- 1Guangdong Key Laboratory for Innovative Development and Utilization of Forest Plant Germplasm, College of Forestry and Landscape Architecture, South China Agricultural University, Guangzhou, China
- 2School of Pharmaceutical Sciences, Guangzhou University of Chinese Medicine, Guangzhou, China
Duclauxin is a heptacyclic oligophenalenone dimer consisting of an isocoumarin and a dihydroisocoumarin unit. These two tricyclic moieties are joined by a cyclopentane ring to form a unique hinge or castanets-like structure. Duclauxin is effective against numerous tumor cell lines because it prevents adenosine triphosphate (ATP) synthesis by inhibiting mitochondrial respiration. There are about 36 reported natural duclauxin analogs mainly produced by 9 Penicillium and Talaromyces species (T. duclauxii, T. aculeatus, T. stipitatus, T. bacillisporus, T. verruculosus, T. macrosporus, P. herquei, P. manginii, and Talaromyces sp.). These metabolites exhibit remarkable biological activities, including antitumor, enzyme inhibition, and antimicrobial, showing tremendous potential in agricultural and medical applications. This review highlights the chemical structures and biological activities of fungal duclauxins, together with biosynthesis, absolute configuration, and mode of action for important duclauxins. Furthermore, phylogenetic analysis and correct names of Penicillium and Talaromyces species producing duclauxins are presented in this review.
Introduction
Natural products are derived from natural sources such as microorganisms, plants, or animals (Jeerapong et al., 2015; Calixto, 2019; Mao et al., 2021). In the past few years, it has been proved that they are a continuing source of novel bioactive compounds and have a significant impact on modern drug discovery (Singh and Chandra, 2014; Abbas et al., 2018). At present, more than 70% of anticancer and antibacterial compounds are natural products or their derivatives that play a vital role in the pharmaceutical sector (Newman and Cragg, 2016, 2020). Among the microorganisms, fungi have attained much attention in recent decades, mainly regarding the discovery of beneficial secondary metabolites. It is estimated that more than 23,000 microbial natural products have been discovered, of which around 45% are from fungi (Cao et al., 2015). Fungal secondary metabolites have wide-ranging applications such as antifungals, antibiotics, antiparasitic, and anticancer agents (Barbero et al., 2018; Lima et al., 2019; Shan et al., 2020; Torres-Mendoza et al., 2020). The most famous example in history is the discovery of penicillin, an antibiotic drug produced by Penicillium chrysogenum that has saved many human lives (Fleming, 1929). Genera Penicillium and Talaromyces produce a wide range of polyketide-derived secondary metabolites with diverse bioactivities. These compounds are promising antitumor and antibacterial agents, leading to their applications in agriculture, environment, forestry, and pharmaceutical sectors (Cao et al., 2015; Chaudhary et al., 2020; Dramae et al., 2020; Jimenez-Arreolaa et al., 2020).
Among fungal secondary metabolites, duclauxin (1) is a well-known antitumor agent because it prevents ATP synthesis by inhibiting mitochondrial respiration (Fuska et al., 1974). Structurally, it is a heptacyclic oligophenalenone dimer consisting of an isocoumarin and a dihydroisocoumarin core (Shibata et al., 1965; Ogihara et al., 1968). Duclauxin (1) was first isolated from the cultures of fungus Talaromyces duclauxii in 1965 (Shibata et al., 1965). After that, a growing number of duclauxin derivatives were isolated from the genera Talaromyces and Penicillium (Yamazaki and Okuyama, 1980; Wu et al., 2015; Zang et al., 2016a; Chaiyosang et al., 2019; Wang et al., 2019; Chaudhary et al., 2020; Dramae et al., 2020; Jimenez-Arreolaa et al., 2020). Duclauxins have attracted particular attention as they have great potential to become remedies against multiple diseases. These compounds possess a wide range of biological properties, including antitumor (Cao et al., 2015), antibacterial (Dramae et al., 2020), cytotoxic (Dethoup et al., 2006), antimalarial (Chaiyosang et al., 2019), and enzyme inhibition (Wu et al., 2015; Wang et al., 2019; Jimenez-Arreolaa et al., 2020). Some of these compounds have been identified as novel inhibitors of cancer cell lines (Shiojiri et al., 1983) and pathogens (Chaiyosang et al., 2019). Thus, they can potentially contribute to the development of new cancer chemotherapies and antibiotics.
This review focuses on chemical structures and biological activities of duclauxin derivatives produced by fungi. Meanwhile, the phylogenetic relationship of Penicillium and Talaromyces species producing duclauxins is described as well. Furthermore, biosynthesis, absolute configuration, and mode of action for some duclauxin derivatives are also discussed here. This review covers the literature related to duclauxins from the year of 1965–2021.
Duclauxin and Its Derivatives Produced by Fungi
Duclauxin (1) (Figure 1) is a heptacyclic bis (oxaphenalenone) heterodimer made up of two functionalized 2-oxaphenalen-1-one units (corymbiferan skeleton) linked by two bonds, which form the cyclopentane. Interestingly, all duclauxin derivatives contain at least one unit of the dihydrocoumarin benzo[de]isochromen-1(3H)-one, and most of them are characterized by a heptacyclic ring system. As shown in Table 1, duclauxin (1) was further produced by seven strains from different Peniclillium and Talaromyces species since the first duclauxin (1) isolated from T. duclauxii in 1965. Until now, 36 duclauxin derivatives are mainly produced by Talaromyces and Penicillium species, isolated from marine sponges, plants endophytes, soil samples, mangroves, and coral reefs (Table 1).
Penicillium and Talaromyces are economically, biotechnologically, and medically important genera that belong to the Eurotiales order and contain many species possessing a worldwide distribution and a vast range of ecological habitats (Tsang et al., 2018). Many Penicillium and Talaromyces species are vital to the pharmaceutical industries as they are robust producers of a diverse spectrum of secondary metabolites with vigorous biological activities (Cai et al., 2020; Rahman and Sarker, 2020). Over the past few years, the taxonomic history of anamorphic species attributed to Penicillium subgenus Biverticillium was reviewed and transferred to the genus Talaromyces (Houbraken et al., 2020). Since taxonomy is a dynamic discipline, and name changes of fungi with medical, biotechnological, and industrial importance are often challenging for researchers in the applied fields to understand. As a result, we added the list of accepted species present in genera Penicillium and Talaromyces that produced duclauxins, based on ITS, BenA, CaM and RPB2 multigene data obtained from the NCBI nucleotide database (GenBank). The list includes accepted names, basionyms, subgenus, section, series, and reproduction attributed to genera Penicillium and Talaromyces (Table 2). To confirm the previous findings, the phylogenetic relationship of Penicillium and Talaromyces species producing duclauxins was analyzed based on the rDNA-ITS sequence obtained from the NCBI database (GenBank). Figure 2 shows the relationship among the species belonging to genera Penicillium and Talaromyces. The phylogenetic tree (Figure 2) revealed that the strain T. duclauxii (accession no. L14534.1, previous name: P. duclauxii) formed a clade with the strain T. duclauxii (accession no. JN899342.1) and had 98.07% similarity. Similarly, the strain T. stipitatus (accession no. JN899348.1), previously known as P. stipitatum had 100% similarity with the strain T. stipitatus (accession no. NR 147424.1) and clustered on the same branch. Moreover, the strain T. aculeatus C08652 (accession no. KT715695.1) was first identified as P. aculeatum based on the 18S rDNA sequence analysis (Huang et al., 2017). However, the latest literature implies that the name of P. aculeatum has been changed into T. aculeatus (Houbraken et al., 2020). The phylogenetic tree (Figure 2) also revealed that the strain T. aculeatus C08652 (accession no. KT715695.1) formed a clade with members of the genus Talaromyces. These results confirmed that species attributed to Penicillium subgenus Biverticillium (P. aculeatum, P. stipitatum, and P. duclauxii) are phylogenetically related to the genus Talaromyces (Figure 2) and were consistent with the article recently published by Houbraken and coworkers (Houbraken et al., 2020). Therefore, we used the currently accepted names of Penicillium and Talaromyces species in this review that produced duclauxins. Based on the structural skeletons, duclauxin derivates can be classified into four types including heptacyclic, octal- and nona-cyclic, N-containing, and asymmetrical.
