- 1Department of Ophthalmology, Sue Anschutz-Rodgers Eye Center, School of Medicine, Aurora, CO, United States
- 2Department of Pharmaceutical Sciences, Skaggs School of Pharmacy and Pharmaceutical Sciences, University of Colorado, Aurora, CO, United States
This review summarizes the latest findings on small heat shock proteins (sHsps) in three major retinal diseases: glaucoma, diabetic retinopathy, and age-related macular degeneration. A general description of the structure and major cellular functions of sHsps is provided in the introductory remarks. Their role in specific retinal diseases, highlighting their regulation, role in pathogenesis, and possible use as therapeutics, is discussed.
Introduction
The small heat shock proteins (sHsps) family comprises 11 members, namely, HspB1 (Hsp27), HspB2 (myotonic dystrophy kinase-binding protein), HspB3 (Hsp17), HspB4 (αA-crystallin), HspB5 (αB-crystallin), HspB6 (Hsp20), HspB7 (cardiovascular heat shock protein), HspB8 (Hsp22), HspB9 (cancer/testis antigen 51), HspB10 (outer dense fiber protein 1) and HspB11 (Hsp16.2) (Kappé et al., 2003; Schmidt et al., 2016; Zhu and Reiser, 2018). sHspB1, B2 (at low levels), B4, B5, B6, B8 and B11 have been identified in the mammalian retina (O’Reilly et al., 2010; Reddy et al., 2013; Schmidt et al., 2016). sHsps are ATP-independent molecular chaperones (Zhu and Reiser, 2018). sHsps are categorized into two classes (Taylor and Benjamin, 2005). Class I sHsps (HspB1, B5, B6, and B8) are widely distributed in various tissues. However, Class II sHsps (HspB2, 3, 4, 7, 9, and 10) exhibit tissue-specific expression. The molecular masses of the sHsp subunits range between 12 and 43 kDa HspB1, B4, B5, and B11 can form large oligomeric species capable of subunit exchange, which is accelerated under various stress conditions. sHsps have a highly conserved, 80–100 amino acid α-crystallin core domain (ACD), with the notable exception of HspB11 containing the least conserved ACD-like region (Kappé et al., 2010; Bartelt-Kirbach and Golenhofen, 2014). This ACD is flanked by a less conserved N-terminal domain and a C-terminal extension (CTE) (Kriehuber et al., 2010). The CTEs of several sHsps contain an IXI/V motif that contains hydrophobic residues, typically isoleucine or valine, separated by a single residue (Baughman et al., 2020).
sHsps interact with partially unfolded proteins through their surface-exposed hydrophobic residues and prevent protein aggregation (Sharma et al., 2000; Jaya et al., 2009). This property is essential in preventing neurodegenerative and neuromuscular diseases caused by protein aggregation (Carra et al., 2013). The anti-aggregation activity of sHsps can be determined in vitro by their ability to inhibit thermal- or chemical-mediated aggregation of client proteins. A comparative analysis has shown that HspB1, B4 and B5 are most effective, B2 and B3 are intermediately effective, and B6, B7, and B8 are moderately effective in their chaperone-like function (Mymrikov et al., 2017).
HspB1, B3, B4 and B5 bind to many heat-sensitive proteins in cells when subjected to thermal stress (Mymrikov et al., 2017). Bcl-2-associated anthanogene (BAG3) is a linker protein of sHsps with large heat shock proteins (Boelens, 2020). BAG3 can interact with HspB2, B5, B6 and B8 and facilitate the removal of misfolded proteins through autophagy (Rusmini et al., 2017; Boelens, 2020). Apart from this, sHsps can also interact with cellular proteins under normal conditions. HspB1, B4, B5 and B6 interact with cytoskeletal proteins such as actin, tubulin, vimentin, nestin, and desmin to maintain the integrity of the cytoskeletal architecture (Mymrikov et al., 2011; Wettstein et al., 2012). In addition, sHsps play an essential regulatory role in maintaining protein homeostasis; by interacting with early misfolded proteins and facilitating their refolding or degradation (mediated by chaperones and co-factors), thereby preventing cell damage (Westerheide and Morimoto, 2005; Webster et al., 2019).
sHsps are also regulators of apoptosis (Takayama et al., 2003; Nagaraj et al., 2016). Several studies have shown that sHsps bind to procaspase-3 and Bax and block apoptosis in cells under various stress conditions (Pasupuleti et al., 2010; Nahomi et al., 2013a; Bakthisaran et al., 2015). sHsps also block TRAIL-, TNF-α- and Fas-induced apoptosis across multiple cell types (Mehlen et al., 1995; Kamradt et al., 2005). Furthermore, sHsps can inhibit apoptosis by activating the cell survival pathway mediated by PI3-K/Akt/PDK1 (Takayama et al., 2003; Pasupuleti et al., 2010).
Previous studies have shown that sHsps are anti-inflammatory proteins. Overexpression of HspB1 ameliorates neuroinflammation caused by ethanol-induced brain injury in mice (Dukay et al., 2021). HspB5 reduces inflammation by decreasing the secretion of proinflammatory cytokines in animal models of multiple sclerosis, ischemia, or nerve crush injury (Kurnellas et al., 2012; Rothbard et al., 2012; Lim et al., 2021). Furthermore, HspB5 protects intestinal mucosa from inflammation in an experimental model in mice (Xu et al., 2019). It also shows anti-inflammatory and otoprotective effects in experimental pneumococcal meningitis (Erni et al., 2019), modulation of the inflammatory response in a mouse model of acute spinal cord injury (Klopstein et al., 2012), and reduction of paralytic symptoms in a mouse model of experimental autoimmune encephalomyelitis through modulation of inflammatory cytokines (Rothbard et al., 2012). Administration of HspB4 showed a reduction in Th1 cytokines in the retina of a mouse model of experimental autoimmune uveitis (Rao et al., 2012).
Apart from the above functions, sHsps are also involved in other cellular processes. HspB1, B5, and B6 have been shown to regulate angiogenesis (Dimberg et al., 2008; Lee et al., 2012; Zhang et al., 2012; Tao et al., 2019), associated with tumor progression and neovascularization in retinal diseases. Several reports are available about the promotion of epithelial to mesenchymal transition in various cells/tissues by HspB1 and B5, which is a phenomenon that supports malignant cell metastasis (Shiota et al., 2013; Cordonnier et al., 2015; Shi et al., 2017a; Li et al., 2017; Chen et al., 2018a; Han et al., 2018; Maksimiuk et al., 2020).
sHsps undergo phosphorylation and other posttranslational modifications in cells. Phosphorylation is mediated through protein kinase A, p38 MAP and MAPKAP kinases (Bakthisaran et al., 2016; Larsen et al., 1997; Edwards et al., 2012), also reviewed in (Bakthisaran et al., 2015). Phosphorylation produces a negative charge, inducing a shift from the oligomeric state to smaller species, as observed in HspB1 and HspB5. The phosphorylation sites identified in sHsps are as follows: HspB1 (serine 15, 78, and 82), HspB4 (serine 45, 122, and serine/threonine 148), HspB5 (serine 19, 45, and 59), HspB6 (serine 16), and HspB8 (serine 24 and threonine 87) (Bakthisaran et al., 2016; Larsen et al., 1997; Shemetov et al., 2011; Edwards et al., 2012; Boelens, 2020; Nath et al., 2021). Phosphorylation of sHsps affects their oligomeric structure and chaperone-like activity. Phosphorylation has been considered a gain-of-function modification in sHsps (Koteiche and McHaourab, 2003; Ecroyd et al., 2007; Ahmad et al., 2008; Jovcevski et al., 2015; Freilich et al., 2018), but there are studies showing conflicting results (Rogalla et al., 1999; Ito et al., 2001; Lelj-Garolla and Mauk, 2006; Shemetov et al., 2008; Mellier et al., 2013). For example, while some studies showed HspB1 phosphomimics (serine residues mutated to aspartic acid) promote the dissociation of oligomers and enhance its chaperone-like activity (Jovcevski et al., 2015; Freilich et al., 2018), other studies showed the opposite effect on the chaperone-like activity (Rogalla et al., 1999; Lelj-Garolla and Mauk, 2006; Mellier et al., 2013). In one study, HspB5 phosphomimics showed more efficient chaperone-like activity against an amyloid-forming protein but reduced activity against another (Ecroyd et al., 2007), suggesting client-specific effects. In another study, a phosphorylation mimic of HspB5 showed a reduced oligomeric size and decreased chaperone-like activity (Ito et al., 2001).
In addition to phosphorylation, other modifications affect the functions of sHsps. HspB1 has been shown to undergo sumoylation, which affects its influence on cell proliferation (Ge et al., 2017). Another modification is glycation. Glycation is a major modification of the human lens proteins HspB4 and HspB5 (Swamy et al., 1992; Cherian and Abraham, 1995), and this modification can either improve or reduce the function of these proteins, depending on which carbonyl compound initiates the glycation (Nagaraj et al., 2012a). Various acyl modifications, such as acetylation, succinylation, malonylation, and propionylation, have also been reported in the human lenses proteins HspB4 and HspB5 (Nagaraj et al., 2012b; Nahomi et al., 2013b; Nandi et al., 2019a; Nahomi et al., 2020). These modifications (acetylation and succinylation) have also been shown to improve the chaperone-like activity of HspB5 (Nahomi et al., 2013a; Nahomi et al., 2013b; Nandi et al., 2019a; Nandi et al., 2019b).
