Tujunga, CA, USA). The scalp was retracted and one burr hole was drilled above the ventral tegmental area in the midbrain (AP, +2.0 mm from the tip of the lambda, 0.4–0.6 mm from midline; V, 7.5–8.5 mm from cortical surface), according to the stereotaxic rat brain atlas (Paxinos and Watson, 2007). Single unit activity of neurons was extracellularly recorded with glass micropipettes filled with 2% Pontamine sky blue (Merck, Sigma-Aldrich C8679) dissolved in 0.5 M sodium acetate. Individual action potentials were amplified (DAM80 World Precision Instruments, Sarasota, FL, USA and A-M Systems 1700) and displayed on a digital storage oscilloscope (HM507, Hameg Instruments). Experiments were sampled online and offline with Spike2 software (Cambridge Electronic Design, Cambridge, UK) by a computer connected to a CED 1401 interface (Cambridge Electronic Design, Cambridge, UK). To estimate cell population spontaneous activity, the electrode was passed in 6–9 predetermined tracks separated by 200 μm and the number of active cells encountered in each track was averaged. At the end of the recording session, direct current was passed through the recording micropipette to mark the position of the electrode.
Single putative dopamine cells were identified according to previously published criteria, such as broad action potential with triphasic positive–negative–positive deflections and duration from the start to the negative trough between 1.6 and 2.4 ms (Ungless et al., 2004). The pattern of activity, with firing rate < 10 Hz, was regular, irregular or bursty. Bursts were estimated as the occurrence of two spikes at interspike interval < 80 ms and terminated at interspike interval > 160 ms (Grace and Bunney, 1984a,b). Response to aversive stimuli was tested by applying a 2 s nociceptive mechanical pinch to the hind paw contralateral to the recording brain hemisphere.
Single putative glutamatergic neurons of the VTA were identified as biphasic with positive–negative deflections and duration from the start to the negative trough between 0.8 and 1.2 ms (Ungless et al., 2004). The pattern of activity, with relatively high spontaneous firing rate < 25 Hz, was regular or irregular (Luo et al., 2008).
Putative GABA-containing neurons were identified according to typical biphasic negative–positive deflections and relatively regular spontaneous firing rate < 10 Hz (Steffensen et al., 1998; Luo et al., 2008).
Immediately after finishing in vivo recording, animals were decapitated, and brains were extracted and placed into an ice-cold artificial cerebrospinal fluid (aCSF) solution composed of (mM): 125 NaCl, 2.5 KCl, 20 NaHCO3, 2.5 CaCl2, 1 MgCl2, 25 D-glucose, 1 NaH2PO4 (pH 7.4). Hippocampi were dissected and slices of 400 μm thickness were sectioned perpendicular to the long axis of the hippocampus using the McIlwain tissue chopper (TC752, Campden Instruments LTD., Loughborough, England). Slices were transferred to customized recovery chamber, submerged in aCSF and the chamber was placed in a water bath set at 30°C. Slices were allowed to recover for at least 1 h before electrophysiological measurements. The aCSF solution was continuously bubbled with a carbogenated gas mixture (95% medical O2 + 5% medical CO2). The recordings were done in a small submersion chamber continuously perfused at 3–4 mL/min with aCSF (30 ± 2°C). Only slices from dorsal hippocampus were used for recordings.
Evoked field excitatory post-synaptic potentials (fEPSPs) were recorded in the stratum radiatum of the hippocampal CA1 area via aCSF-filled glass micropipettes (3 ± 1 MΩ) made of capillary-glass (Harvard Apparatus GmbH, Hugo Sachs Elektronik, Germany) in a horizontal puller (P-87, Sutter Instrument, Novato, CA). Electrical stimulation was delivered via a customized Teflon-coated tungsten wire bipolar electrode (⁓50 μm tip diameter) to the CA3 Schaffer collateral axons. Electrical stimulus was generated by an ISO-STIM 01D stimulator (NPI Electronics, Tamm, Germany). Electrophysiological measurements were obtained using an AxoClamp-2B amplifier and digitalized using a Digidata-1440 interface (both from Axon Instruments, Molecular Devices, Berkshire, UK). Data was acquired and analyzed using the pClamp-11 software (Molecular Devices, Berkshire, UK).
Input/output (I/O) curves were generated by plotting the fEPSPs slope values against the increasing square pulses (200 μs, 15 s inter-pulse interval, 0–9 V and 1 V increments) of electrical stimulation. Input values eliciting 40–50% of the maximum field-slope response were used to examine short-term synaptic plasticity, basal synaptic transmission and to deliver high-frequency stimulation to induce potentiation.
To study hippocampal short-term synaptic plasticity, paired-pulse facilitation (PPF) field responses were examined. To this aim, two electrical stimulation pulses with increasing inter-pulse intervals of 20, 40, 60, 80, and 100 ms were delivered.
The protocol used to induce long-term potentiation (LTP) consisted of 5 bursts of electrical stimulation [each with 10 electrical pulses delivered at 100 Hz (200 μs/pulse)] presented at intervals of 500 ms.
LTP was assessed by examining the temporal evolution of the fEPSP measurements before and after LTP induction. For this, the fEPSP values obtained after LTP-induction were normalized to the average of the fEPSP values obtained before LTP-induction (baseline). Changes in the fEPSP slopes (initial decaying phase) were used for quantitative analyses. fEPSPs measurements for baseline, PPF and those obtained after LTP induction were acquired at a frequency of 0.033 Hz. fEPSP slope values across slices (3 to 5) were averaged to yield a single data point for each animal. Two-way ANOVA with Tukey’s post-hoc test was used to identify differences between experimental groups.
No statistical method was used to predetermine sample size. The sample size was chosen based on previous studies in our laboratory. Old animals were not randomly allocated to experimental groups but based on the criteria described in the results section. All experiments were performed by experimenters who were not aware of the assignment to the experimental groups. Data were tested for normal distribution by Shapiro–Wilk test. Differences between means were considered significant at the p < 0.05. All data are expressed as mean ± SEM and were analysed using GraphPad Prism 7 software (GraphPad Software, CA, USA). Detailed statistical parameters for specific experiments are described in the appropriate sections or figure legends.
The effect of aging on spatial learning and memory in 19–20-months-old male Sprague–Dawley rats was evaluated in the hole-board, a food-motivated spatial learning and memory task. The apparatus and the training protocol are presented in Figure 1A. A group of 88 aged rats was trained in a hole-board, and their performance was scored based on their mean reference memory indices (RMI) derived from trials 6, 10 and 13 of the retrieval phase (trials following 24 h after previous training session) (Figure 1B). Old rats having mean RMI one standard deviation above the mean were characterized as Superior (n = 9), whereas rats having mean RMI one standard deviation below the mean were characterized as Inferior performers (n = 9). “Intermediate” performers were used for treatment with novel dopamine transporter inhibitors (not published). Rats with less than 52 hole entries in total over the 13 trials were excluded from the analysis. Based on this criterium, one animal from the young group and 10 from the aged rats were removed from the analysis. Performance of Young rats (n = 9) was analyzed without separation into groups. No significant differences were detected when comparing the mean RMIs of Young and Old rats (Mann–Whitney test, p > 0.05; Figure 1B).
