- 1Department of Plastic and Burn Surgery, West China Hospital, Sichuan University, Chengdu, China
- 2Department of Central Sterile Supply, West China Hospital, Sichuan University, Chengdu, China
Large skin defects caused by burns, unhealing chronic wounds, and trauma, are still an intractable problem for clinicians and researchers. Ideal skin regeneration includes several intricate and dynamic stages of wound repair and regeneration of skin physiological function. Adipose-derived stem cells (ADSCs), a type of mesenchymal stem cells (MSCs) with abundant resources and micro-invasive extraction protocols, have been reported to participate in each stage of promoting skin regeneration via paracrine effects. As essential products secreted by ADSCs, extracellular vesicles (EVs) derived from ADSCs (ADSC-EVs) inherit such therapeutic potential. However, ADSC-EVs showed much more clinical superiorities than parental cells. ADSC-EVs carry various mRNAs, non-coding RNAs, proteins, and lipids to regulate the activities of recipient cells and eventually accelerate skin regeneration. The beneficial role of ADSCs in wound repair has been widely accepted, while a deep comprehension of the mechanisms of ADSC-EVs in skin regeneration remains unclear. In this review, we provided a basic profile of ADSC-EVs. Moreover, we summarized the latest mechanisms of ADSC-EVs on skin regeneration from the aspects of inflammation, angiogenesis, cell proliferation, extracellular matrix (ECM) remodeling, autophagy, and oxidative stress. Hair follicle regeneration and skin barrier repair stimulated by ADSC-EVs were also reviewed. The challenges and prospects of ADSC-EVs-based therapies were discussed at the end of this review.
Introduction
The skin, the largest organ of the human body, protects the body from exogenous irritation and pathogen invasion as the first barrier between organisms and the environment. Skin damage caused by diseases or trauma threatens the defensive function, leading to the suffering of patients and the burden of public health care (1, 2). Repair of skin, including both structural integrity and physiological function, is essential to maintain its protective property, which remains intractable for clinicians and researchers. Stem cells have been reported to possess considerable potential for skin regeneration through multiple mechanisms (3–5). Adipose-derived stem cells (ADSCs) are a promising type of mesenchymal stem cells (MSCs) for skin regeneration, with abundant resources among human tissue and minimally invasive extraction protocols. However, some severe complications impact the application of stem cells since they are large and sticky, such as elevation in pulmonary arterial pressure or even vascular embolism, along with potential oncogenesis and ethical issues (6, 7). Moreover, some properties of stem cells might impair the beneficial effect of stem cells. For example, restricted delivery of stem cells and uncertain differentiation to proinflammatory or anti-inflammatory phenotype of stem cells in inflammatory conditions might lead to no benefit or even negative effect in the treatment of acute kidney injury after cardiac surgery (8).
Extracellular vesicles (EVs) are natural particles with a phospholipid bilayer membrane secreted by almost all types of cells during vital activities (9). Transferring proteins, nucleic acids, and lipids to recipient cells, EVs derived from stem cells have been deemed to be intercellular communicators and functional executors (10, 11). Compared to stem cells, EVs possess more advantages for clinical application. EVs are safer owing to their smaller size and non-tumorigenic. Because EVs show no immunogenicity and can be stored at −80°C, they are available once patients need, avoiding waiting for cell culture as autologous stem cells therapy (12). In recent years, considerable research efforts on EVs derived from ADSCs (ADSC-EVs) have indicated that ADSC-EVs have a positive impact on skin regeneration, similar to their parental cells. Moreover, ADSC-EVs manifested a superior impact on wound healing than EVs derived from other stem cells, which might be due to their robust angiogenic effect (13). ADSC-EVs might accelerate skin wound repair by participating in inflammation, angiogenesis, cell proliferation, and extracellular matrix (ECM) remodeling (14, 15), regulating cell apoptosis and autophagy (16, 17), and relieving oxidative stress in the wound microenvironment (18). The regeneration of skin appendages and recovery of physiological functions are also promoted by ADSC-EVs (19, 20), which is essential for ideal skin regeneration. Current documents have summarized the promoting effects of ADSC-EVs in skin regeneration, mostly focusing on mechanisms in wound healing but hardly with functional repair involved. In this review, the profile of ADSC-EVs, mechanisms in the promotion of skin regeneration, and potential for clinical applications are discussed (Figure 1). The existing challenges and prospects of ADSC-EVs in regenerative medicine are also discussed here. We hope this work replenishes current comprehension of how ADSC-EVs generate and work, and provides potential inspiration for future research on regenerative medicine.
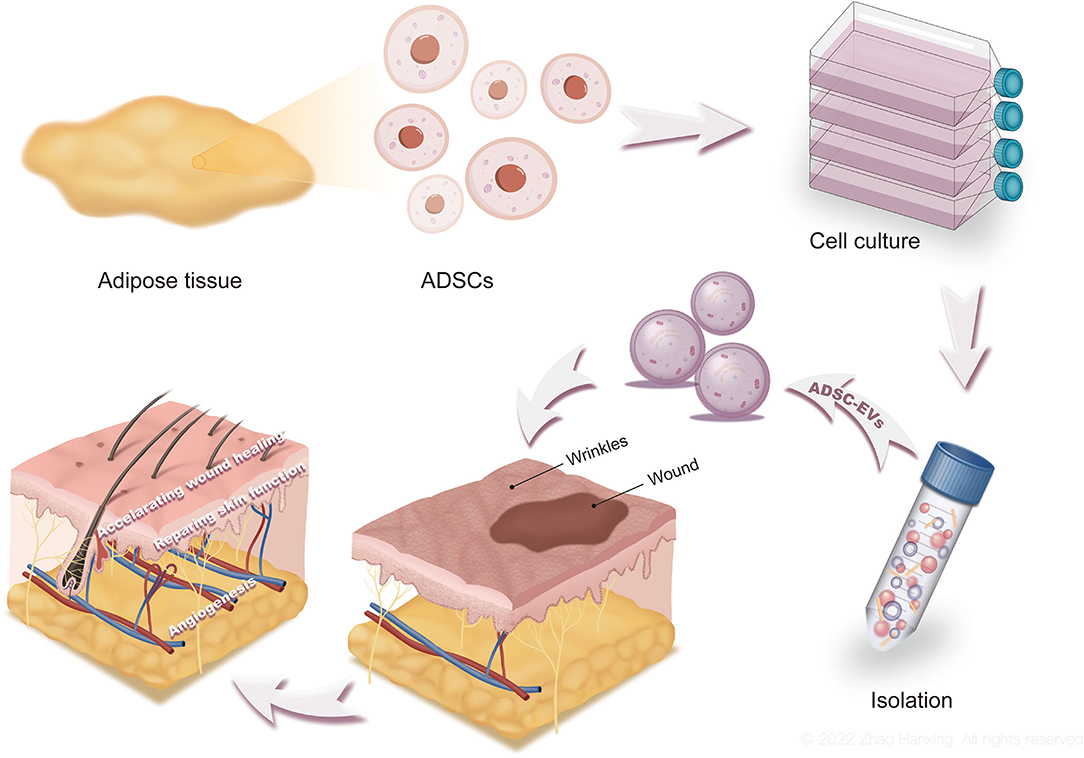
Figure 1. The production, functions, and applications of ADSC-EVs. Culture medium of ADSCs was collected and processed to obtain ADSC-EVs. By accelerating wound healing and repairing skin function, ADSC-EVs promote skin regeneration. ADSC-EVs are promising for clinical applications as well.
Extracellular Vesicle From Adipose-Derived Stem Cells
ADSCs are a subtype of MSCs isolated from adipose tissues with self-renewal and multiple differentiation properties. Through paracrine of a variety of cytokines, ADSCs are known as powerful therapeutics utilized in regenerative medicine (21, 22). In addition to direct secretion, ADSC-EVs have been reported to be functional executors of ADSCs (23). As products of ADSCs during biological activities, ADSC-EVs encapsulate cargoes, including DNA, RNA, proteins, and lipids produced by ADSCs, acting as intercellular communicators and biomolecule transporters (24).
Classification and Biogenesis of EVs
EVs are a generic term for particles with lipid bilayer membranes released by cells in natural activities and are divided into three main subtypes based on the current understanding of their biogenesis, size, and content: exosomes (<150 nm in size), microvesicles (up to 1,000 nm), and apoptotic bodies (more than 1,000 nm) (25–27). Exosomes and microvesicles seem to be generated by almost all types of viable cells (28) and are major subtypes of EVs studied in regenerative medicine to date. Apoptotic bodies, the relatively larger group in size, are products of cell apoptosis and encapsulate contents of cells disassembly. The biogenesis of the three main EV-subtypes is shown here (Figure 2).
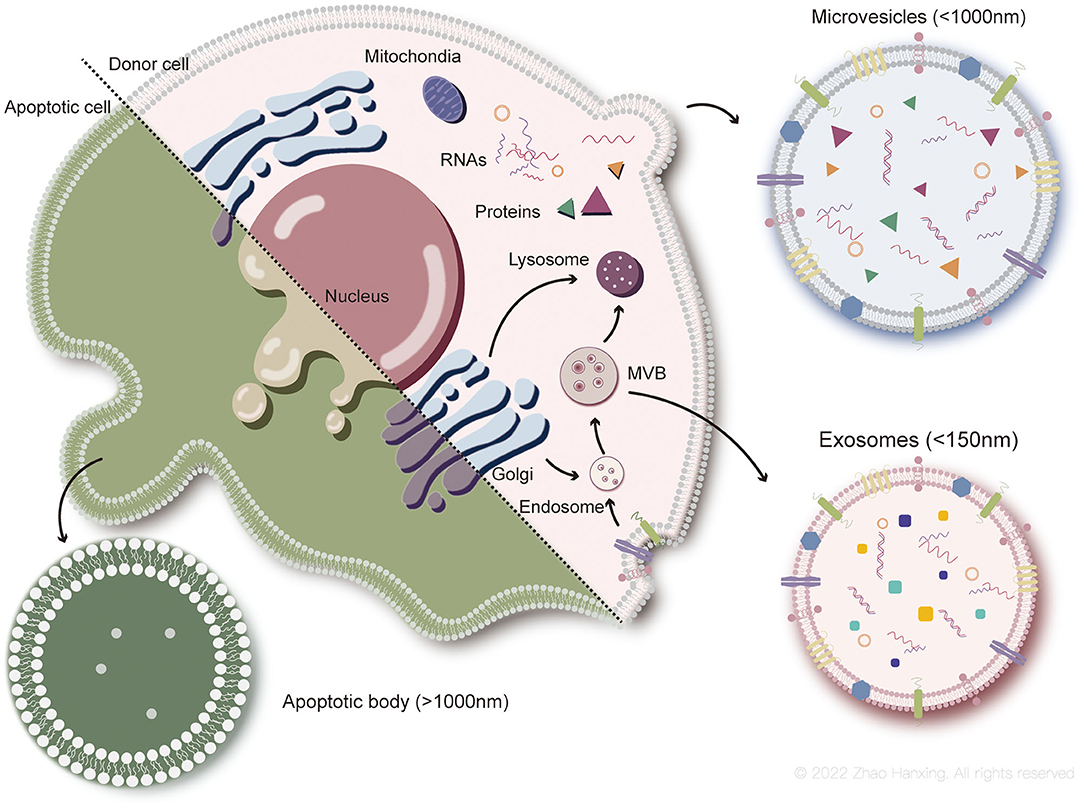
Figure 2. Biogenesis of each subtype of EVs. Exosomes (<150 nm in size) originate from endosome. Microvesicles (up to 1,000 nm) generate from plasma membrane. Apoptotic bodies (more than 1,000 nm) are particles of apoptotic cells disassembly.
