- 1Jilin Provincial Key Laboratory of Radiation Oncology & Therapy, The First Hospital of Jilin University, Changchun, China
- 2Key Laboratory of Pathobiology, Ministry of Education, College of Basic Medical Science, Jilin University, Changchun, China
- 3Department of Gastrointestinal Colorectal and Anal Surgery, China-Japan Union Hospital of Jilin University, Changchun, China
- 4Department of Radiation Oncology, The First Hospital of Jilin University, Changchun, China
- 5NHC Key Laboratory of Radiobiology, School of Public Health, Jilin University, Changchun, China
The prevalence of type 2 diabetes (T2D) is increasing worldwide, with many patients developing long-term complications that affect their cardiovascular, urinary, alimentary, and other systems. A growing body of literature has reported the crucial role of gut microbiota in metabolic diseases, one of which, Akkermansia muciniphila, is considered the “next-generation probiotic” for alleviating metabolic disorders and the inflammatory response. Although extensive research has been conducted on A. muciniphila, none has summarized its regulation in T2D. Hence, this review provides an overview of the effects and multifaceted mechanisms of A. muciniphila on T2D and related diseases, including improving metabolism, alleviating inflammation, enhancing intestinal barrier function, and maintaining microbiota homeostasis. Furthermore, this review summarizes dietary strategies for increasing intestinal A. muciniphila abundance and effective gastrointestinal delivery.
1. Introduction
Diabetes mellitus (DM) refers to a group of metabolic disorders characterized by hyperglycemia, insulin resistance, obesity, and low-grade inflammation (Zimmet et al., 2001). The prevalence of diabetes is increasing at an alarming rate worldwide. In 2017, the International Diabetes Federation estimated that there were 451 million patients with diabetes and 374 million people with impaired glucose tolerance worldwide (Cho et al., 2018). If there is no radical therapy to reverse this expansion, the total number of people with diabetes could increase to 693 million by 2045 (Cho et al., 2018). Type 2 diabetes (T2D) would account for more than 90% of cases in this population (Chatterjee et al., 2017). T2D is a heterogeneous disease, and various factors may contribute to its development, such as unhealthy lifestyle and diet, genetic heritage, aging, and environmental factors (Chatterjee et al., 2017). Due to the long duration of diabetes, T2D patients have higher risks of macrovascular and microvascular damage, as well as other severe complications, such as diabetic nephropathy, diabetic cardiomyopathy, non-alcoholic fatty liver disease (NAFLD), diabetic retinopathy, cognitive impairment, that cause mortality (Gregg et al., 2014). The high incidence of T2D indicates that previous preventive and therapeutic approaches are ineffective. Recent studies have examined the effects of gut microbiota on metabolic diseases, including T2D (Cani, 2018; Gurung et al., 2020). Furthermore, diabetes treatments that target the gut microbiota have become a growing area of investigation.
In the human body, microbial cells outnumber human cells by approximately 10-fold and contribute significantly to host metabolism and immunity (Cani, 2018). As per observational studies in humans, the genera of opportunistic pathogens (i.e., Ruminococcus, Fusobacterium, and Blautia) were enriched in T2D patients, while the genera Bifidobacterium, Bacteroides, Faecalibacterium, and A. muciniphila were negatively associated with T2D (Gurung et al., 2020). Akkermansia muciniphila is a gram-negative, strictly anaerobic, oval-shaped, non-spore-forming mucin-degrading bacterium that belongs to the division Verrucomicrobia and was first isolated from the feces of healthy adults in 2004 through the use of mucin as the only source of carbon and nitrogen (Derrien et al., 2004; Ouwerkerk et al., 2016; Machado et al., 2020). A. muciniphila mostly colonizes the intestinal tract and accounts for approximately 1–3% of the total microbiota (Derrien et al., 2008). The bacteria have been detected in fecal samples of healthy adults of all ages, at levels ranging from 5.00 to 8.80 log cells/g, but their population is reduced among most elderly individuals (Collado et al., 2007). Research on Akkermansia is of growing interest, many animal experiments have determined the positive effects of A. muciniphila supplementation on diabetes and related diseases (Cani and de Vos, 2017). Similarly, the administration of A. muciniphila improved the metabolic parameters of obese patients (Depommier et al., 2019). However, the exact mechanism underlying these positive effects remains unclear. Herein, we summarize research progress on A. muciniphila and describe its role in T2D and related diseases, from its mechanism of action to its therapeutic application.
2. The diversity of Akkermansia muciniphila strains
In light of the known benefits of A. muciniphila, researchers have attempted to determine its genome and the functions of the encoded proteins. A. muciniphila belongs to the phylum Verrucomicrobia and displays significant diversity among the different strains (van Passel et al., 2011). Guo et al. (2017) constructed the genomes of 39 A. muciniphila strains isolated from adult humans and laboratory mice and identified three major phylogroups using maximum likelihood phylogenetic analysis. Later, Kirmiz et al. (2020) reclassified A. muciniphila isolates into four species-level phylogroups based on a predecessor (defined as AmI, AmII, AmIII, AmIV). Becken et al. (2021) suggested that the AmI phylogroup can be phylogenetically divided into two subclades, AmIa and AmIb. At present, the commonly used strain in most researches is A. muciniphila MucT (=ATCC BAA-835T = CIP 107961T), belonging to AmIa. Differences among these four phylogroups include intestinal abundance, physicochemical properties, metabolic characteristics, and immune activation capacity. In a study of 1617 human fecal samples (Karcher et al., 2021), AmI accounted for the highest proportion (47%), followed by AmII and AmIII (27 and 24%, respectively). AmII and AmIII are currently only observed in humans, whereas AmI and AmIV are common in humans and mice in different proportions. Another metagenomic analysis showed that AmIV is mainly distributed in western populations, while AmIII is mainly found in China (Lv et al., 2022).
Genome sequencing has revealed the genes differentially expressed among the four phylogroups, AmI, AmII, AmIII, and AmIV. According to the Kyoto Encyclopedia of Genes and Genomes (KEGG) pathway analysis, genes in the A. muciniphila genome encode proteins involved in membrane transport, drug resistance, and various metabolic activities (Guo et al., 2017). Metabolism-related genes have attracted attention due to their function in improving host metabolism. Several studies have reported differential expression of genes related to glycolipid metabolism and amino acid metabolism among A. muciniphila phylogroups. A principal component analysis of the KEGG pathway showed greater similarities between the AmII and AmIII genomes, whereas AmI genomes differed significantly. Specifically, all four A. muciniphila phylogroups encoded glycoside hydrolases (GHs) and glycosyltransferases (GTs), but the copy numbers of the GH and GT families differed from one another (Becken et al., 2021; Luna et al., 2022). The genomes of the AmI phylogroup contained fewer genes encoding fucosidases (GH29 and GH95) than the other phylogroups that potentially act on the terminal fucose residues that decorate mucin and human milk oligosaccharides (Wu et al., 2021). Notably, AmIb had fewer GH29 genes than AmIa and no GH18 genes (Luna et al., 2022), and galactosidase GH110 was hardly expressed in AmIV (Becken et al., 2021). Similarly, the four phylogroups varied in the proportions of GT2 and GT4, suggesting that they differ in their carbohydrate metabolic levels, especially the ability to synthesize polysaccharides (Karcher et al., 2021). Furthermore, cobalamin (vitamin B12) is an essential cofactor for short-chain fatty acid metabolism. Kirmiz et al. (2020) found that vitamin B12 synthesis genes were absent in AmI. This finding suggests that the vitamin B12 biosynthesis genes were originally present in all strains and were lost by some isolates in the human gut (Karcher et al., 2021). Considering that several intestinal microbes require vitamin B12 as a metabolic substrate (Degnan et al., 2014), these genetic losses may explain the diversity of A. muciniphila and its interactions with the host and other intestinal microbes. Genes for assimilatory sulfate reduction (ASR) were absent in AmII and AmIV. This resulted in the absence of hydrogen sulfide, a critical substrate for cysteine and methionine synthesis, and led to a low growth rate in the mucin medium (Becken et al., 2021). Additionally, AmIV’s high sensitivity to oxygen may be attributed to a deficiency in siderophores (Becken et al., 2021). These results explain the observed differences in the distribution and oligosaccharide utilization of A. muciniphila phylogroups (Luna et al., 2022), and may also explain the reason for AmI and AmII to have different therapeutic efficacies in alleviating metabolic syndrome (Deng et al., 2020).
The relationship between A. muciniphila and colitis is controversial. Several lines of evidence have shown that A. muciniphila administration can ameliorate inflammatory bowel diseases (IBD) or delay colitis-associated tumorigenesis in mice (Wang et al., 2020; Wade and Su, 2021), whereas certain oral treatments for colitis have enriched the abundance of A. muciniphila (Ke et al., 2021). While some studies have suggested that A. muciniphila aggravates IBD (Ganesh et al., 2013), Ring et al. (2019) proposed that ingestion of the A. muciniphila strain ATCC BAA-835 did not exacerbate intestinal inflammation in interleukin (IL)-10-deficient mice. Notably, Cekanaviciute et al. (2017) found increased A. muciniphila levels in MS patients. The investigators hypothesized that this difference might be due to strain specificity (Ring et al., 2019), and Liu’s findings substantiated their hypothesis (Liu Q et al., 2021). A. muciniphila displayed strain-dependent effects on ulcerative colitis in mice. AmII and AmIV exerted stronger immune activation than AmI in HEK-TLR reporter cell lines (Becken et al., 2021). In summary, strain specificity of the gut microbiota is vital for phenotypic studies (Geva-Zatorsky et al., 2017), and A. muciniphila also exhibited strong strain specificity. Most current studies have focused on the strain ATCC BAA-835T (=CIP 107961T), and further investigation should be conducted to gain deeper insights into the interactions between the host and A. muciniphila.
3. The role of Akkermansia muciniphila in T2D and related diseases
Typical manifestations of diabetes include obesity, insulin resistance, impaired glucose tolerance, abnormal lipid metabolism, and low-grade systemic inflammation (Weir and Bonner-Weir, 2004). Furthermore, human gut microbial sequencing revealed a decline in A. muciniphila abundance in T2D patients as early as 10 years ago (Zhang et al., 2013). Numerous studies have also found reduced A. muciniphila levels associated with not only diabetes (Yassour et al., 2016; Chelakkot et al., 2018; Medina-Vera et al., 2019), but also its related diseases, including hyperlipidemia (Yu et al., 2021), non-alcoholic fatty liver disease (Kim et al., 2020; Zhang et al., 2022), and chronic kidney disease (Hsu et al., 2020). Since Everard et al. (2013) discovered the effect of A. muciniphila on alleviating metabolic disorders, researchers have conducted many investigations on this next-generation microorganism, as summarized in Table 1. Several cohort studies have revealed the beneficial effects of A. muciniphila on glucose and lipid metabolism and inflammatory responses in humans (Depommier et al., 2019; Perraudeau et al., 2020). Furthermore, a few animal experiments have also demonstrated multiple benefits of A. muciniphila in liver disease, cardiovascular disease, cognitive impairment, and aging (Grajeda-Iglesias et al., 2021). The diseases related to A. muciniphila and several classes of oral supplements, including polyphenols, saccharides, and flavonoids, that increase the abundance of A. muciniphila are outlined in Figure 1. Furthermore, pasteurized A. muciniphila has been confirmed to be safe and was approved for use by the European Food Safety Authority in 2021 [Efsa Panel on Nutrition, Novel Foods and Food Allergens (NDA) et al., 2021].
