- 1The Affiliated Nanhua Hospital, Hengyang Medical School, University of South China, Hengyang, China
- 2Computer Institute, Hengyang Medical School, University of South China, Hengyang, Hunan, China
- 3Transformation Research Lab, Hengyang Medical School, University of South China, Hengyang, Hunan, China
- 4The Third Affiliated Hospital, Hengyang Medical School, University of South China, Hengyang, China
Traditional immunotherapies mainly focus on αβ T cell-based strategies, which depend on MHC-mediated antigen recognition. However, this approach poses significant challenges in treating recurrent tumors, as immune escape mechanisms are widespread. γδ T cells, with their ability for MHC-independent antigen presentation, offer a promising alternative that could potentially overcome limitations observed in traditional immunotherapies. These cells play a role in tumor immune surveillance through a unique mechanism of antigen recognition and synergistic interactions with other immune effector cells. In this review, we will discuss the biological properties of the Vδ1 and Vδ2 T subsets of γδ T cells, their immunomodulatory role within the tumor microenvironment, and the most recent clinical advances in γδ T cell-based related immunotherapies, including cell engaging strategies and adoptive cell therapy.
1 Introduction
T cells are pivotal in the realm of cancer immunotherapy research (1). They can be classified into αβ T cells and γδ T cells, distinct by their T cell receptor structures. γδ T cells possess a unique TCR composed of γ and δ chains (2), enabling γδ T cells to recognize various antigens, performing multiple roles, including antitumor activities, immune surveillance, and anti-infection capabilities (3–5). Additionally, activated γδ T cells secrete different cytokines depending on the local microenvironment and interact with other cells to participate in the host's antitumor immune response (6, 7). While γδ T cells show immense therapeutic promise, their biological functions and clinical applications remain relatively understudied. Recent research has started to reveal the various roles of γδ T cells in the tumor microenvironment (TME) and explore novel approaches for their clinical application, including the expansion of γδ T cells and the development of chimeric antigen receptor (CAR)-γδ T cells (8–10).
This review provides a comprehensive overview of the current understanding of the mechanisms of γδ T cell recognition and their immunomodulatory role in TME. We will also explore recent advances in γδ T cell-based immunotherapy and discuss the barriers and future directions for γδ T cell research. The primary aim is to connect fundamental research with clinical application to optimize the efficacy of γδ T cell therapy for cancer.
2 Tumor recognition mechanisms of γδ T cells
γδ T cells consist of three main functional subsets: Vδ1, Vδ2, and Vδ3 (11, 12). In humans, Vδ2 T cells mainly express the Vδ2 chain and often pair with Vγ9 to form Vγ9Vδ2 T cells, primarily found in peripheral blood (13, 14). Vγ9Vδ2 T cells have been extensively studied due to multiple tumor cell recognition receptors and their ease of expansion in vitro (15–17). Vδ1 T cells are the second most abundant type in the peripheral blood (18, 19). These cells recognize MHC class irrelated polymorphic molecules through natural killer group 2 member D (NKG2D) receptors (20). Proteins encoded by MHC class I-chain related genes A and B (MICA and MICB), along with UL16-binding proteins (ULBP) (21), are expressed under conditions of cellular stress, damage, or transformation and bind to NKG2D receptors, serving as “kill me” signals to cytotoxic T cells (22, 23). Vδ3 T cells comprise a relatively rare subpopulation in the peripheral blood, liver, and intestines (24, 25). They multiply in reaction to cytomegalovirus infection and are involved in developing dendritic cells (DCs) and B cells. The diverse distribution of different γδ T cell types in various tissues highlights their versatile function in immune responses. More research has been conducted on Vδ2 and Vδ1 T cells in cancer immunotherapy, so this review specifically focuses on these subsets.
2.1 BTN3A1 and BTN2A1 mediate recognition of phosphoantigens by γδ T cells
The process by which γδ T cells identify tumor-associated antigens (TAAs) primarily involves the TCR and NKR pathways (6). Under conditions of cellular stress, Vγ9Vδ2 TCR recognizes phosphoantigens (pAgs) to initiate immune responses (26). pAgs products produced by the isoprene biosynthetic pathway. For instance, a common pAg, isoprene pyrophosphate (IPP), is present in all living organisms. Another potent activator, (E)-4-hydroxy-3-methyl-but-2-enyl pyrophosphate (HMBPP), originating from specific microbes and parasites (27, 28). HMBPP activates the Vγ9Vδ2 T cell receptor much more effectively than IPP (29). The level of pAgs in normal cells is extremely low. However, tumor cell development can lead to the accumulation of endogenous pAgs, making them rapidly identifiable and targetable by Vδ2 T cells. Clinical studies have demonstrated that increasing the IPP levels promotes the activity of farnesyl pyrophosphate synthase in the isoprenoid pathway. Various strategies involve the use of aminobisphosphonates, such as zoledronate (ZOL) and pamidronate, or synthetic pAg analogs to directly activate Vγ9Vδ2 T cells. Studies have shown that γδ T cells exhibit moderate cytotoxicity against tumor cells without pAg. However, when HMBPP or ZOL is added, it induces TCR-dependent cytotoxicity in γδ T cells (30).
Vδ2 T cells do not directly recognize pAgs but depend on the combined action of butyrophilin subfamily 3 member A1 (BTN3A1) and BTN2A1 (31) (Figure 1). The pioneering study by Harly and colleagues first identified a crucial role of BTN3A1 in regulating pAgs responses in Vδ2 T cells (32). BTN3A1 (CD277) and BTN2A1 are members of the butyrophilin family. They are part of the immunoglobulin-like molecules with extracellular segments containing IgV and IgC domains, and intracellular segments consisting of B30.2/SPRY cytoplasmic domains (33). The interaction mechanism among these molecules is still debated, but the prevailing hypothesis supports an “inside-out signaling” model. According to this hypothesis, after the increase of intracellular IPP levels, BTN3A2 or BTN3A3 form heterodimers with a unique surface topology different from the homodimers of BTN3A1. In this process, these heterodimers allow structurally diverse pAg molecules to bind to the intracellular B30.2 domain of BTN3A1, forming a molecular glue complex interface (34). pAgs directly bind to BTN2A1 through this interface. pAgs directly bind to BTN2A1 through this interface. By varying affinities, BTN2A1 articulates onto the Vγ9 chain of the γδ TCR, forming a complex with distinct BTN3A1-V and BTN2A1-V domain topologies (35), initiating TCR-mediated γδ T cell activation (36). This mechanism operates independently of αβ T cells, offering potential for therapeutic development. However, further in-depth studies are required to clarify whether the Vδ2 chain of the Vγ9Vδ2 TCR is involved in recognizing the antigenic process. Recent studies have identified AMPK regulating BTN2A1 and BTN3A interactions within Vδ2 T cells, unveiling a stress-mediated regulatory mechanism that enhances the cytotoxic capabilities of Vδ2 T cells (37). Overall, the mechanism by which Vδ2 T cells recognize TAAs through BTN3A1 and BTN2A1 provides new opportunities for antitumor therapy. The Vg9Vd2 TCR can also recognize F1-ATPase (which binds to apolipoprotein AI, referred to as Apo AI) (38). F1-ATPase is ectopically expressed on the cell membrane of specific tumor cells, for instance human leukemia (K562) cells and lymphoma (Raji) cells. ZOL can bind to ApoA1 as a presenting molecule after high-dose ZOL treatment, enhancing its stimulatory effect on Vδ2 T cells (39). Furthermore, aberrantly expressed MutS homolog two composed complex (MSH2) has also been discovered to mediate γδ T cells recognition, thereby triggering cytolysis of tumor cells (40–42).
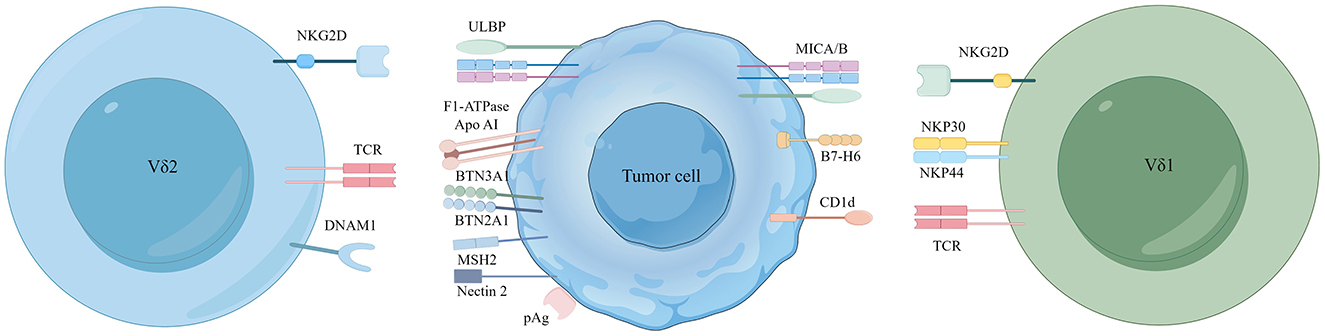
Figure 1. Major receptor-ligand recognition between Vδ2/Vδ1 T cells and tumor cells. Recognition of phosphoantigens by the human Vγ9Vδ2 TCR mainly depends on the synergistic action of BTN3A1/BTN2A1. Vδ2 T cells also recognize a complex of F1-ATPase bound to ApoAI and MutS homolog 2 (MSH2). Nectin-2 from tumor cells can also activate Vδ2 T cells by binding to DNAM-1. The Vδ1 TCR recognizes CD1d and its presented lipids, and its expression of NKp30 and NKp44 receptors can bind to ligands B7-H6. In addition to this, NKG2D receptors expressed on Vδ2 and Vδ1 T cells both recognize MICA/B and ULBP molecular ligands.
2.2 Role of NKG2D and its ligands in γδ T cell activation
γδ T cells recognize TAAs not only through the γδ TCR but also through various natural killer receptors (NKR) expression, such as natural killer group 2 member D (NKG2D) and DNAX accessory molecule-1 (DNAM1) (43). NKG2D in Vδ2 T cells binds to MHC class I polypeptide-related sequence A/B (MICA/B) (44), retinoic acid early inducible 1 (Rae-1) and UL16 binding proteins (ULBP) found on tumor cells (45). Concurrently, DNAM1 interacts with Nectin-5, Nectin-2, and the poliovirus receptor (PVR) on the surface of tumor cells. Such interactions mediate the cytotoxic response, targeting killing tumor cells via the perforin-granzyme pathway (46, 47) (Figure 1).