Figure 1. (A) Schematic demonstration of hole-board maze. Animals have to learn and remember the position of four baited holes during four test days and 13 trials. (B) From a cohort of 88 male Sprague–Dawley rats (19–20 months old) “Superior” (green area) and “Inferior” (red area) performers were selected based on their mean reference memory index (RMI) derived from trial 6, 10, and 13 ± 1 standard deviation. Ten aged rats were excluded from the analysis because they did not fulfill the criteria. Individual points represent specific animals and the line indicates the mean of mean RMIs. Performance of Young, Old (Mean of All), Superior old and Inferior old rats in the hole-board test as expressed by RMIs (C) and latency (D) to find all pellets. Two-way RM-ANOVA followed by Tukey’s post-hoc tests, # Superior old vs. Young, * Superior old vs. Inferior old. Total numbers of hole dips in the first trial (E) and over four days of training (F). (G) Food-deprived animals were habituated to hole-board in two sessions (H1 and H2; one session per day) and distance traveled was recorded. Two-way ANOVA followed by Sidak’s multiple comparison test. Values are expressed as mean ± SEM; n = 9 per group; #/*p < 0.05, ##p < 0.01, ###/***p < 0.001, ****p < 0.0001.
Figures 1C,D presents the behavioral data in terms of RMI and latency to complete the task. A two-way ANOVA for repeated measures (RM) on RMI and latency revealed a significant overall difference between four groups [F(3,101) = 12.72, p < 0.0001; F(3,101) = 13.79, p < 0.0001; RMI and latency, respectively] and a significant day effect [F(2.084,210.5) = 10.57, p = 0.0001; F(2.292,231.5) = 15.98, p < 0.0001; RMI and latency, respectively]. Superior rats performed significantly better than Inferior (Tukey’s post-hoc test, p < 0.0001; RMIs and latency) ones. The group of Young rats and Old rats (a whole group of 78 aged rats) performed significantly worse than Superior old rats (p < 0.05) over all 4 days of the training. No significant differences were detected between Young and Old rats (p > 0.05 over all 4 days). A total number of hole dips in the trial 1 (only trial unaffected by the learning process; Figure 1E) and over 4 days of training (Figure 1F) was not significantly different between the groups; therefore, differences in RMIs between the groups cannot be simply attributed to different motivation, however, also cannot be excluded.
We also compared horizontal activity during two habituation sessions (H1 and H2). Both, young and old animals explored the maze significantly more in the first (H1, novelty) session (p < 0.05). A two-way RM-ANOVA revealed a significant overall difference between the groups [F(2,24) = 9.112, p = 0.0011] and a significant “session” effect [F(1,24) = 71.86, p = 0.0001]. Sidak’s post-hoc test revealed significant differences in distance traveled between Superior and Inferior, as well as between Inferior old and Young (p < 0.05), but not between Superior old and Young (Figure 1G).
At least 1 week after completing the hole-board test, spontaneous locomotor activity was evaluated in the open field apparatus. Unlike habituation sessions of the hole-board task, animals were not deprived of food, so exploration was not motivated by reward. Animals were not habituated to the apparatus; therefore, we refer to the first test as novel open field (NOF) and to the second test as familiar open field (FOF). There was a major difference in distance traveled among the three groups [two-way RM-ANOVA, F(2,24) = 37.53, p < 0.0001]. Aged rats showed a decreased amount of locomotor activity as compared to Young (Tukey’s post-hoc test, p < 0.01); no significant difference between Superior and Inferior groups could be detected (p = 0.28 and p = 0.18 for NOF and FOF, respectively; Figures 2A–C,D1,D2). Young rats showed more decrement in locomotion as a function of repeated tests than aged rats (Sidak’s post-hoc test, Young p = 0.0003, aged p > 0.05). There was no significant difference among the groups in number of entries to the inner zone (Figure 2B). Compared to Young, aged rats were less active and spent more time immobile; a significant difference could be detected between Superior and Inferior (p = 0.008 and p = 0.046 for NOF and FOF, respectively; Figure 2C).
Figure 2. Spontaneous locomotor activity and sucrose-preference test in young and aged male Sprague–Dawley rats. (A) Distance travelled, (B) number of entries to the inner zone, (C) time immobile, and (D1-2) representative traces of locomotor activity recorded in novel open field (NOF) session and familiar open field (FOF) session. Two-way RM-ANOVA followed by Sidak’s post-hoc test for multiple comparisons. (E) Water intake (averaged values over three days when only water was provided), (F) water and 2% sucrose intake when two bottles presented, (G) preference for sucrose. One-way ANOVA with Dunnett’ post-hoc test. Data are expressed as a mean with individual data points, n = 9 per group, *p < 0.05, **p < 0.01, ***p < 0.001, ****p < 0.0001.
Next, we compared the hedonic phenotype using the two-bottle sucrose preference test, an indicator of anhedonia in rodents. Young rats consumed significantly more water than aged rats (p < 0.0001), while water intake did not differ between the two groups of aged rats (Figure 2E). No significant differences in sucrose preference could be detected between the groups (Figures 2F,G).
In the same rats (Young n = 9, Superior old n = 8, Inferior old n = 9), the extracellular electrical activity of single cells from the VTA was recorded, and different neuronal populations were sorted out as putative dopaminergic, glutamatergic and GABAergic neurons (Figures 3A, 4A,E, respectively). The response of dopamine neurons to the contralateral paw pinch was not statistically different in the three experimental groups [χ2(4) = 4.49; p = 0.34], being 35 cells inhibited, 3 stimulated, and 12 not responding in Young rats, 18 inhibited, 1 stimulated, and 4 not responding in Superior old and 30 inhibited, 0 stimulated, and 4 not responding in Inferior old (Figure 3B). Similarly, the number of cells per track did not differ [Young n = 61, Superior old n = 45, Inferior old n = 50; one-way ANOVA F(2, 153) = 0.75, p = 0.47; Figure 3C], suggesting a preserved general activity during aging. The average firing frequency [one way ANOVA F(2, 132) = 4.78, p = 0.009, Young n = 58; Superior old n = 35; Inferior old n = 44 cells] and the burst firing, expressed both as percentage of spikes in burst [one way ANOVA F(2, 132) = 5.119, p = 0.007] and as burst rate [one way ANOVA F(2, 117) = 8.003, p = 0.0006], were lower in the Inferior old group (Figures 3D–F, respectively). The coefficient of variation did not differ [one-way ANOVA F(2, 132) = 1.27, p = 0.28, Figure 3G].
Figure 3. Dopamine neurons in the VTA of young and aged rats. (A) Example of typical action potential (AP) waveform (top left) and extracellular recordings (bottom) from different experimental groups (Young n = 9; Superior old n = 8; Inferior old n = 9 rats). The graph (top right) shows the average action potential (AP) duration as measured from the start to the negative trough. (B) Percentage of responding/not responding dopamine neurons to the contralateral paw pinch. The χ2 test on the number of cells (Young n = 35 inhibited, n = 3 stimulated, n = 12 no effect; Superior old n = 18 inhibited, n = 1 stimulated, n = 4 no effect; Inferior old n = 30 inhibited, n = 0 stimulated, n = 4 no effect) showed no difference among groups [χ2(4) = 4.49; p = 0.34]. (C) Bar histograms showing the average number of cells per electrode track [F(2, 153) = 0.75, p = 0.47]. (D) Individual data points and average firing frequency of dopamine neurons [Young n = 58; Superior old n = 35; Inferior old n = 44; F(2, 132) = 4.78, p = 0.009]. Individual data points and the average (E) percentage of spikes in burst [F(2, 132) = 5.119, p = 0.007], (F) burst rate [F(2, 117) = 8.003, p = 0.0006], and (G) coefficient of variation in percentage [F(2, 132) = 1.27, p = 0.28]. Unless differently stated, data are expressed as mean ± s.e.m. and one-way ANOVA was used to assess differences. Tukey’s multiple comparisons test **p < 0.01, and ***p < 0.001 Inferior old vs. Young.
