- 1Departamento de Microbiología Molecular, Instituto de Biotecnología, Universidad Nacional Autónoma de México (UNAM), Av. Universidad, Col. Chamilpa, Cuernavaca, MO, Mexico
- 2Instituto de Investigaciones Oceanológicas, Universidad Autónoma de Baja California, Ensenada, BC, Mexico
- 3Departamento de Innovación Biomédica, Centro de Investigación Científica y de Educación Superior de Ensenada (CICESE), Ensenada, BC, Mexico
- 4Unidad Universitaria de Secuenciación Masiva y Bioinformática, Instituto de Biotecnología, Universidad Nacional Autónoma de México, Cuernavaca, Mexico
- 5Instituto de Ciencias del Mar y Limnología, Universidad Nacional Autónoma de México, Ciudad Universitaria, Mexico City, Mexico
We describe an assembled marine bacterial consortium designed for bioremediation of oil-contaminated seawater, based on a statistical method using a Plackett-Burman (PB) experimental approach. The final consortium consists of four bacteria isolated from the Gulf of Mexico, from four genera: Pseudomonas, Halopseudomonas, Paenarthrobacter, and Alcanivorax. Individually, bacterial oil removal by these microorganisms was evaluated by gravimetry, reaching 39% at maximum after 75 days, whereas in consortium it was ~62%. We also measured biodegradation levels by Gas Chromatography/Mass Spectrometry (GC-MS) observing 12 polyaromatic hydrocarbons (PAHs) degradation analyzed and n-alkanes degradation with a preference for specific chain length. Consortium population analysis using the V3-V4 region of 16S rRNA showed a stable community, suggesting that the metabolic load was distributed among bacteria and that stable dynamic interactions were achieved. In this work, we show that the use of a factorial method for synthetic consortium design offers the possibility of improving oil degradation efficiency with stable bacterial populations.
1 Introduction
Oil contamination in the ocean represents a risk to the environment, given its complex chemical nature and its ability to have different toxicity levels (Patowary et al., 2016). Currently, physicochemical methods are available for cleanup, but they can only remedy a small fraction of the total oil spilled. During the Deepwater Horizon (DWH) oil spill in 2010, it was estimated that despite having employed a wide range of remediation technologies, only 7-10% of petroleum was recovered and an estimated 10% was chemically dispersed (Committee on the Effects of the Deepwater Horizon Mississippi Canyon-252 Oil Spill on Ecosystem Services et al., 2013). Meanwhile, bioremediation technologies remain as one of the most promising options for oil spill cleanup, where microorganisms that use oil as a source of carbon are used to degrade it. Bioremediation based on aerobic bacterial metabolism is the most rapid way to remediate oil polluted sites (Brzeszcz and Kaszycki, 2018).
It has been demonstrated that oil present in the ocean stimulates the growth of native bacterial communities capable of degrading hydrocarbons (HCs) (Cappello et al., 2007; Hazen et al., 2010), suggesting that their use is convenient for bioremediation in marine environments (Dash et al., 2013). Two major bioremediation approaches are typically referenced, namely biostimulation (addition of nutrients to stimulate the growth of oil-degrading bacteria) and bioaugmentation (addition of microorganisms with oil-degrading capacity). The bioaugmentation strategy uses bacterial consortia or communities, leading to a more efficient process that breaks down a greater range of pollutants (Ebadi et al., 2017). Among the marine bacteria isolated from areas containing petroleum oil, well-known HCs degraders include strains from the genera Alcanivorax, Pseudomonas, Marinobacter, Rhodococcus, Halomonas, and Arthrobacter, among others (Brzeszcz and Kaszycki, 2018).