NKG2D, an activating cell surface receptor, is primarily found in cytotoxic immune cells, including NK cells, NKT cells, and specific γδ T cell subsets (43). The ligand for this receptor is absent in normal cells but is frequently present in malignant cells. Upon encountering tumor cells, the Vδ2 T cell subset undergoes rapid proliferation and upregulates NKG2D expression, bolstering immune surveillance (48). Nadia et al. demonstrated that mice deficient in NKG2D have a higher prevalence of highly malignant prostate cancer and promote tumor progression (49). Moreover, a rapid response of NKG2D to its ligand Rae-1 was observed in mouse γδ T cells. Persistent overexpression of Rae-1 downregulates NKG2D expression, thereby attenuating the antitumor functions of T cells (50). Moreover, the DNAM-1 receptor is pivotal in mediating γδ T cell targeting tumor cells. The antitumor response of human γδ T cells strongly correlates with the presence of DNAM-1 ligands on tumor cells (51, 52). The study found that the inhibition of PVR and Nectin-2 led to a marked decrease in the cytotoxic capabilities and cytokine secretion activities of γδ T cells (47).
2.3 CD1d is the key driver of vδ1 T cell activation
In human Vδ1 T cells, CD1d has emerged as a critical antigen-presenting molecule (53). CD1d, a glycolipid antigen-presenting molecule, is expressed in various cancers, including renal cell (54), medulloblastoma (55), glioma (56), multiple myeloma (57), breast (58), and prostate (59). The Vδ1 TCR can recognize CD1d and its lipid antigens (5), which may facilitate tumor growth by prompting type 1 NKT cells to release immunosuppressive cytokines, thereby aiding protumor NKT cell subsets (60). Besides CD1d, Vδ1 T cells depend on the expression of NKp30 and NKp44 receptors (61). Researchers have shown that the targeted knockdown of the B7-H6 ligand, bound to the NKp30 receptor, utilizing the CRISPR/Cas9 gene-editing technology significantly diminishes the antitumor response of γδ T cells in acute myeloid leukemia (AML) (62). In addition, it is reported that NKp46 is expressed explicitly on intraepithelial Vδ1 T cells in the intestine (63). Remarkably, the Vδ1 T cells can also recognize MICA/B via NKG2D, and direct binding of MICA/B to Vδ1 has been demonstrated (64). These findings provide new insights into the role of Vδ1 T cells in tumor immunity and offer potential new targets for cancer therapy (Figure 1).
3 Immunomodulatory role of γδ T cells in the TME
3.1 Cytokine-mediated modulation of γδ T cell functions
γδ T cells induce apoptosis of tumor cells mainly through the perforin-granzyme mechanism or the Fas/FasL and TRAIL pathways (65, 66). They can also target tumor cells for killing through antibody-dependent cell-mediated cytotoxicity in tumor immunosurveillance (67, 68). γδ T cells stimulate immune responses indirectly by secreting cytokines like interferon-γ (IFN-γ), tumor necrosis factor-α (TNF-α), interleukin (IL)-2, IL-10, IL-12, and IL-15, impacting both tumor cells and the microenvironment (69, 70) (Figure 2).
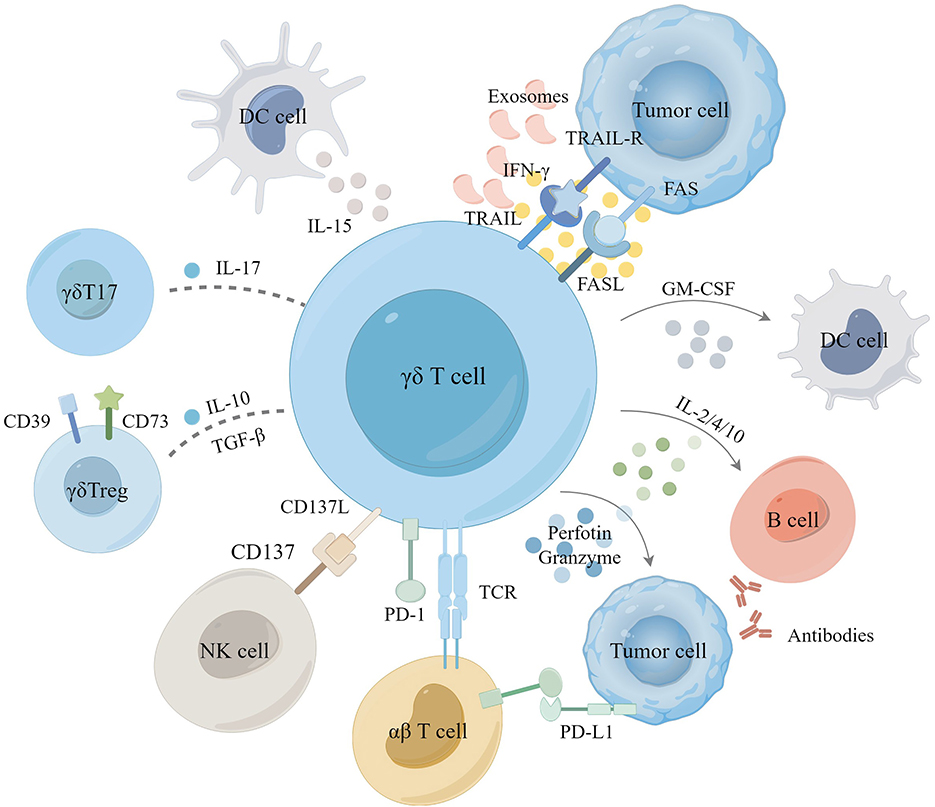
Figure 2. The role of γδ T cell in tumor microenvironment. Activation of γδ T cells induces tumor cell apoptosis primarily through the perforin-granzyme mechanism, Fas/FasL, and TRAIL pathways. Additionally, γδ T cells eliminate tumor cells by releasing cytokines such as IFN-γ and TNF-α, synergizing with the activation of αβ T cells and NK cells and promoting the conversion of antibodies in B cells as well as the antigen-presenting role of DC cells. In certain instances, γδ T cells differentiate into γδ T17 or γδ Treg cells, which secrete IL-17, IL-10, and TGF-β to facilitate the proliferation of tumor cells, playing an immunosuppressive role. Tumor-derived exosomes (TDE), immunosuppressive receptors have also been shown to modulate γδ T cell immunoregulation in TME.
γδ T cells serve as a primary and early source of pro-inflammatory cytokines upon activation, both in vitro and in vivo (71). Activated gd T cells secrete IFN-γ and TNF-α to inhibit tumor cell growth. Upon activation, they secrete IFN-γ and TNF-α, which inhibit tumor cell growth. IFN-γ release stimulates cancer stem cells (CSCs) to upregulate MHC class I molecules and intercellular cell adhesion molecule-1(ICAM-1), enhancing CD8+ T cell-mediated cytotoxicity against tumor cells (72, 73). In the presence of pAgs, IL-15-cultured dendritic cells (DCs) significantly boost the anti-tumor activity of γδ T cells through the secretion of soluble IL-15. This secretion upregulates cytotoxic molecules (CD16) and co-stimulatory molecules (CD80/86) on γδ T cells (74). Adding IL-12 and vitamin C (VitC) to the culture medium significantly enhances proliferation of γδ T cells and production of IFN-γ (75–77). Under the influence of VitC, expanded γδ T cells in vitro display heightened antitumor response in preclinical humanized mouse models and tumor cell assays (78).
γδ T cells producing IL-17 and those producing IFN-γ in the TME have opposing effects on patient prognosis (79). Elevated levels of IL-17 are closely linked with tumor metastasis and poor outcomes (80). IL-10 and transforming growth factor-β (TGF-β) within the TME facilitate the differentiation of γδ T cells into different functional subsets, such as γδT17 cells and γδTregs (81, 82). Numerous studies in mice have demonstrated that IL-17 promotes cancer progression through various mechanisms, including promoting angiogenesis, increasing endothelial cell permeability, and upregulating adhesion molecules (83). Liu et al. further reported that in multiple myeloma (MM), bone marrow stromal cells (BMSC) produce CXCL10, recruiting peripheral blood γδ T cells to the bone marrow microenvironment. Hypoxic conditions within the TME promote IL-17 secretion by γδ T cells via the SRC3/RORγt/IL-17 pathway. Interestingly, there are conflicting findings regarding colorectal cancer (CRC) (84). Wu et al. observed high levels of γδT17 cells in γδ T tumor-infiltrating lymphocytes (TILs) in CRC (83). At the same time, Meraviglia et al. found very low IL-17 secretion by γδ T cells in different CRC patient cohorts (85). However, a recent study suggested that IL-17 could be linked to antitumor activity. In a KIT-driven mouse model of gastrointestinal stromal tumor (GIST), γδ T cells were activated and highly expressed programmed cell death protein-1 (PD-1) and secreted IL-17. It was observed that γδT17 cells could be further activated to release IL-17 with tyrosine kinase inhibitors (86). The improved antitumor efficacy indicated that IL-17 might contribute to antitumor effects. Within the TME, γδTregs may suppress γδ T cell proliferation and cytotoxicity by producing immunosuppressive molecules such as IL-10, IL-8, and adenosine (ADO) (87, 88). γδTreg cells exhibit high surface expression of CD39 and CD73 (89, 90), suppressing other effector cells in an ADO-dependent manner. This suppression involves upregulating programmed cell death ligand-1 (PD-L1) and activating the STAT3 signaling pathway in DCs, leading to DCs senescence to promote tumor growth (91).
3.2 Innate immunity and antigen presentation of γδ T cells
γδ T cells, recognized for their MHC-independent activity, exhibit innate immune functions and antigen-presenting capabilities, similar to NK cells, DCs, macrophages, and B cells (92, 93). Upon activation, γδ T cells secrete pro-inflammatory cytokines and chemokines, creating an inflammatory environment that promotes the presentation of MHC class I- and II-restricted peptides on tumor cells (94). This enhances the expression of co-stimulatory molecules, such as CD80/86, and robustly stimulates CD4+/CD8+ αβ T cell activation and proliferation (95). Additionally, activated γδ T cells indirectly promote αβ T cell proliferation by co-stimulating NK cells via the ICOS/ICOS-L and CD137/CD137L pathways, thereby increasing IFN-γ and TNF-α production (96). Notably, following IL-21 and HMBPP stimulation, γδ T cells induce B cell homing, migration, and aggregation in lymph nodes, facilitating antibody production and class switching (97–99). Mature DCs synergize with ATP, IPP, and BTN3A1 to activate γδ T cells by secreting cytokines like IL-12, IL-18, IFN-γ, and TNF-α (100, 101). In turn, γδ T cells promote DC maturation by secreting IFN-γ and TNF-α, thereby enhancing the activation of both αβ and γδ T cells and amplifying antitumor responses (102). However, COX-2-expressing MSCs and Prostaglandin E2 (PGE2) from tumor cells can inhibit γδ T cell cytotoxicity (81). Furthermore, galectin-9 on γδ T cells and tumor cells drives the polarization of M2-like tumor-associated macrophages, which secrete immunosuppressive molecules that impede the antitumor activity of γδ T cells (103). In conclusion, understanding the intricate relationship between immunity effector cells and γδ T cells within the TME is crucial for harnessing the therapeutic γδ T cells in cancer treatment.
