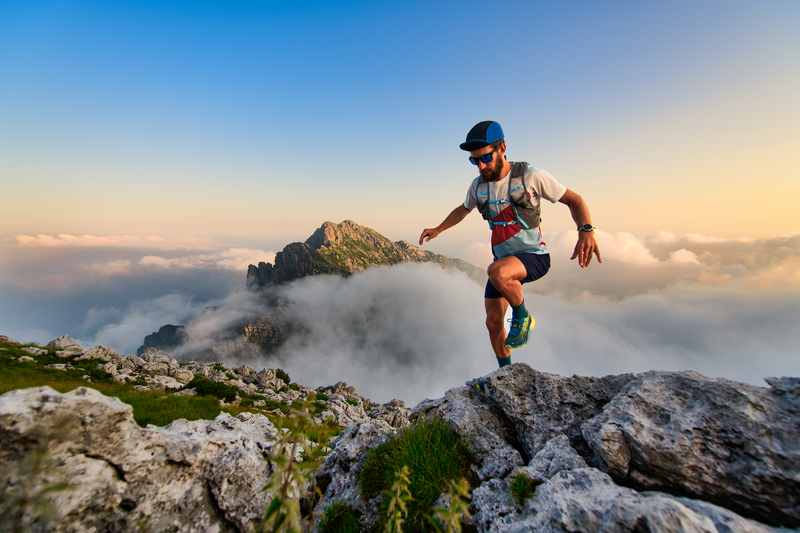
95% of researchers rate our articles as excellent or good
Learn more about the work of our research integrity team to safeguard the quality of each article we publish.
Find out more
ORIGINAL RESEARCH article
Front. Microbiol. , 07 July 2023
Sec. Microbial Symbioses
Volume 14 - 2023 | https://doi.org/10.3389/fmicb.2023.1185661
The close association between bacteria and insect hosts has played an indispensable role in insect diversity and ecology. Thus, continued characterization of such insect-associated-microbial communities is imperative, especially those of saprophagous scarab beetles. The bacterial community of the digestive tract of adults and larvae of the cetoniine scarab species Cotinis nitida is characterized according to life stage, gut structure, and sex via high-throughput 16S rRNA gene amplicon sequencing. Through permutational ANOVAs of the resulting sequences, bacterial communities of the digestive system are shown to differ significantly between adults and larvae in taxon richness, evenness and relatedness. Significant bacterial community-level differences are also observed between the midgut and hindgut in adult beetles, while no significant host-sex differences are observed. The partitioning between bacterial communities in the larval digestive system is shown through significant differences in two distinct hindgut regions, the ileum and the expanded paunch, but not between the midgut and ileum portion of the hindgut region. These data further corroborate the hypothesis of strong community partitioning in the gut of members of the Scarabaeoidea, suggest hypotheses of physiological-digestive association, and also demonstrate the presence of a seemingly unusual non-scarab-associated taxon. These findings contribute to a general portrait of scarabaeoid digestive tract bacterial communities while illuminating the microbiome of a common new world cetoniine of the Gymnetini—a tribe largely neglected in scarab and beetle microbiome and symbiosis literature.
The insect digestive system is morphologically and physiologically dynamic and rich in bacteria and other microbes. The bacteria that comprise these gut communities can be symbiotic and correlate with the host’s diet, and in some cases contribute directly to host nutrient acquisition and other digestive-nutritive metabolic processes (Jing et al., 2020). An important first step in understanding the functionality, interspecific relationships, and ultimate evolutionary significance of the bacterial microbiome, as well as the potential applications, like medicine (Chevrette et al., 2019) and pest management (Qadri et al., 2020), is to determine the diversity and test for the presence of a “core community” within a host species, and to understand how this host-level community can be further delimited according the physiological and morphological variety of the gut. That is, knowledge of the presence of certain organisms at various taxonomic levels and of any predictable patterns relating to their presence enables more precise investigations of these organisms within the system, including the inference of their function with respect to the host.
The scarab beetles are a particularly speciose family of the Coleoptera whose members show a diversity in their diets (McKenna et al., 2019). Additionally, like many other holometabolous insects (insects exhibiting complete metamorphosis), the diets and feeding strategies of scarab beetles differ between adults and larvae, and this distinction is evident in life-stage differences in the alimentary canal (Shukla et al., 2016). Host-species-level diversity and corresponding dietary differences, as well as the differing diets between adults and larvae, the great morphological and physiological compartmentalization of the gut in general and the consequential remodeling of the digestive tract during metamorphosis present an opportunity for us to understand bacterial diversity and ultimately infer and test its function (Hammer and Moran, 2019).
Partitioning of bacterial communities is an apparent trend in scarabs, with each general region of the gut harboring its own distinctive community (Egert et al., 2003; Andert et al., 2010) including even host-species-unique members (Cazemier et al., 1999, 2003). Some studies have indicated that there are differences between the bacterial communities of adult and larval scarabs (Arias-Cordero et al., 2012; Shukla et al., 2016; Suárez-Moo et al., 2020), reflecting the differing habits, as well as varied routes of community transmission. Notably, this is even true among beetles with similar adult and larval diets, such as the dung beetles (Shukla et al., 2016). Indeed, differences can be checked by processes of transmission, which may involve communities passed down from the parents, thus ensuring similarity between developmental stages, as in the case of burying beetles for whom maternal care determines presence or absence of maternal gut bacteria (Wang and Rozen, 2017). While poorly studied, vertical acquisition of the gut microbiome, including members beneficial to their hosts, have been demonstrated in scarabs; for instance, the maternally transmitted community of a dung beetle has been described, and its presence is associated with enhanced development of larvae (Estes et al., 2013; Schwab et al., 2016). Thus, the bacterial microbiota of scarabs encapsulates many of the phenomena observed in such communities in the holometabolans, with a highly diverse assemblage, variable across “regions” of the digestive system (Arias-Cordero et al., 2012), and between life stages and sexes (Shukla et al., 2016). Additionally, in some cases it is vertically transmitted (Estes et al., 2013).
The cetoniine scarab, Cotinis nitida, or green June beetle, is a minor agricultural pest known to damage ripe fruit as an adult and turfgrass as a larva (Potter, 1991) and is widespread across the southeastern United States (Hammons et al., 2008). Like many other scarabs, and especially those of the Cetoniinae or “rose chafers,” it has an above-ground-dwelling imago with well-developed flight capabilities and a diet of sugar-rich foods (particularly ripe or decaying fruit), and a fossorial larval form that dwells, for the entirety of its development, in substrate rich in decaying plant material on which it feeds. Also like other scarabs, it exhibits a radically different adult and larval gut morphology: the adults possess a simplified digestive tract while the larvae have a gut noticeably subdivided into small foregut, midgut with three crowns of gastric caeca, and a hindgut divided into an ileum and a large baglike paunch.
Little is known of its microbiome, and what is known focuses mostly on a few microbial genera in limited contexts. For example, adult C. nitida beetles acquires its gut yeast flora after pupal eclosion (Vishniac and Johnson, 1990). In this gut yeast floral community, Trichosporon cutaneum is most abundant in the gut of adult beetle and has been shown to play a relatively important role in the behavioral biology of adult beetles by producing volatile semiochemicals that serve as aggregation pheromones (Vishniac and Johnson, 1990; Johnson and Vishniac, 1991). Similarly, a species of Spiroplasma, S. clarkii, has been cultured from the guts of both adult and larval C. nitida (Whitcomb et al., 1993). It is the aim of this work to identify the bacterial diversity of the green June beetle, and to test for community partitioning based on gut region, sex, and life stages in C. nitida through the analysis of 16S rRNA gene high-throughput amplicons. Specifically, we test whether the midgut and hindgut structures harbor communities of bacterial ASVs significantly different from each other. We also test if the bacterial microbiomes differ in adult beetles based on sex. Finally, we tested if bacterial microbiomes differ between larval and adult beetles.
Adult beetles were collected after emerging from their pupal cells between June and September of 2018. With the exception of 5 individuals, which were not fed subsequent to capture, adults were placed in plastic containers and supplied with water-soaked paper towels and apple slices between capture and sample processing, which occurred when the beetles were still alive. Third instar larvae were collected from the soil between December of 2018 and February of 2019 and were also stored in plastic containers until processing. All individuals were collected from Clemson, South Carolina, USA. Adult C. nitida are distinguishable from all other scarabs in the region by their gross morphology—no other members of the genus or tribe are known from South Carolina, and the presence of a distinctive hood-like pronotum covering the scutellum distinguishes adults from vaguely similar species like Euphoria fulgida. Cotinis larvae are distinguished from those of other taxa by their size, manner of locomotion, and terminal setae. In total, 12 adults (seven males and five females) and 11 third instar larvae of indeterminate sex were sampled.
