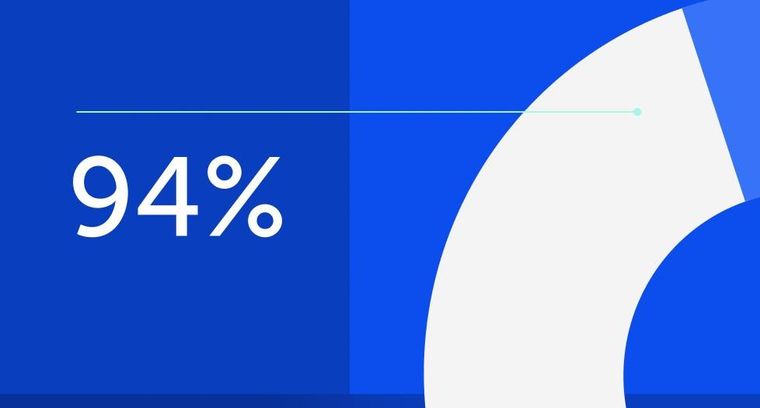
94% of researchers rate our articles as excellent or good
Learn more about the work of our research integrity team to safeguard the quality of each article we publish.
Find out more
REVIEW article
Front. Microbiol., 06 April 2023
Sec. Microorganisms in Vertebrate Digestive Systems
Volume 14 - 2023 | https://doi.org/10.3389/fmicb.2023.1124945
This article is part of the Research TopicGut Microbiota and Gut-Associated Metabolites (GAMs) in Bone HealthView all 5 articles
Gut microbiota is of great importance in human health, and its roles in the maintenance of skeletal homeostasis have long been recognized as the “gut-bone axis.” Recent evidence has indicated intercorrelations between gut microbiota, endocrine system and bone metabolism. This review article discussed the complex interactions between gut microbiota and bone metabolism-related hormones, including sex steroids, insulin-like growth factors, 5-hydroxytryptamine, parathyroid hormone, glucagon-like peptides, peptide YY, etc. Although the underlying mechanisms still need further investigation, the regulatory effect of gut microbiota on bone health via interplaying with endocrine system may provide a new paradigm for the better management of musculoskeletal disorders.
Known as the “second gene pool” of the human body, gut microbiota plays important roles in human health, and the dysbiosis of gut microbiota is closely involved in a variety of diseases, including gastrointestinal diseases (Hold, 2016; Ni et al., 2017), cardiovascular disease (Tang et al., 2017; Wang and Zhao, 2018), metabolic disorder (Maruvada et al., 2017; Makki et al., 2018), and psychiatric disorder (Mangiola et al., 2016; Fattorusso et al., 2019).
Numerous studies have demonstrated the critical roles of gut microbiota in regulating bone homeostasis. By using high-throughput sequencing, the alteration of gut microbiota has been detected in individuals with osteopenia or osteoporosis, characterized with an increased abundance of lipopolysaccharides (LPS)-producing genera (Das et al., 2019; Li et al., 2019; Wei et al., 2021). Germ-free (GF) animals or animals treated with antibiotics have been widely used to investigate the potential roles of microbiota in bone metabolism, revealing the regulatory effect of commensal microbiota on bone mineral density (BMD) and bone property (Sjögren et al., 2012; Guss et al., 2017; Castaneda et al., 2020). In addition, gut microbiota can regulate skeletal system by affecting nutrition absorption, gut barrier functionality and immune response (Lafage Proust, 2017; Li et al., 2021; Tu et al., 2021). Recently, although direct causal effect has not been demonstrated, the regulatory effect of gut microbiota on skeletal homeostasis dependent on the endocrine system, known as “enteroendocrine-osseous axis,” has drawn increasing attentions. In this review, we discussed the evidence of hormone-bone interactions and potential mechanisms of how gut microbiota regulates the enteroendocrine-osseous axis, based on current evidence in this field.
Since a large part of patients with endocrine diseases are accompanied by skeletal disorders, the interaction between endocrine activity and bone metabolism has come into notice. Hepatic osteodystrophy refers to bone diseases in patients with chronic liver disease, and approximately 30% of patients with chronic liver disease suffer from osteoporosis (Lehto-Axtelius et al., 2002; Collier, 2007). Patients who received liver transplants may suffer from accelerated bone loss and elevated fracture rate in the short term after the surgery (European Association for the Study of the Liver, 2016; Kovvuru et al., 2020). Hepatocellular dysfunction leads to the synthesis defect of growth hormone (GH) and insulin-like growth factor 1 (IGF-1), and the endocrine abnormality is perceived as the causation of bone metabolic complications (Baruch et al., 1998; Held et al., 2005; Assy et al., 2008). Chronic kidney disease (CKD) is associated with the development of the mineral and bone disorder (MBD), osteoporosis, and fragility fracture, characterized with abnormal serum levels of calcium, phosphorus, parathyroid hormone (PTH), and vitamin D (Hsu et al., 2020; Pazianas and Miller, 2021). As for primary hyperparathyroidism (PHPT), excessive endogenous PTH promotes bone turnover and results in cortical and trabecular bone loss (Mosekilde, 2008; Silva et al., 2011). Interestingly, being the complication of PHPT and CKD, the aggravated bone turnover generally caused by enhanced PTH level does not always occur as the disease advances (Pierreux and Bravenboer, 2018; Palermo et al., 2020). Additionally, the absence of bone destruction after continuous PTH (cPTH) administration was observed in GF mice (Yu et al., 2020). These findings indicate that gut microbiota might serve as a potential mediator in the enteroendocrine-osseous axis.
Osteoporosis (OP) is the most prevalent skeletal disorder characterized by decreased BMD and bone microarchitecture destruction, which mainly occurs in elderly people, typically postmenopausal women (Lane et al., 2000; Johnston and Dagar, 2020). Strong benefits of sex steroids in bone metabolism have long been recognized. Estrogen is of great importance in bone homeostasis. In addition to directly regulating the development of osteoblasts and osteoclasts via Fas/Fas ligand pathway (Nakamura et al., 2007; Krum et al., 2008), estrogen prevents bone resorption by maintaining systemic and bone marrow T cells homeostasis (Cenci et al., 2003; Jia et al., 2021). Recent studies have revealed the correlations between gut microbiota and postmenopausal osteoporosis (PMO), as gut microbiota dysbiosis was observed under the condition of sex steroid deficiency, whereas the estrogen deficiency-induced bone destruction was not observed in GF mice (Li et al., 2016; He et al., 2020; Wang et al., 2021; Yang et al., 2022). Intricate regulatory effects of gut microbiota in PMO have been demonstrated. On one hand, elevated LPS level caused by gut microbial dysbiosis can stimulate the secretion of inflammatory cytokines, including IL-1β, IL-6, TNF-α, thus promoting bone resorption (Islam et al., 2007; Bandow et al., 2010; Xie et al., 2014; Hirano et al., 2017). On the other hand, short chain fatty acids (SCFAs) derived from gut microbiota exert bone-protective effects by inducing the generation of regulatory T cells (Tregs) (Furusawa et al., 2013; Dalile et al., 2019) and suppressing Th17 cells (Asarat et al., 2016; Luu et al., 2019). Being the most common modulator of gut microbiota, probiotics have been demonstrated to ameliorate estrogen deficiency-induced bone loss in rodents via promoting SCFAs production, restoring the gut permeability, and reducing inflammatory response (Li et al., 2016; Jia et al., 2021). The favorable effects of probiotics on bone health have also demonstrated in clinical trials (Narva et al., 2004; Jafarnejad et al., 2017; Lambert et al., 2017). In addition, it has been shown that administration of brain progesterone can effectively relive the bone loss while maintaining the diversity of the gut microbiomes in estrogen-deficient rats (Park et al., 2018).
Low testosterone level in hypogonadal men is also associated with decreased BMD, and testosterone treatment can effectively improve the bone structure (Kenny et al., 2001; Colleluori et al., 2021). It has been demonstrated that testosterone can stimulate the proliferation of osteoblast precursors, and as well suppress the osteoblasts apoptosis (Damien et al., 2000; Wiren et al., 2006). Approaches that influence gut environment, including probiotics administration and fecal microbiota transplanting, can regulate testosterone level (Flores et al., 2012; Markle et al., 2013), and concurrently alter bone structure (Ai et al., 2021; Han et al., 2022). However, further investigations are still needed to reveal the underlying mechanisms.
The longitudinal bone growth resulting from endochondral ossification is regulated by the GH/insulin-like growth factor (IGF) axis (Wong et al., 2016). IGFs are required for cell survival, proliferation and differentiation, and IGF-1 has long been recognized as a pivotal candidate in osteoblastogenesis (Stewart and Rotwein, 1996; Van Wyk and Smith, 1999). The bone quality is strongly linked to the serum IGF-1 level (van Coeverden et al., 2002; Rosen et al., 2004; Fazeli et al., 2020; Delagrange et al., 2021), and the circulating IGF-1 is mainly synthesized by liver under the regulation of GH (Stewart and Rotwein, 1996; Van Wyk and Smith, 1999). Besides, local production of IGF-1 from osteoblasts, osteoclasts and osteocytes are capable of promoting osteoblastic activity and bone formation, demonstrating the great importance of paracrine/autocrine functions of IGF-1 in bone metabolism (Zhao et al., 2000; Hamrick, 2012; Wang et al., 2013; Kirk et al., 2020). IGF-1 is also required for osteoclastogenesis. Mature osteoclasts express IGF-1 receptor, and IGF-1 deprivation leads to reduction in osteoclast number, size and function, as well as decreased expression of RANKL and RANK (Hou et al., 1997; Wang et al., 2006).
The roles of microbiota in anabolic effects of IGF-1 have been observed in a wide variety of species. In Drosophila, commensal bacteria are responsible for the production of Drosophila insulin-like peptides (dILPs), analogs of mammalian insulin and IGFs, and dILPs control the duration of the larval period as well as the larval growth rate (Hietakangas and Cohen, 2009; Kannan and Fridell, 2013). Axenic fly larvae suffer from defected development, while colonization of Acetobacter pomorum effectively restore the body development and promoted DILP2 expression in larvae (Shin et al., 2011). By optimizing diet-derived branched-chain amino acids (BCAAs) level, the colonization of Lactobacillus plantarum stimulates the TOR kinase activity, leading to increased dILPs production in brain with elevated growth rate (Storelli et al., 2011). In the rodent model, a significant reduction of circulating GH and IGF-1 levels has been observed in both GF and axenic mice (Schwarzer et al., 2016; Yan et al., 2016; Weger et al., 2019), while the colonization of GF mice with gut microbiota from conventional raised mice or Lactobacillus plantarum strains can effectively restore the serum level of IGF-1 (Schwarzer et al., 2016; Yan et al., 2016). Early intestinal intervention with fecal microbiota transplantation promotes growth and elevates serum GH and IGF-1 levels in suckling piglets (Cheng et al., 2019). In addition, the presence of gut microbiota also increases the bone marrow IGF-1 level, indicating the enhanced autocrine and paracrine activity of IGF-1 in bone remodeling (Yan et al., 2016). Of note, the impacts of gut microbiota on the GH/IGF-1 axis depend on the duration of colonization and animal age. A reduced trabecular bone quantity and serum IGF-1 level has been observed in SPF mice as compared to that in GF mice (Novince et al., 2017), and accelerated bone resorption has also been detected after short-term colonization of gut microbiota to GF mice (Yan et al., 2016).
Produced by parathyroid glands, parathyroid hormone (PTH) is an important regulator of calcium metabolism, presenting as a double-edged sword to skeletal homeostasis. The administration of continuous PTH (cPTH) leads to increased level of receptor activator of NF-κB ligand (RANKL) and decreased osteoprotegerin (OPG), which aggravates bone loss (Huang et al., 2004; Jilka et al., 2010). In addition, the T cells-mediated bone resorption can be triggered by cPTH, and enhanced production of TNF is detected when targeting T cells with PTH (Gao et al., 2008; Li et al., 2015). On the contrary, intermittent PTH (iPTH) treatment elevates bone mass by activating the expansion and differentiation of osteoblasts (Nishida et al., 1994; Terauchi et al., 2009; Kim et al., 2012; Wein and Kronenberg, 2018). PTH-related protein (PTHrP) is expressed by chondrocytes in the resting zone of growth plate (Abad et al., 2002). Owing to the structure homology, PTHrP and PTH bind to the shared G-protein coupled receptor, PTH1R, which is expressed on osteoblast (Datta and Abou-Samra, 2009). Consistently, intermittent treatment with PTHrP shows anabolic effects by stimulating the differentiation of subchondral bone marrow-derived mesenchymal stem cell (SMSC), initiating endochondral ossification, and promoting the early osteoblastic cells development (Huch et al., 2003; Datta et al., 2007; Zhang et al., 2022), while continuous PTHrP treatment leads to bone resorption (Teitelbaum, 2000).
The regulatory effects of certain probiotic strains on PTH level have been observed in rodent models and clinical trials (Narva et al., 2004; Takasugi et al., 2011; Jafarnejad et al., 2017; Fernández-Murga et al., 2020), indicating the critical role of gut microbiota in in PTH production. Recent studies have further revealed the essential roles of gut microbiota in bone metabolism regulated by PTH. The absence of bone anabolic effect of iPTH has been observed in axenic mice (Li et al., 2020). Further investigation has revealed that butyrate, one of the gut microbial metabolites, facilitates the expansion of circulating regulatory T cells (Tregs) through GPR43 signaling. Besides, butyrate can potentiate iPTH in inducing bone marrow Treg cells and stimulate the secretion of osteogenic Wnt10b from CD8+ T cells (Li et al., 2020). On the other hand, cPTH only elicits bone catabolic activity in the mice colonized with segmented filamentous bacteria (SFB), rather than axenic mice (Yu et al., 2020). SFB are spore-forming, Gram-positive commensal bacteria that colonize in the murine small intestine, and potentially provoke the intestinal T cell responses (Davis and Savage, 1974; Gaboriau-Routhiau et al., 2009). The presence of SFB promotes cPTH in inducing Th17 and TNF+ T cells development. The elevated level of TNF in bone marrow stimulates the migration of intestinal Th17 cells to the bone marrow, and Th17 cells further promotes the osteoclastogenesis by secreting IL-17A, RANKL, TNF, IL-1, and IL-6 (Yu et al., 2020). As for PTHrP, no evidence has so far implied its correlation with gut microbiota.
