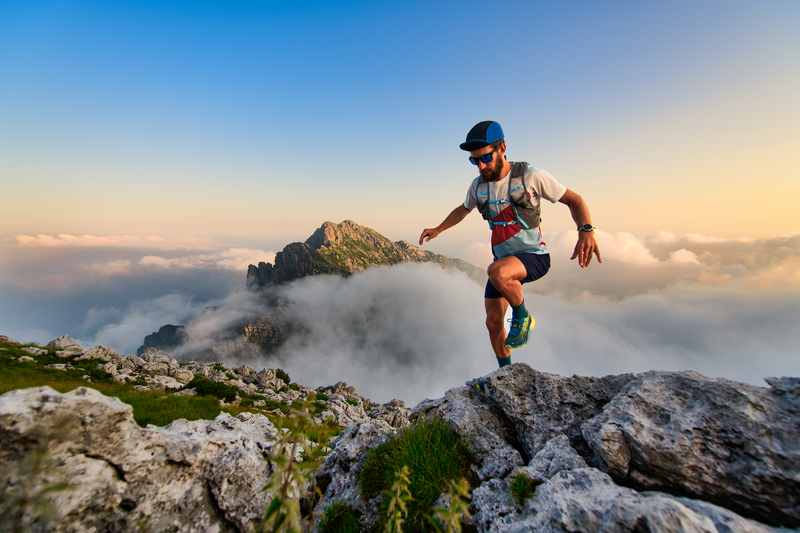
95% of researchers rate our articles as excellent or good
Learn more about the work of our research integrity team to safeguard the quality of each article we publish.
Find out more
ORIGINAL RESEARCH article
Front. Microbiol. , 02 December 2021
Sec. Microbiotechnology
Volume 12 - 2021 | https://doi.org/10.3389/fmicb.2021.780943
This article is part of the Research Topic Microbial Interactions with Nanomaterials in the Environment and their Application View all 6 articles
Honeybees (Apis mellifera) can be exposed via numerous potential pathways to ambient nanoparticles (NPs), including rare earth oxide (REO) NPs that are increasingly used and released into the environment. Gut microorganisms are pivotal in mediating honeybee health, but how REO NPs may affect honeybee health and gut microbiota remains poorly understood. To address this knowledge gap, honeybees were fed pollen and sucrose syrup containing 0, 1, 10, 100, and 1000mgkgā1 of nano-La2O3 for 12days. Nano-La2O3 exerted detrimental effects on honeybee physiology, as reflected by dose-dependent adverse effects of nano-La2O3 on survival, pollen consumption, and body weight (p<0.05). Nano-La2O3 caused the dysbiosis of honeybee gut bacterial communities, as evidenced by the change of gut bacterial community composition, the enrichment of pathogenic Serratia and Frischella, and the alteration of digestion-related taxa Bombella (p<0.05). There were significant correlations between honeybee physiological parameters and the relative abundances of pathogenic Serratia and Frischella (p<0.05), underscoring linkages between honeybee health and gut bacterial communities. Taken together, this study demonstrates that nano-La2O3 can cause detrimental effects on honeybee health, potentially by disordering gut bacterial communities. This study thus reveals a previously overlooked effect of nano-La2O3 on the ecologically and economically important honeybee species Apis mellifera.
Honeybees (e.g., Apis mellifera) provide essential pollination services for agricultural ecosystems and valuable apiary products for human nutritional needs (Klein et al., 2007). Due to their extensive social activities within 14km2 foraging areas, honeybees are exposed to conventional contaminants, for example, pesticides, antibiotics, and respirable suspended particulate matters, that may lead to the decline of honeybee colonies or the deterioration of honeybee health status (BargaÅska et al., 2016; Thimmegowda et al., 2020; Bondarenko et al., 2021; Kapoor et al., 2021). Among the emerging contaminants, rare earth oxide (REO) nanoparticles (NPs), characterized by their unique chemical and physical properties, have been one of most widely used materials in various industries and biotechnology applications (Mastronardi et al., 2015; Gao et al., 2017; Kos et al., 2017). For example, the fertilizers, pesticides, and germination stimulants containing or engineered with REO NPs have been widely used to enhance the efficiency and sustainability of agricultural practices, and nano-La2O3 is reported to account for approximately 30% of REO NPs additives and have higher cytotoxicity compared with other REO NPs (De la Torre Roche et al., 2015; Mastronardi et al., 2015; Servin et al., 2015; Gao et al., 2017). This make honeybees highly susceptible to the exposure and toxicity of REO NPs, through contacting with and ingesting these particles directly or indirectly from the surrounding environments, especially the agricultural ones, such as plant and flower surfaces, pollen and nectar, and soil and dust (Ma et al., 2011; De la Torre Roche et al., 2015; Kos et al., 2017; Radziwill-Bienkowska et al., 2018). Once soil was contaminated by nano-La2O3, plants can serve as a potential intermediary pathway that could bioaccumulate and transport them to primary consumers, for example, Acheta domestica, Tenebrionoidea, and honeybees (Ma et al., 2011; De la Torre Roche et al., 2015). Related studies have also demonstrated that honeybees come in contact with metal oxide NPs (e.g., CeO2, CdO, and PbO) through surface particle adhesion, dust inhalation, foraging on contaminated food, or water (Kos et al., 2017; Al Naggar et al., 2020). Therefore, honeybees may suffer the environmental exposure of terrestrial REO NPs and serve as sensitive indicators of environmental quality. Despite the fact that honeybee gut microorganisms take important roles in maintaining host immunity, metabolism, and health (Kwong and Moran, 2016), it is unknown whether and how REO NPs exposure will cause deterioration of honeybee health and dysbiosis of honeybee gut microbiota and whether the gut dysbiosis will further mediate the toxic effect of environmental contaminants on honeybees health.
Although there are few studies regarding effects of REO NPs on honeybee health, related research implies that metal oxide NPs may adversely affect honeybee health, through mechanisms relating to, for example, signals blocking, reactive oxygen species (ROS), and cell damage (Nel et al., 2006; Benelli, 2018). For example, the mortality rate of Apis mellifera increased with exposure concentrations of REO NPs (nano-TiO2, nano-ZnO-TiO2, and nano-Ag-TiO2), implying a dose-dependent toxic effect of metal oxide NPs on honeybee (Dabour et al., 2019). Also, exposure to nano-CeO2 causes undesirable neurological effects on honeybee Apis mellifera, by inhibiting the activity of membrane acetylcholinesterase (AChE) which further influences cholinergic function of the nervous system (Kos et al., 2017). Also, nano-CdO and nano-PbO can enhance ROS production and thus cause free radical-induced oxidative damage to honeybee Apis millefera, accompanied by anti-oxidative responses, for example, increased catalase production (Al Naggar et al., 2020).
