- 1Division of Cardiac Surgery, University of Ottawa Heart Institute, Ottawa, ON, Canada
- 2Department of Cellular and Molecular Medicine, University of Ottawa, Ottawa, ON, Canada
- 3Department of Biochemistry, Microbiology, and Immunology, Faculty of Medicine, University of Ottawa, Ottawa, ON, Canada
Nanomaterials have attracted the interest of tissue engineers for the last two decades. Their unique properties make them promising for de novo fabrication of bio-inspired hybrid/composite materials with improved regenerative properties, including, for example, the capacity for electric conductivity and the provision of antimicrobial properties. However, to this day, the use of such materials in medical applications is rather limited and most of the studies have only reached the archetypical proof-of-concept stage. Herein, we present a review on the use of nanomaterials in tissue engineering for regenerative therapies of heart, skin, eye, skeletal muscle, and nervous system. The advantages and limitations of nano-engineering materials are presented in this review alongside with the future challenges and milestones nanotechnology must overcome to make an impact in biomedical applications.
Introduction
In the early 2000s, it seemed that materials with improved regenerative properties would come from the combination of biocompatible platforms and nanometric materials (size scale 10−9 m) (Morrow et al., 2007). However, to this day, a relatively small number of materials based on or containing nanoparticles (NPs) are being used in medicine (Morrow et al., 2007; Etheridge et al., 2013). Some examples of nanomaterials used in the clinic are listed in Table 1. For example, the Nanotechnology Characterization Laboratory (NCL) in a joint effort with two pharmaceutical companies (AstraZeneca and Pfizer) (Pan, 2015) has supported the development of new nanotechnologies for cancer therapeutics (Morrow et al., 2007). However, despite the considerable improvement in product manufacturing practices over the past years, safety concerns for human use remain the biggest obstacle for effective translational applications. One of the main reasons for this is the limited amount of reliable, and sometimes contradictory, scientific literature regarding the toxicity of nanomaterials in living organisms, which is due in part to:
• Poor standardization for NP production, which in turn limits reproducibility and scale-up production, particularly in batch-to-batch consistency (Franca et al., 2013).
• Limited knowledge of long-term effects of nanomaterials in living organisms (Buzea et al., 2007; De Jong and Borm, 2008; Bondarenko et al., 2013).
• Most materials are mainly tested at the proof-of-concept stage in vitro, and follow-up publications are rarely seen [see (Pedrosa et al., 2015) for a specific discussion on gold nanoparticles (AuNPs)].
These issues have severely impacted the translation of nano-engineered materials for clinical uses, where incorporation of NPs within the 3D structure, see Figure 1, of the scaffold could help overcoming some of the most important limitations of regenerative scaffolds and therapies that include:
• Enhancement, or modulation, of the mechanical properties of the template (Banquy et al., 2009; Gaharwar et al., 2014).
• Manufacturing of environment responsive scaffolds (pH, ionic strength) see review by Gaharwar et al. (2014).
• Support of electrical conductivity (Dvir et al., 2011; Balint et al., 2014).
• Improved resistance to bacterial colonization (Alarcon et al., 2015, 2016).
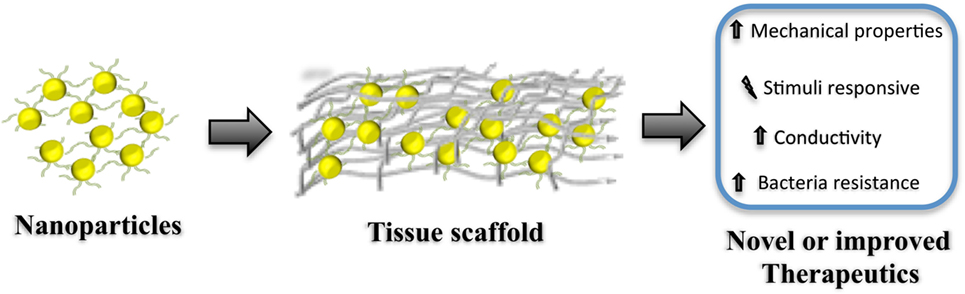
Figure 1. Diagram representation for the fabrication of hybrid regenerative templates (tissue scaffolds) containing nanoparticles. Note that this simplified representation does not consider covalent and non-covalent surface modification of the nanoparticle prior incorporation/preparation of the regenerative scaffold. Such scaffolds can be either applied in situ or used as an in vitro template. Incorporating nanoparticles within the scaffold can modify the mechanical–physical properties, produce stimuli-responsive materials, boost the conductivity of the scaffold, and even provide protection against bacterial colonization. Such materials can be seen as tools for developing new, or de novo, therapeutics for regenerative medicine.
Despite the increasing number of publications using hybrid/composite materials for biomedical applications seen in the past decade, the field is in its infancy in terms of understanding the complexity of nanoscale interactions between biopolymers and NPs. However, before we can fully understand, and eventually manipulate these interactions, we need to review the state-of-the-art on hybrid/composite materials for tissue engineering. Thus, herein, we discuss some relevant advances in regenerative materials/scaffolds that have employed nano-engineered components in their fabrication. We have further targeted inorganic NPs, and peptides reported for use in regeneration of the following organs: heart (see Nano-Engineered Materials for Cardiac Tissue Regeneration), skin (see Nano-Engineered Materials for Skin Wound Healing), eye (Section Nanomaterial Therapeutics for Eye Regeneration), skeletal muscle (SM; see Nano-Engineered Materials for Skeletal Muscle Repair), and nervous system (see Nanomaterials for Peripheral and Central Nervous System Regeneration). Additionally, we focused our literature selection on studies that included in vivo assessment of the materials, which we hope will help the reader to gain a more complete picture of the potential translational application of nano-engineered materials.
Nano-Engineered Materials for Cardiac Tissue Regeneration
Nanoparticle-Based Materials for Cardiac Tissue and Vascular Repair
Cardiovascular tissue engineering strategies have been investigated in order to regenerate or repair scar tissue and/or hibernating myocardium following ischemic injury, in particular myocardial infarction (MI) (Pfeffer and Braunwald, 1990). Heart failure following MI continues to be a prevalent complication, in spite of current surgical revascularization techniques, due to the limited regenerative capacity of cardiac muscle (Sutton and Sharpe, 2000). Nanomaterials have been incorporated as critical components of experimental tissue engineering strategies for regeneration and repair of cardiac tissue (see Nguyen et al., 2015b). Nanomaterials can convey many benefits to cardiac tissue engineering and regeneration strategies including:
i. Metal NPs and carbon nanotubes (CNTs) can increase the conductivity of biomaterial scaffolds (Shin et al., 2013; Shevach et al., 2014; Zhou et al., 2014).
ii. Nanofibers can be used to more closely mimic the nanotopography of the cardiac extracellular matrix (ECM), which allows for better cell connections, differentiation and organization (Davis et al., 2005; Mukherjee et al., 2011).
iii. Some NPs have inherent antioxidant properties, which could be beneficial for cell survival under conditions of oxidative stress within the infarct region (Niu et al., 2007).
In this section, we will review some representative examples for the use of nanomaterials in cardiac regenerative therapeutic applications. Table 2 presents a summary of the main findings of the technologies discussed in this section and in Figure 2, we schematically depicted some of the technologies discussed in this review.
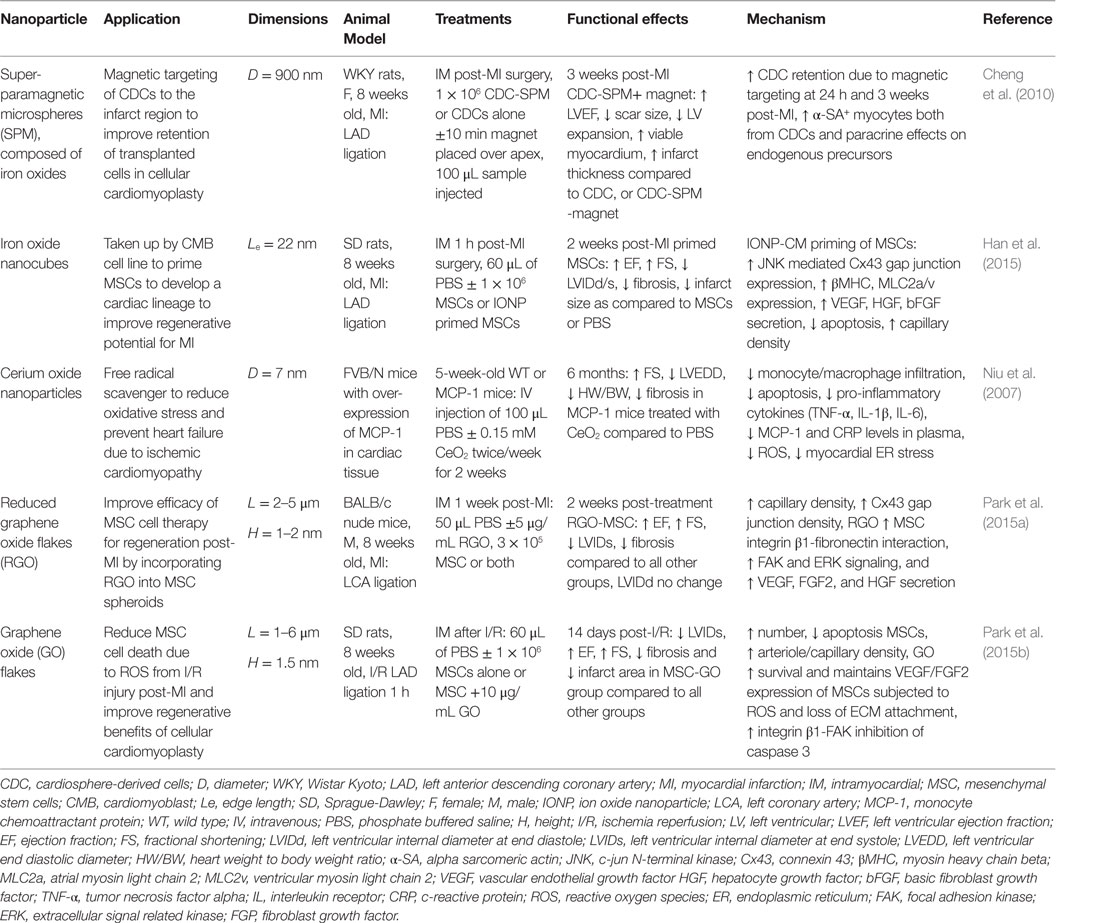
Table 2. Nanoparticles in treatment strategies for heart failure prevention: effects on cardiac function and infarct repair in vivo.
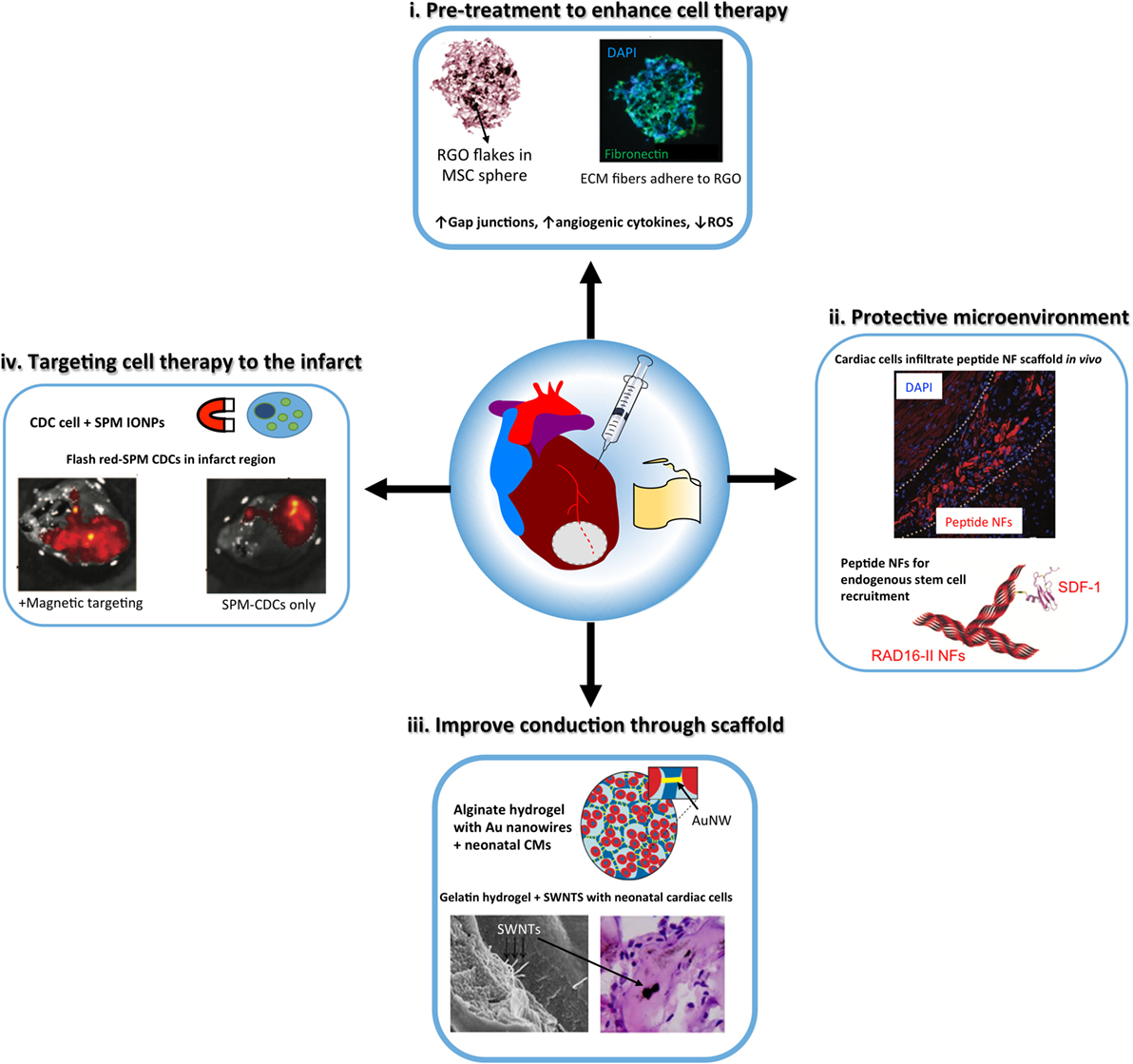
Figure 2. Schematic illustrating the properties of nanoparticles that can enhance the design and function of tissue engineering constructs, either injectable materials or cardiac patches, for cardiac regeneration. (i) metal oxide nanoparticles, including reduced graphene oxide (RGO) flakes have anti-oxtdant properties to protect stem cells [e.g., mesenchymal stem cells (MSCs)] delivered to the infarcted myocardium from reactive oxygen species (ROS). In addition, nanoparticles can prime stem cells to improve therapeutic efficacy [modified with permission from Park et al. (2015b)]. (ii) Peptide nanotiber (NF) scaffolds, including RAD16-II hydrogels form microenvironments that can recruit endogenous stem cells or protect exogenous transplanted cells to improve cardiac repair post-injury. Peptide NFs can be functionalized with growth factors or stem cell recruitment ligands, such as stromal cell-derived factor 1 (SDF-1) [modified with permission from Davis et al. (2005) and Segers et al. (2007)]. (iii) Gold nanowires (NW) and carbon nanotubes [such as single-wall nanotubes (SWNTs)] have been incorporated into tissue-engineered cardiac tissue constructs to improve conduction through the scaffold and cardiomyocyte (CM) maturation [modified with permission from Dvir et al. (2011) and Zhou et al. (2014)]. (iv) Superparamagnetic microspheres (SPM) of iron oxide nanoparticles have been used to reduce post-injection loss ol cardiosphere-derived cells (CDCs) by using a magnel to target the cell therapy to the infarct region [modified with permission from Cheng et al. (2010)].
Iron oxide nanoparticles (IONP) occupy a special place in cardiovascular therapies as diagnosis agents; however, they have also recently sparked interest in the field of regenerative therapies for cardiac tissue by allowing the manipulation of cells containing IONP with an external applied magnetic force (Ito et al., 2005; Cheng et al., 2010; Souza et al., 2010). Several superparamagnetic IONP formulations (a.k.a. SPIO) (3–10 nm diameter) have been approved by the Food and Drug Administration agency (FDA) as magnetic resonance imaging (MRI) contrast agents for inflammatory diseases, as they are readily taken up by monocytes and macrophages following intravenous injection (Reimer and Balzer, 2003; Li et al., 2013). Pre-clinical and clinical studies have shown benefits of SPIO contrast agents in MRI-based detection of the infarct region post-MI (Yilmaz et al., 2012, 2013). The first application of SPIO in cardiovascular regenerative medicine therapies was to label exogenous stem cells in order track these cells after injection into the infarct region in rodent models of MI (Kraitchman et al., 2003). While several studies show that IONPs do not affect cell viability, phenotype or therapeutic potential (Au et al., 2009; Li et al., 2013), other studies have raised concerns on their safety (Mahmoudi et al., 2011, 2012; Ge et al., 2013). Furthermore, their ability to track transplanted cells by MRI is controversial as the uptake of IONP by resident macrophages in the advent of transplanted cell apoptosis results in false-positive signals of cell engraftment in the infarct (Amsalem et al., 2007). In addition to their use in imaging studies, super-paramagnetic iron particles were used to reduce acute wash out of transplanted cells from the infarct region in a rat MI model by iron labeling cardiosphere-derived cells (CDCs) in vitro and then applying a magnetic force over the infarct post-injection (Cheng et al., 2010). This magnetic targeting technique was able to increase retention of CDCs in the infarct region, which lead to improve cardiac function, reduced ventricular remodeling, and reduced infarct expansion post-MI (Cheng et al., 2010). In tissue engineering applications, magnetic force has been used to successfully manipulate IONP labeled cells in culture to create 3D tissue arrangements, including 3D small-diameter vascular grafts with three layers of cells containing IONP (endothelial, smooth muscle, and fibroblast cell layers) (Ito et al., 2005; Souza et al., 2010). A novel application of IONP by (Han et al., 2015) was to enhance the cardiac lineage differentiation of mesenchymal stem cells (MSCs) prior to delivery to the infarct region post-MI in an effort to enhance cardiomyocytes regeneration. It was proposed that IONP triggered an intracellular signaling cascade, which enabled gap junctional coupling of IONP-cardiomyocytes with MSCs, and further activated cross-talk between cells. In this study, a co-culture system of MSCs with IONP-cardiomyoblasts was employed and the presence of IONP effectively increased cardiomyocyte (CM) markers, gap junctions, and pro-angiogenic cytokine expression in MSCs (Han et al., 2015). These primed MSCs were easily separated from the IONP-cardiomyoblasts via magnetic-activated cell sorting and had significantly greater therapeutic efficacy in a mouse MI model, in terms of preserved cardiac function, lower fibrosis, infarct scar area, and survival 2 weeks post-MI compared to unprimed MSCs (Han et al., 2015).
Gold nanoparticles (AuNPs) and gold nanorods (AuNRs) have been investigated in biomaterial scaffolds to boost conductivity in an effort to improve propagation of electrical signals through an engineered cardiac tissue, which will ultimately enhance in vivo integration. AuNRs were incorporated into alginate hydrogels to improve the electrical conductivity of the material and aid in the coupling of cardiomyocytes seeded within the scaffolds (Dvir et al., 2011). The incorporation of the nanorods (NRs) improved the connection and alignment of seeded cardiac cells (cardiomyocytes and fibroblasts), and increased gap junction proteins and expression of proteins involved in contraction and electrical conductivity (Dvir et al., 2011). AuNPs have been incorporated into decellularized omentum tissue scaffolds to decrease scaffold electrical resistance with the goal to produce autologous cardiac patches (Shevach et al., 2014). The incorporation of neonatal cardiac cells into these AuNP-conjugated scaffolds reduced the threshold required for contraction compared to the biomaterial alone, which was accompanied by greater gap junction protein expression, striation formation similar to cardiac muscle, and velocity of calcium of transients in response to electrical stimulation (Shevach et al., 2014). AuNPs have also been incorporated into thermo-responsive hydrogels of collagen nanofibers (Orza et al., 2011) and chitosan polymer (Baei et al., 2016) with the objective of forming injectable formulations for minimally invasive delivery of cells to the infarct. In the study by Orza et al., MSCs cultured AuNP-coated collagen fibers exhibited faster cardiac lineage differentiation after exposure to cardiac differentiation medium as compared to standard tissue culture substrate. Similarly, AuNPs enhanced the expression of cardiac lineage markers in MSCs cultured in chitosan hydrogels along with improving the conductivity of the material (Baei et al., 2016). Furthermore, AuNPs have shown antifibrogenic activity in vitro, by decreasing the percentage of fibroblasts with activated myofibroblast phenotype (Sisco et al., 2008). However, in spite of these advances in AuNPs scaffold development, no in vivo data are available to confirm a benefit of incorporating AuNPs into tissue-engineered scaffold on cardiac function post-MI in animal models. These in vivo studies will be necessary to determine if the injectable scaffold or cardiac patch can effectively propagate electrical signals in vivo without interfering with the directional signal propagation and cardiac contraction, which could produce arrhythmias.
The toxicity of AuNPs in vivo is controlled by many factors including: size, capping agents, and dosage (Khlebtsov and Dykman, 2011). A preliminary study by Spivak et al. indicated that spherical AuNPs of 30-nm diameter (3.19 g/mL metal concentration) could be intrapleurally delivered, which exhibited reduction in hydrothorax incidence and fibrosis in a doxorubicin induced rat model of heart failure similar to that of inotropic agent Simdax (Spivak et al., 2013). No appreciable toxicity due to AuNPs was noted over 6 months. Finally, another in vivo application of AuNPs was to improve the mechanical properties of porcine aorta decellularized vascular grafts by crosslinking them to mercaptoethylamine functionalized spherical AuNPs (100 nm) (Ostdiek et al., 2015). The grafts were able to integrate into the aortic tissue in a pig model, with cellular infiltration into the graft, without signs of inflammation or stenosis.
