- 1Department of Applied Ecology and Phycology, Institute of Biological Sciences, University of Rostock, Rostock, Germany
- 2Guest Science Programme, Biologische Anstalt Helgoland, Alfred-Wegener-Institut Helmholtz-Zentrum für Polar- und Meeresforschung, Helgoland, Germany
- 3Department of Earth Observation and Modelling, Institute of Geography, Christian-Albrechts University of Kiel, Kiel, Germany
- 4Department of Maritime Systems, Interdisciplinary Faculty, University of Rostock, Rostock, Germany
- 5Alfred Wegener Institute, Helmholtz-Centre for Polar and Marine Research, Bremerhaven, Germany
- 6Department of Ecology, Faculty of Sciences, University of Malaga, MƔlaga, Spain
Reliable net primary production (NPP) estimations of kelp forests are important to evaluate their C-fixation potential. Photosynthetic oxygen measurements can be converted to C-fixation using photosynthetic quotients (PQs). Although there is a known high variability in PQs, the extent and the consequences for NPP is understudied in kelp species. Thus, the present study aimed (i) to quantify the variability of PQs, (ii) to model NPP and (iii) to assess the impact of warming on both. The kelp, Laminaria hyperborea, was studied near the island of Helgoland (North Sea, Germany) along a depth gradient (2, 4, 6 m below mean low water spring tide) across all four seasons. Blade discs were cultivated during at least 6 days per season under simulated ambient photosynthetic photon flux density (PPFD) and temperature conditions and, in parallel, in a warming scenario (+ 4°C). PQs were calculated from parallel oxygen production and 14C-fixation measurements at saturating PPFD at the end of the incubation period. Seasonal PQs varied between 1.7 and 4.4, with highest values in summer due to increased oxygen production. The warming scenario stimulated C-fixation in most seasons, lowering the PQ in comparison to ambient temperature conditions, while collection depth had no significant effect on PQs. The seasonal PQs were used to model daily NPP rates for kelp standing stock at 4 m depth. These daily NPP rates were compared between temperature treatments and with daily NPP rates based on fixed PQs. The warming scenario had a stimulating effect on daily NPP rates in the high-light season spring. In the low-light season autumn, warming resulted in negative daily NPP rates, as the high respiration rates could not be compensated by gross photosynthesis. Overall, annual NPP rate under warming conditions (347 g C mā2 yrā1) was 14% higher than the annual NPP rate under ambient conditions (303 g C mā2 yrā1). Modelling daily NPP with fixed PQs, which neglects the seasonal variation of the PQs, led to a high overestimation of up to 255%. We, therefore, recommend modelling NPP rates not with a fixed PQ, but with seasonal PQs determined under different temperature scenarios in order to obtain reliable future predictions.
1 Introduction
By definition, net primary production (NPP) is the total light-dependent carbon assimilated by a given area or biomass in a given period, minus carbon losses (e.g. respiration). Global NPP takes place approximately equally in marine and terrestrial ecosystems, representing a main driver of the biospheric carbon cycle (Kaiser et al., 2020). Phytoplankton contributes approximately 90% of the oceanic NPP, while other communities (e.g. coral reefs, macroalgal forests, seagrass beds and mangroves) make up 10% (Duarte and CebriƔn, 1996). Rough estimations indicate that the marine coastal vegetation is responsible for about half of the carbon burial in the ocean (coastal and global), although they only cover about 2% of the ocean surface (Duarte and CebriƔn, 1996; Duarte et al., 2005). Lately, attention has focused on the contribution of coastal marine habitats, especially kelp forests, to the global carbon budget (e.g. Duarte et al., 2005; Duarte, 2017). The impact of warming on the photosynthetic activity (Burdett et al., 2019; Smale et al., 2020; Franke et al., 2024), the carbon sequestration potential (Pessarrodona et al., 2018; Wright et al., 2022), and distribution ranges (Smale, 2020) of kelps has been extensively studied. However, it is difficult to predict annual NPP estimates of kelps because the seasonal pattern of photosynthetic activity in general and under warming scenarios is not well studied.
Kelp forests and seaweed beds are highly productive coastal ecosystems with an estimated NPP between 197 and 1711 g C m-2 yr-1 (Smale et al., 2020; Pessarrodona et al., 2022a). Most studies estimate NPP of kelps indirectly from standing stock or biomass accumulation (Smale et al., 2020), while photo-respirometry in terms of oxygen production and isotopic carbon assimilation (e.g. O2 and 13C: Miller et al., 2009; O2 and 14C: IƱiguez et al., 2016a, b) provides a direct way to physiologically estimate ātrueā NPP (Pessarrodona et al., 2022b). NPP in units of fixed carbon can be measured directly or converted from units of released oxygen using a known photosynthetic quotient (PQ). The PQ is a molar ratio to convert gross oxygen photosynthesis into assimilated carbon during photosynthesis (IƱiguez et al., 2016a). While PQ values for many algal species are largely unknown, the few available data show high species-specific variability (Buesa, 1980), or even differ within the same species, due to differences in environmental factors such as temperature (Hatcher et al., 1977; IƱiguez et al., 2016b), nutrient availability (Trentman et al., 2023), depth (Miller et al., 2009), and light availability (Hatcher et al., 1977; Weykam et al., 1997). In most studies that deal with macroalgal PQ values, samples were either collected only in one season and experiments were conducted under different levels of abiotic factors (e.g. IƱiguez et al., 2016a, b), or samples were collected at different seasons and experiments were performed only under current environmental conditions (e.g. Hatcher et al., 1977; Weykam et al., 1997). The setup for direct carbon assimilation measurements is expensive and time consuming, in addition to the risks associated with radioisotopic methods. Therefore, to date, conversions of O2 production rates to NPP rates have mostly been performed with a theoretical PQ of around 1 (e.g. Fairhead and Cheshire, 2004; Miller et al., 2011; Rodgers and Shears, 2016), which is insufficient to describe the complex relationship between O2 release and C-fixation (Trentman et al., 2023). The O2 signal derives from the early steps in the photosynthesis process, i.e. cleavage of water at photosystem II, while the C-fixation signal originates from the late carboxylation in the Calvin cycle (Taiz and Zeiger, 2002). Many enzymatic steps are involved in both processes, and their susceptibility to environmental stressors can shift the ratio between oxygen production and carbon fixation. PQ values mainly depend on the photosynthetic end products (Norici et al., 2011; Palmucci et al., 2011), photorespiration (Stewart, 1974; Pokorny et al., 1989), and nitrate or ammonium assimilation (Williams et al., 1979; Bell and Kuparinen, 1984; Smith et al., 2012).
The kelp that contributes most biomass in the rocky mid-sublittoral along the European coastline is Laminaria hyperborea (Gunnerus) Foslie (Smale et al., 2020). This species forms extensive forests from northern Portugal (Araujo et al., 2005) to northern Norway (Kylin, 1947; Lüning, 1990). Climate change is already threatening this unique ecosystem, leading to biomass and range contractions and even to local extinctions at its southern distribution edge (Voerman et al., 2013; PiƱeiro-Corbeira et al., 2016; Casado-AmezĆŗa et al., 2019). In the German Bight, the rocky shore off the island of Helgoland is covered by a dense L. hyperborea forest along a depth gradient between 1 and 6 m with a maximum depth of occurrence of single individuals of down to 12.5 m (Pehlke and Bartsch, 2008). Helgolandic L. hyperborea is exposed to a wide range of monthly mean sea surface temperatures between 4.3°C and 19.0°C annually (Bio-ORACLE v.3.0: Tyberghein et al., 2012; Assis et al., 2024). This temperature range corresponds to the temperature requirements of L. hyperborea over most of its distribution range (e.g. Lauksletta, Norway: 3.8° ā 11.5°C; Vila Praia de Ćncora, Portugal: 12.6° ā 17.9°C; Bio-ORACLE v.3.0: Tyberghein et al., 2012; Assis et al., 2024). Future predictions for Helgoland assume a maximum monthly sea surface temperature of 19.9° and 22.5°C for 2050 and 2100, respectively (SSP585; Bio-ORACLE v.3.0: Tyberghein et al., 2012; Assis et al., 2024). Although this scenario is the most extreme and rather unlikely (Burgess et al., 2023), it exceeds the two-week lethal limit temperature for Helgolandic L. hyperborea (20-21°C; tom Dieck, 1992). However, no study has yet been conducted in which temperature was manipulated during all four seasons in order to assess the consequences of warming on seasonal photosynthetic activity. The Helgolandic L. hyperborea forest provides a well-suited model ecosystem to study the year-round variation of PQ values under ambient and warming scenarios.
The main objectives of the present study were (i) to quantify the seasonal variability of PQ values and (ii) its influence in NPP modelling; and (iii) to assess the effect of a warming scenario on future NPP. We hypothesise that (1) in summer, the excess of photosynthetic photon flux density (PPFD) maximises PQ values; (2) along the depth gradient, decreasing PPFD availability leads to lower PQ values as most of the photosynthetic energy produced will be invested in carbon fixation; (3) warming increases NPP and decreases PQ values, especially in spring when PPFD already saturates O2 production and temperature rise promotes enhanced carbon fixation rates.
2 Materials and methods
2.1 Study site and algal material
Adult Laminaria hyperborea sporophytes were collected along a depth gradient measured at the holdfast (2, 4, 6 m below mean low water spring tide [MLWS]) near the island of Helgoland (North Sea, Germany; 54.1881° N, 7.8699° E) in all four seasons between spring 2021 and winter 2022 (Figure 1, Supplementary Table S1). For logistical reasons, no 2-m algae could be sampled in summer. For details on sampling criteria and material transport, see Franke et al. (2024).
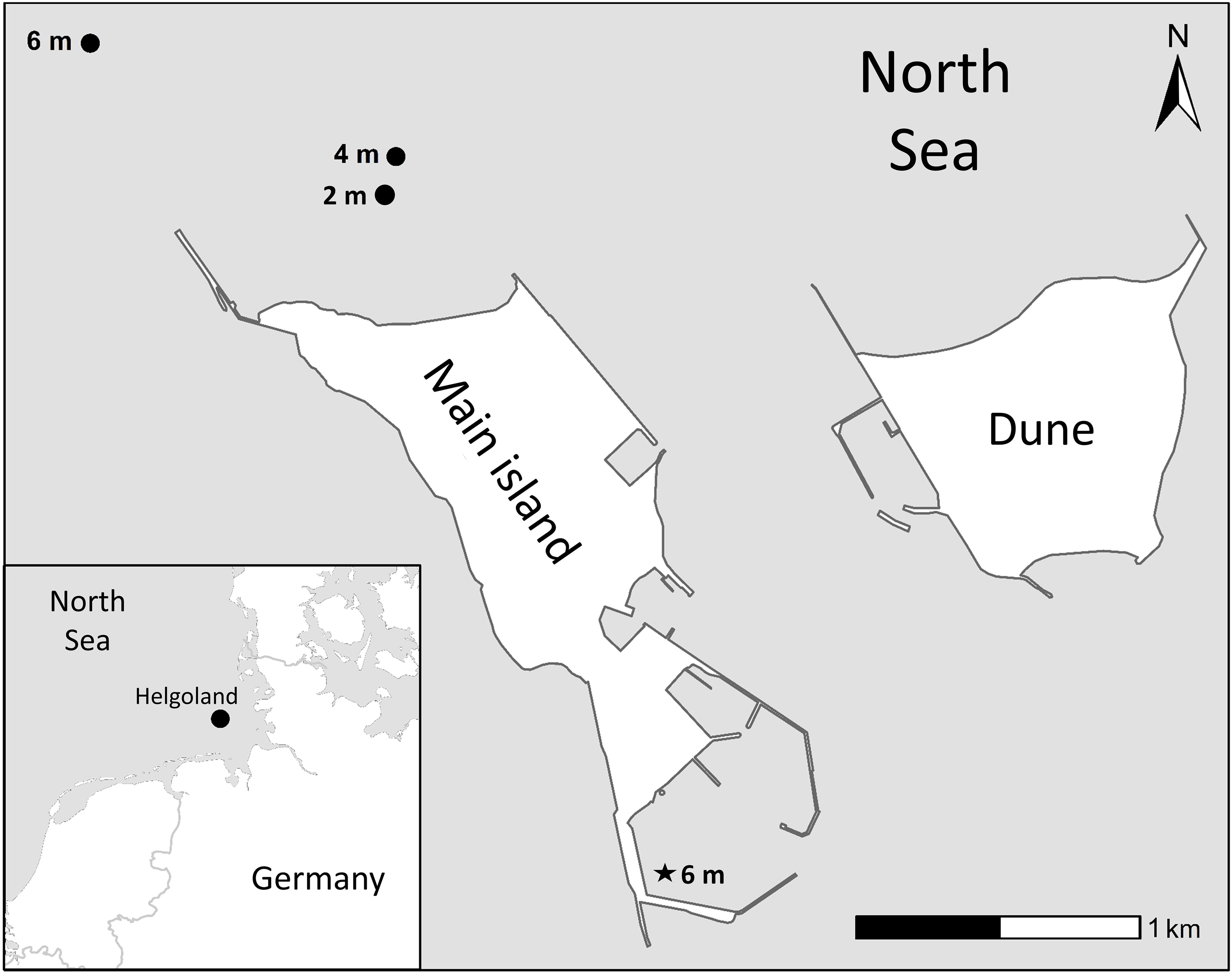
Figure 1. Study site in the north-western rocky sublittoral of the main island off Helgoland (North Sea, Germany; 54.1881° N, 7.8699° E). Black circles: locations of the Laminaria hyperborea station (depths in mean low water spring tide); Black star: 6 m station during winter due to harsh weather conditions. More information is provided in Supplementary Table S1.
