- Interdisciplinary Centre of Marine and Environmental Research (CIIMAR), University of Porto, Matosinhos, Portugal
Biofouling poses a significant challenge to bivalve aquaculture affecting both the target culture and/or the immersed infrastructure. In suspended bivalve cultures (e.g., oysters and scallops), biofouling accumulation leads to additional labor demands and increased costs for the maintenance of underwater structures. Given that the inherent properties of materials used in farming infrastructure influence the formation of fouling communities, evaluating how these materials perform under diverse environmental conditions can help the industry select the most effective materials for preventing or minimizing biofouling growth. This study evaluates the impact of aquaculture material and environmental conditions on biofouling, focusing on two commonly used plastic polymers in marine aquaculture: polyamide (PA) and high-density polyethylene (PE). Both untreated and color-additive treated polymers were tested for their response to fouling development. Performance was gauged by total fouling wet weight and the extent of fouling-induced mesh occlusion. Experimental panels were deployed for 4 months (from May to September 2021) in estuarine (oyster farm) and marine (port) environments on the northern coast of Portugal. The marine sites exhibited greater fouling species diversity, while higher biofouling loads were found in the subtidal estuarine area. Within 3 months, complete mesh occlusion occurred mainly due to colonial hydroids (Obelia dichotoma) in the subtidal site. In contrast, panels deployed in the intertidal estuarine area had lower fouling biomass and mesh occlusion. Notably, significant differences between polymer types and treatments were only evident in the estuarine intertidal area, with long air exposure during low tide. White panels outperformed orange ones in fouling biomass, and PA panels outperformed PE panels in mesh occlusion. These differences were attributed to the settlement and growth of the acorn barnacle Austrominius modestus, known to favor dark-colored and less hydrophilic surfaces. Considering that oyster production in intertidal areas is one of the most important aquaculture industries globally, these findings offer valuable insights into material selection and characteristics that can mitigate fouling loads and their associated impacts. These results could also be relevant for other forms of bivalve aquaculture where infrastructure-related biofouling presents a challenge.
1 Introduction
Biofouling, the undesired colonization of a wide range of micro- and macro-organisms in submerged structures, is responsible for 5-10% of production costs (Lane and Willemsen, 2004), being one of the main barriers to efficient and sustainable aquaculture production (Dürr and Watson, 2010). In the bivalve aquaculture industry, biofouling control may account for over 14.7% of total operating expenses in the USA (Adams et al., 2011) and up to 30% of oyster production costs in Europe (CRAB, 2007). The effects of biofouling on cultivated bivalve species and farming infrastructure may occur by different processes (Fitridge et al., 2012): (1) direct physical damage by fouling organisms (e.g., epibiotic calcareous organisms) that adhere at the shell, weakening the entire structure and affecting bivalves’ vulnerability to disease and/or their visual appearance; (2) mechanical interference in the functioning of the shell, affecting feeding capacity and increasing the susceptibility to predators; (3) biological competition for resources, such as food and space, with negative consequences on growth and condition of the bivalves; (4) modification of the environmental conditions by the colonization on the submerged infrastructures, leading to reduced water flow, accumulation of waste, decreased oxygen levels, and reduced food availability; and (5) increased weight of the aquaculture structures and gears (e.g., panels, nets, trays, ropes and floats) due to biofouling biomass accumulation, causing an increase in the production costs and maintenance frequency. Finally, biofouling may also induce toxic effects on aquaculture species as some of these organisms produce secondary metabolites that act against predators or competitor species, negatively affecting the target aquaculture species.
The most widely used strategy to control biofouling in finfish aquaculture is to apply antifouling biocides to the surface of submerged structures (Chambers et al., 2006; Nikolaou et al., 2014). Despite research efforts to replace biocides with more eco-friendly alternatives (Vilas-Boas et al., 2020; Pereira et al., 2021), copper-based coatings are still generally applied to cages and nets to inhibit the settlement of fouling organisms (Bannister et al., 2019). In shellfish farming, biofouling control requires alternative strategies due to the toxicity, bioaccumulation, and persistence of these metals in target cultured species (Fitridge et al., 2012). Non-toxic coatings, such as wax/silicon-based coatings (Hodson et al., 2000; de Nys and Ison, 2004), have been applied with varying degrees of success against fouling adhesion. However, the mechanical removal of fouling organisms through daily air exposure and manual cleaning remains the most efficient and cost-effective approach (Watson et al., 2009; Reyes-Salarda et al., 2020). Oyster growers commonly place their stock in the intertidal zone to reduce fouling (Moroney and Walker, 1999), and in mussel aquaculture, many farmers expose the mussels for one or two days to air (Papadopoulos et al., 2023). However, this technique may not be feasible for all farming systems.
The formation and development of biofouling communities rely on both extrinsic factors (environmental conditions), including salinity, temperature, light, availability of nutrients, and water circulation (Railkin, 2004), and on intrinsic factors (surface properties), such as composition, roughness, shape, color, among others. Studies investigating the effects of material type and features on the formation and development of fouling communities may provide effective and more environmentally sound solutions for biofouling control. The surface energy and roughness seem to play a significant role in the initial stages of fouling by microorganisms (Characklis and Cooksey, 1983; Artham et al., 2009), while surface color seems to influence the larval settlement of sessile invertebrates (Dahlem et al., 1984; Satheesh and Wesley, 2010) and algal spores (Hodson et al., 2000; Swain et al., 2006). In the specific case of acorn barnacles Amphibalanus amphitrite (Darwin, 1854), previous studies have reported that their recruitment was significantly enhanced on red and blue panels compared to white and yellow ones, confirming a preference of these organisms for darker and less reflective substrates (Su et al., 2007). Conversely, higher abundances of the macroalgae Ulva rigida C.Agardh, 1823 were found in fish cages that used white nets compared to black ones, suggesting a settlement preference for lighter surfaces (Hodson et al., 2000).
Aquaculture cages and netting are generally made from plastic such as polyamide (nylon) and polyethylene (low and high-density polyethylene) (Amara et al., 2018), with the latter being the most commonly used type in shellfish culture infrastructure (e.g., longlines, baskets, floating mesh bags, bottom cages, and trays). High-density polyethylene, compared to polyamide, has a smoother surface structure with the potential to delay the onset of biofouling and facilitate cleaning maintenance (Bloecher and Floerl, 2020). However, there have been limited studies reporting the effect of these polymers on biofouling growth (e.g., Artham et al., 2009; Edwards et al., 2015). Researching the spatiotemporal dynamics of fouling communities and their growth on various aquaculture materials would enhance our understanding of the most effective materials. This, in turn, could guide the development of improved alternatives for controlling biofouling in aquaculture settings (Fitridge et al., 2012). Considering this, the present study aimed to characterize the development of biofouling on widely used plastic materials and to test whether the presence of pigment additives in the materials may contribute to biofouling prevention and/or attenuation. The study was conducted in different environmental settings (marine and estuarine waters), covering a range of conditions experienced in bivalve aquaculture. The results may ultimately be used to minimize the impacts of biofouling by optimizing the grow-out bivalve aquaculture equipment.
2 Materials and methods
2.1 Test materials
For this study, plastic samples in the form of square mesh panels (20 cm x 20 cm, 0.5 cm thickness, 1.24 cm2 mesh) of different polymers and treatments were considered, resulting in four different conditions: polyamide (PA) and high-density polyethylene (PE) polymers, both natural (untreated) and treated with an additive (pigment masterbatch) (Table 1). These plastic samples were manufactured using standardized industrial procedures by an injection molding company (Ernesto São Simão Lda.). The additive of the antioxidant type was supplied by Flexaco company (RNM group) and added to the polymer base to give a specific orange color to the plastic, in contrast with the untreated white panels.
The surface properties of the polymers and treatments were not examined. Previous studies described polyamide as a more hydrophilic (high surface energy and high wettability) material compared to high-density polyethylene (low surface energy and low wettability) (Artham et al., 2009; Carl et al., 2012; Bloecher et al., 2013). Although most materials are susceptible to biofouling, hydrophobic substrates are considered to produce more pronounced fouling than hydrophilic materials (Kang et al., 2006).
2.2 Study sites and experimental design
Panel samples were tested at four study sites with distinct hydrodynamic properties: two in the vicinity of an estuarine oyster aquaculture and the other two under marine conditions associated with a commercial port (Port of Leixões) (Figure 1).