Nanomaterial allotropes of carbon, in particular CNTs and graphene oxide (GO) sheets, have received interest in the design of tissue engineering strategies to regenerate functional myocardium following infarction due to their high conductivity. CNTs have been investigated in vitro by Shin et al. who incorporated CNTs into a gelatin methylacrylate (GelMA) cross-linked hydrogel (Shin et al., 2013). The authors observed that CNTs provided electrical connections, similar to native Purkinje fibers in the heart and allowed conduction in a material that previously was an insulator (Shin et al., 2013). In addition, CM retention, viability, expression of contractile proteins, established striations, and functional gap junctions were enhanced on the scaffolds containing CNTs as compared to hydrogels alone. Furthermore, hydrogels containing CNTs were used as a substrate for the growth of 3D artificial tissues that exhibit beating under pulsatile stimulation (Shin et al., 2013). CNTs have also been investigated as a growth substrate, as they exhibit a topography similar to the native ECM with the ability to relay signals between myocytes (Martinelli et al., 2012). Cardiomyocytes grown on CNT substrates had increased viability, entrance into the cell cycle, and more negative resting membrane potential as compared to standard culture conditions (Martinelli et al., 2012). In vivo evidence in mice indicated that therapeutically relevant concentrations of CNTs are non-toxic; thus, there is promise for further in vivo studies with this nanomaterial (Schipper et al., 2008). Lastly, an engineered cardiac tissue consisting of neonatal rat cardiac cells in a gelatin hydrogel with single-walled CNTs (SWNTs) allowed spontaneous contraction and action potentials in vitro in addition to maturation of cardiomyocytes (Zhou et al., 2014). After suturing the graft onto the infarct region in a rat model, with immunosuppression therapy, improved cardiac function 4 weeks post-application and increase in gap junction protein expression was observed (Zhou et al., 2014). It is noted in this study that there was local macrophage upregulation in SWNT-graft treated mice leading to incorporation of SWNTs into the infarct region. As this study is performed with immunosuppression therapy, further investigation of long-term effects of CNTs in vivo, in particular the activation of an immune response in model with healthy immune function, is still needed.
Graphene oxide (GO) sheets are an alternative carbon-based nanomaterial, which have shown biocompatibility with no acute toxic effects in vivo (Paul et al., 2014; Park et al., 2015b). GO sheets and flakes with a thickness on the nanometer scale and length in the area of 1–6 μm are not taken up by cells but can absorb ECM proteins from culture serum (Lee et al., 2011). Studies by Park et al. demonstrated the benefit of incorporating GO or reduced GO flakes into MSC culture, with the following observations: (1) GO adsorbed ECM components (fibronectin) from the serum and formed GO-cell and cell-ECM interactions, which activate both pro-survival pathways and pro-angiogenic cytokine secretion and (2) the conductivity of GO stimulated expression of cardiac specific makers and gap junctions (Park et al., 2015b). The result in vivo in a rodent MI model showed enhanced retention of the MSC + GO in the infarct, reduced sensitivity to oxidative stress following ischemia reperfusion injury, and improvement of heart function by cellular cardiomyoplasty without an immune response to the GO material (Park et al., 2015a). Furthermore, polyehtylenimine functionalized GO sheets have been investigated as a transfection agent for gene therapy approaches to enhance angiogenic vascular endothelial growth factor (VEGF) gene expression using an injectable GelMA hydrogel (Paul et al., 2014). In a rat MI model, delivery of the composite GO-VEGF hydrogel lead to increased capillary density and decreased infract scar size (Paul et al., 2014). Injectable hydrogel formulations containing GO tethered VEGF plasmid were, therefore, an effective transfection system to enhance VEGF expression in the infarct region. Cerium oxide NPs act as free radical scavengers and have been shown to reduce pro-inflammatory cytokine expression due to reduction of endoplasmic reticulum (ER) activated reactive oxygen species (ROS) in a murine model of heart failure (Niu et al., 2007). The NPs improved heart function and limited ischemic remodeling. These results have been replicated in in vitro systems, including cardiomyocytes exposed to cigarette smoke extract and cardiac progenitor cells in culture in which CeO2 enhanced survival and maintenance of multi-lineage differentiation (Niu et al., 2007; Pagliari et al., 2012).
Nanofibrous Materials for Cardiac Tissue Repairing
Injectable biomaterials composed of hydrated natural or synthetic polymers solutions have been used as therapy for treating hearts post-MI (Christman and Lee, 2006). These hydrogels can limit wall stress that leads to pathological dilative remodeling of the ventricle as well as provide a protective environment to improve retention and function of transplanted cells (Johnson and Christman, 2013). Another ECM therapy approach is the fabrication of nanofiber matrices (Zhang, 2003). Nanofiber diameter can be controlled so that it is to scale with that of native ECM fibers and can offer greater surface area for cell engraftment (Mukherjee et al., 2011). Several nanofiber meshes made from synthetic polymers functionalized with native ECM proteins have shown in vitro the ability to produce scaffolds with similar topology to heart tissue, with aligned rabbit cardiomyocytes that express mature CM contractile protein and gap junction markers as compared to traditional culture techniques (Kai et al., 2011). Furthermore, nanofibers have been implemented in vascular grafts to provide a bioadsorbable scaffold and allow for neo-arteriogenesis and improved long-term graft patency (Pektok et al., 2008; Wu et al., 2012).
Peptide nanofiber injectable hydrogels have been used successfully for both cell and growth factor (GF) delivery in promoting regeneration post-MI (Hynesl et al., 1995). Table 3 summarizes some studies that have shown increased cardiac function post-MI when using peptide nanofiber-based tissue regeneration strategies. Nanofibers are formed by the self-assembly of short oligopeptides, with alternating hydrophilic and hydrophobic amino acid residues, when they introduced to neutral pH and osmolality (Zhang, 2003). Seminal in vivo studies by Davis et al., 2005, indicated that the porous hydrogel material from RAD16-II peptides (AcN-RARADADARARADADA-NH2) could be injected into the healthy myocardium without eliciting an immune response. This material was biodegradable and provided a microenvironment that recruited endothelial, smooth muscle and myocyte precursor cells (Davis et al., 2005). In MI animal models, peptide nanofibers, made from RAD16-II, have shown the capacity to increase exogenous cell retention in the infarct and facilitate the controlled release of GFs to prevent CM apoptosis or recruit endogenous precursor cells; all with the outcome of improving cardiac function (Davis et al., 2006). Furthermore, a study by Lin et al. concluded that in a porcine model the peptide nanofiber scaffold was not only a passive delivery vehicle but also improved diastolic function post-MI through reduction of fibrosis, neo-capillary formation and reduced ventricular remodeling (Lin et al., 2010). Similar studies in small animal models showed positive, yet non-significant, effects of nanofibers alone; however, combined with the incorporation of GFs or endogenous stem cell recruitment factors significantly improved cardiac function was achieved (Davis et al., 2006; Lin et al., 2012). Peptide nanofiber-based scaffolds represent a bottom-up approach to fiber formation where small, chemically synthesized oligopeptides are the building blocks. A benefit of this approach is that the peptide building block sequence can be customized to the needs of the application, as seen in a study by Ban et al. in which amphiphilic peptides were synthesized with a matrix metalloproteinase-2 (MMP2) and integrin binding cell adhesion sequence to replicate native cues for CM attachment prior to material injection (Ban et al., 2014). Further work has aimed to enhance the angiogenic capacity of self-assembling peptides. Kumar et al., for instance, developed a peptide sequence that incorporates a VEGF-165 mimicking peptide that enhanced angiogenesis in vivo without the need for delivery of exogenous GFs (Kumar et al., 2015). A drawback for many of these studies is that material injection must occur directly into the myocardium via an open chest procedure as the material gels instantly once exposed to native pH and osmolarity. Thus, to allow non-invasive delivery, Nguyen et al. designed brush peptide polymer amphiphilic NPs with an MMP degradable peptide sequence. These NPs can be injected intravenously, accumulate through leaky vasculature in the infarct region and due to inflammatory protease activity, transition into a gel that is retained in the infarct (Nguyen et al., 2015a).
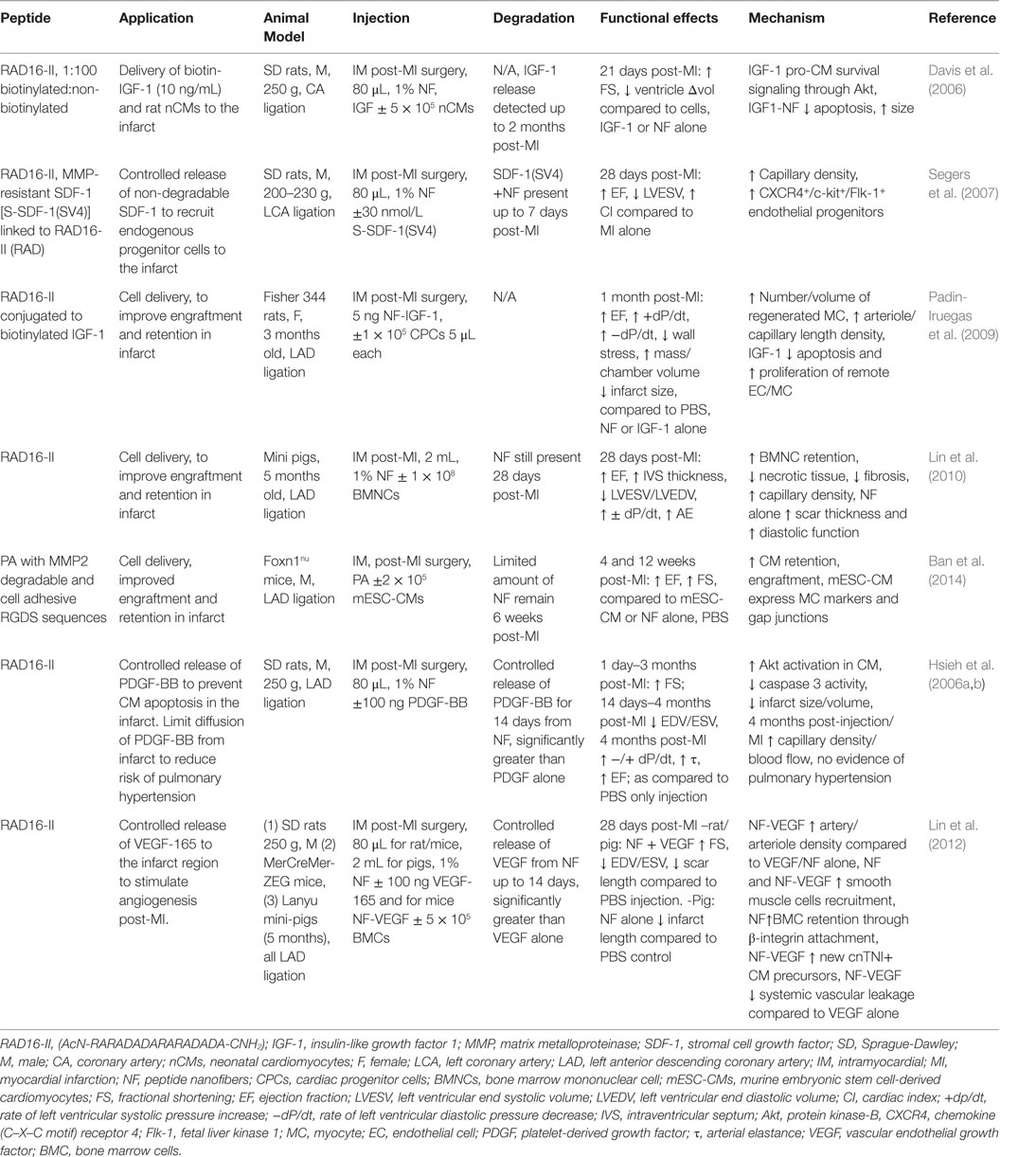
Table 3. Peptide Nanofibers in treatment strategies for myocardial infarction: effects on cardiac function and infarct repair in vivo.
Future Directions for Cardiac and Vascular Tissue Regeneration
In the future, nanomaterials for cardiac regeneration will continue to provide a novel opportunity to enhance the conductivity of biomaterial scaffolds but will require in vivo testing in small and large animal models to test for the possibility of arrhythmias. The development of nanofiber matrices has provided a bottom-up approach to create an ECM-mimicking environment to control cell growth and differentiation. Nanofiber scaffolds as biodegradable vascular grafts could reduce the advent of restenosis and promote regeneration. Nanomaterials, in particular noble metal NPs, are increasingly becoming key components in tissue-engineered materials for cardiac and vascular regeneration in patients with MI, heart failure or coronary artery disease.
Nano-Engineered Materials for Skin Wound Healing
Skin is the largest organ in the human body, which is the natural barrier against external insults and regulates temperature and other vital functions. Wounded skin naturally heals, however, in cases where healing is impaired, as is the case of patients with deficient vascular supply (e.g., diabetic) or those with larger extents of damaged skin (e.g., burns), therapies intended to aid/expedite wound closure are pivotal to reduce morbidity rates (Ulrich, 2014; Uckay et al., 2015). In the following section, we will briefly describe the pathology and medical needs, alongside with the current therapies for two of the most common clinical cases where there is a high demand for regenerative skin scaffolds, which are diabetic foot and burn patients.
Diabetic Foot and Tissue Engineering
Diabetic foot infections (DFI) are part of the pathological profile of diabetic foot ulcers (DFU), where factors, such as arterial insufficiency and immunological disturbances, contribute to their chronic nature (O’Loughlin et al., 2010; Andrews et al., 2015). Statistics point to the fact that DFU and DFI are the major underlying causes for hospitalization and lower limb amputation (Uckay et al., 2015). The current guidelines for treating DFI include systematic antimicrobial therapy, which often does not work in moderate to severe cases (Lipsky et al., 2012; Uckay et al., 2015). This is combined with surgical procedures (Pinney et al., 2002; Mueller et al., 2003; Greenhagen et al., 2012; Colen et al., 2013; Cychosz et al., 2015), or revascularization, in combination with non-surgical wound care therapies, such as custom shoes and orthotics, which to this day remains unclear if they actually prevent the onset of DFU (Cychosz et al., 2015).
Wound healing is a complex biological process that involves inflammation, chemotaxis, angiogenesis, and tissue remodeling (Singer and Clark, 1999; Baum and Arpey, 2005; Gurtner et al., 2008). Chronic wounds are defined as lesions that present impaired (i.e., slow) wound healing (Kirsner and Eaglstein, 1993). Among possible causes for such impaired healing is the persistent inflammation within the actual wound, caused by bacteria colonization, for example (Ehrlich, 1998; Fukai et al., 2005), which is exacerbated in diabetic patients (Lookingbill et al., 1978; Madsen et al., 1996; Robson, 1997; Sibbald et al., 2000; Baltzis et al., 2014; Eming et al., 2014). This permanent inflammatory state results in early degradation of newly forming tissue, creating a never-ending loop of wound healing (Rogers et al., 1995; Yager et al., 1996; Kahari and Saarialho-Kere, 1997; Trengove et al., 1999; Liu et al., 2009). Furthermore, this scenario gets even more complex in the presence of bacteria capable of forming biofilms in diabetic foot wounds such as Pseudomonas aeruginosa (Uckay et al., 2015) and multidrug-resistant Staphylococcus aureus (Banu et al., 2015).
An assessment of the extent of DFU by a wound care expert is followed by debridement, and sequential revaluation of the wound site for surgical reconstruction. Particularly, soft tissue reconstruction can be as simple as letting the wound heal by itself, a.k.a. secondary intention, or healing assisted by microsurgical flaps. The use of skin substitutes (cadaver skin, xenografts, and artificial substitutes) has exponentially improved in quality and regenerative capacity. They offer, in most cases, a temporary solution, especially for large wounds with truncated geometries. Autologous grafting and/or flap transplantation also present some drawbacks since they require mechanical harvesting of donor skin, which causes morbidities, such as pain, risk of infection, discoloration, and scarring. Furthermore, most autologous grafts use only the epidermal coverage of the skin, which together with leaving a scarred donor site, does not provide a completely functional barrier to the wound (Tam et al., 2013).
Thus, over the past few decades, there has been a rise of the so-called biocompatible healing products intended for skin regeneration in the form of skin grafts and wound dressings (O’Loughlin et al., 2010). Amniotic membranes appear promising candidates; however, the risk of infection, as well as their limited availability, makes their use unrealistic as a viable definitive treatment for DFU (Zelen et al., 2013; Lavery et al., 2014; Cychosz et al., 2015). Thus, lab-made living-tissue-engineered products, which were originally designed to act as skin grafts, are now also being employed for filling of DFU, where the ECM of the engineered material promotes an angiogenic response within the wound, which in turn accelerates wound healing (Veves et al., 2001; O’Loughlin et al., 2010). Apligraf® (Novartis, Organogenesis, Inc.) and Dermagraft® (Advanced Biohealing, Inc.) are two of the most popular products for treating skin wounds, including DFU, in North America (Veves et al., 2001; Marston et al., 2003; O’Loughlin et al., 2010). Both are contraindicated in wounds with signs of infection. In addition, they are recommended for limbs with good blood supply, and in some cases several applications of the scaffold are required (Veves et al., 2001; Marston et al., 2003; O’Loughlin et al., 2010; Holmes et al., 2013). Other therapies, such as hyperbaric oxygen and negative pressure, have emerged also as potential non-surgical alternatives for treating DFU; however, clinical evidence does not fully support their use (Londahl et al., 2010; Dumville et al., 2013; Ma et al., 2013; Rhee et al., 2014; Cychosz et al., 2015).
Burns and Tissue Engineering
Full-skin functional substitutes remain an elusive problem for skin tissue engineering in burn units. Depending on the burn degree and extent, the surgeon could decide on using split thickness autograft from the patient, which will result in non-functional transplanted tissue (missing sweat glands) (Tam et al., 2013). This treatment is only suitable when there are enough healthy donor sites in the patient. Thus, as similar to the above discussed for DFUs, laboratory engineered materials had been developed to fulfill the need for alternative strategies for wound covering and tissue regeneration in these patients (Ulrich, 2014). Furthermore, the control/prevention of nosocomial infections in burn patients is a pivotal factor for reducing hospital stays and morbidity (Norbury et al., 2016).
In the following sections, we will highlight the progress made regarding nanomaterial use for skin regeneration. Table 4 summarizes some representative examples of hybrid/composites that were tested in vivo for dermal skin tissue regeneration. Figure 3 displays a representative summary of the technologies presented herein.
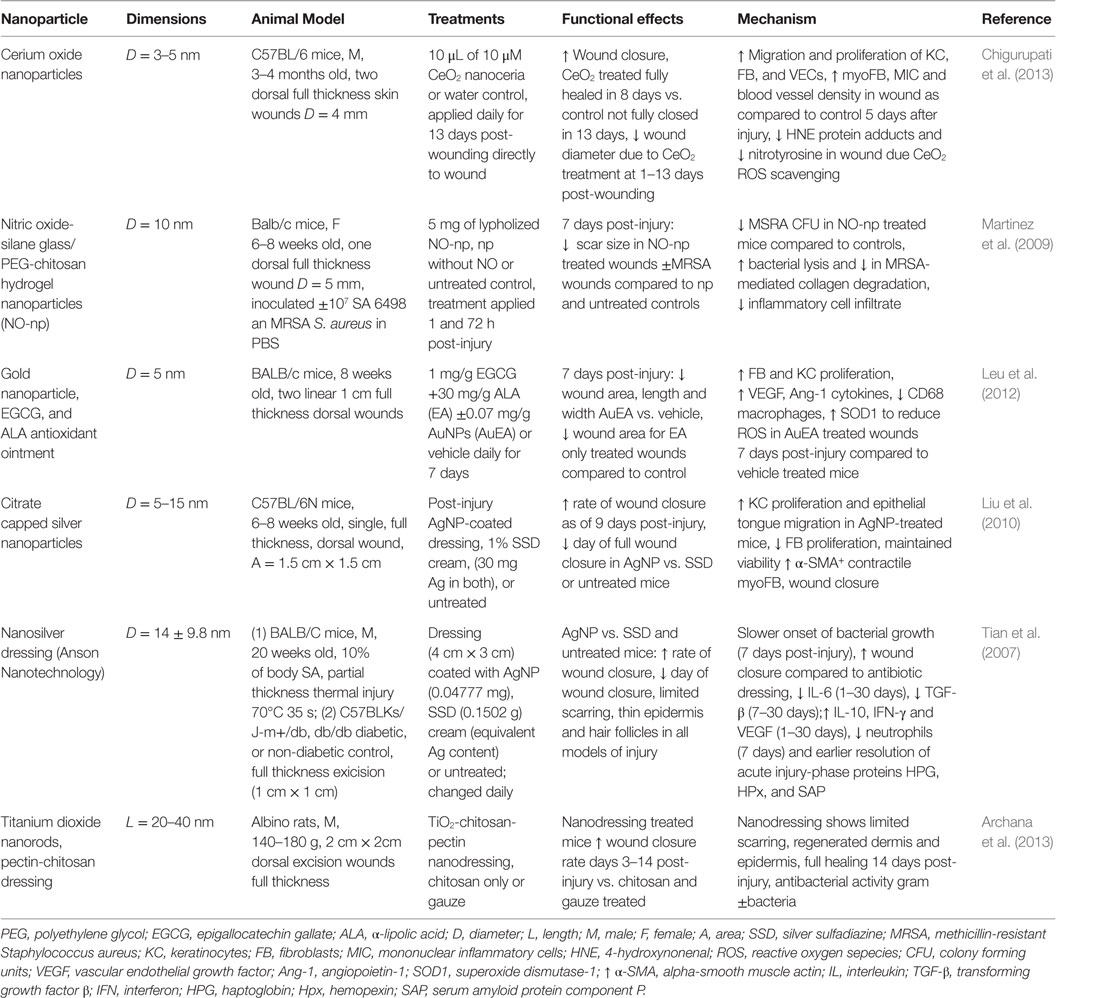
Table 4. Nanoparticles in treatment strategies that significantly improved dermal wound healing in vivo.