3.3 Exosome-mediated modulation of γδ T cells
The interaction between tumor-derived exosomes (TDEs) and γδ T cell responses within the TME plays a dual role in promoting and inhibiting tumor immunity (104). In vitro, stimulation of γδ T cells with TDEs significantly upregulated PD-1 expression, unaffected by miR-21 overexpression or anti-PD-L1 agents, to induce tumor immune escape. Hypoxic TDEs further enhanced the immunosuppressive functions of myeloid-derived suppressor cells (MDSCs) and inhibited γδ T cell proliferation (105). In contrast, gastric cancer cell-derived exosomes enriched with THBS1 enhanced Vγ9Vδ2 T cell cytotoxicity against gastric cancer, increasing the production of IFN-γ, TNF-α, perforin, and granzyme B both in vivo and in vitro (106). Additionally, exosomes from Vδ2 T cells (Vδ2-T-Exos) activate FasL and TRAIL pathways, effectively killing EBV-associated tumor cells while expanding EBV-specific CD4+ and CD8+ T cells. In a mouse model, administration of Vd2-T-Exos effectively controlled EBV-associated tumors (107). Despite these promising findings, further research is necessary to fully utilize exosomes for enhancing the clinical effectiveness of γδ T cells. A thorough understanding of the exact interactions and optimal utilization of TDEs may lead to more efficacious γδ T cell-based immunotherapies.
3.4 PD-1/PD-L1-mediated γδ T cell regulation
While activated γδ T cells can enhance αβ T cell responses, they may also negatively regulate them by upregulating PD-1/PD-L1 (61). Meanwhile, γδT17 cells secrete cytokines like IL-17 and TNF-α, promoting IL-6 secretion and activating the STAT3 pathway, which induces PD-L1 expression and contributes to immunosuppression (108–110). Upon stimulation by ZOL and IL-2, the PD-1 expression of Vδ2 T cells returns to baseline levels after the temporary increase (111). Research shows that PD-1-expressing γδ T cells produce less IFN-γ post-stimulation, reducing their antitumor efficacy (112). In contrast, pembrolizumab treatment rapidly expands γδ T cells, enhancing their recruitment to tumors and IFN-γ and TNF-α secretion (113). Rancan et al. showed that non-Vδ2 T cells are the primary population expressing PD-1, TIGIT, and TIM3 within tumor tissues. Higher transcriptional scores in these cells correlate with improved 5-year survival rates in patients. Additionally, Vδ2– T cells can express 4-1BB, CD39, and CTLA-4, promoting the secretion of IFN-γ, perforin, and granzymes A/K (17). Overall, γδ T cells exert antitumor effects through multiple direct and indirect mechanisms, and their demonstrated function in the tumor microenvironment makes them essential players in cancer therapy.
4 Cancer immunotherapy with γδ T cells
γδ T cells are uniquely positioned to recognize and target killing tumor cells, enriched within tumor tissues are correlated with improved clinical outcomes, under-scoring their potential as a promising target for immunotherapeutic strategies. Currently, immunotherapy for γδ T cell tumors primarily involves killing tumor cells by activated γδ T cells using cell engagers. Another approach is adoptive cellular therapies (ACTs), which involves selectively expanding γδ T cells in patients using small molecule pAgs or reintroducing in vitro-expanded allogeneic γδ T cells into the human body. Moreover, tumor-targeted activation of CAR-γδ T cells have demonstrated potential in addressing both hematological malignancies and solid tumors (114, 115).
4.1 γδ T cell engagers target and kill tumor cells directly
Cell engagers involve using monospecific or bispecific antibodies to connect γδ T cells with tumor targets, resulting in highly targeted tumor destruction. Vγ9Vδ2 T cells can interact with dystrophin via TCR-mediated interactions, and the BTN3A1 antibody induces mimicry of pAg-induced conformational changes to activate the targeting and killing of tumor cells by γδ T cells (116) (Figure 3). Payne et al. demonstrated that anti-BTN3A antibodies induced activation of Vγ9Vδ2 T cells and eliminated inhibition of αβ T cells by BTN3A1 (117). BTN3A1 binds to N-mannosylated residues in CD45 residues on the surface of αβ T cells, hindering their antigen-specific activation. The research on a humanized monoclonal antibody ICT01 targeting BTN3A indicates its ability to rapidly activate non-pAg-dependent Vγ9Vδ2 T cells migrating to tumor tissue. Initial findings from the Phase I/IIa EVICTION trial (NCT04243499; Table 1) of ICT01 in 26 patients with advanced recurrent or refractory cancers revealed a promising safety profile, with no occurrence of serious adverse events. Furthermore, BTN3 antibodies selectively enhance the antitumor function of Vγ9Vδ2 T cells and NK cells without inducing exhaustion of Vγ9Vδ2 T cells caused by ICT01 in vitro studies. These findings suggest that treatment with ICT01 can enhance the recruitment and retention of Vδ2 T cells in the TME. Compared to bisphosphonates, ICT01 has a longer plasma half-life, potentially offering greater tumor penetration (118). The EVICTION trial also explores the use of ICT01 in combination with pembrolizumab. Concurrently, an additional clinical trial EVICTION-2 (NCT05307874; Table 1) aims to assess the synergistic effects of ICT01 combined with subcutaneous IL-2 in augmenting T cell responses.
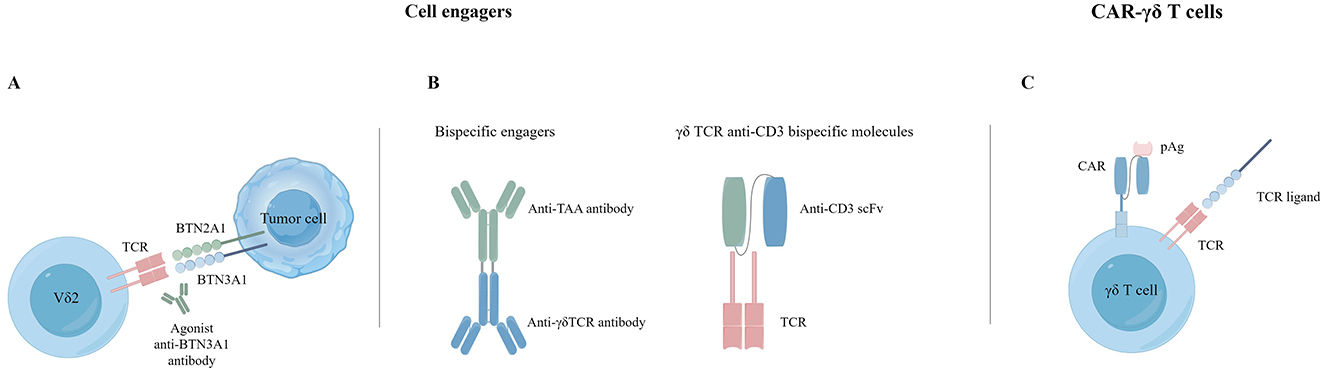
Figure 3. Cancer immunotherapy with γδ T cells. (A) Agonist antibodies targeting BTN3A1. (B) Bispecific T cell engagers. (C) CAR-γδ T cells.
Recent work by Mamedov et al. provided further insight into how AMPK regulates the expression of BTN2A1 and BTN3A, thereby influencing the cytotoxicity of γδ and αβ T cells (37). In cellular models and patient tumor tissues, small molecule activation of AMPK increased the expression of BTN2A1-BTN3A complexes and enhanced Vγ9Vδ2 TCR-mediated cytotoxicity. Ongoing clinical trials focused on TEG001 (a hyperactive Vγ9Vδ2 TCR variant, NTR6541) indicate that AMPK agonist treatment heightened the sensitivity of breast cancer organoids and Duodi cells (a typical B lymphoblast) to Vγ9Vδ2 T cell-mediated antitumor response (37). These results emphasize the profound impact of AMPK-dependent metabolic stress-induced upregulation of ligand expression on the interaction of cancer cells with the Vγ9Vδ2 TCR.
Another type γδ T cell engagers consist of two single-chain variable fragments (scFvs) that combine Vδ2 TCR specificity with tumor-targeting VHH antibodies (Figure 3). These engagers target specific molecules such as CD40, CD1d (119), EGFR, and HER2. CD40 is overexpressed on malignant B cells. Researchers have created bispecific T-cell engagers (BiTEs) targeting CD40, such as the CD40-specific Vγ9Vδ2 T cell engager (LAVA-1278). Study have demonstrated its effectiveness in activating and inducing cytotoxicity against malignant B lymphocytes in vitro and in vivo trials. The fraction targeting the CD40 receptor was observed to inhibit the CD40L-induced pro-survival signaling pathways, subsequently diminishing the resistance of Chronic Lymphocytic Leukemia (CLL) cells toward Bcl-2 inhibitors. A noticeable increase in survival rates was observed in mouse models treated with a combination therapy of both CD40 and Vγ9Vδ2 T-cell dual-specific antibodies, compared to those treated with Vγ9Vδ2 T cells alone (120). CD1d is also expressed upregulated on hematological malignant cells. A BiTE targeting CD1d and Vd2 activates Vg9Vd2 T cells and type 1 NK T cells, leading to the targeting and eliminating malignant and immunosuppressive cells through the perforin/granzyme pathway. In contrast, cytotoxicity against B cells and monocytes is relatively controllable, implying a low risk of on-target-tumor toxicity (60).
Furthermore, the EGFR-Vγ9Vδ2 TCR bispecific engager has successfully activated Vγ9Vδ2 T cells and killed tumor cells in vitro and a mouse model, extending the survival of the mice (121). Presently, LAVA-1207, a BiTE that binds with PSMA, activates Vγ9Vδ2 T cells to eliminate PSMA-expressing tumor cells effectively. This agent is currently being evaluated in a Phase I/IIa clinical trial for treating patients with metastatic castration-resistant prostate cancer (NCT05369000; Table 1).
Novel γδ TCR anti-CD3 bispecific molecules (GABs) present an innovative approach to T cell engagers (Figure 3). They link the extracellular domain of the Vγ9Vδ2 TCR, with the CD3 binding domain to form a Vγ9Vδ2 TCR-CD3 complex (122). Traditional BiTEs face challenges in efficacy against solid tumors and often induce relatively high cytokine release syndrome (CRS) toxicity. GABs can potentially reduce toxicity while achieving better efficacy and safety profiles (123). In mouse xenograft models, the Vγ9Vδ2 TCR of GABs redirects αβ T cells to tumor tissues through pAg recognition, significantly inhibiting tumor growth. Cibisatamab (RO6958688) is a GAB that binds carcinoembryonic antigen (CEA) and CD3, promoting T cell infiltration and cytokine release within tumor tissues (124). Notably, cibisatamab can also convert PD-L1-negative tumor cells to PD-L1-positive (125). Moreover, combining cibisatamab with anti-PD-L1 antibodies has demonstrated better control of tumor progression in various tumor types and mouse models (126). Current clinical trials in cell engagers are summarized in Table 1.