In the laboratory (Dummermuth et al., 2023), blade discs were cut from the meristem and incubated in two temperature scenarios before oxygen and carbon fixation were measured after six days (Supplementary Figure S1).
To measure oxygen production and carbon fixation, blade discs (Ć 2 cm) and rectangular blade pieces (1.5Ć0.8 cm) were cut out using custom-made cork borers (see Supplementary Figure S1). In spring, summer and autumn, material was cut at a 5 cm distance above the stipe-blade transition zone (vital meristematic tissue) as only one blade was present (Supplementary Figure S2A). In winter, algal material was cut from the old blade at a distance of 5 cm above the isthmus zone, as the new blades were not yet large enough for our sampling procedure. Franke et al. (2023) showed that photosynthesis did not change along the thallus of L. hyperborea if data were referred to blade area; therefore, the meristem is representative for most of the thallus. In total, 18 individuals were pooled into six replicates (n=6 per temperature treatment and depth; Franke et al., 2024). From three individuals, blade discs (four discs per individual) and rectangular blade pieces (one piece per individual) were cut and evenly distributed between the two temperature treatments (ambient and a warming scenario of + 4°C for each season).
2.1.1 Laminaria hyperborea areal coverage survey
A concomitant vegetation survey of L. hyperborea areal coverage was conducted in July 2021, in order to relate productivity rates to kelp leaf area. Kelp individuals were harvested by SCUBA divers in 1 m² quadrats at three different depths measured at the holdfast (2, 4, 6 m below MLWS; n=5 in 2 and 4 m, n=10 in 6 m), resulting in a total of 20 quadrats (Table 1).
2.2 Experimental setup
The discs and rectangular pieces were cultivated for six days (Supplementary Figure S1). In spring, 6 m algae were cultivated for 7 days and in summer for 13 days for logistical reasons. The values from longer cultivation periods were taken into account because they were in the same range as other values determined in the same season. Algae were cultivated in aerated natural North Sea water (0.2 µm filtered) in plastic beakers (1.6 L) without the addition of nutrients. The seawater was changed after one night of wound healing and thereafter every three days. The seasonal ambient temperature conditions were averaged as in situ water temperature data measured at 10 m depth (MLWS) between 2016 and 2020 (Data Publisher for Earth & Environmental Science; Fischer et al., 2021a, b, c, d, 2022). For the warming treatment, the ambient temperature was elevated by + 4°C over two days in 2°C steps per day for each season according to Franke et al. (2024, Table 2). The warming treatment was chosen based on the SSP3-7.0 scenario presented in the IPCC report 2023 which estimated an increase by 4°C by the end of the century relative to 1850-1900 (Lee et al., 2023). Both temperatures were maintained at ±0.02°C in water baths fitted with thermostats (DC10 and D3, Thermo Haake) in a constant-temperature room. Light:dark photoperiods and PPFD were adjusted to the respective season and depth (LED Mitras lightbar daylight 150 controlled by ProfiLux 3, GHL Advanced Technology). Daily mean PPFD was calculated based on incoming photon flux density (PFD) measurements at Helgoland between 2014 and 2017 performed by the Helmholtz-Zentrum Hereon (https://codm.hzg.de/codm/) and vertical attenuation coefficients from the water column around Helgoland (Lüning and Dring, 1979) for each depth separately (2, 4 and 6 m MLWS) according to Franke et al., 2024, Table 2).
2.3 Chlorophyll a fluorescence
The maximum quantum yield of in vivo chlorophyll a fluorescence of photosystem II (Fv/Fm) of dark-acclimated (5 min) L. hyperborea discs was measured using pulse amplitude modulated (PAM) fluorometry (PAM 2000, Walz) as described by Schreiber et al. (1986). These measurements were performed prior to the oxygen production incubations to verify that the discs were in a vital physiological state (brown algae: 0.7ā0.8; Hanelt, 2018). The duration of the dark acclimation was tested previously and no significant difference in Fv/Fm values was observed between 5, 10, 15 and 20 min (data not shown).
2.4 Oxygen production
On the 6th day (except: 6 m in spring at day 7 and in summer at day 13), net photosynthetic rates under saturating PPFD levels (Supplementary Table S2), along with dark respiration rates, were measured using an oxygen optode system (Fibox 3, PreSens) following the set-up and method of Franke et al. (2023). The saturating PPFD levels were derived from photosynthesis versus irradiance (P-I) curves performed in each season on day 0 using a custom-made light bar with white LEDs (LUXEON Rebel, Model: LXML-PWN1-0100, Philips) in combination with Schott neutral grey filters (data presented in Franke et al., 2024; Supplementary Table S2). Oxygen production rates were measured in a constantly stirred circular airtight acrylic glass chamber in a constant-temperature room at the respective cultivation temperature according to Franke et al. (2023, Table 2; Supplementary Figures S2B, C). First, the discs were acclimated to darkness for 20 mins and the dark respiration rate was measured during 10 min in the dark. This was followed by the determination of the net photosynthetic rate during a 10-minute exposure to the respective seasonal, depth-dependent, saturating PPFD value to measure maximum photosynthetic activity (Supplementary Table S2). Oxygen concentration was logged by the OxyView software (Presens) and corrected for air pressure, salinity and logged temperature according to Tengberg et al. (2006). During post-processing, the oxygen production rate was normalised to disc area (unit: µmol O2 cmā2 hā1). Disc area was determined after the oxygen measurements via image analysis (Image J: Rasband, W.S., U. S. National Institutes of Health, Bethesda, 1997ā2023).
2.5 Inorganic 14C-fixation
On the 6th day (except: 6 m in spring at day 7 and in summer at day 13), the algal samples were transferred to 8-mL septum-sealed glass vials filled with sterile autoclaved seawater (pre-aerated for approximately 24 h). The glass vials were placed in a custom-made transparent acrylic water bath connected to a thermostat (DC10 and V26, Thermo Haake) keeping the incubation temperature (Table 2). The medium in the glass vials was stirred continuously to reach homogenisation. The six replicates were distributed into four light (Clight fixation; n=4) and two dark (Cdark fixation; n=2) samples. A pre-acclimation period of 15 min in light for reaching steady-state photosynthesis or 15 min in darkness for the dark controls was applied before injecting the H14CO3ā spiked medium (Perkin Elmer). From the H14CO3ā stock solution, 30 µl (~3 µCi mlā1) were injected into each 8-mL septum-sealed glass vial. After injection of the H14CO3ā solution, the L. hyperborea tissue pieces were either incubated at seasonal, depth-dependent, saturating PPFD to achieve maximum photosynthetic rates (Supplementary Table S2) or in darkness (as a control of light-independent C-fixation) for 20 min. Incident PPFD was provided by a custom-made light bar with white LEDs (CREE white light LED: CXA1304/C00A20E8, CreeLED, Inc.; Supplementary Figures S2D, E). After the incubation period, algae were immediately rinsed in unlabelled medium, blotted dry, submersed in liquid nitrogen to stop reactions and settled in 20-ml scintillation vials containing 400 µL of 68% HNO3 (Roth). After 10 min in a mini oven (BG 950, Rommelsbacher) at 50°C, vials were left on an orbital shaker inside a fume hood for approximately 24 h. The procedure was based on the protocol of IƱiguez et al. (2016a).
The next day, after the addition of 15 ml of scintillation cocktail (Insta-gel Plus, Perkin Elmer) and 4 mL MiliQ water, the equivalent C-fixation rates were determined by measuring acid-stable 14C-fixation using a liquid scintillation counter (TriCarb 2910, Perkin Elmer). Additionally, the automatic quench and colour correction of the scintillation counter was used. The C-fixation rates were related to the dissolved inorganic carbon content of the incubation medium. Total alkalinity was determined by potentiometric titrations according to Gran (1952) and for details see Supplementary Materials. Activity levels of dark controls (light-independent C-fixation = Cdark fixation) were subtracted from light C-fixation (Clight fixation) measurements (Supplementary Table S3) to gain the light-dependent gross C-fixation (Cgross fixation) rate. For the calculation of the C-fixation rate, it was further assumed that the uptake of 14C is 5% slower than of 12C (based on Steeman-Nielsen, 1952).
2.6 Photosynthetic quotient
The photosynthetic quotient (PQ) is the molar ratio of the rate of gross oxygen production (Ogross production) to the rate of light-dependent Cgross fixation. Both rates were calculated as follows:
where Ogross production is calculated by subtracting negative dark respiration from net oxygen production under saturating PPFD (Onet production), and light-dependent Cgross fixation is calculated by subtracting light-independent C-fixation measured in darkness (Cdark fixation) from C-fixation under saturating PPFD (Clight fixation). PQ values were calculated as mean and standard deviation according to:
2.7 Leaf area index
Leaf Area Index (LAI) was determined for L. hyperborea by taking digital photographs (Nikon Coolpix L110) of each individual with a calibration area. Subsequently, the blade area was quantified via image analysis (ImageJ: Version 1.53k, 2021) and LAI was calculated. The summer LAI was used for the following annual NPP modelling. Jupp and Drew (1974) found no difference between LAI in Scottish L. hyperborea from different seasons, while Lüning (1971) obtained a peak in blade area in single individuals of Helgolandic L. hyperborea in May-July. Therefore, the NPP rates were presumably modelled with the maximum possible LAI for this location.
