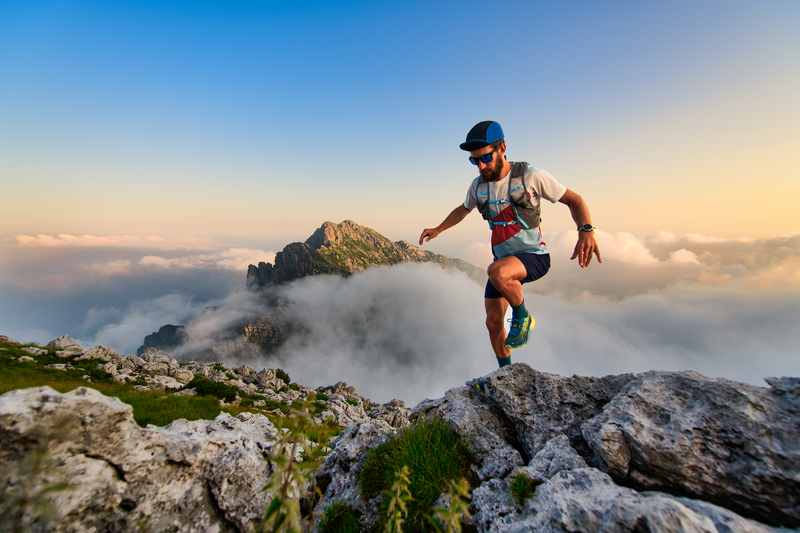
95% of researchers rate our articles as excellent or good
Learn more about the work of our research integrity team to safeguard the quality of each article we publish.
Find out more
ORIGINAL RESEARCH article
Front. Microbiol. , 28 September 2023
Sec. Food Microbiology
Volume 14 - 2023 | https://doi.org/10.3389/fmicb.2023.1253480
Spore-forming probiotic bacteria offer interesting properties as they have an intrinsic high stability, and when consumed, they are able to survive the adverse conditions encountered during the transit thorough the host gastrointestinal (GI) tract. A traditional healthy food, nattō, exists in Japan consisting of soy fermented by the spore-forming bacterium Bacillus subtilis natto. The consumption of nattō is linked to many beneficial health effects, including the prevention of high blood pressure, osteoporosis, and cardiovascular-associated disease. We hypothesize that the bacterium B. subtilis natto plays a key role in the beneficial effects of nattō for humans. Here, we present the isolation of B. subtilis DG101 from nattō and its characterization as a novel spore-forming probiotic strain for human consumption. B. subtilis DG101 was non-hemolytic and showed high tolerance to lysozyme, low pH, bile salts, and a strong adherence ability to extracellular matrix proteins (i.e., fibronectin and collagen), demonstrating its potential application for competitive exclusion of pathogens. B. subtilis DG101 forms robust liquid and solid biofilms and expresses several extracellular enzymes with activity against food diet-associated macromolecules (i.e., proteins, lipids, and polysaccharides) that would be important to improve food diet digestion by the host. B. subtilis DG101 was able to grow in the presence of toxic metals (i.e., chromium, cadmium, and arsenic) and decreased their bioavailability, a feature that points to this probiotic as an interesting agent for bioremediation in cases of food and water poisoning with metals. In addition, B. subtilis DG101 was sensitive to antibiotics commonly used to treat infections in medical settings, and at the same time, it showed a potent antimicrobial effect against pathogenic bacteria and fungi. In mammalians (i.e., rats), B. subtilis DG101 colonized the GI tract, and improved the lipid and protein serum homeostasis of animals fed on the base of a normal- or a deficient-diet regime (dietary restriction). In the animal model for longevity studies, Caenorhabditis elegans, B. subtilis DG101 significantly increased the animal lifespan and prevented its age-related behavioral decay. Overall, these results demonstrate that B. subtilis DG101 is the key component of nattō with interesting probiotic properties to improve and protect human health.
Probiotics are live microorganisms that produce beneficial effects on the host’s health when consumed in the recommended amounts (Raya et al., 2002; Hill et al., 2014). One essential attribute of a probiotic is the proficiency to arrive active (i.e., alive) at the site of action (e.g., the gastrointestinal, GI, tract) (Binda et al., 2020). Typical probiotics are represented by lactic acid bacteria (LAB), such as certain strains belonging to the genus Lactobacilli, Streptococci and Bifidobacterium (Haro et al., 2018; Lemme-Dumit et al., 2018). The beneficial properties of probiotic LAB on humans have some limitations related to the LAB lability and susceptibility to environmental stressors (e.g., temperature changes, dehydration, and the hostile gut environment) (Duary et al., 2011; Pedraza-Reyes et al., 2012; Botta et al., 2014; Gomathi et al., 2014). Other types of human probiotic bacteria that successfully overcome these concerns are represented by spore-forming bacteria of the Bacillus genus (e.g., Bacillus subtilis, Bacillus coagulans, and Bacillus clausii) (Sanders et al., 2003; Kalman et al., 2009; Cutting, 2011; Hanifi et al., 2015; Piewngam et al., 2018; Piewngam and Otto, 2020; Freedman et al., 2021; Rodriguez Ayala et al., 2021; Zeng et al., 2021). These probiotic bacilli possess the ability to form resistant spores protecting them from hostile environmental conditions and preserving their viability as probiotics (De Vecchi and Drago, 2006; Hong et al., 2008; Cutting, 2011; Binda et al., 2020). Interestingly, recent reports have shown the compatibility between probiotic LAB and bacilli, with a focus on the protective role of the bacilli biofilm on LAB survival and functionality (Zhang et al., 2013; VidyaLaxme et al., 2014; Yang et al., 2015; Yahav et al., 2018).
In Asian and African countries, there are several bacilli-fermented foods that produce beneficial effects on human health (Dimidi et al., 2019). One of these healthy fermented foods is nattō, a traditional Japanese food made from whole soybeans that have been fermented with a specific variety of B. subtilis (i.e., B. subtilis var. natto) (Sumi et al., 1987; Ueda, 1989; Fujita et al., 1993; Osawa and Matsumoto, 1997; Fujita et al., 2022). B. subtilis natto produces several molecules associated with human health (Su et al., 2020), including large amounts of vitamin K2 (menaquinone-7), important for bone robustness (Ikeda and Doi, 1990; Tsukamoto et al., 2000; Hu et al., 2020; Wu et al., 2021); pyrroloquinoline quinone (PQQ), a natural antioxidant with energizing and anti-fatigue effects (Nakano et al., 2012, 2015); nattokinase, a protease conferring protection against high blood pressure (Sumi et al., 1987; Fujita et al., 1993; Kim et al., 2008), ischemic stroke (Urano et al., 2001; Hsu et al., 2009; Murakami et al., 2012; Ji et al., 2014; Pham et al., 2020; Suzuki et al., 2023), cardiovascular disease (Urano et al., 2001; Hsu et al., 2009; Murakami et al., 2012; Weng et al., 2017; Suzuki et al., 2023), with anti-neurodegenerative (Hsu et al., 2009; Kumar et al., 2017) and anti-celiac effects (Wei et al., 2016); and cutaneous wound healing properties (Zhang et al., 2019). In addition, B. subtilis produces natural antimicrobials (lipopeptide antibiotics) that demonstrated activity against pathogenic bacteria (Ongena et al., 2005; Cao et al., 2009; Piewngam et al., 2018; Piewngam and Otto, 2020), fungi (Yu et al., 2002; Cawoy et al., 2015; Bartolini et al., 2019), viruses (Itokawa et al., 1994; Vollenbroich et al., 1997; Jung et al., 2000), and cancer cells (Nagata et al., 2002; Wang et al., 2007; Cao et al., 2009; Myung et al., 2009; Zhang et al., 2015; Lu et al., 2017). Therefore, it is not surprising that the regular consumption of nattō (and other soy-fermented foods) is linked to better life quality and lower risk of death (Nagata et al., 2002; Myung et al., 2009; Wu et al., 2012; Zhang et al., 2015; Lu et al., 2017; Sunagawa et al., 2018; Woo et al., 2018; Katagiri et al., 2020; Abe et al., 2022). In particular, the consumption of nattō is related to a significant prevention of cardiovascular disease (Zhang et al., 2003; Kokubo et al., 2007; Nagata et al., 2017; Yan et al., 2017; Katagiri et al., 2020; Rodriguez Ayala et al., 2021; Abe et al., 2022). We envision that the bacterium B. subtilis present in nattō plays a key role in the human beneficial effects of this fermented food (Nagata et al., 2017; Woo et al., 2018; Katagiri et al., 2020). Here, we show that the key human-beneficial component of nattō is the bacterium B. subtilis var. natto (i.e., B. subtilis DG101).
To isolate B. subtilis from nattō, 250 g of nattō were heated at 80°C for 20 min to inactivate (kill) vegetative bacteria and leave only spores as viable cells (Lombardía et al., 2006). Aliquots from serial dilutions of heat-treated nattō were seeded on Luria-Bertani agar (LBA) plates and incubated for 36 h at 37°C. This procedure was repeated three times independently and in all the repetitions only one type of heat-resistant bacterial isolate was obtained. These nattō isolates and the laboratory B. subtilis 168 strains were cultured on Luria-Bertani (LB) broth except when another broth medium is indicated (Pedrido et al., 2013). The growth media for the other bacteria and fungi used in this work were: trypticase soy broth (TSB) for Staphylococcus aureus; brain heart infusion (BHI) broth for Enterococcus faecalis and Listeria monocytogenes; Chapman broth for MR S. aureus; MacConkey broth for Salmonella enterica, Salmonella typhimurium, and Klebsiella pneumoniae; MacConkey Agar with Sorbitol for EHEC O157:H7; thiosulfate-citrate-bile salts-sucrose (TCBS) broth for Vibrio cholerae; King A broth for Pseudomonas aeruginosa; and potato dextrose agar (PDA) for fungi growth. Schaeffer’s sporulation medium (SM) was used to obtain B. subtilis spores (Pedrido et al., 2013). The efficiency of spore formation was measured by counting viable vegetative and spore cells before and after heat treatment at 80°C, respectively, as previously described (Pedrido et al., 2013). To prepare cell suspensions, B. subtilis (168 and DG101 strains) and Escherichia coli DH5α (used as negative control) were grown in SM or LB broth, respectively, at 37°C with shaking (7,258 × g) during 24 h. The B. subtilis cultures were heated at 80°C for 20 min to kill cells that did not form spores. Then, the bacterial cultures (i.e., E. coli and B. subtilis) were centrifuged (3,578 × g, 15 min at 4°C) and the pellets were brought to a final concentration of 5 × 108 colony forming units per milliliter (CFU/mL) in tris buffer solution (10 mM; pH 7.0) and used immediately.
The presumptive B. subtilis natto isolate was microbiologically characterized using routine nutritional and biochemical tests (see Results section). The molecular typification was performed by the Sequencing Service, Molecular Biology Department of The Pasteur Institute of Montevideo, Uruguay.1 Briefly, a sample of chromosomal DNA from the presumptive B. subtilis natto isolate was used for PCR amplification using consensus primers for the 16S ribosomal RNA gene from the B. subtilis group. The sequences of the used forward and reverse primers were 5′-AGAGTTTGATCMTGGCTC-3′ and 5′-ATACTAGCGACTCCGACTTC-3′, respectively. The amplification product (~1,500 bp) was purified, sequenced, and compared with the sequences deposited in GenBank using the BLAST tool2 and in the Ribosomal Database Project.3 A phylogenetic tree was constructed based on 16S rDNA sequences retrieved from the GenBank database of bacilli and LAB (i.e., Lactobacillus and Bifidobacterium). The best match of the isolate from nattō was with the 16S ribosomal coding gene of the B. subtilis natto BEST195 strain. The 1,407 bp DNA sequence corresponding to the 16S ribosomal gene of the new nattō isolate was deposited in GenBank under the accession number OQ813498.4
Five mL aliquots of B. subtilis DG101 or E. coli DH5α (5 × 108 CFU/mL) were independently incubated in the presence of 0, 50, 100, 150, 200, and 250 μ/mL of lysozyme at 37°C. After 20 min (Zheng et al., 1988; Kuwana et al., 2005), an aliquot of each bacterial suspension was taken, and serial dilutions were made in 0.1 M phosphate-buffered saline (PBS) pH 6.2. The corresponding dilutions were seeded with a Drygalsky spatula in LB agar (LBA) plates and after 24 h of incubation at 37°C, the viable cells (CFU) were counted.
