- 1Lumos Consulting, Lititz, PA, United States
- 2Division of Host-Microbe Systems and Therapeutics, Department of Pediatrics, University of California San Diego, La Jolla, CA, United States
- 3Skaggs School of Pharmacy and Pharmaceutical Sciences, University of California San Diego, La Jolla, CA, United States
Introduction: Healthcare-associated infections (HAIs) pose a significant challenge in acute care hospitals, particularly in intensive care units, due to persistent environmental contamination despite existing disinfection protocols and manual cleaning methods. Current disinfection methods are labor-intensive and often ineffective against multidrug-resistant (MDR) pathogens, highlighting the need for new, automated, hands-free approaches.
Methods: This study evaluates the bactericidal efficacy of low concentrations of gaseous ozone (5 ppm) against clinically relevant and often MDR bacteria under various concentrations, contact times, temperatures, and environmental conditions.
Results: We observed a 3 log10-fold reduction in Escherichia coli and Salmonella Typhimurium and a 1–2 log10-fold reduction in group A Streptococcus and methicillin-resistant Staphylococcus aureus upon ozone exposure. The bactericidal effect was dose-dependent, with no significant difference between single and repeated exposures. Environmental conditions such as temperature and humidity had minimal impact on low-dose ozone efficacy, with slightly improved bacterial killing at colder temperatures and higher humidity levels. Gaseous ozone also showed significant bactericidal activity against the broad range of Gram-positive and -negative MDR clinical isolates.
Discussion: These findings highlight the potential of low-dose gaseous ozone as a versatile, effective, and hands-free disinfectant for healthcare and other settings. Further research is needed to establish long-term safety and efficacy guidelines for its use in occupied spaces and to explore potential synergy with other contemporary disinfection strategies.
1 Introduction
Approximately 75,000 individuals acquire healthcare-associated infections (HAIs) annually in U.S. acute care hospitals (Popovich et al., 2019; Halverson et al., 2022). Environmental contamination significantly contributes to the acquisition of HAIs, particularly in intensive care units (ICUs) (Huang et al., 2006; Drees et al., 2008; Dancer, 2014; Cohen et al., 2018) Many clinically relevant pathogens can survive on inanimate surfaces for extended periods (Porter et al., 2024), and the presence of a previously infected occupant significantly increases the risk of subsequent residents developing HAIs (Mitchell et al., 2023). Current disinfection protocols, which include manual and mechanical cleaning with disinfectants, germicides, and ultrasonic cleaners (Rutala and Weber, 2015; Sheppeard et al., 2022), face challenges due to the development of resistance in many clinical isolates, rendering decontamination both expensive and labor-intensive (Dancer, 2014; Lourbopoulos et al., 2021). Given the persistent issues of understaffing and the growing antibiotic resistance crisis, there is a critical need for new, hands-free disinfection methods (Dancer, 2014).
Ozone (O3), a potent oxidant composed of three unstable oxygen atoms, is a naturally occurring, pungent gas found at 10–20 parts per billion (ppb) in the atmosphere and up to 100 ppb in polluted areas. Ozone demonstrates remarkable microbicidal activity by oxidizing bacterial lipids, viral envelopes and capsids, and fungal membranes (Allison et al., 2009; Brié et al., 2018; Xue et al., 2023). Additionally, it generates reactive oxygen species (ROS) that collapse cellular membranes. O3 is frequently employed as an environmental decontaminant, particularly in its aqueous form in water treatment plants (Ding et al., 2019; Epelle et al., 2023).
Ozone has also been used therapeutically for skin and oral pathologies for decades (Kim et al., 2009; Liu et al., 2023). Despite these successes, the use of gaseous ozone as an environmental disinfectant has been limited due to its toxicity to humans at levels exceeding 70 ppb. The severity of these effects can be mitigated by using lower doses, shorter durations, and less frequent applications of ozone (Bette et al., 2022; Singh et al., 2023). While high doses of ozone can be harmful, it also offers advantages as a treatment therapy for various human diseases including cancer, bacterial infections, asthma, and viral diseases, due to its disinfectant properties and anti-inflammatory effects (Singh et al., 2023; Elvis and Ekta, 2011; Zanardi et al., 2016). Additionally, ozone has demonstrated effective antimicrobial properties in sustainable food production, wound healing in healthcare, and public environments by penetrating intracellular components of microorganisms and causing oxidative damage (Epelle et al., 2023; Agarwal et al., 2020; Moraes et al., 2021; Premjit et al., 2022; Roth et al., 2023; Neves et al., 2023).
In recent years, interest in low-dose ozone as a cheap and effective environmental disinfectant has grown, particularly in the agriculture and healthcare industries (Shen et al., 2021). Gaseous ozone is a Generally Recognized as Safe (GRAS) agent and is approved by the U.S. Food and Drug Administration (FDA) for use (Bobka, 1993). Several studies have reported conflicting evidence regarding the efficacy of low-dose gaseous ozone against pathogens, with effectiveness against some bacteria but ineffectiveness against COVID-19 (Fontes et al., 2012; Westover et al., 2022; Misawa et al., 2023). Additionally, several studies have assessed ozone’s capability in decontaminating food and bacterial surfaces (Xue et al., 2023; Coll Cárdenas et al., 2011; Cantalejo et al., 2016). Most of these studies utilized a limited number of targets and exposure conditions, making it difficult to generalize the efficacy of low-dose gaseous ozone as a disinfectant.
We aimed to characterize the impact of environmental conditions, including bacterial growth conditions, temperature, and humidity, on the microbicidal capacity of low-dose ozone on clinically relevant bacterial pathogens. We found that ozone was effective at killing both Gram-positive and Gram-negative species in a dose-dependent manner. Additionally, we found that gaseous ozone was equally effective at low and ambient temperatures and was relatively unaffected by humidity. These studies address the current gap in the literature and provide evidence for the use of low-dose gaseous ozone as a potential environmental disinfectant.
2 Materials and methods
2.1 Bacterial methods
All strains utilized are listed in Table 1. Strains are organized by usage in each figure.
2.1.1 Bacterial cultures
Escherichia coli, Pseudomonas aeruginosa, Salmonella Typhimurium (S. Tm), Serratia marcescens, and Vibrio cholerae were grown in Luria broth (LB) at 37°C with aeration. Acinetobacter baumannii was grown in tryptic soy broth (TSB) at 37°C with aeration. Listeria monocytogenes was grown in brain heart infusion broth (BHI) at 37°C with aeration. Methicillin-resistant Staphylococcus aureus (MRSA), Bacillus subtilis, Enterococcus faecalis, and Lactococcus lactis were grown in Todd Hewitt Broth (THB) at 37°C with aeration. Group A Streptococcus (GAS) and group B Streptococcus (GBS) were grown in THB at 37°C without aeration. Pseudomonas fluorescens Migula was grown in nutrient broth at 30°C with aeration. For stationary phase cultures, 16–20 h cultures were used except for P. fluorescens Migula which was grown for 36 h. For log-phase cultures, stationary phase cultures were diluted 1:20 in fresh media and grown for 2–4 h under appropriate culture conditions until cultures reached mid-logarithmic phase (OD600 ~ 0.4).