2.8 Modelling of net primary production
Annual NPP rates were calculated for L. hyperborea lamina standing stock per m² at 4 m depth as L. hyperborea off Helgoland has the highest LAI at this depth (Pehlke and Bartsch, 2008; present study). Following the approach of Deregibus et al. (2016), daily Ogross production of 4-m L. hyperborea was calculated by correlating the P-I curve parameters obtained in vitro (presented in Franke et al., 2024) with continuous in situ PPFD data at 4 m calculated from incoming solar PFD data between 01.01.2020 00:00 and 31.12.2020 23:50 (measured in 10 min intervals; unit: J cmā2; Helgoland; DWD station-ID: 2115, https://cdc.dwd.de). Data were converted to PPFD (unit: µmol photons mā2 sā1) as follows:
The unit Joule is converted into µmol photon with the conversion factor 4.6 (Lange et al., 1981) and only half of the available PFD is usable for PPFD (Kirk, 1994). PPFD available at the holdfast depths Id(4 m) was estimated, including the monthly vertical attenuation coefficient Kd(PPFD) in mā1 for the water column above 4 m around Helgoland (Lüning and Dring, 1979). The PPFD at 4 m depth was averaged per hour. Daily oxygen production rates were calculated with P-I parameters, PPFD at 4 m and the model proposed by Jassby and Platt (1976):
where P is the Ogross production rate (µmol O2 cmā2 hā1), at a given I (PPFD, µmol photons mā2 sā1). The maximum oxygen production rate is Pmax (µmol O2 cmā2 hā1), α (µmol O2 cmā2 hā1 (µmol photons mā2 sā1)ā1) is the light utilisation coefficient, and R (µmol O2 cmā2 hā1) is the initial dark respiration rate. Gross oxygen production rate (µmol O2 cmā2 hā1) was converted into mol O2 cmā2 hā1, and then, into C-fixation rates using the molar weight of carbon of 12.011 g molā1 and the seasonal PQ values of 4-m L. hyperborea according to Franke et al. (2023).
Next, the hourly NPP was summed over a 24-h period to calculate daily NPP (g C cmā2 dā1). Finally, the daily NPP per m² seafloor (g C mā2 seafloor dā1) was estimated by multiplying the production rate per cm² by the mean in situ LAI determined in July 2021 (Table 1). The seasonal NPP rates were calculated by summing the daily NPP rates for each season (each three months) and annual NPP rates were calculated by summing the daily NPP rates for each temperature treatment. For comparative purposes, seasonal NPP rates were calculated using the P-I curve parameters (Supplementary Table S5) and PQ values obtained from samples exposed to ambient and warming conditions, expressed as NPPambient and NPPwarming, respectively. For comparative purposes, seasonal NPP rates were also calculated using a literature PQ of 1.18 determined by Miller et al. (2009) for L. hyperborea and a mean PQ of 3.24 (mean of the four seasonal PQ values for 4-m sampled L. hyperborea), expressed as NPPMiller and NPPmean, respectively.
2.9 Statistical analysis
Data were analysed using the open-source statistical program R (R Core Team, 2023).
Chlorophyll a fluorescence, oxygen production (Onet & Ogross production & respiration rate), Cgross fixation and PQ values: First, technical outliers were excluded from data using the R package āperformanceā (version 0.10.5; Lüdecke et al., 2021). The Shapiro-Wilk-Test (normality) and Leveneās test (homogeneity of variance) were applied. If the assumptions were met, analyses of variance (ANOVAs) were applied with depth, temperature (categories: ambient and warming) and season as fixed factors; if the assumption of homogeneity was not met the significance level was lowered (alpha <0.01) to reduce the probability of false positive reports (Curran-Everett, 2017). Tukey HSD post-hoc tests were performed when significant differences were found. For the statistical analysis of Cgross fixation and PQ values, a data set was generated in R (R-script in Supplementary Material) based on the mean, standard deviation and replicate size.
Leaf area index: For LAI, a non-parametric Kruskal-Wallis ANOVA and a Dunnās test with Bonferroni-correction (alpha <0.0125) for comparison between different depths were applied.
Net primary production: Intra-seasonal comparison: Daily NPP rates (NPPwarming, NPPMiller, NPPmean) were compared to the daily NPPambient rate in separate, non-parametric Wilcoxon tests for each season and over all seasons. Inter-seasonal comparison: Seasonal effects within each daily NPP rate category (NPPwarming, NPPMiller, NPPmean, NPPambient) were tested with non-parametric Kruskal-Wallis ANOVAs and pairwise Wilcoxon tests with Bonferroni-correction (alpha <0.0083).
3 Results
3.1 Chlorophyll a fluorescence
After six days of culture, spring, autumn and winter samples of Laminaria hyperborea showed Fv/Fm values of 0.76 ± 0.07, reflecting a physiologically healthy state with no significant difference (Figure 2). In summer, the Fv/Fm values exhibited a wide range from 0.19 to 0.81, on average 12% lower than the average of all other seasons, resulting in a significant seasonal effect (Tukey test: p<0.001; Table 3). However, there were neither significant effects of the single factors temperature and depth nor interactions of all three fixed factors depth, temperature and season (Table 3).
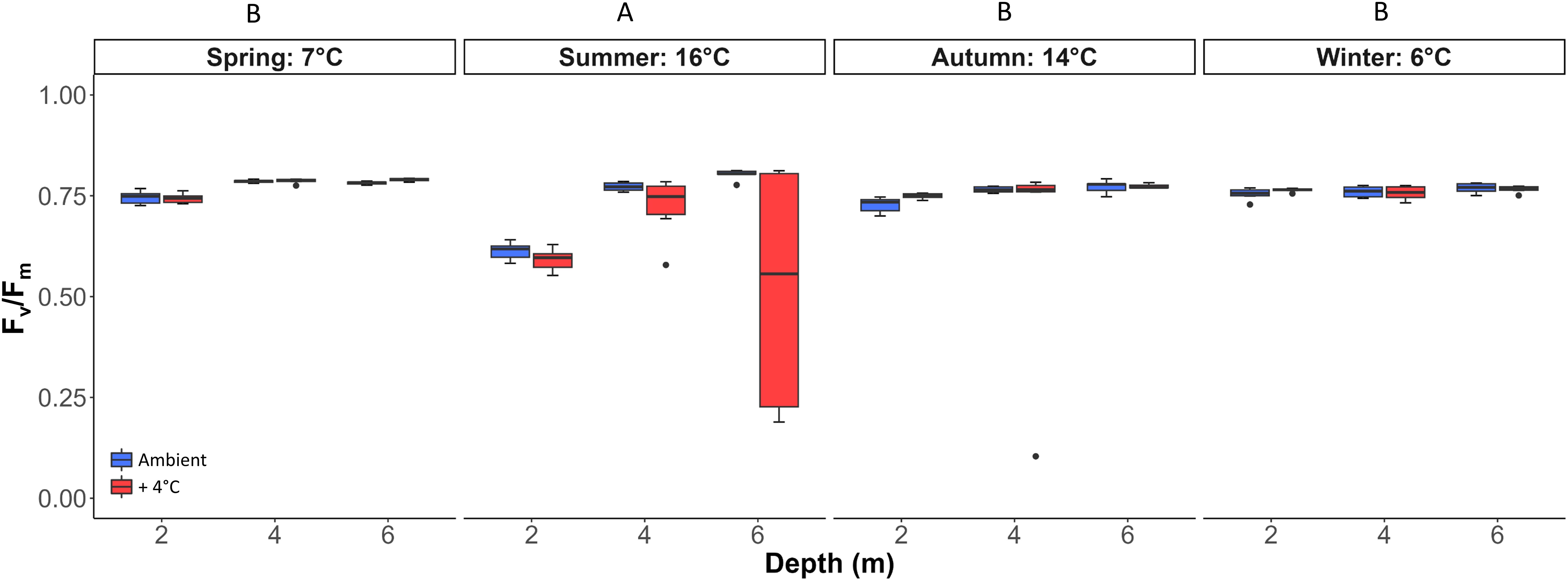
Figure 2. Chlorophyll a fluorescence maximum quantum yield (Fv/Fm) of Laminaria hyperborea after six days of culture at two different temperature treatments (blue: ambient temperature, red: ambient + 4°C as warming scenario). Horizontal lines represent the median; Boxes, the interquartile range; Whiskers, 1.5 à of inter-quartile range. Capital letters show inter-seasonal differences (Statistical results: Table 3; Tukey test: p<0.01; n=6).
3.2 Oxygen evolution, carbon fixation and PQ
Oxygen evolution: The main factor season had a significant effect on all measured parameters (Table 3). In summer, net oxygen production was significantly higher (0.51 ± 0.27 µmol O2 cmā2 hā1) than in autumn (0.25 ± 0.27 µmol O2 cmā2 hā1) and winter (0.30 ± 0.19 µmol O2 cmā2 hā1), while Onet production was intermediate in spring across all depths and temperature treatments (Figure 3A, Supplementary Table S4). Respiration was comparable in spring, summer and autumn (average over three seasons: -0.41 ± 0.24 µmol O2 cmā2 hā1), but was significantly lower in winter (-0.17 ± 0.09 µmol O2 cmā2 hā1; Figure 3B, Table 3; Tukey test: p<0.001). The pattern of oxygen production in the different seasons was reflected by high Ogross production in spring and summer (average over both seasons: 0.87 ± 0.27 µmol O2 cmā2 hā1) and significantly lower rates in autumn (0.65 ± 0.21 µmol O2 cmā2 hā1) and winter (0.47 ± 0.20 µmol O2 cmā2 hā1) across all depths and temperature treatments (Table 3, Tukey test: p<0.001; Figure 4A). The opposing effects of the single factor temperature on Onet production and respiration resulted in a compensation of the calculated Ogross production, and the single factors temperature and depth had no effect on Ogross production (Table 3). Net oxygen production was 31% higher under warming than under ambient conditions (0.31 ± 0.21 µmol O2 cmā2 hā1) in all seasons and depths (Table 3; Tukey test: p<0.004), while respiration was significantly decreased to -0.30 ± 0.14 µmol O2 cmā2 hā1 under warming (ambient: -0.37 ± 0.20 µmol O2 cmā2 hā1; Table 3; Tukey test: p<0.003). Sampling depth significantly affected respiration rates, as 6-m algae had 21% lower respiration (-0.29 ± 0.14 µmol O2 cmā2 hā1) than 4-m algae (-0.37 ± 0.19 µmol O2 cmā2 hā1), while respiration of 2-m algae was comparable to that of 4-m algae (-0.37 ± 0.19 µmol O2 cmā2 hā1; Figure 3B; Table 3; Tukey test: p<0.004).