Guts were dissected from all individual specimens, which were dissected, alive, within 24 h of capture. Dissections were performed with 10% bleach-washed microscissors and forceps, in bleached glassware. Adult beetles were placed in a −20°C freezer for approximately 15 min and upon removal they were surface sterilized in 70% ethanol for one minute. They were then placed in a glass tray with approximately 20 mL of sterile phosphate buffered saline (PBS). Elytra were removed, as were wings. Microscissors were used to trim around the base of the spiracles and the tergites were pulled away, exposing the gut, tracheae, and fat body.
The prothorax was separated from the meso- and metathorax. The meso- and metathorax were removed by tearing out the tergum, pulling away any muscle or fat body tissue, and then pulling away the remaining structure delicately by the legs. The prothorax was removed by cutting the pleural region on either side of the pronotum to cut it in half, and both pieces were gently pulled away. To prevent lumen contents from leaking out of the midgut and small delicate foregut, the head was not removed. After using forceps to clamp the cuticle, a second pair of forceps were used to pull nervous, circulatory and respiratory tissue, fat body, and Malpighian tubules from the gut. The remainder of the beetle’s abdomen was pulled away from the rectum. The whole gut, including the foregut enclosed by the head, was moved to another sanitized tray with approximately 15 mL of sterile PBS. Microscissors were used to separate the hindgut from the midgut (Figure 1), and the midgut from the small foregut with the head.
Figure 1. (A) The gut of an adult C. nitida, head to the right. (B) The gut of a larval C. nitida, head to the right. (C) Detail of the midgut of the larval C. nitida. The anterior portion of the gut midgut is facing the right. (D) Detail of the ileum of the larval C. nitida, which begins just below the posterior most crown of gastric caeca [where the Malpighian tubules arise, faintly visible below this crown in (B) and ends before the greatly expanded hindgut paunch to the bottom left]. Gastric caeca and the greatly expanded hindgut paunch to the bottom left. (E) Detail of the exterior paunch of the larval hindgut C. nitida, showing the off-white bases of papillae.
Like adults, larvae were anaesthetized in a −20°C freezer 15 min prior to dissection and surface sterilized in 70% ethanol prior to being placed in sterile PBS. Incisions were made along the entire pleural region, below the spiracles. A circum-occipital cut was made, thus leaving a dorsal and ventral portion of the integument, which could be pulled apart and away from the head. Circulatory and respiratory tissue, fat body, and Malpighian tubules were pulled away from the gut. Any remaining cuticle was cut away from the around the anus. The gut, with the head still attached to the foregut, was transferred to a clean glass container filled with PBS. The gut was then cut into three sections: the paunch, the ileum, and the midgut (Figure 1). After dissection, all gut sections were immediately stored in a freezer at −80°C. The remains of adults and larvae not processed for extraction were stored in 100% ethanol. Given the partitioning, the adult guts amounted to 24 individual samples (12 midgut samples, and 12 hindgut samples), and the larvae amounted to 33 individual samples (11 midgut samples, 11 ileum samples, and 11 paunch samples). The remaining unused tissues from sampled individuals were saved as voucher specimens and deposited in the Clemson University Arthropod Collection.
DNA from the gut sections of both adult and larval beetles were extracted using the DNeasy PowerSoil kit (QIAGEN Germantown). Given the large volume of liquid harbored by the larval gut sections and the potential to dilute reagents, these were first dehydrated in a vacuum centrifuge for 90 min. Both larval and adult gut sections were pulverized with sterile micropestles (Millipore Sigma St. Louis) prior to extraction. Extractions were carried out according to the protocol of the manufacturer, including suggested incubation steps. Extraction blanks were not used. Extracts were stored in a freezer at −20°C. Extracts were quantified using a Qubit 3.0 Fluorometer (Life Technologies Carlsbad). The 33 samples used had a DNA concentration above 1 ng/μl.
To test for potential inhibitory substances in the sample extracts, PCRs were prepared using 16.875 μl of nuclease-free water, 2.5 μl of Taq buffer, 0.125 μl of Taq polymerase, and 2.5 μl of dNTP mix (10 mM), 1 μl of forward and reverse primer for the V4 hypervariable region (10 μM), and 1 μl of DNA sample. For larval midgut samples, successful amplification required 6 μl of samples diluted to 1:100 of their original concentration. The settings used for the PCR were as follows: 94°C initial denaturation for 3 min (94°C denaturation for 20 s, 50°C annealing for 15 s, 72°C extension for 5 mins) × 25 cycles, 72°C final extension for 10 min, and 4°C incubation. These test runs were carried out on a Mastercycler nexus gradient (Eppendorf). Successful amplification was shown by the presence of a fluorescent band at the 400 bp mark and absence of primer-dimers. Foreguts of both adult and larval beetles were excluded from sequencing due to their small size, structural simplicity, and inability to yield bacterial DNA sufficient for amplification. For all PCRs, negative controls were used. The total number of individual samples suitable for sequencing was 49 (5 larval midgut samples, 11 ileum samples, 10 paunch samples, 12 adult hindgut samples, and 11 adult midgut samples).
Amplicon libraries were assembled using PCR to add sample-specific indexes and adapters to individual samples. Initially, we used 16.75 μl of nuclease-free water, 5 μl of buffer, 0.5 μl of dNTP mix, 2.5 μl of Phusion high fidelity DNA polymerase (New England BioLabs), 0.75 μl of forward and reverse primer, and 1 μl of DNA sample. Test runs were carried out on a C1000 Touch Thermal Cycler (Bio Rad) using Barcoded primers, 515F and 806R (Kozich et al., 2013; Apprill et al., 2015) for the amplification of the V4 hypervariable region to allow single-step multiplexed sequencing and reduce occurrence of chimeras (Callahan et al., 2019). For adult midgut, and adult hindgut samples, 6 and 4 μl of diluted (1:100) DNA extract was used for the PCR amplifications, respectively. The settings for most samples that yielded successful PCR product, without primer-dimers are as follows: 98°C initial denaturation for 3 min (98°C denaturation for 20 s, 61°C annealing for 15 s, 72°C extension for 5 min) × 25 cycles, 72°C final extension for 10 min, and 4°C incubation. The same protocol was used for the adult midgut samples, but 27 cycles were used to increase instances of amplification in these recalcitrant samples. Moreover, to better amplify larval midgut samples, KAPA HiFi DNA polymerase (KAPA Biosystems) was used instead, and the PCR was increased to 30 cycles. All gut samples that did not successfully amplify were omitted from the sequencing run. Consequently, only 4 out of the original 11 larval midgut samples and 11 of the original 12 adult midgut samples were kept. Normalization and library pooling was carried out according to established protocols (Kozich et al., 2013). Barcoded amplicons were sequenced on an in house MiSeq platform using the MiSeq Reagent Kit v2 (500 cycle) (Illumina) according to the protocol of the manufacturer.
Analysis of 16 s rRNA gene sequences from C. nitida samples was carried out in QIIME 2 (version 2018.8; Bolyen et al., 2019). Sequences, as paired-end reads, were first joined using deblur (Amir et al., 2017). Quality filtering, including chimera removal and other denoising was carried out with deblur and representative sequences were generated using the command “representative-sequences.” Taxonomy was assigned with sequences from the SILVA database (version 132; Quast et al., 2013). Alignment of sequences was carried out using MAFFT (Katoh et al., 2002), followed by masking. Fastree (Price et al., 2010) was used to produce an unrooted tree, which was then rooted at the midpoint. To prevent the low frequency midgut samples of adult and larvae from being eliminated, the sampling depth was set at 1,000. This excluded 1 larval midgut sample from analysis on QIIME 2. The resulting sequences are henceforth referred to as amplicon sequence variants (ASVs), defined as different sequences recovered after the removal of erroneous elements generated by both PCR and subsequent amplicon sequencing. Community diversity analysis on QIIME was conducted for sex, gut region, and life stage with “alpha-group-significance” (for Faith’s PD) and “beta-group-significance” (for weighted and unweighted UniFrac, Bray–Curtis dissimilarity and Jaccard beta diversity analyses). To study the association between ASV and life stage or gut region, a multi-level pattern analysis was done using the package “indicspecies” (De Cáceres and Legendre, 2009), this package being chosen for its suitability in accounting for the compositional nature of the gut microbiome dataset (De Cáceres et al., 2012).