To determine the pH resistance of the studied bacteria (B. subtilis DG101 and E. coli DH5α), 10 mL of each bacterial suspension were centrifuged at 3,578 × g for 10 min at 4°C and the cell pellet was reconstituted in the same original volume of sterile distilled water (5 × 108 CFU/mL). The suspensions were brought to different pH (i.e., 1.5, 2.5, and 3.5) with 0.1 M HCl. The bacterial samples were incubated at 37°C for 2 h, considering this is an estimated/average physiological time of gastric emptying with a pH range of 1.5–3.5 (Hellmig et al., 2006; Beasley et al., 2015). The control pH test was carried out at pH 6.2 in 0.1 M PBS. Following the incubation times, serial dilutions of the cells were made in 0.1 M PBS (pH 6.2) to neutralize the acidity of the medium (when necessary), and different aliquots were seeded with a Drygalsky spatula in LBA plates. After 24 h of incubation at 37°C, the viable cells (CFU) were counted. The survival rate was calculated as the percentage of CFU growing in LBA compared to the initial concentration of cells, before exposition to the different pH (Hyronimus et al., 2000).
Briefly, the temperature and salt tolerance assays were performed in 50 mL bottles filled with 10 mL of LB broth supplemented with NaCl at 0.5, 2, 5, 7, and 10%, and B. subtilis DG101 (5 × 105 CFU/mL). Samples were grown at 30, 37, 42, 50, and 54°C with shaking (7.258 × g) for 24 h. After the overnight growth, different dilutions of the salt and temperature-treated cultures were seeded with a Drygalsky spatula in LBA plates and after 24 h of incubation at 37°C, the viable cells (CFU) were counted. The culture that grew in unmodified LB (i.e., LB containing 0.5% NaCl) at 30°C was considered as the control and the final growth yield of the remaining cultures was expressed as a % of the growth yield of the control culture (7.5 × 108 CFU/mL).
Five mL of B. subtilis DG101 or E. coli DH5α suspensions (5 × 108 CFU/mL) were centrifuged at 3,578 × g for 10 min and each pellet was re-suspended in 10 mL of 0.3% bile salt solution (50% sodium cholate and 50% sodium deoxycholate) or in sterile distilled water as a control (Duc et al., 2003). The prepared suspensions were incubated at 37°C for 0, 1, 2, 3, and 4 h. Next, aliquots were taken, and serial dilutions were made in 0.1 M PBS pH 6.2. The corresponding dilutions were seeded with a Drygalsky spatula in LBA plates and after 24 h of incubation at 37°C, the viable cells (CFU) were counted. The survival rate was calculated as the percentage of CFU obtained in LBA compared to the initial cell concentration. The InfoStat 2016 and Sigmaplot 10.0 programs were used to perform the graphs.
B. subtilis DG101 was screened for the production of exoenzymes related to the digestion of nutrients of different natures. Briefly, B. subtilis DG101 was streaked on LBA plates containing one of the following nutrient substrates: gelatin (LBA-gelatin 10 g/L as indicator of protease activity), pectin (LBA-pectine 5 g/L for peptinolytic activity), carboxymethylcellulose (LBA-CMC 10 g/L for cellulolytic activity), Tween-80 (LBA-Tween 80 15 mL/L for lipolytic activity), pythate (LBA-phytate 5 g/L for hydrolytic activity related to release of soluble phosphate), and starch (LBA-soluble starch 10 g/L for amylase activity). The Petri dishes streaked with B. subtilis DG101 were incubated at 37°C for 36 h, and the development of a clear zone around the colonies was indicative of the presence of exoenzyme activity (Lee et al., 2005; Hossain et al., 2020). For the determination of hemolytic activity, suspensions of B. subtilis DG101 were streaked onto blood agar plates (5%–10% sheep blood per plate) and incubated for 24 h at 37°C. The isolates were then examined for the presence of clear zones surrounding the colonies. Clear zones are considered as beta hemolysis, greenish zones as alpha hemolysis, and the absence of hemolysis zones is known as gamma hemolysis (Gerhardt, 1994).
B. subtilis DG101 was incubated in LB broth supplemented with the corresponding metal: cadmium (Cd(II)), chromium (Cr(VI)), or arsenite (As(III)) at 0, 10, 50, 100, 150, 200, 250, and 300 ppm at 37°C with shaking (5 × g). After 20 h, the bacterial cultures were centrifuged and the determination of metal concentration was performed on the cell-free supernatants (i.e., remaining metal in the supernatant). The concentration of Cr(VI) was determined by a colorimetric reaction based on the formation of a complex between Cr(VI) and 2,4-difenylcarbazide in an acid solution to a λ = 540 nm, as previously described (Megharaj et al., 2003). The concentration of As(V) was determined by a colorimetric reaction based on the formation of a red complex, between arsenic hydride with silver diethyl-carbamate, to a λ = 530 nm (Upadhyay et al., 2018). Total chromium (Cr(VI) plus Cr(III)), Cd(II), and As (As(V) plus As(III)) were determined by atomic absorption spectrophotometric determination at λ = 357.3 nm, λ = 228.8 nm, and λ = 193.7 nm, respectively (Nakahara et al., 1973; Megharaj et al., 2003; Liu et al., 2006; Cheng and Li, 2009; Guibaud et al., 2009; Singh and Singh, 2014; Upadhyay et al., 2018).
The selected methodology to test for antimicrobial activity of B. subtilis DG101 against microbial pathogens was adapted from the agar diffusion method (Bauer et al., 1959, 1966; Bonev et al., 2008). Briefly, the antimicrobial activity of B. subtilis DG101 against pathogenic bacteria and fungi (Candida albicans, Fusarium verticillioides, Fusarium graminearum, Aspergillus flavus, Penicillium sp.) was performed on nutrient agar (NA) or PDA plates, respectively. Then, 100 μL of overnight cultures of the different microbial pathogens to be tested were individually poured, using a Drygalsky spatula, on the corresponding type of agar plate (NA or PDA plates). After the seeded microorganisms were totally absorbed in the medium, three sterile paper disks (4 mm in diameter) pre-absorbed with 15 μL of an overnight culture of B. subtilis DG101 grew on LB broth (~2 ×1 06 CFU/paper disk) were loaded equidistantly from each other on each type of agar plate. The NA and PDA plates containing the bacterial and fungi pathogens, respectively, with the B. subtilis DG101-poured paper disks were incubated for 24 h at 37°C or 72–96 h at 30°C, respectively. The clear zone formed around the paper disks indicated the growth inhibition of the tested pathogen. The inhibition halo of each bacterial pathogen produced by B. subtilis DG101 was measured and used to classify the pathogen as sensitive or resistant based on the recommendations of the American Society for Microbiology for antimicrobial control.5 For the determination of susceptibility of fungal pathogens to B. subtilis DG101, the fungal growth inhibition index was calculated from measurements of fungal radial growth toward (X1) versus perpendicular to (X2) the bacterial colony according to the formula [1 − (X1/X2)] *100 (Quecine et al., 2015; Bartolini et al., 2019). Paper disks pre-absorbed with sterile water were used as negative controls of antimicrobial activity.
The antibiotic sensitivity of B. subtilis DG101 was tested against the following antibiotics commonly used in hospitals: amikacin, ampicillin/sulbactam, cephalothin, cefepime, cefoxitin, ceftazidime, cilastatin, ciprofloxacin, erythromycin, gentamicin, imipenem, nitrofurantoin, norfloxacin, oxacillin, penicillin, piperacillin/tazobactam, tetracycline, trimethoprim/sulfamethoxazole and vancomycin. All the selected antibiotics are on the lists of recommended antibiotics by the U.S. Food and Drug Administration (FDA), the Center for Drug Evaluation and Research (CDER), the Clinical and Laboratory Standards Institute (CLSI), and The European Committee on Antimicrobial Susceptibility Testing (EUCAST). Briefly, commercial disks containing an appropriate amount of each antibiotic were added to Mueller Hinton agar plates, previously poured with B. subtilis DG101, and incubated under aerobic conditions at 37°C for 24–48 h. The inhibition zones (clear zone, in mm) were measured using a caliper. All experiments were performed in triplicate on the same day. The breakpoint values used for categorizing the microorganisms as resistant were according to the Clinical and Laboratory Standards Institute (2023).
For biofilm formation, B. subtilis DG101 and B. subtilis 168 cultures were grown in LB medium until they reached the stationary phase. Then, 50 μL of each culture was diluted in 2 mL of fresh LBY medium (LB broth supplemented with 4.0% yeast extract; Pedrido et al., 2013) and statically grown at 37°C for 36 h. For quantification of formed biofilm (biofilm mass), we measured the biofilm mass that adhered to the surface of the microtiter plate wells (Pedrido et al., 2013). To this end, B. subtilis (DG101 or 168) cells were grown in 96-well polyvinylchloride (PVC) microtiter plates at 37°C for 36 h in LBY medium. The bacterial inoculum for the microtiter plates was obtained by growing the cells in LB to mid-exponential phase and then diluting the cells to an OD600 nm of 0.08 in LBY and biofilm formation was monitored by staining with crystal violet (CV) as described by Pedrido et al. (2013). Growth medium and non-adherent cells were carefully removed from the microtiter plate wells. The microtiter plate wells were incubated at 60°C for 30 min. Biofilms were stained with 300 μL of 0.3% CV in 30% methanol at room temperature for 30 min. Excess CV was removed, and the wells were rinsed with water. The CV from stained biofilms was then solubilized in 200 μL of 80% ethanol/20% acetone. Biofilm formation was quantified by measuring the absorbance at 595 nm for each well using a Beckman Coulter Multimode Detector (DTX 880).
Briefly, microtiter plates were coated with 100 μL of each solution containing the following extracellular matrix (ECM) proteins (Sigma-Aldrich): fibronectin, collagen, and gelatine (10 μg/mL in bicarbonate buffer pH 9.6); and subsequently incubated overnight at 4°C. The unfixed proteins were removed, and plates were washed three times with PBS pH 8. After blocking with 0.5% non-fat milk (in PBS), to prevent non-specific bacterial binding, wells were washed again twice with PBS. Finally, bacterial suspensions of B. subtilis DG101 or B. subtilis 168 (100 μL, 1×108 CFU/mL) were added and the plates were incubated on an orbital shaker for 2 h at 37°C. Unbound bacteria were removed by washing the wells five times with PBS. Adhered bacteria to the wells were fixed at 60°C for 20 min and stained with 100 μL/well of CV (0.3% in methanol—H2O 30% v/v) for 45 min as previously described (Styriak et al., 1999; Rodriguez Ayala et al., 2017a). The wells were subsequently washed extensively with PBS to remove the unbound stain. After adding 100 μL of citrate buffer pH 4.3 to each well and 45 min incubation at room temperature (to release the stain bound to bacteria), the absorbance values (A595 nm) were determined in a Multiscan enzyme-linked immunosorbent assay reader as described (Sun et al., 2019). The average values of three independent experiments performed in quadruplicate were calculated. The specificity of binding was tested using BSA as the immobilized protein. Data are expressed as the mean absorbance value (A570 nm), with non-specific binding corrected by subtraction.