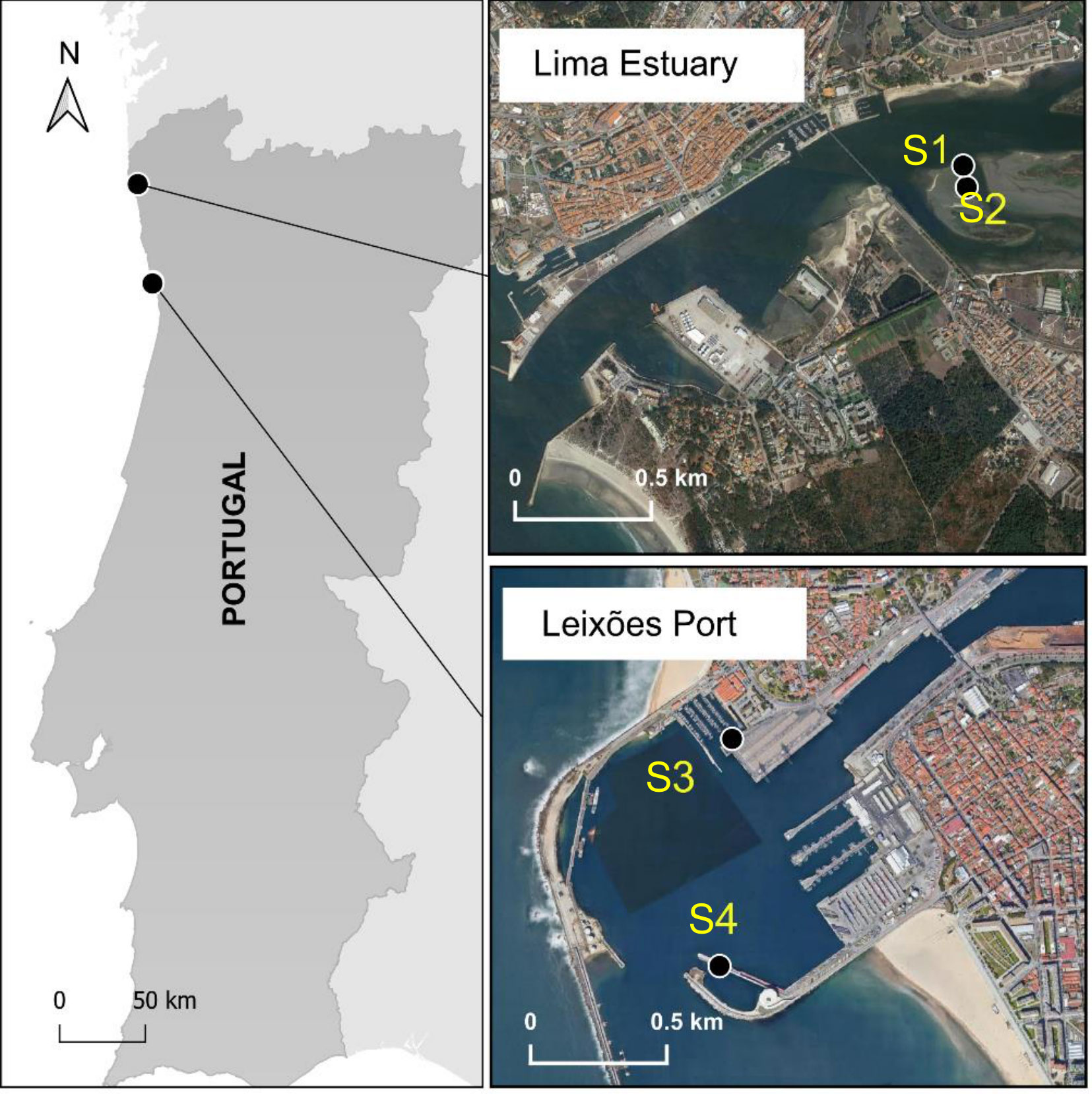
Figure 1 Study sites on the North coast of Portugal: Lima River Estuary (S1 – subtidal and S2 – intertidal) and Port of Leixões (S3 – recreational marina and S4 – cruise-ship terminal).
Estuarine testing was conducted at an oyster farm located in a sandflat in the Lima River Estuary (NW Portugal, 41°41’31” N, 8°48’42” W). The Lima Estuary is a temperate mesotidal estuary with semidiurnal tides (Ramos et al., 2006). Two sites at different tidal heights were selected, one in the subtidal section of the sandflat (S1), a permanently submerged zone, and another in the upper intertidal (S2), with aerial exposure at low tide (4h per low tide). Marine sites were selected in two marinas, one next to the North breakwater (41°11’08”N, 8°42’14”W), used by recreational boats, and the other next to the South breakwater of the Port of Leixões (41°10’42”N, 8°42’15”W), in the cruise-ship terminal (S3 and S4, respectively). Commercial marinas and ports are preferred locations for marine fouling assessments, particularly with regard to the study of colonization by non-indigenous species (Azevedo et al., 2020). The selected locations, in addition to the ease of access and sampling, were previously studied regarding biofouling communities (Azevedo et al., 2020), contributing background information for the present study.
At all study sites, three replicates of each of the four panel types were used, resulting in a total of 12 panels. In the S3 and S4 sites, panels were attached with plastic cable ties to PVC frames, and the frames were deployed vertically at a 2 m depth. In the S1 and S2 sites, panels were individually attached to the longlines used to suspend oyster cages, being exposed to the same tidal variations as farmed oysters.
2.3 Biological colonization assessment and monitoring
All panels were deployed in May 2021 and sampled monthly until the experiment was concluded in September 2021. This time frame encompassed the highest biofouling season (spring-summer) expected at the studied latitude (Azevedo et al., 2020).
The biological colonization of the different test materials was assessed by two methods: 1) measurements of the individual wet weight (WW) of the panels in each month, and 2) image analysis of the panels to estimate the percentage of mesh occlusion (PMO). Once a month, panels were pulled from the water and allowed to drip before being weighed on an electronic balance and photographed. The total wet weight (± 1 g) of attached fouling was calculated by subtracting from each sample the wet weight of the clean (unfouled) panels. Fouling community biomass was then expressed as g/400 cm2, relative to the total area of the panel. In June, at sampling site S2, no weights were recorded due to equipment malfunction.
Images of one side (the same side each time) of each panel were taken, with the panels held up in the air against a contrasting background, using a digital camera attached to a fixed stand to reduce differences in scale between images. The images were analyzed using the open-source ImageJ (Schneider et al., 2012) software to determine the percentage of mesh occlusion (PMO) of the different types of materials. This parameter has been developed and refined to quantify biofouling on aquaculture nets (Braithwaite et al., 2007). The PMO is a direct measure of the degree of accumulation of organisms and is calculated with reference to the mesh size of the panels before being placed in the water. For its calculation, measurements were made of 36 mesh opening areas (6 x 6) of the central zone of each panel, thus obtaining an average value for the area of each material. The edges of the panels were excluded from the analysis as they are subject to handling associated with fixing plastic cable ties.
PMO was then calculated as follows (modified from Braithwaite et al., 2007):
PMO (%) = [ 1 - (Mesh Area at tx/Mesh Area at t0)] x 100,
where Mesh area at t0 corresponds to the mesh area measured before the panels were immersed and Mesh area at tx corresponds to the mesh area measured at each monthly sampling.
Images of the panels were also used for a qualitative assessment of the predominant fouling taxa over the study period. At the end of the study period (September), destructive sampling was conducted with all panels being removed and transported to the laboratory for species identification. All the material collected from the plastic panels was sorted and identified under the stereomicroscope to the lowest possible taxonomic level, by specialists of different groups (see Acknowledgements). Taxa were listed according to the World Register of Marine Species nomenclatural system (WoRMS Editorial Board, 2021).
2.4 Environmental parameters
At each sampling site and time, water physical-chemical parameters such as temperature (°C), salinity, dissolved oxygen concentration (mg L-1), oxygen saturation (%), and pH were measured monthly, with multiparametric probes (HI98194 Hanna Instruments and YSI EXO 1 sonde) properly calibrated. In addition, surface and bottom water samples were collected for further analytical quantification of nutrients (nitrites, phosphates, ammonia, and silica) and chlorophyll a concentrations (Ramos et al., 2012). Water samples were collected using a water sampler (Hydro-Bios), transferred to acid-washed 1.5 L bottles, transported to the laboratory in cool boxes, and processed immediately.
2.5 Data analysis
All biological and environmental data were expressed through means and their respective standard deviations. Statistics and graphics were performed in R (R Core Team, 2019), using the libraries “vegan” and “ggplot2”.
The environmental data measured were first evaluated for collinearity with a correlation matrix and variance inflation factor (VIF) values (Zuur et al., 2010). Parameters with a correlation higher than 0.85 were removed, and the remaining parameters were normalized. Data was then explored with a principal component analysis (PCA) to highlight differences among sites.
Biological data, namely, the total biomass of fouling organisms and the percentage of mesh occlusion (PMO), were analyzed with a permutational analysis of variance (PERMANOVA) (Anderson et al., 2008) applied to univariate data using the “adonis” function within the “vegan” package (Oksanen et al., 2019). This analysis aimed to evaluate differences among sites (S1 to S4), treatments (natural, orange), and polymers (PA and PE) using a 3-way crossed design with fixed factors. Because the panels were repeatedly analyzed over time, we used a “repeated measures” approach to account for the non-independence of the data (Anderson et al., 2008). In this case, we considered each month as a variable, and analyzed the multivariate matrix of the set of 4 months (June, July, August, and September). These factors were also tested for multivariate dispersions using PERMDISP. The data were initially square root-transformed, and the Bray-Curtis dissimilarity matrix was calculated upon which the PERMANOVA was performed (Anderson et al., 2008). Significant differences were visually displayed using Principal Coordinate Analysis (PCoA) plots.
3 Results and discussion
3.1 Environmental conditions
The water quality parameters at the different sites during the 4-month period are shown in Table 2 and Supplementary Table S1. Water temperature was generally higher in the estuarine sites (S1 and S2) compared to the marine sites (Table 2). However, no clear monthly variations were observed, except for a sharp increase in September in all sites (Table S1). The relatively low water temperatures observed in the marine locations (S3 and S4) in summer (15.6 ± 0.8°C) may have been associated with the upwelling events that are frequent in Portuguese coastal waters at this time of the year (Fiúza et al., 1982; Ramos et al., 2006). Estuarine waters presented higher Chl a concentrations (5.58 ± 1.54 μg/L) when compared to marine sites (S3: 2.22 ± 1.59 μg/L; S4: 2.00 ± 1.12 μg/L), presenting a peak value of 7.83 μg/L in July.