The biogenesis of exosomes is a complex process. First, endocytosis of the plasma membrane forms the early sorting endosomes, carrying surface proteins and lipids. Early endosomes subsequently transform to late endosomes under interaction with the Golgi complex (28). Exosomes originate from the inward budding of the endosomal membrane as intraluminal vesicles (IVLs) during maturation of multivesicular endosomes (MVBs), which then enter lysosomes for degradation, or fuse with the plasma membrane to be released as exosomes (27). Exosomal membrane components are derived from plasma or the Golgi complex before the formation of IVLs in endosomes. The cargoes sorted into exosomes are heavily dependent on endosomal sorting mechanisms, which involve the endosomal sorting complex required for transport (ESCRT) proteins (29). ESCRT-independent mechanisms have also been demonstrated, including ceramide and its metabolites and tetraspanin family members (30–32).
Microvesicles released by healthy cells originate from the outward budding of the plasma membrane. Phospholipids on the plasma membrane rearrange with activation of scramblase by the inflow of Ca2+, in which phosphatidylserine flips from the inner leaflet of the bilayer to the outer leaflet. Consequently, budding of the plasma membrane and degradation of the cytoskeleton occur, forming microvesicles (33). However, biogenesis of microvesicles can proceed without the rearrangement of phospholipids (34), indicating that other mechanisms may also be involved, such as the participation of cholesterol-rich lipid rafts (35).
Although the destinies differ between parental cells of apoptotic bodies and microvesicles, the formation of the two subtypes of EVs involves similar changes in the plasma membrane. Biogenesis of apoptotic bodies is described as three sequential well-coordinated steps with corresponding morphological changes: plasma membrane blebbing, thin membrane protrusion formation, and fragmentation (36). During blebbing, phosphatidylserine flips from the inner layer of the plasma membrane to the outer layer, which is induced by caspase-activated scramblase (37). Compared to the former two subtypes, cargoes of apoptotic bodies tend to include intact organelles, chromatin, and higher levels of histones (38). However, recent studies have also shown that some organelles might be enclosed by microvesicles (39). Information on cargoes in EVs needs to be enriched with further research.
Biomarkers of EVs include molecules involved in their biogenesis, such as transmembrane proteins anchored to the plasma membrane or endosomal membrane and cytosolic proteins (27). Non-EV proteins co-isolated with EVs are detected to assess the purity of EVs, such as apolipoproteins A1/2 and B, and albumin (26). According to the biogenesis of exosomes, their biomarkers are conventionally deemed to be ESCRT-associated proteins (Alix, TSG101, Syntenin, and HSC70), tetraspanin family proteins (CD9, CD63, and CD81), and major histocompatibility complex (MHC) class I and class II proteins. However, some of these proteins have also been demonstrated to be contained in other subtypes, such as flotillin-1, HSC70, and MHC class I and II proteins (40). Microvesicles derived from the plasma membrane mainly contain proteins present in the cytoplasm and plasma membrane, especially post-translational modified proteins (41). Apoptotic bodies contain high levels of apoptosis-associated proteins, such as cleaved caspase-3, C1q, and nuclear debris (42). Nevertheless, overlapping biomarkers exist among each group of EVs, making it imprecise for identification. In addition, isolation methods used in current studies, especially for microvesicles and apoptotic bodies, are mainly centrifugation based on the size and density of EVs, which leads to groups overlapping on the very edge of the size scale.
Exosomes and microvesicles transfer information and therapeutic molecules from viable cells to recipient cells (43), rendering them potential options for investigation in regenerative medicine. However, from the orthodox perspective, apoptotic bodies tend to be cell debris responsible for the clearance of dying cells (44). Moreover, cargoes distributed into apoptotic bodies vary in quantity and component (45, 46), along with a relatively large size scale, impeding the identification and mechanical exploration of apoptotic bodies. Hence, studies have paid more attention to the mechanisms and applications of the former two subtypes of EVs. In this review, our discussion of ADSC-EVs is mainly based on exosomes and microvesicles derived from ADSCs.
Isolation and Characterization of EVs
In the past few decades, researchers have isolated EVs by several common strategies (Figure 3). The traditional method is centrifugation, which is based on the density of different groups of EVs, allowing denser particles to sediment out first. Differential ultracentrifugation (DC) is the most frequently used method and is still the “gold standard” for the isolation of EVs (26). Density gradient centrifugation (DGC) is an improved ultracentrifugation method that produces EVs with higher purity, in which a prepared density gradient generally formed by sucrose or iodixanol is required. EVs pass through a gradient with increasing density from top to bottom in the DGC system, and then each subtype of EVs is separated into perspective layers with different densities. Common methods based on the size of EVs include ultrafiltration (UF) and size exclusion chromatography (SEC) (28). In UF, the target group of EVs passes through the filtration membrane with a certain molecular weight cut off (MWCO) while larger particles are retained. In SEC, EVs with different sizes pass through the column filled with porous polymer microspheres that allows smaller particles to penetrate. Routes in those pores take more time for smaller EVs to elute than larger EVs. Immunoaffinity capture (IC) technology relies on the binding between antigens on the surface of EVs and antibodies attached to the surface of tools, such as magnetic beads or plates. IC allows the isolation of EVs originating from a specific source with certain surface proteins. Polymer precipitation (PP), typically polyethylene glycol base, takes advantage of strong hydrophilicity to “grab” the water molecules in the solution, rendering EVs “dehydrated” to aggregate (41). Commercial isolation kits with various strategies described above have also been used in research. However, each method possesses its shortcomings. A large initial volume is required for DC and a long DC duration increases the risk of structural damage to EVs and protein contamination. DGC is associated with extra preparation and a low yield of EVs. When EVs pass through the filter membrane, the pores might be blocked, causing low yield, and the shear force leads to deformation and lysis of EVs. SEC and IC cannot process a large volume of solution. EVs isolated by PP tend to be contaminated by polymers and proteins (41). The isolation method, which is linked to the purity of EVs, is suggested to be chosen according to different research purposes (26).
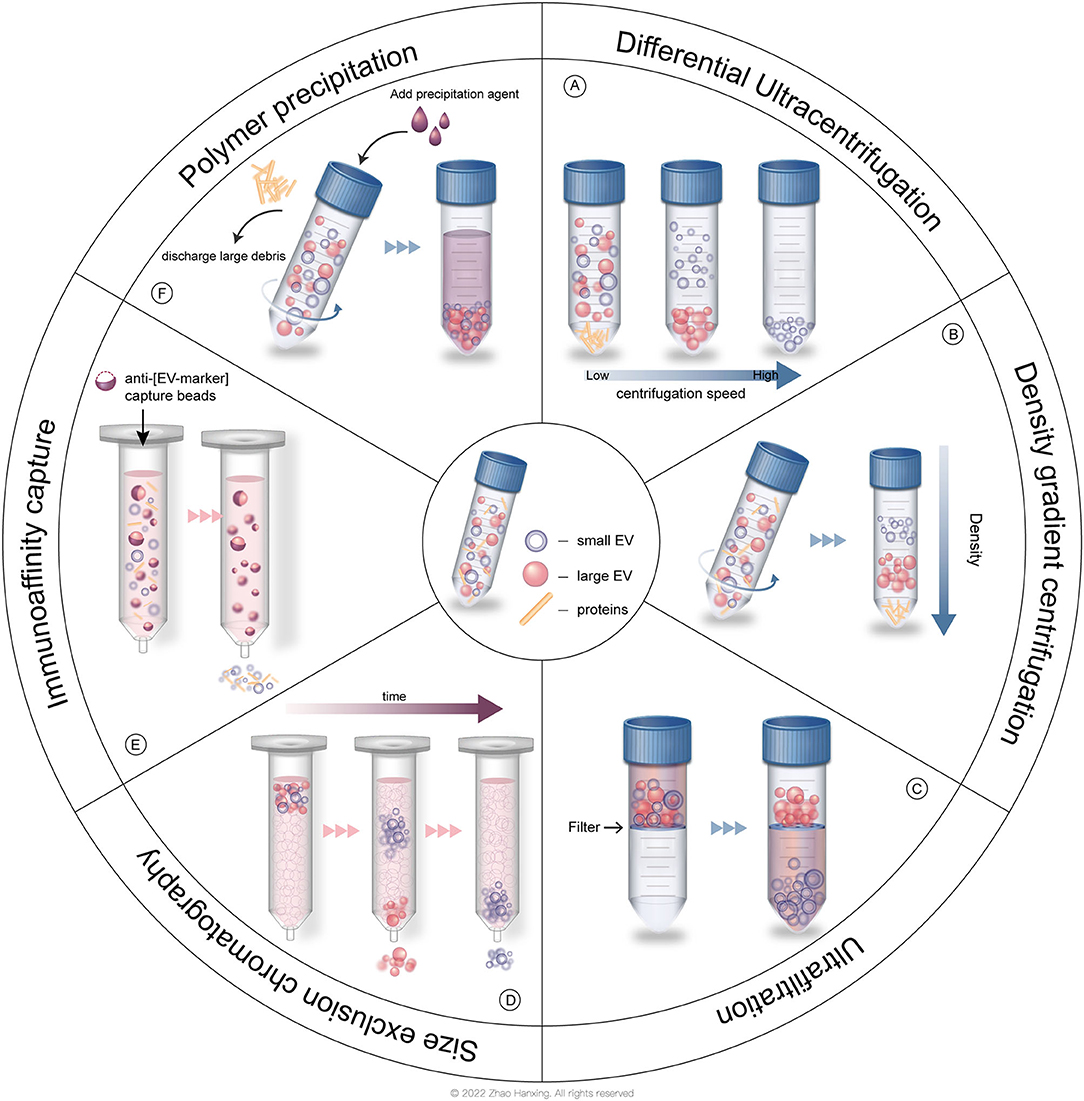
Figure 3. Common isolation strategies of EVs. (A) Differential ultracentrifugation (DC). (B) Density gradient centrifugation (DGC). (C) Ultrafiltration (UF). (D) Size exclusion chromatography (SEC). (E) Immunoaffinity capture (IC). (F) Polymer precipitation (PP).
With the boosted development of technology, novel strategies have been developed to isolate EVs efficiently. Microfluidic techniques allow the isolation of EVs considering their physical and biochemical properties simultaneously. Acoustic (47), electrical (48), and electromagnetic field forces (49) can be addressed inside microfluidic devices to isolate EVs, along with immuno-based (50) and asymmetric flow field flow-based (51) microfluidic techniques. While this technology is still developing, the efficiency, simplicity, and low initial volume of the sample render it a promising method for future clinical application. Other methods of large-scale EV production include the use of bioreactor culture of parental cells, in which the characteristics of EVs need to be clarified with the culture condition in the bioreactor (52). Shear stress and cell extrusion can scale up EV-like vesicles production, while the purity of vesicles is relatively poor (53). Hydrostatic filtration dialysis and cytochalasin B induced vesicles have also been reported to improve EVs yield (54, 55). Immortalization of MSCs is also a potential strategy, along with increased safety concerns (56).
For the characterization of EVs, each subtype has been identified by some detection strategies based on their morphology, size, biomarkers of surface, and contents. The morphology of EVs is frequently observed by scanning electron microscopy and transmission electron microscopy (26). Electron cryo-microscopy and atomic force microscopy are also used (28). The size distribution and concentration of EVs are usually detected by nanoparticle tracking analysis and dynamic light scattering, and tunable resistive pulse sensing (57). Some new technologies, such as light microscopic single EV analysis, have been utilized to analyze the properties of a single EV (58). Common biochemical analysis methods of EVs include western blotting, flow cytometry, and liquid chromatography and mass spectrometry. In recent years, new technologies for EVs analysis have emerged, including small particle flow cytometry, micronuclear magnetic resonance, and thermophoretic profiling (33).
