- 1Instituto de Oceanografía y Cambio Global (IOCAG), Universidad de Las Palmas de Gran Canaria, Unidad Asociada, Universidad de Las Palmas de Gran Canaria-Consejo Superior de Investigaciones Científicas (ULPGC-CSIC), Telde, Spain
- 2Centro Oceanográfico de A Coruña, Instituto Español de Oceanografía (IEO), A Coruña, Spain
Euphausiids, commonly known as krill, are crucial contributors to the ocean’s active carbon pump, impacting carbon export and sequestration through their diel vertical migration. These organisms feed on organic matter in the epipelagic layer at night and release inorganic carbon in the mesopelagic layer during the day via respiration. Measuring respiration in the mesopelagic layer is challenging due to the difficulties in obtaining direct measurements, as well as the lack of comprehensive data, and reliance on conservative estimates. The measurement of the electron transfer system (ETS) activity is used as a proxy to assess respiration in the mesopelagic layer. However, accurate calibration of respiration rates and ETS activity is imperative through experimental measurements and empirical data. Here, we compared the respiration rates with their respective ETS activities of different species of euphausiids captured at night in the epipelagic layer of the Atlantic Ocean along a latitudinal (42-29°N, 25°W) and a longitudinal (25-13°W, 29°N) transect. Our results revealed a spatial trend in respiration rates, and consequently in ETS activities, with rates decreasing southward and increasing slightly towards the African upwelling region. The Generalized Additive Model (GAM) demonstrated that epipelagic oxygen concentration, chlorophyll a, and the interaction between epipelagic temperature and mesopelagic oxygen concentration significantly influenced euphausiids respiration rates. Furthermore, we observed a strong correlation between respiration and specific ETS activities, with R/ETS ratios exceeding the conservative value of 0.5, which is typically used to estimate respiratory flux.
1 Introduction
Zooplankton migrant organisms, such as large copepods and euphausiids (Hernández-León et al., 2019a), play a crucial role in the biological carbon pump by actively exporting carbon. This process is known as the active flux or migrant pump and refers to the active transport of organic matter by zooplankton and micronekton to the deepest areas of the ocean (Longhurst and Harrison, 1988). These organisms consume organic carbon at shallower depths and transport it to the mesopelagic layer through diel vertical migrations, where it is released through respiration (Longhurst et al., 1990), egestion (Angel, 1989), excretion (Steinberg et al., 2000), and mortality (Zhang and Dam, 1997). Zooplankton mortality, excretion, and feeding can be estimated from respiration rates (Ikeda and Motoda, 1978; Steinberg et al., 2000; Ariza et al., 2015). Therefore, respiration rates are of importance to assess the role of these organisms in exporting carbon to the mesopelagic layer.
Euphausiids are significant components of marine ecosystems, conducting vertical and horizontal migrations (Ens et al., 2023) that contribute to nutrient transfer and energy flux in the ocean. These organisms are found throughout the world’s oceans, from coastal seas to the bathypelagic zone, with their distribution influenced by thermal characteristics and water masses (Letessier et al., 2011; Sutton and Beckley, 2022). Furthermore, they are a major contributor to global plankton community biomass (21%), trailing only behind copepods (47%), in terms of their total organic carbon (Longhurst, 1985). Euphausiids are pivotal in driving the biological pump, contributing significantly to the export and sequestration (sensu Lampitt et al., 2008) of atmospheric CO2 into the deep ocean through fast-sinking faecal pellets (Cavan et al., 2019), respiration at depth (Stukel et al., 2023), and moulting (Kobari et al., 2010). Thus, they play a key role in the biological carbon pump and the cycle of essential nutrients in marine ecosystems.
Respiratory flux is estimated from the migrant biomass (night minus day biomass values in the epipelagic zone) and the respiration rates at the residence layer during daytime. These rates are determined by measuring migrant organisms captured during the night in the epipelagic zone and converting them to represent respiration at depth using empirical or published Q10 values (Le Borgne and Rodier, 1997). Alternatively, values from published equations relating respiration, body size, and temperature (Ikeda, 2013a, for euphausiids) are also used. Enzymatic activities related to cell respiration, such as the electron transfer system (ETS) activity, serve as an alternative proxy for estimating respiratory flux. This method offers advantages as samples can be frozen and stored until they are analysed. However, it requires the calibration of enzymatic proxies to physiological rates (Hernández-León et al., 2019b). The respiration to ETS (R/ETS) ratio in marine zooplankton reflects the scope of metabolic activity and typically ranges between 0.5 and 1 mainly depending on factors such as food availability and temperature (Hernández-León and Gómez, 1996). While conservative ratios of 0.5 are commonly used to assess the respiratory flux, Hernández-León et al. (2019b) observed a R/ETS value of 0.96 ± 0.29 for migrant copepods. This result suggests the need for a reassessment of this ratio to accurately evaluate respiratory fluxes and the carbon transported to the deep ocean by migrant organisms.
In this context, we performed respiration measurements on euphausiids captured at night in the epipelagic layer. We then analyzed their specific ETS activity and correlated both sets of measurements to obtain the R/ETS ratio. The aim of the present study was to compare the in situ respiration rates with the specific ETS activities, aiming to derive accurate R/ETS ratios for euphausiids in a longitudinal and latitudinal transect in the North Atlantic Ocean.
2 Materials and methods
2.1 Sampling and on-board experiments
The euphausiids used for experiments were collected during the “DisEntangling Seasonality of Active Flux In the Ocean” (DESAFIO I) cruise on board the R.V. “Sarmiento de Gamboa” during February 2023. The research vessel sailed from Vigo (Spain) to the Canary Islands (Spain), spanning from January 31st to March 2nd, 2023 (Figure 1), sampling eleven oceanographic stations (thereafter ST). Vertical profiles of salinity, temperature, oxygen, conductivity, and fluorescence were obtained using a CTD (Seabird 911 plus) and a Seapoint fluorimeter mounted on a rosette sampler equipped with 12 l Niskin bottles. Euphausiids were captured at night using a Bongo plankton net fitted with a 200 μm mesh and a non-filtering cod-end deployed in horizontal hauls between 0 and 50 m depth. From the plankton sample, euphausiids were sorted and those undamaged with active swimming behavior were selected for the experiments. One single euphausiid was introduced into each experimental bottle (0.5L), which was filled with filtered (0.2 μm Whatman ® Polycap TC encapsulated filter) and oxygenated surface sea water. Oxygen consumption measurements were carried out using two 4-channel FireSting-O2 meter, using 3 channels for the experimental organisms and one for the background respiration (without organism). Oxygen contactless sensors (Pyroscience, OXSP5) were attached to the experimental bottles for contactless oxygen read-out. Incubations were conducted placing the experimental bottles inside thermoregulated baths (15.5 ± 0.5°C) in darkness. Total respiration rates (μl O2·ind-1·h-1) were estimated as the regression slopes of oxygen concentration over time, subtracting background respiration. The first thirty minutes were discarded due to oxygen stabilization inside the bottles and the experiment ranged between 6.5 and 9 hours according to the oxygen concentration in the experimental bottles, that normally decreased from 100% to 85–90% of oxygen saturation.
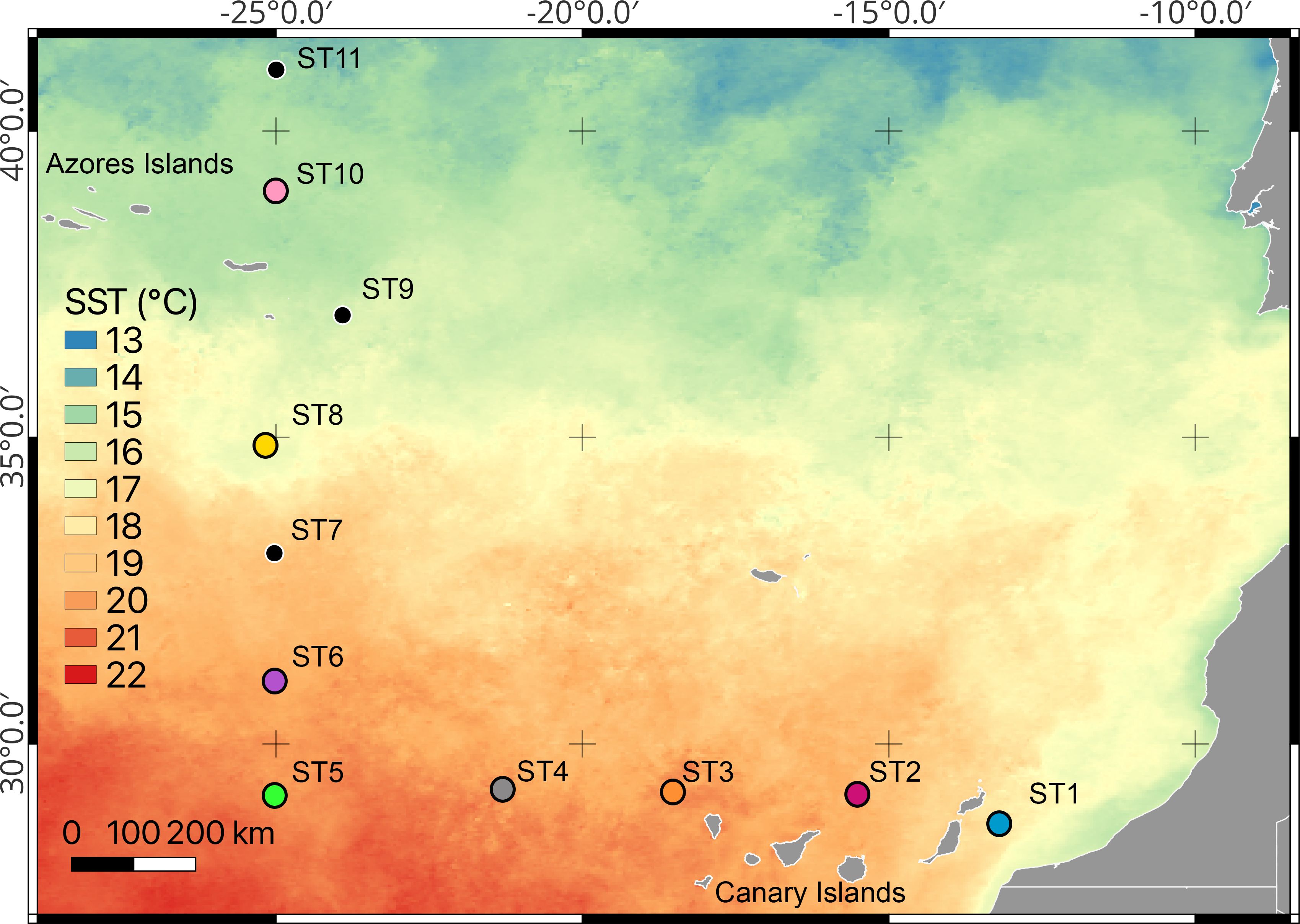
Figure 1. Locations of sampling stations during the DESAFIO 1 cruise conducted in February 2023. The background colours depict the monthly average sea surface temperature (SST, °C). The coloured dots indicate the specific stations where experiments were carried out.