Figure 4. Non-dopaminergic neurons in the VTA of young and aged rats. (A) Example of action potential (AP) waveform (top left) and recordings (bottom) from a putative glutamatergic neuron. The graph (top right) shows the average action potential duration as measured from the start to the negative trough. (B) Bar histograms showing the average number of glutamatergic neurons per electrode track [F(2,151) = 0.49, p = 0.61]. (C) Individual data points and average firing frequency of glutamatergic neurons [Young n = 34; Superior old n = 31; Inferior old n = 34; F(2,96) = 6.09, p = 0.003]. (D) Individual data points and the average of coefficient of variation in percentage [F(2,96) = 1.58, p = 0.21]. (E) Example of action potential waveform (top left) and recordings (bottom) from putative GABAergic neuron. The graph (top right) shows the average action potential duration as measured from the start to the positive trough. (F) Bar histograms showing the average number of GABAergic neurons per electrode track [F(2,148) = 0.58, p = 0.55]. (G) Individual data points and average firing frequency of GABAergic neurons [Young n = 35; Superior old n = 19; Inferior old n = 29; F(2,76) = 5.19, p = 0.007]. (H) Individual data points and the average of coefficient of variation in percentage [F(2,76) = 0.53, p = 0.58]. Data are expressed as mean ± s.e.m. and one-way ANOVA was used to assess differences; Tukey’s multiple comparisons test *p < 0.05 and **p < 0.01 vs. Young; #p < 0.05 vs. Superior old.
Concerning the putative glutamatergic neurons in the VTA, the number of cells per track did not differ between among groups [Young n = 62, Superior old n = 44, Inferior old n = 48; one-way ANOVA F(2,151) = 0.49, p = 0.61; Figure 4B]. The average firing frequency (Young n = 38; Superior old n = 31; Inferior old n = 34 cells) was different [one way ANOVA F(2,96) = 6.09, p = 0.003; Figure 4C], being higher in the Inferior old group (Tukey’s post hoc vs. Young p = 0.0033, vs. Superior old p = 0.036). However, the pattern of activity, as expressed by the coefficient of variation, was not changed [one-way ANOVA F(2,96) = 1.58, p = 0.21; Figure 4D].
Finally, the analysis of putative GABAergic neurons in the VTA showed no difference in the number of cells per track [Young n = 62; Superior old n = 44; Inferior old n = 48; one-way ANOVA F(2,148) = 0.58, p = 0.55; Figure 4F]. The average firing frequency (Young n = 35; Superior old n = 19; Inferior old n = 29 cells) was higher in both Superior and Inferior old as compared with the Young rats [one-way ANOVA F(2,76) = 5.19, p = 0.007; Figure 4G]. The coefficient of variation was not changed among groups [and F(2,76) = 0.53, p = 0.58; Figure 4H].
Basal synaptic transmission and synaptic plasticity were examined by ex vivo electrophysiological recordings from hippocampal slices. Field excitatory postsynaptic potentials (fEPSP) were recorded from the dendritic CA1 layer upon stimulation of the CA3-Schaffer collateral pathway (Figure 5A). We observed that the input - output (I/O) curves of fEPSP slopes in response to different stimulation intensities were significantly depressed in two groups of aged rats when compared with those of Young [two-way RM-ANOVA, F(2,24) = 3.751, p < 0.0382, n = 9 per group; Figures 5B,C], suggesting that basal synaptic transmission was impaired in aged rats. However, there was no difference between the two groups of aged animals. To examine possible differences in presynaptic release mechanisms, a paired-pulse stimulation protocol was applied. In aged animals, PPF was significantly enhanced compared to Young [two-way ANOVA F(2,192) = 31.03, p < 0.0001, n = 9 per group; Figure 5C].
Figure 5. (A) For field potential recordings from hippocampal slices, the stimulating electrode was placed in the Schaffer collateral axons of CA3 and a recording electrode in the Stratum radiatum of CA1 (B) Input/output (I/O) responses were significantly shifted downward in hippocampal slices from Superior and Inferior performing aged rats. Two-way RM-ANOVAwith Tukey’s post-hoc test. (C) Enhanced paired-pulse facilitation in aged animals. Data represent the ratio of the second fEPSP slope to the first fEPSP slope. Two-way ANOVA with Tukey’s post-hoc test. (D) Potentiation induced after high-frequency stimulation (HFS) was significantly enhanced immediately after HFS (first 2 min) and over 40 min of recording in Inferior old rats compared to Young. Two timepoints (2 min and 40 min) are shown as a dot plot. Two-way RM-ANOVA. * Superior old vs. Young, # Inferior old vs. Young. Data are expressed as a mean ± s.e.m., n = 9 per group, #/*p < 0.05, ##p < 0.01, ####/****p < 0.0001.
To identify changes in synaptic plasticity associated with aging or with the cognitive status of old rats, a high-frequency stimulation (HFS) protocol was applied. The HFS protocol induced robust synaptic strengthening responses in all three experimental groups. We found that post-HFS potentiation was significantly enhanced in Inferior old rats, as compared to Young [two-way RM-ANOVA F(1,16) = 5.756, p = 0.029; Figure 5D]. No significant differences were observed between Superior old and Young and between the two groups of aged rats. Post-tetanic potentiation (PTP, the increase of the synaptic response that occurs right after high-frequency stimulation) was significantly higher in Inferior old compared to young rats [two-way RM-ANOVA F(1,16) = 12.70, p = 0.002, n = 9 per group].
Spatial learning and memory are among the cognitive functions that decline with age. Although advanced age is commonly associated with intellectual decline, cognitive impairment is not strictly linked to chronological age, and even in a population with minimal differences in genetic variation and environmental factors, as in our case, there is high variability in how the different cognitive functions are preserved or impaired in each individual. In the present study, our established behavioral procedure (Lubec et al., 2019, 2021) was used to identify aged individuals with Superior and Inferior performance in a hole-board task to address the heterogeneity of cognitive aging. Group of young rats was used as a control; however, based on our previous studies (Lubec et al., 2019, 2021; Pretsch et al., 2020), this group showed unusual heterogeneity in the hole-board test that we cannot explain. Therefore, it is questionable whether the group of young rats is representative of the young population in terms of performance in the hole-board task, which provides a strong argument for increasing the sample size in our future studies.
Old Inferior rats with impaired spatial learning and memory showed reduced exploration in both habituation sessions compared to Young and Superior old animals. Reduced exploration in the first habituation session may be related to decreased response to novelty in aged individuals (Rowe et al., 1998). This was supported by results from the open field where old animals tend to spend more time immobile; however, the environmental novelty did not affect the exploration and/or immobility of old rats (as compared to a second OF test). The reduced general activity was not linked to signs of anhedonia, indicating that depression is not associated with age per se.
Our experimental protocol for spatial learning and memory involves food deprivation as a stimulus to encourage exploratory behavior. Substantial evidence in animals and humans shows that reward prioritizes events for long-term memory storage (Adcock et al., 2006; Wittmann et al., 2007; Braun et al., 2018). Midbrain dopamine neurons are critically involved in reward processing and have been linked to reward location learning (Martig and Mizumori, 2011). Given that dopaminergic signaling is attenuated with age (Backman et al., 2010) and reduced reward sensitivity has been reported in aged rodents and humans (Eppinger et al., 2011; Gilbert et al., 2011; Tryon et al., 2020), this may to be one of the factors contributing to the behavioral outcome in reward-motivated task.