Data tidying and diversity metric calculations used the qiime2R (version 0.99.2; Bisanz et al., 2018), remotes (Hester et al., 2021), “tidyverse,” “stringr” (version 1.3.1; Wickham and RStudio, 2019), “vegan” (version 2.6.0; Oksanen et al., 2020), and “fossil” (version 0.4.0; Vavrek, 2020) packages. Noting that a small number of the adult specimens were processed differently from the others during sample collection (3 adult hindgut samples and 2 adult midgut samples)–these not being provided with a food and water source during their capture–we excluded them from further analysis, leaving 43 suitable samples. We tested whether gut microbiome alpha or beta diversity differed across gut regions between adult and larval C. nitida with perMANOVAs (Anderson, 2017). Both permutation tests were run using the “lm.rrppp” function in the “RRPP” package in R (version 1.2.3; Collyer and Adams, 2021). Because some gut regions are not found in both life stages (i.e., the distinctly modified paunch, a development of the colon, is found in larvae only), a combination variable of life stage and gut region was used as the only explanatory variable. 10,000 permutations were used to reduce the variation inherent to permutation-based methods. Where the ‘stage-region’ was found to significantly affect a given response variable pairwise comparisons were run using the “pairwise” function from the “RRPP” package. Alpha diversity was tested using the following metrics: Chao1, ACE, Shannon diversity, Simpson diversity, Pielou’s evenness index, and Faith’s phylogenetic diversity. This was followed by beta diversity analysis, which used the entire community dataset though ordinations from Jaccard distance, Bray–Curtis dissimilarity, and both weighted and unweighted UniFrac distances (Lozupone et al., 2011). Additional analysis concerning ASV community structuring, namely relative abundance of families and genera at the level of life stage and gut region for the identification of indicator species associated with those regions was conducted using “indicspecies” (De Cáceres and Legendre, 2009).
Univariate metrics (i.e., alpha diversity) were visualized using the tidyverse package (version 1.3.1; Wickham and RStudio, 2021) while beta diversity was visualized with principal coordinates analysis (PCoA) using the “ape” package (Paradis et al., 2004). Figures displaying relative abundances at the phyla, family, and genera levels were visualized using the “ggplot2” package in R (Wickham, 2016). All tidying, analysis, and visualization of the data were performed in version 4.0.3 of the R statistical environment or QIIME2 (Bolyen et al., 2019; R Core Team, 2020). All data and R code will be made available via the version control platform “git” (Torvalds and Hamano, 2021) in a public repository following acceptance for publication (Lyon, 2021; Lyon, 2023).
The data can be found here: https://www.ncbi.nlm.nih.gov/bioproject/PRJNA931270.
A total of 569,124 reads were obtained from 43 samples, yielding 3,622 bacterial and archaeal ASVs (Supplementary Table S1). These resulted in the identification of 285 genera, encompassing 23 phyla and 174 families, with only 2 and 6 of these, respectively, being archaeal.
The gut bacteria of adult and larval beetles showed a marked statistically significant difference in both phylogenetic diversity (Figure 2) and species richness and evenness (Supplementary Figure S1). Larval beetles harbored a taxonomically richer community of bacterial and archaeal ASVs than adults, according to Faith’s PD (H: 28.324696, p < 0.001, q < 0.001) (Figure 2). Additionally, the taxa present in larvae were distinct from those of adults (pseudo-F: 13.222026, p = 0.001, q = 0.001). Within adults, phylogenetic diversity of the midgut region was significantly lower from that of the hindgut (H: 12.595238, p < 0.001, q < 0.001). This distinction in phylogenetic diversity was not observed for the three regions of the larval that were sampled: the midgut was not significantly more diverse than the ileum (H: 2.454545, p = 0.117, q = 0.147) or the paunch (H: 1.28, p = 0.258, q = 0.287), and larval paunches were not significantly more diverse than ileums (H: 1.115702, p = 0.290846). In adult beetles, males and females did not harbor significant differences in the phylogenetic diversity of their gut bacteria (H: 0.46287, p = 0.496, q = 0.496).
Figure 2. Phylogenetic diversity of the life stages (A) and gut regions (B) of C. nitida. The larval gut community consists of overwhelmingly more taxa than that of the adult. Letters (a, b, c) statistically significant distinctiveness.
This pattern was recapitulated in terms of evenness, as demonstrated by Shannon’s Evenness metric, with adults exhibiting less even communities than larvae (F-statistic: 23.4, p = 0.0001), adult midgut communities being less even than adult hindgut ones (p = 0.0414), larval midgut communities not differing significantly from larval ileums (p = 0.102) and paunches (p = 0.4422), and paunches not differing significantly from larval ileums (p = 0.2786). Differences in host sex did not significantly correlate with differences in evenness in terms of Shannon diversity (F: 0.1, p = 0.6975).
The above patterns were also seen in other measures of evenness (Simpson’s), as well as richness (Chao1 and ACE) (Tables 1, 2). That is, larval midgut, ileum, and paunch samples were consistently more diverse than those of adults. Moreover, paunch and midgut samples showed consistently high diversity. Variation was to be found in regard to adult midgut and adult hindgut differences, wherein adult hindguts exhibited more diverse communities than adult midguts according to metrics of phylogenetic diversity and evenness (Faith’s PD, Shannon’s, Simpson’s), and larval paunches exhibited more diverse communities than larval ileums in terms of richness (Chao1, ACE).
Table 1. Summary statistics of alpha and beta diversity analyses of C. nitida among life stage-gut region combinations and between sexes.
Bray-Curtis and weighted UniFrac plots demonstrated a marked separation between adult and larval stages, as well as certain gut regions, namely between the adults and larvae and the hindgut paunch and other regions of the larval gut (Figure 3). Additionally, midgut samples grouped more closely with hindgut ileum samples from the same individual. Similar results were obtained in a Jaccard PCoA (Supplementary Figure S2). Unweighted UniFrac PCoA plots, in addition to demonstrating similar adult and larval differences, showed a distinctively grouped paunch community, as well as a larval midgut community that more closely resembles that of the larval ileum (Supplementary Figure S2). However, as corroborated by a perMANOVA, both models indicated an ileum community that differs from the other regions. Unweighted UniFrac PCoA plots also showed distinctive adult and larval communities as well as a distinction between adult and larval gut communities (Supplementary Figure S2). However, overlap between gut region community members does occur in both larvae adults (Figure 4).
Figure 3. Beta diversity of the gut bacterial and archaeal communities of the sexes of C. nitida according to Bray–Curtis PcoA, and weighted UniFrac. The larval and adult communities are taxonomically distinct from one another, as are individual gut regions, particularly the larval paunch, midgut + ileum, and adult midgut and hindgut regions. Each dot represents the bacterial community of a single gut sample from a single beetle. Ellipses represent confidence regions.
Figure 4. Venn diagram demonstrating overlap of community members (ASVs) in the gut of Adult (A) and larval (B) C. nitida.
Significant differences in community structure between life stages and gut regions, but not sexes, was found between C. nitida gut bacterial communities. There was a significant distinction between the adult midgut and adult hindgut (F statistic: 3.8, value of p = 0.001). However, there was no trend of taxonomic distinctiveness observed between the guts of male and female adult beetles (F statistic: 1.1, p = 0.327). All regions of the larval hindgut differed significantly from the regions of the adult gut. The larval midgut did not show significant taxonomic distinction with respect to the larval ileum (F statistic: 3.8, p = 0.998). However, the bacteria of the paunch showed a marked taxonomic distinction with respect to the midgut (F statistic: 3.8, p = 0.037) and the ileum (F statistic: 3.8, p = 0.001).
Together, the communities of adult and larval C. nitida are dominated by the bacterial phyla Firmicutes, Proteobacteria, and Bacteroidetes (Figure 5), while the most abundant families in C. nitida were Disulfovibrionaceae, Dysgonomonadaceae, Lachnospiraceae, Rikenellaceae, and Ruminococcaceae. However, the most dominant bacterial phyla, in terms of sheer abundance of representative ASVs, varied from one gut region type to the next (Figure 6), a situation also demonstrated by resident bacterial families (Figure 7). The larval midgut harbored communities dominated most heavily by Planctomycetes, followed by Verrucomicrobia, Actinobacteria, and Acidobacteria. The larval ileum of the hindgut harbored communities dominated by Firmicutes, followed by the Planctomycetes, Tenericutes, Verrucomicrobia, and Acidobacteria. The hindgut paunch of the larva differed sharply, with Bacteroidetes as the dominant phylum, followed by Firmicutes, the archaeal group Euarchaeota, and Deferribacteres. Adult beetles harbored Tenericutes as the most abundant midgut clade, followed by Proteobacteria, Firmicutes, and Bacteroidetes, while Bacteroidetes dominated the hindgut as with larvae, followed by Elusimicrobia, Proteobacteria, Firmicutes, and Deferribacteres.
Figure 5. Phyla (A) and families (B) most abundant in the guts of C. nitida, indiscriminate of life stage, gut region, or sex.
