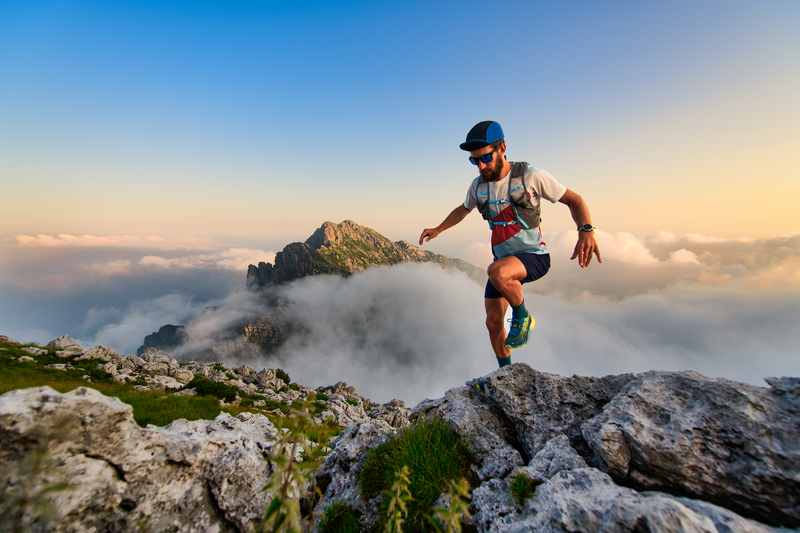
95% of researchers rate our articles as excellent or good
Learn more about the work of our research integrity team to safeguard the quality of each article we publish.
Find out more
REVIEW article
Front. Med. , 31 July 2024
Sec. Hepatobiliary Diseases
Volume 11 - 2024 | https://doi.org/10.3389/fmed.2024.1420281
This article is part of the Research Topic Lipid Metabolism in Metabolic Dysfunction-Associated Steatotic Liver Disease View all 9 articles
The growing prevalence of NAFLD and its global health burden have provoked considerable research on possible diagnostic and therapeutic options for NAFLD. Although various pathophysiological mechanisms and genetic factors have been identified to be associated with NAFLD, its treatment remains challenging. In recent years, exosomes have attracted widespread attention for their role in metabolic dysfunctions and their efficacy as pathological biomarkers. Exosomes have also shown tremendous potential in treating a variety of disorders. With increasing evidence supporting the significant role of exosomes in NAFLD pathogenesis, their theragnostic potential has become a point of interest in NAFLD. Expectedly, exosome-based treatment strategies have shown promise in the prevention and amelioration of NAFLD in preclinical studies. However, there are still serious challenges in preparing, standardizing, and applying exosome-based therapies as a routine clinical option that should be overcome. Due to the great potential of this novel theragnostic agent in NAFLD, further investigations on their safety, clinical efficacy, and application standardization are highly recommended.
• Different exosomes participate in the development of non-alcoholic fatty liver disease.
• Exosomes are ideal biomarkers for the diagnosis of non-alcoholic fatty liver disease.
• Exosomal microRNAs can differentiate the different stages of non-alcoholic fatty liver disease.
• Stem cell-derived exosomes can ameliorate the course of non-alcoholic fatty liver disease.
• Exosomal blocking can prevent non-alcoholic fatty liver disease progression.
Affecting one out of four adults globally, Non-alcoholic fatty liver disease (NAFLD) remains a growing worldwide health issue (1). It is highly associated with metabolic syndrome and commonly accompanies insulin resistance, obesity, and dyslipidemia (1). Moreover, it is increasing due to the Growing obesity and diabetes prevalence (2, 3).
NAFLD is a histological definition describing macrovesicular steatosis in more than 5% of hepatocytes in people with no or little alcohol consumption. It includes two major types: non-alcoholic fatty liver (NAFL) and non-alcoholic steatohepatitis (NASH). Non-alcoholic fatty liver (NAFL), or simple steatosis, is a form of NAFLD. While NAFL is typically accompanied by a lower risk of liver-related mortality, it can progress to non-alcoholic steatohepatitis (NASH) (4). NASH is more commonly associated with cirrhosis and hepatocellular carcinoma (HCC), both of which can lead to liver-associated death (4). Histologically, NASH consists of ballooning degeneration and lobular inflammation along with steatosis, with or without perisinusoidal fibrosis (5). However, present theories propose a dynamic two-way cycling between NAFL and NASH, with fibrosis advancing slowly in most patients (4). The reality that the initiation of progressive fibrosis ultimately determines clinical results raises doubts about the importance of differentiating between NAFL and NASH (4).
Although there has been a lot of effort in developing non-invasive tools for NAFLD diagnosis, the diagnosis of NAFLD is mainly based on liver biopsy (6, 7). However, it is an invasive and costly procedure, and, considering the prevalence of the disease, impractical. Therefore, non-invasive diagnostic methods that could be accurate can be crucial in this area. Along with lifestyle modification and weight loss, several agents, including pioglitazone, semaglutide, and obeticholic acid, have shown promise in the therapy of NAFLD (7). However, with more than 50 treatment agents currently being studied in different clinical phases, the treatment of NAFLD remains challenging (7).
Exosomes are nanosized extracellular vesicles that have significant roles in the pathogenesis of different diseases (8). Recently, their theragnostic potentials have been broadly discussed in various diseases, including cardiac, renal, hepatic, and neurological diseases, as well as neoplasms (8). In the presenting paper, we aimed to review the potential and perspectives of exosomes as a diagnostic and therapeutic agent in NAFLD.
Exosomes are bi-lipid membrane extracellular vesicles sized from 50 to 140 nm (9–11). They are secreted by nearly all cells and can be found in all body fluids (12–15). Exosomes carry a wide variety of proteins, DNAs, RNAs, and lipids, with specific characterization based on the secreting cell (16–19). They have higher levels of specific proteins [such as heat shock protein 70 (HSP 70), tetraspanins (CD9, CD 63, CD 81, and CD 82), ALG-2-interacting protein X (ALIX), tumor susceptibility gene 101 (TSG101)] and lipids [such as flotillin and glycosylphosphatidylinositol-anchored protein (LBPA)] (20–22).
Exosome biogenesis involves complex intracellular pathways with distinct mechanisms for different EV subtypes (23). The endosomal system plays a central role in exosome biogenesis (24). Traditionally, the endosomal sorting complex required for transport (ESCRT) machinery has been considered essential for the biogenesis of exosomes (24). The biogenesis of exosomes involves four key steps: cargo sorting, the development and maturation of MVBs, the transportation of these MVBs, and finally, the fusion of MVBs with the cellular membrane (25). Each process is modulated through the competition or coordination of multiple mechanisms, whereby diverse repertoires of molecular cargos are sorted into distinct subpopulations of exosomes, resulting in the high heterogeneity of exosomes.
Recognition of Ubiquitinated Cargo is the first step in exosome biogenesis (24). The process begins with the recognition of ubiquitinated cargo by the ESCRT-0 complex (24). Ubiquitination is a post-translational modification where a small protein called ubiquitin is attached to a target protein (26). This serves as a recognition signal for ESCRT-dependent cargo sorting. Once the ubiquitinated cargo is recognized, it is sequestered into endosomal microdomains by the ESCRT-I and ESCRT-II complexes (25). These complexes also initiate the budding of the endosomal membrane into the lumen of the endosome, forming the nascent ILV. The ESCRT-III complex is responsible for driving the budding process to completion, leading to the formation of ILVs (24). It does this by assembling into a spiral-shaped structure on the endosomal membrane, which constricts the neck of the budding ILV. ILVs are formed within the lumen of an endosome through the inward budding of the endosomal membrane. When multiple ILVs accumulate within an endosome, it is then referred to as a multivesicular body (MVB). MVBs can either fuse with lysosomes for degradation or with the plasma membrane, releasing ILVs as exosomes.
Recent findings suggest the existence of an alternative pathway for sorting exosomal cargo into MVBs in an ESCRT-independent manner (24). Nonetheless, the pathways may not be completely distinct (27). This ESCRT-independent pathway seems to depend on raft-based microdomains for the lateral segregation of cargo within the endosomal membrane (28). For instance, the nSMase2-ceramide pathway is vital for ESCRT-independent exosome biogenesis (29). Besides, Tetraspanins are a family of proteins that play a crucial role in the biogenesis of exosomes, including the ESCRT-independent pathway (30). They are characterized by their four transmembrane domains, which allow them to interact with various other proteins, cholesterol, and gangliosides. These interactions lead to the formation of tetraspanin-enriched microdomains (TEMs) on the membrane (30). These TEMs can influence membrane bending and actin polymerization, which are essential steps in the formation of multivesicular bodies (MVBs) and, subsequently, exosomes (30). In addition to their role in exosome biogenesis, tetraspanins are also sorted into exosomes in an ALIX- and ESCRT-III-dependent manner (31). This sorting process occurs independently of other ESCRTs but requires lysobisphosphatidic acid (LBPA) in vivo.
Lately, exosomes have got much attention in diagnostics. They constitute a key intracellular communication system and act as a key factor in the pathogenesis of different diseases via intercellular signaling cascades (23, 32–35). Therefore, detecting exosomes, molecules carried by them such as nucleic acids, and their surface proteins may be used as an early, non-invasive, and potentially accurate diagnostic tool in different diseases (8). Their potential diagnostic value has been proven in cancers and diseases of the lungs, kidneys, liver, and central nervous system (36–42).
Exosome offers favorable features as a therapeutic agent. It has the same homing behavior as the secreting parent cell (43). Additionally, it benefits from a bilipid membrane, which allows it to carry considerable amounts of contents and protect its cargo from degradation by chemicals and enzymes. Considering that along with their targeted activity, exosomes are fruitful options in drug delivery applications. Moreover, they have significantly lower immunogenicity than virus-based drug delivery systems and liposomes (44). Besides, exosomes derived from mesenchymal stem cells provide immunomodulatory and anti-inflammatory effects, promote cellular viability, and facilitate cellular proliferation and neoangiogenesis (45–50). In certain settings, exosomes can have regenerative and homeostatic, thus therapeutic effects on diseased tissue (51). On top of that, exosomes lack risks of carcinogenesis and immune response to cancers and infections, compared with cell therapies, offering high therapeutic potential with appreciable safety (9).
The complex pathophysiology of NAFLD has not yet been fully clarified. However, it is believed that it is related to the interaction among genetic, environmental, and individual factors (52).
There are different hypotheses about NAFLD pathophysiology. In the past, the “two-hit hypothesis” was used to explain the mechanism of NAFLD and NASH pathophysiology, but currently, the “multiple-hit model” is suggested (52). According to the two-hit model, the initial phase, often referred to as the “first hit,” involves the build-up of fat, specifically triglycerides, within the liver tissue and the development of insulin resistance. These elements are considered the key drivers of hepatic steatosis when the accumulation surpasses the threshold of 5% (53). The “second hit” is characterized by alterations in the levels of inflammatory cytokines and adipokines, mitochondrial dysfunction, and oxidative stress. These changes can trigger necroinflammation and fibrosis within the liver tissue (54, 55). However, recent investigations have shown that the “two-hit hypothesis” cannot fully explain the exact mechanism of human NAFLD. Therefore, the “multiple-hit model” is currently the most recognized theory. It suggests a broader metabolic dysfunction due to the interplay of genetic and environmental influences, as well as alterations in the crosstalk between the liver and various organs and tissues, such as the adipose tissue, pancreas, and gut (52, 54–57). Despite this, the initial stages, or “first hits,” are still believed to be the accumulation of fat in the liver triggered by obesity and insulin resistance (52).
NASH and NAFLD begin with the excessive accumulation of triglycerides (TG) in the liver (58). The fatty acids used for this hepatic TG accumulation are derived from three sources: (I) dietary fat taken up in the intestine, (II) de novo lipogenesis from glucose and fructose, and (III) dysregulated adipocytes' triglyceride lipolysis leading to excessive fatty acid transport to the liver (58–60). When the liver's capacity to utilize carbohydrates and fatty acids, as the primary metabolic energy substrates, is impaired, toxic lipid species are accumulated in hepatocytes. It, in turn, induces hepatocellular injury and death, which results in genomic instability and fibrogenesis that put the liver at risk of cirrhosis and hepatocellular carcinoma. However, the specific toxic lipid species that promote cell injury are not fully recognized (6). Lipotoxic lipids accumulation causes hepatocellular injury through endoplasmic reticulum (ER) stress (61), a dysregulated unfolded protein response (UPR) (62), activation of the inflammasome and apoptotic pathways (63), an increased hepatic Hedgehog (Hh) signaling, and inflammation (64). There are many external factors contributing to hepatocellular injury, including insulin resistance (65), adipokine dysregulation (66), hepatic ATP depletion (67), uric acid-induced hepatocyte mitochondrial dysfunction (68), and the effects of intestinal microbiota products (69). Fibrogenesis results from extracellular signaling from injured hepatocytes, liver sinusoidal endothelial cells, activated Kupffer cells, T cells, B cells, and natural killer cells, promoting hepatic stellate cells (HSCs) activation. Activated HSCs transdifferentiate into fibrogenic myofibroblasts that can produce extracellular matrix proteins at a higher rate than their degradation (70). Finally, Progressive fibrosis leads to cirrhosis, which subsequently promotes portal hypertension and liver failure, which is the main reason for liver-related mortality in NAFLD (6).
Several recent studies point to a significant role of Extracellular Vesicles (EVs) in the pathophysiology and progression of NAFLD and NASH. EVs play a central role in normal intercellular communication. Different types of liver cells, including human adult liver stem cells, cholangiocytes, hepatocytes, hepatic dendritic cells, and hepatic stellate cells, function as both exosome-secreting and exosome-targeted (42). Various liver conditions, including NAFLD, seem to increase the basal EV's secretion (71). Povero et al. (72) have shown a considerable rise in the concentration of EVs in the liver and blood of diet-induced NAFLD animals. Normal hepatocytes produce exosomes that carry several cargos, such as proteins and miRNAs (73). However, exosomes secreted from damaged hepatocytes are essential in inducing hepatocellular inflammation and fibrosis during liver damage. These effects are through intercellular communication between different cell types (74). The roles of exosomes and exosomal miRNAs in NAFLD pathogenesis and the interactions between different organs are illustrated in Figures 1, 2.
Figure 1. The roles of exosome and exosomal miRNAs in NAFLD pathophysiology. microRNAs, including miR-34a and exosomal miR-199a-5p, that control de novo lipogenesis and dysregulated TG lipolysis in adipocytes significantly induce excessive hepatocyte TG accumulation. Subsequently, TG accumulation in hepatocytes gives rise to impaired hepatic metabolism and toxic lipid species accumulation. The accumulation of toxic lipid species leads to ER stress, apoptosis, and inflammation. These changes contribute to hepatocellular injury and death, followed by HSC activation. MiR-34a, exosomal miR-411-5p and miR-411-5p, miR-17, adipocyte-derived EV, lipotoxic hepatocyte-derived EVs containing DAMPs, ceramides, ICAM1, S100a11, CXCL10 and miR-195-5p and external factors including insulin resistance, intestinal microbiota production, and adipokine dysregulation participate in various parts of this process. HSC activation results in fibrosis and, eventually, cirrhosis. miR, microRNA; TG, triglyceride; ER, endoplasmic reticulum; DAMPs, Damage-associated molecular patterns; ICAM-1, Intercellular Adhesion Molecule 1; HSCs, Hepatic stellate cells; EVs, extracellular vesicles.
Figure 2. The roles of exosomes in the interactions of the liver and pituitary gland, intestine, and adipose tissue in NAFLD. Different organs are involved in the development of NAFLD, and their interactions with the liver play significant roles in NAFLD progression. By TSH regulation, the pituitary gland alters hepatocyte exosome production, which exaggerates steatosis. TSH stimulation of HepG2 cells leads to exosome release and upregulation of proteins involved in steatosis. Adipose tissue induces progression in various steps of NAFLD, including steatosis, apoptosis, inflammation, and hepatocellular injury by exosomal AKR1B7 released from ER stressed adipocytes, active stat3 containing exosome, CD36 containing exosomes and insulin resistance caused by abdominal subcutaneous adipose tissue exosomes. Therefore, adipocytes' metabolism impairment promotes steatosis. Moreover, steatosis can also exaggerate the metabolism impairment of adipocytes through exosomal let-7b-5p. On the other hand, liver-protecting exosomes originating from adipose tissue can ameliorate apoptosis and inflammation. The intestine can also affect NAFLD development in two ways: (1) intestinal microbiota products can amplify hepatocellular injury, and (2) exosomal HMGB1 increases steatosis.
