- 1Sea and Island Research Laboratory, Dongying Institute of Marine Development Research, Dongying, China
- 2Dongying Haimu Agricultural Technology Co., Ltd., Dongying, China
- 3Faculty of Hydraulic Engineering, Environment and Oceanography, Ludong University, Yantai, China
Mariculture ponds are essential components of the coastal wetland, which are often criticized by eutrophication risk for the dissolved inorganic nitrogen (DIN) input to the coastal zone by the culture tailwater. However, the reduce of this DIN pollution was difficult because the tailwater is hard to collect and the treatment is inefficient and expensive. Sesuvium Portulacastrum is a coastal vegetation which has high efficiency in DIN absorption from the seawater and sediment. In this study, we use Sesuvium Portulacastrum as a tool species to study the control behavior of the DIN in mariculture ponds wetland. The change trend of DIN in pond water and benthic species in pond sediment was investigated. The results showed that Sesuvium Portulacastrum reduced NH4+, NO3-, and NO2- in the pond water by 83.21%, 95.22%, and 91.32%, respectively. The species number of benthic organisms was enhanced from 2 to 5 and the species structure was more optimized in Sesuvium Portulacastrum pond than control pond. At the end of the experiment, eutrophication indicator species (Capitella capitata) was disappeared in the Sesuvium Portulacastrum pond. Those suggest that the coastal vegetation (Sesuvium Portulacastrum) have great potential to eliminate DIN pollutants in mariculture pond wetland.
1 Introduction
Mariculture ponds is an essential component of the coastal wetland because the large water body and proximity the coastal area. It supplies a large amount of quality protein for human about 30% of global mariculture production (The State of World Fisheries and Aquaculture, 2022). Meanwhile, it is often criticized by eutrophication risk for the input the effluents rich in N and P to the adjacent coastal zone. According to the World Food and Agriculture Organization (FAO), the growth in global marine and coastal aquaculture production will mainly come from mariculture in the next 30 years. With this rapid development trend, adjacent coastal zones would receive a large amount of mariculture tailwater, which enhance the eutrophication risk in this area (Levy et al., 2017). However, human activities such as overfishing, especially the overharvesting of shellfish, have excessive destructed the coastal sediments. It resulted in the disappearance of indigenous habitats such as seagrass beds, macroalgae bed, and oyster reefs which express the key buffering and degradation capacity of coastal zones for the N and P pollutants (Planque et al., 2010). Furthermore, the restoration of indigenous habitats in coastal zone is often slow and difficult. Therefore, it is important to control the N and P level in mariculture pond wetlands to reduce the input of this contaminant to coastal zone.
Currently, the treatment of mariculture tailwater is difficult because it is rich in variety of pollutants including N, P, and a larger number of organic pollutants such as bait and animal limbs (Chen et al., 2016). These pollutants are prone to clogging treatment equipment, which increases the equipment consumed and cost. Furthermore, the high salinity of the tailwater will corrode treatment equipment made of steel. Taking the normal industrial treatment model, the cost of tailwater treatment is 1.47 RMB m-3 which could increase the cost of mariculture about 17.6 RMB kg-1, about 50% of the original culture cost (Zeng et al., 2019). Hence, industrial treatment model is unsustainable which tends to reduce the expansion of mariculture by the high culture cost. Therefore, it is urgent to develop a low-costly and eco-friendly method to control the N and P pollution level of mariculture tailwater.
Artificial wetlands constructed by the salt marsh vegetations are often eco-friendly method applied for mariculture tailwater treatment (Hu et al., 2017; Cai et al., 2022). The removal rates of N and P pollution can be up to 92% and 72% or more in this kind of system (Wang et al., 2014). High removal capacity of N and P are form two pathways. First are the abundant microorganisms such as Phytophthora nitrite, Vibrio vulnificus, and Platyhelminthes supply by the root system of the salt marsh vegetation. Another was the uptake of N and P by the growth of salt marsh vegetation in wetland. The combination and cooperative interaction of microorganisms and the growth of salt marsh vegetation would make the efficiently removal capacity of artificial wetlands. In addition, the macro-vegetation surviving in salt marshes, such as Salicornia europaea L, Suaeda salsa (L.) Pall., and Sesuvium portulacastrum, has evolved the ability to absorb multiple forms of elemental N. Those vegetations could directly utilize peptide organic nitrogen in seawater, which is important for scavenging N in mariculture tailwater (Quintã et al., 2015; Zheng et al., 2016). However, there are still intractable limits for the application of artificial wetland in treatment mariculture ponds tailwater which constructed based by salt marsh vegetations. For example, the high temperatures and salinity of mariculture water make the salt marsh vegetations difficult survival in this harsh environment. It is necessary to select and breed of vegetations tolerant to high temperatures, high salinity, and high stress tolerance for application in the control of pollutants in mariculture wetland.
Sesuvium portulacastrum is a perennial herbaceous saline vegetations that grows in tropical and subtropical coastal areas (Fan et al., 2010). It often grows in high temperature, high salinity, and long-term flooding adverse environments and have potential to select as the contaminant control species in mariculture wetlands. Some applications of the Sesuvium portulacastrum in mariculture tailwater treatment have been conducted. For example, wetlands constructed based by Sesuvium portulacastrum could increase the removal efficiency of DIN in mariculture effluents (Ma et al., 2021). Sesuvium portulacastrum could remove 98.5% of NH4+ and 55.9% of total nitrogen in mariculture tailwater (Ying et al., 2018). In addition, the use of Sesuvium portulacastrum in a recirculating aquaculture system integrating Chanos chanos and Holothuria scabra allowed ammonia levels to be controlled and reliably decreased to < 1 mg L-1 (Senff et al., 2015). Hence, Sesuvium portulacastrum has great potential applications for controlling N pollution in mariculture water environment.
In this work, we select open-air Holothuria scabra mariculture ponds in the Yellow River Delta as the study area. The role of Sesuvium portulacastrum in controlling inorganic nitrogen (NH4+, NO3-, and NO2-) in pond water during the summer and early autumn growth season of Holothuria scabra was observed. Biodiversity parameters were investigated for the pond substrate. Meanwhile, the water temperature in this system was monitored because it is the key factor in ensuring that the Holothuria scabra safely survives in growing season in summer and early autumn.
2 Materials and methods
2.1 Study location
The Yellow River Delta is the important coastal Holothuria scabra culture zone in China. The culture scale has exceeded 333 km2 and accounts for more than half of the pond culture area of sea cucumbers in Shandong Province. This region has become the largest sea cucumber pond culture area in China (Wang and Liu, 2023). However, the temperatures and rainfall are variable in the Yellow River Delta, which pose a potential threat to sea cucumber mariculture. In the summers of 2013, 2016, and 2017, the production of Holothuria scabra mariculture was significantly decreased due to the high temperatures and lack of oxygen by the short-time strong rainfall. It had seriously affected the development of Holothuria scabra mariculture and the living of mariculturist in this area. Meanwhile, the discharge of tailwater would be urgent problem for the culture of Holothuria scabra with the gradual tightening of environmental protection policies for mariculture. The experiment was conducted in the Holothuria scabra culture ponds in Dongying Kenli District Huilu Aquaculture Co. in the coastal area of Laizhou Bay (E 118°57′50.39″, N 37°37′12.00″) (Figure 1).