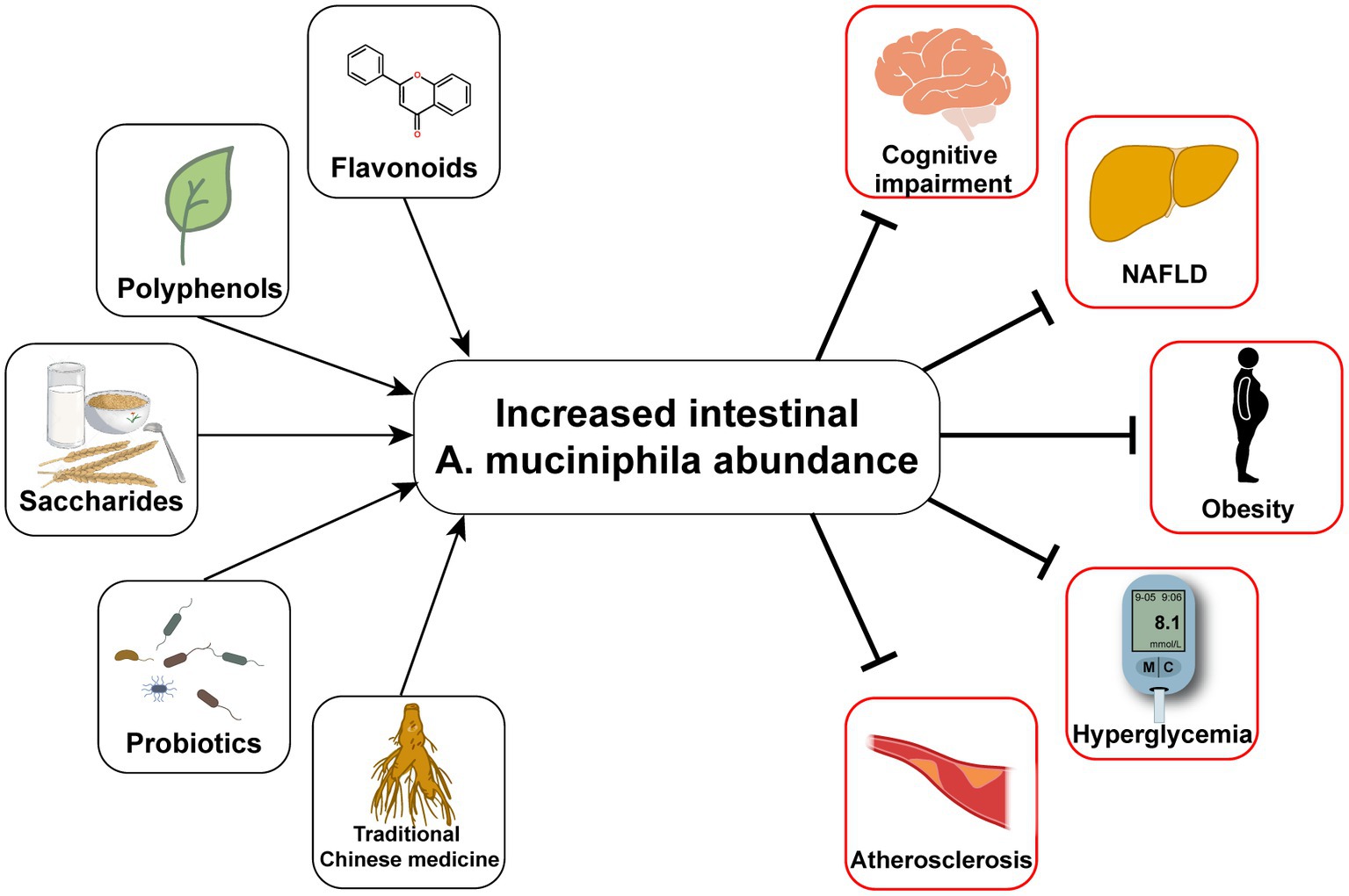
Figure 1. Oral measures to improve the abundance of Akkermansia muciniphila and the consequent improvement of T2D and its complications.
4. Regulatory mechanisms of Akkermansia muciniphila in T2D and related diseases
4.1. Akkermansia muciniphila treatment improves host metabolism
Insulin resistance and metabolic syndrome are the most prominent characteristics of T2D, and A. muciniphila alleviates metabolic syndrome by improving glucose, lipid, and bile acid metabolism. Glucagon-like peptide-1 (GLP-1) is a pleiotropic hormone that has a broad role in metabolism regulation, including the stimulation of insulin secretion and appetite suppression (Muller et al., 2019), both of which contribute to diabetes treatment. Propionate, an A. muciniphila metabolite, has been shown to stimulate GLP-1 secretion (Chambers et al., 2015; Psichas et al., 2015). Furthermore, Yoon et al. (2021) proposed a new GLP-1 activator, intercellular adhesion molecule 2 (ICAM-2), which is an immune cell integrin (Iannacone, 2016). ICAM-2, when directly combined with A. muciniphila-derived protein P9, induced GLP-1 secretion and ameliorated hyperglycemia. Additionally, Xia et al. (2022) observed the activation of the phosphatidylinositol 3-kinase (PI3K)-Akt pathway in mouse liver upon gavage of A. muciniphila. Notably, PI3K-Akt signaling plays an important role in glucose and lipid metabolism (Huang et al., 2018).
Scientists have reported that A. muciniphila regulates lipid metabolism in many ways in mice and organoids (Lukovac et al., 2014; Katiraei et al., 2020), mainly in the liver, small intestine, and adipose tissue. In the intestine, A. muciniphila degrades mucin and produces a variety of bioactive metabolites, such as polysaccharides, short-chain fatty acids (SCFA), and indole derivatives. Propionate modulates the expression of genes involved in fatty acid uptake and oxidation, such as Fiaf, Gpr43, peroxisome proliferator-activated receptor gamma (PPARγ), and histone deacetylases (HDACs) (Lukovac et al., 2014; Yoon et al., 2021). In mouse adipose tissue, A. muciniphila administration reduces white adipose tissue (WAT) volume and enhances thermogenesis by upregulating the uncoupling protein 1 (Ucp1)(Deng et al., 2020; Yoon et al., 2021), and downregulating the expression of lipid-droplet regulator associated protein perilipin2 (Depommier et al., 2020; Juarez-Fernandez et al., 2021). Increased colonization of A. muciniphila regulated a network of genes involved in lipid transportation and oxidation in hepatocytes. Shen et al. (2016) found that the reduction in hepatic low-density lipoprotein (LDL) receptor and apolipoprotein E levels in response to treatment with A. muciniphila contributed to the clearance of the plasma triglyceride-rich lipoprotein and chylomicron remnants in mice. Additionally, both Zhao et al. (2017) and Rao et al. (2021) reported altered expression of fatty acid translocase (FAT) with different trends. Notably, Rao et al. identified several significant differential metabolites in the mouse liver between groups with or without the administration of A. muciniphila. L-aspartate displayed a high fold-change (Rao et al., 2021). Additional research has shown that A. muciniphila facilitates L-aspartate transportation in the gut-liver axis for the activation of the liver kinase B1 (LKB1)-AMPK pathway and increases lipid oxidation, thereby ameliorating liver steatosis (Rao et al., 2021).
Bile acids (BAs) are steroid molecules that are synthesized from cholesterol in the liver and act as metabolic regulators via the nuclear farnesoid X receptor (FXR) and takeda G protein-coupled receptor 5 (TGR5)(Sonne, 2021). After the administration of the synbiotic of A. muciniphila and quercetin, plasma primary bile acids to secondary bile acids ratio was significantly increased in mice (Juarez-Fernandez et al., 2021). Besides, the elevated ratio of free to conjugated BA (CA/GCA + TCA) denoted the enhanced activity of bile salt hydrolase (BSH) (Juarez-Fernandez et al., 2021), which promoted the deconjugation of conjugated BA and conversion to free BA. However, BSH activity has been detected in various other gastrointestinal bacteria but not in A. muciniphila (Horackova et al., 2018). The increased BSH activity was probably due to the regulatory action of A. muciniphila on other intestinal bacteria. An observational study discovered a positive relationship between the proportion of primary and conjugated BAs with NAFLD (Puri et al., 2018); therefore, the changes in BAs induced by synbiotic treatment might have a paradoxical role in NAFLD.
Zhang J. et al. (2021) reported that treatment with A. muciniphila promoted insulin secretion by limiting the availability of 3β-chenodeoxycholic acid (βCDCA) in mice. CDCA was shown to be a high-affinity FXR agonist synthesized in the liver (Makishima et al., 1999), whereas βCDCA was derived from CDCA and had different configurations and functions. CDCA-mediated FXR stimulation occurs in ileal enterocytes and hepatocytes and mediates the expression of fibroblast growth factor 15/19 (FGF 15/19) and small heterodimer protein (SHP) (Sonne, 2021). The FXR-FGF15/19 and FXR-SHP pathways are critical for maintaining metabolic homeostasis. Interestingly, FXR agonists and inhibitors displayed beneficial effects on metabolic disorders, requiring more in-depth research. Zhang J. et al. (2021) observed that treatment with A. muciniphila limited βCDCA synthesis in mice, thereby triggering FGF19 signaling and insulin secretion. Of note, the content of CDCA is low in mice, but the authors also found that serum βCDCA negatively correlated with the relative abundance of A. muciniphila in clinical studies.
These results illustrate that A. muciniphila regulates the host metabolism of glucose, lipids, and bile acids through multiple signaling pathways, thereby alleviating insulin resistance, hyperglycemia, and lipid deposition in various organs, as outlined in Figure 2.
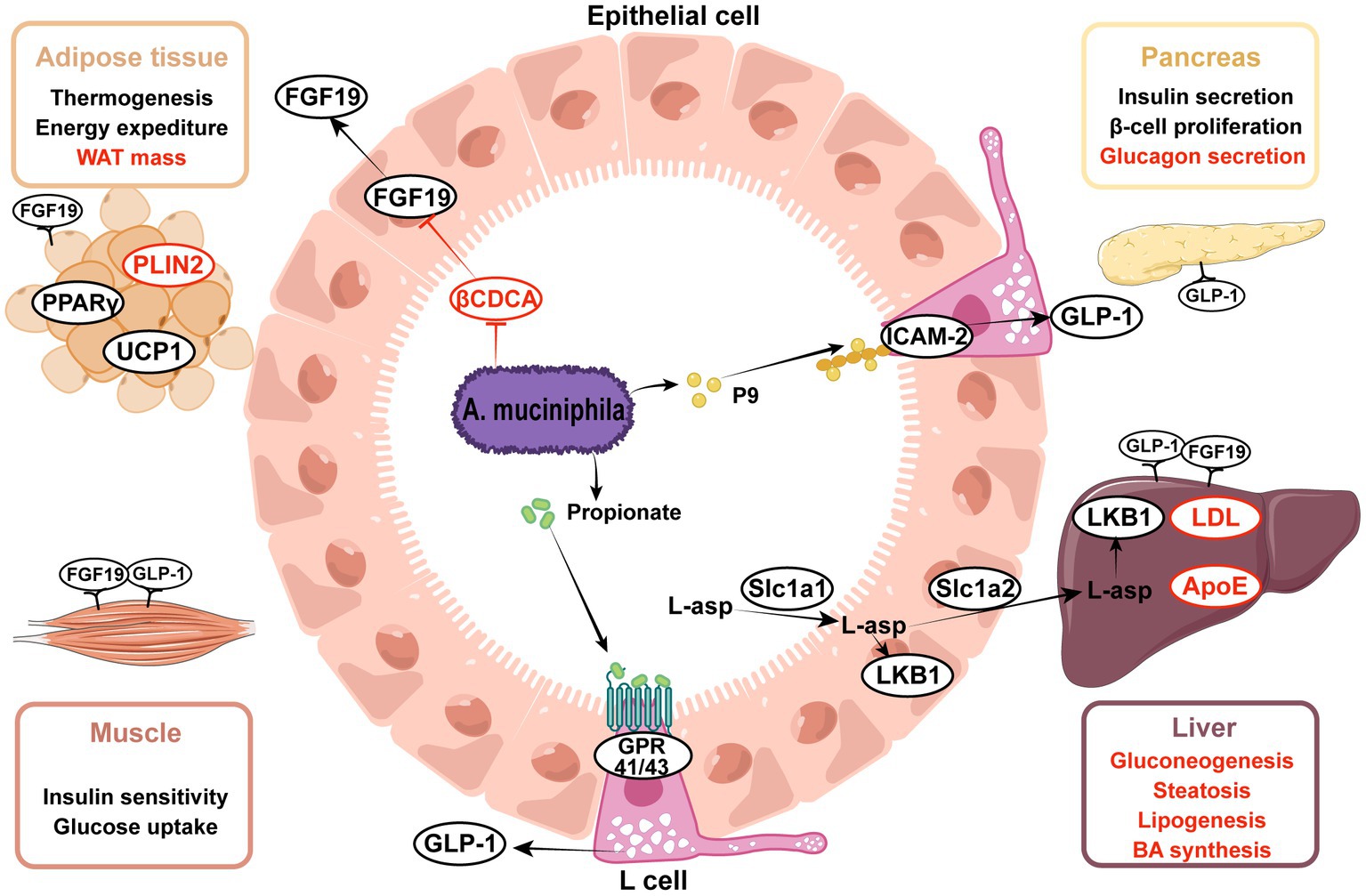
Figure 2. Effects of Akkermansia muciniphila and its derived parts on ameliorating metabolic disorders. (1) A. muciniphila-derived protein P9 directly combined with ICAM-2 and activated the GLP-1 secretion in intestinal L cells, propionate was the metabolite of A. muciniphila and also activated the GLP-1, thereby enhancing insulin secretion and alleviating hyperglycemia. (2) A. muciniphila modulated the expression of genes involved in lipid metabolism, including PPARγ, PLIN2, UCP1, ApoE, LDL. (3) A. muciniphila facilitated the transportation of L-aspartate from gut to liver, L-aspartate upregulated the LKB1-AMPK pathway and ameliorated liver steatosis. (4) A. muciniphila decreased the level of βCDCA, which was one of the FXR inhibitors, thus increasing the intestinal FGF15/19 secretion. FGF15/19 acted on multiple tissues, improved hyperlipidemia and liver steatosis. Upward aspects are indicated in black, downward aspects are indicated in red. ICAM-2, intercellular adhesion molecule 2; GLP-1, glucagon-like peptide-1; FGF15/19, fibroblast growth factor 15/19; βCDCA, 3β-chenodeoxycholic acid; FXR, farnesoid X receptor; L-asp., L-aspartate; LKB1, liver kinase B1; HDAC, histone deacetylases; UCP1, uncoupling protein 1; PLIN2, perilipin2; PPARγ, peroxisome proliferator-activated receptor gamma; LDL, low-density lipoprotein; ApoE, apolipoprotein E.