REO NPs may also disturb honeybee gut microbiota. Previous studies have shown the undesirable effects of various types of NPs on the soil microbiomes and the gut microbiota of animals and insects (Han et al., 2014; Ge et al., 2016; Zhu et al., 2018; Chen et al., 2021). For instance, nano-ZnO and carbonaceous nanoparticles disturb the soil bacterial community structure and change functionally important microbial groups associated with C, N, and S cycling (Ge et al., 2018; Xu et al., 2018a; Wu et al., 2019). Silver NPs alter the gut bacterial communities of Drosophila and Collembola (Han et al., 2014; Zhu et al., 2018). But, it is still unclear whether, and how, REO NPs affect gut microbiota of honeybees, specifically the model species Apis mellifera. Honeybee gut microbiota could be influenced by various factors, including pathogens, antibiotics, pesticides, diet, and host attributes and environmental conditions (Cox-Foster et al., 2007; Raymann et al., 2017; Liu et al., 2019; Ge et al., 2021). Previous study has demonstrated that the shifts of gut bacterial communities in bumblebees may serve as a characteristic of disease states, featuring as low abundance of core species and high incidence of opportunistic environmental bacteria (Cariveau et al., 2014). Given the significant roles of honeybee gut microbiota in maintaining host health and fitness (Kwong and Moran, 2016; Zhang et al., 2020) and the likelihood of honeybee exposure to REOs via ingestion, it is imperative to understand the ecological effects of REO NPs on honeybee gut microbiota to guide the safe design and application of REO NPs.
In this study, the aims were to (1) investigate the toxicity of REO NPs on honeybee health and gut bacterial communities and (2) explore the relationship between REO NP exposure, gut bacterial communities, and host responses. The working hypotheses were that REO NPs would, in a dose-dependent fashion, disturb honeybee gut microbiota and also directly impact honeybee physiology. A further hypothesis was that the overall effects of REO NPs across physiology and gut microbial effects would be additive. To test these hypotheses, honeybees were fed food amended with different concentrations of nano-La2O3. Here, nano-La2O3 was used as a representative REO NP because of its multifunctionality and high cytotoxicity (De la Torre Roche et al., 2015; Gao et al., 2017). Honeybee survival, pollen consumption, and body weight were quantified, and the composition of the honeybee gut bacterial community was investigated. This is the first study to analyze the effects of nano-La2O3 on honeybees and their gut microbiota, and the results contribute new knowledge regarding the environmental risks of REO NPs.
Honeybees (Apis mellifera) used for nano-La2O3 exposure experiment were incubated at the Institute of Apicultural Research apiary, Chinese Academy of Agricultural Science1 following standard protocols (Liu et al., 2019). Briefly, brood frames of a single hive with capped honeybee pupae were placed in an RXZ-380C climate-controlled incubator (Ningbo, China; 34±1°C, 60±10% relative humidity, in darkness) for up to 12h to obtain honeybee specimens. The newly emerged honeybees (less than 12h old) were randomly divided into five rearing cages with 120 honeybees per cage and further incubated for 1week (30±1°C, 45±5% relative humidity, in darkness) by feeding fresh pollen, sterile sucrose solution (50% wt/wt), and deionized water ad libitum to initiate microbial colonization in the gut (Ellegaard and Engel, 2019). After one-week pre-incubation, the adult honeybees were exposed to nano-La2O3 NPs (Day 0).
Nano-La2O3 (25±5nm) was obtained from the University of California Center for the Environmental Implications of Nanotechnology and characterized in a previous study (Qi et al., 2019). Briefly, the nano-La2O3 studied was of >99.99% purity and composed of spherical particles that aggregated in deionized water (pH=6.8) to 589±16nm. The zeta potential of this nano-La2O3 was previously determined to be 9±1mV in deionized water (pH=6.8), and the dissolution extent was determined to be 14% after incubating (24h at 37°C) in an acidic aqueous solution (HCl, 50μgmLā1, pH=4.5; Li et al., 2014).
Fresh pollen grains were collected from Camellia sinensis, freeze-dried under vacuum in a lyophilizer (Songyuan Huaxing, Beijing, China), and ground into a fine powder using a mortar and pestle. To obtain a homogeneous NP distribution, nano-La2O3 powder was thoroughly mixed with the ground pollen with a handheld kitchen mixer for 10min, diluted to a series of concentrations (2.5, 25, 250, and 2500mgkgā1 pollen) using a 10-fold dilution method (Ge et al., 2018), and then stored separately at ā20°C for daily use. Before daily dietary exposure, the mixture of pollen and nano-La2O3 was dispersed (1:1.5 weight ratio) into an aqueous sucrose solution (50% wt/wt in sterile water) to promote ingestion by honeybees (Jack et al., 2016). Therefore, the final target exposure doses of nano-La2O3 in the mixed pollen and sucrose syrup were 1, 10, 100, and 1,000mgkgā1. Negative exposure control was also conducted by treating honeybees with the mixture of pollen (without nano-La2O3) and sucrose solution (1:1.5 weight ratio).
The exposure doses were chosen to represent several scenarios of dietary exposure: possible environmental concentrations (low or medium concentrations), predicted REO NP environmental hotspots (high concentrations), and potential scenarios (the highest concentrations) based on previous studies (Wen et al., 2001; Tyler, 2004; Gottschalk et al., 2009) and some assumptions. Previous studies reported the concentrations of La in plants (0.004ā40mgkgā1; Wen et al., 2001; Tyler, 2004), surface soils (5.5ā44mgkgā1; Tyler, 2004), and sediments (5ā321mgkgā1; Tyler, 2004; Xu et al., 2018b); and the proportion of oxidation state of La was 35ā70% (Wen et al., 2001). Also, La compounds tend to be colloid or nanoclusters (< 200nm) in environmental matrices (Ma et al., 2011; Kulaksız and Bau, 2013), and approximate 1ā30% nanoparticles can be isolated from bulk soil (Theng and Yuan, 2008). We thus assumed that 1ā30% of the La2O3 in environmental matrices was nano-La2O3. Based on this assumption, the estimated concentrations of nano-La2O3 were calculated as 0.00002ā10mgkgā1 in plants, 0.02ā11mgkgā1 in surface soils, and 0.02ā79mgkgā1 in sediments. Therefore, the low and medium concentrations of nano-La2O3 (1 and 10mgkgā1) used in this study were comparable to the estimated concentrations of nano-La2O3 in environmental matrices. Considering that NP distributions in terrestrial environments may be highly heterogeneous such that very high concentrations may occur in localized areas (Gottschalk et al., 2009). The highest dose was also regarded as simulating an extreme endmember concentration within ranges of reported simulated or measured metal oxide NP concentrations in terrestrial environments (Holden et al., 2014). Using the highest concentration here of 1,000mgkgā1 also allows for examining future potential scenarios of NP environmental buildup, as a situation being previously considered (Priester et al., 2013; De la Torre Roche et al., 2015).
To examine the impacts of nano-La2O3 ingestion, adult honeybees in five rearing cages in climate-controlled incubator (Ningbo Jiangnan, Ningbo, China; 30±1°C with 45±5% relative humidity, in darkness) were orally exposed to different concentrations of nano-La2O3 (0, 1, 10, 100, and 1,000mgkgā1) for up to 12days (Liu et al., 2019). Each cage contained three sterile Petri dish feeders: one feeder containing 4g of a freshly prepared pollen and sterile sucrose syrup mixture containing 0, 1, 10, 100, and 1,000mgkgā1 nano-La2O3 for dietary exposure; another feeder containing 20g sucrose solution (50% wt/wt in sterile water) to minimize the indirect effect of insufficient feeding; and the third feeder containing 30ml deionized water (Di Pasquale et al., 2013). The amounts provided were more than sufficient for dietary and water needs and were replaced daily with equal amounts during the exposure experiment. A control cage that contained dietary provisions and water, but no honeybees, was also conducted in the incubator simultaneously to measure water evaporation.