NAFLD is characterized by excessive hepatic lipid accumulation (75). The accumulation of lipids in the liver cells results in the release of stress signals, triggering the activation of inflammatory pathways that, when perpetuated, lead to chronic injury and fibrosis over time. Hepatocytes release EVs in response to lipotoxic fatty acids, which leads to HSCs fibrogenic activation and promotes macrophage chemotaxis (76, 77). Inflammation and fibrosis are essential for NAFLD progression (78).
Li et al. (79) demonstrated that the exosomal miR-199a-5p induces lipid build-up in the liver through a down-regulation of its target gene, hepatic Mammalian sterile 20-like kinase 1 (MST1). This leads to an alteration in Sterol regulatory element-binding protein 1 (SREBP1c), AMP-activated protein kinase (AMPK) signaling cascades and consequently suppresses Carnitine palmitoyltransferase I α (CPT1α) lipolysis gene and induces Fatty acid synthase (FASN) lipogenesis gene expression. In another study, Xu et al. (80) reported that hepatocyte exosomal miR-34a plays an essential role in the development of NAFLD by increasing lipid absorption and synthesis and reducing fatty acid oxidation. It also promotes NAFL's transition to NASH by regulating Kupffer cell activation, which promotes inflammatory responses, increases hepatic ROS levels, and induces hepatic apoptosis.
Lipotoxic hepatocyte-derived EVs cause hepatocellular inflammation. NAFLD is closely related to chronic inflammation associated with macrophages and neutrophils (78). Additionally, innate immune activation is fundamental in triggering hepatic inflammation in NASH (81). Hirsova et al. (82) have demonstrated that incubation of primary hepatocytes with lysophosphatidylcholine (LPC) increased their EV secretion compared with control cells in vitro. EVs derived from hepatocytes, carrying TNF-related apoptosis-inducing ligand, activated IL-1β and IL-6 messenger RNAs expression in macrophages derived from mice bone marrow, leading to inflammation and liver injury.
Regarding macrophage infiltration, Ibrahim et al. (83) have demonstrated that in hepatocyte lipotoxicity with LPC, Mixed lineage kinase 3 (MLK3) signaling induces the secretion of EVs containing elevated levels of CXCL10 from hepatocytes. These EVs induce bone marrow-derived macrophage chemotaxis. Moreover, Hepatocyte-derived ceramide-dependent EVs are enriched in several distinct damage-associated molecular patterns and cellular adhesion molecules, including ICAM1 and S100A11, that may influence immune cell responses, leading to hepatocellular inflammation (84). In addition, Kakazu et al. (77) have demonstrated that hepatocytes treated with palmitic acid (PA), a saturated fatty acid found in NAFLD hepatocytes, release significant amounts of C16:0 ceramide-enriched EVs in an inositol-requiring enzyme-1α (IRE1α)-dependent manner. IRE1A-stimulated hepatocyte-derived EVs, having ceramide-derived sphingosine 1-phosphate, enhance macrophage migration, which results in an inflammatory response in the liver (77, 85).
Moreover, in NAFLD, imbalanced macrophage polarization toward the pro-inflammatory M1 phenotype significantly contributes to disease progression (86). Conversely, M2 macrophages, particularly the M2a and M2c subtypes, exert protective effects in NAFLD (87). M1 macrophages are involved in the immune response and immune monitoring through antigen presentation and the release of pro-inflammatory cytokines like IL-1β and TNF-α (87). On the other hand, M2 macrophages have limited antigen presentation capabilities and contribute to immune regulation by dampening the immune response with the secretion of inhibitory cytokines such as IL-10, TGF-β, and Mrc (88). M2 macrophages can contribute to tissue healing and renewal. The decision of whether macrophages exhibit a pro-inflammatory reaction that causes injury or an anti-inflammatory response that offers protection depends on the balance between M1 and M2 activation tendencies (89).
Liu et al. (90) found that exosomal miR-192-5p and hepatocyte-derived exosome levels are considerably higher in NASH patients' serum, similar to high-fat, high-cholesterol diet (HFHCD)-fed rat NASH models. Progression of NAFLD in HFHCD-fed rats is directly associated with serum miR-192-5p and proinflammatory M1 macrophage amounts along with proinflammatory cytokines expression (90). Another study (91) showed that exosomes originating from lipotoxic hepatocytes and containing miR-192-5p significantly activate macrophages. They polarize macrophages to proinflammatory M1 phenotype by regulating rapamycin-insensitive companion of mammalian target of rapamycin (Rictor)/Akt/Forkhead Box Transcription Factor O1 (FoxO1) signaling pathway. In addition, saturated fatty acids and Cholesterol, via inducing lysosomal dysfunction, promote exosomal miR-122-5p secretion from hepatocytes. It results in pro-inflammatory M1 macrophage polarization and inflammatory activation (92).
The interaction between hepatic stellate cells (HSCs) and macrophages plays a crucial role in the pathogenesis of liver fibrosis (93, 94). Nevertheless, the specific molecular process that facilitates communication between hepatic stellate cells (HSCs) and liver macrophages, particularly the M2 subtype, remains incompletely understood. Exosomes are suggested to mediate the connection between mentioned cells, especially through the miRNAs (94). The polarization of M2 macrophages is strongly linked to the suppression of HSC activation and the reduction of liver fibrosis (95–97). Wan et al. (94) showed that HSC activity was significantly inhibited by exosomes derived from M2 macrophage. They found that exosomal miRNA-411-5p is reduced in the liver tissue and serum of HFHC diet-induced rat model of NASH. They also showed that exosomal miR-411-5p from M2 macrophages hinder the HSCs activity and lead to inactivation of stellate cells through downregulation of the expression of Calmodulin-Regulated Spectrin-Associated Protein 1 (CAMSAP1). The inhibition of HSCs occurred following the knockdown of CAMSAP1 as a direct target of miRNA-411-5p. Furthermore, the amount of miR-411-5p in exosomes derived from M2 macrophages was higher than that in M1 macrophages. Recent literature shows that MiR-411-5p may play a role in the regulation of hepatocellular carcinoma progression (98).
Liu et al., (91) found that arginase 1 (Arg1), a marker of M2 macrophages, was reduced after an 8-week high-fat high-cholesterol diet (HFHCD) in a rat model, but other M2 markers, chitinase 3-like protein 3 (Ym1) and found in inflammatory zone 1 (Fizz1), remained unchanged throughout the 16-week diet. Interestingly, they discovered that hepatocyte-derived exosomal miR-192-5p did not induce M2 macrophage polarization, and neither did exosomes from PA-treated HepG2 cells affect M2 macrophage activation. They also observed a decrease in Ym1 expression in THP-1 macrophages after exposure to serum exosomes from NASH patients, an effect that was reversed with a miR-192-5p inhibitor. In another study, Zhao et al. (92) investigated the potential role of cholesterol-loaded hepatocytes in inducing macrophage polarization through exosome-related cross-talk. They found that exosomes from Huh7 cells loaded with ox-LDL and MβCD-cholesterol did not affect the percentage of M2 macrophages. In conclusion, these studies suggest that while certain exosomes and exosomal miRNAs released by cholesterol-loaded hepatocytes can influence M2 macrophage polarization, their effects are complex and may depend on the specific conditions and markers examined. Further research is needed to fully understand these mechanisms and their implications in NAFLD. This could potentially open new avenues for therapeutic interventions targeting macrophage polarization. Changing the macrophage polarization in hepatic tissue toward M2 macrophages may exert protective effects against NAFLD progression and liver fibrosis. This should be further studied as a potential therapeutic pathway in NAFLD.
Besides, hypoxia can induce NAFLD in obstructive sleep apnea (OSA) syndrome by exosomes. Hypoxia triggers the production of certain exosomes (99). Yang et al. (100) mentioned that OSA-induced exosomes promote hepatocyte steatosis and activate macrophages in liver tissue. These exosomes were observed to enhance fat accumulation. They demonstrated that after the uptake of OSA-induced exosomes by macrophages, the polarization of macrophages toward the M1 type occurred. It led to the inhibition of sirtuin-3 (SIRT3)/AMP-activated protein kinase (AMPK) and autophagy. It also enhanced the activation of the nucleotide-binding domain, leucine-rich-containing family, and pyrin domain-containing-3 (NLRP3) inflammasomes. The use of 3-methyladenine (3-MA) to block autophagy prevented NLRP3 inflammasome activation and hindered M1 macrophage polarization. Moreover, they reported elevated levels of miR-421 in OSA-induced exosomes in OSA plus NAFLD mice and patients. In the liver tissues of OSA and OSA plus NAFLD mice, miR-421 showed the same co-localization with macrophages. Hepatocytes exposed to intermittent hypoxia transferred miR-421 to macrophages through exosomes to inhibit SIRT3 and contribute to macrophage M1 polarization. Accordingly, MiR-421 targeting reduced SIRT3 protein levels in macrophages. They also found that in miR-421–/– mice subjected to OSA and NAFLD modeling, liver steatosis and M1 polarization were notably reduced. Knockout of miR-421 alleviated the inhibitory effects of OSA-induced exosomes on SIRT3 and autophagy, leading to reduced liver steatosis and macrophage M1 polarization. On the other hand, they haven't found any change in the transcription of M2 macrophage genes Arg1 and Fizz1 Following the uptake of OSA exosomes by macrophages.
Other than the above mechanisms, Shen et al. (101) showed that decreased hepatocyte autophagy, found in non-alcoholic steatohepatitis, develops IL-1β/TNF-induced hepatic injury and inflammation through the exosomal release of the damage-associated molecular pattern (DAMPs). In addition, liver inflammation could be caused by EVs secreted from other cells. For example, It has been reported that platelet-derived EVs may have a pro-inflammatory role in the liver (102). However, some studies have demonstrated the opposite (103).
Hepatic stellate cells (HSCs) promote fibrogenesis through EVs intracellular communication. Literature (104) has shown that hepatocytes stimulated by palmitate (PA) secret significantly more exosomes with distinctive miRNA expression patterns, amplifying fibrotic gene expression in HSCs. In this regard, Wei Wang et al. (105) illustrated that hepatocytes treated with PA release more miR-107-enriched exosomes, leading to HSC proliferation and activation by two distinct pathways. These hepatocyte-derived exosomes transfer miR-107 to HSCs, where miR-107, by directly inhibiting Dickkopf-1 (DKK1) expression, activates Wnt signaling. Additionally, these exosomes deliver miR-107 to CD4+ T cells, where miR-107 activates the Raf/MEK/ERK signaling pathway by upregulating IL-9 expression via Forkhead box protein P1 (Foxp1) inhibition. These pathways lead to HSC activation, an essential component of NAFLD pathogenesis.
On the other hand, exosomes may exert protective effects on NAFLD. Studying on a diet-induced rat model of NASH, Qi et al. (106) proved that M2 macrophage-derived exosomes enriched with miR-411-5p directly reduce Calmodulin-Regulated Spectrin-Associated Protein 1 (CAMSAP1) expression. This consequently inhibits hepatic stellate cell activation and fibrosis. In addition, Hou et al. (107) found that miR-223-enriched exosomes are released from myeloid cells in response to IL-6 signaling. They offer protective effects on NASH progression by inhibiting the expression of profibrotic genes that miR-223 targets, including C-X-C motif chemokine (Cxcl10), NOD-, LRR—and pyrin domain-containing protein 3 (Nlrp3), transcriptional activator with PDZ-binding (Taz), and insulin-like Growth Factor 1 Receptor (Igf1r) in the liver.
Adipocyte-hepatocyte interaction through EVs plays an essential role in NAFLD progression. Afrisham et al. (108) demonstrated that plasma exosomes isolated from obese women may play a role in NAFLD development by inducing insulin resistance, increasing hepatocellular TG levels, and decreasing hepatocellular FGF21 secretion. These exosomes promote a significant increase in the expression of integrin ανβ-5 and tissue inhibitor of matrix metalloproteinase-1 (TIMP-1) along with a concomitant decrease in plasminogen activator inhibitor-1 (PAI-1) and matrix metalloproteinase-7 (MMP-7) expression in HepG2 cells. In hepatic stellate cells, these exosomes induce increased integrin ανβ-5 and 8, Smad-3, TIMP-1 and 4, and matrix metalloproteinase-9 (MMP-9) expression. Consequently, these processes dysregulate the tumor growth factor- β (TGF-β) signaling pathway, producing a profibrotic state in liver cells (109).
According to the tissue-cooperative homeostatic model of NAFLD, during the early phases of NAFLD progression, the falling levels of the hepatocellular production of miR-122 are compensated by an increase in the adipose miRNA-containing exosome secretion (110). These molecules are eventually taken up by the hepatocytes and augment these cells' endogenous production of miRNA. Thus, metabolic damage to adipose tissue (due to liver disease progression) eventually decreases the external supply of liver-supporting miRNAs, leading to hepatic fibrosis and carcinogenesis.
Ogur et al. (111) determined that exosomes derived from Endoplasmic Reticulum (ER) stress-induced adipocytes transfer exosomal Aldo-keto-reductase 1b7 (Akr1b7) to hepatocytes. This elevates glycerol levels in liver cells, which gives rise to hepatic steatosis, inflammation, and eventually fibrosis. Additionally, Yan et al. (112) showed that PA-induced AMPKα1 inhibition in adipocytes promotes CD36-containing exosome secretion. These exosomes contribute to HepG2 cell damage and mediate the development of High-fat diet (HFD)-induced NAFL. Furthermore, exosomal let-7b-5p originated from hepatocytes is essential in the interaction between hepatocytes and adipocytes by TGF-β signaling. It impairs the energy balance of adipocytes by regulating Adrb3 gene expression, resulting in hepatic steatosis and obesity (113). Besides, Fuchs et al. (114) found that subcutaneous abdominal adipose tissue-derived exosomes and raised levels of PAI-1 take part in the pathophysiology of insulin resistance. Insulin resistance is a critical factor in the NAFLD pathobiology (115).
Exosomes of adipose tissue may also have beneficial effects on NAFLD. Zhao et al. (116) demonstrated that exosomes from adipose-derived stem cells (ADSCs) promote arginase-1 expression in macrophages by carrying active STAT3 and inducing M2 phenotype macrophage polarization. This results in the inhibition of macrophage inflammatory responses. It suggests the potential efficacy of exosome and stem cell therapy in preventing NAFLD progression.
EVs also contribute to the development of NAFLD through other pathophysiological mechanisms. In a study on Asc −/− mice on a high-fat diet (HFD), Chen et al. (117) revealed a gut-liver axis mechanism in which exosomes play a significant role. In dysbiosis, exosomes act as the transporter of high mobility group box 1 (HMGB1) protein from the intestine to the liver, triggering hepatic steatosis. Besides, a preliminary study (118) on TSH-induced lipotoxicity in NAFLD revealed that TSH stimulation of HepG2 cells significantly increases their exosomal production and alters their exosomal proteomic profile. It leads to upregulation of proteins involved in different biological processes such as metabolism, inflammation, and apoptosis. Hence, it may be involved in NAFLD pathogenesis.
miRNAs are the most abundant cargo molecules transferred by exosomes (119). Specific miRNAs have been associated with NAFLD and may be effectual in its pathogenesis. They may also be used as non-invasive options in NAFLD diagnosis. Zhang and Pan (120) monitored serum exosomal microRNAs in children with NAFLD and identified 2,588 miRNAs. They revealed that in children with NAFLD, the expression of 80 miRNAs, importantly miR-122-5p, miR-335-5p, and miR-27a, differs from that of the control group. In another study, Zhou et al. (121) suggested that exosomal miRNAs might take part in the pathophysiology of NAFLD and revealed an upregulation of miR-146b-3p, miR-155-5p, miR-122-5p, and miR-34a-5p in NAFLD patients. MiR-122, a highly liver-specific miRNA, takes part in lipid metabolism and is detected in the form of exosomes in the serum of NAFLD patients (122, 123). MiR-21 is another miRNA that is increased in the liver of NAFLD patients, as well as animal models of the disease. This miRNA regulates hepatocellular glucose and lipid metabolism. It works through a complex transcription network. At different stages, miR-21 may be involved in NAFLD progression, including early steps of the initiation of hepatocellular steatosis and later steps of inflammation and fibrosis (124). A list of exosomal miRNAs that may be beneficial for NAFLD diagnosis is presented in Table 1.