4.2. Akkermansia muciniphila treatment protects the host from endotoxemia
Previous research has found that patients with diabetes were more susceptible to metabolic endotoxemia than healthy people because of the increased infiltration of lipopolysaccharide (LPS) from the intestine into circulation. Notably, LPS infiltration exacerbated insulin resistance and diabetes and led to various diabetic complications (Cani et al., 2007; Gomes et al., 2017). Improved gut permeability and reduced LPS infiltration after treatment with A. muciniphila have been confirmed in many animal studies (Li et al., 2016; Zhao et al., 2017; Shi et al., 2021; Guo et al., 2022). The intact gut barrier consists of three main interconnected layers: the mucus, gut epithelial, and inner mucosal immune layers. Immunohistochemical staining of colon sections has shown that the colonic mucus layer is significantly thicker in A. muciniphila-treated mice than in vehicle-treated mice (Hanninen et al., 2018; van der Lugt et al., 2019), this may be due to the increased goblet cell count and reduced crypt depth. Furthermore, as a chemical barrier, the mucus layer contains mucin, antimicrobial peptides (AMP), immune cytokines, and digestive secretions. Mucin2 (Muc2) is a basic gel-forming mucin present in the mucus barrier (Johansson et al., 2011). Both in vivo and in vitro experiments have reported that the elevated Muc2 gene expression upon treatment with A. muciniphila corresponds with morphological changes and strengthens mucus barrier function (Ganesh et al., 2016; Ke et al., 2021).
The second layer of the gut barrier is composed of a monolayer of intestinal epithelial cells and intercellular tight junction (TJ) proteins, with a scattered distribution of functionally specialized cells (e.g., Paneth, goblet, and enteroendocrine cells) (Ghosh et al., 2021). After treating chickens with A. muciniphila, Zhu et al. (2020) found the Wnt/β-catenin signaling pathway to be activated in the intestinal stem cells, which is vital for developing and renewing the intestinal epithelium. Kim et al. (2021) further revealed that Wnt signaling activation was mediated by Paneth cells that secreted Wnt3 in the crypt, thereby accelerating intestinal stem cell proliferation. A. muciniphila increase the expression of tight junction proteins in mice, including zonula occludens-1 (ZO-1), occludin, claudin-3, junctional adhesion molecules-3 (jam-3) (Li et al., 2016; Wade and Su, 2021). These proteins form a complex of intercellular junctions that act as an intercellular barrier that separates luminal contents from the subepithelial interstitium (Slifer and Blikslager, 2020). However, the change in claudin-2 expression remains controversial: Liu J. H. et al. (2020) reported increased intestinal expression of claudin-2 in A. muciniphila-treated mice, while several studies found claudin-2 expression to be inhibited (van der Lugt et al., 2019; Wade and Su, 2021). Notably, all these studies confirmed the positive effects of A. muciniphila on intestinal permeability and inflammation. Claudin-2 is a pore-forming claudin that functions as a paracellular channel and increases epithelial layer permeability (Günzel and Yu, 2013). Claudin-2 expression is more likely to have declined considering the improvement in barrier function, but the precise mechanism through which A. muciniphila regulates claudin-2 expression remains to be elucidated.
The last line of defense consists of numerous immunocytes and immunoreactive substances. Several animal experiments have reported that A. muciniphila supplementation induced variations in immune cell composition (Katiraei et al., 2020), and enhanced peritoneal leukocyte functions, including chemotactic activity, phagocytic efficacy, natural killer activity, and lymphoproliferative capacity (Cerro et al., 2022). The known reason may be that A. muciniphila alleviates the intestinal barrier dysfunction through TLR2 activation (Shi et al., 2022), or maintains the function of intestinal barrier by activating alpha kinase 1 and downstream Nuclear Factor-Kappa B (Martin-Gallausiaux et al., 2022). Some anti-inflammatory cytokines, such as IL-10 (Ottman et al., 2017b; Katiraei et al., 2020; Yaghoubfar et al., 2020), α-tocopherol, and β-sitosterol (Zhao et al., 2017), positively correlate with A. muciniphila abundance. In contrast, the levels of several pro-inflammatory factors, such as IL-1β, IL-6, IL-8, and leptin (Ke et al., 2021; Cerro et al., 2022), displayed a downward trend. Ottman et al. (2017b) calculated the ratio of the pro-inflammatory factor TNF-α levels to the anti-inflammatory cytokine IL-10 levels in mice, which is an important parameter for estimating the inflammatory regulation capacity of gut microbiota. Compared to F. prausnitzii and L. plantarum, A. muciniphila showed the lowest ratio, implying it has high anti-inflammatory activity. Aryl hydrocarbon receptor (AhR) is a transcriptional regulator widely expressed in the intestinal epithelium and mediates antimicrobial immunity. Furthermore, impaired AhR agonist activity and lower AhR ligand concentrations are associated with metabolic disorders and intestinal barrier dysfunction (Natividad et al., 2018). AhR ligands include several indole derivatives, such as indoleacetic acid (IAA), indole-3-ethanol, and indole acrylic acid (IA), produced by tryptophan (Trp) metabolism (Gu et al., 2021; Shi et al., 2021). Gu et al. (2021) noted that A. muciniphila raised the plasma concentrations of IAA and IA in mice, both of which combine with AhR and activate its downstream signals. This was evidenced by increased CYP1A1, interleukin-10 (IL-10), and IL-22 levels that consequently alleviate metabolic syndrome and inflammation.
The above literature provides important insights into the mechanism by which A. muciniphila consolidates the intestinal barrier and regulates the balance of pro−/anti-inflammatory factors, thereby protecting the host from endotoxemia and metabolic disorders (Figure 3). However, the role of A. muciniphila in colitis remains controversial. Notably, A. muciniphila cannot be completely classified as anti-or pro-inflammatory, but undoubtedly, plays an essential role in endotoxemia and diabetes-related chronic inflammation (Everard et al., 2013; Plovier et al., 2017; Hanninen et al., 2018).
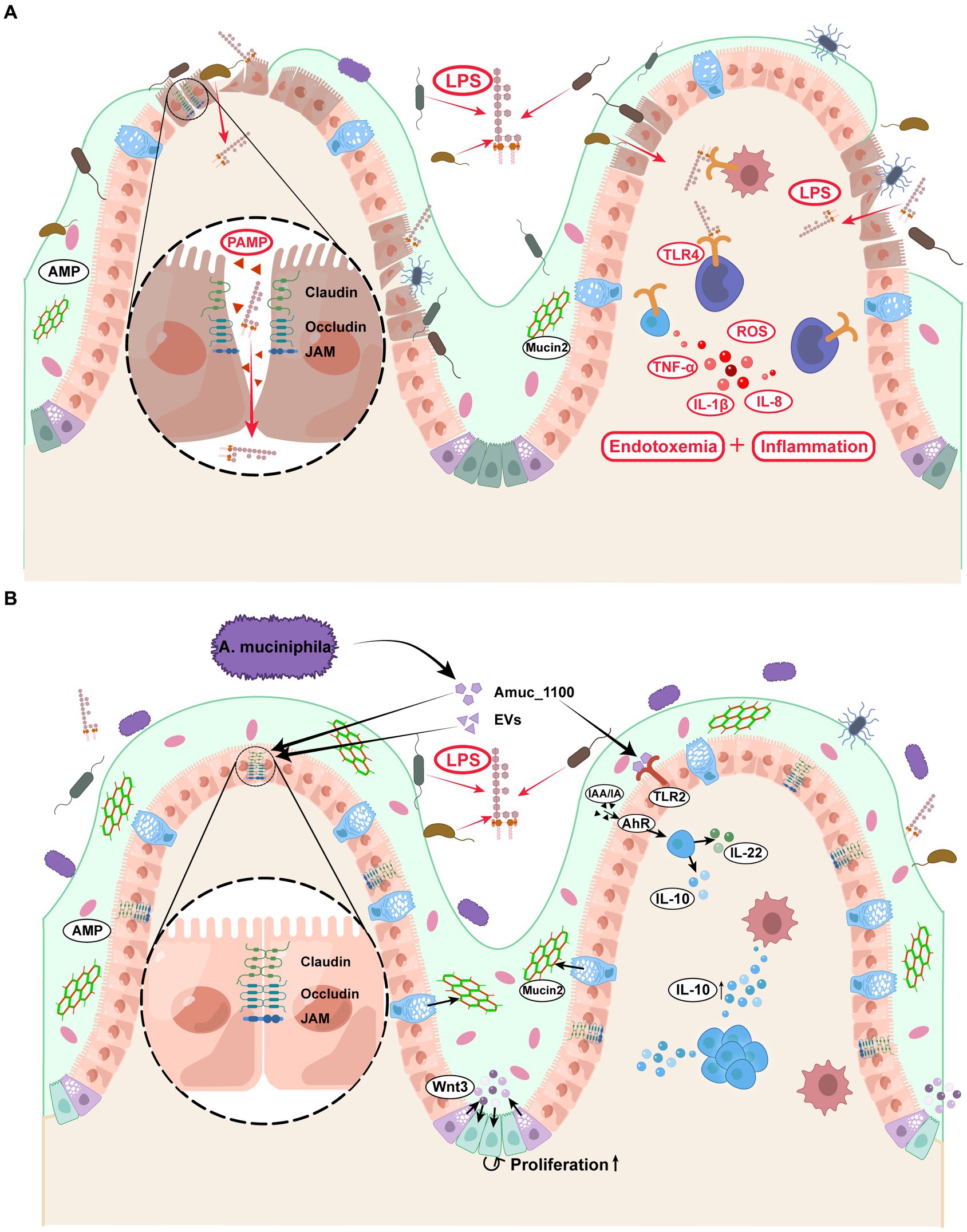
Figure 3. Effects of Akkermansia muciniphila and its derivatives on the enhancement of intestinal barrier and the prevention of endotoxemia. (A) Diabetic patients displayed increased gut permeability and LPS absorption, leading to the accumulation of inflammatory factors and metabolic endotoxemia. (B) (1) A. muciniphila and its derivatives directly activated the intestinal TLR2 and AhR, thereby regulating the ratio of pro−/anti-inflammatory factors. (2) A. muciniphila and its derivatives strengthened the function of tight junction between intestinal epithelial cells and promoted the intestinal stem cell proliferation. (3) A. muciniphila increased the Mucin2 secretion from the goblet cells, which was a major protein in the mucus layer. A. muciniphila consolidated the above three layers of intestinal barrier and prevented the LPS penetration. EVs, extracellular vesicles; LPS, lipopolysaccharide; TLR2, Toll-like receptor 2; AhR, aryl hydrocarbon receptor; IL-10, interleukin-10; IL-22, interleukin-22; AMP, antimicrobial peptide.
