- 1The Interuniversity Institute for Marine Sciences of Eilat, Eilat, Israel
- 2Department of Ecology, Evolution and Behavior, The Hebrew University of Jerusalem, Jerusalem, Israel
- 3Department of Life-Sciences, Ben Gurion University of the Negev, Beer Sheva, Israel
Holling’s classical functional response model describes the mechanistic foundations of the relationships between predation rate and prey density. As such, the model is pertinent to predators that actively search for prey, but not to stationary predators in which additional factors, such as flow speed, determine the rates of prey encounter. The main objective of this study was to measure the rates and corresponding efficiencies of zooplanktivory among different common species of coral-reef fishes under a wide range of prey densities and current speeds. All our experiments were carried out in a flume with different combinations of flow speeds (3-28.5 cm/s) and prey densities (210 - 1050 prey m-3). Nauplii of Artemia salina were used as prey. Despite major differences in the taxonomic origin of the studied species, their morphologies, and the types of shelters they use, the foraging performances of the fish, their predation rates, and the way those rates were affected by prey density and flow speed were surprisingly similar. Under a fixed prey density, capture rates did not change much as function of flow speed. Under conditions of equal prey flux, predation rates were always higher under conditions of high density and weaker flow than under lower density and faster flow. A sharp decline in capture efficiency with increasing flow speed was explained by a corresponding narrowing of the fish’s body orientation relative to the flow. In other words, with increasing flow speed, the fish gradually became more-narrowly oriented head-on onto the flow, exhibiting a decrease in the frequency of body turns (“maneuverability”). These trends, especially the reduced maneuverability under strong currents, can explain our findings that predation rates did not increase when the flow, hence prey flux, increased. Inter-specific differences in predation rates and efficiencies, however small, agree well with observed differences in the type of habitats the different species occupy.
1 Introduction
Much like oases in the desert, coral reefs flourish in oligotrophic seas, where the productivity of the reef commonly exceeds by up to an order of magnitude that of phytoplankton in the surrounding open waters. The enigmatic source(s) of the allochthonous nutrients needed to support this exceptionally high productivity of the reef has long captivated coral-reef researchers (Odum and Odum, 1955; Erez, 1990). One possible source is the consumption of pelagic plankton by benthic animals in the reef. Indeed, Genin et al. (2009) and Wyatt et al. (2010) found that intense consumption of small picoplankton can provide sufficient nutrients to explain this so called “paradox of the reef”. However, the relative contribution of zooplankton predation, is yet poorly known, despite suggestions that such predation is a major link of the reef’s benthic-pelagic coupling (Morais and Bellwood, 2019; Morais et al., 2021).
Generally, predation is a fundamental ecological link that frequently determines key attributes of individuals, populations, and communities (Begon et al., 2006). While this notion is widely recognized, studies in which rates of predation are directly measured are claimed to be infrequent, especially in aquatic systems (Mihalitsis et al., 2022). However, quite a few examples of studies in which predation rates of zooplankton by marine fishes are found in the literature (e.g., Kingsford and MacDiarmid, 1988; Noda et al., 1992; Kiflawi and Genin, 1997; Holzman and Genin, 2003; Clarke et al., 2009; Finelli et al., 2009; Khrizman et al., 2018; Ishikawa et al., 2022). As indicated above, several studies recently highlighted the importance of fish zooplanktivory in the coral reef ecosystem (Brandl et al., 2019; Morais and Bellwood, 2019; Morais et al., 2021; Siqueira et al., 2021; Mihalitsis et al., 2022). However, none of those studies included direct measurements of predation rates. Instead, the alleged intensity of zooplanktivory in those studies was indirectly deduced from measurements of biomass and growth of different fish that, in turn, were incorporated in a Von Bertalanffy Growth Model. Thus, the contribution of zooplankton predation by fish to the overall productivity of the reef is still debatable (Allgeier and Cline, 2019; Brandl et al., 2019), further highlighting the need for direct measurements of that predation.
Planktivorous fish are ubiquitous members of fish communities in many coral reefs, especially across the western Indo-Pacific (Siqueira et al., 2021) and the Red Sea (Fishelson et al., 1974). In some reefs, such as the outer GBR, those fish form a “wall of mouths” (Hamner et al., 1988), that substantially reduces the zooplankton densities down current. Similar down-current depletions of zooplankton also occur in kelp forests, temperate rocky reefs, and over seamounts (Gaines and Roughgarden, 1987; Kingsford and MacDiarmid, 1988; Genin et al., 1994).
In coral reefs, many fishes belonging to this guild are site-attached (Hobson, 1991; Kiflawi and Genin, 1997), keeping their position near a shelter where they capture zooplankton that drift toward them with the currents (Sale, 1971; Fishelson et al., 1974). A similar mode of predation is found in freshwater streams, where many fish capture drifting prey while temporarily keeping a stationary place (O’Brien et al., 2001). The majority of site-attached fishes in the coral reef form social groups, where the group’s fidelity to the same shelter can last several months and beyond (Sale, 1971; Booth, 2016). The shelters used by those site-attached fishes include branching corals, rocky knolls and other complex substrates. The fish maintain their foraging space sufficiently close to the shelter, allowing a fast retreat when threatened (Fishelson et al., 1974; Hobson, 1991). Their foraging space is almost always found up-current of the shelter, with the individual fish commonly facing the oncoming current (Hobson, 1991; Engel et al., 2021). The fish rapidly switch their search direction and position relative to the shelter when the currents change direction (Bray et al., 1981; Hobson, 1991; Engel et al., 2021). While foraging, the fish actively avoid being swept by the currents to a position found down-current of the shelter (Engel et al., 2021; A. Genin unpublished observations).
Site-attached fishes are not filter feeders (e.g., Nonacs et al., 1994); instead, they always strike individual prey. Their capture of a prey consists of three steps: (1) wait & search - slowly swimming near the shelter, waiting for a drifting prey to enter the foraging space; (2) strike - a rapid swim toward a detected prey, bringing their mouth sufficiently close to ingest the prey; (3) ram-jaw suction, an extremely rapid extension of the jaws in order to rapidly suck the prey (Coughlin and Strickler, 1990; Hobson, 1991; Wainwright et al., 2007). The duration of step 1 (wait & search) depends on the flow. Under constant prey densities, the frequency of prey arrival into the fish’s foraging space is a function of current speed (e.g., Finelli et al., 2009; Khrizman et al., 2018; Khrizman et al., 2024). However, the fish do not always increase their predation rates under stronger flows (Kiflawi and Genin, 1997; Clarke et al., 2009), allegedly due to flow-dependent biomechanical constraints that limit the ability of the fish to strike the prey at wide angles in respect to the flow direction (Kiflawi and Genin, 1997; O’Brien et al., 2001; Ella and Genin, 2023). In contrast, the fish’s functional response to changes in zooplankton density, is expected to monotonically increase with increasing prey densities (Kiflawi and Genin, 1997; Clarke et al., 2009), until a yet-unknown saturation level is reached (Holling, 1959).
The main objective of this study was to evaluate the effects of prey density and flow speed on the rates and efficiencies of plankton capture in 3 common species of site-attached coral-reef fishes under a wide, natural range of those parameters. Specifically, we examine the linearity of the fishes’ functional responses to increasing prey density, the occurrence of saturation, and the role of biomechanical limitations in determining the fishes’ functional responses to increasing flow speed.
2 Materials and methods
2.1 Study site
The study was carried out at the Interuniversity Institute for Marine Sciences in Eilat (IUI), northern Red Sea, Israel (29°30´ N, 34° 56´E). Fish were collected at the local coral reef, at 6-14 m depth. Detailed descriptions of the reef’s benthic communities are found in Fishelson (1971), Benayahu and Loya (1977), and Yahel et al. (1998). Briefly, those fringing reefs are dominated by stony corals, growing on a steep slope (10-30°). The currents are generally slow (average speed of 10 cm/s; maximum of ~50 cm/s) exhibiting a strong semidiurnal periodicity during the warm months (Genin and Paldor, 1998; Monismith and Genin, 2004). Northerly winds prevail >90% of the time. Due to a short fetch, the sea around our study site in the northern part of the Gulf is calm with waves < 0.5 m in height, except for rare times of strong southerly winds. The region is extremely arid, with average precipitation rate of 22 mm/y. The water is clear with visibility typically extending 10s of meters. Among the ca. 260 species of fish inhabiting the local reefs, the guild of zooplanktivorous fish is numerically the largest, comprising >40% of the total number of fish (Brokovich et al., 2006; Shaked and Genin, 2023).
2.2 Fish
The 3 site-attached species we studied (Figure 1) included 2 common damselfishes: Dascyllus marginatus (acronym: Dm; Standard length: 52 ± 6.36 mm) and Neopomacentrus miryae (Nm; 94 ± 10.9 mm) and a common serranid Pseudanthias squamipinnis (Ps; 103 ± 13.5 mm) (Figure 1). Dm is endemic to the Red Sea, Gulf of Aden and Gulf of Oman. Nm is endemic to the Red Sea. Ps is found throughout the Indian Ocean and West Pacific. Ps and Nm are the most abundant species in the local coral reefs (Khalaf and Kochzius, 2002; Brokovich et al., 2006; Megdadi et al., 2017). As other species belonging to the guild of diurnal, site-attached, coral-reef planktivores, the fish are generalist visual feeders (Coates, 1980) with diets consisting of meso-zooplankton that are usually larger than 400 µm in length (Noda et al., 1992; Hanson et al., 2016). The fish capture individual zooplankters using ram-jaw suction, as described above (Coughlin and Strickler, 1990; Hobson, 1991).