After the respiration assays, euphausiids were picked and frozen in liquid nitrogen (-196°C) for later analysis. In the laboratory, before the ETS analysis, euphausiids were classified to species level (or genus when further classification was not possible), photographed and digitized using a Nikon digital camera.
2.2 Specific ETS activity and protein content
Electron transfer system (ETS) activity was measured following the method of Packard (1971) modified by Owens and King (1975), Kenner and Ahmed (1975), and Gómez et al. (1996). Frozen samples were homogenized at the laboratory in a Teflon pestle at 0-4°C to avoid degradation of enzyme activity and proteins. Then, the homogenates were centrifuged at 4000 rpm at 4°C for 10 min. An aliquot was subsampled from the homogenate, incubated at 18°C in darkness using NADH, NADPH, succinate, and a tetrazolium salt (INT) as the artificial electron acceptor. After 20 min, the incubation was stopped with a quench solution. The ETS activity was estimated spectrophotometrically at 490 nm with a turbidity baseline of 750 nm. In order to correct ETS activity for in situ temperature, we used the Arrhenius equation and an activation energy of 15 kcal·mol-1 (King and Packard, 1975). Protein content was determined using the method of Lowry et al. (1951) modified by Rutter (1967), and using bovine serum albumin (BSA) as the standard. Finally, protein specific respiration rates (μl O2·prot-1·h-1) were estimated to compared with the specific ETS activities (µl O2·mg prot-1·h-1) and to estimate the R/ETS ratios.
2.3 Estimation of respiration rates and ETS activity in the epipelagic and mesopelagic layer
ETS activity of organisms incubated for respiration experiments and captured in the 0-50 m depth layer were converted to ETS activities in the epipelagic (0-200 m) and mesopelagic (200-1000 m) layers using the Arrhenius equation and the temperature of each layer.
Respiration rates in the upper 50 m were directly measured, while rates for the epipelagic (0-200 m) and mesopelagic (200-1000 m) layers were estimated based on the measured rates from the 0-50 m depth using a Q10 value of 3 (Hernández-León et al., 2019b), which represents the factor by which the respiration rate increases for every 10°C rise in temperature, and the temperature (T) at each layer:
Both the Q10 value and the Arrhenius equation are used to describe the temperature dependence of biological and chemical processes, but they are applied differently based on the nature of the processes they describe and the assumptions behind each model. The Q10 value is commonly used in biological sciences to describe the temperature sensitivity of metabolic processes, including respiration, as it provides a simple and empirical measure of how these rates change with temperature (Mundim et al., 2020). In contrast, the Arrhenius equation is based on the fundamental principles of chemical kinetics and provides insight into the energy barriers that must be overcome for electron transfer reactions to occur, making it directly related to the molecular and energetic properties of the enzymes and substrates involved (Owens and King, 1975).
2.4 Data analysis
The relationships between specific respiration rates and specific ETS activities, and the relationships between protein content and respiration rates were fitted using linear regression. Because of the general trend that metabolism is a power function of body weight, the values were log-transformed. Pearson’s correlation was used to evaluate the relationship between euphausiids respiration rates, ETS activities, and R/ETS ratios against temperature, oxygen concentration, chlorophyll a values, and primary production. Differences in respiration rates between stations were tested using the analysis of the variance (one-way ANOVA). Respiration rates were transformed using a Box-Cox transformation to adjust normality. Normality was tested using the Shapiro-Wilk Test and homoscedasticity using the Bartlett test. Finally, a Generalized Additive Model (GAM) was applied to model the relationship between the response variable (respiration rates measured from euphausiids captured at night in the epipelagic layer) and predictors (individual protein content and environmental conditions in the epipelagic and mesopelagic layer). All analyses were performed in the programming language R (R Core Team, 2024). The map of the sampling region was created using the geographic information system QGIS (V.3.38.2) (QGIS Development Team, 2024).
3 Results
In the latitudinal transect, temperature values (Figure 2A) increased from ST11 to ST5 in the epipelagic layer (0-200 m depth), while in the longitudinal transect (Figure 2B) temperature was similar in all stations but slightly decreasing close to the African coast (i.e., ST1 and ST2). Oxygen vertical profiles (Figure 2C) showed higher oxygen concentration in the epipelagic layer of the latitudinal transect, specially from ST11 to ST8. In the longitudinal transect (Figure 2D), oxygen vertical profiles in the epipelagic layer showed an increase from the open ocean to the coast. On the other hand, mesopelagic oxygen concentration increased from the coast to the open ocean (from ST1 to ST6), reaching the highest values from ST11 to ST8. Vertical profiles of chlorophyll a (Chl a) in the upper 200 m layer showed higher values at the northern stations of the latitudinal transect (Figure 2E) and at the stations close to the African coast (Figure 2F).
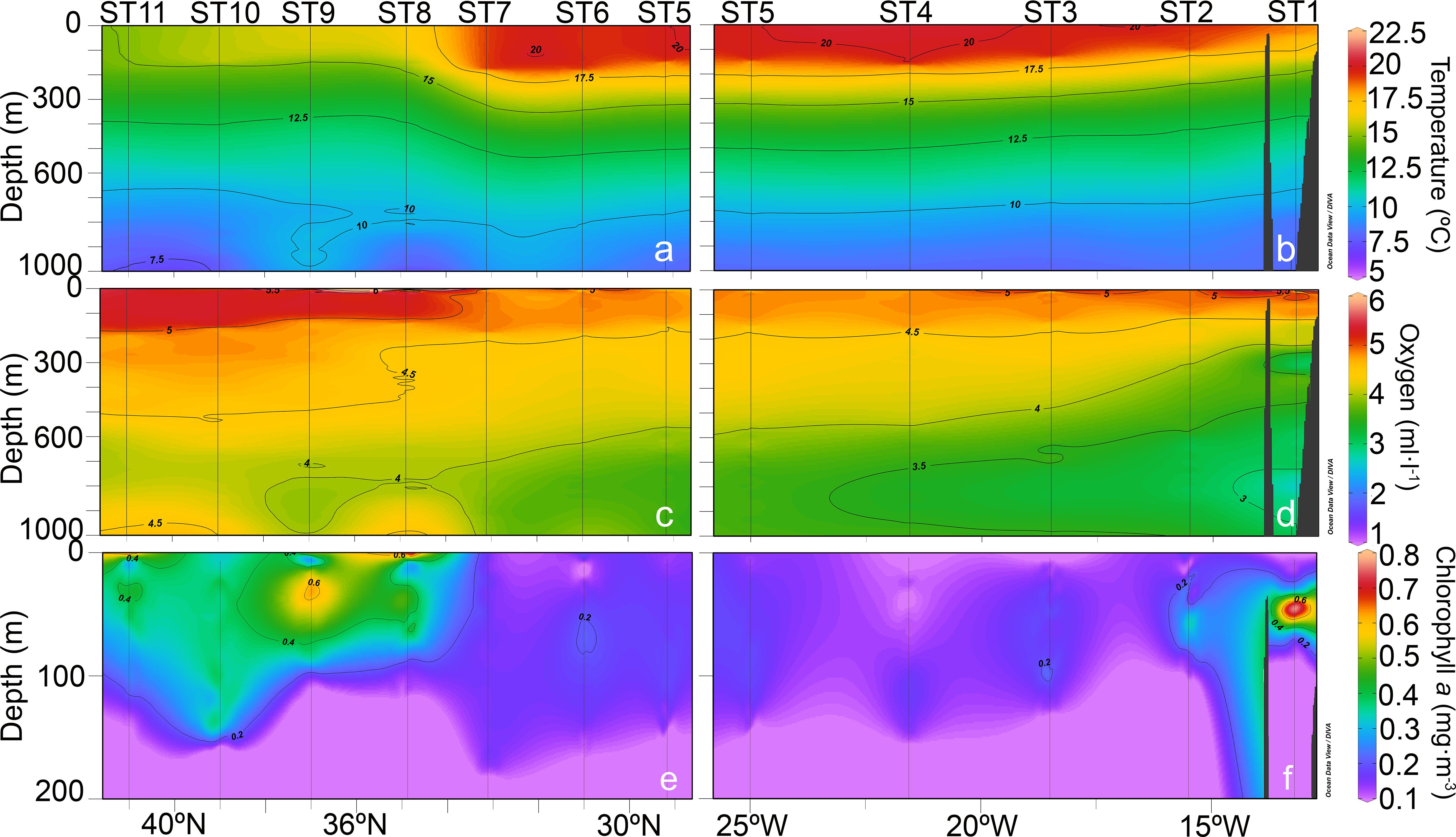
Figure 2. Vertical distribution of (A, B) temperature (°C), (C, D) oxygen (ml·L−¹), and (E, F) chlorophyll a (mg·m−³) along the latitudinal (left panel) and longitudinal (right panel) transects sampled during the DESAFIO 1 cruise. Note the different y-axes for the chlorophyll a vertical profiles.