As midbrain dopamine plays a central role in the regulation of several major biological functions like reward processing, novelty, invigoration of movement and motivation (da Silva et al., 2018) and presumably promotes hippocampal network dynamics associated with memory persistence (McNamara et al., 2014), we examined electrophysiological properties of VTA neurons, including putative dopaminergic, glutamatergic and GABAergic neurons. Many studies suggested that several neuronal subpopulations within the VTA form subcircuits that are differentially involved in specific aspects of behavior and are individually affected in several neuropsychiatric disorders (Bariselli et al., 2016). Results from dopamine neurons in old rats are consistent with previous immunohistochemical and electrophysiological studies, which showed no age-related changes in the number and morphology of TH-positive neurons (Pereira et al., 2021) or in firing parameters (Freeman et al., 1989). However, we evidenced altered electrical properties of dopamine neurons in Inferior aged rats, as expressed by the complete loss of response to an acute nociceptive stimulus, reduced firing activity and bursting activity. This accounts for dopamine system dysfunctions during “unhealthy” aging, possibly associated with cognitive impairments. Reduced dopamine function is consistent with exacerbation of neuropsychiatric symptoms during lifespan, ranging from depressive states to substance use disorders (Skoog, 2011; Alexopoulos, 2019; Tampi et al., 2022). Further, these data are consistent with beneficial electrophysiological and behavioral effects of dopamine enhancement through selective reuptake inhibition in aged rats, including compromised aged rats (Sagheddu et al., 2020; Lubec et al., 2021; Kouhnavardi et al., 2022). To the best of our knowledge, this is the first extensive in vivo investigation of non-dopaminergic neurons in the VTA of aged rats. Our data revealed age-related alterations in putative GABAergic neurons as compared with young rats but status-related alterations in glutamatergic neurons in old age. Interestingly, the increased firing frequency of glutamatergic neurons from Inferior aged rats mirrored the reduced firing activity of dopamine neurons in the same area, though correlation is not proven here. The model used here proved robustness to unveil circuit derangements associated with physiological aging and/or brain deterioration, suggesting that the role of non-dopaminergic VTA projections, and particularly their hippocampal branches, deserves further examination. One limitation is that the identification of the different subpopulations of VTA neurons is based solely on the electrophysiological characteristics of the recorded cells.
It is generally assumed that impairment in spatial learning and memory tasks reflects cellular or synaptic dysfunctions in the hippocampus, a region particularly affected by aging. To access age-or cognitive status-related alterations in synaptic functions, we examined evoked field EPSPs at Schaffer collateral CA1 synapses, an area receiving direct dopamine projections from the midbrain (Tsetsenis et al., 2021). There is strong evidence of mechanisms underlying dopaminergic modulation of hippocampal synaptic plasticity (Tsetsenis et al., 2022) and informational flow (Rosen et al., 2015). However, the locus coeruleus (LC) has been described as another source of dopamine in the hippocampus (Kempadoo et al., 2016; Takeuchi et al., 2016; Yamasaki and Takeuchi, 2017; Duszkiewicz et al., 2019), although the LC’s status in the present aging model remains to be addressed.
Here, we show an altered I/O relationship at the CA3-CA1 synapses of aged rats. We assume that the alteration of basal synaptic transmission in aged rats resulted from the decreased probability of neurotransmitter release, as indicated by an increased facilitation index. Notable, Ca2+ is the major trigger of neurotransmitter release and is essential for a variety of other neuronal functions. Aged neurons show a multitude of defects in Ca2+ homeostasis resulting in an increase of Ca2+ loads, which negatively affects neuronal excitability (Moore and Murphy, 2020), increases the threshold frequency for induction of LTP and enhances the vulnerability to the induction of LTD (Nikoletopoulou and Tavernarakis, 2012). In this context, Ca2+ dyshomeostasis has been described as a crucial mechanism underlying cognitive deficits in aging (Uryash et al., 2020). However, changes in basal synaptic transmission and short-term plasticity were specific to advanced age and did not correlate with cognitive status.
Several studies have reported age-related shifts in synaptic plasticity toward reduction of synaptic transmission and decreased ability to induce and maintain LTP (Watson et al., 2002; Burke and Barnes, 2006; Shetty et al., 2017). In addition, reduced LTP after HFS has been associated with impaired spatial memory performance (Bach et al., 1999; Schulz et al., 2002). Controversially, we observed augmented HFS-induced post-tetanic potentiation and LTP in aged compromised rats as compared to Young, while the induction and persistence of LTP in Inferior aged rats were comparable to young animals. However, it should be taken into account that in an effort to examine different brain components in the very same animals, we implemented a methodological approach combining in vivo electrophysiological recordings followed by ex vivo measurement in acutely dissociated hippocampal slices. Special attention was paid to keeping all the experimental conditions equal across all the studied subjects in order to ensure reproducibility and comparability between the obtained results from the different groups. It is noteworthy to mention that a gradual increase in the degree of cognitive decline and neurodegeneration associated with aging has been analyzed in many different contexts and demonstrated not only in humans but also in various animal models (Kay and Sime, 1962; Ingram et al., 1981; Aggleton et al., 1989; Uttl and Graf, 1993; Chalfonte and Johnson, 1996). Thus, aging itself may influence how different regions of the nervous system respond in different experimental procedures, making it difficult to differentiate up to which extent the observed outputs are due to the physiological nature of the endogenous response at a given age and/or cognitive status or due to how nervous tissue recovers from, e.g., a surgical procedure in an experimental context (Landfield et al., 1978; Deupree et al., 1993; Mori et al., 2000; see also Norris et al., 1996; Kumar and Foster, 2005). The herein-described differences in functional responses observed between experimental groups may thus be due not only to the basal state of the nervous system per se but also to how those nervous systems responded to the methodological procedures and pharmacological treatments used, in our case, used anaesthetics. Compounds like choral hydrate (Adrien et al., 1989; LacKamp et al., 2009; Guo et al., 2017; Tamano et al., 2017), isoflurane (Romero-Barragan et al., 2021) or ketamine xylazine cocktails (Alkire et al., 2008; Laverne et al., 2022) are commonly used anaesthetics in electrophysiological studies of brain neuronal circuits. Like several other general anaesthetics, chloral hydrate and its active metabolite trichloroethanol augments GABAergic inhibitory neurotransmission (Peoples and Weight, 1994). Dysregulation of GABAergic signaling in aging is a widely accepted phenomenon, while reduced inhibition in the hippocampus of aged animals (Vela et al., 2003; Stanley and Shetty, 2004) in some instances has been correlated with the cognitive status of aged rats (Haberman et al., 2013; Spiegel et al., 2013). Thus, as mentioned above, a variety of factors could contribute to the results presented here. Further experiments combining several alternative anaesthetic approaches are in demand to expand further the knowledge on the differential effects of different anaesthetics on the properties of synaptic transmission and plasticity across age and brain regions.
Taken together, this study provides novel insights toward the understanding of healthy versus compromised cerebral aging, with a specific focus on the VTA and hippocampus. In this regard, the synergistic use of experimental animal models in tandem with the comprehensive biophysical characterization of the electrical properties of neuronal circuits at brain regions vital for high-order cognitive functions might improve our understanding of the specific impact that aging has on the central nervous system function and its relation to cognitive performance in different population groups.
The raw data supporting the conclusions of this article will be made available by the authors, without undue reservation.