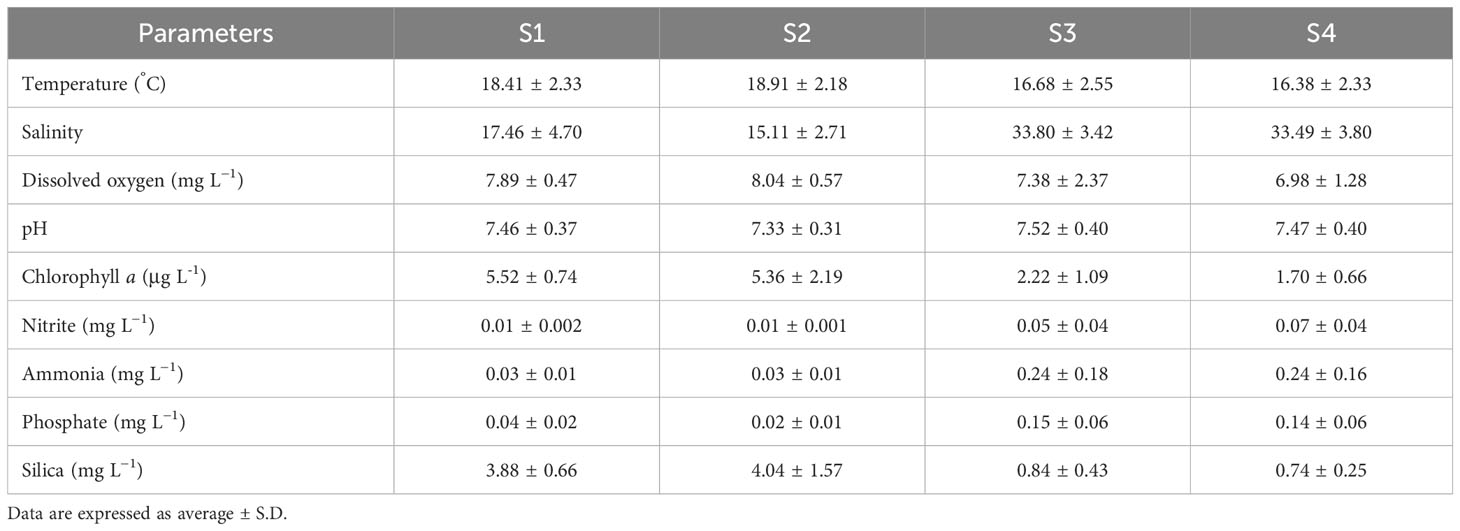
Table 2 Environmental parameters that were measured at the study sites (S1- S4) in the period from May to September 2021.
Overall, the concentration of nitrites, ammonia, phosphates, and silica was considerably lower in the estuary than in the marine sites (Table 2). Together with the reduced oxygenation of the water, particularly in S4, these results indicate lower water quality in the marine sites. This might be related to the fact that these sampling sites are located within the Port of Leixões, a confined area with less hydrodynamics and with potential contamination due to port activities and drainage from a local tributary (Leça River) into the seaport.
The PCA analysis highlighted the differences in the analyzed parameters, particularly on the horizontal axis (PC1, 45% of total variance explained), which distinctly separates estuarine and marine samples (Figure 2). The main parameters responsible for such difference were the higher salinity and nutrient content in marine sites, in contrast to the estuarine waters with lower salinity, higher water temperatures, and concentrations of silica and chlorophyll a. Along the vertical axis (PC2, 22% of explained variance), there seemed to be a separation of the marine samples in July and August, associated with higher dissolved oxygen content (Figure 2).
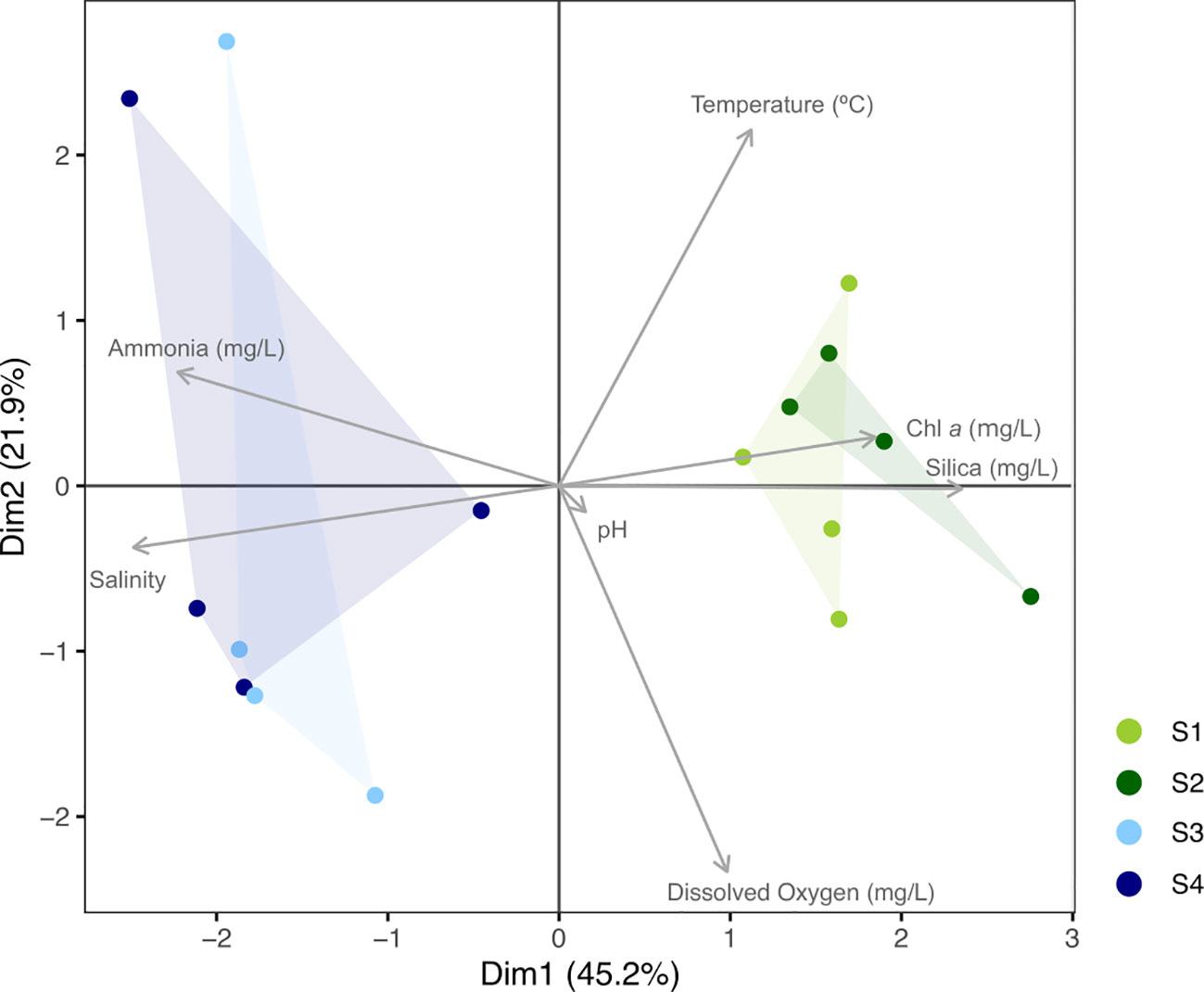
Figure 2 PCA ordination plot showing the position of all sites (color symbols) in the environmental gradient of the non-collinear variables (explained variance: PC1 = 45.2%, PC2 = 21.9%). Estuarine sites (S1 and S2) are in green, and marine sites (S3 and S4) are in blue.
3.2 Biofouling development
Biological colonization of the test panels was observed in the different sampling locations over time (Figure 3; Supplementary Table S2). At the end of the first month of immersion (June), the panels presented an extensive microbial biofilm, composed of organic detritus, diatoms, cyanobacteria, and other microalgae species typical of coastal and estuarine environments (data not shown). Some macrofouling species were also observed, including algal spores, arborescent bryozoans (Bugula sp.), and bivalve spat (Mytilus sp.) at the marine sites (S3 and S4), and newly settled barnacles at the estuarine sites (S1 and S2) (Table S2).
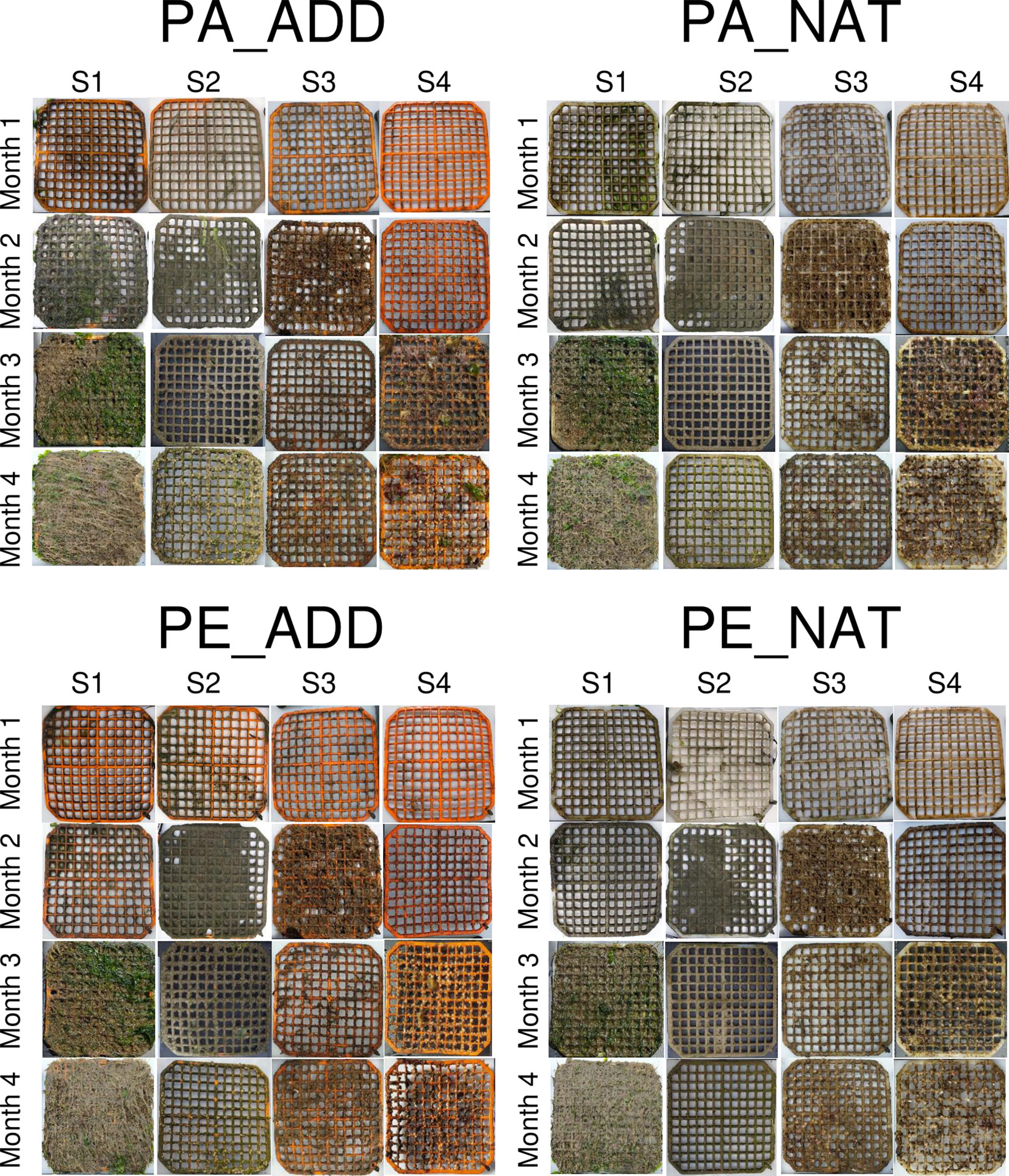
Figure 3 Synoptic image of the test materials at the different sampling sites (S1 to S4), over the immersion period. Top row: polyamide panel with color additive (PA_ADD) and untreated polyamide panel (PA_NAT). Bottom row: high-density polyethylene panel with color additive (PE_ADD) and untreated high-density polyethylene panel (PE_NAT). Images over time are from the same plate randomly selected from the three replicates of each condition.