To assess the naturally environmental exposure of honeybees to La, 3 wild honeybees were randomly collected using a sweep net in the field within Beijing Botanical Garden where the Institute of Apicultural Research is located. To measure the La residue in honeybees under controlled laboratory conditions, 3 honeybees of each treatment were sacrificed after 12days exposure, with each honeybee serving as a biological replicate. Each honeybee was washed and frozen in liquid nitrogen. For each honeybee, the whole body was added with 5ml concentrated nitric acid (HNO3) and 1ml hydrogen peroxide (H2O2, 30% vt/vt) in a 56ml digestion vessel (ZariÄ et al., 2016). The digestion was conducted using a microwave digestion system (Anton Paar GmbH, Graz, Austria) based on the following scheme: 15min from room temperature to 200°C, 15min at 200°C, and cooling down to the room temperature (ZariÄ et al., 2016). The digestion solution was diluted to 10ml with deionized water in volumetric flasks and analyzed with an inductively coupled plasma optical emission spectrometer (PerkinElmer, Palo Alto, CA, United States).
A live census was taken daily, by counting the number of mobile honeybees in each cage, to assess survivorship; any dead honeybees were removed after the census daily. The total mixture consumption of nano-La2O3 was calculated by subtracting the mass of the remaining mixture and water evaporation from the initial 4g mixture supplied daily. The 40% mass of total mixture consumption was divided by the number of surviving honeybees at each 24-h interval in each cage to calculate the pollen consumption per honeybee per day. Further, to determine the mass of the whole honeybee body, 12 live honeybees were randomly sampled from each cage and randomly separated into three groups of 4 honeybees, transferring into individual 50-ml sterile centrifuge tubes and weighed by the subtraction method with a digital balance (Mettler-Toledo, Shanghai, China; ± 0.001g).
The entire exposure test lasted for 12days. After 6 and 12days, five live honeybees as individual replicates were sampled randomly from each cage and cold-anesthetized (ā20°C, 1min). All immobilized honeybees were dissected on ice to collect the entire gut with flame-sterilized forceps under aseptic conditions. Each gut sample was placed into a 2-ml sterile centrifuge tube and immediately frozen in liquid nitrogen for subsequent DNA extraction and gut bacterial community analysis (Liu et al., 2019).
Gut DNA was extracted from the honeybee gut samples using the FastDNA SPIN Kit (MP Biomedicals, Santa Ana, CA, United States) according to manufacturerās protocol. Extraction blanks, containing all the components except gut samples, were used as quality controls. The DNA from the whole gut of one bee was dissolved in 100μl TE buffer, quantified with NanoDrop 2000 (Thermo Scientific, Wilmington, DE, United States), and stored at ā80°C until use.
The V3-V4 hypervariable regions of the bacterial 16S rRNA gene were amplified in triplicates with the primer set 338F (5ā²-ACTCCTACGGGAGGCAGCAG-3ā²) and 806R (5ā²-GGACTACHVGGGTWTCTAAT-3ā²). The oligonucleotides of six-base barcodes were incorporated with the forward and the reverse primers to distinguish sequencing samples. Polymerase Chain Reaction (PCR) was conducted in a 20μl reaction mixture, containing 4μl of 5ĆFastPfu Buffer, 2μl of 2.5mM dNTPs, 0.8μl of each primer (5μm), 0.4μl of FastPfu Polymerase (TransGen Biotech, Beijing, China), and 10ng of template DNA. Each reaction was performed under the following procedures: denaturation at 95°C for 3min, annealing for 25cycles (95°C for 30s, 55°C for 30s, and 72°C for 45s), and a final extension at 72°C for 10min. Reaction mixtures without DNA templates served as negative controls to test for contamination. The size and quality of PCR products were checked by TapeStation (Agilent Technologies, Santa Clara, CA, United States). The triplicate PCR products of each sample were pooled and purified using the AxyPrep DNA Gel Extraction Kit (Axygen Biosciences, Union City, CA, United States) and then quantified using the QuantiFluor-ST (Promega, Madison, WI, United States). After normalization in equimolar amounts, the purified amplicons were sequenced on an Illumina MiSeq platform (Illumina, San Diego, CA, United States) according to standard protocols.
The raw sequencing data were processed using the Quantitative Insights Into Microbial Ecology. First, sequences were filtered according to Liu et al. (2020). Then, the high-quality sequences were merged using FLASH.2 Finally, the merged sequences were clustered into operational taxonomic units (OTUs) at 97% identity threshold using UPARSE,3 while chimeric sequences were removed using UCHIME. The OTUs were assigned to a taxonomic unit by RDP Classifier4 against the SILVA 16S rRNA database (Release 128)5 using a confidence threshold of 70%. The sequences were subsampled to the minimum depth (30,707) prior to analysis.
The āsurvivalā of honeybees was estimated by the Kaplan-Meier (KM) method taking into account the numbers of survivors and dead honeybees, and the samples sacrificed during the study. The KM method is considered efficient and sufficiently general for estimating survival curve (Motta et al., 2018; Al Naggar et al., 2020). Statistical differences following various treatments were assessed by a log-rank paired test, and the p values were adjusted using a Bonferroni procedure. Cumulative pollen consumption indicated the total mass of pollen consumption from the first day to each time point, and the potential maximum honeybee pollen intake was estimated by fitting the cumulative pollen consumption to a first-order kinetic equation. Honeybee weight loss indicated the loss of body weight at each time point relative to the initial weight (Day 0), and the apparently zero-order rate of weight loss was obtained from a linear regression based on untransformed data. Comparisons of equation coefficients between the control and different treatments were achieved by bootstrapping (1000 times) followed by pairwise t test (Zhou et al., 2008).
One-way ANOVA with a post hoc least significant difference test was performed to test the differences among treatments. The contents of La were log-transformed for normality prior to ANOVA. The rarefied sample-OTU matrix was log-transformed to reduce the influence of highly abundant species (Anderson et al., 2006). Principal coordinates analysis (PCoA; using function āpcoaā), permutational multivariate analysis of variance (PERMANOVA; āadonisā), and the distance-based multivariate dispersion test (ābetadisperā) were performed using VEGEN package in R,6 to assess the influence of nano-La2O3 on the gut bacterial community composition.
Due to the simple composition and significant inter-individual variation in the gut samples (Kwong and Moran, 2016), the genera that occurred in more than half of 55 samples were defined as the common ones. Spearman correlation was used to identify affected taxa whose relative abundance was significantly correlated (p<0.05) with nano-La2O3 exposure dose at day 6 and 12. Linear or exponential regression analysis was further conducted to examine the relationship between affected taxa and host physiological parameters (survival, cumulative pollen consumption, and weight loss) and nano-La2O3 exposure dose. Analyses were executed using R,7 SPSS (SPSS, Chicago, IL, United States), or SigmaPlot (Systat Software, Chicago, IL, United States).
