- Renal Medicine, Kolling Institute of Medical Research, Sydney Medical School, University of Sydney, Royal North Shore Hospital, St Leonards, NSW, Australia
Chronic kidney disease (CKD) is rising in global prevalence and has become a worldwide public health problem, with adverse outcomes of kidney failure, cardiovascular disease, and premature death. However, current treatments are limited to slowing rather than reversing disease progression or restoring functional nephrons. Hence, innovative strategies aimed at kidney tissue recovery hold promise for CKD therapy. Mesenchymal stem cells (MSCs) are commonly used for regenerative therapy due to their potential for proliferation, differentiation, and immunomodulation. Accumulating evidence suggests that the therapeutic effects of MSCs are largely mediated by paracrine secretion of extracellular vesicles (EVs), predominantly exosomes. MSC-derived exosomes (MSC-Exos) replicate the functions of their originator MSCs via delivery of various genetic and protein cargos to target cells. More recently, MSC-Exos have also been utilized as natural carriers for targeted drug delivery. Therapeutics can be effectively incorporated into exosomes and then delivered to diseased tissue. Thus, MSC-Exos have emerged as a promising cell-free therapy in CKD. In this paper, we describe the characteristics of MSC-Exos and summarize their therapeutic efficacy in preclinical animal models of CKD. We also discuss the potential challenges and strategies in the use of MSC-Exos-based therapies for CKD in the future.
Introduction
Chronic kidney disease (CKD) is a widespread public health problem, with adverse outcomes of kidney failure, cardiovascular disease, and premature death. CKD is more common than is widely known, affecting approximately 10% of the population worldwide (1). Although the causes of CKD may vary, diabetes and hypertension are still the leading causes (1). Irrespective of the multifactorial etiologies of the initial renal injury, progressive renal fibrosis is common to all forms of CKD (2). Although there have been recent advances in therapeutic strategies for CKD, a significant treatment gap remains. Despite targeted control of diabetes, blood pressure, hyperlipidemia and proteinuria, a large proportion of patients with CKD develop end stage kidney disease (ESKD). Kidney transplantation and dialysis are the only options for the management of ESKD, which results in a significant personal and societal burden (3). Hence innovative therapeutic strategies are urgently needed.
With potent self-renewal capabilities and great potential for differentiation and proliferation, stem cell (SC) therapy has emerged as an option for the preservation of renal function and structural repair in kidney diseases (4). Mounting evidence suggests that SCs exert therapeutic effects mostly by differentiation into tissue-specific cells to replace damaged tissue (5, 6). Amongst different types of SCs, the application of mesenchymal stem cells (MSCs) in treating kidney diseases is widely studied and has been shown to be advantageous over the application of other SCs (7). MSCs are multipotent SCs that differentiate into cells of mesenchymal cell lineages and exert important functions in tissue regeneration and repair by virtue of their wide differentiation capacity as well as anti-inflammatory and immunosuppressive properties (8–10). They can be obtained from virtually any type of tissue (tissue-derived MSCs) including bone marrow (BM), umbilical cord (UC), adipose tissue, dental pulp, amniotic fluid, placenta, Wharton’s jelly (WJ), and organs including kidney, liver, spleen, pancreas, brain, lung, and thymus (11–15). MSCs can also be acquired from cells such as induced pluripotent stem cells (iPSCs)(16). Pluripotent stem cells (PSCs) are cells characterized by the capacity to self-renew and to differentiate into one of the three primary germ cell layers of the early embryo and therefore into specialized cell types (17). There are two types of PSC: embryonic stem cells (ESCs) and iPSCs (18). In 2007, it was reported by Shinya Yamanaka that induced PSCs (iPSCs) could be derived from reprogrammed adult human cells by introducing only a few genes (19). This discovery revolutionized the understanding of cell development and Shinya Yamanaka was thus awarded the Nobel Prize in Physiology or Medicine 2012.
To define MSCs with different origins, minimal criteria required include plastic adherence in standard culture conditions, expression of cluster of differentiation (CD) 105, CD73, and CD90, lack of expression of CD45, CD34, CD14 or CD11b, CD79a or CD19, and human leukocyte antigen-DR isotype (HLA-DR) surface molecules, and differentiation into osteoblasts, adipocytes, and chondroblasts in vitro (20). As immunoprivileged cells, MSCs rapidly home to injured kidneys and act through paracrine pathways to promote repair (21). They are reported to prevent and/or reverse kidney fibrosis and improve renal function in both experimental models and human patients (22, 23).
However, there are disadvantages in using cell based MSC therapy. These include the difficulty in maintaining a consistent source of cells with a stable phenotype (24) and in delivering large cells intravenously associated with a hazard of pulmonary microvasculature entrapment (25). Furthermore, MSCs have a risk of tumor formation through vascularization, immune regulation, and facilitating tumor interstitial remodeling (26). These disadvantages have restricted their clinical use. Thus, alternative MSC-based and complication-free therapeutic strategies are needed.
Numerous lines of evidence have supported that the therapeutic potential of MSCs is mediated by the secretion of soluble paracrine factors-extracellular vesicles (EVs) including apoptotic bodies (1–5 mm), microvesicles (MVs, 0.1–1 μm), and exosomes (30–150 nm)(27–29). Both MVs and apoptotic bodies are formed by direct budding from the plasma membrane. However, exosomes are produced after the fusion of multivesicular bodies (MVB), which are endocytic organelles containing many luminal vesicles, with the plasma membrane and are characterized by surface expression of CD9, CD63, and CD81 (30, 31). Very recent preclinical studies have identified exosomes as a dominant player in the MSC-mediated repair process of injured tissues (Figure 1). MSC-derived exosomes (MSC-Exos) coordinate intercellular communication and tissue repair through transfer of proteins, RNA, DNA and lipids between cells, which is likely to constitute a novel mode of intercellular communication (32, 33). In this review, we will summarize recent advances regarding the therapeutic application of MSC-Exos in preclinical studies in various experimental CKD models including diabetic kidney disease (DKD), hypertensive CKD and kidney fibrosis, aiming to provide novel insights to the treatment of CKD.
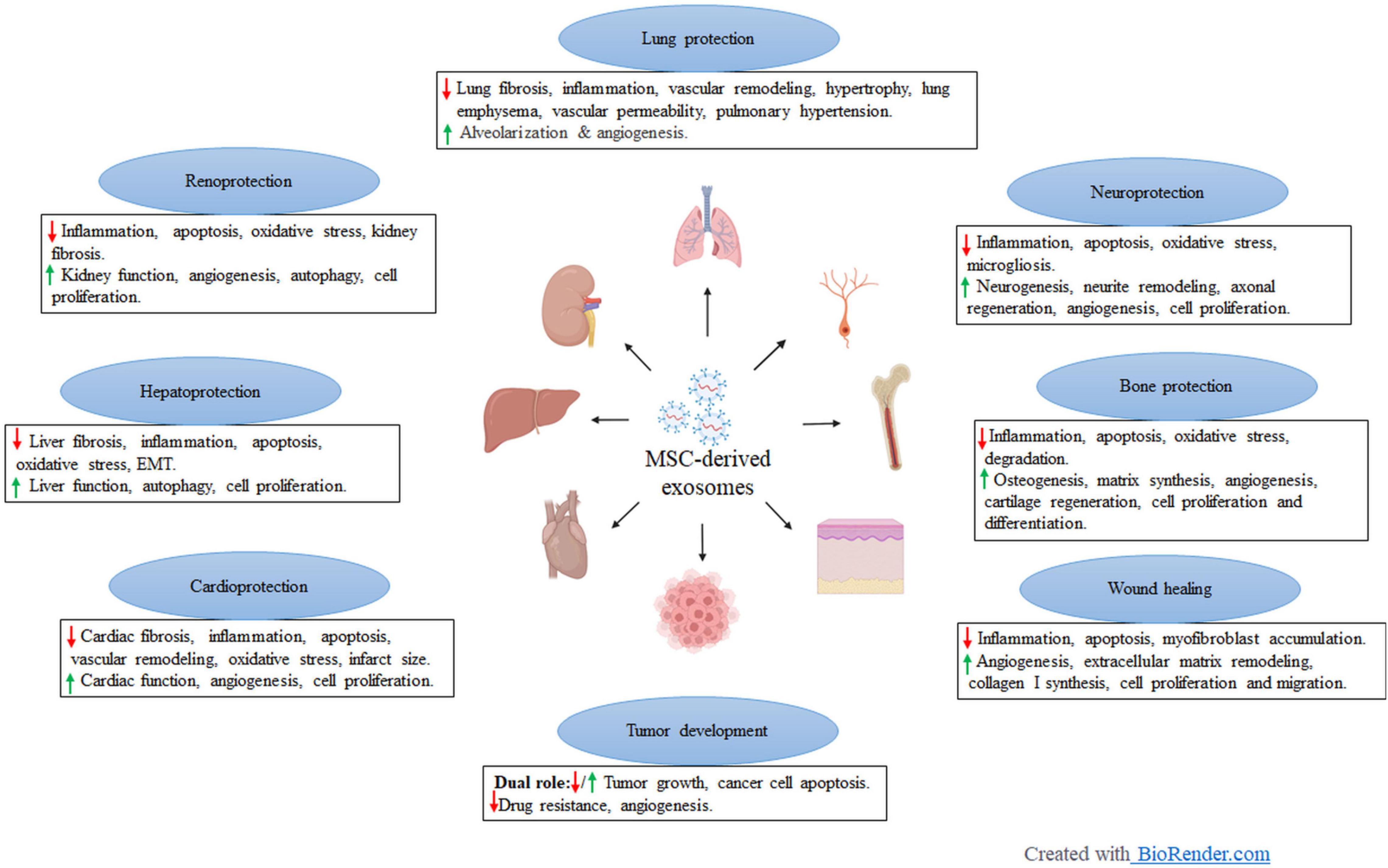
Figure 1. Preclinical application of mesenchymal stem cell-derived exosomes in different disease models. Created with BioRender.com.
Exosomes
Isolation, Identification, and Characterization of Natural Exosomes
Exosomes are small heterogeneous phospholipid-bilayer EVs that can be secreted by almost all type of cells via invagination of the late endosomal membrane (34). Generally, exosomes can now be isolated from conditioned cell culture media or body fluids by differential ultracentrifugation, precipitation, size exclusion chromatography (SEC), filtration, immunoaffinity capture, commercial kits, or microfluidic technologies (35). Each approach has its advantages and disadvantages and there is lack of consensus on a gold standard of isolation. After purification, transmission electron microscopy (TEM) can be used for exosome validation (35). Exosomes contain a wide variety of cytoplasmic or membrane proteins (receptors, enzymes, transcription factors, and ECM components), nucleic acids (mitochondrial DNA, single-stranded DNA, double-stranded DNA, mRNA, and non-coding RNA) and lipids (36, 37). Of note, most exosomes have an evolutionarily conserved set of proteins including tetraspanins (CD81, CD63, and CD9), heat-shock proteins (HSP60, HSP70, and HSP90), ALIX and tumor susceptibility gene 101 (TSG101), which are used as biomarkers to identify exosomes (34).
Naturally, exosomes exhibit the characteristics of their parental cells. Thus, exosomes have been regarded as mini version of the originator cells (34). Emerging evidence has suggested that exosomes are biologically active vesicles regulating physiological and pathological pathways through delivery of functional cargos of proteins, nucleic acids and lipids (34). The cargos of exosomes vary according to the identity and physiological condition of the source cells and the extracellular environment and can be selectively taken up by neighboring or distant cells after the fusion of exosomes to the plasma membrane of recipient cells (38). Once internalized, exosomes fuse with the endosome membrane, followed by the horizontal transfer of their content to the cytoplasm of target cells and modification of their biological activities (39).