Together, these observations suggest that sHsps are important proteins involved in maintaining cellular homeostasis and that they undergo posttranslational modifications that affect their cellular functions. In the following section, we will review the role of sHsps in three major retinal diseases.
sHsps in Glaucoma
Immunostaining experiments have demonstrated an upregulation of HspB1 in retinal ganglion cells (RGCs), retinal vessels, and the optic nerve head of primary open-angle glaucoma (POAG) and normal-tension glaucoma patients (Tezel et al., 2000). Studies on nonhuman primate models of glaucoma have shown elevated levels of HspB1 in RGCs and optic nerve fibers (Sakai et al., 2003). Rats with elevated intraocular pressure (IOP) showed increased expression of HspB1 (Huang et al., 2007; Kalesnykas et al., 2007; Windisch et al., 2009; O’Reilly et al., 2010; Chidlow et al., 2014) and phosphorylated HspB1 (Huang et al., 2007) in the nerve fiber layer (NFL), RGCs, and glial cells. In some studies, dynamic expression of HspB4 and B5 was observed in a rat glaucoma model, with initial downregulation followed by upregulation (Steele et al., 2006; Miyara et al., 2008; Guo et al., 2010; Piri et al., 2013; Thanos et al., 2014; Park et al., 2019). Ischemic injury mediated by optic nerve crush or axotomy and steroid-induced ocular hypertension in rats decreased the expression of HspB4 and HspB5 in the RGC layer (Munemasa et al., 2009).
In contrast to HspB1, transcriptional downregulation of HspB5 has been reported in the trabecular meshwork of postmortem glaucomatous human eyes (Comes and Borrás, 2009). Stankowska et al. observed a reduction in HspB5 in human glaucomatous retinas (Stankowska et al., 2019). In rats, episcleral vein cauterization leading to an elevation of IOP over a 7-week period showed a positive correlation between upregulation of HspB4 and HspB5 and decreased loss of RGCs (Anders et al., 2018). The effects of these changes in sHsp levels on the pathogenesis of glaucoma need further studies.
Recent evidence suggests that an immune-mediated response to sHsps contributes to further glaucomatous cell damage. Immunization of Lewis rats with HspB1 caused reductions in RGC number and axonal density (Wax et al., 2008). It has been observed that the titers of autoantibodies against HspB4, B5, and B1 in sera (Tezel et al., 1998; Wax et al., 1998; Wax et al., 2001; Boehm et al., 2012) and against HspB5 in the aqueous humor of normal pressure glaucoma patients are elevated (Joachim et al., 2007). Since serum levels of these antibodies are not correlated with the degree of neuronal cell loss, it is unlikely that an immune response to sHsps arises as a secondary effect following increasing RGC death (Wax et al., 2001). Furthermore, exogenously applied antibodies against HspB4 and HspB5 or HspB1 to human RGC cultures at concentrations similar to those found in glaucoma patients caused apoptotic cell death (Tezel et al., 1998; Tezel and Wax, 2000). In rats, HspB1 injected intraperitoneally led to an upregulation of anti-HspB1 in sera and was correlated with a reduced RGC density after 6 weeks (Joachim et al., 2009). Given that the IOP did not vary in this experiment, the anti-HspB1 antibodies were the likely initiators of the observed RGC loss (Joachim et al., 2009). This is consistent with the finding that inoculation of rat retinal cells in vivo with HspB1 antibodies resulted in RGC loss and decreased axon density in the optic nerve 1–4 months later. These results suggested a pathogenic role for sHsp antibodies in glaucomatous cell damage.
There are many reports suggesting that exogenously administered sHsps can be beneficial in preventing cellular damage in glaucoma. Intravenous delivery of HspB5 reduced RGC loss after optic nerve injury at the highest concentration tested and inhibited microglial activation at all concentrations for up to 2 weeks (Wu et al., 2014). Further support for a therapeutic role for HspB5 comes from in vivo overexpression of HspB5 in transfected RGCs, which protected against RGC loss for 14 days after injury of optic nerves (Munemasa et al., 2009). Intravitreal injection of HspB5 2 weeks after induction of elevated IOP by episcleral vein cauterization mitigated the loss of rat RGCs and retinal nerve fiber layer thickness (Anders et al., 2017). In ischemic models, intravitreal application of HspB5 improved RGC survival 4 days after acute ocular hypertension (Wu et al., 2012). Intravitreal application of HspB5 immediately after ischemic reperfusion (I/R) injury decreased the RGC loss and retinal thinning 24 h, 1 week, and 1 month later (Yan et al., 2017). Systemic administration of a core peptide of HspB5 in ischemic hypoxic conditions prevented RGC loss in rat retinal explants ex vivo and in vivo. It inhibited RGC axons and soma degeneration in rodent models of glaucoma (Stankowska et al., 2019). Additionally, the neuroprotective effect of HspB5 extends to other cell types. One study showed that anterior ischemic optic neuropathy induces expression of HspB5 at the optic nerve head (Pangratz-Fuehrer et al., 2011); in this study, intravitreally injected HspB5 for 3 days reduced microglial and astrocyte activation. In addition, intravenous administration of HspB5 every other day for 3 weeks led to the full rescue of oligodendrcytes, which are responsible for the myelination of axons (Pangratz-Fuehrer et al., 2011).
HspB1 is another potential therapeutic target due to its protective role against neurodegeneration. Ischemic retinal preconditioning produced selective upregulation of HspB1 in RGCs and inner retinal layers, which was positively correlated with neuroprotective ischemic tolerance in retinal cells for 72 h (Li et al., 2003). In rats with I/R injury, intravitreal delivery followed by electroporation of HspB1 protected apoptosis of RGCs, supporting the neuroprotective effects of HspB1 (Yokoyama et al., 2001). In optic nerve axotomized rats, induction of HspB1 following intravitreal delivery of simvastatin mitigated RGC apoptosis. The application of quercetin, an inhibitor of HspB1 expression, reversed this effect on RGC survival (Kretz et al., 2006). Other studies showed that optic nerve axotomy induced HspB1 expression in a subset of RGCs; the surviving RGCs showed significantly increased HspB1 expression, indicating a protective effect against apoptosis (Krueger-Naug et al., 2002; Krueger-Naug et al., 2003). Additional evidence for the utility of HspB1 comes from research into axonal regeneration. After transected optic nerves were exposed to peripheral nerve autografts, effective axonogenesis in mature RGCs was strongly correlated with the degree of HspB1 expression (Hebb et al., 2006).
Despite showing beneficial effects in glaucoma, HspB1 has also been claimed to induce the pathogenesis of glaucoma. A previous study showed that intravitreal injection of HspB1 in rats resulted in neuronal and optic nerve damages after 21 days (Grotegut et al., 2020). More recently, it was reported that such damages to the retina occurred via the activation of apoptotic pathways through the activation of NF-κB, and induction of immune response (Grotegut et al., 2021). Furthermore, it is shown that a potential mechanism of HspB1 mediated neurodegeneration is through activation of T cells. A transient 3-week elevation of IOP by microbead injection-induced CD4+ T cell migration into the retinal ganglion cell layer (GCL) followed by degeneration of the RGCs and axons up to 8 weeks later. These T cells were shown to be HspB1 specific by cell sorting analysis. Since the retina is immune privileged, one possible explanation for this response is that memory T cells specific to bacterial sHsps gain access to the retina from a compromised retinal blood barrier and are activated by local sHsps. Mice raised without commensal gut microflora did not show these T cell responses or subsequent glaucomatous neural damage, suggesting a role for sensitization of T cells by the gut microbiome in the glaucoma pathogenesis (Chen et al., 2018b). These observations indicate that sHsps play a role in the pathogenesis of glaucoma. The seemingly contrasting effects of HspB1 on RGCs should be investigated further.
sHsps in Diabetic Retinopathy
HspB4 and HspB5 are expressed in the inner and outer nuclear layers in rodent and human retinas, specifically in RGCs, photoreceptors, astrocytes, and Müller cells (Xi et al., 2003; Fort et al., 2009; Heise and Fort, 2011; Kannan et al., 2016; Ruebsam et al., 2018). Several studies have shown that both HspB4 and HspB5 are upregulated in the retinas of rats with streptozotocin-induced diabetes (Heise et al., 2013). Whether such upregulation has a protective role in the retina has yet to be determined. Murine posterior eye cups exposed to advanced glycation end product-modified proteins showed increased expression of HspB4 but decreased expression of HspB5 (Kase et al., 2011). While retinal HspB4 and HspB5 have neuroprotective roles and support retinal neuronal cell survival, diabetes appears to compromise those effects in rats (Losiewicz and Fort, 2011). This could be because diabetes strongly reduces the chaperone-like function of these sHsps by reducing their solubility and disrupting their interactions with Bax (Losiewicz and Fort, 2011). Another study reported that diabetes compromises the solubility of these proteins in the retina (Reddy et al., 2013), which could have additional negative consequences on their function.
It has been shown that phosphorylation of HspB4 at serine 148 (mice) and threonine 148 (human) is dramatically reduced in diabetic retinopathy, which could increase stress and apoptosis in retinal cells (Ruebsam et al., 2018). HspB4 is highly expressed in Müller glia and protects retinal neurons via a paracrine mechanism (Nath et al., 2022). Further, it has been shown that phosphorylation of threonine 148 in HspB4 enhances its cytoprotective function, which could reduce inflammation in the diabetic retina (Nath et al., 2021). A study using retinas from three different diabetic rat strains showed increased phosphorylation on all three residues (serine 19, 45 and 59) of HspB5 (Heise et al., 2013). In human proliferative diabetic retinopathy, endothelial cells in the epiretinal membranes showed strong immunoreactivity for phosphorylated serine 59 in HspB5 compared to the nondiabetic retina (Dong et al., 2016). Additional studies are required to fully understand the specific role of phosphorylation of HspB4 and HspB5 in the pathogenesis of diabetic retinopathy.
