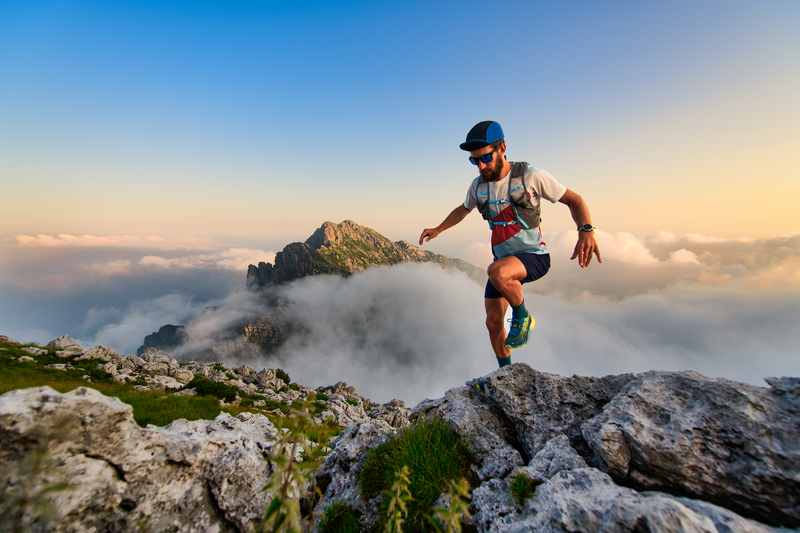
95% of researchers rate our articles as excellent or good
Learn more about the work of our research integrity team to safeguard the quality of each article we publish.
Find out more
ORIGINAL RESEARCH article
Front. Microbiol. , 12 February 2019
Sec. Microbiotechnology
Volume 10 - 2019 | https://doi.org/10.3389/fmicb.2019.00089
This article is part of the Research Topic Microbial Ecotoxicology View all 42 articles
Microcosm experiments with CE-contaminated groundwater from a former industrial site were set-up to evaluate the relationships between biological CE dissipation, dehalogenase genes abundance and bacterial genera diversity. Impact of high concentrations of PCE on organohalide respiration was also evaluated. Complete or partial dechlorination of PCE, TCE, cis-DCE and VC was observed independently of the addition of a reducing agent (Na2S) or an electron donor (acetate). The addition of either 10 or 100 μM PCE had no effect on organohalide respiration. qPCR analysis of reductive dehalogenases genes (pceA, tceA, vcrA, and bvcA) indicated that the version of pceA gene found in the genus Dehalococcoides [hereafter named pceA(Dhc)] and vcrA gene increased in abundance by one order of magnitude during the first 10 days of incubation. The version of the pceA gene found, among others, in the genus Dehalobacter, Sulfurospirillum, Desulfuromonas, and Geobacter [hereafter named pceA(Dhb)] and bvcA gene showed very low abundance. The tceA gene was not detected throughout the experiment. The proportion of pceA(Dhc) or vcrA genes relative to the universal 16S ribosomal RNA (16S rRNA) gene increased by up to 6-fold upon completion of cis-DCE dissipation. Sequencing of 16S rRNA amplicons indicated that the abundance of Operational Taxonomic Units (OTUs) affiliated to dehalogenating genera Dehalococcoides, Sulfurospirillum, and Geobacter represented more than 20% sequence abundance in the microcosms. Among organohalide respiration associated genera, only abundance of Dehalococcoides spp. increased up to fourfold upon complete dissipation of PCE and cis-DCE, suggesting a major implication of Dehalococcoides in CEs organohalide respiration. The relative abundance of pceA and vcrA genes correlated with the occurrence of Dehalococcoides and with dissipation extent of PCE, cis-DCE and CV. A new type of dehalogenating Dehalococcoides sp. phylotype affiliated to the Pinellas group, and suggested to contain both pceA(Dhc) and vcrA genes, may be involved in organohalide respiration of CEs in groundwater of the study site. Overall, the results demonstrate in situ dechlorination potential of CE in the plume, and suggest that taxonomic and functional biomarkers in laboratory microcosms of contaminated groundwater following pollutant exposure can help predict bioremediation potential at contaminated industrial sites.
Extensive industrial use of halogenated volatile organic compounds (VOC) such as tetrachloroethylene (PCE) and trichloroethylene (TCE) has resulted in widespread environmental contamination of soil and groundwater worldwide (Huang et al., 2014). Groundwater at industrial sites producing or using halogenated VOC is often contaminated by chlorinated ethenes (CEs), generally in mixture with other contaminants (Aktaş et al., 2012). Once released into the environment, CEs migrate through the unsaturated zone of the subsurface (vadose zone), and can accumulate in aquifers as a dense non-aqueous phase liquid (DNAPL) due to their solubility, density, and hydrophobicity. Diffusion and transport of CEs in groundwater may result in a contamination plume often characterized by pollutant and redox gradients determining in situ biotransformation (Haack et al., 2004).
Organohalide respiration of CEs occurs under both oxic and anoxic conditions (Vogel and McCarty, 1985; Freedman and Gossett, 1991; Maymó-Gatell et al., 1999; Beeman and Bleckmann, 2002; Atashgahi et al., 2017). Under anoxic conditions, CEs may serve as electron acceptors for microbial metabolism (Holliger et al., 1998). Upon reductive dechlorination, PCE can be enzymatically converted, sequentially to TCE, cis-DCE, VC, and finally to non-toxic ethene. As the number of chlorine substituents decreases, dechlorination rate usually slows down with accumulation of less chlorinated DCE and VC (Abe et al., 2009; Chang et al., 2017).
Bioremediation and monitored natural attenuation are promising approaches for monitoring and removing chlorinated solvents from contaminated aquifers, due to their efficiency, sustainability and relatively low costs (Da Silva and Alvarez, 2008; Kang, 2014; Patil et al., 2014). Biostimulation studies for field remediation of chlorinated ethenes have been recently reported (Santharam et al., 2011; Florey et al., 2017; Sheu et al., 2018). Many bacterial strains capable of reductive dehalogenation of CEs have been characterized (Atashgahi et al., 2016). They typically belong to the genera Dehalococcoides, Dehalobacter, Desulfitobacterium, Sulfurospirillum, and Geobacter. These genera have thus been suggested as potential bioindicators of dechlorination in aquifers (Löffler et al., 2000; Hendrickson et al., 2002; Duhamel and Edwards, 2006; Imfeld et al., 2008; Clark et al., 2018). While many strains of organohalide respiration associated genera are involved in one or several organohalide respiration steps of halogenated VOC, complete reductive dehalogenation of PCE to ethene has only been observed for some strains of the Dehalococcoides genus (Maymó-Gatell et al., 2001; He et al., 2003; Muller et al., 2004; Sung et al., 2006b). Dechlorinating capacities of Dehalococcoides strains may, however, differ widely (Magnuson et al., 1998; Lee et al., 2008). At the functional level, various genes encoding different types of reductive dehalogenases are involved in specific steps of sequential CE dechlorination (Figure 1) (Futagami et al., 2008; Hug, 2016; Saiyari et al., 2018). The dehalogenation of PCE to TCE and DCE (mainly cis-1,2-DCE) by non-obligatory dehalogenating bacteria is encoded by pceA genes. In Dehalococcoides, PCE is dechlorinated to TCE by strains also carrying a pceA gene (Magnuson et al., 1998). More recent findings highlighted the diversity of genes encoding PCE reductive dehalogenase in Dehalococcoides; pteA gene (PCE to TCE) in Dehalococcoides mccartyi strain 11a5 (Zhao et al., 2017), pcbA genes (PCE to TCE and DCE) in three distinct PCB-dechlorinating Dehalococcoides strains (Wang et al., 2014), and mbrA gene in strain MB that produce mainly trans-1,2-DCE (Cheng and He, 2009; Chow et al., 2010). The next steps, i.e., TCE to DCE, VC and ethene, only occur in Dehalococcoides strains and are encoded by three genes, tceA (TCE to DCE), vcrA or bvcA (DCE to CV and ethene) (Zinder, 2016).
Figure 1. Organohalide respiration pathways of chloroethenes, associated bacteria and reductive dehalogenase genes (based on Futagami et al., 2008; Hug, 2016; Zhao et al., 2017; Saiyari et al., 2018).
Knowledge of microbial and gene diversities associated with organohalide respiration of CEs has contributed to develop biomolecular approaches to evaluate biological dehalogenation and guide remediation strategies at contaminated sites (Maphosa et al., 2010; Dugat-Bony et al., 2012). Such approaches mainly rely on sensitive detection and quantification of specific dehalogenating taxa, notably Dehalococcoides (Hendrickson et al., 2002; Lu et al., 2006; Rouzeau-Szynalski et al., 2011; Kranzioch et al., 2013) or of functional genes used as biomarkers of dehlorespiration (Behrens et al., 2008; Da Silva and Alvarez, 2008; Rahm and Richardson, 2008; Carreon-Diazconti et al., 2009; Kranzioch et al., 2013). However, interpretation of currently available biomarkers and identification of novel ones requires a better understanding of the relationship between bacterial taxa associated with organohalide respiration of CEs and genes involved in dechlorination steps (Maphosa et al., 2012).
Here we examined the potential of endogenous groundwater bacterial communities of a former industrial site contaminated with CEs to degrade PCE and other CEs in relation to bacterial diversity and functional genes associated with reductive dechlorination of CEs. The aim was to evaluate the relationships between biological CE dissipation, dehalogenase gene abundance and bacterial genera diversity that could be transposed as biomarkers to the field. The effect of high additions of PCE on organohalide respiration rate and associated bacterial diversity was also addressed. The experimental setup consisted of laboratory microcosms containing groundwater from a multi-contaminated former industrial site. The experimental set-up was designed to evaluate the effect of PCE concentration, and of addition of a carbon source (acetate) and a reducing agent (Na2S) on changes of specific genera associated with organohalide respiration, and reductive dehalogenases genes pceA, tceA, vcrA, and bvcA.
Groundwater samples were taken from the former industrial site of Themeroil (Varennes-le-Grand, France, GPS coordinates, 46.701141 N, 4.843919 E) characterized by historical intensive oil and solvent processing activities. Halogenated solvents and BTEX were released into the site aquifer due to inappropriate storage methods (BRGM, 1998), resulting in high concentrations of chlorinated VOC (mainly CEs) and BTEX, accumulated within DNAPL in the groundwater of the site. The pollutant plume extends over an area of about 5 ⋅ 10-2 km2 (BRGM, 2011). Low concentrations of PCE (20 μg ⋅ L-1) and TCE (11 μg ⋅ L-1) in the contaminant plume compared to the contamination source and the prevalence of cis- over trans-DCE (Table 1) suggest the production of cis-DCE from PCE and TCE reductive dehalogenation and its accumulation (Nijenhuis et al., 2007).