Studies have shown that exosomes, due to their contents, including microRNAs, may serve as potential diagnostic biomarkers for disease progression and severity in NAFLD (142). The content of exosomes can change in various diseases, and this is not only limited to exosomal miRNAs. As mentioned earlier, several exosomal miRNAs are considerably altered in NAFLD, and their potential role in NAFLD pathophysiology remains an important topic for a better understanding of NAFLD development and the mechanisms that help us ameliorate the condition. Meanwhile, the modification of the level of these miRNAs may provide a fruitful non-invasive diagnostic approach for NAFLD, considering the challenges in diagnosing NAFLD. A list of exosomal miRNAs potential for NAFLD diagnosis is presented in Table 1. Moreover, various studies have shown that changes in the level of exosome contents other than miRNAs, including protein FZD-7, can also act as diagnostic and prognostic biomarkers for NAFLD (143, 144). In addition to the content of exosomes, the level of certain EVs in mouse models and human subjects of NASH has been reported to be higher than that of normal individuals, suggesting specific exosomes' levels as potential factors for differentiating the stages of NAFLD (145). However, although exosomes offer favorable non-invasive methods for the diagnosis and prognosis evaluation of NAFLD, there is a lack of comprehensive information regarding the sensitivity and specificity of exosome-based diagnostic methods. There is a need for further investigation to understand better the diagnostic capabilities of exosomes and their practical application in clinical settings for NAFLD assessment.
Resmetirom has been recently approved by the Food and Drug Administration (FDA) and remains the only approved option for NAFLD treatment along with lifestyle modifications, such as diet control and exercise. However, several drugs have shown potential therapeutic effects on NAFLD, such as elafibranor (by inhibiting lipid deposition), emricasan (via lowering cell death), IMMe124 (through regulating intestinal microenvironment and metabolism) (146). Moreover, Probiotics and prebiotics, symbiotic, fecal microbiome transplantation, and fasting-mimicking diet could also be beneficial in patients with NAFLD (147). Due to their unique characteristics, exosomes have gained attention as a new treatment option in different diseases. Exosomes have been studied for various clinical applications, including as a diagnostic/prognostic biomarker, cell-free therapeutic agent, drug delivery carrier, and cancer vaccines (148). Several exosome-based treatment approaches have shown efficacy in liver diseases, including NAFLD, which is described below. Figure 3 demonstrates potential exosome-based treatment approaches in NAFLD.
Figure 3. Diverse potential exosome-based therapeutic strategies in NAFLD. Exosomes originating from different sources, including stem cells, bone marrow, macrophages, and Blueberries, can exert beneficial effects in the treatment of NAFLD. Each source contributes unique therapeutic properties, such as anti-inflammatory and regenerative capabilities, immune modulation, and fibrosis inhibition, making them a promising tool in NAFLD management. They can be administered safely in high doses due to their cell-free nature, exhibit low toxicity, remain stable during circulation, and have minimal immunogenicity. Moreover, researchers are actively exploring exosome-based therapies for NAFLD, including drug delivery (serving exosomes as drug carriers, delivering therapeutic cargo directly to liver cells), targeted approaches (blocking exosomal contents such as microRNAs), and modulation of inflammatory processes.
In recent years, interest in therapeutic usage of cell derivatives such as exosomes over cell therapies has been increasing (149, 150). Exosomes are released by the majority of body cells and have been detected in nearly all fluids of the body, such as blood, cerebrospinal fluid, urine, amniotic fluid, breast milk, saliva, bile, malignant ascites, and lymph (151–158). Among all cell types, Mesenchymal stem cells (MSCs) are the most prolific producer of exosomes (159). MSCs can be derived from different body tissues, including peripheral blood, adipose tissue, bone marrow, umbilical cord, spleen, liver, pancreas, kidney, lung, thymus, and brain (160, 161). They are known as a subgroup of stromal stem cells. MSCs are adults' most researched type of stem cells in regenerative medicine owing to their extensive presence in body tissues and easy expansion procedure in vitro. They have shown the potential to prolong and save lives (162). Mesenchymal stem cell therapy has several disadvantages that must be considered, including the possibility of tracking in pulmonary capillaries, leading to pulmonary embolism, reduction in cell viability of MSCs in cryogenic storage during transport, and the risk of tumorigenesis after administration (163–166).
Exosomes mediate intracellular communication by transferring a variety of biomolecules like microRNAs (miRNAs), messenger RNAs (mRNAs), other non-coding RNAs, and lipids (167). Exosomes derived from stem cells have demonstrated the same therapeutic potential as their parental cells (162). It has been indicated that mesenchymal stem cell-derived exosome can alleviate liver fibrosis, decrease Alanine aminotransferase (ALT) and Aspartate transaminase (AST) levels, and mitigate liver inflammation (168). Thus, these exosomes, such as HUC-MSCs-derived exosomes, can be a promising therapeutic for NAFLD.
Many reports have illustrated that human umbilical cord mesenchymal stem cells (HUC-MSCs) exert therapeutic effects on liver diseases, including chemical-induced liver injury, decompensated liver cirrhosis, liver failure, and autoimmune liver diseases, including primary biliary cholangitis (169–174).
HUC-MSCs improve NAFLD and metabolic syndrome by means of regulating lipid metabolism by promoting the expression of genes that are related to fatty acid oxidation and suppressing adipogenesis-related genes' expression in db/db mice (175). MSCs exert their therapeutic effects through secretory factors, including exosomes. Cheng et al. (176) showed that the treatment of palmitic acid (PA)-treated human normal liver cell line (L-O2 cells), human fetal hepatocyte line, with HUC-MSCs-exosomes improves cell viability and inhibits apoptosis. They showed that the level of miR-627-5p was higher in HUC-MSCs-exosomes compared with HUC-MSCs. Moreover, they found that the expression of G6Pc and PEPCK, gluconeogenesis-related proteins, along with FAS and SREBP-1c, lipid metabolism-related proteins, were repressed in these cells, while the expression of PPARα, another lipid metabolism-related protein, was notably downregulated. Additionally, the study exhibited that the plasma levels of ALT, AST, total cholesterol (TC), triglyceride (TG), and blood glucose in the NAFLD rat model were repressed by exosome treatment. They also reported that MiR-627-5p improves lipid and glucose metabolism, key pathological components of NAFLD, in L-O2 cells by targeting fat mass and obesity-related gene (FTO). The FTO gene facilitates NAFLD development via increasing insulin resistance, oxidative stress, and lipid accumulation in liver cells (177, 178). Furthermore, exosome treatment has also been found to alleviate insulin resistance and liver damage and reduce fat accumulation in NAFLD rat models (176). Another study (179) also confirmed that HUC-MSC-exosome therapy can attenuate liver steatosis and regulate abnormal expression of Fabp5, ACOX, PPAR-α, FAS, SREBP-1c, and CPT1α as lipid metabolism-related genes.
HUC-MSC-Exosomes have also been shown to have beneficial effects against oxidative stress and inflammation. Kang et al. (179) demonstrated that in rat models of NASH, HUC-MSCs exosomes enhanced the Nrf2 (Nuclear factor erythroid 2-related factor 2), a protective factor against oxidative stress and 1 [NAD (P) H quinone dehydrogenase 1], a part of cellular adaptive response to stress, which seems to play a significant part in treating NASH (180). Besides, HUC-MSC-derived exosomes reduce oxidative stress through lowering Malondialdehyde (MDA), CYP2E1, and reactive oxygen species (ROS) levels and elevating Superoxide dismutase (SOD) and GSH function as well (179). Furthermore, HUC-MSC-exosome therapy reduces inflammatory response through decreasing F4/80+ and CD11c+ macrophages and the levels of tumor necrosis factor-alpha (TNF-α) and Interleukins-6 (IL-6) (179).
MSCs are classically separated from bone marrow (181). Bone marrow mesenchymal stem cells (BM-MSCs), also known as BM mesenchymal stromal cells, are multipotent mesenchymal precursor cells that have many favorable characteristics for regenerative therapy, including anti-inflammatory and immune-modulatory properties (182, 183). Studies have revealed that BM-MSCs have therapeutic potential in different diseases, including cardiovascular, lung, neural, hematopoietic, and liver diseases, along with graft-vs.-host disease and cutaneous, tendon ligament, and musculoskeletal tissue repairing (184–186). BM-MSCs release paracrine factors which influence the surrounding microenvironment (187). In contrast with their favorable therapeutic characteristics, a few downsides can restrain their clinical utilization, primarily due to their potential for tumorigenicity and immunogenicity (188). Thus, using BM-MSCs paracrine factors maintains BM-MSCs properties without most disadvantages. Therefore, exosomes can potentially replace BM-MSCs as a safer therapeutic approach for tissue repair (162, 189).
BM-MSCs and BM-MSC-derived exosomes both can provide anti-steatotic effects through downregulating sterol regulatory element binding protein 1 (SREB-1), sterol regulatory element binding protein 2 (SREB-2) and cetyl coenzyme A carboxylase (ACC), suppressing lipid uptake and upregulating peroxisome proliferator-activated receptor alpha (PPAR-α) and carnitine palmitoyltransferase 1 (CPT1) fatty acid oxidation genes. BM-MSC-derived exosomes at 15 μg/kg, 30 μg/kg, and 120 μg/kg have anti-steatotic effects in the HFD-induced NASH model. BM-MSCs or BM-MSCs-exosome co-treatment caused anti-apoptotic impacts via a meaningful reduction in Bax/Bcl2 ratio and a rise in the expression of mitochondrial mitophagy genes, including Parkin RBR E3 Ubiquitin Protein Ligase (Parkin), phosphatase and tensin homolog (PTEN)-induced putative kinase 1 (PINK1), unc-51 like autophagy activating kinase 1 (ULK1), B-cell lymphoma 2/adenovirus E1B 19 kDa protein-interacting protein 3 (BNIP3L), autophagy related gene (ATG5), ATG7 and ATG12. Furthermore, a notable depletion in the levels of AST and ALT has been observed in BM-MSCs or BM-MSCs-exosome co-treatment (190).
El-Derany et al. (190) found that BM-MSC-derived exosome can be an ideal treatment option for NAFLD through a mechanism in which the upregulation of miRNA-96-5p leads to the inhibition of caspase-2. MiRNA-96-5p is found both in peripheral blood and bone marrow (191). Studies have demonstrated that HFD is associated with a downregulation of miRNA-96-5p in NAFLD models (190, 192, 193). Moreover, it has been proven that the suppression of caspase-2 lowers lipo-apoptosis and inhibits fibrogenic Hedgehog ligands production, leading to the transition of NAFL to Nash (190, 194, 195). Furthermore, evidence has revealed that the inhibition of caspase-2 prevents hyperlipidemia and reduces hepatic steatosis, liver apoptosis, and mitochondrial mitophagy (190, 194).
Severe stages of NAFLD are related to hepatic stellate cell (HCS) activation and their interactions with macrophages. Hepatic macrophages have critical roles in the initiation and progression of fibrosis (93, 196, 197). It has been found that the level of miRNA-411-5p is lower in NASH patients' plasma exosomes and liver samples of NAFLD patients (94). Moreover, M2 macrophage-derived exosomes contain a higher amount of miRNA-411-5p than M1 macrophages. Wan et al. (94) revealed that M2-derived exosomes suppress HCS activation by inhibiting the direct target of miRNA-411-5p, Calmodulin-Regulated Spectrin-Associated Protein 1 (CAMSAP1), during the transition of NASH to NAFL.
In macrophages and neutrophils, miR-223 is the amplest miRNA that is functionally active and can be transferred from myeloid cells to other cells by exosomes (198–200). The serum level of miR-223 was reported to be remarkably higher in NAFLD. Moreover, a positive correlation has been seen between the levels of serum miR-223 and IL-6 (107). IL-6 signaling exerts anti-fibrotic effects in NAFLD-associated liver fibrosis by inducing myeloid cells to enhance miR-223-enriched exosome release. IL-6 increases the biogenesis of exosomes by upregulating gene expression without changing the pre-miRNA-223 expression (107). Myeloid cell-derived miRNA-223 enriched exosomes are delivered to the liver and inhibit the expression of miR-223 target pro-fibrotic genes like Igf1r, Cxcl10, Taz, and Nlrp3. Subsequently, they help control Nash's progression. Therefore, macrophage-derived exosomes could be used as a therapeutic option that alters the progression of NASH to NAFL (107).
Exosomes are the communication bridge between cells, thus playing critical roles in the pathological development and progression of numerous diseases (201). Accordingly, changing the level of exosome secretion, inhibiting their activity, or changing their contents could be a potential approach for treating diseases.
Targeting exosomal miRNAs may also be an effective treatment approach in NAFLD. miRNAs are reported to have therapeutic roles in NAFLD by regulating lipid metabolism, inflammation, and fibrosis (90). As mentioned in the pathophysiology part, exosomal miR-192-5p is higher in exosomes derived from NASH hepatocytes and plays a role in NAFLD progression by triggering M1 macrophage polarization (90). It has been found that this mechanism can be reversed using a miR-192-5p inhibitor (91). Hence, targeting serum exosomal miR-192-5p can be a beneficial option for inhibiting the progression of NAFLD (91).
Moreover, MiR-122-5p is another prospective option in exosome blocking. Zhao et al. (92) demonstrated that miR-122-5p level was higher in exosomes originating from Huh7 cells, a hepatic cell line loaded with cholesterol (including ox-LDL and MβCD-cholesterol). They used anti-miR-122-5p to block this exosomal miRNA. Anti-miR-122-5p-treated Huh7 cells-derived exosomes exerted lower effects in inducing TNF-α, IL-6, and IL-1β expression. Additionally, exosome-mediated inflammation of macrophages was blocked.
Adipose tissue exosomes may also be favorable therapeutic targets for NASH. Several studies have supported the role of pro-inflammatory mediators released from visceral adipose tissue (VAT) in the progression of NAFLD (202, 203). A cross-talk between adipose tissue and the liver is significant in NAFLD development and progression. This connection seems to be facilitated through exosomes (204). Stressed AT induces abnormal lipid accumulation in the liver, which may be the major contributor to NASH initiation and progression.
Studies have indicated that NASH development can be a consequence of Endoplasmic Reticulum (ER)-stress, which possibly changes miRNAs and metabolites of secretory exosomes (77, 205). Exosomes derived from ER-stress induced-AT can induce and aggravate NASH by delivering exosomal Aldo-keto reductase family 1 B7 (AKr1b7) (111). They can be taken up by hepatocytes and cause hepatic steatosis, inflammation, and fibrosis. Akr1b7 deficiency protects murine liver from NASH in HFD and methionine-choline-deficient diet (MCD)-fed mice. In addition, suppressing hepatic AKr1b7 reduces TG level and glycerol concentration and alleviates hepatic inflammation, ER stress, and lipid synthesis. Accordingly, Epalrestat, an Akr1b7 inhibitor, can suppress the effects of AT exosomes on ER stress and hepatic inflammation (68). Moreover, Treatment with GW4869, an exosome production inhibitor (by inhibiting sphingomyelinase), has been shown to alleviate ER stress and reduce lipid synthesis and hepatic inflammation (111).
TGF-B signaling plays a major part in various biological processes, such as cellular proliferation, differentiation, migration, and cell death. It is also an essential pathway in the regulation of liver homeostasis. TGF-B signaling helps NAFLD progression from initial lipid accumulation to fibrosis (206). Steatosis, weight gain, and impaired insulin sensitivity have been found to be associated with TGF-B signaling in a mouse model of NASH (207). Moreover, TGF-B signaling can modulate microRNA biogenesis at transcriptional and post-transcriptional levels (208). TGF-B induces hepatic secretion of exosomes carrying let-7b-5p, a miRNA. TGF-β-let-7b-5p pathway contributes to HFD-induced steatosis and obesity through lowering oxidative phosphorylation in mitochondria and inhibiting white adipose tissue (WAT) to brown fat conversion (113). Accordingly, Tgfbr2 loss in hepatocytes ameliorates HFD-induced NAFLD by improving mitochondrial biogenesis. It alleviates lipid accumulation and induces “browning” of WAT via inhibiting miRNA let-7b-5p-containing exosomes release from hepatocytes. In addition, let-7b-5p inhibitor can downregulate the expression of Cd36, Fatp1, and Fabp1 (which are fatty acid transporter genes), upregulate mitochondrial genes' expression, like Cox5b and Atp5a, and reduce lipid accumulation in CL-316,243 (CL)/cold-exposed mice and HFD-induced obese mice (113).
Some drugs are also effective in exosome blockage and provide benefits through exosome modulation. GW4869, a non-competitive neutral sphingomyelinase inhibitor, suppresses exosome secretion. It has been shown to mitigate HFD-induced NAFLD in mice models (112). Moreover, McCommis et al. (209) evaluated the efficacy of next-generation thiazolidinediones (MSDC-0602) in a mouse model of NASH. They have weak binding to peroxisome proliferator-activated receptor γ (PPARγ) and still directly suppress and interact with mitochondrial pyruvate carrier (MPC). The effect of MSDC-0602 was through MPC2. They revealed that MSDC-0602 treatment, directly and indirectly, prevents and reverses stellate cell activation, liver fibrosis, and lipid accumulation in HTF-C mice. It is through the indirect modulation of exosomes derived from hepatocytes in an MPC-dependent manner. Furthermore, Yan et al. (112) found that metformin treatment inhibits HFD-induced NAFLD in mice, thorough activating AMP-activated protein kinase (AMPKα1) in WAT, which leads to the inhibition of exosomes shedding into serum and WAT.