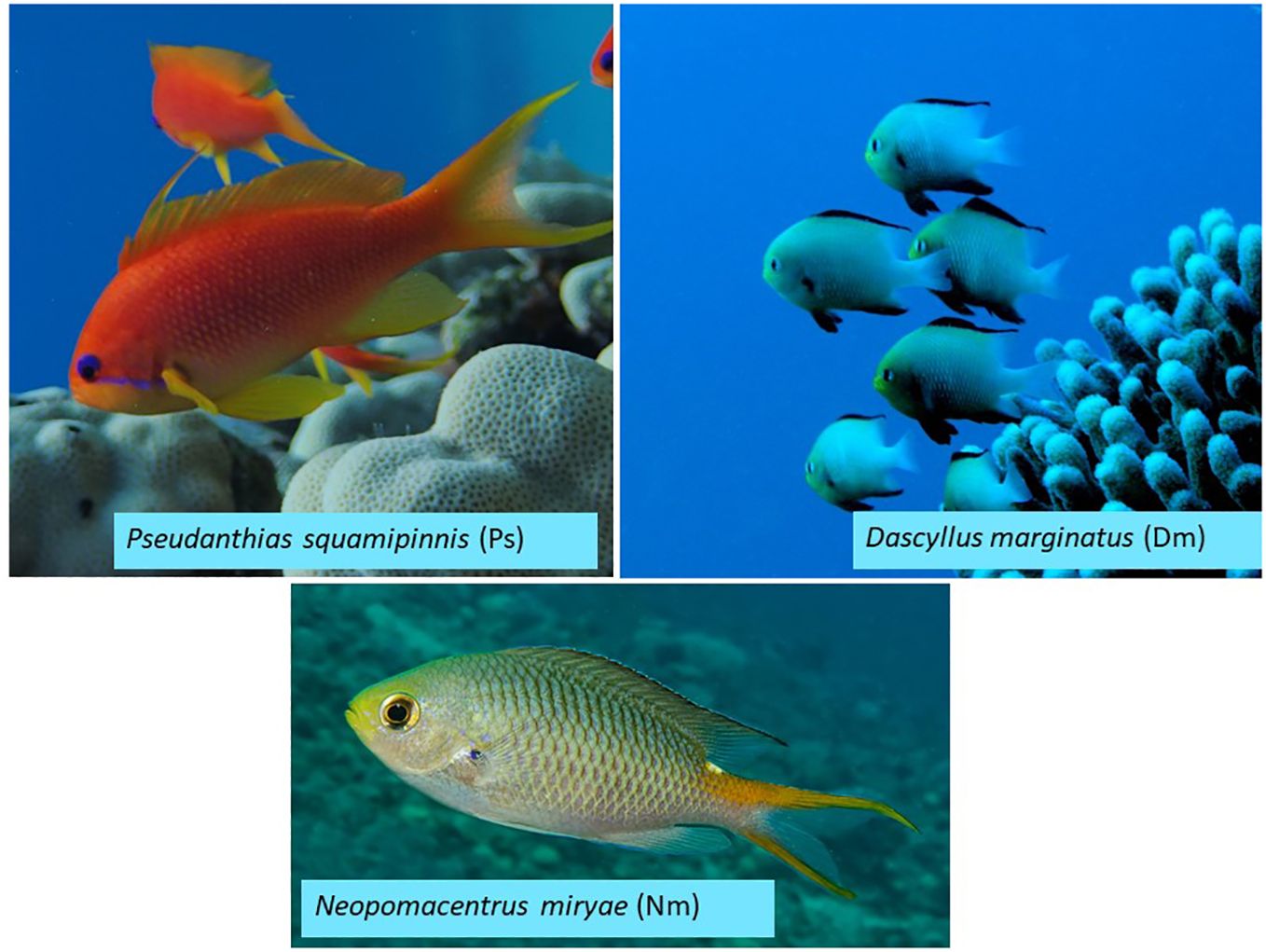
Figure 1 Studied species. Pseudanthias squamipinnis (acronym Ps; mean ± sd standard length 6.2 ± 0.6 cm, N = 5), Dascyllus marginatus (Dm; 4.3 ± 0.5 cm, N = 6), and Neomopacentrus miryae (Nm; 6.8 ± 0.8 cm; N = 5). Fish standard lengths were measured by Ella and Genin (2023) using 3D photography.
The species we studied live in social groups, varying in size from several individuals, in Dm, to hundreds in Ps and Nm (Fricke, 1977; Megdadi et al., 2017). Typical group sizes in those species appear to be related to the type of shelters they use, with those living in association with single coral colonies (Dm) commonly form smaller groups than those associated with large rocky substrates (Ps, Nm) (Fricke, 1977; Khalaf et al., 2006).
Adult individuals were used in our experiments. In Ps, only females were used because males are morphologically different and considerably larger than females and their proportion in the groups are considerably lower (Shapiro and Lubbock, 1980). No such separation was used with the other species, where males and females are morphologically indistinguishable and the gender could not be determined without scarifying the fish.
An additional damselfish, Chromis dimidiata (80-90 mm), was studied as part of a complementary work using the same experimental procedures but different levels of flow speeds and examined parameters. The results of this species are reported in the Supplementary Information.
2.3 The flume
Predation experiments were carried out in a recirculating flume (Figure S1). Briefly, the flume was 330 L in volume, with a glass walled working section, 200 cm in length and 30 × 30 cm in cross-section. The fish were kept inside the working section using plastic-coated wire mesh (1 cm mesh size) 50 cm up-current and a few cm down-current of the work section. A small skeleton of a branching coral or a short piece of PVC pipe, ~4 cm in diameter (Figure S1) were placed as a shelter near the down-current end of the work section. The type of shelter was species dependent, using the coral skeleton for Dm, which in the reef finds shelter inside branching corals, and the pipe for Ps, Nm, and Cd, which typically find shelter in perforated rocks and knolls.
A propeller driven by a 560 W DC motor (Doer Electric Corporation, Cedarburg, Wisconsin, USA) and regulated by an electric motor speed controller generated water movement within the flume. An acoustic Doppler current meter (ADV, Nortek, Norway) was used to calibrate the flume’s controller to generate flows in the range of 3-28.5 cm/s. A 1 cm wide benthic boundary layer was found along the flume’s walls, in which the fish were rarely found. Water temperature was maintained at ± 3° C of the ambient sea-surface temperature. Part of the water in the flume was replaced almost daily, by allowing 1 hr-long exchange with freshly-pumped, pre-filtered seawater. During that time, the propeller was set to high speed and a 65 µm net was tightly inserted in the flume to remove sediments and other suspended particles. Additionally, fouling organisms growing on the walls were scrubbed off every 2-3 weeks, thereby maintaining clear walls for the side-looking video camera. The light intensity in the flume was ~180 µmol m-2 s-1, provided by fluorescent lights from above. This light intensity was similar to that prevailing at the local reef during midday at ~10 m, a depth at which the studied species were common. A natural light-dark cycle was maintained throughout the period of our experiments.
All trials used a single fish in the flume. Different combinations of flow speed and prey density covered the range of 3 to 28.5 cm/s and 210 to 1050 prey m-3, respectively (Table 1). These values were well within those occurring in the coral reef of Eilat (Genin et al., 1995; Genin and Paldor, 1998; Reidenbach et al., 2006; Khrizman et al., 2018) and other coral reefs (e.g., Noda et al., 1992; Gahan et al., 2023).
2.4 Experimental protocol
Fish were collected by scuba divers at depths of 5-14 m in the coral reef off the Interuniversity Institute for Marine Sciences of Eilat (IUI) using either a gill net for fish that reside over rocky substrates (Nm, Ps) or by lightly anesthetizing fish using diluted clove-oil for the coral-associated Dm. The captured fish were transferred to the laboratory for pre-acclimation in 8 L individual holding tanks containing running seawater. Following this initial acclimation, lasting a few days to several weeks, an acclimated fish was transferred to the flume for 3-7 days prior to the onset of its experiments. To avoid acclimation to a certain flow speed, different speeds were used during the acclimation period. A fish was considered acclimated and ready for trials once it readily exited its shelter and commenced feeding immediately after we added prey to the flume.
Throughout that period, the fish were fed with live, 24-36 hrs post-hatching nauplii of Artemia salina. At that stage, their mean [± SD] length was 600 [ ± 70] µm (n = 50), similar to size of the fish’s natural prey in the reef. Note, however, that in comparison with copepods, a common taxon in the fish diet (Hobson, 1991; Noda et al., 1992), Artemia nauplii are poor swimmers and lack an escape response from approaching fish (Trager et al., 1994). However, in the context of this mechanism-oriented study, the use of a single type of non-evasive prey had many advantages, including the logistic ease of getting high numbers of live prey and a use of identical prey in all our experiments, an approach that minimized confounding effects in experiments designed to resolve the effects of only prey density and flow speed.
Each day started with a preparation of syringes (10 ml) filled with the intended numbers of prey for the trials of that day. Using a syringe with a piece of a thin, flexible, transparent tubing attached to its opening, the nauplii were manually collected from a holding tank under a dissecting microscope. Trials using different, haphazardly ordered combinations of prey densities and flow speeds were performed with the same individual fish no more than 4 times a day, with breaks lasting ≥30 min between consecutive trials. To keep the fish hungry during trials, they were kept unfed for at least 12 hrs prior to the initiation of trials. To prevent feeding during the introduction of the nauplii to the flume, the fish were chased into the shelter by introducing a long plastic rod into the flume. During the prey introduction interval, the flow was temporarily raised and the nauplii were gradually ejected from the syringe to the flume near the propellor, assuring their mixing throughout the flume. Following the setting of the controller to the target flow speed, the plastic rod withdrawn, upon which the (now acclimated) fish immediately commenced feeding. Each trial lasted 30 or 60 s, depending on the target flow speed, keeping the trial duration equal to or shorter than the time it took the water to complete a full revolution. Thereby, predation during the trial did not reduce the density of prey in the water flowing by the fish. Upon the completion of a trial, the plastic rod was re-introduced, chasing the fish back to shelter, and the surviving nauplii were collected by filtering the water through a 65 µm plankton net, mounted on an aluminum frame that tightly fitted the flume’s cross section. The filtering stage lasted ~5 min with the flow set at 6 cm/s, thus allowing at least four complete revolutions of the recirculating water through the net. The surviving nauplii were counted using a dissecting microscope. Twenty-eight “control runs” ran under different flow speeds, with no fish in the flume, showed a recapture success of 98 ± 2%.
The difference between the number of nauplii introduced to the flume prior to the trial and those that survived the trial, together with the trail’s duration, were used to calculate predation rates (prey s-1). The values of predation rates were then used to calculate capture efficiency, defined as the percent of prey captured from the total approaching the fish, that is, predation rate divided by the flux. Note that the area used to calculate the flux was the cross section of the flume (width X height of water; 30 X 30 cm).
Details on the setting and number of replicates used are reported in Table 1. The study took about a decade to complete (1999-2009). Within that period, the trials with each species were completed through a single series of back-to-back trials, lasting several months. Within a series, different individuals were collected at the reef one after the other, so that no individual would remain in the lab more than 2-3 months. Long breaks between species allowed interim processing and analyses of data prior to proceeding with the next species. Due to logistic reasons, a break lasting several years was taken prior to the initiation of experiments with Cd.
The collected fish were returned to the reef, preferably to the exact sites where they had been collected. The methods used to collect and handle the fish were carried out under a permit from Israel Nature & Parks Authority and fully complied with the ethical rules of animal treatment at the Hebrew University of Jerusalem.
2.5 Orientation to the flow
A video camera was used to measure the body orientation of the fish with respect to the direction of the flow as viewed from above. Here, our goal was to examine the narrowing of the orientation of the fish with respect to the direction of the oncoming flow as the flow speed was increased, as reported by Kiflawi and Genin (1997) for a few individual fish. Down-looking video recordings of the fish were obtained in the flume during foraging under different flow speeds. Single video frames were digitized using ImageJ in order to measure the angle between the flume’s longitudinal axis (i.e., the flow direction) and the direction of a digitized line connecting the fish’s snout and the base of its tail. The measured angles were converted to absolute values, disregarding left and right with respect to the flow direction. Zero angles referred to a head-on orientation to the flow, while 90° corresponded to a situation where the fish projected its full side to the flow. Video records, each lasting 10 min, were made for each individual (N=3), for each flow speed (3, 6, 9, 12, 15, 18, and 21 cm/s) for each of the 3 studied species. Approximately 50 frames were haphazardly selected from each 10 min record, yielding a total of 3042 data points.