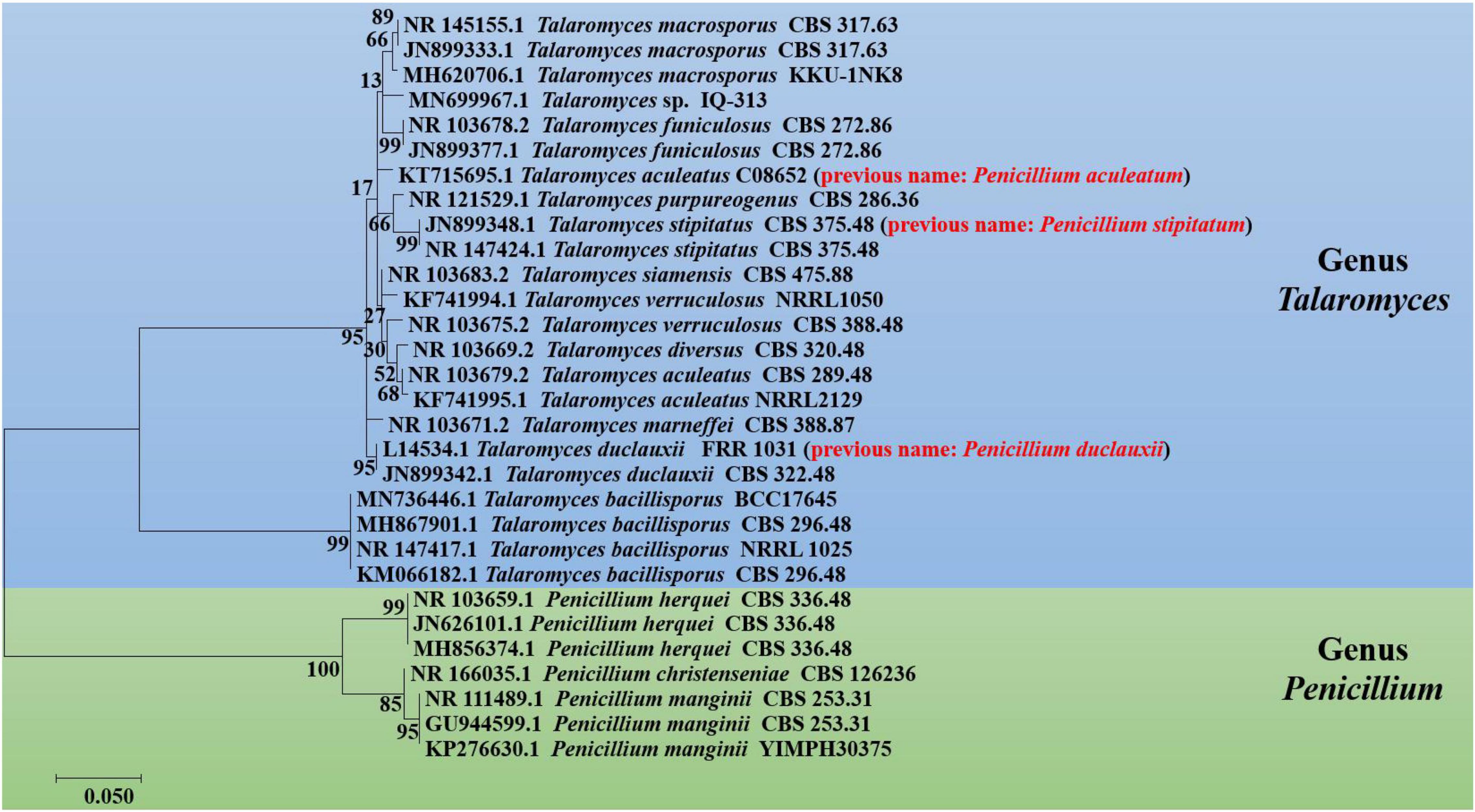
Figure 2. Phylogenetic tree of Penicillium and Talaromyces species regarding duclauxins, based on the rDNA-ITS sequence obtained from the NCBI database. The tree was constructed with the maximum likelihood method in MEGA 7.0 using default parameters, and bootstrap values were calculated after 1,000 replications. The previous or invalid species name is presented with red color between the parenthesis.
Heptacyclic Duclauxin Derivatives
The structures of heptacyclic duclauxins are described as two tricyclic moieties joined by a cyclopentane ring to form a unique hinge-like structure. About 15 heptacyclic duclauxin derivatives were isolated from several fungal strains dwelling in different ecological niches, such as soil, mangrove endophyte, and marine environment (Figure 3 and Table 1). Two new duclauxin derivatives bacillisporins A-B (2–3), were isolated from the ether (Et2O) extract of mycelia of T. bacillisporus NHL 2660 grown on a malt extract medium (Yamazaki and Okuyama, 1980). Afterwards, bacillisporin A (2) was re-isolated from T. stipitatus grown on polished rice medium (Zang et al., 2016b). In another report, bacillisporins A (2) and B (3) were produced by fungus T. macrosporus KKU-1NK8 (Chaiyosang et al., 2019). Interestingly, bacillisporins A (2) and B (3) were isolated from a mangrove-derived endophytic fungus T. aculeatus No. 9EB for the first time. The fungus was obtained from the leaves of mangrove Kandelia candel, collected from Yangjiang, Guangdong province, China (Huang et al., 2017). Further investigations revealed compounds 2 and 3 have also been discovered from the soil fungus T. bacillisporus. The continuing research on duclauxin-like secondary metabolites from T. bacillisporus grown on potato dextrose broth (PDB) media led to the discovery of two new duclauxin derivatives, namely bacillisporins I (4) and J (5), respectively. The skeleton of compounds 2, 3, 4, and 5 is identical except for minor differences at C-9′ and C-1 positions. However, the absolute configuration of compounds 4 and 5 at C-1 could not be determined due to the similar CD patterns of both 1S and 1R configurations. Thus the 1H chemical shift calculation together with DP4 probability analysis may be required to confirm the absolute configuration at C-1 (Figure 3). Heptacyclic oxyphenalenone dimers, bacillisporins D (6) and E (7) were reported first from a soil fungus T. bacillisporus (Dethoup et al., 2006; Dramae et al., 2020). With time, compounds 6–7 were acquired by the chemical investigation of T. macrosporus KKU-1NK8 grown on malt extract peptone broth. The only difference between the structures of compounds 6 and 7 was the C-9′ position (“OH” group for compound 6 and “OAc” group for compound 7). However, the absolute configuration of compounds 6 and 7 at C-9a has not been determined until now. Further experiments such as 1H chemical shift calculation and DP4 probability analysis may be required to confirm the absolute configuration at C-9a (Chaiyosang et al., 2019).
Three new polyketide-derived oligophenalenone dimers, 9a-epi-bacillisporin E (8), bacillisporin F (9), and 1-epi-bacillisporin F (10) were isolated from the soil fungus T. stipitatus ATCC 10500. Their absolute configurations were determined using Gauge-Independent Atomic Orbital (GIAO) Nuclear Magnetic Resonance (NMR) shift calculation followed by DP4 analysis. The differences between compounds 9 and 10 were the chemical shifts of the methoxy group in CD3OD (δH 3.66 for 9 vs. δH 3.58 for 10) and the methine H-1 (δH 6.57 for 9 vs. δH 6.55 for 10) (Zang et al., 2016b). Subsequently, bacillisporin F (9) and 1-epi-bacillisporin F (10) have also been found in soil fungus T. macrosporus. In addition, compound 9 was isolated from a marine fungus T. verruculosus in soft coral. The resonance signals of bacillisporin F (9) and 1-epi-bacillisporin F (10) were similar to those of the macrosporusone E (11) and 1-epi-macrosporusone E (12), except for a hydroxyl group at C-9′ being replaced by an acetoxyl group (Chaiyosang et al., 2019; Wang et al., 2019; Table 1). Cryptoclauxin (13), a well-known duclauxin derivative, was isolated from T. duclauxii for the first time using the silicic acid column chromatography technique (Ogihara et al., 1966). This compound was also re-isolated from T. stipitatus in 2018 (Gao et al., 2018).
Forest soil fungus T. macrosporus KKU-1NK8 yielded three new polyketide-derived oxaphenalenone dimers named macrosporusone E (11), 1-epi-macrosporusone E (12), and macrosporusone D (14) along with xenoclauxin (15). Their structures were established by spectroscopic data and electronic circular dichroism (ECD) analyses. The structure of compound 14 was very similar to that of compound 15, except that an acetoxyl group at C-9′ in 15 was replaced by a hydroxyl group in 14. The sole difference between compounds 11 and 12 was the configuration of C-1 (1S for 11 and 1R for 12). However, the numerical misrepresentation of macrosporusone E (12) and macrosporusone D (14) was found in the published article (doi: 10.1016/j.fitote.2019.03.015) that caused considerable confusion to the readers (Chaiyosang et al., 2019). Further studies also resulted in the identification of xenoclauxin (15) from T. verruculosus, T. duclauxii and a soil fungus Talaromyces sp. IQ-313 (Ogihara et al., 1966; Wang et al., 2019; Jimenez-Arreolaa et al., 2020).
Chemical investigation of marine fungus Talaromyces sp. LF458, isolated from a sponge Axinella verrucosa collected from the Mediterranean Sea, Italy, afforded talaromycesone A (16) (Wu et al., 2015). The continuous research on marine fungus resulted in the discovery of verrucolosin B (17) from a soft coral-associated fungus T. verruculosus collected from the South China Sea. The structure of compound 17 presents a unique methoxycarbonyl-methyl moiety at the C-1 position (Wang et al., 2019; Figure 3).