Piezometer Pz6(10) located in the contaminant plume (Supplementary Figure S1) was selected based upon favorable redox conditions regarding CEs reductive dehalogenation (dissolved oxygen concentration below 0.05 ppm and redox potential about -242 mV) (Table 1). Major CE contaminants were cis-DCE (34.8 mg ⋅ L-1) and VC (7.8 mg ⋅ L-1). Pz6(10) was characterized by high sulfate (128 mg ⋅ L-1) and Fe(III) (7.4 mg ⋅ L-1) and low nitrate (<1 mg ⋅ L-1) aqueous concentrations. Thirty liters of Pz6(10) groundwater were sampled in June 2015 with a Twister pump (Proactive, Bradenton, United States) at a depth of 5 m, after purging the piezometer and ensuring that Eh, pH, and conductivity were constant. Groundwater was stored for 30 days at 4°C until microcosm set-up.
Pz6(10) groundwater was pre-incubated to favor bacterial reductive dechlorination of PCE and increase reaction kinetics. Pz6(10) groundwater (800 mL) was pre-incubated in a 1 L bottle (Schott DURAN®, Germany) with 8 mL of a 30 g ⋅ L-1 NH4HCO3 solution (0.30 g ⋅ L-1 final concentration), 8 mL of a 25 g ⋅ L-1 K2HPO4 solution (0.25 g ⋅ L-1), 8 mL of a 1 g ⋅ L-1 MgSO4 ⋅ 7H2O solution (0.1 g ⋅ L-1); 800 μL of an 80 g ⋅ L-1 CaCl2 solution (0.08 g ⋅ L-1), 500 μL of a 100 μg.L-1 vitamin B12 solution (62 μg ⋅ L-1), 2.4 mL of a 1 M sodium acetate solution (3 mM; 246 mg ⋅ L-1), 640 μL of a 40 g.L-1 sodium sulfide solution (32 mg ⋅ L-1), 1 mL of a 20 g.L-1 yeast extract solution (25 mg ⋅ L-1) and 2.6 μL of pure PCE (99.9%, Sigma-Aldrich) solution (20 μM final concentration). The bottle was hermetically closed with a GL45 teflon-coated bottle cap (Omnifit®), conditioned with a H2/N2 atmosphere (5%/95%) and incubated at 20°C in the dark without shaking. After complete dissipation of PCE to TCE (30 days), this pre-culture served as inoculum to the microcosm experiment.
Microcosms consisted of 800 mL of Pz6(10) groundwater, inoculated with the pre-culture at a ratio of 1:10 in 1 L glass bottles (Schott®) capped with GL45 teflon-coated bottle caps (Omnifit®). Sample handling was performed in a glovebox under a nitrogen atmosphere. Two sets of microcosms were prepared in triplicates with Pz6(10) groundwater and amended with pure PCE (99.9%, Sigma-Aldrich) to obtain final PCE concentrations of, respectively, 10 and 100 μM using a glass syringe (Hamilton Bonaduz AG, Bonaduz, Switzerland). For each condition, microcosms were amended or not with sodium acetate (3 mM final concentration) and sodium sulfide solution (Na2S, 0.4 mM final concentration). Killed controls consisted of site water sterilized by autoclaving (twice at 121°C for 30 min with a 24 h break between cycles), with no or 10 μM PCE added after autoclaving. The microcosms were placed under H2/N2 (5%/95%, 0.5 bar) atmosphere and incubated in the dark at 20°C under agitation (80 rpm). Water samples were taken from microcosms for hydrochemical and DNA analyses immediately after set up (day 0), and after 3, 5, 7, 10, 13, 19, 35, and 55 days of incubation.
pH and redox potential (Eh) were measured from aqueous phase microcosm aliquots using a portable probe (Multi 340i, WTW Instrument) at all sampling times in a N2-purged glove box. Acetate, sulfate, nitrate and chloride were measured at days 5, 10, 19, and 35 in filtered samples (0.22 μm syringe filters, Millipore, United States) by ionic chromatography (Dionex DX-100 equipped with AS19HC column). Quantification limits were 0.1 mg ⋅ L-1 for acetate and 0.5 mg ⋅ L-1 for sulfate, nitrate and chloride. Sulfide was measured after 10 and 35 days of incubation with a Merck Spectroquant kit 114779 (Merck, Germany).
Concentrations of CEs (PCE, TCE, cis-DCE and VC) were determined in 5 mL samples collected at all times. CEs were measured using a gas chromatograph (CP-3008-GC Varian, Walnut Creek, CA, United States) equipped with a headspace sampler and flame ionization detector. Chromatographic separation was performed in a capillary column (Agilent DB-624, 30 m, 0.32 mm inside diameter, 1.80 μm film thickness). Injector and detector temperatures were held at 250 and 300°C, respectively, and the following temperature program was used: hold at 35°C for 5 min, heating to 245°C (10°C/min) and hold for 10 min. Concentrations were determined using external standards (R2 = 0.99). The limit of quantification was 20 μg ⋅ L-1 for all CEs.
Dissipation rates (μM.d-1) for individual CEx were estimated as an average of individual time step as follows:
Where DisCE is the dissipation rate in μM.d-1 and x is the number of chlorine atom substituents for each CEx and n the number of incubation days.
Fifteen mL of microcosm groundwater were sampled at each sampling time and filtered through 0.22 μm sterile membrane filters (∅ 2.5 cm, Millipore, United States). Membranes were stored at -20°C until DNA extraction. DNA was extracted using the FastDNA® Spin Kit for Soil (MP Biomedicals, United States) and the Fastprep® instrument according to the manufacturer’s instructions, with minor modifications (30 s lysis at a speed setting of 5.0, subsequent centrifugation of cell debris for 25 min). Extracted total DNA was quantified using the Quantifluor dsDNA sample kit and the Quantus fluorimeter according to the manufacturer’s instructions (Quantus, Promega, United States).
Quantification of the bacterial 16S rRNA gene and of reductive dehalogenase genes (pceA, tceA, vcrA, and bvcA) was performed by qPCR using a CFX ConnectTM Real-Time PCR Detection System (Bio-Rad, France) with primers and programs listed in Table 2. qPCR reactions were performed in a total volume of 20 μL, with a master mix containing 7.6 μL of RNase- and DNase-free water (MP Biomedicals, CA, United States), 10 μL of SYBR Green IQ Supermix (Bio-Rad), 500 nM of each primer, and 2 μL of template DNA (concentrations of total DNA ranging from 0.1 ng ⋅μL-1 to 5 ng ⋅μL-1). Sterile, nuclease-, RNA- and DNA-free water was added instead of DNA in no template controls (NTC). All samples, controls and standards were analyzed in duplicate. A calibration curve was obtained from serial dilutions of a known quantity of linearized plasmids containing known copy numbers of 16S rrnA, pceA(Dhc), pceA(Dhb), tceA, vcrA, and bvcA gene fragments, respectively. Gene concentrations were reported as gene copies per mL of groundwater preparation. Limits of quantification (LOQ) were 3.2 ⋅ 103 gene copies ⋅ mL-1 culture for the 16S rRNA gene, 6.7 ⋅ 102 gene copies ⋅ mL-1 for pceA, tceA, and vcrA genes, and 6.7 ⋅ 101 gene copies ⋅ mL-1 for bvcA, respectively (Supplementary Table S1). Generation of a specific PCR product was confirmed by melting curve analyses and agarose gel electrophoresis. The effect of PCR inhibitors in DNA was estimated using successive dilutions of the DNA extract mixed with known amounts of DNA standard (pGEM-T easy vector, Promega) for qPCR with vector-specific primers as previously described (Miyata et al., 2010). No PCR inhibition was detected.
For CE-SSCP bacterial community fingerprinting, the V3 region of the 16S rRNA gene was amplified by PCR from DNA extracts with forward primer w49 (5′-ACGGTCCAGACTCCTACGGG-3′) and 5′ FAM-labelled reverse primer w34 (5′-TTACCGCGGCTGCTGGCAC-3′) (Delbès et al., 2001), by 30 s hybridisation at 61°C, and 30 s elongation at 72°C for 28 cycles as described previously. One μL of diluted PCR product (5- to 100-fold in nuclease-free water) was then added to a mixture of 18.6 μL of deionized formamide and 0.4 μL of Genescan-600 LIZ internal DNA standard (Life Technologies, United States). To obtain single-strand DNA, samples were heat-denatured for 10 min at 95°C, and immediately cooled on ice. CE-SSCP analyses were performed on an ABI Prism 310 genetic analyser using a 47-cm long capillary, a non-denaturing 5.6% CAP polymer (Life technologies, United States) and the following electrophoresis conditions: run temperature 32°C, sample injection for 5 s at 15 kV, and data collection for 35 min at 12 kV. Alignment of the profiles using an internal DNA standard and assignment of peak positions were performed with Bionumerics software (Applied Maths, Belgium).
Four samples collected from microcosms on days 0, 5, 10, and 34 were selected for 16S rRNA gene amplicon high-throughput sequencing based on chemical variations and community composition changes as preliminary detected by CE-SSCP analysis according to the distribution and the area of the peaks over time (p < 0.05). For each sample, DNA extracted from biological replicates (n = 3) was pooled for amplification of V4-V5 hypervariable region (Claesson et al., 2010) using an optimized and standardized amplicon-library preparation protocol (Metabiote®, GenoScreen, Lille, France). A positive [artificial bacteria community comprising 17 different bacteria (ABCv2)] and a negative (sterile water) control were also performed. Briefly, PCR reactions were performed using 5 ng of genomic DNA and 192 fusion barcoded primers (at 0.2 μM final concentrations), with an annealing temperature of 50°C for 30 cycles. PCR products were purified using Agencourt AMPure XP magnetic beads (Beckman Coulter, Brea, CA, United States), quantified according to GenoScreen’s protocol, and mixed in an equimolar amount. Sequencing was performed using 250-bp paired-end sequencing chemistry on the Illumina MiSeq platform (Illumina, San Diego, CA, United States) at GenoScreen (Lille, France). The sequence information was deposited to NCBI Sequence Read Archive (SRA) under accession numbers SRP160122.
Raw paired-end reads were demultiplexed per sample and subjected to the following process:
(1) search and removal of both forward and reverse primers using CutAdapt, with no mismatches allowed in the primers sequences;
(2) quality-filtering using the PRINSEQ-lite PERL script (Schmieder and Edwards, 2011), by truncating bases at the 3′ end with Phred quality score <30;
(3) paired-end read assembly using FLASH (Magoč and Salzberg, 2011), with a minimum overlap of 30 bases and >97% overlap identity.