The data were processed to provide the 95th percentile of the angles and their variance for each flow speed. The former value was used as a measure of horizontal width of the up-current “aperture” of the fish’s foraging space. The variance was considered a proxy of the frequency of left-right turnings (hereafter “maneuverability”) of the fish under each flow speed.
2.6 Statistical analysis
The effect of flow speed on predation rates was tested using Mixed-Design Two-Way Repeated Measures ANOVA with 6 levels of flow speed (3, 6, 9, 12, 15, and 21 cm/s), used as Within-Subject Variable. Two levels of prey density, low and medium (210 and 630 prey m-3, respectively) and three species (Ps, Dm, and Nm) were used as Between-Subject Factors, as detailed in Table 1. Bonferroni post hoc was used to test for differences between pairs of species. The same test was used to test the effect of prey density, using its 4-5 levels (210, 420, 630, 840, and, except for Nm, 1050 prey m-3) as Within-Subject Variable, with the two levels of flow speed (4 and 12 cm/s) and species being Between-Subject Factors. The dependent variables, predation rates and capture efficiency, were tested separately using the same tests. For efficiency, log-transformed values were used in order comply with the assumptions of homogeneity of variance (Levene’s test). Following a finding of an overall significant effect of species in the above ANOVA, a Bonferroni Post Hoc test was used to test the differences between pairs of species.
Linear relationships between flow speed and the 95th percentile of the orientation angles and between flow speed and the log-transformed variance of the orientation angles were tested for each flow speed and each species using ANCOVA with flow speed as a covariate and species as a fixed factor and Bonferroni post hoc test of differences between species. To test the equality of the intercepts, flow speed was centered on 12 cm/s by subtracting 12 from the original values.
A logarithmic fit of the relationships between flow speed and the variance of the angles in each category of flow speed was tested in two steps: in the first step, performed separately for each species, the fit to a logarithmic regression was tested. After corroborating that fit, the original values were log-transformed and tested using ANCOVA with flow speed as a covariate and species as a fixed factor. Bonferroni post hoc was used to test for differences between pairs of species.
Assumptions of sphericity and homogeneity of variance were verified in each test, as needed. All statistical tests were carried out using SPSS (v. 28).
3 Results
The results of all statistical tests are listed in Tables 2, 3.
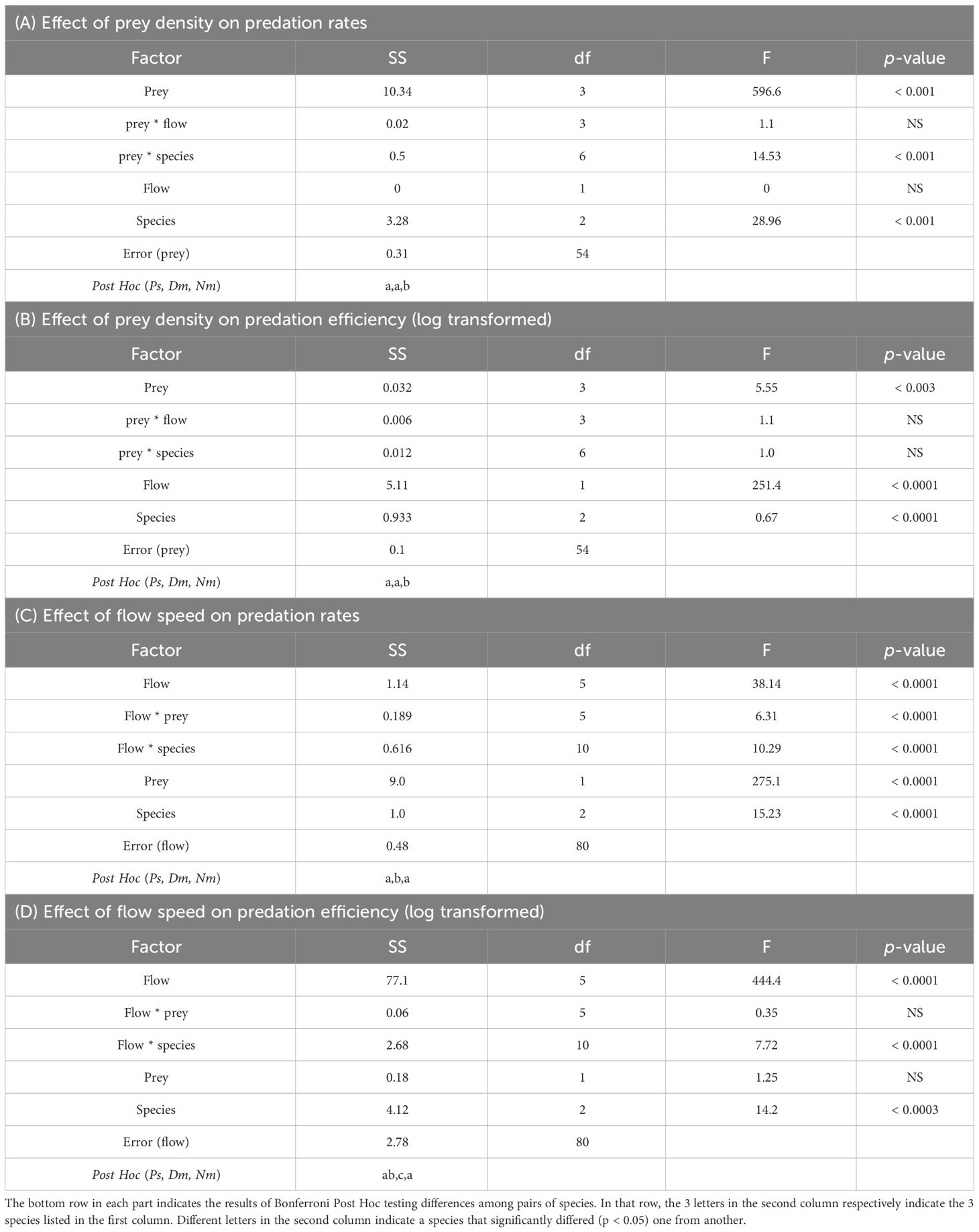
Table 2 Mixed-Design Two-Way Repeated Measures ANOVA testing the effects of prey density (A, B) and of flow speed (C, D) as Within-Subjects factors, on predation rates (A, C) and on predation efficiencies (B, D), with two Between-Subjects Factors: species and flow speed in A, B or species and prey density in C, D.
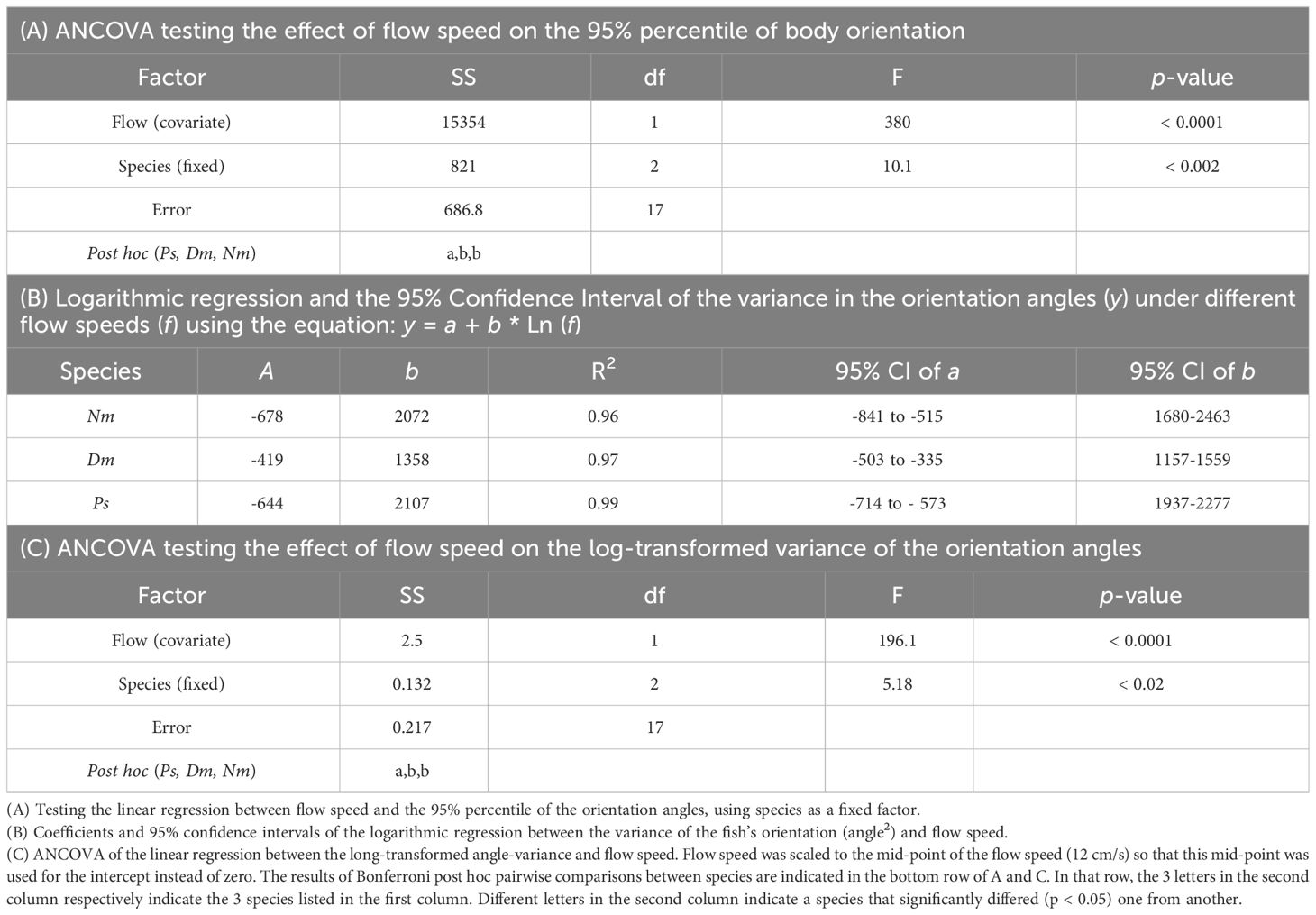
Table 3 Statistical tests of the relationships between flow speed and the fish’s orientation angles with respect to the flow direction.
3.1 Effects of prey density
A nearly linear functional response to increasing density with no apparent saturation was observed in the 3 studied species (Figure 2). Prey density had a significant effect on predation rates (Mixed-Design Two-Way Repeated Measures ANOVA, F1,3 = 596.6, p < 0.0001), with no significant effect of flow speed (two levels) and non-significant interaction between prey density and flow speed. Across all prey densities and two flow speeds included in this analysis, predation rates by Nm were 1.4-1.7 times higher than those of Ps and Dm (Figure 2B), resulting in an overall significant effect of species on predation rates (Mixed-Design Two-Way Repeated Measures ANOVA, F1,2 = 28.96, p < 0.0001) and significant interaction between prey density and species (p < 0.001). However, Bonferroni post hoc test indicated that only the difference between Nm and the other two species was significant (p < 0.05), with no significance difference between Ps and Dm (Table 2).