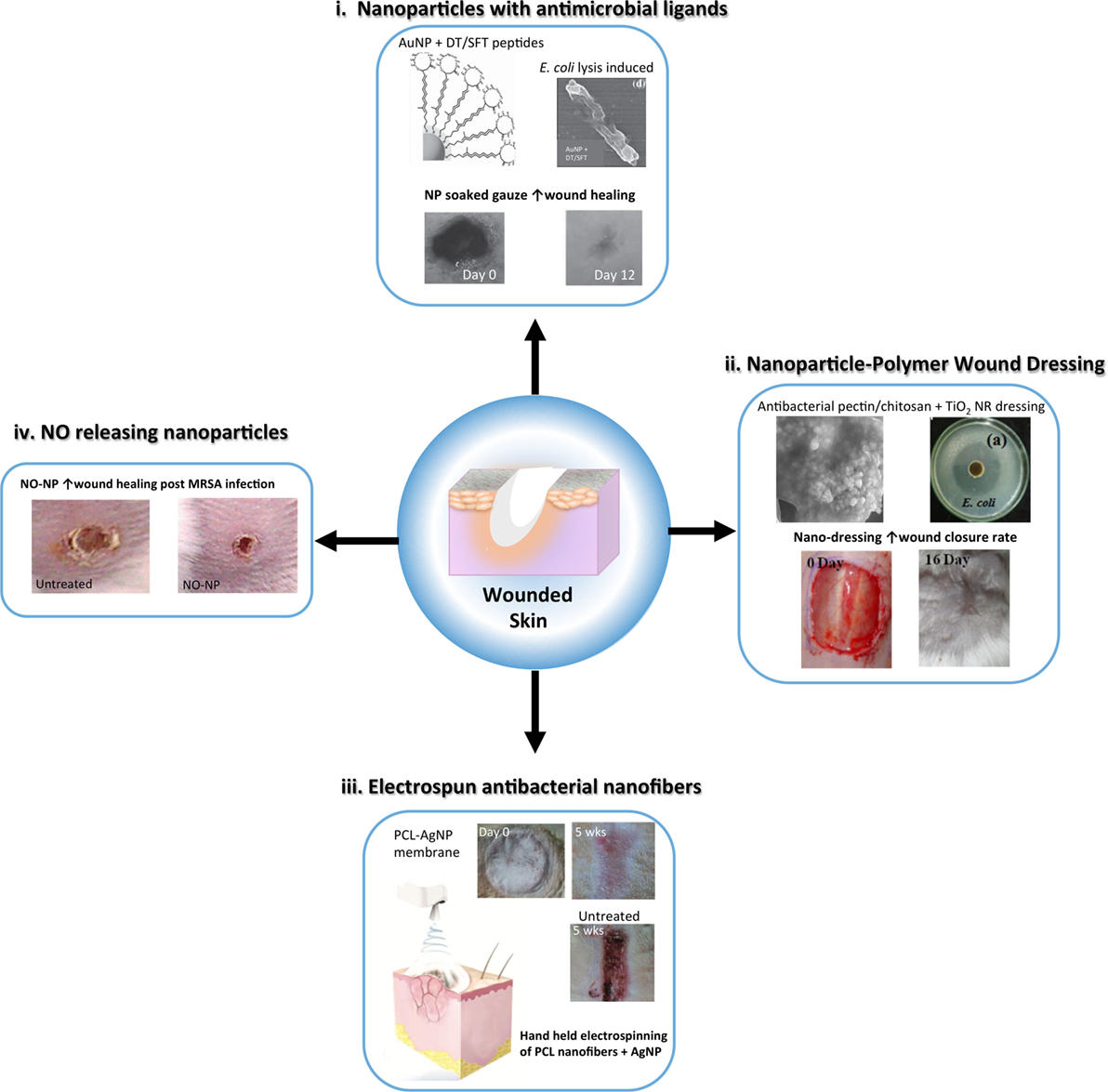
Figure 3. Schematic representing advances in nanoparticle and nanofiber-based strategies to reduce infection and enhance wound healing following skin injury. Nanoparticle and nanofibrous wound dressing have demonstrated antibacterial and anti-inflammatory properties that can improve traditional wound dressing to expedite healing with minimal scarring. (i) Metal nanoparticles, such as gold nanoparticles (AuNP) can be capped with antimicrobial ligands, such as surfactin <SFT) and dodecanethiol (DT) to provide a potent antimicrobial wound dressing [modified with permission from Chen et al. (2015)]. (ii) Nanoparticles can be embedded in polymer based dressing for antibacterial activity, such as TiO2 nanorods (NRs) in a pectin/chitosan hydrogel, which effectively enhanced wound closure rates of full thickness skin wounds [modified with permission from Archana et al. (2013)]. (iii) Nanofibers embedded with silver nanoparticles (AgNPs) can be used as an alternative wound dressing with both antimicrobial and anti-inflammatory properties to improve wound healing and reduce infection. A handheld electrospinning device was constructed for easy fabrication and delivery of the dressing material [modified with permission from Dong et al. (2016)]. (iv) Nitric oxide (NO) releasing nanoparticles made from a hydrogel/glass mixture have been used to safely deliver to skin wounds improving inflammation resolution and angiogenesis. These nanoparticles have also shown antibacterial activity [modified with permission from Martinez et al. (2009)].
Nanomaterials as Therapeutic Agents for Skin Regeneration
Silver as an Antimicrobial and Anti-Inflammatory Agent for Skin Regeneration
When thinking about antimicrobial agents, silver should be considered. The history of silver in medicine as antimicrobial agent goes back over 100 years when silver nitrate (a.k.a. ionic silver) was used in eye drops for newborn babies to prevent gonorrheal ophthalmia (Credé, 1884; Roe, 1915) and micosomal infection in burn patients (Klasen, 2000). However, due to the toxic side effects, such practices were abandoned (Alexander, 2009; Lansdown, 2010; Aziz et al., 2012). During recent decades, however, the alarming rise in the number of antibiotic and multidrug resistant, a.k.a. super-bugs, bacteria strains (Tenover, 2006), has triggered the search for new, more effective, therapies for bacterial infection control, such as nanoparticulated silver (AgNP) (Varner et al., 2010; Rai et al., 2012; Griffith et al., 2015). Despite some studies suggesting that AgNP can present health risks similar to those of ionic silver (Eckhardt et al., 2013; De Alwis Weerasekera et al., 2015), growing evidence indicates otherwise (Asharani et al., 2008; Travan et al., 2009; Moulton et al., 2010; Trickler et al., 2010; Bouwmeester et al., 2011; Stoehr et al., 2011; Alarcon et al., 2012, 2013; Lu et al., 2012b; Simpson et al., 2013; Vignoni et al., 2014).
Functional tissue regeneration requires a balanced orchestration of all the players (e.g., cytokines, macrophages, matrix remodeling) (Eming et al., 2007), to transition from an inflammatory to wound-healing environment. Failure to do so can have possibly irreversible consequences, including tissue scarring, chronic inflammation, and infection (Guirao and Lowry, 1996; Martin, 1997; Branton and Kopp, 1999; Wahl, 1999; Dunn et al., 2001; Eming et al., 2007). Recent in vitro and in vivo work has pointed toward the unprecedented ability of AgNP to reduce inflammatory macrophage and neutrophil infiltration, to inhibit the production of inflammatory cytokines, and to regulate the expression of metalloproteinases (Wright et al., 2002; Bhol and Schechter, 2007; Tian et al., 2007; Wong et al., 2009; Liu et al., 2010; Zhang et al., 2014). Thus, it stands that the incorporation of AgNP in biomaterial dressings is expected not only to add an antimicrobial element to the scaffold but also to further improve wound healing (Lu et al., 2012a; Neibert et al., 2012; Fan et al., 2014; Herron et al., 2014). For example, a chitosan-AgNP dressing by Lu et al. significantly increased the healing rate of dermal wounds 10% of the body surface area in a rat model as compared to silver sulfadiazine or chitosan film alone while the percentage of silver dissemination away from the area of injury was lower for AgNP (Lu et al., 2012a). Recently, our team reported reduced levels of interleukin-6 and other inflammation markers in mice that received subcutaneous implants of type I collagen hydrogels containing AgNP (Alarcon et al., 2015).
Other Nanomaterials for Skin Tissue Regeneration
Nitric oxide (NO) plays an important role in the immune response as well as the proliferation/regeneration phase of wound healing. Friedman et al. developed a NO-releasing nanoparticle (NO-NP) platform from a glass/hydrogel NPs, which contain antimicrobial polysaccharide chitosan (Friedman et al., 2008). These NPs successfully reduced wound closure time, increased wound epithelization, and reduced the burden of methicillin-resistant S. aureus (MSRA) in a murine model of wound infection (Martinez et al., 2009). NO-NP treatment enhanced the secretion of pro-inflammatory cytokines in wounds including IL-6, TNF-α and MCP-1, as well as TGF-β which increased fibroblast proliferation, migration and wound closure. Thus, a cumulative reduction in bacterial-based collagen degradation in the wound by NO-NP-mediated bacterial lysis was observed (Han et al., 2009, 2012). Furthermore, NO-NP treatment of dorsal wounds in a diabetic mouse model was more effective at promoting wound healing than topical therapies for NO release (Blecher et al., 2012).
Gold nanoparticles coated with the antimicrobial peptide surfactin provided a synergistic platform to reduce bacterial burden and enhance wound healing in a rodent model of [MRSA infected wounds (Chen et al., 2015)]. AuNPs have been shown to have synergistic effects in platforms with α-lipolic acid/epigallocatechin gallate antioxidants and hydrocolloid membrane dressings for promoting wound healing through the reduction of oxidative stress and inflammation, and by enhancing angiogenesis in rodent models of cutaneous wounds (Leu et al., 2012; Kim et al., 2015). Cerium oxide NP dressing application alone was shown to have antioxidant and pro-wound-healing effects in rodent models, in part through keratinocyte and fibroblast proliferation and reduction in nitrated protein end products due to ROS (Chigurupati et al., 2013). In addition, carbon allotrope nanomaterials have been applied successfully to enhance wound healing in at least pilot studies in rodent models, including AgNP-loaded graphene hydrogel dressings (Fan et al., 2014), graphene quantum dots that enhance bactericidal effects of hydrogen peroxide (Sun et al., 2014) and a graphene–chitosan nanofiber material that possessed both antibacterial as well as enhanced wound-healing properties with minimal scarring in both mice and rabbits (Lu et al., 2012a). Finally, titanium oxide NPs in combination with chitosan and pectin enhanced antibacterial activity in vitro as well as enhanced wound closure and epithelial regeneration in a mouse model (Archana et al., 2013).
Further advancements in nanofiber development have led to new GF and drug eluting dressings for wound healing. For example, epidermal GF immobilized on PCL/polyethylene glycol (PEG) nanofibers enhanced epithelization of a wound in mice (Choi et al., 2008b), and metformin, an anti-hyperglycemia medication, eluted from poly(lactic-co-glycolic) acid (PLGA) nanofibers improved wound closure in a diabetic mouse model (Lee et al., 2014). In addition, chitosan electrospun nanofibers have been developed and applied to skin wounds in mice leading to enhanced closure of the wound and epidermal regeneration through fibroblast proliferation and angiogenesis (Tchemtchoua et al., 2011). Specialized construction of nanofiber scaffolds and application methods in recent years have lead to the development of a hand-held electrospinning device that can efficiently apply a PCL-AgNP gel to full thickness skin wounds to improve healing and reduce infection (Dong et al., 2016). Fragmented PLLA scaffolds that adhere to uneven surfaces of burn wounds in mice were shown to provide an effective barrier to infection while promoting healing (Okamura et al., 2013). Patterned scaffolds of nanofiber-bioactive glass with controlled micro- and nanotopography (Xu et al., 2015) have been shown to increase pore size for fluid distribution in dressings while mimicking the natural topography of the ECM for cell growth and enhanced wound healing in mice through re-epithelialization, angiogenesis, and collagen deposition, which were superior than non-patterned scaffolds.
Future Directions for Skin Tissue Regeneration and Nanomaterials
The outstanding advancements in nanomaterial therapies for skin regeneration are bringing us closer to the availability of new multi-functional materials that provide multiple wound-healing properties. For example, the incorporation of stable nanosilver to provide anti-inflammatory and antimicrobial properties to any given template presents an interesting and appealing strategy. However, better understanding on the nanoscale interactions between biopolymers and nanomaterials is required to allow de novo engineering of tissue scaffolds for tissue engineering.
Nanomaterial Therapeutics for Eye Regeneration
The human eye is a highly organized and complex sensory organ. The structural and functional features of the eye components cooperatively capture, direct, and process light with a fascinating degree of efficiency and clarity, relayed to the central nervous system (CNS) for interpretation. The tear film, comprised of aqueous, mucin, and lipid layers, consistently nourishes the surface of the eye (Rai et al., 2015). Ocular function is variable between individuals, often corrected with optometric methods (e.g., glasses, contact lenses), yet ophthalmological diseases are relatively common around the world. Physical abrasions or trauma in eye injuries, typical in sports, and ocular surface infections can lead to long-term vision problems; conjunctivitis is frequently a problem that can escalate without treatment or if reoccurrence is common. The aging population is at significant risk of age-related macular degeneration (Rosenthal et al., 2012), cataracts, glaucoma, and dysfunction by hypertension and type II diabetes. Nanotechnology opens a new venue for alternative treatments. The unique characteristics of nano-based materials, such as bioactivity, shape and size, mobility, and delivery potential, are of interest for ocular therapy (Sharaf et al., 2014; Rai et al., 2015). Furthermore, biocompatible scaffolds and NPs minimize immunological reaction and irritation, which supports long-term recovery. In particular, the intrinsic properties of noble metal NPs have substantial success (Jo et al., 2015). The following section will cover nanomaterials and nanoparticulate structures for corneal and retinal treatments, focused on regeneration and vision restoration. Table 5 summarizes the main findings for the technologies herein discussed, while Figure 4 is a selected summary for the technologies here discussed.
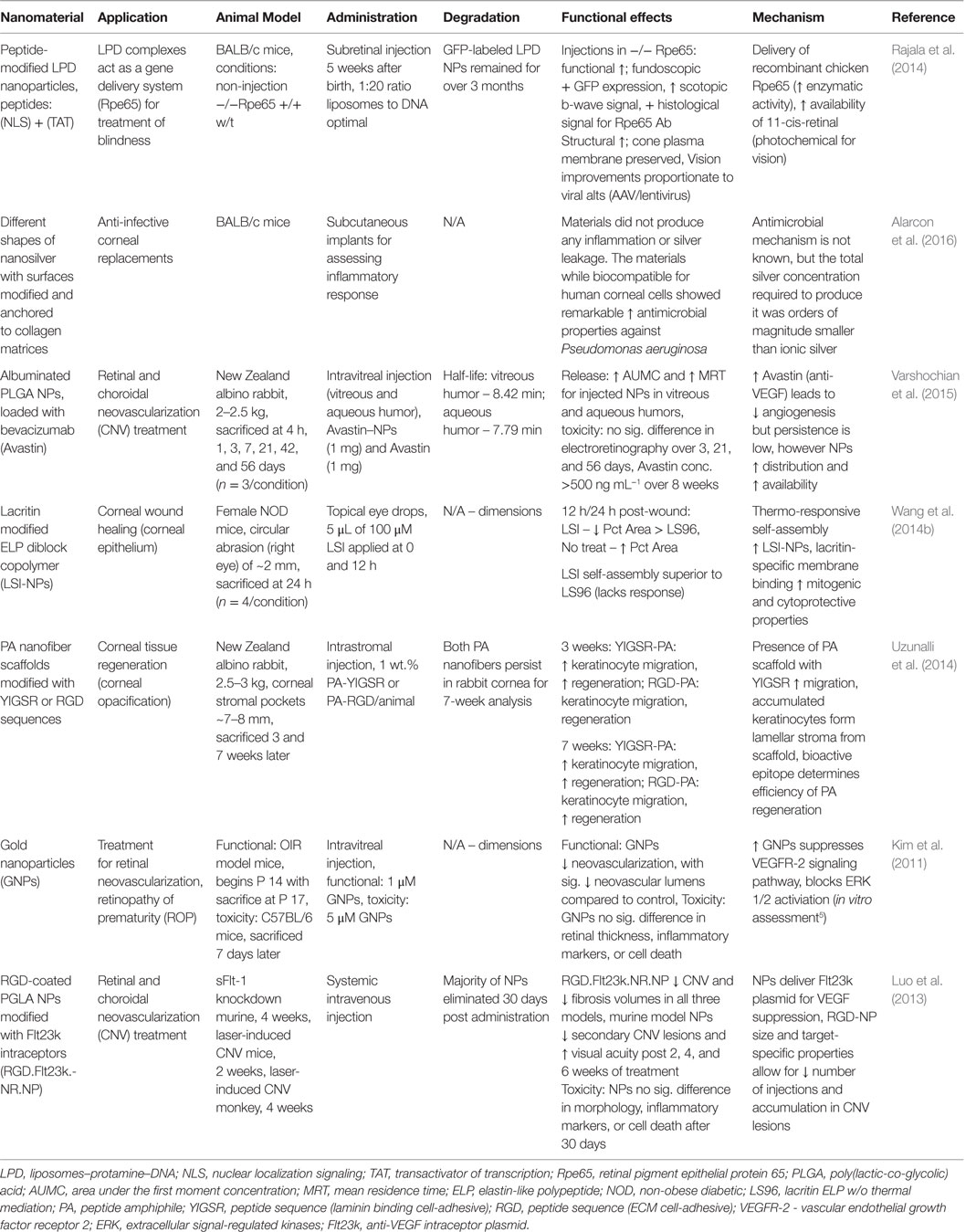
Table 5. Nanomaterials for corneal and retinal regeneration: functional effects on eye structure regeneration and potential mechanisms.
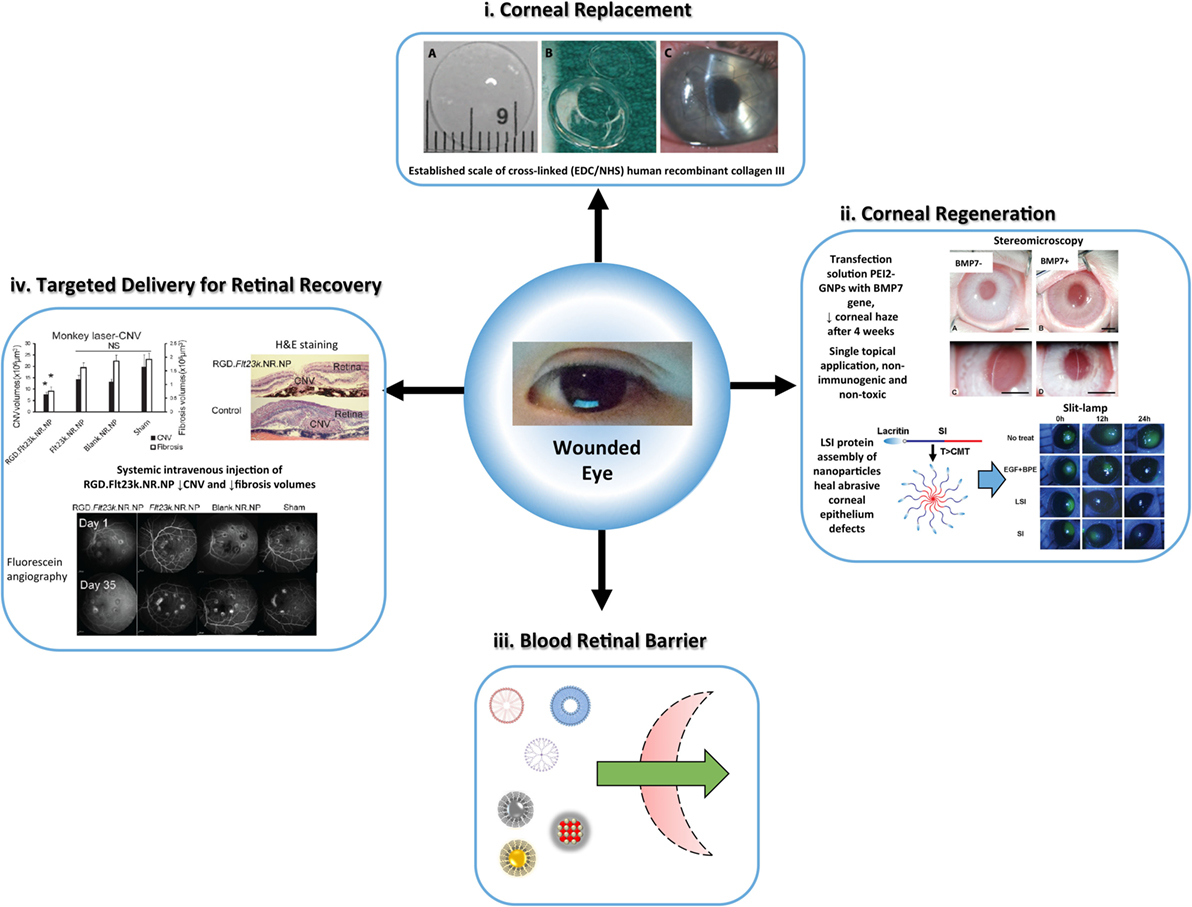
Figure 4. Overview of the versatile nanomaterial applications for corneal and retina defects, established by safe, efficient, and long-term rejuvenation of vision, (i) biosynthetic corneal substitute as an alternative to allogeneic tissue for vision restoration. Recombinant human collagen III is cross-linked (EDC/NHS), shaped (patient-match), and implanted (10–0 mattress sutures) onto damaged host tissue. The structural and lunctional properties of the nanomaterial elicit bioactive regeneration [reproduced with permission from Fagerholm et al. (2010)]. (ii) Hybrid nanoparticles show promise for accelerated corneal regeneration. Polyethylimine-conjugated gold nanoparticles (PEI2-GNPs) transfer bone morphogenelic protein 7 (BMP7) as a gene therapy for corneal fibrosis (Sharma et al., 2011; Tandon et al., 2013) [reproduced with permission from Chaurasia et al. (2015)]. The nanoparticle vector exhibited success in a rabbit model of laser ablation-induced corneal fibrosis. Thermo-responsive lacritin modified elastin-like polypeptide nanoparticles (LSI-NPs) infiltrate the corneal epithelium to facilitate ocular surface wound healing (Wang et al., 2014b). The self-assembly nanoparticles deliver lacritin within the epithelium swiftly and safely. (iii) Schematic of non-viral, nanoparticulate (polymeric micelles, liposomes, dendrimers, AgNPs, AuNPs, CeNPs) advantage for penetrance of the blood retina barrier (Chaurasia et al., 2015). (iv) Gene therapy for retinal defects is one of many methods for regeneration using nanomaterials (inflammatory pathways or reactive oxygen species), and the application of RGD.FH23k.NR.NP in primates and murine models is a prominent case. This method displays the performance of NP vectors with site-specific targeting at a systemic scale, which suppressed retinal angiogenesis and fibrosis after 4 weeks in primates [adapted with permission from Luo et al. (2013)].
