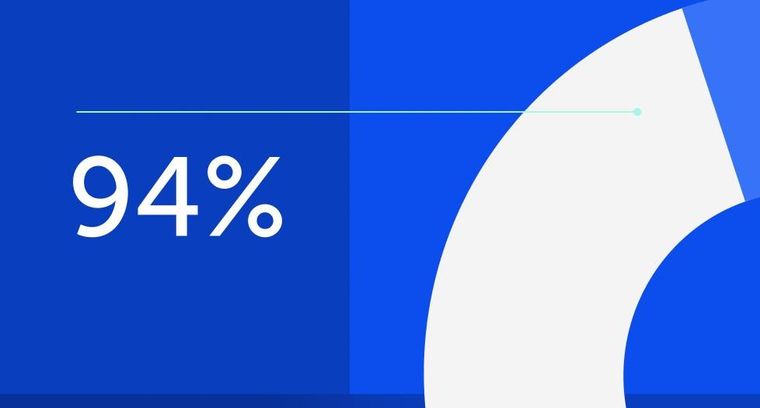
94% of researchers rate our articles as excellent or good
Learn more about the work of our research integrity team to safeguard the quality of each article we publish.
Find out more
MINI REVIEW article
Front. Mater., 19 December 2022
Sec. Carbon-Based Materials
Volume 9 - 2022 | https://doi.org/10.3389/fmats.2022.1090412
A correction has been applied to this article in:
Corrigendum: Nanostructured carbon electrocatalysts for clean energy conversion and storage: A mini review on the structural impact
Electrochemical conversions of carbon dioxide, water, oxygen, and nitrogen have offered effective ways to relieve the problems of carbon dioxide over-emission and fluctuated energy (such as solar, wind, tide, etc.) storage. The key factor that impacts the electrochemical system’s performance is the catalysts employed. Among all the materials, carbon nanomaterials generally exhibit high catalytic activity which is attributed to the high conductivity, large specific surface area, and exposed active sites. Recently, more and more researchers set their sights on applying the carbon nanomaterials in large-scale projects. Herein, it is of great importance to review the most recent studies on carbon nanomaterials in electrochemical applications. This paper summarizes the applications of carbon nanomaterials in electrochemical processes, and the structure impact on the performance. Further, challenges in this field are discussed, which can guide the innovative synthesis of efficient nanostructured carbon electrocatalysts for practical, large-scale energy conversion applications.
Reducing greenhouse gas emissions from energy sources is a reliable way to achieve carbon neutrality (Chen L. et al., 2022). Among all the technologies, using renewable energy to generate electricity is one of the most effective methods to reduce CO2 emissions on a large scale. However, the intermittent, seasonal, and regional characteristics greatly hinder its applications. Recently, researchers have set their eyes on incorporating electrochemical conversion and storage devices into the grid to solve the problem (Yang et al., 2022). Electrocatalytic carbon dioxide reduction reaction (CO2RR), hydrogen evolution reaction (HER), oxygen evolution reaction (OER), oxygen reduction reaction (ORR), and electrocatalytic nitrogen reduction reaction (NRR) are the most commonly used technologies. And the key factor which impacts the electrochemical performance and economic efficiency most is the catalyst employed (Liu et al., 2021a; Deng et al., 2022b).
Carbon nanomaterials with high conductivity, stability, specific surface area, and low cost have drawn great interests in recent years (Khan et al., 2022; Zhang et al., 2022a). These carbon nanomaterials can be divided into zero (0D), also called carbon dots (CDs), one (1D), two (2D), and three (3D) dimensional materials according to their structures (Figure 1). Typical CDs, consisting of an sp2/sp3 hybrid carbon core and an outer layer rich in hydroxyl (−OH), carboxyl (−COOH), and amine (−NH2) functional groups, can be divided into graphene quantum dots (GQDs), carbon nanodots (CNDs), carbon quantum dots (CQDs), and polymer dots (PDs) according to structure (Xia et al., 2019; Wu H. et al., 2022). 1D carbon nanomaterials include carbon nanofibers, nanoneedles, and nanotubes, which are quite malleable; 2D carbon nanomaterials mainly consist of carbon nanosheets graphene, graphene oxide, and other transition metal carbides such as MXene, with ultra-thin layered structures, offering the potential for more active sites and intermediate adsorption (Jin et al., 2021, 2022); 3D carbon nanomaterials primarily include various porous materials and nanoarrays formed by 1D materials like carbon nanotubes or nanosheets (Joo et al., 2001; Asefa et al., 2021). Actually, the pure carbon material does not possess good catalytic activity. In an electrocatalytic process, the activity of the catalyst mainly depends on its adsorption/desorption ability to the key reaction intermediates involved in the reaction, which is related to the active sites’ electronic structure. Therefore, the electronic structure has been optimized, mainly through doping, defect construction, and the formation of heterojunctions, to obtain a more suitable adsorption capacity (Jin et al., 2018b; Wang et al., 2019a). However, the high catalytic activity cannot be obtained with solely well-designed active sites. The number of active sites, the contact between sites and reaction medium, the transport capacity and electron transport capacity of substances in the process, and the durability and adaptability of materials, which are mainly related to the structural properties of the material, are far more important (Wang et al., 2019b; Asefa et al., 2021).
FIGURE 1. Carbon nanomaterials classified by structure (Zhao et al., 2018; Cai et al., 2019; Shah et al., 2019; Kagkoura et al., 2020; Liu F. et al., 2022; Wu Y. et al., 2022; Domingo-Tafalla et al., 2022; Xiao et al., 2022; Zhong et al., 2022).
This review mainly introduces the applications of carbon nanomaterials in CO2RR, HER, OER, ORR, and NRR technologies, and the structural optimization methods to obtain good catalytic performance in terms of the improving of active sites number, electron transport capacity, mass transport capacity, and catalysts’ stability. The challenges in this field are also discussed, and suggestions are given for the future development.
Electrocatalytic water splitting comprises an anodic OER and a cathodic HER in one system. HER is a two-electron transfer process whose energy barrier is relatively low, but the overall kinetics are slow due to the complex four-electron transfer and multi-step reaction behavior of OER.