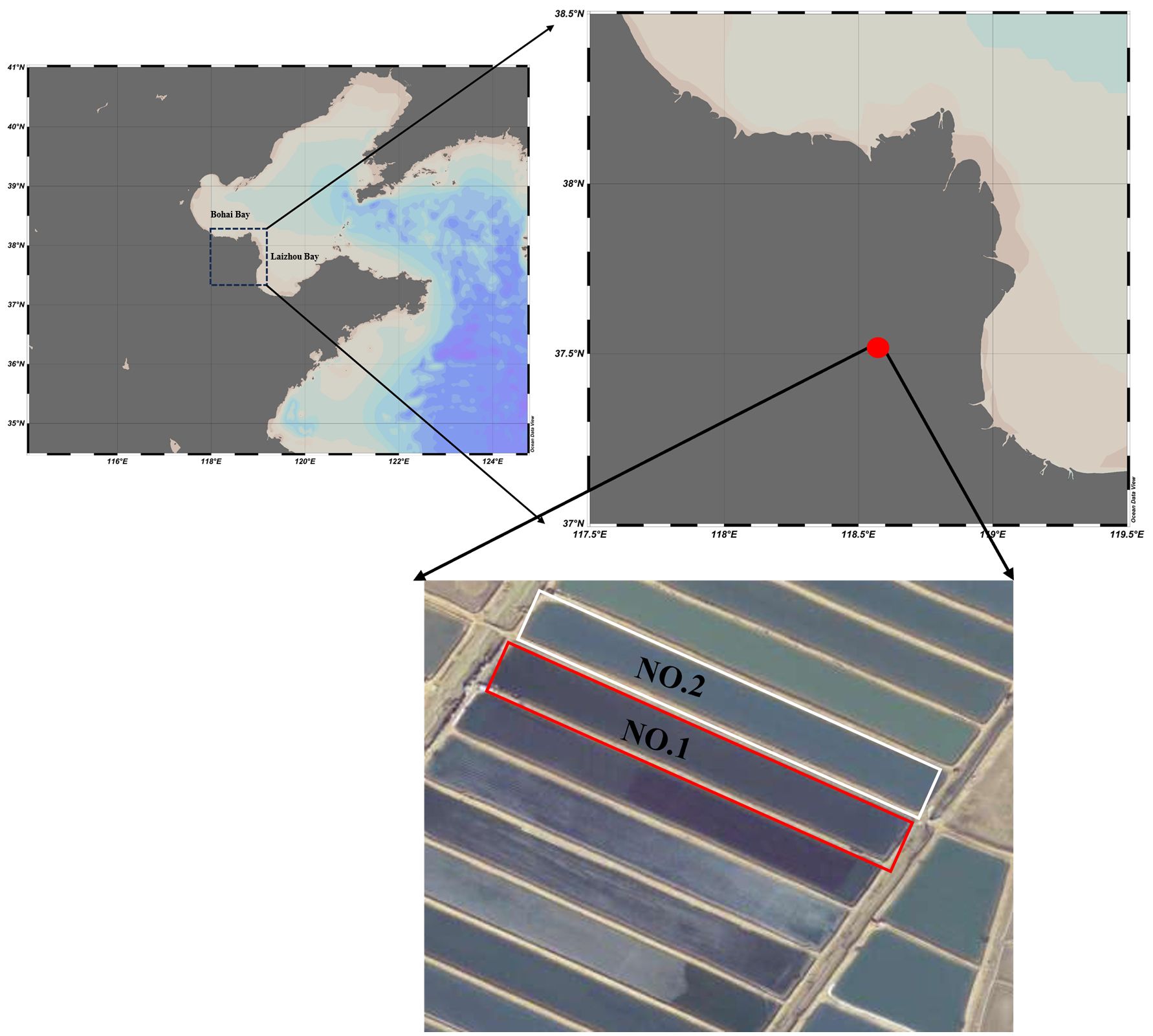
Figure 1. The location of the experiment in the sea cucumber ponds in Dongying Kenli District Huilu Aquaculture Co. in the coastal area of Laizhou Bay (E 118°57′50.39″, N 37°37′12.00″). The red boxes in the figure represent the experimental area (NO. 1). The white boxes in the figure represent the control area (NO. 2).
2.2 Experimental setup
2.2.1 Sesuvium portulacastrum
In summer, Holothuria scabra ponds are characterized by high temperature and salinity. Hence, we collected Sesuvium portulacastrum near the subtidal zone in Wenchang City, Hainan Province, which also has higher temperature and salinity. It is unable to overwinter and will not impact the local ecosystem in the Yellow River Delta. To maintain the activity of the Sesuvium portulacastrum seedlings, we conducted acclimatization process before the begin of the experiment in sea cucumber ponds for 15 d. The brief process was below: Sesuvium portulacastrum were staged in 30 L high-density polyethylene square boxes (60cm×50cm×10cm) contain about 20 L seawater. We used air to supply the dissolved oxygen for the Sesuvium portulacastrum at a rate of 1.5 m3 h-1 and a light period of 16h:8h. Thick and stout Sesuvium portulacastrum with fat leaves was selected for the experiment.
2.2.2 Experiment in the ponds
The experiment was carried out over a period of 120 d from June 5 to October 5 in 2023. The steps were as follows: Two ponds with the same area (1 hm2), specifications, and facilities were selected to carry out the experiment. Pond No. 1 served as the experiment pond and Pond No. 2 was as the control pond (Figure 1). The experimental ponds were rectangular in shape, east-west oriented, water depth up to 2.5 m, and water quality in accordance with The Water Quality Standards for Fishery (GB 11607-89). Six fish cages were set up in the experimental ponds, with specifications of 3m×1.5m×1m and a 30-purpose polyethylene mesh as the cage coat. Cages were fixed using bamboo poles and ropes, with a foam float tied to each of the four corners of the nets. The distance between every fish cage was 6m. The control pond also comprised six fish cages. At the beginning of June (June 5), we used a bench scale (AHC-WL, T-scale®) to weigh about 0.25kg of Holothuria scabra seeding (0.08g/ins-0.10g/ins) and put into every cage. Sesuvium portulacastrum with robust plants and fat leaves were selected and transplanted onto XPS (extruded polystyrene foam board) floating beds in June 10. The floating beds were secured together with ties and then fixed above the Holothuria scabra seeding cages. About 30% of the surface water of the sea cucumber culture cages was covered by floating beds. The same process was followed for the control pond. The initial concentration of DIN in control pond and experiment pond was 0.927 mg L-1 and 0.944 mg L-1, respectively. Figure 2 shows a brief flow of the experiment.
2.3 Sampling and analysis
2.3.1 Sampling
The sampling process is according our previous study and briefly described below (Wang and Liu, 2023). The sample system consists of a collection unit, a filtration unit, and a sampling unit. The collection unit was 125 ml HDPE bottles (Nalgene™, Thermo Fisher Scientific Inc., USA). The filtration unit was a pressure filtration unit (YY3014236, Filter Holder, Millipore®). Since the pond waters contained high concentrations of suspended particulate matter and organic matter, the diameter of the filter membrane (0.22 μm, GPWP14250, Millipore®) was 142 mm. The units were fixed using a C-Flex tube. When sampling, the C-Flex-tube-fixed water intake was directly inserted into the pond water to collected the samples. Two parallel samples were collected. The fixed water intake sampling tubes were attached to a disc filter unit, and washed with ultrapure water before and after each sample. The collected water samples were stored in self-sealing PE bag at -20°C. Sampling devices and bottles were soaked in 3% HNO3 for 48 h and then rinsed five times with ultra-pure water (>18.2 Ω) and blown dry on an ultra-clean table (Class-100). Water samples were collected every 10 days from June 5 to September 5. The last sample was collected every 30d from September 5 to October 5 because the culture in the pond was over.
Benthic organisms were sampled according to the quantitative sampling method in the Zhang et al., 2007. The collection gear was a QNC7-1 box-type mud collector, the sampling area was 0.25m2, and each station was sampled 4 times. Samples were eluted with a 0.5 mm mesh set sieve and macrobenthic samples were collected and stored in 500 ml HDPE bottles containing formaldehyde solution. The samples were preserved, categorized, and weighed in accordance with the Specifications for oceanographic survey-Part 6: Marine biological survey (GB/T 12763.3-2007).
2.3.2 Analysis
Regular parameters such as temperature (T), salinity (S), and dissolved oxygen (DO) were collected using a multiparameter water quality analyzer (ProQuatro, YSI®). The concentrations of DIN (NH4+-N, NO2–N, and NO3–N), active phosphate (PO43-), and DSi (SiO2) of the samples were detected by a continuous-flow nutrient analysis (AutoAnalyzer III, SEAL Analytical™). The quantification limit of the nutrient analysis method for DIN, active phosphate (PO43-), and DSi was 0.012 mg L-1, 0.008 mg L-1, 0.011 mg L-1 and the recoveries ranged from 95.5% to 101.2%. The samples were analyzed three times and the error must be less than 5%.