Corneal Regeneration or Replacement Using Bioactive Materials
The cornea is a transparent layer on the anterior surface of the eye, which overlaps the anterior chamber. In addition to the cornea, the iris, pupil, and lens focus light onto the pigmented interior layer. The human cornea mainly comprises many collagen layers organized to ensure full clarity of light. Furthermore, there are no blood vessels on the corneal epithelial in support of its transparency. However, it is vulnerable to many physical and environmental dangers due to its forefront position. A prominent issue is found with dry eye disease, which directly interferes with corneal function due to tear film imbalances and surface inflammation. Direct damage to the cornea, minor or significant, can have a tremendous effect on vision; thus, a leading cause of blindness, affecting ≈4.9 million individuals who are bilaterally blind, and another ≈ 23 million who are unilaterally blinds (Oliva et al., 2012). The surgical replacement of the damaged cornea by transplantation from a cadaveric donor remains as the gold standard treatment. The severe shortage of donors in most countries, particularly in developing countries, has motivated the search/research for man-made corneal substitutes. Currently, refractive eye surgeries and synthetic corneal replacements (keratoprostheses) are applied to combat this issue but are limited in several ways. Primarily, post-operative complications, such as infection, are rampant for many patients (Griffith et al., 2012). Furthermore, synthetics are incompatible at times depending on the individual and are at risk of biological rejection and induction of further ocular problems (Griffith et al., 2012).
Biocompatible and bioactive nanomaterials can possibly minimize complications associated with corneal regeneration. Intrastromal injection of peptide amphiphile (PA) nanofiber scaffolds modified with YIGSR (fibronectin peptide) or RGD (laminin peptide) sequences, into rabbit cornea were applied for corneal wound healing. Analysis after 3 and 7 weeks post-injection with RGD displayed significant migration of stromal keratinocytes and enhanced regeneration of the damaged cornea (Uzunalli et al., 2014). Furthermore, the opacity of the cornea was unaffected by the treatment. Also, the incorporation of nanosilver into collagen hydrogels can produce collagen mimetic matrices with antimicrobial properties (Alarcon et al., 2016).
Sometimes point-specific corneal healing is insufficient and replacement is necessary. As appealing as natural scaffolds are for replacement, the structural limitations associated with the size of the material (prone to degradation and fracture) remain a problem. Furthermore, the replacement must ensure full compatibility with the surrounding tissues (nerve and muscle) with acceptable refractive transparency. Fagerholm et al. performed a phase I trial of corneal replacement treatment using biosynthetic recombinant human collagen (rHC) type III. This 10% (w/w) rHC type III is EDC/NHS cross-linked within an appropriately sized corneal mold. The assessment over a 2-year period reported no signs of transplant rejection, infection, or immunological reaction. Visual acuity among patients was similar to normal cornea function across the study (Fagerholm et al., 2010). Furthermore, sufficient nerve regeneration and stromal repopulation was found in patients over a 4-year period following the original study (Fagerholm et al., 2014). The long-term safety and efficacy denoted in these studies is promising for future applications.
NP structures, such as liposomes, can also improve the effectiveness of antimicrobial drugs (Chaurasia et al., 2015). Furthermore, NPs and NP structures are excellent drug and gene carriers that can promote site-specific delivery and bioavailability to corneal epithelium (Attama et al., 2009; Sharma et al., 2011). In addition, metal NPs integrated on surface scaffolds are highly effective antimicrobial agents that can reduce post-operative complications (Santoro et al., 2007). Scaffolds made of natural (chitosan) and synthetic (PCL) components are well received in eye transplants for ocular inflammation treatment (Zarbin et al., 2013).
Nanomedicine Impact on Retinal Dysfunction and Associated Blindness
The retina is an expansive neural network, consisting of two layers: a pigmented layer for light absorption and a neural layer dense with photoreceptors. The retinal layers are vital for sensory integration, feedback, and processing of all visual information via chemical and electrical signals. This information is guided by the optic nerve to the brain. Retinal complications are very serious and often are associated with AMD and glaucoma. In addition, genetic abnormalities in photoreceptor function, pigmented cells, and ganglion density can contribute to congenital blindness. A major challenge with current therapies is penetrance of the blood–retina barrier and site-specific distribution of bioactive agents.
Neovascularization of the subretinal space leads to loss of visual acuity. This is treated with continuous intravitreal injections of inhibitors (anti-VEGF) to minimize vascular expansion and thus improve vision. However, the injections are quite costly and invasive. Also many patients experience minimal recovery or vision loss continues to diminish. In a study by Luo et al. (2013), a single injection of RGD-coated PGLA NPs with Flt23k (anti-VEGF plasmid) transcripts was applied to an AMD primate and choroidal neovascularization (CND) murine model. This systemic intravenous injection minimized the administration risk and the NP solution was biocompatible in both animal models. It was found that ~86% of mice displayed a 12.2 ± 5.2% improvement to visual acuity (Luo et al., 2013). Furthermore, CND and fibrotic scar volumes were reduced in both animal models (Luo et al., 2013). In another study, bare titanium dioxide (TiO2) NPs were injected into mice with oxygen-induced retinopathy (Jo et al., 2014). Interestingly, the TiO2 NPs had a low toxicity profile in the trial with signs of reduced retinal neovascularization. There are many proposed nanomaterial scaffolds and drug-carriers that are protective and regenerative for retinal photoreceptors and neurons (Zarbin et al., 2013; Lin et al., 2015; Varshochian et al., 2015).
Degeneration associated with AMD is one of the major causes of blindness in the world. Dry AMD is characterized by gradual loss of vision as drusen accumulates between retinal pigment epithelial and Bruch’s membrane (Cai and McGinnis, 2016). There are many factors associated with this progression, including biochemical and molecular mechanisms. Most therapeutic methods focus on inflammatory pathways or cell-based rejuvenation of tissue. Cerium oxide NPs (CeNPs), commonly known as nanoceria, have antioxidant catalytic activity similar to endogenous defense enzymes. In a study by Kong et al., low-dose nanoceria was systemically injected into mice with retinal defects to observe retinal function and the effects on ROS levels. The nanoceria reduced concentration of ROS in the subretinal space, and preserved photoreceptor integrity by the upregulation of pro-survival genes and downregulation of apoptotic signals (Kong et al., 2011). This work has been assessed in different injury models, including the latest example by Wong et al. (2015). Single-dose CeNPs catalytic activity was consistent over a 7-day period, with a decline by day 14, which was able to prevent photoreceptor degradation in a retinal degeneration rodent model (Wong et al., 2015).
Dramatic Changes in Ocular Treatment Closer than Ever
The advances in ophthalmological nanomedicine are staggering, and few challenges seem to remain before the advent of major innovations. The application of biocompatible, biofunctional materials for corneal regeneration or replacement holds great promise. As described, corneal fibrotic scarring and neovascularization are reversible using various methods, or combinations, of nanomaterials, metal NPs, or nanoparticulate structures. A major advantage provided by noble metal or polymeric NPs, dendrimers, and liposomal nanotechnologies is mobility across the blood retina barrier, allowing for accumulation and sustained bioactivity or release of anti-inflammatory and antivascular drugs (Chaurasia et al., 2015; Jo et al., 2015). In addition, the composition and size of NPs elicit minimal immunological reaction. Ocular therapeutics is more manageable compared to other organ/tissue targets, specifically in terms of administration, cost/utility of materials, and structural penetrance and localization. However, the benefits attributed to ocular nanotechnologies are not without its flaws. Beyond improving therapeutic outcomes, further long-term assessment of NPs distribution and eventual clearance is required to fully evaluate toxicity, bioavailability, and stability (Chaurasia et al., 2015). Nanoemulsions and cell-based therapies for ocular diseases are prime candidates for clinical phase trials (Ramsden et al., 2013; Chaurasia et al., 2015). Nonetheless, it is expected that ocular nanomedicine will cause a shift in translational practices (Etheridge et al., 2013). The number of viable, novel therapeutics will expand considerably following further elucidation of nanomaterial regenerative mechanisms. Furthermore, the technological advances in design tools and integrated nanomaterial components will match this movement. This will guide approaches into a clinical perspective, such as hybrid diagnostic/therapeutic systems, targeted drug systems, and quite possibly gene delivery.
Nano-Engineered Materials for Skeletal Muscle Repair
Nanomaterials for Skeletal Muscle Injuries
Skeletal muscles are responsible for the majority of the active movements in the body that keep everyone in motion. The SMs are organized as bundled (fascicles), layered muscle fibers (myofibers) integrated with connective tissues, nerves, and blood vessels (Grasman et al., 2015). Contraction of these muscles requires substantial energy, and the SM plays a structural (support soft tissues, posture) and functional (temperature regulation, skeletal movement) role in the body. SM hypertrophy is essential to growth/development and lifelong fitness; and these tissues regularly undergo regeneration (Grasman et al., 2015; Wolf et al., 2015). However, recovery from medical problems dictated by atrophy, nerve dysfunction or significant volumetric muscle loss (VML) is limited and typically results in irreversible scar formation (Grasman et al., 2015; Wolf et al., 2015). The application of nanomaterials in tissue engineering is expected to fulfill many niches in SM tissue regeneration and restoration. Key characteristics necessary for the translation of novel materials include: biocompatibility, biodegradability, conductivity, and promotion of cell alignment, vascularization, and innervation. The physical and chemical features of nanomaterials determine functionality, which is often modulated by the addition of GFs, pharmacological drugs, and NPs. The following sections will cover the current nanomaterial progress and challenges in SM tissue regeneration with a focus on in vivo application. Table 6 summarizes the main findings for the literature presented in this section.
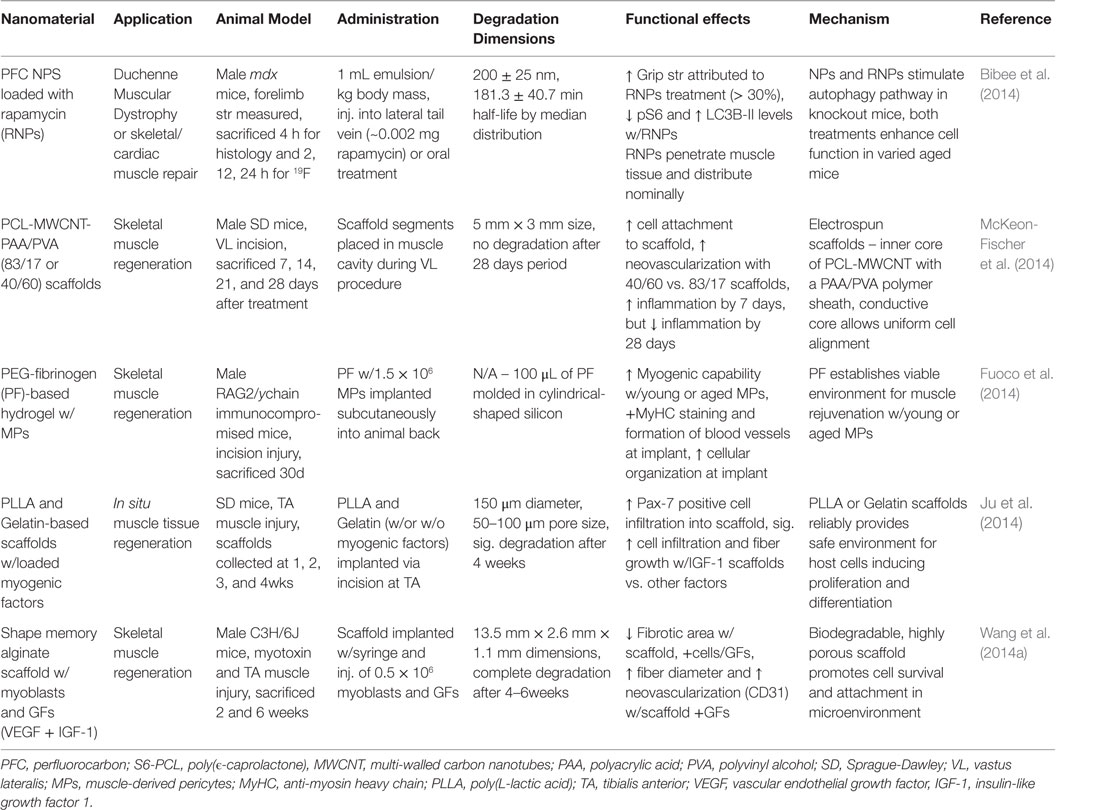
Table 6. Nanomaterials for skeletal muscle regeneration: functional effects on SM regeneration and potential mechanisms.
Natural/Synthetic Hybrid/Composites Biomimetic Materials
Nanomaterials intended for SM tissue regeneration are often categorized as natural or synthetic scaffolds. Currently, significant interest is placed on novel hybrid/composite scaffolds implementing the best features of both types. Natural-based scaffolds comprise essential ECM proteins, such as collagen, fibrin, and hyaluronic acid or decellularized tissue complexes (Wolf et al., 2015). The ECM maintains healthy organ function; thus, scaffolds of this origin are biocompatible, biodegradable, and bioactive for regenerative uses (Kin et al., 2007; Klumpp et al., 2010; Sicari et al., 2014). Furthermore, the inherent nature of these materials can enhance cell-based therapies. However, preparation inconsistencies and material degradation (Koning et al., 2009; Sicari et al., 2014) are frequent issues, in addition to human translational limitations for animal-based natural scaffolds. These materials are manufactured primarily as 2-D sheets from their natural constitutes, designed for external applications. In addition, hydrogel soft materials can develop unique 3D shapes that can be further manipulated with cross-linkers or non-covalent bonding. In a study on in situ muscle regeneration, Ju et al. observed Pax7-positive cells (SCs) infiltrate gelatin-based scaffold implanted in the tibias anterior (TA) of mice (Ju et al., 2014). Myogenic-inducing factors (SDF-1α, HGF, IGF-1, bFGF) were added to separate scaffolds during production. The release of myogenic factors facilitated cell accumulation. In particular, IGF-1 scaffolds showed significant fiber growth, prompted by myogenic differentiation of infiltrated cells, after a 2-week implantation (Ju et al., 2014).
Synthetic scaffolds are reputable for their customization of physical and chemical features and ease of manufacturing process. Nonetheless, these materials are often toxic to cells in vitro and require modifications (coatings, surface proteins) to promote biocompatibility (Grasman et al., 2015; Wolf et al., 2015). Common examples include poly(lactic acid) (PLA), PEG, and poly(ϵ-caprolactone) (PCL). The choice of material determines the capacity of its function, yet difficulties are found in all types for promoting long-term vascularization and myofiber growth (Grasman et al., 2015; Wolf et al., 2015). The production control over synthetic materials allows for an array of configurations. Meshes are designed to maximize surface area and provide incredible structural support. Foams and hydrogels are highly porous, elastic materials capable of interior storage and in situ polymerization. Fiber-based scaffolds are thin, interwoven sheets fabricated typically by electrospinning methods. Fiber length, thickness, and alignment can be predetermined by set electrospinning parameters that allow for abundant choice in design (Grasman et al., 2015; Wolf et al., 2015). Nanofibrous scaffolds can mimic macromolecular sizes to enhance material–ECM interactions (Grasman et al., 2015; Wolf et al., 2015). Furthermore, fiber orientation can promote myoblast adherence and proliferation (Chen et al., 2013). A novel approach in coaxial electrospun scaffolds explored by McKeon-Fischer et al. involves two-layered fibers that induce movement similar to muscle myofibers. The inner core of the fibers comprises PCL and conductive multi-walled carbon nanotubes (MWCNT) and the outside is coated with polyacrylic acid (PAA)/polyvinyl alcohol (PVA) hydrogel that act as an ionic polymer gel (IPG). Following the implantation of these scaffolds into injured vastus lateralis (VL) muscle, myogenic growth was observed at the site (McKeon-Fischer et al., 2014).
Composite scaffolds integrating natural/synthetic properties are showing promise for SM tissue regeneration. These materials are developed with a combination of the individual methods discussed above, usually represented by dual-layered fiber scaffolds or interior/exterior interplay. ECM-derived surface coatings can minimize the foreign body reaction by synthetics. In addition, incorporated PEG or PCL polymers enhance mechanical durability for degradable natural polymers. An electrospun PCL/collagen composite scaffold, seeded with human SM cells, was biocompatible and promoted cellular adhesion and proliferation. In addition, the orientation of scaffold fibers influenced the alignment of muscle cells and facilitated myotube formation (Choi et al., 2008a). As a follow-up, Zhao et al. used the same PCL/collagen scaffold implanted in vivo to resolve diaphragm defective mice over a 6 months period. The material promoted cell migration and adhesion, showing alignment and subsequent tissue formation within and around the scaffold. Physical characteristics of the material matched normal diaphragm tissue, and the implant did not invoke a host response or herniation (Zhao et al., 2013). A PEG-fibrinogen (PF) hydrogel scaffold, with young or aged muscle-derived pericytes, was found to improve myogenic differentiation and angiogenesis (Fuoco et al., 2014). Furthermore, aged pericytes seeded on the scaffold had rejuvenated function that matched young pericytes. This transformation is influenced by the features of the scaffold, which mimic ECM mechanical and functional properties (Fuoco et al., 2014). The capability for hybrid/composite scaffolds to specifically match certain applications by varying a number of parameters on the natural and synthetic components allows for a greater improvement against the challenges of SM regeneration.
Nanoparticle-Based Carriers and Active Agents in Regeneration
There is a relatively expansive outlook on the role NPs can play in SM tissue regeneration. However, the majority of studies are confined to physical/chemical characteristic assessments and in vitro display of biocompatibility, release-kinetics, and cellular functional response. Nonetheless, progress is apparent as our understanding of the structural and functional features these NPs contribute is elucidated. NPs are frequently used as imaging agents (Fang and Zhang, 2009; Adriana et al., 2015) and carriers for GFs or drugs (Arvizo et al., 2012), though this has been limited so far for SM tissue applications. Bibee et al. developed rapamycin-loaded perfluorocarbon NPs that increased physical performance, specifically forelimb grip strength, of both young and old mice. The NPs accumulated at sites of inflammation, where rapamycin induced autophagy that promoted muscle recovery (Bibee et al., 2014). The concentration of drug within NPs, injected via the lateral tail vein, required for this effect is 10 times less than is required with oral supplementation. This is an example where nano-therapeutics can replace existing treatments (corticosteroids, physical therapy) or offer a novel means to promote recovery at a biochemical and molecular level.
Notably, noble metal NPs impart electrical conductive and catalytic functions useful for enhancing nanomaterial effectiveness (Arvizo et al., 2012). However, conductive properties particular to noble metals in SM scaffolds are rarely applied, though the use of multi-walled CNTs has been successful (Quigley et al., 2012; McKeon-Fischer et al., 2014). Parameter changes in pre- and post-production readily alters physical characteristics, such as size, shape, and composition (Arvizo et al., 2012). Metal NPs are usually produced via chemical reduction, followed by downstream purification processes (Arvizo et al., 2012). An area continuously under study is the compatibility of metal NPs in specific tissues, which is heavily focused on NPs concentration and distribution. McKeon-Fischer and Freeman developed electrospun PLLA-AuNPs composite scaffolds with three varying concentrations of AuNPs (7, 13, and 21%) to enhance the healing process (McKeon-Fischer and Freeman, 2011). The proliferation of cells was minimal, yet interestingly the lowest concentration of AuNPs conferred relatively similar results compared to the higher concentration variants. Structural integrity was stable over 4 weeks and the AuNPs did not show signs of toxicity. In regard to coating, metal NPs are capable of promoting cellular interactions and maintaining cell presence at the scaffold surface, which enhances their functional ability (Ishizaki et al., 2011).
The majority of studies on SM regeneration focus on physical injury and loss of SM tissue; however, it may be beneficial to aim at biochemical and molecular dysfunction as well. The role of AuNPs in oxidative stress for exercised-induced muscle damage was evaluated in rats over a 21-day period. Inflammation is active in the presence of ROS, which contributes to SM damage. Phonophoresis (ultrasound-guided delivery) of AuNPs was found to reduce inflammation, denoted by a decrease in many pro-inflammatory markers and oxidative stress markers (superoxide and NO), and an increase in total glutathione levels (Zortea et al., 2015). In addition, it was found that a gold embedded, decellularized porcine diaphragm scaffold promoted fibroblast proliferation and attachment, yet high Au concentration increased free radical levels (Cozad et al., 2011). It would be interesting to see a follow-up involving AuNPs release from hybrid/composite scaffolds for in vivo SM regeneration.
It seems that NPs in SM regenerative applications are in the early stages of development in terms of their potential. The coming years will show how valuable metal NPs features are to modulating scaffold functions and influencing physical/chemical characteristics when based on their extensive foundational study.
Future Directions for Skeletal Muscle Regenerative Materials
Key functional effects prioritized in recent SM regeneration studies include cell migration into/onto nanomaterials, alignment of neighboring cells or newly proliferative cells, and electrical stimulation of scaffolds. Furthermore, elucidation of material–cell–tissue interactions in vivo is vital for facilitating the transition from proof-of-concept design to pre-clinical or FDA-phase trials (Grasman et al., 2015; Wolf et al., 2015). Currently, an incredible variety of nanomaterials are available, yet few boast truly significant in vivo compatibility and regeneration in long-term studies. In part, this is due to limitations in the animal models for SM regeneration. Popular methods of injury include laceration, ischemia, and weighted-trauma. If the induced injury is minimally disruptive, normal SM regeneration takes precedence over potential effects from scaffolds. Furthermore, if the basal membrane remains after injury it can act as a template for satellite cell migration (Wolf et al., 2015). By contrast, VML injuries remove considerable muscle tissue but consistency is difficult to maintain across animal models and injury site (Grasman et al., 2015). Sometimes toxins are introduced to permanently limit SM function. It will be important to fully describe such methods in practice for studies aimed at translational applications.
Skeletal muscle regeneration is a multifaceted endeavor. In order to meet this demand, substantial tissue replacement/healing must be induced by facilitated proliferation and differentiation of neighboring SM cells. Furthermore, promotion of axonal growth and innervation will improve communication with damaged peripheral nerves. In addition to SM regeneration, it will be necessary for nanomaterials to promote both angiogenesis and neurogenesis. Noble metal NPs show promise; however, steps need to be taken to elaborate on their effects in vivo. This advancement will likely involve hybrid/composite materials associated with metal NPs and progenitor cell delivery.