Although the cathode and anode generate different intermediates, the adsorption of all the intermediates can be effectively enhanced with more exposed active sites (Figure 2) through structural optimization (Sultan et al., 2019; Cheng et al., 2022). In HER, nanoparticle aggregation is a serious problem, Liu and co-workers coated the Ni particles with a carbon layer, this effectively inhibited aggregation and increased the exposure of active centers (Liu F. et al., 2022). The modification of carbon nanotubes grown on carbon fiber paper (CFP) effectively promoted the dispersion of the active phase and obtained a larger active surface area, thus enhancing the performance of OER (Qiao et al., 2022). Graphene and g-C3N4-based nanosheets are also well dispersed with active sites due to their ultra-thin layered structure, e.g., graphene oxide (Rh-GO) modified with Rh nanospheres, with HER performance comparable to that of Pt electrodes (Jin et al., 2018a; 2018c; Narwade et al., 2020). Due to special electronic properties and space limitations, the metal inside the core-shell structure has more catalytic activity than the metal deposited on the surface. For example, the core-shell structure prepared by Weber et al. has a graphite-like shell with abundant defect sites, which showed high catalytic performance in HER (Weber et al., 2022).
FIGURE 2. (A) Layered nanosheets uniformly loaded with active particles; (B) porous nanofibers; (C) the core-shell structure with rich active sites (Wang et al., 2021; Liu F. et al., 2022; He M. et al., 2022).
The performances of electrocatalysts depend large on their ability to transfer and exchange electrons in the electrolyte (Qiao et al., 2022). High graphitization can increase catalyts’ inherent electrical conductivity (Yu et al., 2022). Conventional powdered catalysts require polymer binding, which often covers the active center. The 3D self-supporting nanostructures prepared by Song et al. (2022) can effectively reduce the interfacial resistance (Figure 3). The heterojunctions between metals and carbon-based nanostructured materials also promote electron migration (Louis et al., 2022; Yu et al., 2022). Surface functionalization for material can increase the surface-active sites but reduce the conductivity. Li’s team constructed coaxially structured double-walled carbon nanotubes (DWNTs), functionalizing the outer wall while providing a conductive pathway on the inner wall (Li Y. et al., 2022).
FIGURE 3. (A) Self-supporting carbon nanofibers; (B) Graphitized carbon nanofibers; (C) Nanorod arrays; (D) Surface functionalized carbon nanotubes (Zhao et al., 2018; Wu Y. et al., 2022; Yu et al., 2022; Zhong et al., 2022).
The ability to transport substances directly affects the reactants’ and intermediates’ adsorption and the diffusion of products. For HER, the reactants are H+ under acidic conditions and water under alkaline conditions (Zheng et al., 2021; Cheng et al., 2022). In contrast, the reactants are water under acidic conditions and OH− under alkaline conditions for OER (Sultan et al., 2019). Therefore, the hydrophilic or hydrophobic nature of the catalyst influences the efficiency of the reaction (Li et al., 2020). The transfer of intermediates is noticeable, and the hollow or porous structure provides short cuts for fast transportation of mass during the reaction (Liu et al., 2022; Wang et al., 2022). Since both HER and OER products are gaseous, it is essential to avoid bubble accumulation (Chen X. et al., 2022). Wu and co-workers fabricated interlaced carbon nanofibers with large pores, facilitating mass transport and desorption of H2 (Wu Y. et al., 2022). Xiao’s team prepared nanoarrays with good electrolyte penetration and weak bubble adsorption (Xiao et al., 2022).
Enhancing the mechanical strength of the structures is key to obtaining good stability for long-term electrolysis. The bubbles from hydrolysis may damage the nanostructure, herein, many researchers have devoted large efforts to strengthen the mechanical structure through etching and annealing (Wehrhold et al., 2022). Wu Y. et al. (2022) synthesized a robust carbon nanocatalyst with array structures which could maintain stable electrocatalysis for up to 100 h, as well as providing a high current density of 1000 mA cm−2. The self-supported carbon nanofibers also have a stable structure and show long-term performance for tens of hours in both HER and OER (Yu et al., 2022; Zhong et al., 2022). Long reaction times tend to cause aggregation of active particles. Liu F. et al. (2022) prepared graphitic carbon-coated active particles that remain dispersed for up to 60 h in electrolysis reaction. The excellent core-shell structure can also tolerate harsh catalytic environments.
For the water-splitting catalysts, nanostructures such as porous, layered, or arrays not only effectively provide a larger specific surface area to increase intermediate adsorption but also facilitate the diffusive transfer of products, intermediates, or other substances. The construction of heterojunction or self-supporting structures can sufficiently reduce the electron transfer resistance and improve the overall reaction kinetics (Chaitoglou et al., 2022).
ORR is a core reaction of fuel cells. In aqueous solutions, O2 can be reduced to water by a four-electron transfer pathway or to hydrogen peroxide by a two-electron transfer pathway (Luis-Sunga et al., 2020). The complex mechanisms, weak O2 adsorption, and O-O bond activation/cleavage greatly hinder the developments of ORR-based technologies (Xu et al., 2020).
To expose more active sites, obtaining a high specific surface area, improving the catalyst structure, loading active particles on carbon-based substrates, or introducing functional groups are effective strategies. For example, the as-prepared, metal-free mesoporous carbons possessed a high density of N-containing active sites and a high specific surface area (Ilnicka et al., 2021). Zan et al. (2021) prepared an ultrathin carbon nanosheet with a thickness of 1–2 nm, which can incredibly increase the specific surface area and obtain a larger current density. Liu’s team embedded Pt3Co into the unclosed mesopores, which enabled it evenly disperses on the surface and sufficiently in contact with the reaction medium (Liu J. et al., 2022).
ORR is a multi-electron transfer process, in which the timely availability of electrons facilitates the reaction, and highly conductive catalytic materials also increase O2 adsorption (Luis-Sunga et al., 2020). A series of nanoporous carbon were synthesized and compared with Pt/C electrodes, confirming the more excellent electrical conductivity (Fernandes et al., 2020). In the case of utilizing transition metals coupled with carbon-based materials, the synergistic effect between the components enables the catalyst to produce a wealthy heterogeneous interface, which also facilitates electron transfer and enhances the selectivity of four-electron transfer (Zhou et al., 2020; Wu S. et al., 2022). The dendritic carbon structure composed of a carbon sphere core and graphene flap has excellent electrical conductivity, for the carbon sphere skeleton keeping the graphene layer from being squeezed to aggregate in the liquid phase (Feng et al., 2018).