4.2 Adoptive cellular therapies
4.2.1 Expanding Vγ9Vδ2 T cells for enhanced tumor immunotherapy
Compared to αβ T cells, γδ T cells offer unique advantages in ACT due to their distribution across various tissues, rapid target response, and swift effector function production (127). Vδ2 T cells were the initial subset of γδ T cells tested in ACT studies. ZOL and IL-2 are the most commonly utilized stimulants. ZOL affects the isoprene biosynthesis pathway by explicitly targeting farnesyl pyrophosphate synthase (FPPS), leading to the accumulation of intracellular pAgs (128). This approach effectively expands and activates Vγ9Vδ2 T cells in vitro. Another pAg, 2-methyl-3-butenyl-1-pyrophosphate (2M3B1PP), efficiently stimulates and expands Vγ9Vδ2 T cells (129). Several clinical studies have investigated the co-administration of bisphosphonates or synthetic antibodies alongside IL-2, yet the results regarding their effectiveness in vivo have generally failed to meet expectations. Yan Xu et al. combined IL-15 and vitC with ZOL and IL-2, significantly expanding Vγ9Vδ2 T cells in vitro and improving antigen presentation to effector T cells, thus correlating with intense immune response. In addition, experiments in a mouse transplantation tumor model demonstrated that this regimen effectively suppressed tumor growth in vivo and notably extended the survival time of tumor-bearing mice (76). Using bisphosphonate prodrugs and nanotechnology-based Nitrogen-containing bisphosphonate (N-BP) delivery vectors shows great promise in enhancing Vγ9Vδ2 T cell-based immunotherapy. Bisphosphonate prodrugs utilize phosphate groups with chemical masks, facilitating the cellular entry of these compounds (130). On the other hand, nanotechnology-based drugs can enhance the efficacy of killing malignant cells by increasing NKG2D expression in Vγ9Vδ2 T cells and triggering the release of cytokines (131).
4.2.2 Allogeneic Vγ9Vδ2 and vδ1 T cells represent a dual approach to cancer therapy
Allogeneic treatment involves the transfer of allogeneic γδ T cells, which have been grown and activated outside the body, from a healthy donor to a patient with neoplastic conditions. The first team to conduct allogeneic treatment performed a clinical study on 132 patients with advanced liver and lung cancer, utilizing Vγ9Vδ2 T cells from healthy donors. The results demonstrated that after 414 cell infusions, no patients experienced severe adverse effects, with only transient and mild clinical reactions observed in some cases. Furthermore, 18 patients with liver and lung cancer who received multiple cell infusions experienced significantly prolonged survival (76). Vδ1 T cells are also emerging as potential candidates for cancer therapy. However, the lack of reliable methods for their expansion and differentiation has posed a challenge. Sebestyen's team has developed a rapid clinical translation method to produce antitumor effector Vd1 T cells, called Delta One T (DOT) cells. These cells were expanded and differentiated to increase the expression of multiple NKRs, including NKp30, NKG2D, and DNAM-1, and to maintain the expression of immunosuppressive molecules, such as PD-1 and CTLA-4, at low levels or not at all. In vitro and xenograft models have demonstrated that DOT cells exhibit significant anti-AML activity (132). The research team has initiated a clinical trial for relapsed/refractory AML (NCT05886491) and is exploring their potential applications in solid tumors. Additionally, a study has suggested that Vδ1 T cells may possess superior tumor cytotoxicity compared to Vδ2 T cells in mouse xenograft tumor models (133). Hence, a deeper understanding of the functional disparities between these two isoforms could aid in fully exploiting their respective clinical benefits. Current clinical trials in ACT using γδ T cells are summarized in Table 2.
4.2.3 CAR-modified Vδ2 and Vδ1 T cells
Genetically modified γδ T-cells (CAR) are at the forefront of cancer immunotherapy. Initially, CAR-γδ T cell therapies primarily targeted the Vδ2 subset (Figure 3). Around 20 years ago, Rischer and colleagues were the first to describe CAR-γδ T cells. They used recombinant retroviruses to introduce G(D2) or CD19-CARs γδ T cells. These cells were then expanded in a laboratory setting under ZOL activation, resulting in an enriched Vγ9Vδ2 T cell population. Upon encountering antigen-expressing tumor target cells, these cells upregulated CD69 and secreted large amounts of IFN-γ, eliminating Burkitt lymphoma cells in vitro. In subsequent studies, Deniger et al. utilized the Sleeping Beauty transposon system for gene transfer to indicate that CD19-CAR-γδ T cells could form a highly polyclonal population with dual specificity. These cells exhibited continuous proliferation, secretion of proinflammatory cytokines, improved lysis of CD19 tumor targets, and demonstrated anti-leukemic activity in xenograft mouse models. Another approach uses mRNA electroporation to modify γδ T cells, which show potent anticancer activity against CD19-positive cancer cell lines in vitro and in vivo (134).
Compared to Vδ2 T cells, Vδ1 T cells have a reduced sensitivity to their activation-induced cell death (AICD), implying a longer survival time in vivo (135). Hence, allogeneic CAR-Vδ1 T cells have recently been developed. DOT cells transduced with CD123-directed CAR showed high efficiency in inhibiting AML growth in vitro and in vivo (136). A single dose of CAR-DOT cells in combination with IL-15, achieved robust tumor control even after rechallenge. Makkouk et al. has developed CAR-Vδ1 T cells in preclinical studies that were genetically modified to target phosphatidylinositol proteoglycan 3 (glypican-3, GPC-3) and release IL-15 in laboratory conditions. This development aims to treat hepatocellular carcinoma and other solid tumors that may exhibit overexpression of GPC-3. Further research has shown that GPC-3 CAR/sIL-15 Vδ-1 T cells exhibited significant anti-tumor effects in live mouse models without inducing GvHD when used as a standalone treatment (137). Another ADI-001 targeting malignant B cells via the CD20 antigen showed an overall remission rate (ORR) of 75% in eight patients who had received multiple treatments in a Phase I study (NCT04735471; Table 1), with instances of complete remission (CR). ADI-001 exhibited considerable tolerance among subjects without reports of severe adverse reactions, demonstrating a favorable safety profile and substantial preliminary efficacy (138).
While traditional CAR-γδ T cell therapy has shown significant progress in treating certain leukemia and lymphoma patients, its effectiveness in treating solid tumors remains limited (9). Consequently, there remains a persisting necessity to enhance both the structural design and the functional efficacy of CAR-γδ T cells. Recent studies have indicated that second-generation CAR-γδ T cells expressing the CD28 co-stimulatory domain enhance IFN-γ secretion and cytotoxicity against prostate cancer cells. In mouse models, CAR-γδ T cell immunotherapy has been demonstrated to slow tumor growth, and when combined with ZOL, it enhances cytotoxicity and cytokine secretion (139).
Apart from the strategies mentioned above, new therapies based on γδ T cells are continually emerging. Murai et al. have successfully generated nearly unlimited regenerative γδ T cells from γδ T-induced pluripotent stem cells (iPSCs) (140). iPSC-derived γδ T cells (iγδ-T) demonstrate MHC-unrestricted cytotoxicity against cancer cells. However, challenges persist in clinical applications due to using heterologous serum and feeder cells in the iγδ-T induction regimen (141). Lastly, to provide a comprehensive overview of current advances in CAR-γδ T cell immunotherapy, we have compiled ongoing clinical trials as shown in Table 3. These trials cover a wide range of cancer types, further demonstrating the road promise of CAR-γδ T cells immunotherapy.
5 Conclusion
The distinctive antigen recognition mechanism and immunoregulatory function of γδ T cells render them highly advantageous in tumor immunotherapy. Nevertheless, the limited foundational research on γδ T cells restricts their therapeutic efficacy and broader clinical application. Future research should investigate the mechanisms underlying γδ T cell maturation, activation, and proliferation. It is crucial to comprehend the antigen recognition mechanisms of γδ TCRs and to identify TAAs targeted by γδ T cells. Understanding the dynamic functions of different γδ T cell subsets within the complex TME will be pivotal in optimizing their clinical use. Addressing the significant challenges in clinical trials, including the limited expansion of γδ T cells and their exhaustion post-activation, is also essential. Comprehensive development and optimization of γδ T cells from various perspectives are imperative to maximize the therapeutic potential of γδ T cell-based immunotherapy. By overcoming these challenges and leveraging the unique properties of γδ T cells, we can progress toward more effective and personalized cancer immunotherapies.
Author contributions
XL: Conceptualization, Data curation, Formal analysis, Funding acquisition, Methodology, Software, Validation, Writing – original draft, Writing – review & editing. YL: Writing – original draft, Writing – review & editing, Conceptualization. JY: Data curation, Formal analysis, Writing – review & editing. RL: Formal analysis, Methodology, Writing – review & editing. JQ: Formal analysis, Writing – review & editing. YD: Data curation, Writing – review & editing. GT: Methodology, Supervision, Writing – review & editing. CZ: Funding acquisition, Resources, Supervision, Writing – review & editing. JL: Software, Writing – review & editing. JZ: Resources, Writing – review & editing.
Funding
The author(s) declare financial support was received for the research, authorship, and/or publication of this article. This research was funded by the Clinical Research of 4310 Program (20224310NHYCG12).
Acknowledgments
Our study was approved by the ethics committee of Affiliated Nanhua Hospital, University of South China (approval No. 202011). Informed consent was obtained from all individual participants in the study. All methods were performed in accordance with the relevant guidelines.
Conflict of interest
The authors declare that the research was conducted in the absence of any commercial or financial relationships that could be construed as a potential conflict of interest.
Publisher's note
All claims expressed in this article are solely those of the authors and do not necessarily represent those of their affiliated organizations, or those of the publisher, the editors and the reviewers. Any product that may be evaluated in this article, or claim that may be made by its manufacturer, is not guaranteed or endorsed by the publisher.