Engineered Exosomes for Drug Delivery
Recently, natural exosomes have also been engineered as drug carriers to specifically deliver a variety of bioactive molecules, such as short interfering-RNA (siRNA), antagomirs, recombinant proteins, and anti-inflammatory drugs due to their low toxicity, long-term stability, nanoscale size, cargo loading capacity, editable surface and tissue homing capability (40) (Figure 2). The simplest way for cargo loading is to incubate desired cargos with exosome-secreting cells or exosomes to allow diffusion of cargos into exosomes via a concentration gradient (41). Some other strategies include transfection, through which specific plasmids are transduced into cells to ectopically express desired biomolecules in exosomes. In addition to physical treatments (sonication, electroporation, extrusion, freeze-thaw, surfactant treatment and dialysis), in situ synthesis have also been applied to generate reconstituted exosomes (42). It is now recognized that natural exosomes spread via free diffusion and are then randomly internalized into recipient cells (43). To achieve specific targeted delivery of reconstituted exosomes, methodologies based on ligand-receptor binding, pH gradient/surface charge, and magnetism have been applied (44).
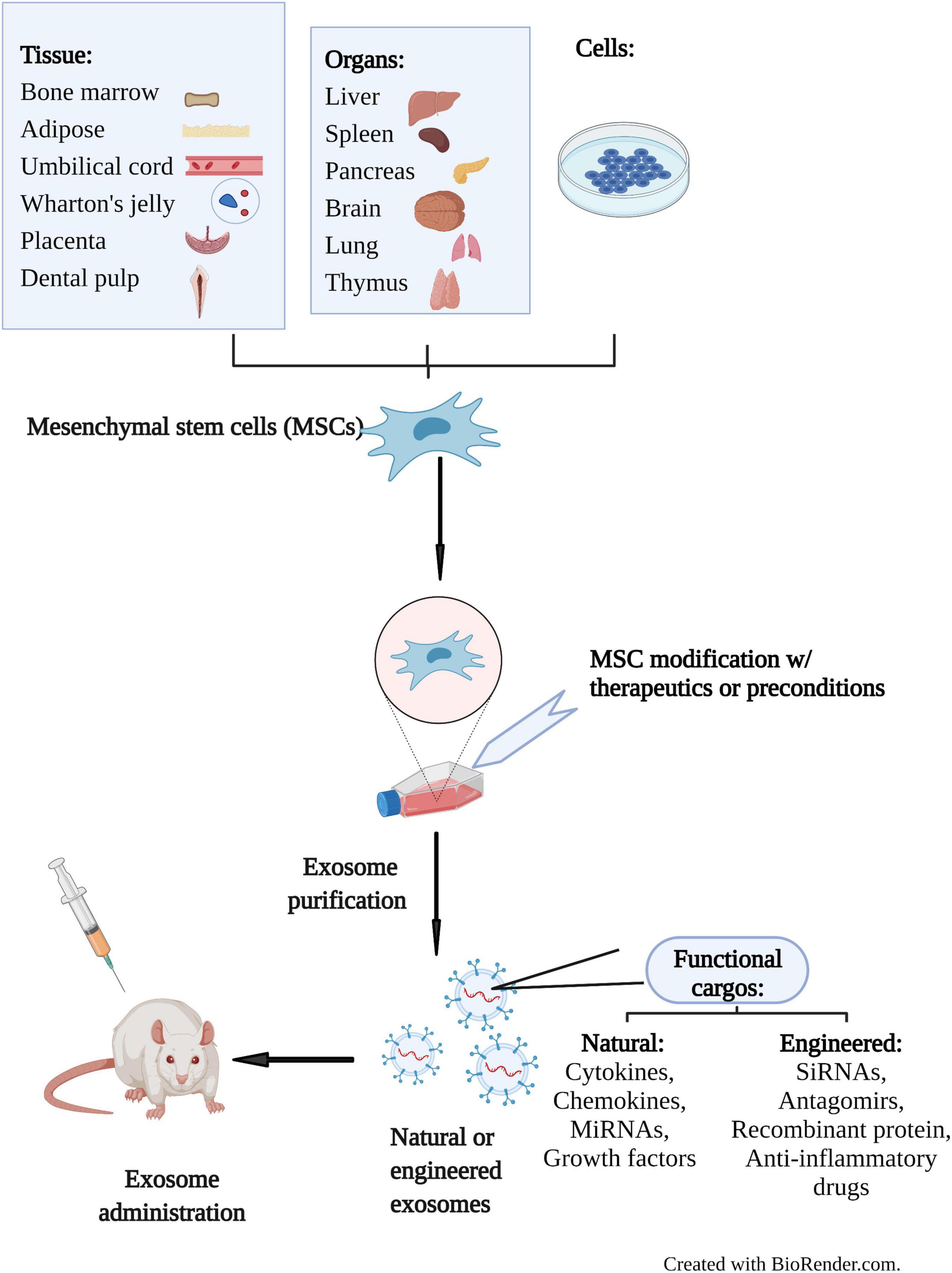
Figure 2. Schematic diagram of therapeutic application of MSC-Exos in preclinical studies. MSCs can be isolated from various sources including tissues, organs, and cells. Exosomes secreted by MSCs can be engineered at the cellular or exosomal level. Natural MSC-Exos exhibit the characteristics of their parental cells through transfer of cargos such as cytokines, chemokines, miRNAs and growth factors. Engineered exosomes can also deliver bioactive siRNAs, antagomirs, recombinant proteins and anti-inflammatory drugs specifically. Administration of MSC-Exos to animal models are used to investigate their therapeutic potential in preclinical studies. Created with BioRender.com.
Compared with synthetic drug carriers, exosomes have several advantages. They can be obtained from patients’ tissues or body fluids with excellent host bio-distribution and biocompatibility, which minimize clearance rate and toxicity (45). For long-distance cell to cell communication, exosomes can also enter the blood and pass through additional biological barriers such as blood-brain barrier to achieve delivery throughout the body (46). Additionally, exosomes can be administrated via different routes (intranasally, intravenously, intraperitoneally, and intracranially), confirming exosome-based drug delivery is highly flexible (40).
Collectively, the utilization of exosomes in therapy has more benefits than their counterpart whole cells. MSCs have shown regenerative potential in the attenuation of kidney injury. Likewise, MSC-Exos represent attractive strategies for the treatment of various kidney diseases including CKD.
Biochemistry and Functions of MSC-Exos
Mesenchymal stem cell-derived exosomes not only have the advantages of exosomes, but also replicate the biological characteristics of MSCs through transfer of functional cargos, mainly microRNAs (miRNAs) and proteins. MiRNAs are short non-coding RNAs that regulate various physiological cellular processes such as cell death, differentiation, proliferation, metabolism, and pathophysiology of many diseases via regulation of target genes (47, 48). To date, more than 150 miRNAs and over 900 proteins have been identified in cargos of MSC-Exos (49, 50), resulting in the alteration of a variety of activities in target cells via different pathways. The tissue-repairing activities of MSC-Exos involve promoting cell proliferation, dedifferentiation and angiogenesis, whilst simultaneously dampening apoptosis and oxidative stress (51, 52). MiRNA cargos such as miRNA-10a, miRNA-486 were regarded as pro-regenerative miRNAs due to their capability to promote cell proliferation (53, 54) while miRNA-199a-3p was found to downregulate apoptosis-related genes and thereby suppress apoptosis (55, 56). Protein cargos like extracellular matrix metalloproteinase inducer (EMMPRIN) and metalloproteinase-9 (MMP-9) have been reported to stimulate angiogenesis (57, 58). Furthermore, MSC-Exos mitigate inflammatory responses by minimizing infiltration of immune cells such as macrophages, T cells, and NK cells (51). For instance, miRNA-155 (59), miRNA-146a (60), some cytokines such as interleukin-6 (IL-6), IL-10, and growth factor (GFs) hepatocyte growth factor (HGF), contribute to MSC-Exos-mediated immunoregulation (61). Nevertheless, exosomes of different MSC origins contain different biomolecules and thus exhibit heterogeneous characteristics (62, 63).
A comparative proteomic-based analysis through mass spectrometry on the secretome of MSCs revealed that BM-derived MSC (BM-MSCs), adipose-derived MSCs (AD-MSCs), and UC-derived MSC (UC-MSCs) differed in their secretion of anti-oxidative stress or anti-apoptosis molecules involved in central nervous system (CNS) injury (62). Another study by Hoang et al identified differential release of GFs responsible for wound healing by MSC-Exos from BM, adipose and UC (64). Notably, BM-MSC-derived exosomes (BM-MSC-Exos) was superior in inducing primary dermal fibroblasts (64). BM-MSC-Exos have enhanced regeneration capacity by virtue of induction of angiogenesis; AD-MSC-derived exosomes (AD-MSC-Exos) function as major immunomodulators and UC-MSC-derived exosomes (UC-MSC-Exos) mostly participate in tissue repair (63, 65). In spite of the heterogeneity, growing evidence demonstrate that MSC-Exos offer a novel cell-free therapeutic opportunity, as an alternative to MSCs, for treatment of various pathological conditions including neurological disorders, liver or lung damage and acute or chronic kidney injury (50, 66).
Therapeutic Potential of MSC-Exos for CKD
Mesenchymal stem cells have exhibited promising efficacy in alleviating kidney injury in experimental CKD (67, 68). MSC-Exos possess repair functions similar to MSCs and have been widely used in CKD particularly DKD and kidney fibrosis to overcome the limitations of MSCs. Although the functions of MSC-Exos may vary depending on the cellular source of MSCs, they are in general therapeutic. Among all preclinical studies, heterogenous experiment settings including different doses and schedules, various routes of administration (tail infusion, organ perfusion, or the direct application in the kidney) and distinct CKD animal models were applied.
MSC-Exos for DKD
Diabetic kidney disease, a microvascular complication of diabetes mellitus (DM), is the most common form of CKD, and is likely to increase in epidemic proportions globally (69). Diabetic patients with kidney disease have a greater mortality risk than those without kidney disease. With the prevalence of DM in the global adult population expected to increase from 8.8% in 2015 to 10.4% in 2040, the impact of DKD is expected to be an increasingly prominent global health issue (69). In DKD, microalbuminuria is an early, although not invariable, clinical manifestation and portends an increased risk for progressive kidney damage. Hyperglycemia activates various inflammatory pathways through direct mechanisms to induce reactive oxygen species, oxidative stress, renin-angiotensin-aldosterone system (RAAS) activation, profibrotic cytokines, including transforming growth factor-beta (TGF-β), and advanced glycation end-products (70). This leads collectively to apoptosis, podocyte and tubular damage and associated albuminuria. The increased matrix protein production and decreased protein degradation leads to deposition of ECM proteins, including collagens and fibronectin (FN) in the glomerular mesangium and tubulointerstitium, resulting in progressive fibrosis (70, 71). To investigate the therapeutic effects of MSC-Exos in DKD, STZ-DKD in vivo model (mice or rats) and in vitro high glucose (HG)-treated cell lines of podocyte, tubular epithelial cells (TECs) and glomerular endothelial cells were commonly used. The efficacy of MSC-Exos in treating rodent DKD is summarized in Table 1.