Bacterial consortium design can be performed using a metabolic analysis approach if there is available knowledge regarding the metabolic capabilities of the individual species, so that interactions among them may be taken into account (Grosskopf and Soyer, 2014; Kong et al., 2018; Karkaria et al., 2021). In the absence of such knowledge, and given the practical impossibility of evaluating all possible combinations of bacterial species in a consortium (Kong et al., 2018), a combinatorial approach based on statistical methods can be used (Lv et al., 2014; Mazzucotelli et al., 2014). One of these methods is the Plackett-Burman (PB) design, which enables the identification of dominant factors in a system through the analysis of their orthogonal arrangements (Box et al., 2005; Lv et al., 2014). Applications of the PB method include the optimization of bacterial culture growth parameters (Jiang et al., 2013), design of microbial culture media (Prajapati et al., 2014; Ghanem and Al-Zahrani, 2016), and setting of parameters that influence metal removal by bacteria (Migahed et al., 2017). In the design of bacterial consortia, PB has been used to improve a synthetic bacterial consortium to treat dairy industry wastewater (Mazzucotelli et al., 2014). In this case, 32,000 possible bacterial strain combinations were narrowed down to carry out just 16 experiments, resulting in a designed consortium of 4 bacterial isolates with increased pollutant degradation levels.
Previously, our research determined the bacterial population baseline in the southwestern Gulf of Mexico (GoM). The taxonomic and metabolic information from different genera found in the GoM, showed a bioremediation potential to develop a strategy that can be used during a possible oil spill event. From marine sediment samples collected in an area of 200,000 km2, and using high throughput sequencing, we have identified at least 450 genera belonging to the bacterial baseline (Godoy-Lozano et al., 2018; Raggi et al., 2020). In addition to this effort, we have isolated some bacterial strains from the GoM (Muriel-Millán et al., 2019; Muriel-Millán et al., 2021; Rodríguez-Salazar et al., 2021; Rosas-Díaz et al., 2021; Rojas-Vargas et al., 2022) and from the Northwest region of the Mexican Pacific Ocean (MPO) (Silva-Jiménez et al., 2018). These strains were isolated from water column and sediment samples collected at locations where crude oil was reported to be present; as such, we expect to find HC degraders among them, which could potentially be used to design a promising bacterial consortium for HC bioremediation in seawater.
In the present study, we describe a strategy based on the PB statistical and predictive approach, involving an experimental design using nineteen native marine bacterial strains from our aforementioned collection, and leading to the final selection of the most significant bacterial strains for light crude oil degradation in seawater. The resulting consortium, consisting of four bacterial strains, showed improved removal and biodegradation levels, suggesting synergic interactions among the isolates. An in silico prediction of such bacterial interactions is also reported.
2 Material and methods
2.1 Reagents
Unless otherwise specified, all reagents used in this study were analytical grade, and commercially available. The dichloromethane (DCM) used for HC extractions and as the mobile phase for column chromatography were HPLC grade, purchased from VWR Chemicals BDH (Radnor, PA, United States).
2.2 Sampling and bacterial strain isolation
Nineteen marine oil-degrading bacteria isolates were selected for this study. Out of these nineteen strains, sixteen strains were obtained from sediment and seawater samples collected from different locations of the GoM (Table 1). Sediment samples were added to mineral medium (MM) composed of (g·L-1): Na2SO4 0.183; CaCl2 0.073; NH4Cl 0,267; MgCl2·6H2O 0.16; NaMoO4·2H2O 0.0002; FeSO4 0.005; NaCl 11.68; K2HPO4 0.8; KH2PO4 0.2; and supplemented with HCs such as kerosene, phenanthrene or oil petroleum as the sole carbon and energy source. Bacterial isolates were plated in LB agar (Bertani, 2004) or EDM agar (Rosas-Díaz et al., 2021). Three additional strains were isolated from sediment samples collected in the coastal area of Rosarito Port, Baja California, in the MPO (Silva-Jiménez et al., 2018) (Table 1). These strains were isolated using Bushnell Haas (BH) broth supplemented with 25 mg·L-1 pyrene or phenanthrene. Glycerol stocks of these bacteria were stored at -80°C.