4.3. Akkermansia muciniphila regulates intestinal microbial homeostasis
A wide variety of microbiota colonize the human body and constitute a complex ecosystem. Maintaining the homeostasis of this ecosystem is essential for human health. Drastic changes in the composition or distribution of the microbiota result in intestinal microbial dysbiosis, which leads to various metabolic and inflammatory diseases. Several studies, having benefited from the advent of metagenomic sequencing, described the different compositions of gut microbiota between patients with T2D and healthy individuals (Qin et al., 2010). Generally, T2D has been correlated with increased levels of opportunistic pathogens (e.g., Ruminococcus, Fusobacterium, and Blautia) and decreased levels of SCFA-producing bacteria (e.g., Bifidobacterium, Bacteroides, Faecalibacterium, A. muciniphila, and Roseburia) (Gurung et al., 2020). The lower intestinal abundance of A. muciniphila has been demonstrated in both T2D mice and patients (Everard et al., 2013; Yassour et al., 2016). Although there is no exact definition of a healthy gut microbiome composition, a high level of microbial diversity is certainly important. After treating mice with A. muciniphila, the α-diversity of their fecal microbiome was found to increase, as measured by Shannon’s diversity index, Ace, and Chao1 (Bian et al., 2019; Kim et al., 2021; Rao et al., 2021). These results imply that A. muciniphila can improve the richness and diversity of gut microbiota, which is a prerequisite for intestinal stability.
Firmicutes and Bacteroidetes are the two most abundant phyla in the human intestine (Qin et al., 2010). Human obesity is thought to be associated with an increased ratio of Firmicutes/Bacteroidetes (Magne et al., 2020), which may be due to differences in energy metabolism and inflammatory response (Krajmalnik-Brown et al., 2012; Bian et al., 2019). Notably, A. muciniphila was found to rescue mice with diabetes and NAFLD from Firmicutes/Bacteroidetes imbalance (Hanninen et al., 2018; Perez-Monter et al., 2022). Firmicutes and Bacteroidetes also differ in their SCFA profiles but may not be involved in the regulation of SCFA by A. muciniphila (Magne et al., 2020). In addition, the abundance of certain potential probiotics, such as Lactobacillus and Verrucomicrobia, has increased upon colonization with A. muciniphila (Bian et al., 2019; Xia et al., 2022).
However, the changes in intestinal microbiota following A. muciniphila administration reported in the current articles were not identical. For example, Hanninen et al. (2018) found that 4-week treatment with oral A. muciniphila reduced the abundance of Ruminococcus, while Bian et al. (2019) drew an opposite conclusion that the relative abundance of Ruminococcus was increased after A. muciniphila treatment compared with the dextran sulfate sodium-treated group. This may be due to the different sample sources for microbiota analysis, Hanninen et al. (2018) isolated bacterial DNA from stools or caecal and colon contents, while Bian et al. (2019) extracted bacterial DNA from fecal samples only. Different frequency and periodicity of A. muciniphila administration also influenced the results. At present, the low reproducibility and high bias of microbial sequencing results may be explained by methodological differences in sample sources and sequencing technologies, as well as the lack of consideration of environmental factors (Cani, 2018). Notably, A. muciniphila is a crucial regulator of the gut microbiota balance, and its precise role in the intestinal ecosystem remains to be explored.
4.4. Components of Akkermansia muciniphila active against T2D
Culture conditions can affect the potency of probiotics, live and heat-killed bacteria may differ in functions. Recent studies have focused on the active components of A. muciniphila. Heat-killed A. muciniphila is generally considered ineffective (Everard et al., 2013). Contrarily, many studies have argued that pasteurized A. muciniphila is equivalent to live A. muciniphila in providing metabolic improvement (Plovier et al., 2017; Depommier et al., 2020), and inflammation relief in obese and diabetic mice (Choi et al., 2021). Furthermore, pasteurized A. muciniphila has displayed increased effectiveness over the live microorganism in certain studies (Ashrafian et al., 2021). Notably, a recent study reported the anti-fibrotic properties of heat-killed A. muciniphila in human LX-2 cells, suggesting a possible role in liver fibrosis (Keshavarz Azizi Raftar et al., 2021). The above experiments indicate that certain critical substances from A. muciniphila are insensitive to heat and remain active after pasteurization or heating at 95°C. To determine the key components, Ottman et al. (2016) identified 79 outer membrane proteins of A. muciniphila using proteomics. Among these, Amuc_1100, encoded by the type IV pili gene cluster, is one of the most abundant proteins. Many studies have suggested that Amuc_1100 could partially recapitulate the beneficial effects of A. muciniphila in mice with diabetes or colitis (Plovier et al., 2017; Ottman et al., 2017b; Wang et al., 2020). Therefore, scientists have explored the underlying mechanism of Amuc_1100 functions, such as the activation of AhR (Gu et al., 2021), Toll-like receptor 2 (TLR2), and TLR4 (Plovier et al., 2017; Ottman et al., 2017b; Wang et al., 2021), reduction of colonic cytotoxic T lymphocytes (Wang et al., 2020), and inhibition of lipid synthesis and transport genes (Zhang F. L. et al., 2021), thereby alleviating T2D and related diseases. A recent study identified a lipid from A. muciniphila’s cell membrane as the main role of regulating immune homeostasis and dissected its structure and the mechanism of binding to toll-like receptors (Bae et al., 2022).
In addition, bacterial extracellular vesicles (EV) mediate signal transmission between the gut microbiota and the host (Villard et al., 2021). A. muciniphila-derived EVs (AmEV) play a significant role in improving the intestinal barrier (Chelakkot et al., 2018; Ashrafian et al., 2019), and regulating serotonin levels (Yaghoubfar et al., 2020). Thus, AmEV exhibits anti-inflammatory action and metabolic regulation. In detail, AmEV relieves inflammation by strengthening tight junctions and regulates metabolic imbalance by improving glucose tolerance (Chelakkot et al., 2018). In summary, AmEV and the outer membrane protein Amuc_1100 possess partial functions of A. muciniphila; however, further experimental investigations are needed to evaluate their potency and full potential as therapeutic agents.
5. Cultivation and measures to enrich the intestinal abundance of Akkermansia muciniphila
The ameliorative effects and mechanisms of A. muciniphila on T2D and related metabolic diseases have been described previously, which remind us that A. muciniphila is a promising probiotic and that oral administration of A. muciniphila may alleviate metabolic disorders. The method of cultivation and administration of A. muciniphila can greatly affect its function. Owing to the potential of A. muciniphila in treating metabolic diseases, many researchers have explored the most suitable growing environment and method for A. muciniphila in the intestine. Suitable culture conditions will allow A. muciniphila to survive better so that they can be administered by gavage to observe their effect on metabolic diseases. When A. muciniphila was first discovered by Derrien et al. (2004) this bacterium was defined as strictly anaerobic and grew well on mucin medium, with an optimal pH of 6.5 and growth temperature of 37°C. However, later studies suggested that A. muciniphila could grow under a low oxygen concentration (Ouwerkerk et al., 2016; Machado et al., 2020). Ottman et al. performed a transcriptomic and proteomic analysis on A. muciniphila cultured in mucus and non-mucus sugars. The results showed that several mucin-derived monosaccharides could be utilized by A. muciniphila. Furthermore, the addition of mucin enhanced the uptake of monosaccharides and the growth rate of A. muciniphila (Ottman et al., 2017a). The investigators also demonstrated that hexosamines, N-acetylgalactosamine (GalNAc), and N-acetylglucosamine (GlcNAc) served as critical nitrogen sources in the mixed sugar medium; Plovier’s discovery also supported this finding. Notably, the researchers found that the A. muciniphila cultured in a medium supplemented with glucose, GlcNAc, soy peptone, and threonine had the same growth efficiency as that in a mucus-based medium (Plovier et al., 2017). Additionally, a recent study reported that only when the concentration of mucin reached 0.5% m/v in the medium could the metabolic characteristics of A. muciniphila be altered (Liu X et al., 2021).
Oral drugs need to pass through the stomach to reach the intestine, so to achieve the purpose of drug delivery, A. muciniphila should be delivered in a way that maintains their activity as much as possible. However, probiotic delivery methods require improvement. Considering the characteristics of A. muciniphila and the gastric oxygen-rich and acidic environment, researchers have attempted to encapsulate live A. muciniphila in a water-in-oil-in-water double emulsion (van der Ark et al., 2017), freeze-dried xanthan, gellan gum matrix (Marcial-Coba et al., 2018), and spray-dried modified sodium alginate (Chang et al., 2020). A. muciniphila microencapsulation reinforced its activity and survival rate in gastrointestinal transit. Further research on microencapsulation would be of great help in improving the next-generation probiotics. In addition to direct A. muciniphila gavage, the intake of multiple dietary components and medicines, classified as polyphenols, flavonoids, probiotics, alkaloids, etc., could increase the abundance of A. muciniphila (Table 2).
6. Conclusions and perspective
Ever since it was isolated, A. muciniphila has been considered a therapeutic target for metabolic diseases, especially T2D. Although accumulating evidence has shown the positive effects of A. muciniphila on T2D and related diseases, the underlying mechanisms remain obscure. In this review, we focused on the mechanisms by which A. muciniphila ameliorates T2D and its potential as a next-generation probiotic. However, certain problems remain to be addressed. First, most controlled experiments were conducted using experimental animals as hosts; thus, more large-scale clinical studies should be performed to validate the function of A. muciniphila in humans. Second, the reproducibility of microbial gene sequencing has been complicated by environmental factors and methodological divergences. Therefore, sampling from both stool samples and different parts of the intestine may be necessary to ensure the consistency of the experimental findings. Furthermore, it would make more sense to focus on the microbial dynamic changes associated with A. muciniphila administration rather than sampling at a fixed time. Third, the discovery of A. muciniphila-derived substances provides a step forward in identifying potential therapeutics, and it is necessary to evaluate their bioequivalences with A. muciniphila itself. Finally, considering the heterogeneity of diabetes, individualized research and treatment options for microbiota are essential.
Author contributions
YX and XJ: conceptualization, validation, writing—review and editing, and funding acquisition. JL: methodology, formal analysis, and writing—original draft preparation. QZ: software. GY: investigation. YX: resources and project administration. ZL, data curation. XJ: visualization and supervision. All authors contributed to the article and approved the submitted version.
Funding
This research was funded by the National Natural Science Foundation of China (No. 82170369), the Jilin Provincial Science and Technology Foundation (Nos. 20210509003RQ and 20210402002GH), and the Changchun Science and Technology Bureau Development Plan project (No. 21ZY29).
Acknowledgments
The authors would like to thank Editage (www.editage.cn) for English language editing.
Conflict of interest
The authors declare that the research was conducted in the absence of any commercial or financial relationships that could be construed as a potential conflict of interest.
Publisher’s note
All claims expressed in this article are solely those of the authors and do not necessarily represent those of their affiliated organizations, or those of the publisher, the editors and the reviewers. Any product that may be evaluated in this article, or claim that may be made by its manufacturer, is not guaranteed or endorsed by the publisher.