Ezetimibe is a lipid-lowering drug that selectively inhibits the absorption of cholesterol through the blockage of NBC1LI-dependent cholesterol transport in the small intestine. NBC1LI is also present in the liver (210–212). Many studies have demonstrated that ezetimibe treatment can significantly improve hepatic steatosis, ballooning score, and NAFLD histological features, even after its fibrotic changes (213, 214). It has been reported that ezetimibe treatment activates autophagy in human hepatocyte cells (215). In addition, Kim et al. (216) observed other beneficial effects of ezetimibe in NAFLD. They reported changes in Hepatocyte-macrophage interaction following ezetimibe treatment. They observed decreased Interleukin 1 Beta (IL-1B) mRNA and protein levels in macrophages cultured with EVs released from ezetimibe/PA-cotreated hepatocytes.
Recently, a variety of different nano-based drug carriers have been used in order to improve the therapeutic efficacy of chemical and biomolecular drugs and ingredients (217). Exosomes have favorable characteristics for being used as a drug delivery agent, including small size, which facilitates its penetration into deep tissues (218), having a slightly negative zeta potential (219), biocompatibility, low immunogenicity, being able to cross the biological barriers such as the BBB (220) and escape immune clearance (221), low systemic toxicity, and long-term existence in the target tissue (221, 222). Moreover, compared to many synthetic drug delivery agents, using exosomes facilitates cellular uptake due to their specific surface proteins (218, 223).
The exosomal-based delivery system has been loaded with a variety of biomolecules for drug delivery like paclitaxel, doxorubicin, curcumin as a peptide or protein-based therapeutics containing STAT3 inhibitors18, catalase, and also genetic material including siRNA (222), an effective carrier of miRNA that also stabilizes the miRNA is exosome (224, 225).
MiRNAs are non-coding RNAs that take part in the regulation of gene expression. Recently, some studies have revealed that miRNA-based therapies are feasible and effective in various diseases, especially cancers (226–228). For instance, miR-16-loaded minicell is in clinical investigation for the treatment of malignant pleural mesothelioma (229). MiRNAs may be potential treatment options for NAFLD as well. In the early stages of NAFLD, decreased intrahepatic miRNA levels are compensated by the elevated production of miRNA in adipose tissue. As NAFLD progresses, the external supply of liver-supporting miRNA falls gradually, which leads to the deterioration of liver function and an increase in hepatic carcinogenesis (110). Therefore, supplying damaged liver with external miRNA may be efficient in hindering the progression of NAFLD. Moreover, Baranova et al. (110) revealed that purified miR-122 exosomes may be potential cell-free therapeutics for preventing HCC in NAFLD. Further research on the efficiency of miRNA therapy in NAFLD is needed.
Blocking pathogenic exosomal miRNAs is also a potential treatment approach for NAFLD. The level of miR-199a-5P is found to be considerably higher in NAFLD. Exosomal miR-199a-5P (Exo-miR-199a-5P) interferes with the metabolism of lipids in the liver by suppressing the expression of hepatic MST1 (79). Additionally, administration of Exo-miR-199a-5P induces hepatic lipid deposition in normo-caloric diet (NCD) and HFD mice (which is possibly via regulating AMPK and SREBP-1c signaling pathways) (79). It also elevates liver weights and plasma TG levels (79). Expectedly, exosomal anti-miR-199 can significantly reduce lipid accumulation in vivo and in vitro. It also downregulates lipogenic genes and lowers serum and hepatic TG and cholesterol in HFD mice (79). Thus, treatment with exosomal anti-miR-199a can offer fruitful effects in the prevention and treatment of liver steatosis and dyslipidemia.
Plant-derived exosome-like nanoparticles (PDNPs) have shown potential in drug delivery and as therapeutic applications in different disease (230, 231). PDNPs are nano-sized vesicles released from edible plants, including lemon, apple, carrot, grapefruit, coconut, broccoli, grape, and ginger (232–241). Increasing evidence indicates that similar to mammalian cell-secreted exosomes, PDNPs have an important in intercellular communication (242, 243). In addition, they have certain superiorities compared to mammalian cell-secreted exosomes or artificial nanoparticles; they do not cause any detectable Immunogenicity or toxicity, they have high potential in delivering biomolecules, and using them is highly economical (95, 107, 110). They have been used in treating alcoholic fatty liver as well as IBD and colon cancer (234, 237, 244–246).
Blueberry nanoparticles have shown promise in the treatment of NAFLD. Blueberry is one of the most consumed berries that contains a wide diversity of bioactive compounds, such as flavonoids, anthocyanins, and polyphenols. Blueberry has antioxidative, anti-inflammatory, and anti-tumor activity and can have protective effects against cancers as well as Alzheimer's cardiovascular diseases, and depression (247–251). Zhao et al. (252) investigated the anti-oxidative impacts of Blueberry-derived exosomes-like nanoparticles (BELNs) in hepatocytes. They demonstrated that BELNs could attenuate oxidative stress by modulating nuclear factor erythroid 2–related factor 2 (Nrf2) distribution, which results in the upregulation of the antioxidative proteins and enzymes expression such as SOD, NADPH/quinone oxidoreductase I (NQ01), Heme Oxygenase-1 (HO-1), glutathione peroxidase (GPx), and catalase (CAT). It also regulates Bcl-2-associated X protein (BAX), Bcl-2, and HO-1 apoptosis-related proteins' expression in the liver of HFD-fed mice and rotenone-induced HepG2 cells. BELN supplementation also improves liver dysfunction and insulin resistance in NAFLD, which is accompanied by a reduction in AST and ALT levels and fat deposition as well as a downregulation in acetyl-CoA carboxylase 1 (ACC1) and fatty acid synthase (FAS) expression (252).
Melatonin can potentiate the therapeutic effects of exosomes; thus, it may be a favorable agent to be administered alongside exosomes. Melatonin is a methoxyindole that is secreted by the pineal gland and gastrointestinal tract during night and daytime, respectively (253). Melatonin has a critical role in the amelioration of different metabolic disorders in various tissues, including adipose and hepatic tissues (254–256). Melatonin exerts its beneficial effects in NAFLD exosomal therapy by activating Bmal1 expression in adipocytes (257). Eventually, Exosome-Melatonin co-treatment alleviates ER-stress-induced hepatic steatosis through a significant downregulation in the expression of adipocyte-derived exosomal resistin (257). Resistin is an adipocytokine that may aggravate hepatic steatosis via stimulating hepatic ER stress. Furthermore, exosome-melatonin supplementation can lower hepatic inflammation, fibrosis, and cell apoptosis (257).
The growing prevalence of NAFLD and its global health burden have provoked considerable research on possible diagnostic and therapeutic options for NAFLD. NAFLD is a multifactorial disease which includes a broad spectrum of liver damage. Although various pathophysiological mechanisms and genetic factors have been proven to be related to NAFLD, its treatment remains challenging. In recent years, exosomes have attracted widespread attention for their role in metabolic dysfunctions and their efficacy as pathological biomarkers. Exosomes have also shown tremendous potential in treating various disorders, such as wound healing and neurological and cardiovascular dysfunctions. With increasing evidence supporting the significant role of exosomes in NAFLD pathogenesis, their theragnostic potential has become a point of interest in NAFLD. Considering the low cost and high feasibility of EV detection and its non-invasiveness, using exosomes as a diagnostic biomarker can be a revolution in NAFLD diagnosis. Exosome-based therapies can also bring favorable results in preventing and treating NAFLD. However, there are still certain limitations that need to be overcome, and many questions need to be answered before exosomes can be utilized as a routine treatment for liver diseases.
Contrary to the satisfactory results of experimental exosome therapy studies, exosomal therapy methods and safety are not entirely clarified (258). Exosome-based therapy methods face both pharmaceutical (production, isolation, and drug loading) and pharmacokinetic (biodistribution and cellular uptake) challenges in becoming clinical. One of the main limitations of exosome therapy is the preparation and purification of a large volume of desired exosomes. Moreover, designing standard methods to isolate and identify the types and sources of exosomes also needs to be investigated more since the purity and physicochemical properties of exosomes are strongly affected by their isolation method, and no globally accepted standard is currently available in this era. Besides, considering exosomes' degradation during isolation and freeze-thaw processes, standardization of isolation and storage conditions is also crucial (148, 259–261). Another emerging challenge in exosomal therapy is that natural exosomes, also known as cell-derived exosomes, contain a multitude of biomolecules with unknown effects. Cells in different environmental conditions release exosomes with diverse cargo, complicating the predictability of cell-derived exosome therapies (262). It also makes finding the effective agent challenging. Therefore, a comprehensive review of the content of exosomes and their effect on pathologic and healthy tissues is also necessary. Additionally, although exosomes are capable of cell targeting, concerns about their intrahepatic and intrasplenic accumulation remain to be solved, as well as their relatively short half-life. These problems may be manageable if different tissue targeting strategies are used in conjunction (168). Finally, suppressing exosome secretion or function as a therapeutic strategy is far from utilization, as it requires a further in-depth understanding of the exosome's role in normal physiology (259). Accordingly, there are still serious challenges in using exosome-based therapies as a routine clinical option that should be overcome.
Recent findings on exosomes have provided promising prospects of using them as feasible, costly, and reliable diagnostic options in NAFLD. In addition, exosomal-based therapies, having low immunogenicity and high biocompatibility, for drug delivery as an effective treatment option or by inhibiting their actions as a treatment strategy have been associated with favorable results in NAFLD. However, despite several pre-clinical studies on exosomes in liver disorders, the passage to the clinical setting remains unsure. There are still certain challenges to using exosomal-based therapies in NAFLD. Besides, the feasibility of exosome-related treatment strategies in NAFLD will await further evaluation due to the critical role of exosomes in numerous physiological conditions. Due to the great potential of this novel theragnostic agent in NAFLD, further investigations on their safety, clinical efficacy, and application standardization are highly recommended.
AT: Conceptualization, Investigation, Supervision, Validation, Visualization, Writing – original draft, Writing – review & editing. MJ: Investigation, Writing – original draft. NS-P: Visualization, Writing – review & editing. AM: Investigation, Writing – original draft.
The author(s) declare that no financial support was received for the research, authorship, and/or publication of this article.
I want to express our sincere gratitude to the members of our research team for their contributions and support throughout this project. Their dedication and hard work were essential in achieving our research goals.
The authors declare that the research was conducted in the absence of any commercial or financial relationships that could be construed as a potential conflict of interest.
All claims expressed in this article are solely those of the authors and do not necessarily represent those of their affiliated organizations, or those of the publisher, the editors and the reviewers. Any product that may be evaluated in this article, or claim that may be made by its manufacturer, is not guaranteed or endorsed by the publisher.
1. Chalasani N, Younossi Z, Lavine JE, Diehl AM, Brunt EM, Cusi K, et al. The diagnosis and management of non-alcoholic fatty liver disease: practice guideline by the American Association for the Study of Liver Diseases, American College of Gastroenterology, and the American Gastroenterological Association. Hepatology. (2012) 55:2005–23. doi: 10.1002/hep.25762
2. Welsh JA, Karpen S, Vos MB. Increasing prevalence of nonalcoholic fatty liver disease among United States adolescents, 1988-1994 to 2007-2010. J Pediatr. (2013) 162:496–500.e1. doi: 10.1016/j.jpeds.2012.08.043
3. Eguchi Y, Hyogo H, Ono M, Mizuta T, Ono N, Fujimoto K, et al. Prevalence and associated metabolic factors of nonalcoholic fatty liver disease in the general population from 2009 to 2010 in Japan: a multicenter large retrospective study. J Gastroenterol. (2012) 47:586–95. doi: 10.1007/s00535-012-0533-z
4. De A, Duseja A. Natural history of simple steatosis or nonalcoholic fatty liver. J Clin Exp Hepatol. (2020) 10:255–62. doi: 10.1016/j.jceh.2019.09.005
5. Loomba R, Sanyal AJ. The global NAFLD epidemic. Nat Rev Gastroenterol Hepatol. (2013) 10:686–90. doi: 10.1038/nrgastro.2013.171
6. Friedman SL, Neuschwander-Tetri BA, Rinella M, Sanyal AJ. Mechanisms of NAFLD development and therapeutic strategies. Nat Med. (2018) 24:908–22. doi: 10.1038/s41591-018-0104-9
7. Rowe IA, Wong VW-S, Loomba R. Treatment candidacy for pharmacologic therapies for NASH. Clin Gastroenterol Hepatol. (2021) 3:5. doi: 10.1016/j.cgh.2021.03.005
8. Muthu S, Bapat A, Jain R, Jeyaraman N, Jeyaraman M. Exosomal therapy-a new frontier in regenerative medicine. Stem Cell Invest. (2021) 8:37. doi: 10.21037/sci-2020-037