Mechanisms by Which ADSC-EVs Play a Therapeutic Role in Skin Regeneration
Recent studies have demonstrated the beneficial role of ADSC-EVs in multiple tissue regeneration, such as skin (59), tendon (60), bone (61), and nerve tissue (62). ADSC-EVs exert a regenerative effect by delivering signals to cells with single or coordinated actions of biomolecules. Wound healing is one of the dominant components of skin regeneration and consists of several intricate and dynamic processes: hemostasis, inflammation, proliferation, and remodeling (63). ADSC-EVs participate in each process of wound healing, along with the regeneration of skin physiological functions to achieve ideal skin regeneration. The comprehensive abstract of current understandings of ADSC-EVs functioning in skin regeneration is shown in Figure 4.
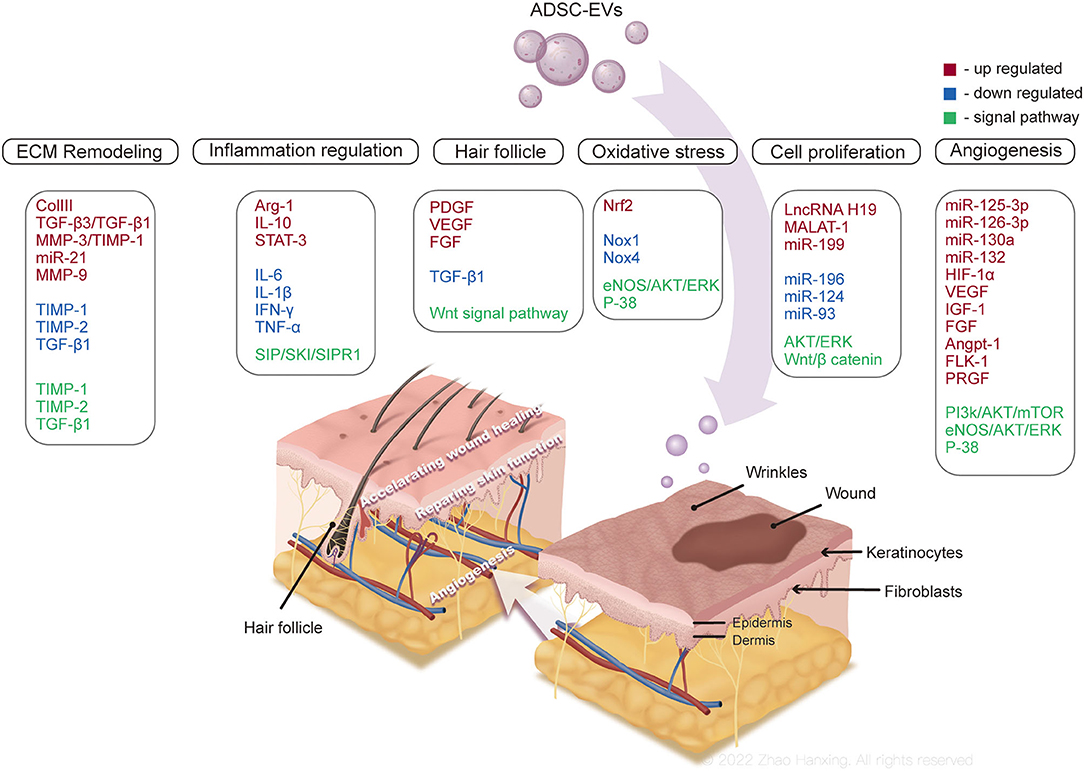
Figure 4. Mechanisms of ADSC-EVs promoting skin regeneration. ADSC-EVs might accelerate skin wound repair by participate in inflammation, angiogenesis, cell proliferation, and extracellular matrix (ECM) remodeling, regulating cell apoptosis and autophagy, and relieving oxidative stress in wound microenvironment. The regeneration of skin appendages and physiological functions are also promoted by ADSC-EVs.
Promote Wound Healing
Inflammation Regulation
In the process of ideal wound healing, the immune system is supposed to defend against the invasion of pathogens by a moderate inflammatory response. When immune homeostasis is compromised, excess and persistent inflammation contributes to impaired wound healing, such as in chronic diabetic wounds (64). ADSC-EVs improve the inflammatory microenvironment at wound sites by regulating the activities of immune cells, thereby accelerating wound repair.
ADSC-EVs can regulate the balance between CD4 T cell subsets (65) and inhibit the proliferation of T cells and the release of inflammatory factor IFN-γ (66). The engulfment of ADSC-EVs by macrophages and subsequent increased expression of Arg-1 and IL-10, soluble markers of anti-inflammatory phenotype macrophages (M2) (67), were observed in obese mice. Mechanically, ADSC-EVs induced transactivation of Arg-1 in macrophages by transferring signal transducer and activator of transcription 3 (68). Additionally, the polarization of M2 macrophages after treatment with ADSC-EVs was found to be associated with the S1P/SK1/S1PR1 signaling pathway, along with reduced expression of inflammatory cytokines IL-6, IL-1β, IFN-γ, and TNF-α (69).
The immunosuppressive activity of ADSC-EVs regulates inflammation in skin wounds, too. Interestingly, another study demonstrated that ADSC-EVs attenuated the polarization of inflammatory M1 macrophages, while hardly inducing M2 polarization (70). Although clarification of this controversy needs to be addressed in further investigation, both inhibited M1 polarization and increased M2 polarization led to improved inflammation. In addition, treatment of ADSC-EVs in ischemia-reperfusion skin flaps resulted in less infiltration of inflammatory cells and ameliorated apoptosis (71).
Current studies have demonstrated the effects of ADSC-EVs as mediators in the inflammatory response, in which the inhibition of inflammation dominates, promoting wound repair. However, it is noteworthy that a necessary inflammatory response also contributes to the protection of the wound microenvironment (72). In the early stage of using ADSC-EVs, they were indicated to cause inflammation, whereas exert pro-adipogenic function and promote collagen synthesis in the late stage (73). From this perspective, homeostasis of inflammation at wound sites needs to be considered in both research and application of ADSC-EVs, rather than absolute inhibition of inflammation.
Angiogenesis
Angiogenesis involves multiple cytokines and intricate signaling pathways, which are essential for the supply of oxygen and nutrients as skin wounds heal. ADSC-EVs have been documented to trigger angiogenesis by transferring contained proangiogenic mediators. Neovascularization of human umbilical vein endothelial cells (HUVECs) was promoted with the treatment of ADSC-EVs, which was more robust when miR-126-3p was overexpressed (74). Other microRNAs carried by ADSC-EVs were also reported to participate in angiogenesis, such as miR-126, miR-130a, and miR-132 (75). Additionally, miR-125a-3p from ADSC-EVs enhanced angiogenesis of HUVECs by activating the PI3K/AKT signaling pathway, targeting the PTEN gene (76). Another study indicated that ADSC-EVs also contributed to angiogenesis in the diabetes microenvironment by increasing HIF-1α and VEGF expression through the PI3K/AKT/mTOR signaling pathway (77). The angiogenic potential of ADSC-EVs might be enhanced in the specific microenvironment. Under hypoxia conditions, ADSC-EVs encapsulated more proangiogenic growth factors, including IGF-1, FGF, VEGF and their receptors (17), angiopoietin-1, and fetal liver kinase-1 (78), and demonstrated more prominent neovascularization and faster wound repair. With the stimulus of PDGF, secretion of ADSC-EVs increased, along with upregulated c-kit and SCF (79). The c-kit is a tyrosine kinase receptor that regulates the differentiation of progenitor cells to blood or vascular endothelial cells. C-kit ligand SCF is a kind of stem cell regulator that plays an important role in angiogenesis and recruitment of MSCs (80). EVs released by ADSCs overexpressing glyoxalase-1 (GLO-1) promoted capillary growth compared with normal ADEC-EVs under high-glucose conditions by upregulating the eNOS/AKT/ERK/P-38 signaling pathway, which regulates the proliferation and migration of HUVECs (17).
ADSC-EVs facilitate angiogenesis by providing cargos that participate in capillary growth or activate angiogenic signaling pathways, thereby promoting wound healing. However, newly formed capillaries are supposed to degrade to form an appropriate vascular density similar to normal skin at the late stage of wound healing without scarring (81). In wound repair, further experiments are necessary to figure out optimal administration time and quantity of ADSC-EVs, avoiding potential side effects, such as scar formation out of excessive angiogenesis caused by the overdose of ADSC-EVs.
Cell Proliferation
During the proliferative phase of wound healing, epithelialization occurs mainly by proliferating and migrating to the wound site of epithelial cells. Fibroblasts are activated to proliferate and produce ECM to repair the defect. ADSC-EVs can be engulfed by human skin fibroblasts (HSFs) and HaCaT keratinocytes, promoting subsequent proliferation and migration (74, 82) in a dose-dependent manner (83). After the uptake of ADSC-EVs, the cell cycle of HSF was stimulated to accelerate re-epithelialization (82, 83) by the activation of AKT and ERK signaling pathways (82). Intriguingly but predictably, the Wnt/β-catenin signaling pathway, which participates closely in cell growth and renewal, was also involved in the proliferative effect of ADSC-EVs (84, 85). Long non-coding RNA (lncRNA) H19 in ADSC-EVs combined with miR-19b and inhibited its expression, targeting SRY-related high-mobility-group box 9, thus promoting wound healing (84). ADSC-EVs promote the proliferation and migration of human dermal fibroblasts (HDFs) and HaCaT keratinocytes by lncRNA MALAT-1 targeting miR-124 (85). Additionally, boosted proliferation and migration of HDFs were induced by upregulated miR-199 and downregulated miR-93 contained in ADSC-EVs (86). Finally, ADSC-EVs-treated M2 macrophages contributed to the proliferation and self-renewal of ADSCs (68).
Extracellular Matrix Remodeling
The synthesis and remodeling of ECM affect the formation of hypertrophic scars and the time of wound healing. Scar, which is composed of ECM, mostly collagen I (87), provides temporary strength to injured skin and will be degraded by matrix metalloproteinases (MMPs) gradually in the wound healing process (88). ADSC-EVs have been demonstrated to regulate the process of ECM remodeling. With the treatment of ADSC-EVs, deposition of collagen I and III with a well-organized histological structure increased at the wound site, along with the regeneration of skin appendages (89, 90). ADSC-EVs facilitated ECM remodeling during wound repair by upregulating the ratio of collagen III/I, TGF-β3/TGF-β1, and MMP-3/tissue inhibitors of MMP-1 (TIMP-1). The differentiation of fibroblasts to myofibroblasts that contribute to scarring was also inhibited by ADSC-EVs (91). Overexpressed miR-21 in ADSC-EVs promoted the expression of MMP-9 and MMP-3 and suppressed that of TIMP-1, TIMP-2 and TGF-β1 by activating the PI3K/AKT signaling pathway to restrain scar formation (92). Although persistent high MMPs levels indicated extra ECM degradation and poor prognosis in diabetic wounds (93), ADSC-EVs boosted the deposition of collagen, which is essential for ECM formation in skin wounds, exerting a positive effect on ECM remodeling (94). In the mouse model, ADSC-EVs were reported to increase the synthesis of collagen to accelerate wound repair, which was inhibited in the late stage to reduce scarring (83). However, with the treatment of ADSC-EVs, HSF produced less collagen I, collagen III, and α-SMA, which differed from other studies, although they all ameliorated scar formation (95). This controversial result might be because that HSF in this study was isolated from hypertrophic scar tissue, in which HSF remained persistently hyperactive (96) while other studies utilized cells from normal skin tissue.