Indicator of coastal eutrophication potential (ICEP) was used to measure the nutrients risk of the N and P in the Sesuvium portulacastrum ponds to the coastal zone. This index was proposed by Billen and Garnier (2007) and modified it to suit the study because the pond water was not exchanged with the coastal area in the culture process. The modified equation is as follows:
In this study, benthic community compositional diversity was expressed using the Shannon–Wiener species diversity index (H’), the Margaief species abundance index (D), and the Pielou species evenness index (J) (Lobon-Cervia et al., 2012). The following formula was used:
Ni is the ratio of the number of individuals of species i to the total number of individuals of all captured organisms (ni/N); N is the total number of individuals of all captured organisms; and S is the total number of species of all captured organisms.
The Mcnaughton dominance index was used to determine the dominant species in the pond sediment (Lobon-Cervia et al., 2012). Community dominance was calculated using the following formula:
S1 and S2 are the biotic densities of the two most abundant species in the community; ST is the total benthic density.
3 Results
3.1 Basic geochemical parameters
The temperature (T), salinity (S), pH, and dissolved oxygen (DO) are key geochemical parameters related to the stability of the environment in Holothuria scabra pond. Especially for the temperature, it is related to the survival rate of the Holothuria scabra seedlings cultured in the pond. Figure 3 shows the change trend of basic geochemical parameters throughout the culture process. In all the ponds, the water temperature showed a trend of increasing and then decreasing, which was similar to the weather temperature changes during the experiment. However, changing amplitude of temperature was different from the experimental and control ponds (Figure 3A). In the control pond, the water temperature increased from 25°C to 30.9°C, whereas that of the experimental pond increased to 29.2°C. This indicates that the Sesuvium portulacastrum floating bed can effectively reduce the pond water temperature. It is important for the Holothuria scabra in the pond to survive the high temperature period in summer and early autumn.
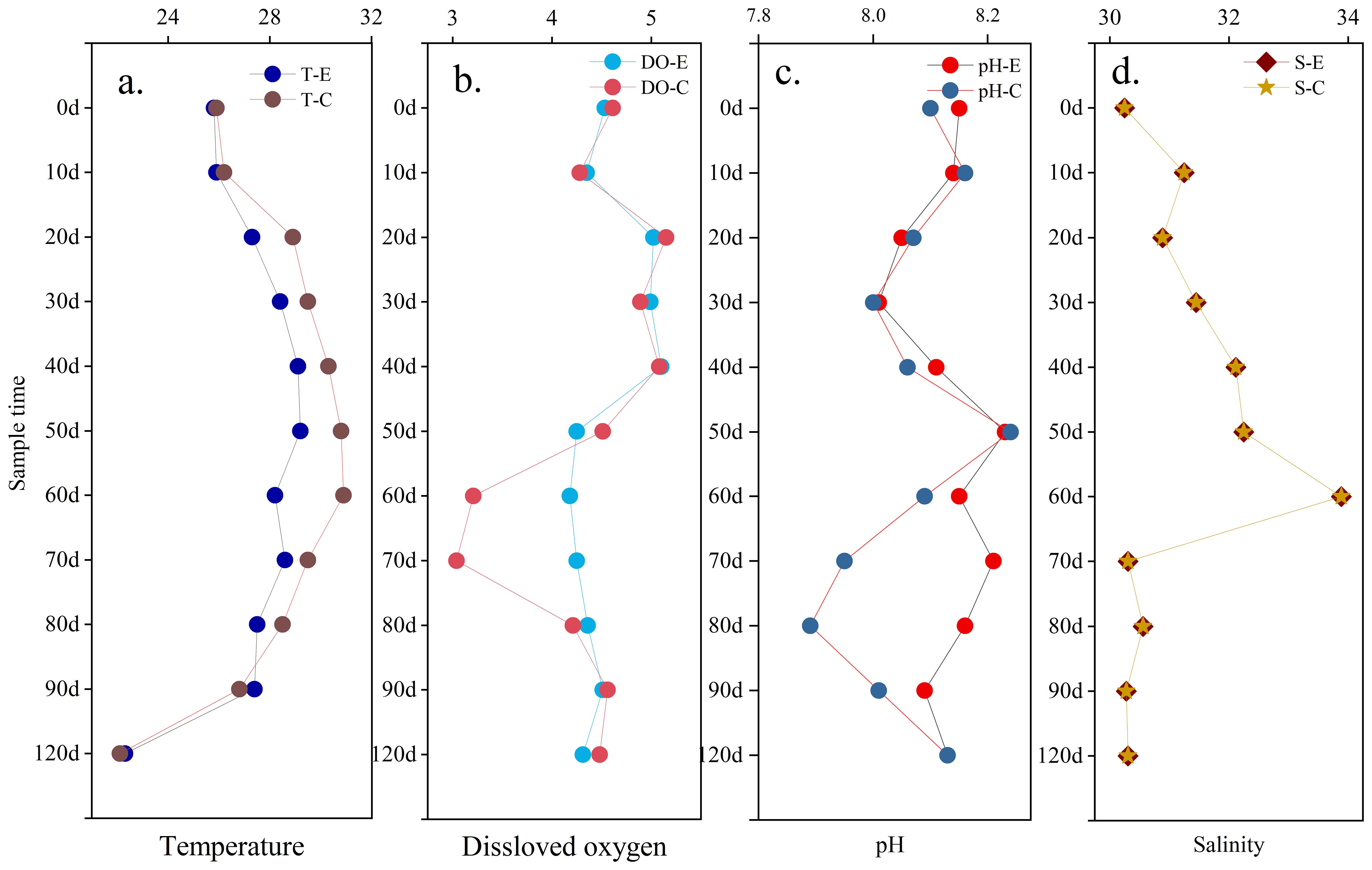
Figure 3. Change trend of basic biogeochemical parameters in ponds with or without Sesuvium portulacastrum.
Changes in DO are strongly influenced by the external environment such as wind around the pond because the water depth of the pond was only about 2m. In the Sesuvium portulacastrum pond, the average concentration of DO fluctuate around 4.53 mg L-1, with a concentration fluctuation range of 0.32 mg L-1 (Figure 3B). However, in the control pond, the average concentration of DO was 4.35 mg L-1, with a fluctuation range of 0.65 mg L-1. This fluctuation mainly occurred around 60d and 70d, and the lowest concentration of DO was 3.04 mg L-1 (Figure 3B). pH showed similar changes trend to DO (Figure 3C). In the Sesuvium portulacastrum pond, pH varied from 8.01 to 8.23 with a fluctuation of 0.06. However, in the control ponds, pH varied from 7.89 to 8.24 with a fluctuation of 0.09, and the severe changes were observed around 60 d and 70 d. For salinity, the change trend was almost identical between different ponds (Figure 3D). Salinity ranged from 30-34 and the highest salinity occurring during the maximum temperature in almost 32°C. Evaporation from the pond water body was high during high-temperature periods, resulting in higher salinity in the pond water (Figure 4D). During this period, Sesuvium portulacastrum still grew vigorously which indicating the salt and heat tolerance of this plant.