One study reported that intravitreally injected adenovirus encoding HspB4 resulted in reduced vascular leakage and pericyte loss in diabetic mice (Kim et al., 2012). Thus, HspB4 could be essential in inhibiting diabetic retinal pericyte loss and, overall, preventing the pathogenesis of diabetic retinopathy. The levels of HspB1 are decreased in the rat diabetic retina, despite an elevation of its mRNA levels (Reddy et al., 2013), suggesting either enhanced degradation or chemical modification. However, one study in mice (after 10 weeks of streptozotocin-induced diabetes) reported a significant increase in HspB1 mRNA and protein levels in the retina (Pinach et al., 2013). Retinal capillary endothelial cells serve as regulators of vascular wall tension and angiogenesis (Mrugacz et al., 2021), and HspB1 has been shown to play a vital role in maintaining the integrity of these cells (Batulan et al., 2016; Shi et al., 2017b; Rada et al., 2021). However, proinflammatory cytokines that are generally upregulated in the diabetic retina (Joussen et al., 2004; Abu El-Asrar, 2012; Wang et al., 2018) reduce the levels of HspB1 in retinal capillary endothelial cells, which could lead to their apoptosis in diabetic retinopathy (Nahomi et al., 2014). Interestingly, it has also been shown that in glial cells of diabetic rats, HspB1 is upregulated to induce vasoprotective mechanisms to preserve the inner nuclear layer of the retina (Feng et al., 2014). Apart from these sHsps, one study on the diabetic rat retina showed a significant reduction in HspB6, both at the mRNA and protein levels (Reddy et al., 2013), and whether that has any effect on the retina needs to be studied.
Angiogenic growth factors, including vascular endothelial growth factor (VEGF) and platelet-derived growth factor (PDGF), have a role in the proliferative phase of diabetic retinopathy. Angiogenesis can have structural and physiological effects on capillaries and render them dysfunctional. One study found a significant increase in HspB5 in the vitreous fluid of patients with proliferative diabetic retinopathy compared to the nondiabetic control group (Chen et al., 2017). Oxygen-induced retinopathy (OIR) in mice has been widely used as a model for angiogenesis in proliferative diabetic retinopathy. A study on this model reported upregulation of HspB4 and HspB5 (Vähätupa et al., 2018). It has also been observed that under hypoxic conditions, HspB5 is phosphorylated at serine 59 (Ito et al., 1997). Under these conditions, VEGF can stimulate the phosphorylation and functions of HspB5 in capillary endothelial cells (Dimberg et al., 2008). It has been observed that phosphorylated HspB5 can promote VEGF folding and secretion under hypoxic conditions, and in the absence of HspB5, misfolded, monoubiquitinated VEGF may be exported to the cytoplasm, where it is degraded (Kase et al., 2010). Thus, sHsps could play a pivotal role in vascular homoeostasis and retinal angiogenesis in diabetic retinopathy. Furthermore, downregulation of HspB5 in the diabetic retina has been proposed to increase oxidative stress in retinal pigment epithelial cells (Wu et al., 2022). Altogether, the above studies suggest that sHsps play an important role in the pathogenesis of diabetic retinopathy.
sHsps in Age-Related Macular Degeneration
The pathogenesis of AMD begins with the thickening of Bruch’s membrane due to lipid and protein accumulation forming subretinal deposits, known as drusen. Drusen interferes with fluid efflux from retinal pigment epithelial cells (RPEs) across Bruch’s membrane, leading to increased stress on RPEs. This increased cellular stress leads to proinflammatory signaling combined with an increase in drusen deposition (Bowes Rickman et al., 2013). HspB4 and HspB5 are concentrated in the drusen of macaque monkeys (Umeda et al., 2005), possibly to reduce oxidative damage to RPEs. HspB4 and HspB5 are present in the inner and outer nuclear layers of the retina and RPE (Xi et al., 2003). HspB4 and HspB5 are expressed in the cytosol and mitochondria of RPE cells, and they protect RPEs against oxidative stress and ER stress-induced by ischemic injury and autophagy (Alge et al., 2002).
Since HspB5 is a modulator of angiogenesis by aiding in VEGF folding and secretion (Kase et al., 2010), it might promote choroidal neovascularization in neovascular AMD. In support of this notion is the study by Kase et al. demonstrating that VEGF-A expression is low in HspB5−/− mouse retinas relative to the wild-type, and that laser-induced choroidal neovascularization is reduced in the absence of HspB5 (Kase et al., 2010).
HspB1 has also been detected in the nerve fiber layer, ganglion cell layer and photoreceptors of the retina (Tezel et al., 2000). Oxidative stress in ARPE-19 cells increases HspB1 expression within membrane blebs (Strunnikova et al., 2001). These membrane blebs are inflammatory injury-induced and cause caspase activation, nuclear fragmentation and apoptosis (Wolf and Green, 1999). Animal studies using RPE isolated from mice and human donors and studies applying hydroquinone as an oxidative agent demonstrated that increased oxidative stress on RPE increased the levels of HspB1 phosphorylation and activation (Pons et al., 2010). Another study showed that hydroquinone-induced nonlethal oxidative injury led to HSPB1 gene transcription and dimer formation and phosphorylation of HspB1 in ARPE-19 cells (Pons et al., 2010). Pons et al. also found that p38 and extracellular signal-regulated kinase (ERK) mediated hydroquinone-induced HspB1 phosphorylation and actin aggregation, revealing ERK to be an upstream regulator of HspB1 (Pons et al., 2010). In addition, it has been shown that HspB5 plays a vital role in subretinal fibrosis associated with neovascular AMD (Ishikawa et al., 2016).
Mini chaperone peptides derived from HspB5 prevent oxidant-induced cell death of RPEs and have therapeutic potential through the binding of proinflammatory mediators (Kannan et al., 2016). The study by Kannan et al. used polycaprolactone nanoparticles loaded with a mini chaperone peptide from either the HspB4 or B5 core domain and demonstrated that this protected primary RPE cells from oxidative stress and was approximately 4-fold more effective than nonencapsulated HspB5 mini chaperone peptide at the same doses (Kannan et al., 2016). Recently, Kannan’s laboratory observed that oxidative stress induces senescence in RPE cells, and a mini chaperone peptide of HspB5 inhibits such senescence (Sreekumar et al., 2022). In addition, Wang et al. demonstrated that recombination of HspB5 with a protein polymer fused with soluble peptide S96 and deblock copolymer SI protected RPE cells from apoptosis induced by chemical stress from H2O2 (Wang et al., 2014) and its intravitreal injection attenuated NaIO3-induced retinal degeneration in mice (Sreekumar et al., 2018). Another study demonstrated that retinal damage was reduced after administration an HspB5 peptide in a NaIO3-induced retinal damage/degeneration model, and ARPE-19 cells (Raju et al., 2016). Together, these observations suggest that sHsps play a protective role in AMD. Further work is required to fully understand at the molecular level how sHsps help maintain homeostasis in the retina and RPE cells and how alterations in their levels affect the pathogenesis of AMD.
Concluding Remarks
The accumulated evidence confirms that sHsps exhibit molecular chaperone-like functions by interacting with partially unfolded proteins, thereby preventing protein aggregation under stress conditions. In addition, sHsps interfere with apoptosis under various stress conditions at several stages in the intrinsic and extrinsic apoptotic pathways. These properties appear to be important in preventing neurodegeneration and vascular abnormalities in the retina. Moreover, the retina is prone to produce high levels of photooxidative radicals due to its high metabolic activity and exposure to light. Therefore, sHsps might be crucial in protecting retinal cells and RPEs from oxidative damage. sHsps might be useful as therapeutics in retinal diseases due to their neurovascular protective abilities. In addition, recent studies have shown beneficial effects of intraocularly delivered sHsps/sHsp peptides in glaucoma and neovascular AMD. However, sHsps could be pathogenic as well. HspB1 can promote VEGF folding and secretion and thus could promote angiogenesis in proliferative diabetic retinopathy and neovascular AMD. Moreover, HspB1 has been shown to promote RGC death in animal models of glaucoma (Figure 1). Therefore, a better understanding of the molecular mechanisms of sHsp functions in specific cell types of the retina and the impact of disease conditions on their functions is necessary to appreciate their role in retinal diseases fully.
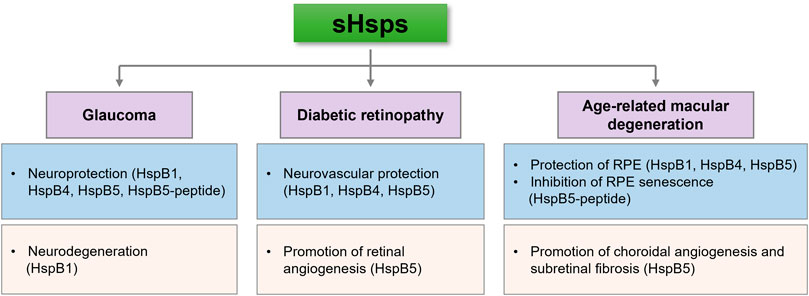
FIGURE 1. sHsps can inhibit or contribute to the pathogenesis of retinal diseases. This seemingly contradictory ability might depend on their cell type expression, response to stress and posttranslational modifications.
Author Contributions
VR, JW, DY, RN, MN, JR and RN wrote the draft. All authors have reviewed and approved the final manuscript.
Conflict of Interest
The authors declare that the research was conducted in the absence of any commercial or financial relationships that could be construed as a potential conflict of interest.
Publisher’s Note
All claims expressed in this article are solely those of the authors and do not necessarily represent those of their affiliated organizations, or those of the publisher, the editors and the reviewers. Any product that may be evaluated in this article, or claim that may be made by its manufacturer, is not guaranteed or endorsed by the publisher.
Acknowledgments
We thank the Research to Prevent Blindness, NY for an unrestricted grant to the Department of Ophthalmology, University of Colorado.