The colonization of the GI tract by B. subtilis DG101 was performed according to Hoa et al. (2001). Briefly, B. subtilis DG101 eliminated in feces was monitored in Sprague Dawley rats (male, 6 to 8 weeks old, n = 12) housed individually in cages with gridded floors. Animals were deprived of drinking water for 6 h and then fed on a single dose of 2 × 107 spores of B. subtilis DG101 contained in a volume of 20 mL of drinking water. After this procedure, the animals were fed ad libitum with an unrestricted drinking water supply. Total feces were collected day to day and weighed for 2 weeks. To determine the total number of B. subtilis DG101 viable cells, including spores and vegetative cells in feces, samples were suspended in 10 to 30 mL of 0.1 M PBS with frequent and rigorous vortexing. Serial dilutions were made with PBS, seeded on LBA plates, and incubated at 37°C for 2 days. B. subtilis DG101 colonies were identified by their characteristic colony morphology (see Figure 1). To determine the total number of B. subtilis DG101 spores, the same procedure was used, except that feces were suspended in 0.1 M PBS and incubated at 80°C for 20 min to inactivate (i.e., kill) vegetative cells. The total CFU count was extrapolated for the total weight of feces collected and represented as a percentage of the initial dose.
Figure 1. Growth of Bacillus subtilis DG101 on LB agar media. Morphological appearance of B. subtilis DG101 isolated from nattō food after incubation for 24 h at 37°C on LB agar (A) compared to the domesticated and non-probiotic strain B. subtilis 168 (B). Shown are the scale bars.
Three-weeks-old Wistar male rats (n = 14) were housed in metal cages (2 animals/cage) in a temperature-controlled room under a 12 h light/dark cycle. After a 1 week adaptation period on powder chow diet (Gepsa Feed Corporation), the animals were randomly assigned to 2 groups (n = 7): Control group (rats fed ad libitum on powder chow) and Treated group (rats fed ad libitum on powder chow supplemented with B. subtilis DG101 1 × 1010 CFU/kg of food). On day 120, blood samples were drawn from the tail rats. Serum was separated by centrifuging the blood at 7,500 × g for 15 min. Triglyceride (TG, Cat. 1780101), total cholesterol (TC, Cat. 1220101), high-density lipoprotein-cholesterol (HDL-c, Cat. 1220103), and low-density lipoprotein-cholesterol (LDL-c, Cat. 1220108) were measured using enzymatic reagent kits from Wiener Lab (Wiener Group Co, Argentina).
For protein quantification under normal and dietary restriction conditions, 20 male Wistar rats, 3 weeks old, were housed in metal cages (2 animals/cage) in a temperature-controlled room under a 12 h light/dark cycle. After a 1 week adaptation period on a powder chow diet, the rats were randomly assigned to 4 groups (n = 5): Control group (rats fed ad libitum, consuming an average amount of 26 g of food/day/animal), Treated group (rats fed ad libitum and supplemented with B. subtilis DG101 1 × 1010 spores/kg of food), Nutritional stress group (rats with a dietary restriction that consisted of ~75% of the average amount of food consumed by day −19.5 g of food/day/animal-under physiological conditions), and Treated nutritional stress group (rats with dietary restriction supplemented with B. subtilis DG101 1 × 1010 spores/kg of food). After 90 days, blood samples were drawn from the tail rats. Serum was separated by centrifuging the blood at 7,500 × g for 15 min. Total proteins (TP, Cat. 1999736), albumin (ALB, Cat. 1009240), and globulins (TG, Cat. 1690004) were measured using enzymatic reagent kits from Wiener Lab. All aspects of the experiment were conducted according to the guidelines provided by the ethical committee of experimental animal care at the National University of Rosario (Santa Fe, Argentina).
Lifespans for the wild-type C. elegans N2 strain (Bristol strain) were monitored at 20°C as previously described (Cogliati et al., 2020). Briefly, worm embryos were isolated by exposing hermaphrodite adult worms to alkaline hypochlorite treatment for 3 min, and synchronized eggs were allowed to develop. In all cases, L4/young adult worms (n = 100) were used at time zero for lifespan analysis; they were transferred to fresh nematode growth medium agar (NGMA) plates previously seeded with E. coli OP50 or B. subtilis DG101 or B. subtilis 168 cells each day until the death of the last worm of each assay (Cogliati et al., 2020). Worms were considered dead when they ceased pharyngeal pumping and did not respond to prodding with a platinum wire. Worms with internal hatching were removed from the plates and excluded from lifespan calculations.
B. subtilis 168- or B. subtilis DG101-fed N2 (10 days-old adults) worms were collected, washed three times with M9 buffer (Cogliati et al., 2020), and seeded in 10 cm Petri dishes prepared with NGMA without food for 1 h. Then, approximately 75 worms were placed in the center of 6 cm plates prepared with NGMA. After all the animals were transferred to the center of the assay plates, 2 μL of attractant or repellent were seeded 2 cm from the center of the plate, and 2 μL of solvent (control) in which the attractant or repellent was diluted were seeded equidistantly. Both the attractant, repellent, and solvent (control) were added with a 1-μL drop of 1 M azide. The plates were incubated for 1 h at 25°C. Then, worms found at each end of the plates were counted, and the chemotaxis index (CI) was calculated (Bargmann et al., 1993). The attractant compounds used for the assays were 0.5% diacetyl (DA, Sigma-Aldrich) diluted in ethanol and 1 mM isoamyl alcohol (IAA; Sigma-Aldrich) diluted in water, 1 mM 2,3-pentanedione and 1 mM 2-butanone (Sigma-Aldrich). The repellent compounds used for the assays were 0.3% octanol and 15% 2-nonanone (Sigma-Aldrich). The CI was defined as the number of worms at the attractant or repellent location—the number of worms at the control location divided by the total number of worms on the plate (Bargmann et al., 1993).
All experiments were performed in triplicate and the mean values along with the standard deviation are given. All the data from laboratory experiments were analyzed separately for each experiment and were subjected to an analysis of variance (ANOVA test). Significant effects were determined by the F value (p ≤ 0.05). For C. elegans survival assays, experiments were performed at least three times in duplicate. Mean survival days, standard error of the mean (S.E.M.), intervals of mean survival days with 95% confidence, and equality p-values to compare averages were calculated by log-rank and Kaplan–Meier tests using the OASIS program. The S.E.M. values are used in the figures; p < 0.1 was considered statistically significant.
The genetic pathways (e.g., the phosphorelay signaling and the Spo0A/SinR/AbrB/LuxS regulatory circuit) controlling the multicellular behavior of the B. subtilis natto strain described here have been previously reported (Lombardía et al., 2006; Pedrido et al., 2013; Grau et al., 2015: Rodriguez Ayala et al., 2017a). Now, we present a short discussion of the biofilm, sliding, and adherence proficiencies of the natto isolate plus a profound and complete description of its in vitro and in vivo probiotic properties. The bacterial nattō isolate (see Material and methods) showed a complex colony architecture, resembling the sophisticated colony (biofilm) architecture of wild (undomesticated) B. subtilis isolates (Branda et al., 2001), (Figure 1A). Accordingly, the biofilm physiognomy of the nattō isolate remarkably differed from the simple and undifferentiated colony (biofilm) architecture of domesticated B. subtilis isolates (Branda et al., 2001), i.e., 168 strain (Figure 1B). The confirmation that the nattō isolate belongs to B. subtilis was achieved through Sanger sequencing and alignment of the 16S ribosomal RNA (rRNA) encoding gene (Figure 2, see Material and methods for details). The nattō isolate exhibited strong homologies (~99.7% similarity and ~99.5% identity over its full length) to the 16S rRNA sequences belonging to different B. subtilis strains, and significant homology with other Gram-positive probiotic bacteria of the genus Lactobacilli and Bifidobacterium (Figure 2). Interestingly, the 16S rRNA gene of the nattō isolate showed the strongest homology with the BEST 195 B. subtilis natto strain previously characterized (Kamada et al., 2014). Therefore, the nattō isolate (Figures 1A, 2) was given initially the name Bacillus subtilis RG4365 (Roberto Grau; Lombardía et al., 2006; Pedrido et al., 2013; Grau et al., 2015; Rodriguez Ayala et al., 2017a) and more recently, because of its approval for consumption as a human probiotic, B. subtilis DG101 (Doctor Grau; Cardinali et al., 2020; Rodriguez Ayala et al., 2021, 2022a, 2022b). Both strain designations (i.e., RG4365 and DG101) refers to the same nattō strain. The 1,407-base sequence fragment of the 16S rRNA gene of B. subtilis DG101 was deposited in GenBank (accession number OQ813498).
Figure 2. Phylogenetic relationship of the 16S rRNA gene of B. subtilis DG101 within representative Bacillaceae bacteria. The genes coding for the 16S rRNA were used to generate a neighbor-joining tree using crustal omega (www.ebi.ac.uk/tools/msa/clustalo). The phylogenetic distance with a gene encoding for 16S rRNA of Bifidobacterium and Lactobacillus is also shown, forming two independent clusters. The numbers are Bootstrap numbers, ranging from 0 to 1, with 0 showing complete identity and no identity by 1.
B. subtilis DG101 was initially characterized by Gram staining, sporulation proficiency, and basic biochemical and physiological tests whose results correlated well with the expected biochemistry and physiology attributes of bacilli (Table 1A). B. subtilis DG101 was proficient to grow in the presence of high salt concentrations at different growth temperatures (Table 1B) and in the production of several exoenzymes (Table 1C) involved in the digestion of diverse types of macromolecules (i.e., polysaccharides, complex lipids, proteins). The in-situ production of these exoenzymes in the host intestine could play a positive role in improving food diet digestion. Importantly, B. subtilis DG101 lacked hemolysis activity (Table 1C) and was sensitive to antibiotics routinely used to treat infections (Table 1D).
B. subtilis DG101 was able to grow in the presence of high concentrations of toxic metals (i.e., Cd (II), Cr(VI), or As(III)) (Figure 3). The minimal inhibitory concentrations (MICs) of B. subtilis DG101 growth in the presence of each metal were ~ 300 ppm for Cd (II) and Cr(VI); and ~250 ppm for As(III) (Figure 3A). These MIC values were significantly higher than the metal limits allowed to be present in drinking water: 5 ppb, 5 ppm, and 10 ppb for Cd (II), Cr(VI) or As(III), respectively (Nakahara et al., 1973; Singh and Singh, 2002; Megharaj et al., 2003; Liu et al., 2006; Cheng and Li, 2009; Guibaud et al., 2009; Upadhyay et al., 2018). The proficiency of B. subtilis DG101 to grow satisfactorily in the presence of 50 ppm of each metal (Figure 3A) correlated with the significant removal of Cd (II), Cr(VI), and As(III) from the liquid phase of the culture (i.e., cell-free supernatant) (Figure 3B). The presence of Cd (II), Cr(VI), and As(III) in the cell-free supernatant of B. subtilis DG101 that grew overnight in the presence of 50 ppm of each metal was reduced more than ~80%, ~ 60%, and ~85%, respectively (Figure 3B). The growth of B. subtilis DG101 in the presence of each metal correlated with the absorption of Cd (II) to the cell envelope, and the reduction or oxidation of Cr(VI) to Cr(III) and As(III) to As(V), respectively (data not shown). The proficiency of B. subtilis DG101 to transform toxic metals into less hazardous species in humans (i.e., conversion of Cr(VI) to Cr(III) and As(III) to As(V)) or a less available form (absorption of Cd(II) to the cell envelope) opens the potential use of this probiotic bacilli for environmental bioremediation uses and/or prevention/treatment of food/water metal poisoning.
Figure 3. Bioremediation of toxic heavy metals Cd (II), Cr (VI), and As (III) by B. subtilis DG101. (A) Effect of different concentrations of Cd(II) (black bar), Cr(VI) (grey bar), and As(III) (white bar) on the final yield growth of B. subtilis DG101 after 24 h of cultivation in LB broth, with or without metal supplementation. (B) Percentage of metal detoxification after 24 h of B. subtilis DG101 grown in LB broth supplemented with 50 ppm de each metal (Cr(VI), Cd(II) or As(III)). See Material and methods for details.