Respiration rates measured in euphausiids captured in the first 0-50 m of the water column ranged from 19.65 ± 9.91 μl O2·mg prot-1·h-1 at ST10 to 4.64 ± 2.11 μl O2·mg prot-1·h-1 at ST3 (Table 1), displaying higher values at those stations where higher values of oxygen and Chl a were found (i.e. from ST10 to ST6). Respiration rates measured in the upper 50 m depth were similar to the rates estimated for the epipelagic layer (0-200 m depth) using the measured ETS and applying a Q10 value of 3 (Figure 3A). In contrast, the estimated respiration rates for the mesopelagic layer (200-1000 m depth) were lower as expected (Figure 3A; Table 1). Specific ETS activity, estimated using the Arrhenius equation as mentioned above (Figure 3B), showed a similar pattern as the respiration rates, with higher values at ST10 decreasing along the latitudinal transect, and slightly increasing close to the African upwelling (ST1). R/ETS ratios in the upper 0-50 m depth ranged from 1.19 ± 0.49 at ST6 to 0.65 ± 0.12 at ST3 in the 0-50 m depth layer (Table 1; Figure 3C), displaying similar values as the R/ETS obtained for the epipelagic layer (ranging from 1.18 ± 0.34 to 0.64 ± 0.12, respectively) and slightly higher than those obtained at depth (ranging from 1.05 ± 0.3 to 0.56 ± 0.11, respectively) (Table 1). Estimated respiration for the epipelagic layer (0-200 m depth) showed a significant relationship with epipelagic specific ETS values (R2 = 0.73, p<0.001, n= 66, see Figure 4) and mesopelagic specific ETS values (R2 = 0.76, p<0.001, n= 66). Total respiration rates (Figure 5A) showed a positive relationship with body weight as protein content, while specific respiration rate (μl O2·mg prot-1·h-1) significantly decreased with the increasing body weight as expected (Figure 5B).
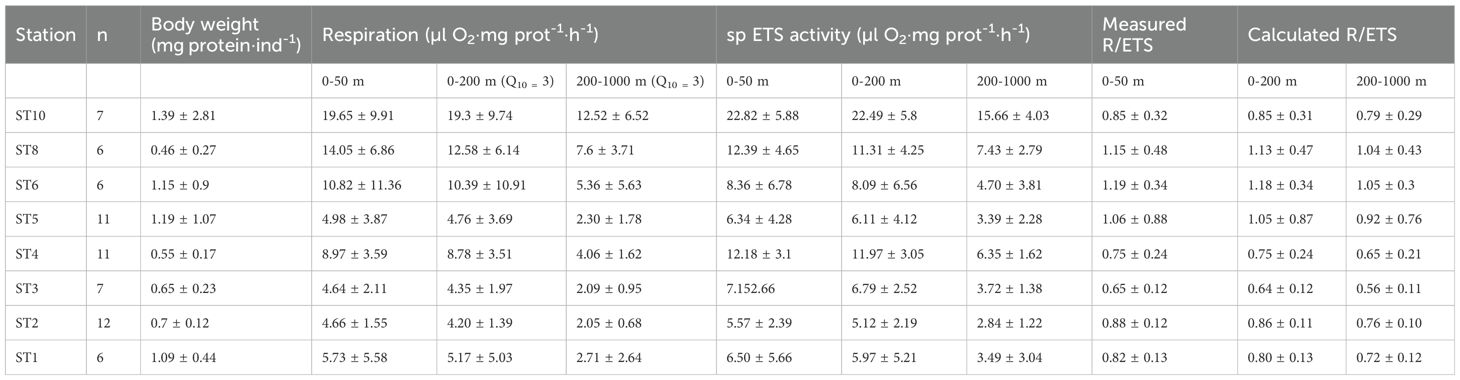
Table 1. Euphausiid biomass (mg protein·ind-1), respiration rates (µl O2·mg prot-1·h-1), specific (sp) ETS activities (µl O2·mg prot-1·h-1), and R/ETS ratios measured in the 0- 50 m depth layer, estimated for the epipelagic (0-200 m depth) and mesopelagic (200-1000 m depth) layers.
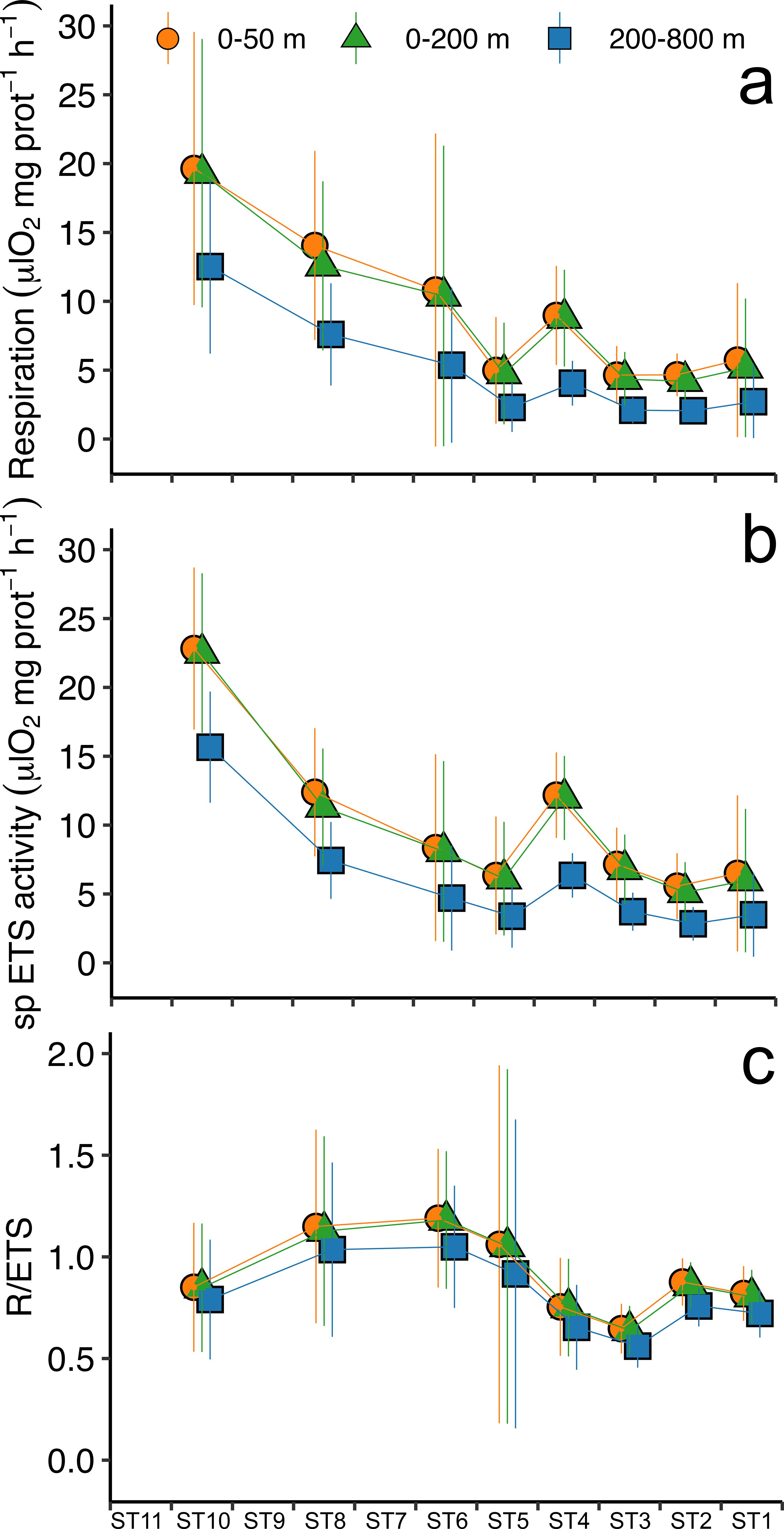
Figure 3. (A) Respiration rates (μl O2·mg prot-1·h-1), (B) protein normalized ETS activity (μl O2·mg prot-1·h-1), and (C) R/ETS ratios of migrant euphausiids obtained from incubations conducted along the oceanographic stations in the Atlantic transect. Measurements were estimated at surface temperature (orange), for the epipelagic layer (green), and for the mesopelagic temperature (blue) at each station (see text). Vertical lines indicate the standard error.