9. Jeyaraman M, Muthu S, Gulati A, Jeyaraman N, Jain R. Mesenchymal stem cell-derived exosomes: a potential therapeutic avenue in knee osteoarthritis. Cartilage. (2021) 13(1_Suppl.):1572S−85S. doi: 10.1177/1947603520962567
10. Théry C, Witwer KW, Aikawa E, Alcaraz MJ, Anderson JD, Andriantsitohaina R, et al. Minimal information for studies of extracellular vesicles 2018 (MISEV2018): a position statement of the International Society for Extracellular Vesicles and update of the MISEV2014 guidelines. J Extracell Vesicl. (2018) 7:1535750. doi: 10.1080/20013078.2018.1535750
11. Cocucci E, Meldolesi J. Ectosomes and exosomes: shedding the confusion between extracellular vesicles. Trends Cell Biol. (2015) 25:364–72. doi: 10.1016/j.tcb.2015.01.004
12. Caby M-P, Lankar D, Vincendeau-Scherrer C, Raposo G, Bonnerot C. Exosomal-like vesicles are present in human blood plasma. Int Immunol. (2005) 17:879–87. doi: 10.1093/intimm/dxh267
13. Pisitkun T, Shen R-F, Knepper MA. Identification and proteomic profiling of exosomes in human urine. Proc Nat Acad Sci USA. (2004) 101:13368–73. doi: 10.1073/pnas.0403453101
14. Michael A, Bajracharya SD, Yuen PS, Zhou H, Star RA, Illei GG, et al. Exosomes from human saliva as a source of microRNA biomarkers. Oral Dis. (2010) 16:34–8. doi: 10.1111/j.1601-0825.2009.01604.x
15. Admyre C, Johansson SM, Qazi KR, Filén J-J, Lahesmaa R, Norman M, et al. Exosomes with immune modulatory features are present in human breast milk. J Immunol. (2007) 179:1969–78. doi: 10.4049/jimmunol.179.3.1969
16. Mathivanan S, Fahner CJ, Reid GE, Simpson RJ. ExoCarta 2012: database of exosomal proteins, RNA and lipids. Nucleic Acids Res. (2012) 40:D1241–D4. doi: 10.1093/nar/gkr828
17. Théry C, Ostrowski M, Segura E. Membrane vesicles as conveyors of immune responses. Nat Rev Immunol. (2009) 9:581–93. doi: 10.1038/nri2567
18. Daaboul GG, Gagni P, Benussi L, Bettotti P, Ciani M, Cretich M, et al. Digital detection of exosomes by interferometric imaging. Sci Rep. (2016) 6:1–10. doi: 10.1038/srep37246
19. Pitt JM, Kroemer G, Zitvogel L. Extracellular vesicles: masters of intercellular communication and potential clinical interventions. J Clin Invest. (2016) 126:1139–43. doi: 10.1172/JCI87316
20. Van Niel G, d'Angelo G, Raposo G. Shedding light on the cell biology of extracellular vesicles. Nat Rev Mol Cell Biol. (2018) 19:213–28. doi: 10.1038/nrm.2017.125
21. Xie F, Zhou X, Fang M, Li H, Su P, Tu Y, et al. Extracellular vesicles in cancer immune microenvironment and cancer immunotherapy. Adv Sci. (2019) 6:1901779. doi: 10.1002/advs.201901779
22. Valadi H, Ekström K, Bossios A, Sjöstrand M, Lee JJ, Lötvall JO. Exosome-mediated transfer of mRNAs and microRNAs is a novel mechanism of genetic exchange between cells. Nat Cell Biol. (2007) 9:654–9. doi: 10.1038/ncb1596
23. Kalluri R, LeBleu VS. The biology, function, and biomedical applications of exosomes. Science. (2020) 367:eaau6977. doi: 10.1126/science.aau6977
24. Krylova SV, Feng D. The machinery of exosomes: biogenesis, release, and uptake. Int J Mol Sci. (2023) 24:1337. doi: 10.3390/ijms24021337
25. Han Q-F, Li W-J, Hu K-S, Gao J, Zhai W-L, Yang J-H, et al. Exosome biogenesis: machinery, regulation, and therapeutic implications in cancer. Mol Cancer. (2022) 21:207. doi: 10.1186/s12943-022-01671-0
26. Xie S, Zhang Q, Jiang L. Current knowledge on exosome biogenesis, cargo-sorting mechanism and therapeutic implications. Membranes. (2022) 12:498. doi: 10.3390/membranes12050498
27. Hessvik NP, Llorente A. Current knowledge on exosome biogenesis and release. Cell Mol Life Sci. (2018) 75:193–208. doi: 10.1007/s00018-017-2595-9
28. Zhang Y, Liu Y, Liu H, Tang WH. Exosomes: biogenesis, biologic function and clinical potential. Cell Biosci. (2019) 9:1–18. doi: 10.1186/s13578-019-0282-2
29. Lau NCH, Yam JWP. From exosome biogenesis to absorption: key takeaways for cancer research. Cancers. (2023) 15:1992. doi: 10.3390/cancers15071992
30. Andreu Z, Yáñez-Mó M. Tetraspanins in extracellular vesicle formation and function. Front Immunol. (2014) 5:442. doi: 10.3389/fimmu.2014.00442
31. Larios J, Mercier V, Roux A, Gruenberg J. ALIX-and ESCRT-III-dependent sorting of tetraspanins to exosomes. J Cell Biol. (2020) 219:4113. doi: 10.1083/jcb.201904113
32. Kalluri R. The biology and function of exosomes in cancer. J Clin Invest. (2016) 126:1208–15. doi: 10.1172/JCI81135
33. Pegtel DM, Gould SJ. Exosomes. Annu Rev Biochem. (2019) 88:487–514. doi: 10.1146/annurev-biochem-013118-111902
34. Robbins PD, Morelli AE. Regulation of immune responses by extracellular vesicles. Nat Rev Immunol. (2014) 14:195–208. doi: 10.1038/nri3622
35. Becker A, Thakur BK, Weiss JM, Kim HS, Peinado H, Lyden D. Extracellular vesicles in cancer: cell-to-cell mediators of metastasis. Cancer Cell. (2016) 30:836–48. doi: 10.1016/j.ccell.2016.10.009
36. Soung YH, Ford S, Zhang V, Chung J. Exosomes in cancer diagnostics. Cancers. (2017) 9:8. doi: 10.3390/cancers9010008
37. Zhang Y, Hu Y-W, Zheng L, Wang Q. Characteristics and roles of exosomes in cardiovascular disease. DNA Cell Biol. (2017) 36:202–11. doi: 10.1089/dna.2016.3496
38. Zhang W, Zhou X, Zhang H, Yao Q, Liu Y, Dong Z. Extracellular vesicles in diagnosis and therapy of kidney diseases. Am J Physiol Renal Physiol. (2016) 311:F844–F51. doi: 10.1152/ajprenal.00429.2016
39. Alipoor SD, Mortaz E, Varahram M, Movassaghi M, Kraneveld AD, Garssen J, et al. The potential biomarkers and immunological effects of tumor-derived exosomes in lung cancer. Front Immunol. (2018) 9:819. doi: 10.3389/fimmu.2018.00819
40. Kanninen KM, Bister N, Koistinaho J, Malm T. Exosomes as new diagnostic tools in CNS diseases. Biochimica et Biophysica Acta. (2016) 1862:403–10. doi: 10.1016/j.bbadis.2015.09.020
41. Fitts CA, Ji N, Li Y, Tan C. Exploiting exosomes in cancer liquid biopsies and drug delivery. Adv Healthc Mater. (2019) 8:1801268. doi: 10.1002/adhm.201801268
42. Masyuk AI, Masyuk TV, LaRusso NF. Exosomes in the pathogenesis, diagnostics and therapeutics of liver diseases. J Hepatol. (2013) 59:621–5. doi: 10.1016/j.jhep.2013.03.028
43. Yang Y, Hong Y, Cho E, Kim GB, Kim I-S. Extracellular vesicles as a platform for membrane-associated therapeutic protein delivery. J Extracell Vesicl. (2018) 7:1440131. doi: 10.1080/20013078.2018.1440131
44. Jiang X-C, Gao J-Q. Exosomes as novel bio-carriers for gene and drug delivery. Int J Pharm. (2017) 521:167–75. doi: 10.1016/j.ijpharm.2017.02.038
45. Zhang B, Yin Y, Lai RC, Tan SS, Choo ABH, Lim SK. Mesenchymal stem cells secrete immunologically active exosomes. Stem Cells Dev. (2014) 23:1233–44. doi: 10.1089/scd.2013.0479
46. Lee C, Mitsialis SA, Aslam M, Vitali SH, Vergadi E, Konstantinou G, et al. Exosomes mediate the cytoprotective action of mesenchymal stromal cells on hypoxia-induced pulmonary hypertension. Circulation. (2012) 126:2601–11. doi: 10.1161/CIRCULATIONAHA.112.114173
47. Kanazawa H, Fujimoto Y, Teratani T, Iwasaki J, Kasahara N, Negishi K, et al. Bone marrow-derived mesenchymal stem cells ameliorate hepatic ischemia reperfusion injury in a rat model. PLoS ONE. (2011) 6:e19195. doi: 10.1371/journal.pone.0019195
48. Lai RC, Arslan F, Lee MM, Sze NSK, Choo A, Chen TS, et al. Exosome secreted by MSC reduces myocardial ischemia/reperfusion injury. Stem Cell Res. (2010) 4:214–22. doi: 10.1016/j.scr.2009.12.003
49. Salomon C, Ryan J, Sobrevia L, Kobayashi M, Ashman K, Mitchell M, et al. Exosomal signaling during hypoxia mediates microvascular endothelial cell migration and vasculogenesis. PLoS ONE. (2013) 8:e68451. doi: 10.1371/journal.pone.0068451
50. Chen J, Liu Z, Hong MM, Zhang H, Chen C, Xiao M, et al. Proangiogenic compositions of microvesicles derived from human umbilical cord mesenchymal stem cells. PLoS ONE. (2014) 9:e115316. doi: 10.1371/journal.pone.0115316
51. Rani S, Ryan AE, Griffin MD, Ritter T. Mesenchymal stem cell-derived extracellular vesicles: toward cell-free therapeutic applications. Mol Ther. (2015) 23:812–23. doi: 10.1038/mt.2015.44
52. Fang YL, Chen H, Wang CL, Liang L. Pathogenesis of non-alcoholic fatty liver disease in children and adolescence: From “two hit theory” to “multiple hit model”. World J Gastroenterol. (2018) 24:2974–83. doi: 10.3748/wjg.v24.i27.2974
53. Schwimmer JB, Behling C, Newbury R, Deutsch R, Nievergelt C, Schork NJ, et al. Histopathology of pediatric nonalcoholic fatty liver disease. Hepatology. (2005) 42:641–9. doi: 10.1002/hep.20842
54. Ratziu V, Bellentani S, Cortez-Pinto H, Day C, Marchesini G. A position statement on NAFLD/NASH based on the EASL 2009 special conference. J Hepatol. (2010) 53:372–84. doi: 10.1016/j.jhep.2010.04.008
55. Berardis S, Sokal E. Pediatric non-alcoholic fatty liver disease: an increasing public health issue. Eur J Pediatr. (2014) 173:131–9. doi: 10.1007/s00431-013-2157-6
56. Alisi A, Cianfarani S, Manco M, Agostoni C, Nobili V. Non-alcoholic fatty liver disease and metabolic syndrome in adolescents: pathogenetic role of genetic background and intrauterine environment. Ann Med. (2012) 44:29–40. doi: 10.3109/07853890.2010.547869
57. Ayonrinde OT, Olynyk JK, Marsh JA, Beilin LJ, Mori TA, Oddy WH, et al. Childhood adiposity trajectories and risk of nonalcoholic fatty liver disease in adolescents. J Gastroenterol Hepatol. (2015) 30:163–71. doi: 10.1111/jgh.12666
58. Cohen JC, Horton JD, Hobbs HH. Human fatty liver disease: old questions and new insights. Science. (2011) 332:1519–23. doi: 10.1126/science.1204265
59. Lomonaco R, Ortiz-Lopez C, Orsak B, Webb A, Hardies J, Darland C, et al. Effect of adipose tissue insulin resistance on metabolic parameters and liver histology in obese patients with nonalcoholic fatty liver disease. Hepatology. (2012) 55:1389–97. doi: 10.1002/hep.25539
60. Donnelly KL, Smith CI, Schwarzenberg SJ, Jessurun J, Boldt MD, Parks EJ. Sources of fatty acids stored in liver and secreted via lipoproteins in patients with nonalcoholic fatty liver disease. J Clin Invest. (2005) 115:1343–51. doi: 10.1172/JCI200523621
61. Han J, Kaufman RJ. The role of ER stress in lipid metabolism and lipotoxicity. J Lipid Res. (2016) 57:1329–38. doi: 10.1194/jlr.R067595
62. Puri P, Mirshahi F, Cheung O, Natarajan R, Maher JW, Kellum JM, et al. Activation and dysregulation of the unfolded protein response in nonalcoholic fatty liver disease. Gastroenterology. (2008) 134:568–76. doi: 10.1053/j.gastro.2007.10.039
63. Szabo G, Petrasek J. Inflammasome activation and function in liver disease. Nat Rev Gastroenterol Hepatol. (2015) 12:387–400. doi: 10.1038/nrgastro.2015.94
64. Guy CD, Suzuki A, Zdanowicz M, Abdelmalek MF, Burchette J, Unalp A, et al. Hedgehog pathway activation parallels histologic severity of injury and fibrosis in human nonalcoholic fatty liver disease. Hepatology. (2012) 55:1711–21. doi: 10.1002/hep.25559
65. Loomba R, Abraham M, Unalp A, Wilson L, Lavine J, Doo E, et al. Association between diabetes, family history of diabetes, and risk of nonalcoholic steatohepatitis and fibrosis. Hepatology. (2012) 56:943–51. doi: 10.1002/hep.25772
66. Marra F, Bertolani C. Adipokines in liver diseases. Hepatology. (2009) 50:957–69. doi: 10.1002/hep.23046
67. Abdelmalek MF, Lazo M, Horska A, Bonekamp S, Lipkin EW, Balasubramanyam A, et al. Higher dietary fructose is associated with impaired hepatic adenosine triphosphate homeostasis in obese individuals with type 2 diabetes. Hepatology. (2012) 56:952–60. doi: 10.1002/hep.25741
68. Lanaspa MA, Sanchez-Lozada LG, Choi YJ, Cicerchi C, Kanbay M, Roncal-Jimenez CA, et al. Uric acid induces hepatic steatosis by generation of mitochondrial oxidative stress: potential role in fructose-dependent and -independent fatty liver. J Biol Chem. (2012) 287:40732–44. doi: 10.1074/jbc.M112.399899
69. Henao-Mejia J, Elinav E, Jin C, Hao L, Mehal WZ, Strowig T, et al. Inflammasome-mediated dysbiosis regulates progression of NAFLD and obesity. Nature. (2012) 482:179–85. doi: 10.1038/nature10809
70. Tsuchida T, Friedman SL. Mechanisms of hepatic stellate cell activation. Nat Rev Gastroenterol Hepatol. (2017) 14:397–411. doi: 10.1038/nrgastro.2017.38
71. Sato K, Meng F, Glaser S, Alpini G. Exosomes in liver pathology. J Hepatol. (2016) 65:213–21. doi: 10.1016/j.jhep.2016.03.004
72. Povero D, Eguchi A, Li H, Johnson CD, Papouchado BG, Wree A, et al. Circulating extracellular vesicles with specific proteome and liver microRNAs are potential biomarkers for liver injury in experimental fatty liver disease. PLoS ONE. (2014) 9:e113651. doi: 10.1371/journal.pone.0113651
73. Yao ZY, Chen WB, Shao SS, Ma SZ, Yang CB, Li MZ, et al. Role of exosome-associated microRNA in diagnostic and therapeutic applications to metabolic disorders. J Zhejiang Univ Sci B. (2018) 19:183–98. doi: 10.1631/jzus.B1600490
74. Sato K, Kennedy L, Liangpunsakul S, Kusumanchi P, Yang Z, Meng F, et al. Intercellular communication between hepatic cells in liver diseases. Int J Mol Sci. (2019) 20:92180. doi: 10.3390/ijms20092180
75. European Association for the Study of the L, European Association for the Study of D, European Association for the Study of O. EASL-EASD-EASO Clinical Practice Guidelines for the management of non-alcoholic fatty liver disease. Diabetologia. (2016) 59:1121–40. doi: 10.1007/s00125-016-3902-y
76. Ban LA, Shackel NA, McLennan SV. Extracellular vesicles: a new frontier in biomarker discovery for non-alcoholic fatty liver disease. Int J Mol Sci. (2016) 17:376. doi: 10.3390/ijms17030376
77. Kakazu E, Mauer AS, Yin M, Malhi H. Hepatocytes release ceramide-enriched pro-inflammatory extracellular vesicles in an IRE1α-dependent manner. J Lipid Res. (2016) 57:233–45. doi: 10.1194/jlr.M063412
78. Schuster S, Cabrera D, Arrese M, Feldstein AE. Triggering and resolution of inflammation in NASH. Nat Rev Gastroenterol Hepatol. (2018) 15:349–64. doi: 10.1038/s41575-018-0009-6
79. Li Y, Luan Y, Li J, Song H, Li Y, Qi H, et al. Exosomal miR-199a-5p promotes hepatic lipid accumulation by modulating MST1 expression and fatty acid metabolism. Hepatol Int. (2020) 14:1057–74. doi: 10.1007/s12072-020-10096-0
80. Xu Y, Zhu Y, Hu S, Pan X, Bawa FC, Wang HH, et al. Hepatocyte miR-34a is a key regulator in the development and progression of non-alcoholic fatty liver disease. Mol Metab. (2021) 51:101244. doi: 10.1016/j.molmet.2021.101244