Figure 6. Relative abundances of bacterial phyla present in the various regions of the guts of adult and larval C. nitida. Only phyla of abundance levels of above 5% are shown here as distinct colored bars. Each column concerns an individual gut fragment (numbers following the gut fragment refer to the individual beetle the gut fragment was excised from).
Figure 7. Relative abundance of bacterial families in the gut of adults (Midgut, Hindgut) and larvae (Midgut, Ileum, Paunch) of C. nitida. Families below 10% relative abundance have been excluded.
Partitioning regarding abundance was observed in bacterial families present in the guts of both larval and adult C. nitida, with the families of greatest abundance (10% relative abundance or greater) in differing both between adults and larvae and between gut regions. However, with the exception of Dysgomonadaceae in the hindguts of adults, no given family-level taxon reached great abundance in all individuals of a particular life stage or gut type. However, a fraction of the most abundant taxa for each life stage or gut type were found in at least one third or more of the beetles sampled. Across sampled adults, these families were Desulfovibrionaceae, Dysgomonadaceae, Enterobacteraceae, Orbaceae, and Ruminococcaceae while in larvae the Bacillaceae, Christensenellaceae, Enterococcaceae, Lachnospiraceae, Rikenellaceae, Ruminococcaceae predominated. Regarding adult gut types, the adult midguts harbored Enterobacteraceae, Enterococcaceae, and Orbaceae, while the hindguts harbored Dysgomonadaceae, Orbaceae, Desulfovibrionaceae, Ruminococcaceae. In the larvae, the midguts harbored no taxa of great abundance in one-third or more the larvae, the ileums harbored Bacillaceae, Enterococcaceae, Lachnospiraceae, and over one-third of paunch samples harbored Christensenellaceae, Rikenellaceae, and Ruminococcaceae.
Partitioning in regard to abundant taxa was also seen in bacterial genera present in the guts of both larval and adult C. nitida, with the families of greatest abundance (here, 5% relative abundance or greater) differing both between adults and larvae and between gut regions (Figure 8). Unlike the case of bacterial family, more genera were found at great abundance in all individuals of a particular life stage or gut type, but this phenomenon, where present, was primarily exhibited in the larval paunch: genera Alistipes, Christensenellaceae of the R-7 group, and Desulfovibrio were found at high abundance in all paunch samples, while Dysgonomonas was found at high abundance in all adult hindgut samples. However, some taxa of comparatively great abundance were also present in at least 1/3 or more of samples. In the case of adults, this was true for Desulfovibrio, Dysgonomonas, Enterococcus, and Gilliamella. In the case of larvae, this was true for Alistipes, Bacillus, Desulfovibrio, Christensenellaceae of the R-7 group and Candidatus Soleaferrea.
Figure 8. Abundance of bacterial genera found in the gut communities of larval (A) and adult (B) C. nitida, featuring taxa of 5% abundance or greater.
Within specific gut regions, some genera were abundant in 1/3 or more of samples as well (Figure 8). In adult midguts, this was the case with Enterococcus, Dysgonomonas Gilliamella, and Lactococcus. In adult hindguts, such taxa were represented by Desulfovibrio, Bacteroides, Dysgonomonas, Enterococcus, Gilliamella, and NK4A214 group Ruminococcaceae. In the comparatively few larval midguts sampled, Udaeobacter and Bacteria of the Pir4 lineage were most abundant in 1/3 or more of samples, while in the ileums this was the case for Anaeroplasma, Bacillus, Enterrococcus, Udaeobacter, and Bacteria of the Pir4 lineage. Finally, in larval paunches, bacteria of the genus Alistipes, Christensenellaceae of the R7 group, Desulfovibrio, Methanobrevibacter, and Candidatus Soleaferrea were found in 1/3 or more of samples.
The guts of C. nitida on the level of life stage and gut region also bear region-specific families of indicator taxa (Table 3). The indicator taxa of each region differed from each other in terms of number as well, with the larvae having a far greater assemblage of indicator families than adults. In both larvae and the adults, these indicator taxa increased posteriorly, with more indicator taxa present in the hindgut than the midgut of adults and likewise in the larvae, with more indicator taxa in the hindgut than the midgut and more such taxa in the paunch than the ileum. Adult indicator families are represented by members of the phyla Firmicutes and Proteobacteria, which are also represented among larval indicator families in addition to 10 other larval indicator phyla (Acidobacteroidota, Actinomycetota, Chloroflexi, Euarchaeota, Gemmatimonadota, Nitrososphaerota, Planctomycetota, Tectomicrobia, and Verrucomicrobiota). Adult midgut families are represented solely by the phylum Firmicutes, while adult hindgut indicator families are represented by the phyla Proteobacteria and Thermodesulfobacteriota. Larval midgut indicator families belong to Bacteroidetes and Proteobacteria, while Ileum indicator families belong to Bacteroidetes and four other phyla (Firmicutes, Tenericutes, Proteobacteria, and Verrucomicrobiota), and the paunch indicator families consisted of the phyla Firmicutes, Bacteroidetes, Euryarchaeota, Gracilibacteria, and Proteobacteria. The indicator families did not overlap much with the most abundant families: in adults only three of the 17 most abundant taxa were indicator taxa (Leuconostocaceae, Orbaceae, Streptococcaceae), while in larvae, whose indicator taxa were represented by 47 families, only six of the 16 most abundant families were also indicator taxa (Bacillaceae, Blastocatellaceae, Cthonobacteraceae, Christensenellaceae, Pirellulaceae, and Xanthobacteraceae). In specific gut regions, only one adult midgut -abundant taxon (Streptococcaceae) and one hindgut taxon (Orbaceae) were also indicator taxa, and in larvae no midgut indicator taxa were abundant, while one ileum taxon (Anaeroplasmataceae) and two paunch taxa (Rhodocyclaceae, Ruminococcaceae) were indicator taxa.
At the genus level, indicator taxa were most numerous in larvae, consisting of 135 different genera, and particularly in the larval paunch and ileum (45 genera and 38 genera, respectively), but were also observed in the larval midgut and in the adult hindgut (just four genera and eight genera, respectively). Few of these were also particularly abundant genera, however, In terms of abundance with overlap, two out of the four most abundant taxa present in adults (Gilliamella and Dysgonomonas) were also indicator taxa of adult guts. In the larval gut, a single highly abundant taxon (R-7 group Christensenellaceae) out of six others was also represented among the numerous indicator taxa. The larval midgut did not have any overlap between its most abundant taxa and its indicator taxa. In larval ileums, a single abundant genus (Bacillus) out of five was also an indicator genus. In the larval paunch, by contrast, abundant genera were more well-represented among indicator taxa among the five most abundant taxa present, four (R-7 group Christensenellaceae, Alistipes, Methanobrevibacter, and Soleaferrea) were also indicators. In both adult hindguts and larval paunches was a shared indicator genus, Desulfovibrio, which was also one of the most abundant and widespread genera in C. nitida overall.
This study characterized the gut community of adult and larval members of the cetoniine scarab beetle C. nitida. Diversity analyses revealed that C. nitida not only possesses a taxonomically rich community of bacteria, but that this richness varies markedly between both adults and larvae and between gut regions within the same life stage. Gut community composition did not significantly differ between adult females and adult males. We observed patterns including gut-morphology-centered community partitioning that largely corroborate the general portrait of scarabaeoid beetle gut communities. Understanding these patterns helps to not only make predictions about the composition of gut communities in other organisms, but also to understand potential functions of the microbial communities along the digestive tract.
Our hypothesis regarding gut bacterial community differences based on life stage was confirmed. Not only did larvae possess more diverse communities phylogenetically (over 11 times more ASVs in their digestive tract than adults), but their microbial communities were also more diverse in terms of evenness and richness. Larva also harbored distinct taxa. Overall, this community partitioning resembles that found in other scarabaeoids (Wang et al., 2020).
The absence of a significant difference between male and female beetles in both alpha and beta diversity is markedly different from other scarab beetles, notably the dung beetles (Shukla et al., 2016). However, this discrepancy could be due to parental behavior differences between the two species. Because female scarab beetles are the sole parent participating in larval feeding, larvae develop a gut bacterial community that is more similar to the female than the male (Shukla et al., 2016). C. nitida does not exhibit parental care, and, therefore, would not be expected to have a gut bacterial community more like one parent than another.