Serotonin, also known as 5-hydroxytryptamine (5-HT), is a candidate hormone in bridging gut microbiota and bone homeostasis. Approximately 95% of body’s 5-HT is synthesized by enterochromaffin (EC) cells in the gastrointestinal tract under the control of tryptophan hydroxylase 1 (TPH1), while only a minor fraction is produced by brainstem spinal neurons (Gershon and Tack, 2007; Ducy and Karsenty, 2010). These 2 types of 5-HT play antagonistic functions in bone remodeling through different pathways. The peripheral 5-HT exerts negative effects on bone formation, for 5-HT not only suppresses the proliferation of osteoblast via Htr1b/PKA/CREB/cyclins signaling cascade, but also enhances the osteoclastic resorption (Yadav et al., 2008; Ducy and Karsenty, 2010; Chabbi-Achengli et al., 2012; Spohn and Mawe, 2017). The Tph1−/− mice exhibits ameliorated bone resorption as compared to the WT mice (Chabbi-Achengli et al., 2012). Consistently, TPH1 inhibition can rescue the bone loss in ovariectomized rodent models (Yadav et al., 2010; Inose et al., 2011). In addition, application of selective serotonin reuptake inhibitors (SSRIs), which are extensively used in treating psychiatric disorders, is associated with reduced BMD and bone formation marker (Feuer et al., 2015; Kumar et al., 2018; Wu et al., 2018). Thus, the inhibition of gut-derived 5-HT is considered as a potential treatment for the bone-loss disorder.
Emerging evidences indicate that the process of ECs producing 5-HT is microbiota-dependent. Down-regulated gene expression of TPH1 with deficient peripheral 5-HT level have been observed in GF mice, accompanied by improved bone mass (Wikoff et al., 2009; Yano et al., 2015). The reconstitution of gut microbiota in GF mice effectively restores the peripheral 5-HT concentration (Yano et al., 2015; Yan et al., 2018). Spore-forming bacteria (Sp) plays pivotal role in regulating EC cells’ function and 5-HT biosynthesis (Yano et al., 2015; Wu et al., 2018). In addition, microbes including Candida spp., Streptococcus spp., Escherichia spp., and Enterococcus spp., also facilitate 5-HT production (Cryan and Dinan, 2012). In addition, excessive ethanol consumption is recognized as an important risk factor for osteopenia (Luo et al., 2017; Wu et al., 2018), as gut microbiota dysbiosis caused by chronic ethanol abuse leads to elevated 5-HT concentration and aggravated bone resorption (Liu et al., 2022). In contrast to peripheral 5-HT, the brain derived central 5-HT acts as a neurotransmitter which promotes bone formation via binding to Htr2c receptors on ventromedial hypothalamic neurons (Yadav et al., 2009). Although no direct link between gut microbiota and central 5-HT has been demonstrated, propionic acid derived from gut bacteria can significantly reduce the brain 5-HT in Western Albino rats (Al-Ghamdi et al., 2014).
The gastrointestinal (GI) tract is an important site of endocrine production, and signaling molecules released from enteroendocrine cells (EECs) act on regulating digestive effect and energy metabolism. Since the clinical symptom of low BMD occasionally occurs after bariatric surgery or during gastrointestinal diseases (Lehto-Axtelius et al., 2002; Brzozowska et al., 2013), the involvement of gastrointestinal hormones in bone homeostasis has drawn increasing attention over the years. In addition, accumulating evidences have demonstrated that functions of EECs are under the regulation of gut microbiota, and the microbiota- hormone interaction is of great importance in the gut-bone axis (Furness et al., 2013; Viswanathan, 2013; Ye et al., 2021).
Ghrelin is a 28 amino acid peptide mainly synthesized in the stomach. Ghrelin was initially identified as the endogenous ligand of GH secretagogue receptor (GHSR), acting as an appetite stimulant and energy regulator (Kojima et al., 1999; Perelló and Zigman, 2012; Huang et al., 2017). In addition to its bone anabolic effect via stimulating GH secretion (Kojima et al., 1999), the GH-independent effects of ghrelin on skeletal metabolism have also been identified. By binding to the GHSR, ghrelin directly stimulates the proliferation and differentiation of osteoblasts (Fukushima et al., 2005; Kim et al., 2005; Maccarinelli et al., 2005), and the mitogen-activated protein kinase (MAPK)/phosphoinositide 3-kinase (PI3K) pathway is involved in this process (Delhanty et al., 2006). However, a negative association between serum ghrelin level and bone mass or bone synthesis marker PINP has been observed in either female suffered from anorexia nervosa or obesity patients who have weight loss, and this is probably attributed to the elevated adrenocorticotropic hormone (ACTH) level induced by ghrelin (Arvat et al., 2001; Misra et al., 2005; Yu et al., 2022).
The interaction between gut microbiota and ghrelin seems controversial. Patients with polycystic ovary syndrome (PCOS) exhibit gut microbial dysbiosis accompanied with decreased serum ghrelin (Liu et al., 2017). A case–control study has revealed that the ghrelin level is negatively correlated with fecal Bifidobacterium, Lactobacillus and Blautia coccoides-Eubacterium rectale group, but positively correlated with Bacteroides and Prevotella (Queipo-Ortuño et al., 2013). The absence of gut microbiota perturbs the secretion of ghrelin (Perry et al., 2016; Weger et al., 2019), while increased acetate production induced by colonization of gut microbiota to GF mice activates the parasympathetic nervous system and enhances ghrelin secretion (Perry et al., 2016). However, a recent study has shown the attenuating effects of SCFAs on ghrelin-mediated signaling through the GHSR-1a, suggesting a negative effect of gut microbiota in ghrelin-induced activity (Torres-Fuentes et al., 2019).
Incretins are a group of insulin-tropic peptides synthesized in small intestine, responding to nutrient intake and stimulating insulin secretion (Baggio and Drucker, 2007; Nauck and Meier, 2018). The most well-known incretins are glucose-dependent insulinotropic polypeptide (GIP) and glucagon-like peptide-1 (GLP-1), which are secreted by K-cells and L-cells respectively, and serve as anabolic signals to the skeletal system. GIP receptors (GIPRs) are widely expressed on osteoblastic lineage and osteoclasts (Bollag et al., 2000), and the activation of GIPRs leads to the release of bone formation markers, inhibition of osteoblasts apoptosis, and suppression of osteoclast functions (Zhong et al., 2007; Berlier et al., 2015; Mabilleau et al., 2016; Stensen et al., 2020). The beneficial effects of GIP on bone have also been observed in vivo, as GIP administration effectively prevents bone resorption (Bollag et al., 2001; Nissen et al., 2014), while GIPR knockout mice exhibits defective bone property and suppressed bone formation (Xie et al., 2005; Gaudin-Audrain et al., 2013; Mieczkowska et al., 2013). GLP-1 was previously believed to attenuate bone resorption by stimulating calcitonin production (Yamada et al., 2008). However, GLP-1 receptors (GLP-1Rs) are widely expressed on osteoblastic cell lines, bone marrow-derived macrophage (BMM), and preosteoclast, and GLP-1 can not only enhance the viability of osteoblast (Pacheco-Pantoja et al., 2011), but also inhibit osteoclastogenesis through the NF-κB/MAPK-nuclear factor of activated T cells (NFATc1) pathway (Li et al., 2020). Additionally, administration of GLP-1 to adipose-derived stem cells (ADSCs) stimulates the osteoblast differentiation (Lee et al., 2015), and upregulated expression of GLP-1R has been detected during osteogenesis (Jeon et al., 2014). The GLP-1 receptor knockout mice exhibit reduced bone quality but increased bone resorption (Yamada et al., 2008; Mabilleau et al., 2013), while GLP-1 treatment improves bone structure in streptozotocin-induced diabetic Wistar rats and hyperlipidemic rats (Nuche-Berenguer et al., 2009, 2011).
Studies have shown that administration of probiotics or prebiotics elevates the serum level of GLP-1 (Shirouchi et al., 2016; Kim et al., 2018; Wang et al., 2020). SCFAs can trigger the secretion of GLP-1 by activating SCFA receptors FFAR2 (GPR43) and FFAR3 (GPR41) in L cells (Tolhurst et al., 2012). Interestingly, elevated level of GLP-1 has also been observed in microbiota-deleted mice as compared to the conventionally raised mice (Wichmann et al., 2013; Zarrinpar et al., 2018; Heiss et al., 2021), and this increment is possibly attributed to the suppressed intestinal transit and altered colonocyte glucose utilization caused by SCFAs deficiency (Donohoe et al., 2011; Zarrinpar et al., 2018).
Belonging to the neuropeptide Y peptide family, peptide YY (PYY) is predominantly secreted from L-cells, and plays pivotal roles in regulating appetite and energy homeostasis (Batterham et al., 2002; Batterham and Bloom, 2003). PYY is regarded as a negative regulator in bone metabolism. A negative correlation between circulating PYY level and BMD has been observed in patients of anorexia nervosa, patients receiving bariatric surgery and premenopausal women (Utz et al., 2008; Scheid et al., 2011; Yu et al., 2016; Kim et al., 2020). PYY binds to Y-receptors, particularly Y1R and Y2R, with high affinity, and Y1R−/− mice exhibit enhanced osteoblastic activity with increased mineral apposition (Lee et al., 2011). However, the deletion of PYY in mice leads to controversial skeletal phenotypes. Replacement of the Pyy coding region with a lacZ reporter gene results in a decreased BMD (Wortley et al., 2007), while either retaining Cre recombinase gene and EGFP reporter gene or deletion of the entire PYY coding region promotes the bone formation (Wong et al., 2012; Leitch et al., 2019). These divergent phenotypes may be attributed to different mice age and gene manipulation approaches. The production of PYY is under the regulation of gut microbiota. The ceftazidime (an anti-Gram-negative bacteria antibiotic) treatment significantly promotes PYY secretion in high-fat diet feed mice (Rajpal et al., 2015). By activating Toll-like receptors (TLRs) in L-cells, the microbiota-derived butyrate strongly promotes the Pyy expression (Larraufie et al., 2017). Moreover, the bile acid G protein-coupled receptor, Takeda G protein receptor 5 (TGR5), stimulates the release of PYY, while H2S, produced by sulfate-reducing commensal bacteria in colon, inhibits the TGR5-dependent PYY release involving PLC-ε/Ca2+ pathway (Bala et al., 2014).
Accumulating evidences have revealed the intimate correlation between adipocytes-derived hormones and skeletal homeostasis. Leptin is considered as a crucial signal in regulating food intake and energy expenditure. By binding to the receptors on bone marrow mesenchymal stem cells (BMSCs) with high affinity, leptin promotes the proliferation and the differentiation of BMSC into the osteoblastic lineage (Thomas et al., 1999; Astudillo et al., 2008), and further stimulates the osteoblast development and bone mineralization (Reseland et al., 2001). In addition to the direct effect on bone cells, leptin exerts beneficial effects on skeletal health by facilitating GH secretion (Carro et al., 1997; Tannenbaum et al., 1998). Adiponectin is an adipocytokine that sensitizes the body to insulin (Kadowaki et al., 2006). Adiponectin has been shown to stimulate the differentiation of osteoblasts via MAKP-cyclooxygenase-2 (COX2) signaling (Luo et al., 2005; Lee et al., 2009). Moreover, leptin and adiponectin also show inhibitory effects on osteoclastogenesis (Holloway et al., 2002; Tu et al., 2011). Leptin level may also be regulated by gut microbiota, as positive correlations of leptin with Bifidobacterium and Lactobacillus, and negative correlations with Clostridium, Bacteroides and Prevotella have been demonstrated in rats under different nutritional status and physical activity (Queipo-Ortuño et al., 2013).
Taken together, although no direct causal effects between gut microbiota and endocrine system in regulating skeletal homeostasis have been well demonstrated, current evidence has shown strong intercorrelations between gut microbiota and the production and activity of hormones related to bone health. Further delineation of the underlying mechanisms by which gut microbiota interplays with endocrine system may provide novel target for the better management of skeletal disorders.
One of the important factors by which gut microbiota interplays with endocrine system is the production of SCFAs (Figure 1). Acetate, butyrate and propionate are the most common SCFAs generated from microbial fermentation of non-digestible polysaccharides. The involvement of SCFAs in skeletal homeostasis has been well reported in recent years (Zaiss et al., 2019; Wallimann et al., 2021). It has been demonstrated that SCFAs suppress the osteoclasts differentiation via inhibiting histone deacetylases (HDACs) and activating FFARs (Rahman et al., 2003; Kim et al., 2018; Yan et al., 2018; Montalvany-Antonucci et al., 2019). Additionally, SCFAs stimulate bone formation by increasing the production of bone sialoprotein (BSP) and osteopontin (OPN) from osteoblasts (Katono et al., 2008). Despite the direct effects on bone cells, SCFAs facilitate Tregs cells development (Furusawa et al., 2013; Smith et al., 2013; Dalile et al., 2019), and in the meantime inhibit osteoclastogenic Th17 cells (Asarat et al., 2016; Luu et al., 2019), maintaining the osteo-immune homeostasis. The administration of probiotics or berberine has been reported to effectively elevate the gut SCFAs level, leading to alleviated bone resorption (Jia et al., 2019, 2021).
SCFAs are capable of selectively promoting L-cell proliferation (Kaji et al., 2011; Petersen et al., 2014), which facilitate the enteroendocrine hormone production. SCFA receptors, especially FFAR2 and FFAR3, are expressed on L-cells that are involved in enteroendocrine hormones secretion (Karaki et al., 2006; Tazoe et al., 2009). SCFAs enhance the production of GLP-1and PYY by activating L-cells through FFARs (Lin et al., 2012; Brooks et al., 2017), and mice lacking of FFAR2 or FFAR3 secret reduced GLP-1 (Samuel et al., 2008; Tolhurst et al., 2012). The impacts of SCFAs on 5-HT secretion seem to be bone-detrimental. SCFAs can stimulate gut 5-HT release in rodent model by activating FFAR2 (Fukumoto et al., 2003; Akiba et al., 2015), and SCFAs can also promote the gene expression of Tph1 (Yano et al., 2015). Moreover, propionate is able to induce the brain toxication and deplete central 5-HT production (Al-Ghamdi et al., 2014). In addition to the well-recognized roles of gut microbiota in producing SCFAs, the expression of FFAR gene can also be regulated by gut microbiota (Han et al., 2021; Zhang et al., 2021).
The production of adipocytes-derived leptin is also stimulated by SCFAs, and Galpha(i) signaling is involved in this process (Zaibi et al., 2010). As for IGF-1, a concomitant decrease of gut SCFAs level and systemic IGF-1 level have been reported in antibiotic-treated mice, while SCFAs supplementation restores the IGF-1 secretion (Yan et al., 2016). However, no evidence has yet confirmed the involvement of FFARs in SCFAs-mediated IGF-1 production. However, the production of some hormones is suppressed by SCFAs. A decreased ghrelin level and attenuated ghrelin receptor signaling were observed with the application of SCFAs (Fukumori et al., 2011; Torres-Fuentes et al., 2019). The inhibition of GIP secretion was also conducted by SCFA binding FFAR3 (Lee et al., 2018), even though SCFAs facilitated the K-cells expansion.