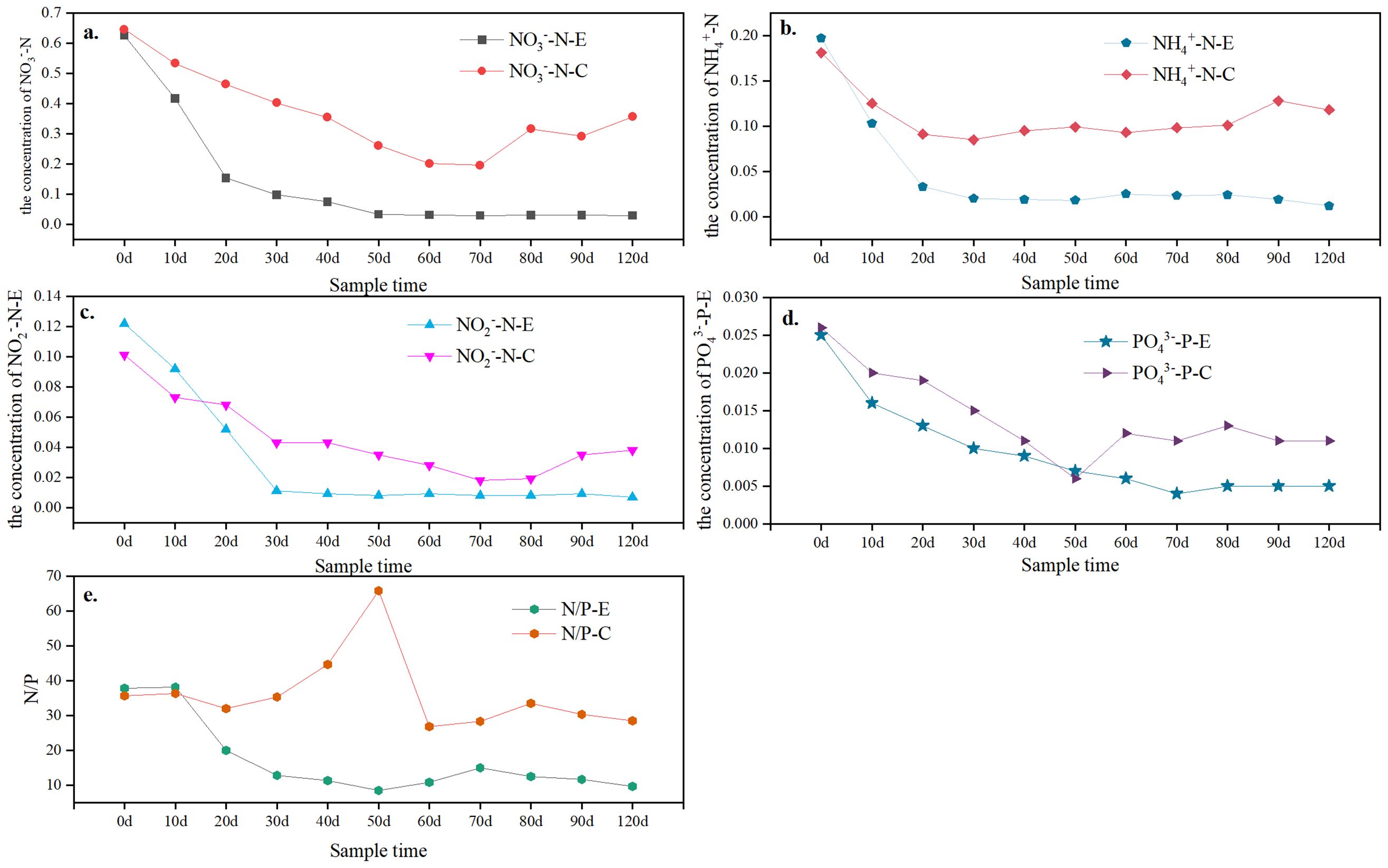
Figure 4. Concentration trend of dissolved inorganic nitrogen in the culture system with or without Sesuvium portulacastrum: (A) NO3–N variation; (B) NO2–N variation; (C) NH4+-N variation; (D) PO43–P variation; (E) N/P ration variation. X-E represent the pond with Sesuvium portulacastrum; X-C represent the pond without Sesuvium portulacastrum..
3.2 Dissolved inorganic nitrogen and reactive phosphate
Nitrate nitrogen (NO3–N) is the most essential forms for vegetation uptake in the pond. It is also one of the more stable states of elemental N. Figure 4A. shows the changes in NO3–N concentration in ponds with or without Sesuvium portulacastrum. The concentration of NO3–N varied with different ponds. In the Sesuvium portulacastrum pond, NO3–N was continued declining but the decrease rate gradually slowed with the decrease in temperature. The maximum concentration of NO3–N was found in June, about 0.625 ± 0.005 mg L-1. During the culture process, the concentration of NO3–N showed three change stages (Figure 4A). There was rapid decline phase from 0 to 40d, a slow decline phase from 40 to 60d, and an equilibrium rise phase from 60 to 90d. About 88.12% of the NO3–N was eliminated in the rapid decline phase, while there was only 5% in the slow decline stage. In the control pond, the minimum concentration of NO3–N was 0.195 ± 0.002 mg L-1 in August and was essentially unchanged by September. Notably, a strenuous fluctuation in NO3–N concentration was observed (about 0.356 ± 0.011 mg L-1) in October. However, the NO3–N in the Sesuvium portulacastrum pond was lower and more stable in the same period than the control pond. The elimination rate of NO3–N was 95.22% in the Sesuvium portulacastrum system but it was 42.56% in the control pond.
The variation of NO2–N was similar to NO3–N but more drastic in the culture system. Figure 4B shows the changes in NO2–N in the culture system with or without Sesuvium portulacastrum. A rapid decline stage was found from 0 to 30d in the Sesuvium portulacastrum system about 0.029 ± 0.003 mg L-1 (Figure 4B). However, in the control pond, this decline continued to 60d at a concentration amplitude of 0.019 ± 0.006 mg L-1. There was no increase in NO2–N in the Sesuvium portulacastrum pond during September and October. However, for the control ponds, NO2–N showed a significant increase and fluctuation. About 91.32% of the NO2–N was consumed in the Sesuvium portulacastrum system in the rapid decline stage (0-30d). However, the removal rate was 81.67% in the control pond.
The change trend of NH4+-N in the culture system was different to that of DIN in other forms. Figure 4C. shows the trend of NH4+-N in the culture system with or without Sesuvium portulacastrum. In the Sesuvium portulacastrum system, NH4+-N showed a very rapid decrease from 0 to 20d, and about 83.21% was removed. After this stage, NH4+-N showed a slow removal rate of only 8-10% from 20d to 40d. After 40d, the NH4+-N stably fluctuated around 0.19 ± 0.07 mg L-1 in the experimental pond. In the control pond, NH4+-N also showed a relatively intense decline, about 50% in 20d. However, the concentration of NH4+-N stably fluctuated around 0.10 ± 0.05 mg L-1 with the culture process.
Reactive phosphate levels in pond water were consistently low (Figure 4D). At the beginning of the experiment, the phosphate concentrations in the two ponds were 0.025 mg L-1 and 0.026 mg L-1, respectively. In the Sesuvium portulacastrum ponds, reactive phosphate had a rapid declining phase from 0 to 30d. It then gradually decreased to minimum of 0.005 mg L-1 in 30-70d stage. After 70d, the phosphate concentration in the ponds fluctuated around 0.005 mg L-1. In the control ponds, different trends of changes in phosphate concentration were observed. A sharp decline in phosphate occurred during the 0-50d period, although most of the decline was lower than in the experimental ponds. At 50d, the phosphate concentration reached minimum concentration (about 0.006mg L-1). However, after 50 days, there was a significant increase in phosphate concentration in the control pond. It eventually rose to 0.18 mg L-1. This trend was similar to the changes in DIN in the control system. The N/P ratio was also express extremely varied in the ponds with the culture (Figure 4E). In the control ponds, the N/P ratio was changed from 26.8 to 65.8 and maximum value was observed in 40d to 50d. It was no found a clear change trend in the N/P ratio. However, in the experiment ponds, the N/P ratio decreased with the culture time. Furthermore, the after 30d, the N/P was maintained in 11.5 ± 1.9. Moreover, the N/P ratio in the experiment pond was lower than in the control pond.
3.3 Eutrophication risk
The ICEP index indicated the eutrophication risk of the water in Holothuria scabra ponds and could use to measuring the impact of Holothuria scabra culture tailwater on the offshore environment. The maximum eutrophication index of DIN (about 4.63) in the Sesuvium portulacastrum pond appeared at the beginning of the experiment (Figure 5). With the culture process, the ICEP gradually decreased until the index became negative after up to 50 d in the experiment pond. It is notably indicated that the eutrophication risk of the experimental ponds disappeared. In the control ponds, the change trend of ICEP was in another way. During the 0-60d, the ICEP changed similarly to the experimental ponds, which gradually decreased but the decreased amplitude was smaller. However, a significant increase of the ICEP about 6.43 was observed in the control ponds at 70d (Figure 5). And the ICEP then decreased and finally stabilized at about 2.4. In the whole experiment process, the ICEP of the control ponds was higher than that in the experimental ponds. These results suggest that Sesuvium portulacastrum plays an important role in controlling eutrophication risk in Holothuria scabra ponds. The ICEP index for phosphate was consistently negative in both the experimental and control ponds. This result clarified that the Holothuria scabra ponds are phosphate-limited in the Yellow River Delta. It is noteworthy that ICEP index for phosphate fluctuated less in the experimental ponds than in the control ponds. It also indicates that Sesuvium portulacastrum plays an important role in phosphate stabilization in Holothuria scabra pond like the control behavior in DIN.