The animal study was approved by the ethics committee of the Medical University of Vienna, Austria and by the Federal Ministry of Education, Science and Culture, Austria. The study was conducted in accordance with the local legislation and institutional requirements.
CS: Conceptualization, Writing – original draft, Writing – review & editing, Formal analysis, Investigation, Methodology, Visualization. TS: Investigation, Methodology, Writing – review & editing. SK: Formal analysis, Investigation, Methodology, Writing – review & editing. AS: Investigation, Methodology, Writing – review & editing. AH: Investigation, Methodology, Writing – review & editing. MP: Funding acquisition, Resources, Writing – review & editing. FM: Methodology, Supervision, Writing – review & editing. RP: Project administration, Supervision, Writing – review & editing. MA: Writing – review & editing. CRS: Resources, Supervision, Writing – review & editing. JL: Conceptualization, Formal analysis, Investigation, Methodology, Visualization, Writing – original draft, Writing – review & editing. GL: Conceptualization, Funding acquisition, Project administration, Resources, Writing – review & editing.
The author(s) declare financial support was received for the research, authorship, and/or publication of this article. The research project was funded from the private bag (GL). The research was partially funded by “Progetti di Rilevante Interesse Nazionale” (PRIN) 2017 (2017YH3SXK) by the Italian Ministry of Research and by Hybrid Hub (H2UB) – POS-T4-AN10 2022 by the Italian Ministry of Health. The project was also partially funded by a VDSEE completion grant.
The authors declare that the research was conducted in the absence of any commercial or financial relationships that could be construed as a potential conflict of interest.
The author(s) declared that they were an editorial board member of Frontiers, at the time of submission. This had no impact on the peer review process and the final decision.
All claims expressed in this article are solely those of the authors and do not necessarily represent those of their affiliated organizations, or those of the publisher, the editors and the reviewers. Any product that may be evaluated in this article, or claim that may be made by its manufacturer, is not guaranteed or endorsed by the publisher.
Adcock, R. A., Thangavel, A., Whitfield-Gabrieli, S., Knutson, B., and Gabrieli, J. D. (2006). Reward-motivated learning: mesolimbic activation precedes memory formation. Neuron 50, 507–517. doi: 10.1016/j.neuron.2006.03.036
Adrien, J., Lanfumey, L., Gozlan, H., Fattaccini, C. M., and Hamon, M. (1989). Biochemical and electrophysiological evidence for an agonist action of CM 57493 at pre-and postsynaptic 5-hydroxytryptamine1A receptors in brain. J. Pharmacol. Exp. Ther. 248, 1222–1230.
Aggleton, J. P., Blindt, H. S., and Candy, J. M. (1989). Working memory in aged rats. Behav. Neurosci. 103, 975–983. doi: 10.1037//0735-7044.103.5.975
Alexopoulos, G. S. (2019). Mechanisms and treatment of late-life depression. Transl. Psychiatry 9:188. doi: 10.1038/s41398-019-0514-6
Alkire, M. T., Hudetz, A. G., and Tononi, G. (2008). Consciousness and anesthesia. Science 322, 876–880. doi: 10.1126/science.1149213
Aron, L., Zullo, J., and Yankner, B. A. (2022). The adaptive aging brain. Curr. Opin. Neurobiol. 72, 91–100. doi: 10.1016/j.conb.2021.09.009
Bach, M. E., Barad, M., Son, H., Zhuo, M., Lu, Y. F., Shih, R., et al. (1999). Age-related defects in spatial memory are correlated with defects in the late phase of hippocampal long-term potentiation in vitro and are attenuated by drugs that enhance the cAMP signaling pathway. Proc. Natl. Acad. Sci. U. S. A. 96, 5280–5285. doi: 10.1073/pnas.96.9.5280
Backman, L., Lindenberger, U., Li, S. C., and Nyberg, L. (2010). Linking cognitive aging to alterations in dopamine neurotransmitter functioning: recent data and future avenues. Neurosci. Biobehav. Rev. 34, 670–677. doi: 10.1016/j.neubiorev.2009.12.008
Bariselli, S., Glangetas, C., Tzanoulinou, S., and Bellone, C. (2016). Ventral tegmental area subcircuits process rewarding and aversive experiences. J. Neurochem. 139, 1071–1080. doi: 10.1111/jnc.13779
Bettio, L. E. B., Rajendran, L., and Gil-Mohapel, J. (2017). The effects of aging in the hippocampus and cognitive decline. Neurosci. Biobehav. Rev. 79, 66–86. doi: 10.1016/j.neubiorev.2017.04.030
Bozzali, M., D'Amelio, M., and Serra, L. (2019). Ventral tegmental area disruption in Alzheimer's disease. Aging (Albany NY) 11, 1325–1326. doi: 10.18632/aging.101852
Braun, E. K., Wimmer, G. E., and Shohamy, D. (2018). Retroactive and graded prioritization of memory by reward. Nat. Commun. 9:4886. doi: 10.1038/s41467-018-07280-0
Burke, S. N., and Barnes, C. A. (2006). Neural plasticity in the ageing brain. Nat. Rev. Neurosci. 7, 30–40. doi: 10.1038/nrn1809
Cabeza, R., Albert, M., Belleville, S., Craik, F. I. M., Duarte, A., Grady, C. L., et al. (2018). Maintenance, reserve and compensation: the cognitive neuroscience of healthy ageing. Nat. Rev. Neurosci. 19, 701–710. doi: 10.1038/s41583-018-0068-2
Cai, Y., and Ford, C. P. (2018). Dopamine cells differentially regulate striatal cholinergic transmission across Regions through corelease of dopamine and glutamate. Cell Rep. 25, 3148–3157 e3143. doi: 10.1016/j.celrep.2018.11.053
Chalfonte, B. L., and Johnson, M. K. (1996). Feature memory and binding in young and older adults. Mem. Cogn. 24, 403–416. doi: 10.3758/bf03200930
Cordella, A., Krashia, P., Nobili, A., Pignataro, A., La Barbera, L., Viscomi, M. T., et al. (2018). Dopamine loss alters the hippocampus-nucleus accumbens synaptic transmission in the Tg2576 mouse model of Alzheimer's disease. Neurobiol. Dis. 116, 142–154. doi: 10.1016/j.nbd.2018.05.006
da Silva, J. A., Tecuapetla, F., Paixao, V., and Costa, R. M. (2018). Dopamine neuron activity before action initiation gates and invigorates future movements. Nature 554, 244–248. doi: 10.1038/nature25457
Deupree, D. L., Bradley, J., and Turner, D. A. (1993). Age-related alterations in potentiation in the CA1 region in F344 rats. Neurobiol. Aging 14, 249–258. doi: 10.1016/0197-4580(93)90009-z
Duszkiewicz, A. J., McNamara, C. G., Takeuchi, T., and Genzel, L. (2019). Novelty and dopaminergic modulation of memory persistence: a tale of two systems. Trends Neurosci. 42, 102–114. doi: 10.1016/j.tins.2018.10.002
Eppinger, B., Hammerer, D., and Li, S. C. (2011). Neuromodulation of reward-based learning and decision making in human aging. Ann. N. Y. Acad. Sci. 1235, 1–17. doi: 10.1111/j.1749-6632.2011.06230.x
Freeman, A. S., Kelland, M. D., Rouillard, C., and Chiodo, L. A. (1989). Electrophysiological characteristics and pharmacological responsiveness of midbrain dopaminergic neurons of the aged rat. J. Pharmacol. Exp. Ther. 249, 790–797.