Taxonomic and diversity analysis were performed with the Metabiote Online v2.0 pipeline (GenoScreen, Lille, France) which is based in part on QIIME software v 1.9.1 (Caporaso et al., 2010). Following pre-processing, full-length 16S rRNA gene sequences were checked for chimera sequences (in-house method based on Usearch 6.1). Similar sequences with a nucleic identity defined threshold (97% identity for an affiliation at the genus level on the V4–V5 regions of the 16S rRNA gene) were clustered with Uclust v1.2.22q (Edgar, 2010) through an open-reference Operational Taxonomic Units (OTU) picking process and complete-linkage method, finally generating groups of sequences or “Operational Taxonomic Units” (OTUs). An OTU cleaning step involving elimination of singletons was performed. The most abundant sequence of each OTU was considered as the reference sequence of its OTU and taxonomically compared to a reference database included in the Greengenes database (release 13_81; DeSantis et al., 2006) by the RDP classifier method v2.2 (Cole et al., 2014). Alpha-diversity metrics (Chao1 index) within samples were computed using QIIME v 1.9.1.
Univariate statistical analyses (Student test, ANOVA) were performed with XLSTAT (Version 2016.02.27390). Multivariate analyses [principal component analysis (PCA)] were performed within R2. Bacterial community composition data obtained from Illumina MiSeq sequencing were analyzed by PCA ordination. Data were first normalized using Hellinger transformation (Legendre and Gallagher, 2001; Ramette, 2007). Explanatory variables consisted of quantitative variables including: CEs concentrations (and sum of total CE) (mg ⋅ L-1), pH, redox potential (mV) temperature (°C), sulfate, chloride and acetate concentrations (mg ⋅ L-1), gene copies number (copies ⋅ mL-1) pceA(Dhc), pceA(Dhb), vcrA and bvcA to 16S rRNA gene ratios (%). Explanatory variables were standardized to provide dimensionless variables and remove undue influence of magnitude differences between scales or units. The relationship between community profiles and biogeochemical variables was investigated by fitting environmental vectors a posteriori onto the PCA. Their significance was assessed with a 1000-step Monte-Carlo permutation test. Significance was inferred at p < 0.05.
16S rRNA gene was amplified with Dehalococcoides specific primers using DNA extracted from the 10 μM_PCE_T8 experiment: forward primer Fp DHC 1 (5′-GATGAACGCTAGCGGCG-3′) and reverse primer Rp DHC 1377 (5′-GGTTGGCACATCGACTTCAA-3′) (Hendrickson et al., 2002), by 30 s hybridisation at 66°C, and 3 min elongation at 72°C for 40 cycles. Then, ligation and transformation were realized according to pGEM-T® easy vector (Promega, United States) protocol. After 37°C overnight incubation on LB media, one plasmid was purified according to NucleoSpin® Plasmid Columns protocol (Macherey-Nagel, Germany). Forward and reverse sequencing was realized by Sanger sequencing on ABI3730XL at GenoScreen (France). Consensus sequence was created from forward and reverse sequences and aligned by ClustalW method thanks to BioEdit v7.0.5.3 (Hall, 1999) with 18 NCBI sequences: 17 sequences of the 16S rRNA gene of Dehalococcoides strains and Desulfitobacterium hafniense DCB-2 strain. Finally, phylogeny was performed on 923 nucleotides with SeaView v4.7 (Gouy et al., 2010) with BioNJ - JC distance method, a 1,000 bootstrap value and without ignoring GAPs.
Redox potential (Eh) values decreased from -130 mV to -350 mV in all microcosms (data not shown), thus confirming suitable conditions for reductive dechlorination (Löffler et al., 2013). The pH in all microcosms remained between 6.9 and 7.9 during the experiment, close to the measured in situ Pz6-(10) groundwater pH of 7.0.
In all microcosms except killed controls, PCE was nearly completely dissipated within 5 days under both 10 and 100 μM PCE spiking conditions (Figure 2 and Supplementary Figure S2). TCE was only partially dissipated, and no further dissipation was observed after 20 days. In contrast, cis-DCE decreased continuously throughout the incubation, down to the detection limit after 60 days for both PCE spiking doses. Transient build-up of VC was observed, with VC first decreasing until day 10, and then increasing until day 35, before decreasing again. This may reflect VC formation from cis-DCE dechlorination with only limited VC dissipation until day 35. Sulfate initially present in these microcosms was completely reduced to sulfide after 10 days (Supplementary Figure S3).
Figure 2. Dissipation of CEs in microcosms spiked with PCE and acetate. Concentrations of PCE (triangles), TCE (squares), cis-DCE (circles), VC (diamonds), and total chlorinated ethenes (white circles), in microcosms spiked with 10 μM PCE (A) or 100 μM PCE (B). Represented values are the average of three replicates. Error bars are not shown for clarity and were below 100% (see Supplementary Figure S1). Data from microcosms that received acetate but no Na2S are shown as a representative example. Results were very similar for other experimental conditions tested.
Maximum dissipation rates were estimated at (mean ± standard deviation) 30 ± 2 μM ⋅ day-1, 10 ± 5 μM ⋅ d-1 and 26 ± 5 μM ⋅ d-1 for PCE, cis-DCE and CV, respectively (Figure 3, results with acetate and no Na2S addition). Highest rates were observed over the 10 first days of incubation. The PCE dissipation rate was 10-fold higher in microcosms spiked with 100 μM PCE than in those spiked with 10 μM PCE. For other CEs, similar rates were observed in microcosms with 10 or 100 μM PCE. Dissipation rate of cis-DCE was lower than that of PCE (10 ± 5 μM.d-1) and decreased by 10 μM.d-1 after 20 days. VC was rapidly dissipated within the first 10 days, with a rate up to 25 μM.d-1, and then decreased to 5 μM.d-1 for the remaining incubation time.
Figure 3. Chlorinated ethene dissipation in microcosms spiked with PCE and acetate. Dissipation rates estimated for PCE (triangles), cis-DCE (circles), VC (diamonds), and total chlorinated ethenes (white circles) are given for microcosms spiked with 10 μM PCE (A) or 100 μM PCE (B).
Killed controls showed low dissipation of CEs (<20%, data not shown), confirming that dissipation was the result of microorganisms activity in non-sterile microcosms.
Addition of acetate and Na2S did not impact PCE dissipation kinetics. All complementary analyses were thus carried out from microcosms amended with acetate only (without Na2S, see Figures 2, 3), and all the results presented hereafter were obtained under those conditions.
Abundance of selected reductive dehalogenase genes (pceA, tceA, vcrA, and bvcA) and total bacterial abundance (assessed from the 16S rRNA gene) were determined by qPCR (Figure 4). Total bacterial abundance increased from 107 to 108 copies ⋅ mL-1 during the first 2 weeks of incubation under both PCE spiking doses, and then remained constant until the end of the experiment. The vcrA gene and the version of the pceA gene carried by Dehalococcoides [pceA(Dhc)], initially present at levels around 105 copies ⋅ mL-1 also increased by one order of magnitude under both PCE spiking conditions. Notably, increase in abundance of pceA(Dhc) occurred during PCE dissipation. Then, after 19 days of incubation, pceA(Dhc) gene copies decreased by one order of magnitude before stabilizing around 2.104 and 4.104 copies ⋅ mL-1 in microcosms spiked with 10 and 100 μM PCE, respectively. The ratio pceA(Dhc)/16S rrnA was relatively low at the beginning of the experiment (around 0.1% relative abundance). However, the ratio pceA(Dhc)/16S rrnA ratio increased during the incubation by at least one order of magnitude, up to 1 and 3.5% for microcosms spiked with 10 μM PCE and 100 μM PCE, respectively (Supplementary Figure S4). Abundance of the vcrA gene, potentially involved in the two organohalide respiration steps from cis-DCE to ethene, followed the same pattern as that of pceA(Dhc) (with a magnitude of about 0.4 log) over the duration of the experiment. The vcrA/16S rrnA ratio increased from about 2.5% relative abundance at the start of the experiment to 6% in microcosms spiked with 10 μM PCE and 12% in microcosms spiked with 100 μM PCE after 34 days of incubation (Supplementary Figure S4). Overall, changes of the abundance of pceA(Dhc) and vcrA genes compared to the total community as monitored using 16S rRNA gene as proxy (Figure 4) paralleled the dynamics observed for complete dissipation of cis-DCE and VC (Figure 2).
Figure 4. Biomarker genes in microcosms. Quantification of 16S rRNA gene (white circles), pceA(Dhc) (diamonds), pceA(Dhb) (squares), vcrA (black circles) and bvcA (triangles) genes in microcosms amended with acetate and spiked with 10 μM (A) or 100 μM (B) PCE. The tceA gene was not detected. Values represent the mean (±standard deviation) of three replicates. Quantification limits of qPCR analysis for functional genes are indicated by red lines. nd, not detected.
Surprisingly, the dehalogenase gene pceA(Dhb) involved in the organohalide respiration of PCE to cis-DCE by strains of Dehalobacter and Desulfitobacterium genera (Regeard et al., 2004) was less abundant than dehalogenases genes pceA(Dhc) and vcrA by two orders of magnitude (Figure 4). At about 103 copies ⋅ mL-1 at the beginning of the experiment, it remained above the limit of quantification (LOQ, 102 copies ⋅ mL-1) during the 10 first days of the experiment. It then decreased and remained below the LOQ. Similarly, the absence of detection of tceA gene was in keeping with the observed incomplete dissipation of TCE. As for gene bvcA involved in VC organohalide respiration, it was only detected at very low copy-numbers, and only during the first 10 days of incubation.
Changes in bacterial community were first assessed by CE-SSCP fingerprinting analyses for all microcosm experiments (i.e., with/without acetate, sodium sulfide and/or PCE). Only minor variations were apparent during the first 19 days. Distinct changes were then observed after 34 days (Supplementary Figure S5), depending on the PCE spiking dose.
Principal component analysis of CE-SSCP fingerprints confirmed the observed variations (Supplementary Figure S6). The first two principal components explained 38% of the variability, the first component (24%) being mainly associated with SSCP peaks only present during the first stages of the incubation. A posteriori fitting of the main physico-chemical variables and gene abundance data onto the PCA ordination plot suggested that these changes were associated to the decrease of PCE, cis-DCE and VC concentrations, and with the increase of pceA(Dhc) and vcrA dehalogenase gene abundances over time (Supplementary Figure S6). Microcosm samples at the beginning of the incubation (0 and 5 days), and at intermediate (10 days) and late (34 days) sampling times were selected for high-throughput sequence analysis of 16S rRNA gene amplicons to identify bacterial taxa present over time and potentially involved in successive steps of organohalide respiration of CEs.