References
Abu El-Asrar, A. (2012). Role of Inflammation in the Pathogenesis of Diabetic Retinopathy. Middle East. Afr. J. Ophthalmol. 19 (1), 70–74. doi:10.4103/0974-9233.92118
Ahmad, M. F., Raman, B., Ramakrishna, T., and Rao, C. M. (2008). Effect of Phosphorylation on αB-crystallin: Differences in Stability, Subunit Exchange and Chaperone Activity of Homo and Mixed Oligomers of αB-Crystallin and its Phosphorylation-Mimicking Mutant. J. Mol. Biol. 375 (4), 1040–1051. doi:10.1016/j.jmb.2007.11.019
Alge, C. S., Priglinger, S. G., Neubauer, A. S., Kampik, A., Zillig, M., Bloemendal, H., et al. (2002). Retinal Pigment Epithelium Is Protected against Apoptosis by alphaB-Crystallin. Invest. Ophthalmol. Vis. Sci. 43 (11), 3575–3582.
Anders, F., Liu, A., Mann, C., Teister, J., Lauzi, J., Thanos, S., et al. (2017). The Small Heat Shock Protein α-Crystallin B Shows Neuroprotective Properties in a Glaucoma Animal Model. Ijms 18 (11), 2418. doi:10.3390/ijms18112418
Anders, F., Mann, C., Liu, A., Teister, J., Funke, S., Thanos, S., et al. (2018). Correlation of Crystallin Expression and RGC Susceptibility in Experimental Glaucoma Rats of Different Ages. Curr. Eye Res. 43 (10), 1267–1273. doi:10.1080/02713683.2018.1485950
Bakthisaran, R., Akula, K. K., Tangirala, R., and Rao, C. M. (2016). Phosphorylation of αB-crystallin: Role in Stress, Aging and Patho-Physiological Conditions. Biochim. Biophys. Acta (Bba) - Gen. Subjects 1860 (1 Pt B), 167–182. doi:10.1016/j.bbagen.2015.09.017
Bakthisaran, R., Tangirala, R., and Rao, C. M. (2015). Small Heat Shock Proteins: Role in Cellular Functions and Pathology. Biochim. Biophys. Acta (Bba) - Proteins Proteomics 1854 (4), 291–319. doi:10.1016/j.bbapap.2014.12.019
Bartelt-Kirbach, B., and Golenhofen, N. (2014). Reaction of Small Heat-Shock Proteins to Different Kinds of Cellular Stress in Cultured Rat Hippocampal Neurons. Cell Stress and Chaperones 19 (1), 145–153. doi:10.1007/s12192-013-0452-9
Batulan, Z., Pulakazhi Venu, V. K., Li, Y., Koumbadinga, G., Alvarez-Olmedo, D. G., Shi, C., et al. (2016). Extracellular Release and Signaling by Heat Shock Protein 27: Role in Modifying Vascular Inflammation. Front. Immunol. 7, 285. doi:10.3389/fimmu.2016.00285
Baughman, H. E. R., Pham, T.-H. T., Adams, C. S., Nath, A., and Klevit, R. E. (2020). Release of a Disordered Domain Enhances HspB1 Chaperone Activity toward Tau. Proc. Natl. Acad. Sci. U.S.A. 117 (6), 2923–2929. doi:10.1073/pnas.1915099117
Boehm, N., Wolters, D., Thiel, U., Lossbrand, U., Wiegel, N., Pfeiffer, N., et al. (2012). New Insights into Autoantibody Profiles from Immune Privileged Sites in the Eye: a Glaucoma Study. Brain Behav. Immun. 26 (1), 96–102. doi:10.1016/j.bbi.2011.07.241
Boelens, W. C. (2020). Structural Aspects of the Human Small Heat Shock Proteins Related to Their Functional Activities. Cell Stress and Chaperones 25 (4), 581–591. doi:10.1007/s12192-020-01093-1
Bowes Rickman, C., Farsiu, S., Toth, C. A., and Klingeborn, M. (2013). Dry Age-Related Macular Degeneration: Mechanisms, Therapeutic Targets, and Imaging. Invest. Ophthalmol. Vis. Sci. 54 (14), ORSF68–80. doi:10.1167/iovs.13-12757
Carra, S., Rusmini, P., Crippa, V., Giorgetti, E., Boncoraglio, A., Cristofani, R., et al. (2013). Different Anti-aggregation and Pro-degradative Functions of the Members of the Mammalian sHSP Family in Neurological Disorders. Phil. Trans. R. Soc. B 368 (1617), 20110409. doi:10.1098/rstb.2011.0409
Chen, D., Cao, G., Qiao, C., Liu, G., Zhou, H., and Liu, Q. (2018). Alpha B‐crystallin Promotes the Invasion and Metastasis of Gastric Cancer via NF‐κB‐induced Epithelial‐mesenchymal Transition. J. Cell. Mol. Medi 22 (6), 3215–3222. doi:10.1111/jcmm.13602
Chen, H., Cho, K.-S., Vu, T. H. K., Shen, C.-H., Kaur, M., Chen, G., et al. (2018). Commensal Microflora-Induced T Cell Responses Mediate Progressive Neurodegeneration in Glaucoma. Nat. Commun. 9 (1), 3209. doi:10.1038/s41467-018-05681-9
Chen, W., Lu, Q., Lu, L., and Guan, H. (2017). Increased Levels of alphaB-Crystallin in Vitreous Fluid of Patients with Proliferative Diabetic Retinopathy and Correlation with Vascular Endothelial Growth Factor. Clin. Exp. Ophthalmol. 45 (4), 379–384. doi:10.1111/ceo.12891
Cherian, M., and Abraham, E. C. (1995). Decreased Molecular Chaperone Property of α-Crystallins Due to Posttranslational Modifications. Biochem. Biophysical Res. Commun. 208 (2), 675–679. doi:10.1006/bbrc.1995.1391
Chidlow, G., Wood, J. P. M., and Casson, R. J. (2014). Expression of Inducible Heat Shock Proteins Hsp27 and Hsp70 in the Visual Pathway of Rats Subjected to Various Models of Retinal Ganglion Cell Injury. PLoS One 9 (12), e114838. doi:10.1371/journal.pone.0114838
Comes, N., and Borrás, T. (2009). Individual Molecular Response to Elevated Intraocular Pressure in Perfused Postmortem Human Eyes. Physiol. Genomics 38 (2), 205–225. doi:10.1152/physiolgenomics.90261.2008
Cordonnier, T., Bishop, J. L., Shiota, M., Nip, K. M., Thaper, D., Vahid, S., et al. (2015). Hsp27 Regulates EGF/β-catenin Mediated Epithelial to Mesenchymal Transition in Prostate Cancer. Int. J. Cancer 136 (6), E496–E507. doi:10.1002/ijc.29122
Dimberg, A., Rylova, S., Dieterich, L. C., Olsson, A.-K., Schiller, P., Wikner, C., et al. (2008). αB-crystallin Promotes Tumor Angiogenesis by Increasing Vascular Survival during Tube Morphogenesis. Blood 111 (4), 2015–2023. doi:10.1182/blood-2007-04-087841
Dong, Y., Dong, Z., Kase, S., Ando, R., Fukuhara, J., Kinoshita, S., et al. (2016). Phosphorylation of alphaB-Crystallin in Epiretinal Membrane of Human Proliferative Diabetic Retinopathy. Int. J. Ophthalmol. 9 (8), 1100–1105. doi:10.18240/ijo.2016.08.03
Dukay, B., Walter, F. R., Vigh, J. P., Barabási, B., Hajdu, P., Balassa, T., et al. (2021). Neuroinflammatory Processes Are Augmented in Mice Overexpressing Human Heat-Shock Protein B1 Following Ethanol-Induced Brain Injury. J. Neuroinflammation 18 (1), 22. doi:10.1186/s12974-020-02070-2
Ecroyd, H., Meehan, S., Horwitz, J., Aquilina, J. A., Benesch, J. L. P., Robinson, C. V., et al. (2007). Mimicking Phosphorylation of αB-crystallin Affects its Chaperone Activity. Biochem. J. 401 (1), 129–141. doi:10.1042/BJ20060981
Edwards, H. V., Scott, J. D., and Baillie, G. S. (2012). PKA Phosphorylation of the Small Heat-Shock Protein Hsp20 Enhances its Cardioprotective Effects. Biochem. Soc. Trans. 40 (1), 210–214. doi:10.1042/BST20110673
Erni, S. T., Fernandes, G., Buri, M., Perny, M., Rutten, R. J., van Noort, J. M., et al. (2019). Anti-inflammatory and Oto-Protective Effect of the Small Heat Shock Protein Alpha B-Crystallin (HspB5) in Experimental Pneumococcal Meningitis. Front. Neurol. 10, 570. doi:10.3389/fneur.2019.00570
Feng, Y., Wang, Y., Yang, Z., Wu, L., Hoffmann, S., Wieland, T., et al. (2014). Chronic Hyperglycemia Inhibits Vasoregression in a Transgenic Model of Retinal Degeneration. Acta Diabetol. 51 (2), 211–218. doi:10.1007/s00592-013-0488-4
Fort, P. E., Freeman, W. M., Losiewicz, M. K., Singh, R. S. J., and Gardner, T. W. (2009). The Retinal Proteome in Experimental Diabetic Retinopathy. Mol. Cell Proteomics 8 (4), 767–779. doi:10.1074/mcp.M800326-MCP200