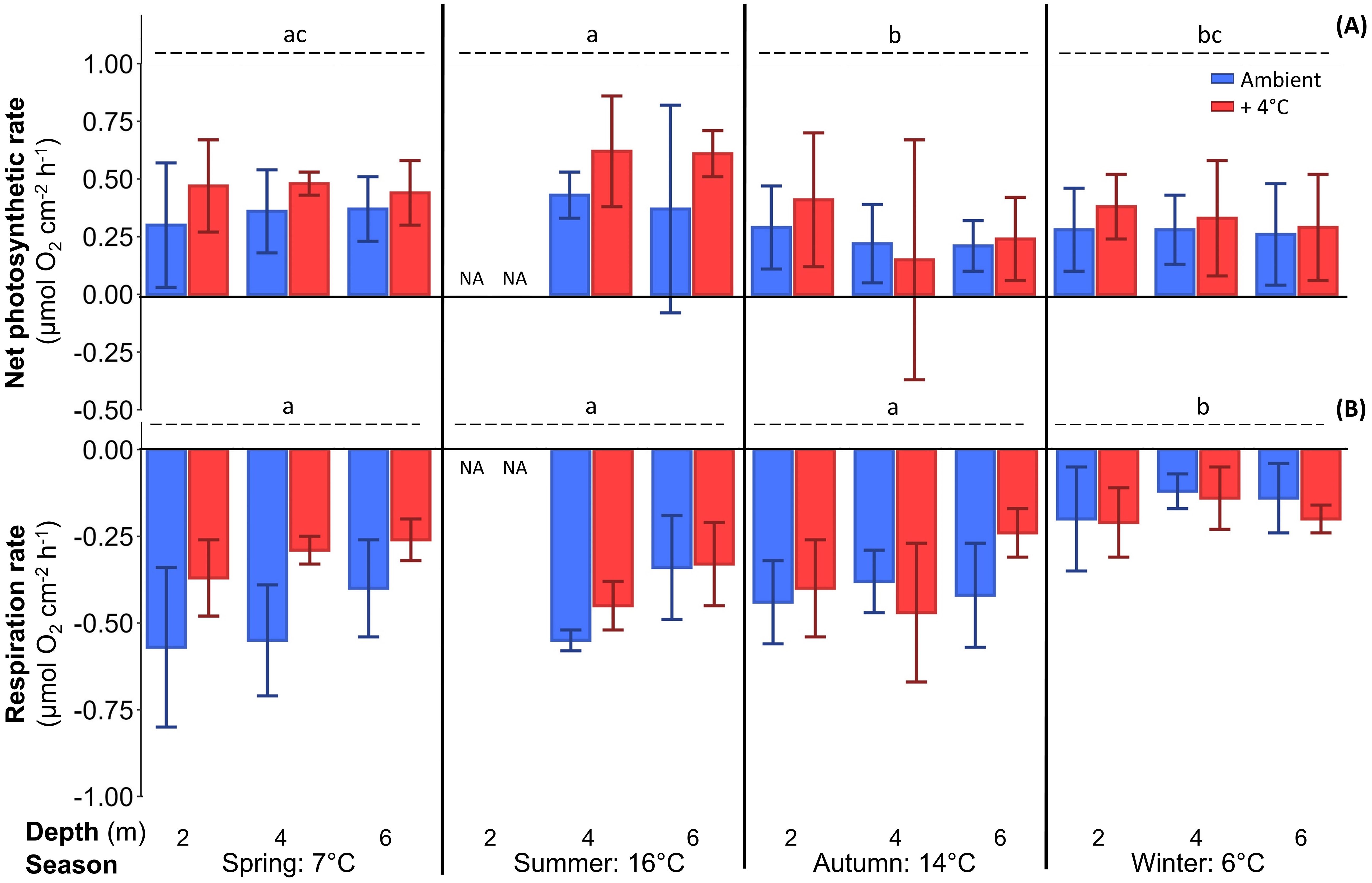
Figure 3. Net photosynthetic rate under saturating light for photosynthesis (A) and respiration rate (B), measured as oxygen evolution, of Laminaria hyperborea after at least six days of cultivation at two temperatures (blue: ambient, red: ambient + 4°C as warming scenario). Lower case letters indicate inter-seasonal differences (further statistical results: Table 3; mean ± SD; n=6).
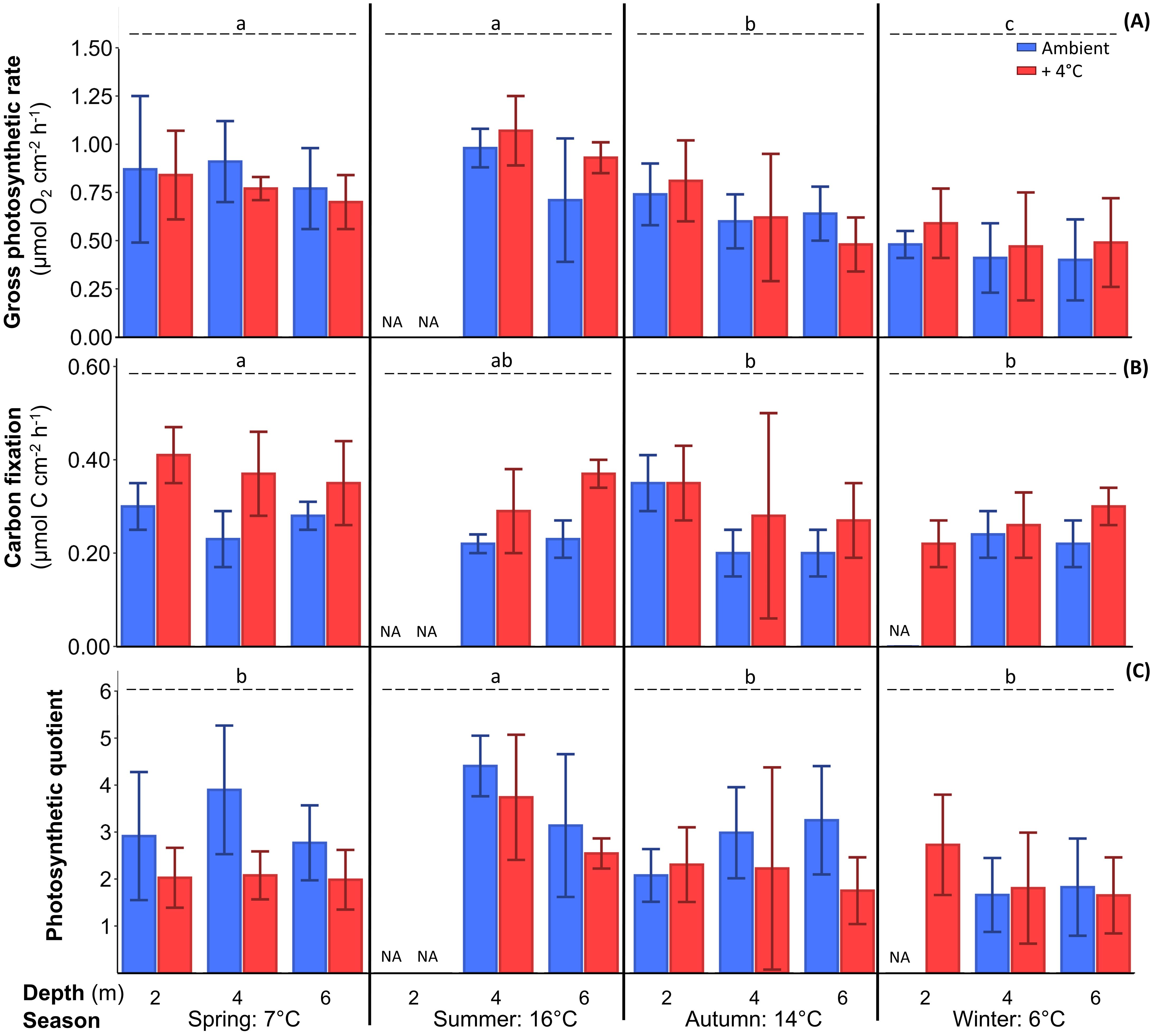
Figure 4. Gross photosynthetic rate (A) measured as oxygen production, gross carbon fixation rate (B) measured as light-dependent 14C fixation, and photosynthetic quotient (C) of Laminaria hyperborea after at least six days of cultivation at two temperatures (blue: ambient, red: ambient + 4°C as warming scenario). C-fixation and oxygen production were measured under saturating light for photosynthesis. Lower case letters indicate inter-seasonal differences (further statistical results: Table 3; Tukey test: p<0.01; mean ± SD; n=6).
The only significant interaction was the temperatureĆseason interaction for the respiration rate, as the respiration rate at ambient temperature was 165% higher at all depths in spring than under warming conditions (-0.31 ± 0.09 µmol O2 cmā2 hā1; Tukey test: p<0.001; Figure 3B). In all other seasons, algae revealed comparable respiration rates between the two treatments across all depths (Table 3).
Carbon fixation: The pattern of oxygen production in the different seasons was also present in the Cgross fixation (Tables 3; Supplementary Table S4; Tukey test: p<0.001; Figure 4B). In spring, Cgross fixation was significantly higher with 0.32 ± 0.07 µmol C cmā2 hā1 than in autumn (0.28 ± 0.11 µmol C cmā2 hā1) and winter (0.22 ± 0.05 µmol C cmā2 hā1; Table 3; Tukey test: p<0.001). Warming significantly increased Cgross fixation (0.32 ± 0.06 µmol C cmā2 hā1) compared to ambient temperature conditions (0.25 ± 0.05 µmol C cmā2 hā1; Table 3; Tukey test: p<0.001). The single factor depth also affected Cgross fixation, as 2-m algae with 0.33 ± 0.07 µmol C cmā2 hā1 had significantly higher Cgross fixation than algae from other depths with 0.27 ± 0.05 µmol C cmā2 hā1 (average over both depths) across all seasons and temperature treatments (Table 3; Tukey test: p<0.001). Only the depthĆseason interaction was significant for Cgross fixation, as rates were -60% lower in 2-m algae in winter than for 2-m algae in spring, for both treatments (Figure 4B; Tukey test: p<0.01).
Photosynthetic quotient: The seasonal variation in oxygen production and Cgross fixation resulted in a seasonal pattern in PQ values with significantly highest values in summer (3.5 ± 1.1) compared to all other seasons (average over all other seasons: 2.3 ± 1.0) across all depths and temperature treatments (Figure 4C, Tables 3, Supplementary Table S4; Tukey test: p<0.001). Under warming conditions PQ values decreased significantly. Samples under warming revealed a PQ of 2.3 ± 1.0 across all depths and seasons, which was 22% lower than under ambient conditions (PQ of 2.9 ± 1.1; Table 3; Tukey test: p<0.001). Along the depth gradient PQ values showed no significant differences, although 4-m discs from the ambient treatment exhibited a trend toward 21% higher PQ values compared to discs from 2 and 6 m across all seasons. For PQ values no significant interactions of the three main factors were present (Table 3).
3.3 Leaf area index
The LAI had a peak at 4 m with 6.70 ± 0.98 m2 lamina m-2 seafloor. This was 14% higher than the LAI at 2 m and 36% higher than the LAI at 6 m (Table 1). However, depth had no significant effect on LAI (Kruskal-Wallis ANOVA: Ļ2 = 5.97, df=3, p=0.051). At 2 m, LAI of L. hyperborea varied greatly between replicates (1.02 to 10.07; raw data not shown).
3.4 Modelled net primary production
Comparison of modelled NPP under ambient and warming conditions at 4 m depth: Seasonal variation was evident in the daily NPPambient and the daily NPPwarming rates (Tables 4, 5; Figure 5B). Daily NPPambient rates were significantly higher in spring and summer than in the other seasons and only negative in winter. Daily NPPwarming was significantly different in all seasons, with the highest rates in spring and lowest in autumn, while rates were negative in autumn and winter (Table 4). No significant difference was observed between the annual NPPambient rate and the annual NPPwarming rate, although the annual NPPwarming rate was 14% higher (Tables 4, 5).
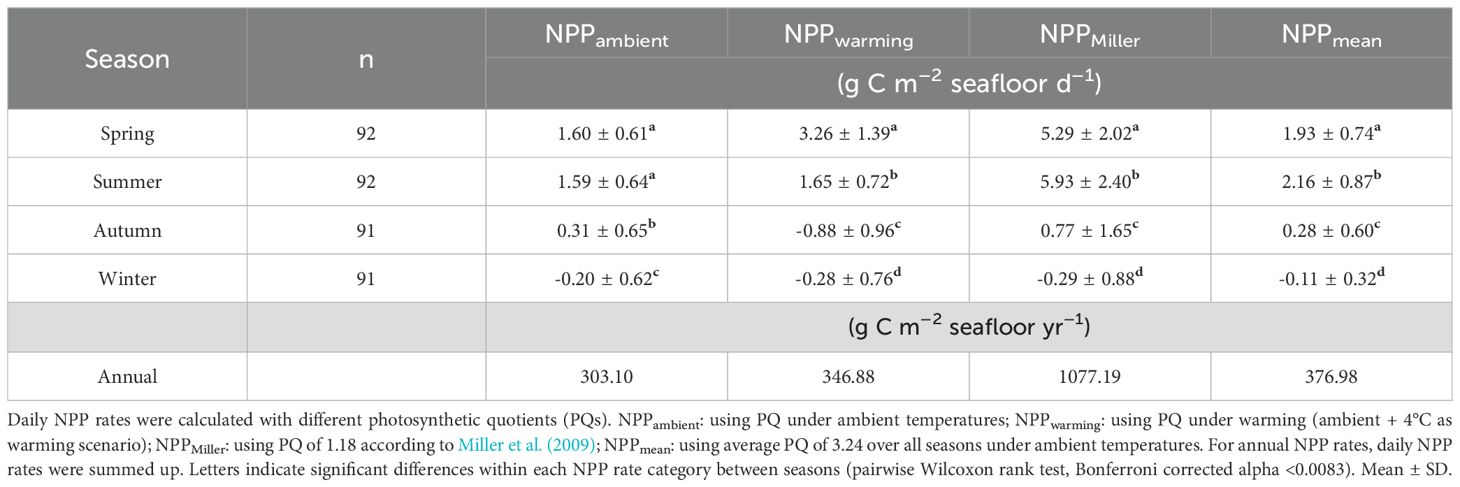
Table 4. Modelled daily net primary production (NPP) rates of the Laminaria hyperborea forest at 4 m water depth.