A total of 412,231 high-quality sequences of the V4–V5 region of the 16S rRNA gene (∼350 bp) were obtained from separately pooled DNA extracted from triplicate microcosms spiked with 10 and 100 μM PCE at the selected four incubation times (0, 5, 10, and 34 days) (Supplementary Table S2). Rarefaction curves did not systematically reach saturation in the 1st days of incubation, although patterns of alpha diversity and overall relative abundances of dominant lineages could be retrieved (Supplementary Figure S8). In the later phase of incubation (10 and 34 days), in contrast, rarefaction curves of diversity indices reached saturation (Supplementary Figure S7), indicating a reduction of bacterial α-diversity through time in laboratory microcosms.
At the start of the experiment, genera Hydrogenophaga, Arcobacter, Dehalococcoides, Desulfovibrio, Sulfurospirillum, and Dechloromonas dominated the microcosms, and were found in similar proportions at both investigated concentrations of spiked PCE (Figure 5). On average, these genera represented 63 and 65% of the total number of sequences for microcosms spiked with 10 μM PCE and 100 μM PCE, respectively. Dominant genus-level taxa belong to Proteobacteria, except Dehalococcoides affiliated to Chloroflexi. Over the course of the experiment, Arcobacter (19.2–2.0%), Hydrogenophaga (8.1–0.76%), Dechloromonas (10.8–0.8%), and Geobacter (2.0–0.4%) strongly decreased under both PCE amendment conditions (Figure 5). On the other hand, several genus-level taxa showed significant increases in relative abundance, e.g., Desulfovibrio (8.2–56.0%) and Dehalococcoides (11.8–35.6%) (Figure 5).
Figure 5. Relative abundance of the 17 most abundant genus-level OTUs during CEs dissipation. Only data obtained for microcosms with acetate amendment were analyzed in detail. A total of 412,231 sequences of the V4–V5 region of the 16S rRNA gene were obtained by Illumina MiSeq and analyzed with Qiime software. 10 μM, 100 μM: concentrations of PCE added. OTUs with <1% abundance were clustered together.
High throughput sequencing of the 16S rRNA gene V4–V5 region revealed changes in taxa associated with dehalogenation coinciding with changes in bacterial community diversity (Figure 6). Using PCA analysis, the main PC1 axis explained 42% of community diversity change, and was mainly linked to cis-DCE concentration, abundance of pceA and vcrA genes and occurrence of Dehalococcoides. The second PC2 axis explained 30% of the variability in bacterial community diversity, and mainly reflect PCE and VC concentration changes. Only slight differences between microbial community patterns were observed in early stages of the experiment (0–5 days) between microcosms spiked with 10 μM or with 100 μM PCE. As already seen by CE-SSCP fingerprinting analysis, larger differences in community composition were observed at later stages (10–35 days) with both 10 and 100 μM PCE amendments.
Figure 6. Principal component analysis (PCA) ordination plot of bacterial communities during CEs dissipation. Largest contributions of bacterial taxa are represented by red arrows. Significant physico-chemical and biomolecular explanatory variables were fitted a posteriori on the PCA and are represented by gray arrows. Values on the axes indicate the percentage of the total variation explained by the corresponding axis (PC1, principal component axis 1; PC2, principal component axis 2). δ-prot., Deltaproteobacteria; Arc, Arcobacter; Dem, Dechloromonas; Dhc, Dehalococcoides; Dsv, Desulfovibrio; Gb, Geobacter; Hgp, Hydrogenophaga; Sul, Sulfurospirillum.
Worthy of note, 3 of the 17 most abundant genera at the beginning of the experiment significantly contributing to PCA ordination were potentially associated to reductive dechlorination (Figure 6), e.g., Dehalococcoides (10% average abundance), Sulfurospirillum (6.4% average abundance), and Geobacter (2.7% average abundance). Interestingly, Sulfurospirillum-related taxa increased in the early stages of incubation in microcosms spiked with 10 μM PCE (6.3–10.5% average abundance) as well as in microcosms spiked with 100 μM PCE (6.6–14.5% average abundance), and then decreased to about 4% under both PCE conditions. Relative abundance of Geobacter remained stable (2.0% average abundance with 10 μM PCE) or slightly increased (2.8–3.5% with 100 μM PCE) and then decreased, especially in microcosms with 100 μM PCE. Relative abundance of Acetobacterium taxa, also associated with reductive dehalogenation (Terzenbach and Blaut, 1994), remained stable (1.0% average abundance) at all sampling times and for both PCE amendments. In contrast, other taxa putatively associated to reductive dechlorination (all affiliated to the class of Dehalococcoidetes) were only found in minor proportions (<0.3% abundance) throughout the experiment (data not shown). Sequences associated with prominent dehalogenating taxa Dehalobacter and Desulfitobacterium were not found.
One of the most abundant taxa identified in microcosms is affiliated to Dehalococcoides, and more specifically to the Dehalococcoides Pinellas group (Figure 7) (Hug and Edwards, 2013). As Miseq sequencing do not allow accurate taxa affiliation down to genus level, the affiliation to Pinellas was confirmed by 16S rRNA gene sequence phylogeny on 923 nucleotides of the corresponding OTU (Genbank MK312632). Relative abundance of this taxon remained stable (about 10% abundance) in the initial phase of the experiment (see Figure 5), independently of PCE amendment. A subsequent decrease to 7% average abundance across microcosms was noted under both conditions, followed by a significant (four–sixfold) increase of Dehalococcoides abundance at a later stage (Figure 5). This paralleled the observed increase in pceA(Dhc) and vcrA genes found in Dehalococcoides (Figure 6).
Figure 7. Phylogenetic affiliation of OTU935 within genus Dehalococcoides. The tree was obtained by the Neighbor-Joining and Jukes and Cantor methods from a 923 nucleotides alignment of 16S rRNA gene sequences. OTU935 of the microcosm is displayed in bold. Dehalococcoides subgroup affiliation is also shown. Bootstrap values are expressed as the percentage of 1000 replications.
The main incentive for this study was to investigate whether laboratory microcosm studies can help identify specific dehalogenation reactions and associated bacterial taxa in groundwater contaminated with CEs that could then be stimulated for bioremediation. Abundances and dynamics of key dehalogenase genes (pceA, tceA, vcrA, and bvcA), bacterial community composition and dehalogenation-specific taxa through the 16S rRNA gene as proxy were thus inventoried in laboratory microcosms of groundwater contaminated with multiple chlorinated solvents (Table 2).
The experiment specifically focused on effects of PCE exposure (10 and 100 μM) on dissipation rates and abundances of selected biomarkers. Complete dissipation of PCE and cis-DCE (in sampled site water and following in labo PCE dissipation), as well as partial dissipation of TCE and VC, were observed in all microcosm experiments. Only minor dissipation of CEs was observed in killed controls, confirming the prevailing role of microorganisms. Notably, organohalide respiration occurred rapidly and independently of acetate and/or addition of a reducing agent (Na2S). This confirms that reductive dehalogenation likely proceeded through hydrogen supplied through the 5% H2/N2 atmosphere as the electron donor, and that sufficient carbon was available for biomass production from the groundwater and the preculture used as inoculum.
Monitoring of key reductive dehalogenase genes in microcosms showed a correlation with CE dissipation. It also suggested the dominant involvement of the “Pinellas” subgroup of the Dehalococcoides genus (Hug and Edwards, 2013) in the process (Figure 7). Dehalogenase genes pceA(Dhc) and vcrA were already abundant at the start of the experiment (Figure 4). Groundwater bacteria carrying these genes were likely enriched in the activated preculture prepared from Pz6(10) groundwater and used as inoculum. Upon laboratory incubation, pceA(Dhc) and vcrA dehalogenase genes abundance increased by one order of magnitude in the initial phase of the experiment, with concomitant complete dissipation of PCE and partial dissipation of cis-DCE and VC. This is in agreement with previous studies (Amos et al., 2008; Ise et al., 2011; Aktaş et al., 2012; Baelum et al., 2013). In addition, increase in the 16S rRNA gene suggested selective growth of dehalogenating strains during dechlorination of CEs (Figure 4). In the later phase of the experiment, no further increase of genes pceA(Dhc) and vcrA was observed (Figure 4), while cis-DCE and VC dissipation continued (Figure 2). This suggested that in the presence of hydrogen, dechlorination was supported by sufficient abundance of bacteria containing vcrA, as reported previously (Cupples et al., 2004).
Dehalococcoides strains may carry several different dehalogenase genes (Behrens et al., 2008). Furthermore, their co-occurrence in CE-degrading enrichment cultures derived from organohalide-contaminated sites has already been observed (Scheutz et al., 2008; Baelum et al., 2013; Kranzioch et al., 2015). Very similar variations and gene copy numbers observed for pceA(Dhc) and vcrA (Figure 4) suggest that these genes are associated with the same bacteria. However, pceA(Dhc) and vcrA genes are usually found in different strains. Dehalococcoides sp. strain VS, the only bacterium reported to date that carries both pceA and vcrA genes (Muller et al., 2004; Behrens et al., 2008; Lee et al., 2008), is able to dechlorinate PCE to TCE or cis-DCE to ethene, but not TCE to cis-DCE (Lee et al., 2008). A comparable situation was encountered here, with complete dehalogenation of PCE and cis-DCE but only partial dissipation of TCE (Figure 2). However, the only Dehalococcoides-related OTU identified in microcosms by high-throughput sequencing (Figure 5) is affiliated to the Pinellas group and therefore appears phylogenetically distinct from that of the VS strain, which belongs to the Victoria group (Nishimura et al., 2008) (Figure 7). These results, together with patterns of dehalogenase genes pceA and vcrA, lead to the hypothesis that a new strain of a dehalogenating Dehalococcoides sp. containing both pceA(Dhc) and vcrA genes is involved in organohalide respiration of CEs in groundwater from the Themeroil site.
Abundance data of other dehalogenase genes also allows some conclusions to be drawn. For instance, low abundance of genes pceA(Dhb) and bvcA suggests an only minor involvement of PCE- and TCE-degrading bacteria carrying these dehalogenases, such as Desulfitobacterium and Dehalobacter strains (Suyama et al., 2002; Daprato et al., 2007; Rupakula et al., 2013). Similarly, Dehalococcoides phylotypes identified in this study likely did not carry the bvcA gene for VC organohalide respiration, unlike other known representatives of this genus (Krajmalnik-Brown et al., 2004). Finally, failure to detect the tceA gene, together with TCE accumulation in microcosms, suggests the absence of TCE-dehalogenating Dehalococcoides sp. Worthy of note, this contrasts with the low TCE concentration (Table 1), and detection of genes pceA(Dhb) and tceA in groundwater when measured directly on-site (data not shown). Hence, growth of tceA-containing bacteria may be limited under the chosen laboratory microcosm conditions.