Freilich, R., Betegon, M., Tse, E., Mok, S.-A., Julien, O., Agard, D. A., et al. (2018). Competing Protein-Protein Interactions Regulate Binding of Hsp27 to its Client Protein Tau. Nat. Commun. 9 (1), 4563. doi:10.1038/s41467-018-07012-4
Ge, H., Du, J., Xu, J., Meng, X., Tian, J., Yang, J., et al. (2017). SUMOylation of HSP27 by Small Ubiquitin-like Modifier 2/3 Promotes Proliferation and Invasion of Hepatocellular Carcinoma Cells. Cancer Biol. Ther. 18 (8), 552–559. doi:10.1080/15384047.2017.1345382
Grotegut, P., Hoerdemann, P. J., Reinehr, S., Gupta, N., Dick, H. B., and Joachim, S. C. (2021). Heat Shock Protein 27 Injection Leads to Caspase Activation in the Visual Pathway and Retinal T-Cell Response. Ijms 22 (2), 513. doi:10.3390/ijms22020513
Grotegut, P., Kuehn, S., Dick, H. B., and Joachim, S. C. (2020). Destructive Effect of Intravitreal Heat Shock Protein 27 Application on Retinal Ganglion Cells and Neurofilament. Ijms 21 (2), 549. doi:10.3390/ijms21020549
Guo, Y., Cepurna, W. O., Dyck, J. A., Doser, T. A., Johnson, E. C., and Morrison, J. C. (2010). Retinal Cell Responses to Elevated Intraocular Pressure: a Gene Array Comparison between the Whole Retina and Retinal Ganglion Cell Layer. Invest. Ophthalmol. Vis. Sci. 51 (6), 3003–3018. doi:10.1167/iovs.09-4663
Han, L., Jiang, Y., Han, D., and Tan, W. (2018). Hsp27 Regulates Epithelial Mesenchymal Transition, Metastasis and Proliferation in Colorectal Carcinoma. Oncol. Lett. 16 (4), 5309–5316. doi:10.3892/ol.2018.9286
Hebb, M. O., Myers, T. L., and Clarke, D. B. (2006). Enhanced Expression of Heat Shock Protein 27 Is Correlated with Axonal Regeneration in Mature Retinal Ganglion Cells. Brain Res. 1073-1074, 146–150. doi:10.1016/j.brainres.2005.12.038
Heise, E. A., and Fort, P. E. (2011). Impact of Diabetes on Alpha-Crystallins and Other Heat Shock Proteins in the Eye. J Ocul. Biol. Dis. Inform. 4 (1-2), 62–69. doi:10.1007/s12177-011-9073-7
Heise, E. A., Marozas, L. M., Grafton, S. A., Green, K. M., Kirwin, S. J., and Fort, P. E. (2013). Strain-independent Increases of Crystallin Proteins in the Retina of Type 1 Diabetic Rats. PLoS One 8 (12), e82520. doi:10.1371/journal.pone.0082520
Huang, W., Fileta, J. B., Filippopoulos, T., Ray, A., Dobberfuhl, A., and Grosskreutz, C. L. (2007). Hsp27 Phosphorylation in Experimental Glaucoma. Invest. Ophthalmol. Vis. Sci. 48 (9), 4129–4135. doi:10.1167/iovs.06-0606
Ishikawa, K., Sreekumar, P. G., Spee, C., Nazari, H., Zhu, D., Kannan, R., et al. (2016). αB-Crystallin Regulates Subretinal Fibrosis by Modulation of Epithelial-Mesenchymal Transition. Am. J. Pathol. 186 (4), 859–873. doi:10.1016/j.ajpath.2015.11.014
Ito, H., Kamei, K., Iwamoto, I., Inaguma, Y., Nohara, D., and Kato, K. (2001). Phosphorylation-induced Change of the Oligomerization State of αB-crystallin. J. Biol. Chem. 276 (7), 5346–5352. doi:10.1074/jbc.M009004200
Ito, H., Okamoto, K., Nakayama, H., Isobe, T., and Kato, K. (1997). Phosphorylation of αB-Crystallin in Response to Various Types of Stress. J. Biol. Chem. 272 (47), 29934–29941. doi:10.1074/jbc.272.47.29934
Jaya, N., Garcia, V., and Vierling, E. (2009). Substrate Binding Site Flexibility of the Small Heat Shock Protein Molecular Chaperones. Proc. Natl. Acad. Sci. U.S.A. 106 (37), 15604–15609. doi:10.1073/pnas.0902177106
Joachim, S. C., Bruns, K., Lackner, K. J., Pfeiffer, N., and Grus, F. H. (2007). Antibodies to α B-Crystallin, Vimentin, and Heat Shock Protein 70 in Aqueous Humor of Patients with Normal Tension Glaucoma and IgG Antibody Patterns against Retinal Antigen in Aqueous Humor. Curr. Eye Res. 32 (6), 501–509. doi:10.1080/02713680701375183
Joachim, S. C., Grus, F. H., Kraft, D., White-Farrar, K., Barnes, G., Barbeck, M., et al. (2009). Complex Antibody Profile Changes in an Experimental Autoimmune Glaucoma Animal Model. Invest. Ophthalmol. Vis. Sci. 50 (10), 4734–4742. doi:10.1167/iovs.08-3144
Joussen, A. M., Poulaki, V., Le, M. L., Koizumi, K., Esser, C., Janicki, H., et al. (2004). A central Role for Inflammation in the Pathogenesis of Diabetic Retinopathy. FASEB j. 18 (12), 1450–1452. doi:10.1096/fj.03-1476fje
Jovcevski, B., Kelly, M. A., Rote, A. P., Berg, T., Gastall, H. Y., Benesch, J. L. P., et al. (2015). Phosphomimics Destabilize Hsp27 Oligomeric Assemblies and Enhance Chaperone Activity. Chem. Biol. 22 (2), 186–195. doi:10.1016/j.chembiol.2015.01.001
Kalesnykas, G., Niittykoski, M., Rantala, J., Miettinen, R., Salminen, A., Kaarniranta, K., et al. (2007). The Expression of Heat Shock Protein 27 in Retinal Ganglion and Glial Cells in a Rat Glaucoma Model. Neuroscience 150 (3), 692–704. doi:10.1016/j.neuroscience.2007.09.078
Kamradt, M. C., Lu, M., Werner, M. E., Kwan, T., Chen, F., Strohecker, A., et al. (2005). The Small Heat Shock Protein αB-crystallin Is a Novel Inhibitor of TRAIL-Induced Apoptosis that Suppresses the Activation of Caspase-3. J. Biol. Chem. 280 (12), 11059–11066. doi:10.1074/jbc.M413382200
Kannan, R., Sreekumar, P. G., and Hinton, D. R. (2016). Alpha Crystallins in the Retinal Pigment Epithelium and Implications for the Pathogenesis and Treatment of Age-Related Macular Degeneration. Biochim. Biophys. Acta (Bba) - Gen. Subjects 1860 (1 Pt B), 258–268. doi:10.1016/j.bbagen.2015.05.016
Kappé, G., Boelens, W. C., and de Jong, W. W. (2010). Why Proteins without an α-crystallin Domain Should Not Be Included in the Human Small Heat Shock Protein Family HSPB. Cell Stress and Chaperones 15 (4), 457–461. doi:10.1007/s12192-009-0155-4
Kappé, G., Franck, E., Verschuure, P., Boelens, W. C., Leunissen, J. A. M., and de Jong, W. W. (2003). The Human Genome Encodes 10 α-crystallin-related Small Heat Shock Proteins: HspB1-10. Cell Stress Chaper 8 (1), 53–61. doi:10.1379/1466-1268(2003)8<53:thgecs>2.0.co;2
Kase, S., He, S., Sonoda, S., Kitamura, M., Spee, C., Wawrousek, E., et al. (2010). αB-crystallin Regulation of Angiogenesis by Modulation of VEGF. Blood 115 (16), 3398–3406. doi:10.1182/blood-2009-01-197095
Kase, S., Ishida, S., and Rao, N. A. (2011). Increased Expression of αA-crystallin in Human Diabetic Eye. Int. J. Mol. Med. 28 (4), 505–511. doi:10.3892/ijmm.2011.708
Kim, Y. H., Park, S. Y., Park, J., Kim, Y. S., Hwang, E. M., Park, J. Y., et al. (2012). Reduction of Experimental Diabetic Vascular Leakage and Pericyte Apoptosis in Mice by Delivery of αA-crystallin with a Recombinant Adenovirus. Diabetologia 55 (10), 2835–2844. doi:10.1007/s00125-012-2625-y
Klopstein, A., Santos-Nogueira, E., Francos-Quijorna, I., Redensek, A., David, S., Navarro, X., et al. (2012). Beneficial Effects of B-Crystallin in Spinal Cord Contusion Injury. J. Neurosci. 32 (42), 14478–14488. doi:10.1523/JNEUROSCI.0923-12.2012
Koteiche, H. A., and McHaourab, H. S. (2003). Mechanism of Chaperone Function in Small Heat-Shock Proteins. J. Biol. Chem. 278 (12), 10361–10367. doi:10.1074/jbc.M211851200
Kretz, A., Schmeer, C., Tausch, S., and Isenmann, S. (2006). Simvastatin Promotes Heat Shock Protein 27 Expression and Akt Activation in the Rat Retina and Protects Axotomized Retinal Ganglion Cells In Vivo. Neurobiol. Dis. 21 (2), 421–430. doi:10.1016/j.nbd.2005.08.003
Kriehuber, T., Rattei, T., Weinmaier, T., Bepperling, A., Haslbeck, M., and Buchner, J. (2010). Independent Evolution of the Core Domain and its Flanking Sequences in Small Heat Shock Proteins. FASEB j. 24 (10), 3633–3642. doi:10.1096/fj.10-156992