Bile Acids (BAs) are another major contributor to the intercorrelations between gut microbiota and endocrine system in maintaining skeletal homeostasis (Figure 1). The primary bile acids (BAs), including cholic acid (CA) and chenodeoxycholic acid (CDCA), are cholesterol-derived metabolites synthesized in the liver, and then enter the duodenum through bile duct. Microbial degradation further coverts primary BAs to secondary BAs, such as lithocholic acid (LCA) and deoxycholic acid (DCA) (Russell, 2003). The systemic BA homeostasis is under the feedback control of farnesoid X receptor (FXR)-fibroblast growth factor 15 (FGF15) axis, which requires the presence of gut microbiota (Sinal et al., 2000; Chiang, 2013; Wang and Yao, 2021). Anaerobic bacteria such as Bacteroides, Eubacterium, and Clostridium are involved in the BAs metabolism (Narushima et al., 2006; Nicholson et al., 2012; Long et al., 2017; Wang and Yao, 2021). A positive correlation between serum level of total BAs and BMD has been reported in the postmenopausal women (Zhao et al., 2020), and FXR−/− mice exhibits reduced BMD (Cho et al., 2013), indicating the critical role of BAs in bone remodeling. BAs not only facilitate osteoblast formation by up-regulating the Runx2 expression and enhancing extracellular signal-regulated kinase (ERK) (Cho et al., 2013), but also promote the osteogenic differentiation of bone marrow-derived mesenchymal stem cells (BMMSCs) by regulating Integrin 5 (ITGA5) (Cha et al., 2016). Despite the direct effects on bone cells, FXR and G protein-coupled bile acid receptor-1 (Gpbar-1, also known as TGR5) are involved in BAs-stimulated GLP-1 and PYY secretion (Katsuma et al., 2005; Bala et al., 2014; Pathak et al., 2018). Lithocholic acid-producing bacteria including Acetatifactor and Bacteroideswere have been found to modulate FXR/TGR5/GLP-1 signaling (Pathak et al., 2018). However, negative effects of BAs on bone metabolism have been also reported. DCA can upregulate tph1 expression by activating TGR5, and thus promote gut 5-HT release, which may result in bone resorption (Alemi et al., 2013; Wang and Yao, 2021). FGF15 is also identified as a gut hormone, and the FGF15 knockout mice exhibits osteopenia phenotype, suggesting its positive effect in bone remodeling (Bozadjieva-Kramer et al., 2021). Application of antibiotics minocycline can lead to impaired skeletal maturation in young mice, likely due to excessive serum conjugated BAs resulted from gut microbiota dysbiosis and disrupted FXR-FGF15 axis (Carson et al., 2022).
The LPS derived from Gram-negative bacteria is known to be a Toll-like receptor (TLR) 4 ligand. By activating TLR4, LPS can stimulate 5-HT production from EC cells (Kidd et al., 2009). In addition to SCFAs, other bacterial metabolites including α-tocopherol, cholate, deoxycholate, p-aminobenzoate (PABA) and tyramine are also capable of stimulating gut-derived 5-HT synthesis (Yano et al., 2015). Furthermore, a recent study reported that extracellular RNAs may serve as an activator of ion channels. By sensing single-stranded RNA (ssRNA) from gut microbiota, Piezo1, a mechanosensitive Ca2+ channel in ECs, can be activated and subsequently triggers the transcription of Thp1 and production of 5-HT (Sugisawa et al., 2020).
Therefore, current evidence has suggested that bacterial metabolites, particularly SCFAs, are the major molecular basis by which gut microbiota regulates the enteroendocrine-osseous axis. In addition, bile acid homeostasis, which is closely associated with gut microbiota, also involved in the secretion of bone-related hormones such as 5-HT and GLP-1. Modulation of gut microbiota to promote SCFAs production and maintain a bile acid homeostasis is promising to a joint management of skeletal and endocrine disorders.
Probiotics are live microorganisms that benefit to human health when consumed in adequate amounts (Pineiro and Stanton, 2007). Mainly by elevating luminal SCFA levels, probiotics administration is capable of reducing intestinal permeability, alleviating inflammatory response and promoting osteoblast formation, thus exerting protective effects against bone loss (Ibáñez et al., 2019; Zaiss et al., 2019; Jia et al., 2021). Relevant studies on the anabolic effect of probiotics via regulating endocrine system are listed Table 1. GH and IGFs are the most commonly affected hormones by probiotics. Lactobacillus plantarum has been shown to sustain the growth of Drosophila and mice under the nutrition scarcity by promoting the production of dILPs or IGF-1 (Storelli et al., 2011; Schwarzer et al., 2016). Likewise, the beneficial impacts of probiotics on bone traits have been demonstrated in zebrafish and broiler chicken, accompanied with enhanced IGF and GH level (Avella et al., 2012; Abdelqader et al., 2020). Probiotics can also regulate PTH production. It has been reported that Bifidobacterium pseudocatenulatum can effectively reduce serum PTH level and meanwhile improve the trabecular architecture in mice fed with high fat diet (Fernández-Murga et al., 2020). In addition, Bacillus subtilis can reduce bone resorption in broilers under thermoneutral conditions, which is mediated by increased central 5-HT level (Yan et al., 2018). The regulatory effects of probiotics on GLP-1, leptin and adiponectin have also been reported, however, the relevant bone phenotypes have not been reported yet (Bagarolli et al., 2017; López-Moreno et al., 2020; Wang et al., 2020).
Prebiotics are defined as selectively fermented ingredients, which lead to specific gut microbiota alteration and confer benefits upon host health (Sheveleva, 1999). Non-digestible oligosaccharides (NDOs), such as galactooligtosaccharides (GOS), fructooligosaccharidesc (FOS), oligofructose, and inulin are regarded as the most beneficial prebiotics to bone health. Application of FOS, GOS and inulin not only promotes growth (Scholz-Ahrens et al., 2007; Edwards et al., 2020), but also effectively prevent the bone loss induced by ovarian hormone deficiency (Mathey et al., 2004; Zafar et al., 2004; Hooshmand et al., 2010; Seijo et al., 2019). Prebiotics also exert favorable impacts on bone health by regulating microbial ecology, promoting SCFAs production, and facilitating nutrition absorption (Scholz-Ahrens et al., 2007; Edwards et al., 2020). In addition, consumption of prebiotics elevates the level of hormones including IGF-1, IGF-2, and GH (Fordjour et al., 2010; Cluny et al., 2015; Kareem et al., 2016; Soumeh et al., 2019), which shows beneficiary effects on bone health. Although there still lacks direct evidence to support the interplay of prebiotics and hormones in maintaining bone health, a clinical study has shown that supplement of FOS-inulin to milk results in decreased serum level of PTH, accompanied with reduced bone resorption in postmenopausal women (Kruger et al., 2016).
Considering the intimate correlation between gut microbiota and skeletal health, microbiota-targeted technique, such as fecal microbiota transplantation (FMT), has become a promising therapeutic strategy in the treatment of bone disorder. FMT refers to the transferring of feces from a healthy donor to the GI tract of a recipient patient, in order to treat a specific disease associated with gut microbiota disorder (Cammarota et al., 2017). Animal studies have demonstrated that FMT can alleviate bone lose by improving intestinal microenvironment and adjusting metabolic functions (Ma et al., 2021; Zhang et al., 2022). A recent study has shown that FMT can sustain IGF-1 production and attenuate bone destruction in sickle cell disease mice by increasing the level of SCFAs (Xiao et al., 2022). FMT can also effectively prevent the estrogen deprivation-induced bone loss, accompanied by elevated SCFAs and decreased pro-osteoclastogenic cytokines release (Zhang et al., 2022). However, cautions should be taken when performing FMT, as it may result in side effects including abdominal discomfort, constipation, diarrhea, etc. (Baxter and Colville, 2016; Wang et al., 2019). In addition, inappropriate donor recruiting or fecal material screening may cause the contamination of patient with pathogenic micro-organisms (Wang et al., 2019; Antushevich, 2020).
Bacteriophages (phages) are viruses that infect bacteria, and can kill the host by its replication within infected bacterium and lysis (Wommack and Colwell, 2000). Since antibiotic resistance has become a severe problem in orthopedics, typically implant-associated infection (Campoccia et al., 2006), phage therapy is now regarded as valuable adjunct. In vitro studies have demonstrated that the utilization of phage exhibits anti-biofilm effect by suppressing the bacterial growth and adhesion (Kaur et al., 2014; Morris et al., 2019), and phage is also effective in alleviating skeletal inflammation in animal studies (Yilmaz et al., 2013; Kaur et al., 2016). However, the phage therapy is generally given by local injection, further studies focused on the treatment of skeletal disorder mediated by gut microbiota are still needed.
In a word, the manipulation of gut microbiota has been proved as a practicable strategy in improving skeletal health, mainly by promoting the production of SCFAs. However, the involved mechanisms, such as target endocrine factors and biological pathways, still needs further investigation. In addition, since every individual owns unique gut microbiota, more precise medical treatment is wait to be developed.
In recent years, the regulatory effects of gut microbiota on bone metabolism have attracted increasing attention. Despite the well-recognized pathways that involve gut barrier and osteoimmunology, emerging evidence has indicated that gut microbiota can impact bone health via interplaying with the endocrine system of host. Gut microbiota profoundly influences the secretion of a variety of bone metabolism-related hormones including IGF-1, 5-HT, PTH, GLP-1 and leptin, which further regulate bone homeostasis. SCFAs and BAs are identified as important mediators of gut microbiota in regulating skeletal health via enteroendocrine-osseous axis. Supplementation of probiotics and prebiotics may serve as a promising adjuvant therapy in promoting bone health. However, more well-controlled clinical trials are still needed to translate these findings to the better management of skeletal disorders.
XX designed and structured the manuscript. YT performed the literature search and wrote the draft of the manuscript. XK and LZ critically revised the manuscript. XX revised and edited the final version of the manuscript. All authors have read and agreed to the published version of the manuscript.
This study was supported by the grant from Science and Technology Department of Sichuan Province (2021YFQ0064), and the grant from Health Commission of Sichuan Province (21PJ058).
The authors declare that the research was conducted in the absence of any commercial or financial relationships that could be construed as a potential conflict of interest.
All claims expressed in this article are solely those of the authors and do not necessarily represent those of their affiliated organizations, or those of the publisher, the editors and the reviewers. Any product that may be evaluated in this article, or claim that may be made by its manufacturer, is not guaranteed or endorsed by the publisher.