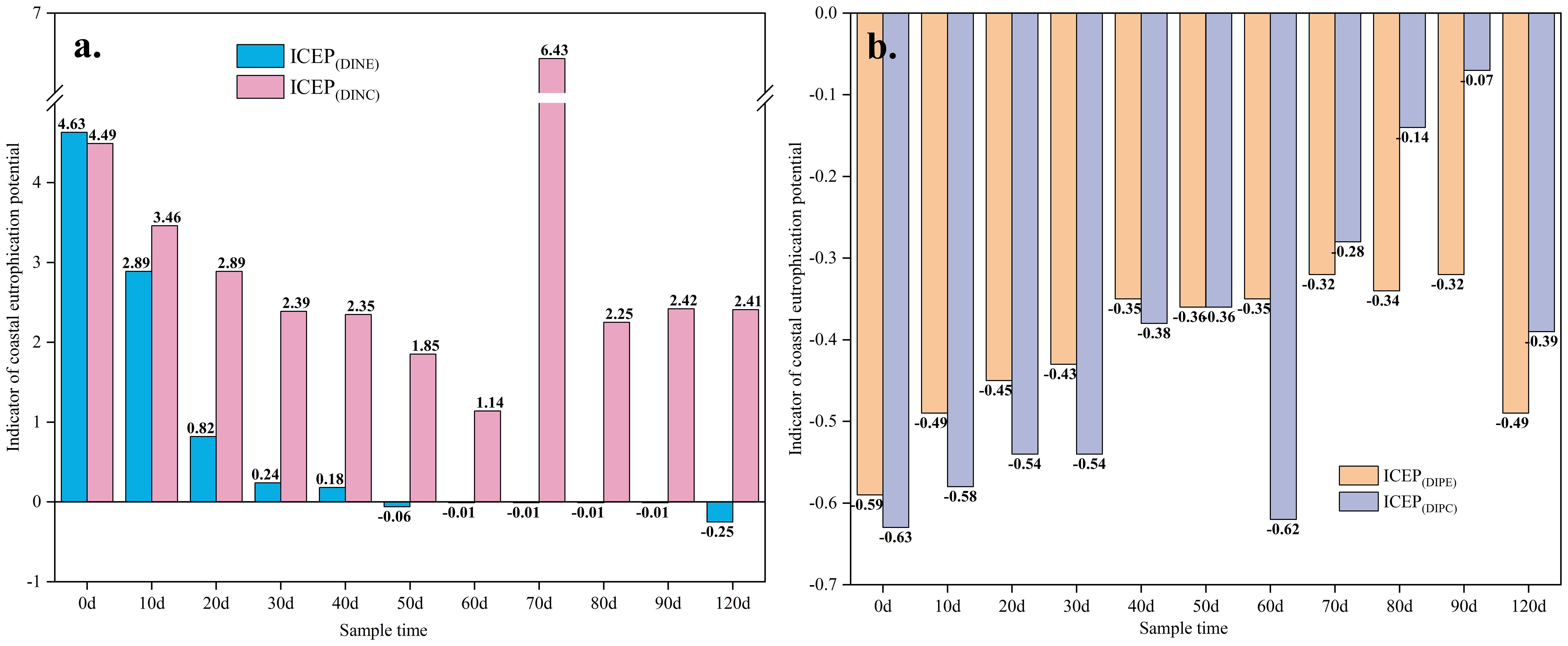
Figure 5. Indicator of coastal eutrophication potential in the culture system with or without Sesuvium portulacastrum. (A) ICEP(DINE) and ICEP(DINC) are the indicators of coastal eutrophication potential of dissolved inorganic nitrogen in the Sesuvium portulacastrum and control systems, respectively; (B) ICEP(DIPE) and ICEP(DIPC) are the indicators of coastal eutrophication potential of active phosphate in the Sesuvium portulacastrum and control systems, respectively.
3.4 Benthos community
The acquisition of benthic species in different ponds was compared at 0d, 30d, 60d, and 90d (Table 1 and Figure 6). In the control pond, only two benthic species was observed, which mainly dominated by Capitella capitata and with a small number of Minicoraphium insidiosum. The composition of benthic species was not change in this pond and the biodiversity parameters at low level. The Shannon–Wiener diversity index (H’) was about 0.13-0.16, the Pielou evenness index (J) was about 0.24-0.26, and the Margaief species abundance index (D) was about 0.19-0.22. It indicated that the control pond was undergoing persistently eutrophication. In the Sesuvium portulacastrum pond, the benthic species was changed with the experiment process. The benthic species was consistent to the control pond in the preliminary stage of the experiment, dominated by Capitella capitata and small number of Minicoraphium insidiosum. However, as the experiment continued, the number of species increased to 3 and Capitella capitata was about 45.23% lower than in the control pond in 30d. The Shannon–Wiener diversity index (H’) increased from 0.17 to 1.06, the Pielou evenness index (J) from 0.24 to 0.96, and the Margaief species abundance index (D) from 0.21 to 0.46. The change of benthic organisms in the Sesuvium portulacastrum pond was shown in Figure 6. Significant variations in species structure occurred during the 60 d. Although the number of species was decreased from 3 to 2, the Capitella capitata, a species indicative of eutrophication, decreased from 38.79% to 0% (Silva et al., 2017). Capitella capitata was replaced by the Perinereis aibuhitensis and Pseudopolydora paucibranchiata. Over the 90d, the number of species increased to 5 and some shellfish such as Theora lata appeared.
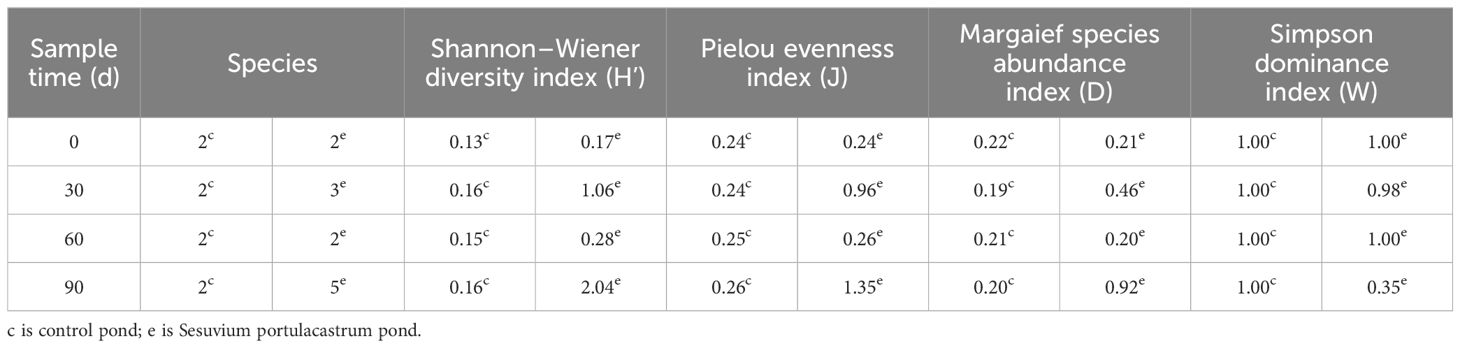
Table 1. Structural parameters of benthic organisms in the Sesuvium portulacastrum pond and control pond at different times.