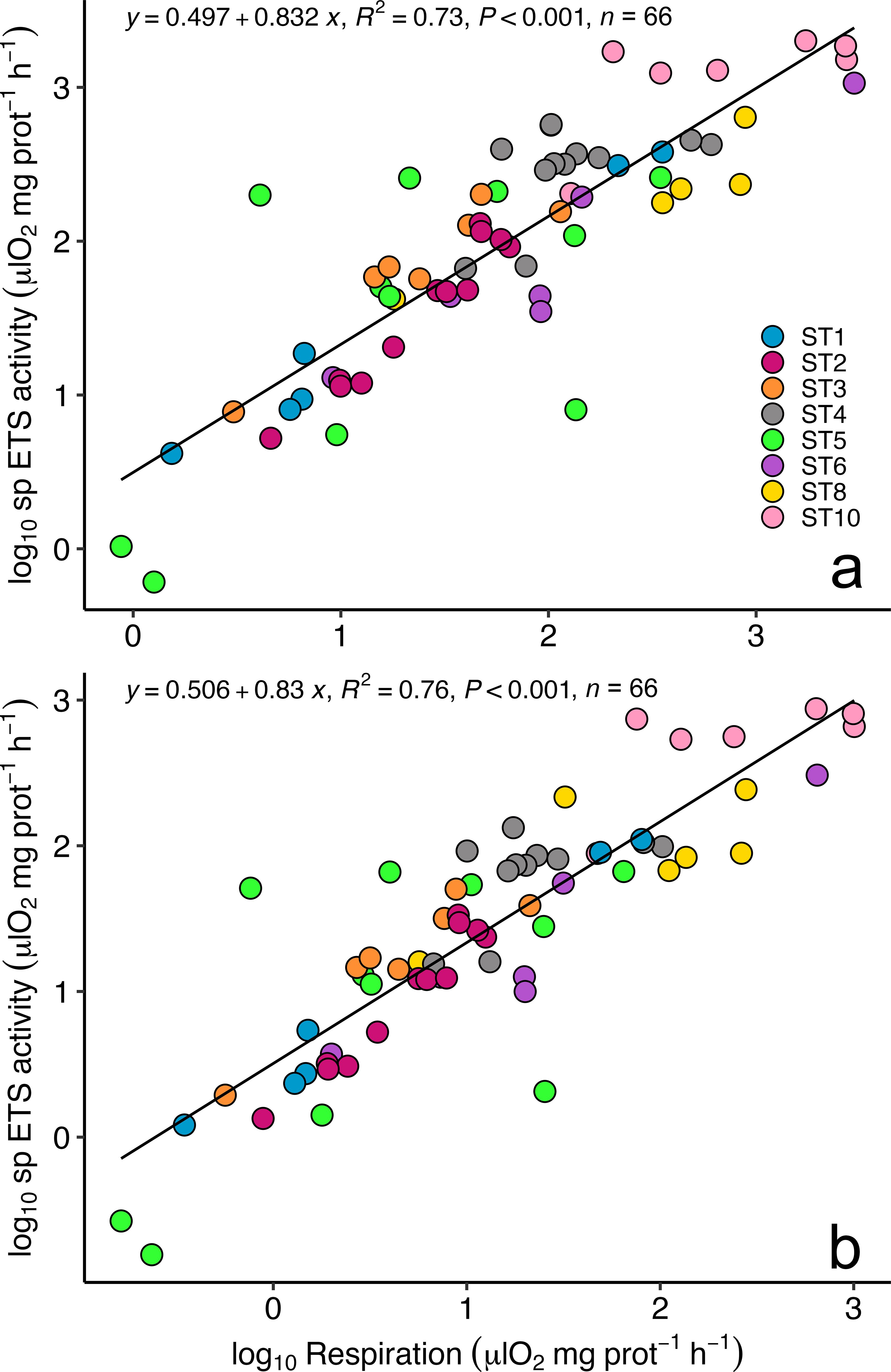
Figure 4. Relationship between euphausiid respiration rates and specific (sp) ETS activity estimated for the (A) epipelagic (0-200 m depth) and the (B) mesopelagic layer (200-1000 m depth). See text for explanation.
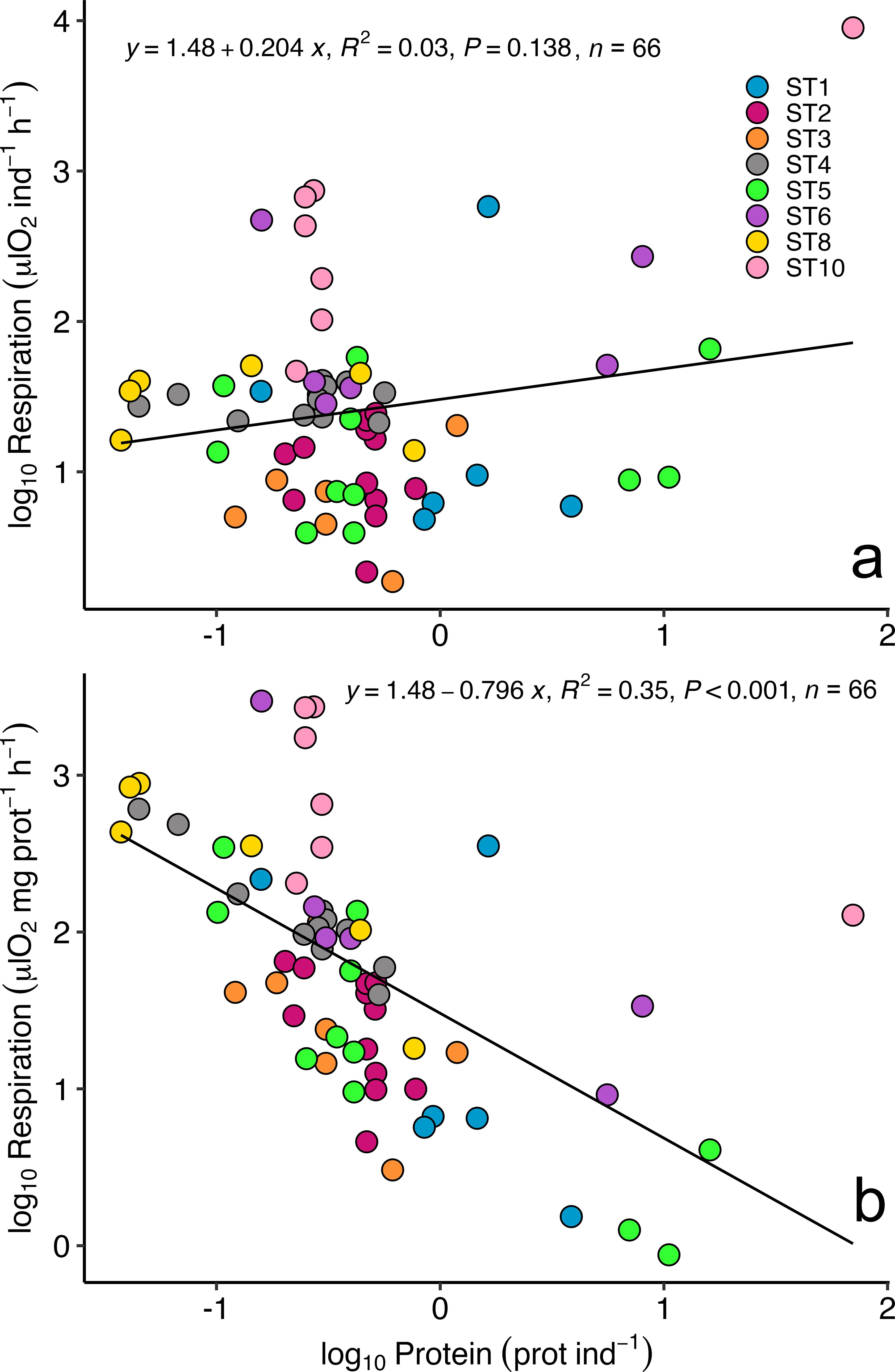
Figure 5. Relationship between euphausiid body weight (as protein content) and (A) total respiration rates (μl O2·ind-1·h-1) and (B) respiration rate (μl O2·mg prot-1·h-1) in the epipelagic layer (0-200 m depth).
Pearson’s correlation showed a significant negative correlation between the averaged epipelagic temperature and the respiration rates estimated for the epipelagic (r=-0.7, p<0.05) and for the mesopelagic layer (r=-0.77, p<0.05) (Figure 6A; Supplementary Table 2). Moreover, specific ETS activity estimated for the mesopelagic layer also showed a significant negative correlation with the epipelagic temperature (r=-0.7, p<0.05) (Figure 6B; Supplementary Table 2). However, no significant correlations were found between the epipelagic temperature and the epipelagic specific ETS activity nor the R/ETS ratios (Figures 6B, C; Supplementary Table 2). On the other hand, a strong significant positive correlation was found between the epipelagic oxygen concentration (ml·L-1) (Figure 6 upper panel) and the estimated respiration rates (μl O2·mg prot-1·h-1), both for the epipelagic (r=0.95, p<0.001) and for the mesopelagic (r=0.96, p<0.001) (Figure 7A; Supplementary Table 2) layers, as well as between the epipelagic oxygen concentration and the specific ETS activity, also for the epipelagic (r=0.94, p<0.001) and for the mesopelagic (r=0.96, p<0.001) (Figure 7B; Supplementary Table 2) layers. Likewise, mesopelagic oxygen concentration (Figure 7 lower panel) also showed a strong significant positive correlation between the estimated respiration rates, both for the epipelagic (r=0.78, p<0.05) and for the mesopelagic (r=0.77, p<0.05) (Figure 7D; Supplementary Table 2) layer, and with the ETS activity for the epipelagic (r=0.71, p<0.05) and mesopelagic (r=0.72, p<0.05) (Figure 7E; Supplementary Table 2) layers. However, no significant correlations were found between the epipelagic and mesopelagic oxygen concentration and the R/ETS ratios (Figures 7C, F; Supplementary Table 2). Finally, average Chl a (Figure 8 upper panel) and net primary production (Figure 8 lower panel) obtained in the epipelagic layer did not show any correlation between the respiration rates, the ETS activities, nor the R/ETS ratios (Figures 8C, F; Supplementary Table 2).