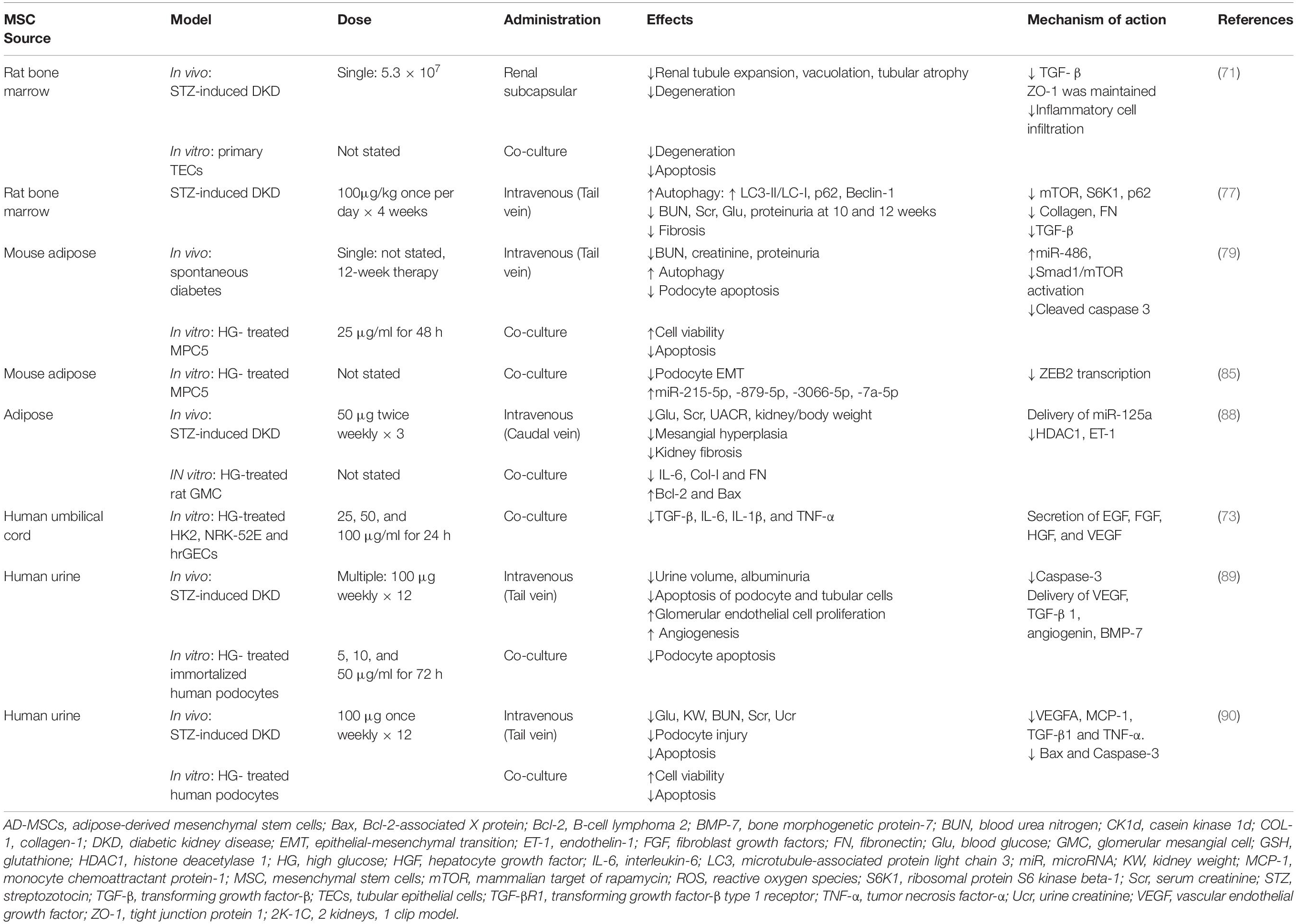
Table 1. Summary of therapeutic effects of MSC-Exos from various sources in preclinical models of DKD.
Involvement of MSC-Exos From Tissues in DKD
The various cargos including GFs and therapeutic miRNAs delivered by MSC-Exos exert significant effects on restoring renal function, enhancing autophagy, attenuating podocyte injury and mitigating kidney fibrosis. MSCs originate from a wide range of sources and were first discovered from BM (72). In the study by Nagaishi et al., exosomes derived from BM-MSCs were delivered to the subcapsular region of the kidney in STZ-induced diabetic rats in vivo (73). These exosomes ameliorated kidney injury, inflammatory cell infiltration and TGF-β production as well as maintained the expression of tight junction protein-1 (ZO-1). Consistently, BM-MSC-Exos also suppressed apoptosis and degeneration of primary TECs from STZ-induced diabetic rats in vitro (73). UC, a conduit between the placenta and the developing embryo, is another popular source of MSCs due to the easy, safe and non-invasive way of collection, low immunogenicity, and high paracrine potential (74). In vitro, UC-MSC-Exos dramatically downregulated HG-induced pro-inflammatory cytokines including TGF-β, IL-6, IL-1β, and TNF-α in both renal TEC cell lines (NRK-52E, HK2) and human renal glomerular endothelial cell line (hrGECs) via their horizontal transfer of large amounts of GFs including epidermal growth factor (EGF), fibroblast growth factor (FGF), HGF and vascular endothelial growth factor (VEGF) (75).
Autophagy is an intracellular lysosome-dependent degradative process, which maintains cellular homeostasis and integrity through removing damaged macromolecules and organelles (76). Additionally, autophagy is critical to provide energy and molecular building blocks by recycling macromolecules in response to nutrient and environmental stress (77). Impairment of autophagy in renal cells in patients with DM contributes to the progression of DKD via mammalian target of rapamycin (mTOR) pathway activation (78). Studies have proven that MSC-Exos can effectively restore autophagy activity by decreasing mTOR. Rats administered BM-MSC-Exos revealed significantly enhanced autophagy markers microtubule-associated protein light chain 3 (LC3)-II/LC3-I and p62 protein expression compared to the animals with DKD (79). Consistently, fibrotic markers including TGF-β and FN were also inhibited, suggesting a potent anti-fibrotic effect of MSC-Exos. The protective impact of MSC-Exos can be blocked by autophagy inhibitors including 3-methyladenine (3-MA) and chloroquine in rats, confirming the involvement of autophagy in the MSC-Exos mediated renoprotection (79).
Adipose-derived stem cells are also MSCs obtained from adipose tissue (80). Unlike BM-MSCs, AD-MSCs can be obtained by a minimally invasive procedure and thus are also promising for tissue regeneration. AD-MSC-Exos enhanced autophagy and reduced podocyte apoptosis, leading to attenuated DKD as evidenced by reduced levels of urine protein, serum creatinine (Scr) and blood urea nitrogen (BUN) in mice. Consistently, AD-MSC-Exos reversed autophagy downregulation and suppressed podocyte apoptosis in vitro (81).
MiRNAs as Major Bioactive Cargos of MSC-Exos
MicroRNAs have been found to be packed and protected from proteases and RNAses in EVs (82, 83) and are the most abundant content in human plasma derived exosomal RNAs (84). Investigations on mechanisms by which MSC-Exos elicit their renoprotection verify that apart from proteins such as various GFs, certain miRNAs are the main contents of exosomes contributing to their regenerative potential (32). In HG-stimulated podocyte in vitro, miRNA-486 from AD-MSC-Exos inhibited Smad1 and mTOR activation, leading to increased autophagy and reduced podocyte apoptosis (81). These beneficial effects can be neutralized in the presence of miRNA-486 inhibitor, further supporting that AD-MSC-Exos promoted survival of podocytes through miRNA-dependent mechanisms.
In most CKD, the breakdown of the glomerular filtration barrier (GFB) manifests as proteinuria and is subsequently associated with loss of normal kidney function (85). Podocytes, which are specialized visceral epithelial cells, are an independent component of the GFB. They plays an essential role in maintaining the integrity of GFB (85). HG induces epithelial-mesenchymal transition (EMT) and may initiate podocyte injury, resulting in GFB destruction (86). Jin et al found that AD-MSC-Exos administration mitigated HG-induced podocyte EMT due to the restoration of miRNAs including miRNA-215-5p, miRNA-879-5p, miRNA-3066-5p, and miRNA-7a-5p (87). As miRNA-215-5p mimics abrogated HG-induced EMT in podocytes and miRNA-215-5p inhibitors counteracted the protective effect of the AD-MSC-Exos, miRNA-251-5p is regarded as a main player facilitating protection of AD-MSC-Exos on podocyte damage (87). Histone deacetylase 1 (HDAC1)/endothelin-1 (ET-1) axis upregulation was observed in DKD rats and HG-stimulated glomerular mesangial cells (GMCs). High ET-1‘expression induces insulin resistance and increases glomerular permeability, thereby promoting the progression of DKD (88, 89). A recent study suggested AD-MSC-Exos alleviated DKD through delivering miRNA-125a, which targeted the HDAC1/ET1 axis directly to block inflammation and fibrosis (90).
Involvement of Exosomes From Urine-Derived Stem Cells in DKD
Urine-derived stem cells (USCs) display classical features of MSCs. Importantly, they can be isolated from urine with a cheap and non-invasive procedure, whereas most adult SCs require invasive procedures (91). Moreover, USCs can differentiate into renal cells, therefore representing huge benefits for application in the treatment of kidney diseases (92, 93). Intravenous injections of USCs-derived exosomes (USC-Exos) alleviated albuminuria in diabetic rats through inhibiting podocytic apoptosis and increasing glomerular endothelial cell proliferation and mesangial angiogenesis in the early stage of DKD (94). The horizontal transfer of podocyte survival factor (bone morphogenetic protein-7, BMP-7) and proangiogenic factors (VEGF, TGF-β, and angiogenin) from USCs-Exos to resident cells mediated nephroprotection by USC-Exos (94). Another study on USC-Exos showed that USC-Exos delivered miRNA-16-5p to the injured kidney and mitigated renal functional impairment (decreased BUN, Scr, and Ucr) in the STZ-DKD rat model (95). The mechanism of renoprotection was attributed to the downregulation of VEGFA, monocyte chemoattracting protein-1 (MCP-1), TGF-β1, TNF-α, and apoptosis-associated protein including B-cell lymphoma-2 (Bcl-2), Bcl-2-associated X protein (Bax) and Caspase-3. In vitro, USC-Exos enriched with miRNA-16-5p also led to inhibition of VEGF and offered protection against HG-induced podocyte apoptosis (95).
MSC-Exos for Hypertensive CKD
Hypertension, a complex multifactorial disease, is also one of the leading causes of CKD due to the deleterious effects of increased blood pressure (BP) on the kidney. Chronic hypertension leads to changes in the systemic and renal macro and microvasculature, resulting in loss of renal auto-regulation, increased glomerular capillary pressure and hyperfiltration-mediated tubular injury (96). Hyperfiltration contributes to glomerular proteinuria, which promotes the release of inflammatory cytokines and GFs by GMCs and TECs (97). In addition, hypertension induces vascular stretch, endothelial dysfunction and the consequent activation of the intra-renal renin-angiotensin system (RAS), which amplifies the release of cytokines and GFs, recruitment of inflammatory cells, increased ECM production and finally progressive glomerular and tubulointerstitial fibrosis (98). Patients with diabetes commonly have hypertension due to chronic hyperglycemia-induced dysfunction of the vasculature (99).
Studies investigating the use of MSC-Exos as therapeutic agents in hypertension-associated CKD are scant. Aliotta et al found that exosomes isolated from both human and murine MSCs were effective in reversing pulmonary hypertension in a mouse model. The beneficial effects may be mediated by cargos of anti-inflammatory and anti-proliferative miRNAs (miRNAs-34a, -122, -124, and -127) that dampen angiogenesis, blunt neoplastic cell proliferation and elicit senescence of vascular smooth muscle cells (SMCs) and endothelial progenitor cells (EPCs) (100). Lindoso et al reported that multiple injections of EVs isolated from adipose-MSCs protected the kidney from hypertensive damage by downregulating the pro-inflammatory molecules MCP-1 and plasminogen activating inhibitor-1 (PAI-1) and reducing macrophage recruitment to the kidney in a hypertensive rat model. Furthermore, the miRNA-200-TGF-β axis was found to be significantly altered after EV administration, thereby reprogramming EMT signaling and preventing renal inflammation and fibrosis (101).
MSC-Exos for Kidney Fibrosis
Kidney fibrosis, and in particular tubulointerstitial fibrosis, is the final common outcome of nearly all forms of progressive CKD (2). The histopathology of tubulointerstitial fibrosis is characterized by the deposition of ECM in the interstitium associated with inflammatory cell infiltration, tubular cell damage, fibroblast activation and expansion and rarefaction of the peritubular microvasculature (2). Many studies have established TGF-β as a major profibrotic factor through various mechanisms (102). Once renal fibrosis supervenes, progressive functional decline occurs, which relentlessly progresses leading to dialysis or renal transplantation.