References
1. Waldman AD, Fritz JM, Lenardo MJ. A guide to cancer immunotherapy: from T cell basic science to clinical practice. Nat Rev Immunol. (2020) 20:651–68. doi: 10.1038/s41577-020-0306-5
2. Hayday AC, Saito H, Gillies SD, Kranz DM, Tanigawa G, Eisen HN, et al. Structure, organization, and somatic rearrangement of T cell gamma genes. Cell. (1985) 40:259–69. doi: 10.1016/0092-8674(85)90140-0
3. Girardi M, Oppenheim DE, Steele CR, Lewis JM, Glusac E, Filler R, et al. Regulation of cutaneous malignancy by gammadelta T Cells. Science. (2001) 294:605–9. doi: 10.1126/science.1063916
4. Strid J, Roberts SJ, Filler RB, Lewis JM, Kwong BY, Schpero W, et al. Acute upregulation of an NKG2D ligand promotes rapid reorganization of a local immune compartment with pleiotropic effects on carcinogenesis. Nat Immunol. (2008) 9:146–54. doi: 10.1038/ni1556
5. Luoma AM, Castro CD, Adams, EJ. Γδ T cell surveillance via CD1 molecules. Trends Immunol. (2014) 35:613–21. doi: 10.1016/j.it.2014.09.003
6. Silva-Santos B, Mensurado S, Coffelt SB. Γδ T cells: pleiotropic immune effectors with therapeutic potential in cancer. Nat Rev Cancer. (2019) 19:392–404. doi: 10.1038/s41568-019-0153-5
7. Ma Y, Aymeric L, Locher C, Mattarollo SR, Delahaye NF, Pereira P, et al. Contribution of IL-17-producing gamma delta T cells to the efficacy of anticancer chemotherapy. J Exp Med. (2011) 208:491–503. doi: 10.1084/jem.20100269
8. Dolgin E. Unconventional Γδ T cells “the new black” in cancer therapy. Nat Biotechnol. (2022) 40:805–8. doi: 10.1038/s41587-022-01363-6
9. Ganapathy T, Radhakrishnan R, Sakshi S, Martin S. CAR Γδ T cells for cancer immunotherapy is the field more yellow than green? Cancer Immunol Immunother. (2023) 72:277–86. doi: 10.1007/s00262-022-03260-y
10. Wang L, Chen X, Zhang L, Niu B, Li L, Sun Y, Yuan X. CAR cell design strategies in solid tumors. Int Immunopharmacol. (2022) 113:109345. doi: 10.1016/j.intimp.2022.109345
11. Hinz T, Wesch D, Halary F, Marx S, Choudhary A, Arden B, et al. Identification of the complete expressed human TCR V gamma repertoire by flow cytometry. Int Immunol. (1997) 9:1065–72. doi: 10.1093/intimm/9.8.1065
12. Gray JI, Caron DP, Wells SB, Guyer R, Szabo P, Rainbow D, et al. Human Γδ T cells in diverse tissues exhibit site-specific maturation dynamics across the life span. Sci Immunol. (2024) 9:eadn3954. doi: 10.1126/sciimmunol.adn3954
13. Nielsen MM, Witherden DA, Havran WL. Γδ T cells in homeostasis and host defence of epithelial barrier tissues. Nat Rev Immunol. (2017) 17:733–45. doi: 10.1038/nri.2017.101
14. McVay LD, Carding SR. Extrathymic origin of human gamma delta T cells during fetal development. J Immunol. (1996) 157:2873–82. doi: 10.4049/jimmunol.157.7.2873
15. Deusch K, Lüling F, Reich K, Classen M, Wagner H. Pfeffer KA. Major fraction of human intraepithelial lymphocytes simultaneously expresses the gamma/delta T cell receptor, the CD8 accessory molecule and preferentially uses the V delta 1 gene segment. Eur J Immunol. (1991) 21:1053–9. doi: 10.1002/eji.1830210429
16. Davey MS, Willcox CR, Hunter S, Kasatskaya SA, Remmerswaal EBM, Salim M, et al. The human Vδ2+ T-cell compartment comprises distinct innate-like Vγ9+ and adaptive Vγ9- subsets. Nat Commun. (2018) 9:1760. doi: 10.1038/s41467-018-04076-0
17. Rancan C, Arias-Badia M, Dogra P, Chen B, Aran D, Yang H, et al. Exhausted intratumoral Vδ2- Γδ T cells in human kidney cancer retain effector function. Nat Immunol. (2023) 24:612–24. doi: 10.1038/s41590-023-01448-7
18. Holtmeier W, Pfänder M, Hennemann A, Zollner TM, Kaufmann R, Caspary WF. The TCR-delta repertoire in normal human skin is restricted and distinct from the TCR-delta repertoire in the peripheral blood. J Invest Dermatol. (2001) 116:275–80. doi: 10.1046/j.1523-1747.2001.01250.x
19. Carding SR, Egan, PJ. Gammadelta T cells: functional plasticity and heterogeneity. Nat Rev Immunol. (2002) 2:336–45. doi: 10.1038/nri797
20. Bauer S, Groh V, Wu J, Steinle A, Phillips JH, Lanier LL, et al. Activation of NK cells and T cells by NKG2D, a receptor for stress-inducible MICA. Science. (1999) 285:727–9. doi: 10.1126/science.285.5428.727
21. Poggi A, Venturino C, Catellani S, Clavio M, Miglino M, Gobbi M, et al. Vdelta1 T lymphocytes from B-CLL patients recognize ULBP3 expressed on leukemic B cells and up-regulated by trans-retinoic acid. Cancer Res. (2004) 64:9172–9. doi: 10.1158/0008-5472.CAN-04-2417
22. Correia DV, Fogli M, Hudspeth K, da Silva MG, Mavilio D, Silva-Santos B. Differentiation of human peripheral blood Vδ1+ T cells expressing the natural cytotoxicity receptor NKp30 for recognition of lymphoid leukemia cells. Blood. (2011) 118:992–1001. doi: 10.1182/blood-2011-02-339135
23. Knight A, Mackinnon S. Lowdell MW. Human Vdelta1 gamma-delta T cells exert potent specific cytotoxicity against primary multiple myeloma cells. Cytotherapy. (2012) 14:1110–8. doi: 10.3109/14653249.2012.700766
24. Kabelitz D, Kalyan S, Oberg H.-H, Wesch D. Human Vδ2 versus non-Vδ2 Γδ T cells in antitumor. Immunity Oncoimmunology. (2013) 2:e23304. doi: 10.4161/onci.23304
25. Rice MT, von Borstel A, Chevour P, Awad W, Howson LJ, Littler DR, et al. Recognition of the antigen-presenting molecule MR1 by a Vδ3+ Γδ T cell receptor. Proc Natl Acad Sci U S A. (2021) 118:e2110288118. doi: 10.1073/pnas.2110288118
26. Shen L, Huang D, Qaqish A, Frencher J, Yang R, Shen H, et al. Fast-acting Γδ T-cell subpopulation and protective immunity against infections. Immunol Rev. (2020) 298:254–63. doi: 10.1111/imr.12927
27. Eberl M, Hintz M, Reichenberg A, Kollas, A.-K., Wiesner J, Jomaa, H. Microbial isoprenoid biosynthesis and human gammadelta T Cell activation. FEBS Lett. (2003) 544:4–10. doi: 10.1016/S0014-5793(03)00483-6
28. Moulin M, Alguacil J, Gu S, Mehtougui A, Adams EJ, Peyrottes S, et al. Vγ9Vδ2 T cell activation by strongly agonistic nucleotidic phosphoantigens. Cell Mol Life Sci. (2017) 74:4353–67. doi: 10.1007/s00018-017-2583-0
29. Garavaglia B, Vallino L, Ferraresi A, Esposito A, Salwa A, Vidoni C, et al. Butyrate inhibits colorectal cancer cell proliferation through autophagy degradation of beta-catenin regardless of APC and beta-catenin mutational status. Biomedicines. (2022) 10:1131. doi: 10.3390/biomedicines10051131
30. Umeyama Y, Taniguchi H, Gyotoku H, Senju H, Tomono H, Takemoto S, et al. Three distinct mechanisms underlying human Γδ T cell-mediated cytotoxicity against malignant pleural mesothelioma. Front Immunol. (2023) 14:1058838. doi: 10.3389/fimmu.2023.1058838
31. Rigau M, Ostrouska S, Fulford TS, Johnson DN, Woods K, Ruan Z, et al. Butyrophilin 2A1 Is essential for phosphoantigen reactivity by Γδ T cells. Science. (2020) 367:eaay5516. doi: 10.1126/science.aay5516
32. Harly C, Guillaume Y, Nedellec S, Peigné C-M, Mönkkönen H, Mönkkönen J, et al. Key implication of CD277/butyrophilin-3(BTN3A) in cellular stress sensing by a major human Γδ T-cell subset. Blood. (2012) 120:2269–79. doi: 10.1182/blood-2012-05-430470
33. Sandstrom A, Peigné C-M, Léger A, Crooks JE, Konczak F, Gesnel M-C, et al. The intracellular B302 domain of butyrophilin 3A1 binds phosphoantigens to mediate activation of human Vγ9Vδ2 T cells. Immunity. (2014) 40:490–500. doi: 10.1016/j.immuni.2014.03.003
34. Karunakaran MM, Subramanian H, Jin Y, Mohammed F, Kimmel B, Juraske C, et al. A distinct topology of BTN3A IgV and B302 domains controlled by juxtamembrane regions favors optimal human Γδ T Cell phosphoantigen sensing. Nat Commun. (2023) 14:7617. doi: 10.1038/s41467-023-41938-8
35. Salim M, Knowles TJ, Baker AT, Davey MS, Jeeves M, Sridhar P, et al. BTN3A1 discriminates Γδ T cell phosphoantigens from nonantigenic small molecules via a conformational sensor in its B302 domain ACS. Chem Biol. (2017) 12:2631–43. doi: 10.1021/acschembio.7b00694
36. Yuan L, Ma X, Yang Y, Qu Y, Li X, Zhu X, et al. Phosphoantigens glue butyrophilin 3A1 and 2A1 to activate Vγ9Vδ2 T cells. Nature. (2023) 621:840–8. doi: 10.1038/s41586-023-06525-3
37. Mamedov MR, Vedova S, Freimer JW, Sahu AD, Ramesh A, Arce MM, et al. CRISPR screens decode cancer cell pathways that trigger Γδ T cell detection. Nature. (2023) 621:188–95. doi: 10.1038/s41586-023-06482-x
38. Scotet E, Martinez LO, Grant E, Barbaras R, Jenö P, Guiraud M, et al. Tumor recognition following Vgamma9Vdelta2 T cell receptor interactions with a surface F1-ATPase-related structure and apolipoprotein A-I. Immunity. (2005) 22:71–80. doi: 10.1016/j.immuni.2004.11.012