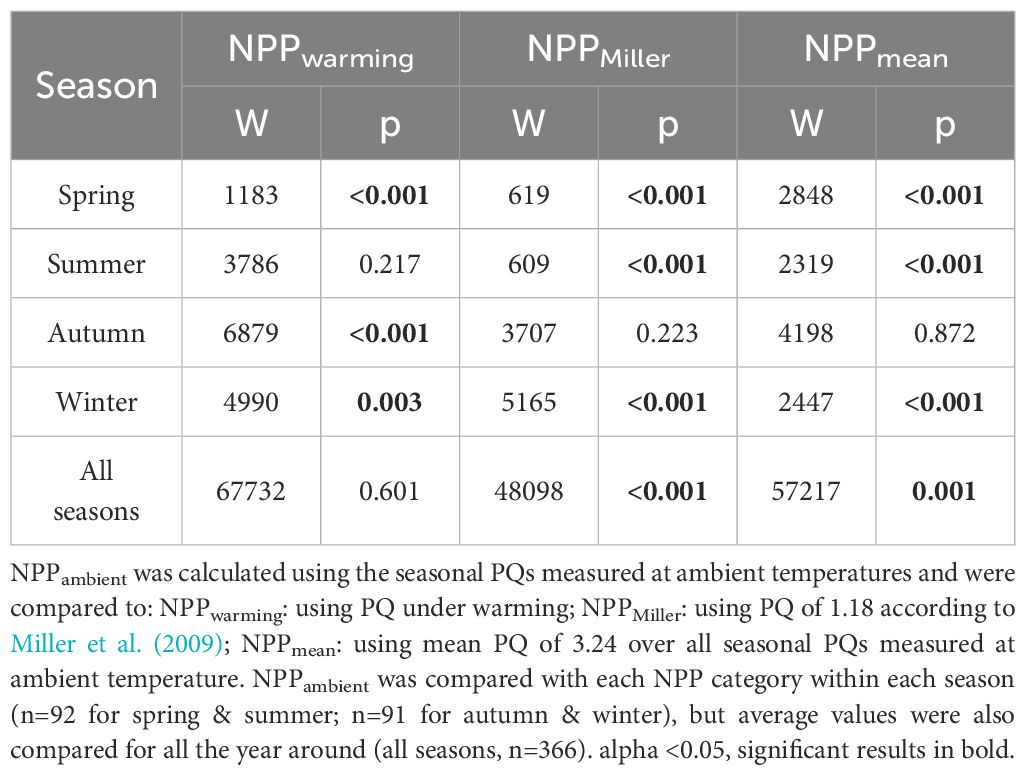
Table 5. Results of non-parametric Wilcoxon tests for the modelled daily net primary production (NPP) rates of Laminaria hyperborea collected at 4 m depth, calculated with different photosynthetic quotients (PQs).
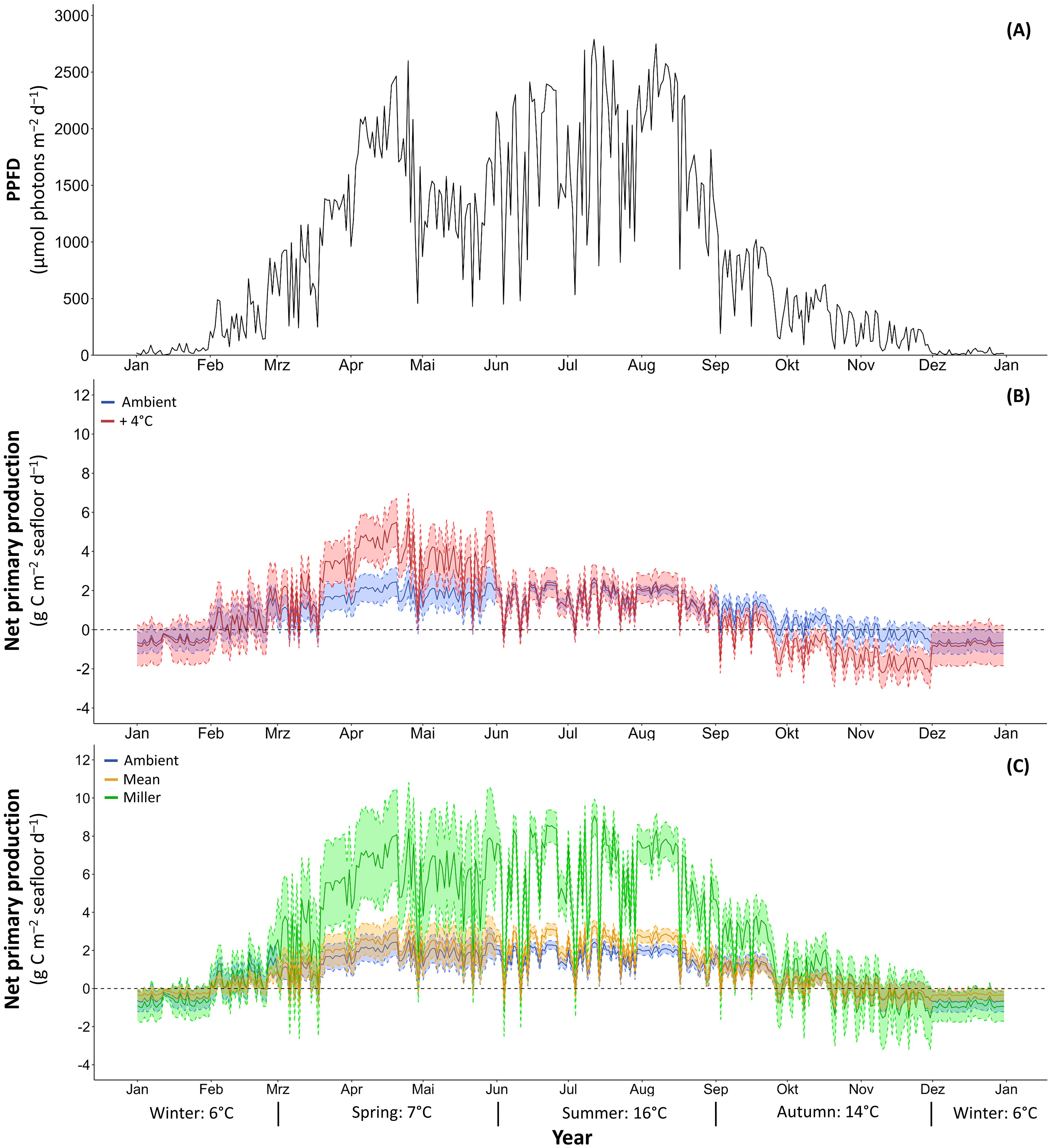
Figure 5. Daily photosynthetic photon flux density (PPFD) (A) used to model net primary production of Laminaria hyperborea collected at 4 m with different photosynthetic quotients (PQs). Blue: using PQ under ambient temperatures & Red: using PQ under warming (ambient + 4°C) (B); Green: using PQ of 1.18 according to Miller et al. (2009) & Orange: using average PQ of 3.24 over all seasons under ambient temperatures (C). Continuous line: mean, dashed line: ± SD; n=6.
The seasonal NPP rates of both scenarios differed significantly in spring, autumn, and winter (Table 5). In spring, the daily NPPwarming rate was twice as high as the daily NPPambient rate, which is due to lower C-fixation at ambient temperature (Table 4; Figure 5). In autumn, daily NPPambient rates were positive, but under warming they turned into significantly lower, negative daily NPPwarming rates (Table 4). In winter, daily NPPwarming rates were 35% lower than daily NPPambient rates (Tables 4, 5; Figure 5B).
Comparison of modelled NPP with different PQ approaches: As we have also shown for daily NPPwarming rates, the daily NPPMiller and NPPmean rates differed significantly between seasons. Both rates were significantly higher in summer and lowest in winter (Table 4; Figure 5C). The daily NPPPQ-Miller rates and the daily NPPmean rates were significantly different from NPPambient rates in spring, summer and winter, but were comparable to NPPambient rates in autumn (Table 5; Tukey test: p<0.001). In spring and summer, the Miller PQ and mean PQ values were lower than the seasonal PQ values, resulting in a 330ā373% higher NPPMiller and a 20ā36% higher NPPmean compared to NPPambient (Figure 5C). In winter, NPPambient was calculated with a PQ of 1.66, which was higher than the Miller PQ of 1.18, but lower than the mean PQ of 3.24, resulting in a significantly lower NPPMiller (41%) and a significantly higher NPPmean (49%; Tables 4, 5; Tukey test: p<0.001). Both modelled annual NPP rates based on fixed PQs were significantly higher than the annual NPPambient rate (Table 5).
4 Discussion
The importance of kelps in the coastal carbon cycle has been known for decades (e.g. Smith, 1981; Duarte, 2017). In most studies, net primary production (NPP) rates were derived from biomass surveys that included the total standing stock, or oxygen production rates that were converted to NPP rates using a fixed, theoretical PQ (Pessarrodona et al., 2022a). However, direct measurements of seasonal C-fixation rates of kelps are missing and using a fixed PQ is unable to reflect dynamic aspects in primary productivity (Wright et al., 2022; Trentman et al., 2023). Our study for the first time highlights the variability of PQ values over the course of the investigated seasons ranging from 1.7 to 4.4 within one important kelp species and evaluates its impact on modelling kelp NPP rates. This aspect has been neglected in other studies. Overall, measured PQ values were highest in high-light season summer, when oxygen production was maximised. While depth effects could be neglected, warming resulted in overall significantly lower PQ values, but seasonally this effect was most prominent in spring and summer.
Modelled daily NPP rates over the year were highly variable and followed the seasonally changing PPFD (Figure 5), revealing highest rates in high-light seasons spring and summer under ambient conditions. Warming intensified the effect of PPFD especially in spring, while caused a switch to negative NPP rates in the low-light season autumn in contrast to positive NPP rates at ambient temperatures due to increased respiration rate at 4 m depth under warming condition (Franke et al., 2024). The potentially higher NPP rates resulting from the lowered PQ during warming were however only apparent in spring, while PQ values were lower under warming conditions also in summer and winter without an effect on NPP rates. Interestingly, daily NPP rates modelled under ambient conditions with a fixed PQ value of 1.18 (Miller et al., 2009 for L. hyperborea), resulted in annual NPP rates three times higher than using seasonally changing PQ values, reflecting a large overestimation. In contrast, daily NPP rates modelled under ambient conditions with a mean PQ for the whole year, based on the seasonal PQ values derived from our measurements, only resulted in a slightly higher annual NPP rate compared to the annual NPP rate using seasonally changing PQ values. We thus suggest to consider this approach as a direct method to measure so-called ātrueā C-fixation (Arnold and Littler, 1985; Kaiser et al., 2020), in addition to the usual standing stock surveys, or at least to include variable PQ values in NPP predictions.