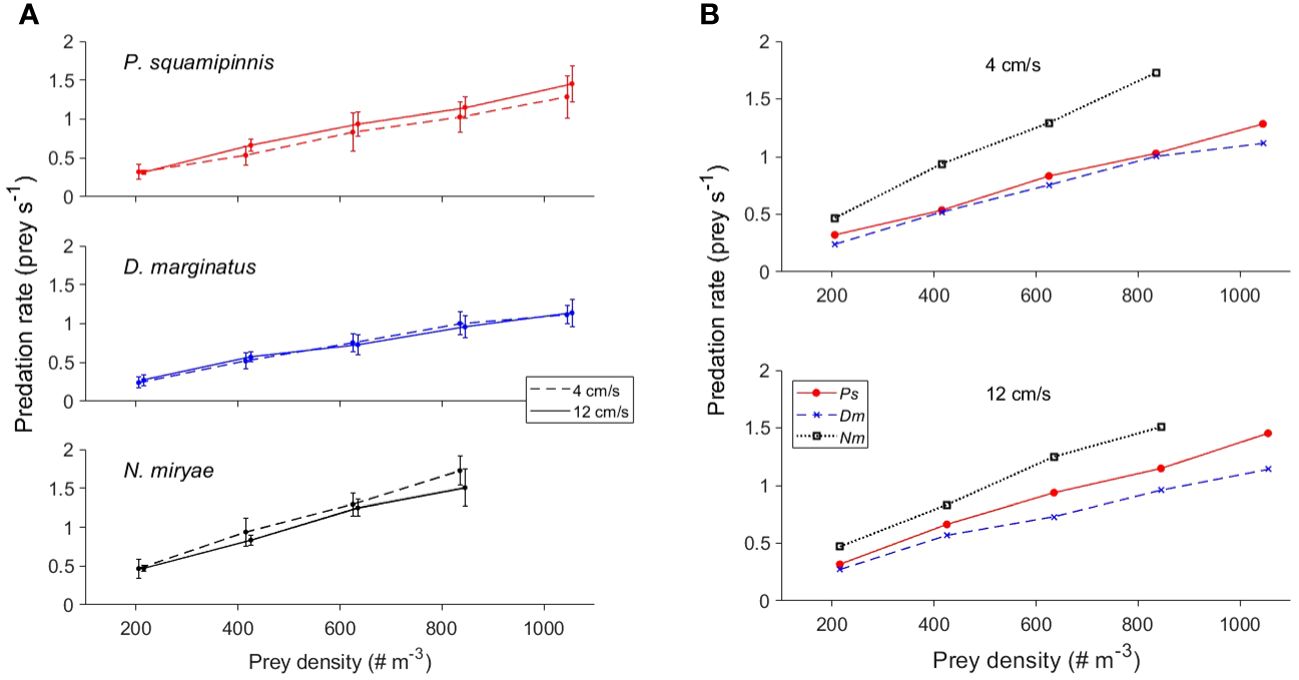
Figure 2 Effects of prey density on predation rate. (A) Mean ( ± sd) predation rates as function of prey density in the 3 species under two levels of flow speed (4 and 12 cm/s; dashed and full lines, respectively). (B) Combined plots of the means of all species (color coded) under flow speeds of 4 (upper panel) and 12 cm/s (lower panel). To improve visualization, error bars are not plotted in (B) For statistics see Table 2.
The linear functional response reported in Figure 2 indicated no saturation up to the maximum prey density examined (1050 prey m-3). The effect of prey density on the efficiency of prey capture (Figure 3) was indiscernible, however statistically significant (Mixed-Design Two-Way Repeated Measures ANOVA, F1,3 = 5.55, p < 0.003). Capture efficiencies were strongly affected by flow speed (p < 0.0001; Table 2), being ~2X higher under slow (4 cm/s) than fast (12 cm/s) flows (p < 0.0001). The overall effect of species on capture efficiency was significant (p < 0.003), however, again, Bonferroni post hoc test indicated that the capture efficiency of only Nm was higher than Ps and Dm, with no significant difference between the latter two. The higher capture efficiencies of Nm were most conspicuous under weak flows (Figure 3B).
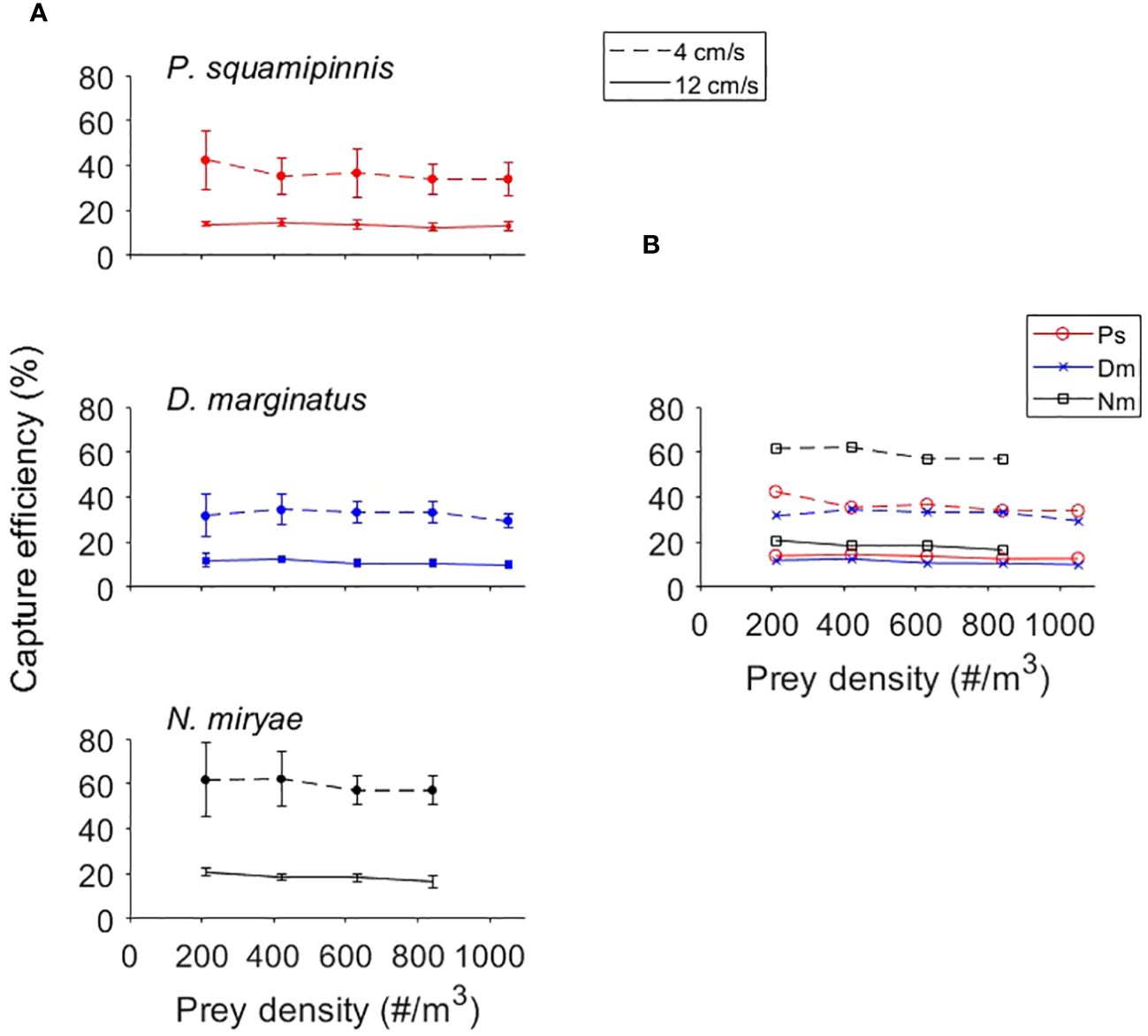
Figure 3 Effects of prey density on capture efficiency, defined as the percent of prey captured by the fish from those approaching it during a trial. (A)- Mean (± sd) efficiencies in the range of 210-1050 prey/m3 under flow speeds of 4 cm/s (dashed lines) and 12 cm/s (full lines) in Ps (top panel), Dm (mid panel) and Nm (lower panel). (B) - Visualization of inter-specific differences for the 3 species (color coded as in A) under flow speeds of 4 cm/s (dashed lines) and 12 cm/s (full lines). Note the higher capture efficiencies of Nm, especially under the weaker flow. For statistics see Table 2.
3.2 Effects of flow speed
The effects of flow speed on predation rates (Figure 4) were generally smaller than those of prey density, especially under conditions of low prey density (210 prey m-3). Under the higher density (630 prey m-3), the functional response was weakly unimodal, exhibiting maxima at different, species-specific speeds. Overall, the effect of flow speed on predation rates was significant (Mixed-Design Two-Way Repeated Measures ANOVA, F1,5 = 9, p < 0.0001; Table 2). Under strong flows (≥ 12 cm/s), Ps exhibited higher predation rates than those of the other species, whereas under weak flows (≤ 9 cm/s) Nm had the highest predation rates (Figure 4B), resulting in an overall significant effect of species (p < 0.0001). However, Bonferroni post hoc test indicated a significant difference only between Dm and the other two species. The interactions between flow speed and prey density and between flow speed and species were highly significant (p < 0.0001; Table 2).
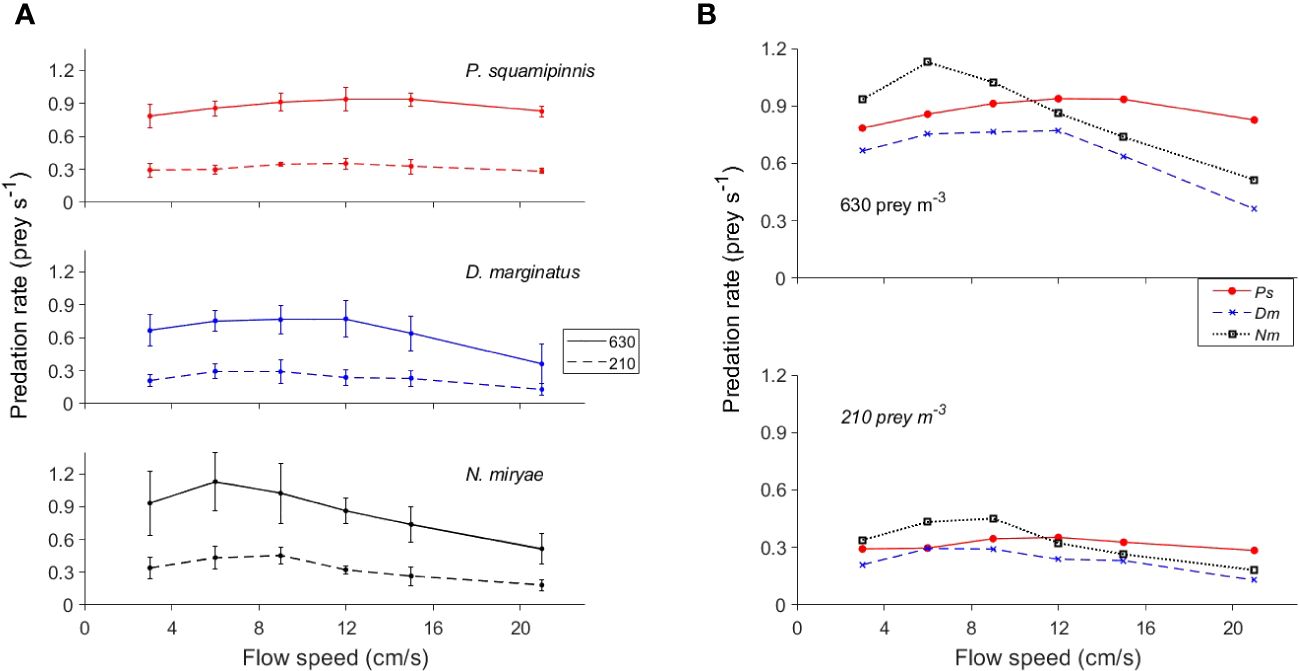
Figure 4 Effects of flow speed on predation rate. (A) Mean ( ± sd) predation rates as function of flow speed in the 3 species under 2 levels of prey density (dashed line: 210 prey m-3; full lines: 630 prey m-3). (B) Combined plots of the means of all species (color coded) under prey density of 210 prey m-3 (upper panel) and 630 prey m-3 (lower panel) prey m-3. To improve visualization, error bars are not plotted in (B). For statistics see Table 2.