Friedman, S. M. (2020). Lifestyle (medicine) and healthy aging. Clin. Geriatr. Med. 36, 645–653. doi: 10.1016/j.cger.2020.06.007
Gasbarri, A., Packard, M. G., Campana, E., and Pacitti, C. (1994). Anterograde and retrograde tracing of projections from the ventral tegmental area to the hippocampal formation in the rat. Brain Res. Bull. 33, 445–452. doi: 10.1016/0361-9230(94)90288-7
Gilbert, R. J., Mitchell, M. R., Simon, N. W., Banuelos, C., Setlow, B., and Bizon, J. L. (2011). Risk, reward, and decision-making in a rodent model of cognitive aging. Front. Neurosci. 5:144. doi: 10.3389/fnins.2011.00144
Gloria, Y., Ceyzeriat, K., Tsartsalis, S., Millet, P., and Tournier, B. B. (2021). Dopaminergic dysfunction in the 3xTg-AD mice model of Alzheimer's disease. Sci. Rep. 11:19412. doi: 10.1038/s41598-021-99025-1
Grace, A. A., and Bunney, B. S. (1984a). The control of firing pattern in nigral dopamine neurons: burst firing. J. Neurosci. 4, 2877–2890. doi: 10.1523/JNEUROSCI.04-11-02877.1984
Grace, A. A., and Bunney, B. S. (1984b). The control of firing pattern in nigral dopamine neurons: single spike firing. J. Neurosci. 4, 2866–2876. doi: 10.1523/JNEUROSCI.04-11-02866.1984
Guo, F., Zhao, J., Zhao, D., Wang, J., Wang, X., Feng, Z., et al. (2017). Dopamine D4 receptor activation restores CA1 LTP in hippocampal slices from aged mice. Aging Cell 16, 1323–1333. doi: 10.1111/acel.12666
Haberman, R. P., Colantuoni, C., Koh, M. T., and Gallagher, M. (2013). Behaviorally activated mRNA expression profiles produce signatures of learning and enhanced inhibition in aged rats with preserved memory. PLoS One 8:e83674. doi: 10.1371/journal.pone.0083674
Ingram, D. K., London, E. D., and Goodrick, C. L. (1981). Age and neurochemical correlates of radial maze performance in rats. Neurobiol. Aging 2, 41–47. doi: 10.1016/0197-4580(81)90058-0
Itoh, K., Fukumori, R., and Suzuki, Y. (1984). Effect of methamphetamine on the locomotor activity in the 6-OHDA dorsal hippocampus lesioned rat. Life Sci. 34, 827–833. doi: 10.1016/0024-3205(84)90199-1
Jaroudi, W., Garami, J., Garrido, S., Hornberger, M., Keri, S., and Moustafa, A. A. (2017). Factors underlying cognitive decline in old age and Alzheimer's disease: the role of the hippocampus. Rev. Neurosci. 28, 705–714. doi: 10.1515/revneuro-2016-0086
Josephs, K. A., Dickson, D. W., Tosakulwong, N., Weigand, S. D., Murray, M. E., Petrucelli, L., et al. (2017). Rates of hippocampal atrophy and presence of post-mortem TDP-43 in patients with Alzheimer's disease: a longitudinal retrospective study. Lancet Neurol. 16, 917–924. doi: 10.1016/S1474-4422(17)30284-3
Kay, H., and Sime, M. E. (1962). Discrimination learning with old and young rats. J. Gerontol. 17, 75–80. doi: 10.1093/geronj/17.1.75
Kempadoo, K. A., Mosharov, E. V., Choi, S. J., Sulzer, D., and Kandel, E. R. (2016). Dopamine release from the locus coeruleus to the dorsal hippocampus promotes spatial learning and memory. Proc. Natl. Acad. Sci. USA 113, 14835–14840. doi: 10.1073/pnas.1616515114
Kouhnavardi, S., Ecevitoglu, A., Dragacevic, V., Sanna, F., Arias-Sandoval, E., Kalaba, P., et al. (2022). A novel and selective dopamine transporter inhibitor, (S)-MK-26, promotes hippocampal synaptic plasticity and restores effort-related motivational dysfunctions. Biomol. Ther. 12:881. doi: 10.3390/biom12070881
Krashia, P., Nobili, A., and D'Amelio, M. (2019). Unifying hypothesis of dopamine neuron loss in neurodegenerative diseases: focusing on Alzheimer's disease. Front. Mol. Neurosci. 12:123. doi: 10.3389/fnmol.2019.00123
Kumar, A., and Foster, T. C. (2005). Intracellular calcium stores contribute to increased susceptibility to LTD induction during aging. Brain Res. 1031, 125–128. doi: 10.1016/j.brainres.2004.10.023
La Barbera, L., Nobili, A., Cauzzi, E., Paoletti, I., Federici, M., Saba, L., et al. (2022). Upregulation of ca(2+)-binding proteins contributes to VTA dopamine neuron survival in the early phases of Alzheimer's disease in Tg2576 mice. Mol. Neurodegener. 17:76. doi: 10.1186/s13024-022-00580-6
La Barbera, L., Vedele, F., Nobili, A., Krashia, P., Spoleti, E., Latagliata, E. C., et al. (2021). Nilotinib restores memory function by preventing dopaminergic neuron degeneration in a mouse model of Alzheimer's disease. Prog. Neurobiol. 202:102031. doi: 10.1016/j.pneurobio.2021.102031
LacKamp, A., Zhang, G. C., Mao, L. M., Fibuch, E. E., and Wang, J. Q. (2009). Loss of surface N-methyl-D-aspartate receptor proteins in mouse cortical neurones during anaesthesia induced by chloral hydrate in vivo. Br. J. Anaesth. 102, 515–522. doi: 10.1093/bja/aep009
Lammel, S., Lim, B. K., and Malenka, R. C. (2014). Reward and aversion in a heterogeneous midbrain dopamine system. Neuropharmacology 76, 351–359. doi: 10.1016/j.neuropharm.2013.03.019
Landfield, P. W., McGaugh, J. L., and Lynch, G. (1978). Impaired synaptic potentiation processes in the hippocampus of aged, memory-deficient rats. Brain Res. 150, 85–101. doi: 10.1016/0006-8993(78)90655-8
Laverne, G., Pesce, J., Reynders, A., Combrisson, E., Gascon, E., Melon, C., et al. (2022). Cholinergic interneuron inhibition potentiates corticostriatal transmission in direct medium spiny neurons and rescues motor learning in parkinsonism. Cell Rep. 40:111034. doi: 10.1016/j.celrep.2022.111034
Lubec, J., Hussein, A. M., Kalaba, P., Feyissa, D. D., Arias-Sandoval, E., Cybulska-Klosowicz, A., et al. (2023). Low-affinity/high-selectivity dopamine transport inhibition sufficient to rescue cognitive functions in the aging rat. Biomol. Ther. 13:467. doi: 10.3390/biom13030467
Lubec, J., Kalaba, P., Hussein, A. M., Feyissa, D. D., Kotob, M. H., Mahmmoud, R. R., et al. (2021). Reinstatement of synaptic plasticity in the aging brain through specific dopamine transporter inhibition. Mol. Psychiatry 26, 7076–7090. doi: 10.1038/s41380-021-01214-x
Lubec, J., Smidak, R., Malikovic, J., Feyissa, D. D., Korz, V., Hoger, H., et al. (2019). Dentate gyrus Peroxiredoxin 6 levels discriminate aged unimpaired from impaired rats in a spatial memory task. Front. Aging Neurosci. 11:198. doi: 10.3389/fnagi.2019.00198
Luo, A. H., Georges, F. E., and Aston-Jones, G. S. (2008). Novel neurons in ventral tegmental area fire selectively during the active phase of the diurnal cycle. Eur. J. Neurosci. 27, 408–422. doi: 10.1111/j.1460-9568.2007.05985.x
Martig, A. K., and Mizumori, S. J. (2011). Ventral tegmental area and substantia nigra neural correlates of spatial learning. Learn. Mem. 18, 260–271. doi: 10.1101/lm.1895211
McLachlan, R. S. (2009). A brief review of the anatomy and physiology of the limbic system. Can. J. Neurol. Sci. 36, S84–S87.