3.5 Holothuria scabra seedling
At 80 d, five Holothuria scabra fishfly cages were randomly selected for weighing in the experiment and control ponds, respectively. This result would best reflect the role of the Sesuvium portulacastrum system in protection of Holothuria scabra seedlings because the high temperature period has just passed. The survival rate was calculated according to the seedling casting of 0.25 kg and the specification of 11,000 heads/kg. The survival rate results are shown in Table 2. In the experiment pond, the average harvest was 2.313 kg, with a single weight of 1.732 g ins-1 and a survival rate of 61.45%. In the control pond, the average harvest was 1.762 kg, with an average weight of 1.598 g ins-1 and a survival rate of 40.29%. The average harvest of the experimental pond was increased by 0.551 kg, the average weight of every seedling was increased by 0.078 g, and the survival rate was increased by 21.16% compared with the control pond.
4 Discussion
From the experiment results, the Sesuvium portulacastrum system in the Holothuria scabra ponds expressed positive influences in reducing pond eutrophication, increasing pond ecological stability, and conserving Holothuria scabra seedlings under high temperatures in summer and early autumn. The Sesuvium portulacastrum might be the key factor to induce those positive influences in the mariculture pond system. Figure 7 shows the potential ecological cycle built by Sesuvium portulacastrum.
4.1 For the dissolved inorganic nitrogen
In the begin of the experiment, the initially high concentration of NO3–N reflected the transformation of NH4+-N and NO2–N by microorganisms in the pond water (Diab et al., 1993). NO3–N is the most stable state of elemental N and was more conservative removal compared to other forms (NH4+-N and NO2–N) in the seawater. It was mainly accomplished through the uptake behavior of algae and vegetations in the pond water (Pereira et al., 2020). Generally, the higher concentration of NO3–N the faster uptake of Sesuvium portulacastrum in the pond water (Hao et al., 2024). When the NO3–N in the system declines to certain level, the uptake of this form will gradually become slower (Pereira et al., 2020; Hao et al., 2024). However, when the NO3–N is gradually depleted, the concentration balance between the sediment-water was broken induced the release of NO3–N from sediment to water (Khoi et al., 2006). This release process is mainly dominated by the microbial activities in the sediment (Zhang et al., 2015). Under the strong adsorption and uptake of the Sesuvium portulacastrum system, the released nitrate will be rapidly consumed (Peng et al., 2022). NO3–N in the pond will eventually reach the equilibrium with a relatively low concentration. In addition, epiphytic algae appeared on the roots of Sesuvium portulacastrum in the late stage of the experiment. This indicated that not only the Sesuvium portulacastrum but also the epiphytic environment created by the root system have an important influence on the NO3–N removal in the system (Dou et al., 2011; Liu et al., 2019). Hence, Sesuvium portulacastrum play powerful absorber for NO3–N in the mariculture pond because this form of nitrogen was easily and rapidly uptake by vegetations (Cai et al., 2022).
As an important intermediate state of nitrification in the Sesuvium portulacastrum pond system, the concentration of NO2–N reflects the influence of the whole system on the cycling of elemental N (Beman et al., 2013). The concentration of NO2–N was in the rapid decline stage (0-20d) when the dissolved oxygen increased in the pond water. High dissolved oxygen would promote more active microbial activity, which lead to the conversion of NO2–N to stable NO3–N and N2 (Rosamond et al., 2012). However, in this study, the decline in NO2–N was smaller in the experimental pond than that in the control pond. This might be the Sesuvium portulacastrum system consumed a certain amount of dissolved oxygen during the early growth stages of this plants. It results in partial hypoxia in the pond where the Sesuvium portulacastrum are deployed which could reduce the level of nitrification and denitrification (Ni et al., 2021). After 20d of the experiment, the level of NO2–N in the Sesuvium portulacastrum system decreased sharply. The nitrification system in the Sesuvium portulacastrum system was being constructed, resulting in a low level of intermediate nitrogen conversion in the system (Dou et al., 2011; Liu et al., 2019). Meanwhile, the damage of plant root by transplant during the construction of the Sesuvium portulacastrum system also caused the release and formation of nitrite in the pond water. When the construction of the microbial nitrification system was completed, the NO2–N was rapidly removed. With the culture process, the free NO2–N was transformed into a complete form in the pond water, and only a small amount was left to decompose continuously. This stable nitrification system would also continuously eliminate NH4+-N in the water, which was rapidly converted to NO3–N and NO2–N under sufficient oxygen (Dou et al., 2011). In addition, the Sesuvium portulacastrum would directly utilize the ammonium nitrogen in the water as a nutrient source for plant growth. The Sesuvium portulacastrum system not only an absorber of the NH4+-N but also comprises a healthy nitrification system to maintain the cycling between different forms of DIN in the pond. This stable cycle would make the changes in nitrate nitrogen, nitrite nitrogen, and ammonia nitrogen leveled off.
4.2 For the microalgae
The Sesuvium portulacastrum system also maintained the microalgae balance in the pond. During the 60d-70d period, abnormal changes in dissolved oxygen, pH, and DIN occurred in the control pond. We believe that this phenomenon is mainly related to the massive death of microalgae in the Holothuria scabra ponds, i.e., algal inversion. Microalgae blooms easily induced a sharp decrease of nutrients in the pond by rapid multiplication uptake amount of nutrients (Bell et al., 2015). However, disordered proliferation of microalga was not stable and often exceeded the survival capacity of the pond which could induce the mass and rapid death of this organism (Silva et al., 2017). In addition, sudden rainstorm following persistent high temperature also induced the algal inversion in the pond (Tayaban et al., 2018). The decomposition of microalgae led to sharply decrease in dissolved oxygen and the release of nutrient. Meanwhile, the microalgae gradually entered extinction also caused decrease in DO and pH in September (Sanchis-Perucho et al., 2018). However, this phenomenon did not occur in the Sesuvium portulacastrum system. This might because the nutrient uptake behavior of Sesuvium portulacastrum suppressed the microalgae in the pond and make them could not disorderly growth (Smith and Horne, 1988). Sesuvium portulacastrum could balance the changes in pH, dissolved oxygen, and nutrients timely when partial algal collapse or algal die-off in the pond. The ecosystem became more stable and the ecological buffering capacity for the DIN pollution were stronger in the Sesuvium portulacastrum pond.
4.3 For the organism
The Sesuvium portulacastrum system played a beneficial role and made positive influence on both cultured and benthic organisms. The continuous high temperature was often fatal to Holothuria scabra. In general, the pond water temperature once exceeded 33°C for more than 2d, the Holothuria scabra cultured in the pond would extinction due to intolerance to high temperatures (≥33°C) (Dong and Dong, 2009; Zhang et al., 2022). Meanwhile, the high temperature would also induce hypoxia of the pond water further leading to the death of Holothuria scabra (Unmuth et al., 2000; Zhang et al., 2022; Hoblyn and Iversen, 2024). Sesuvium portulacastrum community could suppression of high temperatures of pond water by covering water surface to reduce water temperature in the pond. The Sesuvium portulacastrum system diminished the water temperature by 1-2°C to enhance the Holothuria scabra survival rate about 21.6%. Notably, the Sesuvium portulacastrum system changed the organism species and structure in the pond. Aquatic vegetation cover was a significant environmental variable to influence the variance in aquatic organisms in pond sediment (Natsumeda et al., 2015). The vegetation could primarily affect the chemistry characters of pond sediment such as uptake more N and P, and then manage the evolution of ponds benthic species for the direction more species and prefer the vegetation (Sinclair et al., 2021). Hence, the Sesuvium portulacastrum system will induce the ponds environment more biodiversity.
In general, the strong uptake behavior of Sesuvium portulacastrum diminished the DIN in the mariculture pond, which created nutrient deficit between surface waters. This deficit induced the release of DIN, DIP, and other eutrophication factors from sediment (Wu et al., 2021). Meanwhile, it also increased the decomposition of organic debris in sediments. Changes in sediment composition would cause variations in benthos species structure (Posey et al., 1993; Ni et al., 2021). In mariculture pond, the benthic organisms in pond sediment were often single and mostly represented by eutrophication indicator species such as Capitella capitata. The establishment of the Sesuvium portulacastrum system led to a succession of benthic organisms in the mariculture pond (Kaenel et al., 1998). For example, the replacement of the Capitella capitata by the Perinereis aibuhitensis in the pond sediment indicated more stable and health pond depositional environment with the Sesuvium portulacastrum.