In recent years, apart from diabetic and hypertensive CKD models, several other rodent models of CKD such as unilateral ureteral obstruction (UUO), ischemia-reperfusion injury (IRI) and 2 kidney, 1 clip (2K-1C) unilateral renal artery stenosis model have also been utilized to assess the anti-fibrotic efficacy of MSC-Exos (Table 2). UUO induces severe renal injury, characterized by reduced renal blood flow and glomerular filtration rate within 24 h, followed by interstitial inflammation (peak at 2–3 days), tubular dilation, tubular atrophy and fibrosis within a week. It develops interstitial infiltration of macrophages, tubular cell death, the phenotypic transition of resident renal cells and severe interstitial renal fibrosis with excessive ECM accumulation (103). Renal IRI is one of the leading causes of acute kidney injury (AKI), which temporarily suspends the oxygen and nutrient supply to kidney, inducing robust cellular and molecular responses primarily in TECs. After IRI, the acutely damaged kidney experiences a transition from an unresolved self-healing process to maladaptive repair, resulting in incomplete recovery and progression to kidney fibrosis (104, 105). In the 2K-1C model, one renal artery is constricted to chronically reduce renal perfusion, leading to renal hypertension, hypoxia, activation of RAAS and irreversible renal impairment (106, 107).
Natural MSC-Exos
It is now well recognized that the alleviation of the inciting cause of fibrosis alone is not sufficient to restore kidney function as functional nephron tissue is damaged or lost after kidney injury (108, 109). Consequently, MSC-Exos with potential for kidney regeneration might represent an innovative strategy for kidney fibrosis alleviation. Although MSCs have been proven to be derived from virtually all tissues’ adventitial progenitor cells and pericytes, UC-derived MSCs are considered one of the major MSCs sources for clinical and research applications (74). During the prenatal phase, the UC is genetically and physiologically part of the fetus and usually contains two arteries and one vein. These blood vessels are enveloped by mucous connective tissue WJ, which is derived from the extraembryonic mesoderm and exerts a protective function (13). Both UC and WJ are considered as promising sites for MSC collection (13, 74).
A notable mechanism of kidney fibrosis is injury-induced oxidative stress, which is caused by over-production of reactive oxygen species (ROS) that exceed its scavenging capacity (110). Excessive ROS repress the antioxidant enzymes and results in breakdown of cells through lipid peroxidation, DNA fragmentation and protein damage. In addition, ROS can promote the progression of renal interstitial fibrosis by regulating the infiltration of inflammatory cells such as monocytes and macrophages (111). UUO-induced renal damage is associated with oxidative stress-induced renal tubular apoptosis (112). UC-MSC-Exos administered after UUO alleviated kidney fibrosis and restored renal function (decreased BUN and Scr) through inhibition of apoptosis, malondialdehyde (MDA), ROS, and ROS-mediated P38MAPK/ERK signaling pathways. In vitro, similar anti-fibrotic effects were also observed in TGF-β1 treated NRK-52E cells (113). Another study investigating the anti-fibrotic effects of UC-MSC-Exos confirmed the involvement of Hippo and yes associated protein (YAP) signaling, which regulates TGF-β-Smad signaling, podocyte mesenchymal-epithelial trans-differentiation and ECM protein synthesis (114). Once the Hippo pathway is activated, it limits tissue growth and cell proliferation through the degradation of YAP. UC-MSC-Exos deliver two major ubiquitination related enzyme casein kinase 1d (CK1d) and E3 ubiquitin ligase-transducin repeats-containing protein (b-TRCP) to trigger ubiquitination and degradation of YAP in TECs. This reduced ECM deposition and attenuated fibrosis associated with UUO. Knockdown of CK1d and b-TRCP abrogated the repairing effects of UC-MSC-Exos on renal fibrosis, implying that the efficacy of UC-MSC-Exos relies on the transportation of these active proteins (114).
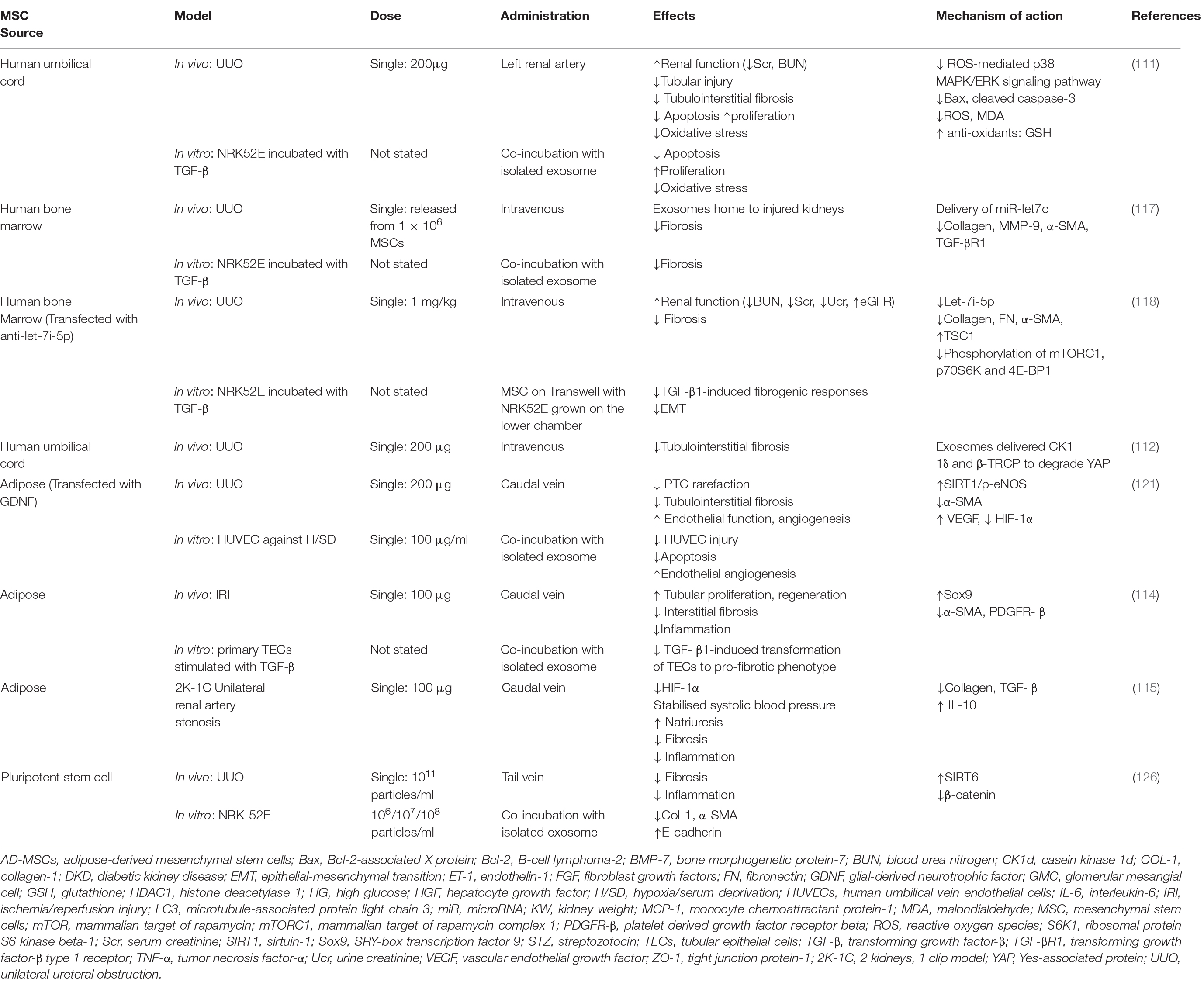
Table 2. Summary of anti-fibrotic effects of MSC-Exos from various sources in preclinical models of kidney fibrosis.
Sex-determining region Y-box transcription factor 9 (Sox-9) is a transcription factor of the sex-determining region Y (SRY) box family and may repair injured kidney (115). AD-MSCs-Exos upregulated Sox9 and prevented TGF-β1-induced transformation of TECs into a pro-fibrotic phenotype in vitro. Moreover, AD-MSC-EVs were capable of attenuating kidney fibrosis through improving kidney hypoxia, reducing inflammatory cell infiltration and inflammatory cytokine secretion, and inhibiting the TGF-β1/Smad 3 signaling pathway in mice subjected to unilateral IRI (116). IRI also occurs in donation after circulatory death (DCD) kidneys. To evaluate the renoprotective effect of MSC-EVs on isolated DCD kidney, MSC-EVs were applied as part of the hypothermic machine perfusion (HMP) procedure in a rat DCD model. The addition of MSC-EVs during HMP attenuated the ischemic kidney injury through maintaining the enzymatic machinery critical for cell survival and reduced the reperfusion damage to kidney (117). Beneficial properties of AD-MSC-Exos were also reported in the 2K-1C (118), a renal artery stenosis model. Administration of AD-MSC-Exos were demonstrated to stabilize the systolic blood pressure (SBP), downregulate hypoxia marker HIF-1a and reduce profibrotic gene collogen and TGF-β expression, thus mitigating kidney fibrosis (118). Interestingly, the treatments with AD-MSC-Exos, AD-MSC or AD-MSC-EVs were equally effective in reducing the expression of the fibrotic markers collagen-1 (COL-1) and TGF-β. However, AD-MSCs were the most effective in elevating the expression of the anti-inflammatory IL-10. These difference may be ascribed to the various cargos released and/or to the ability of the vesicles to reach the damaged tissue, which requires further investigation (118). WJ-derived MSCs (WJ-MSCs) are more immune-privileged and exhibit greater immunosuppressive properties compared to BM-MSCs or AD-MSCs. WJ-MSCs mitigated kidney fibrosis triggered by IRI through downregulating HGF versus TGF-β1 expression (119).
Engineered MSC-Exos
As mentioned earlier, aside from paracrine transferring their natural biological cargos, exosomes including MSC-Exos can also be engineered to carry different biomolecules to various therapeutic targets. The engineered exosomes have a higher therapeutic potential and efficacy and more specific targeting when compared with naive exosomes (40).
Numerous studies have validated that the anti-fibrotic effect of MSC-Exos can be mediated through the transfer of miRNAs such as miRNA-let7c, which targets fibrosis-associated genes. To deliver miRNA-let7c, Wang et al., utilized lentiviral transduction to construct the engineered human BM-MSCs overexpressing miRNA-let7c. The exosomes released from engineered MSC mediated the transfer of miRNA-let7 to diseased kidney and attenuated UUO-induced kidney fibrosis through repression of fibrotic gene collagen-4 (COL-4), MMP-9, alpha-smooth muscle actin (α-SMA), TGF-β1 and its receptor (120). In another study by Jin et al., exosome-secreting MSCs were transfected with let-7i-5p antagomir (anti-let-7i-5p), and then exosomes were isolated from the transfected MSCs to deliver anti-let-7i-5p oligonucleotides to inhibit the level of let-7i-5p. These engineered exosomes reduced the level of let-7i-5p via delivery of anti-let-7i-5p, reduced ECM deposition and attenuated EMT process in TGF-β1-stimulated NRK-52E cells and in the damaged kidneys of UUO mice, thereby attenuating kidney fibrosis (121). Glial-derived neurotrophic factor (GDNF), an effective neurotrophic factor that protects nigral dopaminergic neurons, promoted the therapeutic effect of MSCs (122, 123). Chen et al transfected GDNF into human AD-MSCs via lentiviral transfection and then exosomes (GDNF-AD-MSC-Exos) were collected from those engineered MSCs. Application of the GDNF-AD-MSC-Exos led to the amelioration of kidney fibrosis in mice with UUO, which was mediated by enhancing SIRT1 signaling and its downstream target, phosphorylated endothelial nitric oxide synthase (p-eNOS), which activated endothelial function and angiogenesis and reduced peritubular capillary loss (124).
iPSC-Derived MSC-Exos for CKD
All MSC mentioned above are from tissues. Despite promising therapeutic effects, tissue-derived MSCs have been reported to have several weaknesses, such as limited potential to proliferate, difficult to standardize, loss of differentiation capacity, and decreased regenerative efficacy with expansion (125). As mentioned earlier, MSCs can also be produced from cells such as iPSCs. Those single cell-derived MSCs have the characteristics of both MSCs and PSCs and are capable of expanding with high efficiency (17). iPSC-MSCs revealed comparable effects in renoprotection, such as reducing apoptosis and enhancing vascularization (4). EVs directly isolated from iPSC rescued rats from IRI through maintaining functional mitochondria and inhibiting oxidative stress-relevant genes (126). Sirtuin 6 (Sirt6) is an NAD-dependent deacetylase of the Sirtuin family that has been suggested to effectively reverse the fibrotic process in many organs (127, 128). More recently, a study by Liu et al established that intravenous infusion of human iPSC-derived MSC-Exos (iPSC-MSC-Exos) mitigated kidney fibrosis, reduced inflammatory responses, and improved renal function in mice subjected to UUO (129). These anti-fibrotic effects of iPSC-MSC-Exos are mediated through increasing SIRT6 while decreasing β-catenin and its downstream products (PAI-1, Fsp1 and Axin2), elucidating a novel mechanism of MSC-Exos in nephroprotection (129).