39. Mookerjee-Basu J, Vantourout P, Martinez LO, Perret B, Collet X, Périgaud C, et al. F1-adenosine triphosphatase displays properties characteristic of an antigen presentation molecule for Vgamma9Vdelta2 T cells. J Immunol. (2010) 184:6920–8. doi: 10.4049/jimmunol.0904024
40. Dai Y, Chen H, Mo C, Cui L, He W. Ectopically expressed human tumor biomarker MutS homologue 2 is a novel endogenous ligand that is recognized by human Γδ T cells to induce innate anti-tumor/virus immunity. J Biol Chem. (2012) 287:16812–9. doi: 10.1074/jbc.M111.327650
41. Dai Y-M, Liu HY, Liu Y-F, Zhang Y, He W. EBV transformation induces overexpression of hMSH2/3/6 on B lymphocytes and enhances γδT-cell-mediated cytotoxicity via TCR and NKG2D. Immunology. (2018) 154:673–82. doi: 10.1111/imm.12920
42. Chen H, Ji X, Cui L, Zhang J, He W. Characterization of complementary determinant region 3δ in human MutS homologue 2-specific Γδ T cells. Scand J Immunol. (2015) 81:121–8. doi: 10.1111/sji.12256
43. Dhar P, Wu, JD. NKG2D and its ligands in cancer. Curr Opin Immunol. (2018) 51:55–61. doi: 10.1016/j.coi.2018.02.004
44. Wrobel P, Shojaei H, Schittek B, Gieseler F, Wollenberg B, Kalthoff H, et al. Lysis of a broad range of epithelial tumour cells by human gamma delta T cells: involvement of NKG2D ligands and T-cell receptor- versus NKG2D-dependent recognition. Scand J Immunol. (2007) 66:320–8. doi: 10.1111/j.1365-3083.2007.01963.x
45. El-Gazzar A, Groh V. Spies, immunobiology T, and conflicting roles of the human NKG2D lymphocyte receptor and its ligands in cancer. J Immunol. (2013) 191:1509–15. doi: 10.4049/jimmunol.1301071
46. Mensurado S, Condeço C, Sánchez-Martínez D, Shirley S, Coelho RML, Tirado N, et al. CD155/PVR determines acute myeloid leukemia targeting by delta one T cells. Blood. (2024) 143:1488–95. doi: 10.1182/blood.2023022992
47. Choi H, Lee Y, Park SA, Lee JH, Park J, Park JH, et al. Human allogenic Γδ T cells kill patient-derived glioblastoma cells expressing high levels of DNAM-1 ligands. Oncoimmunology. (2022) 11:2138152. doi: 10.1080/2162402X.2022.2138152
48. Niu C, Jin H, Li M, Xu J, Xu D, Hu J, et al. In vitro analysis of the proliferative capacity and cytotoxic effects of ex vivo induced natural killer cells, cytokine-induced killer cells, and gamma-delta T cells. BMC Immunol. (2015) 16:61. doi: 10.1186/s12865-015-0124-x
49. Guerra N, Tan YX, Joncker NT, Choy A, Gallardo F, Xiong N, et al. NKG2D-deficient mice are defective in tumor surveillance in models of spontaneous malignancy. Immunity. (2008) 28:571–80. doi: 10.1016/j.immuni.2008.02.016
50. Oppenheim DE, Roberts SJ, Clarke SL, Filler R, Lewis JM, Tigelaar RE, et al. Sustained localized expression of ligand for the activating NKG2D receptor impairs natural cytotoxicity in vivo and reduces tumor immunosurveillance. Nat Immunol. (2005) 6:928–37. doi: 10.1038/ni1239
51. Niu C, Jin H, Li M, Zhu S, Zhou L, Jin F, et al. Low-dose bortezomib increases the expression of NKG2D and DNAM-1 ligands and enhances induced NK and Γδ T cell-mediated lysis in multiple myeloma. Oncotarget. (2017) 8:5954–64. doi: 10.18632/oncotarget.13979
52. Wang X, Mou W, Han W, Xi Y, Chen X, Zhang H, et al. Diminished cytolytic activity of Γδ T cells with reduced DNAM-1 expression in neuroblastoma patients. Clin Immunol. (2019) 203:63–71. doi: 10.1016/j.clim.2019.04.006
53. Castro CD, Boughter CT, Broughton AE, Ramesh A, Adams, E. Diversity in recognition and function of human Γδ T cells. Immunol Rev. (2020) 298:134–52. doi: 10.1111/imr.12930
54. Chong TW, Goh FY, Sim MY, Huang HH, Thike AA, Lim WK, et al. CD1d expression in renal cell carcinoma is associated with higher relapse rates, poorer cancer-specific and overall survival. J Clin Pathol. (2015) 68:200–5. doi: 10.1136/jclinpath-2014-202735
55. Liu D, Song L, Brawley VS, Robison N, Wei J, Gao X, et al. Medulloblastoma expresses CD1d and can be targeted for immunotherapy with NKT cells. Clin Immunol. (2013) 149:55–64. doi: 10.1016/j.clim.2013.06.005
56. Hara A, Koyama-Nasu R, Takami M, Toyoda T, Aoki T, Ihara F, et al. CD1d expression in glioblastoma is a promising target for NKT cell-based cancer immunotherapy. Cancer Immunol Immunother. (2021) 70:1239–54. doi: 10.1007/s00262-020-02742-1
57. Dhodapkar MV, Geller MD, Chang DH, Shimizu K, Fujii S-I, Dhodapkar KM, et al. A reversible defect in natural killer T cell function characterizes the progression of premalignant to malignant multiple myeloma. J Exp Med. (2003) 197:1667–76. doi: 10.1084/jem.20021650
58. Shyanti RK, Sehrawat A, Singh SV, Mishra JPN, Singh, R. Zerumbone modulates CD1d expression and lipid antigen presentation pathway in breast cancer cells. Toxicol In vitro. (2017) 44:74–84. doi: 10.1016/j.tiv.2017.06.016
59. Nowak M, Arredouani MS, Tun-Kyi A, Schmidt-Wolf I, Sanda MG, Balk SP, et al. Defective NKT cell activation by CD1d+ TRAMP prostate tumor cells is corrected by interleukin-12 with α-galactosylceramide. PLoS ONE. (2010) 5:e11311. doi: 10.1371/journal.pone.0011311
60. Lameris R, Ruben JM, Iglesias-Guimarais V, de Jong M, Veth M, van de Bovenkamp FS, et al. A bispecific T cell engager recruits both type 1 NKT and Vγ9Vδ2-T cells for the treatment of CD1d-expressing hematological malignancies. Cell Rep Med. (2023) 4:100961. doi: 10.1016/j.xcrm.2023.100961
61. Kabelitz D, Serrano R, Kouakanou L, Peters C, Kalyan, S. Cancer immunotherapy with Γδ T cells: many paths ahead of us. Cell Mol Immunol. (2020) 17:925–39. doi: 10.1038/s41423-020-0504-x
62. Di Lorenzo B, Simões AE, Caiado F, Tieppo P, Correia DV, Carvalho T, et al. Broad cytotoxic targeting of acute myeloid leukemia by polyclonal delta one T cells. Cancer Immunol Res. (2019) 7:552–8. doi: 10.1158/2326-6066.CIR-18-0647
63. Mikulak J, Oriolo F, Bruni E, Roberto A, Colombo FS, Villa A, et al. NKp46-expressing human gut-resident intraepithelial Vδ1 T cell subpopulation exhibits high antitumor activity against colorectal cancer. JCI Insight. (2019) 4:e125884. doi: 10.1172/jci.insight.125884
64. Xu B, Pizarro JC, Holmes MA, McBeth C, Groh V, Spies T, et al. Crystal structure of a gammadelta T-cell receptor specific for the human MHC class I homolog MICA. Proc Natl Acad Sci U S A. (2011) 108:2414–9. doi: 10.1073/pnas.1015433108
65. Hull CM, Larcombe-Young D, Mazza R, George M, Davies DM, Schurich A, et al. Granzyme B-activated IL18 potentiates Aβ and Γδ CAR T cell immunotherapy in a tumor-dependent manner. Mol Ther. (2024) 2014:S1525-0016(24)00315-0. doi: 10.1016/j.ymthe.2024.05.013
66. Zhang T, Wang J, Zhao A, Xia L, Jin H, Xia S, et al. The way of interaction between Vγ9Vδ2 T cells and tumor cells. Cytokine. (2023) 162:156108. doi: 10.1016/j.cyto.2022.156108
67. Meraviglia S, Caccamo N, Guggino G, Tolomeo M, Siragusa S, Stassi G, et al. Optimizing tumor-reactive Γδ T cells for antibody-based cancer immunotherapy. Curr Mol Med. (2010) 10:719–26. doi: 10.2174/156652410793384150
68. Himoudi N, Morgenstern DA, Yan M, Vernay B, Saraiva L, Wu Y, et al. Human Γδ T lymphocytes are licensed for professional antigen presentation by interaction with opsonized target cells. J Immunol. (2012) 188:1708–16. doi: 10.4049/jimmunol.1102654
69. Street SEA, Hayakawa Y, Zhan Y, Lew AM, MacGregor D, Jamieson AM, et al. Innate immune surveillance of spontaneous B cell lymphomas by natural killer cells and gammadelta T cells. J Exp Med. (2004) 199:879–84. doi: 10.1084/jem.20031981
70. Ribot JC, Ribeiro ST, Correia DV, Sousa AE, Silva-Santos B. Human Γδ thymocytes are functionally immature and differentiate into cytotoxic type 1 effector T cells upon IL-2/IL-15 signaling. J Immunol. (2014) 192:2237–43. doi: 10.4049/jimmunol.1303119
71. Chen H-C, Joalland N, Bridgeman JS, Alchami FS, Jarry U, Khan MWA, et al. Synergistic targeting of breast cancer stem-like cells by human Γδ T cells and CD8+ T cells. Immunol Cell Biol. (2017) 95:620–9. doi: 10.1038/icb.2017.21
72. Fiala GJ, Lücke J, Huber S. Pro- and antitumorigenic functions of Γδ T cells. Eur J Immunol. (2024) 2024:e2451070. doi: 10.1002/eji.202451070
73. Todaro M, D'Asaro M, Caccamo N, Iovino F, Francipane MG, Meraviglia S, et al. Efficient killing of human colon cancer stem cells by gammadelta T lymphocytes. J Immunol. (2009) 182:7287–96. doi: 10.4049/jimmunol.0804288
74. Van Acker HH, Anguille S, De Reu H, Berneman ZN, Smits EL, Van Tendeloo, VF. Interleukin-15-cultured dendritic cells enhance anti-tumor gamma delta T cell functions through IL-15 secretion. Front Immunol. (2018) 9:658. doi: 10.3389/fimmu.2018.00658
75. Kabelitz D, Cierna L, Juraske C, Zarobkiewicz M, Schamel WW, Peters, C. Empowering Γδ T-cell functionality with vitamin C. Eur J Immunol. (2024) 54:e2451028. doi: 10.1002/eji.202451028