5 Conclusions
The Sesuvium portulacastrum system established in the Holothuria scabra ponds demonstrated a very positive impact on the pond and around environment. It reduced the NH4+, NO3-, and NO2- in the pond water by 83.21%, 95.22%, and 91.32%, respectively. Active phosphate was suppressed to low levels (<0.005 mg L-1) in the pond water. The number of pond benthic organism species was enhanced from 2 to 5. Capitella capitata, the eutrophication indicator organism, was disappeared and Perinereis aibuhitensis appeared. These results suggest that the Sesuvium Portulacastrum could both reduce DIN pollutants in Holothuria scabra culture pond and regulate the pond environment. However, studies on the effects of Sesuvium portulacastrum and other aquatic vegetations on the stability of mariculture pond ecosystems are still few and focused on nutrient depletion. Its effects on the microbial, microalgal, and elemental geochemical cycles and biodiversity in mariculture ponds still need to be further investigated. In addition, the wetland function of the mariculture pond around the coastal area should be taken seriously in future.
Data availability statement
The raw data supporting the conclusions of this article will be made available by the authors, without undue reservation.
Author contributions
KL: Writing – original draft, Writing – review & editing, Funding acquisition, Methodology, Software. WG: Formal analysis, Investigation, Visualization, Writing – review & editing. ZY: Investigation, Validation, Writing – review & editing. YH: Formal analysis, Investigation, Writing – review & editing. MZ: Formal analysis, Investigation, Writing – review & editing. CS: Investigation, Writing – review & editing. XZ: Investigation, Writing – review & editing. LW: Writing – original draft, Writing – review & editing, Conceptualization.
Funding
The author(s) declare financial support was received for the research, authorship, and/or publication of this article. This research was funded by the Natural Science Foundation of Dongying in China (2023ZR01) and the Foundation of State Environmental Protection Key Laboratory of Marine Ecosystem Restoration (202302).
Acknowledgments
We would like to thank all the group members for their constant field and laboratory assistance, helpful advice, and in-depth discussion. Special thanks to JunQi Chen for the tremendous work on the furthered English-editing and polishing on the manuscript. The Prof. Bingchen Wang and Dr. Fei Cheng were given amount of assistance in sampling and sample analysis.
Conflict of interest
Author XZ was employed by the company Dongying Haimu Agricultural Technology Co., Ltd.
The remaining authors declare that the research was conducted in the absence of any commercial or financial relationships that could be construed as a potential conflict of interest.
Publisher’s note
All claims expressed in this article are solely those of the authors and do not necessarily represent those of their affiliated organizations, or those of the publisher, the editors and the reviewers. Any product that may be evaluated in this article, or claim that may be made by its manufacturer, is not guaranteed or endorsed by the publisher.
References
Bell T., Bruyn W., Marandino C., Miller S., Law C., Smith. M., et al. (2015). Dimethylsulfide gas transfer coefficients from algal blooms in the Southern Ocean. Atmo. Chem. Phys. 15, 1783–1794. doi: 10.5194/acp-15-1783-2015
Beman J. M., Shih J. L., Popp B. N. (2013). Nitrite oxidation in the upper water column and oxygen minimum zone of the eastern tropical North Pacific Ocean. ISME J. 7, 2192–2205. doi: 10.1038/ismej.2013.96
Billen G., Garnier J. (2007). River basin nutrient delivery to the coastal sea: assessing its potential to sustain new production of non-siliceous algae. Mar. Chem. 106, 148–160. doi: 10.1016/j.marchem.2006.12.017
Cai Z., Li Q., Bai H., Zhu C., Tang G., Zhou H., et al. (2022). Interactive effects of aquatic nitrogen and plant biomass on nitrous oxide emission from constructed wetlands. Environ. Res. 213, 113716. doi: 10.1016/j.envres.2022.113716
Chen Y. B., Song G. B., Zhao W. X., Chen J. W. (2016). Estimating pollutant loadings from mariculture in China. Mar. Envir. Sci. 35, 1–12. doi: 10.13634/j.cnki.mes.2016.01.001
Diab S., Kochba M., Avnimelech. Y. (1993). Nitrification pattern in a fluctuating anaerobic-aerobic pond environment. Water Res. 27, 1469–1475. doi: 10.1016/0043-1354(93)90027-F
Dong Y. W., Dong S. L. (2009). Advances of ecological physiology in sea cucumber, apostichopus japonicus selenka. Period Ocean U. China 39, 908–912. doi: 10.16441/j.cnki.hdxb.2009.05.017
Dou B. X., Huang J. R., Li L. C., Qu H. T., Zhou Q. Y., Li Z. F. (2011). Research on Effects of nutrient and phosphate removal from marine aquaculture system by Sesuvium portulacastru. J. Hydroecol. 32, 94–99. doi: 10.15928/j.1674-3075.2011.05.012
Fan W., Li W. J., Fu G., Zhang Z. L. (2010). Sesuvium portulacastrum L., A promising halophyte in research and application. J. Trop. Subtrop. Bot. 18, 689–695. doi: 10.3969/j.issn.1005-3395.2010.06.017
Hao M. M., Li B., Li H. J., Qiao P., Zhang M. L., Xiang Z. W., et al. (2024). Effects of salinity and nitrogen and phosphorus concentrations on water quality and growth of Sesuvium portulacastrum. Trans. Oceanol. Limnol. 46, 125–133. doi: 10.13984/j.cnki.cn37-1141.2024.02.015
Hoblyn A., Iversen L. L. (2024). Effects on local oxygen conditions by the invasive macrophyte Myriophyllum spicatum. Aquat. Bot. 192, 103739. doi: 10.1016/j.aquabot.2023.103739
Hu J., Liu J., Fan J., Hu Z., Xie H., Wang S., et al. (2017). Effect of salinity on the performance of constructed wetlands treating mariculture wastewater with different halophytes and its molecular biological mechanism. Desalin. Water Treat. 99, 255–265. doi: 10.5004/dwt.2017.21702
Kaenel B. R., Kaenel B. R., Matthaei C. D., Uehlinger U. (1998). Disturbance by aquatic plant management in streams: effects on benthic invertebrates. Regul. Rivers Res. Manage. 14, 341–356. doi: 10.1002/(ISSN)1099-1646
Khoi C. M., Guong V. T., Merckx R. (2006). Predicting the release of mineral nitrogen from hypersaline pond sediments used for brine shrimp Artemia franciscana production in the Mekong Delta. Aquaculture 257, 221–231. doi: 10.1016/j.aquaculture.2006.02.075
Levy A., Milstein A., Neori A., Harpaz S., Shpigel M., Guttman L. (2017). Marine periphyton biofilters in mariculture effluents: Nutrient uptake and biomass development. Aquaculture 473, 513–520. doi: 10.1016/j.aquaculture.2017.03.018
Liu X., Pu X., Luo D., Lu J., Liu Z. L. (2019). Model assessment of nutrient removal via planting Sesuvium portulacastrum in floating beds in eutrophic marine waters: the case of aquaculture areas of Dongshan Bay. Acta Oceanol. Sin. 38, 91–100. doi: 10.1007/s13131-019-1492-5
Lobon-Cervia J., Rezende C. F., CastelLaos C. (2012). High species diversity and low density typify drift and benthos composition in neotropical streams. Fund. Appl. Limnol. 181, 129–142. doi: 10.1127/1863-9135/2012/0242
Ma X. N., Li X., Li J., Ren J. L., Chi L., Cheng X. W. (2021). Iron-carbon could enhance nitrogen removal in Sesuvium portulacastrum constructed wetlands for treating mariculture effluents. Biores. Technol. 352, 124602. doi: 10.1016/j.biortech.2020.124602