2.2 Gaseous ozone
2.2.1 Gaseous ozone chamber
We constructed a 28-liter3 ozone chamber for this study, capable of controlling both ozone and humidity levels. Ozone was produced by an ozone generator (Model: 1000 mg/h; Ambohr Electric Limited, Fengdong New Town, Xi’an, Shaanxi, China), and vented into a humidity- controlled chamber. Ozone concentration was measured using an ozone sensor (Model:110-4xx, Interlink Electronics, Irvine California) and flow rate (0.1–5 ppm) was adjusted to maintain the desired concentration. We built an ozone destructor (MINSLITE-B, Hunan, China) that decomposed residual ozone immediately after reaching the desired contact time. Humidity was measured using a humidity and temperature sensor (Model: SHT-31, Sensiron AG, Stäfa Switzerland) and the humidity was adjusted with molecular grade H2O. Ambient humidity (~55–70%) was used unless otherwise indicated.
2.2.2 Refrigerated ozone chamber
Ozone was produced by an ozone generator (Model: 1000 mg/h; Ambohr Electric Limited, Fengdong New Town, Xi’an, Shaanxi, China) placed inside a compact refrigerator (74 liters3, Walmart). Ozone concentration was measured using an ozone sensor (Model ZE14-O3, Winsen Electronics Technology Co., Ltd., Zhengzhou, China) and flow rate (0.1–5 ppm) was adjusted to maintain the indicated concentration.
2.2.3 Gaseous ozone treatment
Note: to compare gaseous ozone to other published studies, ozone concentration can be expressed in multiple ways. We report concentrations in volumetric ppm O3 and contact time (CT) = ppm x exposure time. Equivalent measures are listed in Table 2. Additional ozone calculation information can be found at Oxidation Technologies (Ozone Equipment Manufacturer and Ozone System Integrators, 2024).
Unless otherwise indicated, stationary-phase cultures were serially diluted in PBS and spot-plated onto appropriate agar. Petri dishes were placed in the chamber at the indicated ozone contact times and humidities with the lid off, exposing the surface for the duration of the CT. For humidity experiments, control plates were placed in the chamber for the same amount of time with the tested humidity in the absence of ozone. For refrigerated experiments, control plates were placed in the refrigerated chamber for the same duration without ozone exposure to account for the impact of temperature on bacterial growth inhibition.
2.2.3.1 Dose response experiments
Stationary-phase E. coli, S. Tm, GAS, and MRSA were serially diluted in PBS in triplicate, plated on Luria agar (LA) or Todd Hewitt Agar (THA) plates, and exposed to 5 ppm O3 for 0, 25, 50, 100,200, or 400 O3 CT.
2.2.3.2 Repeated exposure experiments
Stationary-phase E. coli, S. Tm, GAS, and MRSA were serially diluted in PBS in triplicate, plated on LA or THA plates, and exposed to a single dose of 5 ppm O3 for 360 CT or three doses of 5 ppm O3 200 CT with 30–60 min recovery time at normal O2 concentrations between exposures. Control plates were left untreated at ambient O2.
2.2.3.3 Logarithmic vs. stationary phase experiments
E. coli, S. Tm, GAS, and MRSA in stationary or mid-log phase (OD600 0.4–0.6) were serially diluted in PBS in triplicate, plated on Luria agar (LA) or Todd Hewitt agar (THA) plates and exposed to 0, 25, 100, or 400 O3 CT.
2.2.3.4 Refrigerated exposure experiments
Stationary phase E. coli, S. Tm, GAS, and MRSA were serially diluted in PBS in triplicate, plated on LA or THA plates and exposed to 5 ppm O3 for 0, 25, 100, or 400 O3 CT at 4°C or room temperature (RT, 21–23°C). For extended refrigerated exposures, plates were exposed to 1 ppm O3 for 0 or 360 O2 CT. Untreated plates were incubated in the absence of ozone at 4°C or RT for the equivalent exposure time. All plates were then incubated overnight at 37°C.
2.2.3.5 Humidity exposure experiments
Stationary phase E. coli, S. Tm, GAS, and MRSA were serially diluted in PBS in triplicate, plated on LA or THA plates and exposed to 5 ppm O3 200 CT at low (<40%), ambient (40–60%), and high (>70%) humidity.
2.2.3.6 Clinical isolate experiments
Stationary phase MRSA, E. coli, P. aeruginosa, GAS, GBS, S. marcescens, E. faecium, V. cholerae, Salmonella, L. monocytogenes, L. lactis, B. subtilis, P. fluorescens and A. baumannii were grown in appropriate media, serially diluted in PBS in triplicate, and plated on appropriate agar as described above. Plates were exposed to 5 ppm O3 for a 400 CT or 1 ppm O3 360CT. Control plates were left untreated at ambient O2. All plates were then incubated overnight at 37°C except P. fluorescens which was incubated at 30°C.
2.2.3.7 Fitness studio pilot experiment
An ozone generator was placed inside a fitness studio, and the room was exposed to 0.5 ppm for 1 h for three consecutive evenings. Six sites were swabbed with pre-moistened sterile cotton swabs for microbial growth prior to exposure and after the final exposure. Swabs were vigorously inoculated into Letheen broth (3M) to transfer microbes, TSA was serially diluted, plated in triplicate on TSA plates, and grown at 37°C overnight for enumeration.
2.2.3.8 Data analysis
Statistical analysis was performed using GraphPad Prism v10. Comparisons between two groups were conducted using a two-tailed Student’s t-test, while comparisons among three or more groups were conducted using a one-way analysis of variance (ANOVA). Unless otherwise indicated in the figure legends, graphs display the means of technical replicates from three or more independent biological replicates ± standard error of the mean (SEM). p values <0.05 were considered statistically significant.
3 Results
We first tested the microbicidal activity of gaseous ozone, selecting E. coli and S. typhimurium as representative Gram-negative strains, and GAS and MRSA as representative Gram-positive strains. Strains were exposed to gaseous ozone at 5 ppm for varying CT. We observed a 3 log10-fold reduction in both E. coli and S. typhimurium after exposure compared to controls (Figures 1A,B). GAS and MRSA showed a 1–2 log10-fold reduction after exposure to increasing concentrations of O3 (Figures 1C,D), indicating that gaseous ozone kills in a dose-dependent manner. To assess whether repeated exposure enhances killing, we exposed strains to a single 360 CT dose or three doses of 200 CT with recovery periods between each dose. We found no significant difference in bacterial survival between single and multiple exposures, suggesting that a single potent dose is sufficient to reduce bacterial survival (Figures 1E–H).