Abad, V., Meyers, J. L., Weise, M., Gafni, R. I., Barnes, K. M., Nilsson, O., et al. (2002). The role of the resting zone in growth plate chondrogenesis. Endocrinology 143, 1851–1857. doi: 10.1210/endo.143.5.8776
Abdelqader, A., Abuajamieh, M., Hayajneh, F., and Al-Fataftah, A. R. (2020). Probiotic bacteria maintain normal growth mechanisms of heat stressed broiler chickens. J. Therm. Biol. 92:102654. doi: 10.1016/j.jtherbio.2020.102654
Ai, T., Hao, L., Shang, L., Wang, L., Li, B., and Li, J. (2021). Konjac oligosaccharides modulate the gut environment and promote bone health in calcium-deficient mice. J. Agric. Food Chem. 69, 4412–4422. doi: 10.1021/acs.jafc.0c07839
Akiba, Y., Inoue, T., Kaji, I., Higashiyama, M., Narimatsu, K., Iwamoto, K., et al. (2015). Short-chain fatty acid sensing in rat duodenum. J. Physiol. 593, 585–599. doi: 10.1113/jphysiol.2014.280792
Alemi, F., Poole, D. P., Chiu, J., Schoonjans, K., Cattaruzza, F., Grider, J. R., et al. (2013). The receptor TGR5 mediates the prokinetic actions of intestinal bile acids and is required for normal defecation in mice. Gastroenterology 144, 145–154. doi: 10.1053/j.gastro.2012.09.055
Al-Ghamdi, M., Al-Ayadhi, L., and El-Ansary, A. (2014). Selected biomarkers as predictive tools in testing efficacy of melatonin and coenzyme Q on propionic acid - induced neurotoxicity in rodent model of autism. BMC Neurosci. 15:34. doi: 10.1186/1471-2202-15-34
Antushevich, H. (2020). Fecal microbiota transplantation in disease therapy. Clin. Chim. Acta 503, 90–98. doi: 10.1016/j.cca.2019.12.010
Arvat, E., Maccario, M., Di Vito, L., Broglio, F., Benso, A., Gottero, C., et al. (2001). Endocrine activities of ghrelin, a natural growth hormone secretagogue (GHS), in humans: Comparison and interactions with hexarelin, a nonnatural peptidyl GHS, and GH-releasing hormone. J. Clin. Endocrinol. Metab. 86, 1169–1174. doi: 10.1210/jcem.86.3.7314
Asarat, M., Apostolopoulos, V., Vasiljevic, T., and Donkor, O. (2016). Short-chain fatty acids regulate cytokines and Th17/Treg cells in human peripheral blood mononuclear cells in vitro. Immunol. Investig. 45, 205–222. doi: 10.3109/08820139.2015.1122613
Assy, N., Pruzansky, Y., Gaitini, D., Shen Orr, Z., Hochberg, Z., and Baruch, Y. (2008). Growth hormone-stimulated IGF-1 generation in cirrhosis reflects hepatocellular dysfunction. J. Hepatol. 49, 34–42. doi: 10.1016/j.jhep.2008.02.013
Astudillo, P., Ríos, S., Pastenes, L., Pino, A. M., and Rodríguez, J. P. (2008). Increased adipogenesis of osteoporotic human-mesenchymal stem cells (MSCs) characterizes by impaired leptin action. J. Cell. Biochem. 103, 1054–1065. doi: 10.1002/jcb.21516
Avella, M. A., Place, A., Du, S. J., Williams, E., Silvi, S., Zohar, Y., et al. (2012). Lactobacillus rhamnosus accelerates zebrafish backbone calcification and gonadal differentiation through effects on the GnRH and IGF systems. PLoS One 7:e45572. doi: 10.1371/journal.pone.0045572
Bagarolli, R. A., Tobar, N., Oliveira, A. G., Araújo, T. G., Carvalho, B. M., Rocha, G. Z., et al. (2017). Probiotics modulate gut microbiota and improve insulin sensitivity in DIO mice. J. Nutr. Biochem. 50, 16–25. doi: 10.1016/j.jnutbio.2017.08.006
Baggio, L. L., and Drucker, D. J. (2007). Biology of incretins: GLP-1 and GIP. Gastroenterology 132, 2131–2157. doi: 10.1053/j.gastro.2007.03.054
Bala, V., Rajagopal, S., Kumar, D. P., Nalli, A. D., Mahavadi, S., Sanyal, A. J., et al. (2014). Release of GLP-1 and PYY in response to the activation of G protein-coupled bile acid receptor TGR5 is mediated by Epac/PLC-ε pathway and modulated by endogenous H2S. Front. Physiol. 5:420. doi: 10.3389/fphys.2014.00420
Bandow, K., Maeda, A., Kakimoto, K., Kusuyama, J., Shamoto, M., Ohnishi, T., et al. (2010). Molecular mechanisms of the inhibitory effect of lipopolysaccharide (LPS) on osteoblast differentiation. Biochem. Biophys. Res. Commun. 402, 755–761. doi: 10.1016/j.bbrc.2010.10.103
Baruch, Y., Assy, N., Amit, T., Krivoy, N., Strickovsky, D., Orr, Z. S., et al. (1998). Spontaneous pulsatility and pharmacokinetics of growth hormone in liver cirrhotic patients. J. Hepatol. 29, 559–564. doi: 10.1016/s0168-8278(98)80150-5
Batterham, R. L., and Bloom, S. R. (2003). The gut hormone peptide YY regulates appetite. Ann. N. Y. Acad. Sci. 994, 162–168. doi: 10.1111/j.1749-6632.2003.tb03176.x
Batterham, R. L., Cowley, M. A., Small, C. J., Herzog, H., Cohen, M. A., Dakin, C. L., et al. (2002). Gut hormone PYY(3-36) physiologically inhibits food intake. Nature 418, 650–654. doi: 10.1038/nature00887
Baxter, M., and Colville, A. (2016). Adverse events in faecal microbiota transplant: A review of the literature. J. Hosp. Infect. 92, 117–127. doi: 10.1016/j.jhin.2015.10.024
Berlier, J. L., Kharroubi, I., Zhang, J., Dalla Valle, A., Rigutto, S., Mathieu, M., et al. (2015). Glucose-dependent insulinotropic peptide prevents serum deprivation-induced apoptosis in human bone marrow-derived mesenchymal stem cells and osteoblastic cells. Stem Cell Rev. Rep. 11, 841–851. doi: 10.1007/s12015-015-9616-6
Bollag, R. J., Zhong, Q., Ding, K. H., Phillips, P., Zhong, L., Qin, F., et al. (2001). Glucose-dependent insulinotropic peptide is an integrative hormone with osteotropic effects. Mol. Cell. Endocrinol. 177, 35–41. doi: 10.1016/s0303-7207(01)00405-1
Bollag, R. J., Zhong, Q., Phillips, P., Min, L., Zhong, L., Cameron, R., et al. (2000). Osteoblast-derived cells express functional glucose-dependent insulinotropic peptide receptors. Endocrinology 141, 1228–1235. doi: 10.1210/endo.141.3.7366
Bozadjieva-Kramer, N., Shin, J. H., Shao, Y., Gutierrez-Aguilar, R., Li, Z., Heppner, K. M., et al. (2021). Intestinal-derived FGF15 protects against deleterious effects of vertical sleeve gastrectomy in mice. Nat. Commun. 12:4768. doi: 10.1038/s41467-021-24914-y
Brooks, L., Viardot, A., Tsakmaki, A., Stolarczyk, E., Howard, J. K., Cani, P. D., et al. (2017). Fermentable carbohydrate stimulates FFAR2-dependent colonic PYY cell expansion to increase satiety. Mol. Metab. 6, 48–60. doi: 10.1016/j.molmet.2016.10.011
Brzozowska, M. M., Sainsbury, A., Eisman, J. A., Baldock, P. A., and Center, J. R. (2013). Bariatric surgery, bone loss, obesity and possible mechanisms. Obes. Rev. 14, 52–67. doi: 10.1111/j.1467-789X.2012.01050.x
Cammarota, G., Ianiro, G., Tilg, H., Rajilić-Stojanović, M., Kump, P., Satokari, R., et al. (2017). European consensus conference on faecal microbiota transplantation in clinical practice. Gut 66, 569–580. doi: 10.1136/gutjnl-2016-313017
Campoccia, D., Montanaro, L., and Arciola, C. R. (2006). The significance of infection related to orthopedic devices and issues of antibiotic resistance. Biomaterials 27, 2331–2339. doi: 10.1016/j.biomaterials.2005.11.044
Carro, E., Señaris, R., Considine, R. V., Casanueva, F. F., and Dieguez, C. (1997). Regulation of in vivo growth hormone secretion by leptin. Endocrinology 138, 2203–2206. doi: 10.1210/endo.138.5.5238
Carson, M. D., Warner, A. J., Hathaway-Schrader, J. D., Geiser, V. L., Kim, J. D., Gerasco, J. E., et al. (2022). Minocycline-induced disruption of the intestinal FXR-FGF15 axis impairs osteogenesis in mice. JCI Insight 8:e160578. doi: 10.1172/jci.insight.160578
Castaneda, M., Strong, J. M., Alabi, D. A., and Hernandez, C. J. (2020). The gut microbiome and bone strength. Curr. Osteoporos. Rep. 18, 677–683. doi: 10.1007/s11914-020-00627-x
Cenci, S., Toraldo, G., Weitzmann, M. N., Roggia, C., Gao, Y., Qian, W. P., et al. (2003). Estrogen deficiency induces bone loss by increasing T cell proliferation and lifespan through IFN-gamma-induced class II transactivator. Proc. Natl. Acad. Sci. U. S. A. 100, 10405–10410. doi: 10.1073/pnas.1533207100
Cha, B. H., Jung, M. J., Moon, B. K., Kim, J. S., Ma, Y., Arai, Y., et al. (2016). Administration of tauroursodeoxycholic acid enhances osteogenic differentiation of bone marrow-derived mesenchymal stem cells and bone regeneration. Bone 83, 73–81. doi: 10.1016/j.bone.2015.10.011
Chabbi-Achengli, Y., Coudert, A. E., Callebert, J., Geoffroy, V., Côté, F., Collet, C., et al. (2012). Decreased osteoclastogenesis in serotonin-deficient mice. Proc. Natl. Acad. Sci. U. S. A. 109, 2567–2572. doi: 10.1073/pnas.1117792109
Cheng, C. S., Wei, H. K., Wang, P., Yu, H. C., Zhang, X. M., Jiang, S. W., et al. (2019). Early intervention with faecal microbiota transplantation: An effective means to improve growth performance and the intestinal development of suckling piglets. Animal 13, 533–541. doi: 10.1017/s1751731118001611
Chiang, J. Y. (2013). Bile acid metabolism and signaling. Compr. Physiol. 3, 1191–1212. doi: 10.1002/cphy.c120023
Cho, S. W., An, J. H., Park, H., Yang, J. Y., Choi, H. J., Kim, S. W., et al. (2013). Positive regulation of osteogenesis by bile acid through FXR. J. Bone Miner. Res. 28, 2109–2121. doi: 10.1002/jbmr.1961
Cluny, N. L., Eller, L. K., Keenan, C. M., Reimer, R. A., and Sharkey, K. A. (2015). Interactive effects of oligofructose and obesity predisposition on gut hormones and microbiota in diet-induced obese rats. Obesity 23, 769–778. doi: 10.1002/oby.21017
Colleluori, G., Aguirre, L., Napoli, N., Qualls, C., Villareal, D. T., and Armamento-Villareal, R. (2021). Testosterone therapy effects on bone mass and turnover in hypogonadal men with type 2 diabetes. J. Clin. Endocrinol. Metab. 106, e3058–e3068. doi: 10.1210/clinem/dgab181
Collier, J. (2007). Bone disorders in chronic liver disease. Hepatology 46, 1271–1278. doi: 10.1002/hep.21852
Cryan, J. F., and Dinan, T. G. (2012). Mind-altering microorganisms: The impact of the gut microbiota on brain and behaviour. Nat. Rev. Neurosci. 13, 701–712. doi: 10.1038/nrn3346
Dalile, B., Van Oudenhove, L., Vervliet, B., and Verbeke, K. (2019). The role of short-chain fatty acids in microbiota-gut-brain communication. Nat. Rev. Gastroenterol. Hepatol. 16, 461–478. doi: 10.1038/s41575-019-0157-3
Damien, E., Price, J. S., and Lanyon, L. E. (2000). Mechanical strain stimulates osteoblast proliferation through the estrogen receptor in males as well as females. J. Bone Miner. Res. 15, 2169–2177. doi: 10.1359/jbmr.2000.15.11.2169
Das, M., Cronin, O., Keohane, D. M., Cormac, E. M., Nugent, H., Nugent, M., et al. (2019). Gut microbiota alterations associated with reduced bone mineral density in older adults. Rheumatology 58, 2295–2304. doi: 10.1093/rheumatology/kez302
Datta, N. S., and Abou-Samra, A. B. (2009). PTH and PTHrP signaling in osteoblasts. Cell. Signal. 21, 1245–1254. doi: 10.1016/j.cellsig.2009.02.012
Datta, N. S., Pettway, G. J., Chen, C., Koh, A. J., and McCauley, L. K. (2007). Cyclin D1 as a target for the proliferative effects of PTH and PTHrP in early osteoblastic cells. J. Bone Miner. Res. 22, 951–964. doi: 10.1359/jbmr.070328
Davis, C. P., and Savage, D. C. (1974). Habitat, succession, attachment, and morphology of segmented, filamentous microbes indigenous to the murine gastrointestinal tract. Infect. Immun. 10, 948–956. doi: 10.1128/iai.10.4.948-956.1974
Delagrange, M., Rousseau, V., Cessans, C., Pienkowski, C., Oliver, I., Jouret, B., et al. (2021). Low bone mass in Noonan syndrome children correlates with decreased muscle mass and low IGF-1 levels. Bone 153:116170. doi: 10.1016/j.bone.2021.116170
Delhanty, P. J., van der Eerden, B. C., van der Velde, M., Gauna, C., Pols, H. A., Jahr, H., et al. (2006). Ghrelin and unacylated ghrelin stimulate human osteoblast growth via mitogen-activated protein kinase (MAPK)/phosphoinositide 3-kinase (PI3K) pathways in the absence of GHS-R1a. J. Endocrinol. 188, 37–47. doi: 10.1677/joe.1.06404
Donohoe, D. R., Garge, N., Zhang, X., Sun, W., O’Connell, T. M., Bunger, M. K., et al. (2011). The microbiome and butyrate regulate energy metabolism and autophagy in the mammalian colon. Cell Metab. 13, 517–526. doi: 10.1016/j.cmet.2011.02.018
Ducy, P., and Karsenty, G. (2010). The two faces of serotonin in bone biology. J. Cell Biol. 191, 7–13. doi: 10.1083/jcb.201006123
Edwards, P. T., Kashyap, P. C., and Preidis, G. A. (2020). Microbiota on biotics: Probiotics, prebiotics, and synbiotics to optimize growth and metabolism. Am. J. Physiol. Gastrointest. Liver Physiol. 319, G382–G390. doi: 10.1152/ajpgi.00028.2020
European Association for the Study of the Liver (2016). EASL Clinical Practice Guidelines: Liver transplantation. J. Hepatol. 64, 433–485. doi: 10.1016/j.jhep.2015.10.006
Fattorusso, A., Di Genova, L., Dell’Isola, G. B., Mencaroni, E., and Esposito, S. (2019). Autism Spectrum disorders and the gut microbiota. Nutrients 11:521. doi: 10.3390/nu11030521