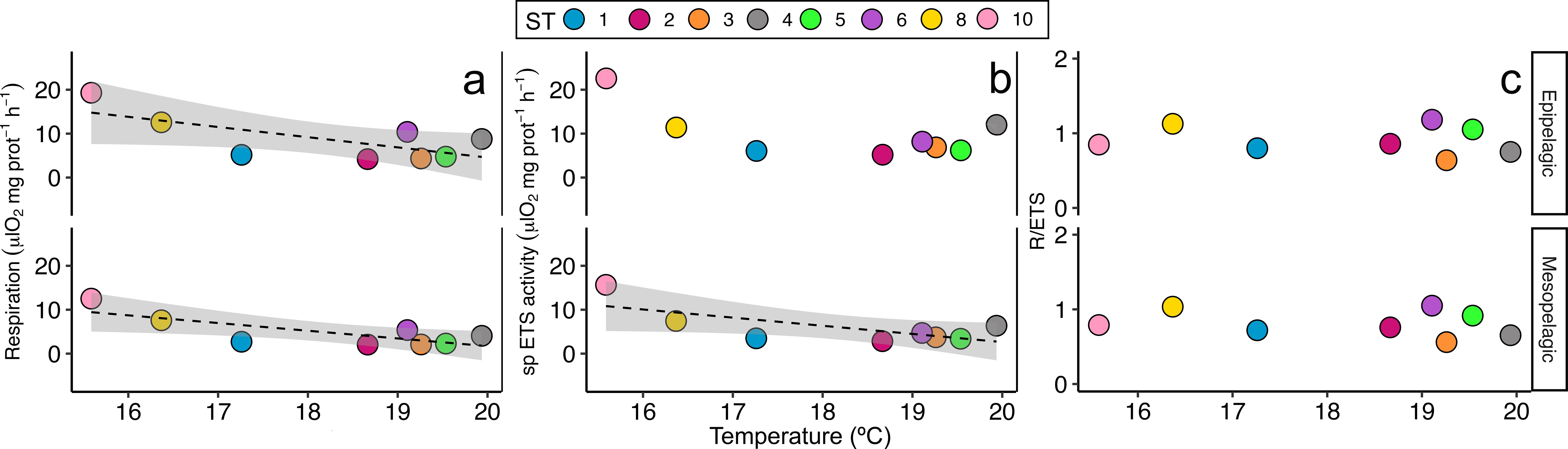
Figure 6. Pearson’s correlation between temperature (°C) in the epipelagic layer and average (A) respiration (μl O2·mg prot-1·h-1), (B) specific (sp) ETS activity (μl O2·mg prot-1·h-1), and (C) the R/ETS ratio in the epipelagic and mesopelagic layers per station. Only significant correlations are indicated with a line and standard deviation. Colours and numbers represent the stations.
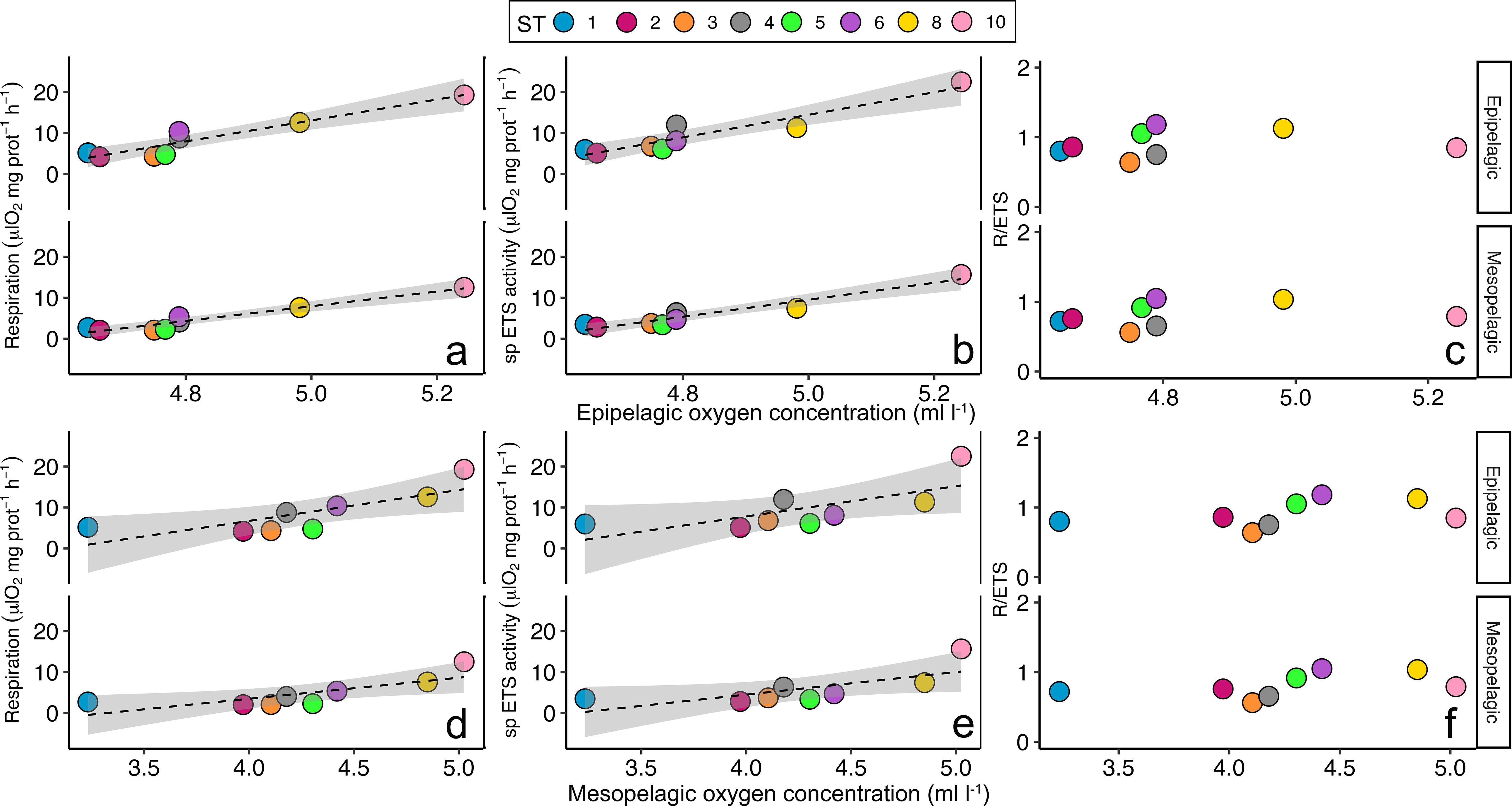
Figure 7. Pearson’s correlation between oxygen (ml·l-1) in the epipelagic (upper panel) and mesopelagic (lower panel) layers and average (A, D) respiration (μl O2·mg prot-1·h-1), (B, E) specific (sp) ETS activity (μl O2·mg prot-1·h-1), and (C, F) the R/ETS ratio in the epipelagic and mesopelagic layers per station. Only significant correlations are indicated with a line and standard deviation. Colours and numbers represent the stations.
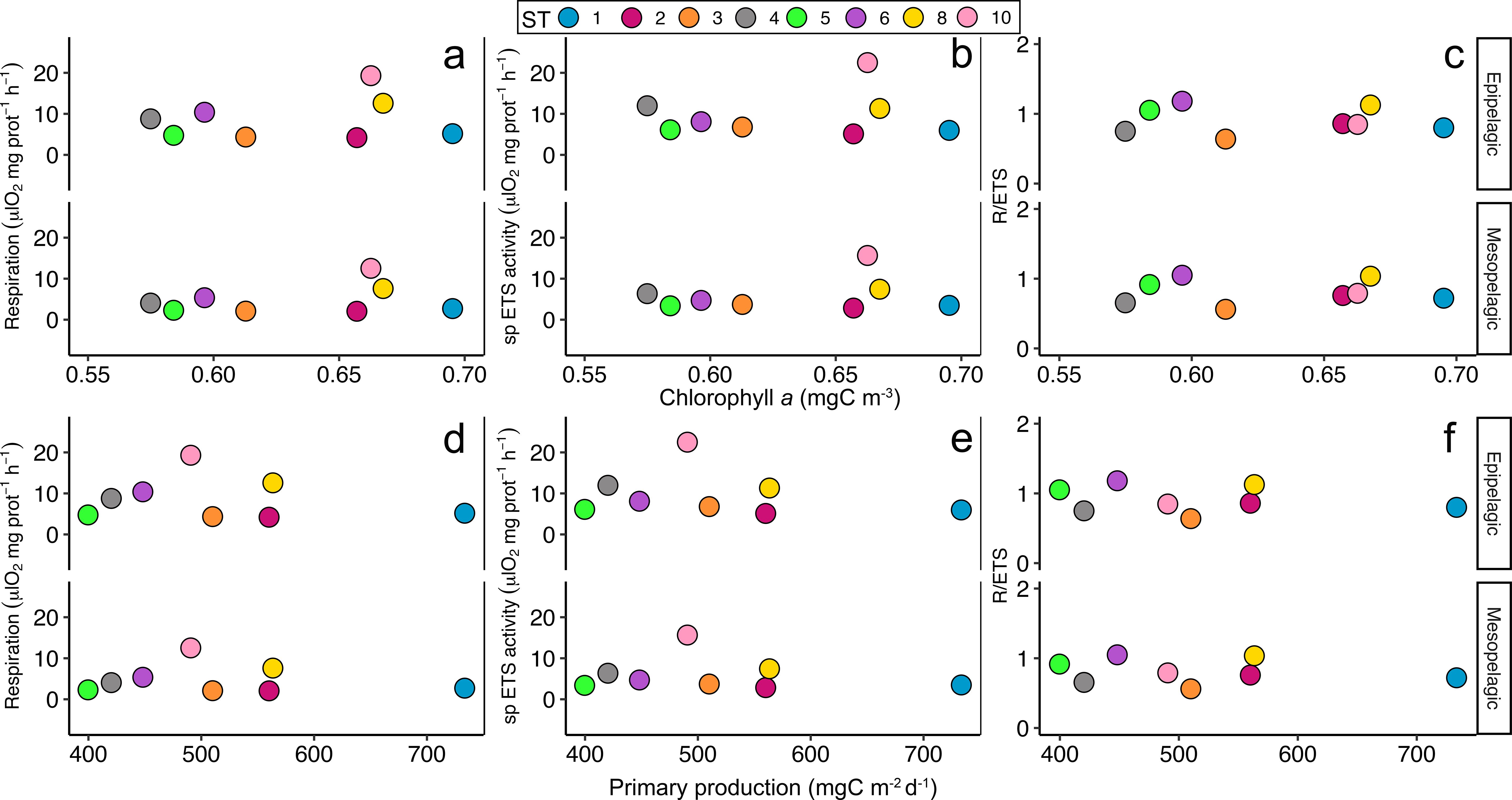
Figure 8. Upper panel: Pearson’s correlation between chlorophyll a (mg·m-3) in the epipelagic layer and average (A) respiration (μl O2·mg prot-1·h-1), (B) specific (sp) ETS activity (μl O2·mg prot-1·h-1), and (C) the R/ETS ratio in the epipelagic and mesopelagic layers per station. Lower panel: Pearson’s correlation between net primary production (mg C·m-2·d-1) in the epipelagic layer and average (D) respiration (μl O2·mg prot-1·h-1), (E) specific (sp) ETS activity (μl O2·mg prot-1·h-1), and (F) the R/ETS ratio in the epipelagic and mesopelagic layers per station. Only significant correlations are indicated with a line and standard deviation. Colours and numbers represent the stations.