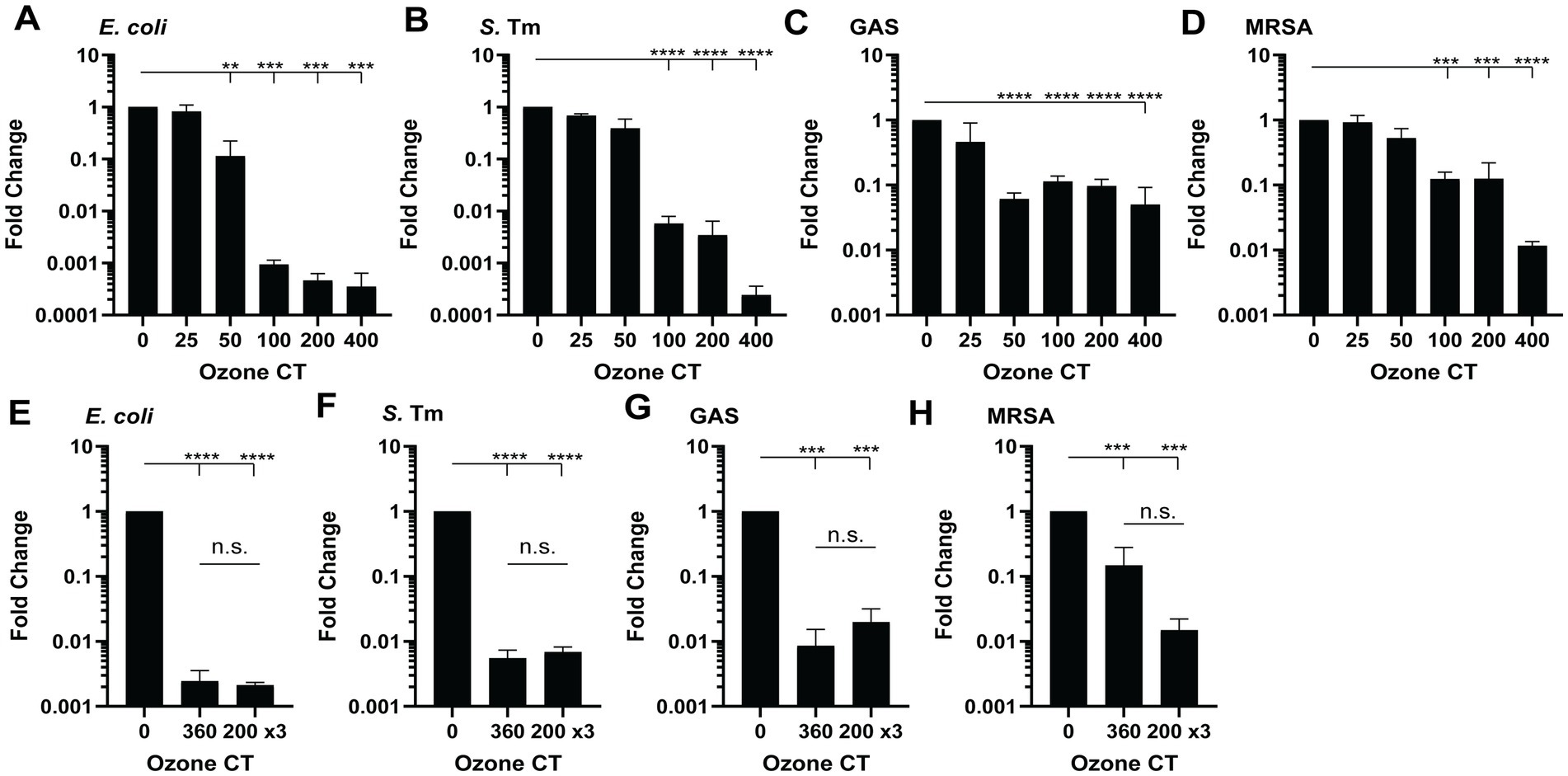
Figure 1. Gaseous ozone is effective against Gram-negative and Gram-positive bacteria in a dose-dependent manner. (A–D) Fold change in colony forming units (CFUs) from stationary phase (A) E. coli, (B) Salmonella enterica serovar Typhimurium (S. Tm), (C) Group A Streptococcus (GAS), and (D) methicillin-resistant Staphylococcus aureus (MRSA) treated with 5 ppm gaseous O3 to 25, 100, 200, and 400 CT. (E–H) Fold change in CFUs of (E) E. coli, (F) S. Tm, (G) GAS, and (H) MRSA treated with 5 ppm to 360 CT once or 200 CT three times with 30–60 min rest between each cycle. Pooled means ± SEM from three independent experiments. n.s., not significant. * p < 0.05, ** p < 0.01, *** p < 0.001, **** p < 0.0001.
Strains were exposed to gaseous ozone at 5 ppm for varying contact times (CT). We observed a three log10-fold reduction in both E. coli and S. typhimurium after exposure compared to controls (Figures 1A,B). GAS and MRSA showed a 1–2 log10-fold reduction after exposure to increasing concentrations of O3 (Figures 1C,D), indicating that gaseous ozone kills in a dose-dependent manner. To assess whether repeated exposure enhances killing, we exposed strains to a single 360 CT dose or three doses of 200 CT with recovery periods between each dose. We found no significant difference in bacterial survival between single and multiple exposures, suggesting that a single potent dose is sufficient to reduce bacterial survival (Figures 1E–H).
Next, we tested the impact of environmental conditions on the bactericidal capacity of gaseous ozone by exposing bacteria grown in logarithmic and stationary phases to O3. We did not find consistent differences in killing between growth phases of E. coli and MRSA, although stationary-phase MRSA was modestly, though not significantly, more resistant to killing (Figures 2A,B). Given the significant interest in applying ozone to refrigerated food products, we examined the impact of temperature on gaseous ozone efficacy by exposing E. coli and MRSA to gaseous O3 at ambient temperature (~21–22°C) and at 4°C. Gaseous ozone was slightly more effective at killing MRSA and E. coli at colder temperatures, though the difference did not achieve statistical significance (Figures 2C–F). We also investigated the impact of humidity on ozone’s killing capacity by testing low humidity (<40%), ambient humidity (40–60%), and high humidity (>70%). Different humidity levels did not impact the ability of ozone to kill E. coli (Figure 2G). There was a modest, though not statistically significant, improvement in the bactericidal activity of gaseous O3 against MRSA at higher humidity levels (Figure 2H). Collectively, these results indicate that gaseous ozone is effective against bacteria in both quiescent and active growth phases and that its killing capacity is relatively unaffected by low temperatures and varying humidity levels.