Octal- and Nona-Cyclic Duclauxin Derivatives
This class of compounds comprises tetra- and penta-cyclic moieties on one side of the structure joined via two C-C bonds with the tricyclic benzannulated moieties. Only three compounds have been reported in this class and they were isolated from several sources of genus Talaromyces (Figure 4 and Table 1). Talaroketals A (18) and B (19) were isolated from the soil fungus T. stipitatus ATCC 10500. Their structures and absolute configurations were determined based on X-ray diffraction and ECD experiments. Talaroketals A (18) and B (19) represent the first example of bis (oxaphenalenone) 5,6-spiroketal and 5,6-fused ketal, respectively. Compound 18 is a new member of the rare benzannulated [6,5]-spiroketal class of natural products (Zang et al., 2016a). Chemical investigation of the marine-derived fungus, T. verruculosus, isolated from the soft coral Goniopora sp. collected from the South China Sea, yielded a new oligophenalenone dimer, named verrucolosin A (20). The detailed structure and absolute configuration were solved with the aid of spectroscopic data, X-ray crystallography, optical rotation, ECD analysis, and NMR calculations. Compound 20 possesses a unique/distinctive octacyclic skeleton in the oxaphenelenone dimer family (Wang et al., 2019).
Nitrogen-Containing Duclauxin Derivatives
Nitrogen-containing duclauxin derivatives are scarcely found in nature, and thus until now, 9 N-containing duclauxin derivatives have been discovered from nature, as shown in Table 1 and Figure 5. The structures of these compounds consist of a heptacyclic dimer in which a nitrogen atom is present in the upper 6/6/6 tri-ring system. Bacillisporin H (21), a new nitrogenated bis-oxaphenalenone was isolated from EtOAc extract of T. stipitatus obtained from rice-based media (Zang et al., 2016b). Studies carried out by Cao et al. (2015) presented the isolation of new polyketide-derived heptacyclic oligophenalenone dimer duclauxamide A1 (22) bearing an N-2-hydroxyethyl moiety from P. manginii YIM PH30375, which was isolated from the elder root of Panax notoginseng, China. X-ray single-crystal diffraction technique and 13C NMR DFT calculation confirmed that compound 22 and other duclauxin analogs possess a unified S-configuration at C-9′, which corrected a long-standing misrepresentation of duclauxin and its analogs as C-9′ R epimers (Cao et al., 2015). A few years later, duclauxamide A1 (22) and two new N-containing oxaphenalenone dimers, duclauxamides B-C (23–24), were discovered from the soil fungus T. bacillisporus BCC17645 (Dramae et al., 2020). Five new oxyphenalenone amino acid hybrids, talauxins E, Q, V, L, and I (25–29), have been identified from the culture of T. stipitatus FP2248C on pearl barley medium. The fungus was recovered as a quiescent contaminant from the Australian fungus Aspergillus banksianus isolated from a soil sample collected in Collaroy, New South Wales, Australia (Chaudhary et al., 2020).
Asymmetrical Duclauxin Derivatives
This class of monomer compounds presents an irregular shape in their structures as compared to other duclauxin derivatives. 7 duclauxins have been identified in this class from fungi so far (Figure 6 and Table 1). Bacillisporin C (30) was isolated for the first time from the mycelia of T. bacillisporus NHL 2660 grown on malt extract medium (Yamazaki and Okuyama, 1980). Likewise, isolation of bacillisporin C (30) was reported twice from soil fungus T. bacillisporus. Compound 30 was declared as the first compound in the duclauxin family, in which one C-C bond is connected with oxygen (O) between the two tricyclic moieties of a heptacyclic dimer (Dethoup et al., 2006; Dramae et al., 2020). Chemical evaluation of a soil fungus T. stipitatus ATCC 10500 collected from Thailand afforded bacillisporin G (31) (Zang et al., 2016b). With time, compound 31 was reported from other Talaromyces species such as Talaromyces sp. IQ-313 and T. macrosporus KKU-1NK8, both were isolated from soil (Chaiyosang et al., 2019; Jimenez-Arreolaa et al., 2020). Chromatographic separation of the EtOAc extract of a forest soil fungus T. macrosporus KKU-1NK8 resulted in three new polyketide-derived oxaphenalenone dimers, macrosporusones A-C (32–34). The fungus was isolated from the forest soil collected in the Pha Nok Kao Silvicultural Station, Khon Kaen Province, Thailand. The NMR spectroscopic data of compound 32 were similar to bacillisporin G (31) except for the absence of an aldehyde group at C-9a and that a hydroxyl group replaced an acetyl group at C-9′. The 1H and 13C NMR spectroscopic data of compound 33 were similar to those of compound 32, except that the hydroxyl group at C-9′ was replaced by an acetoxyl group (δC carbonyl ester group 170.6 and methyl group δH/C 1.99 (s)/20.8). Macrosporusone C (34) is the first oxaphenalenone dimer reported with a chlorine atom in the molecule (Chaiyosang et al., 2019).
Talaromycesone B (35) was isolated as a brown powder from the culture broth and mycelia of a marine fungus Talaromyces sp. LF458 on WSP30 medium. Strain LF458 was isolated from tissues of the sponge Axinella verrucosa collected from the Mediterranean Sea, Italy, and tentatively classified as a T. funiculosum. Compound 35 represents the first case of a 1-nor oxaphenalenone dimer carbon skeleton in nature (Wu et al., 2015; Imhoff, 2016). Additionally, talaromycesone B (35) and the new oxaphenalenone dimer talaromycesone C (36) were isolated from the EtOAc extracts of the mycelia of a soil fungus T. macrosporus KKU-1NK8. Compound 36 was reported as the second compound of oxaphenalenone dimer with a 5-membered lactone ring on one side (Chaiyosang et al., 2019). Recently, compound 35 was re-isolated from Talaromyces sp. IQ-313, an anthill soil-dwelling fungus around the Huasteca Hidalguense, Hidalgo State, Mexico. The cultivation of Talaromyces sp. IQ-313 was carried out in potato dextrose agar (PDA) and PDB (Jimenez-Arreolaa et al., 2020).
The cost of production and yield of duclauxin (1) on different culture media are different. The production cost of duclauxin (1) from P. herquei culture on Gostar media (peanut hulls and potato starch substrates) was less than enriched media. However, the yield of duclauxin (1) from Gostar medium (7.71 mg/flask) was less than enriched media (8.57 mg/flask). The cost comparison greatly favors the Gostar-type medium for this process (Bryant et al., 1993). Fourteen in vitro growth media were used to cultivate the fungi to produce duclauxin analogs in the present report, showing that fungi have different compounds on different artificial growth media under different conditions (Table 1).
Biological Activities of Duclauxin Metabolites
Chemical investigation of genera Talaromyces and Penicillium led to the isolation of 36 duclauxin analogs, in which only 22 compounds were evaluated for bioactivities. These 22 compounds showed multiple biological activities such as antimicrobial, antimalarial, enzyme inhibition, and antitumor activities. The distribution of bioactive compounds is described in Figure 7. In this section, the detailed bioactivities of duclauxin analogs were summarized, mainly focused on their activities against cancer, infectious disease, Alzheimer’s disease, diabetes, and malaria (Tables 3–6).
Antitumor Activities
According to the World Health Organization (WHO), cancer is a hazardous disease primarily caused by malignant tumors resulting from abnormal cell growth. Cancer is a leading cause of death around the globe; nearly 10 million people have died from cancer in 2020 worldwide (World Health Organization, 2021).1 Various techniques have been used to control cancer, such as radiotherapy, chemotherapy, and surgery. Among these techniques, chemotherapy is the most helpful approach to treat cancer so far (Farce et al., 2004). Therefore, finding effective antitumor agents from a natural source is necessary due to their lower toxicity.
Duclauxin (1), a metabolite that occurs naturally in fungi, is the most effective against numerous tumor cell lines because it prevents ATP synthesis by inhibiting mitochondrial respiration and therefore exhibits potential as a leading anticancer molecule. The antitumor activity of duclauxin (1) was evaluated in vitro against Ehrlich ascites carcinoma (EAC), lymphadenoma L-5178, and HeLa cells. The inhibitory effect was evaluated according to the decreased number of nucleic acids in tested tumor cells. It suppressed the growth of all tested tumor cell lines with cytotoxic effect ED50 from 20 to 50 μg/mL (Fuska et al., 1974). Duclauxin (1) was evaluated for the effect on murine leukemia L1210 culture cells, and it was strongly active with the ID50 value of ca. 3.5 μg/mL (6.6 μM) (Shiojiri et al., 1983). Similarly, duclauxin (1) was examined using an Agrobacterium tumefaciens potato disc assay for crown gall tumor/antitumor induction at the concentrations of 5 μg/disc, 10 μg/disc, 25 μg/disc, and 50 μg/disc, respectively. The inhibitory potential values at the given concentrations were –67, –74, –95, and –94%, respectively. This observation concurs that duclauxin (1) was an effective antitumor agent at the concentrations of 25 μg/disc (camptothecin, positive control, –100% at 25 μg/disc) (Bryant et al., 1994). Duclauxin (1) isolated from T. bacillisporus was intensely active against three human cancer cell lines (MCF-7, NCI-H460 and SF-286) with the following GI50 values of 15.0 ± 1.3, 40.3 ± 1.7, and 78.3 ± 1.9 μM, respectively. However, all the cancer cell lines were less inhibited by the positive control doxorubin with the GI50 values of 42.8 ± 8.2, 93 ± 7.0, and 94.0 ± 8.7 μM, respectively (Dethoup et al., 2006). The cytotoxicity of duclauxin (1) was recently examined against NS-1 (ATCC TIB-18) mouse myeloma cells and found to be inactive (Chaudhary et al., 2020; Table 3).