MSC-Exos in Lupus Nephritis
Systemic lupus erythematosus (SLE) is a common autoimmune disease. It is characterized by multi-organ damage resulting from abnormal activation of autoreactive T cells, the presence of pathogenic autoantibodies and deposition of immune complexes (130). LN is the most common and severe organ injury in SLE (131). Over the past decades, there has been several publications investigating the therapeutic application of MSCs in LN in both animal models and humans. BM-MSCs alleviated LN and improved mice survival rate by effectively inhibiting IL-21 production and follicular helper T cell differentiation (132). The combination of MSCs with prednisone or mycophenolate mofetil (MMF) improved survival, reduced the secretion of autoantibody and inflammatory cytokines, and decreased the infiltration of inflammatory cells in the kidney in a mouse model of lupus nephritis (133). In LN patients, allogeneic MSC transplantation (MSCT) resulted in an increased glomerular filtration rate (GFR) and renal remission over 12 months, confirming its therapeutic potential for LN (134). It has been well established that MSC-Exos exert immunomodulatory effects through delivery of immunosuppressive molecules that inhibit infiltration, proliferation, differentiation and activation of immune cells or induce anti-inflammatory cells (135). Additionally, MSC-Exos promote the chemotaxis of anti-inflammatory non-coding RNAs to accelerate tissue healing (136). However, despite the efficacy and clinical potential for therapeutic application in inflammatory glomerular disease indicated by these studies, there are few publications applying MSC-Exos directly in LN animal models or in human patients. Recently, Wei et al reported that miR-20a-containing exosomes are responsible for the alleviation of LN in the mouse lupus model through enhancing autophagy (137). In another study by Chen et al., UC-MSC-Exos attenuated SLE-associated diffuse alveolar hemorrhage (DAH) by regulating macrophage polarization in murine lupus (138). In summary, further studies are warranted for a better understanding of the application of MSC-Exos-based therapy in LN and more generally in glomerular disease.
Conclusion
Chronic kidney disease is a world-wide pandemic, and its prevalence is rising annually. MSC-Exos transfer a variety of growth factors and non-coding miRNAs to injured renal cells, which attenuate kidney injury and restore kidney function through promoting proliferation, autophagy and angiogenesis, and suppressing inflammation, oxidative stress, apoptosis, EMT, and tubulointerstitial fibrosis. Thus, MSC-Exos represent a novel cell-free therapeutic strategy for the treatment of CKD.
Despite advances in understanding the therapeutic capacity of MSC-Exos in CKD, major issues surrounding large-scale production and purification must be overcome before translation of MSC-Exos therapy to clinical application occurs. The amount of MSC-Exos required for clinical application is high. Recently, new technologies such as 3D culture conditions using hydrogels, spheroid or hollow fibers and bioreactors have been introduced to allow large-scale production of exosomes (139). To optimize the procedures of isolation/purification of exosomes, new approaches such as tangential flow filtration (TFF) and asymmetrical field-flow fractionation (AsFFF) have been applied (140, 141). However, there is a lack of standard techniques to quickly isolate, purify, quantitate, and identify exosomes. Moreover, it still requires further investigation to fully understand the biodistribution and clearance of MSC-Exos upon administration. Biodistribution of systemically administered exosomes is a dynamic process. Although several in vivo tracking strategies have been employed, current knowledge of the biodistribution of MSC-Exos is limited.
To conclude, advances in MSC-Exos studies hold a great promise for the regenerative treatment of CKD. Future studies focusing on the standardization of MSC-Exos production, purification, and characterization to improve quality and safety will enable the translation of MSC-Exos into the clinic as efficient therapeutics for CKD.
Author Contributions
QC conceived and wrote the manuscript. X-MC and CH reviewed the manuscript. CP revised and reviewed the manuscript. All authors have read and agreed to the published version of the manuscript.
Conflict of Interest
The authors declare that the research was conducted in the absence of any commercial or financial relationships that could be construed as a potential conflict of interest.
Publisher’s Note
All claims expressed in this article are solely those of the authors and do not necessarily represent those of their affiliated organizations, or those of the publisher, the editors and the reviewers. Any product that may be evaluated in this article, or claim that may be made by its manufacturer, is not guaranteed or endorsed by the publisher.
Abbreviations
AD-MSCs, adipose-derived mesenchymal stem cells; AD-MSC-Exos, AD-MSC-derived exosomes; AKI: acute kidney injury; AsFFF, asymmetrical field-flow fractionation; α -SMA, α -smooth muscle actin; Bax, Bcl-2-associated X protein; Bcl-2, B-cell lymphoma-2; BM, bone marrow; BM-MSC, bone marrow-derived mesenchymal stem cell; BM-MSC-Exos, BM-MSC-derived exosomes; BMP-7, bone morphogenetic protein-7; b-TRCP, E3 ubiquitin ligase-transducin repeats-containing protein; BUN, blood urea nitrogen; CD, cluster of differentiation; CNS, central nervous system; CK1d, casein kinase 1d; CKD, chronic kidney disease; COL-1, collagen-1; COL-4, collagen-4; DKD, diabetic kidney disease; EGF, epidermal growth factor; EMMPRIN, extracellular matrix metalloproteinase inducer; EMT, epithelial-mesenchymal transition; EPC, endothelial progenitor cells; ESC, embryonic stem cells; ESKD, end stage kidney disease; ET-1, endothelin-1; EV, extracellular vesicles; FGF, fibroblast growth factor; FN, fibronectin; GDNF, glial-derived neurotrophic factor; GF, growth factor; GFB, glomerular filtration barrier; GMCs, glomerular mesangial cells; HDAC1, histone deacetylase 1; HGF, hepatocyte growth factor; HLA-DR, human leukocyte antigen-DR isotype; HSP, heat-shock proteins; IL-6, interleukin-6; iPSC, induced pluripotent stem cells; iPSC-MSC-Exos, iPSC-MSC-derived Exosomes; IRI, ischemia-reperfusion injury; LN, lupus nephritis; MCP-1, monocyte chemoattracting protein-1; MDA, malondialdehyde; MMF, mycophenolate mofetil; MiRNAs, microRNAs; MMP-9, metalloproteinase-9; MSC, mesenchymal stem cells; MSC-Exos, MSC-derived exosomes; MSCT, MSC transplantation; mTOR, mammalian target of rapamycin; MVB, multivesicular bodies; PAI-1, plasminogen activating inhibitor-1; P-eNOS, phosphorylated endothelial nitric oxide synthase; PSC, pluripotent stem cells; RAAS, renin-angiotensin-aldosterone system; ROS, reactive oxygen species; SBP, systolic blood pressure; SC, stem cell; Scr, serum creatinine; SMC, smooth muscle cell; SEC, size exclusion chromatography; SiRNA, short interfering-RNA; Sirt6, sirtuin 6; SLE, systemic lupus erythematosus; Sox9, SRY-box transcription factor 9; SRY, sex-determining region Y; TECs, tubular epithelial cells; TEM, transmission electron microscopy; TFF, tangential flow filtration; TGF- β : transforming growth factor-beta; TSG101, tumor susceptibility gene 101; UC, umbilical cord; USC, urine-derived stem cell; UC-MSC, umbilical cord-derived mesenchymal stem cell; UC-MSC-Exos, UC-MSC-derived exosomes; UUO, unilateral ureteral obstruction; VEGF, vascular endothelial growth factor; WJ, Wharton’s jelly; WJ-MSC, WJ-derived MSC; YAP, yes associated protein; 2K-1C, 2 kidney, 1 clip; 3-MA, 3-methyladenine.