A sharp, nearly exponential decrease in the efficiency of prey capture with increasing flow speed was observed in all species (Figure 5), declining from 40-50% at 3 cm/s to approximately 10% at 15-21 cm/s (Mixed-Design Two-Way Repeated Measures ANOVA, F1,5 = 9, p < 0.0001; Table 2). As the effect of flow speed on the rate of predation was small, especially under low prey densities (Figure 4), the observed decline in efficiency was due to the fish’s inability to effectively utilize higher fluxes of prey when the density of prey remained constant but the number of prey passing by the fish per unit of time (i.e., prey flux) increased due to the increasing flow speeds. Neither prey density nor the interaction between flow speed and prey density had a significant effect on the capture efficiency, but the effect of the interaction between flow speed and species was (p < 0.0001; Table 2). Despite a general similarity among the 3 studied species (Figure 5), the small inter-specific differences in the capture efficiency between them were statistically significant (p < 0.0003). Bonferroni post hoc test indicated significant differences (p < 0.05) between Dm and the two other species but not between Ps and Nm (Table 2).
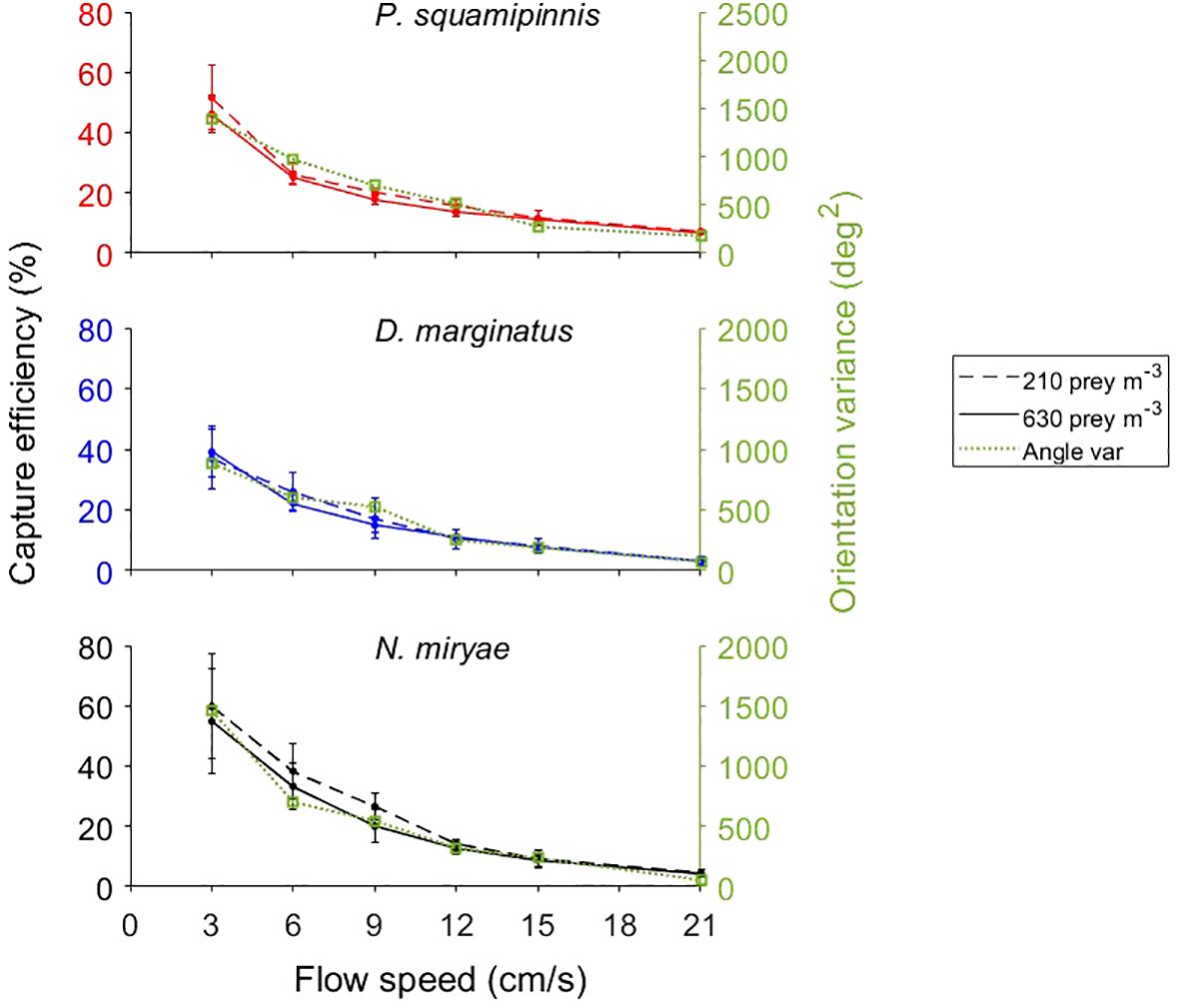
Figure 5 Effects of flow speed on the capture efficiency of prey and on the variance of the orientation angle. Left axes (color coded dashed and full lines): average ( ± sd) capture efficiency as function of flow speed under two levels of prey density: 210 prey m-3 (dashed lines) and 630 prey m-3 (full lines) for Ps, Dm, and Nm. Right axes (green dotted lines): the variance of the orientation angles was copied from Figure 7B) to demonstrate the fit between the three plotted lines for each species. The scale of the right axes was set so that the plots of the orientation variance would be positioned close to the respective plots of capture efficiencies. For statistics see Table 2.
Note that efficiency was calculated using the flux of prey across the full cross section of the flume, disregarding the observation that fish’s foraging space narrowed down with increasing flow speed, as presented by Ella and Genin (2023). In that paper, we show that the decrease in capture efficiency with increasing flow speed (Figure 5) does not always occur when the fluxes are calculated using the actual, flow-dependent “apertures” of the foraging space (addressed below in the Discussion).
3.3 Prey flux
Under identical levels of prey flux, the combinations of weak flow (3-6 cm/s) and high density (630 prey m-3) resulted in 2 to 4 times higher predation rates than in combinations of fast flow (10-21 cm/s) and low prey density (210 prey m-3) (Figure 6). This difference is consistent with the aforementioned findings that predation rates were strongly affected by prey density but not by flow speed (Figures 2, 3).
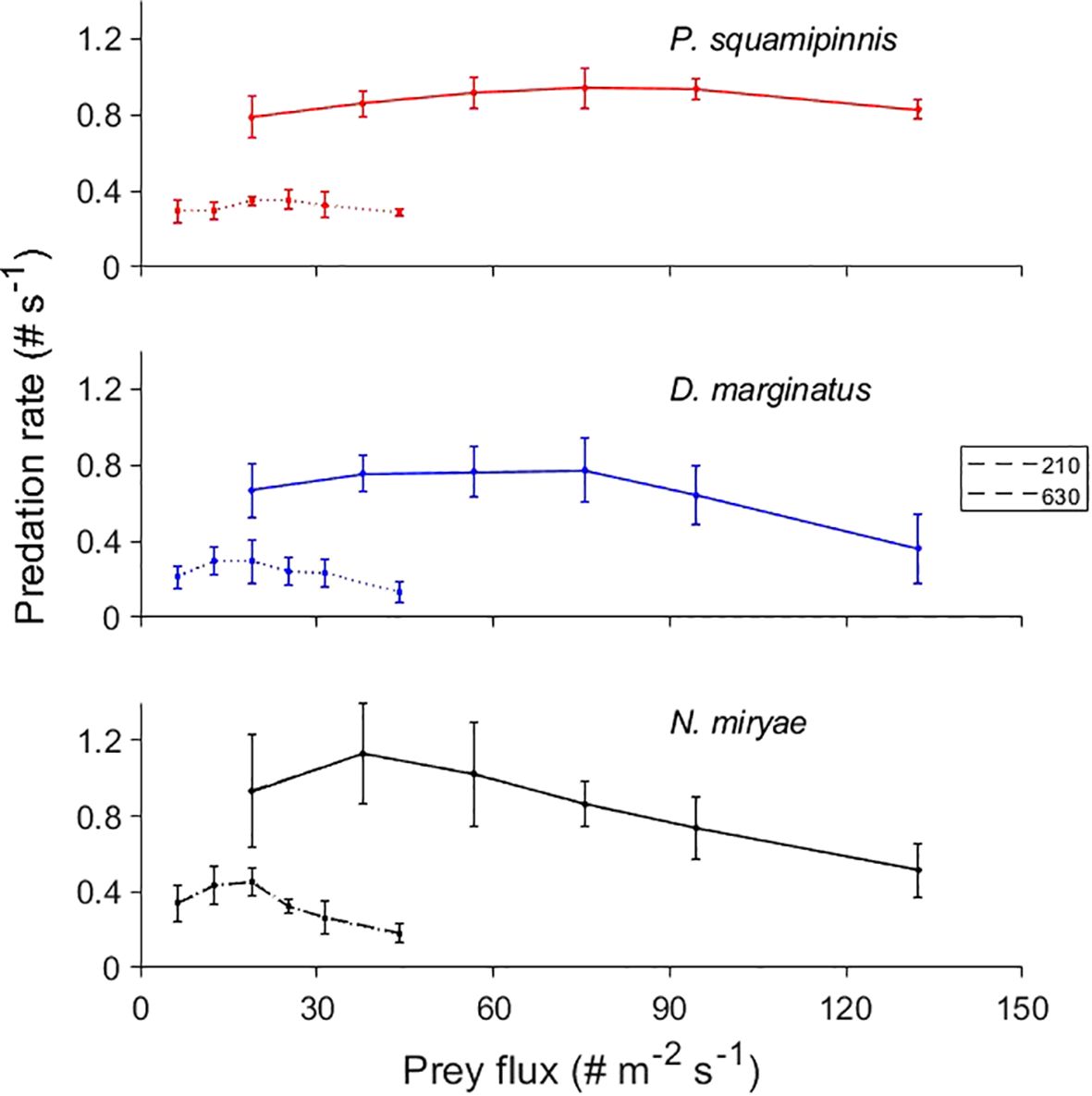
Figure 6 Effects of prey flux on predation rate. Mean ( ± sd) predation rates as function of prey flux in the studied species under prey densities of 210 prey -3 (dotted lines) and 630 prey-3 (full lines). Each point indicates a different combination of prey density and flow speed.