Investigations of the dynamics of bacterial composition as a function of CE organohalide respiration in microcosms may help to associate observed patterns of dehalogenation with bacterial taxa identified in microcosms. CE-SSCP fingerprinting analyses proved useful for initial characterisation of bacterial community dynamics in microcosm experiments, and allowed to select key samples for taxonomic characterisation of communities by Illumina MiSeq sequencing of 16S rRNA gene amplicons. Despite being less precise than sequencing, CE-SSCP analysis yielded a similar picture of the main determinants shaping the evolution of the bacterial community in microcosms (Supplementary Figure S6) to high-throughput sequencing (Figure 5).
Overall, microcosms sustained diverse bacterial populations capable of different terminal electron-accepting processes. Bacterial communities were dominated by Proteobacteria and Chloroflexi, as previously reported for chlorinated hydrocarbon-contaminated groundwater (Abbai and Pillay, 2013; Kotik et al., 2013). A large proportion of recovered microorganisms was putatively associated with iron and sulfate reduction, in agreement with physico-chemical conditions in microcosms (Table 1). Only a few dominant taxa significantly contributed to the overall change of bacterial communities over time.
Regarding taxa associated with dehalogenation, increasing proportions of Dehalococcoides were observed (Figure 5). In contrast, the proportion of Sulfurospirillum and Geobacter, known to inhabit contaminated aquifers (Duhamel and Edwards, 2006; Maillard et al., 2011; Kranzioch et al., 2013; Lee et al., 2015), decreased throughout the experiment. These two genera include members capable of respiratory reductive dehalogenation of PCE, and may thus have contributed to dechlorination of PCE to TCE and DCE at the very beginning of the experiment. Furthermore, Sulfurospirillum and Geobacter strains capable of reductive dehalogenation of PCE to cis-DCE usually contain pceA genes more closely related to pceA(Dhb) of Dehalobacter and Desulfitobacterium strains (Neumann et al., 1998; Wagner et al., 2012; Buttet et al., 2013), which was detected in microcosms in early stage of the experiment. But more likely, as these taxa are non-obligatory organohalide-respirers (Wagner et al., 2012; Goris et al., 2014), they could also have used alternative endogenous electron acceptors, such as Fe(III) initially present in the site groundwater, and produced hydrogen necessary to Dehalococcoides to go further in the organohalide respiration pathway. This is likely to have occurred since recovered sequences associated with these OTUs were affiliated to non-dehalogenating strains of these genera (Sung et al., 2006a; Goris et al., 2014). Members of these taxa can also produce corinoïd cofactors that could be used for Dehalococcoides growth (Yan et al., 2012).
In addition, OTUs related to Acetobacterium, a genus including strains degrading PCE to TCE (Terzenbach and Blaut, 1994), were systematically found in low abundance (<1%) throughout the experiment. Although data obtained so far do not allow to conclude on their involvement in PCE dehalogenation in the present study, strains of Acetobacterium woodii have been reported to produce vitamin B12, an essential co-factor for reductive dechlorination (He et al., 2007). Hence, their presence could potentially benefit dissipation of CEs by reductive dehalogenation. Identifying taxa in groundwater microcosms whose activity would depend on the presence of CEs would require additional experiments as well as complementary approaches, such as, e.g., stable isotope probing (Chen and Murrell, 2010).
Operational Taxonomic Units corresponding to other genera often associated with reductive dehalogenation, such as Desulfitobacterium, Dehalobacter (Maillard et al., 2011; Rouzeau-Szynalski et al., 2011) and Dehalogenimonas (Maness et al., 2012) were detected at very low abundances, as expected from the low abundance of dehalogenase pceA(Dhb) typical of these genera (Regeard et al., 2004). In addition, these genera are not necessarily associated to organohalide respiration of CEs (Holscher et al., 2004; Dugat-Bony et al., 2012). Increase in abundance of an unclassified taxon associated with Deltaproteobacteria also occurred in the later phase of incubation (Figure 5). Indeed, Deltaproteobacteria are often associated with key dechlorinators in contaminated groundwater (Kotik et al., 2013; Adetutu et al., 2015; Kaown et al., 2016).
Some taxa, not usually associated with dehalogenation, also showed large changes in abundance in our experiments. Hydrogenophaga, Treponema, and Arcobacter, observed previously in contaminated groundwater (Kotik et al., 2013; Lee et al., 2015), decreased after the early stages of incubation (5 days). This could result from detrimental effects of microcosm conditions on their growth, such as the toxicity of sulfide produced by sulfate-reducing bacteria in the initial phase of microcosm experiments (Supplementary Figure S2). Indeed, the main changes in bacterial communities other than those associated with dehalogenation occurred for taxa related to sulfate reduction, in particular Desulfovibrio spp. (Barton and Fauque, 2009). The increase in relative abundance of sulfate reducers, such as strains of the genera Desulfovibrio, Desulfobulbus, and Desulfomicrobium (Kleikemper et al., 2002), suggests that they grew during the early stages of the experiment. Reductive dehalogenation of CEs is known to compete with sulfate reduction for electron donors and in particular for hydrogen (Davis et al., 2002; Aulenta et al., 2007, 2008). It is thus interesting that both reductive dehalogenation and sulfate reduction occurred in the early phases of the microcosm experiments, suggesting that its establishment was possible due to modest competition between these two metabolisms.
The only OTU associated with Dehalococcoides increased in abundance in the later stages of the experiment, which coincided with higher relative abundances of pceA and vcrA (Figure 4). An almost twofold increase in abundance of this Dehalococcoides OTU in microcosms spiked with 100 μM PCE compared to those amended with 10 μM further suggested that it was involved in PCE utilization for growth. Increase in Dehalococcoides following a decline of sulfate-reducing bacteria (mainly Desulfovibrio) and the decrease of sulfate in microcosms (Figure 5 and Supplementary Figure S3) suggests that relative abundance of sulfate-reducing bacteria, reduction of sulfate and development of Dehalococcoides may be related. Possibly, hydrogen transfer between Desulfovibrio and Dehalococcoides strains upon sulfate depletion may be occurring under these conditions (Drzyzga et al., 2001; Men et al., 2012).
Principal component analysis allowed integrative analysis of chemical and biomolecular data (Figure 6), and how functional gene abundance, bacterial diversity and CEs dissipation may be correlated. Microcosms were mainly discriminated according to the concentration of pollutants PCE, cis-DCE and VC (p < 0.05) (Figure 6), and time (Supplementary Figure S6). Overall, no clear differentiation of the bacterial community for most of the identified taxa was observed, although a correlation with time, pollutant concentrations or physico-chemical parameters such as redox potential and sulfate concentrations could be identified for a few of them (Figure 6). For example, relative abundance of Arcobacter, Dechloromonas, and Hydrogenophaga associated OTUs was correlated with the early stages of incubation, together with higher concentrations of CEs, sulfate and redox potential (Figures 5, 6). Several other genera such as Geobacter, Treponema, and Sulfurospirillum were also linked to the early stages of incubation (Figure 5) and physico-chemical conditions at the beginning of the experiment (Figure 6). In contrast, high relative abundance of taxa related to Desulfovibrio correlated to a later incubation time (10 days) (Figure 5).
Regarding dehalogenation, low relative abundance of pceA(Dhb) in microcosms was correlated with high concentrations of PCE, thus questioning its association with PCE dissipation. Similarly, abundance of bvcA was not significantly associated with changes in physico-chemical parameters or taxonomy. Key shifts observed in bacterial community composition were thus clearly associated with relative abundance of dehalogenase genes pceA(Dhc) and vcrA, and negatively or not correlated to pollutant concentrations (Figure 6), as expected for genes involved in pollutant transformation. In addition, chloride, sulfate and redox potential were correlated with abundances of Dehalococcoides-associated OTUs identified as a potential biomarker of organohalide respiration of CEs in microcosms of groundwater from the contaminated site of interest, as expected for reductive dehalogenation metabolism.
In summary, an active dechlorinating bacterial community was evidenced and characterized in groundwater samples from the contaminated Themeroil site. Molecular investigations of groundwater microcosms allowed to assess changes of functional genes associated with organohalide respiration of CEs and associated bacterial community composition. Analysis of the relationship between key dehalogenase genes and taxonomic profiling highlighted the importance of specific genera associated with dehalogenation of PCE, cis-DCE and VC, as dehalogenation of CEs. Concomitant changes in bacterial community composition revealed different compositions through time, and changes in Dehalococcoides and sulfate-reducing bacteria.
Taken together, our results provide new evidence that endogenous Dehalococcoides sp. in multi-contaminated groundwater from the investigated site of interest predominantly grows through CE organohalide respiration under anoxic conditions. This, together with patterns of pceA(Dhc) and vcrA genes, led to hypothesize that a potentially novel Dehalococcoides sp. taxon belonging to the Pinellas subgroup and containing both pceA(Dhc) and vcrA genes is related to dissipation of PCE, cis-DCE and VC. This hypothesis remains to be further examined by isolation of dehalogenating strains and experiments in pure cultures. Metagenome sequencing on groundwater samples of the site as well as attempts at isolation and characterisation of this strain in pure cultures and by multi-element compound specific isotope analysis (CSIA) may also help to identify biological pathways and genes associated with dissipation of CEs at the Themeroil site.
LH contributed to the experimental design, carried out experimental work, data analysis, and drafted the paper. JH, CJ, and SV contributed to the experimental setup, data analysis, and paper drafting and revision. JD and SF carried out metagenomics sequencing, data analysis, and paper revision. GI contributed to data analysis and paper drafting and revision. CU carried out cloning, sequencing and phylogenetic analyses and paper revision.
This project was funded through a ADEME-BRGM Ph.D. grant to LH (No. TEZ14-31) and the BIODISSPOL project funded by ADEME (No. 1572C0006).
The authors declare that the research was conducted in the absence of any commercial or financial relationships that could be construed as a potential conflict of interest.
We wish to thank Cécile Grand (ADEME) for providing access to the Themeroil site and scientific discussions. We are also grateful to Kevin Kuntze and Yvonne Nijenhuis [Helmholtz Centre for Environmental Research (UFZ), Leipzig, Germany] for providing plasmids with tceA, vcrA and bvcA reference genes, and to Julien Maillard (Environmental Engineering Institute, EPF Lausanne, Switzerland) for the plasmid with the pceA reference gene. We also thank the LIGAN-PM Genomics platform (Lille, France) supported by the ANR Equipex 2010 session (ANR-10-EQPX-07-01; ‘LIGAN-PM’), FEDER, and the Region Nord-Pas-de-Calais-Picardie, for their contribution to the high-throughput sequencing analysis.