Wild honeybees in the field were assayed to evaluate the environmental background of La in honeybees. Incubated honeybees with nano-La2O3 exposure were also assayed to assess treatment effects on uptake of dietary exposure of nano-La2O3. The background La residue (0.20μg beeā1) in wild honeybees was significantly higher than the lab-reared ones with no (exposure, 0mgkgā1; residual, 0.06μg beeā1) or low-dosage (1mgkgā1; 0.04μg beeā1) exposure of nano-La2O3 (p<0.05; Figure 1), suggesting that honeybees did suffer La exposure under field condition. For incubated honeybees, the La residues increased significantly with La2O3 exposure doses (Spearmanās R=0.86, p<0.001; Figure 1). Notably, La residue in the honeybees treated with medium dosage (10mgkgā1) nano-La2O3 exposure were comparable to the background La residue in wild honeybees (p>0.05; Figure 1). Therefore, the medium dosage of nano-La2O3 used in this study could be taken into account to predict the natural La exposure to honeybees, although it remains a challenge to characterize the complex forms of La during translocation in environmental matrices (Ma et al., 2011; Li et al., 2014). La residues in high (100mgkgā1) and the highest dosage (1,000mgkgā1) treatments were approximately 7 and 55 times that of wild honeybees (p<0.05; Figure 1). It is reported that high concentrations of La could result in irreversibly adverse impacts to plants and Daphnia magna, despite of its neutral effects on Chlorella sp. (Balusamy et al., 2015; Yue et al., 2017). In addition, the measured La residue was lower than the corresponding cumulative consumption (Supplementary Figure 1), indicating that nano-La2O3 was released to the intestinal environment and partially excreted through the gut. Therefore, we further conducted honeybee toxicity assays to explore the potential effects of nano-La2O3 on honeybee health and gut microbiota.
Figure 1. Environmental background of La content in wild honeybee (collected from field) and cumulative La residues in lab-reared honeybees after 12-day nano-La2O3 exposure. Error bars indicate the standard error of the mean (n=3). Different letters near bars indicate significant differences among samples (p<0.05; ANOVA-LSD; data were log-transformed for variance homogeneity prior to comparison).
The Kaplan-Meier survival curves of honeybees showed that survival significantly decreased in the high or highest NP concentration treatments compared to the control (p<0.001), while there were negligible decreases in low and medium treatments (p=1 and p=0.07, respectively; Figure 2; Supplementary Table 1). This suggests a dose-dependent toxic effect of nano-La2O3 on honeybeeās survival, which is in line with a previous study (Dabour et al., 2019). Previous studies indicated that nano-La2O3 is chronically toxic to the lung that can strip membrane phosphate groups in acidifying lysosomes and induce cellular and pulmonary damage (Li et al., 2014). Pollen provides most of the nutrients (e.g., proteins, amino acids, and lipids) for honeybee physiological development (Di Pasquale et al., 2013). The results revealed major differences across the time course of cumulative pollen consumption in different treatments (Figure 3A,B; Supplementary Table 2), indicating dose-dependent toxic effects of nano-La2O3 on honeybee nutrition intake (Glavan et al., 2017). In addition, there were positive relationships between weight loss and exposure days, such that the rate of the weight losses for the two highest dose treatments was significantly greater than that of the control (p<0.05, Figures 3C,D; Supplementary Table 3). Thus, exposure to sufficient doses of nano-La2O3 can decrease the cumulative body weight of honeybees. Body weight is a sensitive indicator of nutritional and energetic effects, which are tightly linked with gut microbiota (Zheng et al., 2017). Thus, the effect of nano-La2O3 exposure on the honeybee gut microbiota was worthy of investigation.
Figure 2. The Kaplan-Meier survival curves of honeybees under different concentrations of nano-La2O3 exposure. Survival was monitored and recorded each day for 12days. Different lower-case letters indicate significant differences among samples (log-rank (Mantel-Cox) paired test and Bonferroni correction, p<0.05). Detailed numbers of survivors are listed in Supplementary Table 1.
Figure 3. Effects of nano-La2O3 exposure on honeybeesā pollen consumption and weight loss. (A) cumulative pollen consumption per honeybee per day; (B) maximum pollen intake estimated by first-order kinetic fitting (Y0, mg beeā1, in A); (C) honeybee weight loss (the loss of the body weight per honeybee compared with the weight at day 0); and (D) weight loss rate estimated using linear regression (k, mg beeā1 dā1, in C). Error bars indicated 95% confidence intervals. *indicates significant difference between treatment and control (p<0.05; tested using bootstrapping with 1000 randomizations). Detailed pollen consumption and body weight for each treatment are listed in Supplementary Tables 2 and 3.
To access whether honeybee gut bacterial community will respond to nano-La2O3 exposure, we extracted gut DNA (Supplementary Figure 2) and conducted bacterial 16S rRNA gene sequencing. The results of PCoA, PERMANOVA, and distance-based multivariate dispersion showed that nano-La2O3 exposure caused significant shift of gut bacterial community composition (p <0.05; Figures 4AāF), with or without the consideration of the effect of exposure days (day 6 and 12; p <0.05; Figures 4A,D). These results suggest that Nano-La2O3 exposure can cause significant honeybee gut bacterial compositional dysbiosis in a relative short term of within 6-day exposure. When the dose of nano-La2O3 exposure was assessed, a gradual shift of gut bacterial community composition with increasing exposure dose of nano-La2O3 was observed at both day 6 (linear regression, p <0.05) and day 12 (p =0.07), while the significant effects were only observed for the highest dose (1,000mgkgā1) at both days 6 and 12 as evidenced by the pairwise comparison of gut community compositional differences (p <0.05; multivariate dispersion test; Figures 4E,F). These further suggest the dose-dependent effect of nano-La2O3 on honeybee gut microbiota and that a relative high threshold of nano-La2O3 exposure (e g., a diet concentration of 100ā1,000mgkgā1 or an intake rate of 0.1ā1.0μg beeā1 dayā1; Figure 1) are necessary to generate substantial and sharp shifts of gut microbial communities in a short term (within 6days).
Figure 4. Nano-La2O3 exposure perturbs gut microbiota as evidenced by principal coordinates analysis (AāC), nonparametric multivariate analysis of variance (AāC), and multivariate dispersion test (DāF). (A,D) analyses based on all samples; (B,E) samples of day 6; (C,F) samples of day 12. Different symbols (# or ā ) under x-axis in panel (C,D) indicate significant differences of gut bacterial community composition among treatments (p<0.05; multivariate dispersion test).
Besides the overall shift of honeybee gut bacterial communities, the response of specific members within the communities to nano-La2O3 exposure was also examined. In total, 64 genera comprised of 96 OTUs were identified, while the gut bacterial community composition was mainly dominated by nine genera (Figure 5), accounting for ca. 95.87% total abundances of the whole communities. Notably, the genera Serratia, Frischella, and Bombella were significantly related with nano-La2O3 exposure dose (p<0.05; Figure 5). Specifically, nano-La2O3 exposure enriched gut pathogen genera Serratia and Frischella, among which, the genus Serratia was found to be the most sensitive taxa whose relative abundance increased most significantly with increasing exposure dose (p<0.001 at day 12, Figure 5). It is important to note that the genus Serratia is an intrinsically opportunistic pathogen which becomes highly abundant when hosts are stressed or become diseased (Glavan et al., 2017; Raymann et al., 2018). The genus Serratia contains antimicrobial resistance genes acquired through horizontal gene transfer, which likely contributes to its high tolerance against stresses (Sandner-Miranda et al., 2018). Hence, the opportunistic pathogen Serratia has a survival advantage relative to other bacteria, for example, when nano-La2O3 induces cellular phospholipid damage and bacterial death (Li et al., 2014). In addition, the genus Frischella is often reported as a rare gut bacterial taxa that is less abundant and irregularly occurring (Kwong and Moran, 2016). The abundance of Frischella in our study varied largely across individual honeybees, but positively correlated with the exposure dose of nano-La2O3 at day 12 (Figure 5). This is supported by the fact that the Frischella is an opportunistic pathogen that can establish in a specific niche, for example, when the host suffers tissue damage and pathogen invasion (Maes et al., 2016; Emery et al., 2017). We also found that the typically acetic acid forming genus Bombella (Yun et al., 2017) was negatively related to nano-La2O3 exposure at day 12 (Figure 5).