In the sampling times of July, August, and September, an increase in colonization by macrofoulers (macroalgae and invertebrates) was observed, and after 4 months of immersion, panels located at different sampling sites presented their own specific community composition (Figure 3, Supplementary Table S2). As expected, marine sites exhibited a greater diversity of taxonomic groups, including native and non-indigenous species, which is consistent with findings from Marins et al. (2010) and McNaught and Norden (2011). On the contrary, estuarine sites had a lower diversity dominated by one or two taxa. The main fouling groups in the marine sites were macroalgae (green, brown, and red), encrusting and arborescent bryozoans, ascidians, barnacles Amphibalanus spp., and calcareous tube-dwelling polychaetes. In the estuarine sites, the dominant fouling organisms were filamentous green algae (Ulva spp.), acorn barnacles Austrominius modestus (Darwin, 1854), and colonial hydroids of the species Obelia dichotoma (Linnaeus, 1758). The dominant fouling groups identified in this study are recognized as typical common fouling organisms (Fitridge et al., 2012; Azevedo et al., 2020; Vinagre et al., 2020).
3.3 Biofouling biomass and mesh occlusion
The change in biofouling composition over time, from microfoulers to macrofoulers, is reflected in the total biomass (wet weight - WW), with relatively low values in the first month of immersion, followed by larger increases in the following months (Figure 4).
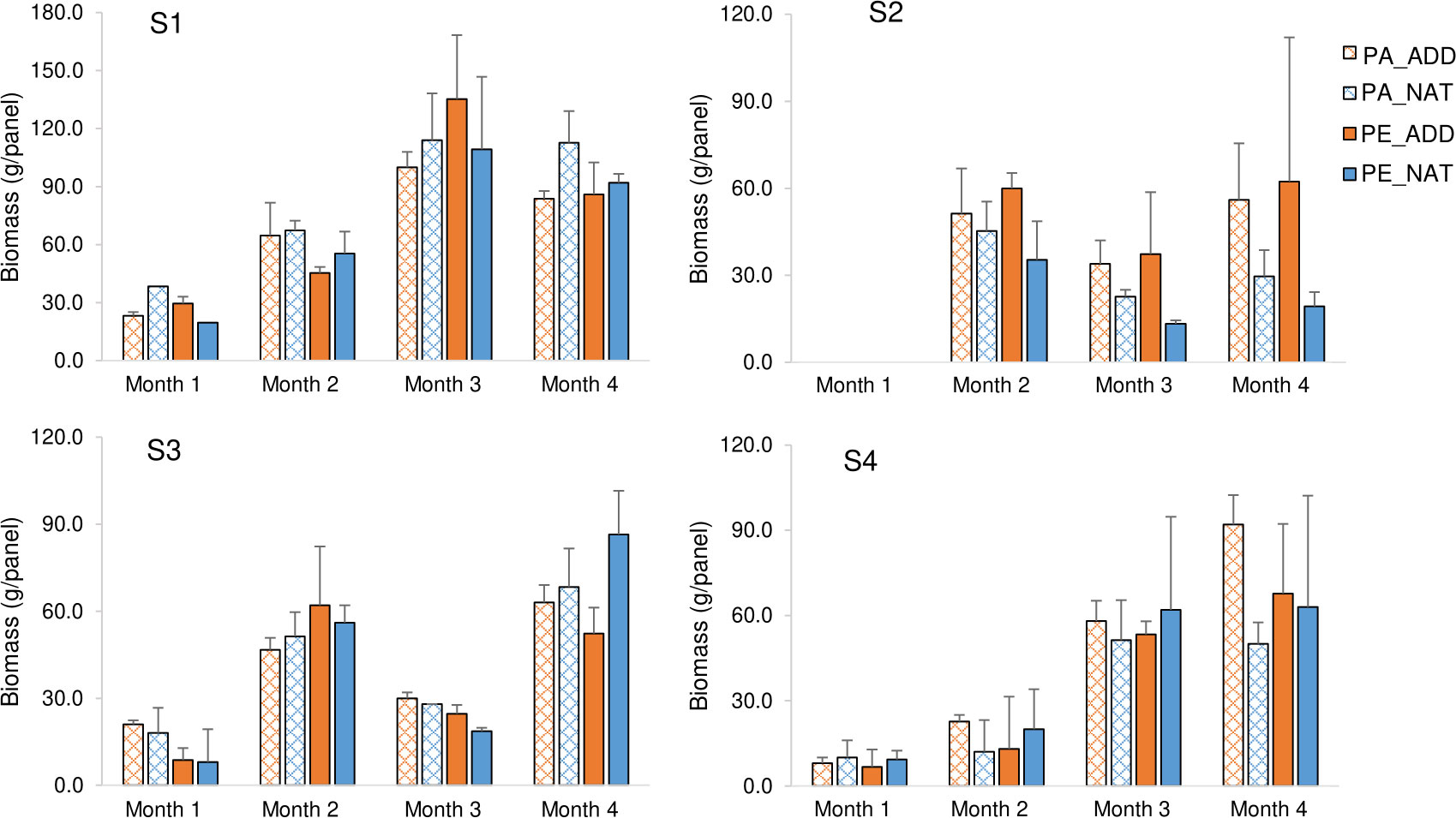
Figure 4 Fouling biomass (g wet weight per panel) on the tested plastic panels, over the 4 months of immersion at the different study sites (S1 to S4). Each column represents the mean of three replicate panels. Bars=standard deviation. PA_ADD: Polyamide with color additive; PA_NAT: Natural (untreated) polyamide; PE_ADD: High-density polyethylene with color additive; PE_NAT: Natural (untreated) high-density polyethylene. In site S2, biomass from June was not recorded due to equipment malfunction.
There were no consistent temporal or spatial patterns in the fouling community biomass. Panels deployed at the estuarine site, near the oyster farm, with longer immersion times (S1), had the highest fouling load over the study period (WW= 80 ± 36 g), while at the other sites, fouling biomasses were about half of that value (S2: 39 ± 22 g; S3: 39 ± 21 g; and S4: 38 ± 30 g) (Figure 4). This pattern is consistent with previous reports showing that continuously submerged structures at or near aquaculture farms typically exhibit greater biofouling biomass than sites distant from aquaculture activity, probably due to the enhanced food supply associated with mariculture (Cook et al., 2006; Fernandez-Gonzalez et al., 2021).
The temporal development of fouling communities differed according to site (Figure 4). Sites S1 and S4 showed greater biomass growth between July and August. In sites S3 and S2, a decrease in biomass was observed during the third month of immersion. As also observed by Marques et al. (2022) for ascidian communities, some fouling populations can proliferate rapidly and then retreat. This change in the fouling accumulation pattern might be indicative of a disturbance event or other differences in the environmental settings affecting marine fouling recruitment locally. Because there was no significant change in water quality parameters, this phenomenon might have been caused by biotic interactions within the fouling communities. In fact, the visual inspection of the fouling communities at that specific time (Table S2) showed an overall decrease in the abundance of dominant groups, namely, filamentous green algae in S2, and the arborescent bryozoan Bugula sp. in S3. In the case of bryozoans, Chang and Marshall (2016) also found that, in a 3-month study of marine fouling communities, this functional group had their maximum cover during mid-succession, declining the following month. This is probably linked to the relatively weak competitive abilities of arborescent bryozoans, relying on rapid colonization of free space before being overgrown by other species.
Results from the PERMANOVA analysis of biomass across the entire study period showed a significant interaction for treatment by site (p < 0.05; Table 3). Pairwise comparisons showed significant differences in biomass between natural (white) and treated (orange) panels only at the estuarine intertidal site, S2 (Table 3). At this site, the orange-colored panels had significantly larger biomass than the white (untreated) panels (50.17 ± 23.52 gWW vs. 27.61 ± 12.86 gWW), and the analysis of the fouling composition showed that the difference was due to the presence of the acorn barnacle Austrominius modestus, which settled more abundantly on colored panels (Figure 5).
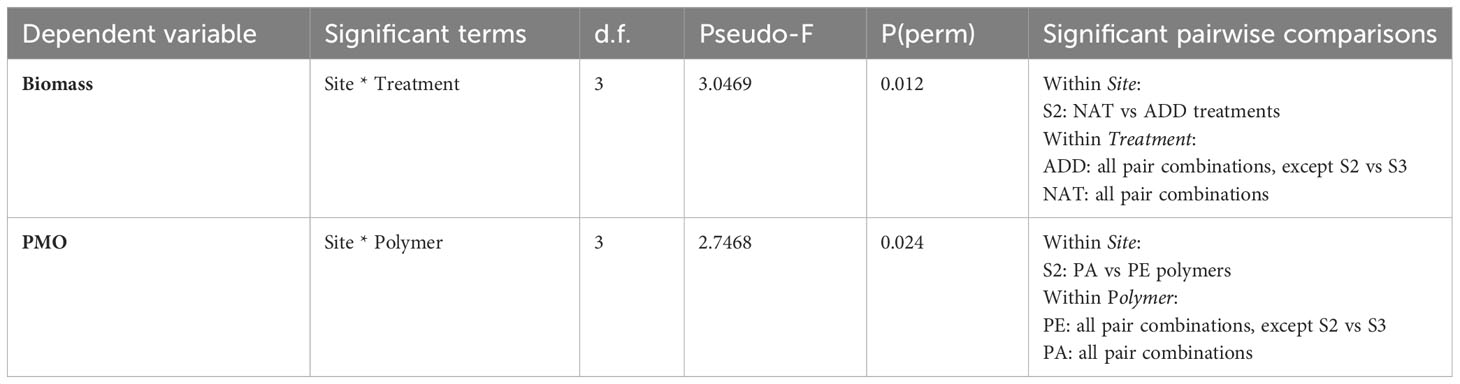
Table 3 Summary of significant terms for the PERMANOVA on the fouling biomass and on percentage mesh occlusion (PMO) as dependent variables, and site, treatment, and polymer as independent variables, with the indication of the significant terms and pairwise combinations (p<0.05).