The growth rate (μ) of B. subtilis DG101 cultured in LB broth with shaking at 37°C was ~0.0231 min−1 with a generation time (tg) of 30 min (Figure 4A). This growth showed two distinctive features that distinguish B. subtilis DG101 from other B. subtilis strains previously described (e.g., strains 168 and NCIB3610; Branda et al., 2001). First, as a key difference with the swimming proficiency of the B. subtilis 168 and NCIB3610 strains (Branda et al., 2001), B. subtilis DG101 was not motile in the liquid medium and this phenotype correlated with the absence of visible flagella after sample staining with a specific dye for flagella visualization (Table 1A and data not shown). Associated with the observed non-motility phenotype in liquid broth with shaking, B. subtilis DG101 showed a tendency to form long strings (longer than 50 μm each) constituted of individual cells lined up one after the other (Figure 4B). The tendency to form long cords of cells was observed during the entire log phase of growth and reached the maximal expression of cords formation at the transition from T−1 to T0 (the end of the log phase of growth, Figure 4B). At this developmental time (T0) in LB broth, a small portion (~ 15%) of the culture initiated the sporulation program, and later (approximately 2–3 h after T0) the cellular cords were completely dissembled, and free vegetative cells and sporangia (similar to the ones of B. subtilis 168 or B. subtilis NCIB3610, data not shown) were released into the culture medium (data not shown). Surprisingly, B. subtilis DG101 was able to perform surface-associated motility similar to the flagella-dependent swarming motility exhibited by B. subtilis NCIB3610 (Branda et al., 2001) but by a flagella-independent mechanism of sliding motility (Grau et al., 2015; Figure 4C).
Figure 4. Growth behavior of B. subtilis DG101 in liquid and in solid media at 37°C. (A) Growth curve of B. subtilis DG101 in LB broth with shaking. (B) Pictures of the B. subtilis DG101 cellular cords observed during the log phase of growth (pictures were taken at the time indicated by the arrow in A). Scale bar is 10 μm. (C) Surface-associated motility of B. subtilis DG101 on soft LB agar plates. B. subtilis DG101 cells were cultured and inoculated on LB-0.7% agar plates and the surface translocation of the expanding cellular swarm was photographed at different times as indicated. The control showed the inability of B. subtilis 168 to swarm on LB-0.7% agar plates (bottom picture). Scale bar is 1 cm. (D) Liquid biofilm (pellicle) development of B. subtilis DG101 and B. subtilis 168 seeded in microplates containing LBY broth. Top-view pictures were taken after 20 h of incubation without agitation. Scale bar is 1 mm. Right panel, mass quantification of the biofilms produced by B. subtilis 168 and B. subtilis DG101 after 24 h of incubation (see Material and methods for details). (E) Solid biofilm (colony) development of B. subtilis DG101 on LBY agar plates. Panoramic appearance of typical B. subtilis DG101 colonies (biofilms) developed after 24 h of incubation are shown. Scale bar is 1 mm. (F) Proficiency of B. subtilis DG101 and B. subtilis 168 to bind to representative proteins of the extracellular matrix of tissues. Immobilized collagen (left panel), fibronectin (middle panel), gelatin (right panel), or BSA (not shown) were incubated in the presence of B. subtilis DG101 or B. subtilis 168 as indicated. Each data point contains the mean ± S.E.M. of a representative experiment performed by quadruplicate. NS: no significant difference, ***p < 0.001 (ANOVA with Bonferroni test).
Under sessile conditions of growth (i.e., without shaking) in liquid and solid medium, B. subtilis DG101, but not B. subtilis 168, formed (liquid and solid) robust, sophisticated, and long-lasting hydrophobic biofilms (Branda et al., 2004; Pedrido et al., 2013; Grau et al., 2015; and Figures 4D,E). The proficiency in biofilm formation depended on the adherence ability of the bacterium to different substrates (Pedrido et al., 2013). For example, B. subtilis DG101 showed a strong and specific adherence to diverse proteins present in the extracellular matrix of the human intestinal mucosa (e.g., collagen and fibronectin), an adhesive property that would be important for the competitive exclusion of pathogen adherence (VidyaLaxme et al., 2014; Rodriguez Ayala et al., 2017a; and Figure 4F).
The antimicrobial activity of B. subtilis DG101 was assessed by measuring the growth inhibition zone diameter of different pathogenic bacteria and fungi that grew in the presence of the probiotic (see Material and methods for details). The results shown in Table 2 indicate the effectiveness of B. subtilis DG101 in controlling the growth of pathogenic Gram-positive and Gram-negative bacteria (Table 2A) as well as the proficiency of the probiotic to inhibit the growth of yeasts and mycotoxin-producer filamentous fungi (Table 2B).
A probiotic bacterium must be able to remain alive in the hostile environment of the host GI tract (Cutting, 2011). To this end, the probiotic must resist the lytic activity of the lysozyme during its transit through the buccal cavity and the low pH and bile salts present in the stomach and intestine, respectively (Zheng et al., 1988; Duc et al., 2003; Kuwana et al., 2005; De Vecchi and Drago, 2006; Hellmig et al., 2006; Beasley et al., 2015). As shown in Figure 5A, B. subtilis DG101 spores were resistant to the lysozyme’s hydrolysis at similar concentrations present or secreted in the human buccal cavity (Figure 5A), the low pH that resembled the exposure and transit through the stomach acidity (Figure 5B), and bile salts concentrations that correlated with physiological bile concentrations present in the human GI tract (Figure 5C).
Figure 5. Survival of B. subtilis DG101 to stressful conditions of the gastrointestinal tract. (A) Effect of lysozyme. E. coli DH5α (white circles) and B. subtilis DG101 (black circles) suspensions (5 × 108 CFU/mL) in PBS buffer were incubated in the presence of different lysozyme concentrations at 37°C for 20 min. After 20 min, the survival % for each strain was calculated. (B) Effect of pH. E. coli DH5α and B. subtilis DG101 (5 × 108 CFU/mL) were exposed to different pH before to determine the survival expresses as %. (C) Effect of bile salts. B. subtilis DG101 and E. coli DH5α suspensions (5 × 108 CFU/mL) were incubated in PBS buffer with and without (black and with circles, respectively) 0.3% bile salts (BS) and the survival expresses as % was determined at different times. (D) In vivo gut colonization. Persistence of B. subtilis DG101 in the feces of male Sprague Dawley rats fed with a single dose of B. subtilis DG101 at time zero. For (A–D), the average % values of three independent repetitions ± S.E.M. (n = 3) are shown. See Material and methods for details.
The in vitro stress resistance of B. subtilis DG101 to GI-simulated conditions (Figures 5A–C) correlated well with its proficiency in in vivo gut colonization in animal models (Figure 5D). In this assay, 60 days-old Sprague Dawley male rats were fed with a single dose of 2 × 107 spores of B. subtilis DG101 present in drinking water (20 mL), and the fecal elimination of the probiotic strain was quantified day to day for 2 weeks (see Material and methods for details). B. subtilis DG101 started to be present in the feces of inoculated animals approximately 15 h after the feeding, and after ~40 h, the CFU of B. subtilis DG101 in animal feces passed the initial inoculum of the probiotic (Figure 5D), indicating that the ingested spores were able to germinate and grow (data not shown). After 2 weeks, the total CFU present in the feces of B. subtilis DG101-fed animals (a total of 3.0 × 108 CFU, 10% vegetative cells, and 90% spores, data not shown) exceeded by ~1.5 log the total CFU of B. subtilis DG101 ingested with the drinking water (2.0 × 107 CFU). Interestingly, the B. subtilis DG101-fed animals continued with the fecal elimination of the probiotic (spores and vegetative cells, data not shown) at a rate of ~1 × 103 to 5 × 103 CFU/gram of fecal matter during 3 months after the end of the experiment (the CFU of spore-forming indigenous bacilli different to B. subtilis DG101 in rat feces was around 1 × 101 to 1 × 102 CFU/gram of fecal matter, data not shown). Overall, these results show that B. subtilis DG101 is able to persist, grow, and colonize the GI tract.
To analyze the potential beneficial effects of B. subtilis DG101 on blood biochemical parameters, the food of Sprague Dawley male rats, from day 1 after weaning, was supplemented with a daily dose of 1 × 1010 CFU of B. subtilis DG101/kg food (treated group). After 120 days, blood samples were taken from the animal tails to analyze and compare the lipid levels present in the serum with the lipid levels present in animals of similar age fed without the probiotic supplementation (control group). The analysis included the determination of triglycerides, total cholesterol, HDL cholesterol, and LDL cholesterol. As shown in Figure 6A, the animals of the treated group (compared with the control group animals) did not modify their triglyceride content although they showed a significant reduction of the levels of total cholesterol and LDL cholesterol (“bad cholesterol”). Accordingly, the animals of the treated group showed higher serum levels of HDL cholesterol (“good cholesterol”) compared with the control group animals (Figure 6A). These results provide experimental evidence of the beneficial effect of B. subtilis DG101 on serum lipid homeostasis.
Figure 6. Beneficial effect of B. subtilis DG101 on lipid and protein serum homeostasis in an animal model. (A) B. subtilis DG101 significantly decreases total cholesterol and LDL-cholesterol levels and increases HDL-cholesterol levels in the serum of male rats (Sprague Dawley) fed with B. subtilis DG101 supplementation. (B-D) B. subtilis DG101 improves serum protein levels in animals under physiological and nutritional stress (dietary restriction) conditions. Figures show the level of total protein (B), albumin (C), and globulins (D), present in the sera of 120 days-old Wistar rats fed with or without B. subtilis DG101 supplementation, see Material and methods for details. The bars represent the average of 2 determinations carried out each in duplicate ± S.E.M. (n = 5 rats per group). Asterisks indicate statistical significance (**p < 0.01 ***p < 0.001; ns, no significant difference, p > 0.5).
Next, it was interesting to analyze the performance of B. subtilis DG101-fed animals under nutritional stress conditions (dietary restriction). It is known that dietary restriction (or caloric restriction) represents nutritional stress that decreases the level of serum proteins (Alexopoulos et al., 2004). Therefore, we were intrigued to evaluate if B. subtilis DG101 could manage this nutritional stress (e.g., maintenance of a physiologic level of plasmatic proteins) to benefit host health. To answer this question, 30 days-old male Wistar rats were divided into four groups as indicated in the Material and methods section. As shown in Figures 6B,C, the subgroup of animals fed with the supplement of B. subtilis DG101 and under nutritional stress showed higher levels of total serum proteins and albumin than the respective group of animals under nutritional stress and without B. subtilis DG101 supplementation (p < 0.01). The other main protein component of the serum, apart from albumin, are the globulins which are divided into alpha-1, alpha-2, beta, and gamma globulins (Abbas et al., 2011). As shown in Figure 6D, the serum concentration of globulins of rats complemented in their diet with B. subtilis DG101 was significantly higher in animals with or without nutritional stress. Interestingly, there were no significant differences in the levels of total serum proteins, albumin, and globulins in B. subtilis DG101-fed animals without nutritional stress and B. subtilis DG101-fed animals under nutritional stress (Figures 6B–D). These findings suggest that hosts colonized by B. subtilis DG101 were well-equipped to respond to the stressful condition of nutrient limitation. Overall, these results provide experimental evidence of the beneficial effect of B. subtilis DG101 on the maintenance of serum protein levels in healthy individuals under physiological conditions of growth or subjected to nutritional stress.
One interesting property of some probiotic strains is the attribute to extend the host lifespan in different animal models (e.g., flies, mice, and worms), and we wondered if B. subtilis DG101 would possess this anti-aging property. To this end, we assayed the lifespan extension of the animal model Caenorhabditis elegans (Cogliati et al., 2020) when its gut was colonized by different bacterial strains including B. subtilis DG101. One-day-old wild-type worms of the Bristol N2 strain were grown on NGMA plates seeded with E. coli OP50 or B. subtilis DG101 (the ingested bacteria that survived the transit from the worm grinder to the gut constituted the gut flora of the animal). The NGMA plates seeded with the different bacteria were incubated at 20°C and the worm survival was monitored every day as described (see Material and methods and Donato et al., 2017). As shown in Figure 7A, N2 worms fed on B. subtilis DG101 gained ~45% in lifespan extension (mean survival 33.22 ± 0.82 days) when compared with the lifespan extension of the N2 worms colonized by the lab routine and the non-probiotic strain E. coli OP50 used to feed C. elegans (mean survival 18.37 ± 0.63 days, p < 0.001). Figure 7A also shows the lifespan extension of N2 worms fed the domesticated and non-probiotic B. subtilis 168 (mean survival 20.34 ± 0.71 days) compared with the lifespan of N2 worms fed E. coli OP50 (p < 0.001). Importantly, B. subtilis 168 and E. coli OP50 were much less effective than B. subtilis DG101 in prolonging the life expectancy of C. elegans when colonizing its gut.