Figure 5. Heatmap illustrating the relative sequence abundances (log2-transformed; 30,707 depth basis) of common bacterial genera in the guts of honeybees of different treatments. Asterisk indicate significant relationship (Spearmanās correlation) between the relative abundances of core genera and exposure dose of nano-La2O3 (*p<0.05, **p<0.01, ***p<0.001).
There are specific relationships between environmental stressors, honeybee gut microbiota, and honeybee colonial resistance to pathogens (Doublet et al., 2015; Bonilla-Rosso and Engel, 2018; Radziwill-Bienkowska et al., 2018). Nano-La2O3 could dissolve in the gut and strip phosphates from the phospholipids on bacterial membranes (Zheng et al., 2019), which could cause cell damage and impose enhanced selection pressure on gut bacteria. When the bacterial community is unstable, honeybee colony disease resistance may decrease with the thrive of existing gut pathogens (Maes et al., 2016). The significance here is that the enteric pathogens, which were involved in specific and functionally distinct interactions within the bacterial community, could cause gut dysbiosis. The prevalence of pathogenic Serratia and Frischella indicate a disrupted gut homeostasis, thus serving as a diagnostic signature of dysbiosis (Emery et al., 2017; Raymann et al., 2018). Therefore, nano-La2O3 exposure may not only induce dysbiosis of honeybee gut bacterial communities, but also enhance the competitive advantages of pathogens.
Gut microbiota play crucial roles in host health (Engel et al., 2016; Zhang et al., 2020). The relationships between the abundances of main bacterial taxa and the physiological parameters of honeybees (survival, cumulative pollen consumption, and weight loss) were assessed with linear or exponential regression (Supplementary Table 4). The result showed that the abundances of pathogenic Serratia and Frischella were significantly related to honeybee physiological parameters at either day 6 or day 12 (p<0.05; Figure 6).
Figure 6. Linear or exponential regressions showing the relationships between honeybee physiological parameters (survival, cumulative pollen consumption, and weight loss) and the relative abundances (log2-transformed) of genera Serratia (A,C,E) and Frischella (B,D,F) after 6-day and 12-day nano-La2O3 exposures. Error bars indicate the standard error of the mean (n=5). The solid and dotted lines represent significant (p<0.05) and nonsignificant (p>0.05) relationships, respectively.
GRAPHICAL ABSTRACT. The effects of nano-La2O3 on honeybee physiology and gut bacterial communities are additive: nano-La2O3 can deteriorate honeybee health potentially by enriching for pathogens in gut bacterial communities.
Our result showed a significant decrease of honeybee survival with the increasing abundances of the pathogenic genera Serratia and Frischella at day 12 (Figures 6A,B). This is in line with previous studies, showing that the enrichment of pathogens, for example, the genera Serratia and Frischella, deteriorates host development and increases host mortality (Doublet et al., 2015;Maes et al., 2016; Raymann et al., 2018). Our results, as well as the previous evidence, imply that the response of the gut microbiota and the specific functional taxa, including the pathogenic ones, may act important roles in mediating the effects of contaminants, such as nano-La2O3 assessed in this study, on host health (Raymann et al., 2017; Motta et al., 2018). Notably, these correlations did not provide conclusive evidence that the enrichment of the pathogenic Serratia and Frischella directly cause honeybee death, but they are suggestive. Further, perhaps in vivo, infection experiments are needed to establish the virulence of pathogens from various contaminant exposures and to assess whether pathogenesis derives directly from contaminant-promoted thrive of pathogen or indirectly from contaminant-induced inhibition of beneficial taxa.
Gut dysbiosis induces dramatic effects on honeybee health (Hamdi et al., 2011). Gut microbiota affect host weight by mediating host nutritional physiology (e.g., vitellogenin level; Zheng et al., 2017). Hence, gut dysbiosis can cause metabolic disorders and impair host development through altering hormone production (Cryan and Dinan, 2012). In this study, the abundance of Serratia was significantly related to cumulative pollen consumption and weight loss at day 12 (Figures 6C,E). The abundance of Frischella exponentially increased, while the body weight decreased under nano-La2O3 exposure (Figures 6D,F). According to these results, an inference is that the markers of gut dysbiosis, pathogenic Serratia and Frischella, may affect hormonal signaling-related gene expression and subsequently induce pathophysiological responses (e.g., impaired nutrition intake and body weight; Maes et al., 2016; Raymann et al., 2018). However, testing this hypothesis requires studying the relationship between hormone gene expression and gut pathogen abundance. Generally, it may be difficult to disentangle the effects of nano-La2O3 on gut bacterial communities independent of the direct effects on the host, which may, in turn, alter the tolerance to pathogens (Raymann et al., 2017). For example, nano-La2O3 exposure inhibited honeybee pollen intake and thus affected the resistance threshold of honeybees to pathogen stress (Di Pasquale et al., 2013). Although several studies have explored how contaminant-induced shifts in gut microbiota affect host physiology (Raymann et al., 2017; Motta et al., 2018), evidence of direct effects is currently lacking. In the future, it would be important to investigate how changes in honeybee health following environment disturbances may trigger changes to gut microbiota.
In summary, this study examined the effects of nano-La2O3 on honeybee health and gut bacterial communities. Our results provide evidence that nano-La2O3 exerted dose-dependent detrimental effects on honeybee physiology as reflected by the decrease in honeybee survival, pollen consumption, and body weight. Further, the exposures of 0 to 100mgkgā1 nano-La2O3 had no significant effects on gut bacterial community, while the exposure dose of 1,000mgkgā1 caused a significant community compositional shift. Besides, the specific genera within the community, including the pathogenic Serratia and Frischella, and the digestion-related bacteria Bombella, also responded significantly to nano-La2O3 exposure. Moreover, honeybee physiological impairments were significantly related to the enrichment of Serratia and Frischella. Collectively, these findings suggest that pathogen enrichment and gut dysbiosis may be at least partially responsible for adverse effects of nano-La2O3 exposure to honeybee health, thus extending our knowledge regarding the effects of nano-La2O3 on honeybee (Apis mellifera).
The 16S rRNA datasets generated in this study can be found in the SRA archive in GenBank under the BioProject PRJNA764692.
This study was reviewed and approved by the ethics committee of Institute of Apicultural Research, Chinese Academy of Agricultural Sciences (IAR, CAAS).