The physiological and ecological consequences of a sub-lethal year-round global warming on L. hyperborea and kelps in general are unknown. Our study provides a first insight about possible negative and positive effects of warming in L. hyperborea over the seasons. In summer, higher temperatures increased the variance in maximum quantum yield which indicating onset of sub-lethal heat stress (Graiff et al., 2015). This may become lethal depending on the duration of the heat stress, as juvenile L. hyperborea has a two-week lethal temperature limit of 20°C (tom Dieck, 1992). In autumn, negative C-fixation rates as indicated by our model, may however lead to the incorporation of less carbon reserves (Schiener et al., 2015) which are crucial for winter survival in low light conditions (Scheschonk et al., 2019) and for nourishing the newly emerging blade via translocation from old to new blade (Lüning et al., 1972). Although the removal of the old blade does not prevent formation of a new blade in spring (Lüning, 1969), the new blade will become much smaller. This situation may also be the case if carbon reserves become diminished due to autumn warming in an elongated negative carbon balance situation. The situation changes again from mid-winter onwards when blade growth is initiated (Schaffelke and Lüning, 1994) and will profit from increased temperatures as optimum growth temperatures of L. hyperborea are at 10-17°C (tom Dieck, 1992). As we could show that higher temperatures in spring substantially increased the primary production rates, this, in combination with increased growth rates, may potentially result in larger and more vigorous thalli entering summer. As a consequence, NPP may possibly even increase with warming over the year, under the condition that temperatures do not surpass critical high limits over extended periods.
4.1 Oxygen production, carbon fixation and the photosynthetic quotient
By definition, the PQ describes the molar ratio of oxygen released during the assimilation of carbon into organic matter (Kaiser et al., 2020). The methods used in the present study provide reliable estimates of photosynthetic activities in terms of moles of oxygen produced per mol of fixed carbon (Hurd et al., 2014). In the present study, Helgolandic L. hyperborea exhibited PQ values ranging from 1.65 ± 0.81 to 4.41 ± 0.64. The comparison of PQ values in literature is quite difficult because of the different methodologies used (relative atomic compositions: e.g. Ryther, 1956 used data from Brandt and Raben, 1919; C:N ratios: Gerard, 1988; combination of oxygen production measurements and isotopic C-fixation: Hatcher et al., 1977; Miller et al., 2009; IƱiguez et al., 2016b). Therefore, the PQ values reported for macroalgae vary widely from 0.67 to 5.20 (Ryther, 1956 used data from Brandt and Raben, 1919: Saccharina latissima; Hatcher et al., 1977: Laminaria longicruris; Gerard, 1988: S. latissima; Weykam et al., 1997: Iridaea cordata; IƱiguez et al., 2016b: S. latissima, Laminaria solidungula). Compared to Norwegian L. hyperborea, our PQ values were considerably higher (1.18 and 1.3; Miller et al., 2009). While the oxygen production rates were comparable to those of our study, the higher 13C-fixation rates at saturated PPFD could be caused by differences in the methodology, as the high background level of 13C, while 14C signal is almost completely added throughout the experiment (Miller et al., 2009; Beer et al., 2014). Miller et al. (2009) used 13C, a stable isotope that is more common in nature and requires significant 13C enrichment to produce measurable uptake signals in kelp tissue. In contrast, 14C only occurs in low abundance in nature, making detection very sensitive, even with short incubation times (Miller and Dunton, 2007; Beer et al., 2014; Kaiser et al., 2020). Our 14C-fixation rates under saturating light conditions ranged from 1.2 to 3.4 µmol C gā1 FM hā1 (corresponding to 0.25 to 0.42 µmol C cmā2 hā1) and were comparable to 14C-fixation rates presented in Wiencke and Bischof (2012: summarised from Kremer, 1981: 2.1 µmol C gā1 FM hā1) measured under saturating PPFD for Helgolandic L. hyperborea.
Laminaria hyperborea revealed a strong seasonality in its photosynthetic activity as measured by both oxygen production and 14C-fixation, with peak values in spring and summer, which is confirmed by other studies on this kelp (Drew, 1983; White et al., 2021; Franke et al., 2024) and other Laminariales (Blain and Shears, 2019). In summer, an enhanced activity of the light-dependent reactions provides more photosynthetic energy, but C-fixation rates were not significantly increased (Kirk, 1994; Wiencke and Bischof, 2012), leading to the highest PQ values. The photosynthetic energy is distributed between C-uptake and fixation on the one hand, and other nutrient assimilation mechanisms (such as essential enzymes and transporters involved in nitrate and phosphate uptake and assimilation) on the other (Gordillo et al., 2002). Although nutrient levels are low in summer, kelps accumulate mainly carbohydrates such as mannitol and laminarin as storage compounds for being used during the growth period in late winter and early spring (Kain and Jones, 1976; Küppers and Weidner, 1980; Kirk, 1994; Schaffelke and Lüning, 1994), but also expend more photosynthetic energy in maintaining active inorganic carbon acquisition mechanisms under saturating light conditions. In spring, the combination of high PPFD and elevated nutrient availability resulted in higher C-fixation rates (Kirk, 1994). In summer, higher PQ values were measured when nutrients were low and the assimilation of nitrate was the main source of nitrogen, which also increases PQ (Beer et al., 2014; Trentman et al., 2023). The high impact of the nitrogen source on PQ values was also demonstrated in studies using nitrate instead of ammonium, which determined 50% higher PQ values due to lower C-fixation as more energy was required to reduce nitrate to ammonium (Beer et al., 2014; Hurd et al., 2014; Kaiser et al., 2020).
Along the depth gradient, gross oxygen production and PQ values did not differ significantly, indicating a high acclimatisation potential to the different PPFD conditions of the photosynthetic processes of L. hyperborea, which has already been discussed by Franke et al. (2023, 2024). However, 14C-fixation was significantly higher in 2-m kelps compared to other depths across all seasons and temperature treatments, confirming the previously observed increase in C-fixation rates in Norwegian L. hyperborea sampled at mean low water (= 0 m) compared to 10 m depth (below mean low water; Miller et al., 2009). Although gross oxygen production was not significantly higher in 2-m kelps, it was 8ā25% higher than at other depths, most likely due to higher PPFD availability.
Warming resulted in increased Fv/Fm variances and a partial decrease in Fv/Fm values only in summer, indicating a moderate stress response. Burdett et al. (2019) exposed L. hyperborea from Plymouth Sound, UK, to short-term warming events (+ 4°C) and observed an insensitive photo-physiological response with no significant change in net oxygen production during the cultivation period of three days. Nevertheless, kelp studies in which temperature was manipulated during all four seasons in an experimental setup with simultaneous measurements of oxygen production and C-fixation are missing. In the present study, warming had no significant impact on gross oxygen production measured under saturating PPFD, while 14C-fixation was stimulated in all seasons, which was also observed in L. solidungula under increased temperature (Iñiguez et al., 2016b). In the present study, short-term warming may have increased enzyme activity, including carbon assimilation, which is supported by the study of Küppers and Weidner (1980), who showed an increase in RubisCO activity of L. hyperborea in response to seasonally increasing temperatures. As a result, the higher 14C-fixation at elevated temperatures resulted in lower PQ values.
4.2 Net primary production
Our study modelled daily NPP rates with seasonally changing PQ values as well as P-I curve parameters (Franke et al., 2024), hourly PPFD data and a fixed LAI. The LAI was obtained during the summer campaign as Lüning (1971) measured a peak in blade area during early summer. The daily NPP rate of our blade disc was up scaled with this fixed LAI in a linear equation. A lower LAI would directly led to reduced daily NPP rates, indicating that negative rates become less negative and positive rates are less positive, resulting in an overall lower NPP rate. In the present study, these potential differences in LAI were neglected. However, variable LAI should be taken into account in future studies to get a more reliable NPP. Nevertheless, the highest variability in daily NPP rates is driven by the PPFD. Franke et al. (2023) evaluated the impact of changing underwater PPFD on kelp daily NPP rates during one summer, which can lead to negative rates already in >3-4 m during a storm period leading to enhanced turbidity with low PPFD.
The annual NPP rate presented was comparable to annual NPP rates based on a biomass survey conducted by Smale et al. (2020) in L. hyperborea forests in Great Britain. They investigated the productivity based on biomass at two sites with different temperatures and revealed a decrease in productivity at the warmer site (Smale et al., 2020). Our temperature manipulation experiment was conducted on a kelp population at one site and revealed a trend towards a higher annual NPP rate under warming and highlighted significant differences in seasonal NPP rates in spring, autumn and winter. Warming had an intensifying effect on the seasonal variability of NPP rates due to higher C-fixation and therefore lower PQ values under warming conditions. The overall shape of the daily NPP curve is driven by PPFD (Deregibus et al., 2016; Franke et al., 2023, 2024). In both temperature scenarios, NPP rates were highest in spring compared to the other seasons, which may be a consequence of the annual growth rhythm of L. hyperborea (Kain and Jones, 1976; Schaffelke and Lüning, 1994). Due to lower PPFD in autumn and winter, NPP rates (ambient and warming) were up to 127% lower than the NPP rates in spring. This seasonality in NPP rates was also observed for Ecklonia radiata (Rodgers and Shears, 2016) and Macrocystis pyrifera (Miller et al., 2011), which were modelled using biomass surveys and theoretical fixed PQ values, respectively, resulting in highest production rates in spring and summer. We also observed a change from positive NPP rates under ambient conditions to negative NPP rates under warming conditions in autumn, which is attributed to a three-time higher respiration rate and the typical low PPFD of this season.
The Helgolandic L. hyperborea forest exhibits LAI values comparable to other L. hyperborea populations along the NE-Atlantic coastline ranging from 1-12.7 m2 lamina m-2 seafloor pointing to a highly productive ecosystem (Jupp and Drew, 1974; Kain and Jones, 1977; Pehlke and Bartsch, 2008). White et al. (2021) modelled daily NPP rates between 3.9 and 24.5 g C m-2 d-1 in sub-canopy and canopy blades, respectively, in summer and between -10.1 and 1.5 g C m-2 d-1 in canopy and sub-canopy blades, respectively, in autumn with a fixed PQ (1.2), in situ P-I curves and PPFD data for a L. hyperborea forest at 4 m depth in France. Daily NPP rates for these two seasons in the present study were at the lower end of the above-mentioned ranges. Our seasonal PQ values were up to three times higher than the theoretical PQ value used by White et al. (2021). Thus, low PQ values result in higher NPP rates and a possible overestimation of the C-fixation of kelps. Estimations of kelp NPP based on oxygen measurements combined with a single theoretical PQ value (Fairhead and Cheshire, 2004; White et al., 2021; Franke et al., 2023) neglect seasonal differences in PQ values and their impact on NPP rates. This became apparent when we compared modelled annual NPP rates with the PQ value of 1.18 from Miller et al. (2009) with NPP rates resulting from our seasonal PQ values. The approach of Miller et al. (2009) resulted in three-time higher annual NPP rate, as the PQ value was much lower than our seasonal PQ values. As it is common knowledge that Laminaria species have a pronounced seasonality in a series of parameters such as growth (Kain and Jones, 1976; Schaffelke and Lüning, 1994), carbon storage (Schiener et al., 2015; Scheschonk et al., 2019), photosynthesis (Franke et al., 2024) or enzyme activity (Küppers and Weidner, 1980), we assume that the seasonal difference in PQs which we identified, is a general feature of kelps, although this may need verification at least in one or two other kelps species from other locations. In the end, the high variability in PQ values directly affected NPP rates and was neglected in former models. Therefore, we recommend modelling NPP rates not with a fixed PQ, but with variable PQ values to obtain reliable future predictions.