Figure 7. B. subtilis DG101 extends the C. elegans lifespan and improves its behavioral response. (A) C. elegans longevity fed on B. subtilis DG101 cells. Fifty late L4/young-adult-stage N2 worms were fed on B. subtilis DG101 or B. subtilis 168 cells (black squares and grey triangles, respectively) on NGM agar plates, and survival was monitored. The OP50 E. coli strain (white circles) was used as a control. The survival graph is the average ± S.E.M. (n = 3), and the survival increase is expressed as a percentage of the total number of worms fed on OP50 E. coli. (B) Chemotaxis indexes (CI) for N2 worms to diacetyl (DA), isoamyl alcohol (IA), 2,3-pentanedione, 2-butanone (attractants); octanol, and 2-nonanone (repellents) scored for 1 h. A typical result out of three independent experiments (performed in duplicate) is presented. Bars represent the CI response of N2 worms to each odorant ± S.E.M. Asterisks indicate statistical significance **p < 0.01; ***p < 0.001; ns, no significant difference, p > 0.5.
In addition to an extension of the host life expectancy, a healthy life extension (i.e., a host aging accompanied by a delay in the behavioral decay associated with the age progression) is also desirable (Cogliati et al., 2020). One sensory behavior we examined was chemotaxis. The age-synchronized wild-type control strain (N2) of C. elegans was cultured at 20°C from egg hatching, using B. subtilis 168 or B. subtilis DG101 as a food source. Then, worms of 10 days of age, were starved of food (168 or DG101) for 1 h before to compare their chemotactic response toward the attractants. As shown in Figure 7B, B. subtilis 168-colonized N2 worms exhibited a normal chemotactic response toward DA (CI = 0.69 ± 0.02; n = 75), IAA (CI = 0.75 ± 0.02; n = 75), 2,3-pentadione (CI = 0.65 ± 0.01; n = 75), and 2-butanone (CI = 0.41 ± 0.02; n = 75) (Figure 7B, attractants, white bars). Importantly, the colonization of C. elegans gut by B. subtilis DG101 significantly improved the behavioral responses (chemotaxis index) of this host model organism to the chemical attractants [DA (CI = 092 ± 0.01; n = 75), IAA (CI = 0.88 ± 0.02; n = 75), 2,3-pentadione (CI = 0.90 ± 0.02; n = 75), and 2-butanone (CI = 071 ± 0.03; n = 75)] (Figure 7B, attractants, black bars).
Accordingly, N2 worms fed on B. subtilis DG101 significantly improved the response repellent stimuli [2-nonanone (CI = −0.71 ± 0.01; n = 75), and octanol (CI = −0.91 ± 0.02; n = 75) (Figure 7B, repellents, white bars)] when compared with worms colonized by B. subtilis 168 2-nonanone (CI = −0.41 ± 0.02; n = 75) and octanol (CI = −0.66 ± 0.01; n = 75) (Figure 7B, repellents, black bars). Therefore, B. subtilis DG101 possesses the potential attribute to extend the host’s healthy lifespan.
Probiotic bacteria do not always produce in vivo beneficial effects for the host. The ability to reach and colonize (in the desired number) the GI tract is sometimes compromised (Duary et al., 2011; Botta et al., 2014; Gomathi et al., 2014). For example, LAB probiotics have shown differences in their ability to survive GI conditions depending on the source of their isolation, preservation form, or packaging (e.g., powdered, or liquid form, microencapsulated, lyophilized) (Ozawa et al., 2012). Also, the accessibility of alive probiotic bacteria to the gut, and the subsequent adhesion (i.e., adherence to extracellular matrix components of the mucosa) and colonization (e.g., favored by the proficiency in biofilm formation), are important for probiotics to colonize the host gut and produce their beneficial effects (Horie et al., 2005; VidyaLaxme et al., 2014; Volstatova et al., 2016; Rodriguez Ayala et al., 2017a).
Here, we show that B. subtilis DG101 (Figures 1, 2) expresses many desirable attributes of a probiotic, e.g., antibiotic susceptibility, antimicrobial activity, proficiency in biofilm formation, translocation over surfaces, and adherence to ECM proteins (Figure 4 and Tables 1, 2). One expected consequence of these B. subtilis DG101 attributes is the protection and increment of the beneficial portion of the indigenous gut flora (i.e., LAB). In this regard, we and others have demonstrated that B. subtilis is able to improve the growth and survival of LAB probiotics because of the proficiency of B. subtilis to form biofilms protecting LAB from the stressful gut environment (Zhang et al., 2013; VidyaLaxme et al., 2014; Yang et al., 2015; Yahav et al., 2018).
B. subtilis DG101 possesses an extra beneficial effect on the LAB population. It has been previously reported that the ability of B. subtilis natto to degrade starch (i.e., proficiency in amylase activity, Table 1C) provides LAB (unable to degrade starch) with the simple sugars needed for their metabolism and energy production (Horie et al., 2018). B. subtilis DG101 would be able to collaborate in food digestion and host metabolism because of the plethora of other hydrolytic exoenzymes with activity against macromolecules (e.g., proteins, lipids, and polysaccharides) present in the regular human diet (Table 1C). The beneficial gut-associated attributes of B. subtilis DG101 could be the reasons for the recognized efficacy of B. subtilis in alleviating GI discomfort, gas production, and bloating, and the improvement of the production of healthy polyunsaturated fatty acids in the gut (VidyaLaxme et al., 2014; Penet et al., 2021; Garvey et al., 2022).
B. subtilis DG101 shows the potential for the detoxification (i.e., bioremediation) of metal-contaminated foods or drinking water (Alotaibi et al., 2012) because of its proficiency in growing in the presence of toxic heavy metals (CdII, CrVI, and AsIII) and turning them into less toxic (transformation of CrVI and AsIII into CrIII and AsV, respectively) or less available forms (absorbed CdII) to impair the host intestinal absorption (Figures 3A,B).
B. subtilis DG101 possesses a high tolerance for growth at high temperatures in the presence of high salt concentrations (Table 1B), however, it lacks hemolytic activity (Table 1C and data not shown) and antimicrobial resistance (Table 1E). B. subtilis DG101 expresses a potent antimicrobial activity against pathogenic bacteria and fungi of medical concern (Table 2). The in vitro antimicrobial activity of B. subtilis DG101 (Table 2) correlates with the reported human protection against pathogenic Salmonella enterica and Staphylococcus aureus because of the production of natural lipopeptide antibiotics by B. subtilis (Piewngam et al., 2018; Piewngam and Otto, 2020).
Besides the demonstrated desirable attributes of B. subtilis DG101 (Figures 1–4 and Tables 2 and 1), this strain is able to survive the hostile environmental conditions (e.g., exposure to lysozyme, low pH, and bile salts) that it would encounter during transit through and colonization of the GI tract (Figure 5). In addition, B. subtilis DG101 was able to improve lipid homeostasis (e.g., decrease LDL and increase HDL levels) and the protein concentrations (e.g., albumin and globulins) present in the serum of animals fed with a supplement of the probiotic bacterium. These healthy lipid and serum protein patterns were maintained in animals exposed to nutritional stress (Figure 6). The in vitro and in vivo proficiencies of B. subtilis DG101 correlates well with the recently reported anti-type 2 diabetes mellitus and anti-obesity effects of B. subtilis DG01 in humans (Patterson et al., 2016; Cardinali et al., 2020; Rodriguez Ayala et al., 2022a, 2022b).
A high and regular human intake of nattō, but not total soy or isoflavonoids intake, has been associated with a lower risk of high blood pressure and stroke (Kim et al., 2008; Yan et al., 2017), and, importantly, with a significant reduction (~10%) of any cause of natural death, in particular cardiovascular disease (CVD) mortality (Zhang et al., 2003; Kokubo et al., 2007; Nagata et al., 2017; Yan et al., 2017; Katagiri et al., 2020; Rodriguez Ayala et al., 2021; Abe et al., 2022). The human beneficial compounds produced by B. subtilis DG101 and present in nattō food (e.g., nattoquinase, PQQ, vitamin K2, and lipopeptide antibiotics) and the results shown here, led us to hypothesize that the nattō-making bacterium B. subtilis DG101 can play an important role in the reported human healthy prolongevity effect of this traditional food (Rodriguez Ayala et al., 2017b, 2021). The proof of concept of this hypothesis was the antiage and healthy cognitive effects produced in C. elegans colonized by B. subtilis DG101 compared with the life expectancy of worms colonized by the non-probiotic bacteria E. coli OP50 or B. subtilis 168 (Figure 7).
The datasets presented in this study can be found in online repositories. The names of the repository/repositories and accession number(s) can be found below: https://www.ncbi.nlm.nih.gov/genbank/, OQ813498.
The animal study was approved by Comité de ética Universidad Nacional de Rosario. The study was conducted in accordance with the local legislation and institutional requirements.
CL: Investigation, Methodology, Writing – original draft. FR: Investigation, Methodology, Writing – original draft. AG: Investigation, Methodology, Writing – original draft. LR: Investigation, Methodology, Writing – original draft. AN: Conceptualization, Methodology, Writing – original draft. RG: Conceptualization, Funding acquisition, Supervision, Validation, Writing – review & editing.
This work was supported by FONCyT (Fondo para la Investigación Científica y Tecnológica) of Argentina and the Pew Latin-American Program in Biological Sciences (Philadelphia, United States).
The authors would like to thank all former lab members (especially Ester Sabal, Marcela Salvarrey, Gustavo Rosso, Carlos Bauman, Tamara Spalding and Lucía Speroni) for thoughtful discussions and technical assistance.
The authors declare that the research was conducted in the absence of any commercial or financial relationships that could be construed as a potential conflict of interest.
All claims expressed in this article are solely those of the authors and do not necessarily represent those of their affiliated organizations, or those of the publisher, the editors and the reviewers. Any product that may be evaluated in this article, or claim that may be made by its manufacturer, is not guaranteed or endorsed by the publisher.
The Supplementary material for this article can be found online at: https://www.frontiersin.org/articles/10.3389/fmicb.2023.1253480/full#supplementary-material
1. ^https://pasteur.uy/en/units/molecular-biology
2. ^www.ncbi.nlm.nih.gov/blast
4. ^https://www.ncbi.nlm.nih.gov/nuccore/OQ813498
5. ^https://asm.org/Articles/2022/February/Updating-Breakpoints-in-Antimicrobial-Susceptibili
Abbas, A., Lichtman, A., and Pillai, S. (2011). Cellular and molecular inmunology. Elsevier Saunders. Philadelphia, PA. 19–46.
Abe, C., Imai, T., Sezaki, A., Miyamoto, K., Kawase, F., Shirai, Y., et al. (2022). Global association between traditional Japanese diet score and all-cause, cardiovascular disease, and total cancer mortality: a cross-sectional and longitudinal ecological study. J. Am. Nutr. Assoc. 11, 1–8. doi: 10.1080/27697061.2022.2130472
Alotaibi, B., Khan, M., and Shamin, S. (2012). Unraveling the underlying heavy metal detoxification mechanisms of Bacillus species. Microorganisms 9:1628. doi: 10.3390/microorganisms9081628
Alexopoulos, C., Georgoulakis, I. E., Tzivara, A., Kritas, S. K., Siochu, A., Kyriakis, S. C., et al. (2004). Field evaluation of the efficacy of a probiotic containing Bacillus licheniformis and Bacillus subtilis spores, on the health status and performance of sows and their litters. J Anim Physiol Anim Nutr (Berl). 88, 381–92.