The ANOVA results showed significant differences between stations (F value = 6.55, p<0.001, df=7), thus we modeled the variability of the respiration rates in the epipelagic layer between stations according to the environmental variables in the epipelagic and mesopelagic layers using a GAM, as mentioned above. Estimated coefficients from the best-fitting GAM based on different combinations of explanatory variables for the response variables are summarized in Table 2. The best-fitting GAM was selected using the Akaike information criterium (AIC, see Akaike, 1974). The optimal model included the effect of body weight, oxygen, and chlorophyll a in the epipelagic layer, and the interaction of temperature in the epipelagic layer and the oxygen concentration in the mesopelagic layer:
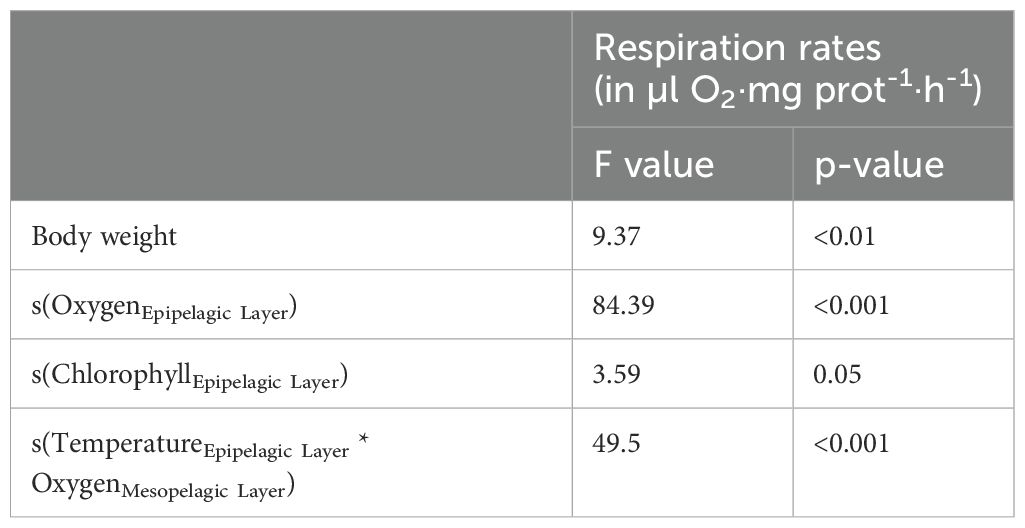
Table 2. Estimated coefficients for selected variables from the best-fitting Generalized Additive Model (GAM) for the response variables.
where g() stands for the function of the response variable (Box-Cox transformed, here respiration rates) and s() denotes the smoother functions.
4 Discussion
Euphausiid respiration rates, specific ETS activity, and R/ETS were estimated along a latitudinal and a longitudinal transect in the North Atlantic Ocean. To our knowledge, this is the first attempt to calibrate respiration rates and ETS activity through experimental measurements in euphausiids. We observed higher R/ETS ratios than the conservative value of 0.5 commonly used to assess the respiratory flux (Table 1) and found a close relationship between respiration rates and specific ETS activity (Figure 4), with rates highly influenced by the oxygen concentration (Figure 7).
Respiration rates in euphausiids are influenced by various environmental factors, such as temperature, body size, feeding rates, and the number of daylight hours (Clarke and Morris, 1983). Significant variations were also observed among different species and seasons (Small and Hebard, 1967; Tremblay et al., 2014; Tarling, 2020). As previously reported by Ikeda (1970), we also obtained an increase of total respiration rates with weight (Figure 5A) and a decrease of specific respiration rate with the increasing of body weight (Figure 5B), as body mass is one of the major determinants of zooplankton respiration rates (Hernández-León and Ikeda, 2005). Our results showed significant correlations between temperature (Figure 6) and oxygen concentration (Figure 7) with specific respiration rates and ETS activities. However, the correlations between chlorophyll a values and primary production with respiration rates, specific ETS activity, or R/ETS ratios were not statistically significant (Figure 8), although the GAM results indicated that chlorophyll a significantly influences respiration rates (Table 2). This disagreement suggests that chlorophyll a, while not the primary driver, provides additional explanatory power in predicting euphausiid respiration rates (Chen and Smith, 2018). Indeed, the relationship between primary production and euphausiids’ respiration rates, abundance, and species diversity is not straightforward (Tremblay et al., 2020). The GAM approach allows the detection of nonlinear relationships and the interactions between multiple environmental variables, thus revealing the indirect influence of chlorophyll a on euphausiid metabolism. In any case, our findings indicated that chlorophyll a does not directly influence respiration rates. Instead, other environmental factors such as temperature and oxygen concentration appear to have a more direct impact on respiration rates and specific ETS activity.
Temperature has different impacts on euphausiids respiration rates depending on the oxygen concentration (Tremblay et al., 2020). Moreover, oxygen in the mesopelagic layer also affects zooplankton by influencing their community structure, vertical distribution (Ekau et al., 2010), metabolic activity (Kiko and Hauss, 2019), feeding, and excretion rates (Robinson et al., 2010). Our findings, supported by the Generalized Additive Model (GAM) results (Table 2) and the Pearson’s correlations (Figures 6, 7), indicated that temperature and oxygen concentration significantly influenced euphausiids respiration rates. However, we observed a negative correlation between temperature and both euphausiid respiration rates and ETS activity, which contrasts with previous research on the effects of temperature on euphausiids metabolism (Iguchi and Ikeda, 2005; Ikeda, 2013b; Kiko and Hauss, 2019). This discrepancy may be attributed to oxygen concentration having a greater impact on the metabolism of these organisms than temperature, as we obtained the highest respiration rates in areas with high mesopelagic oxygen concentration, lower epipelagic temperatures, and high chlorophyll a values (i.e., ST10 and ST8, Table 1). Similar results were previusly reported by Kiko and Hauss (2019) in the Tropical Pacific, where theirs results indicated that oxygen is a key environmental variable that scales metabolic activity. However, research has focused on scenarios involving increasing temperatures and low oxygen concentrations, which could negatively impact hypoxia tolerance due to increased energy expenditures (Ekau et al., 2018). Yet, in this study, the higher respiration rates were observed in areas characterized by low temperature but high oxygen concentrations. Further research is needed to unravel the combined effects of temperature and oxygen concentrations on euphausiid physiological rates.
The influence of mesopelagic oxygen concentration on the euphausiids’ respiration rates and enzymatic activity might be attributed to the diverse responses to varying oxygen levels. Some species exhibit a high degree of oxyconformity, decreasing their oxygen consumption rates as oxygen levels decline. Conversely, other species display strong oxyregulation, maintaining a consistent oxygen uptake irrespective of ambient oxygen levels (Herrera et al., 2019; Tremblay et al., 2020). Other individuals, at the limit of their aerobic scope (the difference between the routine and the maximum metabolic rate), reach a point where oxygen levels in the body fluids begin to decline and the capacity to adjust respiration becomes gradually limited (Pörtner, 2002). At this point, they reverted to anaerobic mode of mitochondrial metabolism caused by an excessive oxygen demand if reached the critical threshold temperature (Ollier et al., 2018; Tarling, 2020). The different adaptations of euphausiids to low dissolved oxygen concentrations affect the respiration rates reflecting their ecophysiological plasticity (Werner, 2013) and are linked to the temperature they experience during the diel vertical migration (Tremblay et al., 2020). This leads to a vertical limitation of habitats for these diel vertical migrant organisms, shaping the different species’ behavior, distribution and physiological processes (Tremblay et al., 2020). Under the actual global warming scenario of expanding and intensifying oxygen minimum zones (Stramma et al., 2008), and considering the key position of euphausiids in the trophic web dynamics (Lee et al., 2022), the physiological and behavioral adaptions, and strategies of these organisms to different levels of oxygen and temperatures should be further addressed.
The R/ETS ratio has been subject of debate since Packard et al. (1974). It appears that the R/ETS ratio in marine zooplankton is influenced by a combination of factors, including zooplankton size, temperature, metabolic activity, primary production, and the limitations of the measurement techniques used (Hernández-León and Gómez, 1996). However, the available database on R/ETS ratios remains relatively limited, which hampers the comprehensive study of the variables affecting respiration rates, thus the ETS activities and R/ETS ratios. High R/ETS ratios were associated with fed organisms exhibiting higher average respiration but lower ETS activity at the end of incubation, while lower R/ETS ratios are associated with low primary production scenarios (Hernández-León and Gómez, 1996). Despite these findings, the influence of oxygen concentration on R/ETS ratios in zooplankton was not previously studied. To explore the impact of environmental variables on the estimated epipelagic and mesopelagic R/ETS ratios, we applied the Generalized Additive Model (GAM) fitted for respiration rates. This approach was chosen due to the lack of significant correlations between temperature, oxygen concentration, chlorophyll a, or primary production values and the R/ETS ratios. While epipelagic oxygen and chlorophyll a significantly influenced (p<0.05 and p<0.0001, respectively) the epipelagic R/ETS ratios, both the epipelagic oxygen concentration (p=0.05), chlorophyll a (p<0.001), and the interaction between epipelagic temperature and mesopelagic oxygen concentration (p<0.05) influenced the mesopelagic R/ETS ratios. These results suggest a dependence between the respiration rates in the epipelagic layer during the nighttime (expected to be higher with increasing chloropyll a) and the mesopelagic R/ETS during the daytime (due to increased metabolism at their residence depth), supporting the indirect influence of chlorophyll a on euphausiid metabolism.