References
Anhe, F. F., Roy, D., Pilon, G., Dudonne, S., Matamoros, S., Varin, T. V., et al. (2015). A polyphenol-rich cranberry extract protects from diet-induced obesity, insulin resistance and intestinal inflammation in association with increased Akkermansia spp. population in the gut microbiota of mice. Gut 64, 872–883. doi: 10.1136/gutjnl-2014-307142
Anhe, F. F., Varin, T. V., Le Barz, M., Pilon, G., Dudonne, S., Trottier, J., et al. (2018). Arctic berry extracts target the gut-liver axis to alleviate metabolic endotoxaemia, insulin resistance and hepatic steatosis in diet-induced obese mice. Diabetologia 61, 919–931. doi: 10.1007/s00125-017-4520-z
Ashrafian, F., Keshavarz Azizi Raftar, S., Shahryari, A., Behrouzi, A., Yaghoubfar, R., Lari, A., et al. (2021). Comparative effects of alive and pasteurized Akkermansia muciniphila on normal diet-fed mice. Sci. Rep. 11:17898. doi: 10.1038/s41598-021-95738-5
Ashrafian, F., Shahriary, A., Behrouzi, A., Moradi, H. R., Keshavarz Azizi Raftar, S., Lari, A., et al. (2019). Akkermansia muciniphila-derived extracellular vesicles as a mucosal delivery vector for amelioration of obesity in mice. Front. Microbiol. 10:2155. doi: 10.3389/fmicb.2019.02155
Bae, M., Cassilly, C. D., Liu, X., Park, S. M., Tusi, B. K., Chen, X., et al. (2022). Akkermansia muciniphila phospholipid induces homeostatic immune responses. Nature 608, 168–173. doi: 10.1038/s41586-022-04985-7
Bang, S. J., Lee, E. S., Song, E. J., Nam, Y. D., Seo, M. J., Kim, H. J., et al. (2019). Effect of raw potato starch on the gut microbiome and metabolome in mice. Int. J. Biol. Macromol. 133, 37–43. doi: 10.1016/j.ijbiomac.2019.04.085
Becken, B., Davey, L., Middleton, D. R., Mueller, K. D., Sharma, A., Holmes, Z. C., et al. (2021). Genotypic and phenotypic diversity among human isolates of Akkermansia muciniphila. MBio 12:e00478-21. doi: 10.1128/mBio.00478-21
Bian, X., Wu, W., Yang, L., Lv, L., Wang, Q., Li, Y., et al. (2019). Administration of Akkermansia muciniphila ameliorates dextran sulfate sodium-induced ulcerative colitis in mice. Front. Microbiol. 10:2259. doi: 10.3389/fmicb.2019.02259
Bu, F., Ding, Y., Chen, T., Wang, Q., Wang, R., Zhou, J. Y., et al. (2021). Total flavone of Abelmoschus Manihot improves colitis by promoting the growth of Akkermansia in mice. Sci. Rep. 11:20787. doi: 10.1038/s41598-021-00070-7
Cani, P. D. (2018). Human gut microbiome: hopes, threats and promises. Gut 67, 1716–1725. doi: 10.1136/gutjnl-2018-316723
Cani, P. D., Amar, J., Iglesias, M. A., Poggi, M., Knauf, C., Bastelica, D., et al. (2007). Metabolic endotoxemia initiates obesity and insulin resistance. Diabetes 56, 1761–1772. doi: 10.2337/db06-1491
Cani, P. D., and de Vos, W. M. (2017). Next-generation beneficial microbes: the case of Akkermansia muciniphila. Front. Microbiol. 8:1765. doi: 10.3389/fmicb.2017.01765
Cekanaviciute, E., Yoo, B. B., Runia, T. F., Debelius, J. W., Singh, S., Nelson, C. A., et al. (2017). Gut bacteria from multiple sclerosis patients modulate human T cells and exacerbate symptoms in mouse models. Proc. Natl. Acad. Sci. U. S. A. 114, 10713–10718. doi: 10.1073/pnas.1711235114
Cerro, E. D., Lambea, M., Felix, J., Salazar, N., Gueimonde, M., and De la Fuente, M. (2022). Daily ingestion of Akkermansia mucciniphila for one month promotes healthy aging and increases lifespan in old female mice. Biogerontology 23, 35–52. doi: 10.1007/s10522-021-09943-w
Chambers, E. S., Viardot, A., Psichas, A., Morrison, D. J., Murphy, K. G., Zac-Varghese, S. E., et al. (2015). Effects of targeted delivery of propionate to the human colon on appetite regulation, body weight maintenance and adiposity in overweight adults. Gut 64, 1744–1754. doi: 10.1136/gutjnl-2014-307913
Chang, C. J., Lin, C. S., Lu, C. C., Martel, J., Ko, Y. F., Ojcius, D. M., et al. (2015). Ganoderma lucidum reduces obesity in mice by modulating the composition of the gut microbiota. Nat. Commun. 6:7489. doi: 10.1038/ncomms8489
Chang, Y., Yang, Y., Xu, N., Mu, H., Zhang, H., and Duan, J. (2020). Improved viability of Akkermansia muciniphila by encapsulation in spray dried succinate-grafted alginate doped with epigallocatechin-3-gallate. Int. J. Biol. Macromol. 159, 373–382. doi: 10.1016/j.ijbiomac.2020.05.055
Chatterjee, S., Khunti, K., and Davies, M. J. (2017). Type 2 diabetes. Lancet 389, 2239–2251. doi: 10.1016/s0140-6736(17)30058-2
Chelakkot, C., Choi, Y., Kim, D. K., Park, H. T., Ghim, J., Kwon, Y., et al. (2018). Akkermansia muciniphila-derived extracellular vesicles influence gut permeability through the regulation of tight junctions. Exp. Mol. Med. 50:e450. doi: 10.1038/emm.2017.282
Chen, H., Cheng, J., Zhou, S., Chen, D., Qin, W., Li, C., et al. (2021). Arabinoxylan combined with different glucans improve lipid metabolism disorder by regulating bile acid and gut microbiota in mice fed with high-fat diet. Int. J. Biol. Macromol. 168, 279–288. doi: 10.1016/j.ijbiomac.2020.12.036
Cho, N. H., Shaw, J. E., Karuranga, S., Huang, Y., da Rocha Fernandes, J. D., Ohlrogge, A. W., et al. (2018). IDF diabetes atlas: global estimates of diabetes prevalence for 2017 and projections for 2045. Diabetes Res. Clin. Pract. 138, 271–281. doi: 10.1016/j.diabres.2018.02.023
Choi, Y., Bose, S., Seo, J., Shin, J. H., Lee, D., Kim, Y., et al. (2021). Effects of live and pasteurized forms of Akkermansia from the human gut on obesity and metabolic dysregulation. Microorganisms 9:2039. doi: 10.3390/microorganisms9102039
Collado, M. C., Derrien, M., Isolauri, E., de Vos, W. M., and Salminen, S. (2007). Intestinal integrity and Akkermansia muciniphila, a mucin-degrading member of the intestinal microbiota present in infants, adults, and the elderly. Appl. Environ. Microbiol. 73, 7767–7770. doi: 10.1128/AEM.01477-07
Degnan, P. H., Taga, M. E., and Goodman, A. L. (2014). Vitamin B12 as a modulator of gut microbial ecology. Cell Metab. 20, 769–778. doi: 10.1016/j.cmet.2014.10.002
Deng, L., Ou, Z., Huang, D., Li, C., Lu, Z., Liu, W., et al. (2020). Diverse effects of different Akkermansia muciniphila genotypes on brown adipose tissue inflammation and whitening in a high-fat-diet murine model. Microb. Pathog. 147:104353. doi: 10.1016/j.micpath.2020.104353
Deng, Z., Wu, N., Wang, J., Geng, L., Yue, Y., Wang, F., et al. (2021). Low molecular weight fucoidan fraction LF2 improves metabolic syndrome via up-regulating PI3K-AKT-mTOR axis and increasing the abundance of Akkermansia muciniphila in the gut microbiota. Int. J. Biol. Macromol. 193, 789–798. doi: 10.1016/j.ijbiomac.2021.10.188
Depommier, C., Everard, A., Druart, C., Plovier, H., Van Hul, M., Vieira-Silva, S., et al. (2019). Supplementation with Akkermansia muciniphila in overweight and obese human volunteers: a proof-of-concept exploratory study. Nat. Med. 25, 1096–1103. doi: 10.1038/s41591-019-0495-2
Depommier, C., Van Hul, M., Everard, A., Delzenne, N. M., De Vos, W. M., and Cani, P. D. (2020). Pasteurized Akkermansia muciniphila increases whole-body energy expenditure and fecal energy excretion in diet-induced obese mice. Gut Microbes 11, 1231–1245. doi: 10.1080/19490976.2020.1737307
Derrien, M., Collado, M. C., Ben-Amor, K., Salminen, S., and de Vos, W. M. (2008). The mucin degrader Akkermansia muciniphila is an abundant resident of the human intestinal tract. Appl. Environ. Microbiol. 74, 1646–1648. doi: 10.1128/AEM.01226-07
Derrien, M., Vaughan, E. E., Plugge, C. M., and de Vos, W. M. (2004). Akkermansia muciniphila gen. Nov., sp. nov., a human intestinal mucin-degrading bacterium. Int. J. Syst. Evol. Microbiol. 54, 1469–1476. doi: 10.1099/ijs.0.02873-0
Dong, S., Zhu, M., Wang, K., Zhao, X., Hu, L., Jing, W., et al. (2021). Dihydromyricetin improves DSS-induced colitis in mice via modulation of fecal-bacteria-related bile acid metabolism. Pharmacol. Res. 171:105767. doi: 10.1016/j.phrs.2021.105767
Duan, R., Guan, X., Huang, K., Zhang, Y., Li, S., Xia, J., et al. (2021). Flavonoids from whole-grain oat alleviated high-fat diet-induced hyperlipidemia via regulating bile acid metabolism and gut microbiota in mice. J. Agric. Food Chem. 69, 7629–7640. doi: 10.1021/acs.jafc.1c01813
Efsa Panel on Nutrition, Novel Foods and Food Allergens (NDA)Turck, D., Bohn, T., Castenmiller, J., De Henauw, S., Hirsch-Ernst, K. I., et al. (2021). Safety of pasteurised Akkermansia muciniphila as a novel food pursuant to regulation (EU) 2015/2283. EFSA J. 19:e06780. doi: 10.2903/j.efsa.2021.6780
Everard, A., Belzer, C., Geurts, L., Ouwerkerk, J. P., Druart, C., Bindels, L. B., et al. (2013). Cross-talk between Akkermansia muciniphila and intestinal epithelium controls diet-induced obesity. Proc. Natl. Acad. Sci. U. S. A. 110, 9066–9071. doi: 10.1073/pnas.1219451110
Everard, A., Plovier, H., Rastelli, M., Van Hul, M., de Wouters d'Oplinter, A., Geurts, L., et al. (2019). Intestinal epithelial N-acylphosphatidylethanolamine phospholipase D links dietary fat to metabolic adaptations in obesity and steatosis. Nat. Commun. 10:457. doi: 10.1038/s41467-018-08051-7
Ganesh, B. P., Engevik, M. A., and Versalovic, J. (2016). Su1896 Akkermansia muciniphila secretory product actively increases mucus secretion and sialylation in human goblet-like cells. Gastroenterology 150:S582. doi: 10.1016/s0016-5085(16)31995-3
Ganesh, B. P., Klopfleisch, R., Loh, G., and Blaut, M. (2013). Commensal Akkermansia muciniphila exacerbates gut inflammation in Salmonella Typhimurium-infected gnotobiotic mice. PLoS One 8:e74963. doi: 10.1371/journal.pone.0074963
Gao, J., Mao, K., Wang, X., Mi, S., Fu, M., Li, X., et al. (2021). Tibet kefir Milk regulated metabolic changes induced by high-fat diet via amino acids, bile acids, and Equol metabolism in human-microbiota-associated rats. J. Agric. Food Chem. 69, 6720–6732. doi: 10.1021/acs.jafc.1c02430
Geva-Zatorsky, N., Sefik, E., Kua, L., Pasman, L., Tan, T. G., Ortiz-Lopez, A., et al. (2017). Mining the human gut microbiota for immunomodulatory organisms. Cells 168, 928–943.e911. doi: 10.1016/j.cell.2017.01.022
Ghosh, S., Whitley, C. S., Haribabu, B., and Jala, V. R. (2021). Regulation of intestinal barrier function by microbial metabolites. Cell. Mol. Gastroenterol. Hepatol. 11, 1463–1482. doi: 10.1016/j.jcmgh.2021.02.007