The Supplementary Material for this article can be found online at: https://www.frontiersin.org/articles/10.3389/fmicb.2019.00089/full#supplementary-material
Abbai, N. S., and Pillay, B. (2013). Analysis of hydrocarbon-contaminated groundwater metagenomes as revealed by high-throughput sequencing. Mol. Biotechnol. 54, 900–912. doi: 10.1007/s12033-012-9639-z
Abe, Y., Aravena, R., Zopfi, J., Parker, B., and Hunkeler, D. (2009). Evaluating the fate of chlorinated ethenes in streambed sediments by combining stable isotope, geochemical and microbial methods. J. Contam. Hydrol. 107, 10–21. doi: 10.1016/j.jconhyd.2009.03.002
Adetutu, E. M., Gundry, T. D., Patil, S. S., Golneshin, A., Adigun, J., Bhaskarla, V., et al. (2015). Exploiting the intrinsic microbial degradative potential for field-based in situ dechlorination of trichloroethene contaminated groundwater. J. Hazard. Mater. 300, 48–57. doi: 10.1016/j.jhazmat.2015.06.055
Aktaş,Ö, Schmidt, K. R., Mungenast, S., Stoll, C., and Tiehm, A. (2012). Effect of chloroethene concentrations and granular activated carbon on reductive dechlorination rates and growth of Dehalococcoides spp. Bioresour. Technol. 103, 286–292. doi: 10.1016/j.biortech.2011.09.119
Amos, B. K., Suchomel, E. J., Pennell, K. D., and Löffler, F. E. (2008). Microbial activity and distribution during enhanced contaminant dissolution from a NAPL source zone. Water Res. 42, 2963–2974. doi: 10.1016/j.watres.2008.03.015
Atashgahi, S., Lu, Y., Ramiro-Garcia, J., Peng, P., Maphosa, F., Sipkema, D., et al. (2017). Geochemical parameters and reductive dechlorination determine aerobic cometabolic VS aerobic metabolic vinyl chloride biodegradation at oxic/anoxic interface of hyporheic zones. Environ. Sci. Technol. 51, 1626–1634. doi: 10.1021/acs.est.6b05041
Atashgahi, S., Lu, Y., and Smidt, H. (2016). “”Overview of known organohalide-respiring bacteria—phylogenetic diversity and environmental distribution”,” in Organohalide-Respiring Bacteria, eds L. Adrian and F. Löffler (Berlin: Springer), 63–105. doi: 10.1007/978-3-662-49875-0_5
Aulenta, F., Beccari, M., Majone, M., Papini, M. P., and Tandoi, V. (2008). Competition for H2 between sulfate reduction and dechlorination in butyrate-fed anaerobic cultures. Process Biochem. 43, 161–168. doi: 10.1016/j.procbio.2007.11.006
Aulenta, F., Pera, A., Rossetti, S., Papini, M. P., and Majone, M. (2007). Relevance of side reactions in anaerobic reductive dechlorination microcosms amended with different electron donors. Water Res. 41, 27–38. doi: 10.1016/j.watres.2006.09.019
Baelum, J., Chambon, J. C., Scheutz, C., Binning, P. J., Laier, T., Bjerg, P. L., et al. (2013). A conceptual model linking functional gene expression and reductive dechlorination rates of chlorinated ethenes in clay rich groundwater sediment. Water Res. 47, 2467–2478. doi: 10.1016/j.watres.2013.02.016
Barton, L. L., and Fauque, G. D. (2009). “Biochemistry, physiology and biotechnology of sulfate-reducing bacteria,” in Advances in Applied Microbiology, Vol. 68, eds A. I. Laskin, S. Sariaslani, and G. M. Gadd (San Diego, CA: Elsevier Academic Press Inc), 41–98. doi: 10.1016/S0065-2164(09)01202-7
Beeman, R. E., and Bleckmann, C. A. (2002). Sequential anaerobic–aerobic treatment of an aquifer contaminated by halogenated organics: field results. J. Contam. Hydrol. 57, 147–159. doi: 10.1016/S0169-7722(02)00008-6
Behrens, S., Azizian, M. F., McMurdie, P. J., Sabalowsky, A., Dolan, M. E., Semprini, L., et al. (2008). Monitoring abundance and expression of “Dehalococcoides” species chloroethene-reductive dehalogenases in a tetrachloroethene-dechlorinating flow column. Appl. Environ. Microbiol. 74, 5695–5703. doi: 10.1128/AEM.00926-08
BRGM (1998). Pollution du site industriel THEMEROIL à Varennes-le- Grand (71) Expertise géologique et hydrogéologique du dossier. Orléans: BRGM, 111.
BRGM (2011). Synthèse des connaissances sur l’hydrogéologie des sites de l’entreprise THEMEROIL et des aires de service de l’autoroute A6 sur les communes de Saint-Ambreuil et de Varennes-le-Grand (Saône-et-Loire). Orléans: BRGM, 101.
Buttet, G. F., Holliger, C., and Maillard, J. (2013). Functional genotyping of Sulfurospirillum spp. in mixed cultures allowed the identification of a new tetrachloroethene reductive dehalogenase. Appl. Environ. Microbiol. 79, 6941–6947. doi: 10.1128/AEM.02312-13
Caporaso, J. G., Kuczynski, J., Stombaugh, J., Bittinger, K., Bushman, F. D., Costello, E. K., et al. (2010). QIIME allows analysis of high-throughput community sequencing data. Nat. Methods 7, 335–336. doi: 10.1038/nmeth.f.303
Carreon-Diazconti, C., Santamaria, J., Berkompas, J., Field, J. A., and Brusseau, M. L. (2009). Assessment of in situ reductive dechlorination using compound-specific stable isotopes, functional gene PCR, and geochemical data. Environ. Sci. Technol. 43, 4301–4307. doi: 10.1021/es803308q
Chang, C.-H., Yang, H.-Y., Hung, J.-M., Lu, C.-J., and Liu, M.-H. (2017). Simulation of combined anaerobic/aerobic bioremediation of tetrachloroethylene in groundwater by a column system. Int. Biodeterior. Biodegrad. 117, 150–157. doi: 10.1016/j.ibiod.2016.12.014
Chen, Y., and Murrell, J. C. (2010). When metagenomics meets stable-isotope probing: progress and perspectives. Trends Microbiol. 18, 157–163. doi: 10.1016/j.tim.2010.02.002
Cheng, D., and He, J. (2009). Isolation and characterization of “Dehalococcoides” sp. strain MB, which dechlorinates tetrachloroethene to trans-1,2-dichloroethene. Appl. Environ. Microbiol. 75, 5910. doi: 10.1128/AEM.00767-09
Chow, W. L., Cheng, D., Wang, S., and He, J. (2010). Identification and transcriptional analysis of trans-DCE-producing reductive dehalogenases in Dehalococcoides species. ISME J. 4, 1020–1030. doi: 10.1038/ismej.2010.27
Claesson, M. J., Wang, Q., O’Sullivan, O., Greene-Diniz, R., Cole, J. R., Ross, R. P., et al. (2010). Comparison of two next-generation sequencing technologies for resolving highly complex microbiota composition using tandem variable 16S rRNA gene regions. Nucleic Acids Res. 38:e200. doi: 10.1093/nar/gkq873
Clark, K., Taggart, D. M., Baldwin, B. R., Ritalahti, K. M., Murdoch, R. W., Hatt, J. K., et al. (2018). Normalized quantitative PCR measurements as predictors for ethene formation at sites impacted with chlorinated ethenes. Environ. Sci. Technol. 52, 13410–13420. doi: 10.1021/acs.est.8b04373
Cole, J. R., Wang, Q., Fish, J. A., Chai, B., McGarrell, D. M., Sun, Y., et al. (2014). Ribosomal database project: data and tools for high throughput rRNA analysis. Nucleic Acids Res. 42, D633–D642. doi: 10.1093/nar/gkt1244
Cupples, A. M., Spormann, A. M., and McCarty, P. L. (2004). Vinyl chloride and cis-dichloroethene dechlorination kinetics and microorganism growth under substrate limiting conditions. Environ. Sci. Technol. 38, 1102–1107. doi: 10.1021/es0348647
Da Silva, M. L. B., and Alvarez, P. J. J. (2008). Exploring the correlation between halorespirer biomarker concentrations and TCE dechlorination rates. J. Environ. Eng. 134, 895–901. doi: 10.1061/(asce)0733-9372
Daprato, R. C., Löffler, F. E., and Hughes, J. B. (2007). Comparative analysis of three tetrachloroethene to ethene halorespiring consortia suggests functional redundancy. Environ. Sci. Technol. 41, 2261–2269. doi: 10.1021/es061544p
Davis, J. W., Odom, J. M., DeWeerd, K. A., Stahl, D. A., Fishbain, S. S., West, R. J., et al. (2002). Natural attenuation of chlorinated solvents at Area 6, Dover Air Force Base: characterization of microbial community structure. J. Contam. Hydrol. 57, 41–59. doi: 10.1016/S0169-7722(01)00217-0
Delbès, C., Moletta, R., and Godon, J. (2001). Bacterial and archaeal 16S rDNA and 16S rRNA dynamics during an acetate crisis in an anaerobic digestor ecosystem. FEMS Microbiol. Ecol. 35, 19–26. doi: 10.1111/j.1574-6941
DeSantis, T. Z., Hugenholtz, P., Larsen, N., Rojas, M., Brodie, E. L., Keller, K., et al. (2006). Greengenes, a chimera-checked 16S rRNA gene database and workbench compatible with ARB. Appl. Environ. Microbiol. 72, 5069–5072. doi: 10.1128/aem.03006-05
Drzyzga, O., Gerritse, J., Dijk, J. A., Elissen, H., and Gottschal, J. C. (2001). Coexistence of a sulphate-reducing Desulfovibrio species and the dehalorespiring Desulfitobacterium frappieri TCE1 in defined chemostat cultures grown with various combinations of sulphate and tetrachloroethene. Environ. Microbiol. 3, 92–99. doi: 10.1046/j.1462-2920
Dugat-Bony, E., Biderre-Petit, C., Jaziri, F., David, M. M., Denonfoux, J., Lyon, D. Y., et al. (2012). In situ TCE degradation mediated by complex dehalorespiring communities during biostimulation processes. Microb. Biotechnol. 5, 642–653. doi: 10.1111/j.1751-7915.2012.00339.x
Duhamel, M., and Edwards, E. A. (2006). Microbial composition of chlorinated ethene-degrading cultures dominated by Dehalococcoides. FEMS Microbiol. Ecol. 58, 538–549. doi: 10.1111/j.1574-6941.2006.00191.x
Edgar, R. C. (2010). Search and clustering orders of magnitude faster than BLAST. Bioinformatics 26, 2460–2461. doi: 10.1093/bioinformatics/btq461
Florey, J., Adams, A., and Michel, L. (2017). Reductive dechlorination of a chlorinated solvent plume in Houston. Texas. Remed J. 28, 5–54. doi: 10.1002/rem.21541
Freedman, D. L., and Gossett, J. M. (1991). Biodegradation of dichloromethane and its utilization as a growth substrate under methanogenic conditions. Appl. Environ. Microbiol. 57, 2847–2857.