76. Xu Y, Xiang Z, Alnaggar M, Kouakanou L, Li J, He J, et al. Allogeneic Vγ9Vδ2 T-cell immunotherapy exhibits promising clinical safety and prolongs the survival of patients with late-stage lung or liver cancer. Cell Mol Immunol. (2021) 18:427–39. doi: 10.1038/s41423-020-0515-7
77. Assy L, Khalil SM, Attia M, Salem ML. IL-12 conditioning of peripheral blood mononuclear cells from breast cancer patients promotes the zoledronate-induced expansion of Γδ T cells in vitro and enhances their cytotoxic activity and cytokine production. Int Immunopharmacol. (2023) 114:109402. doi: 10.1016/j.intimp.2022.109402
78. Kouakanou L, Xu Y, Peters C, He J, Wu Y, Yin Z, et al. Vitamin C promotes the proliferation and effector functions of human Γδ T cells. Cell Mol Immunol. (2020) 17:462–73. doi: 10.1038/s41423-019-0247-8
79. Patil RS, Shah SU, Shrikhande SV, Goel M, Dikshit RP, Chiplunkar SV. IL17 producing γδT cells induce angiogenesis and are associated with poor survival in gallbladder cancer patients. Int J Cancer. (2016) 139:869–81. doi: 10.1002/ijc.30134
80. Jin C, Lagoudas GK, Zhao C, Bullman S, Bhutkar A, Hu B, et al. Commensal microbiota promote lung cancer development via Γδ T cells. Cell. (2019) 176:998–1013.e16. doi: 10.1016/j.cell.2018.12.040
81. Wesch D, Kabelitz D, Oberg H-H. Tumor resistance mechanisms and their consequences on Γδ T cell activation. Immunol Rev. (2020) 298:84–98. doi: 10.1111/imr.12925
82. Zuberbuehler MK, Parker ME, Wheaton JD, Espinosa JR, Salzler HR, Park E. Ciofani, M. The transcription factor C-Maf is essential for the commitment of IL-17-producing Γδ T cells. Nat Immunol. (2019) 20:73–85. doi: 10.1038/s41590-018-0274-0
83. Wu P, Wu D, Ni C, Ye J, Chen W, Hu G, et al. γδT17 cells promote the accumulation and expansion of myeloid-derived suppressor cells in human colorectal cancer. Immunity. (2014) 40:785–800. doi: 10.1016/j.immuni.2014.03.013
84. Wang J, Peng Z, Guo J, Wang Y, Wang S, Jiang H, et al. CXCL10 recruitment of Γδ T cells into the hypoxic bone marrow environment leads to IL17 expression and multiple myeloma progression. Cancer Immunol Res. (2023) 11:1384–99. doi: 10.1158/2326-6066.CIR-23-0088
85. Meraviglia S, Lo Presti E, Tosolini M, La Mendola C, Orlando V, Todaro M, et al. Distinctive features of tumor-infiltrating Γδ T lymphocytes in human colorectal cancer. Oncoimmunology. (2017) 6:e1347742. doi: 10.1080/2162402X.2017.1347742
86. Etherington MS, Hanna AN, Medina BD, Liu M, Tieniber AD, Kwak HV, et al. Tyrosine kinase inhibition activates intratumoral Γδ T cells in gastrointestinal stromal tumor. Cancer Immunol Res. (2024) 12:107–19. doi: 10.1158/2326-6066.CIR-23-0061
87. Jeong J.-H, Zhong S, Li F, Huang C, Chen X, Liu Q, et al. Tumor-derived OBP2A promotes prostate cancer castration resistance. J Exp Med. (2023) 220:e20211546. doi: 10.1084/jem.20211546
88. Ye J, Ma C, Wang F, Hsueh EC, Toth K, Huang Y, et al. Specific recruitment of Γδ regulatory T cells in human breast cancer. Cancer Res. (2013) 73:6137–48. doi: 10.1158/0008-5472.CAN-13-0348
89. Weimer P, Wellbrock J, Sturmheit T, Oliveira-Ferrer L, Ding Y, Menzel S, et al. Tissue-specific expression of TIGIT, PD-1, TIM-3, and CD39 by Γδ T cells in ovarian cancer. Cells. (2022) 11:964. doi: 10.3390/cells11060964
90. Hu G, Cheng P, Pan J, Wang S, Ding Q, Jiang Z, et al. An IL6-adenosine positive feedback loop between CD73+ γδTregs and CAFs promotes tumor progression in human breast cancer. Cancer Immunol Res. (2020) 8:1273–86. doi: 10.1158/2326-6066.CIR-19-0923
91. Si F, Liu X, Tao Y, Zhang Y, Ma F, Hsueh EC, et al. Peng, blocking senescence G, and tolerogenic function of dendritic cells induced by Γδ Treg cells enhances tumor-specific immunity for cancer immunotherapy. J Immunother Cancer. (2024) 12:e008219. doi: 10.1136/jitc-2023-008219
92. Peng G, Wang HY, Peng W, Kiniwa Y, Seo KH, Wang R-F. Tumor-infiltrating gammadelta T cells suppress T and dendritic cell function via mechanisms controlled by a unique toll-like receptor signaling pathway. Immunity. (2007) 27:334–48. doi: 10.1016/j.immuni.2007.05.020
93. Petrasca A, Melo AM, Breen EP, Doherty DG. Human Vδ3+ Γδ T cells induce maturation and IgM secretion by B cells. Immunol Lett. (2018) 196:126–34. doi: 10.1016/j.imlet.2018.02.002
94. Tirier SM, Mallm J-P, Steiger S, Poos AM, Awwad MHS, Giesen N, et al. Subclone-specific microenvironmental impact and drug response in refractory multiple myeloma revealed by single-cell transcriptomics. Nat Commun. (2021) 12:6960. doi: 10.1038/s41467-021-26951-z
95. Ye J, Ma C, Hsueh EC, Eickhoff CS, Zhang Y, Varvares MA, et al. Tumor-derived Γδ regulatory T cells suppress innate and adaptive immunity through the induction of immunosenescence. J Immunol. (2013) 190:2403–14. doi: 10.4049/jimmunol.1202369
96. Cairo C, Surendran N, Harris KM, Mazan-Mamczarz K, Sakoda Y, Diaz-Mendez F, et al. Vγ2Vδ2 T cell costimulation increases NK cell killing of monocyte-derived dendritic cells. Immunology. (2014) 144:422–30. doi: 10.1111/imm.12386
97. Bansal RR, Mackay CR, Moser B, Eberl, M. IL-21 enhances the potential of human Γδ T cells to provide B-cell help. Eur J Immunol. (2012) 42:110–9. doi: 10.1002/eji.201142017
98. Rezende RM, Lanser AJ, Rubino S, Kuhn C, Skillin N, Moreira TG, et al. Γδ T Cells control humoral immune response by inducing T follicular helper cell differentiation. Nat Commun. (2018) 9:3151. doi: 10.1038/s41467-018-05487-9
99. Vermijlen D, Ellis P, Langford C, Klein A, Engel R, Willimann K, et al. Distinct cytokine-driven responses of activated blood gammadelta T cells: insights into unconventional T cell pleiotropy. J Immunol. (2007) 178:4304–14. doi: 10.4049/jimmunol.178.7.4304
100. Devilder MC, Maillet S, Bouyge-Moreau I, Donnadieu E, Bonneville M, Scotet E. Potentiation of antigen-stimulated V gamma 9V delta 2 T cell cytokine production by immature dendritic cells(DC) and reciprocal effect on DC maturation. J Immunol. (2006) 176:1386–93. doi: 10.4049/jimmunol.176.3.1386
101. Münz C, Steinman RM, Fujii S. Dendritic cell maturation by innate lymphocytes: coordinated stimulation of innate and adaptive immunity. J Exp Med. (2005) 202:203–7. doi: 10.1084/jem.20050810
102. Galati D, Zanotta S, Bocchino M, De Filippi R, Pinto A. The subtle interplay between gamma delta t lymphocytes and dendritic cells: is there a role for a therapeutic cancer vaccine in the era of combinatorial strategies? Cancer Immunol Immunother. (2021) 70:1797–809. doi: 10.1007/s00262-020-02805-3
103. Mao Y, Yin S, Zhang J, Hu Y, Huang B, Cui L, et al. A new effect of IL-4 on human Γδ T cells: promoting regulatory Vδ1 T cells via IL-10 production and inhibiting function of Vδ2 T cells. Cell Mol Immunol. (2016) 13:217–28. doi: 10.1038/cmi.2015.07
104. Yang E, Wang X, Gong Z, Yu M, Wu H, Zhang D. Exosome-mediated metabolic reprogramming: the emerging role in tumor microenvironment remodeling and its influence on cancer progression signal. Transduct Target Ther. (2020) 5:242. doi: 10.1038/s41392-020-00359-5
105. Li L, Cao B, Liang X, Lu S, Luo H, Wang Z, et al. Microenvironmental oxygen pressure orchestrates an anti- and pro-tumoral Γδ T cell equilibrium via tumor-derived exosomes. Oncogene. (2019) 38:2830–43. doi: 10.1038/s41388-018-0627-z
106. Li J, Feng H, Zhu J, Yang K, Zhang G, Gu Y, et al. Gastric cancer derived exosomal THBS1 enhanced Vγ9Vδ2 T-cell function through activating RIG-I-like receptor signaling pathway in a N6-methyladenosine methylation dependent manner. Cancer Lett. (2023) 576:216410. doi: 10.1016/j.canlet.2023.216410
107. Wang X, Xiang Z, Liu Y, Huang C, Pei Y, Wang X, et al. Exosomes derived from Vδ2-T cells control epstein-barr virus-associated tumors and induce T cell antitumor immunity. Sci Transl Med. (2020) 12:eaaz3426. doi: 10.1126/scitranslmed.aaz3426
108. Zhang Z, Yang C, Li L, Zhu Y, Su K, Zhai L, et al. “γδT Cell-IL17A-Neutrophil” axis drives immunosuppression and confers breast cancer resistance to high-dose anti-VEGFR2 therapy. Front Immunol. (2021) 12:699478. doi: 10.3389/fimmu.2021.699478
109. Lopes N. Silva-Santos B. Functional B, and metabolic dichotomy of murine Γδ T cell subsets in cancer immunity. Eur J Immunol. (2021) 51:17–26. doi: 10.1002/eji.201948402
110. Aotsuka A, Matsumoto Y, Arimoto T, Kawata A, Ogishima J, Taguchi A, et al. Interleukin-17 is associated with expression of programmed cell death 1 ligand 1 in ovarian carcinoma. Cancer Sci. (2019) 110:3068–78. doi: 10.1111/cas.14174
111. Zumwalde NA, Sharma A, Xu X, Ma S, Schneider CL, Romero-Masters JC, et al. Adoptively transferred Vγ9Vδ2 T cells show potent antitumor effects in a preclinical B cell lymphomagenesis model. JCI Insight. (2017) 2:e93179. doi: 10.1172/jci.insight.93179