81. Arrese M, Cabrera D, Kalergis AM, Feldstein AE. Innate immunity and inflammation in NAFLD/NASH. Dig Dis Sci. (2016) 61:1294–303. doi: 10.1007/s10620-016-4049-x
82. Hirsova P, Ibrahim SH, Krishnan A, Verma VK, Bronk SF, Werneburg NW, et al. Lipid-induced signaling causes release of inflammatory extracellular vesicles from hepatocytes. Gastroenterology. (2016) 150:956–67. doi: 10.1053/j.gastro.2015.12.037
83. Ibrahim SH, Hirsova P, Tomita K, Bronk SF, Werneburg NW, Harrison SA, et al. Mixed lineage kinase 3 mediates release of C-X-C motif ligand 10-bearing chemotactic extracellular vesicles from lipotoxic hepatocytes. Hepatology. (2016) 63:731–44. doi: 10.1002/hep.28252
84. Nakao Y, Fukushima M, Mauer AS, Liao CY, Ferris A, Dasgupta D, et al. A comparative proteomic analysis of extracellular vesicles associated with lipotoxicity. Front Cell Dev Biol. (2021) 9:735001. doi: 10.3389/fcell.2021.735001
85. Dasgupta D, Nakao Y, Mauer AS, Thompson JM, Sehrawat TS, Liao CY, et al. IRE1A stimulates hepatocyte-derived extracellular vesicles that promote inflammation in mice with steatohepatitis. Gastroenterology. (2020) 159:1487–503.e17. doi: 10.1053/j.gastro.2020.06.031
86. Vonderlin J, Chavakis T, Sieweke M, Tacke F. The multifaceted roles of macrophages in NAFLD pathogenesis. Cell Mol Gastroenterol Hepatol. (2023) 15:1311–24. doi: 10.1016/j.jcmgh.2023.03.002
87. Wang C, Ma C, Gong L, Guo Y, Fu K, Zhang Y, et al. Macrophage polarization and its role in liver disease. Front Immunol. (2021) 12:803037. doi: 10.3389/fimmu.2021.803037
88. Shen M, Shen Y, Fan X, Men R, Ye T, Yang L. Roles of macrophages and exosomes in liver diseases. Front Med. (2020) 7:583691. doi: 10.3389/fmed.2020.583691
89. Lu W, Bai L, Chen Y. The role of macrophage-derived exosomes in liver diseases. Infect Dis Immunity. (2022) 2:34. doi: 10.1097/ID9.0000000000000034
90. Liu XL, Cao HX, Fan JG. MicroRNAs as biomarkers and regulators of nonalcoholic fatty liver disease. J Dig Dis. (2016) 17:708–15. doi: 10.1111/1751-2980.12408
91. Liu X-L, Pan Q, Cao H-X, Xin F-Z, Zhao Z-H, Yang R-X, et al. Lipotoxic hepatocyte-derived exosomal MicroRNA 192-5p activates macrophages through Rictor/Akt/Forkhead box transcription factor O1 signaling in nonalcoholic fatty liver disease. Hepatology. (2020) 72:454–69. doi: 10.1002/hep.31050
92. Zhao Z, Zhong L, Li P, He K, Qiu C, Zhao L, et al. Cholesterol impairs hepatocyte lysosomal function causing M1 polarization of macrophages via exosomal miR-122-5p. Exp Cell Res. (2020) 387:111738. doi: 10.1016/j.yexcr.2019.111738
93. Cheng D, Chai J, Wang H, Fu L, Peng S, Ni X. Hepatic macrophages: key players in the development and progression of liver fibrosis. Liver Int. (2021) 41:2279–94. doi: 10.1111/liv.14940
94. Wan Z, Yang X, Liu X, Sun Y, Yu P, Xu F, et al. M2 macrophage-derived exosomal microRNA-411-5p impedes the activation of hepatic stellate cells by targeting CAMSAP1 in NASH model. iScience. (2022) 25:104597. doi: 10.1016/j.isci.2022.104597
95. Sakai Y, Chen G, Ni Y, Zhuge F, Xu L, Nagata N, et al. DPP-4 inhibition with anagliptin reduces lipotoxicity-induced insulin resistance and steatohepatitis in male mice. Endocrinology. (2020) 161:bqaa139. doi: 10.1210/endocr/bqaa139
96. Su SB, Qin SY, Xian XL, Huang FF, Huang QL, Zhang Di HJ, et al. Interleukin-22 regulating Kupffer cell polarization through STAT3/Erk/Akt crosstalk pathways to extenuate liver fibrosis. Life Sci. (2021) 264:118677. doi: 10.1016/j.lfs.2020.118677
97. Yang Y, Wu XQ, Li WX, Huang HM, Li HD, Pan XY, et al. PSTPIP2 connects DNA methylation to macrophage polarization in CCL4-induced mouse model of hepatic fibrosis. Oncogene. (2018) 37:6119–35. doi: 10.1038/s41388-018-0383-0
98. Chen T, Liu R, Niu Y, Mo H, Wang H, Lu Y, et al. HIF-1α-activated long non-coding RNA KDM4A-AS1 promotes hepatocellular carcinoma progression via the miR-411-5p/KPNA2/AKT pathway. Cell Death Dis. (2021) 12:1152. doi: 10.1038/s41419-021-04449-2
99. Duan WJ, Li YF, Liu FL, Deng J, Wu YP, Yuan WL, et al. A SIRT3/AMPK/autophagy network orchestrates the protective effects of trans-resveratrol in stressed peritoneal macrophages and RAW 2647 macrophages. Free Rad Biol Med. (2016) 95:230–42. doi: 10.1016/j.freeradbiomed.2016.03.022
100. Yang L, Liu S, He Y, Gan L, Ni Q, Dai A, et al. Exosomes regulate SIRT3-related autophagy by delivering miR-421 to regulate macrophage polarization and participate in OSA-related NAFLD. J Transl Med. (2024) 22:475. doi: 10.1186/s12967-024-05283-8
101. Shen Y, Malik SA, Amir M, Kumar P, Cingolani F, Wen J, et al. Decreased hepatocyte autophagy leads to synergistic IL-1β and TNF mouse liver injury and inflammation. Hepatology. (2020) 72:595–608. doi: 10.1002/hep.31209
102. Balaphas A, Meyer J, Sadoul K, Fontana P, Morel P, Gonelle-Gispert C, et al. Platelets and platelet-derived extracellular vesicles in liver physiology and disease. Hepatol Commun. (2019) 3:855–66. doi: 10.1002/hep4.1358
103. Chauhan A, Adams DH, Watson SP, Lalor PF. Platelets: no longer bystanders in liver disease. Hepatology. (2016) 64:1774–84. doi: 10.1002/hep.28526
104. Lee Y-S, Kim SY, Ko E, Lee J-H, Yi H-S, Yoo YJ, et al. Exosomes derived from palmitic acid-treated hepatocytes induce fibrotic activation of hepatic stellate cells. Sci Rep. (2017) 7:3710. doi: 10.1038/s41598-017-03389-2
105. Wang W, Li F, Lai X, Liu H, Wu S, Han Y, et al. Exosomes secreted by palmitic acid-treated hepatocytes promote LX-2 cell activation by transferring miRNA-107. Cell Death Discov. (2021) 7:174. doi: 10.1038/s41420-021-00536-7
106. Qi Y, Ren S, Ye J, Tian Y, Wang G, Zhang S, et al. Infection microenvironment-activated core-shell nanoassemblies for photothermal/chemodynamic synergistic wound therapy and multimodal imaging. Acta Biomater. (2022) 143:445–58. doi: 10.1016/j.actbio.2022.02.034
107. Hou X, Yin S, Ren R, Liu S, Yong L, Liu Y, et al. Myeloid-cell-specific IL-6 signaling promotes MicroRNA-223-enriched exosome production to attenuate NAFLD-associated fibrosis. Hepatology. (2021) 74:116–32. doi: 10.1002/hep.31658
108. Afrisham R, Sadegh-Nejadi S, Meshkani R, Emamgholipour S, Paknejad M. Effect of circulating exosomes derived from normal-weight and obese women on gluconeogenesis, glycogenesis, lipogenesis and secretion of FGF21 and fetuin A in HepG2 cells. Diabetol Metab Syndr. (2020) 12:32. doi: 10.1186/s13098-020-00540-4
109. Koeck ES, Iordanskaia T, Sevilla S, Ferrante SC, Hubal MJ, Freishtat RJ, et al. Adipocyte exosomes induce transforming growth factor beta pathway dysregulation in hepatocytes: a novel paradigm for obesity-related liver disease. J Surg Res. (2014) 192:268–75. doi: 10.1016/j.jss.2014.06.050
110. Baranova A, Maltseva D, Tonevitsky A. Adipose may actively delay progression of NAFLD by releasing tumor-suppressing, anti-fibrotic miR-122 into circulation. Obes Rev. (2019) 20:108–18. doi: 10.1111/obr.12765
111. Ogur HU, Kapukaya R, Çiloglu O, Külahci Ö, Tekbaş VT, Yörükoglu A. Treatment of open wounds secondary to trauma using polyurethane foams with boric acid particles. Ulusal travma ve acil cerrahi dergisi. (2021) 27:624–30. doi: 10.14744/tjtes.2020.38613
112. Yan C, Tian X, Li J, Liu D, Ye D, Xie Z, et al. A high-fat diet attenuates AMPK α1 in adipocytes to induce exosome shedding and nonalcoholic fatty liver development in vivo. Diabetes. (2021) 70:577–88. doi: 10.2337/db20-0146
113. Zhao J, Hu L, Gui W, Xiao L, Wang W, Xia J, et al. Hepatocyte TGF-β signaling inhibiting WAT browning to promote NAFLD and obesity is associated with Let-7b-5p. Hepatol Commun. (2022) 6:1301–21. doi: 10.1002/hep4.1892
114. Fuchs A, Samovski D, Smith GI, Cifarelli V, Farabi SS, Yoshino J, et al. Associations among adipose tissue immunology, inflammation, exosomes and insulin sensitivity in people with obesity and nonalcoholic fatty liver disease. Gastroenterology. (2021) 161:968–81.e12. doi: 10.1053/j.gastro.2021.05.008
115. Sakurai Y, Kubota N, Yamauchi T, Kadowaki T. Role of insulin resistance in MAFLD. Int J Mol Sci. (2021) 22:4156. doi: 10.3390/ijms22084156
116. Zhao H, Shang Q, Pan Z, Bai Y, Li Z, Zhang H, et al. Exosomes from adipose-derived stem cells attenuate adipose inflammation and obesity through polarizing M2 macrophages and beiging in white adipose tissue. Diabetes. (2018) 67:235–47. doi: 10.2337/db17-0356
117. Chen Y, Sun H, Bai Y, Zhi F. Gut dysbiosis-derived exosomes trigger hepatic steatosis by transiting HMGB1 from intestinal to liver in mice. Biochem Biophys Res Commun. (2019) 509:767–72. doi: 10.1016/j.bbrc.2018.12.180
118. Ma S, Shao S, Yang C, Yao Z, Gao L, Chen W, et al. preliminary study: proteomic analysis of exosomes derived from thyroid-stimulating hormone-stimulated HepG2 cells. J Endocrinol Invest. (2020) 43:1229–38. doi: 10.1007/s40618-020-01210-y
119. Zheng D, Huo M, Li B, Wang W, Piao H, Wang Y, et al. The role of exosomes and exosomal MicroRNA in cardiovascular disease. Front Cell Develop Biol. (2021) 8:616161. doi: 10.3389/fcell.2020.616161
120. Zhang JW, Pan HT. microRNA profiles of serum exosomes derived from children with nonalcoholic fatty liver. Genes Genomics. (2022) 44:879–88. doi: 10.1007/s13258-021-01150-8
121. Zhou X, Huang K, Jia J, Ni Y, Yuan J, Liang X, et al. Exosomal miRNAs profile in children's nonalcoholic fatty liver disease and the correlation with transaminase and uric acid. Ann Nutr Metab. (2020) 76:44–53. doi: 10.1159/000506665
122. Lewis AP, Jopling CL. Regulation and biological function of the liver-specific miR-122. Biochem Soc Trans. (2010) 38:1553–7. doi: 10.1042/BST0381553
123. Pirola CJ, Fernández Gianotti T, Castaño GO, Mallardi P, San Martino J, Mora Gonzalez Lopez Ledesma M, et al. Circulating microRNA signature in non-alcoholic fatty liver disease: from serum non-coding RNAs to liver histology and disease pathogenesis. Gut. (2015) 64:800–12. doi: 10.1136/gutjnl-2014-306996
124. Benhamouche-Trouillet S, Postic C. Emerging role of miR-21 in non-alcoholic fatty liver disease. Gut. (2016) 65:1781–3. doi: 10.1136/gutjnl-2015-310044
125. Loyer X, Paradis V, Hénique C, Vion AC, Colnot N, Guerin CL, et al. Liver microRNA-21 is overexpressed in non-alcoholic steatohepatitis and contributes to the disease in experimental models by inhibiting PPARα expression. Gut. (2016) 65:1882–94. doi: 10.1136/gutjnl-2014-308883
126. Ahn J, Lee H, Jung CH, Ha T. Lycopene inhibits hepatic steatosis via microRNA-21-induced downregulation of fatty acid-binding protein 7 in mice fed a high-fat diet. Mol Nutr Food Res. (2012) 56:1665–74. doi: 10.1002/mnfr.201200182
127. Sun C, Huang F, Liu X, Xiao X, Yang M, Hu G, et al. miR-21 regulates triglyceride and cholesterol metabolism in non-alcoholic fatty liver disease by targeting HMGCR. Int J Mol Med. (2015) 35:847–53. doi: 10.3892/ijmm.2015.2076
128. Wu H, Ng R, Chen X, Steer CJ, Song G. MicroRNA-21 is a potential link between non-alcoholic fatty liver disease and hepatocellular carcinoma via modulation of the HBP1-p53-Srebp1c pathway. Gut. (2016) 65:1850–60. doi: 10.1136/gutjnl-2014-308430
129. Dattaroy D, Pourhoseini S, Das S, Alhasson F, Seth RK, Nagarkatti M, et al. Micro-RNA 21 inhibition of SMAD7 enhances fibrogenesis via leptin-mediated NADPH oxidase in experimental and human nonalcoholic steatohepatitis. Am J Physiol Gastrointest Liver Physiol. (2015) 308:G298–312. doi: 10.1152/ajpgi.00346.2014
130. Caviglia JM, Yan J, Jang MK, Gwak GY, Affo S, Yu L, et al. MicroRNA-21 and Dicer are dispensable for hepatic stellate cell activation and the development of liver fibrosis. Hepatology. (2018) 67:2414–29. doi: 10.1002/hep.29627
131. Long JK, Dai W, Zheng YW, Zhao SP. miR-122 promotes hepatic lipogenesis via inhibiting the LKB1/AMPK pathway by targeting Sirt1 in non-alcoholic fatty liver disease. Mol Med. (2019) 25:26. doi: 10.1186/s10020-019-0085-2
132. Ding RB, Bao J, Deng CX. Emerging roles of SIRT1 in fatty liver diseases. Int J Biol Sci. (2017) 13:852–67. doi: 10.7150/ijbs.19370
133. Zhang M, Sun W, Zhou M, Tang Y. MicroRNA-27a regulates hepatic lipid metabolism and alleviates NAFLD via repressing FAS and SCD1. Sci Rep. (2017) 7:14493. doi: 10.1038/s41598-017-15141-x
134. Luo X, Xu ZX, Wu JC, Luo SZ, Xu MY. Hepatocyte-derived exosomal miR-27a activateshepatic stellate cells through the inhibitionof PINK1-mediated mitophagy in MAFLD. Mol Ther Nucleic Acids. (2021) 26:1241–54. doi: 10.1016/j.omtn.2021.10.022
135. Shen M, Pan H, Ke J, Zhao F. NF-κB-upregulated miR-155-5p promotes hepatocyte mitochondrial dysfunction to accelerate the development of nonalcoholic fatty liver disease through downregulation of STC1. J Biochem Mol Toxicol. (2022) 36:e23025. doi: 10.1002/jbt.23025
136. López-Pastor AR, Infante-Menéndez J, Escribano Ó, Gómez-Hernández A. miRNA dysregulation in the development of non-alcoholic fatty liver disease and the related disorders type 2 diabetes mellitus and cardiovascular disease. Front Med. (2020) 7:527059. doi: 10.3389/fmed.2020.527059
137. González-López P, Ares-Carral C, López-Pastor AR, Infante-Menéndez J, González Illaness T, Vega de Ceniga M, et al. Implication of miR-155-5p and miR-143-3p in the vascular insulin resistance and instability of human and experimental atherosclerotic plaque. Int J Mol Sci. (2022) 23:1810253. doi: 10.3390/ijms231810253
138. Ding J, Li M, Wan X, Jin X, Chen S, Yu C, et al. Effect of miR-34a in regulating steatosis by targeting PPARα expression in nonalcoholic fatty liver disease. Sci Rep. (2015) 5:13729. doi: 10.1038/srep13729
139. Lin Y, Ding D, Huang Q, Liu Q, Lu H, Lu Y, et al. Downregulation of miR-192 causes hepatic steatosis and lipid accumulation by inducing SREBF1: Novel mechanism for bisphenol A-triggered non-alcoholic fatty liver disease. Biochim Biophys Acta Mol Cell Biol Lipids. (2017) 1862:869–82. doi: 10.1016/j.bbalip.2017.05.001
140. Du X, Yang Y, Xu C, Peng Z, Zhang M, Lei L, et al. Upregulation of miR-181a impairs hepatic glucose and lipid homeostasis. Oncotarget. (2017) 8:91362–78. doi: 10.18632/oncotarget.20523
141. Yang YL, Wang PW, Wang FS, Lin HY, Huang YH. miR-29a Modulates GSK3β/SIRT1-linked mitochondrial proteostatic stress to ameliorate mouse non-alcoholic steatohepatitis. Int J Mol Sci. (2020) 21:186884. doi: 10.3390/ijms21186884