Gomes, J. M. G., Costa, J. A., and Alfenas, R. C. G. (2017). Metabolic endotoxemia and diabetes mellitus: a systematic review. Metabolism 68, 133–144. doi: 10.1016/j.metabol.2016.12.009
Grajeda-Iglesias, C., Durand, S., Daillère, R., Iribarren, K., Lemaitre, F., Derosa, L., et al. (2021). Oral administration of Akkermansia muciniphila elevates systemic antiaging and anticancer metabolites. Aging (Albany NY) 13, 6375–6405. doi: 10.18632/aging.202739
Grander, C., Adolph, T. E., Wieser, V., Lowe, P., Wrzosek, L., Gyongyosi, B., et al. (2018). Recovery of ethanol-induced Akkermansia muciniphila depletion ameliorates alcoholic liver disease. Gut 67, 891–901. doi: 10.1136/gutjnl-2016-313432
Gregg, E. W., Li, Y., Wang, J., Burrows, N. R., Ali, M. K., Rolka, D., et al. (2014). Changes in diabetes-related complications in the United States, 1990-2010. N. Engl. J. Med. 370, 1514–1523. doi: 10.1056/NEJMoa1310799
Gu, Z., Pei, W., Shen, Y., Wang, L., Zhu, J., Zhang, Y., et al. (2021). Akkermansia muciniphila and its outer protein Amuc_1100 regulates tryptophan metabolism in colitis. Food Funct. 12, 10184–10195. doi: 10.1039/d1fo02172a
Günzel, D., and Yu, A. S. (2013). Claudins and the modulation of tight junction permeability. Physiol. Rev. 93, 525–569. doi: 10.1152/physrev.00019.2012
Guo, X., Li, S., Zhang, J., Wu, F., Li, X., Wu, D., et al. (2017). Genome sequencing of 39 Akkermansia muciniphila isolates reveals its population structure, genomic and functional diverisity, and global distribution in mammalian gut microbiotas. BMC Genomics 18:800. doi: 10.1186/s12864-017-4195-3
Guo, D., Park, C., Li, Y., Li, B., Yang, Q., Deng, Y., et al. (2022). Akkermansia muciniphila ameliorates depressive disorders in a murine alcohol-LPS (mALPS) model. Food Funct. 13, 12766–12776. doi: 10.1039/d2fo01478e
Gurung, M., Li, Z., You, H., Rodrigues, R., Jump, D. B., Morgun, A., et al. (2020). Role of gut microbiota in type 2 diabetes pathophysiology. EBioMedicine 51:102590. doi: 10.1016/j.ebiom.2019.11.051
Han, X., Guo, J., Yin, M., Liu, Y., You, Y., Zhan, J., et al. (2020). Grape Extract Activates Brown Adipose Tissue Through Pathway Involving the Regulation of Gut Microbiota and Bile Acid. Mol. Nutr. Food Res. 64, e2000149. doi: 10.1002/mnfr.202000149
Hanninen, A., Toivonen, R., Poysti, S., Belzer, C., Plovier, H., Ouwerkerk, J. P., et al. (2018). Akkermansia muciniphila induces gut microbiota remodelling and controls islet autoimmunity in NOD mice. Gut 67, 1445–1453. doi: 10.1136/gutjnl-2017-314508
Henning, S. M., Summanen, P. H., Lee, R. P., Yang, J., Finegold, S. M., Heber, D., et al. (2017). Pomegranate ellagitannins stimulate the growth of Akkermansia muciniphila in vivo. Anaerobe 43, 56–60. doi: 10.1016/j.anaerobe.2016.12.003
Higarza, S. G., Arboleya, S., Arias, J. L., Gueimonde, M., and Arias, N. (2021). Akkermansia muciniphila and environmental enrichment reverse cognitive impairment associated with high-fat high-cholesterol consumption in rats. Gut Microbes 13, 1–20. doi: 10.1080/19490976.2021.1880240
Horackova, S., Plockova, M., and Demnerova, K. (2018). Importance of microbial defence systems to bile salts and mechanisms of serum cholesterol reduction. Biotechnol. Adv. 36, 682–690. doi: 10.1016/j.biotechadv.2017.12.005
Hsu, C. N., Chang-Chien, G. P., Lin, S., Hou, C. Y., Lu, P. C., and Tain, Y. L. (2020). Association of Trimethylamine, trimethylamine N-oxide, and dimethylamine with cardiovascular risk in children with chronic kidney disease. J. Clin. Med. 9:336. doi: 10.3390/jcm9020336
Huang, X., Liu, G., Guo, J., and Su, Z. (2018). The PI3K/AKT pathway in obesity and type 2 diabetes. Int. J. Biol. Sci. 14, 1483–1496. doi: 10.7150/ijbs.27173
Iannacone, M. (2016). Platelet-mediated modulation of adaptive immunity. Semin. Immunol. 28, 555–560. doi: 10.1016/j.smim.2016.10.008
Jeong, H. W., Kim, J. K., Kim, A. Y., Cho, D., Lee, J. H., Choi, J. K., et al. (2020). Green tea encourages growth of Akkermansia muciniphila. J. Med. Food 23, 841–851. doi: 10.1089/jmf.2019.4662
Johansson, M. E., Larsson, J. M., and Hansson, G. C. (2011). The two mucus layers of colon are organized by the MUC2 mucin, whereas the outer layer is a legislator of host-microbial interactions. Proc. Natl. Acad. Sci. U. S. A. 108 Suppl 1(Suppl 1), 4659–4665. doi: 10.1073/pnas.1006451107
Juarez-Fernandez, M., Porras, D., Petrov, P., Roman-Saguillo, S., Garcia-Mediavilla, M. V., Soluyanova, P., et al. (2021). The Synbiotic combination of Akkermansia muciniphila and quercetin ameliorates early obesity and NAFLD through gut microbiota reshaping and bile acid metabolism modulation. Antioxidants (Basel) 10:2001. doi: 10.3390/antiox10122001
Karcher, N., Nigro, E., Puncochar, M., Blanco-Miguez, A., Ciciani, M., Manghi, P., et al. (2021). Genomic diversity and ecology of human-associated Akkermansia species in the gut microbiome revealed by extensive metagenomic assembly. Genome Biol. 22:209. doi: 10.1186/s13059-021-02427-7
Katiraei, S., de Vries, M. R., Costain, A. H., Thiem, K., Hoving, L. R., van Diepen, J. A., et al. (2020). Akkermansia muciniphila exerts lipid-lowering and immunomodulatory effects without affecting Neointima formation in Hyperlipidemic APOE*3-Leiden. CETP Mice. Mol Nutr Food Res 64:e1900732. doi: 10.1002/mnfr.201900732
Ke, H., Li, F., Deng, W., Li, Z., Wang, S., Lv, P., et al. (2021). Metformin exerts anti-inflammatory and mucus barrier protective effects by enriching Akkermansia muciniphila in mice with ulcerative colitis. Front. Pharmacol. 12:726707. doi: 10.3389/fphar.2021.726707
Keshavarz Azizi Raftar, S., Abdollahiyan, S., Azimirad, M., Yadegar, A., Vaziri, F., Moshiri, A., et al. (2021). The anti-fibrotic effects of heat-killed Akkermansia muciniphila Muc T on liver fibrosis markers and activation of hepatic stellate cells. Probiotics Antimicrob Proteins 13, 776–787. doi: 10.1007/s12602-020-09733-9
Kim, S., Lee, Y., Kim, Y., Seo, Y., Lee, H., Ha, J., et al. (2020). Akkermansia muciniphila prevents fatty liver disease, decreases serum triglycerides, and maintains gut homeostasis. Appl. Environ. Microbiol. 86:e03004-19. doi: 10.1128/aem.03004-19
Kim, S., Shin, Y. C., Kim, T. Y., Kim, Y., Lee, Y. S., Lee, S. H., et al. (2021). Mucin degrader Akkermansia muciniphila accelerates intestinal stem cell-mediated epithelial development. Gut Microbes 13, 1–20. doi: 10.1080/19490976.2021.1892441
Kirmiz, N., Galindo, K., Cross, K. L., Luna, E., Rhoades, N., Podar, M., et al. (2020). Comparative genomics guides elucidation of vitamin B (12) biosynthesis in novel human-associated Akkermansia strains. Appl. Environ. Microbiol. 86:e02117-19. doi: 10.1128/aem.02117-19
Krajmalnik-Brown, R., Ilhan, Z. E., Kang, D. W., and DiBaise, J. K. (2012). Effects of gut microbes on nutrient absorption and energy regulation. Nutr. Clin. Pract. 27, 201–214. doi: 10.1177/0884533611436116
Li, X., Chu, L., Liu, S., Zhang, W., Lin, L., and Zheng, G. (2022). Smilax china L. flavonoid alleviates HFHS-induced inflammation by regulating the gut-liver axis in mice. Phytomedicine 95:153728. doi: 10.1016/j.phymed.2021.153728
Li, D., Cui, Y., Wang, X., Liu, F., and Li, X. (2021). Apple polyphenol extract improves high-fat diet-induced hepatic steatosis by regulating bile acid synthesis and gut microbiota in C57BL/6 male mice. J. Agric. Food Chem. 69, 6829–6841. doi: 10.1021/acs.jafc.1c02532
Li, J., Lin, S., Vanhoutte, P. M., Woo, C. W., and Xu, A. (2016). Akkermansia Muciniphila protects against atherosclerosis by preventing metabolic Endotoxemia-induced inflammation in Apoe−/− mice. Circulation 133, 2434–2446. doi: 10.1161/CIRCULATIONAHA.115.019645
Li, T., Zhang, Y., Song, J., Chen, L., Du, M., and Mao, X. (2022). Yogurt enriched with inulin ameliorated reproductive functions and regulated gut microbiota in Dehydroepiandrosterone-induced polycystic ovary syndrome mice. Nutrients 14:279. doi: 10.3390/nu14020279
Liu, Q., Lu, W., Tian, F., Zhao, J., Zhang, H., Hong, K., et al. (2021). Akkermansia muciniphila exerts strain-specific effects on DSS-induced ulcerative colitis in mice. Front. Cell. Infect. Microbiol. 11:698914. doi: 10.3389/fcimb.2021.698914
Liu, F., Wang, X., Li, D., Cui, Y., and Li, X. (2021). Apple polyphenols extract alleviated dextran sulfate sodium-induced ulcerative colitis in C57BL/6 male mice by restoring bile acid metabolism disorder and gut microbiota dysbiosis. Phytother. Res. 35, 1468–1485. doi: 10.1002/ptr.6910
Liu, J. H., Yue, T., Luo, Z. W., Cao, J., Yan, Z. Q., Jin, L., et al. (2020). Akkermansia muciniphila promotes type H vessel formation and bone fracture healing by reducing gut permeability and inflammation. Dis. Model. Mech. 13:dmm043620. doi: 10.1242/dmm.043620
Liu, X., Zhao, K., Jing, N., Zhao, Y., and Yang, X. (2020). EGCG regulates fatty acid metabolism of high-fat diet-fed mice in association with enrichment of gut Akkermansia muciniphila. J. Funct. Foods 75:104261. doi: 10.1016/j.jff.2020.104261
Liu, X., Zhao, F., Liu, H., Xie, Y., Zhao, D., and Li, C. (2021). Transcriptomics and metabolomics reveal the adaption of Akkermansia muciniphila to high mucin by regulating energy homeostasis. Sci. Rep. 11:9073. doi: 10.1038/s41598-021-88397-z
Lu, F., Li, Y., Wang, X., Hu, X., Liao, X., and Zhang, Y. (2021). Early-life polyphenol intake promotes Akkermansia growth and increase of host goblet cells in association with the potential synergistic effect of Lactobacillus. Food Res. Int. 149:110648. doi: 10.1016/j.foodres.2021.110648
Lukovac, S., Belzer, C., Pellis, L., Keijser, B. J., de Vos, W. M., Montijn, R. C., et al. (2014). Differential modulation by Akkermansia muciniphila and Faecalibacterium prausnitzii of host peripheral lipid metabolism and histone acetylation in mouse gut organoids. MBio 5:e01438-14. doi: 10.1128/mBio.01438-14
Luna, E., Parkar, S. G., Kirmiz, N., Hartel, S., Hearn, E., Hossine, M., et al. (2022). Utilization efficiency of human Milk oligosaccharides by human-associated Akkermansia is strain dependent. Appl. Environ. Microbiol. 88:e0148721. doi: 10.1128/AEM.01487-21