Duclauxamide A1 (22) isolated from P. manginii, exhibited moderate cytotoxicity against SW480, MCF-7, A-549, MCF-7 SMML-7721, and HL-60 cancer cell lines with inhibitory concentration (IC50) values in the range of 11–32 μM (Cao et al., 2015). The cytotoxic properties of compounds 8, 9, 21, and 31 were determined against HeLa cell line by the MTT assay, in which cisplatin was used as a positive control. Only bacillisporin H (21) displayed modest cytotoxicity against the HeLa cell line with an IC50 value of 49.5 μM, while the other compounds were inactive (cisplatin, positive control, IC50: 10.6 ± 6.6 μM) (Zang et al., 2016b). Biological evaluation against HeLa cell line showed that talaroketals A and B (18–19) exhibited moderate cytotoxicity with IC50 values 36 ± 2.0 μM and 44 ± 2.0 μM, respectively (cisplatine, positive control, IC50: 5.6 ± 2.0 μM) (Zang et al., 2016a). Kawai et al. (1985) assessed the in vitro cytotoxicity effect of xenoclauxin (15) against murine leukemia (L-1210 culture cells), and it exhibited the strongest inhibitory activity by causing a complete growth inhibition at approximately 20 μM. Compound 15 exhibited the same mode of inhibition as that of duclauxin on the ATP synthesis in mitochondria, displaying uncoupling of oxidative phosphorylation and inhibition of state 3 respiration (Kawai et al., 1985; Table 3).
Oxaphenalenones 2–3, 7, and 30 were screened for in vitro cytotoxicity against three human cancer cell lines MCF-7, NCI-H460, and SF-286. Among them, bacillisporin A (2) showed strong growth inhibitory effects against MCF-7 and NCI-H460 with GI50 values of 10.2 ± 0.9 and 7.9 ± 0.3 μM, respectively. It also exhibited a moderate GI50 on SF-268 with a value of 14.7 ± 0.3 μM. Bacillisporins B (3) and C (30) also showed an average mild growth inhibitory effect against all cancer cell lines (MCF-7, NCI-H460, and SF-286) with GI50 from 15.0 ± 1.3 to 78.3 ± 1.9 μM. However, bacilisporin E (7) exhibited a weak activity compared to the positive control and other tested compounds. GI50 values of these compounds were determined by the National Cancer Institute in vitro anticancer molecule discovery screen using protein-binding dye sulforhodamine B (SRB) (Skehan et al., 1990; Dethoup et al., 2006). In another study, bacillisporins A-B (2–3) and duclauxamide B (23) were evaluated against cancerous (MCF-7 and NCI-H187) and non-cancerous (Vero) cells. Only compound 2 displayed notable activity against the MCF-7 cell line with an IC50 value of 35.56 μM as compared to positive control tamoxifen (IC50, 27.64 μM), while compound 3 demonstrated weak cytotoxicity against both cancerous and non-cancerous cells with IC50 values ranging from 78.06 to 103.12 μM. In contrast, duclauxamide B (23) demonstrated moderate cytotoxicity exclusively against NCI-H187 with IC50 35.58 μM (ellipticine, positive control, IC50: 9.87 μM, Table 3; Dramae et al., 2020).
Macrosporusone B (33) exhibited significant cytotoxic activity against NCI-H187 (human small cell lung cancer) and primate (Vero) cells with the IC50 values of 16.73 and 13.74 μM, respectively (ellipticine, positive control, IC50: 9.26 and 3.45 μM). At the same time, bacillisporin G (31) showed remarkable cytotoxic activity against NCI-H187, MCF-7 (human breast adenocarcinoma), KB (human epidermoid carcinoma in the mouth) and primate (Vero) cell lines with IC50 values of 7.29, 9.16, 5.86, and 7.50 μM, respectively. In this study, ellipticine was used as a positive control, displayed cytotoxicity againstNCI-H187, KB, and Vero cells with the IC50 values of 9.26, 13.80, and 3.45 μM, respectively. The IC50 value of standard control doxorubicin against the MCF-7 cell line was 17.44 μM. This information concurs that compound 31 might be used as a leading hit against the cancer lines. Furthermore, compounds 2, 3, 14–15, and 32 exhibited weak cytotoxicity against Vero cells with IC50 values in the range of 33.55–93.27 μM. In comparison, the KB cell line was slightly inhibited by compound 9 with the IC50 of 33.55 μM (ellipticine, positive control, IC50: 13.80 μM). Resazurin microplate assay (REMA) was employed to perform the activity against NCI-H187, MCF-7, and KB cell lines. In addition, cytotoxicity against Vero cells was tested using the green fluorescent protein (GFP) detection method (Chaiyosang et al., 2019). Talaromycesones A (16) and B (35) isolated from marine fungus Talaromyces sp. LF458 were evaluated for cytotoxic activity against HepG2 (human hepatocellular liver carcinoma cell line) and NIH 3T3 (mouse fibroblasts cell line). Both compounds were inactive against tested cancer cell lines (Wu et al., 2015; Table 3).
Antimicrobial Activities
Antimicrobial resistance (AMR) has emerged as one of the most serious human health concerns of the twenty-first century. The Center for Disease Control and Prevention (CDC) reported that 23,000 people die every year in developing countries due to AMR. The emergence of multidrug-resistant strains, such as methicillin-resistant Staphylococcus aureus (MRSA), significantly contributes to AMR incidence. Antimicrobials are the most effective way to protect human beings from infectious diseases (Basak et al., 2016; Cruz et al., 2020; Health Research and Educational Trust, 2020).2 Thus, fungi-derived secondary metabolites are a potential pool for antimicrobial agent screening, which holds a great promise for new antibiotics.
Duclauxin (1) was evaluated against pathogenic bacteria and fungi using the 96-well microtiter plate method. However, it was not active against Bacillus subtilis ATCC 6633, Escherichia coli ATCC 25922, Candida albicans ATCC 10231, and Saccharomyces cerevisiae ATCC 9763, respectively (Chaudhary et al., 2020). Bacillisporins A (2) and B (3) were inactive against Acinetobacter baumannii, Escherichia coli, Pseudomonas aeruginosa, and Enterococcus faecium at a concentration of 50 μg/mL, but bacillisporin A (2) possessed potent antibacterial activity against Gram-positive bacteria such as S. aureus and B. cereus with the same MIC value of 3.13 μg/mL (vencomycin, positive control, MIC: 2.0 and 1.0 μg/mL). However, bacillisporin B (3) exhibited moderate antibacterial activity against B. cereus and S. aureus with MIC values of 6.25 and 12.50 μg/mL, respectively (vencomycin, positive control, MIC: 2.0 and 1.0 μg/mL), while bacillisporin C (30) displayed weak antibacterial activity against both strains with MIC values of 25.0 and 50.0 μg/mL, respectively. Duclauximide B (23), a N-containing oxaphenalenone dimer, displayed moderate inhibitory activity against Mycobacterium tuberculosis, S. aureus and B. cereus with the same MIC value of 12.5 μg/mL. The standard positive control values against Mycobacterium tuberculosis, S. aureus and B. cereus are listed in Table 4 (Dramae et al., 2020). In another report, bacillisporins A (2) and B (3) were tested for antimicrobial activities against B. cereus, Sarcina lutea, S. albus, E. coli, and Salmonella, using the microplate assay method. Both compounds displayed strong inhibitory activities against B. subtilis with the same MIC values of 0.13 ± 0.02 μM, whereas the MIC value of compound 2 against Salmonella was 2.00 ± 0.02 μM (Huang et al., 2017). Substantial antibacterial activities against clinically relevant pathogenic bacterial strains S. epidermidis DSM 20044 and methicillin-resistant S. aureus (MRSA) DSM 18827 were observed for talaromycesone A (16) with an IC50 3.70 and 5.4 μM, respectively (chloramphenicol, positive control, IC50, 1.81 and 2.46 μM). At the same time, talaromycesone B (35) showed moderate inhibitory effects against S. epidermidis and S. aureus with IC50 values of 17.36 and 19.50 μM, respectively (Wu et al., 2015; Table 4).