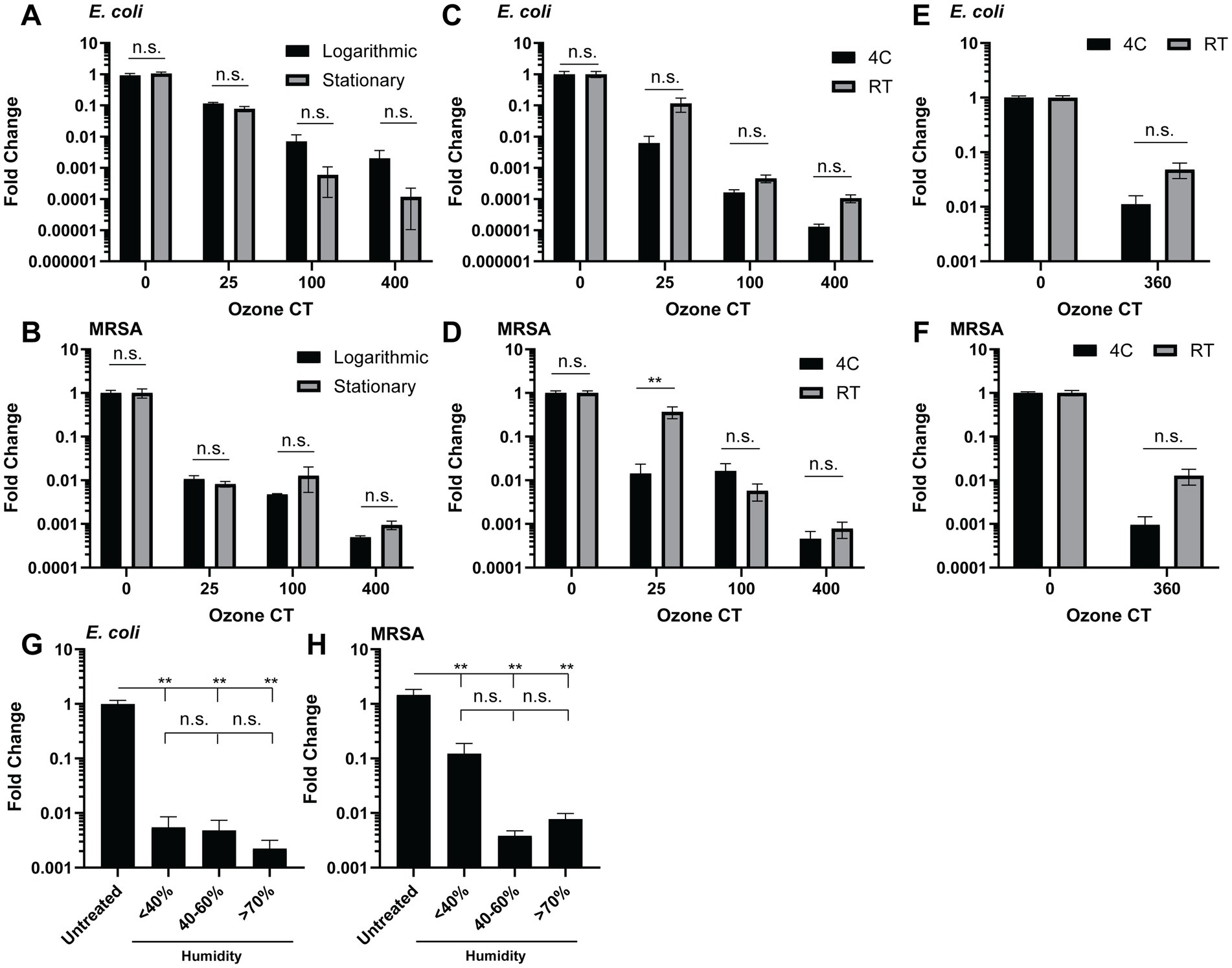
Figure 2. Ozone killing efficacy is largely indifferent to microbial growing conditions, ambient temperature, and humidity. (A,B) CFUs of (A) E. coli and (B) MRSA grown at stationary and logarithmic phase treated with 5 ppm O3 to 200 CT. (C–F) Colony forming units (CFUs) from stationary phase E. coli and MRSA treated with (C,D) 5 ppm O3 to 0, 25, 100, and 400 CT at 4°C or room temperature (RT) or (E,F) 1 ppm O3 360 CT at 4C and RT. (G,H) CFUs of (G) E. coli and (H) MRSA treated with 5 ppm O3 to 200 CT at low (<40%), Ambient (40–60%), or high (>70%) humidity. (A,B) Representative of two independent experiments. (C–F) three experiments pooled. n.s., not significant. * p < 0.05, ** p < 0.01, *** p < 0.001, **** p < 0.0001.
Lastly, we examined the ability of ozone to kill a panel of clinical isolates of important human pathogens. We exposed strains of A. baumannii, MRSA, E. coli, P. aeruginosa, GAS, GBS, E. faecium, and S. marcescens to a single dose of gaseous ozone and found significant bactericidal activity against all strains tested (Figure 3A). We further tested a broader panel of isolates relevant to both human health and the food industry. Consistent with previous findings, a single low-dose exposure of gaseous ozone effectively reduced microbial counts by at least 10-fold (Figure 3B). As a proof of principle, we conducted a pilot study where an ozone generator was placed in a fitness studio over a weekend. Six sites were sampled for microbial counts before and after exposure to gaseous ozone at 0.5 ppm for 1 h over three consecutive nights. All six sites showed reduced bacterial counts after O3 exposure (Figure 3C). These findings collectively demonstrate the potential of gaseous ozone as a hands-free microbicide effective against MDR bacterial species.
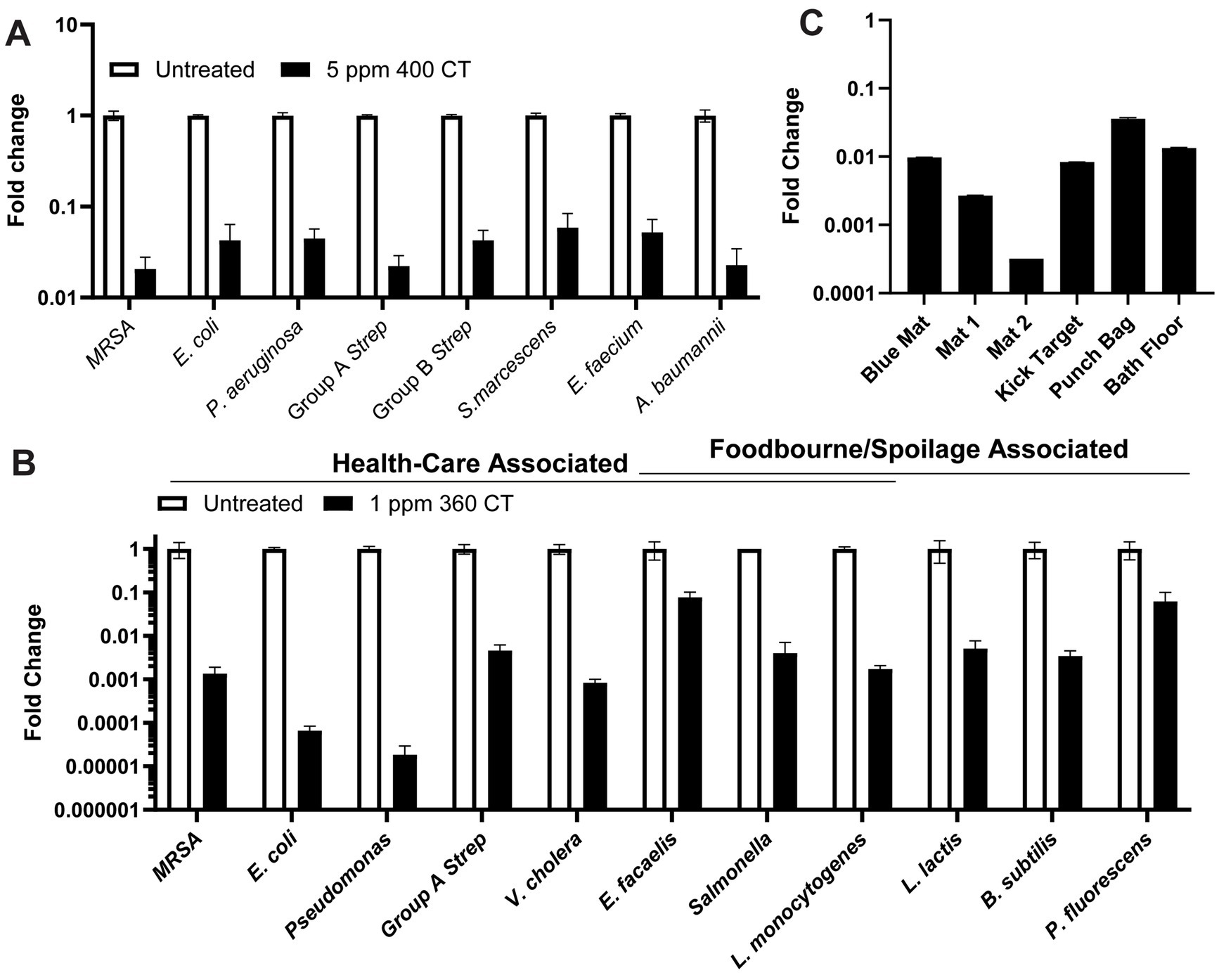
Figure 3. Gaseous ozone is effective against clinically relevant bacterial pathogens. (A) CFUs from clinical isolates treated with 5 ppm 400 CT. (B) CFUs from healthcare associated and foodborne/spoilage associated bacteria treated with 1 ppm O3 360 CT. Healthcare associated pathogens include MRSA, E. coli, Pseudomonas aeruginosa, GAS, Vibrio cholerae, Enterococcus faecalis, S. Tm, and Listeria monocytogenes. Foodborne pathogens include E. faecalis, S. Tm, L. mono, Lactococcus lactis, Bacillus subtilis, and Pseudomonas fluorescens. Pooled means ± SEM from two-five independent experiments. (C) Fold change in CFUs from six sampled sites at a yoga studio after exposure to 0.5 ppm for 1 h on three consecutive nights over a weekend in a pilot application study. n.s., not significant. * p < 0.05, ** p < 0.01, *** p < 0.001, **** p < 0.0001.
4 Discussion
The findings from our study demonstrate the robust bactericidal efficacy of low-dose gaseous ozone against a variety of MDR, clinically relevant pathogens, highlighting its potential as a hands-free environmental disinfectant. Our results showed that a single potent dose of ozone was sufficient to achieve significant reductions in bacterial counts. The lack of enhanced killing with repeated exposures suggests that ozone’s bactericidal effect is dose-dependent rather than frequency-dependent.
Ozone’s microbial inactivation stems from its ability to induce microorganisms such as bacteria, fungi, and mold, to generate reactive oxygen species (ROS), which then attack their cell membranes, particularly the polyunsaturated fatty acids, leading to lipid peroxidation and microbial inactivation (Brié et al., 2018; Xue et al., 2023; Epelle et al., 2023; Elvis and Ekta, 2011; Agarwal et al., 2020; Moraes et al., 2021; Premjit et al., 2022; Roth et al., 2023; Neves et al., 2023; Pagès et al., 2020; Rangel et al., 2021). This effect is more evident in humidified ozone applications, where spore swelling occurs more severely, enhancing the passage of ROS and resulting in the collapse or rupture of the cell membrane (Epelle et al., 2023; Agarwal et al., 2020; Moraes et al., 2021; Premjit et al., 2022; Roth et al., 2023; Neves et al., 2023; Bhilwadikar et al., 2019; Tizaoui et al., 2022). When ozone is used against viruses, it damages their lipid envelopes and protein capsids, rendering them unable to infect hosts (Allison et al., 2009). This damage extends to the genome and RNA, impairing the virus’s ability to reproduce. Furthermore, the reaction with ROS can produce secondary reactive species, which can intensify the inactivation process (Farooq and Tizaoui, 2023).