YG and Y-JL proposed the project and designed the experiments. Q-YD, ZJ, Y-YW, and Y-JL performed the exposure experiment and collected honeybee physiological data. X-TB, JW, and Y-JL collected the sequencing data. TX characterized the nanoparticles. X-TB and ZJ analyzed and visualized the data. X-TB and YG wrote the original draft. QZ, TX, BX, ZJ, and PH reviewed and edited the manuscript. All authors contributed to the data interpretation and paper writing.
This work was supported by National Natural Science Foundation of China (41671254 and 31772683), Chinese Academy of Agricultural Sciences (Elite Youth Program to Y-JL), and State Key Laboratory of Urban and Regional Ecology (SKLURE2017-1\137).
The authors declare that the research was conducted in the absence of any commercial or financial relationships that could be construed as a potential conflict of interest.
All claims expressed in this article are solely those of the authors and do not necessarily represent those of their affiliated organizations, or those of the publisher, the editors and the reviewers. Any product that may be evaluated in this article, or claim that may be made by its manufacturer, is not guaranteed or endorsed by the publisher.
The Supplementary Material for this article can be found online at: https://www.frontiersin.org/articles/10.3389/fmicb.2021.780943/full#supplementary-material
Al Naggar, Y., Dabour, K., Masry, S., Sadek, A., Naiem, E., and Giesy, J. P. (2020). Sublethal effects of chronic exposure to CdO or PbO nanoparticles or their binary mixture on the honey bee (Apis millefera L.). Environ. Sci. Pollut. Res. 27, 19004ā19015. doi: 10.1007/s11356-018-3314-2
Anderson, M. J., Ellingsen, K. E., and McArdle, B. H. (2006). Multivariate dispersion as a measure of beta diversity. Ecol. Lett. 9, 683ā693. doi: 10.1111/j.1461-0248.2006.00926.x
Balusamy, B., Tastan, B. E., Ergen, S. F., Uyar, T., and Tekina, T. (2015). Toxicity of lanthanum oxide (La2O3) nanoparticles in aquatic environments. Environ. Sci.-Process Impacts 17, 1265ā1270. doi: 10.1039/c5em00035a
BargaÅska, Å»., Ålebioda, M., and NamieÅnik, J. (2016). Honey bees and their products: bioindicators of environmental contamination. Crit. Rev. Environ. Sci. Technol. 46, 235ā248. doi: 10.1080/10643389.2015.1078220
Benelli, G. (2018). Mode of action of nanoparticles against insects. Environ. Sci. Pollut. Res. 25, 12329ā12341. doi: 10.1007/s11356-018-1850-4
Bondarenko, O., Mortimer, M., Kahru, A., Feliu, N., Javed, I., Kakinen, A., et al. (2021). Nanotoxicology and nanomedicine: The yin and Yang of nano-bio interactions for the new decade. Nano Today 39:101184. doi: 10.1016/j.nantod.2021.101184
Bonilla-Rosso, G., and Engel, P. (2018). Functional roles and metabolic niches in the honey bee gut microbiota. Curr. Opin. Microbiol. 43, 69ā76. doi: 10.1016/j.mib.2017.12.009
Cariveau, D. P., Elijah Powell, J., Koch, H., Winfree, R., and Moran, N. A. (2014). Variation in gut microbial communities and its association with pathogen infection in wild bumble bees (Bombus). ISME J. 8, 2369ā2379. doi: 10.1038/ismej.2014.68
Chen, P., Huang, J., Rao, L., Zhu, W., Yu, Y., Xiao, F., et al. (2021). Resistance and resilience of fish gut microbiota to silver nanoparticles. mSystems 6:e00630-00621. doi: 10.1128/mSystems.00630-21
Cox-Foster, D. L., Conlan, S., Holmes, E. C., Palacios, G., Evans, J. D., Moran, N. A., et al. (2007). A metagenomic survey of microbes in honey bee colony collapse disorder. Science 318, 283ā287. doi: 10.1126/science.1146498
Cryan, J. F., and Dinan, T. G. (2012). Mind-altering microorganisms: the impact of the gut microbiota on brain and behaviour. Nat. Rev. Neurosci. 13, 701ā712. doi: 10.1038/nrn3346
Dabour, K., Al Naggar, Y., Masry, S., Naiem, E., and Giesy, J. P. (2019). Cellular alterations in midgut cells of honey bee workers (Apis millefera L.) exposed to sublethal concentrations of CdO or PbO nanoparticles or their binary mixture. Sci. Total Environ. 651, 1356ā1367. doi: 10.1016/j.scitotenv.2018.09.311
De la Torre Roche, R., Servin, A., Hawthorne, J., Xing, B., Newman, L. A., Ma, X., et al. (2015). Terrestrial trophic transfer of bulk and nanoparticle La2O3 does not depend on particle size. Environ. Sci. Technol. 49, 11866ā11874. doi: 10.1021/acs.est.5b02583
Di Pasquale, G., Salignon, M., Le Conte, Y., Belzunces, L. P., Decourtye, A., Kretzschmar, A., et al. (2013). Influence of pollen nutrition on honey bee health: do pollen quality and diversity matter? PLoS One 8:e72016. doi: 10.1371/journal.pone.0072016
Doublet, V., Labarussias, M., de Miranda, J. R., Moritz, R. F. A., and Paxton, R. J. (2015). Bees under stress: sublethal doses of a neonicotinoid pesticide and pathogens interact to elevate honey bee mortality across the life cycle. Environ. Microbiol. 17, 969ā983. doi: 10.1111/1462-2920.12426
Ellegaard, K. M., and Engel, P. (2019). Genomic diversity landscape of the honey bee gut microbiota. Nat. Commun. 10:446. doi: 10.1038/s41467-019-08303-0
Emery, O., Schmidt, K., and Engel, P. (2017). Immune system stimulation by the gut symbiont Frischella perrara in the honey bee (Apis mellifera). Mol. Ecol. 26, 2576ā2590. doi: 10.1111/mec.14058
Engel, P., Kwong, W. K., McFrederick, Q., Anderson, K. E., Barribeau, S. M., Chandler, J. A., et al. (2016). The bee microbiome: impact on bee health and model for evolution and ecology of host-microbe interactions. mBio 7:e02164-15. doi: 10.1128/mBio.02164-15
Gao, J., Li, R., Wang, F., Liu, X., Zhang, J., Hu, L., et al. (2017). Determining the cytotoxicity of rare earth element nanoparticles in macrophages and the involvement of membrane damage. Environ. Sci. Technol. 51, 13938ā13948. doi: 10.1021/acs.est.7b04231
Ge, Y., Horst, A. M., Kim, J., Priester, J. H., Welch, Z. S., and Holden, P. A. (2016). āToxicity of manufactured nanomaterials to microorganisms,ā in Engineered Nanoparticles and the Environment: Biophysicochemical Processes and Toxicity. eds. B. Xing, C. D. Vecitis, and N. Senesi (Hoboken: John Wiley & Sons), 320ā346.