Compounds 2, 3, 7, 9, 15, 31–33, and 35 isolated from T. macrosporus KKU-1NK8, evaluated for antibacterial activity against Gram-positive (B. cereus ATCC 11778, S. aureus ATCC 6538, MRSA) and Gram-negative bacteria (E. coli ATCC 25922, P. aeruginosa ATCC 27853, and Salmonella enterica serovar Typhimurium ATCC 13311). Among them, bacillisporins A (2) and E (7) displayed the most vigorous antibacterial activities against B. cereus with MIC of 1.94 and 3.76 μM, respectively. Therefore, they can be used as anti-B. cereus candidate molecule due to their intense effects compared to standard drugs vancomycin (MIC 1.38 μM) and kanamycin (MIC 4.13 μM), respectively. Moreover, compound (3) was slightly active against Gram-positive bacteria, while the other tested compounds were inactive against Gram-positive and Gram-negative bacteria. The dilution method was used to evaluate the minimum inhibitory concentrations (MICs), as described in the M07-A9 of the Clinical and Laboratory Standards Institute (Balouiri et al., 2016; Chaiyosang et al., 2019; Table 4).
Talaroketals A (18) and B (19) were examined for their antimicrobial activities against four strains such as E. coli ATCC 8739, S. aureus ATCC 6538, S. haemolyticus MNHN26, and Enterococcus faecalis CIP 103014. These compounds exhibited weak antimicrobial activity against S. aureus with an IC50 value of around 50 μg mL–1 but no action against the other bacterial strains (Zang et al., 2016a). Bacillisporins A (2), F (9), G (31), and H (21) were evaluated against human pathogenic bacteria E. coli ATCC 8739, S. aureus ATCC 6538, S. hemolyticus MNHN26, and E. faecalis CIP 103014. Among them, bacillisporins A (2) and H (21) revealed potent antimicrobial activity against S. hemolyticus with MIC values of 9.5 ± 0.4 and 20.4 ± 6.5 μg/mL, respectively. Tetracycline, the positive control (MIC 29.5 ± 0.3 μM), was less active than the compounds 2 and 21. The MIC value of bacillisporin H (21) against S. aureus was 5.0 μg/mL, and the other compounds displayed weak activity against the panel of human pathogenic bacteria (Zang et al., 2016b; Table 4).
Enzyme Inhibition Activities
Alzheimer’s disease is a progressive neurodegenerative disease that causes brain cells to waste away and die. It is characterized by progressive cognitive deterioration and continuous decline in thinking, behavioral, and social skills that disrupts a person’s ability to function independently (Khan et al., 2016). Around 50 million people are affected by Alzheimer’s disease across the globe, and it is likely to present an increasing demand for the innovation of small molecules for this type of dementia (World Health Organization, 2020).3 Acetylcholinesterase (AChE) is an enzyme that catalyzes acetylcholine hydrolysis. Inhibition of this enzyme leads to an increased level of the neurotransmitter acetylcholine, which improves cholinergic functions in patients (Li et al., 2019). Talaromycesone A (16), isolated from the broth and mycelia of a marine fungus Talaromyces sp. LF458 was the first oxaphenalenone structure that exhibited AChE inhibitory activity with an IC50 of 7.49 μM (huperzine, positive control, IC50 11.6 μM). Therefore, compound 16 is a potential AChE inhibitor that could be a candidate molecule against Alzheimer’s disease (Figure 8; Wu et al., 2015).
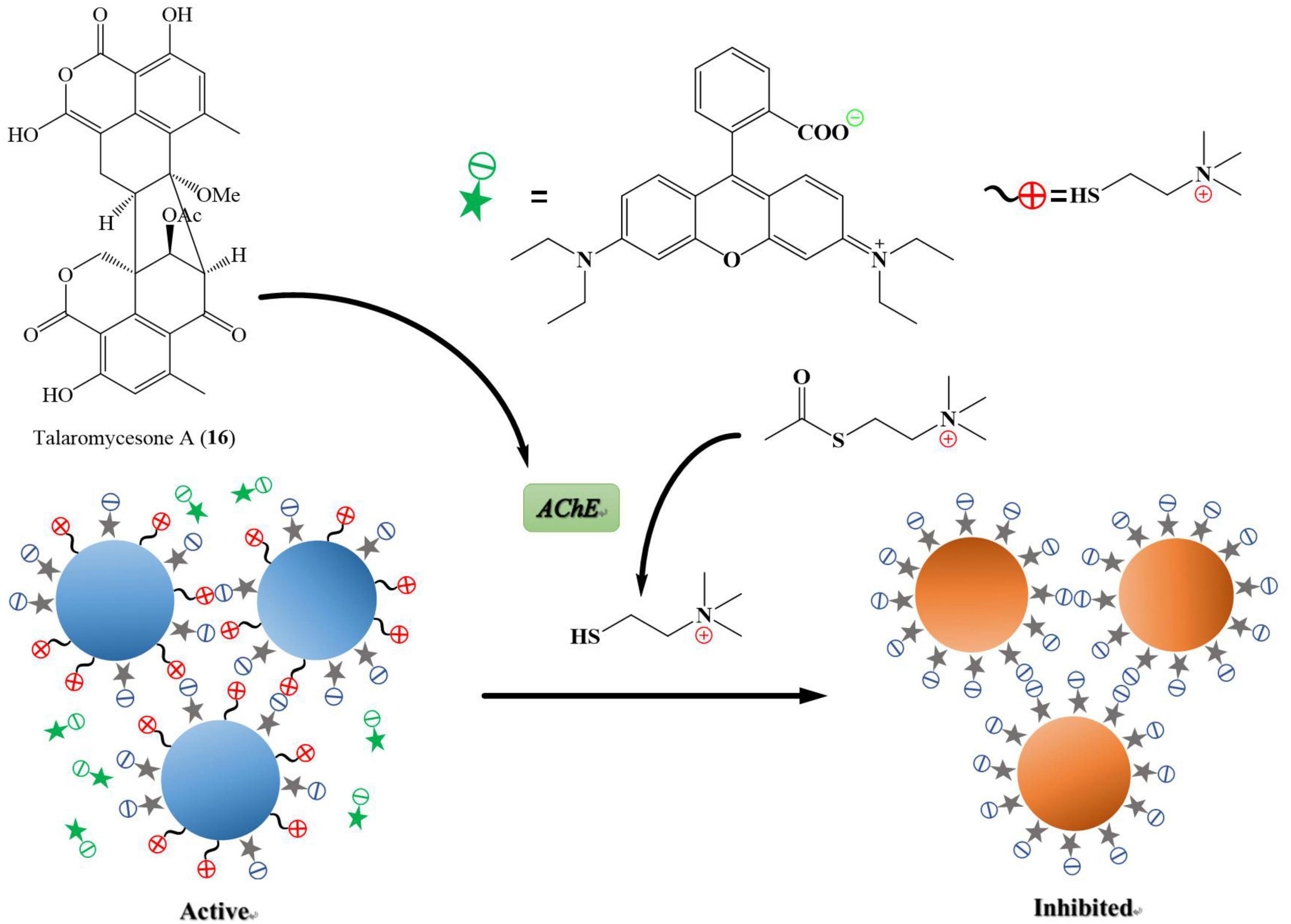
Figure 8. Acetylcholinesterase (AChE) is an enzyme that catalyzes acetylcholine into choline and acetate, resulting in the termination of synaptic transmission, responsible for Alzheimer’s disease. AChE is an enzymatic target for the treatment of Alzheimer’s disease. It has been shown in Figure 7 that the compound named talaromycesone A (16) inhibited the enzyme AChE from breaking down acetylcholine into choline and acetate.
Bacillisporins A (2) and B (3) produced by T. aculeatus exhibited potent inhibitory activity against α-glucosidase enzyme with IC50 values of 33.55 ± 0.63 and 95.81 ± 1.12 μM, respectively (acarbose, positive control, IC50 1075.53 ± 11.94 μM). The α-glucosidase inhibition activity of these compounds was determined using the method described by Jeon et al. (2013) with slight modifications (Huang et al., 2017; Table 4). Novel molecules against α-glucosidase are urgently needed because of their essential role in glucose absorption in the gastrointestinal tract, the inhibition of which prevents postprandial hyperglycemia. Compounds 2 and 3 are potential candidates of lead compounds for the treatment of type II diabetes.
Oligophenalenone dimers such as 1, 9, 15, 17, and 20 were assayed for their effects against cell division cycle 25B (CDC25B) tyrosine phosphatase. Among them, verruculosin A (20), bacillisporin F (9), and xenocluaxin (15) showed strong inhibitory activity against CDC25B with IC50 values of 0.38 ± 0.03, 0.40 ± 0.02, and 0.26 ± 0.06 μM, respectively (Na3VO4, positive control, IC50 0.52 ± 0.02 μM). The CDC25B regulates cell cycle progression in various cancer cell lines and has contributed to tumor growth. However, the specific mechanism by which increased CDC25B impacts tumor progression is not clear. The results indicated that oligophenalenone dimers might be used for screening as the new CDC25B inhibitor candidates. In addition, compounds 1, 9, 15, 17, and 20 displayed weak inhibitory activity toward epidermal growth factor receptor (EGFR) tyrosine kinase with IC50 values ranging from 0.92 ± 0.25 to 4.41 ± 2.32 μM (afatinib, positive control, IC50 0.0005 ± 0.00002 μM) (Wang et al., 2019; Table 5).