112. Iwasaki M, Tanaka Y, Kobayashi H, Murata-Hirai K, Miyabe H, Sugie T, et al. Minato, expression N, and function of PD-1 in human Γδ T cells that recognize phosphoantigens. Eur J Immunol. (2011) 41:345–55. doi: 10.1002/eji.201040959
113. Lien SC, Ly D, Yang SYC, Wang BX, Clouthier DL, St Paul M, et al. Tumor reactive Γδ T Cells contribute to a complete response to PD-1 blockade in a merkel cell carcinoma patient. Nat Commun. (2024) 15:1094. doi: 10.1038/s41467-024-45449-y
114. Rimailho L, Faria C, Domagala M, Laurent C, Bezombes C, Poupot, M. Γδ T cells in immunotherapies for B-cell malignancies. Front Immunol. (2023) 14:1200003. doi: 10.3389/fimmu.2023.1200003
115. Papadakos SP, Arvanitakis K, Stergiou IE, Koutsompina ML, Germanidis G, Theocharis S. Γδ T cells: a game changer in the future of hepatocellular carcinoma immunotherapy. Int J Mol Sci. (2024) 25:1381. doi: 10.3390/ijms25031381
116. Palakodeti A, Sandstrom A, Sundaresan L, Harly C, Nedellec S, Olive D, et al. The molecular basis for modulation of human Vγ9Vδ2 T cell responses by CD277/butyrophilin-3(BTN3A)-specific antibodies. J Biol Chem. (2012) 287:32780–90. doi: 10.1074/jbc.M112.384354
117. Payne KK, Mine JA, Biswas S, Chaurio RA, Perales-Puchalt A, Anadon CM, et al. BTN3A1 governs antitumor responses by coordinating Aβ and Γδ T cells. Science. (2020) 369:942–9. doi: 10.1126/science.aay2767
118. De Gassart A, Le, K.-S., Brune P, Agaugué S, Sims J, Goubard A, et al. Development of ICT01, a first-in-class, anti-BTN3A antibody for activating Vγ9Vδ2 T cell-mediated antitumor immune response. Sci Transl Med. (2021) 13:eabj0835. doi: 10.1126/scitranslmed.abj0835
119. de Weerdt I, Lameris R, Ruben JM, de Boer R, Kloosterman J, King LA, et al. A bispecific single-domain antibody boosts autologous Vγ9Vδ2-T cell responses toward CD1d in chronic lymphocytic leukemia. Clin Cancer Res. (2021) 27:1744–55. doi: 10.1158/1078-0432.CCR-20-4576
120. de Weerdt I, Lameris R, Scheffer GL, Vree J, de Boer R, Stam AG, et al. A bispecific antibody antagonizes prosurvival CD40 signaling and promotes Vγ9Vδ2 T Cell-mediated antitumor responses in human B-Cell malignancies. Cancer Immunol Res. (2021) 9:50–61. doi: 10.1158/2326-6066.CIR-20-0138
121. de Bruin RCG, Veluchamy JP, Lougheed SM, Schneiders FL, Lopez-Lastra S, Lameris R, et al. A bispecific nanobody approach to leverage the potent and widely applicable tumor cytolytic capacity of Vγ9Vδ2-T cells. Oncoimmunology. (2017) 7:e1375641. doi: 10.1080/2162402X.2017.1375641
122. Xin W, Huang B, Chi X, Liu Y, Xu M, Zhang Y, et al. Structures of human Γδ T cell receptor-CD3 complex. Nature. (2024) 630:222–9. doi: 10.1038/s41586-024-07439-4
123. van Diest E, Hernández López P, Meringa AD, Vyborova A, Karaiskaki F, Heijhuurs S, et al. Gamma delta TCR anti-CD3 bispecific molecules(GABs) as novel immunotherapeutic compounds. J Immunother Cancer. (2021) 9:e003850. doi: 10.1136/jitc-2021-003850
124. Bacac M, Klein C, Umana, P. CEA TCB: a novel head-to-tail 2:1 T cell bispecific antibody for treatment of CEA-positive solid tumors. Oncoimmunology. (2016) 5:e1203498. doi: 10.1080/2162402X.2016.1203498
125. Segal NH, Melero I, Moreno V, Steeghs N, Marabelle A, Rohrberg K, et al. CEA-CD3 bispecific antibody cibisatamab with or without atezolizumab in patients with CEA-positive solid tumours: results of two multi-institutional phase 1 trials. Nat Commun. (2024) 15:4091. doi: 10.1038/s41467-024-48479-8
126. Sam J, Colombetti S, Fauti T, Roller A, Biehl M, Fahrni L, et al. Combination of T-cell bispecific antibodies with PD-l1 checkpoint inhibition elicits superior anti-tumor activity. Front Oncol. (2020) 10:575737. doi: 10.3389/fonc.2020.575737
127. Edwards SC, Sutton CE, Ladell K, Grant EJ, McLaren JE, Roche F, et al. A population of proinflammatory T cells coexpresses Aβ and Γδ T cell receptors in mice and humans. J Exp Med. (2020) 217:e20190834. doi: 10.1084/jem.20190834
128. Gober HJ, Kistowska M, Angman L, Jenö P, Mori L, De Libero, G. Human T cell receptor gammadelta cells recognize endogenous mevalonate metabolites in tumor cells. J Exp Med. (2003) 197:163–8. doi: 10.1084/jem.20021500
129. Kondo M, Izumi T, Fujieda N, Kondo A, Morishita T, Matsushita H, et al. Expansion of human peripheral blood Γδ T cells using zoledronate. J Vis Exp. (2011) 55:3182. doi: 10.3791/3182
130. Harmon NM, Huang X, Schladetsch MA, Hsiao CH, Wiemer AJ, Wiemer DF. Potent double prodrug forms of synthetic phosphoantigens. Bioorg Med Chem. (2020) 28:115666. doi: 10.1016/j.bmc.2020.115666
131. Lin L, He J, Li J, Xu Y, Li J, Wu, Y. Chitosan nanoparticles strengthen Vγ9Vδ2 T-cell cytotoxicity through upregulation of killing molecules and cytoskeleton polarization. Int J Nanomedicine. (2019) 14:9325–36. doi: 10.2147/IJN.S212898
132. Almeida AR, Correia DV, Fernandes-Platzgummer A, da Silva CL, da Silva MG, Anjos DR, et al. Delta one T cells for immunotherapy of chronic lymphocytic leukemia: clinical-grade expansion/differentiation and preclinical proof of concept. Clin Cancer Res. (2016) 22:5795–804. doi: 10.1158/1078-0432.CCR-16-0597
133. Deniger DC, Maiti SN Mi T, Switzer KC, Ramachandran V, Hurton LV, et al. Activating and propagating polyclonal gamma delta T cells with broad specificity for malignancies. Clin Cancer Res. (2014) 20:5708–19. doi: 10.1158/1078-0432.CCR-13-3451
134. Deniger DC, Switzer K, Mi T, Maiti S, Hurton L, Singh H, et al. Bispecific T-cells expressing polyclonal repertoire of endogenous Γδ T-cell receptors and introduced CD19-specific chimeric antigen receptor. Mol Ther. (2013) 21:638–47. doi: 10.1038/mt.2012.267
135. Reis BS, Darcy PW, Khan IZ, Moon CS, Kornberg AE, Schneider VS, et al. TCR-Vγδ usage distinguishes protumor from antitumor intestinal Γδ T cell subsets. Science. (2022) 377:276–84. doi: 10.1126/science.abj8695
136. Sánchez Martínez D, Tirado N, Mensurado S, Martínez-Moreno A, Romecín P, Gutiérrez Agüera F, et al. Generation and proof-of-concept for allogeneic CD123 CAR-delta one T(DOT) cells in acute myeloid leukemia. J Immunother Cancer. (2022) 10:e005400. doi: 10.1136/jitc-2022-005400
137. Makkouk A, Yang X, Barca T, Lucas A, Turkoz M, Wong JTS, et al. Off-the-shelf Vδ1 gamma delta T cells engineered with glypican-3(gpc-3)-specific chimeric antigen receptor(CAR) and soluble IL-15 display robust antitumor efficacy against hepatocellular carcinoma. J Immunother Cancer. (2021) 9:e003441. doi: 10.1136/jitc-2021-003441
138. Nishimoto KP, Barca T, Azameera A, Makkouk A, Romero JM, Bai L, et al. Allogeneic CD20-targeted Γδ T cells exhibit innate and adaptive antitumor activities in preclinical B-cell lymphoma models. Clin Transl Immunology. (2022) 11:e1373. doi: 10.1002/cti2.1373
139. Frieling JS, Tordesillas L, Bustos XE, Ramello MC, Bishop RT, Cianne JE, et al. Γδ-enriched CAR-T cell therapy for bone metastatic castrate-resistant prostate cancer. Sci Adv. (2023) 9:eadf0108. doi: 10.1126/sciadv.adf0108
140. Ueda T, Shiina S, Iriguchi S, Terakura S, Kawai Y, Kabai R, et al. Optimization of the proliferation and persistency of CAR T cells derived from human induced pluripotent stem cells. Nat Biomed Eng. (2023) 7:24–37. doi: 10.1038/s41551-022-00969-0
Keywords: gamma delta T cell, tumor microenvironment, immunotherapy, adoptive cell therapy, CAR-γδ T cell
Citation: Luo X, Lv Y, Yang J, Long R, Qiu J, Deng Y, Tang G, Zhang C, Li J and Zuo J (2024) Gamma delta T cells in cancer therapy: from tumor recognition to novel treatments. Front. Med. 11:1480191. doi: 10.3389/fmed.2024.1480191
Received: 13 August 2024; Accepted: 09 December 2024;
Published: 19 December 2024.
Edited by:
Forat Lutfi, University of Kansas Medical Center, United StatesReviewed by:
Sanpreet Singh, University of Pittsburgh, United StatesCheng-Rong Yu, National Eye Institute (NIH), United States
Kenth Gustafsson, University College London, United Kingdom
Copyright © 2024 Luo, Lv, Yang, Long, Qiu, Deng, Tang, Zhang, Li and Zuo. This is an open-access article distributed under the terms of the Creative Commons Attribution License (CC BY). The use, distribution or reproduction in other forums is permitted, provided the original author(s) and the copyright owner(s) are credited and that the original publication in this journal is cited, in accordance with accepted academic practice. No use, distribution or reproduction is permitted which does not comply with these terms.
*Correspondence: Jianhong Zuo, MjAwMDAwMDQwN0B1c2MuZWR1LmNu
†These authors share first authorship