Nanomaterials for Peripheral and Central Nervous System Regeneration
A Gap Bridged by Nanomaterials for Peripheral and Central Nervous System Repair
The nervous system is essential to the functional relay and processing of information outside and within the human body. It is categorized by two divisions: the CNS, which includes the brain and spinal cord, and the peripheral nervous system (PNS) that comprises all other neural tissue in the body (Goldberg and Barres, 2000). The basic neural unit, the neuron, is capable of receiving and sending signals at rapid speeds. The CNS integrates, processes, and coordinates sensory and motor information to enable a functional response. The PNS primarily sends signals to the CNS, via the spinal cord, and delivers signals to other cells within the body (Goldberg and Barres, 2000). However, the nervous system is susceptible to acute or chronic damage alike to the other tissues in the body. People of all ages suffer from neural complications, induced by infection, physical trauma, toxins, and hypoxic conditions. Furthermore, neurodegenerative diseases are prominent in the elderly population. Commonly, neural degradation is associated with a diminished neuron population or severed neuron-tissue connections. Clinical therapies for neural regeneration range from successful, PNS axonal gap connections <5.0 mm, to non-treatable, many neural degenerative diseases (Orive et al., 2009; Cunha et al., 2011). Nanomaterials are expected to revolutionize novel therapies and provide improvements to current cell-based or immunological therapies. The following sections will cover current nanomaterial compositions demonstrating CNS and PNS regeneration, and representative examples for those materials are summarized in Table 7 and some of them depicted in Figure 5.
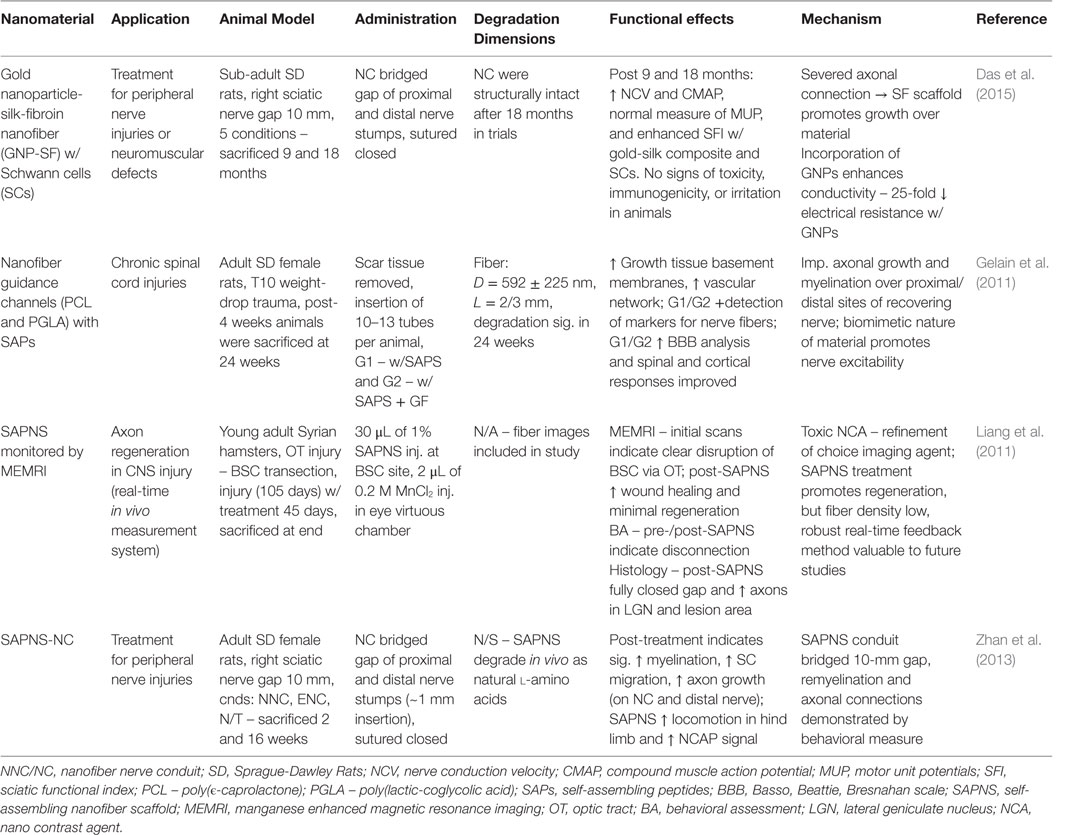
Table 7. Nanomaterials for peripheral and neural nervous system: functional effects on NS regeneration and potential mechanisms.
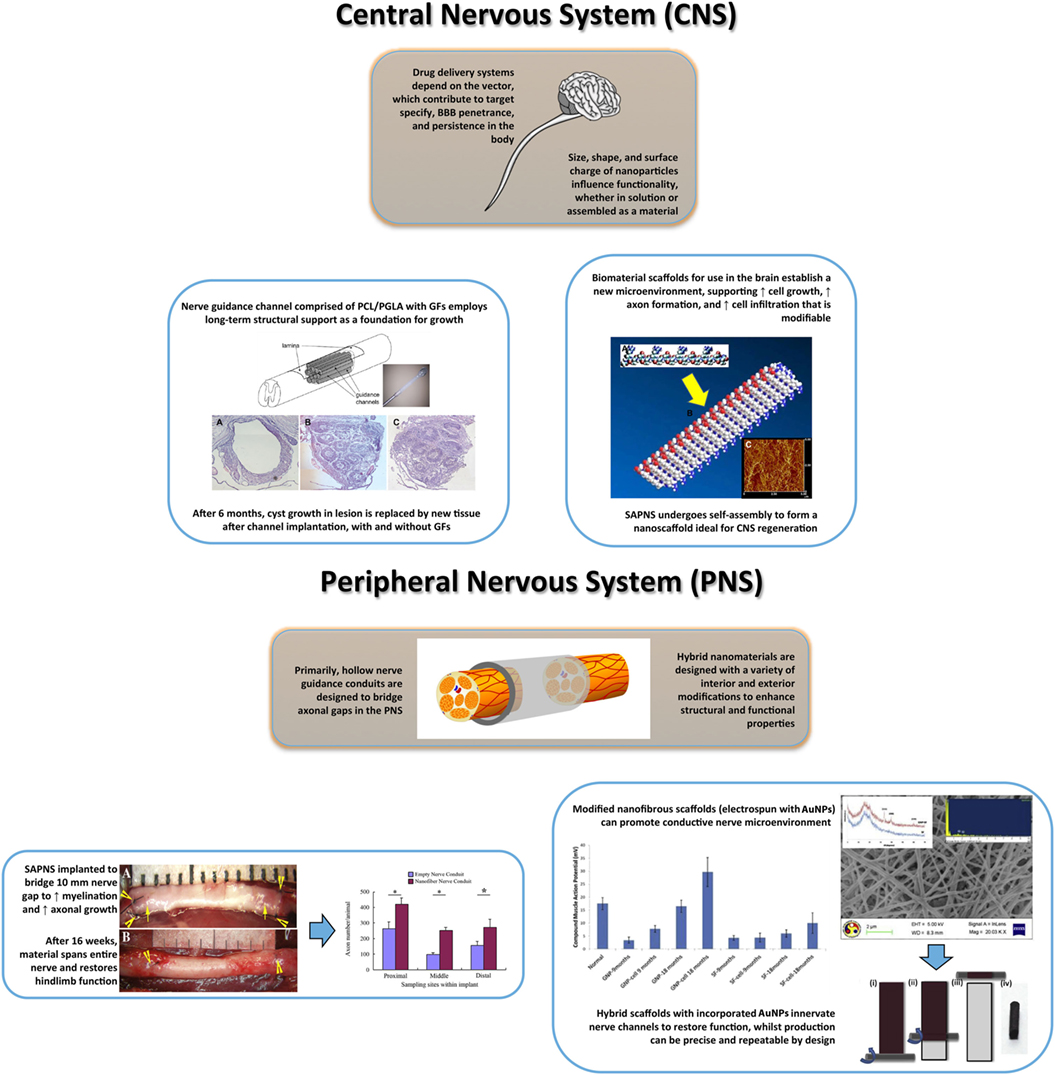
Figure 5. Summary illustration of nanomaterials for central nervous system (top) and peripheral nervous system (bottom) regeneration involving restored structure and function. Top: regenerative medicine for CNS recovery often follows the Four P. (Preserve, Permit, Promote, and Plasticity) as guidelines in developing novel nanomaterials (Ellis-Behnke, 2007). The importance of these guidelines depends on variables of treatment time and severity of injury, which contribute to themes of maximal cellular regeneration and minimal complication. Current pharmaceuticals intended for neural regeneration are associated with novel drug delivery platforms (polymeric nanoparticles, dendrimers, liposomes) that can modulate dose and target specificity within the CNS, without consequence (Orive et al., 2009). The design flexibility of nanoparticles allows for diverse application, dependent on uses for cellular uptake, dispersion, target binding, penetrance, and bioactivity modified from (Jo et al., 2015). Top-Left: spinal cord injuries have numerous complications that are possibly overcome using hybrid scaffolds with growth factors. The implantation of this nanomaterial melds within the damaged region, providing tissue reconstruction and neural regeneration [adapted with permission from Gelain et al. (2011)]. Top-Right: delivery platforms are garnering heightened attention, yet scaffolds can accelerate regeneration in specific cranial regions. For long-term treatment, hybrid scaffolds or SAPNS interact with the microenvironment conferring a biocompatible, biodegradable platform. Porosity, surface dimensions, and fibrous orientation contribute to positive material–cell interactions [reproduced with permission from Liang et al. (2011)]. Bottom: the majority of peripheral nerve injuries are associated with severed neural regions, with limited axonal growth and myelination. Highlighted, nerve guidance conduits are inspired to create a stable structural bridge between nerve gaps while promoting cellular growth and initiating electrical stimulation [adapted with permission from Daly et al. (2012)]. Bottom-Left: representative image of the implantation (a) and post-16 weeks (b) of a nerve conduit for sciatic nerve repair in rats followed by the increase in axon growth across the conduit dimensions [adapted with permission from Zhan et al. (2013)]. Bottom-Right: example of the incorporation of nanohybrid materials (gold nanoparticle-silk-fibroin nanotiber.Ai GNP-SF) for peripheral nerve regeneration. The nerve conduit preparation is completed using a sheet rolling method. Importantly, the GNP-SF shows that GNPs enhance nerve conductance as measured by compound muscle action potential over the study period in rats [adapted with permission from Das et al. (2015)]. Figures used in illustration are referred by use in caption, except generic brain/spine image.
Self-Assembly Peptide Scaffold, Nanoparticles, and Nanofiber Composite Scaffolds for Nerve Regeneration
The nervous system is vulnerable to injury, yet it is surrounded by impressive structural defenses. The skull and surrounding cerebrospinal fluid (CSF) protects the brain from trauma, and the blood–brain barrier (BBB) semi-permeable membrane guards against ROS and pathogens. The spinal vertebrates encase the vital nerves and provide structural support. The peripheral nerves lack the same protective measures, but have intrinsic regenerative capabilities (Cunha et al., 2011). Nonetheless, the CNS is at serious risk when its defenses are overwhelmed and peripheral nerve regeneration is unreliable in moderate-severe destruction. Most cell-based therapies are limited by poor cell engraftment and survival (Orive et al., 2009). Nanofiber scaffolds can establish an environment suitable for cell proliferation and survival, and promote proper neurite and axonal architecture (Ellis-Behnke et al., 2009; Orive et al., 2009). Neuroprotective drugs that are intended to minimize inflammation are ineffective via systemic injections due to the BBB and non-specific tissue distribution. The physical characteristics, primarily size and shape, of metal NPs and nano-structures confer penetrance of the BBB. Furthermore, metallic NPs are chemical stable and readily modifiable for surface functionality (Jo et al., 2015; Paviolo and Stoddart, 2015). Nanotechnology is demonstrating its potential to maximize cellular regeneration and minimize complications attributed to current methodologies (Orive et al., 2009; Cunha et al., 2011; Paviolo and Stoddart, 2015).
Peripheral nerve dysfunction is usually gradual, starting with pain sensations that progress to inconsistent coordination, which can lead to paralysis. In a study by Zhan and colleagues, a self-assembly peptide nanofiber scaffold (SAPNS) with an artery conduit sheath was implanted on the proximal and distal nerve stumps to bridge a 10 mm gap in adult rats (Zhan et al., 2013). It was found that after 16 weeks, the artificial nerve graft promoted myelination, satellite cell migration, and axonal growth over the conduit. In addition, assessment of the injured hindlimb motor function displayed significant improvement just after 6 weeks and followed throughout the 15-week period (Zhan et al., 2013). SAPNS have been of interest for axon regeneration over the last decade, initiated by Ellis-Behnke and colleagues (Ellis-Behnke et al., 2006, 2009). The self-assembly peptide hydrogels resolve the architectural issue associated with the degraded tissue environment, suitable for CNS and PNS repair. The dynamic features of self-assembling peptides have been shown to induce functional recovery in different CNS injury models (Sang et al., 2015). Modifications to SAPNS are currently being investigated to enhance their regenerative potential (Cunha et al., 2011). A nanofiber guidance channels comprised PCL/PLGA with SAPs were implanted into cysts of mice with spinal cord injury. The assessment after 6 months displayed new tissue formation, comprising nerve fibers, ECM, and a vascular network. Furthermore, the motor function had improved in the animals after the procedure. The neuro-prosthetics was positively received with and without the addition of GFs, and inflammatory response was decreased during the period (Gelain et al., 2011).
The implications of NPs and NP structures (dendrimers, liposomes) on neural regeneration are showing great promise (Provenzale and Silva, 2009; Paviolo and Stoddart, 2015; Vidal and Guzman, 2015). NPs are very flexible in design ranging from solid or hollow cores, varying shapes and sizes, and different compositions (Jo et al., 2015). It is this leniency in parameters that allows for a certain type of NP to function in a particular manner (Provenzale and Silva, 2009; Jo et al., 2015). Metallic NPs, specifically gold, have intrinsic bioactivity promoting angiogenesis and cell differentiation (Paviolo and Stoddart, 2015). Furthermore, AuNPs have bioelectric functionality providing modulation of action potentials and ion channels in nerves (Zhang et al., 2012; Paviolo and Stoddart, 2015). In a study by Das et al. (2015), a composite scaffold comprised AuNPs and silk-fibroin nanofibers (GNP-SF) was implanted into the sciatic nerve of Sprague-Dawley rats to bridge a 10 mm gap. The GNP-SF scaffolds, seeded with Schwann cells, provided significant improvements to motor function and electrical stimulation in 9- and 18-month studies. The scaffold was biocompatible and structurally stable over the study period, exemplary based on the sciatic nerve index (SNI) analysis (Das et al., 2015). Alternatively, polymeric NPs and liposomes are excellent carriers for drugs, genes, and GFs for CNS/PNS treatments (Paviolo and Stoddart, 2015; Takeda and Xu, 2015). The surface–environment interactions, modified or unmodified, with these materials are suitable for membrane penetrance, targeted tissue distribution, and biofunctional activity. Encapsulation of neuro-regenerative contents is in parallel with the material fabrication, making the procedure relatively straightforward. In addition, NPs are modifiable for in vivo monitoring, which is beneficial for assessing material regenerative potential or distribution in long-term studies.
Methods for CNS and PNS neural regeneration share a common goal, but the differences in complexity and injury severity between systems leads to divergence in applicable nanomaterials. Most PNS injury models involve precise incision and removal of segments in sciatic and peroneal nerves (Siemionow et al., 2010). Nanomaterials are designed as nerve conduits or artificial prosthetics that bridge the gap and propagate cellular proliferation, migration, and differentiation at the treated site (Siemionow et al., 2010; Cunha et al., 2011). CNS injury is far more diverse depending on the associated cause(s). For spinal cord injuries, dorsal vertebrate trauma (1–2 vertebrate at most) is common in animal models. Anatomical and functional analysis is prioritized with scaffolds employing properties that establish neo-tissue environments. Brain injury depends on the treatment, varying in degree with hypoxia-induced models or severance of specific neural circuits and cell populations (Orive et al., 2009). Primarily in brain injuries, nanotechnologies are used for sustained delivery of neurotrophic drugs or ECM-mimetic scaffolds for localized cell populations (Orive et al., 2009; Mishra et al., 2014; Jo et al., 2015). Regenerative solutions for severe spinal injury and widespread degradation of neuronal cell populations in the brain are the most challenging tasks. This movement will be facilitated in the presence of new tools and techniques that interplay with intercellular and extracellular mechanisms involved in axonal growth and extension afflicted by disease.
Challenges in Nervous Tissue Regeneration and Upcoming Approaches
Treatment for structural and functional nervous tissue restoration is a massive undertaking. There is still much to learn from the biochemical, cellular, and genomic/proteomic mechanisms involving neural regeneration. This is a clear limitation in understanding nanomaterial-tissue interactions. Future studies need to elaborate on the endogenous mechanisms at play on the material interface to properly assert regenerative significance (Orive et al., 2009). Essentially, this understanding can perpetuate the novelty of nanomaterial designs to mimic these mechanisms. In addition, hybrid/composite nanomaterials can hopefully exploit bioelectric cell features and reestablish the myelin sheath. Nonetheless, nanomaterials under in vivo investigation are already complying with necessary properties for clinical translation; biocompatibility, biodegradation, low toxicity and immunogenicity, and functional surfaces/interiors are key for advancing model cases into treatment options.
The progression in nanomedicine for CNS/PNS regeneration is exciting and substantial, yet realistically the gains only permit small-scale clinical relevance. At this time, encapsulated cell-based therapies with neurotrophic factors or GFs are in clinical phase trials for CNS disorders (Orive et al., 2009). Biomaterial nerve guidance conduits for PNIs are clinically approved and in phase trials, yet most are quite short or are quickly degradable (Daly et al., 2012). The foundation for nanotechnology is being built everyday, and eventually their unique features will be fully understood and applied in next generation nervous system therapies.
Concluding Remarks
The rationale integration of nanomaterials to the current therapies for tissue engineering will bring unprecedented strategies for tissue regeneration. Some examples of the actual potential, and impact, of that engineered materials were discussed in this review for heart, skin, eye, SM, and nervous system. In cardiac regeneration, enhancing the biomaterial conductivity upon incorporation of the nanomaterial presents a unique venue of NPs. However, in vivo testing in animal models capable to evaluate cardiac tissue regeneration, including onset for arrhythmias, is required. For skin regeneration, the use of multi-functional materials where by incorporating NPs produce materials with anti-inflammatory and antimicrobial are appealing strategies for the next generation of tissue scaffolds. The use of biocompatible materials for corneal regeneration including noble metal, polymeric NPs, dendrimers, and liposomal nanotechnologies will allow mobility across the retina barrier, which could act as a reservoir for anti-inflammatory and antivascular drugs. For SM, promotion of axonal growth and innervation are pivotal for improving communication with damaged peripheral nerves. Finally, central and PSS would be benefited from the fabrication of biodegradable scaffolds capable to rapidly integrate within the lesion and promote cell angiogenesis and tissue regeneration. It has to be recognized, however, that better understanding on the nanoscale interactions between biopolymers and nanomaterials is required to more effectively harnessing and de novo engineering regenerative templates. Additionally, other aspects that to this day still hinder use of nanomaterials in the clinic are:
I. Lack, or little, regulatory standards to assure stability of engineered nanomaterials prior clinical evaluation. This should also account for evaluating the impact of batch-to-batch variability in the preparation of the nanomaterial. Assuring stability of nanocomposites and/or materials containing them in biological systems is pivotal to assess with precision the regenerative potential of the new materials.
II. Almost non-existing standards for animal models to be used for assessing the bioactivity of nanomaterials, including biodistribution and accumulation of the nanomaterial. This becomes even more critical for cases where a specific medical lesion/injury has to be mimicked, as the case of MI or corneal wounds. Without clear standards or guidelines, inadequate choice of the in vivo assays in animals can lead to misleading data interpretation.
Although there is still a long way to go to better understanding the real impact of nanotechnology, a elucidating nanoscale interactions, including dynamics of surface oxidation, capping agent replacement, and formation of supramolecular structures of nanomaterials in living organisms will pave the future of tissue engineering. Where macroscopic materials will be engineered from the nanoscale. Thus, once we fully understand those phenomena, we will be able to better design the next generation of tissue scaffolds, or even artificial organs, a Scientific legacy will redefine the field of regenerative medicine.
Author Contributions
Dr. EA and Dr. ES supervised the writing of the review. They also edited the text and guided the trainees in the bibliographical search for literature. Dr. MR and Dr. BM provided with critical revisions and suggestions on the manuscript. They also contributed with their expertise in tissue engineering to the final version. Ms. SM and Mr. JP performed the bibliographical search for the article, wrote sections for the review, and provided their critical vision on the problematic of nanomaterials to tissue engineering. They both are trainees in our research group, and part of their theses are related to nanomaterials in tissue engineering.
Conflict of Interest Statement
The authors declare that the research was conducted in the absence of any commercial or financial relationships that could be construed as a potential conflict of interest.
Funding
This work was funded by the Natural Sciences and Engineering Research Council (Discovery Grants #342107 to EJS and RGPIN-2015-06325 to EA). EA was also supported by UOHI. JP was supported by an Alexander Graham Bell/Canada Graduate Award (CGS-M/NSERC) and an Ontario Graduate Scholarship (OGS).