Fazeli, P. K., Faje, A. T., Meenaghan, E., Russell, S. T., Resulaj, M., Lee, H., et al. (2020). IGF-1 is associated with estimated bone strength in anorexia nervosa. Osteoporos. Int. 31, 259–265. doi: 10.1007/s00198-019-05193-2
Fernández-Murga, M. L., Olivares, M., and Sanz, Y. (2020). Bifidobacterium pseudocatenulatum CECT 7765 reverses the adverse effects of diet-induced obesity through the gut-bone axis. Bone 141:115580. doi: 10.1016/j.bone.2020.115580
Feuer, A. J., Demmer, R. T., Thai, A., and Vogiatzi, M. G. (2015). Use of selective serotonin reuptake inhibitors and bone mass in adolescents: An NHANES study. Bone 78, 28–33. doi: 10.1016/j.bone.2015.04.042
Flores, R., Shi, J., Fuhrman, B., Xu, X., Veenstra, T. D., Gail, M. H., et al. (2012). Fecal microbial determinants of fecal and systemic estrogens and estrogen metabolites: A cross-sectional study. J. Transl. Med. 10:253. doi: 10.1186/1479-5876-10-253
Fordjour, L., D’Souza, A., Cai, C., Ahmad, A., Valencia, G., Kumar, D., et al. (2010). Comparative effects of probiotics, prebiotics, and synbiotics on growth factors in the large bowel in a rat model of formula-induced bowel inflammation. J. Pediatr. Gastroenterol. Nutr. 51, 507–513. doi: 10.1097/MPG.0b013e3181df5ff2
Fukumori, R., Sugino, T., Hasegawa, Y., Kojima, M., Kangawa, K., Obitsu, T., et al. (2011). Plasma ghrelin concentration is decreased by short chain fatty acids in wethers. Domest. Anim. Endocrinol. 41, 50–55. doi: 10.1016/j.domaniend.2011.04.001
Fukumoto, S., Tatewaki, M., Yamada, T., Fujimiya, M., Mantyh, C., Voss, M., et al. (2003). Short-chain fatty acids stimulate colonic transit via intraluminal 5-HT release in rats. Am. J. Physiol. Regul. Integr. Comp. Physiol. 284, R1269–R1276. doi: 10.1152/ajpregu.00442.2002
Fukushima, N., Hanada, R., Teranishi, H., Fukue, Y., Tachibana, T., Ishikawa, H., et al. (2005). Ghrelin directly regulates bone formation. J. Bone Miner. Res. 20, 790–798. doi: 10.1359/jbmr.041237
Furness, J. B., Rivera, L. R., Cho, H. J., Bravo, D. M., and Callaghan, B. (2013). The gut as a sensory organ. Nat. Rev. Gastroenterol. Hepatol. 10, 729–740. doi: 10.1038/nrgastro.2013.180
Furusawa, Y., Obata, Y., Fukuda, S., Endo, T. A., Nakato, G., Takahashi, D., et al. (2013). Commensal microbe-derived butyrate induces the differentiation of colonic regulatory T cells. Nature 504, 446–450. doi: 10.1038/nature12721
Gaboriau-Routhiau, V., Rakotobe, S., Lécuyer, E., Mulder, I., Lan, A., Bridonneau, C., et al. (2009). The key role of segmented filamentous bacteria in the coordinated maturation of gut helper T cell responses. Immunity 31, 677–689. doi: 10.1016/j.immuni.2009.08.020
Gao, Y., Wu, X., Terauchi, M., Li, J. Y., Grassi, F., Galley, S., et al. (2008). T cells potentiate PTH-induced cortical bone loss through CD40L signaling. Cell Metab. 8, 132–145. doi: 10.1016/j.cmet.2008.07.001
Gaudin-Audrain, C., Irwin, N., Mansur, S., Flatt, P. R., Thorens, B., Baslé, M., et al. (2013). Glucose-dependent insulinotropic polypeptide receptor deficiency leads to modifications of trabecular bone volume and quality in mice. Bone 53, 221–230. doi: 10.1016/j.bone.2012.11.039
Gershon, M. D., and Tack, J. (2007). The serotonin signaling system: From basic understanding to drug development for functional GI disorders. Gastroenterology 132, 397–414. doi: 10.1053/j.gastro.2006.11.002
Guss, J. D., Horsfield, M. W., Fontenele, F. F., Sandoval, T. N., Luna, M., Apoorva, F., et al. (2017). Alterations to the gut microbiome impair bone strength and tissue material properties. J. Bone Miner. Res. 32, 1343–1353. doi: 10.1002/jbmr.3114
Hamrick, M. W. (2012). The skeletal muscle secretome: An emerging player in muscle-bone crosstalk. Bonekey Rep. 1:60. doi: 10.1038/bonekey.2012.60
Han, X., Feng, Z., Chen, Y., Zhu, L., Li, X., Wang, X., et al. (2022). Effects of high-fructose corn syrup on bone health and gastrointestinal microbiota in growing male mice. Front. Nutr. 9:829396. doi: 10.3389/fnut.2022.829396
Han, X., Wang, Y., Zhang, P., Zhu, M., Li, L., Mao, X., et al. (2021). Kazak faecal microbiota transplantation induces short-chain fatty acids that promote glucagon-like peptide-1 secretion by regulating gut microbiota in db/db mice. Pharm. Biol. 59, 1077–1087. doi: 10.1080/13880209.2021.1954667
He, J., Xu, S., Zhang, B., Xiao, C., Chen, Z., Si, F., et al. (2020). Gut microbiota and metabolite alterations associated with reduced bone mineral density or bone metabolic indexes in postmenopausal osteoporosis. Aging 12, 8583–8604. doi: 10.18632/aging.103168
Heiss, C. N., Mannerås-Holm, L., Lee, Y. S., Serrano-Lobo, J., Håkansson Gladh, A., Seeley, R. J., et al. (2021). The gut microbiota regulates hypothalamic inflammation and leptin sensitivity in Western diet-fed mice via a GLP-1R-dependent mechanism. Cell Rep. 35:109163. doi: 10.1016/j.celrep.2021.109163
Held, M. A., Cosme-Blanco, W., Difedele, L. M., Bonkowski, E. L., Menon, R. K., and Denson, L. A. (2005). Alterations in growth hormone receptor abundance regulate growth hormone signaling in murine obstructive cholestasis. Am. J. Physiol. Gastrointest. Liver Physiol. 288, G986–G993. doi: 10.1152/ajpgi.00287.2004
Hietakangas, V., and Cohen, S. M. (2009). Regulation of tissue growth through nutrient sensing. Annu. Rev. Genet. 43, 389–410. doi: 10.1146/annurev-genet-102108-134815
Hirano, S., Zhou, Q., Furuyama, A., and Kanno, S. (2017). Differential regulation of IL-1β and IL-6 release in murine macrophages. Inflammation 40, 1933–1943. doi: 10.1007/s10753-017-0634-1
Hold, G. L. (2016). Gastrointestinal microbiota and colon cancer. Dig. Dis. 34, 244–250. doi: 10.1159/000443358
Holloway, W. R., Collier, F. M., Aitken, C. J., Myers, D. E., Hodge, J. M., Malakellis, M., et al. (2002). Leptin inhibits osteoclast generation. J. Bone Miner. Res. 17, 200–209. doi: 10.1359/jbmr.2002.17.2.200
Hooshmand, S., Juma, S., and Arjmandi, B. H. (2010). Combination of genistin and fructooligosaccharides prevents bone loss in ovarian hormone deficiency. J. Med. Food 13, 320–325. doi: 10.1089/jmf.2009.0059
Hou, P., Sato, T., Hofstetter, W., and Foged, N. T. (1997). Identification and characterization of the insulin-like growth factor I receptor in mature rabbit osteoclasts. J. Bone Miner. Res. 12, 534–540. doi: 10.1359/jbmr.1997.12.4.534
Hsu, C. Y., Chen, L. R., and Chen, K. H. (2020). Osteoporosis in patients with chronic kidney diseases: A systemic review. Int. J. Mol. Sci. 21:6846. doi: 10.3390/ijms21186846
Huang, J. C., Sakata, T., Pfleger, L. L., Bencsik, M., Halloran, B. P., Bikle, D. D., et al. (2004). PTH differentially regulates expression of RANKL and OPG. J. Bone Miner. Res. 19, 235–244. doi: 10.1359/jbmr.0301226
Huang, H. J., Zhu, X. C., Han, Q. Q., Wang, Y. L., Yue, N., Wang, J., et al. (2017). Ghrelin alleviates anxiety- and depression-like behaviors induced by chronic unpredictable mild stress in rodents. Behav. Brain Res. 326, 33–43. doi: 10.1016/j.bbr.2017.02.040
Huch, K., Kleffner, S., Stöve, J., Puhl, W., Günther, K. P., and Brenner, R. E. (2003). PTHrP, PTHr, and FGFR3 are involved in the process of endochondral ossification in human osteophytes. Histochem. Cell Biol. 119, 281–287. doi: 10.1007/s00418-003-0519-2
Ibáñez, L., Rouleau, M., Wakkach, A., and Blin-Wakkach, C. (2019). Gut microbiome and bone. Joint Bone Spine 86, 43–47. doi: 10.1016/j.jbspin.2018.02.008
Inose, H., Zhou, B., Yadav, V. K., Guo, X. E., Karsenty, G., and Ducy, P. (2011). Efficacy of serotonin inhibition in mouse models of bone loss. J. Bone Miner. Res. 26, 2002–2011. doi: 10.1002/jbmr.439
Islam, S., Hassan, F., Tumurkhuu, G., Dagvadorj, J., Koide, N., Naiki, Y., et al. (2007). Bacterial lipopolysaccharide induces osteoclast formation in RAW 264.7 macrophage cells. Biochem. Biophys. Res. Commun. 360, 346–351. doi: 10.1016/j.bbrc.2007.06.023
Jafarnejad, S., Djafarian, K., Fazeli, M. R., Yekaninejad, M. S., Rostamian, A., and Keshavarz, S. A. (2017). Effects of a multispecies probiotic supplement on bone health in osteopenic postmenopausal women: A randomized, double-blind. Controlled Trial. J. Am. Coll. Nutr. 36, 497–506. doi: 10.1080/07315724.2017.1318724
Jeon, Y. K., Bae, M. J., Kim, J. I., Kim, J. H., Choi, S. J., Kwon, S. K., et al. (2014). Expression of glucagon-like peptide 1 receptor during osteogenic differentiation of adipose-derived stem cells. Endocrinol. Metab. 29, 567–573. doi: 10.3803/EnM.2014.29.4.567
Jia, X., Jia, L., Mo, L., Yuan, S., Zheng, X., He, J., et al. (2019). Berberine ameliorates periodontal bone loss by regulating gut microbiota. J. Dent. Res. 98, 107–116. doi: 10.1177/0022034518797275
Jia, L., Tu, Y., Jia, X., Du, Q., Zheng, X., Yuan, Q., et al. (2021). Probiotics ameliorate alveolar bone loss by regulating gut microbiota. Cell Prolif. 54:e13075. doi: 10.1111/cpr.13075
Jilka, R. L., O’Brien, C. A., Bartell, S. M., Weinstein, R. S., and Manolagas, S. C. (2010). Continuous elevation of PTH increases the number of osteoblasts via both osteoclast-dependent and -independent mechanisms. J. Bone Miner. Res. 25, 2427–2437. doi: 10.1002/jbmr.145
Johnston, C. B., and Dagar, M. (2020). Osteoporosis in older adults. Med. Clin. North Am. 104, 873–884. doi: 10.1016/j.mcna.2020.06.004
Kadowaki, T., Yamauchi, T., Kubota, N., Hara, K., Ueki, K., and Tobe, K. (2006). Adiponectin and adiponectin receptors in insulin resistance, diabetes, and the metabolic syndrome. J. Clin. Invest. 116, 1784–1792. doi: 10.1172/jci29126
Kaji, I., Karaki, S., Tanaka, R., and Kuwahara, A. (2011). Density distribution of free fatty acid receptor 2 (FFA2)-expressing and GLP-1-producing enteroendocrine L cells in human and rat lower intestine, and increased cell numbers after ingestion of fructo-oligosaccharide. J. Mol. Histol. 42, 27–38. doi: 10.1007/s10735-010-9304-4
Kannan, K., and Fridell, Y. W. (2013). Functional implications of Drosophila insulin-like peptides in metabolism, aging, and dietary restriction. Front. Physiol. 4:288. doi: 10.3389/fphys.2013.00288
Karaki, S., Mitsui, R., Hayashi, H., Kato, I., Sugiya, H., Iwanaga, T., et al. (2006). Short-chain fatty acid receptor, GPR43, is expressed by enteroendocrine cells and mucosal mast cells in rat intestine. Cell Tissue Res. 324, 353–360. doi: 10.1007/s00441-005-0140-x
Kareem, K. Y., Loh, T. C., Foo, H. L., Akit, H., and Samsudin, A. A. (2016). Effects of dietary postbiotic and inulin on growth performance, IGF1 and GHR mRNA expression, faecal microbiota and volatile fatty acids in broilers. BMC Vet. Res. 12:163. doi: 10.1186/s12917-016-0790-9
Katono, T., Kawato, T., Tanabe, N., Suzuki, N., Iida, T., Morozumi, A., et al. (2008). Sodium butyrate stimulates mineralized nodule formation and osteoprotegerin expression by human osteoblasts. Arch. Oral Biol. 53, 903–909. doi: 10.1016/j.archoralbio.2008.02.016
Katsuma, S., Hirasawa, A., and Tsujimoto, G. (2005). Bile acids promote glucagon-like peptide-1 secretion through TGR5 in a murine enteroendocrine cell line STC-1. Biochem. Biophys. Res. Commun. 329, 386–390. doi: 10.1016/j.bbrc.2005.01.139
Kaur, S., Harjai, K., and Chhibber, S. (2014). Bacteriophage mediated killing of Staphylococcus aureus in vitro on orthopaedic K wires in presence of linezolid prevents implant colonization. PLoS One 9:e90411. doi: 10.1371/journal.pone.0090411
Kaur, S., Harjai, K., and Chhibber, S. (2016). In vivo assessment of phage and linezolid based implant coatings for treatment of methicillin resistant S. aureus (MRSA) mediated orthopaedic device related infections. PLoS One 11:e0157626. doi: 10.1371/journal.pone.0157626
Kenny, A. M., Prestwood, K. M., Gruman, C. A., Marcello, K. M., and Raisz, L. G. (2001). Effects of transdermal testosterone on bone and muscle in older men with low bioavailable testosterone levels. J. Gerontol. A Biol. Sci. Med. Sci. 56, M266–M272. doi: 10.1093/gerona/56.5.m266
Kidd, M., Gustafsson, B. I., Drozdov, I., and Modlin, I. M. (2009). IL1beta- and LPS-induced serotonin secretion is increased in EC cells derived from Crohn’s disease. Neurogastroenterol. Motil. 21, 439–450. doi: 10.1111/j.1365-2982.2008.01210.x
Kim, S. W., Her, S. J., Park, S. J., Kim, D., Park, K. S., Lee, H. K., et al. (2005). Ghrelin stimulates proliferation and differentiation and inhibits apoptosis in osteoblastic MC3T3-E1 cells. Bone 37, 359–369. doi: 10.1016/j.bone.2005.04.020
Kim, Y. A., Keogh, J. B., and Clifton, P. M. (2018). Probiotics, prebiotics, synbiotics and insulin sensitivity. Nutr. Res. Rev. 31, 35–51. doi: 10.1017/s095442241700018x