A good structure increases the adsorption of O2 and facilitates the transfer of intermediates. Feng et al. (2018) synthesized catalysts that have more than twice the current density of Pt/C electrodes at the same catalyst loading due to the super absorption properties of graphene layers and oxygen bubbles stabilizing ability of dendritic interstices. The porous structure provides similar properties, with strong interaction with O2 bubbles (Lu et al., 2016; Ilnicka et al., 2021). In the porous nanosheet catalyst synthesized by Li et al. (2021) 17.4% of the macropores were for substance transport, and 82.6% of the mesopores were for the diffusion of reactants and products. Carbon nanohorns have conical tips where most of the defects are located and need to open the tips with modification to facilitate material transfer (Kagkoura et al., 2020) (Figure 4).
FIGURE 4. (A) Tip-opened carbon nanohorn; (B) dendritic structures consist of carbon sphere cores and graphene sheets (Feng et al., 2018; Kagkoura et al., 2020).
Stability is an important property for industrial applications, and the encapsulation of metal particles with excellent ORR catalytic activity can effectively avoid recrystallization (He M. et al., 2022). Guo’s team prepared the leaf-like porous nanosheet with a core-shell structure that has more remarkable stability than the Pt/C electrode, for its potential decreases almost negligible after reacting at the constant current of 5 mA cm−2 exceeds 110 h, the Pt/C electrode exhibited a significant potential drop within 70 h (Guo et al., 2022). Anchoring small-sized CoN nanoparticles between ultra-thin nanosheets provide both active sites and less nanosheet aggregation, which remains stable after 350 h (Wu S. et al., 2022).
For ORR, the utilization of carbon nanomaterials not only enables fully exposure of active sites, but also increases O2 adsorption. These characteristics are benefit to the opening of O-O bond. And the high electron transfer rate on the surface of the carbon-based catalysts can largely improve the reaction efficiency. The carbon-based catalysts’ stability can be easily achieved by coating or loading various particles.
In CO2RR, CO2 is converted into fuels or other value added products such as methanol, formic acid, methane (Deng et al., 2022a; Xuan et al., 2022). CO2RR typically involves three steps. First, the adsorption and activation of CO2 to form intermediates. Second, proton coupling and electron transferring, Third, desorption of the resulting products (Tan et al., 2022).
CO2 molecule has a stable linear structure which requires large energy input to evoke and low solubility in commonly used electrolytes. Therefore, the highly active CO2RR catalysts should possess the characteristics of suitable CO2 thermodynamic adsorption strength and sufficient exposed active sites (Melchionna et al., 2021; Li L. et al., 2022). Liang et al. (2022) found that the sample with the largest micropore-specific surface area has a larger current density. Zhu’s team prepared arrays to prevent the aggregation of nanorods and nanosheets, and the optimal specific surface area result in greater current density under the same voltage and the best CO selectivity (Zhu et al., 2019). In addition, supporting the active particles or single atoms on carbon material can expose the active site to the reaction medium sufficiently and obtain better catalytic efficiency. For example, Wu’s team embedded the nickel (Ni) monatomic site in carbon nanotubes which the FE of CO can be as high as 98% at a low potential of −0.65 V. Since the abundant mesopores fix the metal nanoparticles in the pores evenly, effectively preventing migration and aggregation (Wu Y. et al., 2022).
For CO2RR, a multi-electron transfer process, improving the conductivity of the catalyst material can accelerate the reaction and ensure the ability to provide enough protons and electrons consistently, which is relevant to the product (Askins et al., 2021). The rich porous structure is beneficial for electron transport, and the layered and array structure can also improve the electron transport capacity (Tuo et al., 2019; Zhu et al., 2019; He C. et al., 2022). Compared with adsorption or drop coating, the structure loaded with active particles has lower interface resistance and better electron transport ability (Liu et al., 2020). The GQDs consist of single or few graphene sheets with a considerable number of edge sites that make them conductive (Hoang et al., 2019). Therefore, the graphitization of materials can also effectively accelerate electron transmission (He C. et al., 2022).
CO2RR is usually performed in an aqueous electrolyte, forming a gas-liquid-solid three-phase interface with low CO2 solubility, which requires consideration of mass transfer, which is also key to the proton coupling step (Tan et al., 2022). MXene has a stable layering which, in addition to exposing the active site, facilitates the diffusion of the substance (VahidMohammadi et al., 2021). Sun et al. (2022) found that materials with rich micropores have a higher specific surface area for absorbing CO2, and bigger diameter pores are conducive to accelerating the diffusion of electrolytes and increasing the wet electrode area. The hydrophobic structure creates a microenvironment that facilitates the diffusion of CO2 (Xing et al., 2021). For example, the rich mesoporous nanotubes prepared by Du’s team have good catalytic properties due to the hydrophobicity, which also inhibits HER (Du et al., 2022).
The collapse of the catalyst structure will result in the active site’s absence (Xuan et al., 2020). The active particles fixed by pores are stable for their uniform dispersion, and both CO FEs and partial current density retain about 90% of the original value during a 60 h electrolysis (He C. et al., 2022). The arrays reacted for 30 h, showing an almost negligible reduction in current density attenuation, indicating excellent durability (Zhu et al., 2019). Graphene sheets provide a significant specific surface with abundant active sites but are destroyed easily during the reaction. Etching not only accelerates electron transfer but also further increases strength (Shao et al., 2022).
Micropores are the best for CO2 adsorption and facilitate CO2 activation, while macropores allow for better mass transfer. The increased electron transfer capacity of the graphitized and active particle-loaded structures also increases the selectivity of CO2RR, determined by the multiple electron transfer and product diversity. At the same time, these structures also effectively improve stability and are a guideline for designing catalysts for industrial applications where long catalytic times are required.