Additionally, the hypothesis regarding bacterial community diversity based on gut region was also confirmed. Within adults, the bacterial communities of the midgut and hindgut differed in terms of phylogenetic diversity and evenness, with the hindgut being more phylogenetically diverse and even. Within larvae, the paunch had a distinct community signature. The larval ileum and midgut showed more similarities in community dynamics. Several occurrences could explain this phenomenon. This less stringent grouping of communities may indicate a far more transient community in the midgut and ileum. For example, the wide passage of the anterior ileum and the physiological similarities between this region and the anterior midgut may naturally facilitate a community crossover between the midgut and ileum. The poor clustering of ileum samples suggests a less consistent community of organisms compared to that of the paunch. It is possible that the ileum simply shares taxa by virtue of several physiological and morphological conditions of the beetle gut, including but not limited to condition of ingested substrate, gut pH, oxygen level, host digestive enzymes, and host immune response, as seen in other insect taxa (Maire et al., 2018; Lampert et al., 2019). Additionally, the presence or absence of certain taxa may be influenced by the microbial community itself. It is possible that hindgut paunch physiology favors some taxa over others. For example, oxygen content can influence community makeup, i.e., the lack of oxygen promotes the domination of anerobic bacteria such as Christensenellacae and Methanosaetacae (Chen and He, 2015; Kropp et al., 2021). Such patterns are observed in other animals, including some other insects (Engel and Moran, 2013), and indicates settlement of bacterial communities along the digestive tract is not a consequence of ingestion, but rather that underlying patterns relating to the host’s environment and specific selection processes are at play. The lack of statistically significant overlap between bacterial communities of the paunch, which was more morphologically delimited from the other two segments and thus less prone to disturbance during the dissection process further bolsters this hypothesis. Moreover, morphology should also be considered when hypothesizing about community localization, for the paunch is separated from the ileum by a greatly constricted length of hindgut. It is also internally lined with papillae, the basis of which can be observed through the translucent colon, and whose facilitation of retaining digested material can be hypothesized to affect residential microbial consortia. However, the crossover between taxa found in the larval ileum and midgut may be indicative of human error regarding dissection approach and gut morphology. Although the gut is filled with large pieces of plant material that do not move readily, lumen fluid can easily shift from one portion of the gut to another during dissection. Though, the notion that dissection error is solely responsible for these results can be overturned when considering the clustering of ileum samples with midguts from the same individual, compared to ileums from other individuals.
While mirroring most trends observed in other insects, the differences in taxon richness and community partitioning based on gut region and life stage in C. nitida is emblematic of what is known of the scarab gut microbiome. However, as stated previously, the lack of significant difference in taxon richness between midgut and hindgut regions of larvae in this study differs from that of other scarabs (Andert et al., 2010; Arias-Cordero et al., 2012). The distinctiveness of bacterial communities according to measures of alpha and beta diversity regarding life stage and individual gut region is not unprecedented, and other insects, including scarab beetles, exhibit similar patterns of bacterial diversity (Egert et al., 2003). This is also the case for other animals that have highly complex, compartmentalized digestive systems, including ruminating mammals and humans (Albenberg et al., 2014; Donaldson et al., 2016). Moreover, many relatively abundant bacterial families in the guts of C. nitida have representatives associated with particular physiological processes in the digestive systems of other organisms including Ruminococcaceae, Lachnospiraceae, Desulfovibrionaceae, Rikenellaceae (abundant in the hindguts of adults and the hindguts of larvae, although most abundant in the paunch) with cellulolytic activity in the digestive systems of other scarabs as well as ungulates (Biddle et al., 2013; Handique et al., 2017; Alonso-Pernas et al., 2017a,b; La Reau and Suen, 2018; Wang et al., 2022), Bacillaceae (abundant in the ileums of larvae) with iron reduction in the midguts of other scarabs (Hobbie et al., 2012), Burkholderiaceae (abundant in all regions of the larval gut, but most abundant in the hindgut paunch) and Orbaceae (abundant in adult midguts and hindguts) with nutritional symbiosis, respectively, in other insects (Kikuchi et al., 2012; Zheng et al., 2016), and Spiroplasmataceae (abundant in the adult midgut) with defensive symbiosis and reproductive manipulation in other insects (Xie et al., 2014).
In terms of most abundant and region-indicative genera of larval C. nitida, we find numerous taxa that are generally expected of animal digestive tracts. Among the most prominent of these “usual suspects” can be found in the larval paunch. For example, the R-7 group of the Christensenellaceae has been characterized as a volatile fatty acid producer (Goodrich et al., 2014), as well as a digestor of complex carbohydrates and amino acids, respectively converting these into acetate and ammonia (Chen et al., 2020). Alistipes, in addition to being potentially influential member of human guts (Parker et al., 2020), is well-represented in fungus-growing termites (Schnorr et al., 2019), suggesting a role as a gut symbiont in general. The paunch-centric Candidatus Soleaferrea, which also appears in the guts of numerous organisms, from mammals (Cai et al., 2020) to insects (McManus et al., 2018), has been found in hindguts of scarabs, and is noted for a fermentative habit (Ebert et al., 2021)—a habit that befits the environmental conditions of the scarab hindgut (Andert et al., 2010; Huang and Zhang, 2013; Ebert et al., 2021). Desulfovibrio, an anaerobic sulfate-reducer, is yet another common gut resident in animal taxa (Rey et al., 2013; Holman et al., 2022), including non-scarab insects (Mikaelyan et al., 2016), but in particular has been demonstrated to colonize the same region in the hindguts of scarabs of the subfamilies Melolonthinae and Scarabaeinae (Egert et al., 2005; Huang and Zhang, 2013; Ebert et al., 2021) at similarly great levels of abundance, further bolstering a gut-centric habit and sulfate-reducing service in C. nitida. Methanobrevibacter, an anaerobic methanogen (Enzmann et al., 2018), was also detected from the hindguts of a melolonthine scarab, further suggesting a broader preference for this specific anatomical region on the part of anaerobes (Egert et al., 2005).
In the distinctive larval ileum, the genus Bacillus was one of the few genera of over 5% abundance to be found in more than 1/3 of samples, mirroring overall significant presence in larval guts, but not specific region localization–this genus in particular is associated with iron reduction in midguts of larval scarabs (Hobbie et al., 2012), rather than hindguts. However, this may indeed may be a consequence of inclusion of the larval ileum with the midgut, which calls attention to possible level of gut localization that may be occurring, even in seemingly uniform or similar structures. In ileums as well as some larval midguts we also see Udaeobacter, a genus common in soil (Willms et al., 2020), which suggests a relationship between general larval habitat and the organisms that colonize the gut. This is also true of the Pir4 lineage (also present in the midguts of larvae); a group known for its anaerobic associations, including the habitation of low-oxygen soil (Dedysh et al., 2020).
In adult beetles, few of the most abundant taxa were found in more than 1/3 of the samples, the exceptional cases being Gilliamella, Dysgonomonas, and, as in larvae, the genus Desulfovibrio. With the exception of Gilliamella, the most abundant of these were restricted to the hindgut of adults, suggesting, as in larvae, a more selective hindgut community at the generic level. As in the cases of larvae, the adult beetles possess among their most abundant and widespread residents generally animal-gut-associated taxa like Dysgonomonas, which, while found in various terrestrial animals, including humans (Hofstad et al., 2000) has known insect associations, including residence in the guts of termites (Pramono et al., 2015) as well as other cetoniine scarabs (Schroeder et al., 2022). The most intriguing of the widespread and abundant genera in adult guts was the genus Gilliamella. Gilliamella of the Orbaceae, known as a bee symbiont, is a particularly unprecedented finding, although recent work, describing the genus and its close relatives from brachyceran flies (Jose et al., 2021; Hong et al., 2022) and tenebrionid beetles (Huang et al., 2019; Kuo et al., 2021), suggests a more extensive presence in insects, although not necessarily a mutualistic one.
Overall, whether or not the relatedness of certain taxa to studied organisms with similar gut localizations and cooccurrences with other microbial taxa reflects any manner of functional similarity is tenuous; at best the suggestion of such similarities provides a basis for future hypothesis testing. Additionally, the great diversity of C. nitida gut communities, particularly in larvae, makes functional hypothesizing weak without at least metagenomic scrutiny, as well as thorough culturing and proteomics approaches.
Additional factors which were not considered for the present study include the relationship between age of the sampled organisms (time since eclosion for either larvae or adults) and ag -related physiological processes. Though metamorphosis ceases after the imago stage, feeding behavior, as well as certain physiological shifts, incumbent on maturation may alter the bacterial community profile. Other limitations of this study include location of sampling, host diet variation, and small sample size. The gut communities of some scarabs change across instars (Alonso-Pernas et al., 2017a,b; Chouaia et al., 2019), as well as the provenance of taxa that appear to be common or abundant across one gut region or another, may be influenced by shifts in host diet or the geographic location from which the individual was sampled—our samples were taken from a very small geographical area, and thus their gut communities may reflect microhabitat in addition to broader geographical associations. Future work should seek to test how this occurs in C. nitida and to ultimately test for uniformities of microbial community structure in the scarabaeoids overall. Nevertheless, this study adds to the growing body of literature on gut bacterial community dynamics in C. nitida.