Kim, D. S., Kwon, J. E., Lee, S. H., Kim, E. K., Ryu, J. G., Jung, K. A., et al. (2018). Attenuation of rheumatoid inflammation by sodium butyrate through reciprocal targeting of HDAC2 in osteoclasts and HDAC8 in T cells. Front. Immunol. 9:1525. doi: 10.3389/fimmu.2018.01525
Kim, S. W., Pajevic, P. D., Selig, M., Barry, K. J., Yang, J. Y., Shin, C. S., et al. (2012). Intermittent parathyroid hormone administration converts quiescent lining cells to active osteoblasts. J. Bone Miner. Res. 27, 2075–2084. doi: 10.1002/jbmr.1665
Kim, T. Y., Shoback, D. M., Black, D. M., Rogers, S. J., Stewart, L., Carter, J. T., et al. (2020). Increases in PYY and uncoupling of bone turnover are associated with loss of bone mass after gastric bypass surgery. Bone 131:115115. doi: 10.1016/j.bone.2019.115115
Kirk, B., Feehan, J., Lombardi, G., and Duque, G. (2020). Muscle, bone, and fat crosstalk: The biological role of myokines, osteokines, and adipokines. Curr. Osteoporos. Rep. 18, 388–400. doi: 10.1007/s11914-020-00599-y
Kojima, M., Hosoda, H., Date, Y., Nakazato, M., Matsuo, H., and Kangawa, K. (1999). Ghrelin is a growth-hormone-releasing acylated peptide from stomach. Nature 402, 656–660. doi: 10.1038/45230
Kovvuru, K., Kanduri, S. R., Vaitla, P., Marathi, R., Gosi, S., Garcia Anton, D. F., et al. (2020). Risk factors and management of osteoporosis post-transplant. Medicina 56:302. doi: 10.3390/medicina56060302
Kruger, M. C., Chan, Y. M., Kuhn-Sherlock, B., Lau, L. T., Lau, C., Chin, Y. S., et al. (2016). Differential effects of calcium- and vitamin D-fortified milk with FOS-inulin compared to regular milk, on bone biomarkers in Chinese pre- and postmenopausal women. Eur. J. Nutr. 55, 1911–1921. doi: 10.1007/s00394-015-1007-x
Krum, S. A., Miranda-Carboni, G. A., Hauschka, P. V., Carroll, J. S., Lane, T. F., Freedman, L. P., et al. (2008). Estrogen protects bone by inducing Fas ligand in osteoblasts to regulate osteoclast survival. EMBO J. 27, 535–545. doi: 10.1038/sj.emboj.7601984
Kumar, M., Wadhwa, R., Kothari, P., Trivedi, R., and Vohora, D. (2018). Differential effects of serotonin reuptake inhibitors fluoxetine and escitalopram on bone markers and microarchitecture in Wistar rats. Eur. J. Pharmacol. 825, 57–62. doi: 10.1016/j.ejphar.2018.02.026
Lafage Proust, M. H. (2017). How the gut affects bone metabolism. Joint Bone Spine 84, 515–519. doi: 10.1016/j.jbspin.2016.12.015
Lambert, M. N. T., Thybo, C. B., Lykkeboe, S., Rasmussen, L. M., Frette, X., Christensen, L. P., et al. (2017). Combined bioavailable isoflavones and probiotics improve bone status and estrogen metabolism in postmenopausal osteopenic women: A randomized controlled trial. Am. J. Clin. Nutr. 106, 909–920. doi: 10.3945/ajcn.117.153353
Lane, J. M., Russell, L., and Khan, S. N. (2000). Osteoporosis. Clin. Orthop. Relat. Res. 372, 139–150. doi: 10.1097/00003086-200003000-00016
Larraufie, P., Doré, J., Lapaque, N., and Blottière, H. M. (2017). TLR ligands and butyrate increase Pyy expression through two distinct but inter-regulated pathways. Cell. Microbiol. 19:e12648. doi: 10.1111/cmi.12648
Lee, H. M., Joo, B. S., Lee, C. H., Kim, H. Y., Ock, J. H., and Lee, Y. S. (2015). Effect of glucagon-like peptide-1 on the differentiation of adipose-derived stem cells into osteoblasts and adipocytes. J. Menopausal Med. 21, 93–103. doi: 10.6118/jmm.2015.21.2.93
Lee, H. W., Kim, S. Y., Kim, A. Y., Lee, E. J., Choi, J. Y., and Kim, J. B. (2009). Adiponectin stimulates osteoblast differentiation through induction of COX2 in mesenchymal progenitor cells. Stem Cells 27, 2254–2262. doi: 10.1002/stem.144
Lee, N. J., Nguyen, A. D., Enriquez, R. F., Doyle, K. L., Sainsbury, A., Baldock, P. A., et al. (2011). Osteoblast specific Y1 receptor deletion enhances bone mass. Bone 48, 461–467. doi: 10.1016/j.bone.2010.10.174
Lee, E. Y., Zhang, X., Miyamoto, J., Kimura, I., Taknaka, T., Furusawa, K., et al. (2018). Gut carbohydrate inhibits GIP secretion via a microbiota/SCFA/FFAR3 pathway. J. Endocrinol. 239, 267–276. doi: 10.1530/joe-18-0241
Lehto-Axtelius, D., Chen, D., Surve, V. V., and Håkanson, R. (2002). Post-gastrectomy osteopenia in the rat: Bone structure is preserved by retaining 10-30% of the oxyntic gland area. Scand. J. Gastroenterol. 37, 437–443. doi: 10.1080/003655202317316079
Leitch, V. D., Brassill, M. J., Rahman, S., Butterfield, N. C., Ma, P., Logan, J. G., et al. (2019). PYY is a negative regulator of bone mass and strength. Bone 127, 427–435. doi: 10.1016/j.bone.2019.07.011
Li, J. Y., Chassaing, B., Tyagi, A. M., Vaccaro, C., Luo, T., Adams, J., et al. (2016). Sex steroid deficiency-associated bone loss is microbiota dependent and prevented by probiotics. J. Clin. Invest. 126, 2049–2063. doi: 10.1172/jci86062
Li, J. Y., D’Amelio, P., Robinson, J., Walker, L. D., Vaccaro, C., Luo, T., et al. (2015). IL-17A is increased in humans with primary hyperparathyroidism and mediates PTH-induced bone loss in mice. Cell Metab. 22, 799–810. doi: 10.1016/j.cmet.2015.09.012
Li, C., Huang, Q., Yang, R., Dai, Y., Zeng, Y., Tao, L., et al. (2019). Gut microbiota composition and bone mineral loss-epidemiologic evidence from individuals in Wuhan, China. Osteoporos Int. 30, 1003–1013. doi: 10.1007/s00198-019-04855-5
Li, Z., Li, S., Wang, N., Xue, P., and Li, Y. (2020). Liraglutide, a glucagon-like peptide-1 receptor agonist, suppresses osteoclastogenesis through the inhibition of NF-κB and MAPK pathways via GLP-1R. Biomed. Pharmacother. 130:110523. doi: 10.1016/j.biopha.2020.110523
Li, C., Pi, G., and Li, F. (2021). The role of intestinal flora in the regulation of bone homeostasis. Front. Cell. Infect. Microbiol. 11:579323. doi: 10.3389/fcimb.2021.579323
Li, J. Y., Yu, M., Pal, S., Tyagi, A. M., Dar, H., Adams, J., et al. (2020). Parathyroid hormone-dependent bone formation requires butyrate production by intestinal microbiota. J. Clin. Invest. 130, 1767–1781. doi: 10.1172/jci133473
Lin, H. V., Frassetto, A., Kowalik, E. J. Jr., Nawrocki, A. R., Lu, M. M., Kosinski, J. R., et al. (2012). Butyrate and propionate protect against diet-induced obesity and regulate gut hormones via free fatty acid receptor 3-independent mechanisms. PLoS One 7:e35240. doi: 10.1371/journal.pone.0035240
Liu, Z., Xu, X., Shen, Y., Hao, Y., Cui, W., Li, W., et al. (2022). Altered gut microbiota and metabolites profile are associated with reduced bone metabolism in ethanol-induced osteoporosis. Cell Prolif. 55:e13245. doi: 10.1111/cpr.13245
Liu, R., Zhang, C., Shi, Y., Zhang, F., Li, L., Wang, X., et al. (2017). Dysbiosis of gut microbiota associated with clinical parameters in polycystic ovary syndrome. Front. Microbiol. 8:324. doi: 10.3389/fmicb.2017.00324
Long, S. L., Gahan, C. G. M., and Joyce, S. A. (2017). Interactions between gut bacteria and bile in health and disease. Mol. Asp. Med. 56, 54–65. doi: 10.1016/j.mam.2017.06.002
López-Moreno, A., Suárez, A., Avanzi, C., Monteoliva-Sánchez, M., and Aguilera, M. (2020). Probiotic strains and intervention total doses for modulating obesity-related microbiota dysbiosis: A systematic review and meta-analysis. Nutrients 12:1921. doi: 10.3390/nu12071921
Luo, X. H., Guo, L. J., Yuan, L. Q., Xie, H., Zhou, H. D., Wu, X. P., et al. (2005). Adiponectin stimulates human osteoblasts proliferation and differentiation via the MAPK signaling pathway. Exp. Cell Res. 309, 99–109. doi: 10.1016/j.yexcr.2005.05.021
Luo, Z., Liu, Y., Liu, Y., Chen, H., Shi, S., and Liu, Y. (2017). Cellular and molecular mechanisms of alcohol-induced osteopenia. Cell. Mol. Life Sci. 74, 4443–4453. doi: 10.1007/s00018-017-2585-y
Luu, M., Pautz, S., Kohl, V., Singh, R., Romero, R., Lucas, S., et al. (2019). The short-chain fatty acid pentanoate suppresses autoimmunity by modulating the metabolic-epigenetic crosstalk in lymphocytes. Nat. Commun. 10:760. doi: 10.1038/s41467-019-08711-2
Ma, S., Wang, N., Zhang, P., Wu, W., and Fu, L. (2021). Fecal microbiota transplantation mitigates bone loss by improving gut microbiome composition and gut barrier function in aged rats. PeerJ 9:e12293. doi: 10.7717/peerj.12293
Mabilleau, G., Mieczkowska, A., Irwin, N., Flatt, P. R., and Chappard, D. (2013). Optimal bone mechanical and material properties require a functional glucagon-like peptide-1 receptor. J. Endocrinol. 219, 59–68. doi: 10.1530/joe-13-0146
Mabilleau, G., Perrot, R., Mieczkowska, A., Boni, S., Flatt, P. R., Irwin, N., et al. (2016). Glucose-dependent insulinotropic polypeptide (GIP) dose-dependently reduces osteoclast differentiation and resorption. Bone 91, 102–112. doi: 10.1016/j.bone.2016.07.014
Maccarinelli, G., Sibilia, V., Torsello, A., Raimondo, F., Pitto, M., Giustina, A., et al. (2005). Ghrelin regulates proliferation and differentiation of osteoblastic cells. J. Endocrinol. 184, 249–256. doi: 10.1677/joe.1.05837
Makki, K., Deehan, E. C., Walter, J., and Bäckhed, F. J. (2018). The impact of dietary fiber on gut microbiota in host health and disease. Cell Host Microbe 23, 705–715. doi: 10.1016/j.chom.2018.05.012
Mangiola, F., Ianiro, G., Franceschi, F., Fagiuoli, S., Gasbarrini, G., and Gasbarrini, A. (2016). Gut microbiota in autism and mood disorders. World J. Gastroenterol. 22, 361–368. doi: 10.3748/wjg.v22.i1.361
Markle, J. G., Frank, D. N., Mortin-Toth, S., Robertson, C. E., Feazel, L. M., Rolle-Kampczyk, U., et al. (2013). Sex differences in the gut microbiome drive hormone-dependent regulation of autoimmunity. Science 339, 1084–1088. doi: 10.1126/science.1233521
Maruvada, P., Leone, V., Kaplan, L. M., and Chang, E. B. (2017). The human microbiome and obesity: Moving beyond associations. Cell Host Microbe 22, 589–599. doi: 10.1016/j.chom.2017.10.005
Mathey, J., Puel, C., Kati-Coulibaly, S., Bennetau-Pelissero, C., Davicco, M. J., Lebecque, P., et al. (2004). Fructooligosaccharides maximize bone-sparing effects of soy isoflavone-enriched diet in the ovariectomized rat. Calcif. Tissue Int. 75, 169–179. doi: 10.1007/s00223-004-0128-7
Mieczkowska, A., Irwin, N., Flatt, P. R., Chappard, D., and Mabilleau, G. (2013). Glucose-dependent insulinotropic polypeptide (GIP) receptor deletion leads to reduced bone strength and quality. Bone 56, 337–342. doi: 10.1016/j.bone.2013.07.003
Misra, M., Miller, K. K., Stewart, V., Hunter, E., Kuo, K., Herzog, D. B., et al. (2005). Ghrelin and bone metabolism in adolescent girls with anorexia nervosa and healthy adolescents. J. Clin. Endocrinol. Metab. 90, 5082–5087. doi: 10.1210/jc.2005-0512
Montalvany-Antonucci, C. C., Duffles, L. F., de Arruda, J. A. A., Zicker, M. C., de Oliveira, S., Macari, S., et al. (2019). Short-chain fatty acids and FFAR2 as suppressors of bone resorption. Bone 125, 112–121. doi: 10.1016/j.bone.2019.05.016
Morris, J., Kelly, N., Elliott, L., Grant, A., Wilkinson, M., Hazratwala, K., et al. (2019). Evaluation of bacteriophage anti-biofilm activity for potential control of orthopedic implant-related infections caused by Staphylococcus aureus. Surg. Infect. 20, 16–24. doi: 10.1089/sur.2018.135
Mosekilde, L. (2008). Primary hyperparathyroidism and the skeleton. Clin. Endocrinol. 69, 1–19. doi: 10.1111/j.1365-2265.2007.03162.x
Nakamura, T., Imai, Y., Matsumoto, T., Sato, S., Takeuchi, K., Igarashi, K., et al. (2007). Estrogen prevents bone loss via estrogen receptor alpha and induction of Fas ligand in osteoclasts. Cells 130, 811–823. doi: 10.1016/j.cell.2007.07.025
Narushima, S., Itoha, K., Miyamoto, Y., Park, S. H., Nagata, K., Kuruma, K., et al. (2006). Deoxycholic acid formation in gnotobiotic mice associated with human intestinal bacteria. Lipids 41, 835–843. doi: 10.1007/s11745-006-5038-1
Narva, M., Nevala, R., Poussa, T., and Korpela, R. (2004). The effect of Lactobacillus helveticus fermented milk on acute changes in calcium metabolism in postmenopausal women. Eur. J. Nutr. 43, 61–68. doi: 10.1007/s00394-004-0441-y
Nauck, M. A., and Meier, J. J. (2018). Incretin hormones: Their role in health and disease. Diabetes Obes. Metab. 20, 5–21. doi: 10.1111/dom.13129
Ni, J., Wu, G. D., Albenberg, L., and Tomov, V. T. (2017). Gut microbiota and IBD: Causation or correlation? Nat. Rev. Gastroenterol. Hepatol. 14, 573–584. doi: 10.1038/nrgastro.2017.88
Nicholson, J. K., Holmes, E., Kinross, J., Burcelin, R., Gibson, G., Jia, W., et al. (2012). Host-gut microbiota metabolic interactions. Science 336, 1262–1267. doi: 10.1126/science.1223813
Nishida, S., Yamaguchi, A., Tanizawa, T., Endo, N., Mashiba, T., Uchiyama, Y., et al. (1994). Increased bone formation by intermittent parathyroid hormone administration is due to the stimulation of proliferation and differentiation of osteoprogenitor cells in bone marrow. Bone 15, 717–723. doi: 10.1016/8756-3282(94)90322-0