Ammonia synthesis using NRR allows efficient energy storage and is an alternative to the Haber-Bosch process that requires high-pressure and high-temperature conditions (Lv et al., 2018; Wu et al., 2021). The NRR process is similar to CO2RR while the triple bond structure of N2 is more stable than the CO2 molecule.
Performing adsorption and dissociation for chemically inert N2 molecules is still a challenge to overcome (Westhead et al., 2021). In addition to constructing effective active sites, the key to increasing contact between active sites and N2 molecules is the active specific surface area (Lv et al., 2018). Synthesizing a mesopore-rich structure is a direct way (Hu et al., 2020). 1D CDs have a high specific surface area due to their size characteristics. Han et al. (2022) reported the successful CQDs with only about 2 nm diameter, and the generous oxygen-containing groups covered the surface, which dispersed them in aqueous or non-aqueous media uniformly to promote the contact of active site and reaction medium. The monoatomic loading of the bimetal on the carbon matrix results in the abundance and dispersion of active sites (Zhang et al., 2022).
The electron transport capacity is critical after dissociation and affects the overall rate of the reaction. The B/N codoped porous carbon nanotubes have a tubular structure that not only allows them to expose more active sites but also maximizes the use of electrode materials and shortens the electron diffusion path (Shi et al., 2022). MXene relies on structurally optimized smaller layer spacing to support electron transfer (VahidMohammadi et al., 2021). Besides, the better the graphitization, the higher the FE of NH3, which correlates with good electron transport ability (Wen et al., 2021).
The accumulation of products on the electrode surface will increase the resistance, and the stable supply of protons is related to both N2 adsorption and NH3 formation (Westhead et al., 2021). Ma et al. (2022) found that Fe and N-doped porous carbon has the lowest Faraday efficiency and NH3 yield at the highest specific surface area of the micropores, which might be attributed to the too-small voids hindering the mass transfer. The structures of average pore diameter concentrate between 1.7 and 5 nm can provide abundant channels for the transmission of gas molecules and the diffusion of active ions (Shi et al., 2022).
Catalysts tend to structurally collapse during catalysis, appearing to undergo a catastrophic loss of efficiency (Westhead et al., 2021). Liu et al. (2021b) used Co and Mo doping to immobilize the active N atoms, forming a single-atom catalyst, that is, stable and efficient. Wang’s team prepared the GO nanoflakes covered C=O group on the surface can effectively adsorb and activate N2 and maintain a stable current density response without conspicuous fluctuation within 30 h (Wang et al., 2020). The B-enriched BCN nanomesh prepared by Chang et al. (2021) retains catalytic activity after a electrolytic process for 36 h.
NRR, as an emerging electrocatalytic process, has drawn increasing attention. However, only few researchers have employed carbon nanomaterials in NRR. And according to the reports recently, most of the researchers mainly focused on increasing the nitrogen molecules adsorption and activation, which is similar to that of the CO2RR. It is reasonable to predict that carbon nanomaterials will play an important role in the future studies on NRR.
In summary, carbon nanomaterials with remarkable properties such as large specific surface area, good electrical conductivity, abundant surface functional groups, high plasticity, and low price have great potential for application in electrocatalysis. Currently, researchers mainly prepare graded porous structures to increase the exposure of active sites and improve mass transfer; grapheneize the materials or hybridize with graphene structures and reduce the interfacial resistance to improve electron transport; use nanocarbon as a substrate to support the active sites to increase the stability. However, there are still numerous challenges waiting to be conquered.
1) Different properties are difficult to ensure at the same time. For example, graphitization facilitates electron transport, but the original structure is hardly maintained at high temperatures, resulting in the absence of active sites.
2) It is uncontrollable to prepare needed structure. Some carbon nanomaterials with high selectivity, such as single atoms and nanoarrays carbon nanomaterials, are hard to prepare and require relatively harsh preparation conditions, leading to low economic efficiency.
3) It is difficult for industrial applications. For industrial applications, catalysts require higher current density and prolonged stability, as well as better durability to cope with the possible strong acid and alkali environment caused by uneven diffusion in industry.
MY: Resources, writing—original draft. MW: Resources. MZ: Graph editing, writing—review and editing. XS: supervision, writing—review and editing. XX: Investigation, writing—review and editing, conceptualization, supervision.
This work was financially supported by the National Natural Science Foundation of China (Grant No. 52106257), the Natural Science Foundation of Jiangsu Province (Grant No. BK20210112), the Natural Science Foundation of Shandong Province (Grant No. ZR2021QE017), Hong Kong Scholars Program (Grant No. XJ2021030), and the Fundamental Research Funds for the Central Universities (Grant No. 2020GN050).
The authors declare that the research was conducted in the absence of any commercial or financial relationships that could be construed as a potential conflict of interest.
All claims expressed in this article are solely those of the authors and do not necessarily represent those of their affiliated organizations, or those of the publisher, the editors and the reviewers. Any product that may be evaluated in this article, or claim that may be made by its manufacturer, is not guaranteed or endorsed by the publisher.