References
Adriana, F., Monica, P., Adrian, F., Ioana, B., Diana, O., Pompei, B., et al. (2015). Comparative evaluation by scanning confocal Raman spectroscopy and transmission electron microscopy of therapeutic effects of noble metal nanoparticles in experimental acute inflammation. RSC Adv. 5, 67435–67448. doi:10.1039/C5RA10376B
Alarcon, E. I., Bueno-Alejo, C. J., Noel, C. W., Stamplecoskie, K. G., Pacioni, N. L., Poblete, H., et al. (2013). Human serum albumin as protecting agent of silver nanoparticles: role of the protein conformation and amine groups in the nanoparticle stabilization. J. Nanopart. Res. 15, 1374–1377. doi:10.1007/s11051-012-1374-7
Alarcon, E. I., Udekwu, K., Noel, C. W., Gagnon, L. B.-P., Taylor, P. K., Vulesevic, B., et al. (2015). Safety and efficacy of composite collagen-silver nanoparticle hydrogels as tissue engineering scaffolds. Nanoscale 7, 18789–18798. doi:10.1039/c5nr03826j
Alarcon, E. I., Udekwu, K., Skog, M., Pacioni, N. L., Stamplecoskie, K. G., Gonzalez-Bejar, M., et al. (2012). The biocompatibility and antibacterial properties of collagen-stabilized, photochemically prepared silver nanoparticles. Biomaterials 33, 4947–4956. doi:10.1016/j.biomaterials.2012.03.033
Alarcon, E. I., Vulesevic, B., Argawal, A., Ross, A., Bejjani, P., Podrebarac, J., et al. (2016). Coloured cornea replacements with anti-infective properties: expanding the safe use of silver nanoparticles in regenerative medicine. Nanoscale 8, 6484–6489. doi:10.1039/c6nr01339b
Alexander, J. W. (2009). History of the medical use of silver. Surg. Infect. 10, 289–292. doi:10.1089/sur.2008.9941
Amsalem, Y., Mardor, Y., Feinberg, M. S., Landa, N., Miller, L., Daniels, D., et al. (2007). Iron-oxide labeling and outcome of transplanted mesenchymal stem cells in the infarcted myocardium. Circulation 116, I38–I45. doi:10.1161/CIRCULATIONAHA.106.680231
Andrews, K. L., Houdek, M. T., and Kiemele, L. J. (2015). Wound management of chronic diabetic foot ulcers: from the basics to regenerative medicine. Prosthet. Orthot. Int. 39, 29–39. doi:10.1177/0309364614534296
Archana, D., Dutta, J., and Dutta, P. K. (2013). Evaluation of chitosan nano dressing for wound healing: characterization, in vitro and in vivo studies. Int. J. Biol. Macromol. 57, 193–203. doi:10.1016/j.ijbiomac.2013.03.002
Arvizo, R. R., Bhattacharyya, S., Kudgus, R. A., Giri, K., Bhattacharya, R., and Mukherjee, P. (2012). Intrinsic therapeutic applications of noble metal nanoparticles: past, present and future. Chem. Soc. Rev. 41, 2943–2970. doi:10.1039/c2cs15355f
Arya, S. K., Lim, B., and Rahman, A. R. (2013). Enrichment, detection and clinical significance of circulating tumor cells. Lab. Chip 13, 1995–2027. doi:10.1039/c3lc00009e
Asharani, P., Low Kah Mun, G., Hande, M. P., and Valiyaveettil, S. (2008). Cytotoxicity and genotoxicity of silver nanoparticles in human cells. ACS Nano 3, 279–290. doi:10.1021/nn800596w
Attama, A. A., Reichl, S., and Muller-Goymann, C. C. (2009). Sustained release and permeation of timolol from surface-modified solid lipid nanoparticles through bioengineered human cornea. Curr. Eye Res. 34, 698–705. doi:10.1080/02713680903017500
Au, K.-W., Liao, S.-Y., Lee, Y.-K., Lai, W.-H., Ng, K.-M., Chan, Y.-C., et al. (2009). Effects of iron oxide nanoparticles on cardiac differentiation of embryonic stem cells. Biochem. Biophys. Res. Commun. 379, 898–903. doi:10.1016/j.bbrc.2008.12.160
Aziz, Z., Abu, S. F., and Chong, N. J. (2012). A systematic review of silver-containing dressings and topical silver agents (used with dressings) for burn wounds. Burns 38, 307–318. doi:10.1016/j.burns.2011.09.020
Baei, P., Jalili-Firoozinezhad, S., Rajabi-Zeleti, S., Tafazzoli-Shadpour, M., Baharvand, H., and Aghdami, N. (2016). Electrically conductive gold nanoparticle-chitosan thermosensitive hydrogels for cardiac tissue engineering. Mater. Sci. Eng. C 63, 131–141. doi:10.1016/j.msec.2016.02.056
Balint, R., Cassidy, N. J., and Cartmell, S. H. (2014). Conductive polymers: towards a smart biomaterial for tissue engineering. Acta Biomater. 10, 2341–2353. doi:10.1016/j.actbio.2014.02.015
Baltzis, D., Eleftheriadou, I., and Veves, A. (2014). Pathogenesis and treatment of impaired wound healing in diabetes mellitus: new insights. Adv. Ther. 31, 817–836. doi:10.1007/s12325-014-0140-x
Ban, K., Park, H.-J., Kim, S., Andukuri, A., Cho, K.-W., Hwang, J. W., et al. (2014). Cell therapy with embryonic stem cell-derived cardiomyocytes encapsulated in injectable nanomatrix gel enhances cell engraftment and promotes cardiac repair. ACS Nano 8, 10815–10825. doi:10.1021/nn504617g
Banquy, X., Suarez, F., Argaw, A., Rabanel, J.-M., Grutter, P., Bouchard, J.-F., et al. (2009). Effect of mechanical properties of hydrogel nanoparticles on macrophage cell uptake. Soft Matter 5, 3984–3991. doi:10.1039/b821583a
Banu, A., Noorul Hassan, M. M., Rajkumar, J., and Srinivasa, S. (2015). Spectrum of bacteria associated with diabetic foot ulcer and biofilm formation: a prospective study. Australas. Med. J. 8, 280–285. doi:10.4066/AMJ.2015.2422
Baum, C. L., and Arpey, C. J. (2005). Normal cutaneous wound healing: clinical correlation with cellular and molecular events. Dermatol. Surg. 31, 674–686. doi:10.1111/j.1524-4725.2005.31612
Bhol, K., and Schechter, P. (2007). Effects of nanocrystalline silver (NPI 32101) in a rat model of ulcerative colitis. Dig. Dis. Sci. 52, 2732–2742. doi:10.1007/s10620-006-9738-4
Bibee, K. P., Cheng, Y. J., Ching, J. K., Marsh, J. N., Li, A. J., Keeling, R. M., et al. (2014). Rapamycin nanoparticles target defective autophagy in muscular dystrophy to enhance both strength and cardiac function. FASEB J. 28, 2047–2061. doi:10.1096/fj.13-237388
Blecher, K., Martinez, L. R., Tuckman-Vernon, C., Nacharaju, P., Schairer, D., Chouake, J., et al. (2012). Nitric oxide-releasing nanoparticles accelerate wound healing in NOD-SCID mice. Nanomedicine 8, 1364–1371. doi:10.1016/j.nano.2012.02.014
Bondarenko, O., Juganson, K., Ivask, A., Kasemets, K., Mortimer, M., and Kahru, A. (2013). Toxicity of Ag, CuO and ZnO nanoparticles to selected environmentally relevant test organisms and mammalian cells in vitro: a critical review. Arch. Toxicol. 87, 1181–1200. doi:10.1007/s00204-013-1079-4
Bouwmeester, H., Poortman, J., Peters, R. J., Wijma, E., Kramer, E., Makama, S., et al. (2011). Characterization of translocation of silver nanoparticles and effects on whole-genome gene expression using an in vitro intestinal epithelium coculture model. ACS Nano 5, 4091–4103. doi:10.1021/nn2007145
Branton, M. H., and Kopp, J. B. (1999). TGF-beta and fibrosis. Microbes Infect. 1, 1349–1365. doi:10.1016/S1286-4579(99)00250-6
Buzea, C., Pacheco, I. I., and Robbie, K. (2007). Nanomaterials and nanoparticles: sources and toxicity. Biointerphases 2, MR17–MR71. doi:10.1116/1.2815690
Cai, X., and McGinnis, J. F. (2016). Nanoceria: a potential therapeutic for dry AMD. Adv. Exp. Med. Biol. 854, 111–118. doi:10.1007/978-3-319-17121-0_16
Chaurasia, S. S., Lim, R. R., Lakshminarayanan, R., and Mohan, R. R. (2015). Nanomedicine approaches for corneal diseases. J. Funct. Biomater. 6, 277–298. doi:10.3390/jfb6020277
Chen, M. C., Sun, Y. C., and Chen, Y. H. (2013). Electrically conductive nanofibers with highly oriented structures and their potential application in skeletal muscle tissue engineering. Acta Biomater. 9, 5562–5572. doi:10.1016/j.actbio.2012.10.024
Chen, W.-Y., Chang, H.-Y., Lu, J.-K., Huang, Y.-C., Harroun, S. G., Tseng, Y.-T., et al. (2015). Self-assembly of antimicrobial peptides on gold nanodots: against multidrug-resistant bacteria and wound-healing application. Adv. Funct. Mater. 25, 7189–7199. doi:10.1002/adfm.201503248
Cheng, K., Li, T. S., Malliaras, K., Davis, D. R., Zhang, Y., and Marban, E. (2010). Magnetic targeting enhances engraftment and functional benefit of iron-labeled cardiosphere-derived cells in myocardial infarction. Circ. Res. 106, 1570–1581. doi:10.1161/CIRCRESAHA.109.212589
Chigurupati, S., Mughal, M. R., Okun, E., Das, S., Kumar, A., McCaffery, M., et al. (2013). Effects of cerium oxide nanoparticles on the growth of keratinocytes, fibroblasts and vascular endothelial cells in cutaneous wound healing. Biomaterials 34, 2194–2201. doi:10.1016/j.biomaterials.2012.11.061
Choi, J. S., Lee, S. J., Christ, G. J., Atala, A., and Yoo, J. J. (2008a). The influence of electrospun aligned poly(epsilon-caprolactone)/collagen nanofiber meshes on the formation of self-aligned skeletal muscle myotubes. Biomaterials 29, 2899–2906. doi:10.1016/j.biomaterials.2008.03.031
Choi, J. S., Leong, K. W., and Yoo, H. S. (2008b). In vivo wound healing of diabetic ulcers using electrospun nanofibers immobilized with human epidermal growth factor (EGF). Biomaterials 29, 587–596. doi:10.1016/j.biomaterials.2007.10.012
Christman, K. L., and Lee, R. J. (2006). Biomaterials for the treatment of myocardial infarction. J. Am. Coll. Cardiol. 48, 907–913. doi:10.1016/j.jacc.2006.06.005
Colen, L. B., Kim, C. J., Grant, W. P., Yeh, J. T., and Hind, B. (2013). Achilles tendon lengthening: friend or foe in the diabetic foot? Plast. Reconstr. Surg. 131, 37e–43e. doi:10.1097/PRS.0b013e3182729e0b
Cozad, M. J., Bachman, S. L., and Grant, S. A. (2011). Assessment of decellularized porcine diaphragm conjugated with gold nanomaterials as a tissue scaffold for wound healing. J. Biomed. Mater. Res. A. 99, 426–434. doi:10.1002/jbm.a.33182
Credé, C. S. F. (1884). Die Verhutung der Augenentzundung der Neugeborenen (ophthalmoblennorrhoea neonatorum), der haufigsten und wichtigsten Ursache de Blindheit. Berlin: Verlag von August Hirschwald.
Cunha, C., Panseri, S., and Antonini, S. (2011). Emerging nanotechnology approaches in tissue engineering for peripheral nerve regeneration. Nanomedicine 7, 50–59. doi:10.1016/j.nano.2010.07.004
Cychosz, C. C., Phisitkul, P., Belatti, D. A., and Wukich, D. K. (2015). Current concepts review: preventive and therapeutic strategies for diabetic foot ulcers. Foot Ankle Int. doi:10.1177/1071100715611951
Daly, W., Yao, L., Zeugolis, D., Windebank, A., and Pandit, A. (2012). A biomaterials approach to peripheral nerve regeneration: bridging the peripheral nerve gap and enhancing functional recovery. J. R. Soc. Interface 9, 202–221. doi:10.1098/rsif.2011.0438
Das, S., Sharma, M., Saharia, D., Sarma, K. K., Sarma, M. G., Borthakur, B. B., et al. (2015). In vivo studies of silk based gold nano-composite conduits for functional peripheral nerve regeneration. Biomaterials 62, 66–75. doi:10.1016/j.biomaterials.2015.04.047
Davis, M. E., Hsieh, P. C. H., Takahashi, T., Song, Q., Zhang, S., Kamm, R. D., et al. (2006). Local myocardial insulin-like growth factor 1 (IGF-1) delivery with biotinylated peptide nanofibers improves cell therapy for myocardial infarction. Proc. Natl. Acad. Sci. U.S.A. 103, 8155–8160. doi:10.1073/pnas.0602877103
Davis, M. E., Motion, J. P., Narmoneva, D. A., Takahashi, T., Hakuno, D., Kamm, R. D., et al. (2005). Injectable self-assembling peptide nanofibers create intramyocardial microenvironments for endothelial cells. Circulation 111, 442–450. doi:10.1161/01.CIR.0000153847.47301.80
De Alwis Weerasekera, H., Griffith, M., and Alarcon, E. I. (2015). “Chapter 5. Biomedical uses of silver nanoparticles: from Roman wine cups to biomedical devices,” in Silver Nanoparticle Applications: In the Fabrication and Design of Medical and Biosensing Devices, eds E. I. Alarcon, M. Griffith, and K. Udekwu (UK: Springer), 93–125.
De Jong, W. H., and Borm, P. J. A. (2008). Drug delivery and nanoparticles: applications and hazards. Int. J. Nanomedicine 3, 133–149. doi:10.2147/IJN.S596
Dong, R. H., Jia, Y. X., Qin, C. C., Zhan, L., Yan, X., Cui, L., et al. (2016). In situ deposition of a personalized nanofibrous dressing via a handy electrospinning device for skin wound care. Nanoscale 8, 3482–3488. doi:10.1039/c5nr08367b
Dumville, J. C., Hinchliffe, R. J., Cullum, N., Game, F., Stubbs, N., Sweeting, M., et al. (2013). Negative pressure wound therapy for treating foot wounds in people with diabetes mellitus. Cochrane Database Syst. Rev. 10, Cd010318. doi:10.1002/14651858.CD010318.pub2
Dunn, R., Lyman, M. D., Edelman, P. G., and Campbell, P. K. (2001). Evaluation of the SprayGel adhesion barrier in the rat cecum abrasion and rabbit uterine horn adhesion models. Fertil. Steril. 75, 411–416. doi:10.1016/S0015-0282(00)01677-0
Dvir, T., Timko, B. P., Brigham, M. D., Naik, S. R., Karajanagi, S. S., Levy, O., et al. (2011). Nanowired three-dimensional cardiac patches. Nat. Nanotechnol. 6, 720–725. doi:10.1038/nnano.2011.160
Eckhardt, S., Brunetto, P. S., Gagnon, J., Priebe, M., Giese, B., and Fromm, K. M. (2013). Nanobio silver: its interactions with peptides and bacteria, and its uses in medicine. Chem. Rev. 113, 4708–4754. doi:10.1021/cr300288v
Ehrlich, H. P. (1998). The physiology of wound healing. A summary of normal and abnormal wound healing processes. Adv. Wound Care 11, 326–328.
Ellis-Behnke, R. (2007). Nano neurology and the four P’s of central nervous system regeneration: preserve, permit, promote, plasticity. Med. Clin. North Am. 91, 937–962.
Ellis-Behnke, R. G., Liang, Y. X., Guo, J., Tay, D. K., Schneider, G. E., Teather, L. A., et al. (2009). Forever young: how to control the elongation, differentiation, and proliferation of cells using nanotechnology. Cell Transplant. 18, 1047–1058. doi:10.3727/096368909X471242
Ellis-Behnke, R. G., Liang, Y. X., You, S. W., Tay, D. K., Zhang, S., So, K. F., et al. (2006). Nano neuro knitting: peptide nanofiber scaffold for brain repair and axon regeneration with functional return of vision. Proc. Natl. Acad. Sci. U.S.A. 103, 5054–5059. doi:10.1073/pnas.0600559103
Eming, S. A., Krieg, T., and Davidson, J. M. (2007). Inflammation in wound repair: molecular and cellular mechanisms. J. Invest. Dermatol. 127, 514–525. doi:10.1038/sj.jid.5700701
Eming, S. A., Martin, P., and Tomic-Canic, M. (2014). Wound repair and regeneration: mechanisms, signaling, and translation. Sci. Transl. Med. 6, 265sr266. doi:10.1126/scitranslmed.3009337
Etheridge, M. L., Campbell, S. A., Erdman, A. G., Haynes, C. L., Wolf, S. M., and McCullough, J. (2013). The big picture on nanomedicine: the state of investigational and approved nanomedicine products. Nanomedicine 9, 1–14. doi:10.1016/j.nano.2012.05.013
Fagerholm, P., Lagali, N. S., Merrett, K., Jackson, W. B., Munger, R., Liu, Y., et al. (2010). A biosynthetic alternative to human donor tissue for inducing corneal regeneration: 24-month follow-up of a phase 1 clinical study. Sci. Transl. Med. 2, 46ra61. doi:10.1126/scitranslmed.3001022
Fagerholm, P., Lagali, N. S., Ong, J. A., Merrett, K., Jackson, W. B., Polarek, J. W., et al. (2014). Stable corneal regeneration four years after implantation of a cell-free recombinant human collagen scaffold. Biomaterials 35, 2420–2427. doi:10.1016/j.biomaterials.2013.11.079
Fan, Z., Liu, B., Wang, J., Zhang, S., Lin, Q., Gong, P., et al. (2014). A novel wound dressing based on Ag/graphene polymer hydrogel: effectively kill bacteria and accelerate wound healing. Adv. Funct. Mater. 24, 3933–3943. doi:10.1002/adfm.201304202
Fang, C., and Zhang, M. (2009). Multifunctional magnetic nanoparticles for medical imaging applications. J. Mater. Chem. 19, 6258–6266. doi:10.1039/b902182e
Franca, R., Zhang, X. F., Veres, T., Yahia, L., and Sacher, E. (2013). Core-shell nanoparticles as prodrugs: possible cytotoxicological and biomedical impacts of batch-to-batch inconsistencies. J. Colloid Interface Sci. 389, 292–297. doi:10.1016/j.jcis.2012.08.065
Friedman, A. J., Han, G., Navati, M. S., Chacko, M., Gunther, L., Alfieri, A., et al. (2008). Sustained release nitric oxide releasing nanoparticles: characterization of a novel delivery platform based on nitrite containing hydrogel/glass composites. Nitric Oxide 19, 12–20. doi:10.1016/j.niox.2008.04.003
Fukai, T., Takeda, A., and Uchinuma, E. (2005). Wound healing in denervated rat skin. Wound Repair Regen. 13, 175–180. doi:10.1111/j.1067-1927.2005.130208.x
Fuoco, C., Sangalli, E., Vono, R., Testa, S., Sacchetti, B., Latronico, M. V., et al. (2014). 3D hydrogel environment rejuvenates aged pericytes for skeletal muscle tissue engineering. Front. Physiol. 5:203. doi:10.3389/fphys.2014.00203
Gaharwar, A. K., Peppas, N. A., and Khademhosseini, A. (2014). Nanocomposite hydrogels for biomedical applications. Biotechnol. Bioeng. 111, 441–453. doi:10.1002/bit.25160
Ge, G., Wu, H., Xiong, F., Zhang, Y., Guo, Z., Bian, Z., et al. (2013). The cytotoxicity evaluation of magnetic iron oxide nanoparticles on human aortic endothelial cells. Nanoscale Res. Lett. 8, 215. doi:10.1186/1556-276X-8-215
Gelain, F., Panseri, S., Antonini, S., Cunha, C., Donega, M., Lowery, J., et al. (2011). Transplantation of nanostructured composite scaffolds results in the regeneration of chronically injured spinal cords. ACS Nano 5, 227–236. doi:10.1021/nn102461w
Goldberg, J. L., and Barres, B. A. (2000). The relationship between neuronal survival and regeneration. Annu. Rev. Neurosci. 23, 579–612. doi:10.1146/annurev.neuro.23.1.579
Grasman, J. M., Zayas, M. J., Page, R. L., and Pins, G. D. (2015). Biomimetic scaffolds for regeneration of volumetric muscle loss in skeletal muscle injuries. Acta Biomater. 25, 2–15. doi:10.1016/j.actbio.2015.07.038
Greenhagen, R. M., Johnson, A. R., and Bevilacqua, N. J. (2012). Gastrocnemius recession or tendo-achilles lengthening for equinus deformity in the diabetic foot? Clin. Podiatr. Med. Surg. 29, 413–424. doi:10.1016/j.cpm.2012.04.005
Griffith, M., Polisetti, N., Kuffova, L., Gallar, J., Forrester, J., Vemuganti, G. K., et al. (2012). Regenerative approaches as alternatives to donor allografting for restoration of corneal function. Ocul. Surf. 10, 170–183. doi:10.1016/j.jtos.2012.04.004
Griffith, M., Udekwu, K., Gkotzis, S., Mah, T. F., and Alarcon, E. I. (2015). “Chapter 6. Anti-microbiological and anti-infective activities of silver,” in Silver Nanoparticle Applications: In the Fabrication and Design of Medical and Biosensing Devices (UK: Springer), 127–146.