Nissen, A., Christensen, M., Knop, F. K., Vilsbøll, T., Holst, J. J., and Hartmann, B. (2014). Glucose-dependent insulinotropic polypeptide inhibits bone resorption in humans. J. Clin. Endocrinol. Metab. 99, E2325–E2329. doi: 10.1210/jc.2014-2547
Novince, C. M., Whittow, C. R., Aartun, J. D., Hathaway, J. D., Poulides, N., Chavez, M. B., et al. (2017). Commensal gut microbiota immunomodulatory actions in bone marrow and liver have catabolic effects on skeletal homeostasis in health. Sci. Rep. 7:5747. doi: 10.1038/s41598-017-06126-x
Nuche-Berenguer, B., Lozano, D., Gutiérrez-Rojas, I., Moreno, P., Mariñoso, M. L., Esbrit, P., et al. (2011). GLP-1 and exendin-4 can reverse hyperlipidic-related osteopenia. J. Endocrinol. 209, 203–210. doi: 10.1530/joe-11-0015
Nuche-Berenguer, B., Moreno, P., Esbrit, P., Dapía, S., Caeiro, J. R., Cancelas, J., et al. (2009). Effect of GLP-1 treatment on bone turnover in normal, type 2 diabetic, and insulin-resistant states. Calcif. Tissue Int. 84, 453–461. doi: 10.1007/s00223-009-9220-3
Pacheco-Pantoja, E. L., Ranganath, L. R., Gallagher, J. A., Wilson, P. J., and Fraser, W. D. (2011). Receptors and effects of gut hormones in three osteoblastic cell lines. BMC Physiol. 11:12. doi: 10.1186/1472-6793-11-12
Palermo, A., Naciu, A. M., Tabacco, G., Falcone, S., Santonati, A., Maggi, D., et al. (2020). Clinical, biochemical, and radiological profile of normocalcemic primary hyperparathyroidism. J. Clin. Endocrinol. Metab. 105, e2609–e2616. doi: 10.1210/clinem/dgaa174
Park, S., Kim, D. S., Kang, E. S., Kim, D. B., and Kang, S. (2018). Low-dose brain estrogen prevents menopausal syndrome while maintaining the diversity of the gut microbiomes in estrogen-deficient rats. Am. J. Physiol. Endocrinol. Metab. 315, E99–e109. doi: 10.1152/ajpendo.00005.2018
Pathak, P., Xie, C., Nichols, R. G., Ferrell, J. M., Boehme, S., Krausz, K. W., et al. (2018). Intestine farnesoid X receptor agonist and the gut microbiota activate G-protein bile acid receptor-1 signaling to improve metabolism. Hepatology 68, 1574–1588. doi: 10.1002/hep.29857
Pazianas, M., and Miller, P. D. (2021). Osteoporosis and chronic kidney disease-mineral and bone disorder (CKD-MBD): Back to basics. Am. J. Kidney Dis. 78, 582–589. doi: 10.1053/j.ajkd.2020.12.024
Perelló, M., and Zigman, J. M. (2012). The role of ghrelin in reward-based eating. Biol. Psychiatry 72, 347–353. doi: 10.1016/j.biopsych.2012.02.016
Perry, R. J., Peng, L., Barry, N. A., Cline, G. W., Zhang, D., Cardone, R. L., et al. (2016). Acetate mediates a microbiome-brain-β-cell axis to promote metabolic syndrome. Nature 534, 213–217. doi: 10.1038/nature18309
Petersen, N., Reimann, F., Bartfeld, S., Farin, H. F., Ringnalda, F. C., Vries, R. G., et al. (2014). Generation of L cells in mouse and human small intestine organoids. Diabetes 63, 410–420. doi: 10.2337/db13-0991
Pierreux, J., and Bravenboer, B. (2018). Normocalcemic primary hyperparathyroidism: A comparison with the hypercalcemic form in a tertiary referral population. Horm. Metab. Res. 50, 797–802. doi: 10.1055/a-0752-4533
Pineiro, M., and Stanton, C. (2007). Probiotic bacteria: Legislative framework— requirements to evidence basis. J. Nutr. 137, 850S–853S. doi: 10.1093/jn/137.3.850S
Queipo-Ortuño, M. I., Seoane, L. M., Murri, M., Pardo, M., Gomez-Zumaquero, J. M., Cardona, F., et al. (2013). Gut microbiota composition in male rat models under different nutritional status and physical activity and its association with serum leptin and ghrelin levels. PLoS One 8:e65465. doi: 10.1371/journal.pone.0065465
Rahman, M. M., Kukita, A., Kukita, T., Shobuike, T., Nakamura, T., and Kohashi, O. (2003). Two histone deacetylase inhibitors, trichostatin A and sodium butyrate, suppress differentiation into osteoclasts but not into macrophages. Blood 101, 3451–3459. doi: 10.1182/blood-2002-08-2622
Rajpal, D. K., Klein, J. L., Mayhew, D., Boucheron, J., Spivak, A. T., Kumar, V., et al. (2015). Selective spectrum antibiotic modulation of the gut microbiome in obesity and diabetes rodent models. PLoS One 10:e0145499. doi: 10.1371/journal.pone.0145499
Reseland, J. E., Syversen, U., Bakke, I., Qvigstad, G., Eide, L. G., Hjertner, O., et al. (2001). Leptin is expressed in and secreted from primary cultures of human osteoblasts and promotes bone mineralization. J. Bone Miner. Res. 16, 1426–1433. doi: 10.1359/jbmr.2001.16.8.1426
Rosen, C. J., Ackert-Bicknell, C. L., Adamo, M. L., Shultz, K. L., Rubin, J., Donahue, L. R., et al. (2004). Congenic mice with low serum IGF-I have increased body fat, reduced bone mineral density, and an altered osteoblast differentiation program. Bone 35, 1046–1058. doi: 10.1016/j.bone.2004.07.008
Russell, D. W. (2003). The enzymes, regulation, and genetics of bile acid synthesis. Annu. Rev. Biochem. 72, 137–174. doi: 10.1146/annurev.biochem.72.121801.161712
Samuel, B. S., Shaito, A., Motoike, T., Rey, F. E., Backhed, F., Manchester, J. K., et al. (2008). Effects of the gut microbiota on host adiposity are modulated by the short-chain fatty-acid binding G protein-coupled receptor, Gpr41. Proc. Natl. Acad. Sci. U. S. A. 105, 16767–16772. doi: 10.1073/pnas.0808567105
Scheid, J. L., Toombs, R. J., Ducher, G., Gibbs, J. C., Williams, N. I., and De Souza, M. J. (2011). Estrogen and peptide YY are associated with bone mineral density in premenopausal exercising women. Bone 49, 194–201. doi: 10.1016/j.bone.2011.04.011
Scholz-Ahrens, K. E., Ade, P., Marten, B., Weber, P., Timm, W., Açil, Y., et al. (2007). Prebiotics, probiotics, and synbiotics affect mineral absorption, bone mineral content, and bone structure. J. Nutr. 137, 838S–846S. doi: 10.1093/jn/137.3.838S
Schwarzer, M., Makki, K., Storelli, G., Machuca-Gayet, I., Srutkova, D., Hermanova, P., et al. (2016). Lactobacillus plantarum strain maintains growth of infant mice during chronic undernutrition. Science 351, 854–857. doi: 10.1126/science.aad8588
Seijo, M., Bryk, G., Zeni Coronel, M., Bonanno, M., Río, M. E., Martín, P., et al. (2019). Effect of adding a galacto-oligosaccharides/fructo-oligosaccharides (GOS/FOS®) mixture to a normal and low calcium diet, on calcium absorption and bone health in ovariectomy-induced osteopenic rats. Calcif. Tissue Int. 104, 301–312. doi: 10.1007/s00223-018-0490-5
Sheveleva, S. A. (1999). Probiotics, prebiotics and probiotic products. Current status. Vopr. Pitan. 68, 32–40.
Shin, S. C., Kim, S. H., You, H., Kim, B., Kim, A. C., Lee, K. A., et al. (2011). Drosophila microbiome modulates host developmental and metabolic homeostasis via insulin signaling. Science 334, 670–674. doi: 10.1126/science.1212782
Shirouchi, B., Nagao, K., Umegatani, M., Shiraishi, A., Morita, Y., Kai, S., et al. (2016). Probiotic Lactobacillus gasseri SBT2055 improves glucose tolerance and reduces body weight gain in rats by stimulating energy expenditure. Br. J. Nutr. 116, 451–458. doi: 10.1017/s0007114516002245
Silva, B. C., Costa, A. G., Cusano, N. E., Kousteni, S., and Bilezikian, J. P. (2011). Catabolic and anabolic actions of parathyroid hormone on the skeleton. J. Endocrinol. Investig. 34, 801–810. doi: 10.3275/7925
Sinal, C. J., Tohkin, M., Miyata, M., Ward, J. M., Lambert, G., and Gonzalez, F. J. (2000). Targeted disruption of the nuclear receptor FXR/BAR impairs bile acid and lipid homeostasis. Cells 102, 731–744. doi: 10.1016/s0092-8674(00)00062-3
Sjögren, K., Engdahl, C., Henning, P., Lerner, U. H., Tremaroli, V., Lagerquist, M. K., et al. (2012). The gut microbiota regulates bone mass in mice. J. Bone Miner. Res. 27, 1357–1367. doi: 10.1002/jbmr.1588
Smith, P. M., Howitt, M. R., Panikov, N., Michaud, M., Gallini, C. A., Bohlooly, Y. M., et al. (2013). The microbial metabolites, short-chain fatty acids, regulate colonic Treg cell homeostasis. Science 341, 569–573. doi: 10.1126/science.1241165
Soumeh, E. A., Mohebodini, H., Toghyani, M., Shabani, A., Ashayerizadeh, A., and Jazi, V. (2019). Synergistic effects of fermented soybean meal and mannan-oligosaccharide on growth performance, digestive functions, and hepatic gene expression in broiler chickens. Poult. Sci. 98, 6797–6807. doi: 10.3382/ps/pez409
Spohn, S. N., and Mawe, G. M. (2017). Non-conventional features of peripheral serotonin signalling - the gut and beyond. Nat. Rev. Gastroenterol. Hepatol. 14, 412–420. doi: 10.1038/nrgastro.2017.51
Stensen, S., Gasbjerg, L. S., Helsted, M. M., Hartmann, B., Christensen, M. B., and Knop, F. K. (2020). GIP and the gut-bone axis - Physiological, pathophysiological and potential therapeutic implications. Peptides 125:170197. doi: 10.1016/j.peptides.2019.170197
Stewart, C. E., and Rotwein, P. (1996). Growth, differentiation, and survival: Multiple physiological functions for insulin-like growth factors. Physiol. Rev. 76, 1005–1026. doi: 10.1152/physrev.1996.76.4.1005
Storelli, G., Defaye, A., Erkosar, B., Hols, P., Royet, J., and Leulier, F. (2011). Lactobacillus plantarum promotes Drosophila systemic growth by modulating hormonal signals through TOR-dependent nutrient sensing. Cell Metab. 14, 403–414. doi: 10.1016/j.cmet.2011.07.012
Sugisawa, E., Takayama, Y., Takemura, N., Kondo, T., Hatakeyama, S., Kumagai, Y., et al. (2020). RNA sensing by gut piezo1 is essential for systemic serotonin synthesis. Cells 182, 609–624.e21. doi: 10.1016/j.cell.2020.06.022
Takasugi, S., Ashida, K., Maruyama, S., Komaba, Y., Kaneko, T., and Yamaji, T. (2011). A dairy product fermented by lactobacilli cancels the adverse effects of hypochlorhydria induced by a proton pump inhibitor on bone metabolism in growing rats. Br. J. Nutr. 106, 1487–1494. doi: 10.1017/s0007114511002017
Tang, W. H., Kitai, T., and Hazen, S. L. (2017). Gut microbiota in cardiovascular health and disease. Circ. Res. 120, 1183–1196. doi: 10.1161/circresaha.117.309715
Tannenbaum, G. S., Gurd, W., and Lapointe, M. (1998). Leptin is a potent stimulator of spontaneous pulsatile growth hormone (GH) secretion and the GH response to GH-releasing hormone. Endocrinology 139, 3871–3875. doi: 10.1210/endo.139.9.6206
Tazoe, H., Otomo, Y., Karaki, S., Kato, I., Fukami, Y., Terasaki, M., et al. (2009). Expression of short-chain fatty acid receptor GPR41 in the human colon. Biomed. Res. 30, 149–156. doi: 10.2220/biomedres.30.149
Teitelbaum, S. L. (2000). Bone resorption by osteoclasts. Science 289, 1504–1508. doi: 10.1126/science.289.5484.1504
Terauchi, M., Li, J. Y., Bedi, B., Baek, K. H., Tawfeek, H., Galley, S., et al. (2009). T lymphocytes amplify the anabolic activity of parathyroid hormone through Wnt10b signaling. Cell Metab. 10, 229–240. doi: 10.1016/j.cmet.2009.07.010
Thomas, T., Gori, F., Khosla, S., Jensen, M. D., Burguera, B., and Riggs, B. L. (1999). Leptin acts on human marrow stromal cells to enhance differentiation to osteoblasts and to inhibit differentiation to adipocytes. Endocrinology 140, 1630–1638. doi: 10.1210/endo.140.4.6637
Tolhurst, G., Heffron, H., Lam, Y. S., Parker, H. E., Habib, A. M., Diakogiannaki, E., et al. (2012). Short-chain fatty acids stimulate glucagon-like peptide-1 secretion via the G-protein-coupled receptor FFAR2. Diabetes 61, 364–371. doi: 10.2337/db11-1019
Torres-Fuentes, C., Golubeva, A. V., Zhdanov, A. V., Wallace, S., Arboleya, S., Papkovsky, D. B., et al. (2019). Short-chain fatty acids and microbiota metabolites attenuate ghrelin receptor signaling. FASEB J. 33, 13546–13559. doi: 10.1096/fj.201901433R
Tu, Y., Yang, R., Xu, X., and Zhou, X. (2021). The microbiota-gut-bone axis and bone health. J. Leukoc. Biol. 110, 525–537. doi: 10.1002/jlb.3mr0321-755r
Tu, Q., Zhang, J., Dong, L. Q., Saunders, E., Luo, E., Tang, J., et al. (2011). Adiponectin inhibits osteoclastogenesis and bone resorption via APPL1-mediated suppression of Akt1. J. Biol. Chem. 286, 12542–12553. doi: 10.1074/jbc.M110.152405
Utz, A. L., Lawson, E. A., Misra, M., Mickley, D., Gleysteen, S., Herzog, D. B., et al. (2008). Peptide YY (PYY) levels and bone mineral density (BMD) in women with anorexia nervosa. Bone 43, 135–139. doi: 10.1016/j.bone.2008.03.007
van Coeverden, S. C., Netelenbos, J. C., de Ridder, C. M., Roos, J. C., Popp-Snijders, C., and Delemarre-van de Waal, H. A. (2002). Bone metabolism markers and bone mass in healthy pubertal boys and girls. Clin. Endocrinol. 57, 107–116. doi: 10.1046/j.1365-2265.2002.01573.x
Van Wyk, J. J., and Smith, E. P. (1999). Insulin-like growth factors and skeletal growth: Possibilities for therapeutic interventions. J. Clin. Endocrinol. Metab. 84, 4349–4354. doi: 10.1210/jcem.84.12.6201