142. Gim JA, Bang SM, Lee YS, Lee Y, Yim SY, Jung YK, et al. Evaluation of the severity of nonalcoholic fatty liver disease through analysis of serum exosomal miRNA expression. PLoS ONE. (2021) 16:e0255822. doi: 10.1371/journal.pone.0255822
143. Nguyen HQ, Lee D, Kim Y, Bang G, Cho K, Lee YS, et al. Label-free quantitative proteomic analysis of serum extracellular vesicles differentiating patients of alcoholic and nonalcoholic fatty liver diseases. J Proteomics. (2021) 245:104278. doi: 10.1016/j.jprot.2021.104278
144. Scavo MP, Depalo N, Rizzi F, Carrieri L, Serino G, Franco I, et al. Exosomal FZD-7 expression is modulated by different lifestyle interventions in patients with NAFLD. Nutrients. (2022) 14:61133. doi: 10.3390/nu14061133
145. Sung S, Kim J, Jung Y. Liver-derived exosomes and their implications in liver pathobiology. Int J Mol Sci. (2018) 19:123715. doi: 10.3390/ijms19123715
146. Sumida Y, Yoneda M. Current and future pharmacological therapies for NAFLD/NASH. J Gastroenterol. (2018) 53:362–76. doi: 10.1007/s00535-017-1415-1
147. Chen J, Vitetta L. Gut microbiota metabolites in NAFLD pathogenesis and therapeutic implications. Int J Mol Sci. (2020) 21:155214. doi: 10.3390/ijms21155214
148. Rezaie J, Feghhi M, Etemadi T. A review on exosomes application in clinical trials: perspective, questions, and challenges. Cell Commun Signal. (2022) 20:145. doi: 10.1186/s12964-022-00959-4
149. Phinney DG, Pittenger MF. Concise review: MSC-derived exosomes for cell-free therapy. Stem Cells. (2017) 35:851–8. doi: 10.1002/stem.2575
150. Ibrahim AG, Cheng K, Marbán E. Exosomes as critical agents of cardiac regeneration triggered by cell therapy. Stem Cell Reports. (2014) 2:606–19. doi: 10.1016/j.stemcr.2014.04.006
151. Blans K, Hansen MS, Sørensen LV, Hvam ML, Howard KA, Möller A, et al. Pellet-free isolation of human and bovine milk extracellular vesicles by size-exclusion chromatography. J Extracell Vesicl. (2017) 6:1294340. doi: 10.1080/20013078.2017.1294340
152. Clayton A, Boilard E, Buzas EI, Cheng L, Falcón-Perez JM, Gardiner C, et al. Considerations towards a roadmap for collection, handling and storage of blood extracellular vesicles. J Extracell Vesicl. (2019) 8:1647027. doi: 10.1080/20013078.2019.1647027
153. Gallo A, Tandon M, Alevizos I, Illei GG. The majority of microRNAs detectable in serum and saliva is concentrated in exosomes. PLoS ONE. (2012) 7:e30679. doi: 10.1371/journal.pone.0030679
154. Iwai K, Minamisawa T, Suga K, Yajima Y, Shiba K. Isolation of human salivary extracellular vesicles by iodixanol density gradient ultracentrifugation and their characterizations. J Extracell Vesicl. (2016) 5:30829. doi: 10.3402/jev.v5.30829
155. Simpson RJ, Lim JW, Moritz RL, Mathivanan S. Exosomes: proteomic insights and diagnostic potential. Expert Rev Proteom. (2009) 6:267–83. doi: 10.1586/epr.09.17
156. Srinivasan S, Duval MX, Kaimal V, Cuff C, Clarke SH. Assessment of methods for serum extracellular vesicle small RNA sequencing to support biomarker development. J Extracell Vesicl. (2019) 8:1684425. doi: 10.1080/20013078.2019.1684425
157. Taylor DD, Gercel-Taylor C. MicroRNA signatures of tumor-derived exosomes as diagnostic biomarkers of ovarian cancer. Gynecol Oncol. (2008) 110:13–21. doi: 10.1016/j.ygyno.2008.04.033
158. Zonneveld MI, Brisson AR, van Herwijnen MJ, Tan S, van de Lest CH, Redegeld FA, et al. Recovery of extracellular vesicles from human breast milk is influenced by sample collection and vesicle isolation procedures. J Extracell Vesicl. (2014) 3:24215. doi: 10.3402/jev.v3.24215
159. Yeo RW, Lai RC, Zhang B, Tan SS, Yin Y, Teh BJ, et al. Mesenchymal stem cell: an efficient mass producer of exosomes for drug delivery. Adv Drug Deliv Rev. (2013) 65:336–41. doi: 10.1016/j.addr.2012.07.001
160. Kwon SG, Kwon YW, Lee TW, Park GT, Kim JH. Recent advances in stem cell therapeutics and tissue engineering strategies. Biomater Res. (2018) 22:36. doi: 10.1186/s40824-018-0148-4
161. Lai RC, Yeo RW, Lim SK. Mesenchymal stem cell exosomes. Semin Cell Dev Biol. (2015) 40:82–8. doi: 10.1016/j.semcdb.2015.03.001
162. Han C, Sun X, Liu L, Jiang H, Shen Y, Xu X, et al. Exosomes and their therapeutic potentials of stem cells. Stem Cells Int. (2016) 2016:7653489. doi: 10.1155/2016/7653489
163. Barbash IM, Chouraqui P, Baron J, Feinberg MS, Etzion S, Tessone A, et al. Systemic delivery of bone marrow-derived mesenchymal stem cells to the infarcted myocardium: feasibility, cell migration, and body distribution. Circulation. (2003) 108:863–8. doi: 10.1161/01.CIR.0000084828.50310.6A
164. Braid LR, Wood CA, Wiese DM, Ford BN. Intramuscular administration potentiates extended dwell time of mesenchymal stromal cells compared to other routes. Cytotherapy. (2018) 20:232–44. doi: 10.1016/j.jcyt.2017.09.013
165. Jung JW, Kwon M, Choi JC, Shin JW, Park IW, Choi BW, et al. Familial occurrence of pulmonary embolism after intravenous, adipose tissue-derived stem cell therapy. Yonsei Med J. (2013) 54:1293–6. doi: 10.3349/ymj.2013.54.5.1293
166. Yong KW, Wan Safwani WK, Xu F, Wan Abas WA, Choi JR, Pingguan-Murphy B. Cryopreservation of human mesenchymal stem cells for clinical applications: current methods and challenges. Biopreserv Biobank. (2015) 13:231–9. doi: 10.1089/bio.2014.0104
167. Keerthikumar S, Chisanga D, Ariyaratne D, Al Saffar H, Anand S, Zhao K, et al. ExoCarta: a web-based compendium of exosomal cargo. J Mol Biol. (2016) 428:688–92. doi: 10.1016/j.jmb.2015.09.019
168. Wu R, Fan X, Wang Y, Shen M, Zheng Y, Zhao S, et al. Mesenchymal stem cell-derived extracellular vesicles in liver immunity and therapy. Front Immunol. (2022) 13:833878. doi: 10.3389/fimmu.2022.833878
169. Zhang Z, Lin H, Shi M, Xu R, Fu J, Lv J, et al. Human umbilical cord mesenchymal stem cells improve liver function and ascites in decompensated liver cirrhosis patients. J Gastroenterol Hepatol. (2012) 27(Suppl.2):112–20. doi: 10.1111/j.1440-1746.2011.07024.x
170. Chai NL, Zhang XB, Chen SW, Fan KX, Linghu EQ. Umbilical cord-derived mesenchymal stem cells alleviate liver fibrosis in rats. World J Gastroenterol. (2016) 22:6036–48. doi: 10.3748/wjg.v22.i26.6036
171. Shi M, Zhang Z, Xu R, Lin H, Fu J, Zou Z, et al. Human mesenchymal stem cell transfusion is safe and improves liver function in acute-on-chronic liver failure patients. Stem Cells Transl Med. (2012) 1:725–31. doi: 10.5966/sctm.2012-0034
172. Yan W, Li D, Chen T, Tian G, Zhou P, Ju X. Umbilical cord MSCs reverse D-galactose-induced hepatic mitochondrial dysfunction via activation of Nrf2/HO-1 pathway. Biol Pharm Bull. (2017) 40:1174–82. doi: 10.1248/bpb.b16-00777
173. Yun JW, Ahn JH, Kwon E, Kim SH, Kim H, Jang JJ, et al. Human umbilical cord-derived mesenchymal stem cells in acute liver injury: Hepatoprotective efficacy, subchronic toxicity, tumorigenicity, and biodistribution. Regul Toxicol Pharmacol. (2016) 81:437–47. doi: 10.1016/j.yrtph.2016.09.029
174. Fan J, Tang X, Wang Q, Zhang Z, Wu S, Li W, et al. Mesenchymal stem cells alleviate experimental autoimmune cholangitis through immunosuppression and cytoprotective function mediated by galectin-9. Stem Cell Res Ther. (2018) 9:237. doi: 10.1186/s13287-018-0979-x
175. Li B, Cheng Y, Yu S, Zang L, Yin Y, Liu J, et al. Human umbilical cord-derived mesenchymal stem cell therapy ameliorates nonalcoholic fatty liver disease in obese type 2 diabetic mice. Stem Cells Int. (2019) 2019:8628027. doi: 10.1155/2019/8628027
176. Cheng L, Yu P, Li F, Jiang X, Jiao X, Shen Y, et al. Human umbilical cord-derived mesenchymal stem cell-exosomal miR-627-5p ameliorates non-alcoholic fatty liver disease by repressing FTO expression. Hum Cell. (2021) 34:1697–708. doi: 10.1007/s13577-021-00593-1
177. Schuhladen K, Stich L, Schmidt J, Steinkasserer A, Boccaccini AR, Zinser E. Cu, Zn doped borate bioactive glasses: antibacterial efficacy and dose-dependent in vitro modulation of murine dendritic cells. Biomater Sci. (2020) 8:2143–55. doi: 10.1039/C9BM01691K
178. Mizuno TM. Fat mass and obesity associated (FTO) gene and hepatic glucose and lipid metabolism. Nutrients. (2018) 10:166. doi: 10.20944/preprints201810.0166.v1
179. Kang Y, Song Y, Luo Y, Song J, Li C, Yang S, et al. Exosomes derived from human umbilical cord mesenchymal stem cells ameliorate experimental non-alcoholic steatohepatitis via Nrf2/NQO-1 pathway. Free Radic Biol Med. (2022) 192:25–36. doi: 10.1016/j.freeradbiomed.2022.08.037
180. Ross D, Siegel D. Functions of NQO1 in cellular protection and CoQ(10) metabolism and its potential role as a redox sensitive molecular switch. Front Physiol. (2017) 8:595. doi: 10.3389/fphys.2017.00595
181. Pittenger MF, Mackay AM, Beck SC, Jaiswal RK, Douglas R, Mosca JD, et al. Multilineage potential of adult human mesenchymal stem cells. Science. (1999) 284:143–7. doi: 10.1126/science.284.5411.143
182. Wada N, Gronthos S, Bartold PM. Immunomodulatory effects of stem cells. Periodontol. (2013) 63:198–216. doi: 10.1111/prd.12024
183. Nguyen TM, Arthur A, Hayball JD, Gronthos S. EphB and Ephrin-B interactions mediate human mesenchymal stem cell suppression of activated T-cells. Stem Cells Dev. (2013) 22:2751–64. doi: 10.1089/scd.2012.0676
184. Badiavas EV, Abedi M, Butmarc J, Falanga V, Quesenberry P. Participation of bone marrow derived cells in cutaneous wound healing. J Cell Physiol. (2003) 196:245–50. doi: 10.1002/jcp.10260
185. Awad HA, Butler DL, Boivin GP, Smith FN, Malaviya P, Huibregtse B, et al. Autologous mesenchymal stem cell-mediated repair of tendon. Tissue Eng. (1999) 5:267–77. doi: 10.1089/ten.1999.5.267
186. Squillaro T, Peluso G, Galderisi U. Clinical trials with mesenchymal stem cells: an update. Cell Transplant. (2016) 25:829–48. doi: 10.3727/096368915X689622
187. Pittenger MF, Discher DE, Péault BM, Phinney DG, Hare JM, Caplan AI. Mesenchymal stem cell perspective: cell biology to clinical progress. NPJ Regen Med. (2019) 4:22. doi: 10.1038/s41536-019-0083-6
188. Musiał-Wysocka A, Kot M, Majka M. The pros and cons of mesenchymal stem cell-based therapies. Cell Transplant. (2019) 28:801–12. doi: 10.1177/0963689719837897
189. Yin K, Wang S, Zhao RC. Exosomes from mesenchymal stem/stromal cells: a new therapeutic paradigm. Biomark Res. (2019) 7:8. doi: 10.1186/s40364-019-0159-x
190. El-Derany MO, AbdelHamid SG. Upregulation of miR-96-5p by bone marrow mesenchymal stem cells and their exosomes alleviate non-alcoholic steatohepatitis: Emphasis on caspase-2 signaling inhibition. Biochem Pharmacol. (2021) 190:114624. doi: 10.1016/j.bcp.2021.114624
191. Davis C, Dukes A, Drewry M, Helwa I, Johnson MH, Isales CM, et al. MicroRNA-183-5p increases with age in bone-derived extracellular vesicles, suppresses bone marrow stromal (stem) cell proliferation, and induces stem cell senescence. Tissue Eng A. (2017) 23:1231–40. doi: 10.1089/ten.tea.2016.0525
192. Desgagné V, Bouchard L, Guérin R. microRNAs in lipoprotein and lipid metabolism: from biological function to clinical application. Clin Chem Lab Med. (2017) 55:667–86. doi: 10.1515/cclm-2016-0575
193. Green CD, Huang Y, Dou X, Yang L, Liu Y, Han JJ. Impact of dietary interventions on noncoding RNA networks and mRNAs encoding chromatin-related factors. Cell Rep. (2017) 18:2957–68. doi: 10.1016/j.celrep.2017.03.001
194. Machado MV, Michelotti GA, Pereira Tde A, Boursier J, Kruger L, Swiderska-Syn M, et al. Reduced lipoapoptosis, hedgehog pathway activation and fibrosis in caspase-2 deficient mice with non-alcoholic steatohepatitis. Gut. (2015) 64:1148–57. doi: 10.1136/gutjnl-2014-307362
195. El-Derany MO, El-Demerdash E. Pyrvinium pamoate attenuates non-alcoholic steatohepatitis: Insight on hedgehog/Gli and Wnt/β-catenin signaling crosstalk. Biochem Pharmacol. (2020) 177:113942. doi: 10.1016/j.bcp.2020.113942
196. Seki E, Schwabe RF. Hepatic inflammation and fibrosis: functional links and key pathways. Hepatology. (2015) 61:1066–79. doi: 10.1002/hep.27332
197. Tacke F, Zimmermann HW. Macrophage heterogeneity in liver injury and fibrosis. J Hepatol. (2014) 60:1090–6. doi: 10.1016/j.jhep.2013.12.025
198. Ismail N, Wang Y, Dakhlallah D, Moldovan L, Agarwal K, Batte K, et al. Macrophage microvesicles induce macrophage differentiation and miR-223 transfer. Blood. (2013) 121:984–95. doi: 10.1182/blood-2011-08-374793
199. Li M, He Y, Zhou Z, Ramirez T, Gao Y, Gao Y, et al. MicroRNA-223 ameliorates alcoholic liver injury by inhibiting the IL-6-p47 (phox)-oxidative stress pathway in neutrophils. Gut. (2017) 66:705–15. doi: 10.1136/gutjnl-2016-311861
200. Neudecker V, Brodsky KS, Clambey ET, Schmidt EP, Packard TA, Davenport B, et al. Neutrophil transfer of miR-223 to lung epithelial cells dampens acute lung injury in mice. Sci Transl Med. (2017) 9:aah5360. doi: 10.1126/scitranslmed.aah5360
201. Isola AL, Chen S. Exosomes: the messengers of health and disease. Curr Neuropharmacol. (2017) 15:157–65. doi: 10.2174/1570159X14666160825160421
202. Abenavoli L, Peta V. Role of adipokines and cytokines in non-alcoholic fatty liver disease. Rev Recent Clin Trials. (2014) 9:134–40. doi: 10.2174/1574887109666141216102458
203. Stojsavljević S, Gomerčić Palčić M, Virović Jukić L, Smirčić Duvnjak L, Duvnjak M. Adipokines and proinflammatory cytokines, the key mediators in the pathogenesis of nonalcoholic fatty liver disease. World J Gastroenterol. (2014) 20:18070–91. doi: 10.3748/wjg.v20.i48.18070
204. Azzu V, Vacca M, Virtue S, Allison M, Vidal-Puig A. Adipose tissue-liver cross talk in the control of whole-body metabolism: implications in nonalcoholic fatty liver disease. Gastroenterology. (2020) 158:1899–912. doi: 10.1053/j.gastro.2019.12.054
205. Wang S, Luan J, Lv X. Inhibition of endoplasmic reticulum stress attenuated ethanol-induced exosomal miR-122 and acute liver injury in mice. Alcohol Alcohol. (2019) 54:465–71. doi: 10.1093/alcalc/agz058
206. Fabregat I, Moreno-Càceres J, Sánchez A, Dooley S, Dewidar B, Giannelli G, et al. TGF-β signalling and liver disease. FEBS J. (2016) 283:2219–32. doi: 10.1111/febs.13665
207. Yang L, Roh YS, Song J, Zhang B, Liu C, Loomba R, et al. Transforming growth factor beta signaling in hepatocytes participates in steatohepatitis through regulation of cell death and lipid metabolism in mice. Hepatology. (2014) 59:483–95. doi: 10.1002/hep.26698