Krueger-Naug, A. M. R., Emsley, J. G., Myers, T. L., Currie, R. W., and Clarke, D. B. (2003). Administration of Brain-Derived Neurotrophic Factor Suppresses the Expression of Heat Shock Protein 27 in Rat Retinal Ganglion Cells Following Axotomy. Neuroscience 116 (1), 49–58. doi:10.1016/s0306-4522(02)00582-1
Krueger-Naug, A. M. R., Emsley, J. G., Myers, T. L., Currie, R. W., and Clarke, D. B. (2002). Injury to Retinal Ganglion Cells Induces Expression of the Small Heat Shock Protein Hsp27 in the Rat Visual System. Neuroscience 110 (4), 653–665. doi:10.1016/s0306-4522(01)00453-5
Kurnellas, M. P., Brownell, S. E., Su, L., Malkovskiy, A. V., Rajadas, J., Dolganov, G., et al. (2012). Chaperone Activity of Small Heat Shock Proteins Underlies Therapeutic Efficacy in Experimental Autoimmune Encephalomyelitis. J. Biol. Chem. 287 (43), 36423–36434. doi:10.1074/jbc.M112.371229
Larsen, J. K., Yamboliev, I. A., Weber, L. A., and Gerthoffer, W. T. (1997). Phosphorylation of the 27-kDa Heat Shock Protein via P38 MAP Kinase and MAPKAP Kinase in Smooth Muscle. Am. J. Physiology-Lung Cell Mol. Physiol. 273 (5), L930–L940. doi:10.1152/ajplung.1997.273.5.L930
Lee, Y.-J., Lee, H.-J., Choi, S.-h., Jin, Y. B., An, H. J., Kang, J.-H., et al. (2012). Soluble HSPB1 Regulates VEGF-Mediated Angiogenesis through Their Direct Interaction. Angiogenesis 15 (2), 229–242. doi:10.1007/s10456-012-9255-3
Lelj-Garolla, B., and Mauk, A. G. (2006). Self-association and Chaperone Activity of Hsp27 Are Thermally Activated. J. Biol. Chem. 281 (12), 8169–8174. doi:10.1074/jbc.M512553200
Li, Q., Wang, Y., Lai, Y., Xu, P., and Yang, Z. (2017). HspB5 Correlates with Poor Prognosis in Colorectal Cancer and Prompts Epithelial-Mesenchymal Transition through ERK Signaling. PLoS One 12 (8), e0182588. doi:10.1371/journal.pone.0182588
Li, Y., Roth, S., Laser, M., Ma, J.-x., and Crosson, C. E. (2003). Retinal Preconditioning and the Induction of Heat-Shock Protein 27. Invest. Ophthalmol. Vis. Sci. 44 (3), 1299–1304. doi:10.1167/iovs.02-0235
Lim, E.-M. F., Hoghooghi, V., Hagen, K. M., Kapoor, K., Frederick, A., Finlay, T. M., et al. (2021). Presence and Activation of Pro-inflammatory Macrophages Are Associated with CRYAB Expression In Vitro and after Peripheral Nerve Injury. J. Neuroinflammation 18 (1), 82. doi:10.1186/s12974-021-02108-z
Losiewicz, M. K., and Fort, P. E. (2011). Diabetes Impairs the Neuroprotective Properties of Retinal Alpha-Crystallins. Invest. Ophthalmol. Vis. Sci. 52 (9), 5034–5042. doi:10.1167/iovs.10-6931
Maksimiuk, M., Sobiborowicz, A., Tuzimek, A., Deptała, A., Czerw, A., and Badowska-Kozakiewicz, A. (2020). αB-crystallin as a Promising Target in Pathological Conditions - A Review. Ann. Agric. Environ. Med. 27 (3), 326–334. doi:10.26444/aaem/111759
Mehlen, P., Preville, X., Chareyron, P., Briolay, J., Klemenz, R., and Arrigo, A. P. (1995). Constitutive Expression of Human Hsp27, Drosophila Hsp27, or Human Alpha B-Crystallin Confers Resistance to TNF- and Oxidative Stress-Induced Cytotoxicity in Stably Transfected Murine L929 Fibroblasts. J. Immunol. 154 (1), 363–374.
Mellier, G., Liu, D., Bellot, G., Lisa Holme, A., and Pervaiz, S. (2013). Small Molecule Sensitization to TRAIL Is Mediated via Nuclear Localization, Phosphorylation and Inhibition of Chaperone Activity of Hsp27. Cell Death Dis 4, e890. doi:10.1038/cddis.2013.413
Miyara, N., Shinzato, M., Yamashiro, Y., Iwamatsu, A., Kariya, K.-i., and Sawaguchi, S. (2008). Proteomic Analysis of Rat Retina in a Steroid-Induced Ocular Hypertension Model: Potential Vulnerability to Oxidative Stress. Jpn. J. Ophthalmol. 52 (2), 84–90. doi:10.1007/s10384-007-0507-5
Mrugacz, M., Bryl, A., and Zorena, K. (2021). Retinal Vascular Endothelial Cell Dysfunction and Neuroretinal Degeneration in Diabetic Patients. Jcm 10 (3), 458. doi:10.3390/jcm10030458
Munemasa, Y., Kwong, J. M. K., Caprioli, J., and Piri, N. (2009). The Role of αA- and αB-Crystallins in the Survival of Retinal Ganglion Cells after Optic Nerve Axotomy. Invest. Ophthalmol. Vis. Sci. 50 (8), 3869–3875. doi:10.1167/iovs.08-3138
Mymrikov, E. V., Daake, M., Richter, B., Haslbeck, M., and Buchner, J. (2017). The Chaperone Activity and Substrate Spectrum of Human Small Heat Shock Proteins. J. Biol. Chem. 292 (2), 672–684. doi:10.1074/jbc.M116.760413
Mymrikov, E. V., Seit-Nebi, A. S., and Gusev, N. B. (2011). Large Potentials of Small Heat Shock Proteins. Physiol. Rev. 91 (4), 1123–1159. doi:10.1152/physrev.00023.2010
Nagaraj, R. H., Linetsky, M., and Stitt, A. W. (2012). The Pathogenic Role of Maillard Reaction in the Aging Eye. Amino Acids 42 (4), 1205–1220. doi:10.1007/s00726-010-0778-x
Nagaraj, R. H., Nahomi, R. B., Mueller, N. H., Raghavan, C. T., Ammar, D. A., and Petrash, J. M. (2016). Therapeutic Potential of α-crystallin. Biochim. Biophys. Acta (Bba) - Gen. Subjects 1860 (1 Pt B), 252–257. doi:10.1016/j.bbagen.2015.03.012
Nagaraj, R. H., Nahomi, R. B., Shanthakumar, S., Linetsky, M., Padmanabha, S., Pasupuleti, N., et al. (2012). Acetylation of αA-crystallin in the Human Lens: Effects on Structure and Chaperone Function. Biochim. Biophys. Acta (Bba) - Mol. Basis Dis. 1822 (2), 120–129. doi:10.1016/j.bbadis.2011.11.011
Nahomi, R. B., Huang, R., Nandi, S. K., Wang, B., Padmanabha, S., Santhoshkumar, P., et al. (2013). Acetylation of Lysine 92 Improves the Chaperone and Anti-apoptotic Activities of Human αB-Crystallin. Biochemistry 52 (45), 8126–8138. doi:10.1021/bi400638s
Nahomi, R. B., Nandi, S. K., Rakete, S., Michel, C., Fritz, K. S., and Nagaraj, R. H. (2020). Lysine Malonylation and Propionylation Are Prevalent in Human Lens Proteins. Exp. Eye Res. 190, 107864. doi:10.1016/j.exer.2019.107864
Nahomi, R. B., Oya-Ito, T., and Nagaraj, R. H. (2013). The Combined Effect of Acetylation and Glycation on the Chaperone and Anti-apoptotic Functions of Human α-crystallin. Biochim. Biophys. Acta (Bba) - Mol. Basis Dis. 1832 (1), 195–203. doi:10.1016/j.bbadis.2012.08.015
Nahomi, R. B., Palmer, A., Green, K. M., Fort, P. E., and Nagaraj, R. H. (2014). Pro-inflammatory Cytokines Downregulate Hsp27 and Cause Apoptosis of Human Retinal Capillary Endothelial Cells. Biochim. Biophys. Acta (Bba) - Mol. Basis Dis. 1842 (2), 164–174. doi:10.1016/j.bbadis.2013.11.011
Nandi, S. K., Nahomi, R. B., Harris, P. S., Michel, C. R., Fritz, K. S., and Nagaraj, R. H. (2019). The Absence of SIRT3 and SIRT5 Promotes the Acetylation of Lens Proteins and Improves the Chaperone Activity of α-crystallin in Mouse Lenses. Exp. Eye Res. 182, 1–9. doi:10.1016/j.exer.2019.02.024
Nandi, S. K., Rakete, S., Nahomi, R. B., Michel, C., Dunbar, A., Fritz, K. S., et al. (2019). Succinylation Is a Gain-Of-Function Modification in Human Lens αB-Crystallin. Biochemistry 58 (9), 1260–1274. doi:10.1021/acs.biochem.8b01053
Nath, M., Shan, Y., Myers, A. M., and Fort, P. E. (2021). HspB4/αA-Crystallin Modulates Neuroinflammation in the Retina via the Stress-specific Inflammatory Pathways. Jcm 10 (11), 2384. doi:10.3390/jcm10112384
Nath, M., Sluzala, Z. B., Phadte, A. S., Shan, Y., Myers, A. M., and Fort, P. E. (2022). Evidence for Paracrine Protective Role of Exogenous αA-Crystallin in Retinal Ganglion Cells. eNeuro 9, 0045–122. doi:10.1523/ENEURO.0045-22.2022