Futagami, T., Goto, M., and Furukawa, K. (2008). Biochemical and genetic bases of dehalorespiration. Chem. Rec. 8, 1–12. doi: 10.1002/tcr.20134
Goris, T., Schubert, T., Gadkari, J., Wubet, T., Tarkka, M., Buscot, F., et al. (2014). Insights into organohalide respiration and the versatile catabolism of Sulfurospirillum multivorans gained from comparative genomics and physiological studies. Environ. Microbiol. 16, 3562–3580. doi: 10.1111/1462-2920.12589
Gouy, M., Guindon, S., and Gascuel, O. (2010). SeaView version 4: a multiplatform graphical user interface for sequence alignment and phylogenetic tree building. Mol. Biol. Evol. 27, 221–224. doi: 10.1093/molbev/msp259
Haack, S. K., Fogarty, L. R., West, T. G., Alm, E. W., McGuire, J. T., Long, D. T., et al. (2004). Spatial and temporal changes in microbial community structure associated with recharge-influenced chemical gradients in a contaminated aquifer. Environ. Microbiol. 6, 438–448. doi: 10.1111/j.1462-2920.2003.00563.x
Hall, T. (1999). BioEdit: a user-friendly biological sequence alignment editor and analysis program for Windows 95/NT. Nucleic Acids Symp. Ser. 41, 95–98.
He, J., Holmes, V. F., Lee, P. K. H., and Alvarez-Cohen, L. (2007). Influence of vitamin B12 and cocultures on the growth of Dehalococcoides isolates in defined medium. Appl. Environ. Microbiol. 73, 2847–2853. doi: 10.1128/AEM.02574-06
He, J. Z., Ritalahti, K. M., Yang, K. L., Koenigsberg, S. S., and Löffler, F. E. (2003). Detoxification of vinyl chloride to ethene coupled to growth of an anaerobic bacterium. Nature 424, 62–65. doi: 10.1038/nature01717
Hendrickson, E. R., Payne, J. A., Young, R. M., Starr, M. G., Perry, M. P., Fahnestock, S., et al. (2002). Molecular analysis of Dehalococcoides 16S ribosomal DNA from chloroethene-contaminated sites throughout North America and Europe. Appl. Environ. Microbiol. 68, 485–495. doi: 10.1128/AEM.68.2.485-495.2002
Holliger, C., Wohlfarth, G., and Diekert, G. (1998). Reductive dechlorination in the energy metabolism of anaerobic bacteria. FEMS Microbiol. Rev. 22, 383–398. doi: 10.1016/S0168-6445(98)00030-8
Holscher, T., Krajmalnik-Brown, R., Ritalahti, K. M., von Wintzingerode, F., Gorisch, H., Löffler, F. E., et al. (2004). Multiple non-identical reductive-dehalogenase-homologous genes are common in Dehalococcoides. Appl. Environ. Microbiol. 70, 5290–5297. doi: 10.1128/AEM.70.9.5290-5297.2004
Huang, B., Lei, C., Wei, C., and Zeng, G. (2014). Chlorinated volatile organic compounds (Cl-VOCs) in environment — sources, potential human health impacts, and current remediation technologies. Environ. Int. 71, 118–138. doi: 10.1016/j.envint.2014.06.013
Hug, L. A. (2016). “Diversity, evolution, and environmental distribution of reductive dehalogenase genes,” in Organohalide-Respiring Bacteria, eds L. Adrian and F. Löffler (Berlin: Springer), 377–393. doi: 10.1007/978-3-662-49875-0-16
Hug, L. A., and Edwards, E. A. (2013). Diversity of reductive dehalogenase genes from environmental samples and enrichment cultures identified with degenerate primer PCR screens. Front. Microbiol. 4:341. doi: 10.3389/fmicb.2013.00341
Imfeld, G., Nijenhuis, I., Nikolausz, M., Zeiger, S., Paschke, H., Drangmeister, J., et al. (2008). Assessment of in situ degradation of chlorinated ethenes and bacterial community structure in a complex contaminated groundwater system. Water Res. 42, 871–882. doi: 10.1016/j.watres.2007.08.035
Ise, K., Suto, K., and Inoue, C. (2011). Microbial diversity and changes in the distribution of dehalogenase genes during dechlorination with different concentrations of cis-DCE. Environ. Sci. Technol. 45, 5339–5345. doi: 10.1021/es104199y
Kang, J. W. (2014). Removing environmental organic pollutants with bioremediation and phytoremediation. Biotechnol. Lett. 36, 1129–1139. doi: 10.1007/s10529-014-1466-9
Kaown, D., Jun, S.-C., Kim, R.-H., Woosik, S., and Lee, K.-K. (2016). Characterization of a site contaminated by chlorinated ethenes and ethanes using multi-analysis. Environ. Earth Sci. 75:745. doi: 10.1007/s12665-016-5536-2
Kleikemper, J., Schroth, M. H., Sigler, W. V., Schmucki, M., Bernasconi, S. M., and Zeyer, J. (2002). Activity and diversity of sulfate-reducing bacteria in a petroleum hydrocarbon-contaminated aquifer. Appl. Environ. Microbiol. 68, 1516–1523. doi: 10.1128/AEM.68.4.1516-1523.2002
Kotik, M., Davidova, A., Voriskova, J., and Baldrian, P. (2013). Bacterial communities in tetrachloroethene-polluted groundwaters: a case study. Sci. Total Environ. 454, 517–527. doi: 10.1016/j.scitotenv.2013.02.082
Krajmalnik-Brown, R., Holscher, T., Thomson, I. N., Saunders, F. M., Ritalahti, K. M., and Löffler, F. E. (2004). Genetic identification of a putative vinyl chloride reductase in Dehalococcoides sp. strain BAV1. Appl. Environ. Microbiol. 70, 6347–6351. doi: 10.1128/AEM.70.10.6347-6351.2004
Kranzioch, I., Ganz, S., and Tiehm, A. (2015). Chloroethene degradation and expression of Dehalococcoides dehalogenase genes in cultures originating from Yangtze sediments. Environ. Sci. Pollut. Res. 22, 3138–3148. doi: 10.1007/s11356-014-3574-4
Kranzioch, I., Stoll, C., Holbach, A., Chen, H., Wang, L., Zheng, B., et al. (2013). Dechlorination and organohalide-respiring bacteria dynamics in sediment samples of the Yangtze Three Gorges Reservoir. Environ. Sci. Pollut. Res. 20, 7046–7056. doi: 10.1007/s11356-013-1545-9
Lee, P. K. H., Macbeth, T. W., Sorenson, J., Kent, S., Deeb, R. A., and Alvarez-Cohen, L. (2008). Quantifying genes and transcripts to assess the in situ physiology of “Dehalococcoides” spp. in a trichloroethene-contaminated groundwater site. Appl. Environ. Microbiol. 74, 2728–2739. doi: 10.1128/AEM.02199-07
Lee, S.-S., Kaown, D., and Lee, K.-K. (2015). Evaluation of the fate and transport of chlorinated ethenes in a complex groundwater system discharging to a stream in Wonju. Korea. J. Contam. Hydrol. 182, 231–243. doi: 10.1016/j.jconhyd.2015.09.005
Legendre, P., and Gallagher, E. D. (2001). Ecologically meaningful transformations for ordination of species data. Oecologia 129, 271–280. doi: 10.1007/s004420100716
Löffler, F. E., Sun, Q., Li, J. R., and Tiedje, J. M. (2000). 16S rRNA gene-based detection of tetrachloroethene-dechlorinating Desulfuromonas and Dehalococcoides species. Appl. Environ. Microbiol. 66, 1369–1374. doi: 10.1128/AEM.66.4.1369-1374.2000
Löffler, F. E., Yan, J., Ritalahti, K. M., Adrian, L., Edwards, E. A., Konstantinidis, K. T., et al. (2013). Dehalococcoides mccartyi gen. nov., sp. nov., obligately organohalide-respiring anaerobic bacteria relevant to halogen cycling and bioremediation, belong to a novel bacterial class, Dehalococcoidia classis nov., order Dehalococcoidales ord. nov. and family Dehalococcoidaceae fam. nov., within the phylum Chloroflexi. Int. J. Syst. Evol. Microbiol. 63, 625–635. doi: 10.1099/ijs.0.034926-0
López-Gutiérrez, J. C., Henry, S., Hallet, S., Martin-Laurent, F., Catroux, G., and Philippot, L. (2004). Quantification of a novel group of nitrate-reducing bacteria in the environment by real-time PCR. J. Microbiol. Methods 57, 399–407. doi: 10.1016/j.mimet.2004.02.009
Lu, X., Wilson, J. T., and Kampbell, D. H. (2006). Relationship between Dehalococcoides DNA in ground water and rates of reductive dechlorination at field scale. Water Res. 40, 3131–3140. doi: 10.1016/j.watres.2006.05.030
Magnuson, J. K., Stern, R. V., Gossett, J. M., Zinder, S. H., and Burris, D. R. (1998). Reductive dechlorination of tetrachloroethene to ethene by two-component enzyme pathway. Appl. Environ. Microbiol. 64, 1270–1275.
Magoč, T., and Salzberg, S. L. (2011). FLASH: fast length adjustment of short reads to improve genome assemblies. Bioinformatics 27, 2957–2963. doi: 10.1093/bioinformatics/btr507
Maillard, J., Charnay, M.-P., Regeard, C., Rohrbach-Brandt, E., Rouzeau-Szynalski, K., Rossi, P., et al. (2011). Reductive dechlorination of tetrachloroethene by a stepwise catalysis of different organohalide respiring bacteria and reductive dehalogenases. Biodegradation 22, 949–960. doi: 10.1007/s10532-011-9454-4
Maness, A. D., Bowman, K. S., Yan, J., Rainey, F. A., and Moe, W. M. (2012). Dehalogenimonas spp. can reductively dehalogenate high concentrations of 1,2-dichloroethane, 1,2-dichloropropane, and 1,1,2-trichloroethane. AMB Express 2:54. doi: 10.1186/2191-0855-2-54
Maphosa, F., Lieten, S. H., Dinkla, I., Stams, A. J., Smidt, H., and Fennell, D. E. (2012). Ecogenomics of microbial communities in bioremediation of chlorinated contaminated sites. Front. Microbiol. 3:351. doi: 10.3389/fmicb.2012.00351
Maphosa, F., Smidt, H., De Vos, W. M., and Roling, W. F. M. (2010). Microbial community- and metabolite dynamics of an anoxic dechlorinating bioreactor. Environ. Sci. Technol. 44, 4884–4890. doi: 10.1021/es903721s
Maymó-Gatell, X., Anguish, T., and Zinder, S. H. (1999). Reductive dechlorination of chlorinated ethenes and 1,2-dichloroethane by “Dehalococcoides ethenogenes” 195. Appl. Environ. Microbiol. 65, 3108–3113.