A critical aspect of our study was evaluating the impact of environmental conditions on ozone’s efficacy. Previous studies have found mixed effects of microclimate on O3 (Pironti et al., 2021; Blanco et al., 2021; Grignani et al., 2020; Hudson et al., 2009). Our findings indicate that gaseous ozone maintains its bactericidal properties across various temperatures and humidity levels. Specifically, ozone was slightly more effective at colder temperatures (~4°C) compared to ambient temperature (~21–22°C), and higher humidity levels modestly improved killing, particularly for MRSA, though these differences were not statistically significant. Our findings are consistent with those of De Caro et al., which showed mild impacts of humidity and temperature on the bactericidal activity of O3 against E. coli (Pironti et al., 2021). Furthermore, we observed a slight but nonsignificant increase in microbicidal efficacy at higher humidity levels compared to low humidity, aligning with previous reports demonstrating maximal antiviral O3 activity against COVID-19 at high humidity (Hudson et al., 2009). Collectively, these results suggest that ozone can be an effective disinfectant in diverse environmental settings, including refrigerated environments. This is particularly relevant to the food industry, which continually combats mold and bacterial growth in walk-in refrigerators and refrigerated trucks where food is stored and transported. Regular low-dose gaseous ozone application to these spaces could reduce microbial contamination, prevent food spoilage, and result in cost savings.
The effectiveness of ozone against a broad range of clinical isolates, including A. baumannii, P. aeruginosa, and S. marcescens, underscores its potential application in healthcare settings to combat healthcare-associated infections (HAIs). The growing threat of MDR infections is difficult to overstate, with projections estimating 10 million deaths annually by 2050 if infection trends continue unchecked (O’Neil, 2014). Of the 22 new antibiotics approved between 2012 and 2022, only two were considered first-in-class, or novel, antibiotics (García-Castro et al., 2023), and five are no longer available (McKenna, 2020). Despite standardized cleaning protocols, a previously room occupancy by infected patients remains a major risk factor for HAIs due to the ability of MDR pathogens to leverage virulence factors that facilitate their persistence in highly inhospitable healthcare environments (Mitchell et al., 2023). Given the dwindling supply of antibiotics and limitations of current disinfection methods, ozone offers a promising alternative that could be integrated into infection control protocols in a cost-effective manner.
While gaseous O3 cannot completely replace manual cleaning, we envision its use in conjunction with current treatments to reduce the risk of HAIs and eliminate environmental reservoirs of nosocomial pathogens. O3 could be particularly useful in disinfecting hard-to-reach spaces or areas where manual cleaning is impractical. As a proof of concept, we conducted a pilot study in which an ozone generator was placed in a fitness studio. Three one-hour exposures to 0.5 ppm gaseous O3 on successive evenings reduced microbial counts by 2–3 log10-fold across multiple surfaces. These findings support the practical application of ozone and suggest it could be implemented in clinics, schools, and other high-risk settings.