The datasets presented in this study can be found in online repositories. The names of the repository/repositories and accession number(s) can be found at: https://www.ncbi.nlm.nih.gov/, PRJNA931270.
RK: project design, sample collection, data analysis, and manuscript preparation. BC: methodological guidance and manuscript drafting. NL: data analysis, writing (methodology), and public repository provisioning. ES: statistical analysis and figure generation. MC: project design, methodological guidance, and manuscript preparation. All authors contributed to the article and approved the submitted version.
This publication was supported by the John and Suzanne Morse Endowment for Arthropod Biodiversity.
The authors declare that the research was conducted in the absence of any commercial or financial relationships that could be construed as a potential conflict of interest.
All claims expressed in this article are solely those of the authors and do not necessarily represent those of their affiliated organizations, or those of the publisher, the editors and the reviewers. Any product that may be evaluated in this article, or claim that may be made by its manufacturer, is not guaranteed or endorsed by the publisher.
The Supplementary material for this article can be found online at: https://www.frontiersin.org/articles/10.3389/fmicb.2023.1185661/full#supplementary-material
Albenberg, L., Esipova, T. V., Judge, C. P., Bittinger, K., Chen, J., Laughlin, A., et al. (2014). Correlation between intraluminal oxygen gradient and radial partitioning of intestinal microbiota. Gastroenterology 147, 1055–63.e8. doi: 10.1053/j.gastro.2014.07.020
Alonso-Pernas, P., Arias-Cordero, E., Novoselov, A., Ebert, C., Rybak, J., Kaltenpoth, M., et al. (2017a). Bacterial community and PHB-accumulating Bacteria associated with the wall and specialized niches of the hindgut of the Forest cockchafer (Melolontha hippocastani). Front. Microbiol. 8:291. doi: 10.3389/fmicb.2017.00291
Alonso-Pernas, P., Bartram, S., Arias-Cordero, E. M., Novoselov, A. L., Halty-deLeon, L., Shao, Y., et al. (2017b). In vivo isotopic labeling of symbiotic Bacteria involved in cellulose degradation and nitrogen recycling within the gut of the Forest cockchafer (Melolontha hippocastani). Front. Microbiol. 8:1970. doi: 10.3389/fmicb.2017.01970
Amir, A., McDonald, D., Navas-Molina, J. A., Kopylova, E., Morton, J. T., Zech, X. Z., et al. (2017). Deblur Rapidly Resolves Single-Nucleotide Community Sequence Patterns. mSystems 2, e00191–e00116.
Anderson, M. J. (2017). “Permutational multivariate analysis of variance (PERMANOVA)” in Wiley StatsRef: statistics reference online. eds. N. Balakrishnan, T. Colton, B. Everitt, W. Piegorsch, F. Ruggeri, and J. L. Teugels
Andert, J., Marten, A., Brandl, R., and Brune, A. (2010). Inter- and intraspecific comparison of the bacterial assemblages in the hindgut of humivorous scarab beetle larvae (Pachnoda spp.). FEMS Microbiol Ecol. 74, 439–449.
Apprill, A., McNally, S., Parsons, R., and Weber, L. (2015). Minor revision to v4 region rRNA 806R gene primer greatly increases detection of SAR11 bacterioplankton. Aquat. Microb. Ecol. 75, 129–137. doi: 10.3354/ame01753
Arias-Cordero, E., Ping, L., Reichwald, K., Delb, H., Platzer, M., and Boland, W. (2012). Comparative evaluation of the gut microbiota associated with the below- and above-ground life stages (larvae and beetles) of the forest cockchafer. Melolontha hippocastani. PLoS One. 7:e51557.
Biddle, A., Stewart, L., Blanchard, J., and Leschine, S. (2013). Untangling the genetic basis of Fibrolytic specialization by Lachnospiraceae and Ruminococcaceae in diverse gut communities. Diversity 5, 627–640. doi: 10.3390/d5030627
Bisanz, J. E. (2018). qiime2R: Importing QIIME2 artifacts and associated data into R sessions. Version 2.
Bolyen, E., Rideout, J. R., Dillon, M. R., Bokulich, N. A., Abnet, C. C., Al-Ghalith, G. A., et al. (2019). Reproducible, interactive, scalable and extensible microbiome data science using QIIME 2. Nat Biotechnol. 37, 852–857. Erratum in: Nat Biotechnol. 37:1091.
Cai, J., Zhou, L., Song, X., Yin, M., Liang, G., Xu, H., et al. (2020). Alteration of intestinal microbiota in 3-deoxyglucosone-induced Prediabetic rats. Biomed. Res. Int. 2020:8406846. doi: 10.1155/2020/8406846
Callahan, B. J., Wong, J., Heiner, C., Oh, S., Theriot, C. M., Gulati, A. S., et al. (2019). High-throughput amplicon sequencing of the full-length 16S rRNA gene with single-nucleotide resolution. Nucleic Acids Res. 47:e103.
Cazemier, A. E., Verdoes, J. C., Reubsaet, F. A. G., Hackstein, J. H. P., van der Drift, C., and Op den Camp, H. J. (2003). Promicromonospora pachnodae sp. nov., a member of the (hemi)cellulolytic hindgut flora of larvae of the scarab beetle Pachnoda marginata. Antonie Van Leeuwenhoek 83, 135–148. doi: 10.1023/A:1023325817663
Cazemier, A. E., Verdoes, J. C., Van Ooyen, A. J. J., and Op den Camp, H. J. M. (1999). Molecular and biochemical characterization of two xylanase-encoding genes from Cellulomonas pachnodae. Appl. Environ. Microbiol. 65, 4099–4107. doi: 10.1128/AEM.65.9.4099-4107.1999
Chen, S., and He, Q. (2015). Persistence of Methanosaeta populations in anaerobic digestion during process instability. J. Ind. Microbiol. Biotechnol. 42, 1129–1137. doi: 10.1007/s10295-015-1632-7
Chen, R., Li, Z., Feng, J., Zhao, L., and Yu, J. (2020). Effects of digestate recirculation ratios on biogas production and methane yield of continuous dry anaerobic digestion. Bioresour. Technol. 316:123963. doi: 10.1016/j.biortech.2020.123963
Chevrette, M. G., Carlson, C. M., Ortega, H. E., Thomas, C., Ananiev, G. E., Barns, K. J., et al. (2019). The antimicrobial potential of Streptomyces from insect microbiomes. Nat. Commun. 10:516.
Chouaia, B., Goda, N., Mazza, G., Alali, S., Florian, F., Gionechetti, F., et al. (2019). Developmental stages and gut microenvironments influence gut microbiota dynamics in the invasive beetle Popillia japonica Newman (Coleoptera: Scarabaeidae). Environ. Microbiol. 21, 4343–4359. doi: 10.1111/1462-2920.14797
Collyer, M., and Adams, D. (2021). RRPP: Linear model evaluation with randomized residuals in a permutation procedure. Vienna, Austria: R Foundation for Statistical Computing.