Bargmann, C. I., Hartwieg, E., and Horvitz, H. R. (1993). Odorant-selective genes and neurons mediate olfaction in C. elegans. Cells 74, 515–527. doi: 10.1016/0092-8674(93)80053-H
Bartolini, M., Cogliati, S., Vileta, D., Bauman, C., Ramirez, W., and Grau, R. (2019). Stress-responsive alternative sigma factor SigB plays a positive role in the antifungal proficiency of Bacillus subtilis. Appl. Environ. Microbiol. 85, e00178–e00119. doi: 10.1128/AEM.00178-19
Bauer, A. W., Kirby, W. M., Sherris, J. C., and Turck, M. (1966). Antibiotic susceptibility testing by a standardized single disk method. Am. J. Clin. Pathol. 45, 493–496. doi: 10.1093/ajcp/45.4_ts.493
Bauer, A. W., Perry, D. M., and Kirby, W. M. (1959). Single-disk antibiotic-sensitivity testing of staphylococci: an analysis of technique and results. Arch. Intern. Med. 104, 208–216. doi: 10.1001/archinte.1959.00270080034004
Beasley, D. E., Koltz, A. M., Lambert, J. E., Fierer, N., and Dunn, R. R. (2015). The evolution of stomach acidity and its relevance to the human microbiome. PLoS One 10:e0134116. doi: 10.1371/journal.pone.0134116
Binda, S., Hill, C., Johansen, E., Obis, D., Pot, B., Sanders, M. E., et al. (2020). Criteria to qualify microorganisms as “probiotic” in foods and dietary supplements. Front. Microbiol. 11:1662. doi: 10.3389/fmicb.2020.01662
Bonev, B., Hooper, J., and Parisot, J. (2008). Principles of assessing bacterial susceptibility to antibiotics using the agar diffusion method. J. Antimicrob. Chemother. 61, 1295–1301. doi: 10.1093/jac/dkn090
Botta, C., Langerholc, T., Cenci, A., and Cocolin, L. (2014). In vitro selection and characterization of new probiotic candidates from table olive microbiota. PLoS One 9:e94457. doi: 10.1371/journal.pone.0094457
Branda, S. S., González-Pastor, J. E., Ben-Yehuda, S., Losick, R., and Kolter, R. (2001). Fruiting body formation by Bacillus subtilis. Proc. Natl. Acad. Sci. U. S. A. 98, 11621–11626. doi: 10.1073/pnas.191384198
Branda, S. S., González-Pastor, J. E., Dervyn, E., Ehrlich, S. D., Losick, R., and Kolter, R. (2004). Genes involved in formation of structured multicellular communities by Bacillus subtilis. J. Bacteriol. 186, 3970–3979. doi: 10.1128/JB.186.12.3970-3979.2004
Cao, X.-H., Liao, Z.-Y., Wang, C.-L., Yang, W.-Y., and Lu, M.-F. (2009). Evaluation of a lipopeptide biosurfactant from Bacillus natto TK-1 as a potential source of anti-adhesive, antimicrobial, and antitumor activities. Braz. J. Microbiol. 40, 373–379. doi: 10.1590/S1517-83822009000200030
Cardinali, N., Bauman, C., Rodriguez Ayala, F., and Grau, R. (2020). Two cases of type 2 diabetes mellitus successfully treated with probiotics. Clin. Case Rep. 8, 3119–3124. doi: 10.1002/ccr3.3354
Cawoy, H., Debois, D., Franzil, L., De Pauw, E., Thonart, P., and Ongena, M. (2015). Lipopeptides as main ingredients for inhibition of fungal phytopathogens by Bacillus subtilis/amyloliquefaciens: lipopeptides as inhibitors of phytopathogens. Mol. Microbiol. 8, 281–295. doi: 10.1111/1751-7915.12238
Cheng, G., and Li, X. (2009). Bioreduction of chromium (VI) by Bacillus sp. isolated from soils of iron mineral area. Eur. J. Soil Biol. 42, 483–487. doi: 10.1016/j.ejsobi.2009.06.009
Clinical and Laboratory Standards Institute. (2023). Performance standards for antimicrobial susceptibility testing CLSI document M100. 33. Wayne, PA: Clinical and Laboratory Standards Institute (CLSI); (2023)
Cogliati, S., Clementi, V., Francisco, M., Crespo, C., Argañaraz, F., and Grau, R. (2020). Bacillus subtilis delays neurodegeneration and behavioral impairment in the Alzheimer’s disease model Caenorhabditis Elegans. J. Alzheimers Dis. 73, 1035–1052. doi: 10.3233/JAD-190837
Cutting, S. M. (2011). Bacillus probiotics. Food Microbiol. 28, 214–220. doi: 10.1016/j.fm.2010.03.007
De Vecchi, E., and Drago, L. (2006). Lactobacillus sporogenes or Bacillus coagulans: misidentification or mislabeling? Int. J. Probiotics Prebiotics 1, 3–10. doi: 10.1179/joc.2009.21.4.371
Dimidi, E., Cox, S. R., Rossi, M., and Whelan, K. (2019). Fermented foods: definitions and characteristics, impact on the gut microbiota and effects on gastrointestinal health and disease. Nutrients 11:1806. doi: 10.3390/nu11081806
Donato, V., Ayala, F. R., Cogliati, S., Bauman, C., Costa, J. G., Leñini, C., et al. (2017). Bacillus subtilis biofilm extends Caenorhabditis elegans longevity through downregulation of the insulin-like signalling pathway. Nat. Commun. 30:14332. doi: 10.1038/ncomms14332
Duary, R., Rajput, Y., Batish, V., and Grover, S. (2011). Assessing the adhesion of putative indigenous probiotic lactobacilli to human colonic epithelial cells. Indian J. Med. Res. 134, 664–671. doi: 10.4103/0971-5916.90992
Duc, L. H., Hong, H. A., and Cutting, S. M. (2003). Germination of the spore in the gastrointestinal tract provides a novel route for heterologous antigen delivery. Vaccine 21, 4215–4224. doi: 10.1016/s0264-410x(03)00492-4
Raya, M., Morelli, L., Reid, G., Sanders, M., Stanton, C. G., Pineiro, M., et al. (2002). Working Group report on drafting guidelines for the evaluation of probiotics in food. London, Ontario, Canada, (WHO Publisher), April 30 and May 1.
Freedman, K., Hill, J., Wei, Y., Vazquez, A., Grubb, D., Trotter, R., et al. (2021). Examining the gastrointestinal and immunomodulatory effects of the novel probiotic Bacillus subtilis DE111. Int. J. Mol. Sci. 22:2453. doi: 10.3390/ijms22052453
Fujita, M., Nomura, K., Hong, K., Ito, Y., Asada, A., and Nishimuro, S. (1993). Purification and characterization of a strong fibrinolytic enzyme (nattokinase) in the vegetable cheese natto, a popular soybean fermented food in Japan. Biochem. Biophys. Res. Commun. 197, 1340–1347. doi: 10.1006/bbrc.1993.2624
Fujita, C., Usui, Y., and Inoue, M. (2022). Effects of Bacillus subtilis var. natto products on capillary blood flow in healthy subjects with peripheral coldness: a double-blind, placebo-controlled, randomized parallel study. Food Nutr. Sci. 13, 211–223. doi: 10.4236/fns.2022.133018
Garvey, S., Mah, E., Blonquist, T., Kaden, V., and Spears, J. (2022). The probiotic Bacillus subtilis BS50 decreases gastrointestinal symptoms in healthy adults: a randomized, doble-blind, placebo-controlled trial. Gut Microbes 14:2122668. doi: 10.1080/19490976.2022.2122668
Gerhardt, P., R. G. E. Murray, W. A. Wood, and N. R. Krieg. (1994). Methods for general and molecular bacteriology. Am. Soc. Microbiol. 619, 642–647. doi: 10.1002/food.19960400226
Gomathi, S., Sasikumar, P., Anbazhagan, K., Sasikumar, S., Kavitha, M., Selvi, M. S., et al. (2014). Screening of indigenous oxalate degrading lactic acid bacteria from human faeces and south Indian fermented foods: assessment of probiotic potential. Sci. World J. 2014:648059. doi: 10.1155/2014/648059
Grau, R. R., de Oña, P., Kunert, M., Leñini, C., Gallegos-Monterrosa, R., Mhatre, E., et al. (2015). A duo of potassium-responsive histidine kinases govern the multicellular destiny of Bacillus subtilis. mBio 6:e00581. doi: 10.1128/mBio.00581-15
Guibaud, G., van Hullebusch, E., Bordas, F., d’Abzac, P., and Joussein, E. (2009). Sorption of Cd(II) and Pb(II) by exopolymeric substances (EPS) extracted from activated sludges and pure bacterial strains: modeling of the metal/ligand ratio effect and role of the mineral fraction. Bioresour. Technol. 100, 2959–2968. doi: 10.1016/j.biortech.2009.01.040
Hanifi, A., Culpepper, T., Mai, V., Anand, A., Ford, A., Ukhanova, M., et al. (2015). Evaluation of Bacillus subtilis RO179 on gastrointestinal viability and general wellness: a randomized double-blind, placebo-controlled trial in healthy adults. Benef. Microbes 6, 19–27. doi: 10.3920/BM2014.0031
Haro, C., Mónaco, M. E., and Medina, M. (2018). Lactobacillus casei beneficially modulates immune-coagulative response in an endotoxemia model. Blood Coagul. Fibrinolysis 29, 104–110. doi: 10.1097/MBC.0000000000000684
Hellmig, S., Von Schöning, F., Gadow, C., Katsoulis, S., Hedderich, J., Fölsch, U. R., et al. (2006). Gastric emptying time of fluids and solids in healthy subjects determined by 13C breath tests: influence of age, sex and body mass index. J. Gastroenterol. Hepatol. 21, 1832–1838. doi: 10.1111/j.1440-1746.2006.04449.x
Hill, C., Guarner, F., Reid, G., Gibson, G. R., Merenstein, D. J., Pot, B., et al. (2014). Expert consensus document. The International Scientific Association for Probiotics and Prebiotics consensus statement on the scope and appropriate use of the term probiotic. Nat. Rev. Gastroenterol. Hepatol. 11, 506–514. doi: 10.1038/nrgastro.2014.66
Hoa, T. T., Duc, L. H., Isticato, R., Baccigalupi, L., Ricca, E., Van, P. H., et al. (2001). Fate and dissemination of Bacillus subtilis spores in a murine model. Appl. Environ. Microbiol. 67, 3819–3823. doi: 10.1128/AEM.67.9.3819-3823.2001
Hong, H. A., Huang, J.-M., Khaneja, R., Hiep, L. V., Urdaci, M. C., and Cutting, S. M. (2008). The safety of Bacillus subtilis and Bacillus indicus as food probiotics. Appl. Environ. Microbiol. 105, 510–520. doi: 10.1111/j.1365-2672.2008.03773.x
Horie, M., Koike, T., Sugino, S., Umeno, A., and Yoshida, Y. (2018). Evaluation of probiotic and prebiotic-like effects of Bacillus subtilis BN on growth of lactobacilli. J. Gen. Appl. Microbiol. 64, 26–33. doi: 10.2323/jgam.2017.03.002
Horie, M., Murakami, T., Sato, T., Tarusawa, Y., Nakamura, S., and Toba, T. (2005). Anaerobic induction of adherence to laminin in Lactobacillus gasseri strains by contact with solid surface. Curr. Microbiol. 51, 275–282. doi: 10.1007/s00284-005-4572-z
Hossain, T. J., Chowdhury, S. I., Mozumder, H. A., Chowdhury, M. N. A., Ali, F., Rahman, N., et al. (2020). Hydrolytic exoenzymes produced by bacteria isolated and identified from the gastrointestinal tract of Bombay duck. Front. Microbiol. 26:2097. doi: 10.3389/fmicb.2020.02097
Hsu, R. L., Lee, K. T., Wang, J. H., Lee, L. Y., and Chen, R. P. (2009). Amyloid-degrading ability of nattokinase from Bacillus subtilis natto. J. Agric. Food Chem. 57, 503–508. doi: 10.1021/jf803072r
Hu, L., Feng, J., Wu, J., Li, W., Gningue, S., Yang, Z., et al. (2020). Identification of six important amino acid residues of MenA from Bacillus subtilis natto for enzyme activity and formation of menaquinone. Enzym. Microb. Technol. 138:109583. doi: 10.1016/j.enzmictec.2020.109583
Hyronimus, B., Le Marrec, C., Sassi, A. H., and Deschamps, A. (2000). Acid and bile tolerance of spore-forming lactic acid bacteria. Int. J. Food Microbiol. 61, 193–197. doi: 10.1016/S0168-1605(00)00366-4