2.3 Bacterial genotyping by 16S rRNA analysis
A glycerol stock inoculum of each strain was streaked on an LB agar plate and incubated overnight at 30°C. Isolated colonies of each strain were cultured overnight in 25 mL of LB medium in a 125 mL flask, at 30°C and 180 RPM. Cells were harvested by centrifugation for 15 min, at 6,000 RPM and 4°C. Bacterial DNA was extracted using the Quick-DNA™ Miniprep Kit from Zymo Research (Irvine, CA, United States). A polymerase chain reaction (PCR) was carried out with Taq DNA polymerase (NZYTech, Lisboa, Portugal) using degenerate primers SD-Bact-0008-dS-20 (F27, 5′-AGAGTTTGATCMTGGCTCAG-3′) and SD-Bact-1492-aA-22 (R1492, 5′-TACGGYTACCTTGTTACGACTT-3′) to amplify the bacterial 16S rRNA gene (Lane, 1991; Monciardini et al., 2002). The PCR products were purified with DNA Clean & Concentrator-25 from Zymo Research and subsequently sequenced using the Sanger method at the DNA Synthesis and Sequencing Unit of the Institute of Biotechnology (IBt), UNAM, Mexico. Sequences were cleaned using Chromas v.2.6.6 (http://technelysium.com.au/wp/chromas/), assembled by CAP in BioEdit v7.1 (Hall and Others, 1999), and annotated based on a Blastn search of the NCBI database 16S ribosomal RNA sequences for Bacteria and Archaea (McGinnis and Madden, 2004).
2.4 Plackett-Burman experimental design
The nineteen isolated strains were used as assessed factors (A, B, C, …T) to feed into the PB fractional factorial experimental design; PB codes were randomly assigned and are indicated in Table 1. These factors were sorted in a PB orthogonal matrix (Table 2), which is composed of values of 1 (strain present, at an initial concentration of 1x108 CFU·mL-1) and -1 (strain absent), for a total of 20 runs (19 consortia of 10 isolates each, plus the abiotic control). The PB orthogonal matrix was obtained by arranging a pre-designed PB generating vector as the first column and off-setting by one vector element for each new column (Antony, 2014). The type of isolates in the given runs were assigned in accordance with the matrix.