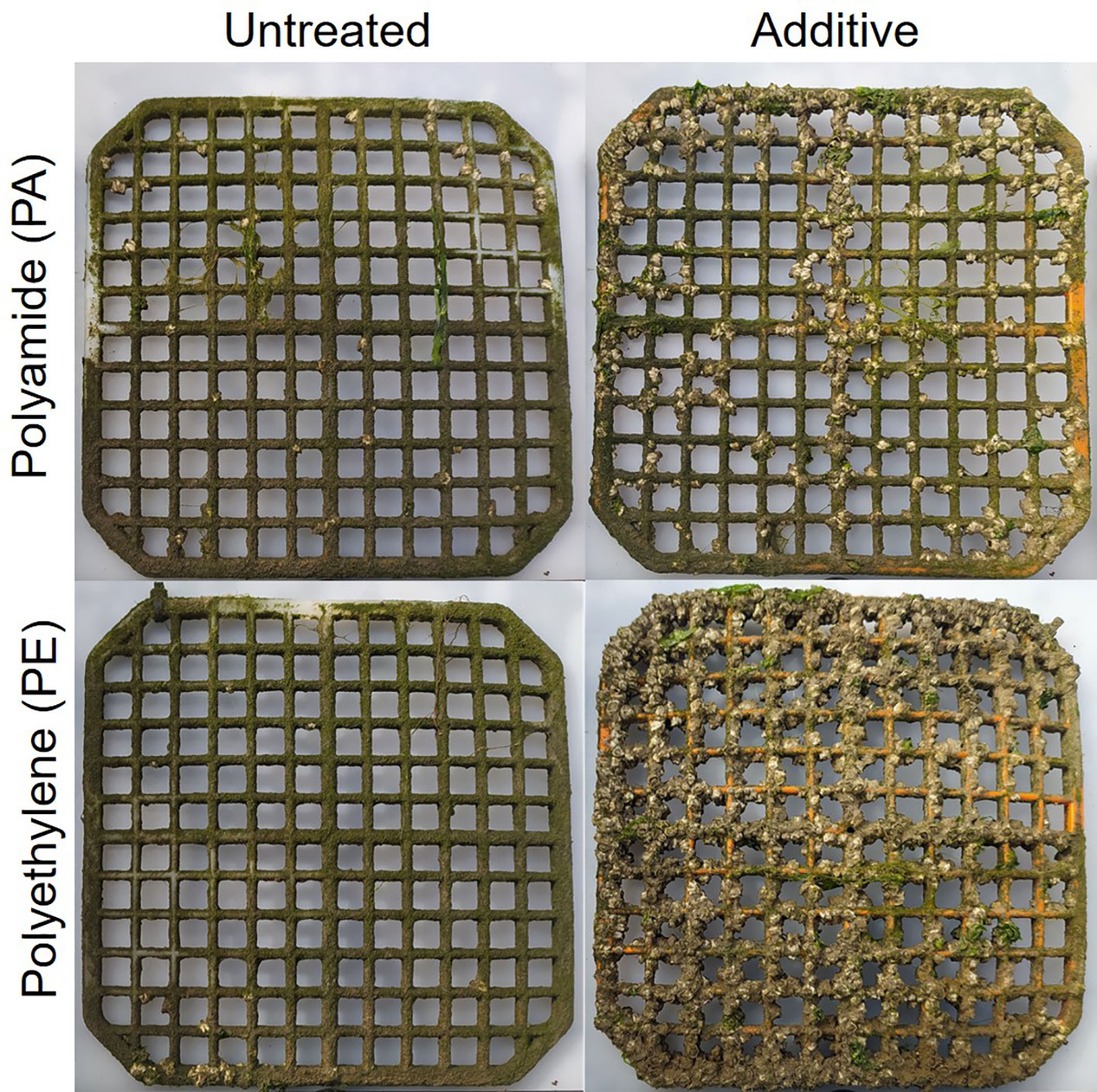
Figure 5 Barnacle cover on the tested plastic panels at the estuarine intertidal site (S2) after 4 months of immersion.
The percentage of mesh occlusion (PMO) closely followed the patterns observed in fouling biomass (Figure 6). The greatest amount of clogging occurred at the subtidal estuarine site (S1) with 100% clogging in treated panels (either of polyamide or high-density polyethylene) after 4 months of immersion. In other locations, the occlusion of the openings was less than 50% in most months. In the subtidal estuarine site (S1), mesh occlusion was caused by colonial hydroids (Obelia dichotoma) (Table S2), which tended to form dense mats colonizing the entire surface area of all plastic panels. The hydroid O. dichotoma is a well-known fouling species, forming locally abundant colonies and found almost everywhere in aquaculture facilities (Braithwaite and McEvoy, 2005; Fitridge et al., 2012; Bloecher et al., 2013; Martell et al., 2018), particularly in suspended culture. In addition to causing problems related to occlusion of cage mesh apertures, Obelia spp. has been found to colonize shell surfaces in mussels (Antoniadou et al., 2013) and pearl oysters (Guenther et al., 2006; Ye et al., 2019), leading to reduced growth rates and lower marketability of the bivalves.
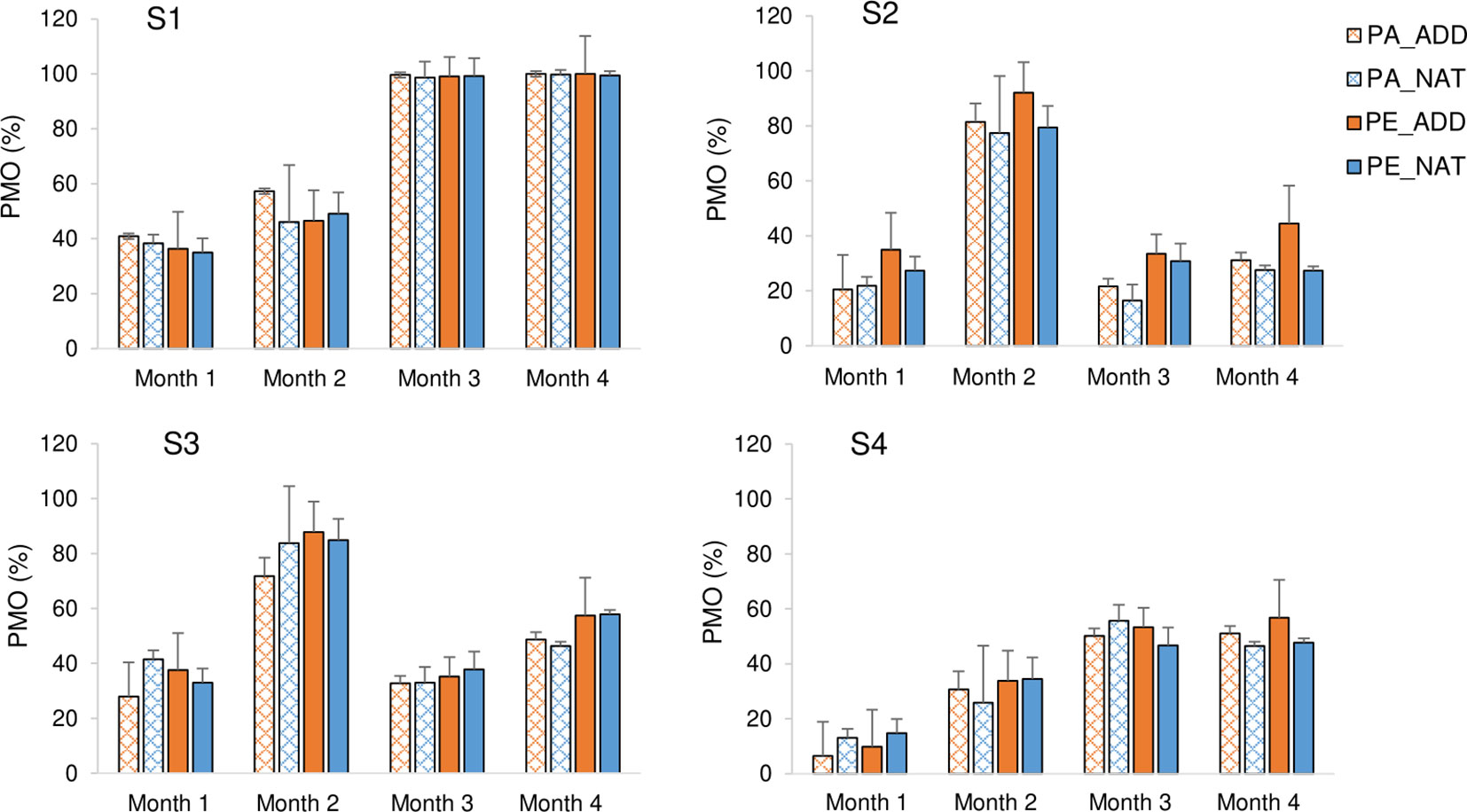
Figure 6 Percentage mesh occlusion (PMO, %) of the tested plastic panels, over the 4 months of immersion at the different study sites (S1 to S4). Each column represents the mean of three replicate panels. Bars=standard deviation. PA_ADD: Polyamide with color additive; PA_NAT: Natural (untreated) polyamide; PE_ADD: High-density polyethylene with color additive; PE_NAT: Natural (untreated) high-density polyethylene.