Lv, Q. B., Li, S., Zhang, Y., Guo, R., Wang, Y. C., Peng, Y., et al. (2022). A thousand metagenome-assembled genomes of Akkermansia reveal phylogroups and geographical and functional variations in the human gut. Front. Cell. Infect. Microbiol. 12:957439. doi: 10.3389/fcimb.2022.957439
Ma, Y., Hu, C., Yan, W., Jiang, H., and Liu, G. (2020). Lactobacillus pentosus increases the abundance of Akkermansia and affects the serum metabolome to alleviate DSS-induced colitis in a murine model. Front. Cell Dev. Biol. 8:591408. doi: 10.3389/fcell.2020.591408
Machado, D., Almeida, D., Seabra, C. L., Andrade, J. C., Gomes, A. M., and Freitas, A. C. (2020). Uncovering Akkermansia muciniphila resilience or susceptibility to different temperatures, atmospheres and gastrointestinal conditions. Anaerobe 61:102135. doi: 10.1016/j.anaerobe.2019.102135
Magne, F., Gotteland, M., Gauthier, L., Zazueta, A., Pesoa, S., Navarrete, P., et al. (2020). The Firmicutes/Bacteroidetes ratio: a relevant marker of gut Dysbiosis in obese patients? Nutrients 12:1474. doi: 10.3390/nu12051474
Makishima, M., Okamoto, A. Y., Repa, J. J., Tu, H., Learned, R. M., Luk, A., et al. (1999). Identification of a nuclear receptor for bile acids. Science 284, 1362–1365. doi: 10.1126/science.284.5418.1362
Marcial-Coba, M. S., Cieplak, T., Cahu, T. B., Blennow, A., Knochel, S., and Nielsen, D. S. (2018). Viability of microencapsulated Akkermansia muciniphila and Lactobacillus plantarum during freeze-drying, storage and in vitro simulated upper gastrointestinal tract passage. Food Funct. 9, 5868–5879. doi: 10.1039/c8fo01331d
Martin-Gallausiaux, C., Garcia-Weber, D., Lashermes, A., Larraufie, P., Marinelli, L., Teixeira, V., et al. (2022). Akkermansia muciniphila upregulates genes involved in maintaining the intestinal barrier function via ADP-heptose-dependent activation of the ALPK1/TIFA pathway. Gut Microbes 14:2110639. doi: 10.1080/19490976.2022.2110639
Medina-Vera, I., Sanchez-Tapia, M., Noriega-López, L., Granados-Portillo, O., Guevara-Cruz, M., Flores-López, A., et al. (2019). A dietary intervention with functional foods reduces metabolic endotoxaemia and attenuates biochemical abnormalities by modifying faecal microbiota in people with type 2 diabetes. Diabetes Metab. 45, 122–131. doi: 10.1016/j.diabet.2018.09.004
Muller, T. D., Finan, B., Bloom, S. R., D'Alessio, D., Drucker, D. J., Flatt, P. R., et al. (2019). Glucagon-like peptide 1 (GLP-1). Mol. Metab. 30, 72–130. doi: 10.1016/j.molmet.2019.09.010
Natividad, J. M., Agus, A., Planchais, J., Lamas, B., Jarry, A. C., Martin, R., et al. (2018). Impaired aryl hydrocarbon receptor ligand production by the gut microbiota is a key factor in metabolic syndrome. Cell Metab. 28, 737–749 e734. doi: 10.1016/j.cmet.2018.07.001
Ondee, T., Pongpirul, K., Visitchanakun, P., Saisorn, W., Kanacharoen, S., Wongsaroj, L., et al. (2021). Lactobacillus acidophilus LA5 improves saturated fat-induced obesity mouse model through the enhanced intestinal Akkermansia muciniphila. Sci. Rep. 11:6367. doi: 10.1038/s41598-021-85449-2
Org, E., Parks, B. W., Joo, J. W., Emert, B., Schwartzman, W., Kang, E. Y., et al. (2015). Genetic and environmental control of host-gut microbiota interactions. Genome Res. 25, 1558–1569. doi: 10.1101/gr.194118.115
Ottman, N., Davids, M., Suarez-Diez, M., Boeren, S., Schaap, P. J., Martins Dos Santos, V. A. P., et al. (2017a). Genome-scale model and omics analysis of metabolic capacities of Akkermansia muciniphila reveal a preferential mucin-degrading lifestyle. Appl. Environ. Microbiol. 83:e01014-17. doi: 10.1128/AEM.01014-17
Ottman, N., Huuskonen, L., Reunanen, J., Boeren, S., Klievink, J., Smidt, H., et al. (2016). Characterization of outer membrane proteome of Akkermansia muciniphila reveals sets of novel proteins exposed to the human intestine. Front. Microbiol. 7:1157. doi: 10.3389/fmicb.2016.01157
Ottman, N., Reunanen, J., Meijerink, M., Pietila, T. E., Kainulainen, V., Klievink, J., et al. (2017b). Pili-like proteins of Akkermansia muciniphila modulate host immune responses and gut barrier function. PLoS One 12:e0173004. doi: 10.1371/journal.pone.0173004
Ouwerkerk, J. P., van der Ark, K. C. H., Davids, M., Claassens, N. J., Finestra, T. R., de Vos, W. M., et al. (2016). Adaptation of Akkermansia muciniphila to the Oxic-anoxic Interface of the mucus layer. Appl. Environ. Microbiol. 82, 6983–6993. doi: 10.1128/AEM.01641-16
Palomba, A., Tanca, A., Abbondio, M., Sau, R., Serra, M., Marongiu, F., et al. (2021). Time-restricted feeding induces Lactobacillus-and Akkermansia-specific functional changes in the rat fecal microbiota. NPJ Biofilms Microbiomes 7:85. doi: 10.1038/s41522-021-00256-x
Perez-Monter, C., Alvarez-Arce, A., Nuno-Lambarri, N., Escalona-Nandez, I., Juarez-Hernandez, E., Chavez-Tapia, N. C., et al. (2022). Inulin improves diet-induced hepatic steatosis and increases intestinal Akkermansia genus level. Int. J. Mol. Sci. 23:991. doi: 10.3390/ijms23020991
Perraudeau, F., McMurdie, P., Bullard, J., Cheng, A., Cutcliffe, C., Deo, A., et al. (2020). Improvements to postprandial glucose control in subjects with type 2 diabetes: a multicenter, double blind, randomized placebo-controlled trial of a novel probiotic formulation. BMJ Open Diabetes Res. Care 8:e001319. doi: 10.1136/bmjdrc-2020-001319
Plovier, H., Everard, A., Druart, C., Depommier, C., Van Hul, M., Geurts, L., et al. (2017). A purified membrane protein from Akkermansia muciniphila or the pasteurized bacterium improves metabolism in obese and diabetic mice. Nat. Med. 23, 107–113. doi: 10.1038/nm.4236
Psichas, A., Sleeth, M. L., Murphy, K. G., Brooks, L., Bewick, G. A., Hanyaloglu, A. C., et al. (2015). The short chain fatty acid propionate stimulates GLP-1 and PYY secretion via free fatty acid receptor 2 in rodents. Int. J. Obes. 39, 424–429. doi: 10.1038/ijo.2014.153
Puri, P., Daita, K., Joyce, A., Mirshahi, F., Santhekadur, P. K., Cazanave, S., et al. (2018). The presence and severity of nonalcoholic steatohepatitis is associated with specific changes in circulating bile acids. Hepatology 67, 534–548. doi: 10.1002/hep.29359
Qin, J., Li, R., Raes, J., Arumugam, M., Burgdorf, K. S., Manichanh, C., et al. (2010). A human gut microbial gene catalogue established by metagenomic sequencing. Nature 464, 59–65. doi: 10.1038/nature08821
Rao, Y., Kuang, Z., Li, C., Guo, S., Xu, Y., Zhao, D., et al. (2021). Gut Akkermansia muciniphila ameliorates metabolic dysfunction-associated fatty liver disease by regulating the metabolism of L-aspartate via gut-liver axis. Gut Microbes 13, 1–19. doi: 10.1080/19490976.2021.1927633
Regnier, M., Rastelli, M., Morissette, A., Suriano, F., Le Roy, T., Pilon, G., et al. (2020). Rhubarb supplementation prevents diet-induced obesity and diabetes in association with increased Akkermansia muciniphila in mice. Nutrients 12:2932. doi: 10.3390/nu12102932
Ribo, S., Sanchez-Infantes, D., Martinez-Guino, L., Garcia-Mantrana, I., Ramon-Krauel, M., Tondo, M., et al. (2021). Increasing breast milk betaine modulates Akkermansia abundance in mammalian neonates and improves long-term metabolic health. Sci. Transl. Med. 13:eabb0322. doi: 10.1126/scitranslmed.abb0322
Ring, C., Klopfleisch, R., Dahlke, K., Basic, M., Bleich, A., and Blaut, M. (2019). Akkermansia muciniphila strain ATCC BAA-835 does not promote short-term intestinal inflammation in gnotobiotic interleukin-10-deficient mice. Gut Microbes 10, 188–203. doi: 10.1080/19490976.2018.1511663
Roopchand, D. E., Carmody, R. N., Kuhn, P., Moskal, K., Rojas-Silva, P., Turnbaugh, P. J., et al. (2015). Dietary polyphenols promote growth of the gut bacterium Akkermansia muciniphila and attenuate high-fat diet-induced metabolic syndrome. Diabetes 64, 2847–2858. doi: 10.2337/db14-1916
Roshanravan, N., Mahdavi, R., Alizadeh, E., Ghavami, A., Rahbar Saadat, Y., Mesri Alamdari, N., et al. (2017). The effects of sodium butyrate and inulin supplementation on angiotensin signaling pathway via promotion of Akkermansia muciniphila abundance in type 2 diabetes; a randomized, double-blind, placebo-controlled trial. J. Cardiovasc. Thorac. Res. 9, 183–190. doi: 10.15171/jcvtr.2017.32
Shang, Q., Song, G., Zhang, M., Shi, J., Xu, C., Hao, J., et al. (2017). Dietary fucoidan improves metabolic syndrome in association with increased Akkermansia population in the gut microbiota of high-fat diet-fed mice. J. Funct. Foods 28, 138–146. doi: 10.1016/j.jff.2016.11.002
Shang, Q., Wang, Y., Pan, L., Niu, Q., Li, C., Jiang, H., et al. (2018). Dietary polysaccharide from Enteromorpha Clathrata modulates gut microbiota and promotes the growth of Akkermansia muciniphila, Bifidobacterium spp. and Lactobacillus spp. Mar. Drugs 16:167. doi: 10.3390/md16050167
Shen, W., Shen, M., Zhao, X., Zhu, H., Yang, Y., Lu, S., et al. (2017). Anti-obesity effect of capsaicin in mice fed with high-fat diet is associated with an increase in population of the gut bacterium Akkermansia muciniphila. Front. Microbiol. 8:272. doi: 10.3389/fmicb.2017.00272
Shen, J., Tong, X., Sud, N., Khound, R., Song, Y., Maldonado-Gomez, M. X., et al. (2016). Low-density lipoprotein receptor signaling mediates the triglyceride-lowering action of Akkermansia muciniphila in genetic-induced hyperlipidemia. Arterioscler. Thromb. Vasc. Biol. 36, 1448–1456. doi: 10.1161/ATVBAHA.116.307597
Sheng, L., Jena, P. K., Liu, H. X., Hu, Y., Nagar, N., Bronner, D. N., et al. (2018). Obesity treatment by epigallocatechin-3-gallate-regulated bile acid signaling and its enriched Akkermansia muciniphila. FASEB J. 32:fj201800370R. doi: 10.1096/fj.201800370R
Shi, Z., Lei, H., Chen, G., Yuan, P., Cao, Z., Ser, H. L., et al. (2021). Impaired intestinal Akkermansia muciniphila and aryl hydrocarbon receptor ligands contribute to nonalcoholic fatty liver disease in mice. mSystems 6:e00985-20. doi: 10.1128/mSystems.00985-20
Shi, M., Yue, Y., Ma, C., Dong, L., and Chen, F. (2022). Pasteurized Akkermansia muciniphila ameliorate the LPS-induced intestinal barrier dysfunction via modulating AMPK and NF-κB through TLR2 in Caco-2 cells. Nutrients 14:764. doi: 10.3390/nu14040764
Slifer, Z. M., and Blikslager, A. T. (2020). The integral role of tight junction proteins in the repair of injured intestinal epithelium. Int. J. Mol. Sci. 21:972. doi: 10.3390/ijms21030972