Guirao, X., and Lowry, S. F. (1996). Biologic control of injury and inflammation: much more than too little or too late. World J. Surg. 20, 437–446. doi:10.1007/s002689900069
Gurtner, G. C., Werner, S., Barrandon, Y., and Longaker, M. T. (2008). Wound repair and regeneration. Nature 453, 314–321. doi:10.1038/nature07039
Han, G., Martinez, L. R., Mihu, M. R., Friedman, A. J., Friedman, J. M., and Nosanchuk, J. D. (2009). Nitric oxide releasing nanoparticles are therapeutic for Staphylococcus aureus abscesses in a murine model of infection. PLoS ONE 4:e7804. doi:10.1371/journal.pone.0007804
Han, G., Nguyen, L. N., Macherla, C., Chi, Y., Friedman, J. M., Nosanchuk, J. D., et al. (2012). Nitric oxide-releasing nanoparticles accelerate wound healing by promoting fibroblast migration and collagen deposition. Am. J. Pathol. 180, 1465–1473. doi:10.1016/j.ajpath.2011.12.013
Han, J., Kim, B., Shin, J.-Y., Ryu, S., Noh, M., Woo, J., et al. (2015). Iron oxide nanoparticle-mediated development of cellular gap junction crosstalk to improve mesenchymal stem cells’ therapeutic efficacy for myocardial infarction. ACS Nano 9, 2805–2819. doi:10.1021/nn506732n
Herreros, E., Morales, S., Cortes, C., Cabana, M., Penaloza, J. P., Jara, L., et al. (2014). Advances in nanomedicine towards clinical application in oncology and immunology. Curr. Pharm. Biotechnol. 15, 864–879. doi:10.2174/1389201015666140909122727
Herron, M., Agarwal, A., Kierski, P. R., Calderon, D. F., Teixeira, L. B., Schurr, M. J., et al. (2014). Reduction in wound bioburden using a silver-loaded dissolvable microfilm construct. Adv. Healthc. Mater. 3, 916–928. doi:10.1002/adhm.201300537
Holmes, C., Wrobel, J. S., Maceachern, M. P., and Boles, B. R. (2013). Collagen-based wound dressings for the treatment of diabetes-related foot ulcers: a systematic review. Diabetes Metab. Syndr. Obes. 6, 17–29. doi:10.2147/DMSO.S36024
Hsieh, P. C., Davis, M. E., Gannon, J., Macgillivray, C., and Lee, R. T. (2006a). Controlled delivery of PDGF-BB for myocardial protection using injectable self-assembling peptide nanofibers. J. Clin. Invest. 116, 237–248. doi:10.1172/JCI25878
Hsieh, P. C., Macgillivray, C., Gannon, J., Cruz, F. U., and Lee, R. T. (2006b). Local controlled intramyocardial delivery of platelet-derived growth factor improves postinfarction ventricular function without pulmonary toxicity. Circulation 114, 637–644. doi:10.1161/CIRCULATIONAHA.106.639831
Hynesl, R. O., Su, X., and Rich, A. (1995). Self-complementary oligopeptide matrices support mammalian cell. Biomaterials 16, 1385–1393. doi:10.1016/0142-9612(95)96874-Y
Ishizaki, K., Sugita, Y., Iwasa, F., Minamikawa, H., Ueno, T., Yamada, M., et al. (2011). Nanometer-thin TiO(2) enhances skeletal muscle cell phenotype and behavior. Int. J. Nanomedicine 6, 2191–2203. doi:10.2147/IJN.S24839
Ito, A., Ino, K., Hayashida, M., Kobayashi, T., Matsunuma, H., Kagami, H., et al. (2005). Novel methodology for fabrication of tissue-engineered tubular constructs using magnetite nanoparticles and magnetic force. Tissue Eng. 11, 1553–1561. doi:10.1089/ten.2005.11.1553
Jo, D. H., Kim, J. H., Lee, T. G., and Kim, J. H. (2015). Size, surface charge, and shape determine therapeutic effects of nanoparticles on brain and retinal diseases. Nanomedicine 11, 1603–1611. doi:10.1016/j.nano.2015.04.015
Jo, D. H., Kim, J. H., Son, J. G., Song, N. W., Kim, Y. I., Yu, Y. S., et al. (2014). Anti-angiogenic effect of bare titanium dioxide nanoparticles on pathologic neovascularization without unbearable toxicity. Nanomedicine 10, 1109–1117. doi:10.1016/j.nano.2014.02.007
Johnson, T. D., and Christman, K. L. (2013). Injectable hydrogel therapies and their delivery strategies for treating myocardial infarction. Expert Opin. Drug Deliv. 10, 59–72. doi:10.1517/17425247.2013.739156
Ju, Y. M., Atala, A., Yoo, J. J., and Lee, S. J. (2014). In situ regeneration of skeletal muscle tissue through host cell recruitment. Acta Biomater. 10, 4332–4339. doi:10.1016/j.actbio.2014.06.022
Kahari, V. M., and Saarialho-Kere, U. (1997). Matrix metalloproteinases in skin. Exp. Dermatol. 6, 199–213. doi:10.1111/j.1600-0625.1997.tb00164.x
Kai, D., Prabhakaran, M. P., Jin, G., and Ramakrishna, S. (2011). Guided orientation of cardiomyocytes on electrospun aligned nanofibers for cardiac tissue engineering. J. Biomed. Mater. Res. Part B Appl. Biomater. 98B, 379–386. doi:10.1002/jbm.b.31862
Keefe, A. D., Pai, S., and Ellington, A. (2010). Aptamers as therapeutics. Nat. Rev. Drug Discov. 9, 537–550. doi:10.1038/nrd3141
Khlebtsov, N., and Dykman, L. (2011). Biodistribution and toxicity of engineered gold nanoparticles: a review of in vitro and in vivo studies. Chem. Soc. Rev. 40, 1647–1671. doi:10.1039/c0cs00018c
Kim, J. E., Lee, J., Jang, M., Kwak, M. H., Go, J., Kho, E. K., et al. (2015). Accelerated healing of cutaneous wounds using phytochemically stabilized gold nanoparticle deposited hydrocolloid membranes. Biomater. Sci. 3, 509–519. doi:10.1039/c4bm00390j
Kim, J. H., Kim, M. H., Jo, D. H., Yu, Y. S., Lee, T. G., and Kim, J. H. (2011). The inhibition of retinal neovascularization by gold nanoparticles via suppression of VEGFR-2 activation. Biomaterials 32, 1865–1871. doi:10.1016/j.biomaterials.2010.11.030
Kin, S., Hagiwara, A., Nakase, Y., Kuriu, Y., Nakashima, S., Yoshikawa, T., et al. (2007). Regeneration of skeletal muscle using in situ tissue engineering on an acellular collagen sponge scaffold in a rabbit model. ASAIO J. 53, 506–513. doi:10.1097/MAT.0b013e3180d09d81
Kirsner, R. S., and Eaglstein, W. H. (1993). The wound healing process. Dermatol. Clin. 11, 629–640.
Klasen, H. J. (2000). A historical review of the use of silver in the treatment of burns. II. Renewed interest for silver. Burns 26, 131–138. doi:10.1016/S0305-4179(99)00108-4
Klumpp, D., Horch, R. E., Kneser, U., and Beier, J. P. (2010). Engineering skeletal muscle tissue – new perspectives in vitro and in vivo. J. Cell. Mol. Med. 14, 2622–2629. doi:10.1111/j.1582-4934.2010.01183.x
Kong, L., Cai, X., Zhou, X., Wong, L. L., Karakoti, A. S., Seal, S., et al. (2011). Nanoceria extend photoreceptor cell lifespan in tubby mice by modulation of apoptosis/survival signaling pathways. Neurobiol. Dis. 42, 514–523. doi:10.1016/j.nbd.2011.03.004
Koning, M., Harmsen, M. C., Van Luyn, M. J., and Werker, P. M. (2009). Current opportunities and challenges in skeletal muscle tissue engineering. J. Tissue Eng. Regen. Med. 3, 407–415. doi:10.1002/term.190
Kraitchman, D. L., Heldman, A. W., Atalar, E., Amado, L. C., Martin, B. J., Pittenger, M. F., et al. (2003). In vivo magnetic resonance imaging of mesenchymal stem cells in myocardial infarction. Circulation 107, 2290–2293. doi:10.1161/01.CIR.0000070931.62772.4E
Kumar, V. A., Taylor, N. L., Shi, S., Wang, B. K., Jalan, A. A., Kang, M. K., et al. (2015). Highly angiogenic peptide nanofibers. ACS Nano 9, 860–868. doi:10.1021/nn506544b
Lansdown, A. B. G. (2010). “Chapter 1 silver in health and disease,” in Silver in Healthcare: Its Antimicrobial Efficacy and Safety in Use, ed. A. B. G. Lansdown (Boca Raton, FL: CRC Press), 1–8.
Lavery, L. A., Fulmer, J., Shebetka, K. A., Regulski, M., Vayser, D., Fried, D., et al. (2014). The efficacy and safety of Grafix((R)) for the treatment of chronic diabetic foot ulcers: results of a multi-centre, controlled, randomised, blinded, clinical trial. Int. Wound J. 11, 554–560. doi:10.1111/iwj.12329
Lee, C. H., Hsieh, M. J., Chang, S. H., Lin, Y. H., Liu, S. J., Lin, T. Y., et al. (2014). Enhancement of diabetic wound repair using biodegradable nanofibrous metformin-eluting membranes: in vitro and in vivo. ACS Appl. Mater. Interfaces 6, 3979–3986. doi:10.1021/am405329g
Lee, W. C., Lim, C. H. Y. X., Shi, H., Tang, L. A. L., Wang, Y., Lim, C. T., et al. (2011). Origin of enhanced stem cell growth and differentiation on graphene and graphene oxide. ACS Nano 5, 7334–7341. doi:10.1021/nn202190c
Leu, J. G., Chen, S. A., Chen, H. M., Wu, W. M., Hung, C. F., Yao, Y. D., et al. (2012). The effects of gold nanoparticles in wound healing with antioxidant epigallocatechin gallate and alpha-lipoic acid. Nanomedicine 8, 767–775.
Li, L., Jiang, W., Luo, K., Song, H., Lan, F., Wu, Y., et al. (2013). Superparamagnetic iron oxide nanoparticles as MRI contrast agents for non-invasive stem cell labeling and tracking. Theranostics 3, 595–615. doi:10.7150/thno.5366
Liang, Y. X., Cheung, S. W., Chan, K. C., Wu, E. X., Tay, D. K., and Ellis-Behnke, R. G. (2011). CNS regeneration after chronic injury using a self-assembled nanomaterial and MEMRI for real-time in vivo monitoring. Nanomedicine 7, 351–359. doi:10.1016/j.nano.2010.12.001
Lin, T. C., Hung, K. H., Peng, C. H., Liu, J. H., Woung, L. C., Tsai, C. Y., et al. (2015). Nanotechnology-based drug delivery treatments and specific targeting therapy for age-related macular degeneration. J. Chin. Med. Assoc. 78, 635–641. doi:10.1016/j.jcma.2015.07.008
Lin, Y.-D., Luo, C.-Y., Hu, Y.-N., Yeh, M.-L., Hsueh, Y.-C., Chang, M.-Y., et al. (2012). Instructive nanofiber scaffolds with VEGF create a microenvironment for arteriogenesis and cardiac repair. Sci. Transl. Med. 4, 146ra109. doi:10.1126/scitranslmed.3003841
Lin, Y.-D., Yeh, M.-L., Yang, Y.-J., Tsai, D.-C., Chu, T.-Y., Shih, Y.-Y., et al. (2010). Intramyocardial peptide nanofiber injection improves postinfarction ventricular remodeling and efficacy of bone marrow cell therapy in pigs. Circulation 122, S132–S141. doi:10.1161/CIRCULATIONAHA.110.939512
Lipsky, B. A., Berendt, A. R., Cornia, P. B., Pile, J. C., Peters, E. J. G., Armstrong, D. G., et al. (2012). 2012 Infectious diseases society of America clinical practice guideline for the diagnosis and treatment of diabetic foot infections. Clin. Infect. Dis. 54, e132–e173. doi:10.1093/cid/cis460
Liu, X., Lee, P. Y., Ho, C. M., Lui, V. C. H., Chen, Y., Che, C. M., et al. (2010). Silver nanoparticles mediate differential responses in keratinocytes and fibroblasts during skin wound healing. ChemMedChem 5, 468–475. doi:10.1002/cmdc.200900502
Liu, Y., Min, D., Bolton, T., Nube, V., Twigg, S. M., Yue, D. K., et al. (2009). Increased matrix metalloproteinase-9 predicts poor wound healing in diabetic foot ulcers. Diabetes Care 32, 117–119. doi:10.2337/dc08-0763
Londahl, M., Katzman, P., Nilsson, A., and Hammarlund, C. (2010). Hyperbaric oxygen therapy facilitates healing of chronic foot ulcers in patients with diabetes. Diabetes Care 33, 998–1003. doi:10.2337/dc09-1754
Lookingbill, D. P., Miller, S. H., and Knowles, R. C. (1978). Bacteriology of chronic leg ulcers. Arch. Dermatol. 114, 1765–1768. doi:10.1001/archderm.114.12.1765
Lu, B., Li, T., Zhao, H., Li, X., Gao, C., Zhang, S., et al. (2012a). Graphene-based composite materials beneficial to wound healing. Nanoscale 4, 2978–2982. doi:10.1039/c2nr11958g
Lu, R., Yang, D., Cui, D., Wang, Z., and Guo, L. (2012b). Egg white-mediated green synthesis of silver nanoparticles with excellent biocompatibility and enhanced radiation effects on cancer cells. Int. J. Nanomedicine 7, 2101. doi:10.2147/IJN.S29762
Luo, L., Zhang, X., Hirano, Y., Tyagi, P., Barabas, P., Uehara, H., et al. (2013). Targeted intraceptor nanoparticle therapy reduces angiogenesis and fibrosis in primate and murine macular degeneration. ACS Nano 7, 3264–3275. doi:10.1021/nn305958y
Ma, L., Li, P., Shi, Z., Hou, T., Chen, X., and Du, J. (2013). A prospective, randomized, controlled study of hyperbaric oxygen therapy: effects on healing and oxidative stress of ulcer tissue in patients with a diabetic foot ulcer. Ostomy Wound Manage. 59, 18–24.
Madsen, S. M., Westh, H., Danielsen, L., and Rosdahl, V. T. (1996). Bacterial colonization and healing of venous leg ulcers. APMIS 104, 895–899. doi:10.1111/j.1699-0463.1996.tb04955.x
Mahmoudi, M., Hofmann, H., Rothen-Rutishauser, B., and Petri-Fink, A. (2012). Assessing the in vitro and in vivo toxicity of superparamagnetic iron oxide nanoparticles. Chem. Rev. 112, 2323–2338. doi:10.1021/cr2002596
Mahmoudi, M., Laurent, S., Shokrgozar, M. A., and Hosseinkhani, M. (2011). Toxicity evaluations of superparamagnetic iron oxide nanoparticles: cell “vision” versus physicochemical properties of nanoparticles. ACS Nano 5, 7263–7276. doi:10.1021/nn2021088
Marston, W. A., Hanft, J., Norwood, P., Pollak, R., Dermagraft Diabetic Foot Ulcer Study Group. (2003). The efficacy and safety of dermagraft in improving the healing of chronic diabetic foot ulcers: results of a prospective randomized trial. Diabetes Care 26, 1701–1705. doi:10.2337/diacare.26.6.1701
Martin, P. (1997). Wound healing – aiming for perfect skin regeneration. Science 276, 75–81. doi:10.1126/science.276.5309.75
Martinelli, V., Cellot, G., Toma, F. M., Long, C. S., Caldwell, J. H., Zentilin, L., et al. (2012). Carbon nanotubes promote growth and spontaneous electrical activity in cultured cardiac myocytes. Nano Lett. 12, 1831–1838. doi:10.1021/nl204064s
Martinez, L. R., Han, G., Chacko, M., Mihu, M. R., Jacobson, M., Gialanella, P., et al. (2009). Antimicrobial and healing efficacy of sustained release nitric oxide nanoparticles against Staphylococcus aureus skin infection. J. Invest. Dermatol. 129, 2463–2469. doi:10.1038/jid.2009.95
McKeon-Fischer, K. D., and Freeman, J. W. (2011). Characterization of electrospun poly(L-lactide) and gold nanoparticle composite scaffolds for skeletal muscle tissue engineering. J. Tissue Eng. Regen. Med. 5, 560–568. doi:10.1002/term.348
McKeon-Fischer, K. D., Rossmeisl, J. H., Whittington, A. R., and Freeman, J. W. (2014). In vivo skeletal muscle biocompatibility of composite, coaxial electrospun, and microfibrous scaffolds. Tissue Eng. Part A 20, 1961–1970. doi:10.1089/ten.TEA.2013.0283
Mehta, P., Justo, L., Walsh, S., Arshad, M. S., Wilson, C. G., O’sullivan, C. K., et al. (2015). New platforms for multi-functional ocular lenses: engineering double-sided functionalized nano-coatings. J. Drug Target. 23, 305–310. doi:10.3109/1061186X.2014.1001395
Mishra, M. K., Beaty, C. A., Lesniak, W. G., Kambhampati, S. P., Zhang, F., Wilson, M. A., et al. (2014). Dendrimer brain uptake and targeted therapy for brain injury in a large animal model of hypothermic circulatory arrest. ACS Nano 8, 2134–2147. doi:10.1021/nn404872e
Morrow, K. J. Jr., Bawa, R., and Wei, C. (2007). Recent advances in basic and clinical nanomedicine. Med. Clin. North Am. 91, 805–843. doi:10.1016/j.mcna.2007.05.009
Moulton, M. C., Braydich-Stolle, L. K., Nadagouda, M. N., Kunzelman, S., Hussain, S. M., and Varma, R. S. (2010). Synthesis, characterization and biocompatibility of “green” synthesized silver nanoparticles using tea polyphenols. Nanoscale 2, 763–770. doi:10.1039/c0nr00046a
Mueller, M. J., Sinacore, D. R., Hastings, M. K., Strube, M. J., and Johnson, J. E. (2003). Effect of Achilles tendon lengthening on neuropathic plantar ulcers. A randomized clinical trial. J. Bone Joint Surg. Am. 85-A, 1436–1445.
Mukherjee, S., Reddy Venugopal, J., Ravichandran, R., Ramakrishna, S., and Raghunath, M. (2011). Evaluation of the biocompatibility of PLACL/collagen nanostructured matrices with cardiomyocytes as a model for the regeneration of infarcted myocardium. Adv. Funct. Mater. 21, 2291–2300. doi:10.1002/adfm.201002434
Nanjwade, B. K., Bechra, H. M., Derkar, G. K., Manvi, F. V., and Nanjwade, V. K. (2009). Dendrimers: emerging polymers for drug-delivery systems. Eur. J. Pharm. Sci. 38, 185–196. doi:10.1016/j.ejps.2009.07.008
Neibert, K., Gopishetty, V., Grigoryev, A., Tokarev, I., Al-Hajaj, N., Vorstenbosch, J., et al. (2012). Wound-healing with mechanically robust and biodegradable hydrogel fibers loaded with silver nanoparticles. Adv. Healthc. Mater. 1, 621–630. doi:10.1002/adhm.201200075
Nguyen, M. M., Carlini, A. S., Chien, M.-P., Sonnenberg, S., Luo, C., Braden, R. L., et al. (2015a). Enzyme-responsive nanoparticles for targeted accumulation and prolonged retention in heart tissue after myocardial infarction. Adv. Mater. Weinheim 27, 5547–5552. doi:10.1002/adma.201570243
Nguyen, M. M., Gianneschi, N. C., and Christman, K. L. (2015b). Developing injectable nanomaterials to repair the heart. Curr. Opin. Biotechnol. 34, 225–231. doi:10.1016/j.copbio.2015.03.016
Niu, J., Azfer, A., Rogers, L., Wang, X., and Kolattukudy, P. (2007). Cardioprotective effects of cerium oxide nanoparticles in a transgenic murine model of cardiomyopathy. Cardiovasc. Res. 73, 549–559. doi:10.1016/j.cardiores.2006.11.031
Norbury, W., Herndon, D. N., Tanksley, J., Jeschke, M. G., and Finnerty, C. C. (2016). Infection in burns. Surg. Infect. 17, 250–255. doi:10.1089/sur.2013.134
O’Brien, B., Zafar, H., Ibrahim, A., Zafar, J., and Sharif, F. (2015). Coronary stent materials and coatings: a technology and performance update. Ann. Biomed. Eng. 44, 523–535. doi:10.1007/s10439-015-1380-x
Okamura, Y., Kabata, K., Kinoshita, M., Miyazaki, H., Saito, A., Fujie, T., et al. (2013). Fragmentation of poly(lactic acid) nanosheets and patchwork treatment for burn wounds. Adv. Mater. 25, 545–551. doi:10.1002/adma.201202851
Oliva, M. S., Schottman, T., and Gulati, M. (2012). Turning the tide of corneal blindness. Indian J. Ophthalmol. 60, 423–427. doi:10.4103/0301-4738.100540
O’Loughlin, A., McIntosh, C., Dinneen, S. F., and O’Brien, T. (2010). Review paper: basic concepts to novel therapies: a review of the diabetic foot. Int. J. Low. Extrem. Wounds 9, 90–102. doi:10.1177/1534734610371600
Orive, G., Anitua, E., Pedraz, J. L., and Emerich, D. F. (2009). Biomaterials for promoting brain protection, repair and regeneration. Nat. Rev. Neurosci. 10, 682–692. doi:10.1038/nrn2685
Orza, A., Soritau, O., Olenic, L., Diudea, M., Florea, A., Rus Ciuca, D., et al. (2011). Electrically conductive gold-coated collagen nanofibers for placental-derived mesenchymal stem cells enhanced differentiation and proliferation. ACS Nano 5, 4490–4503. doi:10.1021/nn1035312
Ostdiek, A. M., Ivey, J. R., Grant, D. A., Gopaldas, J., and Grant, S. A. (2015). An in vivo study of a gold nanocomposite biomaterial for vascular repair. Biomaterials 65, 175–183. doi:10.1016/j.biomaterials.2015.06.045
Padin-Iruegas, M. E., Misao, Y., Davis, M. E., Segers, V. F., Esposito, G., Tokunou, T., et al. (2009). Cardiac progenitor cells and biotinylated insulin-like growth factor-1 nanofibers improve endogenous and exogenous myocardial regeneration after infarction. Circulation 120, 876–887. doi:10.1161/CIRCULATIONAHA.109.852285
Pagliari, F., Mandoli, C., Forte, G., Magnani, E., Pagliari, S., Nardone, G., et al. (2012). Cerium oxide nanoparticles protect cardiac progenitor cells from oxidative stress. ACS Nano 6, 3767–3775. doi:10.1021/nn2048069
Park, J., Kim, B., Han, J., Oh, J., Park, S., Ryu, S., et al. (2015a). Graphene oxide flakes as a cellular adhesive: prevention of reactive oxygen species mediated death of implanted cells for cardiac repair. ACS Nano 9, 4987–4999. doi:10.1021/nn507149w
Park, J., Kim, Y. S., Ryu, S., Kang, W. S., Park, S., Han, J., et al. (2015b). Graphene potentiates the myocardial repair efficacy of mesenchymal stem cells by stimulating the expression of angiogenic growth factors and gap junction protein. Adv. Funct. Mater. 25, 2590–2600. doi:10.1002/adfm.201500365
Paul, A., Hasan, A., Kindi, H. A., Gaharwar, A. K., Rao, V. T. S., Nikkhah, M., et al. (2014). Injectable graphene oxide/hydrogel-based angiogenic gene delivery system for vasculogenesis and cardiac repair. ACS Nano 8, 8050–8062. doi:10.1021/nn5020787
Paviolo, C., and Stoddart, P. R. (2015). Metallic nanoparticles for peripheral nerve regeneration: is it a feasible approach? Neural Regen. Res. 10, 1065–1066. doi:10.4103/1673-5374.160083
Pedrosa, P., Vinhas, R., Fernandes, A., and Baptista, V. P. (2015). Gold nanotheranostics: proof-of-concept or clinical tool? Nanomaterials 5, 1853–1879. doi:10.3390/nano5041853
Pektok, E., Nottelet, B., Tille, J. C., Gurny, R., Kalangos, A., Moeller, M., et al. (2008). Degradation and healing characteristics of small-diameter poly(epsilon-caprolactone) vascular grafts in the rat systemic arterial circulation. Circulation 118, 2563–2570. doi:10.1161/CIRCULATIONAHA.108.795732
Pfeffer, M. A., and Braunwald, E. (1990). Ventricular remodeling after myocardial infarction. Experimental observations and clinical implications. Circulation 81, 1161–1172. doi:10.1161/01.CIR.81.4.1161
Pinney, S. J., Hansen, S. T. Jr., and Sangeorzan, B. J. (2002). The effect on ankle dorsiflexion of gastrocnemius recession. Foot Ankle Int. 23, 26–29.