Autophagy and Apoptosis Modulation
From the orthodox perspective, autophagy and apoptosis were merely considered to be essential parts during skin wound healing. However, some studies have documented that ADSC-EVs promote wound repair with autophagy and apoptosis involved in the microenvironment of the wound site. Overexpressed circular RNA mmu_circ_0000250 in ADSC-EVs suppressed the expression of miR-128-3p in endothelial progenitor cells (EPCs), hence activating autophagy of EPCs and attenuating apoptosis of skin tissue (16). Moderate autophagy has been demonstrated to augment angiogenesis by recovering the function of EPCs (97), thus promoting wound healing. ADSC-EVs also ameliorated apoptosis of skin cells under irritations, acting as a protective buffer in the wound microenvironment (17, 98). Interestingly, MSCs underwent remarkable apoptosis after transplantation in vivo, but they still exerted prominent therapeutic effects and prevented hypertrophic scar formation (99). ADSCs might participate in wound healing by secreting EVs in healthy conditions, but also transferring therapeutic information when they are dying. ADSC-EVs produced during apoptosis are potential functional parts, which need further investigations.
Oxidative Stress Relief
The generation of reactive oxygen species (ROS) in the processes of wound healing is required to defend against the invasion of pathogenic microbes and active cell survival signaling (100). Nevertheless, excessive ROS in the microenvironment of skin wounds causes oxidative damage and impaired wound healing. For example, in diabetic wounds, persistent high glucose level activates protein kinase C in smooth muscle and endothelial cells, increasing the activity of NDPH and the production of ROS, which leads to impairment of the viability of dermal fibroblasts and keratinocytes (64). After long-term exposure to high glucose, endothelial cells tend to reduce the secretion of vasoactive factor endothelial nitric oxide synthase (eNOS), resulting in restricted blood flow and difficult wound healing (101). ADSC-EVs have been investigated to exert protective effects in such an oxidative stress microenvironment, maintaining the biological activities of cells. ADSC-EVs relieved ROS damage in EPCs induced by high glucose via the reduced expression of oxidative stress-related proteins NOX1 and NOX4, which could be inhibited by the EV inhibitor GW4869 and enhanced by overexpression of nuclear factor erythroid 2-related factor 2 (102). When facing oxidative stress caused by hydrogen peroxide (H2O2), ADSC-EVs also maintained the viability and metabolic activity of keratinocytes and HSF (18), which might be attributed to lncRNA MALAT-1 contained in ADSC-EVs (85, 103). Additionally, EVs derived from ADSCs pretreated with H2O2 possessed a more prominent effect on oxidative stress relief and microvessel formation (71). Some studies have documented that pretreatment with H2O2 enables MSCs to produce higher levels of miR-21 to relieve cell death caused by oxidative stress (104, 105). Irritation seems to change the cargos of ADSC-EVs, thereby affecting their functions. GLO-1 is the rate-limiting enzyme in the glyoxalase system that plays an important role in the detoxification of advanced glycation end products (106, 107), accelerating the clearance of ROS in endothelial cells (108). After coculture with ADSC-EVs containing GLO-1, HUVECs accumulated less ROS and inflammatory cytokine IL-1β in a high glucose wound environment, with the involvement of the eNOS/AKT/ERK/P-38 signaling pathways (17).
Regeneration of Skin Physiological Function
The closure of skin wounds is not the end of perfect skin regeneration, in which the recovery of normal structure and physiological function are also important. The barrier function of the skin mainly relies on the external layer, the epidermis that lies openings of appendages (hair follicles, sweat glands, and sebaceous glands) and intercellular lipids (ceramides, filaggrin, and cholesterol) (109–111). Recent studies have demonstrated that ADSC-EVs function as positive regulators in recovering skin physiological function, at least partly.
Hair Follicle Regeneration
Hair deficiency is one of the major aesthetic complaints not only in patients with alopecia but also in those who have healed skin wounds without hair follicle regeneration. Moreover, skin with more hair follicles heals faster than that with less hair or without hair, which is due to the involvement of hair follicle stem cells in wound healing (112, 113). However, wound repair of adult mammals is likely to form scars without skin appendage regeneration (114). ADSC-EVs seemed to rescue hair regeneration in some way. In nude mice models, additional 50 μg/ml ADSC-EVs in experimental groups were grafted with dermal cells and epidermal cells to skin wounds, in which more hairs with normal structure and mature hair follicles were observed, along with higher expression of PDGF and VEGF and lower TGF-β1 expression in skin tissue (20). Although morphological observation cannot provide a detailed explanation mechanically, PDGF and VEGF are deemed to be growth regulators of hair follicles in the anagen phase (115). In addition, the reduced expression of TGF-β1 might contribute to hair maintenance, since it participates in the catagen phase of hair development and affects hair follicle apoptosis-associated molecules (116, 117). ADSC-EVs themselves also contain cytokines that stimulate hair follicle growth, including VEGF and FGF (17, 118), and enable the activation of the Wnt signaling pathway essential to hair follicle induction (85, 98, 119, 120). Even so, more research in this field is required to decipher the specific molecular mechanism of hair follicle regeneration promoted by ADSC-EVs. Current studies are largely based on rodent models, however, the structure of the skin and mechanisms of wound healing in humans and rodents are different.
Skin Barrier Repair
The epidermal barrier of the skin is supplied by stratum corneum (SC), which consists of corneocytes and an intercellular lipid mixture of ceramides, free fatty acids, and cholesterol (111). Among lipids in SC, ceramides are the dominant content, with weight over 50% (121), the defect of which is a critical part of etiology in atopic dermatitis (AD). Recently, ADSC-EVs have been indicated to promote skin barrier repair in AD mouse models. With the injection of ADSC-EVs, impaired SC hydration induced by oxazolone was normalized. Meanwhile, the quantity of long-chain dihydroceramide, a precursor component during de novo synthesis of ceramides, was significantly increased (19, 122). Predictably, enhanced synthesis of sphingosine-1-phosphate (S1P), a metabolite of ceramides, was also observed in this study. S1P has been reported to inhibit ceramide-associated apoptosis and stimulate the viability and differentiation of keratinocytes (123, 124). As mentioned before, ADSC-EVs activated the S1P / SK1 / S1PR1 signaling pathway to promote M2 macrophages polarization, thus attenuating inflammation (69), which is in accordance with the reduced inflammatory cytokines, such as TNF-α and IFN-γ (19). The lipid regenerative function of ADSC-EVs might be partly due to their regulatory role in inflammation. The particular actions of contents in ADSC-EVs helping skin barrier repair remain elusive, but ADSC-EVs may be a potential cell-free therapeutic approach for regeneration of skin barrier function.
Preclinical and Clinical Studies of ADSC-EVs for Skin Regeneration and Repair After Injury
Wound Healing
After long-term exploration, researchers gradually found the advantages and appropriate drug delivery methods of ADSC-EVs in skin regeneration and repair. Initially, the application of human fibrocyte-derived EVs in wound models of diabetic mice showed that all wound healing was significantly enhanced (125). EVs derived from other MSCs were also found to play a critical role in promoting re-epithelialization, collagen synthesis and angiogenesis in skin wound healing (126, 127). Considering that it would be easier to obtain autologous ADSC when it is finally applied to human wound treatment because liposuction has been very common and mature, researchers mixed ADSC-EVs with fibroblasts and demonstrated that this synergistic effect was helpful to induce the enrichment of miRNAs related to promoting wound healing in fibroblasts (86). Earlier it was shown that direct IV administration of ADSC-EVs in a murine wound model was beneficial to ameliorate cutaneous repair by regulating ECM remodeling (91). Furthermore, local injection of ADSC-EVs into mouse full-thickness cutaneous wounds significantly increased re-epithelialization, collagen deposition, and neovascularization and induced accelerated wound closure (82). To explore the therapeutic potential of ADSC-EVs more accurately, BMSC-EVs and ADSC-EVs were applied, respectively to diabetic wounds, and the results demonstrated that ADSC-EVs possess the more potent pro-angiogenic activity and can promote the wound healing of diabetic ulcers (13). Considering the availability of adipose tissue and fewer ethical concerns of EVs, emerging skin regenerative studies tend to focus on ADSC-EVs to better transform to future clinical trials (128).
Since the regeneration and repair mechanism of swine skin is similar to that of humans, swine skin is more promising than rodent skin for studying skin wounds. The topical conditioned medium of ADSC therapy displayed increased angiogenesis and a diminished inflammatory response and improved the wound closure rates in the full-thickness dorsal wound models in pigs (129). However, such research reports are very scarce. We analyze the possible reasons from two aspects. On the one side, it is technically and economically more difficult to operate diabetic or burn wound models in large animals. On the other side, the application of EVs in large animals requires a larger dose of EVs, which is limited by current EV isolation methods. Researchers need to accelerate the maturation of methods producing large-scale EVs or find new delivery methods to improve local retention of EVs, which is more conducive to the research on EVs application in the future.
At the time of this review writing, only a few trials related to applying EVs to treat skin wounds can be searched on the web clinicaltrials.gov (accessed on Nov. 20, 2021). Unfortunately, no trial related to ADSC-EVs is included until now, however, the following summary of other EVs applied to skin wound repair has important reminder and reference values for the subsequent direct application of ADSC-EVs in this field. Autologous serum-derived EVs will be evaluated to determine whether they could play a positive role in cutaneous wound healing (NCT02565264) and venous ulcers not responsive to conventional treatments (NCT04652531). One trial will investigate the therapeutic potential of stem cell-conditioned medium as an additional growth factor in chronic skin ulcer healing (NCT04134676). Another trial that has completed patient recruitment aims to develop a safe and reasonable method of administering BMSC-EVs to burn wounds (NCT05078385). Although no EV product had been approved by the FDA to date, it is firmly believed that more clinical studies will be included soon, and high-quality clinical trial results can energetically promote the final clinical application of EVs.
Skin Photoaging and Senescence
As the most commonly used stem cell therapeutics, the application of ADSCs in skin rejuvenation has been widely investigated. The paracrine effects of ADSCs, which are characterized by the release of cytokines in the form of EVs, are recognized as critical mechanisms in skin tissue repair and regeneration. Drawing support from their paracrine effects, ADSC-free derivatives, including ADSC-EVs and ADSC conditioned medium (ADSC-CM), have gained attention as novel therapeutics in ameliorating skin health (24, 130). As is universally acknowledged, human dermal fibroblasts (HDFs) work as essential objects in anti-wrinkle, wound healing, skin aging, and overall homeostasis studies. The ADSC-EVs and ADSC-CM acted as therapeutic agents in skin rejuvenation by improving proliferation, migration, and collagen synthesis in HDFs without adverse effects (131, 132). Because ADSC-CM could effectively downregulate the activation and transcription of UVB-related signaling pathways and upregulate antioxidant response agent expression, it was regarded to play a positive role in keeping HDFs away from UVB-induced photoaging damage (133). By decreasing ROS production and MMPs overexpression, which are directly linked to ECM proteins degradation, ADSC-EVs slowed wrinkle formation and skin photoaging (70, 134). Morphometric and morphological assessment of histological changes showed that ADSC-EVs could decrease UV-mediated epidermal thickening and prevent skin damage caused by UV photoaging (135). Because research on ADSC-EVs is still limited, the majority of studies on the therapeutic function of ADSC derivatives in skin rejuvenation focus on ADSC-CM. Despite slight differences in protein properties that exist between ADSC-EVs and ADSC-CM, these two main components in ADSC derivatives contain factors linked to ECM remodeling and immunoregulation, which are crucial for maintaining skin homeostasis and antiaging (136). Furthermore, the removal of EVs from ADSC-CM significantly weakened its positive effects on cell proliferation, migration, and scar prevention (137, 138). This demonstrates that EVs are crucial components in ADSC-CM and may possess an independent or synergistic role that is beneficial for anti-skin photoaging.