3.4 Body orientation
The linear decrease in the 95th percentile of the orientation angles with increasing flow speed (Figure 7A) was highly significant (p < 0.0001; Table 3). Similarly significant (ANCOVA, p < 0.0001) was the decrease in the variance of those angles with increasing flow speed (Figure 7B), with a significant effect of species (p < 0.02) and a non-significant interaction between species and flow speed (Table 3). Bonferroni post hoc tests indicated that the angles of the 95th percentile and the variance were significantly higher (p < 0.05) in Ps than in the other two species (Figure 7), while Dm and Nm were not significantly different one from another (Table 3).
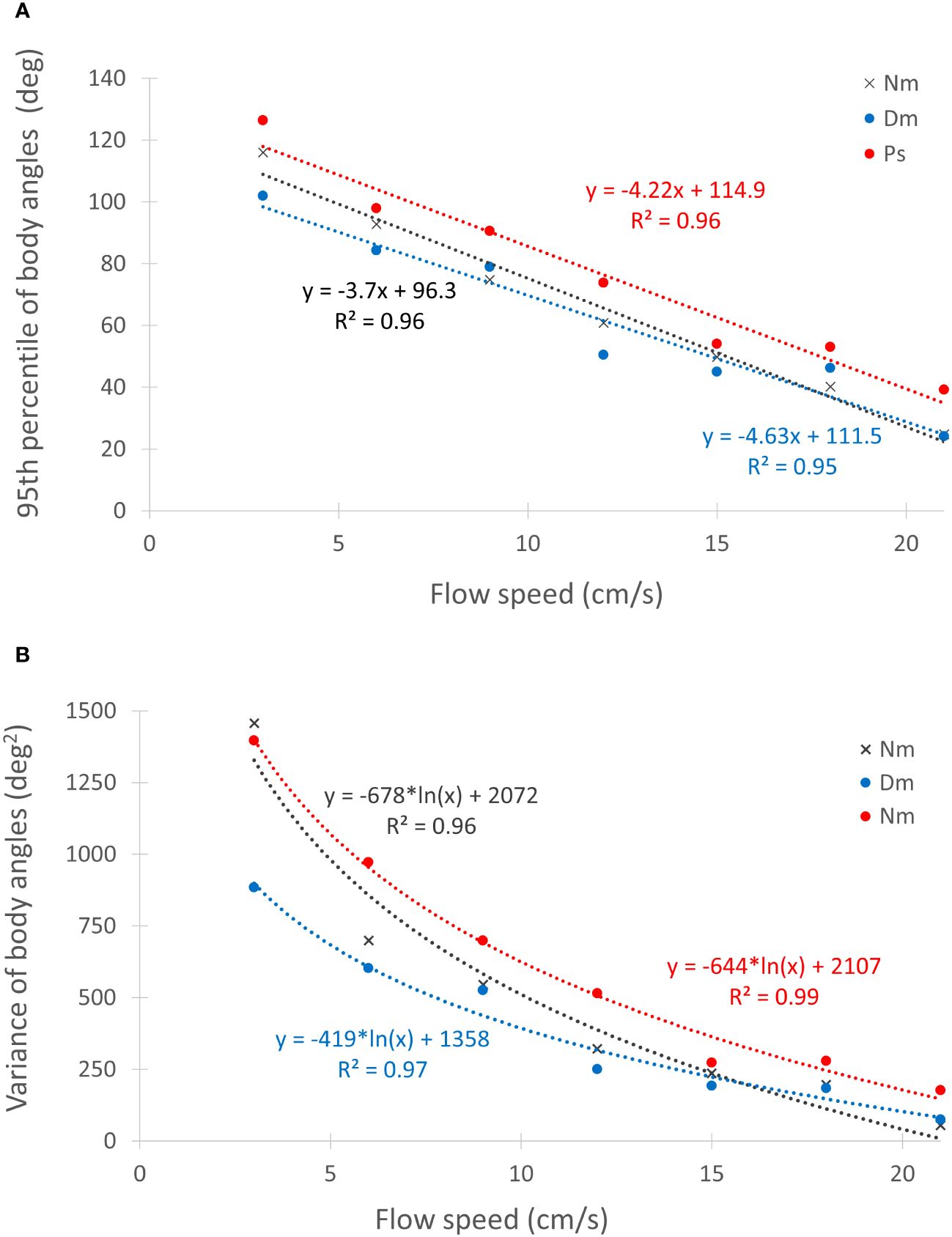
Figure 7 Effect of flow speed on body orientation during foraging. (A) - The 95th percentiles of the orientation angles under different flow speeds for the 3 different species (color coded). Linear regression equations and R2 values are indicated next to each line using the same coded colors. Angles exceeding 90° under weak flows indicate down-current orientations. (B) - The corresponding variance of the orientation angles and their respective logarithmic regression lines. Regression equations and R2 values are color-coded as in (A). For statistics see Table 3.
3.5 C. dimidiata
In general, the trends of predation rates as function of prey density and flow speed observed for Chromis dimidiata (Supplementary Material) were similar to those reported above for Ps, Nm, and Dm.
4 Discussion
The site-attached coral reef fishes we studied were surprisingly similar in their flow-dependent body orientation (rheotaxis), their predation rates and the way those rates were affected by prey density and flow speed. This similarity prevails despite major differences in their taxonomic origin at the family level, their morphologies, the group sizes they typically form, and the types of shelters they use. On a much larger scale, McLean et al. (2021) found that similar environments host similar trait compositions in reef fish assemblages across the world despite the species’ separation by thousands of kilometers and millions of years of evolution. Thus, environmental conditions have likely shaped global patterns in reef fish traits regardless of geography, species identity, or evolutionary history (Bellwood et al., 2017), as observed among the different species explored in our study. This similarity, together with ample evidence that such planktivorous fishes are generalist feeders (Hobson, 1991; Noda et al., 1992; A. Genin unpublished data), suggest that feeding biology is not the principal contributor to the separation of their niches. Other factors such as waves, biomechanical constraints imposed by rapid currents and waves (Fulton et al., 2005; Finelli et al., 2009), different types of shelters the fishes use, and their own exposure to predation (e.g., Hixon, 1991; Hixon and Beets, 1993; Holbrook and Schmitt, 2002) and parasites (e.g., Mikheeva et al., 2020) may have a greater role in determining the habitats the species occupy. For example, Dm is an obligatory resident of live branching corals (Fricke, 1980; Goldshmid et al., 2004), rendering their group sizes limited by the size of the home coral. Therefore, this species cannot form groups of hundreds of individuals, as Ps and Nm do because they occupy large rocky substrates and knolls. Nevertheless, in both types of habitats the fish use a similar rheotactic behavior and exhibit similar responses to changes in prey density and flow speed.
On the other hand, some of the inter-specific differences observed in this study, however small, can contribute to the understanding of the fishes’ adaptations to different types of habitats. For example, differences in predation rates under different flow speeds (Figure 4) indicate that Nm is best adapted to conditions of slow currents while Ps to fast currents. This conclusion agrees well with our extensive, qualitative survey carried out in exposed and sheltered coral reefs around the Straits of Tiran in the southern Gulf of Aqaba (Genin et al., 1994). While in the exposed reefs (mean current speed of ~40 cm/s), Ps flourishes, no Nm is found at those sites. Both species are similarly abundant at sheltered habitats (10-15 cm/s) throughout the Gulf.
A saturation of predation rates under conditions of high prey densities is expected under the classical model of functional response (Holling, 1959). However, no saturation was apparent in our experiments (Figure 2). The most likely explanation is that the range of prey densities we used (210-1050 m-3) did not reach a saturation level. Higher densities sometimes occur over coral reefs, both in Eilat (Holzman et al., 2005) and elsewhere (Noda et al., 1992).
The functional response model characterizes the rates of predation as function of prey density (Holling, 1959; Denny, 2014). There, the density of the prey determines the rate at which a predator encounters prey through a coefficient α, relating capture rate (C) to prey density (N). This scenario applies to predators that actively search for prey across some area or space. Different mechanisms are likely for predators in which prey encounter depends on additional parameters, such as flow speed. Examples include a flow-depended feeding by passive suspension feeders such as crinoids (Leonard et al., 1988) and corals (Sebens and Johnson, 1991; Fabricius et al., 1995) and by site-attached fishes (Kiflawi and Genin, 1997; O’Brien et al., 2001; Clarke et al., 2009; Finelli et al., 2009). Therefore, our findings (Figure 4) that flow speed only had a minor effect on predation rates were surprising. The major effect of prey density on the fish’s functional response (Figure 2), render those fish similar to active, terrestrial predators for which the classical functional response model had been developed. That flow had such a small effect of the fish’s functional response (Figure 4), requires an explanation.
A simple explanation was suggested by Kiflawi and Genin, 1997, based on their observation that the fish’s body orientations to the right and left of the oncoming current became more narrowly distributed around the flow direction as flow speed increased (O’Brien et al., 2001). That is, the “aperture” through which the prey drifts into the fish’s foraging space shrinks as flow becomes stronger. This conclusion was corroborated by our observations, using the 95th percentile of the fish orientation angles (Figure 7A). When such shrinking occurs, an “effective” flux should be considered for each flow speed; that is, the flux through the actual, flow-dependent size of the aperture. This idea was tested in our companion paper (Ella and Genin, 2023), indicating that the above explanation applies for Dm, but not for Ps and Nm. In fact, in Nm predation rates decreased when the flow speed exceeded 9 cm/s despite a corresponding increase in the effective flux. Obviously, mechanisms other than the shrinkage of the aperture should be considered in order to explain the absence of flow-driven increase in predation rates. An alternative explanation is related to the fish’s maneuverability during its foraging movements. If turnings to the left or right of the oncoming current becomes bio-mechanically more limited as flow speed increases, it may restrict the ability of the fish to turn sufficiently fast in order to strike a prey that appears far on its right (or left). Figure 7 showed that wide turns (>70°) with respect to the flow direction rarely occurred when the flow was stronger than 15 cm/s. The close similarity between the logarithmic slopes of the declines in the “maneuverability” and the capture efficiency (Figure 5) supports this explanation. In other words, under the assumption that the variance in the orientation angle can be used as a proxy of foraging maneuverability, the sharp decrease in that maneuverability with increasing flow speeds can explain the absence of increasing predation rates under higher prey fluxes.