While our study provides compelling evidence for the use of low-dose gaseous ozone as an environmental disinfectant, it also highlights areas for future research. Long-term studies assessing the safety and efficacy of continuous ozone use in occupied spaces are necessary to establish implementation guidelines. Additionally, exploring the synergistic effects of ozone with other disinfection methods could further extend its utility in various settings.
Data availability statement
The raw data supporting the conclusions of this article will be made available by the authors, without undue reservation.
Author contributions
BB: Conceptualization, Funding acquisition, Methodology, Writing – review & editing. CT: Formal analysis, Funding acquisition, Investigation, Writing – original draft, Writing – review & editing. VN: Conceptualization, Funding acquisition, Writing – original draft, Writing – review & editing. EB: Conceptualization, Formal analysis, Funding acquisition, Methodology, Writing – original draft, Writing – review & editing.
Funding
The author(s) declare that financial support was received for the research, authorship, and/or publication of this article. CT was supported by NIH Grant T32HD087978. EB was supported in part by an A.P. Giannini Postdoctoral Fellowship.
Acknowledgments
We thank the members of the Nizet lab for their scientific discussion.
Conflict of interest
BB was employed by Lumos Consulting.
The remaining authors declare that the research was conducted in the absence of any commercial or financial relationships that could be construed as a potential conflict of interest.
Publisher’s note
All claims expressed in this article are solely those of the authors and do not necessarily represent those of their affiliated organizations, or those of the publisher, the editors and the reviewers. Any product that may be evaluated in this article, or claim that may be made by its manufacturer, is not guaranteed or endorsed by the publisher.
References
Agarwal, S., Tyagi, P., Deshpande, A., Yadav, S., Jain, V., and Rana, K. S. (2020). Comparison of antimicrobial efficacy of aqueous ozone, green tea, and normal saline as irrigants in pulpectomy procedures of primary teeth. J. Indian Soc. Pedod. Prev. Dent. 38, 164–170. doi: 10.4103/JISPPD.JISPPD_119_20
Allison, K., Hook, J., Cardis, D., and Rice, R. G. (2009). Quantification of the bactericidal, fungicidal, and sporicidal efficacy of the JLA ltd. ozone laundering system. Ozone Sci. Eng. 31, 369–378. doi: 10.1080/01919510903155816
Bette, M., Cors, E., Kresse, C., and Schütz, B. (2022). Therapeutic treatment of superoxide dismutase 1 (G93A) amyotrophic lateral sclerosis model mice with medical ozone decelerates trigeminal motor neuron degeneration, attenuates microglial proliferation, and preserves monocyte levels in mesenteric lymph nodes. Int. J. Mol. Sci. 23:3403. doi: 10.3390/ijms23063403
Bhilwadikar, T., Pounraj, S., Manivannan, S., Rastogi, N. K., and Negi, P. S. (2019). Decontamination of microorganisms and pesticides from fresh fruits and vegetables: a comprehensive review from common household processes to modern techniques. Compr. Rev. Food Sci. Food Saf. 18, 1003–1038. doi: 10.1111/1541-4337.12453
Blanco, A., Ojembarrena, F. B., Clavo, B., Negro, C., Ojembarrena, F. D. B., Clavo, B., et al. (2021). Ozone potential to fight against SAR-COV-2 pandemic: facts and research needs. Environ. Sci. Pollut. Res. Int. 28, 16517–16531. doi: 10.1007/s11356-020-12036-9
Bobka, M. (1993). The 21 CFR (code of Federal Regulations) online database: Food and Drug Administration regulations full text. Med. Ref. Serv. Q. 12, 7–15. doi: 10.1300/J115V12N01_02
Bosi, E., Monk, J. M., Aziz, R. K., Fondi, M., Nizet, V., and Palsson, B. Ø. (2016). Comparative genome-scale modelling of Staphylococcus aureus strains identifies strain-specific metabolic capabilities linked to pathogenicity. Proc. Natl. Acad. Sci. USA 113, E3801–E3809. doi: 10.1073/pnas.1523199113
Brié, A., Boudaud, N., Mssihid, A., Loutreul, J., Bertrand, I., and Gantzer, C. (2018). Inactivation of murine norovirus and hepatitis a virus on fresh raspberries by gaseous ozone treatment. Food Microbiol. 70, 1–6. doi: 10.1016/j.fm.2017.08.010
Cantalejo, M. J., Zouaghi, F., and Pérez-Arnedo, I. (2016). Combined effects of ozone and freeze-drying on the shelf-life of broiler chicken meat. LWT Food Sci. Technol. 68, 400–407. doi: 10.1016/j.lwt.2015.12.058
Chatellier, S., Ihendyane, N., Kansal, R. G., Khambaty, F., Basma, H., Norrby-Teglund, A., et al. (2000). Genetic relatedness and superantigen expression in group a Streptococcus serotype M1 isolates from patients with severe and nonsevere invasive diseases. Infect. Immun. 68, 3523–3534. doi: 10.1128/IAI.68.6.3523-3534.2000
Cohen, B., Liu, J., Cohen, A. R., and Larson, E. (2018). Association between healthcare-associated infection and exposure to hospital roommates and previous bed occupants with the same organism. Infect. Control Hosp. Epidemiol. 39, 541–546. doi: 10.1017/ice.2018.22
Coll Cárdenas, F., Andrés, S., Giannuzzi, L., and Zaritzky, N. (2011). Antimicrobial action and effects on beef quality attributes of a gaseous ozone treatment at refrigeration temperatures. Food Control 22, 1442–1447. doi: 10.1016/j.foodcont.2011.03.006
Dahesh, S., Hensler, M. E., Van Sorge, N. M., Gertz, R. E. Jr., Schrag, S., Nizet, V., et al. (2008). Point mutation in the group B streptococcal pbp2x gene conferring decreased susceptibility to beta-lactam antibiotics. Antimicrob. Agents Chemother. 52, 2915–2918. doi: 10.1128/AAC.00461-08
Dancer, S. J. (2014). Controlling hospital-acquired infection: focus on the role of the environment and new technologies for decontamination. Clin. Microbiol. Rev. 27, 665–690. doi: 10.1128/CMR.00020-14
Datta, S. K., Okamoto, S., Hayashi, T., Shin, S. S., Mihajlov, I., Fermin, A., et al. (2006). Vaccination with irradiated Listeria induces protective T cell immunity. Immunity 25, 143–152. doi: 10.1016/j.immuni.2006.05.013