Ikeda, H., and Doi, Y. (1990). A vitamin-K2-binding factor secreted from Bacillus subtilis. Eur. J. Biochem. 192, 219–224. doi: 10.1111/j.1432-1033.1990.tb19218.x
Itokawa, H., Miyashita, T., Morita, H., Takeya, K., Hirano, T., Homma, M., et al. (1994). Structural and conformational studies of Ile7 and Leu7 surfactins from Bacillus subtilis natto. Chem. Pharm. Bull. 42, 604–607. doi: 10.1248/cpb.42.604
Ji, H., Yu, L., Liu, K., Yu, Z., Zhang, Q., Zou, F., et al. (2014). Mechanisms of nattokinase in protection of cerebral ischemia. Eur. J. Pharmacol. 745, 144–151. doi: 10.1016/j.ejphar.2014.10.024
Jung, M., Lee, S., Kim, H., and Kim, H. (2000). Recent studies on natural products as anti-HIV agents. Curr. Med. Chem. 7, 649–661. doi: 10.2174/0929867003374822
Kalman, D., Schwartz, H., Alvarez, P., Feldman, S., Pezzullo, J., and Krieger, D. (2009). A prospective, randomized double-blind, placebo-controlled parallel-group dual site trial to evaluate the effects of a Bacillus coagulans-based product on functional intestinal gas symptoms. BMC Gastroenterol. 9:85. doi: 10.1186/1471-230X-9-85
Kamada, M., Hase, S., Sato, K., Toyoda, A., Fujiyama, A., and Sakakibara, Y. (2014). Whole genome complete resequencing of Bacillus subtilis natto by combining long reads with high-quality short reads. PLoS One 9:e109999. doi: 10.1371/journal.pone.0109999
Katagiri, R., Sawada, N., Goto, A., Yamaji, T., Iwasaki, M., Noda, M., et al. (2020). Association of soy and fermented soy product intake with total and cause specific mortality: prospective cohort study. BMJ 29:m34. doi: 10.1136/bmj.m34
Kim, J. Y., Gum, S. N., Paik, J. K., Lim, H. H., Kim, K. C., Ogasawara, K., et al. (2008). Effects of nattokinase on blood pressure: a randomized, controlled trial. Hypertens. Res. 31, 1583–1588. doi: 10.1291/hypres.31.1583
Kokubo, Y., Iso, H., Ishihara, J., Okada, K., Inoue, M., Tsugane, S., et al. (2007). Association of dietary intake of soy, beans, and isoflavones with risk of cerebral and myocardial infarctions in Japanese populations. Circulation 116, 2553–2562. doi: 10.1161/CIRCULATIONAHA.106.683755
Kumar, V., Bhatt, P., Pathak, S., and Panda, B. (2017). Attenuation of neurobehavioral and neurochemical abnormalities in animal model of cognitive deficits of Alzheimer’s disease by fermented soybean nanonutraceutical. Inflammopharmacol 26, 105–118. doi: 10.1007/s10787-017-0381-9
Kuwana, R., Okumura, T., Takamatsu, H., and Watabe, K. (2005). The ylbO gene product of Bacillus subtilis is involved in the coat development and lysozyme resistance of spore. FEMS Microbiol. Lett. 242, 51–57. doi: 10.1016/j.femsle.2004.10.038
Lee, D. H., Choi, S. U., and Hwang, Y. I. (2005). Culture conditions and characterizations of a new phytase-producing fungal isolate, Aspergillus sp. L117. Mycobiology 33, 223–229. doi: 10.4489/MYCO.2005.33.4.223
Lemme-Dumit, J. M., Polti, M. A., Perdigón, G., and Galdeano, C. M. (2018). Probiotic bacteria cell walls stimulate the activity of the intestinal epithelial cells and macrophage functionality. Benef. Microbes 9, 153–164. doi: 10.3920/BM2016.0220
Liu, Y., Xu, W., Zeng, G., Li, X., and Gao, H. (2006). Cr (VI) reduction by Bacillus sp. isolated from chromium landfill. Process Biochem. 9, 21–28. doi: 10.1016/j.procbio.2006.04.020
Lombardía, E., Rovetto, A. J., Arabolaza, A. L., and Grau, R. R. (2006). A LuxS-dependent cell-to-cell language regulates social behavior and development in Bacillus subtilis. J. Bacteriol. 188, 4442–4452. doi: 10.1128/JB.00165-06
Lu, D., Pan, C., Ye, C., Duan, H., Xu, F., Yin, L., et al. (2017). Meta-analysis of soy consumption and gastrointestinal cancer risk. Sci. Rep. 7:4048. doi: 10.1038/s41598-017-03692-y
Megharaj, M., Avudainayagam, S., and Naidu, R. (2003). Toxicity of hexavalent chromium and its reduction by bacteria isolated from soil contaminated with tannery waste. Curr. Microbiol. 47, 51–54. doi: 10.1007/s00284-002-3889-0
Murakami, K., Yamanaka, N., Ohnishi, K., Fukayama, M., and Yoshino, M. (2012). Inhibition of angiotensin I converting enzyme by subtilisin NAT (nattokinase) in natto, a Japanese traditional fermented food. Food Funct. 3, 674–678. doi: 10.1039/c2fo10245e
Myung, S. K., Ju, W., Choi, H. J., and Kim, S. C., Korean Meta-Analysis (KORMA) Study Group (2009). Soy intake and risk of endocrine-related gynaecological cancer: a meta-analysis. BJOG 116, 116, 1697–1705. doi: 10.1111/j.1471-0528.2009.02322.x
Nagata, C., Takatsuka, N., Kawakami, N., and Shimizu, H. (2002). A prospective cohort study of soy product intake and stomach cancer death. Br. J. Cancer 87, 31–36. doi: 10.1038/sj.bjc.6600349
Nagata, C., Wada, K., Tamura, T., Konishi, K., Goto, Y., Koda, S., et al. (2017). Dietary soy and natto intake and cardiovascular disease mortality in Japanese adults: the Takayama study. Am. J. Clin. Nutr. 105, 426–431. doi: 10.3945/ajcn.116.137281
Nakahara, T., Nishino, H., Munemori, M., and Musha, S. (1973). The atomic absorption spectrophotometric determination of arsenic and selenium in premixed inert gas (entrained air)-hydrogen flames with a multi-flame burner. Bull. Chem. Soc. Japan 46, 1706–1711.
Nakano, M., Kawasaki, Y., Suzuki, N., and Takara, T. (2015). Effects of pyrroloquinoline quinone disodium salt intake on the serum cholesterol levels of healthy Japanise adults. J. Nutr. Sci. Vitaminol. 61, 233–240. doi: 10.3177/jnsv.61.233
Nakano, M., Yamamoto, T., Okamura, H., Tsuda, A., and Kowatari, Y. (2012). Effects of oral supplementation with pyrroloquinoline quinone on stress, fatigue, and sleep. Funct. Foods Health Dis. 2, 307–324. doi: 10.31989/ffhd.v2i8.81
Ongena, M., Jacques, P., Touré, Y., Destain, J., Jabrane, A., and Thonart, P. (2005). Involvement of fengycin-type lipopeptides in the multifaceted biocontrol potential of Bacillus subtilis. Appl. Microbiol. Biotechnol. 69, 29–38. doi: 10.1007/s00253-005-1940-3
Osawa, R., and Matsumoto, K. (1997). Digestion of staphylococcal enterotoxin by Bacillus natto. Antonie Van Leeuwenhoek 71, 307–311. doi: 10.1023/A:1000131908089
Ozawa, K., Fujiwara, R., Watanabe, K., and Sonoyama, K. (2012). Persistence of orally administered Lactobacillus strains in the gut of infant mice. Biosci. Microbiota Food Health 31, 85–91. doi: 10.12938/bmfh.31.85
Patterson, E., Ryan, P. M., Cryan, J. F., Dinan, T. G., Ross, R. P., Fitzgerald, G. F., et al. (2016). Gut microbiota, obesity and diabetes. Postgrad. Med. J. 92, 286–300. doi: 10.1136/postgradmedj-2015-133285
Pedraza-Reyes, M., Ramírez-Ramírez, N., Vidales-Rodríguez, L., and Robleto, E. (2012). “Chapter 6: mechanisms of bacterial spore survival” in Bacterial spores: current research and applications. ed. E. Abel-Santos (Norfolk: Caister Academic Press), 282.
Pedrido, M. E., de Oña, P., Ramirez, W., Leñini, C., Goñi, A., and Grau, R. (2013). Spo0A links de novo fatty acid synthesis to sporulation and biofilm development in Bacillus subtilis. Mol. Microbiol. 87, 348–367. doi: 10.1111/mmi.12102
Penet, C., Kramer, R., Little, R., Spears, J., Parker, J., Iyer, J., et al. (2021). A randomized, double-blind, placebo-controlled, parallel study evaluating the efficacy of Bacillus subtilis MB40 to reduce abdominal discomfort, gas and bloating. Alter. Ther. Health Med. 27, 146–157.
Pham, P. T., Han, B., and Hoang, B. X. (2020). Nattospes as effective and safe functional supplements in management of stroke. J. Med. Food 23, 879–885. doi: 10.1089/jmf.2019.0183
Piewngam, P., and Otto, M. (2020). Probiotics to prevent Staphylococcus aureus disease? Gut Microbes 11, 94–101. doi: 10.1080/19490976.2019.1591137
Piewngam, P., Zheng, Y., Nguyen, T., Dickey, S., Joo, H., Villaruz, A., et al. (2018). Pathogen elimination by probiotic Bacillus via signalling interference. Nature 562, 532–537. doi: 10.1038/s41586-018-0616-y
Quecine, M. C., Kidarsa, T. A., Goebel, N. C., Shaffer, B. T., Henkels, M. D., Zabriskie, T. M., et al. (2015). An interspecies signaling system mediated by fusaric acid has parallel effects on antifungal metabolite production by Pseudomonas protegens strain Pf-5 and antibiosis of Fusarium spp. Appl. Environ. Microbiol. 82, 1372–1382. doi: 10.1128/AEM.02574-15
Rodriguez Ayala, F., Bauman, C., Bartolini, M., Saball, E., Salvarrey, M., Leñini, C., et al. (2017a). Transcriptional regulation of adhesive properties of Bacillus subtilis to extracellular matrix proteins through the fibronectin-binding protein YloA. Mol. Microbiol. 104, 804–821. doi: 10.1111/mmi.13666
Rodriguez Ayala, F., Bauman, C., Cogliati, S., Leñini, C., Bartolini, M., and Grau, R. (2017b). Microbial flora, probiotics, Bacillus subtilis and the search for a long and healthy human longevity. Microb. Cell 4, 133–136. doi: 10.15698/mic2017.04.569
Rodriguez Ayala, F., Cardinali, N., and Grau, R. (2022a). Efficient weight loss and type II diabetes control in overweight and obese patients consuming the probiotic Bacillus subtilis DG101: a randomized double-blind placebo-controlled study. Asploro J. Biomed. Clin. Case Rep. 5, 51–58. doi: 10.36502/2022/ASJBCCR.6263
Rodriguez Ayala, F., Cardinali, N., and Grau, R. (2022b). Efectiveness of the probiotic Bacillus subtilis DG101 to treat type II diabetes mellitus triguered by SARS-CoV-2 infection. J. Clin. Imag. Med. Case Rep. 3:1847. doi: 10.52768/2766-7820/1847
Rodriguez Ayala, F., Francisco, M., Argañaraz, F., Crespo, C., Clementi, V., and Grau, R. (2021). Healthy aging, neuroprotection and decreased risk of cardiovascular death associated with the consumption of probiotic Bacillus subtilis. Geront. Ger. Stud. 7. doi: 10.31031/GGS.2021.07.000662
Sanders, M. E., Morelli, L., and Tompkins, T. A. (2003). Sporeformers as human probiotics: Bacillus, Sporolactobacillus, and Brevibacillus. Compr. Rev. Food Sci. Food Saf. 2, 101–110. doi: 10.1111/j.1541-4337.2003.tb00017.x
Singh, I. B., and Singh, D. R. (2002). Influence of dissolved oxygen on aqueous Cr(VI) removal by ferrous ion. Environ. Technol. 23, 1347–1353. doi: 10.1080/09593332508618450
Singh, B., and Singh, R. (2014). Axone, an ethnic probiotic containing food, reduces age of sexual maturity and increases poultry production. Probiotics Antimicrob. Proteins 6, 88–94. doi: 10.1007/s12602-014-9160-8
Styriak, I., Lauková, A., Fallgren, C., and Wadström, T. (1999). Binding of extracellular matrix proteins by animal strains of staphylococcal species. Vet. Microbiol. 67, 99–112.