Provenzale, J. M., and Silva, G. A. (2009). Uses of nanoparticles for central nervous system imaging and therapy. AJNR Am. J. Neuroradiol. 30, 1293–1301. doi:10.3174/ajnr.A1590
Quigley, A. F., Razal, J. M., Kita, M., Jalili, R., Gelmi, A., Penington, A., et al. (2012). Electrical stimulation of myoblast proliferation and differentiation on aligned nanostructured conductive polymer platforms. Adv. Healthc. Mater. 1, 801–808. doi:10.1002/adhm.201200102
Rai, M., Ingle, A. P., Gaikwad, S., Padovani, F. H., and Alves, M. (2015). The role of nanotechnology in control of human diseases: perspectives in ocular surface diseases. Crit. Rev. Biotechnol. doi:10.3109/07388551.2015.1036002
Rai, M. K., Deshmukh, S. D., Ingle, A. P., and Gade, A. K. (2012). Silver nanoparticles: the powerful nanoweapon against multidrug-resistant bacteria. J. Appl. Microbiol. 112, 841–852. doi:10.1111/j.1365-2672.2012.05253.x
Rajala, A., Wang, Y., Zhu, Y., Ranjo-Bishop, M., Ma, J. X., Mao, C., et al. (2014). Nanoparticle-assisted targeted delivery of eye-specific genes to eyes significantly improves the vision of blind mice in vivo. Nano Lett. 14, 5257–5263. doi:10.1021/nl502275s
Ramsden, C. M., Powner, M. B., Carr, A. J., Smart, M. J., Da Cruz, L., and Coffey, P. J. (2013). Stem cells in retinal regeneration: past, present and future. Development 140, 2576–2585. doi:10.1242/dev.092270
Reimer, P., and Balzer, T. (2003). Ferucarbotran (Resovist): a new clinically approved RES-specific contrast agent for contrast-enhanced MRI of the liver: properties, clinical development, and applications. Eur. Radiol. 13, 1266–1276.
Rhee, S. M., Valle, M. F., Wilson, L. M., Lazarus, G., Zenilman, J. M., and Robinson, K. A. (2014). “AHRQ technology assessments,” in Negative Pressure Wound Therapy Technologies for Chronic Wound Care in the Home Setting (Rockville, MD: Agency for Healthcare Research and Quality (US)).
Robson, M. C. (1997). Wound infection. A failure of wound healing caused by an imbalance of bacteria. Surg. Clin. North Am. 77, 637–650. doi:10.1016/S0039-6109(05)70572-7
Roe, A. L. (1915). Collosol argentum and its ophthalmic uses. Br. Med. J. 1, 104–104. doi:10.1136/bmj.1.2820.104
Rogers, A. A., Burnett, S., Moore, J. C., Shakespeare, P. G., and Chen, W. Y. (1995). Involvement of proteolytic enzymes – plasminogen activators and matrix metalloproteinases – in the pathophysiology of pressure ulcers. Wound Repair Regen. 3, 273–283. doi:10.1046/j.1524-475X.1995.30307.x
Rosenthal, V. D., Bijie, H., Maki, D. G., Mehta, Y., Apisarnthanarak, A., Medeiros, E. A., et al. (2012). International nosocomial infection control consortium (INICC) report, data summary of 36 countries, for 2004-2009. Am. J. Infect. Control 40, 396–407. doi:10.1016/j.ajic.2011.05.020
Sang, L. Y., Liang, Y. X., Li, Y., Wong, W. M., Tay, D. K., So, K. F., et al. (2015). A self-assembling nanomaterial reduces acute brain injury and enhances functional recovery in a rat model of intracerebral hemorrhage. Nanomedicine 11, 611–620. doi:10.1016/j.nano.2014.05.012
Santoro, C. M., Duchsherer, N. L., and Grainger, D. W. (2007). Antimicrobial efficacy and ocular cell toxicity from silver nanoparticles. Nanobiotechnology 3, 55–65. doi:10.1007/s12030-008-9007-z
Schipper, M. L., Nakayama-Ratchford, N., Davis, C. R., Kam, N. W. S., Chu, P., Liu, Z., et al. (2008). A pilot toxicology study of single-walled carbon nanotubes in a small sample of mice. Nat. Nanotechnol. 3, 216–221. doi:10.1038/nnano.2008.68
Segers, V. F., Tokunou, T., Higgins, L. J., Macgillivray, C., Gannon, J., and Lee, R. T. (2007). Local delivery of protease-resistant stromal cell derived factor-1 for stem cell recruitment after myocardial infarction. Circulation 116, 1683–1692. doi:10.1161/CIRCULATIONAHA.107.718718
Seil, J. T., and Webster, T. J. (2010). Electrically active nanomaterials as improved neural tissue regeneration scaffolds. Wiley Interdiscip. Rev. Nanomed. Nanobiotechnol. 2, 635–647. doi:10.1002/wnan.109
Sharaf, M. G., Cetinel, S., Heckler, L., Damji, K., Unsworth, L., and Montemagno, C. (2014). Nanotechnology-based approaches for ophthalmology applications: therapeutic and diagnostic strategies. Asia Pac. J. Ophthalmol. (Phila.) 3, 172–180. doi:10.1097/APO.0000000000000059
Sharma, A., Tandon, A., Tovey, J. C., Gupta, R., Robertson, J. D., Fortune, J. A., et al. (2011). Polyethylenimine-conjugated gold nanoparticles: gene transfer potential and low toxicity in the cornea. Nanomedicine 7, 505–513. doi:10.1016/j.nano.2011.01.006
Shevach, M., Fleischer, S., Shapira, A., and Dvir, T. (2014). Gold nanoparticle-decellularized matrix hybrids for cardiac tissue engineering. Nano Lett. 14, 5792–5796. doi:10.1021/nl502673m
Shin, S. R., Jung, S. M., Zalabany, M., Kim, K., Zorlutuna, P., Kim, S. B., et al. (2013). Carbon-nanotube-embedded hydrogel sheets for engineering cardiac constructs and bioactuators. ACS Nano 7, 2369–2380. doi:10.1021/nn305559j
Sibbald, R. G., Williamson, D., Orsted, H. L., Campbell, K., Keast, D., Krasner, D., et al. (2000). Preparing the wound bed – debridement, bacterial balance, and moisture balance. Ostomy Wound Manage. 46, 14–22, 24–8, 30–5.
Sicari, B. M., Dearth, C. L., and Badylak, S. F. (2014). Tissue engineering and regenerative medicine approaches to enhance the functional response to skeletal muscle injury. Anat. Rec. (Hoboken) 297, 51–64. doi:10.1002/ar.22794
Siemionow, M., Bozkurt, M., and Zor, F. (2010). Regeneration and repair of peripheral nerves with different biomaterials: review. Microsurgery 30, 574–588. doi:10.1002/micr.20799
Simpson, M. J., Poblete, H., Griffith, M., Alarcon, E. I., and Scaiano, J. C. (2013). Impact of dye-protein interaction and silver nanoparticles on rose Bengal photophysical behavior and protein photocrosslinking. Photochem. Photobiol. 89, 1433–1441. doi:10.1111/php.12119
Singer, A. J., and Clark, R. A. (1999). Cutaneous wound healing. N. Engl. J. Med. 341, 738–746. doi:10.1056/NEJM199909023411006
Sisco, P. N., Wilson, C. G., Mironova, E., Baxter, S. C., Murphy, C. J., and Goldsmith, E. C. (2008). The effect of gold nanorods on cell-mediated collagen remodeling. Nano Lett. 8, 3409–3412. doi:10.1021/nl802142h
Smith, D. M., Simon, J. K., and Baker, J. R. Jr. (2013). Applications of nanotechnology for immunology. Nat. Rev. Immunol. 13, 592–605. doi:10.1038/nri3488
Souza, G. R., Molina, J. R., Raphael, R. M., Ozawa, M. G., Stark, D. J., Levin, C. S., et al. (2010). Three-dimensional tissue culture based on magnetic cell levitation. Nat. Nanotechnol. 5, 291–296. doi:10.1038/nnano.2010.23
Spivak, M. Y., Bubnov, R. V., Yemets, I. M., Lazarenko, L. M., Tymoshok, N. O., and Ulberg, Z. R. (2013). Development and testing of gold nanoparticles for drug delivery and treatment of heart failure: a theranostic potential for PPP cardiology. EPMA J. 4, 20. doi:10.1186/1878-5085-4-20
Stoehr, L. C., Gonzalez, E., Stampfl, A., Casals, E., Duschl, A., Puntes, V., et al. (2011). Shape matters: effects of silver nanospheres and wires on human alveolar epithelial cells. Part. Fibre Toxicol. 8, 3–15. doi:10.1186/1743-8977-8-36
Sun, H., Gao, N., Dong, K., Ren, J., and Qu, X. (2014). Graphene quantum dots-band-aids used for wound disinfection. ACS Nano 8, 6202–6210. doi:10.1021/nn501640q
Sutton, M. G. S. J., and Sharpe, N. (2000). Left ventricular remodeling after myocardial infarction pathophysiology and therapy. Circulation 101, 2981–2988. doi:10.1161/01.CIR.101.25.2981
Takeda, Y. S., and Xu, Q. (2015). Synthetic and nature-derived lipid nanoparticles for neural regeneration. Neural Regen. Res. 10, 689–690. doi:10.4103/1673-5374.156946
Tam, J., Wang, Y., Farinelli, W. A., Jimenez-Lozano, J., Franco, W., Sakamoto, F. H., et al. (2013). Fractional skin harvesting: autologous skin grafting without donor-site morbidity. Plast. Reconstr. Surg. Glob. Open 1, e47. doi:10.1097/GOX.0b013e3182a85a36
Tandon, A., Sharma, A., Rodier, J. T., Klibanov, A. M., Rieger, F. G., and Mohan, R. R. (2013). BMP7 gene transfer via gold nanoparticles into stroma inhibits corneal fibrosis in vivo. PLoS One 8:e66434. doi:10.1371/journal.pone.0066434
Tchemtchoua, V. T., Atanasova, G., Aqil, A., Filee, P., Garbacki, N., Vanhooteghem, O., et al. (2011). Development of a chitosan nanofibrillar scaffold for skin repair and regeneration. Biomacromolecules 12, 3194–3204. doi:10.1021/bm200680q
Tenover, F. C. (2006). Mechanisms of antimicrobial resistance in bacteria. Am. J. Med. 119, S3–S10. doi:10.1016/j.amjmed.2006.03.011
Tian, J., Wong, K. K. Y., Ho, C.-M., Lok, C.-N., Yu, W.-Y., Che, C.-M., et al. (2007). Topical delivery of silver nanoparticles promotes wound healing. ChemMedChem 2, 129–136. doi:10.1002/cmdc.200600171
Travan, A., Pelillo, C., Donati, I., Marsich, E., Benincasa, M., Scarpa, T., et al. (2009). Non-cytotoxic silver nanoparticle-polysaccharide nanocomposites with antimicrobial activity. Biomacromolecules 10, 1429–1435. doi:10.1021/bm900039x
Trengove, N. J., Stacey, M. C., Macauley, S., Bennett, N., Gibson, J., Burslem, F., et al. (1999). Analysis of the acute and chronic wound environments: the role of proteases and their inhibitors. Wound Repair Regen. 7, 442–452. doi:10.1046/j.1524-475X.1999.00442.x
Trickler, W. J., Lantz, S. M., Murdock, R. C., Schrand, A. M., Robinson, B. L., Newport, G. D., et al. (2010). Silver nanoparticle induced blood-brain barrier inflammation and increased permeability in primary rat brain microvessel endothelial cells. Toxicol. Sci. 118, 160–170. doi:10.1093/toxsci/kfq244
Uckay, I., Aragon-Sanchez, J., Lew, D., and Lipsky, B. A. (2015). Diabetic foot infections: what have we learned in the last 30 years? Int. J. Infect. Dis. 40, 81–91. doi:10.1016/j.ijid.2015.09.023
Ulrich, M. W. (2014). Regenerative medicine in burn wound healing: aiming for the perfect skin. EWMA J. 14, 25.
Uzunalli, G., Soran, Z., Erkal, T. S., Dagdas, Y. S., Dinc, E., Hondur, A. M., et al. (2014). Bioactive self-assembled peptide nanofibers for corneal stroma regeneration. Acta Biomater. 10, 1156–1166. doi:10.1016/j.actbio.2013.12.002
Varner, K. E., El-Badawy, A., Feldhake, D., and Venkatapathy, R. (2010). State-of-the-Science Review: Everything Nanosilver and More. Washington, DC: U.S. Environmental Protection Agency.
Varshochian, R., Riazi-Esfahani, M., Jeddi-Tehrani, M., Mahmoudi, A. R., Aghazadeh, S., Mahbod, M., et al. (2015). Albuminated PLGA nanoparticles containing bevacizumab intended for ocular neovascularization treatment. J. Biomed. Mater. Res. A. 103, 3148–3156. doi:10.1002/jbm.a.35446
Ventola, C. L. (2012). The nanomedicine revolution: part 2: current and future clinical applications. P T 37, 582–591.
Veves, A., Falanga, V., Armstrong, D. G., Sabolinski, M. L., Apligraf Diabetic Foot Ulcer Study. (2001). Graftskin, a human skin equivalent, is effective in the management of noninfected neuropathic diabetic foot ulcers: a prospective randomized multicenter clinical trial. Diabetes Care 24, 290–295. doi:10.2337/diacare.24.2.290
Vidal, F., and Guzman, L. (2015). Dendrimer nanocarriers drug action: perspective for neuronal pharmacology. Neural Regen. Res. 10, 1029–1031. doi:10.4103/1673-5374.160063
Vignoni, M., De Alwis Weerasekera, H., Simpson, M. J., Phopase, J., Mah, T.-F., Griffith, M., et al. (2014). LL37 peptide@silver nanoparticles: combining the best of the two worlds for skin infection control. Nanoscale 6, 5725–5728. doi:10.1039/c4nr01284d
Wahl, S. M. (1999). TGF-beta in the evolution and resolution of inflammatory and immune processes. Introduction. Microbes Infect. 1, 1247–1249. doi:10.1016/S1286-4579(99)00261-0
Wang, L., Cao, L., Shansky, J., Wang, Z., Mooney, D., and Vandenburgh, H. (2014a). Minimally invasive approach to the repair of injured skeletal muscle with a shape-memory scaffold. Mol. Ther. 22, 1441–1449. doi:10.1038/mt.2014.78
Wang, W., Despanie, J., Shi, P., Edman-Woolcott, M. C., Lin, Y. A., Cui, H., et al. (2014b). Lacritin-mediated regeneration of the corneal epithelia by protein polymer nanoparticles. J. Mater. Chem. B Mater. Biol. Med. 2, 8131–8141.
Walkey, C., Das, S., Seal, S., Erlichman, J., Heckman, K., Ghibelli, L., et al. (2015). Catalytic properties and biomedical applications of cerium oxide nanoparticles. Environ. Sci. Nano 2, 33–53. doi:10.1039/C4EN00138A
Wolf, M. T., Dearth, C. L., Sonnenberg, S. B., Loboa, E. G., and Badylak, S. F. (2015). Naturally derived and synthetic scaffolds for skeletal muscle reconstruction. Adv. Drug Deliv. Rev. 84, 208–221. doi:10.1016/j.addr.2014.08.011
Wong, K. K. Y., Cheung, S. O. F., Huang, L., Niu, J., Tao, C., Ho, C. M., et al. (2009). Further evidence of the anti-inflammatory effects of silver nanoparticles. ChemMedChem 4, 1129–1135. doi:10.1002/cmdc.200900049
Wong, L. L., Pye, Q. N., Chen, L., Seal, S., and McGinnis, J. F. (2015). Defining the catalytic activity of nanoceria in the P23H-1 rat, a photoreceptor degeneration model. PLoS ONE 10:e0121977. doi:10.1371/journal.pone.0121977
Wright, J. B., Lam, K., Buret, A. G., Olson, M. E., and Burrell, R. E. (2002). Early healing events in a porcine model of contaminated wounds: effects of nanocrystalline silver on matrix metalloproteinases, cell apoptosis, and healing. Wound Repair Regen. 10, 141–151. doi:10.1046/j.1524-475X.2002.10308.x
Wu, W., Allen, R. A., and Wang, Y. (2012). Fast-degrading elastomer enables rapid remodeling of a cell-free synthetic graft into a neoartery. Nat. Med. 18, 1148–1153. doi:10.1038/nm.2821
Xu, H., Lv, F., Zhang, Y., Yi, Z., Ke, Q., Wu, C., et al. (2015). Hierarchically micro-patterned nanofibrous scaffolds with a nanosized bio-glass surface for accelerating wound healing. Nanoscale 7, 18446–18452. doi:10.1039/c5nr04802h
Yager, D. R., Zhang, L. Y., Liang, H. X., Diegelmann, R. F., and Cohen, I. K. (1996). Wound fluids from human pressure ulcers contain elevated matrix metalloproteinase levels and activity compared to surgical wound fluids. J. Invest. Dermatol. 107, 743–748. doi:10.1111/1523-1747.ep12365637
Yilmaz, A., Rösch, S., Klingel, K., Kandolf, R., Helluy, X., Hiller, K.-H., et al. (2013). Magnetic resonance imaging (MRI) of inflamed myocardium using iron oxide nanoparticles in patients with acute myocardial infarction – preliminary results. Int. J. Cardiol. 163, 175–182. doi:10.1016/j.ijcard.2011.06.004
Yilmaz, A., Rösch, S., Yildiz, H., Klumpp, S., and Sechtem, U. (2012). First multiparametric cardiovascular magnetic resonance study using ultrasmall superparamagnetic iron oxide nanoparticles in a patient with acute myocardial infarction new vistas for the clinical application of ultrasmall superparamagnetic iron oxide. Circulation 126, 1932–1934. doi:10.1161/CIRCULATIONAHA.112.108167
Yin, H. Q., Langford, R., and Burrell, R. E. (1999). Comparative evaluation of the antimicrobial activity of ACTICOAT antimicrobial barrier dressing. J. Burn Care Rehabil. 20, 195–200. doi:10.1097/00004630-199905000-00006
Zarbin, M. A., Arlow, T., and Ritch, R. (2013). Regenerative nanomedicine for vision restoration. Mayo Clin. Proc. 88, 1480–1490. doi:10.1016/j.mayocp.2013.05.025
Zelen, C. M., Serena, T. E., Denoziere, G., and Fetterolf, D. E. (2013). A prospective randomised comparative parallel study of amniotic membrane wound graft in the management of diabetic foot ulcers. Int. Wound J. 10, 502–507. doi:10.1111/iwj.12097
Zhan, X., Gao, M., Jiang, Y., Zhang, W., Wong, W. M., Yuan, Q., et al. (2013). Nanofiber scaffolds facilitate functional regeneration of peripheral nerve injury. Nanomedicine 9, 305–315. doi:10.1016/j.nano.2012.08.009
Zhang, H., Shih, J., Zhu, J., and Kotov, N. A. (2012). Layered nanocomposites from gold nanoparticles for neural prosthetic devices. Nano Lett. 12, 3391–3398. doi:10.1021/nl3015632
Zhang, S. (2003). Fabrication of novel biomaterials through molecular self-assembly. Nat. Biotechnol. 21, 1171–1178. doi:10.1038/nbt874
Zhang, S., Liu, X., Wang, H., Peng, J., and Wong, K. K. Y. (2014). Silver nanoparticle-coated suture effectively reduces inflammation and improves mechanical strength at intestinal anastomosis in mice. J. Pediatr. Surg. 49, 606–613. doi:10.1016/j.jpedsurg.2013.12.012
Zhao, W., Ju, Y. M., Christ, G., Atala, A., Yoo, J. J., and Lee, S. J. (2013). Diaphragmatic muscle reconstruction with an aligned electrospun poly(epsilon-caprolactone)/collagen hybrid scaffold. Biomaterials 34, 8235–8240. doi:10.1016/j.biomaterials.2013.07.057
Zhou, J., Chen, J., Sun, H., Qiu, X., Mou, Y., Liu, Z., et al. (2014). Engineering the heart: evaluation of conductive nanomaterials for improving implant integration and cardiac function. Sci. Rep. 4, 3733. doi:10.1038/srep03733
Keywords: nanomaterials, tissue regeneration, regenerative therapies
Citation: Mclaughlin S, Podrebarac J, Ruel M, Suuronen EJ, McNeill B and Alarcon EI (2016) Nano-Engineered Biomaterials for Tissue Regeneration: What Has Been Achieved So Far? Front. Mater. 3:27. doi: 10.3389/fmats.2016.00027
Received: 16 April 2016; Accepted: 09 June 2016;
Published: 27 June 2016
Edited by:
Aldo Ferrari, ETH Zürich – Eidgenössische Technische Hochschule Zürich, SwitzerlandReviewed by:
Hirak Kumar Patra, Linköping University, SwedenAhmed El-Fiqi, Dankook University, South Korea
Copyright: © 2016 Mclaughlin, Podrebarac, Ruel, Suuronen, McNeill and Alarcon. This is an open-access article distributed under the terms of the Creative Commons Attribution License (CC BY). The use, distribution or reproduction in other forums is permitted, provided the original author(s) or licensor are credited and that the original publication in this journal is cited, in accordance with accepted academic practice. No use, distribution or reproduction is permitted which does not comply with these terms.
*Correspondence: Erik J. Suuronen, ZXN1dXJvbmVuQG90dGF3YS5jYQ==;
Emilio I. Alarcon, ZWFsYXJjb25Ab3R0YXdhaGVhcnQuY2E=