Data availability statement
The raw data supporting the conclusions of this article will be made available by the authors, without undue reservation.
Author contributions
KF: Writing ā review & editing, Writing ā original draft, Visualization, Validation, Project administration, Methodology, Investigation, Formal analysis, Data curation, Conceptualization. FK: Writing ā review & editing, Writing ā original draft, Visualization, Investigation, Formal analysis, Data curation. UK: Writing ā review & editing, Resources, Funding acquisition, Conceptualization. IB: Writing ā review & editing, Validation, Resources, Conceptualization. CI: Writing ā review & editing, Methodology, Formal analysis, Conceptualization. AG: Writing ā review & editing, Writing ā original draft, Supervision, Project administration, Methodology, Investigation, Formal analysis, Conceptualization.
Funding
The author(s) declare financial support was received for the research, authorship, and/or publication of this article. KF, AG and FK were supported by the Deutsche Forschungsgemeinschaft (DFG) within the project āSeasonal kelp primary production at a rocky shore site: Integrating physiology and biochemistry into ecological modellingā (GR5088/2-1). The LAI investigation was part of the M.Sc. thesis of FK at the Carl-von-Ossietzky University Oldenburg, Germany, which was performed at the Alfred Wegener Institute on Helgoland. FK was further supported by the DFG within the project āRemote sensing based assessment and modelling of macrophyte growth in small lakes integrating spectral-seasonal traits and abiotic factorsā (GE 2169/11-1).
Acknowledgments
The authors thank P. Fischer and the Centre for Scientific Diving as well as the Biological Institute Helgoland of the Alfred Wegener Institute for their support and infrastructure during sampling and laboratory measurements (Grant number: AWI_BAH_06). We also thank M. Lucassen for his help with the coordination of the isotope laboratory and the stock solution. We thank I. Bussmann for her support with the isotope laboratory on Helgoland. The authors thank the submaris scientific diving team for support during sampling.
Conflict of interest
The authors declare that the research was conducted in the absence of any commercial or financial relationships that could be construed as a potential conflict of interest.
Publisherās note
All claims expressed in this article are solely those of the authors and do not necessarily represent those of their affiliated organizations, or those of the publisher, the editors and the reviewers. Any product that may be evaluated in this article, or claim that may be made by its manufacturer, is not guaranteed or endorsed by the publisher.
Supplementary material
The Supplementary Material for this article can be found online at: https://www.frontiersin.org/articles/10.3389/fmars.2024.1455706/full#supplementary-material
References
Araujo R., BĆ”rbara I., Sousa-Pinto I., Quintino V. (2005). Spatial variability of intertidal rocky shore assemblages in the northwest coast of Portugal. Estuar. Coast. Shelf Sci. 64, 658ā670. doi: 10.1016/j.ecss.2005.03.020
Arnold K. E., Littler M. M. (1985). āThe carbon-14 method for measuring primary productivity,ā in Handbook of phycological methods, ecological field methods: macroalgae. Eds. Littler M. M., Littler D. S. (Cambridge, UK: Cambridge University Press), 377ā396.
Assis J., FernÔndez Bejarano S. J., Salazar V. W., Schepers L., Gouvêa L., Fragkopoulou E., et al. (2024). Bio-ORACLE v3.0. Pushing marine data layers to the CMIP6 Earth system models of climate change research. Global Ecol. Biogeogr. 33, e13813. doi: 10.1111/geb.13813
Beer S., Bjƶrk M., Beardall J. (2014). Photosynthesis in the marine environment (New Jersey: John Wiley & Sons).
Bell R. T., Kuparinen J. (1984). Assessing phytoplankton and bacterioplankton production during early spring in Lake Erken. Sweden. Appl. Environ. Microbiol. 48, 12211230. doi: 10.1128/aem.48.6.1221-1230.1984
Blain C. O., Shears N. T. (2019). Seasonal and spatial variation in photosynthetic response of the kelp Ecklonia radiata across a turbidity gradient. Photosynth. Res. 140, 21ā38. doi: 10.1007/s11120-019-00636-7
Brandt K., Raben E. (1919). Zur Kenntnis der chemischen Zusammensetzung des Planktons und einiger Bodenorganismen. Wiss. Meeresuntersuch. 19, 175ā209.
Burdett H. L., Wright H., Smale D. A. (2019). Photophysiological responses of canopy-forming kelp species to short-term acute warming. Front. Mar. Sci. 6. doi: 10.3389/fmars.2019.00516
Burgess M. G., Becker S. L., Langendorf R. E., Fredston A., Brooks C. M. (2023). Climate change scenarios in fisheries and aquatic conservation research. ICES J. Mar. Sci. 80, 1163ā1178. doi: 10.1093/icesjms/fsad045
Casado-AmezĆŗa P., Araujo R., Criado I. B., Bermejo R., Borja A., DĆez I., et al. (2019). Distributional shifts of canopy-forming seaweeds from the Atlantic coast of Southern. Europe. Biodivers. Conserv. 28, 1151ā1172. doi: 10.1007/s10531-019-01716-9
Curran-Everett D. (2017). CORP: Minimizing the chances of false positives and false negatives. J. Appl. Physiol. 122, 91ā95. doi: 10.1152/japplphysiol.00937.2016
Deregibus D., Quartino M. L., Campana G. L., Momo F. R., Wiencke C., Zacher K. (2016). Photosynthetic light requirements and vertical distribution of macroalgae in newly ice-free areas in Potter Cove, South Shetland Islands, Antarctica. Polar Biol. 39, 153ā166. doi: 10.1007/s00300-015-1679-y
Drew E. A. (1983). Physiology of Laminaria. Mar. Ecol. 4, 227ā250. doi: 10.1111/j.1439-0485.1983.tb00298.x
Duarte C. M. (2017). Reviews and syntheses: Hidden forests, the role of vegetated coastal habitats in the ocean carbon budget. Biogeosciences 14, 301ā310. doi: 10.5194/bg-14-301-2017
Duarte C. M., CebriĆ”n J. (1996). The fate of marine autotrophic production. Limnol. Oceanogr. 41, 1758ā1766. doi: 10.4319/lo.1996.41.8.1758
Duarte C. M., Middelburg J. J., Caraco N. (2005). Major role of marine vegetation on the oceanic carbon cycle. Biogeosciences 2, 1ā8. doi: 10.5194/bg-2-1-2005
Dummermuth A., Wiltshire K. H., Kirstein I., Brodte E. M., Wichels A., Shama L., et al. (2023). Marine Stations Helgoland and Sylt operated by the Alfred Wegener Institute Helmholtz Centre for Polar and Marine Research. J. Large Scale Res. Facil. 8, A184. doi: 10.17815/jlsrf-8-184
Fairhead V. A., Cheshire A. C. (2004). Rates of primary productivity and growth in Ecklonia radiata measured at different depths, over an annual cycle, at West Island, South Australia. Mar. Biol. 145, 41ā50. doi: 10.1007/s00227-004-1308-8
Fischer P., Brand M., Friedrich M., Eickelmann L., LienkƤmper M., Spotowitz L., et al. (2021b). Hydrographical time series data of Helgoland, Southern North Sea 2018 (PANGAEA). doi: 10.1594/PANGAEA.934740
Fischer P., Brand M., Friedrich M., Eickelmann L., LienkƤmper M., Spotowitz L., et al. (2021c). Hydrographical time series data of Helgoland, Southern North Sea 2019 (PANGAEA). doi: 10.1594/PANGAEA.927379
Fischer P., Brand M., Friedrich M., Eickelmann L., LienkƤmper M., Spotowitz L., et al. (2021d). Hydrographical time series data of Helgoland, Southern North Sea 2020 (PANGAEA). doi: 10.1594/PANGAEA.933714
Fischer P., Happel L., Anselm N., Bussmann I., LienkƤmper M., Brand M., et al. (2022). Hydrographical time series data of Helgoland, Southern North Sea 2016 (PANGAEA). doi: 10.1594/PANGAEA.942188
Fischer P., Happel L., Brand M., Eikelmann L., LienkƤmper M., Spotowitz L., et al. (2021a). Hydrographical time series data of Helgoland, Southern North Sea 2017 (PANGAEA). doi: 10.1594/PANGAEA.935808
Franke K., Bartsch I., Karsten U., Graiff A. (2024). Seasonality influences the effect of warming on kelp photosynthesis. MEPS 739, 15ā29. Available at: https://www.int-res.com/prepress/m14623.html.
Franke K., Matthes L. C., Graiff A., Karsten U., Bartsch I. (2023). The challenge of estimating kelp production in a turbid marine environment. J. Phycol. 59, 518ā537. doi: 10.1111/jpy.13327
Gerard V. A. (1988). Ecotypic differentiation in light-related traits of the kelp Laminaria saccharina. Mar. Biol. 97, 25ā36. doi: 10.1007/BF00391242
Gordillo F. J., Dring M. J., Savidge G. (2002). Nitrate and phosphate uptake characteristics of three species of brown algae cultured at low salinity. Mar. Ecol. Prog. Ser. 234, 111ā118. doi: 10.3354/meps234111
Graiff A., Liesner D., Karsten U., Bartsch I. (2015). Temperature tolerance of western Baltic Sea Fucus vesiculosusāgrowth, photosynthesis and survival. J. Exp. Mar. Biol. Ecol. 471, 8ā16. doi: 10.1016/j.jembe.2015.05.009
Gran G. (1952). Determination of the equivalence point in potentiometric titrations. Part II. Anal. 77, 661ā671. doi: 10.1039/AN9527700661
Hanelt D. (2018). āPhotosynthesis assessed by chlorophyll fluorescence,ā in Bioassays, advanced Methods and Applications. Eds. HƤder D. P., Erzinger G. S. (Elsevier, The Netherlands), 169ā198.
Hatcher B. G., Chapman A. O., Mann K. H. (1977). An annual carbon budget for the kelp Laminaria longicruris. Mar. Biol. 44, 85ā96. doi: 10.1007/BF00386909
Hurd C. L., Harrison P. J., Bischof K., Lobban C. S. (2014). Seaweed ecology and physiology. 2nd (GB: Cambridge University Press).
IƱiguez C., Carmona R., Lorenzo M. R., Niell F. X., Wiencke C., Gordillo F. J. (2016a). Increased CO2 modifies the carbon balance and the photosynthetic yield of two common Arctic brown seaweeds: Desmarestia aculeata and Alaria esculenta. Polar Biol. 39, 1979ā1991. doi: 10.1007/s00300-015-1724-x
IƱiguez C., Carmona R., Lorenzo M. R., Niell F. X., Wiencke C., Gordillo F. J. (2016b). Increased temperature, rather than elevated CO2, modulates the carbon assimilation of the Arctic kelps Saccharina latissima and Laminaria solidungula. Mar. Biol. 163, 1ā18. doi: 10.1007/s00227-016-3024-6
Jassby A. D., Platt T. (1976). Mathematical formulation of the relationship between photosynthesis and light for phytoplankton. Limnol. Oceanogr. 21, 540ā547. doi: 10.4319/lo.1976.21.4.0540
Jupp B. P., Drew E. A. (1974). Studies on the growth of Laminaria hyperborea (Gunn.) Fosl. I. Biomass and productivity. J. Exp. Mar. Biol. Ecol. 15, 185ā196. doi: 10.1016/0022-0981(74)90044-6
Kain J. M., Jones N. S. (1976). The biology of Laminaria hyperborea IX. Growth pattern of fronds. J. Mar. Biol. Assoc. U. K. 56, 603ā628. doi: 10.1017/S0025315400020683
Kain J. M., Jones N. S. (1977). The biology of Laminaria hyperborea X. The effect of depth on some populations. J. Mar. Biol. Assoc. U. K. 57, 587ā607. doi: 10.1017/S0025315400025054
Kaiser M. J., Attrill M. J., Jennings S., Thomas D. N., Barnes D. K. A., Brierley A. S., et al. (2020). Marine ecology: Processes, systems, and impacts. 3rd (New York: Oxford University Press).