The heavy fouling observed at the subtidal estuarine site contrasts with the significantly less clogged panels at the nearby intertidal site. Aerial exposure is one of the ways to control biofouling in shellfish cultivation, and innovative designs in culture equipment that allow air-drying have been evolving to tackle this problem (Mizuta and Wikfors, 2019).
The two dimensions of the PCO ordination (Figure 7) effectively captured the general structure of the variability in fouling biomass (95%) and mesh occlusion (96%), with samples clustering according to the testing site. The PCO plots showed a smaller variation in the biomass and percentage of mesh occlusion of samples from site S1 (estuarine subtidal), while the other sites presented a larger variation and a general overlap of samples from sites S2 and S3, in accordance with the PERMANOVA results (Table 3).
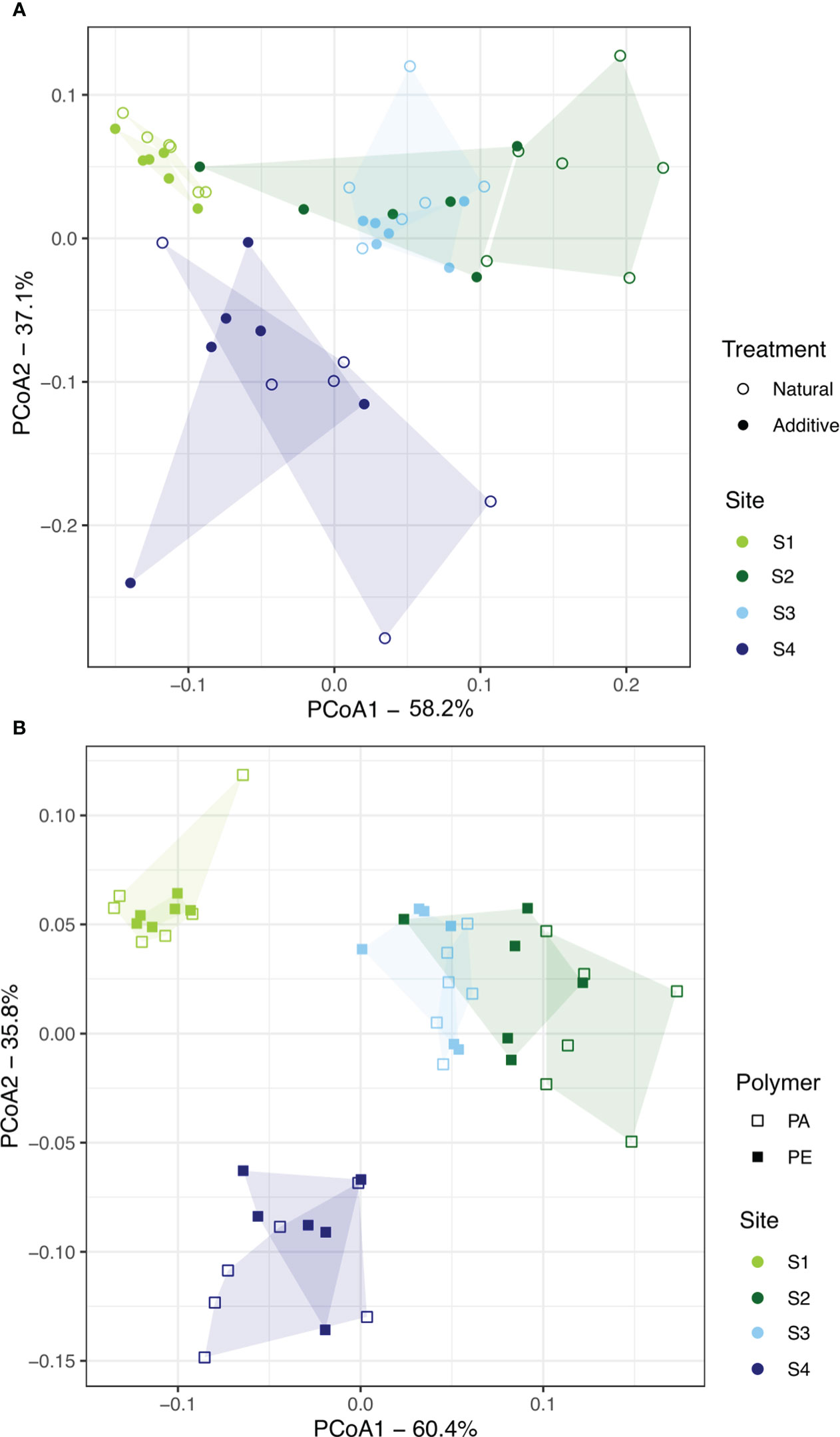
Figure 7 Principal coordinate analysis (PCoA) plots for the (A) biomass of biofouling community, evidencing sites and treatments, and the (B) percent mesh occlusion (PMO), evidencing sites and polymer types, in accordance with the PERMANOVA results, with an indication of the variance explained by each axis. Convex hulls were drawn to represent the area occupied by each group.
The PERMANOVA results indicated a significant interactive effect of “site” and “polymer” types on PMO, preventing the analysis of the main effects (Table 3). This interaction term was primarily driven by differences in panels deployed at the estuarine intertidal site S2, where panels made of high-density polyethylene (PE) were significantly more occluded than polyamide (PA) ones. As observed in the case of fouling biomass, these results were also attributed to an increased settlement of A. modestus in PE panels (Figure 5).
The acorn barnacle A. modestus is a non-native species typically found in intertidal and shallow subtidal estuarine areas (Ashton et al., 2017), and commonly associated with shellfish aquaculture (Rech et al., 2018). As an intertidal barnacle, A. modestus is able to tolerate fluctuations in salinity and temperature, and due to its fast growth rates and short generation times, it can form dense aggregations affecting shellfish, gear, and aquaculture equipment (Katsanevakis et al., 2018).
Dark-colored surfaces have been found to promote higher settlement of barnacle larvae (Yule and Walker, 1984; Robson et al., 2009) compared to lighter, more reflective colors such as white and yellow. The results presented here also confirm this pattern, reinforcing that substrate color is an important factor in the formation of macrofouling communities (Robson et al., 2009; Satheesh and Wesley, 2010; Dobretsov et al., 2013).
A number of studies have reported that different marine invertebrates show a preference for surfaces with a certain initial wettability (Schmidt et al., 1987; Rittschof and Costlow, 1989; Roberts et al., 1991). Some species of barnacles such as Amphibalanus amphitrite avoid surfaces with low wettability (Rittschof and Costlow, 1989; Dahlstrom et al., 2004), while bryozoans such as Bugula sp. settle in a higher percentage on surfaces with lower initial wettability (Rittschof and Costlow, 1989; Roberts et al., 1991). In contrast, the barnacle species A. modestus and Balanus crenatus, exhibit a preference for surfaces with lower initial wettability (Brown et al., 2001). Polyamide is a more hydrophilic (high-energy and high wettability) material compared to high-density polyethylene (Artham et al., 2009; Carl et al., 2012; Bloecher et al., 2013). While we have not analyzed the physicochemical characteristics of the surfaces of plastic materials and treatments, the differential settlement response of barnacle larvae is likely influenced by the surface properties of different polymers. However, it should be noted that the pristine surfaces will undergo some degree of modification upon immersion before macrofouler adhesion events occur (Callow and Fletcher, 1994). Plastic material exposed to UV-B radiation in the intertidal zone and subjected to tidal immersion-emersion cycles can also undergo surface modifications through mechanical abrasion and chemical processes (Cooper and Corcoran, 2010; Weinstein et al., 2016). This could explain why differences in polymer performance were only evident at the intertidal site, where complex interactions in abiotic and biotic factors could cause the greatest changes in plastic surfaces depending on the resistance to degradation of the specific polymers. Overall, these results underscore the need for field tests to assess gear-related plastics performance.
A final observation pertains to the utilization of plastics in aquaculture infrastructure. While plastics are valued for their robustness, cost-effectiveness, durability, affordability, and versatility, they can degrade into smaller particles, releasing microplastics and/or chemical compounds. Additionally, plastics can act as carriers for pathogenic agents (e.g., Botterell et al., 2019; Almeida et al., 2020), posing environmental risks to both cultivated species and human food security. To mitigate the risks associated with microplastic release and its potential hazards, it is essential to implement proper maintenance and disposal practices for plastics employed in aquaculture settings (e.g., Hingant et al., 2023).
4 Conclusions
Overall, the development of biofouling organisms in the different study locations was highly dynamic, varying temporally and spatially, even among sites that were in close proximity. These results highlight the need for conducting studies on seasonal changes in biofouling communities near aquaculture farms to anticipate and devise effective strategies to mitigate biofouling impacts. Although natural variability may have obscured the effects of surface properties in some of the sites, it is evident that polymer type and color play an important role, particularly in estuarine intertidal areas, where barnacle settlement, the main fouling organism, appears to be influenced by these two properties. This study suggests that the use of reflective colors and higher hydrophilic materials may help attenuate biofouling development. These findings offer valuable insights for selecting materials that can effectively reduce fouling loads and associated impacts, especially in bivalve aquaculture within intertidal estuarine regions. Future studies are planned, including the incorporation of natural effective antifouling compounds in plastic prototypes having those advantageous traits highlighted here.
Employing appropriate materials and proactive fouling control strategies will be essential in ensuring efficient and sustainable aquaculture practices in the future. By understanding and managing biofouling dynamics, the aquaculture industry can maintain its productivity and minimize the challenges posed by fouling organisms.
Data availability statement
The original contributions presented in the study are included in the article/Supplementary Material. Further inquiries can be directed to the corresponding author.
Author contributions
Conceptualization, VF, SR, JA, and MD; Data curation, VF, and OG; Formal analysis, VF, OG, and MD; Funding acquisition, VF, JA, SR, MD, RO, and IM; Investigation, VF, OG, JA, MD, SR, and JM; Project administration, VF; Supervision, VF; Writing – original draft, VF and JA; Writing – review & editing, VF, JA, SR, MD, RO, IM, and JM. All authors contributed to the article and approved the submitted version.