An additional explanation refers to the time a fish would need to strike the “next prey”. In situ observations of prey strikes by Dm made by Engel et al. (2021) indicated that under strong currents of 13-17 and >17 cm/s, almost all strikes (95th and 100%, respectively) targeted prey that was found up-current relative to the strikes’ initiation points. Therefore, we suggest that a fish would avoid striking a “next” prey, once it drifted past (down-current of) its present location. Occurrence of such “pass-over” cases become more likely under higher flow speeds. Figure 2 of Ella and Genin (2023) shows that under flow speeds of 15 and 20 cm/s, the mean duration of a strike by Nm, Ps, and Dm is ~380 ms, or a maximum of 2.6 prey/s when prey are caught continuously, one after the other. Under prey density of 630 m-3 and flow speed of 15 cm/s, the flux of prey across the flume (0.3 X 0.3 m) is ~8.5 s-1, whereas under a slower flow (3 cm/s) and the same prey density the flux is 1.7 s-1. This difference means that under a continuous series of strikes, the fish would be able to capture all the prey drifting by it under the weaker flow (3 cm/s) and higher density (630 m-3) but only ~30% of the flux under the stronger flow.
Likely, each of the three explanations suggested above can contribute to the observation that the functional responses of the fish we studied does not depend on prey flux, only on prey density. In our opinion, based on a visualization of the fish foraging movements (Video S1), the shrinking aperture and the reduced maneuverability contribute the most.
Several caveats should be considered. The first, as mentioned above, is our use of Artemia nauplii as prey. Those nauplii are poor swimmers and do not use an escape response, as copepods do (Trager et al., 1994). Copepods are the dominant taxon in the diet in fishes belonging to the guild of the species we studied (Hobson, 1991; Noda et al., 1992; A. Genin unpublished observations). The advantage of using those readily available nauplii was their similarity in size and general morphology to the fish’s natural prey and the uniformity of the prey used in different trials. A second caveat is introduced by our use of a single fish at a time. In nature, all those fish live in social groups, never alone. Our attempts to add more fish to the flume failed because they always incited enduring aggressive interactions, inhibiting predation by both subordinate and dominant individuals. A third caveat that should be considered is our use of a flume with walls that could restrict the fish movements. Obviously, the use of a flume was the only way to independently control flow speed and prey density. On the other hand, the use of a flume with a 30 X 30 cm cross section seems to introduce some, however not an extreme restriction on the foraging of fish in which prey strikes are, on average, 2-6 cm long (Ella and Genin, 2023).
Taken together, those 3 caveats render our results pertinent to the mechanisms underlying the effects of flow speed and prey density, rather than the absolute values of predation rates that are found in situ. While we are confident that the nearly linear increase in predation rate with increasing prey density (Figure 2) holds under in-situ conditions, the slope of that increase and the predation-rate values may be different.
Our study provides the most extensive quantitative information to-date on predation rates by zooplanktivorous coral-reef fishes and the effects of prey density and flow speed on those rates. The rapid advent of ocean optics, including an in-situ visualization of zooplankton (Orenstein et al., 2020; Robinson et al., 2021), together with recent advances in computerized tracking of fish in situ (Engel et al., 2021), assure the prospects of extending our flume study to in-situ conditions.
Data availability statement
The raw data supporting the conclusions of this article will be made available by the authors, without undue reservation.
Ethics statement
The animal study was approved by (1) Israel Nature & Parks Authority and by (2) The ethical committee of animal treatment at the Hebrew University of Jerusalem. The study was conducted in accordance with the local legislation and institutional requirements.
Author contributions
AG: Conceptualization, Formal analysis, Funding acquisition, Investigation, Methodology, Project administration, Resources, Supervision, Validation, Visualization, Writing – original draft, Writing – review & editing. SR: Conceptualization, Formal analysis, Investigation, Methodology, Writing – original draft. MZ: Data curation, Formal analysis, Investigation, Methodology, Validation, Writing – original draft, Writing – review & editing. MK: Conceptualization, Formal analysis, Investigation, Methodology, Supervision, Writing – review & editing.
Funding
The author(s) declare financial support was received for the research, authorship, and/or publication of this article. Israel Science Foundation (ISF) grants 576/96, 455/99, 1211/14.
Acknowledgments
We thank Moty Ohevia for his extensive assistance with the flume, video recording, and other technical tasks. For discussions and comments, we thank R. Holzman, O. Ben-Tzvi, D. Cohencius, S. Eckstein, B. Farstey, R. Goldshmid, R. Kent, S. Sabah, R. Yahel, G. Yahel, D. Genin, and two reviewers. We are indebted to the staff of the Interuniversity Institute (IUI) for general support and logistics and to the Underwater Observatory Park for their help in collecting the fish at the reef. Finally, we thank the Israel Science Foundation for their continuous support.
Conflict of interest
The authors declare that the research was conducted in the absence of any commercial or financial relationships that could be construed as a potential conflict of interest.
Publisher’s note
All claims expressed in this article are solely those of the authors and do not necessarily represent those of their affiliated organizations, or those of the publisher, the editors and the reviewers. Any product that may be evaluated in this article, or claim that may be made by its manufacturer, is not guaranteed or endorsed by the publisher.
Supplementary material
The Supplementary Material for this article can be found online at: https://www.frontiersin.org/articles/10.3389/fmars.2024.1330477/full#supplementary-material
References
Allgeier J. E., Cline T. J. (2019). Comment on “Demographic dynamics of the smallest marine vertebrates fuel coral reef ecosystem functioning”. Science 366. doi: 10.1126/science.aay9321
Begon M., Townsend C. R., Harper J. L. (2006). Ecology: From Individuals to Ecosystems (New York: Wiley).
Bellwood D. R., Goatley C. H. R., Bellwood O. (2017). The evolution of fishes and corals on reefs: Form, function and interdependence. Biol. Rev. 92, 878–901. doi: 10.1111/brv.12259
Benayahu Y., Loya Y. (1977). Space partitioning by stony corals soft corals and benthic algae on the coral reefs of the northern Gulf of Eilat (Red Sea). Helgol. Wiss. Meer. 30, 362–382. doi: 10.1007/BF02207848
Booth D. J. (2016). Ability to home in small site-attached coral reef fishes. J. Fish Biol. 89, 1501–1506. doi: 10.1111/jfb.13043
Brandl S. J., Tornabene L., Goatley C. H. R., Casey J. M., Morais R. A., Côté I. M., et al. (2019). Demographic dynamics of the smallest marine vertebrates fuel coral reef ecosystem functioning. Science 364, 1189–1192. doi: 10.1126/science.aav3384
Bray R. N., Miller A. C., Geesey G. G. (1981). The fish connection: A trophic link between planktonic and rocky reef communities? Science 214, 204–205. doi: 10.1126/science.214.4517.204
Brokovich E., Baranes A., Goren M. (2006). Habitat structure determines coral reef fish assemblages at the northern tip of the Red Sea. Ecol. Indic. 6, 494–507. doi: 10.1016/j.ecolind.2005.07.002
Clarke R. D., Finelli C. M., Buskey E. J. (2009). Water flow controls distribution and feeding behavior of two co-occurring coral reef fishes: II. Laboratory experiments. Cor. Reef. 28, 475–488. doi: 10.1007/s00338-009-0479-7
Coates D. (1980). Prey-size intake in humbug damselfish, Dascyllus aruanus (Pisces, Pomacentridae) living within social groups. J. Anim. Ecol. 49, 335–340. doi: 10.2307/4292
Coughlin D. J., Strickler J. R. (1990). Zooplankton capture by a coral reef fish: an adaptive response to evasive prey. Environ. Biol. Fishes. 29, 35–42. doi: 10.1007/BF00000566
Denny M. (2014). Buzz holling and the functional response. Bull. Ecolog. Soc Am. 95, 200–203. doi: 10.1890/0012-9623-95.3.200
Ella H., Genin A. (2023). Capture of zooplankton by site-attached fish: striking dynamics under different flow speeds and prey paths. Front. Mar. Sci. 10. doi: 10.3389/fmars.2023.1327581
Engel A., Reuben Y., Kolesnikov I., Churilov D., Nathan R., Genin A. (2021). In situ three-dimensional video tracking of tagged individuals within site-attached social groups of coral-reef fish. Limnol. Ocean. Meth. 19, 579–588. doi: 10.1002/lom3.10444
Erez J. (1990). “On the importance of food sources in coral reef ecosystems,” in Coral reefs. Ed. Dubinsky Z. (Elsevier, Amsterdam), 411–418.
Fabricius K. E., Benayahu Y., Genin A. (1995). Herbivory in asymbiotic soft corals. Science 268, 90–92. doi: 10.1126/science.268.5207.90
Finelli C. M., Clarke R. D., Robinson H. E., Buskey E. J. (2009). Water flow controls distribution and feeding behavior of two co-occurring coral reef fishes: I. Field measurements. Cor. Reef. 28, 461–473. doi: 10.1007/s00338-009-0481-0
Fishelson L. (1971). Ecology and distribution of the benthic fauna in the shallow waters of the Red Sea. Mar. Biol. 10, 113–133. doi: 10.1007/BF00354828
Fishelson L., Popper D., Avidor A. (1974). Biosociology and ecology of pomacentrid fishes around the Sinai Peninsula (northern Red Sea). J. Fish Biol. 6, 119–133. doi: 10.1111/j.1095-8649.1974.tb04532.x
Fricke H. W. (1977). Community structure, social organization and ecological requirements of coral reef fish (Pomacentridae). Helgol. Wiss. Meer. 30, 412–426. doi: 10.1007/BF02207851
Fricke H. W. (1980). Control of different mating systems in a coral reef fish by one environmental factor. Anim. Behav. 28, 561–569. doi: 10.1016/S0003-3472(80)80065-0
Fulton C. J., Bellwood D. R., Wainwright P. C. (2005). Wave energy and swimming performance shape coral reef fish assemblages. Proc. R. Soc B 272, 827–832. doi: 10.1098/rspb.2004.3029
Gahan J., Bellwood D. R., Nankervis L., Tebbett S. B. (2023). Spatial and temporal variability in tropical off-reef zooplankton across broad spatial and temporal scales. Mar. Env. Res. 191. doi: 10.1016/j.marenvres.2023.106169
Gaines S. D., Roughgarden J. (1987). Fish in offshore kelp forests affect recruitment to intertidal barnacle populations. Science 235, 479–478. doi: 10.1126/science.235.4787.479
Genin A., Gal G., Haury L. (1995). Copepod carcasses in the ocean. II. Near coral reefs. Mar. Ecol. Prog. Ser. 123, 65–71. doi: 10.3354/meps123065
Genin A., Karp L., Miroz A. (1994). Effects of flow on competitive superiority in scleractinian corals. Limn. Ocean. 39, 913–924. doi: 10.4319/lo.1994.39.4.0913
Genin A., Monismith S. G., Reidenbach M. A., Yahel G., Koseff J. R. (2009). Intense benthic grazing of phytoplankton in a coral reef. Limn. Ocean. 54, 938–951. doi: 10.4319/lo.2009.54.3.0938
Genin A., Paldor N. (1998). Changes in the circulation and current spectrum near the tip of the narrow, seasonally mixed, Gulf of Elat. Isr. J. Earth Sci. 47, 87–92.