A critical biological event that contributes to the appearance and progress of cancer and diabetes is the reversible phosphorylation of proteins, a process controlled by protein tyrosine phosphatase (PTPs). Within the PTPs, PTP1B has gained significant interest from a pharmacological point of view since it is an emerging therapeutic target in treating cancer and diabetes. Until now, more than 300 PTP1B1–300 inhibitors have been identified from nature, including microorganisms and plants (Verma et al., 2017). Particularly, chemical investigation of a soil fungus Talaromyces sp. IQ-313 has resulted in the isolation of many different types of PTP1B inhibitors, such as duclauxin (1), bacillisporin G (31), and xenoclauxin (15), which showed potent inhibitory activity toward human protein tyrosine phosphatase 1B (hPTP1B1–400) in a concentration-dependent manner with IC50 values of 12.7, 13.4, and 21.8 μM, respectively (Ursolic acid, positive control, IC50 26.7 μM). These compounds induce conformational changes in the protein, which directly affect its activity, suggesting these compounds are allosteric modulators of the protein. Thus, compounds 1, 15, and 31 have great potential to treat obesity and chronic diseases such as diabetes and cancer (Jimenez-Arreolaa et al., 2020; Table 5).
Antimalarial and Other Activities
Malaria is a parasitic disease caused by Plasmodium spp. resulting from the bites of infected mosquitoes. Approximately 220 million people are infected by malaria, resulting in 435,000 deaths worldwide per annum. The emergence of drug-resistant malaria strains to classical synthetic drugs requires a continuous search for new compounds from alternative niches to introduce new and efficient therapies (Cadamuro et al., 2021). Bacillisporin G (31) and macrosporusone B (33) produced by the fungus T. macrosporus displayed weak antimalarial activity against Plasmodium falciparum (IC50 values were 8.07 and 10.28 μM, respectively) in comparison with positive control dihydroartemisinin (IC50 value was 0.003 μM) (Chaiyosang et al., 2019). It was observed that duclauxin (1) significantly inhibited the growth of etiolated wheat coleoptiles in a concentration-dependent manner. The percentage of inhibition at selected concentrations of duclauxin relative to controls were: 100% at 10–3 M, 80% at 10–4 M, 39% at 10–5 M, and 0% at 10–6 M (Bryant et al., 1993; Table 6).
Biosynthesis of Duclauxin Derivatives
The biosynthetic investigations of duclauxin and its derivatives have been partially clarified. Gao and co-workers studied the biosynthesis of duclauxin (1) at the molecular level and identified the respective responsible biosynthetic gene clusters in P. herquei and T. stipitatus (Gao et al., 2016, 2018). Briefly, biosynthesis of duclauxin (1) includes three extensive redox steps: (i) The phenalenone (38) is biosynthesized through redox reactions from an acetyl-CoA and malonyl-CoAs as the first key intermediate in duclauxin biosynthesis; (ii) A series of oxidordeuctases catalyze redox reactions on phenalenone (38) to yield the second key intermediate oxaphenalenone (SF226, 40); (iii) Dimerization of SF226 and/or lamellicolic anhydride can yield duclauxin (1) and its derivatives as shown in Figure 9.
The phenalenone (38) is a key intermediate in duclauxin biosynthesis (Figure 9). Phenalenone (38) is an aromatic polyketide with a 6/6/6 ring system essential in biosynthetic studies. In P. herquei, a gene cluster named phn was associated with phenalenone (38) production, and only two genes in this cluster, phnA and phnB, were sufficient for the formation of phenalenone (38). phnA encoding a non-reducing polyketide synthase, which has a domain architecture of SAT-KS-MAT-PT-ACP-ACP-TE-CLC (Gao et al., 2016), used 6 malonyl-CoAs and an acetyl-CoA as building blocks to generate the prephenalenone (37) core. During this process, the PT domain of phnA catalyzed the aldol condensation of C4-C9 cyclization of the polyketide chain, and the TE/CLC domain was responsible for C1-C10 Claisen condensation (Gao et al., 2016). After the formation of prephenalenone (37), a FAD-dependent hydroxylase encoded by phnB was used to form phenalenone (38) (Gao et al., 2016). Using phenalenone (38) as an essential precursor or intermediate, enzymes encoded by downstream genes facilitate redox reactions on phenalenone to yield diversified duclauxin analogs. In T. stipitatus, a gene cluster named dux1 possessed two counterparts to phnA and phnB: duxI and duxE, and they were likewise responsible for phenalenone formation. In this gene cluster, some additional genes were also found, including predicted P450 monooxygenases (DuxD and DuxL), oxidoreductase (DuxB), NAD(P)H-dependent reductases (DuxA, DuxG, and DuxJ), and a cupin family oxygenase (DuxM), transcription factor (DuxC), hydrolase (DuxH), and O-methyltransferase (DuxK) (Gao et al., 2018). Firstly, DuxM catalyzed phenalenone to another important intermediate 39, 39 can be further transformed to lamellicolic anhydride (41) by DuxM. Secondly, a series of sequential redox reactions catalyzed by DuxJ, DuxD and DuxG took place on 39 to yield SF226 (40), another key building block of duclauxin and its analogs (Gao et al., 2018). After the formation of oxaphenalenone (40) and lamellicolic anhydride (41), mediated by P450 monooxygenase DuxL through a diradical-coupling mechanism, two oxaphenalenones (40) formed dimer 42, which can finally nonenzymatically convert to bacillisporin C (30), or possibly enzymatically convert to 43 (Gao et al., 2018; Liu et al., 2021). However, further functional experiments are required to prove it. Intriguingly, in T. stipitatus, two identical genes (duxB and duxB’) were involved in transforming 43 to duclauxin (1). In comparison, a molecule of oxaphenalenone (40) and a molecule of lamellicolic anhydride (41) can finally lead to the formation of cryptoclauxin (13) (Gao et al., 2018).
Zang et al. (2016a) proposed the biosynthetic pathway for talaroketals A and B (18 and 19). In short, the upper 6/6/6 tri-ring system of duclauxin (1) can be modified into duclauxin derivatives (Figure 9). For example, a nucleophilic species can react with the upper ring to give the spiroketal moiety of talaroketal A (18) and the fused ketal part of talaroketal B (19) (Zang et al., 2016a). In another case, the upper moiety present in the structure of heptacyclic duclauxin (1) can react with different amino acids to yield talauxins Q, L, and I (26, 28–29), which could happen without the presence of specific enzymes (Figure 9; Chaudhary et al., 2020). The amino acids present in these talauxins possess hydrophobic and hydrophilic side chains, indicating that other amino acids probably react with duclauxin (1) to produce more talauxin derivatives.
Conclusion and Prospect
Over the past few years, about 36 duclauxin derivates have been identified from at least 9 fungal species mainly distributed in the genera Penicillium and Talaromyces (T. duclauxii, T. aculeatus, T. stipitatus, T. bacillisporus, T. verruculosus, T. macrosporus, P. herquei, P. manginii, and Talaromyces sp.). These fungal species were isolated from various niches, including soil, mangroves, plants endophytes, and marine ecosystem. According to the latest nomenclature and phylogenetic analysis, Penicillium species classified in the subgenus Biverticillium belong to genus Talaromyces. The species such as P. duclauxii, P. aculeatum, and P. stipitatum involved in duclauxins isolation are now named T. duclauxii, T. aculeatus and T. stipitatus, respectively. In recent years, significant strides of progress have been made in deciphering the mechanism and functions of duclauxin and its derivatives. Numerous studies showed that duclauxin derivatives revealed various significant biological activities, including antitumor, antimicrobial, and enzyme inhibition. Talaromycesone A (16), isolated from marine fungus Talaromyces sp. LF458, is the first oxaphenalenone with potent AChE inhibitory activity, signifying its potential for further research against Alzheimer’s disease. Similarly, bacillisporin A (2) is critically important to the development of leading hit for the treatment of type II diabetes, owing to its inhibitory activity against α-glucosidase, an enzyme responsible for postprandial hyperglycemia. Until now, to the best of our knowledge, total synthesis has not been successfully achieved to construct the delicate architectures of duclauxin derivatives. However, in this review, important information was provided in discovering essential genes and enzymes responsible for the biosynthesis of duclauxin, which would be helpful to achieve novel duclauxin derivatives biosynthetically.
Modern experimental approaches and computing tools are expected to unravel mechanisms of action (MOA) of duclauxins. Techniques such as RNA-seq and bioinformatics would provide a theoretical basis for the MOA study of duclauxins. Research to date indicates that more profound studies are needed to understand the biosynthetic pathway, metabolic regulation, and structure-activity relationship of duclauxins. Lastly, the physiological and ecological roles of the duclauxin metabolites and their practical applications in medicine, forestry, and agriculture will be confidently expected.