O’Reilly, A. M., Currie, R. W., and Clarke, D. B. (2010). HspB1 (Hsp 27) Expression and Neuroprotection in the Retina. Mol. Neurobiol. 42 (2), 124–132. doi:10.1007/s12035-010-8143-3
Pangratz-Fuehrer, S., Kaur, K., Ousman, S. S., Steinman, L., and Liao, Y. J. (2011). Functional rescue of Experimental Ischemic Optic Neuropathy with αB-crystallin. Eye 25 (6), 809–817. doi:10.1038/eye.2011.42
Park, Y. H., Snook, J. D., Ostrin, E. J., Kim, S., Chen, R., and Frankfort, B. J. (2019). Transcriptomic Profiles of Retinal Ganglion Cells Are Defined by the Magnitude of Intraocular Pressure Elevation in Adult Mice. Sci. Rep. 9 (1), 2594. doi:10.1038/s41598-019-39141-1
Pasupuleti, N., Matsuyama, S., Voss, O., Doseff, A. I., Song, K., Danielpour, D., et al. (2010). The Anti-apoptotic Function of Human αA-crystallin Is Directly Related to its Chaperone Activity. Cel Death Dis 1, e31. doi:10.1038/cddis.2010.3
Pinach, S., Burt, D., Berrone, E., Barutta, F., Bruno, G., Porta, M., et al. (2013). Retinal Heat Shock Protein 25 in Early Experimental Diabetes. Acta Diabetol. 50 (4), 579–585. doi:10.1007/s00592-011-0346-1
Piri, N., Kwong, J. M. K., and Caprioli, J. (2013). Crystallins in Retinal Ganglion Cell Survival and Regeneration. Mol. Neurobiol. 48 (3), 819–828. doi:10.1007/s12035-013-8470-2
Pons, M., Cousins, S. W., Csaky, K. G., Striker, G., and Marin-Castaño, M. E. (2010). Cigarette Smoke-Related Hydroquinone Induces Filamentous Actin Reorganization and Heat Shock Protein 27 Phosphorylation through P38 and Extracellular Signal-Regulated Kinase 1/2 in Retinal Pigment Epithelium. Am. J. Pathol. 177 (3), 1198–1213. doi:10.2353/ajpath.2010.091108
Rada, C. C., Mejia-Pena, H., Grimsey, N. J., Canto Cordova, I., Olson, J., Wozniak, J. M., et al. (2021). Heat Shock Protein 27 Activity Is Linked to Endothelial Barrier Recovery after Proinflammatory GPCR-Induced Disruption. Sci. Signal. 14 (698), eabc1044. doi:10.1126/scisignal.abc1044
Raju, M., Santhoshkumar, P., and Krishna Sharma, K. (2016). Alpha-crystallin-derived Peptides as Therapeutic Chaperones. Biochim. Biophys. Acta (Bba) - Gen. Subjects 1860 (1 Pt B), 246–251. doi:10.1016/j.bbagen.2015.06.010
Rao, N. A., Saraswathy, S., Pararajasegaram, G., and Bhat, S. P. (2012). Small Heat Shock Protein αA-Crystallin Prevents Photoreceptor Degeneration in Experimental Autoimmune Uveitis. PLoS One 7 (3), e33582. doi:10.1371/journal.pone.0033582
Reddy, V. S., Raghu, G., Reddy, S. S., Pasupulati, A. K., Suryanarayana, P., and Reddy, G. B. (2013). Response of Small Heat Shock Proteins in Diabetic Rat Retina. Invest. Ophthalmol. Vis. Sci. 54 (12), 7674–7682. doi:10.1167/iovs.13-12715
Rogalla, T., Ehrnsperger, M., Preville, X., Kotlyarov, A., Lutsch, G., Ducasse, C., et al. (1999). Regulation of Hsp27 Oligomerization, Chaperone Function, and Protective Activity against Oxidative Stress/Tumor Necrosis Factor α by Phosphorylation. J. Biol. Chem. 274 (27), 18947–18956. doi:10.1074/jbc.274.27.18947
Rothbard, J. B., Kurnellas, M. P., Brownell, S., Adams, C. M., Su, L., Axtell, R. C., et al. (2012). Therapeutic Effects of Systemic Administration of Chaperone αB-Crystallin Associated with Binding Proinflammatory Plasma Proteins. J. Biol. Chem. 287 (13), 9708–9721. doi:10.1074/jbc.M111.337691
Ruebsam, A., Dulle, J. E., Myers, A. M., Sakrikar, D., Green, K. M., Khan, N. W., et al. (2018). A Specific Phosphorylation Regulates the Protective Role of αA-crystallin in Diabetes. JCI Insight 3 (4). doi:10.1172/jci.insight.97919
Rusmini, P., Cristofani, R., Galbiati, M., Cicardi, M. E., Meroni, M., Ferrari, V., et al. (2017). The Role of the Heat Shock Protein B8 (HSPB8) in Motoneuron Diseases. Front. Mol. Neurosci. 10, 176. doi:10.3389/fnmol.2017.00176
Sakai, M., Sakai, H., Nakamura, Y., Fukuchi, T., and Sawaguchi, S. (2003). Immunolocalization of Heat Shock Proteins in the Retina of normal Monkey Eyes and Monkey Eyes with Laser-Induced Glaucoma. Jpn. J. Ophthalmol. 47 (1), 42–52. doi:10.1016/s0021-5155(02)00627-5
Schmidt, T., Fischer, D., Andreadaki, A., Bartelt-Kirbach, B., and Golenhofen, N. (2016). Induction and Phosphorylation of the Small Heat Shock Proteins HspB1/Hsp25 and HspB5/αB-Crystallin in the Rat Retina upon Optic Nerve Injury. Cell Stress and Chaperones 21 (1), 167–178. doi:10.1007/s12192-015-0650-8
Sharma, K. K., Kumar, R. S., Kumar, G. S., and Quinn, P. T. (2000). Synthesis and Characterization of a Peptide Identified as a Functional Element in αA-crystallin. J. Biol. Chem. 275 (6), 3767–3771. doi:10.1074/jbc.275.6.3767
Shemetov, A. A., Seit-Nebi, A. S., Bukach, O. V., and Gusev, N. B. (2008). Phosphorylation by Cyclic AMP-dependent Protein Kinase Inhibits Chaperone-like Activity of Human HSP22 In Vitro. Biochem. Mosc. 73 (2), 200–208. doi:10.1134/s0006297908020120
Shemetov, A. A., Seit-Nebi, A. S., and Gusev, N. B. (2011). Phosphorylation of Human Small Heat Shock Protein HspB8 (Hsp22) by ERK1 Protein Kinase. Mol. Cel Biochem 355 (1-2), 47–55. doi:10.1007/s11010-011-0837-y
Shi, C., Yang, X., Bu, X., Hou, N., and Chen, P. (2017). Alpha B-Crystallin Promotes the Invasion and Metastasis of Colorectal Cancer via Epithelial-Mesenchymal Transition. Biochem. Biophysical Res. Commun. 489 (4), 369–374. doi:10.1016/j.bbrc.2017.05.070
Shi, Y., Jiang, X., Zhang, L., Pu, H., Hu, X., Zhang, W., et al. (2017). Endothelium-targeted Overexpression of Heat Shock Protein 27 Ameliorates Blood-Brain Barrier Disruption after Ischemic Brain Injury. Proc. Natl. Acad. Sci. U.S.A. 114 (7), E1243–E1252. doi:10.1073/pnas.1621174114
Shiota, M., Bishop, J. L., Nip, K. M., Zardan, A., Takeuchi, A., Cordonnier, T., et al. (2013). Hsp27 Regulates Epithelial Mesenchymal Transition, Metastasis, and Circulating Tumor Cells in Prostate Cancer. Cancer Res. 73 (10), 3109–3119. doi:10.1158/0008-5472.CAN-12-3979
Sreekumar, P. G., Li, Z., Wang, W., Spee, C., Hinton, D. R., Kannan, R., et al. (2018). Intra-vitreal αB Crystallin Fused to Elastin-like Polypeptide Provides Neuroprotection in a Mouse Model of Age-Related Macular Degeneration. J. Controlled Release 283, 94–104. doi:10.1016/j.jconrel.2018.05.014
Sreekumar, P. G., Reddy, S. T., Hinton, D. R., and Kannan, R. (2022). Mechanisms of RPE Senescence and Potential Role of αB Crystallin Peptide as a Senolytic Agent in Experimental AMD. Exp. Eye Res. 215, 108918. doi:10.1016/j.exer.2021.108918
Stankowska, D. L., Nam, M.-H., Nahomi, R. B., Chaphalkar, R. M., Nandi, S. K., Fudala, R., et al. (2019). Systemically Administered Peptain-1 Inhibits Retinal Ganglion Cell Death in Animal Models: Implications for Neuroprotection in Glaucoma. Cell Death Discov. 5, 112. doi:10.1038/s41420-019-0194-2
Steele, M. R., Inman, D. M., Calkins, D. J., Horner, P. J., and Vetter, M. L. (2006). Microarray Analysis of Retinal Gene Expression in the DBA/2J Model of Glaucoma. Invest. Ophthalmol. Vis. Sci. 47 (3), 977–985. doi:10.1167/iovs.05-0865
Strunnikova, N., Baffi, J., Gonzalez, A., Silk, W., Cousins, S. W., and Csaky, K. G. (2001). Regulated Heat Shock Protein 27 Expression in Human Retinal Pigment Epithelium. Invest. Ophthalmol. Vis. Sci. 42 (9), 2130–2138.