Goldshmid R., Holzman R., Weihs D., Genin A. (2004). Aeration of corals by sleep-swimming fish. Limn. Ocean. 49, 1832–1839. doi: 10.4319/lo.2004.49.5.1832
Hamner W. M., Jones M. S., Carleton J. H., Hauri I. R., Williams D.M. (1988). Zooplankton, Planktivorous Fish, and water currents on a Windward Reef Face: great barrier reef, Australia. Bull. Mar. Sci. 42, 459–479.
Hanson K. M., Schnarr E. L., Leichter J. J. (2016). Non-random feeding enhances the contribution of oceanic zooplankton to the diet of the planktivorous coral reef fish Dascyllus flavicaudus. Mar. Biol. 163, 77. doi: 10.1007/s00227-016-2849-3
Hixon M. A. (1991). “Predation as a process structuring coral-reef fish communities,” in The ecology of fishes on coral reefs. Ed. Sale P. (San Diego, USA: Academic Press). doi: 10.1016/C2009-0-02443-X
Hixon M. A., Beets J. P. (1993). Predation, prey refuges, and the structure of coral-reef fish assemblages. Ecol. Mon. 63, 77–101. doi: 10.2307/2937124
Hobson E. S. (1991). “Trophic relationships of fishes specialized to feed on zooplankters above coral reefs,” in The ecology of fishes on coral reefs. Ed. Sale P. (San Diego, USA: Academic Press). doi: 10.1016/C2009-0-02443-X
Holbrook S. J., Schmitt R. J. (2002). Competition for shelter space causes density-dependent predation mortality in damselfishes. Ecology 83, 2855–2868. doi: 10.1890/0012-9658(2002)083[2855:CFSSCD]2.0.CO;2
Holling C. S. (1959). Some characteristics of simple types of predation and parasitism. Canad. Entom. 91, 385–398. doi: 10.4039/Ent91385-7
Holzman R., Genin A. (2003). Zooplanktivory by a nocturnal coral-reef fish: Effects of light, flow, and prey density. Limnol. Ocean. 48, 1367–1375. doi: 10.4319/lo.2003.48.4.1367
Holzman R., Reidenbach M. A., Monismith S. G., Koseff J. R., Genin A. (2005). Near-bottom depletion of zooplankton over a coral reef: II. Relationships with zooplankton swimming ability. Cor. Reef. 24, 87–94. doi: 10.1007/s00338-004-0450-6
Ishikawa K., Wu H., Mitarai S., Genin A. (2022). Effects of prey density and flow speed on plankton feeding by garden eels: A flume study. J. Exp. Biol. 225, 1–10. doi: 10.1242/jeb.243655
Khalaf M. A., Al-Horani F. A., Al-Rousan S. A., Manasrah R. S. (2006). Community structure of the family Pomacentridae along the Jordanian coast, Gulf of Aqaba, Red Sea. Zool. Mid. East 37, 47–62. doi: 10.1080/09397140.2006.10638148
Khalaf M. A., Kochzius M. (2002). Community structure and biogeography of shore fishes in the Gulf of Aqaba, Red Sea. in the Gulf of Aqaba, Red Sea. Helgol. Wiss. Meer. 55, 252–284. doi: 10.1007/s10152-001-0090-y
Khrizman A., Kolesnikov I., Churilov D., Genin A. (2024). Zooplanktivory in garden eels: benefits and shortcomings of being “anchored” compared with other coral-reef fish. Front. Mar. Sci. 10. doi: 10.3389/fmars.2024.1330379
Khrizman A., Ribak G., Churilov D., Kolesnikov I., Genin A. (2018). Life in the flow: Unique adaptations for feeding on drifting zooplankton in garden eels. J. Exp. Biol. 221. doi: 10.1242/jeb.179523
Kiflawi M., Genin A. (1997). Prey flux manipulation and the feeding rates of reef-dwelling planktivorous fish. Ecology 78, 1062–1077. doi: 10.1890/0012-9658(1997)078[1062:PFMATF]2.0.CO;2
Kingsford M. J., MacDiarmid A. B. (1988). Interrelations between planktivorous reef fish and zooplankton in temperate waters. Mar. Ecol. Prog. Ser. 48, 103–117. doi: 10.3354/meps048103
Leonard A. B., Strickler J. R., Holland N. D. (1988). Effects of current speed on filtration during suspension feeding in Oligometra serripinna (Echinodermata: Crinoidea). Mar. Biol. 97, 111–125. doi: 10.1007/BF00391251
McLean M., Stuart-Smith R. D., Villéger S., Auber A., Edgar G. J., MacNeil M. A., et al. (2021). Trait similarity in reef fish faunas across the world’s oceans. Proc. Nat. Acad. Sci. 118, 1–10. doi: 10.1073/pnas.2012318118
Megdadi J. M., Khalaf M. A., Al-Horani F. A., Manasrah R. S. (2017). Community structure of coral reef fishes in relation to habitat and depth in the northern Gulf of Aqaba, Red Sea. Fres. Env. Bull. 26, 1824–1834.
Mihalitsis M., Morais R. A., Bellwood D. R. (2022). Small predators dominate fish predation in coral reef communities. PloS Biol. 20, e3001898. doi: 10.1371/journal.pbio.3001898
Mikheeva V. N., Zhokhovb A. E., Britaeva T. A. (2020). Macroparasite burden of obligate and facultative symbionts in symbiotic communities of scleractinian corals. Biol. Bull. Rev. 10, 456–463. doi: 10.1134/S2079086420050059
Monismith S. G., Genin A. (2004). Tides and sea level in the Gulf of Aqaba (Eilat). J. Geo. Res. 109, C04015. doi: 10.1029/2003JC002069
Morais R. A., Bellwood D. R. (2019). Pelagic subsidies underpin fish productivity on a degraded coral reef. Curr. Biol. 29, 1521–1527. doi: 10.1016/j.cub.2019.03.044
Morais R. A., Siqueira A. C., Smallhorn-West P. F., Bellwood D. R. (2021). Spatial subsidies drive sweet spots of tropical marine biomass production. PloS Biol. 19, e3001435. doi: 10.1371/journal.pbio.3001435
Noda M., Kawabata K., Gushima K., Kakuda S. (1992). Importance of zooplankton patches in foraging ecology of the planktivorous reef fish Chromis chrysurus (Pomacentridae) at Kuchinoerabu Island, Japan. Mar. Ecol. Prog. Ser. 87, 251–263. doi: 10.3354/meps087251
Nonacs P., Smith P. E., Bouskila A., Luttbeg B. (1994). Modeling the behavior of the northern anchovy, Engraulis mordax, as a schooling predator exploiting patchy prey. Deep-Sea Res. 41, 147–169. doi: 10.1016/0967-0645(94)90065-5
O’Brien W. J., Barfield M., Sigler K. (2001). The functional response of drift-feeding Arctic grayling: the effects of prey density, water velocity, and location efficiency. Can. J. Fish. Aquat. Sci. 58, 1957–1963. doi: 10.1139/f01-138
Odum H. T., Odum E. P. (1955). Trophic structure and productivity of a windward coral reef community on Eniwetok Atoll. Ecol. Monog. 25, 291–320. doi: 10.2307/1943285
Orenstein E. C., Ratelle D., Briseño-Avena C., Carter M. L., Franks P. J. S., Jaffe J. S., et al. (2020). The Scripps Plankton Camera system: A framework and platform for in situ microscopy. Limnol. Ocean. Meth. 18, 681–695. doi: 10.1002/lom3.10394
Reidenbach M. A., Monismith S. G., Koseff J. R., Yahel G., Genin A. (2006). Boundary layer turbulence and flow structure over a fringing coral reef. Limnol. Ocean. 51, 1956–1968. doi: 10.4319/lo.2006.51.5.1956
Robinson K. L., Sponaugle S., Luo J. Y., Gleiber M. R., Cowen R. K. (2021). Big or small, patchy all: Resolution of marine plankton patch structure at micro- to submesoscales for 36 taxa. Sci. Adv. 7, (47). doi: 10.1126/sciadv.abk2904
Sale P. F. (1971). Extremely limited home range in a coral reef fish, dascyllus aruanus (Pisces; pomacentridae). Copeia 1971, 324–327. doi: 10.2307/1442839
Sebens K. P., Johnson A. S. (1991). Effects of water movement on prey capture and distribution of reef corals. Hydrobiol 226, 91–101. doi: 10.1007/BF00006810
Shaked Y., Genin A. (2023). The Israel National Monitoring Program in the Northern Gulf of Aqaba – scientific report (Jerusalem, Israel: Israel Ministry of Environmental Protection). Available at: https://iui-eilat.huji.ac.il/uploaded/NMP/reports/NMP%20Report%202022.pdf.
Shapiro D. Y., Lubbock R. (1980). Group sex ratio and sex reversal. J. Theor. Biol. 83, 411–426. doi: 10.1016/0022-5193(80)90048-X
Siqueira A. C., Morais R. A., Bellwood D. R., Cowman P. F. (2021). Planktivores as trophic drivers of global coral reef fish diversity patterns. Proc. Nat. Acad. Sci. 118, e2019404118. doi: 10.1073/pnas.2019404118
Trager G., Achituv Y., Genin A. (1994). Effects of prey escape ability, flow speed, and predator feeding mode on zooplankton capture by barnacles. Mar. Biol. 120, 251–259. doi: 10.1007/BF00349685
Wainwright P., Carroll A. M., Collar D. C., Day S. W., Higham T. H., Holzman R. A. (2007). Suction feeding mechanics, performance, and diversity in fishes. Integ. Comp. Biol. 47, 96–106. doi: 10.1093/icb/icm032
Wyatt A. S. J., Lowe R. J., Humphries S., Waite A. M. (2010). Particulate nutrient fluxes over a fringing coral reef: Relevant scales of phytoplankton Production and mechanisms of supply. Mar. Ecol. Prog. Ser. 405, 113–130. doi: 10.3354/meps08508
Keywords: zooplankton, predation, foraging, site-attached, movement, Red Sea
Citation: Genin A, Rickel S, Zarubin M and Kiflawi M (2024) Effects of flow speed and prey density on the rate and efficiency of prey capture in zooplanktivorous coral-reef fishes. Front. Mar. Sci. 11:1330477. doi: 10.3389/fmars.2024.1330477
Received: 30 October 2023; Accepted: 23 February 2024;
Published: 21 March 2024.
Edited by:
Stuart Humphries, University of Lincoln, United KingdomReviewed by:
Jesse Balaban-Feld, University of Saint Joseph, United StatesGeoffrey Mazue, The University of Sydney, Australia
Copyright © 2024 Genin, Rickel, Zarubin and Kiflawi. This is an open-access article distributed under the terms of the Creative Commons Attribution License (CC BY). The use, distribution or reproduction in other forums is permitted, provided the original author(s) and the copyright owner(s) are credited and that the original publication in this journal is cited, in accordance with accepted academic practice. No use, distribution or reproduction is permitted which does not comply with these terms.
*Correspondence: Amatzia Genin, YS5nZW5pbkBtYWlsLmh1amkuYWMuaWw=