Author Contributions
HS, PD, and TS performed the review design. TC and YW performed the drawing of the compounds structure. HS and YY constructed the phylogenetic tree. HS and TS completed the manuscript draft. YW, CZ, and ZM finished the review and editing. All authors have read and agreed to the published version of the manuscript.
Funding
This research was co-financed by the National Natural Science Foundation of China (32071766), the Natural Science Foundation of Guangdong Province (2019A1515011554), the Key Research, Development Projects of Guangdong Province (2020B020214001), and the National College Students’ Innovation and Entrepreneurship Training Program (202110564061).
Conflict of Interest
The authors declare that the research was conducted in the absence of any commercial or financial relationships that could be construed as a potential conflict of interest.
Publisher’s Note
All claims expressed in this article are solely those of the authors and do not necessarily represent those of their affiliated organizations, or those of the publisher, the editors and the reviewers. Any product that may be evaluated in this article, or claim that may be made by its manufacturer, is not guaranteed or endorsed by the publisher.
Acknowledgments
We thank to Jun Wang for the productive suggestions. We are very grateful and appreciate Zhuo Shang (University of Western Australia, Australia), Babar Hassan (University of Sunshine Coast, Australia), Juniad Zafar (South China Agricultural University, China), and Muhammad Zaryab Khalid (University of Agriculture Faisalabad, Pakistan) for their constructive efforts on the manuscript.
Footnotes
- ^ https://www.who.int/news-room/fact-sheets/detail/cancer
- ^ www.hret-hiin.org
- ^ https://www.who.int/news-room/fact-sheets/detail/dementia
References
Abbas, M., Elshahawi, S. I., Wang, X., Ponomareva, L. V., Sajid, I., Shaaban, K. A., et al. (2018). Puromycins B–E, naturally occurring amino-nucleosides produced by the Himalayan isolate Streptomyces sp, PU-14G. J. Nat. Prod. 81, 2560–2566. doi: 10.1021/acs.jnatprod.8b00720
Balouiri, M., Sadiki, M., and Ibnsouda, S. K. (2016). Methods for in vitro evaluating antimicrobial activity. A review. J. Pharm. Anal. 6, 71–79. doi: 10.1016/j.jpha.2015.11.005
Barbero, M., Artuso, E., and Prandi, C. (2018). Fungal anticancer metabolites: synthesis towards drug discovery. Curr. Med. Chem. 25, 141–185. doi: 10.2174/0929867324666170511112815
Basak, S., Singh, P., and Rajurkar, M. (2016). Multidrug resistant and extensively drug resistant bacteria: a study. J. Pathog. 2016, 1–5. doi: 10.1155/2016/4065603
Bryant, F. O., Cutler, H. G., and Jazyno, J. M. (1993). Properties and cost effective method for production of the antitumor agent duclauxin from sporulating Penicillium herquei. J. Pharm. Sci. 82, 1214–1217. doi: 10.1002/jps.2600821206
Bryant, F. O., Cutler, H. G., and Parker, S. R. (1994). Effect of fungal natural products in an Agrobacterium tumefaciens potato disc assay. J. Nat. Prod. 57, 640–643. doi: 10.1021/np50107a012
Cadamuro, R. D., da Silveira Bastos, I. M. A., da Silva, I. T., da Cruz, A. C. C., Robl, D., Sandjo, L. P., et al. (2021). Bioactive compounds from mangrove endophytic fungus and their uses for microorganism control. J. Fungi 7, 455–473. doi: 10.3390/jof7060455
Cai, J., Zhou, X. M., Yang, X., Tang, M. M., Liao, Q. Y., Meng, B. Z., et al. (2020). Three new bioactive natural products from the fungus Talaromyces assiutensis JTY2. Bioorg. Chem. 94:103362. doi: 10.1016/j.bioorg.2019.103362
Calixto, J. B. (2019). The role of natural products in modern drug discovery. An. Acad. Bras. Cienc. 91, 1678–2690. doi: 10.1590/0001-3765201920190105
Cao, P., Yang, J., Miao, C. P., Yan, Y., Ma, Y. T., Li, X. N., et al. (2015). New duclauxamide from Penicillium manginii YIM PH30375 and structure revision of the duclauxin family. Org. Lett. 17, 1146–1149. doi: 10.1002/chin.201529258
Chaiyosang, B., Kanokmedhakul, K., Sanmanoch, W., Boonlue, S., Hadsadee, S., Jungsuttiwong, S., et al. (2019). Bioactive oxaphenalenone dimers from the fungus Talaromyces macrosporus KKU-1NK8. Fitoterapia 134, 429–434. doi: 10.1016/j.fitote.2019.03.015
Chaudhary, N. K., Crombie, A., Vuong, D., Lacey, E., Piggott, A. M., and Karuso, P. (2020). Talauxins: hybrid phenalenone dimers from Talaromyces stipitatus. J. Nat. Prod. 83, 1051–1060. doi: 10.1021/acs.jnatprod.9b01066
Cruz, J. S., da Silva, C. A., and Hamerski, L. (2020). Natural products from endophytic fungi associated with Rubiaceae Species. J Fungi 6:128. doi: 10.3390/jof6030128
Dethoup, T., Manoch, L., Kijjoa, A., Nascimento, M., Puaparoj, P., Silva, A., et al. (2006). Bacillisporins D and E, new oxyphenalenone dimers from Talaromyces bacillisporus. Planta Med. 72, 957–960. doi: 10.1055/s-2006-947188
Dramae, A., Intaraudom, C., Bunbamrung, N., Saortep, W., Srichomthong, K., and Pittayakhajonwut, P. (2020). Heptacyclic oligophenalenones from the soil fungus Talaromyces bacillisporus BCC17645. Tetrahedron 76:130980. doi: 10.1016/j.tet.2020.130980
Farce, A., Loge, C., Gallet, S., Lebegue, N., Carato, P., Chavatte, P., et al. (2004). Docking study of ligands into the colchicine binding site of tubulin. J. Enzym. Inhib. Med. Chem. 19, 541–547. doi: 10.1080/14756360412331280545
Fleming, A. (1929). On the antibacterial action of cultures of a Penicillium, with special reference to their use in the isolation of B. influenzae. Br. J. Exp. Pathol. 10, 226–236. doi: 10.1093/clinids/2.1.129
Fuska, J., Kuhr, I., Nemec, P., and Fuskova, A. (1974). Antitumor antibiotics produced by Penicillium Stipitatum Thom. J. Antibiot. 27, 123–127. doi: 10.7164/antibiotics.27.123
Gao, S. S., Duan, A., Xu, W., Yu, P., Hang, L., Houk, K. N., et al. (2016). Phenalenone polyketide cyclization catalyzed by fungal polyketide synthase and flavin-dependent monooxygenase. J. Am. Chem. Soc. 138, 4249–4259. doi: 10.1021/jacs.6b01528
Gao, S. S., Zhang, T., Garcia-Borràs, M., Hung, Y. S., Billingsley, J. M., Houk, K. N., et al. (2018). Biosynthesis of heptacyclic duclauxins requires extensive redox modifications of the phenalenone aromatic polyketide. J. Am. Chem. Soc. 140, 6991–6997. doi: 10.1021/jacs.8b03705
Health Research and Educational Trust (2020). Multi-Drug Resistant Organism Infection Change Package: 2017 Update. Chicago. Available online at: www.hret-hiin.org (accessed September 20, 2020).