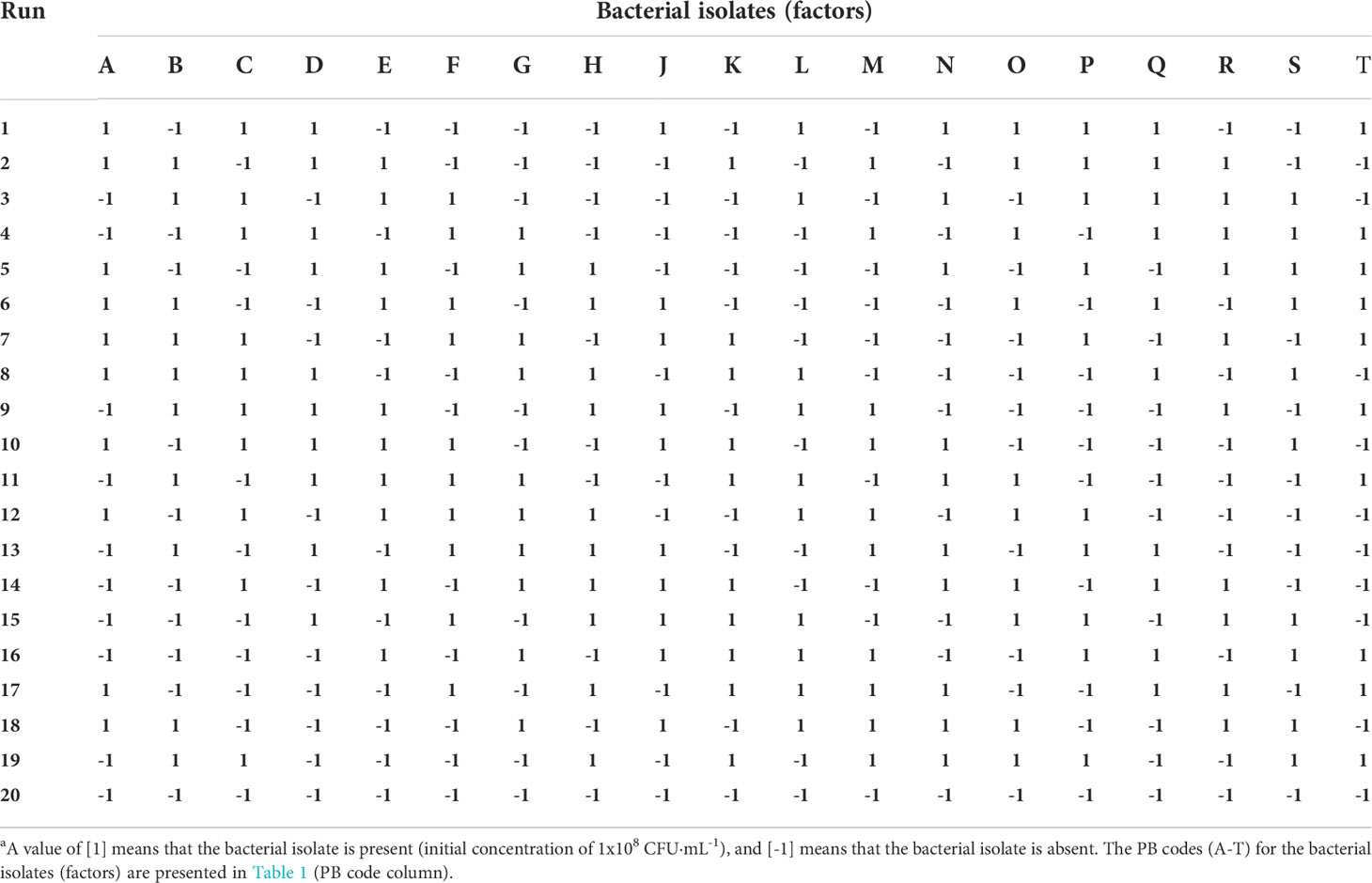
Table 2 Orthogonal Plackett-Burman matrixa.
Bacteria were separately grown by inoculating 100 mL of MM supplemented with peptone and yeast extract (PY) 0.3% w/v, and incubating at 30°C, 180 RPM, 20 h. Cells were recovered and washed three times with sterile MgSO4 10 mM, and were used to assemble each consortium with an initial concentration of 1x108 CFU·mL-1 of each strain. Marine medium was prepared by filtered-sterilizing seawater with a 0.22 μm membrane (Merck Millipore, Darmstadt, DEU), and after autoclaving, 0.5 g·L-1 of sterile API 35 crude oil from GoM was added as the sole carbon source, along with nitrogen (NH4Cl) and phosphorus (KH2PO4 and K2HPO4) in a 100:10:1 C:N:P ratio. Glass flasks containing 20 mL of marine medium were inoculated with each consortium and incubated for 75 days at 30°C, 180 RPM. An additional flask containing only marine medium was also incubated as abiotic control. Every run was performed in triplicate.
2.5 HC extraction and total crude oil removal measurements
Liquid-liquid extraction of total HCs was performed by adding to each 20-mL culture (in marine medium containing API 35 crude oil as the sole carbon source, as described above) 15 mL of HPLC-grade dichloromethane (DCM) (CH2Cl2, Sigma-Aldrich Inc., MO, USA). The total volume was collected in glassware previously washed with DCM, and combined with three more washes of each culture flask, 5 mL of DCM each. After allowing to stand 12–14 h at room temperature for phase separation, the organic phases were separated by manual pipetting and concentrated to ~1 mL using the Rotavapor R-300 (Buchi, Flawil, Suiza), then run through a self-packed glass column containing anhydrous sodium sulfate (built in a 9-inch Pasteur pipette with a fiberglass plug) to remove any possible water residue. The volume was harvested in a 1.5-mL Wheaton glass vial and allowed to stand in a fume hood until all DCM was evaporated. Total crude oil removal was determined by the gravimetric method (Richter, 1997).