Maymó-Gatell, X., Nijenhuis, I., and Zinder, S. H. (2001). Reductive dechlorination of cis-1,2-dichloroethene and vinyl chloride by “Dehalococcoides ethenogenes.”. Environ. Sci. Technol. 35, 516–521. doi: 10.1021/es001285i
Men, Y., Feil, H., VerBerkmoes, N. C., Shah, M. B., Johnson, D. R., Lee, P. K. H., et al. (2012). Sustainable syntrophic growth of Dehalococcoides ethenogenes strain 195 with Desulfovibrio vulgaris Hildenborough and Methanobacterium congolense: global transcriptomic and proteomic analyses. ISME J. 6, 410–421. doi: 10.1038/ismej.2011.111
Miyata, R., Adachi, K., Tani, H., Kurata, S., Nakamura, K., Tsuneda, S., et al. (2010). Quantitative detection of chloroethene-reductive bacteria Dehalococcoides spp. using alternately binding probe competitive polymerase chain reaction. Mol. Cell. Probes 24, 131–137. doi: 10.1016/j.mcp.2009.11.005
Muller, J. A., Rosner, B. M., von Abendroth, G., Meshulam-Simon, G., McCarty, P. L., and Spormann, A. M. (2004). Molecular identification of the catabolic vinyl chloride reductase from Dehalococcoides sp. strain VS and its environmental distribution. Appl. Environ. Microbiol. 70, 4880–4888. doi: 10.1128/AEM.70.8.4880-4888.2004
Neumann, A., Wohlfarth, G., and Diekert, G. (1998). Tetrachloroethene dehalogenase from Dehalospirillum multivorans: cloning, sequencing of the encoding genes, and expression of the pceA gene in Escherichia coli. J. Bacteriol. 180, 4140–4145.
Nijenhuis, I., Nikolausz, M., Koeth, A., Felfoeldi, T., Weiss, H., Drangmeister, J., et al. (2007). Assessment of the natural attenuation of chlorinated ethenes in an anaerobic contaminated aquifer in the Bitterfeld/Wolfen area using stable isotope techniques, microcosm studies and molecular biomarkers. Chemosphere 67, 300–311. doi: 10.1016/j.chemosphere.2006.09.084
Nishimura, M., Ebisawa, M., Sakihara, S., Kobayashi, A., Nakama, T., Okochi, M., et al. (2008). Detection and identification of Dehalococcoides species responsible for in situ dechlorination of trichloroethene to ethene enhanced by hydrogen-releasing compounds. Biotechnol. Appl. Biochem. 51, 1–7. doi: 10.1042/BA20070171
Patil, S. S., Adetutu, E. M., Sheppard, P. J., Morrison, P., Menz, I. R., and Ball, A. S. (2014). Site-specific pre-evaluation of bioremediation technologies for chloroethene degradation. Int. J. Environ. Sci. Technol. 11, 1869–1880. doi: 10.1007/s13762-013-0383-0
Rahm, B. G., and Richardson, R. E. (2008). Dehalococcoides’ gene transcripts as quantitative bioindicators of tetrachloroethene, trichloroethene, and cis-1,2-dichloroethene dehalorespiration rates. Environ. Sci. Technol. 42, 5099–5105. doi: 10.1021/es702912t
Ramette, A. (2007). Multivariate analyses in microbial ecology. FEMS Microbiol Ecol. 62, 142–160. doi: 10.1111/j.1574-6941.2007.00375.x
Regeard, C., Maillard, J., and Holliger, C. (2004). Development of degenerate and specific PCR primers for the detection and isolation of known and putative chloroethene reductive dehalogenase genes. J. Microbiol. Methods 56, 107–118. doi: 10.1016/j.mimet.2003.09.019
Rouzeau-Szynalski, K., Maillard, J., and Holliger, C. (2011). Frequent concomitant presence of Desulfitobacterium spp. and “Dehalococcoides” spp. in chloroethene-dechlorinating microbial communities. Appl. Microbiol. Biotechnol. 90, 361–368. doi: 10.1007/s00253-010-3042-0
Rupakula, A., Kruse, T., Boeren, S., Holliger, C., Smidt, H., and Maillard, J. (2013). The restricted metabolism of the obligate organohalide respiring bacterium Dehalobacter restrictus: lessons from tiered functional genomics. Philos. Trans. R. Soc. B Biol. Sci. 368:20120325. doi: 10.1098/rstb.2012.0325
Saiyari, D. M., Chuang, H.-P., Senoro, D. B., Lin, T.-F., Whang, L.-M., Chiu, Y.-T., et al. (2018). A review in the current developments of genus Dehalococcoides, its consortia and kinetics for bioremediation options of contaminated groundwater. Sustain. Environ. Res. 28, 149–157. doi: 10.1016/j.serj.2018.01.006
Santharam, S., Ibbini, J., Davis, L., and Erickson, L. (2011). Field study of biostimulation and bioaugmentation for remediation of tetrachloroethene in groundwater. Remed. J. 21, 51–68. doi: 10.1002/rem.20281
Scheutz, C., Durant, N. D., Dennis, P., Hansen, M. H., Jørgensen, T., Jakobsen, R., et al. (2008). Concurrent ethene generation and growth of Dehalococcoides containing vinyl chloride reductive dehalogenase genes during an enhanced reductive dechlorination field demonstration. Environ. Sci. Technol. 42, 9302–9309. doi: 10.1021/es800764t
Schmieder, R., and Edwards, R. (2011). Quality control and preprocessing of metagenomic datasets. Bioinformatics 27, 863–864. doi: 10.1093/bioinformatics/btr026
Sheu, Y., Tsang, D., Dong, C., Chen, C., Luo, S., and Kao, C. (2018). Enhanced bioremediation of TCE-contaminated groundwater using gamma poly-glutamic acid as the primary substrate. J. Clean Prod. 178, 108–118. doi: 10.1016/j.jclepro.2017.12.212
Sung, Y., Fletcher, K. E., Ritalahti, K. M., Apkarian, R. P., Ramos-Hernández, N., Sanford, R. A., et al. (2006a). Geobacter lovleyi sp. nov. strain SZ, a novel metal-reducing and tetrachloroethene-dechlorinating bacterium. Appl. Environ. Microbiol. 72, 2775–2782. doi: 10.1128/AEM.72.4.2775-2782.2006
Sung, Y., Ritalahti, K. M., Apkarian, R. P., and Löffler, F. E. (2006b). Quantitative PCR confirms purity of strain GT, a novel trichloroethene-to-ethene-respiring Dehalococcoides isolate. Appl. Environ. Microbiol. 72, 1980–1987. doi: 10.1128/AEM.72.3.1980-1987.2006
Suyama, A., Yamashita, M., Yoshino, S., and Furukawa, K. (2002). Molecular characterization of the pceA reductive dehalogenase of Desulfitobacterium sp. strain Y51. J. Bacteriol. 184, 3419–3425. doi: 10.1128/JB.184.13.3419-3425.2002
Terzenbach, D. P., and Blaut, M. (1994). Transformation of tetrachloroethylene to trichloroethylene by homoacetogenic bacteria. FEMS Microbiol. Lett. 123, 213–218. doi: 10.1111/j.1574-6968.1994.tb07224.x
Vogel, T. M., and McCarty, P. L. (1985). Biotransformation of tetrachloroethylene to trichloroethylene, dichloroethylene, vinyl chloride, and carbon dioxide under methanogenic conditions. Appl. Environ. Microbiol. 49, 1080–1083.
Wagner, D. D., Hug, L. A., Hatt, J. K., Spitzmiller, M. R., Padilla-Crespo, E., Ritalahti, K. M., et al. (2012). Genomic determinants of organohalide-respiration in Geobacter lovleyi, an unusual member of the Geobacteraceae. BMC Genomics 13:200. doi: 10.1186/1471-2164-13-200
Wang, S., Chng, K. R., Wilm, A., Zhao, S., Yang, K.-L., Nagarajan, N., et al. (2014). Genomic characterization of three unique Dehalococcoides that respire on persistent polychlorinated biphenyls. PNAS 111, 12103–12108. doi: 10.1073/pnas.1404845111
Yan, J., Ritalahti, K. M., Wagner, D. D., and Löffler, F. E. (2012). Unexpected specificity of interspecies cobamide transfer from Geobacter spp. to organohalide-respiring Dehalococcoides mccartyi strains. Appl. Environ. Microbiol. 78, 6630–6636. doi: 10.1128/AEM.01535-12
Zhao, S., Ding, C., and He, J. (2017). Genomic characterization of Dehalococcoides mccartyi strain 11a5 reveals a circular extrachromosomal genetic element and a new tetrachloroethene reductive dehalogenase gene. FEMS Microbiol. Ecol. 93:fiw235. doi: 10.1093/femsec/fiw235
Keywords: perchloroethylene (PCE), chloroethenes (CEs), contaminated groundwater, dehalogenase genes, organohalide respiration
Citation: Hermon L, Hellal J, Denonfoux J, Vuilleumier S, Imfeld G, Urien C, Ferreira S and Joulian C (2019) Functional Genes and Bacterial Communities During Organohalide Respiration of Chloroethenes in Microcosms of Multi-Contaminated Groundwater. Front. Microbiol. 10:89. doi: 10.3389/fmicb.2019.00089
Received: 11 September 2018; Accepted: 16 January 2019;
Published: 12 February 2019.
Edited by:
Stéphane Pesce, National Research Institute of Science and Technology for Environment and Agriculture (IRSTEA), FranceReviewed by:
Lise Barthelmebs, Université de Perpignan Via Domitia, FranceCopyright © 2019 Hermon, Hellal, Denonfoux, Vuilleumier, Imfeld, Urien, Ferreira and Joulian. This is an open-access article distributed under the terms of the Creative Commons Attribution License (CC BY). The use, distribution or reproduction in other forums is permitted, provided the original author(s) and the copyright owner(s) are credited and that the original publication in this journal is cited, in accordance with accepted academic practice. No use, distribution or reproduction is permitted which does not comply with these terms.
*Correspondence: Catherine Joulian, Yy5qb3VsaWFuQGJyZ20uZnI=
Disclaimer: All claims expressed in this article are solely those of the authors and do not necessarily represent those of their affiliated organizations, or those of the publisher, the editors and the reviewers. Any product that may be evaluated in this article or claim that may be made by its manufacturer is not guaranteed or endorsed by the publisher.
Research integrity at Frontiers
Learn more about the work of our research integrity team to safeguard the quality of each article we publish.