Houbraken, J., Kocsubé, A. S., Visagie, C. M., Yilmaz, N., Wang, X. C., Meijer, M., et al. (2020). Classification of Aspergillus, Penicillium, Talaromyces and related genera (Eurotiales): an overview of families, genera, subgenera, sections, series and species. Stud. Mycol. 95, 5–169. doi: 10.1016/j.simyco.2020.05.002
Huang, H. R., Liu, T., Wu, X. E., Guo, J. X., Lan, X., Zhu, Q., et al. (2017). A new antibacterial chromone derivative from mangrove-derived fungus Penicillium aculeatum (No. 9EB). Nat. Prod. Res. 31, 2593–2598. doi: 10.1080/14786419.2017.1283498
Imhoff, J. F. (2016). Natural products from marine fungi—still an underrepresented resource. Mar. Drugs 14, 19. doi: 10.3390/md14010019
Jeerapong, C., Phupong, W., Bangrak, P., Intana, W., and Tuchinda, P. (2015). Trichoharzianol, a new antifungal from Trichoderma harzianum F031. J. Agric. Food Chem. 63, 3704–3708. doi: 10.1021/acs.jafc.5b0125
Jeon, S. Y., Oh, S., Kim, E., and Imm, J. Y. (2013). α-Glucosidase inhibiton and antiglycation activity of laccasecatalyzed catechin polymers. J. Agric. Food Chem. 61, 4577–4584. doi: 10.1021/jf400791r
Jimenez-Arreolaa, B. S., Aguilar-Ramireza, E., Cano-Sancheza, P., Morales-Jimenezb, J., Gonzalez-Andradec, M., Medina-Francod, J. L., et al. (2020). Dimeric phenalenones from Talaromyces sp. (IQ-313) inhibit hPTP1B1-400. Bioor. Chem. 101:103893. doi: 10.1016/j.bioorg.2020.103893
Kawai, K., Shiojiri, H., Nakamaru, T., Nozawa, Y., Sugie, S., Mori, H., et al. (1985). Cytotoxicity and genotoxicity of xenoclauxin and desacetyl duclauxin from Penicillium duclauxii (Delacroix). Cell Biol. Tonicol. 1, 1–10. doi: 10.1007/BF00717786
Khan, M. M., Ahsan, F., Ahmad, U., Akhtar, J., and Badruddeen</snm>, and Mujahid, M. (2016). Alzheimer disease: a review. World J. Pharm. Pharm. Sci. 5, 649–666. doi: 10.20959/wjpps20166-7045
Kuhr, I., Fuska, J., Sedmera, P., Podojil, M., Vokoun, J., and Vanek, Z. (1973). An antitumor antibiotic produced by Penicillium stipitatum Thom, its identity with duclauxin. J. Antibiot. 26, 535–536. doi: 10.7164/antibiotics.26.535
Li, Z., Ma, N., and Zhao, P. J. (2019). Acetylcholinesterase inhibitory active metabolites from the endophytic fungus Colletotrichum sp. YMF432. Nat. Prod. Res. 33, 1794–1797. doi: 10.1080/14786419.2018.1434648
Lima, M. A., de Oliveira, M. C., Pimenta, A., and Uchoa, P. (2019). Aspergillus niger: a hundred years of contribution to the natural products chemistry. J. Braz. Chem. Soc. 30, 2029–2059. doi: 10.21577/0103-5053.20190080
Liu, J. W., Liu, A. A., and Hu, Y. C. (2021). Enzymatic dimerization in the biosynthetic pathway of microbial natural products. Nat. Prod. Rep. 38, 1469–1505. doi: 10.1039/d0np00063a
Mao, Z., Zhang, W., Wu, C., Feng, H., Peng, Y., Shahid, H., et al. (2021). Diversity and antibacterial activity of fungal endophytes from Eucalyptus exserta. BMC Microbiol. 21:155. doi: 10.1186/s12866-021-02229-8
Newman, D. J., and Cragg, G. M. (2016). Natural products as sources of new drugs from 1981 to 2014. J. Nat. Prod. 79, 629–661. doi: 10.1021/acs.jnatprod.5b01055
Newman, D. J., and Cragg, G. M. (2020). Natural products as sources of new drugs over the nearly four decades from 01/1981 to 09/2019. J. Nat. Prod. 83, 770–803. doi: 10.1021/acs.jnatprod.9b01285
Ogihara, Y., Iitaka, Y., and Shibata, S. (1968). The crystal and molecular structure of monobromoduclauxin. Acta Crystallogr. 24, 1037–1047. doi: 10.1107/S0567740868003675
Ogihara, Y., Tanaka, O., and Shibata, S. (1966). On the metabolites of Penicillium duclauxi (Delacroix). III. The reaction of duclauxin with ammonia and primary amines. The structure of desacetylduclauxin, neoclauxin, xenocluxin, and cryptoclauxin. Tetrahedron Lett. 7, 2867–2873. doi: 10.1016/S0040-4039(01)99876-4
Rahman, M., and Sarker, S. D. (2020). Antimicrobial natural products. Annu. Rep. Med. Chem. 55, 77–113. doi: 10.1016/bs.armc.2020.06.001
Shan, T., Wang, Y., Wang, S., Xie, Y., Cui, Z., Wu, C., et al. (2020). A new p-terphenyl derivative from the insect-derived fungus Aspergillus candidus Bdf-2 and the synergistic effects of terphenyllin. PeerJ 8:e8221. doi: 10.7717/peerj.8221
Shibata, S., Ogihara, Y., Tokutake, N., and Tanaka, O. (1965). Duclauxin, A metabolite of Penicillium Duclauxi (Delacroix). Tetrahedron Lett. 18, 1287–1288. doi: 10.1016/S0040-4039(00)77197-8
Shiojiri, H., Kawai, K., Kato, T., Ogihara, Y., and Nozawa, Y. (1983). Cytotoxicities on culture cells and inhibitory effects on mitochondrial respiration by iluuclauxin and related compounds. Proc. Jpn. Assoc. Mycotoxicol. 1983, 38–41. doi: 10.2520/myco1975.1983.18_38
Singh, N., and Chandra, R. (2014). “Endophytes: unexplored reservoir of bioactive natural products,” in Handbook of Medicinal Plants and their Bioactive Compounds, ed. N. Gupta (Kerala: Research Signpost), 1–10.
Skehan, P., Storeng, R., Scudiero, D., Monks, A., McMahon, J., and Vistica, D. (1990). New colorimetric cytotoxicty assay for anticancer drug screening. JNCI J. Natl. Cancer Inst. 82, 1107–1112. doi: 10.1093/jnci/82.13.1107
Torres-Mendoza, D., Ortega, H. E., and Cubilla-Rios, L. (2020). Patents on endophytic fungi related to secondary metabolites and biotransformation applications. J. Fungi 6:58. doi: 10.3390/jof6020058
Tsang, C. C., Tang, J. Y. M., Lau, S. K. P., and Woo, P. C. Y. (2018). Taxonomy and evolution of Aspergillus, Penicillium and Talaromyces in the omics era - Past, present and future. Comput. Struct. Biotechnol. J. 16, 197–210. doi: 10.1016/j.csbj.2018.05.003
Verma, M., Gupta, S. J., Chaudhary, A., and Garg, V. K. (2017). Protein tyrosine phosphatase 1B inhibitors as antidiabetic agents - A brief review. Bioorg. Chem. 70, 267–283. doi: 10.1016/j.bioorg.2016.12.004
Wang, M., Yang, L., Feng, L., Hu, F., Zhang, F., Ren, J., et al. (2019). Verruculosins A–B, new oligophenalenone dimers from the soft coral - derived fungus Talaromyces verruculosus. Mar. Drugs 17:516. doi: 10.3390/md17090516
World Health Organization (2021). Fact Sheets. Cancer. Available online at: https://www.who.int/news-room/fact-sheets/detail/cancer (accessed September 21, 2021).
World Health Organization (WHO) (2020). Fact Sheets. Dementia. Available online at: https://www.who.int/news-room/fact-sheets/detail/dementia (accessed September 21, 2020).
Wu, B., Ohlendorf, B., Oesker, V., Wiese, J., Malien, S., Schmaljohann, R., et al. (2015). Acetylcholinesterase inhibitors from a marine fungus Talaromyces sp. strain LF458. Mar. Biotechnol. 17, 110–119. doi: 10.1007/s10126-014-9599-3
Yamazaki, M., and Okuyama, E. (1980). Isolation and structures of oxaphenalenone dimers from Talaromyces bacillisporus. Chem. Pharm. Bull. 28, 3649–3655. doi: 10.1248/cpb.28.3649
Zang, Y., Genta-Jouve, G., Retailleau, P., Escargueil, A., Mann, S., Nay, B., et al. (2016a). Talaroketals A and B, unusual bis(oxaphenalenone) spiro and fused ketals from the soil fungus Talaromyces stipitatus ATCC 10500. Org. Biomol. Chem. 14, 2691–2697. doi: 10.1039/c5ob02657a
Keywords: duclauxin derivatives, fungi, secondary metabolites, biological activities, biosynthesis
Citation: Shahid H, Cai T, Wang Y, Zheng C, Yang Y, Mao Z, Ding P and Shan T (2021) Duclauxin Derivatives From Fungi and Their Biological Activities. Front. Microbiol. 12:766440. doi: 10.3389/fmicb.2021.766440
Received: 13 October 2021; Accepted: 06 December 2021;
Published: 22 December 2021.
Edited by:
Maria Jorge Campos, Polytechnic Institute of Leiria, PortugalReviewed by:
Sebastian Simonetti, Consejo Nacional de Investigaciones Científicas y Técnicas (CONICET), ArgentinaGuo-Dong Chen, Jinan University, China
Copyright © 2021 Shahid, Cai, Wang, Zheng, Yang, Mao, Ding and Shan. This is an open-access article distributed under the terms of the Creative Commons Attribution License (CC BY). The use, distribution or reproduction in other forums is permitted, provided the original author(s) and the copyright owner(s) are credited and that the original publication in this journal is cited, in accordance with accepted academic practice. No use, distribution or reproduction is permitted which does not comply with these terms.
*Correspondence: Ping Ding, ZGluZ3BpbmdnekAxMjYuY29t; Tijiang Shan, dGpzaGFuc2NhdUAxNjMuY29t