Funding
This work was developed within the scope of the Research and Innovation (R&I) contract of the project ERGOMARINE (PT-INNOVATION-0006), promoted by Ernesto São Simão Lda, and funded by EEA Grants. CIIMAR research unit acknowledges FCT Fundação para a Ciência e a Tecnologia – for the strategic fund support (UIDB/04423/2020 and UIDP/04423/2020). This research was also supported by the Norte Portugal Regional Operational Programme (NORTE 2020), under the PORTUGAL 2020 Partnership Agreement and through the ERDF, as a result of the project ATLANTIDA (NORTE-01-0145-FEDER-000040); and by the Innovation Pact, Project No. C644915664-00000026 (WP2 Vertical Bivalves), under the “Blue Bioeconomy Pact”, resulting from the submission of the application to Notice No. 02/C05-i01/2022, within the scope of theRecovery and Resilience Plan (PRR), co-funded by the Portuguese Republic and the European Union. JRA (2022.03876.CEECIND) and IM (2021.03721.CEECIND) acknowledge their work contracts by FCT- Scientific Employment Stimulus Individual Call, MD (CEECINST/00027/2021/CP2789/CT0001) by the FCT-institutional call, and SR (DL57/2016/CP1344/CT0020) and VF (DL57/2016/CP1344/CT0027) through the FCT-DL57.
Acknowledgments
The authors gratefully acknowledge the support of Aquagoma – Produtos do Rio e Mar, Lda, especially Armando Gomes, for allowing access to their oyster farm and equipment in the Lima estuary. We also thank Francisco Arenas for making available the biofouling monitoring structures and helping with installating the panels. Thanks are also due to Marta Martins and Dimitri Costa for helping with taxa identification.
Conflict of interest
The authors declare that the research was conducted in the absence of any commercial or financial relationships that could be construed as a potential conflict of interest.
Publisher’s note
All claims expressed in this article are solely those of the authors and do not necessarily represent those of their affiliated organizations, or those of the publisher, the editors and the reviewers. Any product that may be evaluated in this article, or claim that may be made by its manufacturer, is not guaranteed or endorsed by the publisher.
Supplementary material
The Supplementary Material for this article can be found online at: https://www.frontiersin.org/articles/10.3389/fmars.2023.1229634/full#supplementary-material
References
Adams C. M., Shumway S. E., Whitlatch R. B., Getchis T. (2011). Biofouling in marine molluscan shellfish aquaculture: a survey assessing the business and economic implications of mitigation. J. World Aquacult. Soc 42 (2), 242–252. doi: 10.1111/j.1749-7345.2011.00460.x
Almeida C. M. R., Manjate E., Ramos S. (2020). Adsorption of Cd and Cu to different types of microplastics in estuarine salt marsh medium. Mar. pollut. Bull. 151, 110797. doi: 10.1016/j.marpolbul.2019.110797
Amara I., Miled W., Slama R. B., Ladhari N. (2018). Antifouling processes and toxicity effects of antifouling paints on marine environment. A review. Environ. Toxicol. Pharmacol. 57, 115–130. doi: 10.1016/j.etap.2017.12.001
Anderson M. J., Gorley R. N., Clarke K. R. (2008). PERMANOVA+ for PRIMER: Guide to software and statistical methods (UK: Plymouth). doi: 10.13564/j.cnki.issn.1672-9382.2013.01.010
Antoniadou C., Voultsiadou E., Rayann A., Chintiroglou C. (2013). Sessile biota fouling farmed mussels: diversity, spatio-temporal patterns, and implications for the basibiont. J. Mar. Biolog. Assoc. U.K. 93 (6), 1593–1607. doi: 10.1017/S0025315412001932
Artham T., Sudhakar M., Venkatesan R., Nair C. M., Murty K. V. G. K., Doble M. (2009). Biofouling and stability of synthetic polymers in sea water. Int. Biodeterior. Biodegrad. 63 (7), 884–890. doi: 10.1016/j.ibiod.2009.03.003
Ashton G. V., Brandt A., Isaac M. J., Makings P., Moyse J., Naylor E., et al. (2017). “Crustaceans,” in Handbook of the Marine Fauna of North-West Europe, 2nd ed. Eds. Hayward P. J., Ryland J. S. (Oxford, UK: Oxford University Press), 283–440.
Azevedo J., Antunes J. T., MaChado A. M., Vasconcelos V., Leão P. N., Froufe E. (2020). Monitoring of biofouling communities in a Portuguese port using a combined morphological and metabarcoding approach. Sci. Rep. 10 (1), 13461. doi: 10.1038/s41598-020-70307-4
Bannister J., Sievers M., Bush F., Bloecher N. (2019). Biofouling in marine aquaculture: a review of recent research and developments. Biofouling 35 (6), 631–648. doi: 10.1080/08927014.2019.1640214
Bloecher N., de Nys R., Poole A. J., Guenther J. (2013). The fouling hydroid Ectopleura larynx: a lack of effect of next generation antifouling technologies. Biofouling 29 (3), 237–246. doi: 10.1080/08927014.2013.763228
Bloecher N., Floerl O. (2020). Efficacy testing of novel antifouling coatings for pen nets in aquaculture: How good are alternatives to traditional copper coatings? Aquaculture 519, 734936.
Botterell Z. L., Beaumont N., Dorrington T., Steinke M., Thompson R. C., Lindeque P. K. (2019). Bioavailability and effects of microplastics on marine zooplankton: A review. Environ. pollut. 245, 98–110. doi: 10.1016/j.envpol.2018.10.065
Braithwaite R. A., Carrascosa M. C. C., McEvoy L. A. (2007). Biofouling of salmon cage netting and the efficacy of a typical copper-based antifoulant. Aquaculture 262 (2-4), 219–226. doi: 10.1016/j.aquaculture.2006.11.027
Braithwaite R. A., McEvoy L. A. (2005). Marine biofouling on fish farms and its remediation. Adv. Mar. Biol. 47, 215–252. doi: 10.1016/S0065-2881(04)47003-5
Brown C. J., Eaton R. A., Thorp C. H. (2001). Effects of chromated copper arsenate (CCA) wood preservative on early fouling community formation. Mar. pollut. Bull. 42 (11), 1103–1113.
Callow M. E., Fletcher R. L. (1994). The influence of low surface energy materials on bioadhesion–a review. Int. biodeterioration biodegradation 34 (3-4), 333–348.
Carl C., Poole A. J., Sexton B. A., Glenn F. L., Vucko M. J., Williams M. R., et al. (2012). Enhancing the settlement and attachment strength of pediveligers of Mytilus galloprovincialis by changing surface wettability and microtopography. Biofouling 28, 175–186. doi: 10.1080/08927014.2012.662676
Chambers L. D., Stokes K. R., Walsh F. C., Wood R. J. (2006). Modern approaches to marine antifouling coatings. Surf. Coat. Technol. 201 (6), 3642–3652. doi: 10.1016/j.surfcoat.2006.08.129
Chang C. Y., Marshall D. J. (2016). Spatial pattern of distribution of marine invertebrates within a subtidal community: do communities vary more among patches or plots? Ecol. Evol. 6 (22), 8330–8337.
Characklis W. G., Cooksey K. E. (1983). Biofilms and microbial fouling. Adv. Appl. Microbiol. 29, 93–138. doi: 10.1016/S0065-2164(08)70355-1
Cook E. J., Black K. D., Sayer M. D. J., Cromey C. J., Angel D. L., Spanier E., et al. (2006). The influence of caged mariculture on the early development of sublittoral fouling communities: a pan-European study. ICES J. Mar. Sci. 63 (4), 637–649. doi: 10.1016/j.icesjms.2005.12.007
Cooper D. A., Corcoran P. L. (2010). Effects of mechanical and chemical processes on the degradation of plastic beach debris on the island of Kauai, Hawaii. Mar. pollut. Bull. 60 (5), 650–654. doi: 10.1016/j.marpolbul.2009.12.026
CRAB (2007) European Best Practice in Aquaculture Biofouling. Available at: www.crabproject.com.
Dahlem C., Moran P. J., Grant T. R. (1984). Larval settlement of marine sessile invertebrates on surfaces of different colour and position. Ocean Sci. Eng. 9, 225–236.
Dahlström M., Jonsson H., Jonsson P. R., Elwing H. (2004). Surface wettability as a determinant in the settlement of the barnacle balanus improvisus (Darwin). J. Exp. Mar. Biol. Ecol. 305 (2), 223–232.
de Nys R., Ison O. (2004). Evaluation of antifouling products developed for the Australian pearl industry. Fisheries Res. Dev. Corporation., 1–114.
Dobretsov S., Abed R. M., Voolstra C. R. (2013). The effect of surface colour on the formation of marine micro and macrofouling communities. Biofouling 29 (6), 617–627. doi: 10.1080/08927014.2013.784279
Dürr S., Watson D. I. (2010). Biofouling and antifouling in aquaculture. Biofouling 12, 267–287. doi: 10.1002/9781444315462.ch19
Edwards C. D., Pawluk K. A., Cross S. F. (2015). The effectiveness of several commercial antifouling treatments at reducing biofouling on finfish aquaculture cages in british columbia. Aquaculture Res. 46, 2225–2235. doi: 10.1111/are.12380
Fernandez-Gonzalez V., Navarro-Mayoral S., Sanchez-Jerez P. (2021). Connectivity patterns for direct developing invertebrates in fragmented marine habitats: fish farms fouling as source population in the establishment and maintenance of local metapopulations. Front. Mar. Sci. 8, 785260. doi: 10.3389/fmars.2021.785260
Fitridge I., Dempster T., Guenther J., De Nys R. (2012). The impact and control of biofouling in marine aquaculture: a review. Biofouling 28 (7), 649–669. doi: 10.1080/08927014.2012.700478
Fiúza A. F. G., Macedo M. E., Guerreiro M. R. (1982). Climatological space and time-variation of the Portuguese coastal upwelling. Oceanol. Acta 5 (1), 31–40.