Ding, W., Jin, W., Cao, S., Zhou, X., Wang, C., Jiang, Q., et al. (2019). Ozone disinfection of chlorine-resistant bacteria in drinking water. Water Res. 160, 339–349. doi: 10.1016/j.watres.2019.05.014
Drees, M., Snydman, D. R., Schmid, C. H., Barefoot, L., Hansjosten, K., Vue, P. M., et al. (2008). Prior environmental contamination increases the risk of acquisition of vancomycin-resistant enterococci. Clin. Infect. Dis. 46, 678–685. doi: 10.1086/527394
Elvis, A. M., and Ekta, J. S. (2011). Ozone therapy: a clinical review. J. Nat. Sci. Biol. Med. 2, 66–70. doi: 10.4103/0976-9668.82319
Epelle, E. I., Macfarlane, A., Cusack, M., Burns, A., Okolie, J. A., Mackay, W., et al. (2023). Ozone application in different industries: a review of recent developments. Chem. Eng. J. 454:140188. doi: 10.1016/j.cej.2022.140188
Farooq, S., and Tizaoui, C. (2023). A critical review on the inactivation of surface and airborne SARS-CoV-2 virus by ozone gas. Crit. Rev. Environ. Sci. Technol. 53, 87–109. doi: 10.1080/10643389.2022.2043094
Fontes, B., Cattani Heimbecker, A. M., de Souza, B. G., Costa, S. F., van der Heijden, I. M., Levin, A. S., et al. (2012). Effect of low-dose gaseous ozone on pathogenic bacteria. BMC Infect. Dis. 12:358. doi: 10.1186/1471-2334-12-358
García-Castro, M., Sarabia, F., Díaz-Morilla, A., and López-Romero, J. M. (2023). Approved antibacterial drugs in the last 10 years: from the bench to the clinic. Explor. Drug Sci. 1, 180–209. doi: 10.37349/eds.2023.00013
Gonzalez, B. E., Martinez-Aguilar, G., Hulten, K. G., Hammerman, W. A., Coss-Bu, J., Avalos-Mishaan, A., et al. (2005). Severe staphylococcal sepsis in adolescents in the era of community-acquired methicillin-resistant Staphylococcus aureus. Pediatrics 115, 642–648. doi: 10.1542/peds.2004-2300
Grignani, E., Mansi, A., Cabella, R., Castellano, P., Tirabasso, A., Sisto, R., et al. (2020). Safe and effective use of ozone as air and surface disinfectant in the conjuncture of covid-19. Gases 1, 19–32. doi: 10.3390/gases1010002
Halverson, T., Mikolajczak, A., Mora, N., Silkaitis, C., and Stout, S. (2022). Impact of COVID-19 on hospital-acquired infections. Am. J. Infect. Control 50, 831–833. doi: 10.1016/j.ajic.2022.02.030
Harris, G., Kuo Lee, R., Lam, C. K., Kanzaki, G., Patel, G. B., Xu, H. H., et al. (2013). A mouse model of Acinetobacter baumannii-associated pneumonia using a clinically isolated hypervirulent strain. Antimicrob. Agents Chemother. 57, 3601–3613. doi: 10.1128/AAC.00944-13
Huang, S. S., Datta, R., and Platt, R. (2006). Risk of acquiring antibiotic-resistant bacteria from prior room occupants. Arch. Intern. Med. 166, 1945–1951. doi: 10.1001/archinte.166.18.1945
Hudson, J. B., Sharma, M., and Vimalanathan, S. (2009). Development of a practical method for using ozone gas as a virus decontaminating agent. Ozone Sci. Eng. 31, 216–223. doi: 10.1080/01919510902747969
Kim, H. S., Noh, S. U., Han, Y. W., Kim, K. M., Kang, H., Kim, H. O., et al. (2009). Therapeutic effects of topical application of ozone on acute cutaneous wound healing. J. Korean Med. Sci. 24, 368–374. doi: 10.3346/jkms.2009.24.3.368
Kuipers, O. P., Beerthuyzen, M. M., Siezen, R. J., and De Vos, W. M. (1993). Characterization of the nisin gene cluster nisABTCIPR of Lactococcus lactis. Requirement of expression of the nisA and nisI genes for development of immunity. Eur. J. Biochem. 216, 281–291. doi: 10.1111/j.1432-1033.1993.tb18143.x
Lin, L., Nonejuie, P., Munguia, J., Hollands, A., Olson, J., Dam, Q., et al. (2015). Azithromycin synergizes with cationic antimicrobial peptides to exert bactericidal and therapeutic activity against highly multidrug-resistant gram-negative bacterial pathogens. EBioMedicine 2, 690–698. doi: 10.1016/j.ebiom.2015.05.021
Liu, W.-T., Yang, Y.-L., Xu, Y., Lamsa, A., Haste, N. M., Yang, J. Y., et al. (2010). Imaging mass spectrometry of intraspecies metabolic exchange revealed the cannibalistic factors of Bacillus subtilis. Proc. Natl. Acad. Sci. USA 107, 16286–16290. doi: 10.1073/pnas.1008368107
Liu, L., Zeng, L., Gao, L., Zeng, J., and Lu, J. (2023). Ozone therapy for skin diseases: cellular and molecular mechanisms. Int. Wound J. 20, 2376–2385. doi: 10.1111/iwj.14060
Lourbopoulos, A. I., Mourouzis, I. S., Trikas, A. G., Tseti, I. K., and Pantos, C. I. (2021). Effects of thyroid hormone on tissue hypoxia: relevance to sepsis therapy. J. Clin. Med. Res. 10:5855. doi: 10.3390/jcm10245855
McKay, F. C., McArthur, J. D., Sanderson-Smith, M. L., Gardam, S., Currie, B. J., Sriprakash, K. S., et al. (2004). Plasminogen binding by group a streptococcal isolates from a region of hyperendemicity for streptococcal skin infection and a high incidence of invasive infection. Infect. Immun. 72, 364–370. doi: 10.1128/IAI.72.1.364-370.2004
McKenna, M. (2020). The antibiotic paradox: why companies can’t afford to create life-saving drugs. Nature 584, 338–341. doi: 10.1038/d41586-020-02418-x
Misawa, K., Nishimura, T., Kashimura, S., and Hasegawa, N. (2023). Inactivation of nontuberculous mycobacteria by gaseous ozone treatment. J. Infect. Chemother. 29, 628–630. doi: 10.1016/j.jiac.2023.03.004
Mitchell, B. G., McDonagh, J., Dancer, S. J., Ford, S., Sim, J., Thottiyil Sultanmuhammed Abdul Khadar, B., et al. (2023). Risk of organism acquisition from prior room occupants: an updated systematic review. Infect dis. Health 28, 290–297. doi: 10.1016/j.idh.2023.06.001
Moraes, M. M., Coelho, M. S., Nascimento, W. M., Nogales, C. G., de Campos, F. U. F., de Jesus, S. A., et al. (2021). The antimicrobial effect of different ozone protocols applied in severe curved canals contaminated with Enterococcus faecalis: ex vivo study. Odontology 109, 696–700. doi: 10.1007/s10266-021-00592-6
Neves, E. S., Ng, C. T., Pek, H. B., Goh, V. S. L., Mohamed, R., Osman, S., et al. (2023). Field trial assessing the antimicrobial decontamination efficacy of gaseous ozone in a public bus setting. Sci. Total Environ. 876:162704. doi: 10.1016/j.scitotenv.2023.162704
O’Neil, J. (2014). Review on antimicrobial resistance. Antimicrobial resistance: tackling a crisis for the health and wealth of nations. London: Review on Antimicrobial Resistance.