Skin Pigmentation After Trauma
In posttraumatic skin regeneration, the non-Caucasian race is more susceptible to pigmentation and/or scar formation. For such patients, anti-scar treatment while desalinating the pigment as far as possible will be conducive to the appearance of regenerated skin closer to normal. The increased expression of S1P induced by ADSC-EVs was negatively correlated with the production of melanin, which implies that ADSC-EVs have potential application value in skin-brightening (19). As shown in a prospective, double-blind, randomized, placebo-controlled study, a cosmetic formulation containing ADSC-EVs decreased skin melanin contents and reversed hyperpigmentation in human volunteers (139). Although the effect of ADSC-EVs on improving skin brightness becomes weak with time due to the limitation of transdermal delivery, the actuation duration of ADSC-EVs will be expected to be ameliorated with the continuous development of new drug delivery agents such as nano biomaterials. In the early stage of skin injury repair, the application of ADSC-EVs can promote scarless healing (83), but whether similar effects will appear in colored people and whether they could dilute pigmentation while reducing scarring need to be further studied.
Due to the different types of skin damage, the diversity of the involved skin layers also exists. Further research will be needed to locate which cells in the skin damage microenvironment are targets of ADSC-EVs. It is foreseeable that accurately applying EVs to selectively act on target cells in the epidermis or dermis will greatly contribute to further explaining the specific mechanism of EVs' regenerative function.
Challenges and Prospects
ADSCs have been demonstrated to play a beneficial role in skin regeneration and rejuvenation due to their participation in multiple biological activities of skin cells. ADSC-EVs, the products, and the information disseminators of ADSCs, seem to inherit similar therapeutic effects from their parental cells but are safer and more convenient to use. ADSC-EVs may promote skin regeneration, including structural repair and functional recovery, by accelerating the canonical wound repair process and regulating the skin microenvironment. The regenerative effect of ADSC-EVs renders them a potential option for clinical application in wound treatment and skin cosmetology. With multiple signal recognition molecules anchoring to the natural lipid membrane, ADSC-EVs are underlying carriers delivering drugs in vivo. EVs have been modified to carry therapeutic molecules by multiple loading methods, such as transfection of parental cells for endogenous loading (16), electroporation (140), co-incubation (141), and freeze-thawing (142) for exogenous loading. Furthermore, the combination between ADSC-EVs and bioactive scaffolds is an effective strategy to improve the quick clearance of ADSC-EVs at the wound site. Meanwhile, hydrogel dressings containing ADSC-EVs can be modified to possess functions promoting wound repair, such as antibacterial (89) and antioxidation (18).
Nevertheless, as mentioned above, current comprehension of ADSC-EVs themselves and the mechanisms of their actions remain elusive, and challenges exist in their manufacture and application. With skin structure more similar to human skin, pigs and guinea pigs are preferred animal models for skin wound research than rodent models mostly used in current studies of ADSC-EVs in skin regeneration. More convincing evidence needs to be uncovered to clarify the current enigma existing in the mechanisms of ADSC-EVs. For example, regulation of ADSC-EVs on the proliferation and differentiation of fibroblasts, which is essential for wound repair but results in scar formation when overwhelming. From a current perspective, the effect of ADSC-EVs is strongly associated with their contents, which are influenced by the physiological conditions of their parental cells (14, 143). Diverse isolation methods also lead to discrepancies in cargo (25). Quality control of ADSC-EVs is fundamental to probe the particular portion of ADSC-EVs functioning directly or mediately as key therapeutics in skin regeneration, thus further formulating instructive protocols to study or manufacture in the future. Likewise, a low yield of ADSC-EVs is also a crucial issue, which is attributed to the limited culture medium of ADSCs and repeated centrifugation processes in conventional isolation methods. Strategies for the production with large quantities and long-term storage of ADSC-EVs must be developed for clinical application. To date, research on ADSC-EVs has mainly remained at the laboratory level. To utilize the regenerative and therapeutic functions of ADSC-EVs, much more comprehensive information needs to be uncovered.
Author Contributions
YW, LC, and ZZ: conceptualization. YW and ZZ: validation. YW, LC, HZ, ZL, and JC: investigation. YC and ZZ: resources. YW: original draft preparation. LC and ZZ: review and editing. HZ, YC, and JC: visualization. ZZ: supervision and project administration. ZZ and ZL: funding acquisition. All authors have read and agreed to the published version of the manuscript, accepted responsibility for the entire content of this manuscript, and approved its submission.
Funding
This work was supported by grants from the National Natural Science Foundation of China (No. 81871574), and Scientific Research Projects of Sichuan Health Commission (No. 19PJ097).
Conflict of Interest
The authors declare that the research was conducted in the absence of any commercial or financial relationships that could be construed as a potential conflict of interest.
Publisher's Note
All claims expressed in this article are solely those of the authors and do not necessarily represent those of their affiliated organizations, or those of the publisher, the editors and the reviewers. Any product that may be evaluated in this article, or claim that may be made by its manufacturer, is not guaranteed or endorsed by the publisher.
References
1. Saeedi P, Petersohn I, Salpea P, Malanda B, Karuranga S, Unwin N, et al. Global and regional diabetes prevalence estimates for 2019 and projections for 2030 and 2045: results from the international diabetes federation diabetes atlas, 9(th) edition. Diabetes Res Clin Pract. (2019) 157:107843. doi: 10.1016/j.diabres.2019.107843
2. Walsh JW, Hoffstad OJ, Sullivan MO, Margolis DJ. Association of diabetic foot ulcer and death in a population-based cohort from the United Kingdom. Diabet Med. (2016) 33:1493–8. doi: 10.1111/dme.13054
3. Nourian Dehkordi A, Mirahmadi Babaheydari F, Chehelgerdi M, Raeisi Dehkordi S. Skin tissue engineering: wound healing based on stem-cell-based therapeutic strategies. Stem Cell Res Ther. (2019) 10:111. doi: 10.1186/s13287-019-1212-2
4. Welz PS. Clock regulation of skin regeneration in stem cell aging. J Invest Dermatol. (2021)141:1024–30. doi: 10.1016/j.jid.2020.10.009
5. Tompkins BA, DiFede DL, Khan A, Landin AM, Schulman IH, Pujol MV, et al. Allogeneic mesenchymal stem cells ameliorate aging frailty: a phase II randomized, double-blind, placebo-controlled clinical trial. J Gerontol A Biol Sci Med Sci. (2017) 72:1513–22. doi: 10.1093/gerona/glx137
6. Trounson A, McDonald C. Stem cell therapies in clinical trials: progress and challenges. Cell Stem Cell. (2015) 17:11–22. doi: 10.1016/j.stem.2015.06.007
7. Liu A, Zhang X, He H, Zhou L, Naito Y, Sugita S, et al. Therapeutic potential of mesenchymal stem/stromal cell-derived secretome and vesicles for lung injury and disease. Expert Opin Biol Ther. (2020) 20:125–40. doi: 10.1080/14712598.2020.1689954
8. Swaminathan M, Stafford-Smith M, Chertow GM, Warnock DG, Paragamian V, Brenner RM, et al. Allogeneic mesenchymal stem cells for treatment of AKI after cardiac surgery. J Am Soc Nephrol. (2018) 29:260–7. doi: 10.1681/ASN.2016101150
9. EL Andaloussi S, Mäger I, Breakefield XO, Wood MJ. Extracellular vesicles: biology and emerging therapeutic opportunities. Nat Rev Drug Discov. (2013) 12:347–57. doi: 10.1038/nrd3978
10. Keshtkar S, Azarpira N, Ghahremani MH. Mesenchymal stem cell-derived extracellular vesicles: novel frontiers in regenerative medicine. Stem Cell Res Ther. (2018) 9:63. doi: 10.1186/s13287-018-0791-7
11. Park JH, Hwang I, Hwang SH, Han H, Ha H. Human umbilical cord blood-derived mesenchymal stem cells prevent diabetic renal injury through paracrine action. Diabetes Res Clin Pract. (2012) 98:465–73. doi: 10.1016/j.diabres.2012.09.034
12. Jeyaram A, Jay SM. Preservation and storage stability of extracellular vesicles for therapeutic applications. AAPS J. (2017) 20:1. doi: 10.1208/s12248-017-0160-y
13. Pomatto M, Gai C, Negro F, Cedrino M, Grange C, Ceccotti E, et al. Differential therapeutic effect of extracellular vesicles derived by bone marrow and adipose mesenchymal stem cells on wound healing of diabetic ulcers and correlation to their cargoes. Int J Mol Sci. (2021) 22:3851. doi: 10.3390/ijms22083851
14. Casado-Díaz A, Quesada-Gómez JM, Dorado G. Extracellular vesicles derived from mesenchymal stem cells (MSC) in regenerative medicine: applications in skin wound healing. Front Bioeng Biotechnol. (2020) 8:146. doi: 10.3389/fbioe.2020.00146
15. Kim H, Lee JW, Han G, Kim K, Yang Y, Kim SH. extracellular vesicles as potential theranostic platforms for skin diseases and aging. Pharmaceutics. (2021) 13:760. doi: 10.3390/pharmaceutics13050760
16. Qu Y, Zhang Q, Cai X, Li F, Ma Z, Xu M, et al. Exosomes derived from miR-181-5p-modified adipose-derived mesenchymal stem cells prevent liver fibrosis via autophagy activation. J Cell Mol Med. (2017) 21:2491–502. doi: 10.1111/jcmm.13170
17. Zhang X, Jiang Y, Huang Q, Wu Z, Pu H, Xu Z, et al. Exosomes derived from adipose-derived stem cells overexpressing glyoxalase-1 protect endothelial cells and enhance angiogenesis in type 2 diabetic mice with limb ischemia. Stem Cell Res Ther. (2021) 12:403. doi: 10.1186/s13287-021-02475-7