208. Ottaviani S, Stebbing J, Frampton AE, Zagorac S, Krell J, de Giorgio A, et al. TGF-β induces miR-100 and miR-125b but blocks let-7a through LIN28B controlling PDAC progression. Nat Commun. (2018) 9:1845. doi: 10.1038/s41467-018-03962-x
209. McCommis KS, Hodges WT, Brunt EM, Nalbantoglu I, McDonald WG, Holley C, et al. Targeting the mitochondrial pyruvate carrier attenuates fibrosis in a mouse model of nonalcoholic steatohepatitis. Hepatology. (2017) 65:1543–56. doi: 10.1002/hep.29025
210. Park SW. Intestinal and hepatic niemann-pick c1-like 1. Diabetes Metab J. (2013) 37:240–8. doi: 10.4093/dmj.2013.37.4.240
211. van Heek M, Farley C, Compton DS, Hoos L, Davis HR. Ezetimibe selectively inhibits intestinal cholesterol absorption in rodents in the presence and absence of exocrine pancreatic function. Br J Pharmacol. (2001) 134:409–17. doi: 10.1038/sj.bjp.0704260
212. Sudhop T, von Bergmann K. Cholesterol absorption inhibitors for the treatment of hypercholesterolaemia. Drugs. (2002) 62:2333–47. doi: 10.2165/00003495-200262160-00002
213. Park H, Shima T, Yamaguchi K, Mitsuyoshi H, Minami M, Yasui K, et al. Efficacy of long-term ezetimibe therapy in patients with nonalcoholic fatty liver disease. J Gastroenterol. (2011) 46:101–7. doi: 10.1007/s00535-010-0291-8
214. Yoneda M, Fujita K, Nozaki Y, Endo H, Takahashi H, Hosono K, et al. Efficacy of ezetimibe for the treatment of non-alcoholic steatohepatitis: An open-label, pilot study. Hepatol Res. (2010) 40:566–73. doi: 10.1111/j.1872-034X.2010.00644.x
215. Yamamura T, Ohsaki Y, Suzuki M, Shinohara Y, Tatematsu T, Cheng J, et al. Inhibition of Niemann-Pick-type C1-like1 by ezetimibe activates autophagy in human hepatocytes and reduces mutant α1-antitrypsin Z deposition. Hepatology. (2014) 59:1591–9. doi: 10.1002/hep.26930
216. Kim SH, Kim G, Han DH, Lee M, Kim I, Kim B, et al. Ezetimibe ameliorates steatohepatitis via AMP activated protein kinase-TFEB-mediated activation of autophagy and NLRP3 inflammasome inhibition. Autophagy. (2017) 13:1767–81. doi: 10.1080/15548627.2017.1356977
217. Luan X, Sansanaphongpricha K, Myers I, Chen H, Yuan H, Sun D. Engineering exosomes as refined biological nanoplatforms for drug delivery. Acta Pharmacol Sin. (2017) 38:754–63. doi: 10.1038/aps.2017.12
218. Vader P, Mol EA, Pasterkamp G, Schiffelers RM. Extracellular vesicles for drug delivery. Adv Drug Deliv Rev. (2016) 106:148–56. doi: 10.1016/j.addr.2016.02.006
219. Malhotra H, Sheokand N, Kumar S, Chauhan AS, Kumar M, Jakhar P, et al. Exosomes: tunable nano vehicles for macromolecular delivery of transferrin and lactoferrin to specific intracellular compartment. J Biomed Nanotechnol. (2016) 12:1101–14. doi: 10.1166/jbn.2016.2229
220. Wiklander OP, Nordin JZ, O'Loughlin A, Gustafsson Y, Corso G, Mäger I, et al. Extracellular vesicle in vivo biodistribution is determined by cell source, route of administration and targeting. J Extracell Vesicles. (2015) 4:26316. doi: 10.3402/jev.v4.26316
221. Hood JL. Post isolation modification of exosomes for nanomedicine applications. Nanomedicine. (2016) 11:1745–56. doi: 10.2217/nnm-2016-0102
222. Goh WJ, Zou S, Ong WY, Torta F, Alexandra AF, Schiffelers RM, et al. Bioinspired cell-derived nanovesicles versus exosomes as drug delivery systems: a cost-effective alternative. Sci Rep. (2017) 7:14322. doi: 10.1038/s41598-017-14725-x
223. Marcus ME, Leonard JN. FedExosomes: engineering therapeutic biological nanoparticles that truly deliver. Pharmaceuticals. (2013) 6:659–80. doi: 10.3390/ph6050659
224. Zhang L, Zhang S, Yao J, Lowery FJ, Zhang Q, Huang WC, et al. Microenvironment-induced PTEN loss by exosomal microRNA primes brain metastasis outgrowth. Nature. (2015) 527:100–4. doi: 10.1038/nature15376
225. Deng ZB, Poliakov A, Hardy RW, Clements R, Liu C, Liu Y, et al. Adipose tissue exosome-like vesicles mediate activation of macrophage-induced insulin resistance. Diabetes. (2009) 58:2498–505. doi: 10.2337/db09-0216
226. Xue J, Yang J, Luo M, Cho WC, Liu X. MicroRNA-targeted therapeutics for lung cancer treatment. Expert Opin Drug Discov. (2017) 12:141–57. doi: 10.1080/17460441.2017.1263298
227. Pretti MAM, Bernardes SS, da Cruz JGV, Boroni M, Possik PA. Extracellular vesicle-mediated crosstalk between melanoma and the immune system: Impact on tumor progression and therapy response. J Leukoc Biol. (2020) 108:1101–15. doi: 10.1002/JLB.3MR0320-644R
228. Jin JO, Kim G, Hwang J, Han KH, Kwak M, Lee PCW. Nucleic acid nanotechnology for cancer treatment. Biochim Biophys Acta Rev Cancer. (2020) 1874:188377. doi: 10.1016/j.bbcan.2020.188377
229. van Zandwijk N, Pavlakis N, Kao SC, Linton A, Boyer MJ, Clarke S, et al. Safety and activity of microRNA-loaded minicells in patients with recurrent malignant pleural mesothelioma: a first-in-man, phase 1, open-label, dose-escalation study. Lancet Oncol. (2017) 18:1386–96. doi: 10.1016/S1470-2045(17)30621-6
230. Yang C, Zhang M, Merlin D. Advances in plant-derived edible nanoparticle-based lipid nano-drug delivery systems as therapeutic nanomedicines. J Mater Chem B. (2018) 6:1312–21. doi: 10.1039/C7TB03207B
231. Zhang M, Viennois E, Xu C, Merlin D. Plant derived edible nanoparticles as a new therapeutic approach against diseases. Tissue Barriers. (2016) 4:e1134415. doi: 10.1080/21688370.2015.1134415
232. Ju S, Mu J, Dokland T, Zhuang X, Wang Q, Jiang H, et al. Grape exosome-like nanoparticles induce intestinal stem cells and protect mice from DSS-induced colitis. Mol Ther. (2013) 21:1345–57. doi: 10.1038/mt.2013.64
233. Wang B, Zhuang X, Deng ZB, Jiang H, Mu J, Wang Q, et al. Targeted drug delivery to intestinal macrophages by bioactive nanovesicles released from grapefruit. Mol Ther. (2014) 22:522–34. doi: 10.1038/mt.2013.190
234. Wang Q, Ren Y, Mu J, Egilmez NK, Zhuang X, Deng Z, et al. Grapefruit-derived nanovectors use an activated leukocyte trafficking pathway to deliver therapeutic agents to inflammatory tumor sites. Cancer Res. (2015) 75:2520–9. doi: 10.1158/0008-5472.CAN-14-3095
235. Brahmbhatt M, Gundala SR, Asif G, Shamsi SA, Aneja R. Ginger phytochemicals exhibit synergy to inhibit prostate cancer cell proliferation. Nutr Cancer. (2013) 65:263–72. doi: 10.1080/01635581.2013.749925
236. Raimondo S, Naselli F, Fontana S, Monteleone F, Lo Dico A, Saieva L, et al. Citrus limon-derived nanovesicles inhibit cancer cell proliferation and suppress CML xenograft growth by inducing TRAIL-mediated cell death. Oncotarget. (2015) 6:19514–27. doi: 10.18632/oncotarget.4004
237. Deng Z, Rong Y, Teng Y, Mu J, Zhuang X, Tseng M, et al. Broccoli-derived nanoparticle inhibits mouse colitis by activating dendritic cell AMP-activated protein kinase. Mol Ther. (2017) 25:1641–54. doi: 10.1016/j.ymthe.2017.01.025
238. Mu J, Zhuang X, Wang Q, Jiang H, Deng ZB, Wang B, et al. Interspecies communication between plant and mouse gut host cells through edible plant derived exosome-like nanoparticles. Mol Nutr Food Res. (2014) 58:1561–73. doi: 10.1002/mnfr.201300729
239. Zhao Z, Yu S, Li M, Gui X, Li P. Isolation of exosome-like nanoparticles and analysis of MicroRNAs derived from coconut water based on small RNA high-throughput sequencing. J Agric Food Chem. (2018) 66:2749–57. doi: 10.1021/acs.jafc.7b05614
240. Yu S, Zhao Z, Xu X, Li M, Li P. Characterization of three different types of extracellular vesicles and their impact on bacterial growth. Food Chem. (2019) 272:372–8. doi: 10.1016/j.foodchem.2018.08.059
241. Fujita D, Arai T, Komori H, Shirasaki Y, Wakayama T, Nakanishi T, et al. Apple-derived nanoparticles modulate expression of organic-anion-transporting polypeptide (OATP) 2B1 in Caco-2 cells. Mol Pharm. (2018) 15:5772–80. doi: 10.1021/acs.molpharmaceut.8b00921
242. An Q, Hückelhoven R, Kogel KH, van Bel AJ. Multivesicular bodies participate in a cell wall-associated defence response in barley leaves attacked by the pathogenic powdery mildew fungus. Cell Microbiol. (2006) 8:1009–19. doi: 10.1111/j.1462-5822.2006.00683.x
243. An Q, van Bel AJ, Hückelhoven R. Do plant cells secrete exosomes derived from multivesicular bodies? Plant Signal Behav. (2007) 2:4–7. doi: 10.4161/psb.2.1.3596
244. Zhang M, Viennois E, Prasad M, Zhang Y, Wang L, Zhang Z, et al. Edible ginger-derived nanoparticles: A novel therapeutic approach for the prevention and treatment of inflammatory bowel disease and colitis-associated cancer. Biomaterials. (2016) 101:321–40. doi: 10.1016/j.biomaterials.2016.06.018
245. Zhang M, Xiao B, Wang H, Han MK, Zhang Z, Viennois E, et al. Edible ginger-derived nano-lipids loaded with doxorubicin as a novel drug-delivery approach for colon cancer therapy. Mol Ther. (2016) 24:1783–96. doi: 10.1038/mt.2016.159
246. Zhuge Q, Zhang Y, Liu B, Wu M. Blueberry polyphenols play a preventive effect on alcoholic fatty liver disease C57BL/6 J mice by promoting autophagy to accelerate lipolysis to eliminate excessive TG accumulation in hepatocytes. Ann Palliat Med. (2020) 9:1045–54. doi: 10.21037/apm.2020.03.38
247. Khalid S, Barfoot KL, May G, Lamport DJ, Reynolds SA, Williams CM. Effects of acute blueberry flavonoids on mood in children and young adults. Nutrients. (2017) 9:20158. doi: 10.3390/nu9020158
248. Shi M, Loftus H, McAinch AJ, Su XQ. Blueberry as a source of bioactive compounds for the treatment of obesity, type 2 diabetes and chronic inflammation. J Funct Foods. (2017) 30:16–29. doi: 10.1016/j.jff.2016.12.036
249. Wu T, Gao Y, Guo X, Zhang M, Gong L. Blackberry and blueberry anthocyanin supplementation counteract high-fat-diet-induced obesity by alleviating oxidative stress and inflammation and accelerating energy expenditure. Oxid Med Cell Longev. (2018) 2018:4051232. doi: 10.1155/2018/4051232
250. Xu N, Meng H, Liu T, Feng Y, Qi Y, Zhang D, et al. Blueberry phenolics reduce gastrointestinal infection of patients with cerebral venous thrombosis by improving depressant-induced autoimmune disorder via miR-155-mediated brain-derived neurotrophic factor. Front Pharmacol. (2017) 8:853. doi: 10.3389/fphar.2017.00853
251. Zepeda A, Aguayo LG, Fuentealba J, Figueroa C, Acevedo A, Salgado P, et al. Blueberry extracts protect testis from hypobaric hypoxia induced oxidative stress in rats. Oxid Med Cell Longev. (2012) 2012:975870. doi: 10.1155/2012/975870
252. Zhao WJ, Bian YP, Wang QH, Yin F, Yin L, Zhang YL, et al. Blueberry-derived exosomes-like nanoparticles ameliorate nonalcoholic fatty liver disease by attenuating mitochondrial oxidative stress. Acta Pharmacol Sin. (2022) 43:645–58. doi: 10.1038/s41401-021-00681-w
253. Mortezaee K. Human hepatocellular carcinoma: protection by melatonin. J Cell Physiol. (2018) 233:6486–508. doi: 10.1002/jcp.26586
254. Xu P, Wang J, Hong F, Wang S, Jin X, Xue T, et al. Melatonin prevents obesity through modulation of gut microbiota in mice. J Pineal Res. (2017) 62:12399. doi: 10.1111/jpi.12399
255. Liu Z, Gan L, Xu Y, Luo D, Ren Q, Wu S, et al. Melatonin alleviates inflammasome-induced pyroptosis through inhibiting NF-κB/GSDMD signal in mice adipose tissue. J Pineal Res. (2017) 63:12414. doi: 10.1111/jpi.12414
256. Fernández Vázquez G, Reiter RJ, Agil A. Melatonin increases brown adipose tissue mass and function in Zücker diabetic fatty rats: implications for obesity control. J Pineal Res. (2018) 64:e12472. doi: 10.1111/jpi.12472
257. Rong B, Feng R, Liu C, Wu Q, Sun C. Reduced delivery of epididymal adipocyte-derived exosomal resistin is essential for melatonin ameliorating hepatic steatosis in mice. J Pineal Res. (2019) 66:e12561. doi: 10.1111/jpi.12561
258. Sun L, Xu R, Sun X, Duan Y, Han Y, Zhao Y, et al. Safety evaluation of exosomes derived from human umbilical cord mesenchymal stromal cell. Cytotherapy. (2016) 18:413–22. doi: 10.1016/j.jcyt.2015.11.018
259. Ding J, Wang J, Chen J. Exosomes as therapeutic vehicles in liver diseases. Ann Transl Med. (2021) 9:735. doi: 10.21037/atm-20-5422
260. Pritchard CC, Kroh E, Wood B, Arroyo JD, Dougherty KJ, Miyaji MM, et al. Blood cell origin of circulating microRNAs: a cautionary note for cancer biomarker studies. Cancer Prev Res. (2012) 5:492–7. doi: 10.1158/1940-6207.CAPR-11-0370
261. Wisgrill L, Lamm C, Hartmann J, Preißing F, Dragosits K, Bee A, et al. Peripheral blood microvesicles secretion is influenced by storage time, temperature, and anticoagulants. Cytometry A. (2016) 89:663–72. doi: 10.1002/cyto.a.22892
Keywords: NASH, non-alcoholic fatty liver, exosome, NAFLD, extracellular vesicle (EV), microRNA
Citation: Tamimi A, Javid M, Sedighi-Pirsaraei N and Mirdamadi A (2024) Exosome prospects in the diagnosis and treatment of non-alcoholic fatty liver disease. Front. Med. 11:1420281. doi: 10.3389/fmed.2024.1420281
Received: 19 April 2024; Accepted: 16 July 2024;
Published: 31 July 2024.
Edited by:
Nirajan Shrestha, Massachusetts General Hospital and Harvard Medical School, United StatesReviewed by:
Hao Du, UCONN Health, United StatesCopyright © 2024 Tamimi, Javid, Sedighi-Pirsaraei and Mirdamadi. This is an open-access article distributed under the terms of the Creative Commons Attribution License (CC BY). The use, distribution or reproduction in other forums is permitted, provided the original author(s) and the copyright owner(s) are credited and that the original publication in this journal is cited, in accordance with accepted academic practice. No use, distribution or reproduction is permitted which does not comply with these terms.
*Correspondence: Amirhossein Tamimi, YW1pcmhvc3NlaW50YW1pbWk5OTdAZ21haWwuY29t
†ORCID: Amirhossein Tamimi orcid.org/0000-0002-0228-3409
Mona Javid orcid.org/0000-0003-1361-0812
Nasrin Sedighi-Pirsaraei orcid.org/0000-0002-4595-5973
Arian Mirdamadi orcid.org/0000-0002-6608-8477
Disclaimer: All claims expressed in this article are solely those of the authors and do not necessarily represent those of their affiliated organizations, or those of the publisher, the editors and the reviewers. Any product that may be evaluated in this article or claim that may be made by its manufacturer is not guaranteed or endorsed by the publisher.
Research integrity at Frontiers
Learn more about the work of our research integrity team to safeguard the quality of each article we publish.