Ozone Equipment Manufacturer and Ozone System Integrators. Ozone conversions and calculations ozone integration experts. (2024). Available at: https://www.oxidationtech.com/ozone/ozone-calculations.html (Accessed June 3, 2024).
Pagès, M., Kleiber, D., and Violleau, F. (2020). Ozonation of three different fungal conidia associated with apple disease: importance of spore surface and membrane phospholipid oxidation. Food Sci. Nutr. 8, 5292–5297. doi: 10.1002/fsn3.1618
Pironti, C., Moccia, G., Motta, O., Boccia, G., Franci, G., Santoro, E., et al. (2021). The influence of microclimate conditions on ozone disinfection efficacy in working places. Environ. Sci. Pollut. Res. Int. 28, 64687–64692. doi: 10.1007/s11356-021-15457-2
Popovich, K. J., Calfee, D. P., Patel, P. K., Lassiter, S., Rolle, A. J., Hung, L., et al. (2019). The Centers for Disease Control and Prevention STRIVE initiative: construction of a national program to reduce health care–associated infections at the local level. Ann. Intern. Med. 171, S2–S6. doi: 10.7326/M18-3529
Porter, L., Sultan, O., Mitchell, B. G., Jenney, A., Kiernan, M., Brewster, D. J., et al. (2024). How long do nosocomial pathogens persist on inanimate surfaces? A scoping review. J. Hosp. Infect. 147, 25–31. doi: 10.1016/j.jhin.2024.01.023
Premjit, Y., Sruthi, N. U., Pandiselvam, R., and Kothakota, A. (2022). Aqueous ozone: chemistry, physiochemical properties, microbial inactivation, factors influencing antimicrobial effectiveness, and application in food. Compr. Rev. Food Sci. Food Saf. 21, 1054–1085. doi: 10.1111/1541-4337.12886
Rahme, L. G., Stevens, E. J., Wolfort, S. F., Shao, J., Tompkins, R. G., and Ausubel, F. M. (1995). Common virulence factors for bacterial pathogenicity in plants and animals. Science 268, 1899–1902. doi: 10.1126/science.7604262
Rangel, K., Cabral, F. O., Lechuga, G. C., Carvalho, J. P. R. S., Villas-Bôas, M. H. S., Midlej, V., et al. (2021). Detrimental effect of ozone on pathogenic bacteria. Microorganisms 10:40. doi: 10.3390/microorganisms10010040
Roth, A., Krishnakumar, A., and Rahimi, R. (2023). Ozone as a topical treatment for infected dermal wounds. Front. Biosci. 15:9. doi: 10.31083/j.fbe1502009
Rutala, W. A., and Weber, D. J. (2015). “Disinfection, sterilization, and control of hospital waste” in Mandell, Douglas, and Bennett’s principles and practice of infectious diseases. eds. Bennett, J. E., Dolin, R., and Blaser, M. J., Elsevier Inc., 3294–3309.
Shen, X., Su, Y., Hua, Z., Sheng, L., Mendoza, M., He, Y., et al. (2021). Effectiveness of low-dose continuous gaseous ozone in controlling Listeria innocua on red delicious apples during 9-month commercial cold storage. Front. Microbiol. 12:712757. doi: 10.3389/fmicb.2021.712757
Sheppeard, R., Mitchell, C., Woo, W., and Simmonds, R. (2022). A suspicious skin nodule with dilated chest veins: the importance of a full skin examination. Clin. Exp. Dermatol. 47, 1721–1722. doi: 10.1111/ced.15252
Singh, S. A., Suresh, S., and Vellapandian, C. (2023). Ozone-induced neurotoxicity: in vitro and in vivo evidence. Ageing Res. Rev. 91:102045. doi: 10.1016/j.arr.2023.102045
Son, M. S., Megli, C. J., Kovacikova, G., Qadri, F., and Taylor, R. K. (2011). Characterization of Vibrio cholerae O1 El Tor biotype variant clinical isolates from Bangladesh and Haiti, including a molecular genetic analysis of virulence genes. J. Clin. Microbiol. 49, 3739–3749. doi: 10.1128/JCM.01286-11
Tizaoui, C., Stanton, R., Statkute, E., Rubina, A., Lester-Card, E., Lewis, A., et al. (2022). Ozone for SARS-CoV-2 inactivation on surfaces and in liquid cell culture media. J. Hazard. Mater. 428:128251. doi: 10.1016/j.jhazmat.2022.128251
Tran, T. T., Panesso, D., Gao, H., Roh, J. H., Munita, J. M., Reyes, J., et al. (2013). Whole-genome analysis of a daptomycin-susceptible Enterococcus faecium strain and its daptomycin-resistant variant arising during therapy. Antimicrob. Agents Chemother. 57, 261–268. doi: 10.1128/AAC.01454-12
Welch, R. A., Burland, V., Plunkett, G. 3rd, Redford, P., Roesch, P., Rasko, D., et al. (2002). Extensive mosaic structure revealed by the complete genome sequence of uropathogenic Escherichia coli. Proc. Natl. Acad. Sci. USA 99, 17020–17024. doi: 10.1073/pnas.252529799
Westover, C., Rahmatulloev, S., Danko, D., Afshin, E. E., O’Hara, N. B., Ounit, R., et al. (2022). Ozone disinfection for elimination of bacteria and degradation of SARS-CoV2 RNA for medical environments. Genes 14:85. doi: 10.3390/genes14010085
Xue, W., Macleod, J., and Blaxland, J. (2023). The use of ozone technology to control microorganism growth, enhance foods afety and extend shelf life: a promising food decontamination technology. Food Secur. 12:814. doi: 10.3390/foods12040814
Keywords: gaseous ozone disinfection, multidrug-resistant bacteria, alternatives to antibiotics, low dose ozone, environmental decontamination
Citation: Banerjee B, Thompson C, Nizet V and Bjånes E (2024) Bactericidal efficacy of low dose gaseous ozone against clinically relevant multidrug-resistant bacteria. Front. Microbiol. 15:1480433. doi: 10.3389/fmicb.2024.1480433
Edited by:
Fu Qiao, Sichuan University, ChinaReviewed by:
Erica De Vita, University of Pisa, ItalyMohamad Hamad, University of Sharjah, United Arab Emirates
Copyright © 2024 Banerjee, Thompson, Nizet and Bjånes. This is an open-access article distributed under the terms of the Creative Commons Attribution License (CC BY). The use, distribution or reproduction in other forums is permitted, provided the original author(s) and the copyright owner(s) are credited and that the original publication in this journal is cited, in accordance with accepted academic practice. No use, distribution or reproduction is permitted which does not comply with these terms.
*Correspondence: Elisabet Bjånes, ZWJqYW5lc0BoZWFsdGgudWNzZC5lZHU=; Victor Nizet, dm5pemV0QGhlYWx0aC51Y3NkLmVkdQ==
‡Present address: Christine Thompson, Children’s Hospital of Los Angeles, Los Angeles, CA, United States
†These authors have contributed equally to this work and share first authorship