18. Shiekh PA, Singh A, Kumar A. Exosome laden oxygen releasing antioxidant and antibacterial cryogel wound dressing OxOBand alleviate diabetic and infectious wound healing. Biomaterials. (2020) 249:120020. doi: 10.1016/j.biomaterials.2020.120020
19. Shin KO, Ha DH, Kim JO, Crumrine DA, Meyer JM, Wakefield JS, et al. Exosomes from human adipose tissue-derived mesenchymal stem cells promote epidermal barrier repair by inducing de novo synthesis of ceramides in atopic dermatitis. Cells. (2020) 9:680. doi: 10.3390/cells9030680
20. Wu J, Yang Q, Wu S, Yuan R, Zhao X, Li Y, et al. Adipose-derived stem cell exosomes promoted hair regeneration. Tissue Eng Regen Med. (2021) 18:685–91. doi: 10.1007/s13770-021-00347-y
21. Wolf DA, Beeson W, Rachel JD, Keller GS, Hanke CW, Waibel J, et al. Mesothelial stem cells and stromal vascular fraction for skin rejuvenation. Facial Plast Surg Clin North Am. (2018) 26:513–32. doi: 10.1016/j.fsc.2018.06.011
22. Zarei F, Abbaszadeh A. Stem cell and skin rejuvenation. J Cosmet Laser Ther. (2018) 20:193–7. doi: 10.1080/14764172.2017.1383615
23. Shukla L, Yuan Y, Shayan R, Greening DW, Karnezis T. Fat therapeutics: the clinical capacity of adipose-derived stem cells and exosomes for human disease and tissue regeneration. Front Pharmacol. (2020) 11:158. doi: 10.3389/fphar.2020.00158
24. Cai Y, Li J, Jia C, He Y, Deng C. Therapeutic applications of adipose cell-free derivatives: a review. Stem Cell Res Ther. (2020) 11:312. doi: 10.1186/s13287-020-01831-3
25. Gurunathan S, Kang MH, Jeyaraj M, Qasim M, Kim JH. Review of the isolation, characterization, biologial function, and multifarious therapeutic approaches of exosomes. Cells. (2019) 8:307. doi: 10.3390/cells8040307
26. Théry C, Witwer KW, Aikawa E, Alcaraz MJ, Anderson JD, Andriantsitohaina R, et al. Minimal information for studies of extracellular vesicles 2018 (MISEV2018): a position statement of the international society for extracellular vesicles and update of the MISEV2014 guidelines. J Extracell Vesicles. (2018) 7:1535750. doi: 10.1080/20013078.2018.1535750
27. van Niel G, D'Angelo G, Raposo G. Shedding light on the cell biology of extracellular vesicles. Nat Rev Mol Cell Biol. (2018) 19:213–28. doi: 10.1038/nrm.2017.125
28. Wu P, Zhang B, Ocansey DKW, Xu W, Qian H. Extracellular vesicles: a bright star of nanomedicine. Biomaterials. (2021) 269:120467. doi: 10.1016/j.biomaterials.2020.120467
29. Colombo M, Moita C, van Niel G, Kowal J, Vigneron J, Benaroch P, et al. Analysis of ESCRT functions in exosome biogenesis, composition and secretion highlights the heterogeneity of extracellular vesicles. J Cell Sci. (2013) 126:5553–65. doi: 10.1242/jcs.128868
30. Kajimoto T, Okada T, Miya S, Zhang L, Nakamura S. Ongoing activation of sphingosine 1-phosphate receptors mediates maturation of exosomal multivesicular endosomes. Nat Commun. (2013) 4:2712. doi: 10.1038/ncomms3712
31. Trajkovic K, Hsu C, Chiantia S, Rajendran L, Wenzel D, Wieland F, et al. Ceramide triggers budding of exosome vesicles into multivesicular endosomes. Science. (2008) 319:1244–7. doi: 10.1126/science.1153124
32. Andreu Z, Yáñez-Mó M. Tetraspanins in extracellular vesicle formation and function. Front Immunol. (2014) 5:442. doi: 10.3389/fimmu.2014.00442
33. Shao H, Im H, Castro CM, Breakefield X, Weissleder R, Lee H. New technologies for analysis of extracellular vesicles. Chem Rev. (2018) 118:1917–50. doi: 10.1021/acs.chemrev.7b00534
34. Connor DE, Exner T, Ma DD, Joseph JE. The majority of circulating platelet-derived microparticles fail to bind annexin V, lack phospholipid-dependent procoagulant activity and demonstrate greater expression of glycoprotein Ib. Thromb Haemost. (2010) 103:1044–52. doi: 10.1160/TH09-09-0644
35. Del Conde I, Shrimpton CN, Thiagarajan P, López JA. Tissue-factor-bearing microvesicles arise from lipid rafts and fuse with activated platelets to initiate coagulation. Blood. (2005) 106:1604–11. doi: 10.1182/blood-2004-03-1095
36. Atkin-Smith GK, Poon IKH. Disassembly of the dying: mechanisms and functions. Trends Cell Biol. (2017) 27:151–62. doi: 10.1016/j.tcb.2016.08.011
37. Lemke G. How macrophages deal with death. Nat Rev Immunol. (2019) 19:539–49. doi: 10.1038/s41577-019-0167-y
38. Li M, Liao L, Tian W. Extracellular vesicles derived from apoptotic cells: an essential link between death and regeneration. Front Cell Dev Biol. (2020) 8:573511. doi: 10.3389/fcell.2020.573511
39. Gomzikova MO, James V, Rizvanov AA. Therapeutic application of mesenchymal stem cells derived extracellular vesicles for immunomodulation. Front Immunol. (2019) 10:2663. doi: 10.3389/fimmu.2019.02663
40. Kowal J, Arras G, Colombo M, Jouve M, Morath JP, Primdal-Bengtson B, et al. Proteomic comparison defines novel markers to characterize heterogeneous populations of extracellular vesicle subtypes. Proc Natl Acad Sci U S A. (2016) 113:E968–77. doi: 10.1073/pnas.1521230113
41. Doyle LM, Wang MZ. Overview of extracellular vesicles, their origin, composition, purpose, and methods for exosome isolation and analysis. Cells. (2019) 8:727. doi: 10.3390/cells8070727
42. Kakarla R, Hur J, Kim YJ, Kim J, Chwae YJ. Apoptotic cell-derived exosomes: messages from dying cells. Exp Mol Med. (2020) 52:1–6. doi: 10.1038/s12276-019-0362-8
43. Tkach M, Théry C. Communication by extracellular vesicles: where we are and where we need to go. Cell. (2016) 164:1226–32. doi: 10.1016/j.cell.2016.01.043
44. Caruso S, Poon IKH. Apoptotic cell-derived extracellular vesicles: more than just debris. Front Immunol. (2018) 9:1486. doi: 10.3389/fimmu.2018.01486
45. Atkin-Smith GK, Tixeira R, Paone S, Mathivanan S, Collins C, Liem M, et al. A novel mechanism of generating extracellular vesicles during apoptosis via a beads-on-a-string membrane structure. Nat Commun. (2015) 6:7439. doi: 10.1038/ncomms8439
46. Lleo A, Zhang W, McDonald WH, Seeley EH, Leung PS, Coppel RL, et al. Shotgun proteomics: identification of unique protein profiles of apoptotic bodies from biliary epithelial cells. Hepatology. (2014) 60:1314–23. doi: 10.1002/hep.27230
47. Lee K, Shao H, Weissleder R, Lee H. Acoustic purification of extracellular microvesicles. ACS Nano. (2015) 9:2321–7. doi: 10.1021/nn506538f
48. Ibsen SD, Wright J, Lewis JM, Kim S, Ko SY, Ong J, et al. Rapid isolation and detection of exosomes and associated biomarkers from plasma. ACS Nano. (2017) 11:6641–51. doi: 10.1021/acsnano.7b00549
49. Davies RT, Kim J, Jang SC, Choi EJ, Gho YS, Park J. Microfluidic filtration system to isolate extracellular vesicles from blood. Lab Chip. (2012) 12:5202–10. doi: 10.1039/c2lc41006k
50. Dorayappan KDP, Gardner ML, Hisey CL, Zingarelli RA, Smith BQ, Lightfoot MDS, et al. A microfluidic chip enables isolation of exosomes and establishment of their protein profiles and associated signaling pathways in ovarian cancer. Cancer Res. (2019) 79:3503–13. doi: 10.1158/0008-5472.CAN-18-3538
51. Zhang H, Lyden D. Asymmetric-flow field-flow fractionation technology for exomere and small extracellular vesicle separation and characterization. Nat Protoc. (2019) 14:1027–53. doi: 10.1038/s41596-019-0126-x
52. Patel DB, Luthers CR, Lerman MJ, Fisher JP, Jay SM. Enhanced extracellular vesicle production and ethanol-mediated vascularization bioactivity via a 3D-printed scaffold-perfusion bioreactor system. Acta Biomater. (2019) 95:236–44. doi: 10.1016/j.actbio.2018.11.024
53. Thone MN, Kwon YJ. Extracellular blebs: artificially-induced extracellular vesicles for facile production and clinical translation. Methods. (2020) 177:135–45. doi: 10.1016/j.ymeth.2019.11.007
54. Nair A, Bu J, Rawding PA, Do SC, Li H, Hong S. Cytochalasin B treatment and osmotic pressure enhance the production of extracellular vesicles (EVs) with improved drug loading capacity. Nanomaterials. (2021) 12:3. doi: 10.3390/nano12010003
55. Musante L, Tataruch-Weinert D, Kerjaschki D, Henry M, Meleady P, Holthofer H. Residual urinary extracellular vesicles in ultracentrifugation supernatants after hydrostatic filtration dialysis enrichment. J Extracell Vesicles. (2017) 6:1267896. doi: 10.1080/20013078.2016.1267896
56. Chen TS, Arslan F, Yin Y, Tan SS, Lai RC, Choo AB, et al. Enabling a robust scalable manufacturing process for therapeutic exosomes through oncogenic immortalization of human ESC-derived MSCs. J Transl Med. (2011) 9:47. doi: 10.1186/1479-5876-9-47
57. An Y, Lin S, Tan X, Zhu S, Nie F, Zhen Y, et al. Exosomes from adipose-derived stem cells and application to skin wound healing. Cell Prolif. (2021) 54:e12993. doi: 10.1111/cpr.12993
58. Lee K, Fraser K, Ghaddar B, Yang K, Kim E, Balaj L, et al. Multiplexed profiling of single extracellular vesicles. ACS Nano. (2018) 12:494–503. doi: 10.1021/acsnano.7b07060
59. Xiao S, Xiao C, Miao Y, Wang J, Chen R, Fan Z, et al. Human acellular amniotic membrane incorporating exosomes from adipose-derived mesenchymal stem cells promotes diabetic wound healing. Stem Cell Res Ther. (2021) 12:255. doi: 10.1186/s13287-021-02333-6
60. Liu H, Zhang M, Shi M, Zhang T, Lu W, Yang S, et al. Adipose-derived mesenchymal stromal cell-derived exosomes promote tendon healing by activating both SMAD1/5/9 and SMAD2/3. Stem Cell Res Ther. (2021) 12:338. doi: 10.1186/s13287-021-02410-w
61. Li Q, Yu H, Sun M, Yang P, Hu X, Ao Y, et al. The tissue origin effect of extracellular vesicles on cartilage and bone regeneration. Acta Biomater. (2021) 125:253–66. doi: 10.1016/j.actbio.2021.02.039
62. Rau CS, Kuo PJ, Wu SC, Huang LH, Lu TH, Wu YC, et al. Enhanced nerve regeneration by exosomes secreted by adipose-derived stem cells with or without FK506 stimulationInt. J Mol Sci. (2021) 22:8545. doi: 10.3390/ijms22168545
63. Rodrigues M, Kosaric N, Bonham CA, Gurtner GC. Wound healing: a cellular perspective. Physiol Rev. (2019) 99:665–706. doi: 10.1152/physrev.00067.2017