Natsumeda T., Takamura N., Nakagawa M., Kadono Y., Tanaka T., Mitsuhashi H. (2015). Environmental and biotic characteristics to discriminate farm ponds with and without exotic largemouth bass and bluegill in western Japan. Limnology 16, 139–148. doi: 10.1007/s10201-015-0453-8
Ni M., Liang X., Hou L. J., Li W. P., He C. Q. (2021). Submerged macrophytes regulate diurnal nitrous oxide emissions from a shallow eutrophic lake: A case study of Lake Wuliangsuhai in the temperate arid region of China. Sci. Total Environ. 811, 152451. doi: 10.1016/j.scitotenv.2021.152451
Peng C., Gao Y., Tan Y., Sheng G., Yang Y., Huang J., et al. (2022). Pollution and release characteristics of nitrogen, phosphorus and organic carbon in pond sediments in a typical polder area of the Lake Taihu Basin. Water 14, 820. doi: 10.3390/w14050820
Pereira D. T., Ouriques L. C., Bouzon Z. L., Simioni C. (2020). Effects of high nitrate concentrations on the germination of carpospores of the red seaweed Pyropia acanthophora var. brasiliensis (Rhodophyta, Bangiales). Hydrobiologia 847, 217–228. doi: 10.1007/s10750-019-04083-2
Planque B., Fromentin J. M., Cury P., Drinkwater K. F., Jennings S., Perry R. I., et al. (2010). How does fishing alter marine populations and ecosystems sensitivity to climate? J. Mar. Syst. 79, 403–417. doi: 10.1016/j.jmarsys.2008.12.018
Posey M. H., Wigand C., Stevenson J. C. (1993). Effects of an introduced aquatic plant, hydrilla verticillata, on Benthic Communities in the upper Chesapeake Bay. Estuar. Coast. Shelf S. 37, 539–555. doi: 10.1006/ecss.1993.1072
Quintã R., Hill P. W., Jones D. L., Santos R., Thomas D. N., LeVay L. (2015). Uptake of an amino acid (alanine) and its peptide (trialanine) by the saltmarsh halophytes Salicornia europaea and Aster tripolium and its potential role in ecosystem N cycling and marine aquaculture wastewater treatment. Ecol. Eng. 75, 145–154. doi: 10.1016/j.ecoleng.2014.11.049
Rosamond M. S., Thuss S. J., Schiff S. L. (2012). Dependence of riverine nitrous oxide emissions on dissolved oxygen levels. Nat. Geosci. 5, 715–718. doi: 10.1038/ngeo1556
Sanchis-Perucho P., Duran F., Barat R., Pachés M., Aguado D. (2018). Microalgae population dynamics growth with AnMBR effluent: effect of light and phosphorus concentration. Water Sci. Technol. 77, 2566–2577. doi: 10.2166/wst.2018.207
Senff P., Blanc P. P., Slater M., Kunzmann A. (2015). Low-technology recirculating aquaculture system integrating milkfish Chanos chanos, sea cucumber Holothuria scabra and sea purslane Sesuvium portulacastrum. Aquac. Environ. Interact. 75, 145–154. doi: 10.3354/aei00377
Silva C. F., Seixas V. C., Barroso R., Di Domenico M., Amaral A. C. Z., Paiva P. C. (2017). Demystifying the Capitella capitata complex (Annelida, Capitellidae) diversity by morphological and molecular data along the Brazilian coast. Plos. One 12, e0177760. doi: 10.1371/journal.pone.0177760
Sinclair J. S., Reisinger L. S., Adams C. R., Bean E., Reisinger A. J., Iannone B. V. (2021). Vegetation management and benthic macroinvertebrate communities in urban stormwater ponds: implications for regional biodiversity. URBAN Ecosyst. 24, 725–735. doi: 10.1007/s11252-020-01072-5
Smith D. W., Horne A. J. (1988). Experimental measurement of resource competition between planktonic microalgae and macroalgae (seaweeds) in mesocosms simulating the San Francisco Bay-Estuary, California. Hydrobiologia 159, 259–268. doi: 10.1007/BF00008239
Tayaban K. M. M., Pintor K. L., Vital P. G. (2018). Detection of potential harmful algal bloom-causing microalgae from freshwater prawn farms in Central Luzon, Philippines, for bloom monitoring and prediction. Environ. Dev. Sustain. 20, 1134–1328. doi: 10.1007/s10668-017-9942-8
The State of World Fisheries and Aquaculture (2022). Available online at: https://www.fao.org/family-farming/detail/ru/c/1565527/ (accessed 2024/5/15).
Unmuth J. M. L., Lillie R. A., Dreikosen D. S., Marshall D. W. (2000). Influence of dense growth of Eurasian watermilfoil on lake water temperature and dissolved oxygen. J. Freshwater Ecol. 15, 497–503. doi: 10.1080/02705060.2000.9663772
Wang J. P., Cui Z. G., Zhou Q., Ma S. S., Qu K. M., Mao C. Q. (2014). Removal effect of mariculture wastewater and analysis of microbial communities in constructed wetlands. Prog. Fish. Sci. 35, 1–9. doi: 10.11758/yykxjz.20140601
Wang Z. H., Liu K. (2023). Nutrients transport behavior in Inlet River in the Yellow River Delta in winter. Mar. Pollut. Bull. 197, 115815. doi: 10.1016/j.marpolbul.2023.115815
Wu H. P., Hao B. B., Cai Y. P., Liu G. H., Xing W. (2021). Effects of submerged vegetation on sediment nitrogen-cycling bacterial communities in Honghu Lake (China). Sci. Total Environ. 755, 142541. doi: 10.1016/j.scitotenv.2020.142541
Ying R., Chen J. F., Gao S. S., Li Z. F., Feng J. X. (2018). Single and synergistic effects of Ulva lactuca and Sesuvium portulacastrum on the purification of mariculture wastewater. Chin. J. Ecol. 37, 2745–2753. doi: 10.13292/j.1000-4890.201809.014
Zeng D., Lin F. M., Wu G. Y., Hu L. Q., Shen K. J., Zhou C. Y., et al. (2019). Mariculture wastewater treatment by integrated process of hybridmesh, continuous flow sand filter, and activated carbon adsorption. China. Water Wastewater 35, 97–102. doi: 10.19853/j.zgjsps.1000-4602.2019.16.020
Zhang Y. S., Yang Q. L., Chen R. X. (2007). Specifications for oceanographic survey - Part 6: Marine biological survey. (GB/T 12763.6-2007). Available online at: https://std.samr.gov.cn/gb/search/gbDetailed?id=71F772D788FDD3A7E05397BE0A0AB82A (accessed 2023/04/13).
Zhang Y. Y., Yu S. E., Liao M. L., Dong Y. W. (2022). Evaluation and prediction of the effects of extreme high temperatures on sea cucumber (Apostichopus japonicus) pond aquaculture in China. J. Fish. Sci. China 29, 408–420. doi: 10.12264/JFSC2021-0376
Zhang Z., Lo I. M. C., Zheng G. Y., Woon K. S., Rao P. H. (2015). Effect of autotrophic denitrification on nitrate migration in sulfide-rich marine sediments. J. Soil Sediment 15, 1019–1028. doi: 10.1007/s11368-015-1078-6
Keywords: Sesuvium portulacastrum, reduction, inorganic nitrogen, mariculture, pond water
Citation: Liu K, Gao W, Yu Z, Hu Y, Zuo M, Sun C, Zou X and Wang L (2024) The effect of Sesuvium portulacastrum for reducing inorganic nitrogen pollution in coastal mariculture wetland. Front. Mar. Sci. 11:1460272. doi: 10.3389/fmars.2024.1460272
Received: 05 July 2024; Accepted: 21 August 2024;
Published: 19 September 2024.
Edited by:
Qing Wang, Beijing Normal University, ChinaReviewed by:
Jia Jia, Yellow River Institute of Hydraulic Research, ChinaZhengyi Liu, Chinese Academy of Sciences (CAS), China
Copyright © 2024 Liu, Gao, Yu, Hu, Zuo, Sun, Zou and Wang. This is an open-access article distributed under the terms of the Creative Commons Attribution License (CC BY). The use, distribution or reproduction in other forums is permitted, provided the original author(s) and the copyright owner(s) are credited and that the original publication in this journal is cited, in accordance with accepted academic practice. No use, distribution or reproduction is permitted which does not comply with these terms.
*Correspondence: Kai Liu, a2xpdUB5aWMuYWMuY24=