Swamy, M. S., Abraham, A., and Abraham, E. C. (1992). Glycation of Human Lens Proteins: Preferential Glycation of αA Subunits. Exp. Eye Res. 54 (3), 337–345. doi:10.1016/0014-4835(92)90046-u
Takayama, S., Reed, J. C., and Homma, S. (2003). Heat-shock Proteins as Regulators of Apoptosis. Oncogene 22 (56), 9041–9047. doi:10.1038/sj.onc.1207114
Tao, X., Cheng, L., Li, Y., Ci, H., Xu, J., Wu, S., et al. (2019). Expression of CRYAB with the Angiogenesis and Poor Prognosis for Human Gastric Cancer. Medicine (Baltimore) 98 (45), e17799. doi:10.1097/MD.0000000000017799
Taylor, R. P., and Benjamin, I. J. (2005). Small Heat Shock Proteins: a New Classification Scheme in Mammals. J. Mol. Cell Cardiol. 38 (3), 433–444. doi:10.1016/j.yjmcc.2004.12.014
Tezel, G., Seigel, G. M., and Wax, M. B. (1998). Autoantibodies to Small Heat Shock Proteins in Glaucoma. Invest. Ophthalmol. Vis. Sci. 39 (12), 2277–2287.
Tezel, G., Hernandez, R., and Wax, M. B. (2000). Immunostaining of Heat Shock Proteins in the Retina and Optic Nerve Head of normal and Glaucomatous Eyes. Arch. Ophthalmol. 118 (4), 511–518. doi:10.1001/archopht.118.4.511
Tezel, G., and Wax, M. B. (2000). The Mechanisms of Hsp27 Antibody-Mediated Apoptosis in Retinal Neuronal Cells. J. Neurosci. 20 (10), 3552–3562. doi:10.1523/jneurosci.20-10-03552.2000
Thanos, S., Böhm, M. R. R., Meyer zu Hörste, M., Prokosch-Willing, V., Hennig, M., Bauer, D., et al. (2014). Role of Crystallins in Ocular Neuroprotection and Axonal Regeneration. Prog. Retin. Eye Res. 42, 145–161. doi:10.1016/j.preteyeres.2014.06.004
Umeda, S., Suzuki, M. T., Okamoto, H., Ono, F., Mizota, A., Terao, K., et al. (2005). Molecular Composition of Drusen and Possible Involvement of Anti‐retinal Autoimmunity in Two Different Forms of Macular Degeneration in Cynomolgus Monkey ( Macaca fascicularis ). FASEB j. 19 (12), 1683–1685. doi:10.1096/fj.04-3525fje
Vähätupa, M., Nättinen, J., Jylhä, A., Aapola, U., Kataja, M., Kööbi, P., et al. (2018). SWATH-MS Proteomic Analysis of Oxygen-Induced Retinopathy Reveals Novel Potential Therapeutic Targets. Invest. Ophthalmol. Vis. Sci. 59 (8), 3294–3306. doi:10.1167/iovs.18-23831
Wang, W., Zhang, Y., Jin, W., Xing, Y., and Yang, A. (2018). Catechin Weakens Diabetic Retinopathy by Inhibiting the Expression of NF-Κb Signaling Pathway-Mediated Inflammatory Factors. Ann. Clin. Lab. Sci. 48 (5), 594–600.
Wang, W., Sreekumar, P. G., Valluripalli, V., Shi, P., Wang, J., Lin, Y.-A., et al. (2014). Protein Polymer Nanoparticles Engineered as Chaperones Protect against Apoptosis in Human Retinal Pigment Epithelial Cells. J. Controlled Release 191, 4–14. doi:10.1016/j.jconrel.2014.04.028
Wax, M. B., Tezel, G., and Edward, P. D. (1998). Clinical and Ocular Histopathological Findings in a Patient with normal-pressure Glaucoma. Arch. Ophthalmol. 116 (8), 993–1001. doi:10.1001/archopht.116.8.993
Wax, M. B., Tezel, G., Yang, J., Peng, G., Patil, R. V., Agarwal, N., et al. (2008). Induced Autoimmunity to Heat Shock Proteins Elicits Glaucomatous Loss of Retinal Ganglion Cell Neurons via Activated T-Cell-Derived Fas-Ligand. J. Neurosci. 28 (46), 12085–12096. doi:10.1523/JNEUROSCI.3200-08.2008
Wax, M., Tezel, G., Kawase, K., and Kitazawa, Y. (2001). Serum Autoantibodies to Heat Shock Proteins in Glaucoma Patients from Japan and the United States. Ophthalmology 108 (2), 296–302. doi:10.1016/s0161-6420(00)00525-x
Webster, J. M., Darling, A. L., Uversky, V. N., and Blair, L. J. (2019). Small Heat Shock Proteins, Big Impact on Protein Aggregation in Neurodegenerative Disease. Front. Pharmacol. 10, 1047. doi:10.3389/fphar.2019.01047
Westerheide, S. D., and Morimoto, R. I. (2005). Heat Shock Response Modulators as Therapeutic Tools for Diseases of Protein Conformation. J. Biol. Chem. 280 (39), 33097–33100. doi:10.1074/jbc.R500010200
Wettstein, G., Bellaye, P. S., Micheau, O., and Bonniaud, P. (2012). Small Heat Shock Proteins and the Cytoskeleton: an Essential Interplay for Cell Integrity? Int. J. Biochem. Cel Biol. 44 (10), 1680–1686. doi:10.1016/j.biocel.2012.05.024
Windisch, B. K., LeVatte, T. L., Archibald, M. L., and Chauhan, B. C. (2009). Induction of Heat Shock Proteins 27 and 72 in Retinal Ganglion Cells after Acute Pressure-Induced Ischaemia. Clin. Exp. Ophthalmol. 37 (3), 299–307. doi:10.1111/j.1442-9071.2009.02032.x
Wolf, B. B., and Green, D. R. (1999). Suicidal Tendencies: Apoptotic Cell Death by Caspase Family Proteinases. J. Biol. Chem. 274 (29), 20049–20052. doi:10.1074/jbc.274.29.20049
Wu, D., Kase, S., Liu, Y., Kanda, A., Murata, M., and Ishida, S. (2022). Downregulation of AlphaB-Crystallin in Retinal Pigment Epithelial Cells Exposed to Diabetes-Related Stimuli In Vivo and In Vitro. In Vivo 36 (1), 132–139. doi:10.21873/invivo.12684
Wu, N., Yu, J., Chen, S., Xu, J., Ying, X., Ye, M., et al. (2014). α-Crystallin Protects RGC Survival and Inhibits Microglial Activation after Optic Nerve Crush. Life Sci. 94 (1), 17–23. doi:10.1016/j.lfs.2013.10.034
Wu, Z., Wang, L., and Hou, S. (2012). Alpha B-Crystallin Improved Survival of Retinal Ganglion Cells in a Rat Model of Acute Ocular Hypertension. Neural Regen. Res. 7 (19), 1493–1497. doi:10.3969/j.issn.1673-5374.2012.19.008
Xi, J., Farjo, R., Yoshida, S., Kern, T. S., Swaroop, A., and Andley, U. P. (2003). A Comprehensive Analysis of the Expression of Crystallins in Mouse Retina. Mol. Vis. 9, 410–419.
Xu, W., Guo, Y., Huang, Z., Zhao, H., Zhou, M., Huang, Y., et al. (2019). Small Heat Shock Protein CRYAB Inhibits Intestinal Mucosal Inflammatory Responses and Protects Barrier Integrity through Suppressing IKKβ Activity. Mucosal Immunol. 12 (6), 1291–1303. doi:10.1038/s41385-019-0198-5
Yan, H., Peng, Y., Huang, W., Gong, L., and Li, L. (2017). The Protective Effects ofαB-Crystallin on Ischemia-Reperfusion Injury in the Rat Retina. J. Ophthalmol. 2017, 1–10. doi:10.1155/2017/7205408
Yokoyama, A., Oshitari, T., Negishi, H., Dezawa, M., Mizota, A., and Adachi-Usami, E. (2001). Protection of Retinal Ganglion Cells from Ischemia-Reperfusion Injury by Electrically Applied Hsp27. Invest. Ophthalmol. Vis. Sci. 42 (13), 3283–3286.
Zhang, X., Wang, X., Zhu, H., Kranias, E. G., Tang, Y., Peng, T., et al. (2012). Hsp20 Functions as a Novel Cardiokine in Promoting Angiogenesis via Activation of VEGFR2. PLoS One 7 (3), e32765. doi:10.1371/journal.pone.0032765
Keywords: small heat shock proteins, glaucoma, diabetic retinopathy, age-related macular degeneration, retina
Citation: Rajeswaren V, Wong JO, Yabroudi D, Nahomi RB, Rankenberg J, Nam M-H and Nagaraj RH (2022) Small Heat Shock Proteins in Retinal Diseases. Front. Mol. Biosci. 9:860375. doi: 10.3389/fmolb.2022.860375
Received: 22 January 2022; Accepted: 11 March 2022;
Published: 11 April 2022.
Edited by:
Alok Kumar Panda, KIIT University, IndiaReviewed by:
Santhoshkumar Puttur, University of Missouri, United StatesSuraj Bhat, UCLA Stein Eye Institute, United States
Copyright © 2022 Rajeswaren, Wong, Yabroudi, Nahomi, Rankenberg, Nam and Nagaraj. This is an open-access article distributed under the terms of the Creative Commons Attribution License (CC BY). The use, distribution or reproduction in other forums is permitted, provided the original author(s) and the copyright owner(s) are credited and that the original publication in this journal is cited, in accordance with accepted academic practice. No use, distribution or reproduction is permitted which does not comply with these terms.
*Correspondence: Mi-Hyun Nam, TWktaHl1bi5uYW1AY3VhbnNjaHV0ei5lZHU=; Ram H. Nagaraj, cmFtLm5hZ2FyYWpAY3VhbnNjaHV0ei5lZHU=
†These authors have contributed equally to this work