64. Moura J, Madureira P, Leal EC, Fonseca AC, Carvalho E. Immune aging in diabetes and its implications in wound healing. Clin Immunol. (2019) 200:43–54. doi: 10.1016/j.clim.2019.02.002
65. Bolandi Z, Mokhberian N, Eftekhary M, Sharifi K, Soudi S, Ghanbarian H, et al. Adipose derived mesenchymal stem cell exosomes loaded with miR-10a promote the differentiation of Th17 and Treg from naive CD4(+) T cell. Life Sci. (2020) 259:118218. doi: 10.1016/j.lfs.2020.118218
66. Blazquez R, Sanchez-Margallo FM, de la Rosa O, Dalemans W, Alvarez V, Tarazona R, et al. Immunomodulatory potential of human adipose mesenchymal stem cells derived exosomes on in vitro stimulated T cells. Front Immunol. (2014) 5:556. doi: 10.3389/fimmu.2014.00556
67. Fernandes TL, Gomoll AH, Lattermann C, Hernandez AJ, Bueno DF, Amano MT. Macrophage: a potential target on cartilage regeneration. Front Immunol. (2020) 11:111. doi: 10.3389/fimmu.2020.00111
68. Zhao H, Shang Q, Pan Z, Bai Y, Li Z, Zhang H, et al. Exosomes from adipose-derived stem cells attenuate adipose inflammation and obesity through polarizing M2 macrophages and beiging in white adipose tissue. Diabetes. (2018) 67:235–47. doi: 10.2337/db17-0356
69. Deng S, Zhou X, Ge Z, Song Y, Wang H, Liu X, et al. Exosomes from adipose-derived mesenchymal stem cells ameliorate cardiac damage after myocardial infarction by activating S1P/SK1/S1PR1 signaling and promoting macrophage M2 polarization. Int J Biochem Cell Biol. (2019) 114:105564. doi: 10.1016/j.biocel.2019.105564
70. Xu P, Xin Y, Zhang Z, Zou X, Xue K, Zhang H, et al. Extracellular vesicles from adipose-derived stem cells ameliorate ultraviolet B-induced skin photoaging by attenuating reactive oxygen species production and inflammation. Stem Cell Res Ther. (2020) 11:264. doi: 10.1186/s13287-020-01777-6
71. Bai Y, Han YD, Yan XL, Ren J, Zeng Q, Li XD, et al. Adipose mesenchymal stem cell-derived exosomes stimulated by hydrogen peroxide enhanced skin flap recovery in ischemia-reperfusion injury. Biochem Biophys Res Commun. (2018) 500:310–7. doi: 10.1016/j.bbrc.2018.04.065
72. Xia Y, Rao L, Yao H, Wang Z, Ning P, Chen X. Engineering macrophages for cancer immunotherapy and drug delivery. Adv Mat. (2020) 32:e2002054. doi: 10.1002/adma.202002054
73. Chen B, Cai J, Wei Y, Jiang Z, Desjardins HE, Adams AE, et al. Exosomes are comparable to source adipose stem cells in fat graft retention with up-regulating early inflammation and angiogenesis. Plast Reconstr Surg. (2019) 144:816e−27. doi: 10.1097/PRS.0000000000006175
74. Ma J, Zhang Z, Wang Y, Shen H. Investigation of miR-126-3p loaded on adipose stem cell-derived exosomes for wound healing of full-thickness skin defects. Exp Dermatol. (2021). doi: 10.1111/exd.14480
75. Zhu LL, Huang X, Yu W, Chen H, Chen Y, Dai YT. Transplantation of adipose tissue-derived stem cell-derived exosomes ameliorates erectile function in diabetic rats. Andrologia. (2018) 50:e12871. doi: 10.1111/and.12871
76. Pi L, Yang L, Fang BR, Meng XX, Qian L. Exosomal microRNA-125a-3p from human adipose-derived mesenchymal stem cells promotes angiogenesis of wound healing through inhibiting PTEN. Mol Cell Biochem. (2021). doi: 10.1007/s11010-021-04251-w
77. Liu W, Yuan Y, Liu D. Extracellular vesicles from adipose-derived stem cells promote diabetic wound healing via the PI3K-AKT-mTOR-HIF-1α signaling pathway. Tissue Eng Regen Med. (2021) 18:1035–44. doi: 10.1007/s13770-021-00383-8
78. Xue C, Shen Y, Li X, Li B, Zhao S, Gu J, et al. Exosomes derived from hypoxia-treated human adipose mesenchymal stem cells enhance angiogenesis through the PKA signaling pathway. Stem Cells Dev. (2018) 27:456–65. doi: 10.1089/scd.2017.0296
79. Lopatina T, Bruno S, Tetta C, Kalinina N, Porta M, Camussi G. Platelet-derived growth factor regulates the secretion of extracellular vesicles by adipose mesenchymal stem cells and enhances their angiogenic potential. Cell Commun Signal. (2014) 12:26. doi: 10.1186/1478-811X-12-26
80. Abu El-Asrar AM, Struyf S, Opdenakker G, Van Damme J, Geboes K. Expression of stem cell factor/c-kit signaling pathway components in diabetic fibrovascular epiretinal membranes. Mol Vis. (2010) 16:1098–107. doi: 10.1111/j.1755-3768.2010.2444.x
81. DiPietro LA. Angiogenesis and wound repair: when enough is enough. J Leukoc Biol. (2016) 100:979–84. doi: 10.1189/jlb.4MR0316-102R
82. Ren S, Chen J, Duscher D, Liu Y, Guo G, Kang Y, et al. Microvesicles from human adipose stem cells promote wound healing by optimizing cellular functions via AKT and ERK signaling pathways. Stem Cell Res Ther. (2019) 10:47. doi: 10.1186/s13287-019-1152-x
83. Hu L, Wang J, Zhou X, Xiong Z, Zhao J, Yu R, et al. Exosomes derived from human adipose mensenchymal stem cells accelerates cutaneous wound healing via optimizing the characteristics of fibroblasts. Sci Rep. (2016) 6:32993. doi: 10.1038/srep32993
84. Qian L, Pi L, Fang BR, Meng XX. Adipose mesenchymal stem cell-derived exosomes accelerate skin wound healing via the lncRNA H19/miR-19b/SOX9 axis. Lab Invest. (2021) 101:1254–66. doi: 10.1038/s41374-021-00611-8
85. He L, Zhu C, Jia J, Hao XY, Yu XY, Liu XY, et al. ADSC-Exos containing MALAT1 promotes wound healing by targeting miR-124 through activating Wnt/β-catenin pathway. Biosci Rep. (2020) 40:BSR20192549. doi: 10.1042/BSR20192549
86. Choi EW, Seo MK, Woo EY, Kim SH, Park EJ, Kim S. Exosomes from human adipose-derived stem cells promote proliferation and migration of skin fibroblasts. Exp Dermatol. (2018) 27:1170–2. doi: 10.1111/exd.13451
87. Verhaegen PD, van Zuijlen PP, Pennings NM, van Marle J, Niessen FB, van der Horst CM, et al. Differences in collagen architecture between keloid, hypertrophic scar, normotrophic scar, and normal skin: an objective histopathological analysis. Wound Repair Regen. (2009) 17:649–56. doi: 10.1111/j.1524-475X.2009.00533.x
88. Rohani MG, Parks WC. Matrix remodeling by MMPs during wound repair. Matrix Biol. (2015) 44–46:113–21. doi: 10.1016/j.matbio.2015.03.002
89. Wang C, Wang M, Xu T, Zhang X, Lin C, Gao W, et al. Engineering bioactive self-healing antibacterial exosomes hydrogel for promoting chronic diabetic wound healing and complete skin regeneration. Theranostics. (2019) 9:65–76. doi: 10.7150/thno.29766
90. Wang M, Wang C, Chen M, Xi Y, Cheng W, Mao C, et al. Efficient angiogenesis-based diabetic wound healing/skin reconstruction through bioactive antibacterial adhesive ultraviolet shielding nanodressing with exosome release. ACS Nano. (2019) 13:10279–93. doi: 10.1021/acsnano.9b03656
91. Wang L, Hu L, Zhou X, Xiong Z, Zhang C, Shehada HMA, et al. Exosomes secreted by human adipose mesenchymal stem cells promote scarless cutaneous repair by regulating extracellular matrix remodelling. Sci Rep. (2017) 7:13321. doi: 10.1038/s41598-017-12919-x
92. Yang C, Luo L, Bai X, Shen K, Liu K, Wang J, et al. Highly-expressed micoRNA-21 in adipose derived stem cell exosomes can enhance the migration and proliferation of the HaCaT cells by increasing the MMP-9 expression through the PI3K/AKT pathway. Arch Biochem Biophys. (2020) 681:108259. doi: 10.1016/j.abb.2020.108259
93. Jones JI, Nguyen TT, Peng Z, Chang M. Targeting MMP-9 in diabetic foot ulcers. Pharmaceuticals. (2019) 12:79. doi: 10.3390/ph12020079
94. Wang J, Yi Y, Zhu Y, Wang Z, Wu S, Zhang J, et al. Effects of adipose-derived stem cell released exosomes on wound healing in diabetic mice. Chin J Reparative Reconstr Surg. (2020) 34:124–31. doi: 10.7507/1002-1892.201903058
95. Li Y, Zhang J, Shi J, Liu K, Wang X, Jia Y, et al. Exosomes derived from human adipose mesenchymal stem cells attenuate hypertrophic scar fibrosis by miR-192-5p/IL-17RA/Smad axis. Stem Cell Res Ther. (2021) 12:221. doi: 10.1186/s13287-021-02290-0
96. Shao T, Tang W, Li Y, Gao D, Lv K, He P, et al. Research on function and mechanisms of a novel small molecule WG449E for hypertrophic scar. J Eur Acad Dermatol Venereol. (2020) 34:608–18. doi: 10.1111/jdv.16028
97. Dai X, Zeng J, Yan X, Lin Q, Wang K, Chen J, et al. Sitagliptin-mediated preservation of endothelial progenitor cell function via augmenting autophagy enhances ischaemic angiogenesis in diabetes. J Cell Mol Med. (2018) 22:89–100. doi: 10.1111/jcmm.13296
98. Ma T, Fu B, Yang X, Xiao Y, Pan M. Adipose mesenchymal stem cell-derived exosomes promote cell proliferation, migration, and inhibit cell apoptosis via Wnt/β-catenin signaling in cutaneous wound healing. J Cell Biochem. (2019) 120:10847–54. doi: 10.1002/jcb.28376
99. Liu S, Jiang L, Li H, Shi H, Luo H, Zhang Y, et al. Mesenchymal stem cells prevent hypertrophic scar formation via inflammatory regulation when undergoing apoptosis. J Invest Dermatol. (2014) 134:2648–57. doi: 10.1038/jid.2014.169
100. Cano Sanchez M, Lancel S, Boulanger E, Neviere R. Targeting oxidative stress and mitochondrial dysfunction in the treatment of impaired wound healing: a systematic review. Antioxidants. (2018) 7:98. doi: 10.3390/antiox7080098
101. Okonkwo UA, DiPietro LA. Diabetes and wound angiogenesis. Int J Mol Sci. (2017) 18:1419. doi: 10.3390/ijms18071419
102. Li X, Xie X, Lian W, Shi R, Han S, Zhang H, et al. Exosomes from adipose-derived stem cells overexpressing Nrf2 accelerate cutaneous wound healing by promoting vascularization in a diabetic foot ulcer rat model. Exp Mol Med. (2018) 50:1–14. doi: 10.1038/s12276-018-0058-5
103. Cooper DR, Wang C, Patel R, Trujillo A, Patel NA, Prather J, et al. Human adipose-derived stem cell conditioned media and exosomes containing MALAT1 promote human dermal fibroblast migration and ischemic wound healing. Adv Wound Care. (2018) 7:299–308. doi: 10.1089/wound.2017.0775
104. Shi B, Wang Y, Zhao R, Long X, Deng W, Wang Z. Bone marrow mesenchymal stem cell-derived exosomal miR-21 protects C-kit+ cardiac stem cells from oxidative injury through the PTEN/PI3K/Akt axis. PLoS One. (2018) 13:e0191616. doi: 10.1371/journal.pone.0191616