Kirk J. T. (1994). Light and photosynthesis in aquatic ecosystems. 2nd (GB: Cambridge University Press).
Kremer B. P. (1981). Metabolic implications of non-photosynthetic carbon fixation in brown macroalgae. Phycologia 20, 242ā250. doi: 10.2216/i0031-8884-20-3-242.1
Küppers U., Weidner M. (1980). Seasonal variation of enzyme activities in Laminaria hyperborea. Planta 148, 222ā230. doi: 10.1007/BF00380031
Kylin H. (1947). Die Phaeophyceen der schwedischen Westküste. Lunds Univ. Ć rsskr. (Avd. 2) (Lund, Sweden: C.W.K. Gleerup) 43, 1ā99.
Lange O. L., Nobel P. S., Osmond C. B., Ziegler H. (1981). Physiological Plant Ecology I (Berlin: Springer Verlag).
Lee H., Calvin K., Dasgupta D., Krinner G., Mukherji A., Thorne P., et al. (2023). IPCC 2023: Climate Change 2023: Synthesis Report, Summary for Policymakers. Contribution of Working Groups I, II and III to the Sixth Assessment Report of the Intergovernmental Panel on Climate Change. Eds. Lee H., Romero J. (Geneva, Switzerland: IPCC). doi: 10.59327/IPCC/AR6-9789291691647.001
Lüdecke D., Ben-Shachar M., Patil I., Waggoner P., Makowski D. (2021). performance: An R package for assessment, comparison and testing of statistical models. J. Open source. Software 6, 3139. doi: 10.21105/joss.03139
Lüning K. (1969). Growth of amputated and dark-exposed individuals of the brown alga Laminaria hyperborea. Mar. Biol. 2, 218ā223. doi: 10.1007/BF00351143
Lüning K. (1971). āSeasonal growth of Laminaria hyperborea under recorded underwater light conditions near Helgoland,ā in Fourth Europ. Mar. Biol. symp, vol. 4. (University Press, Cambridge), 347ā361.
Lüning K. (1990). Seaweeds: their environment, biogeography, and ecophysiology (New Jersey: John Wiley & Sons).
Lüning K., Dring M. J. (1979). Continuous underwater light measurement near Helgoland (North Sea) and its significance for characteristic light limits in the sublittoral region. Helgol. Wiss. Meeresunters 32, 403ā424. doi: 10.1007/BF02277985
Lüning K., Schmitz K., Willenbring J. (1972). Translocation of 14C-labeled assimilates in two Laminaria species. Proc. Int. Seaweed Symp. 7, 420ā425.
Miller H. L. III, Dunton K. H. (2007). Stable isotope (13C) and O2 micro-optode alternatives for measuring photosynthesis in seaweeds. Mar. Ecol. Prog. Ser. 329, 85ā97. doi: 10.3354/meps329085
Miller H. L. III, Neale P. J., Dunton K. H. (2009). Biological weighting functions for UV inhibition of photosynthesis in kelp Laminaria hyperborea (Phaeophyceae). J. Phycol. 45, 571ā584. doi: 10.1111/j.1529-8817.2009.00694.x
Miller R. J., Reed D. C., Brzezinski M. A. (2011). Partitioning of primary production among giant kelp (Macrocystis pyrifera), understory macroalgae, and phytoplankton on a temperate reef. Limnol. Oceanogr. 56, 119ā132. doi: 10.4319/lo.2011.56.1.0119
Norici A., Bazzoni A. M., Pugnetti A., Raven J. A., Giordano M. (2011). Impact of irradiance on the C allocation in the coastal marine diatom Skeletonema marinoi Sarno and Zingone. Plant Cell Environ. 34, 1666ā1677. doi: 10.1111/j.1365-3040.2011.02362.x
Palmucci M., Ratti S., Giordano M. (2011). Ecological and evolutionary implications of carbon allocation in marine phytoplankton as a function of nitrogen availability: A Fourier transform infrared spectroscopy approach. J. Phycol. 47, 313ā323. doi: 10.1111/j.1529-8817.2011.00963.x
Pehlke C., Bartsch I. (2008). Changes in depth distribution and biomass of sublittoral seaweeds at Helgoland (North Sea) between 1970 and 2005. Clim. Res. 37, 135ā147. doi: 10.3354/cr00767
Pessarrodona A., Assis J., Filbee-Dexter K., Burrows M. T., Gattuso J. P., Duarte C. M., et al. (2022a). Global seaweed productivity. Sci. Adv. 8, eabn2465. doi: 10.1126/sciadv.abn2465
Pessarrodona A., Filbee-Dexter K., Krumhansl K. A., Pedersen K. A., Moore P. J., Wernberg T. (2022b). A global dataset of seaweed net primary productivity. Sci. Data 9, 484. doi: 10.1038/s41597-022-01554-5
Pessarrodona A., Moore P. J., Sayer M. D., Smale D. A. (2018). Carbon assimilation and transfer through kelp forests in the NE Atlantic is diminished under a warmer ocean climate. Glob. Change Biol. 24, 4386ā4398. doi: 10.1111/gcb.14303
PiƱeiro-Corbeira C., Barreiro R., Cremades J. (2016). Decadal changes in the distribution of common intertidal seaweeds in Galicia (NW Iberia). Mar. Environ. Res. 113, 106ā115. doi: 10.1016/j.marenvres.2015.11.012
Pokorny J., Orr P., Ondok J., Denny P. (1989). Photosynthetic quotients of some aquatic macrophyte species. Photosynthetica 23, 494ā506.
Rodgers K. L., Shears N. T. (2016). Modelling kelp forest primary production using in situ photosynthesis, biomass and light measurements. Mar. Ecol. Prog. Ser. 553, 67ā79. doi: 10.3354/meps11801
Ryther J. H. (1956). The Measurement of Primary Production. Limnol. Oceanogr. 1, 72ā84. doi: 10.4319/lo.1956.1.2.0072
Schaffelke B., Lüning K. (1994). A circannual rhythm controls seasonal growth in the kelps Laminaria hyperborea and L. digitata from Helgoland (North Sea). Eur. J. Phycol. 29, 49ā56. doi: 10.1080/09670269400650471
Scheschonk L., Becker S., Hehemann J. H., Diehl N., Karsten U., Bischof K. (2019). Arctic kelp eco-physiology during the polar night in the face of global warming: a crucial role for laminarin. Mar. Ecol. Prog. Ser. 611, 59ā74. doi: 10.3354/meps12860
Schiener P., Black K. D., Stanley M. S., Green D. H. (2015). The seasonal variation in the chemical composition of the kelp species Laminaria digitata, Laminaria hyperborea, Saccharina latissima and Alaria esculenta. J. Appl. Phycol. 27, 363ā373. doi: 10.1007/s10811-014-0327-1
Schreiber U., Schliwa U., Bilger W. (1986). Continuous recording of photochemical and non-photochemical chlorophyll fluorescence quenching with a new type of modulation fluorometer. Photosynth. Res. 10, 51ā62. doi: 10.1007/BF00024185
Smale D. A. (2020). Impacts of ocean warming on kelp forest ecosystems. New Phytol. 225, 1447ā1454. doi: 10.1111/nph.16107
Smale D. A., Pessarrodona A., King N., Burrows M. T., Yunnie A., Vance T., et al. (2020). Environmental factors influencing primary productivity of the forest-forming kelp Laminaria hyperborea in the northeast Atlantic. Sci. Rep. 10, 12161. doi: 10.1038/s41598-020-69238-x
Smith S. V. (1981). Marine macrophytes as a global carbon sink. Science 211, 838ā840. doi: 10.1126/science.211.4484.83
Smith L. M., Silver C. M., Oviatt C. A. (2012). Quantifying variation in water column photosynthetic quotient with changing field conditions in Narragansett Bay, RI, USA. J. Plankton Res. 34, 437ā442. doi: 10.1093/plankt/fbs011
Steeman-Nielsen E. (1952). The use of radioactive carbon (14C) for measuring organic production in the sea. J. Cons. Int. Explor. Mer. 18, 117ā140. doi: 10.1093/icesjms/18.2.117
Stewart W. D. P. (1974). Algal physiology and biochemistry (California: University of California Press).
Tengberg A., Hovdenes J., Andersson H. J., Brocandel O., Diaz R., Hebert D., et al. (2006). Evaluation of a lifetime-based optode to measure oxygen in aquatic systems. Limnol. Oceanogr.: Methods 4, 7ā17. doi: 10.4319/lom.2006.4.7
tom Dieck I. (1992). North Pacific and North Atlantic digitate Laminaria species (Phaeophyta): hybridization experiments and temperature responses. Phycologia 31, 147ā163. doi: 10.2216/i0031-8884-31-2-147.1
Trentman M. T., Hall R. O. Jr., Valett H. M. (2023). Exploring the mismatch between the theory and application of photosynthetic quotients in aquatic ecosystems. Limnol. Oceanogr.: Lett. 8, 565ā579. doi: 10.1002/lol2.10326
Tyberghein L., Verbruggen H., Pauly K., Troupin C., Mineur F., De Clerck O. (2012). Bio-ORACLE: A global environmental dataset for marine species distribution modelling. Glob. Ecol. Biogeogr. 21, 272ā281. doi: 10.1111/j.1466-8238.2011.00656.x
Voerman S. E., Llera E., Rico J. M. (2013). Climate driven changes in subtidal kelp forest communities in NW Spain. Mar. Environ. Res. 90, 119ā127. doi: 10.1016/j.marenvres.2013.06.006
Weykam G., Thomas D. N., Wiencke C. (1997). Growth and photosynthesis of the Antarctic red algae Palmaria decipiens (Palmariales) and Iridaea cordata (Gigartinales) during and following extended periods of darkness. Phycologia 36, 395ā405. doi: 10.2216/i0031-8884-36-5-395.1
White L., Loisel S., Sevin L., Davoult D. (2021). In situ estimates of kelp forest productivity in macro-tidal environments. Limnol. Oceanogr. 66, 4227ā4239. doi: 10.1002/lno.11955
Williams P. J. B., Raine R. C. T., Bryan J. R. (1979). Agreement between 14C and oxygen methods of measuring phytoplankton production: Reassessment of the photosynthetic quotient. Oceanol. Acta 2, 411ā416.
Keywords: net primary production, Laminaria hyperborea, 14C-fixation, oxygen production, photosynthetic quotient, Helgoland, warming scenario
Citation: Franke K, Kroth F, Karsten U, Bartsch I, IƱiguez C and Graiff A (2024) Varying photosynthetic quotients strongly influence net kelp primary production and seasonal differences increase under warming. Front. Mar. Sci. 11:1455706. doi: 10.3389/fmars.2024.1455706
Received: 27 June 2024; Accepted: 09 September 2024;
Published: 09 October 2024.
Edited by:
Ricardo A. Melo, University of Lisbon, PortugalReviewed by:
Araceli Puente, IHCantabria - Instituto de HidrƔulica Ambiental de la Universidad de Cantabria, SpainJanet Kubler, California State University, Northridge, United States
Copyright © 2024 Franke, Kroth, Karsten, Bartsch, Iñiguez and Graiff. This is an open-access article distributed under the terms of the Creative Commons Attribution License (CC BY). The use, distribution or reproduction in other forums is permitted, provided the original author(s) and the copyright owner(s) are credited and that the original publication in this journal is cited, in accordance with accepted academic practice. No use, distribution or reproduction is permitted which does not comply with these terms.
*Correspondence: Kiara Franke, kiara.franke@uni-rostock.de