Asefa, T., Tang, C., and Ramírez-Hernández, M. (2021). Nanostructured carbon electrocatalysts for energy conversions. Small 17, 2007136. doi:10.1002/smll.202007136
Askins, E. J., Zoric, M. R., Li, M., Luo, Z., Amine, K., and Glusac, K. D. (2021). Toward a mechanistic understanding of electrocatalytic nanocarbon. Nat. Commun. 12, 3288. doi:10.1038/s41467-021-23486-1
Cai, R., You, B., Chen, M., and Wu, L. (2019). Metal-free core-shell structured N-doped carbon/carbon heterojunction for efficient CO2 capture. Carbon 150, 43–51. doi:10.1016/j.carbon.2019.05.001
Chaitoglou, S., Amade, R., and Bertran, E. (2022). Insights into the inherent properties of vertical graphene flakes towards hydrogen evolution reaction. Appl. Surf. Sci. 592, 153327. doi:10.1016/j.apsusc.2022.153327
Chang, B., Li, L., Shi, D., Jiang, H., Ai, Z., Wang, S., et al. (2021). Metal-free boron carbonitride with tunable boron Lewis acid sites for enhanced nitrogen electroreduction to ammonia. Appl. Catal. B Environ. 283, 119622. doi:10.1016/j.apcatb.2020.119622
Chen, L., Msigwa, G., Yang, M., Osman, A. I., Fawzy, S., Rooney, D. W., et al. (2022a). Strategies to achieve a carbon neutral society: A review. Environ. Chem. Lett. 20, 2277–2310. doi:10.1007/s10311-022-01435-8
Chen, X., Yu, B., Dong, Y., Zhu, X., Zhang, W., Ramakrishna, S., et al. (2022b). Electrospun porous carbon nanofibers decorated with iron-doped cobalt phosphide nanoparticles for hydrogen evolution. J. Alloys Compd. 918, 165733. doi:10.1016/j.jallcom.2022.165733
Cheng, C., Deng, M., Li, L., and Wei, Z. (2022). The contribution of water molecules to the hydrogen evolution reaction. Sci. China Chem. 65, 1854–1866. doi:10.1007/s11426-022-1371-x
Deng, C., Lin, R., Kang, X., Wu, B., Ning, X., Wall, D., et al. (2022a). Co-production of hydrochar, levulinic acid and value-added chemicals by microwave-assisted hydrothermal carbonization of seaweed. Chem. Eng. J. 441, 135915. doi:10.1016/j.cej.2022.135915
Deng, C., Lin, R., Kang, X., Wu, B., Wall, D., and Murphy, J. D. (2022b). Improvement in biohydrogen and volatile fatty acid production from seaweed through addition of conductive carbon materials depends on the properties of the conductive materials. Energy 239, 122188. doi:10.1016/j.energy.2021.122188
Domingo-Tafalla, B., Martínez-Ferrero, E., Franco, F., and Palomares-Gil, E. (2022). Applications of carbon dots for the photocatalytic and electrocatalytic reduction of CO2. Molecules 27, 1081. doi:10.3390/molecules27031081
Du, J., Chen, A., Hou, S., and Guan, J. (2022). CNT modified by mesoporous carbon anchored by Ni nanoparticles for CO2 electrochemical reduction. Carbon Energy 223, 1274–1284. doi:10.1002/cey2.223
Feng, W.-J., Lin, Y.-X., Zhao, T.-J., Zhang, P.-F., Su, H., Lv, L.-B., et al. (2018). Direct reduction of oxygen gas over dendritic carbons with hierarchical porosity: Beyond the diffusion limitation. Inorg. Chem. Front. 5, 2023–2030. doi:10.1039/C8QI00356D
Fernandes, D. M., Mestre, A. S., Martins, A., Nunes, N., Carvalho, A. P., and Freire, C. (2020). Biomass-derived nanoporous carbons as electrocatalysts for oxygen reduction reaction. Catal. Today 357, 269–278. doi:10.1016/j.cattod.2019.02.048
Guo, F., He, Y., Zeng, H., Liu, H., Yang, D., Chen, H., et al. (2022). Surface engineering of ZIF-L renders multidoped leaf-like porous carbon nanosheets for highly efficient oxygen reduction reaction in both alkaline and acidic media. Colloids Surf. Physicochem. Eng. Asp. 648, 129417. doi:10.1016/j.colsurfa.2022.129417
Han, Y., Ding, X., Han, J., Fang, Y., Jin, Z., Kong, W., et al. (2022). Oxygen-regulated carbon quantum dots as an efficient metal-free electrocatalyst for nitrogen reduction. Nanoscale 14, 9893–9899. doi:10.1039/D2NR01551J
He, C., Wang, S., Jiang, X., Hu, Q., Yang, H., and He, C. (2022a). Bimetallic cobalt–copper nanoparticle-decorated hollow carbon nanofibers for efficient CO2 electroreduction. Front. Chem. 10, 904241. doi:10.3389/fchem.2022.904241
He, M., Song, S., Wang, P., Fang, Z., Wang, W., Yuan, X., et al. (2022b). Carbon doped cobalt nanoparticles stabilized by carbon shell for highly efficient and stable oxygen reduction reaction. Carbon 196, 483–492. doi:10.1016/j.carbon.2022.05.019
Hoang, V. C., Dave, K., and Gomes, V. G. (2019). Carbon quantum dot-based composites for energy storage and electrocatalysis: Mechanism, applications and future prospects. Nano Energy 66, 104093. doi:10.1016/j.nanoen.2019.104093
Ilnicka, A., Skorupska, M., Tyc, M., Kowalska, K., Kamedulski, P., Zielinski, W., et al. (2021). Green algae and gelatine derived nitrogen rich carbon as an outstanding competitor to Pt loaded carbon catalysts. Sci. Rep. 11, 7084. doi:10.1038/s41598-021-86507-5
Jin, H., Guo, C., Liu, X., Liu, J., Vasileff, A., Jiao, Y., et al. (2018a). Emerging two-dimensional nanomaterials for electrocatalysis. Chem. Rev. 118, 6337–6408. doi:10.1021/acs.chemrev.7b00689
Jin, H., Liu, X., Jiao, Y., Vasileff, A., Zheng, Y., and Qiao, S.-Z. (2018b). Constructing tunable dual active sites on two-dimensional C3N4@Mon hybrid for electrocatalytic hydrogen evolution. Nano Energy 53, 690–697. doi:10.1016/j.nanoen.2018.09.046