Most studies quantifying the carbon transported to the deep ocean due to zooplankton and micronekton diel vertical migrations use a conservative R/ETS ratio of 0.5 (e.g. Ariza et al., 2015; Hernández-León et al., 2019a, 2019c; Sarmiento-Lezcano et al., 2022). However, it is known that R/ETS values are quite variable, usually ranging from 0.5 to 1.0, or even reaching ratios over 1 during periods of high primary production or nutrient-rich conditions (Hernández-León and Gómez, 1996). Using a single, fixed R/ETS ratio to estimate respiration from ETS measurements may lead to over- or underestimation of carbon flux, given that the ratio is not constant. In this context, we obtained a mean R/ETS value of 0.91 ± 0.19 (n=66) in the epipelagic layer for different species of euphausiids (Supplementary Table 1), similarly to the R/ETS value of 0.96 ± 0.29 obtained by Hernández-León et al. (2019b) for migrant copepods. The estimated mean R/ETS ratio for the mesopelagic layer (Table 1) obtained in this study was 0.81 ± 0.18, indicating that respiration rates are higher compared to the conservative value of 0.5 commonly used. Consequently, a larger quantity of carbon is likely to be transported to the deep ocean due to migrant zooplankton and micronekton respiration at depth. The respiration of the mesopelagic organisms is a key controlling factor of carbon export to the deep ocean and plays a significant role in active carbon flux. Understanding the processes and mechanisms of mesopelagic respiration is crucial for accurately modeling and predicting the carbon cycle in the ocean.
In summary, we conducted respiration rate measurements on euphausiids captured in the epipelagic layer at night in areas with different environmental conditions. The resulting R/ETS ratios, both in the epipelagic and the mesopelagic layers, were higher than the conservative value of 0.5 typically used to assess active flux. We employed a GAM model to explore the relationship between the respiration rates obtained in the epipelagic layer at night and the environmental variables, revealing the influence of epipelagic oxygen concentration, chlorophyll a, and the interaction of epipelagic temperature and mesopelagic oxygen concentration on euphausiids respiration rates. However, further research is needed to evaluate the combined effects of the environmental variables on the respiration rates, as well as R/ETS ratios, in both the epipelagic and mesopelagic layer, and their influence on the biological carbon pump.
Data availability statement
The original contributions presented in the study are included in the article/Supplementary Material. Further inquiries can be directed to the corresponding author.
Ethics statement
Ethical approval was not required for the study involving animals in accordance with the local legislation and institutional requirements because no need of an ethical approval if not vertebrate.
Author contributions
MC: Investigation, Writing – original draft, Writing – review & editing. JD-P: Investigation, Writing – review & editing. AS-L: Investigation, Writing – review & editing. JL: Writing – review & editing. SH-L: Funding acquisition, Resources, Writing – review & editing.
Funding
The author(s) declare financial support was received for the research, authorship, and/or publication of this article. This work was supported by projects “DisEntangling Seasonality of Active Flux In the Ocean” (DESAFÍO, PID 2020- 118118RB-100) from the Spanish Ministry of Science and Innovation, and IMDEEP (ref: 2022CLISA15) from the Fundación CajaCanarias-La Caixa. MC was supported by a postgraduate grant (TESIS2022010116) co-financed by the Agencia Canaria de Investigación, Innovación y Sociedad de la Información de la Consejería de Universidades, Ciencia e Innovación y Cultura and by the Fondo Social Europeo Plus (FSE+) Programa Operativo Integrado de Canarias 2021-2027, Eje 3 Tema Prioritario 74 (85%). AS-L was supported by a postdoctoral grant (IN606B-2024/018) from the “Agencia Gallega de Innovación”. JD-P by a postgraduate grant (ULPGC2022-2) from Universidad de Las Palmas de Gran Canaria.
Acknowledgments
The authors wish to thank the crew and other scientists on board the R/V “Sarmiento de Gamboa” as well as the technicians of the “Unidad de Tecnología Marina” (UTM) for their support and help during the cruise.
Conflict of interest
The authors declare that the research was conducted in the absence of any commercial or financial relationships that could be construed as a potential conflict of interest.
Publisher’s note
All claims expressed in this article are solely those of the authors and do not necessarily represent those of their affiliated organizations, or those of the publisher, the editors and the reviewers. Any product that may be evaluated in this article, or claim that may be made by its manufacturer, is not guaranteed or endorsed by the publisher.
Supplementary material
The Supplementary Material for this article can be found online at: https://www.frontiersin.org/articles/10.3389/fmars.2024.1469587/full#supplementary-material
References
Akaike H. (1974). A new look at the statistical model identification. IEEE transactions on automatic control, 19, 716–723.
Angel M. V. (1989). Vertical profiles of pelagic communities in the vicinity of the Azores Front and their implications to deep ocean ecology. Prog. Oceanogr 22, 1–46. doi: 10.1016/0079-6611(89)90009-8
Ariza A., Garijo J. C., Landeira J. M., Bordes F., Hernández-León S. (2015). Migrant biomass and respiratory carbon flux by zooplankton and micronekton in the subtropical northeast Atlantic Ocean (Canary Islands). Prog. Oceanogr 134, 330–342. doi: 10.1016/j.pocean.2015.03.003
Cavan E. L., Belcher A., Atkinson A., Hill S. L., Kawaguchi S., McCormack S., et al. (2019). The importance of Antarctic krill in biogeochemical cycles. Nat. Commun. 10, 1 10, 1–13. doi: 10.1038/s41467-019-12668-7
Chen B., Smith S. L. (2018). Optimality-based approach for computationally efficient modeling of phytoplankton growth, chlorophyll-to-carbon, and nitrogen-to-carbon ratios. Ecol. Model. 385, 197–212. doi: 10.1016/j.ecolmodel.2018.08.001
Clarke A., Morris D. J. (1983). Towards an energy budget for krill: the physiology and biochemistry of Euphausia superba Dana. Polar Biol. 2, 69–86. doi: 10.1007/BF00303172
Ekau W., Auel H., Hagen W., Koppelmann R., Wasmund N., Bohata K., et al. (2018). Pelagic key species and mechanisms driving energy flows in the northern Benguela upwelling ecosystem and their feedback into biogeochemical cycles. J. Mar. Syst. 188, 49–62. doi: 10.1016/j.jmarsys.2018.03.001
Ekau W., Auel H., Püortner H. O., Gilbert D. (2010). Impacts of hypoxia on the structure and processes in pelagic communities (zooplankton, macro-invertebrates and fish). Biogeosciences 7, 1669–1699. doi: 10.5194/BG-7-1669-2010
Ens N. J., Do W Er J. F., Gauthier S. (2023). Geographic variability in the seasonality of euphausiid diel vertical migrations among three locations in coastal British Columbia, Canada. ICES J. Mar. Sci. fsad177, 1–12. doi: 10.1093/ICESJMS/FSAD177
Gómez M., Torres S., Hernández-León S. (1996). Modification of the electron transport system (ETS) method for routine measurements of respiratory rates of zooplankton. South Afr. J. Mar. Sci. 17, 15–20. doi: 10.2989/025776196784158446
Hernández-León S., Calles S., de Puelles M. L. F. (2019b). The estimation of metabolism in the mesopelagic zone: disentangling deep-sea zooplankton respiration. Prog. Oceanography 178, 102163. doi: 10.1016/j.pocean.2019.102163
Hernández-León S., Gómez M. (1996). Factors affecting the respiration/ETS ratio in marine zooplankton. J. Plankton Res. 18, 239–255. doi: 10.1093/plankt/18.2.239
Hernández-León S., Ikeda T. (2005). A global assessment of mesozooplankton respiration in the ocean. J. Plankton Res. 27 (2), 153–158.
Hernández-León S., Olivar M. P., Fernández de Puelles M. L., Bode A., Castellón A., López-Pérez C., et al. (2019a). Zooplankton and micronekton active flux across the tropical and subtropical atlantic ocean. Front. Mar. Sci. 6. doi: 10.3389/fmars.2019.00535
Hernández-León S., Putzeys S., Almeida C., Bécognée P., Marrero-Díaz A., Arístegui J., et al. (2019c). Carbon export through zooplankton active flux in the Canary Current. J. Mar. Syst. 189, 12–21. doi: 10.1016/j.jmarsys.2018.09.002
Herrera I., Yebra L., Antezana T., Giraldo A., Färber-Lorda J., Hernández-León S. (2019). Vertical variability of Euphausia distinguenda metabolic rates during diel migration into the oxygen minimum zone of the Eastern Tropical Pacific off Mexico. J. Plankton Res. 41, 165–176. doi: 10.1093/plankt/fbz004
Iguchi N., Ikeda T. (2005). Effects of temperature on metabolism, growth and growth efficiency of Thysanoessa longipes (Crustacea: Euphausiacea) in the Japan Sea. J. Plankton Res. 27 (1), 1–10.
Ikeda T. (1970). Relationship between respiration rate and body size in marine plankton animals as a function of the temperature of habitat. Bull. Fac. Fish. Hokkaido Univ. 21 (2), 91–112.