McNamara, C. G., Tejero-Cantero, A., Trouche, S., Campo-Urriza, N., and Dupret, D. (2014). Dopaminergic neurons promote hippocampal reactivation and spatial memory persistence. Nat. Neurosci. 17, 1658–1660. doi: 10.1038/nn.3843
Moore, S. J., and Murphy, G. G. (2020). The role of L-type calcium channels in neuronal excitability and aging. Neurobiol. Learn. Mem. 173:107230. doi: 10.1016/j.nlm.2020.107230
Mori, K., Togashi, H., Matsumoto, M., and Yoshioka, M. (2000). Deficits in nitric oxide production after tetanic stimulation are related to the reduction of long-term potentiation in Schaffer-CA1 synapses in aged Fischer 344 rats. Acta Physiol. Scand. 169, 79–85. doi: 10.1046/j.1365-201x.2000.00691.x
Nikoletopoulou, V., and Tavernarakis, N. (2012). Calcium homeostasis in aging neurons. Front. Genet. 3:200. doi: 10.3389/fgene.2012.00200
Nobili, A., Latagliata, E. C., Viscomi, M. T., Cavallucci, V., Cutuli, D., Giacovazzo, G., et al. (2017). Dopamine neuronal loss contributes to memory and reward dysfunction in a model of Alzheimer's disease. Nat. Commun. 8:14727. doi: 10.1038/ncomms14727
Norris, C. M., Korol, D. L., and Foster, T. C. (1996). Increased susceptibility to induction of long-term depression and long-term potentiation reversal during aging. J. Neurosci. 16, 5382–5392. doi: 10.1523/JNEUROSCI.16-17-05382.1996
Ntamati, N. R., and Luscher, C. (2016). VTA projection neurons releasing GABA and glutamate in the dentate gyrus. eNeuro 3, ENEURO.0137–ENEU16.2016. doi: 10.1523/ENEURO.0137-16.2016
Paxinos, G., and Watson, C. (2007). The rat brain in stereotaxic coordinates. Amsterdam; Boston: Academic Press/Elsevier.
Penner, M. R., and Mizumori, S. J. (2012). Age-associated changes in the hippocampal-ventral striatum-ventral tegmental loop that impact learning, prediction, and context discrimination. Front. Aging Neurosci. 4:22. doi: 10.3389/fnagi.2012.00022
Peoples, R. W., and Weight, F. F. (1994). Trichloroethanol potentiation of gamma-aminobutyric acid-activated chloride current in mouse hippocampal neurones. Br. J. Pharmacol. 113, 555–563. doi: 10.1111/j.1476-5381.1994.tb17025.x
Pereira, P. A., Coelho, J., Silva, A., and Madeira, M. D. (2021). Effects of aging on the cholinergic innervation of the rat ventral tegmental area: a stereological study. Exp. Gerontol. 148:111298. doi: 10.1016/j.exger.2021.111298
Pretsch, G., Sanadgol, N., Smidak, R., Lubec, J., Korz, V., Hoger, H., et al. (2020). Doublecortin and IGF-1R protein levels are reduced in spite of unchanged DNA methylation in the hippocampus of aged rats. Amino Acids 52, 543–553. doi: 10.1007/s00726-020-02834-3
Rao, Y. L., Ganaraja, B., Murlimanju, B. V., Joy, T., Krishnamurthy, A., and Agrawal, A. (2022). Hippocampus and its involvement in Alzheimer's disease: a review. Biotech 12:55. doi: 10.1007/s13205-022-03123-4
Romero-Barragan, M. T., Gruart, A., and Delgado-Garcia, J. M. (2021). Transsynaptic Long-term potentiation in the Hippocampus of behaving mice. Front Synaptic Neurosci 13:811806. doi: 10.3389/fnsyn.2021.811806
Rosen, Z. B., Cheung, S., and Siegelbaum, S. A. (2015). Midbrain dopamine neurons bidirectionally regulate CA3-CA1 synaptic drive. Nat. Neurosci. 18, 1763–1771. doi: 10.1038/nn.4152
Rowe, W. B., Spreekmeester, E., Meaney, M. J., Quirion, R., and Rochford, J. (1998). Reactivity to novelty in cognitively-impaired and cognitively-unimpaired aged rats and young rats. Neuroscience 83, 669–680. doi: 10.1016/s0306-4522(97)00464-8
Sagheddu, C., Cancedda, E., Bagheri, F., Kalaba, P., Muntoni, A. L., Lubec, J., et al. (2023). The atypical dopamine transporter inhibitor CE-158 enhances dopamine neurotransmission in the prefrontal cortex of male rats: a behavioral, electrophysiological and microdialysis study. Int. J. Neuropsychopharmacol. 26, 784–795. doi: 10.1093/ijnp/pyad056
Sagheddu, C., Pintori, N., Kalaba, P., Dragacevic, V., Piras, G., Lubec, J., et al. (2020). Neurophysiological and neurochemical effects of the putative cognitive enhancer (S)-CE-123 on Mesocorticolimbic dopamine system. Biomol. Ther. 10:779. doi: 10.3390/biom10050779
Scatton, B., Simon, H., Le Moal, M., and Bischoff, S. (1980). Origin of dopaminergic innervation of the rat hippocampal formation. Neurosci. Lett. 18, 125–131. doi: 10.1016/0304-3940(80)90314-6
Scheltens, P., De Strooper, B., Kivipelto, M., Holstege, H., Chetelat, G., Teunissen, C. E., et al. (2021). Alzheimer's disease. Lancet 397, 1577–1590. doi: 10.1016/S0140-6736(20)32205-4
Schulz, D., Huston, J. P., Jezek, K., Haas, H. L., Roth-Harer, A., Selbach, O., et al. (2002). Water maze performance, exploratory activity, inhibitory avoidance and hippocampal plasticity in aged superior and inferior learners. Eur. J. Neurosci. 16, 2175–2185. doi: 10.1046/j.1460-9568.2002.02282.x
Shetty, M. S., Sharma, M., and Sajikumar, S. (2017). Chelation of hippocampal zinc enhances long-term potentiation and synaptic tagging/capture in CA1 pyramidal neurons of aged rats: implications to aging and memory. Aging Cell 16, 136–148. doi: 10.1111/acel.12537
Skoog, I. (2011). Psychiatric disorders in the elderly. Can. J. Psychiatr. 56, 387–397. doi: 10.1177/070674371105600702
Spiegel, A. M., Koh, M. T., Vogt, N. M., Rapp, P. R., and Gallagher, M. (2013). Hilar interneuron vulnerability distinguishes aged rats with memory impairment. J. Comp. Neurol. 521, 3508–3523. doi: 10.1002/cne.23367
Spoleti, E., Krashia, P., La Barbera, L., Nobili, A., Lupascu, C. A., Giacalone, E., et al. (2022). Early derailment of firing properties in CA1 pyramidal cells of the ventral hippocampus in an Alzheimer's disease mouse model. Exp. Neurol. 350:113969. doi: 10.1016/j.expneurol.2021.113969
Spoleti, E., La Barbera, L., Cauzzi, E., De Paolis, M. L., Saba, L., Marino, R., et al. (2024). Dopamine neuron degeneration in the ventral tegmental area causes hippocampal hyperexcitability in experimental Alzheimer's disease. Mol. Psychiatry. doi: 10.1038/s41380-024-02408-9