De Cáceres, M., and Legendre, P. (2009). Associations between species and groups of sites: indices and statistical inference. Ecology 90, 3566–3574. doi: 10.1890/08-1823.1
De Cáceres, M., Legendre, P., Wiser, S. K., and Brotons, L. (2012). Using species combinations in indicator value analyses. Methods Ecol. Evol. 3, 973–982. doi: 10.1111/j.2041-210X.2012.00246.x
Dedysh, S. N., Kulichevskaya, I. S., Beletsky, A. V., Ivanova, A. A., Rijpstra, W. I. C., Damsté, J. S. S., et al. (2020). Lacipirellula parvula gen. nov., sp. nov., representing a lineage of planctomycetes widespread in low-oxygen habitats, description of the family Lacipirellulaceae fam. nov. and proposal of the orders Pirellulales ord. nov., Gemmatales ord. nov. and Isosphaerales ord. nov. Syst. Appl. Microbiol. 43:126050. doi: 10.1016/j.syapm.2019.126050
Donaldson, G. P., Lee, S. M., and Mazmanian, S. K. (2016). Gut biogeography of the bacterial microbiota. Nat. Rev. Microbiol. 14, 20–32. doi: 10.1038/nrmicro3552
Ebert, K. M., Arnold, W. G., Ebert, P. R., and Merritt, D. J. (2021). Hindgut microbiota reflects different digestive strategies in dung beetles (Coleoptera: Scarabaeidae: Scarabaeinae). Appl. Environ. Microbiol. 87, e02100–e02120. doi: 10.1128/AEM.02100-20
Egert, M., Stingl, U., Bruun, L. D., Pommerenke, B., Brune, A., and Friedrich, M. W. (2005). Structure and topology of microbial communities in the major gut compartments of Melolontha larvae (Coleoptera: Scarabaeidae). Appl. Environ. Microbiol. 71, 4556–4566. doi: 10.1128/AEM.71.8.4556-4566.2005
Egert, M., Wagner, B., Lemke, T., Brune, A., and Friedrich, M. W. (2003). Microbial community structure in midgut and hindgut of the humus-feeding larva of Pachnoda ephippiata (Coleoptera: Scarabaeidae). Appl. Environ. Microbiol. 69, 6659–6668. doi: 10.1128/AEM.69.11.6659-6668.2003
Engel, P., and Moran, N. A. (2013). The gut microbiota of insects – diversity in structure and function. FEMS Microbiol. Rev. 37, 699–735. doi: 10.1111/1574-6976.12025
Enzmann, F., Mayer, F., Rother, M., and Holtmann, D. (2018). Methanogens: biochemical background and biotechnological applications. AMB Express 8:1. doi: 10.1186/s13568-017-0531-x
Estes, A. M., Hearn, D. J., Snell-Rood, E. C., Feindler, M., Feeser, K., Abebe, T., et al. (2013). Brood ball-mediated transmission of microbiome members in the dung beetle, Onthophagus taurus (Coleoptera: Scarabaeidae). PLoS One 8:e79061. doi: 10.1371/journal.pone.0079061
Goodrich, J. K., Waters, J. L., Poole, A. C., Sutter, J. L., Koren, O., Blekhman, R., et al. (2014). Human genetics shape the gut microbiome. Cells 159, 789–799. doi: 10.1016/j.cell.2014.09.053
Hammer, T. J., and Moran, N. A. (2019). Links between metamorphosis and symbiosis in holometabolous insects. Philos. Trans. R. Soc. Lond. Ser. B Biol. Sci. 374:20190068. doi: 10.1098/rstb.2019.0068
Hammons, D., Kurtural, S., and Potter, D. (2008). Japanese beetles facilitate feeding by green June beetles (Coleoptera: Scarabaeidae) on ripening grapes. Environ. Entomol. 37, 608–614. doi: 10.1603/0046-225x(2008)37[608:jbffbg]2.0.co;2
Handique, G., Phukan, A., Bhattacharyya, B., Baruah, A. A., Rahman, S. W., and Baruah, R. (2017). Characterization of cellulose degrading bacteria from the larval gut of the white grub beetle Lepidiota mansueta (Coleoptera: Scarabaeidae). Arch. Insect Biochem. Physiol. 94:e21370. doi: 10.1002/arch.21370
Hester, J., Csárdi, G., Wickham, H., Chang, W., RStudio, Morgan, M., et al. (2021). Remotes: R Package Installation from Remote Repositories, Including “GitHub.” Available at: https://cran.r-project.org/package=remotes
Hobbie, S., Li, X., Basen, M., Stingl, U., and Brune, A. (2012). Humic substance-mediated Fe(III) reduction by a fermenting Bacillus strain from the alkaline gut of a humus-feeding scarab beetle larva. Syst. Appl. Microbiol. 35, 226–232. doi: 10.1016/j.syapm.2012.03.003
Hofstad, T., Olsen, I., Eribe, E. R., Falsen, E., Collins, M. D., and Lawson, P. A. (2000). Dysgonomonas gen. nov. to accommodate Dysgonomonas gadei sp. nov., an organism isolated from a human gall bladder, and Dysgonomonas capnocytophagoides (formerly CDC group DF-3). Int. J. Syst. Evol. Microbiol. 50 Pt 6, 2189–2195. doi: 10.1099/00207713-50-6-2189
Holman, D. B., Kommadath, A., Tingley, J. P., and Abbott, D. W. (2022). Novel Insights into the Pig Gut Microbiome Using Metagenome-Assembled Genomes. Microbiol Spectr. 10:e0238022.
Hong, S., Sun, Y., Sun, D., and Wang, C. (2022). Microbiome assembly on Drosophila body surfaces benefits the flies to combat fungal infections. iScience 25:104408. doi: 10.1016/j.isci.2022.104408
Huang, Q., Lopez, D., and Evans, J. D. (2019). Shared and unique microbes between small hive beetles (Aethina tumida) and their honey bee hosts. Microbiology 8:e899. doi: 10.1002/mbo3.899
Huang, S., and Zhang, H. (2013). The impact of environmental heterogeneity and life stage on the hindgut microbiota of Holotrichia parallela larvae (Coleoptera: Scarabaeidae). PLoS One 8:e57169. doi: 10.1371/journal.pone.0057169
Jing, T. Z., Qi, F. H., and Wang, Z. Y. (2020). Most dominant roles of insect gut bacteria: digestion, detoxification, or essential nutrient provision? Microbiome 8:38. doi: 10.1186/s40168-020-00823-y
Johnson, D. T., and Vishniac, H. S. (1991). The role of Trichosporon cutaneum in eliciting aggregation behavior in Cotinis nitida. (Coleoptera: Scarabeidae). Environ. Entomol. 20, 15–21.
Jose, P. A., Ben-Yosef, M., Lahuatte, P., Causton, C. E., Heimpel, G. E., Jurkevitch, E., et al. (2021). Shifting microbiomes complement life stage transitions and diet of the bird parasite Philornis downsi from the Galapagos Islands. Environ. Microbiol. 23, 5014–5029. doi: 10.1111/1462-2920.15435
Katoh, K., Misawa, K., Kuma, K., and Miyata, T. (2002). MAFFT: a novel method for rapid multiple sequence alignment based on fast Fourier transform. Nucleic Acids Res. 30, 3059–3066.
Kikuchi, Y., Hayatsu, M., Hosokawa, T., Nagayama, A., Tago, K., and Fukatsu, T. (2012). Symbiont-mediated insecticide resistance. Proc. Natl. Acad. Sci. U. S. A. 109, 8618–8622. doi: 10.1073/pnas.1200231109
Kozich, J. J., Westcott, S. L., Baxter, N. T., Highlander, S. K., and Schloss, P. D. (2013). Development of a dual-index sequencing strategy and curation pipeline for analyzing amplicon sequence data on the MiSeq Illumina sequencing platform. Appl. Environ. Microbiol. 79, 5112–5120. doi: 10.1128/AEM.01043-13
Kropp, C., Le Corf, K., Relizani, K., Tambosco, K., Martinez, C., Chain, F., et al. (2021). The keystone commensal bacterium Christensenella minuta DSM 22607 displays anti-inflammatory properties both in vitro and in vivo. Sci. Rep. 11:11494. doi: 10.1038/s41598-021-90885-1
Kuo, C. H., Huang, P. Y., Sheu, S. Y., Sheu, D. S., Jheng, L. C., and Chen, W. M. (2021). Zophobihabitans entericus gen. Nov., sp. nov., a new member of the family Orbaceae isolated from the gut of a superworm Zophobas morio. Int. J. Syst. Evol. Microbiol. 71:005081. doi: 10.1099/ijsem.0.005081
La Reau, A. J., and Suen, G. (2018). The Ruminococci: key symbionts of the gut ecosystem. J. Microbiol. 56, 199–208. doi: 10.1007/s12275-018-8024-4
Lampert, N., Mikaelyan, A., and Brune, A. (2019). Diet is not the primary driver of bacterial community structure in the gut of litter-feeding cockroaches. BMC Microbiol. 19:238. doi: 10.1186/s12866-019-1601-9
Lozupone, C., Lladser, M. E., Knights, D., Stombaugh, J., and Knight, R. (2011). UNiFrac: an effective distance metric for microbial community comparison. ISME J. 5, 169–172. doi: 10.1038/ismej.2010.133
Lyon, N. J. (2021). Gut bacteria of adult and larval Cotinis nitida Linnaeus (Coleoptera: Scarabaeidae) demonstrate community differences according to life stage and gut region: tidy, analysis, and plotting code. Available at: https://github.com/NJLyon-projects/Kucuk-Cotinis-Collab
Lyon, N. J. (2023). supportR: support functions for wrangling and visualization. R package version 1.0.0.