Jin, H., Liu, X., Vasileff, A., Jiao, Y., Zhao, Y., Zheng, Y., et al. (2018c). Single-crystal nitrogen-rich two-dimensional Mo5N6 nanosheets for efficient and stable seawater splitting. ACS Nano 12, 12761–12769. doi:10.1021/acsnano.8b07841
Jin, H., Song, T., Paik, U., and Qiao, S.-Z. (2021). Metastable two-dimensional materials for electrocatalytic energy conversions. Acc. Mat. Res. 2, 559–573. doi:10.1021/accountsmr.1c00115
Jin, H., Yu, H., Li, H., Davey, K., Song, T., Paik, U., et al. (2022). MXene analogue: A 2D nitridene solid solution for high-rate hydrogen production. Angew. Chem. Int. Ed. 61, e202203850. doi:10.1002/anie.202203850
Joo, S. H., Choi, S. J., Oh, I., Kwak, J., Liu, Z., Terasaki, O., et al. (2001). Ordered nanoporous arrays of carbon supporting high dispersions of platinum nanoparticles. Nature 412, 169–172. doi:10.1038/35084046
Kagkoura, A., Arenal, R., and Tagmatarchis, N. (2020). Sulfur-doped carbon nanohorn bifunctional electrocatalyst for water splitting. Nanomaterials 10, 2416. doi:10.3390/nano10122416
Khan, A., Sarfraz, S., Rahman, A. U., and Muhammad, S. (2022). Correction: Novel crystalline reduced graphene oxide/adhesive nanocomposites for enhanced electrical, thermal, dielectric properties and electromagnetic energy absorption application. Carbon Res. 1, 28. doi:10.1007/s44246-022-00028-y
Li, C., Lv, Q., Wu, Y., Wu, X., and Tropea, C. (2020). Measurement of transient evaporation of an ethanol droplet stream with phase rainbow refractometry and high-speed microscopic shadowgraphy. Int. J. Heat Mass Transf. 146, 118843. doi:10.1016/j.ijheatmasstransfer.2019.118843
Li, L., Sun, Y., and Xie, Y. (2022a). Catalysts design for CO2 electroreduction. Sci. China Chem. 65, 425–427. doi:10.1007/s11426-021-1137-3
Li, Y., Li, W., Liu, D., Chen, T., Jia, S., Yang, F., et al. (2022b). Carboxyl functionalized double-walled carbon nanotubes for oxygen evolution reaction. Electrochimica Acta 419, 140395. doi:10.1016/j.electacta.2022.140395
Liang, X., Tian, N., Zhou, Z., and Sun, S. (2022). N, P dual-doped porous carbon nanosheets for high-efficiency CO2 electroreduction. ACS Sustain. Chem. Eng. 10, 1880–1887. doi:10.1021/acssuschemeng.1c07601
Liu, F., Tang, Y., Zhao, J., Bai, Y., Chen, J., Tian, L., et al. (2022a). Carbon dots-induced carbon-coated Ni and Mo2N nanosheets for efficient hydrogen production. Electrochimica Acta 424, 140671. doi:10.1016/j.electacta.2022.140671
Liu, H., Guan, J., Yang, S., Yu, Y., Shao, R., Zhang, Z., et al. (2020). Metal–organic-framework-derived Co2P nanoparticle/multi-doped porous carbon as a trifunctional electrocatalyst. Adv. Mat. 32, 2003649. doi:10.1002/adma.202003649
Liu, J., Zhang, J., Xu, M., Tian, C., Zeng, X., and Wang, C. (2022b). Highly dispersed PT3CO nanocatalysts embedded in porous hollow carbon spheres with efficient electrocatalytic O2-reduction and H2-evolution activities. ACS Appl. Energy Mat. 5, 4496–4504. doi:10.1021/acsaem.1c04081
Liu, K., Jin, H., Huang, L., Luo, Y., Zhu, Z., Dai, S., et al. (2022). Puffing ultrathin oxides with nonlayered structures. Sci. Adv. 8, eabn2030. doi:10.1126/sciadv.abn2030
Liu, Y., Roy, S., Sarkar, S., Xu, J., Zhao, Y., and Zhang, J. (2021a). A review of carbon dots and their composite materials for electrochemical energy technologies. Carbon Energy 3, 795–826. doi:10.1002/cey2.134
Liu, Y., Zhang, S., Li, W., Zhou, H., Wang, G., and Zhang, H. (2021b). Metal (Co/Mo)–N bond anchor-doped N in porous carbon for electrochemical nitrogen reduction. Inorg. Chem. Front. 8, 1476–1481. doi:10.1039/D0QI01324B
Louis, H., Ikenyirimba, O. J., Unimuke, T. O., Mathias, G. E., Gber, T. E., and Adeyinka, A. S. (2022). Electrocatalytic activity of metal encapsulated, doped, and engineered fullerene-based nanostructured materials towards hydrogen evolution reaction. Sci. Rep. 12, 15608. doi:10.1038/s41598-022-20048-3
Lu, Z., Xu, W., Ma, J., Li, Y., Sun, X., and Jiang, L. (2016). Superaerophilic carbon-nanotube-array electrode for high-performance oxygen reduction reaction. Adv. Mat. 28, 7155–7161. doi:10.1002/adma.201504652
Luis-Sunga, M., Rodríguez, J. L., García, G., and Pastor, E. (2020). Oxygen electroreduction reaction at bidimensional materials. Curr. Opin. Electrochem. 23, 139–144. doi:10.1016/j.coelec.2020.05.015
Lv, C., Qian, Y., Yan, C., Ding, Y., Liu, Y., Chen, G., et al. (2018). Defect engineering metal-free polymeric carbon nitride electrocatalyst for effective nitrogen fixation under ambient conditions. Angew. Chem. Int. Ed. 57, 10246–10250. doi:10.1002/anie.201806386
Ma, X., Gao, L., Zhang, Q., Hua, W., Zhang, Y., Li, X., et al. (2022). Nano-CaCO3 templated porous carbons anchored with Fe single atoms enable high-efficiency N2 electroreduction to NH3. Electrochimica Acta 426, 140805. doi:10.1016/j.electacta.2022.140805
Melchionna, M., Fornasiero, P., Prato, M., and Bonchio, M. (2021). Electrocatalytic CO2 reduction: Role of the cross-talk at nano-carbon interfaces. Energy Environ. Sci. 14, 5816–5833. doi:10.1039/D1EE00228G