Su, Y., Liu, C., Fang, H., and Zhang, D. (2020). Bacillus subtilis: a universal cell factory for industry, agriculture, biomaterials and medicine. Microb. Cell Factories 19:173. doi: 10.1186/s12934-020-01436-8
Sumi, H., Hamada, H., Tsushima, H., Mihara, H., and Muraki, H. (1987). A novel fibrinolytic enzyme (nattokinase) in the vegetable cheese natto, a typical and popular soybean food in Japanise diet. Experientia 43, 1110–1111. doi: 10.1007/BF01956052
Sun, L. P., Huang, Y., Huang, T., Yuan, Z., Lin, W., Sun, Z., et al. (2019). Optical microfiber reader for enzyme-linked immunosorbent assay. Anal. Chem. 91, 14141–14148. doi: 10.1021/acs.analchem.9b04119
Sunagawa, Y., Okamura, N., Miyazaki, Y., Shimizu, K., Genpei, M., Funamoto, M., et al. (2018). Effects of products containing Bacillus subtilis var natto on healthy subjects with neck and shoulder stiffness, a double-blind, placebo-controlled, randomized crossover study. Biol. Pharm. Bull. 41, 504–509. doi: 10.1248/bpb.b17-00780
Suzuki, K., Nakamura, M., Sato, N., Futamura, K., Matsunaga, K., and Yagami, A. (2023). Nattokinase (Bac s 1), a subtilisin family serine protease, is a novel allergen contained in the traditional Japanese fermented food natto. Allergol. Int. 72, 279–285. doi: 10.1016/j.alit.2022.11.010
Tsukamoto, Y., Ichise, H., Kakuda, H., and Yamaguchi, M. (2000). Intake of fermented soybean (natto) increases circulating vitamin K2 (menaquinone-7) and gamma-carboxylated osteocalcin concentration in normal individuals. J. Bone Miner. Metab. 18, 216–222. doi: 10.1007/s007740070023
Ueda, S. (1989). “Industrial applications of B. subtilis: utilization of soybean as natto, a traditional Japanese food” in Bacillus subtilis: molecular biology and industrial applications. eds. B. Mauro and H. Yoshikawa (Amsterdam: Elsevier), 143–161.
Upadhyay, M., Yadav, P., Shukla, A., and Srivastava, S. (2018). Utilizing the potential of microorganisms for managing arsenic contamination: a feasible and sustainable approach. Front. Environ. Sci. 6:24. doi: 10.3389/fenvs.2018.00024
Urano, T., Ihara, H., Umemura, K., Suzuki, Y., Oike, M., Akita, S., et al. (2001). The profibrinolytic enzyme subtilisin NAT purified from Bacillus subtilis cleaves and inactivates plasminogen activator inhibitor type 1. J. Biol. Chem. 276, 24690–24696. doi: 10.1074/jbc.M101751200
VidyaLaxme, B., Rovetto, A., Grau, R., and Agrawal, R. (2014). Synergistic effects of probiotic Leuconostoc mesenteroides and Bacillus subtilis in malted ragi (Eleucine corocana) food for antagonistic activity against V. cholerae and other beneficial properties. J. Food Sci. Technol. 51, 3072–3082. doi: 10.1007/s13197-012-0834-5
Vollenbroich, D., Őzel, M., Vater, J., Kamp, R., and Pauli, G. (1997). Mechanism of inactivation of enveloped viruses by the biosurfactant surfactin from Bacillus subtilis. Biologicals 25, 289–297. doi: 10.1006/biol.1997.0099
Volstatova, T., Havlik, J., Potuckova, M., and Geigerova, M. (2016). Milk digesta and milk protein fractions influence the adherence of Lactobacillus gasseri R and Lactobacillus casei FMP to human cultured cells. Food Funct. 7, 3531–3538. doi: 10.1039/C6FO00545D
Wang, C., Ng, T., Yuan, F., Liu, Z., and Liu, F. (2007). Induction of apoptosis in human leukemia K562 cells by cyclic lipopeptide from Bacillus subtilis natto T-2. Peptides 28, 1344–1350. doi: 10.1016/j.peptides.2007.06.014
Wei, G., Tian, N., Siezen, R., Schuppan, D., and Helmerhorst, E. (2016). Identification of food-grade subtilisins as gluten-degrading enzymes to treat celiac disease. Am. J. Physiol. Gastrointest. Liver Physiol. 311, G571–G580. doi: 10.1152/ajpgi.00185.2016
Weng, Y., Yao, J., and Wang, K. (2017). Nattokinase: an oral antithrombotic agent for the prevention of cardiovascular disease. Int. J. Mol. Sci. 18:523. doi: 10.3390/ijms18030523
Woo, H., Kim, M., Lee, Y.-H., Shn, D.-H., Shin, M.-H., and Choi, B. (2018). Habitual consumption of soy protein and isoflavones and risk of metabolic syndrome in adults ≥40 years old: a prospective analysis of the Korean multi-rural communities cohort study (MRcohort). Eur. J. Nut. 58, 2835–2850. doi: 10.1007/s00394-018-1833-8
Wu, J., Li, W., Zhao, S. G., Qian, S. H., Wang, Z., Zhou, M. J., et al. (2021). Site-directed mutagenesis of the quorum-sensing transcriptional regulator SinR affects the biosynthesis of menaquinone in Bacillus subtilis. Microb. Cell Factories 20:113. doi: 10.1186/s12934-021-01603-5
Wu, S., Shu, X., Chow, W.-H., Xiang, Y.-b., Zhang, X., Li, H.-L., et al. (2012). Soy food intake and circulating levels of inflammatory markers in Chinese women. J. Acad. Nutr. Diet. 112, 996–1004. doi: 10.1016/j.jand.2012.04.001
Yahav, S., Berkovich, Z., Ostrov, I., Reifen, R., and Shemesh, M. (2018). Encapsulation of beneficial probiotic bacteria in extracellular matrix from biofilm-forming Bacillus subtilis. Artif. Cells Nanomed. Biotechnol. 46, 974–5982. doi: 10.1080/21691401.2018.1476373
Yan, Z., Zhang, X., Li, C., Jiao, S., and Dong, W. (2017). Association between consumption of soy and risk of cardiovascular disease: a meta-analysis of observational studies. Eur. J. Prev. Cardiol. 24, 735–747. doi: 10.1177/2047487316686441
Yang, J., Niu, C., and Guo, X. (2015). Mixed culture models for predicting intestinal interactions between Escherichia coli and Lactobacillus in the presence of probiotic Bacillus subtilis. Benef. Microbies 6, 871–877. doi: 10.3920/BM2015.0033
Yu, G., Sinclair, J., Hartman, G., and Bertagnolli, B. (2002). Production of iturin a by Bacillus amyloliquefaciens suppressing Rhizoctonia solani. Soil Biol. Biochem. 34, 955–963. doi: 10.1016/S0038-0717(02)00027-5
Zeng, Z., He, X., Li, F., Zhang, Y., Huang, Z., Wang, Y., et al. (2021). Probiotic properties of Bacillus proteolyticus isolated from tibetan yaks, China. Front. Microbiol. 12:649207. doi: 10.3389/fmicb.2021.649207
Zhang, G. Q., Chen, J. L., Liu, Q., Zhang, Y., Zeng, H., and Zhao, Y. (2015). Soy intake is associated with lower endometrial cancer risk: a systematic review and meta-analysis of observational studies. Medicine 94:e2281. doi: 10.1097/MD.0000000000002281
Zhang, B., Liu, Y., Ji, Q., Zhao, M., Zeng, J., Liu, L., et al. (2019). Nattokinase crude extract enhances cutaneous wound healing. J. Biomaterial Tissue Eng. 7, 1281–1286. doi: 10.1166/jbt.2017.1697
Zhang, X., Shu, X., Gao, Y.-T., Yang, G., Li, Q., Li, H., et al. (2003). Soy food consumption is associated with lower risk of coronary heart disease in Chinese women. J. Nutr. 133, 2874–2878. doi: 10.1093/jn/133.9.2874
Zhang, Y.-R., Xiong, H.-R., and Guo, X.-H. (2013). Enhanced viability of Lactobacillus reuteri for probiotics production in mixed solid-state fermentation in the presence of Bacillus subtilis. Folia Microbiol. 59, 31–36. doi: 10.1007/s12223-013-0264-4
Keywords: probiotics, fermented soybean, nattō, Bacillus subtilis DG101, beneficial biofilms, metal bioremediation, gut health
Citation: Leñini C, Rodriguez Ayala F, Goñi AJ, Rateni L, Nakamura A and Grau RR (2023) Probiotic properties of Bacillus subtilis DG101 isolated from the traditional Japanese fermented food nattō. Front. Microbiol. 14:1253480. doi: 10.3389/fmicb.2023.1253480
Received: 05 July 2023; Accepted: 28 August 2023;
Published: 28 September 2023.
Edited by:
Fernanda Mozzi, CONICET Centro de Referencia para Lactobacilos (CERELA), ArgentinaReviewed by:
Mongkol Thirabunyanon, Maejo University, ThailandCopyright © 2023 Leñini, Rodriguez Ayala, Goñi, Rateni, Nakamura and Grau. This is an open-access article distributed under the terms of the Creative Commons Attribution License (CC BY). The use, distribution or reproduction in other forums is permitted, provided the original author(s) and the copyright owner(s) are credited and that the original publication in this journal is cited, in accordance with accepted academic practice. No use, distribution or reproduction is permitted which does not comply with these terms.
*Correspondence: Roberto Ricardo Grau, cm9iZXJ0b2dyYXVAZnVsYnJpZ2h0bWFpbC5vcmc=
†Present addresses: Cecilia Leñini, Kyojin S.A. (R&D), Santa Fe, Argentina
Facundo Rodriguez Ayala, Kyojin S.A. (R&D), Santa Fe, Argentina
Anibal Juan Goñi, Escuela de Educación Técnica Profesional 465 General Manuel Belgrano, Ministerio de Educación de la Provincia de Santa Fe, Rosario, ArgentinaLiliana Rateni, Cátedra de Microbiología, Parasitología y Virología, Facultad de Ciencias Médicas, Universidad Nacional de Rosario, Rosario, Argentina
Disclaimer: All claims expressed in this article are solely those of the authors and do not necessarily represent those of their affiliated organizations, or those of the publisher, the editors and the reviewers. Any product that may be evaluated in this article or claim that may be made by its manufacturer is not guaranteed or endorsed by the publisher.
Research integrity at Frontiers
Learn more about the work of our research integrity team to safeguard the quality of each article we publish.