Ge, Y., Jing, Z., Diao, Q., He, J.-Z., and Liu, Y.-J. (2021). Host species and geography differentiate honeybee gut bacterial communities by changing the relative contribution of community assembly processes. mBio 12:e0075121. doi: 10.1128/mBio.00751-21
Ge, Y., Shen, C., Wang, Y., Sun, Y. Q., Schimel, J. P., Gardea-Torresdey, J. L., et al. (2018). Carbonaceous nanomaterials have higher effects on soybean rhizosphere prokaryotic communities during the reproductive growth phase than during vegetative growth. Environ. Sci. Technol. 52, 6636ā6646. doi: 10.1021/acs.est.8b00937
Glavan, G., Milivojevic, T., Bozic, J., Sepcic, K., and Drobne, D. (2017). Feeding preference and sub-chronic effects of ZnO nanomaterials in honey bees (Apis mellifera carnica). Arch. Environ. Contam. Toxicol. 72, 471ā480. doi: 10.1007/s00244-017-0385-x
Gottschalk, F., Sonderer, T., Scholz, R. W., and Nowack, B. (2009). Modeled environmental concentrations of engineered nanomaterials (TiO2, ZnO, Ag, CNT, fullerenes) for different regions. Environ. Sci. Technol. 43, 9216ā9222. doi: 10.1021/es9015553
Hamdi, C., Balloi, A., Essanaa, J., Crotti, E., Gonella, E., Raddadi, N., et al. (2011). Gut microbiome dysbiosis and honeybee health. J. Appl. Entomol. 135, 524ā533. doi: 10.1111/j.1439-0418.2010.01609.x
Han, X., Geller, B., Moniz, K., Das, P., Chippindale, A. K., and Walker, V. K. (2014). Monitoring the developmental impact of copper and silver nanoparticle exposure in drosophila and their microbiomes. Sci. Total Environ. 487, 822ā829. doi: 10.1016/j.scitotenv.2013.12.129
Holden, P. A., Klaessig, F., Turco, R. F., Priester, J. H., Rico, C. M., Avila-Arias, H., et al. (2014). Evaluation of exposure concentrations used in assessing manufactured nanomaterial environmental hazards: are they relevant? Environ. Sci. Technol. 48, 10541ā10551. doi: 10.1021/es502440s
Jack, C. J., Uppala, S. S., Lucas, H. M., and Sagili, R. R. (2016). Effects of pollen dilution on infection of Nosema ceranae in honey bees. J. Insect Physiol. 87, 12ā19. doi: 10.1016/j.jinsphys.2016.01.004
Kapoor, R. T., Salvadori, M. R., Rafatullah, M., Siddiqui, M. R., Khan, M. A., and Alshareef, S. A. (2021). Exploration of microbial factories for synthesis of nanoparticles ā a sustainable approach for bioremediation of environmental contaminants. Front. Microbiol. 12:1404. doi: 10.3389/fmicb.2021.658294
Klein, A.-M., VaissiĆØre, B. E., Cane, J. H., Steffan-Dewenter, I., Cunningham, S. A., Kremen, C., et al. (2007). Importance of pollinators in changing landscapes for world crops. Proc. R. Soc. B-Biol. Sci. 274, 303ā313. doi: 10.1098/rspb.2006.3721
Kos, M., Jemec Kokalj, A., Glavan, G., Marolt, G., Zidar, P., BožiÄ, J., et al. (2017). Cerium(IV) oxide nanoparticles induce sublethal changes in honeybees after chronic exposure. Environ. Sci. Nano 4, 2297ā2310. doi: 10.1039/c7en00596b
Kulaksız, S., and Bau, M. (2013). Anthropogenic dissolved and colloid/nanoparticle-bound samarium, lanthanum and gadolinium in the Rhine River and the impending destruction of the natural rare earth element distribution in rivers. Earth Planet. Sci. Lett. 362, 43ā50. doi: 10.1016/j.epsl.2012.11.033
Kwong, W. K., and Moran, N. A. (2016). Gut microbial communities of social bees. Nat. Rev. Microbiol. 14, 374ā384. doi: 10.1038/nrmicro.2016.43
Li, R., Ji, Z., Chang, C. H., Dunphy, D. R., Cai, X., Meng, H., et al. (2014). Surface interactions with compartmentalized cellular phosphates explain rare earth oxide nanoparticle hazard and provide opportunities for safer design. ACS Nano 8, 1771ā1783. doi: 10.1021/nn406166n
Liu, W., Graham, E. B., Zhong, L., Zhang, J., Li, S., Lin, X., et al. (2020). Long-term stochasticity combines with short-term variability in assembly processes to underlie rice paddy sustainability. Front. Microbiol. 11:873. doi: 10.3389/fmicb.2020.00873
Liu, Y. J., Qiao, N. H., Diao, Q. Y., Jing, Z., Vukanti, R., Dai, P. L., et al. (2019). Thiacloprid exposure perturbs the gut microbiota and reduces the survival status in honeybees. J. Hazard. Mater. 389:121818. doi: 10.1016/j.jhazmat.2019.121818
Ma, Y., He, X., Zhang, P., Zhang, Z., Guo, Z., Tai, R., et al. (2011). Phytotoxicity and biotransformation of La2O3 nanoparticles in a terrestrial plant cucumber (Cucumis sativus). Nanotoxicology 5, 743ā753. doi: 10.3109/17435390.2010.545487
Maes, P. W., Rodrigues, P. A. P., Oliver, R., Mott, B. M., and Anderson, K. E. (2016). Diet-related gut bacterial dysbiosis correlates with impaired development, increased mortality and Nosema disease in the honeybee (Apis mellifera). Mol. Ecol. 25, 5439ā5450. doi: 10.1111/mec.13862
Mastronardi, E., Tsae, P., Zhang, X., Monreal, C., and DeRosa, M. C. (2015). āStrategic role of nanotechnology in fertilizers: potential and limitationsā in Nanotechnologies in Food and Agriculture. eds. M. Rai, C. Ribeiro, L. Mattoso, and N. Duran (Cambridge: Springer International Publishing), 25ā67.