Narwade, S. S., Mali, S. M., Sapner, V. S., and Sathe, B. R. (2020). Graphene oxide decorated with rh nanospheres for electrocatalytic water splitting. ACS Appl. Nano Mat. 3, 12288–12296. doi:10.1021/acsanm.0c02762
Qiao, Y., Pan, Y., Zhang, J., Wang, B., Wu, T., Fan, W., et al. (2022). Multiple carbon interface engineering to boost oxygen evolution of NiFe nanocomposite electrocatalyst. Chin. J. Catal. 43, 2354–2362. doi:10.1016/S1872-2067(21)63916-5
Shah, S. A., Shen, X., Xie, M., Zhu, G., Ji, Z., Zhou, H., et al. (2019). Nickel@nitrogen-doped carbon@MoS2 nanosheets: An efficient electrocatalyst for hydrogen evolution reaction. Small 15, 1804545. doi:10.1002/smll.201804545
Shao, S., Wen, T., Wang, Z., Yin, X., Liu, Y., Yang, W., et al. (2022). Fabrication of SnSe2-graphene nanosheets for highly effectively electrocatalytic reduction of CO2. Electrochimica Acta 434, 141331. doi:10.1016/j.electacta.2022.141331
Shi, L., Bi, S., Qi, Y., He, R., Ren, K., Zheng, L., et al. (2022). Anchoring Mo single-atom sites on B/N codoped porous carbon nanotubes for electrochemical reduction of N2 to NH3. ACS Catal. 12, 7655–7663. doi:10.1021/acscatal.2c01293
Song, H., Yu, J., Tang, Z., Yang, B., and Lu, S. (2022). Halogen-doped carbon dots on amorphous cobalt phosphide as robust electrocatalysts for overall water splitting. Adv. Energy Mat. 12, 2102573. doi:10.1002/aenm.202102573
Sultan, S., Tiwari, J. N., Singh, A. N., Zhumagali, S., Ha, M., Myung, C. W., et al. (2019). Single atoms and clusters based nanomaterials for hydrogen evolution, oxygen evolution reactions, and full water splitting. Adv. Energy Mat. 9, 1900624. doi:10.1002/aenm.201900624
Sun, M., Pan, D., Ye, T., Gu, J., Zhou, Y., and Wang, J. (2022). Ionic porous polyamide derived N-doped carbon towards highly selective electroreduction of CO2. Chin. J. Chem. Eng. S100495412200249X. doi:10.1016/j.cjche.2022.05.030
Tan, X., Sun, X., and Han, B. (2022). Ionic liquid-based electrolytes for CO2 electroreduction and CO2 electroorganic transformation. Natl. Sci. Rev. 9, nwab022. doi:10.1093/nsr/nwab022
VahidMohammadi, A., Rosen, J., and Gogotsi, Y. (2021). The world of two-dimensional carbides and nitrides (MXenes). Science 372, eabf1581. doi:10.1126/science.abf1581
Wang, S., Zhu, J., Zhang, Y., Liu, Q., Chen, G., and Kong, X. (2020). Identifying the active site on graphene oxide nanosheets for ambient electrocatalytic nitrogen reduction. Inorg. Chem. 59, 11108–11112. doi:10.1021/acs.inorgchem.0c01596
Wang, X., Vasileff, A., Jiao, Y., Zheng, Y., and Qiao, S. (2019a). Electronic and structural engineering of carbon-based metal-free electrocatalysts for water splitting. Adv. Mat. 31, 1803625. doi:10.1002/adma.201803625
Wang, X., Vasileff, A., Jiao, Y., Zheng, Y., and Qiao, S. (2019b). Electronic and structural engineering of carbon-based metal-free electrocatalysts for water splitting. Adv. Mat. 31, 1803625. doi:10.1002/adma.201803625
Wang, X., Zhang, X., Fu, G., and Tang, Y. (2021). Recent progress of electrospun porous carbon-based nanofibers for oxygen electrocatalysis. Mat. Today Energy 22, 100850. doi:10.1016/j.mtener.2021.100850
Wang, Z., Shen, K., Chen, L., and Li, Y. (2022). Scalable synthesis of multi-shelled hollow N-doped carbon nanosheet arrays with confined Co/CoP heterostructures from MOFs for pH-universal hydrogen evolution reaction. Sci. China Chem. 65, 619–629. doi:10.1007/s11426-021-1175-2
Weber, D., Rui, N., Zhang, F., Zhang, H., Vovchok, D., Wildy, M., et al. (2022). Carbon nanosphere-encapsulated fe core–shell structures for catalytic co2 hydrogenation. ACS Appl. Nano Mat. 5, 11605–11616. doi:10.1021/acsanm.2c02602
Wehrhold, M., Neubert, T. J., Grosser, T., Vondráček, M., Honolka, J., and Balasubramanian, K. (2022). A highly durable graphene monolayer electrode under long-term hydrogen evolution cycling. Chem. Commun. 58, 3823–3826. doi:10.1039/D2CC00220E
Wen, Y., Zhu, H., Hao, J., Lu, S., Zong, W., Lai, F., et al. (2021). Metal-free boron and sulphur co-doped carbon nanofibers with optimized p-band centers for highly efficient nitrogen electroreduction to ammonia. Appl. Catal. B Environ. 292, 120144. doi:10.1016/j.apcatb.2021.120144
Westhead, O., Jervis, R., and Stephens, I. E. L. (2021). Is lithium the key for nitrogen electroreduction? Science 372, 1149–1150. doi:10.1126/science.abi8329
Wu, A., Yang, J., Xu, B., Wu, X.-Y., Wang, Y., Lv, X., et al. (2021). Direct ammonia synthesis from the air via gliding arc plasma integrated with single atom electrocatalysis Appl. Catal. B Environ. 299, 120667. doi:10.1016/j.apcatb.2021.120667
Wu, H., Lu, S., and Yang, B. (2022a). Carbon-dot-enhanced electrocatalytic hydrogen evolution. Acc. Mat. Res. 3, 319–330. doi:10.1021/accountsmr.1c00194
Wu, S., Deng, D., Zhang, E., Li, H., and Xu, L. (2022b). CoN nanoparticles anchored on ultra-thin N-doped graphene as the oxygen reduction electrocatalyst for highly stable zinc-air batteries. Carbon 196, 347–353. doi:10.1016/j.carbon.2022.04.043
Wu, Y., Liu, Y., Liu, B., Jiang, W., Zhou, T., Li, H., et al. (2022c). Vanadium nitride/carbon nanotube vertical nanoarrays on iron foam for oxygen evolution reaction. ACS Appl. Nano Mat. 5, 7714–7722. doi:10.1021/acsanm.2c00363