Sonne, D. P. (2021). MECHANISMS IN ENDOCRINOLOGY: FXR signalling: a novel target in metabolic diseases. Eur. J. Endocrinol. 184, R193–R205. doi: 10.1530/EJE-20-1410
Su, Q. (2020). Phytochemicals in fenugreek seed prevent high fat diet induced metabolic inflammation and NAFLD via the mediation of Akkermansia muciniphila. Proc. Nutr. Soc. 79:E485. doi: 10.1017/s0029665120004334
Tian, M., Li, D., Ma, C., Feng, Y., Hu, X., and Chen, F. (2021). Barley leaf insoluble dietary Fiber alleviated dextran sulfate sodium-induced mice colitis by modulating gut microbiota. Nutrients 13:846. doi: 10.3390/nu13030846
van der Ark, K. C. H., Nugroho, A. D. W., Berton-Carabin, C., Wang, C., Belzer, C., de Vos, W. M., et al. (2017). Encapsulation of the therapeutic microbe Akkermansia muciniphila in a double emulsion enhances survival in simulated gastric conditions. Food Res. Int. 102, 372–379. doi: 10.1016/j.foodres.2017.09.004
van der Lugt, B., van Beek, A. A., Aalvink, S., Meijer, B., Sovran, B., Vermeij, W. P., et al. (2019). Akkermansia muciniphila ameliorates the age-related decline in colonic mucus thickness and attenuates immune activation in accelerated aging Ercc 1 (−/Delta7) mice. Immun. Ageing 16:6. doi: 10.1186/s12979-019-0145-z
van Passel, M. W., Kant, R., Zoetendal, E. G., Plugge, C. M., Derrien, M., Malfatti, S. A., et al. (2011). The genome of Akkermansia muciniphila, a dedicated intestinal mucin degrader, and its use in exploring intestinal metagenomes. PLoS One 6:e16876. doi: 10.1371/journal.pone.0016876
Villard, A., Boursier, J., and Andriantsitohaina, R. (2021). Microbiota-derived extracellular vesicles and metabolic syndrome. Acta Physiol (Oxf.) 231:e13600. doi: 10.1111/apha.13600
Wade, H., and Su, Q. (2021). Akkermansia muciniphila ameliorates inflammatory bowel disease by modulating gut tight junctions in mice. Proc. Nutr. Soc. 80:E144. doi: 10.1017/s0029665121002676
Wang, J. H., Bose, S., Kim, H. G., Han, K. S., and Kim, H. (2015). Fermented Rhizoma Atractylodis Macrocephalae alleviates high fat diet-induced obesity in association with regulation of intestinal permeability and microbiota in rats. Sci. Rep. 5:8391. doi: 10.1038/srep08391
Wang, L., Tang, L., Feng, Y., Zhao, S., Han, M., Zhang, C., et al. (2020). A purified membrane protein from Akkermansia muciniphila or the pasteurised bacterium blunts colitis associated tumourigenesis by modulation of CD8(+) T cells in mice. Gut 69, 1988–1997. doi: 10.1136/gutjnl-2019-320105
Wang, L., Wu, Y., Zhuang, L., Chen, X., Min, H., Song, S., et al. (2019). Puerarin prevents high-fat diet-induced obesity by enriching Akkermansia muciniphila in the gut microbiota of mice. PLoS One 14:e0218490. doi: 10.1371/journal.pone.0218490
Wang, J., Xu, W., Wang, R., Cheng, R., Tang, Z., and Zhang, M. (2021). The outer membrane protein Amuc_1100 of Akkermansia muciniphila promotes intestinal 5-HT biosynthesis and extracellular availability through TLR2 signalling. Food Funct. 12, 3597–3610. doi: 10.1039/d1fo00115a
Weir, G. C., and Bonner-Weir, S. (2004). Five stages of evolving beta-cell dysfunction during progression to diabetes. Diabetes 53, S16–S21. doi: 10.2337/diabetes.53.suppl_3.s16
Wu, F., Guo, X., Zhang, M., Ou, Z., Wu, D., Deng, L., et al. (2020). An Akkermansia muciniphila subtype alleviates high-fat diet-induced metabolic disorders and inhibits the neurodegenerative process in mice. Anaerobe 61:102138. doi: 10.1016/j.anaerobe.2019.102138
Wu, W., Lv, L., Shi, D., Ye, J., Fang, D., Guo, F., et al. (2017). Protective effect of Akkermansia muciniphila against immune-mediated liver injury in a mouse model. Front. Microbiol. 8:1804. doi: 10.3389/fmicb.2017.01804
Wu, H., Rebello, O., Crost, E. H., Owen, C. D., Walpole, S., Bennati-Granier, C., et al. (2021). Fucosidases from the human gut symbiont Ruminococcus gnavus. Cell. Mol. Life Sci. 78, 675–693. doi: 10.1007/s00018-020-03514-x
Xi, M., Li, J., Hao, G., An, X., Song, Y., Wei, H., et al. (2020). Stachyose increases intestinal barrier through Akkermansia muciniphila and reduces gut inflammation in germ-free mice after human fecal transplantation. Food Res. Int. 137:109288. doi: 10.1016/j.foodres.2020.109288
Xia, J., Lv, L., Liu, B., Wang, S., Zhang, S., Wu, Z., et al. (2022). Akkermansia muciniphila ameliorates acetaminophen-induced liver injury by regulating gut microbial composition and metabolism. Microbiol. Spectr. 10:e0159621. doi: 10.1128/spectrum.01596-21
Yaghoubfar, R., Behrouzi, A., Ashrafian, F., Shahryari, A., Moradi, H. R., Choopani, S., et al. (2020). Modulation of serotonin signaling/metabolism by Akkermansia muciniphila and its extracellular vesicles through the gut-brain axis in mice. Sci. Rep. 10:22119. doi: 10.1038/s41598-020-79171-8
Yang, M., Bose, S., Lim, S., Seo, J., Shin, J., Lee, D., et al. (2020). Beneficial effects of newly isolated Akkermansia muciniphila strains from the human gut on obesity and metabolic dysregulation. Microorganisms 8:1413. doi: 10.3390/microorganisms8091413
Yang, J., Kurnia, P., Henning, S. M., Lee, R., Huang, J., Garcia, M. C., et al. (2021). Effect of standardized grape powder consumption on the gut microbiome of healthy subjects: a pilot study. Nutrients 13:3965. doi: 10.3390/nu13113965
Yang, Y., Zhong, Z., Wang, B., Xia, X., Yao, W., Huang, L., et al. (2019). Early-life high-fat diet-induced obesity programs hippocampal development and cognitive functions via regulation of gut commensal Akkermansia muciniphila. Neuropsychopharmacology 44, 2054–2064. doi: 10.1038/s41386-019-0437-1
Yassour, M., Lim, M. Y., Yun, H. S., Tickle, T. L., Sung, J., Song, Y. M., et al. (2016). Sub-clinical detection of gut microbial biomarkers of obesity and type 2 diabetes. Genome Med. 8:17. doi: 10.1186/s13073-016-0271-6
Yde, C. C., Jensen, H. M., Christensen, N., Servant, F., Lelouvier, B., Lahtinen, S., et al. (2021). Polydextrose with and without Bifidobacterium animalis ssp. lactis 420 drives the prevalence of Akkermansia and improves liver health in a multi-compartmental obesogenic mice study. PLoS One 16:e0260765. doi: 10.1371/journal.pone.0260765
Yoon, H. S., Cho, C. H., Yun, M. S., Jang, S. J., You, H. J., Kim, J. H., et al. (2021). Akkermansia muciniphila secretes a glucagon-like peptide-1-inducing protein that improves glucose homeostasis and ameliorates metabolic disease in mice. Nat. Microbiol. 6, 563–573. doi: 10.1038/s41564-021-00880-5
Yu, Y., Lu, J., Sun, L., Lyu, X., Chang, X. Y., Mi, X., et al. (2021). Akkermansia muciniphila: a potential novel mechanism of nuciferine to improve hyperlipidemia. Biomed. Pharmacother. 133:111014. doi: 10.1016/j.biopha.2020.111014
Zhai, Z., Niu, K. M., Liu, Y., Lin, C., and Wu, X. (2021). The gut microbiota-bile acids-TGR5 Axis mediates Eucommia ulmoides leaf extract alleviation of injury to colonic epithelium integrity. Front. Microbiol. 12:727681. doi: 10.3389/fmicb.2021.727681
Zhang, J., Ni, Y., Qian, L., Fang, Q., Zheng, T., Zhang, M., et al. (2021). Decreased abundance of Akkermansia muciniphila leads to the impairment of insulin secretion and glucose homeostasis in lean type 2 diabetes. Adv. Sci. (Weinh) 8:e2100536. doi: 10.1002/advs.202100536
Zhang, L., Ouyang, Y., Li, H., Shen, L., Ni, Y., Fang, Q., et al. (2019). Metabolic phenotypes and the gut microbiota in response to dietary resistant starch type 2 in normal-weight subjects: a randomized crossover trial. Sci. Rep. 9:4736. doi: 10.1038/s41598-018-38216-9
Zhang, X., Shen, D., Fang, Z., Jie, Z., Qiu, X., Zhang, C., et al. (2013). Human gut microbiota changes reveal the progression of glucose intolerance. PLoS One 8:e71108. doi: 10.1371/journal.pone.0071108
Zhang, L., Wang, Y., Wu, F., Wang, X., Feng, Y., and Wang, Y. (2022). MDG, an Ophiopogon japonicus polysaccharide, inhibits non-alcoholic fatty liver disease by regulating the abundance of Akkermansia muciniphila. Int. J. Biol. Macromol. 196, 23–34. doi: 10.1016/j.ijbiomac.2021.12.036
Zhang, F. L., Yang, Y. L., Zhang, Z., Yao, Y. Y., Xia, R., Gao, C. C., et al. (2021). Surface-displayed Amuc_1100 from Akkermansia muciniphila on Lactococcus lactis ZHY1 improves hepatic steatosis and intestinal health in high-fat-fed zebrafish. Front. Nutr. 8:726108. doi: 10.3389/fnut.2021.726108
Zhao, S., Liu, W., Wang, J., Shi, J., Sun, Y., Wang, W., et al. (2017). Akkermansia muciniphila improves metabolic profiles by reducing inflammation in chow diet-fed mice. J. Mol. Endocrinol. 58, 1–14. doi: 10.1530/JME-16-0054
Zhu, L., Lu, X., Liu, L., Voglmeir, J., Zhong, X., and Yu, Q. (2020). Akkermansia muciniphila protects intestinal mucosa from damage caused by S. pullorum by initiating proliferation of intestinal epithelium. Vet. Res. 51:34. doi: 10.1186/s13567-020-00755-3
Zhu, L., Zhang, D., Zhu, H., Zhu, J., Weng, S., Dong, L., et al. (2018). Berberine treatment increases Akkermansia in the gut and improves high-fat diet-induced atherosclerosis in Apoe(−/−) mice. Atherosclerosis 268, 117–126. doi: 10.1016/j.atherosclerosis.2017.11.023
Keywords: Akkermansia muciniphila, type 2 diabetes mellitus, gut microbiota, metabolic diseases, endotoxemia, prebiotics
Citation: Li J, Yang G, Zhang Q, Liu Z, Jiang X and Xin Y (2023) Function of Akkermansia muciniphila in type 2 diabetes and related diseases. Front. Microbiol. 14:1172400. doi: 10.3389/fmicb.2023.1172400
Edited by:
Hao Zhong, Zhejiang University of Technology, ChinaReviewed by:
Munhyung Bae, Gachon University, Republic of KoreaXiaoshuang Dai, Beijing Genomics Institute (BGI), China
Copyright © 2023 Li, Yang, Zhang, Liu, Jiang and Xin. This is an open-access article distributed under the terms of the Creative Commons Attribution License (CC BY). The use, distribution or reproduction in other forums is permitted, provided the original author(s) and the copyright owner(s) are credited and that the original publication in this journal is cited, in accordance with accepted academic practice. No use, distribution or reproduction is permitted which does not comply with these terms.
*Correspondence: Xin Jiang, amlhbmd4QGpsdS5lZHUuY24=; Ying Xin, eGlueUBqbHUuZWR1LmNu