References
1. Webster AC, Nagler EV, Morton RL, Masson P. Chronic kidney disease. Lancet. (2017) 389:1238–52. doi: 10.1016/s0140-6736(16)32064-5
2. Duffield JS. Cellular and molecular mechanisms in kidney fibrosis. J Clin Invest. (2014) 124:2299–306. doi: 10.1172/JCI72267
3. Levin A, Tonelli M, Bonventre J, Coresh J, Donner JA, Fogo AB, et al. Global kidney health 2017 and beyond: a roadmap for closing gaps in care, research, and policy. Lancet. (2017) 390:1888–917. doi: 10.1016/s0140-6736(17)30788-2
4. Rota C, Morigi M, Imberti B. Stem cell therapies in kidney diseases: progress and challenges. Int J Mol Sci. (2019) 20:2790. doi: 10.3390/ijms20112790
5. Ilic D, Ogilvie C. Concise review: human embryonic stem cells—what have we done? What are we doing? Where are we going? Stem Cells. (2017) 35:17–25. doi: 10.1002/stem.2450
6. Zakrzewski W, Dobrzyñski M, Szymonowicz M, Rybak Z. Stem cells: past, present, and future. Stem Cell Res Ther. (2019) 10:68. doi: 10.1186/s13287-019-1165-5
7. Uccelli A, Moretta L, Pistoia V. Mesenchymal stem cells in health and disease. Nat Rev Immunol. (2008) 8:726–36. doi: 10.1038/nri2395
8. Campagnoli C, Roberts IA, Kumar S, Bennett PR, Bellantuono I, Fisk NM. Identification of mesenchymal stem/progenitor cells in human first-trimester fetal blood, liver, and bone marrow. Blood. (2001) 98:2396–402. doi: 10.1182/blood.v98.8.2396
9. Liu S, Liu F, Zhou Y, Jin B, Sun Q, Guo S. Immunosuppressive property of MSCs mediated by cell surface receptors. Front Immunol. (2020) 11:1076. doi: 10.3389/fimmu.2020.01076
10. Jiang W, Xu J. Immune modulation by mesenchymal stem cells. Cell Prolif. (2020) 53:e12712. doi: 10.1111/cpr.12712
11. Zuk PA, Zhu M, Ashjian P, De Ugarte DA, Huang JI, Mizuno H, et al. Human adipose tissue is a source of multipotent stem cells. Mol Biol Cell. (2002) 13:4279–95. doi: 10.1091/mbc.e02-02-0105
12. Nemeth K, Mezey E. Bone marrow stromal cells as immunomodulators. A primer for dermatologists. J Dermatol Sci. (2015) 77:11–20. doi: 10.1016/j.jdermsci.2014.10.004
13. Troyer DL, Weiss ML. Concise review: Wharton’s jelly-derived cells are a primitive stromal cell population. Stem Cells. (2008) 26:591–9. doi: 10.1634/stemcells.2007-0439
14. Hassan G, Kasem I, Soukkarieh C, Aljamali M. A simple method to isolate and expand human umbilical cord derived mesenchymal stem cells: using explant method and umbilical cord blood serum. Int J Stem Cells. (2017) 10:184–92. doi: 10.15283/ijsc17028
15. Nancarrow-Lei R, Mafi P, Mafi R, Khan W. A systemic review of adult mesenchymal stem cell sources and their multilineage differentiation potential relevant to musculoskeletal tissue repair and regeneration. Curr Stem Cell Res Ther. (2017) 12:601–10. doi: 10.2174/1574888x12666170608124303
16. Lian Q, Zhang Y, Zhang J, Zhang HK, Wu X, Zhang Y, et al. Functional mesenchymal stem cells derived from human induced pluripotent stem cells attenuate limb ischemia in mice. Circulation. (2010) 121:1113–23. doi: 10.1161/circulationaha.109.898312
17. Tabar V, Studer L. Pluripotent stem cells in regenerative medicine: challenges and recent progress. Nat Rev Genet. (2014) 15:82–92. doi: 10.1038/nrg3563
18. Romito A, Cobellis G. Pluripotent stem cells: current understanding and future directions. Stem Cells Int. (2016) 2016:9451492. doi: 10.1155/2016/9451492
19. Takahashi K, Tanabe K, Ohnuki M, Narita M, Ichisaka T, Tomoda K, et al. Induction of pluripotent stem cells from adult human fibroblasts by defined factors. Cell. (2007) 131:861–72. doi: 10.1016/j.cell.2007.11.019
20. Dominici M, Le Blanc K, Mueller I, Slaper-Cortenbach I, Marini F, Krause D, et al. Minimal criteria for defining multipotent mesenchymal stromal cells. The international society for cellular therapy position statement. Cytotherapy. (2006) 8:315–7. doi: 10.1080/14653240600855905
21. Spees JL, Lee RH, Gregory CA. Mechanisms of mesenchymal stem/stromal cell function. Stem Cell Res Ther. (2016) 7:125. doi: 10.1186/s13287-016-0363-7
22. Liu D, Cheng F, Pan S, Liu Z. Stem cells: a potential treatment option for kidney diseases. Stem Cell Res Ther. (2020) 11:249. doi: 10.1186/s13287-020-01751-2
23. Morigi M, Imberti B, Zoja C, Corna D, Tomasoni S, Abbate M, et al. Mesenchymal stem cells are renotropic, helping to repair the kidney and improve function in acute renal failure. J Am Soc Nephrol. (2004) 15:1794–804. doi: 10.1097/01.asn.0000128974.07460.34
24. Musial-Wysocka A, Kot M, Majka M. The pros and cons of mesenchymal stem cell-based therapies. Cell Transpl. (2019) 28:801–12. doi: 10.1177/0963689719837897
25. Mäkelä T, Takalo R, Arvola O, Haapanen H, Yannopoulos F, Blanco R, et al. Safety and biodistribution study of bone marrow-derived mesenchymal stromal cells and mononuclear cells and the impact of the administration route in an intact porcine model. Cytotherapy. (2015) 17:392–402. doi: 10.1016/j.jcyt.2014.12.004
26. Barkholt L, Flory E, Jekerle V, Lucas-Samuel S, Ahnert P, Bisset L, et al. Risk of tumorigenicity in mesenchymal stromal cell-based therapies–bridging scientific observations and regulatory viewpoints. Cytotherapy. (2013) 15:753–9. doi: 10.1016/j.jcyt.2013.03.005
27. Rani S, Ryan AE, Griffin MD, Ritter T. Mesenchymal stem cell-derived extracellular vesicles: toward cell-free therapeutic applications. Mol Ther. (2015) 23:812–23. doi: 10.1038/mt.2015.44
28. Xin H, Li Y, Chopp M. Exosomes/miRNAs as mediating cell-based therapy of stroke. Front Cell Neurosci. (2014) 8:377. doi: 10.3389/fncel.2014.00377
29. Park JH, Hwang I, Hwang SH, Han H, Ha H. Human umbilical cord blood-derived mesenchymal stem cells prevent diabetic renal injury through paracrine action. Diabetes Res Clin Pract. (2012) 98:465–73. doi: 10.1016/j.diabres.2012.09.034
30. Yáñez-Mó M, Siljander PR, Andreu Z, Zavec AB, Borràs FE, Buzas EI, et al. Biological properties of extracellular vesicles and their physiological functions. J Extracell Vesicles. (2015) 4:27066. doi: 10.3402/jev.v4.27066
31. Doyle LM, Wang MZ. Overview of extracellular vesicles, their origin, composition, purpose, and methods for exosome isolation and analysis. Cells. (2019) 8:727. doi: 10.3390/cells8070727
32. Wei W, Ao Q, Wang X, Cao Y, Liu Y, Zheng SG, et al. Mesenchymal stem cell-derived exosomes: a promising biological tool in nanomedicine. Front Pharmacol. (2021) 11:590470. doi: 10.3389/fphar.2020.590470
33. Phinney DG, Pittenger MF. Concise review: MSC-derived exosomes for cell-free therapy. Stem Cells. (2017) 35:851–8. doi: 10.1002/stem.2575
34. Zhang Y, Liu Y, Liu H, Tang WH. Exosomes: biogenesis, biologic function and clinical potential. Cell Biosci. (2019) 9:19. doi: 10.1186/s13578-019-0282-2
35. Sidhom K, Obi PO, Saleem A. A review of exosomal isolation methods: is size exclusion chromatography the best option? Int J Mol Sci. (2020) 21:6466. doi: 10.3390/ijms21186466
36. Simpson RJ, Lim JWE, Moritz RL, Mathivanan S. Exosomes: proteomic insights and diagnostic potential. Expert Rev Proteomics. (2009) 6:267–83. doi: 10.1586/epr.09.17
37. Valadi H, Ekström K, Bossios A, Sjöstrand M, Lee JJ, Lötvall JO. Exosome-mediated transfer of mRNAs and microRNAs is a novel mechanism of genetic exchange between cells. Nat Cell Biol. (2007) 9:654–9. doi: 10.1038/ncb1596
38. Kalluri R, LeBleu VS. The biology, function, and biomedical applications of exosomes. Science. (2020) 367:eaau6977. doi: 10.1126/science.aau6977
39. Tian T, Wang Y, Wang H, Zhu Z, Xiao Z. Visualizing of the cellular uptake and intracellular trafficking of exosomes by live-cell microscopy. J Cell Biochem. (2010) 111:488–96. doi: 10.1002/jcb.22733
40. Herrmann IK, Wood MJA, Fuhrmann G. Extracellular vesicles as a next-generation drug delivery platform. Nat Nanotechnol. (2021) 16:748–59. doi: 10.1038/s41565-021-00931-2
41. Oskouie MN, Aghili Moghaddam NS, Butler AE. Therapeutic use of curcumin-encapsulated and curcumin-primed exosomes. J Cell Physiol. (2019) 234:8182–91. doi: 10.1002/jcp.27615
42. Luan X, Sansanaphongpricha K, Myers I, Chen H, Yuan H, Sun D. Engineering exosomes as refined biological nanoplatforms for drug delivery. Acta Pharmacol Sin. (2017) 38:754–63. doi: 10.1038/aps.2017.12
43. Lai CP, Mardini O, Ericsson M, Prabhakar S, Maguire CA, Chen JW, et al. Dynamic biodistribution of extracellular vesicles in vivo using a multimodal imaging reporter. ACS Nano. (2014) 8:483–94. doi: 10.1021/nn404945r
44. Fu S, Wang Y, Xia X, Zheng JC. Exosome engineering: current progress in cargo loading and targeted delivery. Nanoimpact. (2020) 20:100261. doi: 10.1016/j.impact.2020.100261
45. Batrakova EV, Kim MS. Using exosomes, naturally-equipped nanocarriers, for drug delivery. J Control Release. (2015) 219:396–405. doi: 10.1016/j.jconrel.2015.07.030
46. Elliott RO, He M. Unlocking the power of exosomes for crossing biological barriers in drug delivery. Pharmaceutics. (2021) 13:122. doi: 10.3390/pharmaceutics13010122
47. Chua JH, Armugam A, Jeyaseelan K. MicroRNAs: biogenesis, function and applications. Curr Opin Mol Ther. (2009) 11:189–99.
48. Cao Q, Chen XM, Huang C, Pollock CA. MicroRNA as novel biomarkers and therapeutic targets in diabetic kidney disease: an update. FASEB Bioadv. (2019) 1:375–88. doi: 10.1096/fba.2018-00064
49. Chen TS, Lai RC, Lee MM, Choo AB, Lee CN, Lim SK. Mesenchymal stem cell secretes microparticles enriched in pre-microRNAs. Nucleic Acids Res. (2010) 38:215–24. doi: 10.1093/nar/gkp857
50. Cheng L, Zhang K, Wu S, Cui M, Xu T. Focus on mesenchymal stem cell-derived exosomes: opportunities and challenges in cell-free therapy. Stem Cells Int. (2017) 2017:6305295. doi: 10.1155/2017/6305295
51. Harrell CR, Jovicic N, Djonov V, Arsenijevic N, Volarevic V. Mesenchymal stem cell-derived exosomes and other extracellular vesicles as new remedies in the therapy of inflammatory diseases. Cells. (2019) 8:1605. doi: 10.3390/cells8121605
52. Harrell CR, Jovicic N, Djonov V, Volarevic V. Therapeutic use of mesenchymal stem cell-derived exosomes: from basic science to clinics. Pharmaceutics. (2020) 12:474. doi: 10.3390/pharmaceutics12050474
53. Tapparo M, Bruno S, Collino F, Togliatto G, Deregibus MC, Provero P, et al. Renal regenerative potential of extracellular vesicles derived from miRNA-engineered mesenchymal stromal cells. Int J Mol Sci. (2019) 20:2381. doi: 10.3390/ijms20102381
54. Luo L, Yu Z-P, Qin H, Zhu Z-X, Liao M-H, Liao H-T, et al. Exosomal microRNA-10a is associated with liver regeneration in rats through downregulation of EphA4. Chin Med J (Engl). (2018) 131:454–60. doi: 10.4103/0366-6999.225057
55. Zhang R, Qin L, Shi J. MicroRNA-199a-3p suppresses high glucose-induced apoptosis and inflammation by regulating the IKKβ/NF−κB signaling pathway in renal tubular epithelial cells. Int J Mol Med. (2020) 46:2161–71. doi: 10.3892/ijmm.2020.4751
56. Li Z, Zhou Y, Zhang L, Jia K, Wang S, Wang M, et al. microRNA-199a-3p inhibits hepatic apoptosis and hepatocarcinogenesis by targeting PDCD4. Oncogenesis. (2020) 9:95. doi: 10.1038/s41389-020-00282-y
57. Abu El-Asrar AM, Ahmad A, Alam K, Siddiquei MM, Mohammad G, Hertogh GD, et al. Extracellular matrix metalloproteinase inducer (EMMPRIN) is a potential biomarker of angiogenesis in proliferative diabetic retinopathy. Acta Ophthalmol. (2017) 95:697–704. doi: 10.1111/aos.13284
58. Bergers G, Brekken R, McMahon G, Vu TH, Itoh T, Tamaki K, et al. Matrix metalloproteinase-9 triggers the angiogenic switch during carcinogenesis. Nat Cell Biol. (2000) 2:737–44. doi: 10.1038/35036374
59. Pers Y-M, Bony C, Duroux-Richard I, Bernard L, Maumus M, Assou S, et al. miR-155 contributes to the immunoregulatory function of human mesenchymal stem cells. Front Immunol. (2021) 12:624024. doi: 10.3389/fimmu.2021.624024
60. Tavasolian F, Hosseini AZ, Soudi S, Naderi M. miRNA-146a improves immunomodulatory effects of MSC-derived exosomes in rheumatoid arthritis. Curr Gene Ther. (2020) 20:297–312. doi: 10.2174/1566523220666200916120708
61. Wu H, Fan H, Shou Z, Xu M, Chen Q, Ai C, et al. Extracellular vesicles containing miR-146a attenuate experimental colitis by targeting TRAF6 and IRAK1. Int Immunopharmacol. (2019) 68:204–12. doi: 10.1016/j.intimp.2018.12.043
62. Assunção-Silva RC, Mendes-Pinheiro B, Patrício P, Behie LA, Teixeira FG, Pinto L, et al. Exploiting the impact of the secretome of MSCs isolated from different tissue sources on neuronal differentiation and axonal growth. Biochimie. (2018) 155:83–91. doi: 10.1016/j.biochi.2018.07.026
63. Pires AO, Mendes-Pinheiro B, Teixeira FG, Anjo SI, Ribeiro-Samy S, Gomes ED, et al. Unveiling the differences of secretome of human bone marrow mesenchymal stem cells, adipose tissue-derived stem cells, and human umbilical cord perivascular cells: a proteomic analysis. Stem Cells Dev. (2016) 25:1073–83. doi: 10.1089/scd.2016.0048
64. Hoang DH, Nguyen TD, Nguyen H-P, Nguyen X-H, Do PTX, Dang VD, et al. Differential wound healing capacity of mesenchymal stem cell-derived exosomes originated from bone marrow, adipose tissue and umbilical cord under serum- and xeno-free condition. Front Mol Biosci. (2020) 7:119. doi: 10.3389/fmolb.2020.00119
65. Wang Z-G, He Z-Y, Liang S, Yang Q, Cheng P, Chen A-M. Comprehensive proteomic analysis of exosomes derived from human bone marrow, adipose tissue, and umbilical cord mesenchymal stem cells. Stem Cell Res Ther. (2020) 11:511. doi: 10.1186/s13287-020-02032-8
66. Nikfarjam S, Rezaie J, Zolbanin NM, Jafari R. Mesenchymal stem cell derived-exosomes: a modern approach in translational medicine. J Transl Med. (2020) 18:449. doi: 10.1186/s12967-020-02622-3
67. Gregorini M, Corradetti V, Rocca C, Pattonieri EF, Valsania T, Milanesi S, et al. Mesenchymal stromal cells prevent renal fibrosis in a rat model of unilateral ureteral obstruction by suppressing the renin-angiotensin system via HuR. PLoS One. (2016) 11:e0148542. doi: 10.1371/journal.pone.0148542
68. Gregorini M, Maccario R, Avanzini MA, Corradetti V, Moretta A, Libetta C, et al. Antineutrophil cytoplasmic antibody-associated renal vasculitis treated with autologous mesenchymal stromal cells: evaluation of the contribution of immune-mediated mechanisms. Mayo Clin Proc. (2013) 88:1174–9. doi: 10.1016/j.mayocp.2013.06.021
69. Gheith O, Farouk N, Nampoory N, Halim MA, Al-Otaibi T. Diabetic kidney disease: world wide difference of prevalence and risk factors. J Nephropharmacol. (2015) 5:49–56.