Motta, E. V. S., Raymann, K., and Moran, N. A. (2018). Glyphosate perturbs the gut microbiota of honey bees. Proc. Natl. Acad. Sci. U. S. A. 115, 10305ā10310. doi: 10.1073/pnas.1803880115
Nel, A., Xia, T., MƤdler, L., and Li, N. (2006). Toxic potential of materials at the nanolevel. Science 311, 622ā627. doi: 10.1126/science.1114397
Priester, J. H., Ge, Y., Chang, V., Stoimenov, P. K., Schimel, J. P., Stucky, G. D., et al. (2013). Assessing interactions of hydrophilic nanoscale TiO2 with soil water. J. Nanopart. Res. 15:1899. doi: 10.1007/s11051-013-1899-4
Qi, L., Ge, Y., Xia, T., He, J. Z., Shen, C., Wang, J. L., et al. (2019). Rare earth oxide nanoparticles promote soil microbial antibiotic resistance by selectively enriching antibiotic resistance genes. Environ. Sci.-Nano 6, 456ā466. doi: 10.1039/C8EN01129J
Radziwill-Bienkowska, J. M., Talbot, P., Kamphuis, J. B. J., Robert, V., Cartier, C., Fourquaux, I., et al. (2018). Toxicity of food-grade TiO2 to commensal intestinal and transient food-borne bacteria: new insights using Nano-SIMS and synchrotron UV fluorescence imaging. Front. Microbiol. 9:794. doi: 10.3389/fmicb.2018.00794
Raymann, K., Coon, K. L., Shaffer, Z., Salisbury, S., and Moran, N. A. (2018). Pathogenicity of Serratia marcescens strains in honey bees. mBio 9:e01649-18. doi: 10.1128/mBio.01649-18
Raymann, K., Shaffer, Z., and Moran, N. A. (2017). Antibiotic exposure perturbs the gut microbiota and elevates mortality in honeybees. PLoS Biol. 15:e2001861. doi: 10.1371/journal.pbio.2001861
Sandner-Miranda, L., Vinuesa, P., Cravioto, A., and Morales-Espinosa, R. (2018). The genomic basis of intrinsic and acquired antibiotic resistance in the genus Serratia. Front. Microbiol. 9:828. doi: 10.3389/fmicb.2018.00828
Servin, A., Elmer, W., Mukherjee, A., De la Torre-Roche, R., Hamdi, H., White, J. C., et al. (2015). A review of the use of engineered nanomaterials to suppress plant disease and enhance crop yield. J. Nanopart. Res. 17:92. doi: 10.1007/s11051-015-2907-7
Theng, B. K. G., and Yuan, G. (2008). Nanoparticles in the soil environment. Elements 4, 395ā399. doi: 10.2113/gselements.4.6.395
Thimmegowda, G. G., Mullen, S., Sottilare, K., Sharma, A., Mohanta, S. S., Brockmann, A., et al. (2020). A field-based quantitative analysis of sublethal effects of air pollution on pollinators. Proc. Natl. Acad. Sci. U. S. A. 117, 20653ā20661. doi: 10.1073/pnas.2009074117
Tyler, G. (2004). Rare earth elements in soil and plant systems - A review. Plant Soil 267, 191ā206. doi: 10.1007/s11104-005-4888-2
Wen, B., Yuan, D. A., Shan, X. Q., Li, F. L., and Zhang, S. Z. (2001). The influence of rare earth element fertilizer application on the distribution and bioaccumulation of rare earth elements in plants under field conditions. Chem. Speciation Bioavail. 13, 39ā48. doi: 10.3184/095422901783726825
Wu, F., You, Y., Zhang, X., Zhang, H., Chen, W., Yang, Y., et al. (2019). Effects of various carbon nanotubes on soil bacterial community composition and structure. Environ. Sci. Technol. 53, 5707ā5716. doi: 10.1021/acs.est.8b06909
Xu, J., Luo, X., Wang, Y., and Feng, Y. (2018a). Evaluation of zinc oxide nanoparticles on lettuce (Lactuca sativa L.) growth and soil bacterial community. Environ. Sci. Pollut. Res. 25, 6026ā6035. doi: 10.1007/s11356-017-0953-7
Xu, N., Morgan, B., and Rate, A. W. (2018b). From source to sink: rare-earth elements trace the legacy of sulfuric dredge spoils on estuarine sediments. Sci. Total Environ. 637, 1537ā1549. doi: 10.1016/j.scitotenv.2018.04.398
Yue, L., Ma, C., Zhan, X., White, J. C., and Xing, B. (2017). Molecular mechanisms of maize seedling response to La2O3 NP exposure: water uptake, aquaporin gene expression and signal transduction. Environ. Sci.-Nano 4, 843ā855. doi: 10.1039/C6EN00487C
Yun, J. H., Lee, J. Y., Hyun, D. W., Jung, M. J., and Bae, J. W. (2017). Bombella apis sp nov., an acetic acid bacterium isolated from the midgut of a honey bee. Int. J. Syst. Evol. Microbiol. 67, 2184ā2188. doi: 10.1099/ijsem.0.001921
ZariÄ, N. M., IlijeviÄ, K., StanisavljeviÄ, L., and GržetiÄ, I. (2016). Metal concentrations around thermal power plants, rural and urban areas using honeybees (Apis mellifera L.) as bioindicators. Int. J. Environ. Sci. Technol. 13, 413ā422. doi: 10.1007/s13762-015-0895-x
Zhang, Y. R., Mortimer, M., and Guo, L. H. (2020). Interplay between engineered nanomaterials and microbiota. Environ. Sci.-Nano 7, 2454ā2485. doi: 10.1039/d0en00557f
Zheng, H., Ji, Z., Roy, K. R., Gao, M., Pan, Y., Cai, X., et al. (2019). Engineered graphene oxide nanocomposite capable of preventing the evolution of antimicrobial resistance. ACS Nano 13, 11488ā11499. doi: 10.1021/acsnano.9b04970
Zheng, H., Powell, J. E., Steele, M. I., Dietrich, C., and Moran, N. A. (2017). Honeybee gut microbiota promotes host weight gain via bacterial metabolism and hormonal signaling. Proc. Natl. Acad. Sci. U. S. A. 114, 4775ā4780. doi: 10.1073/pnas.1701819114
Zhou, J., Kang, S., Schadt, C. W., and Garten, C. T. (2008). Spatial scaling of functional gene diversity across various microbial taxa. Proc. Natl. Acad. Sci. U. S. A. 105, 7768ā7773. doi: 10.1073/pnas.0709016105
Zhu, D., Zheng, F., Chen, Q. L., Yang, X. R., Christie, P., Ke, X., et al. (2018). Exposure of a soil collembolan to Ag nanoparticles and AgNO3 disturbs its associated microbiota and lowers the incidence of antibiotic resistance genes in the gut. Environ. Sci. Technol. 52, 12748ā12756. doi: 10.1021/acs.est.8b02825
Keywords: Apis mellifera, rare earth oxide nanoparticles, honeybee gut microbiota, nano-La2O3, honeybee health
Citation: Liu Y-J, Jing Z, Bai X-T, Diao Q-Y, Wang J, Wu Y-Y, Zhao Q, Xia T, Xing B, Holden PA and Ge Y (2021) Nano-La2O3 Induces Honeybee (Apis mellifera) Death and Enriches for Pathogens in Honeybee Gut Bacterial Communities. Front. Microbiol. 12:780943. doi: 10.3389/fmicb.2021.780943
Received: 22 September 2021; Accepted: 29 October 2021;
Published: 02 December 2021.
Edited by:
Shiying He, Jiangsu Academy of Agricultural Sciences (JAAS), ChinaReviewed by:
Roberta Fulthorpe, University of Toronto Scarborough, CanadaCopyright Ā© 2021 Liu, Jing, Bai, Diao, Wang, Wu, Zhao, Xia, Xing, Holden and Ge. This is an open-access article distributed under the terms of the Creative Commons Attribution License (CC BY). The use, distribution or reproduction in other forums is permitted, provided the original author(s) and the copyright owner(s) are credited and that the original publication in this journal is cited, in accordance with accepted academic practice. No use, distribution or reproduction is permitted which does not comply with these terms.
*Correspondence: Yong-Jun Liu liuyongjun@caas.cn Yuan Ge yuange@rcees.ac.cn
ā These authors have contributed equally to this work and share first authorship
Disclaimer: All claims expressed in this article are solely those of the authors and do not necessarily represent those of their affiliated organizations, or those of the publisher, the editors and the reviewers. Any product that may be evaluated in this article or claim that may be made by its manufacturer is not guaranteed or endorsed by the publisher.
Research integrity at Frontiers
Learn more about the work of our research integrity team to safeguard the quality of each article we publish.