Stanley, D. P., and Shetty, A. K. (2004). Aging in the rat hippocampus is associated with widespread reductions in the number of glutamate decarboxylase-67 positive interneurons but not interneuron degeneration. J. Neurochem. 89, 204–216. doi: 10.1111/j.1471-4159.2004.02318.x
Steffensen, S. C., Svingos, A. L., Pickel, V. M., and Henriksen, S. J. (1998). Electrophysiological characterization of GABAergic neurons in the ventral tegmental area. J. Neurosci. 18, 8003–8015. doi: 10.1523/JNEUROSCI.18-19-08003.1998
Sulzer, D., Joyce, M. P., Lin, L., Geldwert, D., Haber, S. N., Hattori, T., et al. (1998). Dopamine neurons make glutamatergic synapses in vitro. J. Neurosci. 18, 4588–4602. doi: 10.1523/JNEUROSCI.18-12-04588.1998
Swanson, L. W. (1982). The projections of the ventral tegmental area and adjacent regions: a combined fluorescent retrograde tracer and immunofluorescence study in the rat. Brain Res. Bull. 9, 321–353. doi: 10.1016/0361-9230(82)90145-9
Takeuchi, T., Duszkiewicz, A. J., Sonneborn, A., Spooner, P. A., Yamasaki, M., Watanabe, M., et al. (2016). Locus coeruleus and dopaminergic consolidation of everyday memory. Nature 537, 357–362. doi: 10.1038/nature19325
Tamano, H., Nishio, R., Shakushi, Y., Sasaki, M., Koike, Y., Osawa, M., et al. (2017). In vitro and in vivo physiology of low nanomolar concentrations of Zn(2+) in artificial cerebrospinal fluid. Sci. Rep. 7:42897. doi: 10.1038/srep42897
Tampi, R. R., Tampi, D. J., and Elson, A. (2022). Substance use disorders in the elderly. Psychiatr. Clin. North Am. 45, 707–716. doi: 10.1016/j.psc.2022.07.005
Tritsch, N. X., Ding, J. B., and Sabatini, B. L. (2012). Dopaminergic neurons inhibit striatal output through non-canonical release of GABA. Nature 490, 262–266. doi: 10.1038/nature11466
Tryon, V. L., Baker, P. M., Long, J. M., Rapp, P. R., and Mizumori, S. J. Y. (2020). Loss of sensitivity to rewards by dopamine neurons may underlie age-related increased probability discounting. Front. Aging Neurosci. 12:49. doi: 10.3389/fnagi.2020.00049
Tsetsenis, T., Badyna, J. K., Wilson, J. A., Zhang, X., Krizman, E. N., Subramaniyan, M., et al. (2021). Midbrain dopaminergic innervation of the hippocampus is sufficient to modulate formation of aversive memories. Proc. Natl. Acad. Sci. U. S. A. 118:e2111069118. doi: 10.1073/pnas.2111069118
Tsetsenis, T., Broussard, J. I., and Dani, J. A. (2022). Dopaminergic regulation of hippocampal plasticity, learning, and memory. Front. Behav. Neurosci. 16:1092420. doi: 10.3389/fnbeh.2022.1092420
Ungless, M. A., Magill, P. J., and Bolam, J. P. (2004). Uniform inhibition of dopamine neurons in the ventral tegmental area by aversive stimuli. Science 303, 2040–2042. doi: 10.1126/science.1093360
Uryash, A., Flores, V., Adams, J. A., Allen, P. D., and Lopez, J. R. (2020). Memory and learning deficits are associated with ca(2+) Dyshomeostasis in Normal aging. Front. Aging Neurosci. 12:224. doi: 10.3389/fnagi.2020.00224
Uttl, B., and Graf, P. (1993). Episodic spatial memory in adulthood. Psychol. Aging 8, 257–273. doi: 10.1037//0882-7974.8.2.257
Vela, J., Gutierrez, A., Vitorica, J., and Ruano, D. (2003). Rat hippocampal GABAergic molecular markers are differentially affected by ageing. J. Neurochem. 85, 368–377. doi: 10.1046/j.1471-4159.2003.01681.x
Verney, C., Baulac, M., Berger, B., Alvarez, C., Vigny, A., and Helle, K. B. (1985). Morphological evidence for a dopaminergic terminal field in the hippocampal formation of young and adult rat. Neuroscience 14, 1039–1052. doi: 10.1016/0306-4522(85)90275-1
Watson, J. B., Khorasani, H., Persson, A., Huang, K. P., Huang, F. L., and O'Dell, T. J. (2002). Age-related deficits in long-term potentiation are insensitive to hydrogen peroxide: coincidence with enhanced autophosphorylation of Ca2+/calmodulin-dependent protein kinase II. J. Neurosci. Res. 70, 298–308. doi: 10.1002/jnr.10427
Wittmann, B. C., Bunzeck, N., Dolan, R. J., and Duzel, E. (2007). Anticipation of novelty recruits reward system and hippocampus while promoting recollection. NeuroImage 38, 194–202. doi: 10.1016/j.neuroimage.2007.06.038
Keywords: dopamine, LTP, learning and memory, in vivo electrophysiology, VTA GABAergic and VTA glutamatergic neurons, dementia
Citation: Sagheddu C, Stojanovic T, Kouhnavardi S, Savchenko A, Hussein AM, Pistis M, Monje FJ, Plasenzotti R, Aufy M, Studenik CR, Lubec J and Lubec G (2024) Cognitive performance in aged rats is associated with differences in distinctive neuronal populations in the ventral tegmental area and altered synaptic plasticity in the hippocampus. Front. Aging Neurosci. 16:1357347. doi: 10.3389/fnagi.2024.1357347
Received: 17 December 2023; Accepted: 12 February 2024;
Published: 26 February 2024.
Edited by:
Touqeer Ahmed, National University of Sciences & Technology, PakistanReviewed by:
Jeffrey Long, National Institutes of Health (NIH), United StatesCopyright © 2024 Sagheddu, Stojanovic, Kouhnavardi, Savchenko, Hussein, Pistis, Monje, Plasenzotti, Aufy, Studenik, Lubec and Lubec. This is an open-access article distributed under the terms of the Creative Commons Attribution License (CC BY). The use, distribution or reproduction in other forums is permitted, provided the original author(s) and the copyright owner(s) are credited and that the original publication in this journal is cited, in accordance with accepted academic practice. No use, distribution or reproduction is permitted which does not comply with these terms.
*Correspondence: Gert Lubec, Z2VydC5sdWJlY0BsdWJlY2xhYi5jb20=
†These authors have contributed equally to this work and share first authorship
Disclaimer: All claims expressed in this article are solely those of the authors and do not necessarily represent those of their affiliated organizations, or those of the publisher, the editors and the reviewers. Any product that may be evaluated in this article or claim that may be made by its manufacturer is not guaranteed or endorsed by the publisher.
Research integrity at Frontiers
Learn more about the work of our research integrity team to safeguard the quality of each article we publish.