105. Xiao J, Pan Y, Li XH, Yang XY, Feng YL, Tan HH, et al. Cardiac progenitor cell-derived exosomes prevent cardiomyocytes apoptosis through exosomal miR-21 by targeting PDCD4. Cell Death Dis. (2016) 7:e2277. doi: 10.1038/cddis.2016.181
106. Aragonès G, Rowan S, Francisco SG, Yang W, Weinberg J, Taylor A, et al. Glyoxalase system as a therapeutic target against diabetic retinopathy. Antioxidants. (2020) 9:1062. doi: 10.3390/antiox9111062
107. Morgenstern J, Campos Campos M, Nawroth P, Fleming T. The glyoxalase system-new insights into an ancient metabolism. Antioxidants. (2020) 9:939. doi: 10.3390/antiox9100939
108. Sachdeva R, Schlotterer A, Schumacher D, Matka C, Mathar I, Dietrich N, et al. TRPC proteins contribute to development of diabetic retinopathy and regulate glyoxalase 1 activity and methylglyoxal accumulation. Mol Metab. (2018) 9:156–67. doi: 10.1016/j.molmet.2018.01.003
109. Jia Y, Gan Y, He C, Chen Z, Zhou C. The mechanism of skin lipids influencing skin status. J Dermatol Sci. (2018) 89:112–9. doi: 10.1016/j.jdermsci.2017.11.006
110. Arda O, Göksügür N, Tüzün Y. Basic histological structure and functions of facial skin. Clin Dermatol. (2014) 32:3–13. doi: 10.1016/j.clindermatol.2013.05.021
111. Egawa G, Kabashima K. Multifactorial skin barrier deficiency and atopic dermatitis: Essential topics to prevent the atopic march. J Allergy Clin Immunol. (2016) 138:350–8.e1. doi: 10.1016/j.jaci.2016.06.002
112. Nuutila K. Hair follicle transplantation for wound repair. Adv Wound Care. (2021) 10:153–63. doi: 10.1089/wound.2019.1139
113. Joost S, Jacob T, Sun X, Annusver K, La Manno G, Sur I, et al. Single-cell transcriptomics of traced epidermal and hair follicle stem cells reveals rapid adaptations during wound healing. Cell Rep. (2018) 25:585–97.e7. doi: 10.1016/j.celrep.2018.09.059
114. Takeo M, Lee W, Ito M. Wound healing and skin regeneration. Cold Spring Harb Perspect Med. (2015) 5:a023267. doi: 10.1101/cshperspect.a023267
115. Tomita Y, Akiyama M, Shimizu H. PDGF isoforms induce and maintain anagen phase of murine hair follicles. J Dermatol Sci. (2006) 43:105–15. doi: 10.1016/j.jdermsci.2006.03.012
116. Boisvert WA, Yu M, Choi Y, Jeong GH, Zhang YL, Cho S, et al. Hair growth-promoting effect of Geranium sibiricum extract in human dermal papilla cells and C57BL/6 mice. BMC Complement Altern Med. (2017) 17:109. doi: 10.1186/s12906-017-1624-4
117. Zhu HL, Gao YH, Yang JQ Li JB, Gao J. Serenoa repens extracts promote hair regeneration and repair of hair loss mouse models by activating TGF-β and mitochondrial signaling pathway. Eur Rev Med Pharmacol Sci. (2018) 22:4000–8. doi: 10.26355/eurrev_201806_15285
118. Harshuk-Shabso S, Dressler H, Niehrs C, Aamar E, Enshell-Seijffers D. Fgf and Wnt signaling interaction in the mesenchymal niche regulates the murine hair cycle clock. Nat Commun. (2020) 11:5114. doi: 10.1038/s41467-020-18643-x
119. Gentile P, Garcovich S. Advances in regenerative stem cell therapy in androgenic alopecia and hair loss: wnt pathway, growth-factor, and mesenchymal stem cell signaling impact analysis on cell growth and hair follicle development. Cells. (2019) 8:466. doi: 10.3390/cells8050466
120. Rishikaysh P, Dev K, Diaz D, Qureshi WM, Filip S, Mokry J. Signaling involved in hair follicle morphogenesis and development. Int J Mol Sci. (2014) 15:1647–70. doi: 10.3390/ijms15011647
121. Boiten W, Absalah S, Vreeken R, Bouwstra J, van Smeden J. Quantitative analysis of ceramides using a novel lipidomics approach with three dimensional response modelling. Biochim Biophys Acta. (2016) 1861:1652–61. doi: 10.1016/j.bbalip.2016.07.004
122. Di Nardo A, Wertz P, Giannetti A, Seidenari S. Ceramide and cholesterol composition of the skin of patients with atopic dermatitis. Acta Derm Venereol. (1998) 78:27–30. doi: 10.1080/00015559850135788
123. Gomez-Larrauri A, Presa N, Dominguez-Herrera A, Ouro A, Trueba M, Gomez-Muñoz A. Role of bioactive sphingolipids in physiology and pathology. Essays Biochem. (2020) 64:579–89. doi: 10.1042/EBC20190091
124. Borodzicz S, Rudnicka L, Mirowska-Guzel D, Cudnoch-Jedrzejewska A. The role of epidermal sphingolipids in dermatologic diseases. Lipids Health Dis. (2016) 15:13. doi: 10.1186/s12944-016-0178-7
125. Geiger A, Walker A, Nissen E. Human fibrocyte-derived exosomes accelerate wound healing in genetically diabetic mice. Biochem Biophys Res Commun. (2015) 467:303–9. doi: 10.1016/j.bbrc.2015.09.166
126. Zhang J, Guan J, Niu X, Hu G, Guo S, Li Q, et al. Exosomes released from human induced pluripotent stem cells-derived MSCs facilitate cutaneous wound healing by promoting collagen synthesis and angiogenesis. J Transl Med. (2015) 13:49. doi: 10.1186/s12967-015-0417-0
127. Zhang B, Wang M, Gong A, Zhang X, Wu X, Zhu Y, et al. HucMSC-exosome mediated-wnt4 signaling is required for cutaneous wound healing. Stem Cells. (2015) 33:2158–68. doi: 10.1002/stem.1771
128. Bray ER, Oropallo AR, Grande DA, Kirsner RS, Badiavas EV. Extracellular vesicles as therapeutic tools for the treatment of chronic wounds. Pharmaceutics. (2021) 13:1543. doi: 10.3390/pharmaceutics13101543
129. Irons RF, Cahill KW, Rattigan DA, Marcotte JH, Fromer MW, Chang S, et al. Acceleration of diabetic wound healing with adipose-derived stem cells, endothelial-differentiated stem cells, and topical conditioned medium therapy in a swine model. J Vasc Surg. (2018) 68:115s−25. doi: 10.1016/j.jvs.2018.01.065
130. Basu J, Ludlow JW. Exosomes for repair, regeneration and rejuvenation. Expert Opin Biol Ther. (2016) 16:489–506. doi: 10.1517/14712598.2016.1131976
131. Xiong M, Zhang Q, Hu W, Zhao C, Lv W, Yi Y, et al. Exosomes from adipose-derived stem cells: the emerging roles and applications in tissue regeneration of plastic and cosmetic surgery. Front Cell Dev Biol. (2020) 8:574223. doi: 10.3389/fcell.2020.574223
132. Ha DH, Kim SD, Lee J, Kwon HH, Park GH, Yang SH, et al. Toxicological evaluation of exosomes derived from human adipose tissue-derived mesenchymal stem/stromal cells. Regul Toxicol Pharmacol. (2020) 115:104686. doi: 10.1016/j.yrtph.2020.104686
133. Li L, Ngo HTT, Hwang E, Wei X, Liu Y, Liu J, et al. Conditioned medium from human adipose-derived mesenchymal stem cell culture prevents UVB-induced skin aging in human keratinocytes and dermal fibroblasts. Int J Mol Sci. (2019) 21:49. doi: 10.3390/ijms21010049
134. Choi JS, Cho WL, Choi YJ, Kim JD, Park HA, Kim SY, et al. Functional recovery in photo-damaged human dermal fibroblasts by human adipose-derived stem cell extracellular vesicles. J Extracell Vesicles. (2019) 8:1565885. doi: 10.1080/20013078.2019.1565885
135. Syromiatnikova V, Idrisova K, Masgutova G, Gomzikova M, Kabwe E, Bek J, et al. Analyzing the effectiveness of adipose tissue stem cell and microvesicle therapy in premature skin aging caused by chronic exposure to ultraviolet radiation. Bionanoscience. (2020) 10:991–7. doi: 10.1007/s12668-020-00793-3
136. Niada S, Giannasi C, Magagnotti C, Andolfo A, Brini AT. Proteomic analysis of extracellular vesicles and conditioned medium from human adipose-derived stem/stromal cells and dermal fibroblasts. J Proteomics. (2021) 232:104069. doi: 10.1016/j.jprot.2020.104069
137. Zhu YZ, Hu X, Zhang J, Wang ZH, Wu S, Yi YY. Extracellular vesicles derived from human adipose-derived stem cell prevent the formation of hypertrophic scar in a rabbit model. Ann Plast Surg. (2020) 84:602–7. doi: 10.1097/SAP.0000000000002357
138. Lu Z, Chen Y, Dunstan C, Roohani-Esfahani S, Zreiqat H. Priming adipose stem cells with tumor necrosis factor-alpha preconditioning potentiates their exosome efficacy for bone regeneration. Tissue Eng Part A. (2017) 23:1212–20. doi: 10.1089/ten.tea.2016.0548
139. Cho BS, Lee J, Won Y, Duncan DI, Jin RC, Lee J, et al. Skin brightening efficacy of exosomes derived from human adipose tissue-derived stem/stromal cells: a prospective, split-face, randomized placebo-controlled study. Cosmetics. (2020) 7:12. doi: 10.3390/cosmetics7040090
140. Alvarez-Erviti L, Seow Y, Yin H, Betts C, Lakhal S, Wood MJ. Delivery of siRNA to the mouse brain by systemic injection of targeted exosomes. Nat Biotechnol. (2011) 29:341–5. doi: 10.1038/nbt.1807
141. Zhuang X, Xiang X, Grizzle W, Sun D, Zhang S, Axtell RC, et al. Treatment of brain inflammatory diseases by delivering exosome encapsulated anti-inflammatory drugs from the nasal region to the brain. Mol Ther. (2011) 19:1769–79. doi: 10.1038/mt.2011.164
142. Haney MJ, Klyachko NL, Zhao Y, Gupta R, Plotnikova EG, He Z, et al. Exosomes as drug delivery vehicles for Parkinson's disease therapy. J Control Release. (2015) 207:18–30. doi: 10.1016/j.jconrel.2015.03.033
Keywords: skin regeneration, extracellular vesicles, adipose-derived stem cells, stem cells therapy, wound healing
Citation: Wang Y, Cheng L, Zhao H, Li Z, Chen J, Cen Y and Zhang Z (2022) The Therapeutic Role of ADSC-EVs in Skin Regeneration. Front. Med. 9:858824. doi: 10.3389/fmed.2022.858824
Received: 20 January 2022; Accepted: 20 May 2022;
Published: 09 June 2022.
Edited by:
Xing-Hua Gao, The First Affiliated Hospital of China Medical University, ChinaReviewed by:
Teng Su, Duke University, United StatesMarina Gomzikova, Kazan Federal University, Russia
Copyright © 2022 Wang, Cheng, Zhao, Li, Chen, Cen and Zhang. This is an open-access article distributed under the terms of the Creative Commons Attribution License (CC BY). The use, distribution or reproduction in other forums is permitted, provided the original author(s) and the copyright owner(s) are credited and that the original publication in this journal is cited, in accordance with accepted academic practice. No use, distribution or reproduction is permitted which does not comply with these terms.
*Correspondence: Zhenyu Zhang, emhhbmd6eS53Y2gmI3gwMDA0MDtmb3htYWlsLmNvbQ==
†These authors have contributed equally to this work and share first authorship