Ikeda T. (2013a). Respiration and ammonia excretion of euphausiid crustaceans: synthesis toward a global-bathymetric model. Mar. Biol. 160, 251–262. doi: 10.1007/s00227-012-2150-z
Ikeda T. (2013b). Metabolism and chemical composition of pelagic decapod shrimps: synthesis toward a global bathymetric model. J Oceanogr. 69 (6), 671–686.
Ikeda T., Motoda S. (1978). Estimated zooplankton production and their ammonia excretion in the Kuroshio and adjacent seas. Fish. Bull. 76, 357–367.
Kenner R. A., Ahmed S. I. (1975). Measurements of electron transport activities in marine phytoplankton. Mar. Biol. 33, 119–127. doi: 10.1007/BF00390716
Kiko R., Hauss H. (2019). On the estimation of zooplankton-mediated active fluxes in oxygen minimum zone regions. Front. Mar. Sci. 6. doi: 10.3389/FMARS.2019.00741/BIBTEX
King F. D., Packard T. T. (1975). Respiration and the activity of the respiratory electron transport system in marine zooplankton. Limnol Oceanogr 20, 849–854. doi: 10.4319/lo.1975.20.5.0849
Kobari T., Ueda A., Nishibe Y. (2010). Development and growth of ontogenetically migrating copepods during the spring phytoplankton bloom in the Oyashio region. Deep Sea Res. Part II: Topical Stud. Oceanography 57, 1715–1726. doi: 10.1016/j.dsr2.2010.03.015
Lampitt R. S., Achterberg E. P., Anderson T. R., Hughes J. A., Iglesias-Rodriguez M. D., Kelly-Gerreyn B. A., et al. (2008). Ocean fertilization: a potential means of geoengineering? Philos. Trans. R. Soc. A: Mathematical Phys. Eng. Sci. 366, 3919–3945. doi: 10.1098/rsta.2008.0139
Le Borgne R., Rodier M. (1997). Net zooplankton and the biological pump: A comparison between the oligotrophic and mesotrophic equatorial Pacific. Deep Sea Res. 2 Top. Stud. Oceanogr 44, 2003–2023. doi: 10.1016/S0967-0645(97)00034-9
Lee H., Choi J., Im Y., Oh W., Hwang K., Lee K. (2022). Spatial–temporal distribution of the euphausiid euphausia pacifica and fish schools in the coastal southwestern east sea. Water 14, 203. doi: 10.3390/W14020203
Letessier T. B., Falkenhaug T., Debes H., Bergstad O. A., Brierley A. S. (2011). Abundance patterns and species assemblages of euphausiids associated with the Mid-Atlantic Ridge, North Atlantic. J. Plankton Res. 33, 1510–1525. doi: 10.1093/PLANKT/FBR056
Longhurst A. R. (1985). Relationship between diversity and the vertical structure of the upper ocean. Deep Sea Res. Part A. Oceanographic Res. Papers 32, 1535–1570. doi: 10.1016/0198-0149(85)90102-5
Longhurst A. R., Bedo A. W., Harrison W. G., Head E. J. H., Sameoto D. D. (1990). Vertical flux of respiratory carbon by oceanic diel migrant biota. Deep-Sea Res I. 37, 685–694.
Longhurst A. R., Harrison G. W. (1988). Vertical nitrogen flux from the oceanic photic zone by diel migrant zooplankton and nekton. Deep Sea Res. Part A Oceanographic Res. Papers 35, 881–889. doi: 10.1016/0198-0149(88)90065-9
Lowry O. H., Rosebrough N. J., Farr A. L., Randall R. J. (1951). Protein measurement with the Folin phenol reagent. J. Biol. Chem. 193, 265–275. doi: 10.1016/S0021-9258(19)52451-6
Mundim K. C., Baraldi S., MaChado H. G., Vieira F. M. (2020). Temperature coefficient (Q10) and its applications in biological systems: Beyond the Arrhenius theory. Ecol. Model. 431, 109127. doi: 10.1016/j.ecolmodel.2020.109127
Ollier A., Chabot D., Audet C., Winkler G. (2018). Metabolic rates and spontaneous swimming activity of two krill species (Euphausiacea) under different temperature regimes in the St. Lawrence Estuary, Canada. J. Crustacean Biol. 38, 697–706. doi: 10.1093/jcbiol/ruy028
Owens T. G., King F. D. (1975). The measurement of respiratory electron-transport-system activity in marine zooplankton. Mar. Biol. 30, 27–36. doi: 10.1007/BF00393750
Packard T. T. (1971). The measurement of respiratory electron-transport activity in marine phytoplankton. J. Mar. Res. 29, (3). doi: 10.1098/rsta.2008.0139
Packard T., Harmon D., Boucher J. (1974). Respiratory electron transport activity in plankton from upwelled waters. Tethys 6, 213–222.
Pörtner H. O. (2002). Climate variations and the physiological basis of temperature dependent biogeography: systemic to molecular hierarchy of thermal tolerance in animals. Comp. Biochem. Phys. A 132, 739–761. doi: 10.1016/S1095-6433(02)00045-4
QGIS Development Team. (2024). QGIS geographic information system (Open-Source Geospatial Foundation Project). Available at: http://qgis.osgeo.org.
Robinson C., Steinberg D. K., Anderson T. R., Arístegui J., Carlson C. A., Frost J. R., et al. (2010). Mesopelagic zone ecology and biogeochemistry – a synthesis. Deep Sea Res. 2 Top. Stud. Oceanogr. 57 (16), 1504–1518. doi: 10.1016/j.dsr2.2010.02.018
Rutter W. J. (1967). “Methods in developmental biology,” in Methods in Developmental Biology. Eds. Wilt H. F., Wessels N. K. (Academic Press, London), 671–684.
Sarmiento-Lezcano A. N., Busquets-Vass G., Rubio-Rodríguez U., Pilar Olivar M., Peña M., Medina-Suárez I., et al. (2022). Active flux seasonality of the small dominant migratory crustaceans and mesopelagic fishes in the Gulf of California during June and October. Prog. Oceanogr 208, 102894. doi: 10.1016/J.POCEAN.2022.102894
Small L. F., Hebard J. F. (1967). Respiration of a vertically migrating marine crustacean euphausia pacifica hansen. Limnol Oceanogr 12, 272–280. doi: 10.4319/lo.1967.12.2.0272
Steinberg D. K., Carlson C. A., Bates N. R., Goldthwait S. A., Madin L. P., Michaels A. F. (2000). Zooplankton vertical migration and the active transport of dissolved organic and inorganic carbon in the Sargasso Sea. Deep Sea Res. Part I: Oceanographic Res. Papers 47, 137–158. doi: 10.1016/S0967-0637(99)00052-7
Stramma L., Johnson G. C., Sprintall J., Mohrholz V. (2008). Expanding oxygen-minimum zones in the tropical oceans. Science 1979 320, 655–658. doi: 10.1126/SCIENCE.1153847
Stukel M. R., Irving J. P., Kelly T. B., Ohman M. D., Fender C. K., Yingling N. (2023). Carbon sequestration by multiple biological pump pathways in a coastal upwelling biome. Nat. Commun. 14, 1 14, 1–10. doi: 10.1038/s41467-023-37771-8
Sutton A. L., Beckley L. E. (2022). Krill along the 110°E meridian: Oceanographic influences on assemblages in the eastern Indian Ocean. Deep Sea Res. Part II: Topical Stud. Oceanography 202, 105133. doi: 10.1016/J.DSR2.2022.105133
Tarling G. A. (2020). Routine metabolism of Antarctic krill (Euphausia superba) in South Georgia waters: absence of metabolic compensation at its range edge. Mar. Biol. 167, 108. doi: 10.1007/s00227-020-03714-w
Tremblay N., Hünerlage K., Werner T. (2020). Hypoxia tolerance of 10 euphausiid species in relation to vertical temperature and oxygen gradients. Front. Physiol. 11. doi: 10.3389/FPHYS.2020.00248/BIBTEX
Tremblay N., Werner T., Huenerlage K., Buchholz F., Abele D., Meyer B., et al. (2014). Euphausiid respiration model revamped: Latitudinal and seasonal shaping effects on krill respiration rates. Ecol. Modell 291, 233–241. doi: 10.1016/j.ecolmodel.2014.07.031
Werner T. (2013). Trophic positioning, diel vertical migration and physiological constraints in euphausiid species of the Namibian upwelling system (Doctoral dissertation, Staats-und Universitätsbibliothek Hamburg Carl von Ossietzky).
Keywords: euphausiids, respiration rates, specific ETS activity, R/ETS ratio, vertical migration, active carbon pump
Citation: Couret M, Díaz-Pérez J, Sarmiento-Lezcano AN, Landeira JM and Hernández-León S (2024) Respiration rates and its relationship with ETS activity in euphausiids: implications for active flux estimations. Front. Mar. Sci. 11:1469587. doi: 10.3389/fmars.2024.1469587
Received: 24 July 2024; Accepted: 16 September 2024;
Published: 03 October 2024.
Edited by:
Martin F. Soto-Jimenez, National Autonomous University of Mexico, MexicoReviewed by:
Martin Frías-Espericueta, Autonomous University of Sinaloa, MexicoHelmut Maske, Center for Scientific Research and Higher Education in Ensenada (CICESE), Mexico
Copyright © 2024 Couret, Díaz-Pérez, Sarmiento-Lezcano, Landeira and Hernández-León. This is an open-access article distributed under the terms of the Creative Commons Attribution License (CC BY). The use, distribution or reproduction in other forums is permitted, provided the original author(s) and the copyright owner(s) are credited and that the original publication in this journal is cited, in accordance with accepted academic practice. No use, distribution or reproduction is permitted which does not comply with these terms.
*Correspondence: María Couret, bWFyaWEuY291cmV0QHVscGdjLmVz; bWFyaWFjb3VodWVydGFzQGdtYWlsLmNvbQ==
†ORCID: Javier Díaz-Pérez, orcid.org/0000-0002-7268-4398