70. Reidy K, Kang HM, Hostetter T, Susztak K. Molecular mechanisms of diabetic kidney disease. J Clin Invest. (2014) 124:2333–40. doi: 10.1172/JCI72271
71. Badal SS, Danesh FR. New insights into molecular mechanisms of diabetic kidney disease. Am J Kidney Dis. (2014) 63(2 Suppl. 2):S63–83. doi: 10.1053/j.ajkd.2013.10.047
72. Friedenstein AJ, Chailakhjan RK, Lalykina KS. The development of fibroblast colonies in monolayer cultures of guinea-pig bone marrow and spleen cells. Cell Tissue Kinet. (1970) 3:393–403. doi: 10.1111/j.1365-2184.1970.tb00347.x
73. Nagaishi K, Mizue Y, Chikenji T, Otani M, Nakano M, Konari N, et al. Mesenchymal stem cell therapy ameliorates diabetic nephropathy via the paracrine effect of renal trophic factors including exosomes. Sci Rep. (2016) 6:34842. doi: 10.1038/srep34842
74. Arutyunyan I, Elchaninov A, Makarov A, Fatkhudinov T. Umbilical cord as prospective source for mesenchymal stem cell-based therapy. Stem Cells Int. (2016) 2016:6901286. doi: 10.1155/2016/6901286
75. Xiang E, Han B, Zhang Q, Rao W, Wang Z, Chang C, et al. Human umbilical cord-derived mesenchymal stem cells prevent the progression of early diabetic nephropathy through inhibiting inflammation and fibrosis. Stem Cell Res Ther. (2020) 11:336. doi: 10.1186/s13287-020-01852-y
76. Chun Y, Kim J. Autophagy: an essential degradation program for cellular homeostasis and life. Cells. (2018) 7:278. doi: 10.3390/cells7120278
77. Filomeni G, De Zio D, Cecconi F. Oxidative stress and autophagy: the clash between damage and metabolic needs. Cell Death Differ. (2015) 22:377–88. doi: 10.1038/cdd.2014.150
78. Yang D, Livingston MJ, Liu Z, Dong G, Zhang M, Chen J-K, et al. Autophagy in diabetic kidney disease: regulation, pathological role and therapeutic potential. Cell Mol Life Sci. (2018) 75:669–88. doi: 10.1007/s00018-017-2639-1
79. Ebrahim N, Ahmed IA, Hussien NI, Dessouky AA, Farid AS, Elshazly AM, et al. Mesenchymal stem cell-derived exosomes ameliorated diabetic nephropathy by autophagy induction through the mTOR signaling pathway. Cells. (2018) 7:226. doi: 10.3390/cells7120226
80. Gimble JM, Katz AJ, Bunnell BA. Adipose-derived stem cells for regenerative medicine. Circ Res. (2007) 100:1249–60. doi: 10.1161/01.RES.0000265074.83288.09
81. Jin J, Shi Y, Gong J, Zhao L, Li Y, He Q, et al. Exosome secreted from adipose-derived stem cells attenuates diabetic nephropathy by promoting autophagy flux and inhibiting apoptosis in podocyte. Stem Cell Res Ther. (2019) 10:95. doi: 10.1186/s13287-019-1177-1
82. Pomatto MAC, Bussolati B, D’Antico S, Ghiotto S, Tetta C, Brizzi MF, et al. Improved loading of plasma-derived extracellular vesicles to encapsulate antitumor miRNAs. Mol Ther Methods Clin Dev. (2019) 13:133–44. doi: 10.1016/j.omtm.2019.01.001
83. Ridder K, Keller S, Dams M, Rupp AK, Schlaudraff J, Del Turco D, et al. Extracellular vesicle-mediated transfer of genetic information between the hematopoietic system and the brain in response to inflammation. PLoS Biol. (2014) 12:e1001874. doi: 10.1371/journal.pbio.1001874
84. Huang X, Yuan T, Tschannen M, Sun Z, Jacob H, Du M, et al. Characterization of human plasma-derived exosomal RNAs by deep sequencing. BMC Genomics. (2013) 14:319. doi: 10.1186/1471-2164-14-319
85. Daehn IS, Duffield JS. The glomerular filtration barrier: a structural target for novel kidney therapies. Nat Rev Drug Discov. (2021) 20:770–88. doi: 10.1038/s41573-021-00242-0
86. Loeffler I, Wolf G. Epithelial-to-mesenchymal transition in diabetic nephropathy: fact or fiction? Cells. (2015) 4:631–52. doi: 10.3390/cells4040631
87. Jin J, Wang Y, Zhao L, Zou W, Tan M, He Q. Exosomal miRNA-215-5p derived from adipose-derived stem cells attenuates epithelial-mesenchymal transition of podocytes by inhibiting ZEB2. Biomed Res Int. (2020) 2020:2685305. doi: 10.1155/2020/2685305
88. Kasztan M, Pollock David M. Impact of ET-1 and sex in glomerular hyperfiltration in humanized sickle cell mice. Clin Sci. (2019) 133:1475–86. doi: 10.1042/cs20190215
89. Ahlborg G, Lindström J. Insulin sensitivity and big ET-1 conversion to ET-1 after ETA- or ETB-receptor blockade in humans. J Appl Physiol. (2002) 93:2112–21. doi: 10.1152/japplphysiol.00477.2002
90. Hao Y, Miao J, Liu W, Cai K, Huang X, Peng L. Mesenchymal stem cell-derived exosomes carry microRNA-125a to protect against diabetic nephropathy by targeting histone deacetylase 1 and downregulating endothelin-1. Diabetes Metab Syndr Obes. (2021) 14:1405–18. doi: 10.2147/DMSO.S286191
91. Bento G, Shafigullina AK, Rizvanov AA, Sardao VA, Macedo MP, Oliveira PJ. Urine-derived stem cells: applications in regenerative and predictive medicine. Cells. (2020) 9:573. doi: 10.3390/cells9030573
92. Zhang C, George SK, Wu R, Thakker PU, Abolbashari M, Kim T-H, et al. Reno-protection of urine-derived stem cells in a chronic kidney disease rat model induced by renal ischemia and nephrotoxicity. Int J Biol Sci. (2020) 16:435–46. doi: 10.7150/ijbs.37550
93. Pavathuparambil Abdul Manaph N, Al-Hawwas M, Bobrovskaya L, Coates PT, Zhou X-F. Urine-derived cells for human cell therapy. Stem Cell Res Ther. (2018) 9:189. doi: 10.1186/s13287-018-0932-z
94. Jiang ZZ, Liu YM, Niu X, Yin JY, Hu B, Guo SC, et al. Exosomes secreted by human urine-derived stem cells could prevent kidney complications from type I diabetes in rats. Stem Cell Res Ther. (2016) 7:24. doi: 10.1186/s13287-016-0287-2
95. Duan YR, Chen BP, Chen F, Yang SX, Zhu CY, Ma YL, et al. Exosomal microRNA-16-5p from human urine-derived stem cells ameliorates diabetic nephropathy through protection of podocyte. J Cell Mol Med. (2021) 25:10798–813. doi: 10.1111/jcmm.14558
96. Griffin KA. Hypertensive kidney injury and the progression of chronic kidney disease. Hypertension. (2017) 70:687–94. doi: 10.1161/HYPERTENSIONAHA.117.08314
97. Chagnac A, Zingerman B, Rozen-Zvi B, Herman-Edelstein M. Consequences of glomerular hyperfiltration: the role of physical forces in the pathogenesis of chronic kidney disease in diabetes and obesity. Nephron. (2019) 143:38–42. doi: 10.1159/000499486
98. Lopez-Novoa JM, Martinez-Salgado C, Rodriguez-Pena AB, Lopez-Hernandez FJ. Common pathophysiological mechanisms of chronic kidney disease: therapeutic perspectives. Pharmacol Ther. (2010) 128:61–81. doi: 10.1016/j.pharmthera.2010.05.006
99. de Boer IH, Bangalore S, Benetos A, Davis AM, Michos ED, Muntner P, et al. Diabetes and hypertension: a position statement by the american diabetes association. Diabetes Care. (2017) 40:1273–84. doi: 10.2337/dci17-0026
100. Aliotta JM, Pereira M, Wen S, Dooner MS, Del Tatto M, Papa E, et al. Exosomes induce and reverse monocrotaline-induced pulmonary hypertension in mice. Cardiovasc Res. (2016) 110:319–30. doi: 10.1093/cvr/cvw054
101. Lindoso RS, Lopes JA, Binato R, Abdelhay E, Takiya CM, Miranda KR, et al. Adipose mesenchymal cells-derived EVs alleviate DOCA-salt-induced hypertension by promoting cardio-renal protection. Mol Ther Methods Clin Dev. (2020) 16:63–77. doi: 10.1016/j.omtm.2019.11.002
102. Meng XM, Nikolic-Paterson DJ, Lan HY. TGF-beta: the master regulator of fibrosis. Nat Rev Nephrol. (2016) 12:325–38. doi: 10.1038/nrneph.2016.48
103. Martínez-Klimova E, Aparicio-Trejo OE, Tapia E, Pedraza-Chaverri J. Unilateral ureteral obstruction as a model to investigate fibrosis-attenuating treatments. Biomolecules. (2019) 9:141. doi: 10.3390/biom9040141
104. Le Clef N, Verhulst A, D’Haese PC, Vervaet BA. Unilateral renal ischemia-reperfusion as a robust model for acute to chronic kidney injury in mice. PLoS One. (2016) 11:e0152153. doi: 10.1371/journal.pone.0152153
105. Guan Y, Nakano D, Zhang Y, Li L, Tian Y, Nishiyama A. A mouse model of renal fibrosis to overcome the technical variability in ischaemia/reperfusion injury among operators. Sci Rep. (2019) 9:10435. doi: 10.1038/s41598-019-46994-z
106. Chelko SP, Schmiedt CW, Lewis TH, Lewis SJ, Robertson TP. A novel vascular clip design for the reliable induction of 2-kidney, 1-clip hypertension in the rat. J Appl Physiol. (2012) 112:362–6. doi: 10.1152/japplphysiol.01015.2011
107. Li L-Q, Zhang J, Wang R, Li J-X, Gu Y-Q. Establishment and evaluation of a reversible two-kidney, one-clip renovascular hypertensive rat model. Exp Ther Med. (2017) 13:3291–6. doi: 10.3892/etm.2017.4386
108. Allinovi M, De Chiara L, Angelotti ML, Becherucci F, Romagnani P. Anti-fibrotic treatments: a review of clinical evidence. Matrix Biol. (2018) 68-69:333–54. doi: 10.1016/j.matbio.2018.02.017
109. Becherucci F, Mazzinghi B, Allinovi M, Angelotti ML, Romagnani P. Regenerating the kidney using human pluripotent stem cells and renal progenitors. Expert Opin Biol Ther. (2018) 18:795–806. doi: 10.1080/14712598.2018.1492546
110. Liu Y. Cellular and molecular mechanisms of renal fibrosis. Nat Rev Nephrol. (2011) 7:684–96. doi: 10.1038/nrneph.2011.149
111. Ratliff BB, Abdulmahdi W, Pawar R, Wolin MS. Oxidant mechanisms in renal injury and disease. Antioxid Redox Signal. (2016) 25:119–46. doi: 10.1089/ars.2016.6665
112. Dendooven A, Ishola DA Jr, Nguyen TQ, Van der Giezen DM, Kok RJ, Goldschmeding R, et al. Oxidative stress in obstructive nephropathy. Int J Exp Pathol. (2011) 92:202–10. doi: 10.1111/j.1365-2613.2010.00730.x
113. Liu B, Hu D, Zhou Y, Yu Y, Shen L, Long C, et al. Exosomes released by human umbilical cord mesenchymal stem cells protect against renal interstitial fibrosis through ROS-mediated P38MAPK/ERK signaling pathway. Am J Transl Res. (2020) 12:4998–5014.