Xuan, X., Cheng, J., Yang, X., and Zhou, J. (2020). Highly Selective Electrochemical Reduction of CO2 to CH4 over Vacancy–Metal–Nitrogen Sites in an Artificial Photosynthetic Cell. ACS Sustain. Chem. Eng. 8, 1679–1686. doi:10.1021/acssuschemeng.9b07258
Xuan, X., Wang, M., Zhang, M., Kaneti, Y. V., Xu, X., Sun, X., et al. (2022). Nanoarchitectonics of low-dimensional metal-organic frameworks toward photo/electrochemical CO2 reduction reactions. J. CO2 Util. 57, 101883. doi:10.1016/j.jcou.2022.101883
Xia, C., Zhu, S., Feng, T., Yang, M., and Yang, B. (2019). Evolution and synthesis of carbon dots: From carbon dots to carbonized polymer dots. Adv. Sci. 6, 1901316. doi:10.1002/advs.201901316
Xiao, J., Tan, R., Guo, Y., Zhao, P., and Luo, X. (2022). Novel peapod-like Ni2P@N-doped carbon nanorods array on carbon fiber for efficient electrocatalytic urea oxidation and hydrogen evolution reaction. Int. J. Hydrog. Energy 47, 28730–28739. doi:10.1016/j.ijhydene.2022.06.181
Xing, Z., Hu, L., Ripatti, D. S., Hu, X., and Feng, X. (2021). Enhancing carbon dioxide gas-diffusion electrolysis by creating a hydrophobic catalyst microenvironment. Nat. Commun. 12, 136. doi:10.1038/s41467-020-20397-5
Xu, Z., Zhao, H., Liang, J., Wang, Y., Li, T., Luo, Y., et al. (2020). Noble-metal-free electrospun nanomaterials as electrocatalysts for oxygen reduction reaction. Mat. Today Phys. 15, 100280. doi:10.1016/j.mtphys.2020.100280
Yang, M., Lian, Z., Si, C., Jan, F., and Li, B. (2022). Revealing the intrinsic relation between heteroatom dopants and graphene quantum dots as a bi-functional ORR/OER catalyst. Mol. Catal. 518, 112109. doi:10.1016/j.mcat.2021.112109
Yu, S., Zou, Y., Wang, Q., Xu, J., Xiang, C., Xu, F., et al. (2022). Self-supported Co–Mo sulfide in electrospun carbon nanofibers as electrocatalysts for hydrogen evolution reaction in alkaline medium. J. Alloys Compd. 911, 165094. doi:10.1016/j.jallcom.2022.165094
Zan, Y., Zhang, Z., Zhu, B., Dou, M., and Wang, F. (2021). Ultrathin two-dimensional phosphorus and nitrogen Co-doped carbon nanosheet as efficient oxygen reduction electrocatalyst. Carbon 174, 404–412. doi:10.1016/j.carbon.2020.12.058
Zhang, S., Han, M., Shi, T., Zhang, H., Lin, Y., Zheng, X., et al. (2022). Atomically dispersed bimetallic Fe–Co electrocatalysts for green production of ammonia. Nat. Sustain., 1–11. doi:10.1038/s41893-022-00993-7
Zhang, W., Qiu, X., Wang, C., Zhong, L., Fu, F., Zhu, J., et al. (2022a). Lignin derived carbon materials: current status and future trends. Carbon Res. 1, 14. doi:10.1007/s44246-022-00009-1
Zhao, D., Li, L., Xie, L., Zhou, N., and Chen, S. (2018). Sulfur codoping enables efficient oxygen electroreduction on FeCo alloy encapsulated in N-Doped carbon nanotubes. J. Alloys Compd. 741, 368–376. doi:10.1016/j.jallcom.2018.01.144
Zheng, J., Xu, A., Wu, A., and Li, X. (2021). Plasma-engraved Co2N nanostructures toward high-performance alkaline hydrogen evolution. ACS Appl. Mater. Interfaces 13, 21231–21240. doi:10.1021/acsami.1c01698
Zhong, M., Song, N., Li, C., Wang, C., Chen, W., and Lu, X. (2022). Controllable growth of Fe-doped NiS2 on NiFe-carbon nanofibers for boosting oxygen evolution reaction. J. Colloid Interface Sci. 614, 556–565. doi:10.1016/j.jcis.2022.01.134
Zhou, Y., Tao, X., Chen, G., Lu, R., Wang, D., Chen, M.-X., et al. (2020). Multilayer stabilization for fabricating high-loading single-atom catalysts. Nat. Commun. 11, 5892. doi:10.1038/s41467-020-19599-8
Keywords: carbon nanomaterials, electrochemical, water splitting, ORR, CO2RR, NRR
Citation: Yang M, Wang M, Zhang M, Sun X and Xuan X (2022) Nanostructured carbon electrocatalysts for clean energy conversion and storage: A mini review on the structural impact. Front. Mater. 9:1090412. doi: 10.3389/fmats.2022.1090412
Received: 05 November 2022; Accepted: 08 December 2022;
Published: 19 December 2022.
Edited by:
Zaka Ullah, University of Education Lahore, PakistanReviewed by:
Huanyu Jin, University of Adelaide, AustraliaCopyright © 2022 Yang, Wang, Zhang, Sun and Xuan. This is an open-access article distributed under the terms of the Creative Commons Attribution License (CC BY). The use, distribution or reproduction in other forums is permitted, provided the original author(s) and the copyright owner(s) are credited and that the original publication in this journal is cited, in accordance with accepted academic practice. No use, distribution or reproduction is permitted which does not comply with these terms.
*Correspondence: Xiaoxu Xuan, eGlhb3h1eHVhbkBzZHUuZWR1LmNu
Disclaimer: All claims expressed in this article are solely those of the authors and do not necessarily represent those of their affiliated organizations, or those of the publisher, the editors and the reviewers. Any product that may be evaluated in this article or claim that may be made by its manufacturer is not guaranteed or endorsed by the publisher.
Research integrity at Frontiers
Learn more about the work of our research integrity team to safeguard the quality of each article we publish.