Maire, J., Vincent-Monégat, C., Masson, F., Zaidman-Rémy, A., and Heddi, A. (2018). An IMD-like pathway mediates both endosymbiont control and host immunity in the cereal weevil Sitophilus spp. Microbiome. 6:6. doi: 10.1186/s40168-017-0397-9
McKenna, D. D., Shin, S., Ahrens, D., Balke, M., Beza-Beza, C., Clarke, D. J., et al. (2019). The evolution and genomic basis of beetle diversity. Proc. Natl. Acad. Sci. U. S. A. 116, 24729–24737. doi: 10.1073/pnas.1909655116
McManus, R., Ravenscraft, A., and Moore, W. (2018). Bacterial associates of a gregarious riparian beetle with explosive defensive chemistry. Front. Microbiol. 9:2361. doi: 10.3389/fmicb.2018.02361
Mikaelyan, A., Thompson, C. L., Hofer, M. J., and Brune, A. (2016). Deterministic assembly of complex bacterial communities in guts of germ-free cockroaches. Appl. Environ. Microbiol. 82, 1256–1263. doi: 10.1128/AEM.03700-15
Oksanen, J., Blanchet, F. G., Friendly, M., Kindt, R., Legendre, P., McGlinn, D., et al. (2020). vegan: Community Ecology Package. Available at: https://CRAN.R-project.org/package=vegan
Paradis, E., Claude, J., and Strimmer, K. (2004). APE: analyses of phylogenetics and evolution in R language. Bioinformatics 20, 289–290. doi: 10.1093/bioinformatics/btg412
Parker, B. J., Wearsch, P. A., Veloo, A. C. M., and Rodriguez-Palacios, A. (2020). The genus Alistipes: gut Bacteria with emerging implications to inflammation, Cancer, and mental health. Front. Immunol. 11:906. doi: 10.3389/fimmu.2020.00906
Potter, D. A. (1991). Ecology and management of turfgrass insects. Annu. Rev. Entomol. 36, 383–406. doi: 10.1146/annurev.en.36.010191.002123
Pramono, A. K., Sakamoto, M., Iino, T., Hongoh, Y., and Ohkuma, M. (2015). Dysgonomonas termitidis sp. nov., isolated from the gut of the subterranean termite Reticulitermes speratus. Int. J. Syst. Evol. Microbiol. 65, 681–685. doi: 10.1099/ijs.0.070391-0
Price, M. N., Dehal, P. S., and Arkin, A. P. (2010). FastTree 2–approximately maximum-likelihood trees for large alignments. PLoS One 5:e9490. doi: 10.1371/journal.pone.0009490
Qadri, M., Short, S., Gast, K., Hernandez, J., and Wong, A. C.-N. (2020). Microbiome innovation in agriculture: development of microbial based tools for insect pest management. Front. Sustain. Food Syst. 4.
Quast, C., Pruesse, E., Yilmaz, P., Gerken, J., Schweer, T., Yarza, P., et al. (2013). The SILVA ribosomal RNA gene database project: improved data processing and web-based tools. Nucleic Acids Res. 41, D590–D596. doi: 10.1093/nar/gks1219
R Core Team (2020). R: A language and environment for statistical computing. R Foundation for Statistical Computing, Vienna, Austria. Available at: https://www.R-project.org/.
Rey, F. E., Gonzalez, M. D., Cheng, J., Wu, M., Ahern, P. P., and Gordon, J. I. (2013). Metabolic niche of a prominent sulfate-reducing human gut bacterium. Proc. Natl. Acad. Sci. U S A. 110, 13582–13587.
Schnorr, S. L., Hofman, C. A., Netshifhefhe, S. R., Duncan, F. D., Honap, T. P., Lesnik, J., et al. (2019). Taxonomic features and comparisons of the gut microbiome from two edible fungus-farming termites (Macrotermes falciger; M. natalensis) harvested in the Vhembe district of Limpopo, South Africa. BMC Microbiol. 19:164. doi: 10.1186/s12866-019-1540-5
Schroeder, B. G., Logroño, W., Rocha, U. N. D., Harms, H., and Nikolausz, M. (2022). Enrichment of anaerobic microbial communities from Midgut and hindgut of Sun beetle larvae (Pachnoda marginata) on wheat straw: effect of inoculum preparation. Microorganisms. 10:761. doi: 10.3390/microorganisms10040761
Schwab, D. B., Riggs, H. E., Newton, I. L., and Moczek, A. P. (2016). Developmental and ecological benefits of the maternally transmitted microbiota in a dung beetle. Am. Nat. 188, 679–692. doi: 10.1086/688926
Shukla, S. P., Sanders, J. G., Byrne, M. J., and Pierce, N. E. (2016). Gut microbiota of dung beetles correspond to dietary specializations of adults and larvae. Mol. Ecol. 25, 6092–6106. doi: 10.1111/mec.13901
Suárez-Moo, P., Cruz-Rosales, M., Ibarra-Laclette, E., Desgarennes, D., Huerta, C., and Lamelas, A. (2020). Diversity and composition of the gut microbiota in the developmental stages of the dung beetle Copris incertus say (Coleoptera, Scarabaeidae). Front. Microbiol. 11:1698. doi: 10.3389/fmicb.2020.01698
Torvalds, L., and Hamano, J. (2021). Git – fast, scalable, distributed revision control system. Available at: https://git-scm.com/
Vavrek, M. J. (2020). Fossil: Palaeoecological and Palaeogeographical analysis tools. Palaeontologia Electronica. 14:16.
Vishniac, H., and Johnson, D. (1990). Development of a yeast Flora in the adult green June beetle (Cotinis nitida). Mycologia 82, 471–479. doi: 10.1080/00275514.1990.12025910
Wang, K., Gao, P., Geng, L., Liu, C., Zhang, J., and Shu, C. (2022). Lignocellulose degradation in Protaetia brevitarsis larvae digestive tract: refining on a tightly designed microbial fermentation production line. Microbiome. 10:90. doi: 10.1186/s40168-022-01291-2
Wang, Y., and Rozen, D. E. (2017). Gut microbiota colonization and transmission in the burying beetle Nicrophorus vespilloides throughout development. Appl. Environ. Microbiol. 83, e03250–e03216.
Wang, M., Xiang, X., and Wan, X. (2020). Divergence in gut bacterial community among life stages of the rainbow stag beetle Phalacrognathus muelleri (Coleoptera: Lucanidae). Insects. 11:719. doi: 10.3390/insects11100719
Whitcomb, R. F., Vignault, J.-C., Tully, J. G., Rose, D. L., Carle, P., Bove, J. M., et al. (1993). Spiroplasma clarkii sp. nov. from the Green June Beetle (Coleoptera: Scarabaeidae). Int. J. Syst. Bacteriol. 43, 261–265.
Wickham, H. (2016). ggplot2: elegant graphics for data analysis. Available at: https://ggplot2.tidyverse.org
Wickham, H., RStudio. (2019). Stringr: simple, Consistent Wrappers for Common String Operations. Available at: https://CRAN.R-project.org/package=stringr
Wickham, H., RStudio. (2021). Tidyverse: Easily Install and Load the “Tidyverse.” Available at: https://cran.r-project.org/package=tidyverse
Willms, I. M., Rudolph, A. Y., Göschel, I., Bolz, S. H., Schneider, D., Penone, C., et al. (2020). Globally abundant "Candidatus Udaeobacter" benefits from release of antibiotics in soil and potentially performs trace gas scavenging. mSphere 5:e00186-20. doi: 10.1128/mSphere.00186-20
Xie, J., Butler, S., Sanchez, G., and Mateos, M. (2014). Male killing Spiroplasma protects Drosophila melanogaster against two parasitoid wasps. Heredity (Edinb). 112, 399–408. doi: 10.1038/hdy.2013.118
Keywords: cetoniine, gut bacteria composition patterns, scarab beetle, gut microbiome, life stage analysis
Citation: Kucuk RA, Campbell BJ, Lyon NJ, Shelby EA and Caterino MS (2023) Gut bacteria of adult and larval Cotinis nitida Linnaeus (Coleoptera: Scarabaeidae) demonstrate community differences according to respective life stage and gut region. Front. Microbiol. 14:1185661. doi: 10.3389/fmicb.2023.1185661
Received: 13 March 2023; Accepted: 13 June 2023;
Published: 07 July 2023.
Edited by:
Matan Shelomi, National Taiwan University, TaiwanReviewed by:
Marie Braad Lund, Aarhus University, DenmarkCopyright © 2023 Kucuk, Campbell, Lyon, Shelby and Caterino. This is an open-access article distributed under the terms of the Creative Commons Attribution License (CC BY). The use, distribution or reproduction in other forums is permitted, provided the original author(s) and the copyright owner(s) are credited and that the original publication in this journal is cited, in accordance with accepted academic practice. No use, distribution or reproduction is permitted which does not comply with these terms.
*Correspondence: Roy A. Kucuk, cmFrMTI4MzVAdWdhLmVkdQ==
Disclaimer: All claims expressed in this article are solely those of the authors and do not necessarily represent those of their affiliated organizations, or those of the publisher, the editors and the reviewers. Any product that may be evaluated in this article or claim that may be made by its manufacturer is not guaranteed or endorsed by the publisher.
Research integrity at Frontiers
Learn more about the work of our research integrity team to safeguard the quality of each article we publish.