Guenther J., Southgate P. C., De Nys R. (2006). The effect of age and shell size on accumulation of fouling organisms on the Akoya pearl oyster Pinctada fucata (Gould). Aquaculture 253 (1-4), 366–373. doi: 10.1016/j.aquaculture.2005.08.003
Hingant M., Mallarino S., Conforto E., Dubillot E., Barbier P., Bringer A., et al. (2023). Artificial weathering of plastics used in oyster farming. Sci. Total Environ. 868, 161638. doi: 10.1016/j.scitotenv.2023.161638
Hodson S. L., Burke C. M., Bissett A. P. (2000). Biofouling of fish-cage netting: the efficacy of a silicone coating and the effect of netting colour. Aquaculture 184 (3-4), 277–290. doi: 10.1016/S0044-8486%2899%2900328-2
Kang S., Hoek E. M., Choi H., Shin H. (2006). Effect of membrane surface properties during the fast evaluation of cell attachment. Sep. Sci. Technol. 41, 1475–1487. doi: 10.1080/01496390600634673
Katsanevakis S., Rilov G., Edelist D. (2018). Impacts of marine invasive alien species on European fisheries and aquaculture–plague or boon? CIESM Monograph 50, 125–132.
Lane A., Willemsen P. (2004). Collaborative effort looks into biofouling. Fish Farming Int. 44, 34–35.
Marins F. O., Novaes R. L. M., Rocha R. M., Junqueira A. O. R. (2010). Non indigenous ascidians in port and natural environments in a tropical Brazilian bay. Zoologia 27, 213–221. doi: 10.1590/S1984-46702010000200009
Marques L., Teixeira G., Calado R., Lillebø A. I. (2022). Using oyster shells for customized 3-D structures for monitoring ecosystem shifts on ascidians diversity. Front. Mar. Sci. 9, 1–16. doi: 10.3389/fmars.2022.921094
Martell L., Bracale R., Carrion S. A., Giangrande A., Purcell J. E., Lezzi M., et al. (2018). Successional dynamics of marine fouling hydroids (Cnidaria: Hydrozoa) at a finfish aquaculture facility in the Mediterranean Sea. PloS One 13 (4), e0195352. doi: 10.1371/journal.pone.0195352
McNaught D. C., Norden W. S. (2011). Generalized regional spatial patterns of larval recruitment of invasive ascidians, mussels, and other organisms along the coast of maine. Aquat. Invasions 6, 519–523. doi: 10.3391/ai.2011.6.4.18
Mizuta D. D., Wikfors G. H. (2019). Seeking the perfect oyster shell: a brief review of current knowledge. Rev. Aquaculture 11 (3), 586–602.
Moroney D. A., Walker R. L. (1999). The effects of tidal and bottom placement on the growth, survival and fouling of the eastern oyster Crassostrea virginica. J. World Aquac. Soc 30 (4), 433–442. doi: 10.1111/j.1749-7345.1999.tb00991.x
Nikolaou M., Neofitou N., Skordas K., Castritsi-Catharios I., Tziantziou L. (2014). Fish farming and anti-fouling paints: a potential source of Cu and Zn in farmed fish. Aquac. Environ. Interact. 5 (2), 163–171. doi: 10.3354/aei00101
Oksanen J., Blanchet F. G., Friendly M., Kindt R., Legendre P., McGlinn D., et al. (2019). “Package ‘vegan’,” in Community ecology package, version, vol. 2. .
Papadopoulos D. K., Lattos A., Giantsis I. A., Theodorou J. A., Michaelidis B., Feidantsis K. (2023). The impact of ascidian biofouling on the farmed mediterranean mussel mytilus galloprovincialis physiology and welfare, revealed by stress biomarkers. Biofouling 39 (3), 271–288.
Pereira D., Gonçalves C., Martins B. T., Palmeira A., Vasconcelos V., Pinto M., et al. (2021). Flavonoid glycosides with a triazole moiety for marine antifouling applications: synthesis and biological activity evaluation. Mar. Drugs 19 (1), 19010005. doi: 10.3390/md19010005
R Core Team (2019). R: A language and environment for statistical computing (Vienna, Austria: R Foundation for Statistical Computing).
Railkin A. I. (2004). Marine Biofouling: Colonization Processes and Defenses (Boca Raton, Florida: CRC Press), 303. pp.
Ramos S., Cowen R. K., Paris C., Ré P., Bordalo A. A. (2006). Environmental forcing and larval fish assemblage dynamics in the Lima River estuary (NW Portugal). J. Plankton Res. 28 (3), 275–286. doi: 10.1093/plankt/fbi104
Ramos S., Amorim E., Elliott M., Cabral H., Bordalo A. A. (2012). Early life stages of fishes as indicators of estuarine ecosystem health. Ecol. Indic. 19, 172–183.
Rech S., Salmina S., Pichs Y. J. B., García-Vazquez E. (2018). Dispersal of alien invasive species on anthropogenic litter from European mariculture areas. Mar. pollut. Bull. 131, 10–16. doi: 10.1016/j.marpolbul.2018.03.038
Reyes R., del Norte‐Campos A., Añasco N. C., Santander‐de Leon S. M. S. (2020). Biofouling development in marine fish farm influenced by net colour, immersion period and environmental conditions. Aquac. Res. 51 (8), 3129–3138. doi: 10.1111/are.14648
Rittschof D., Costlow J. D. (1989). Bryozoan and barnacle settlement in relation to initial surface wettability: a comparison of laboratory and field studies. Sci. Mar. 53, 411–416.
Roberts D., Rittschof D., Holm E., Schmidt A. R. (1991). Factors influencing initial larval settlement: temporal, spatial and surface molecular components. J. Exp. Mar. Biol. Ecol. 150 (2), 203–221. doi: 10.1016/0022-0981(91)90068-8
Robson M. A., Williams D., Wolff K., Thomason J. C. (2009). The effect of surface colour on the adhesion strength of Elminius modestus Darwin on a commercial non-biocidal antifouling coating at two locations in the UK. Biofouling 25 (3), 215–227. doi: 10.1080/08927010802712879
Satheesh S., Wesley S. G. (2010). Influence of substratum colour on the recruitment of macrofouling communities. J. Mar. Biolog. Assoc. U.K. 90 (5), 941–946. doi: 10.1017/S0025315410000032
Schneider C. A., Rasband W. S., Eliceiri K. W. (2012). NIH Image to ImageJ: 25 years of image analysis. Nat. Methods 9 (7), 671–675. doi: 10.1038/nmeth.2089
Schmidt A. R., Rittschof D., Hooper I. R., Gerhart D. J., Hill L., Bonaventura J., et al. (1987). “Wettability affects settlement of barnacles and bryozoans in the field,” in AMERICAN ZOOLOGIST, vol. 27. (NEW HAMPSHIRE ST, LAWRENCE, KS 66044: AMER SOC ZOOLOGISTS), 1041.
Su Z., Huang L., Yan Y., Li H. (2007). The effect of different substrates on pearl oyster Pinctada martensii (Dunker) larvae settlement. Aquaculture 271 (1-4), 377–383. doi: 10.1016/j.aquaculture.2007.02.039
Swain G., Herpe S., Ralston E., Tribou M. (2006). Short-term testing of antifouling surfaces: the importance of colour. Biofouling 22 (6), 425–429. doi: 10.1080/08927010601037163
Vilas-Boas C., Carvalhal F., Pereira B., Carvalho S., Sousa E., Pinto M., et al. (2020). One step forward towards the development of eco-friendly coatings: Immobilization of a sulfated marine marine-inspired compound. Mar. Drugs 18 (10), 489. doi: 10.3390/md18100489
Vinagre P. A., Simas T., Cruz E., Pinori E., Svenson J. (2020). Marine biofouling: A European database for the marine renewable energy sector. J. Mar. Sci. Eng. 8 (7), 495. doi: 10.3390/jmse8070495
Watson D. I., Shumway S. E., Whitlatch R. B. (2009). “Biofouling and the shellfish industry,” in Shellfish Safety and quality (Cambridge, UK: Woodhead Publishing), 317–337.
Weinstein J. E., Crocker B. K., Gray A. D. (2016). From macroplastic to microplastic: Degradation of high‐density polyethylene, polypropylene, and polystyrene in a salt marsh habitat. Environ. Toxicol. Chem. 35 (7), 1632–1640. doi: 10.1002/etc.3432
WoRMS Editorial Board (2021) World Register of Marine Species (VLIZ). Available at: https://www.marinespecies.org (Accessed 2021-09-01).
Ye L., Wu L., Wang Z., Jiang J., Wang J. (2019). Control of biofouling on pearl oysters Pinctada imbricata using wax and Chinese herbs. Biofouling 35 (6), 649–657. doi: 10.1080/08927014.2019.1641201
Yule A. B., Walker G. (1984). The temporary adhesion of barnacle cyprids: effects of some differing surface characteristics. J. Mar. Biolog. Assoc. U.K. 64 (2), 429–439. doi: 10.1017/S0025315400030101
Keywords: biofouling, color, oyster aquaculture, ports, estuary, plastics
Citation: Freitas V, Gonçalves O, Dolbeth M, Ramos S, Morais J, Ozorio ROdA, Martins I and Almeida JR (2023) Optimization of plastic polymers for shellfish aquaculture infrastructures: in situ antifouling performance assessment. Front. Mar. Sci. 10:1229634. doi: 10.3389/fmars.2023.1229634
Received: 26 May 2023; Accepted: 10 October 2023;
Published: 30 October 2023.
Edited by:
Hideyuki Kanematsu, Suzuka College, JapanReviewed by:
Adriana Giangrande, University of Salento, ItalyIoannis A. Giantsis, University of Western Macedonia, Greece
James Scott Maki, Marquette University, United States
Copyright © 2023 Freitas, Gonçalves, Dolbeth, Ramos, Morais, Ozorio, Martins and Almeida. This is an open-access article distributed under the terms of the Creative Commons Attribution License (CC BY). The use, distribution or reproduction in other forums is permitted, provided the original author(s) and the copyright owner(s) are credited and that the original publication in this journal is cited, in accordance with accepted academic practice. No use, distribution or reproduction is permitted which does not comply with these terms.
*Correspondence: Joana R. Almeida, amFsbWVpZGFAY2lpbWFyLnVwLnB0