Viswanathan, V. K. (2013). Sensing bacteria, without bitterness? Gut Microbes 4, 91–93. doi: 10.4161/gmic.23776
Wallimann, A., Magrath, W., Thompson, K., Moriarty, T., Richards, R. G., Akdis, C. A., et al. (2021). Gut microbial-derived short-chain fatty acids and bone: A potential role in fracture healing. Eur. Cell. Mater. 41, 454–470. doi: 10.22203/eCM.v041a29
Wang, Y., Bikle, D. D., and Chang, W. (2013). Autocrine and paracrine actions of IGF-I signaling in skeletal development. Bone Res. 1, 249–259. doi: 10.4248/br201303003
Wang, Z., Chen, K., Wu, C., Chen, J., Pan, H., Liu, Y., et al. (2021). An emerging role of Prevotella histicola on estrogen deficiency-induced bone loss through the gut microbiota-bone axis in postmenopausal women and in ovariectomized mice. Am. J. Clin. Nutr. 114, 1304–1313. doi: 10.1093/ajcn/nqab194
Wang, Y., Dilidaxi, D., Wu, Y., Sailike, J., Sun, X., and Nabi, X. H. (2020). Composite probiotics alleviate type 2 diabetes by regulating intestinal microbiota and inducing GLP-1 secretion in db/db mice. Biomed. Pharmacother. 125:109914. doi: 10.1016/j.biopha.2020.109914
Wang, J. W., Kuo, C. H., Kuo, F. C., Wang, Y. K., Hsu, W. H., Yu, F. J., et al. (2019). Fecal microbiota transplantation: Review and update. J. Formos. Med. Assoc. 118, S23–S31. doi: 10.1016/j.jfma.2018.08.011
Wang, Y., Nishida, S., Elalieh, H. Z., Long, R. K., Halloran, B. P., and Bikle, D. D. (2006). Role of IGF-I signaling in regulating osteoclastogenesis. J. Bone Miner. Res. 21, 1350–1358. doi: 10.1359/jbmr.060610
Wang, J. K., and Yao, S. K. (2021). Roles of gut microbiota and metabolites in pathogenesis of functional constipation. Evid. Based Complement. Alternat. Med. 2021, 5560310–5560312. doi: 10.1155/2021/5560310
Wang, Z., and Zhao, Y. (2018). Gut microbiota derived metabolites in cardiovascular health and disease. Protein Cell 9, 416–431. doi: 10.1007/s13238-018-0549-0
Weger, B. D., Gobet, C., Yeung, J., Martin, E., Jimenez, S., Betrisey, B., et al. (2019). The mouse microbiome is required for sex-specific diurnal rhythms of gene expression and metabolism. Cell Metab. 29, 362–82.e8. doi: 10.1016/j.cmet.2018.09.023
Wei, M., Li, C., Dai, Y., Zhou, H., Cui, Y., Zeng, Y., et al. (2021). High-throughput absolute quantification sequencing revealed osteoporosis-related gut microbiota alterations in Han Chinese elderly. Front. Cell. Infect. Microbiol. 11:630372. doi: 10.3389/fcimb.2021.630372
Wein, M. N., and Kronenberg, H. M. (2018). Regulation of bone remodeling by parathyroid hormone. Cold Spring Harb. Perspect. Med. 8:a031237. doi: 10.1101/cshperspect.a031237
Wichmann, A., Allahyar, A., Greiner, T. U., Plovier, H., Lundén, G., Larsson, T., et al. (2013). Microbial modulation of energy availability in the colon regulates intestinal transit. Cell Host Microbe 14, 582–590. doi: 10.1016/j.chom.2013.09.012
Wikoff, W. R., Anfora, A. T., Liu, J., Schultz, P. G., Lesley, S. A., Peters, E. C., et al. (2009). Metabolomics analysis reveals large effects of gut microflora on mammalian blood metabolites. Proc. Natl. Acad. Sci. U. S. A. 106, 3698–3703. doi: 10.1073/pnas.0812874106
Wiren, K. M., Toombs, A. R., Semirale, A. A., and Zhang, X. (2006). Osteoblast and osteocyte apoptosis associated with androgen action in bone: Requirement of increased Bax/Bcl-2 ratio. Bone 38, 637–651. doi: 10.1016/j.bone.2005.10.029
Wommack, K. E., and Colwell, R. R. (2000). Virioplankton: Viruses in aquatic ecosystems. Microbiol. Mol. Biol. Rev. 64, 69–114. doi: 10.1128/mmbr.64.1.69-114.2000
Wong, S. C., Dobie, R., Altowati, M. A., Werther, G. A., Farquharson, C., and Ahmed, S. F. (2016). Growth and the growth hormone-insulin like growth factor 1 axis in children with chronic inflammation: Current evidence, gaps in knowledge, and future directions. Endocr. Rev. 37, 62–110. doi: 10.1210/er.2015-1026
Wong, I. P., Driessler, F., Khor, E. C., Shi, Y. C., Hörmer, B., Nguyen, A. D., et al. (2012). Peptide YY regulates bone remodeling in mice: A link between gut and skeletal biology. PLoS One 7:e40038. doi: 10.1371/journal.pone.0040038
Wortley, K. E., Garcia, K., Okamoto, H., Thabet, K., Anderson, K. D., Shen, V., et al. (2007). Peptide YY regulates bone turnover in rodents. Gastroenterology 133, 1534–1543. doi: 10.1053/j.gastro.2007.08.024
Wu, J. Z., Liu, P. C., Liu, R., and Cai, M. (2018). Icariin restores bone structure and strength in a rat model of chronic high-dose alcohol-induced osteopenia. Cell. Physiol. Biochem. 46, 1727–1736. doi: 10.1159/000489248
Xiao, L., Zhou, Y., Bokoliya, S., Lin, Q., and Hurley, M. (2022). Bone loss is ameliorated by fecal microbiota transplantation through SCFA/GPR41/ IGF1 pathway in sickle cell disease mice. Sci. Rep. 12:20638. doi: 10.1038/s41598-022-25244-9
Xie, D., Cheng, H., Hamrick, M., Zhong, Q., Ding, K. H., Correa, D., et al. (2005). Glucose-dependent insulinotropic polypeptide receptor knockout mice have altered bone turnover. Bone 37, 759–769. doi: 10.1016/j.bone.2005.06.021
Xie, Q., Shen, W. W., Zhong, J., Huang, C., Zhang, L., and Li, J. (2014). Lipopolysaccharide/adenosine triphosphate induces IL-1β and IL-18 secretion through the NLRP3 inflammasome in RAW264.7 murine macrophage cells. Int. J. Mol. Med. 34, 341–349. doi: 10.3892/ijmm.2014.1755
Yadav, V. K., Balaji, S., Suresh, P. S., Liu, X. S., Lu, X., Li, Z., et al. (2010). Pharmacological inhibition of gut-derived serotonin synthesis is a potential bone anabolic treatment for osteoporosis. Nat. Med. 16, 308–312. doi: 10.1038/nm.2098
Yadav, V. K., Oury, F., Suda, N., Liu, Z. W., Gao, X. B., Confavreux, C., et al. (2009). A serotonin-dependent mechanism explains the leptin regulation of bone mass, appetite, and energy expenditure. Cells 138, 976–989. doi: 10.1016/j.cell.2009.06.051
Yadav, V. K., Ryu, J. H., Suda, N., Tanaka, K. F., Gingrich, J. A., Schütz, G., et al. (2008). Lrp5 controls bone formation by inhibiting serotonin synthesis in the duodenum. Cells 135, 825–837. doi: 10.1016/j.cell.2008.09.059
Yamada, C., Yamada, Y., Tsukiyama, K., Yamada, K., Udagawa, N., Takahashi, N., et al. (2008). The murine glucagon-like peptide-1 receptor is essential for control of bone resorption. Endocrinology 149, 574–579. doi: 10.1210/en.2007-1292
Yan, J., Herzog, J. W., Tsang, K., Brennan, C. A., Bower, M. A., Garrett, W. S., et al. (2016). Gut microbiota induce IGF-1 and promote bone formation and growth. Proc. Natl. Acad. Sci. U. S. A. 113, E7554–E7563. doi: 10.1073/pnas.1607235113
Yan, J., Takakura, A., Zandi-Nejad, K., and Charles, J. F. (2018). Mechanisms of gut microbiota-mediated bone remodeling. Gut Microbes 9, 84–92. doi: 10.1080/19490976.2017.1371893
Yan, F. F., Wang, W. C., and Cheng, H. W. (2018). Bacillus subtilis based probiotic improved bone mass and altered brain serotoninergic and dopaminergic systems in broiler chickens. J. Funct. Foods 49, 501–509. doi: 10.1016/j.jff.2018.09.017
Yang, X., Chang, T., Yuan, Q., Wei, W., Wang, P., Song, X., et al. (2022). Changes in the composition of gut and vaginal microbiota in patients with postmenopausal osteoporosis. Front. Immunol. 13:930244. doi: 10.3389/fimmu.2022.930244
Yano, J. M., Yu, K., Donaldson, G. P., Shastri, G. G., Ann, P., Ma, L., et al. (2015). Indigenous bacteria from the gut microbiota regulate host serotonin biosynthesis. Cells 161, 264–276. doi: 10.1016/j.cell.2015.02.047
Ye, L., Bae, M., Cassilly, C. D., Jabba, S. V., Thorpe, D. W., Martin, A. M., et al. (2021). Enteroendocrine cells sense bacterial tryptophan catabolites to activate enteric and vagal neuronal pathways. Cell Host Microbe 29, 179–96.e9. doi: 10.1016/j.chom.2020.11.011
Yilmaz, C., Colak, M., Yilmaz, B. C., Ersoz, G., Kutateladze, M., and Gozlugol, M. (2013). Bacteriophage therapy in implant-related infections: An experimental study. J. Bone Joint Surg. Am. 95, 117–125. doi: 10.2106/jbjs.K.01135
Yu, D., Chen, W., Zhang, J., Wei, L., Qin, J., Lei, M., et al. (2022). Effects of weight loss on bone turnover, inflammatory cytokines, and adipokines in Chinese overweight and obese adults. J. Endocrinol. Investig. 45, 1757–1767. doi: 10.1007/s40618-022-01815-5
Yu, M., Malik Tyagi, A., Li, J. Y., Adams, J., Denning, T. L., Weitzmann, M. N., et al. (2020). PTH induces bone loss via microbial-dependent expansion of intestinal TNF(+) T cells and Th17 cells. Nat. Commun. 11:468. doi: 10.1038/s41467-019-14148-4
Yu, E. W., Wewalka, M., Ding, S. A., Simonson, D. C., Foster, K., Holst, J. J., et al. (2016). Effects of gastric bypass and gastric banding on bone remodeling in obese patients with type 2 diabetes. J. Clin. Endocrinol. Metab. 101, 714–722. doi: 10.1210/jc.2015-3437
Zafar, T. A., Weaver, C. M., Zhao, Y., Martin, B. R., and Wastney, M. E. (2004). Nondigestible oligosaccharides increase calcium absorption and suppress bone resorption in ovariectomized rats. J. Nutr. 134, 399–402. doi: 10.1093/jn/134.2.399
Zaibi, M. S., Stocker, C. J., O’Dowd, J., Davies, A., Bellahcene, M., Cawthorne, M. A., et al. (2010). Roles of GPR41 and GPR43 in leptin secretory responses of murine adipocytes to short chain fatty acids. FEBS Lett. 584, 2381–2386. doi: 10.1016/j.febslet.2010.04.027
Zaiss, M. M., Jones, R. M., Schett, G., and Pacifici, R. (2019). The gut-bone axis: How bacterial metabolites bridge the distance. J. Clin. Invest. 129, 3018–3028. doi: 10.1172/jci128521
Zarrinpar, A., Chaix, A., Xu, Z. Z., Chang, M. W., Marotz, C. A., Saghatelian, A., et al. (2018). Antibiotic-induced microbiome depletion alters metabolic homeostasis by affecting gut signaling and colonic metabolism. Nat. Commun. 9:2872. doi: 10.1038/s41467-018-05336-9
Zhang, Y. W., Cao, M. M., Li, Y. J., Lu, P. P., Dai, G. C., Zhang, M., et al. (2022). Fecal microbiota transplantation ameliorates bone loss in mice with ovariectomy-induced osteoporosis via modulating gut microbiota and metabolic function. J. Orthop. Translat. 37, 46–60. doi: 10.1016/j.jot.2022.08.003
Zhang, J. M., Liu, X. Y., Gu, W., Xu, H. Y., Jiao, H. C., Zhao, J. P., et al. (2021). Different effects of probiotics and antibiotics on the composition of microbiota, SCFAs concentrations and FFAR2/3 mRNA expression in broiler chickens. J. Appl. Microbiol. 131, 913–924. doi: 10.1111/jam.14953
Zhang, J., Pi, C., Cui, C., Zhou, Y., Liu, B., Liu, J., et al. (2022). PTHrP promotes subchondral bone formation in TMJ-OA. Int. J. Oral Sci. 14:37. doi: 10.1038/s41368-022-00189-x
Zhao, G., Monier-Faugere, M. C., Langub, M. C., Geng, Z., Nakayama, T., Pike, J. W., et al. (2000). Targeted overexpression of insulin-like growth factor I to osteoblasts of transgenic mice: Increased trabecular bone volume without increased osteoblast proliferation. Endocrinology 141, 2674–2682. doi: 10.1210/endo.141.7.7585
Zhao, Y. X., Song, Y. W., Zhang, L., Zheng, F. J., Wang, X. M., Zhuang, X. H., et al. (2020). Association between bile acid metabolism and bone mineral density in postmenopausal women. Clinics 75:e1486. doi: 10.6061/clinics/2020/e1486
Keywords: gut microbiota, bone metabolism, gut-bone axis, endocrine system, hormone
Citation: Tu Y, Kuang X, Zhang L and Xu X (2023) The associations of gut microbiota, endocrine system and bone metabolism. Front. Microbiol. 14:1124945. doi: 10.3389/fmicb.2023.1124945
Received: 15 December 2022; Accepted: 16 March 2023;
Published: 06 April 2023.
Edited by:
Rupesh K. Srivastava, All India Institute of Medical Sciences, IndiaReviewed by:
Abdul Malik Tyagi, Central Drug Research Institute (CSIR), IndiaCopyright © 2023 Tu, Kuang, Zhang and Xu. This is an open-access article distributed under the terms of the Creative Commons Attribution License (CC BY). The use, distribution or reproduction in other forums is permitted, provided the original author(s) and the copyright owner(s) are credited and that the original publication in this journal is cited, in accordance with accepted academic practice. No use, distribution or reproduction is permitted which does not comply with these terms.
*Correspondence: Ling Zhang, emhhbmdsaW5nMTk4MzA4MjRAMTYzLmNvbQ==; Xin Xu, eGluLnh1QHNjdS5lZHUuY24=
Disclaimer: All claims expressed in this article are solely those of the authors and do not necessarily represent those of their affiliated organizations, or those of the publisher, the editors and the reviewers. Any product that may be evaluated in this article or claim that may be made by its manufacturer is not guaranteed or endorsed by the publisher.
Research integrity at Frontiers
Learn more about the work of our research integrity team to safeguard the quality of each article we publish.