114. Ji C, Zhang J, Zhu Y, Shi H, Yin S, Sun F, et al. Exosomes derived from hucMSC attenuate renal fibrosis through CK1delta/beta-TRCP-mediated YAP degradation. Cell death Dis. (2020) 11:327. doi: 10.1038/s41419-020-2510-4
115. Kumar S, Liu J, Pang P, Krautzberger AM, Reginensi A, Akiyama H, et al. Sox9 activation highlights a cellular pathway of renal repair in the acutely injured mammalian kidney. Cell Rep. (2015) 12:1325–38. doi: 10.1016/j.celrep.2015.07.034
116. Zhu F, Chong Lee Shin OLS, Pei G, Hu Z, Yang J, Zhu H, et al. Adipose-derived mesenchymal stem cells employed exosomes to attenuate AKI-CKD transition through tubular epithelial cell dependent Sox9 activation. Oncotarget. (2017) 8:70707–26. doi: 10.18632/oncotarget.19979
117. Gregorini M, Corradetti V, Pattonieri EF, Rocca C, Milanesi S, Peloso A, et al. Perfusion of isolated rat kidney with mesenchymal stromal cells/extracellular vesicles prevents ischaemic injury. J Cell Mol Med. (2017) 21:3381–93. doi: 10.1111/jcmm.13249
118. Ishiy C, Ormanji MS, Maquigussa E, Ribeiro RS, da Silva Novaes A, Boim MA. Comparison of the effects of mesenchymal stem cells with their extracellular vesicles on the treatment of kidney damage induced by chronic renal artery stenosis. Stem Cells Int. (2020) 2020:8814574. doi: 10.1155/2020/8814574
119. Du T, Zou X, Cheng J, Wu S, Zhong L, Ju G, et al. Human Wharton’s jelly-derived mesenchymal stromal cells reduce renal fibrosis through induction of native and foreign hepatocyte growth factor synthesis in injured tubular epithelial cells. Stem Cell Res Ther. (2013) 4:59. doi: 10.1186/scrt215
120. Wang B, Yao K, Huuskes BM, Shen HH, Zhuang J, Godson C, et al. Mesenchymal stem cells deliver exogenous microRNA-let7c via exosomes to attenuate renal fibrosis. Mol Ther. (2016) 24:1290–301. doi: 10.1038/mt.2016.90
121. Jin J, Qian F, Zheng D, He W, Gong J, He Q. Mesenchymal stem cells attenuate renal fibrosis via exosomes-mediated delivery of microRNA Let-7i-5p antagomir. Int J Nanomed. (2021) 16:3565–78. doi: 10.2147/ijn.s299969
122. Åkerud P, Canals JM, Snyder EY, Arenas E. Neuroprotection through delivery of glial cell line-derived neurotrophic factor by neural stem cells in a mouse model of Parkinson’s disease. J Neurosci. (2001) 21:8108–18. doi: 10.1523/jneurosci.21-20-08108.2001
123. Sun S, Zhang Q, Li M, Gao P, Huang K, Beejadhursing R, et al. GDNF promotes survival and therapeutic efficacy of human adipose-derived mesenchymal stem cells in a mouse model of Parkinson’s disease. Cell Transpl. (2020) 29:0963689720908512. doi: 10.1177/0963689720908512
124. Chen L, Wang Y, Li S, Zuo B, Zhang X, Wang F, et al. Exosomes derived from GDNF-modified human adipose mesenchymal stem cells ameliorate peritubular capillary loss in tubulointerstitial fibrosis by activating the SIRT1/eNOS signaling pathway. Theranostics. (2020) 10:9425–42. doi: 10.7150/thno.43315
125. Larson BL, Ylostalo J, Lee RH, Gregory C, Prockop DJ. Sox11 is expressed in early progenitor human multipotent stromal cells and decreases with extensive expansion of the cells. Tissue Eng Part A. (2010) 16:3385–94. doi: 10.1089/ten.tea.2010.0085
126. Zhang ZY, Hou YP, Zou XY, Xing XY, Ju GQ, Zhong L, et al. Oct-4 enhanced the therapeutic effects of mesenchymal stem cell-derived extracellular vesicles in acute kidney injury. Kidney Blood Press Res. (2020) 45:95–108. doi: 10.1159/000504368
127. Tian K, Chen P, Liu Z, Si S, Zhang Q, Mou Y, et al. Sirtuin 6 inhibits epithelial to mesenchymal transition during idiopathic pulmonary fibrosis via inactivating TGF-β1/Smad3 signaling. Oncotarget. (2017) 8:61011–24. doi: 10.18632/oncotarget.17723
128. Cai J, Liu Z, Huang X, Shu S, Hu X, Zheng M, et al. The deacetylase sirtuin 6 protects against kidney fibrosis by epigenetically blocking β-catenin target gene expression. Kidney Int. (2020) 97:106–18. doi: 10.1016/j.kint.2019.08.028
129. Liu L, Wu Y, Wang P, Shi M, Wang J, Ma H, et al. PSC-MSC-derived exosomes protect against kidney fibrosis in vivo and in vitro through the SIRT6/β-catenin signaling pathway. Int J Stem Cells. (2021) 14:310–9. doi: 10.15283/ijsc20184
130. Maidhof W, Hilas O. Lupus: an overview of the disease and management options. P T. (2012) 37:240–9.
131. Anders HJ, Saxena R, Zhao MH, Parodis I, Salmon JE, Mohan C. Lupus nephritis. Nat Rev Dis Primers. (2020) 6:7. doi: 10.1038/s41572-019-0141-9
132. Yang X, Yang J, Li X, Ma W, Zou H. Bone marrow-derived mesenchymal stem cells inhibit T follicular helper cell in lupus-prone mice. Lupus. (2018) 27:49–59. doi: 10.1177/0961203317711013
133. Lee HK, Kim KH, Kim HS, Kim JS, Lee JH, Ji A, et al. Effect of a combination of prednisone or mycophenolate mofetil and mesenchymal stem cells on lupus symptoms in MRL.Fas(lpr) mice. Stem Cells Int. (2018) 2018:4273107. doi: 10.1155/2018/4273107
134. Gu F, Wang D, Zhang H, Feng X, Gilkeson GS, Shi S, et al. Allogeneic mesenchymal stem cell transplantation for lupus nephritis patients refractory to conventional therapy. Clin Rheumatol. (2014) 33:1611–9. doi: 10.1007/s10067-014-2754-4
135. Shen B, Liu J, Zhang F, Wang Y, Qin Y, Zhou Z, et al. CCR2 Positive exosome released by mesenchymal stem cells suppresses macrophage functions and alleviates ischemia/reperfusion-induced renal injury. Stem Cells Int. (2016) 2016:1240301. doi: 10.1155/2016/1240301
136. Fatima F, Ekstrom K, Nazarenko I, Maugeri M, Valadi H, Hill AF, et al. Non-coding RNAs in mesenchymal stem cell-derived extracellular vesicles: deciphering regulatory roles in stem cell potency, inflammatory resolve, and tissue regeneration. Front Genet. (2017) 8:161. doi: 10.3389/fgene.2017.00161
137. Wei S, Zhang Z, Yan L, Mo Y, Qiu X, Mi X, et al. miR-20a overexpression in adipose-derived mesenchymal stem cells promotes therapeutic efficacy in murine lupus nephritis by regulating autophagy. Stem Cells Int. (2021) 2021:3746335. doi: 10.1155/2021/3746335
138. Chen X, Wei Q, Sun H, Zhang X, Yang C, Tao Y, et al. Exosomes derived from human umbilical cord mesenchymal stem cells regulate macrophage polarization to attenuate systemic lupus erythematosus-associated diffuse alveolar hemorrhage in mice. Int J Stem Cells. (2021) 14:331–40. doi: 10.15283/ijsc20156
139. Correa RR, Juncosa EM, Masereeuw R, Lindoso RS. Extracellular vesicles as a therapeutic tool for kidney disease: current advances and perspectives. Int J Mol Sci. (2021) 22:5787. doi: 10.3390/ijms22115787
140. Busatto S, Vilanilam G, Ticer T, Lin WL, Dickson DW, Shapiro S, et al. Tangential flow filtration for highly efficient concentration of extracellular vesicles from large volumes of fluid. Cells. (2018) 7:273. doi: 10.3390/cells7120273
Keywords: exosome, chronic kidney disease, mesenchymal stem cells, therapy, new advances, regeneration
Citation: Cao Q, Huang C, Chen X-M and Pollock CA (2022) Mesenchymal Stem Cell-Derived Exosomes: Toward Cell-Free Therapeutic Strategies in Chronic Kidney Disease. Front. Med. 9:816656. doi: 10.3389/fmed.2022.816656
Received: 17 November 2021; Accepted: 24 February 2022;
Published: 21 March 2022.
Edited by:
Maik Gollasch, Charité Universitätsmedizin Berlin, GermanyReviewed by:
Mario Ollero, INSERM U955 Institut Mondor de Recherche Biomédicale (IMRB), FranceTeresa Rampino, San Matteo Hospital Foundation (IRCCS), Italy
Copyright © 2022 Cao, Huang, Chen and Pollock. This is an open-access article distributed under the terms of the Creative Commons Attribution License (CC BY). The use, distribution or reproduction in other forums is permitted, provided the original author(s) and the copyright owner(s) are credited and that the original publication in this journal is cited, in accordance with accepted academic practice. No use, distribution or reproduction is permitted which does not comply with these terms.
*Correspondence: Carol A. Pollock, Y2Fyb2wucG9sbG9ja0BzeWRuZXkuZWR1LmF1