2.6 Plackett-Burman statistical analysis
Total crude oil removal results from the 20-run PB experimental design were analyzed using the Pareto chart and the analysis of variances (ANOVA) using the Design-Expert software v7.0.0 (Stat-Ease Inc., MN, USA). The model significance was evaluated with the F-test and the standardized effects of each factor (Vatsal et al., 2016). The oil-removal contributions of the 19 isolates were calculated, identifying those that had the greatest contribution to the standard deviation and with a p-value less than 0.05, for the formulation of the final bacterial consortium.
2.7 Biodegradation kinetics of selected isolates
Each of the most significant isolates, determined by PB analysis, was grown individually, and in consortium, under the same culture conditions described in section 2.4 with an initial concentration of 1x108 CFU·mL-1 for each strain. For each sample, five culture sets were grown in triplicate to be used for total crude oil removal determination at days 7, 15, 30, 50, and 75. To monitor bacterial growth, one additional set of cultures was grown both with and without (negative control) crude oil; their protein content was measured with the Lowry method (Lowry et al., 1951) by taking 300-μL aliquots from each culture at the same five time points, plus a day 0 sample.
2.8 Gas chromatography-mass spectrometry
The 12 Environmental Protection Agency (EPA) priority polyaromatic hydrocarbons (PAHs) along with all C8-C38 n-alkanes were analyzed using gas chromatography-mass spectrometry (GC-MS). HC extracts were reconstituted with 1 mL HPLC grade DCM, then diluted with the same grade DCM in a 1:23 ratio, followed by addition of internal standards. These solutions were injected into an Agilent 6890N Gas Chromatograph (Santa Clara, CA, USA) with a 30-m HP-5ms column, carried with Helium gas. Once each sample was separated based on column selectivity and temperature ranges, it was driven into the coupled mass spectrometer. Mass spectra were defined using the built-in Mass Hunter software. The GC-MS results were used to determine crude oil biodegradation levels. n-Alkanes were divided into 5 different groups based on chain lengths. In the present study, they were classified as follows: C8-C12 (short-chain alkanes), C13-C17 (medium-short-chain alkanes), C18-C22 (medium-chain alkanes), C23-C27 (medium-long chain alkanes), C28-C32 (long-chain alkanes), C33-C38 (very long-chain alkanes).
2.9 Sequencing and bioinformatic analysis
Additional sets of cultures of the final consortium, just as those used for bacterial growth kinetics with crude oil, were grown and cells were harvested at 0, 7, 15, 30, 50 and 75 days by centrifuging for 15 min at 6,000 RPM, 4°C for a time-series experiment. Total DNA extraction was performed with the ZymoBIOMICS DNA Miniprep Kit from Zymo Research (Irvine, CA, United States). The extracted DNA was used to amplify the V3-V4 regions from the 16S rRNA gene. These 16S rRNA V3-V4 amplicons were obtained using Taq DNA polymerase (NZYTech, Lisboa, Portugal) and primers SD-Bact-0341-bS-17 (Bakt_341F, 5’-CCTACGGGNGGCWGCAG-3’) and SD-Bact-0785-aA-21 (Bakt_805R, 5′-GACTACHVGGGTATCTAATCC-3’) (Herlemann et al., 2011). PCR products were purified with the DNA Clean & Concentrator-25 from Zymo Research, quantified in NanoDrop One (Thermo Fisher Scientific Inc. Waltham, MA USA) and Qubit (Invitrogen, Waltham, MA USA), and subsequently used to prepare the library for sequencing using the Illumina MiSeq platform using a 600 cycles sequencing kit with a paired-end configuration to generate a 300 bp read length.
To obtain the relative abundances of the four bacterial strains in the final consortium, raw reads were concatenated by Flash v1.2.11 (Magoč and Salzberg, 2011), and the extended fragments were pooled in 99% similarity to CD-HIT (Li and Godzik, 2006). Subsequently, the taxonomic annotation was performed using Megablast (Chen et al., 2015) with an e-value of 1x10-10 against the NCBI 16S ribosomal database (02-2021). The taxonomic profile was corrected by dividing by the 16S copy number for each organism (Stoddard et al., 2015).
2.10 Bacterial community interactions
We used the time-series Lotka-Volterra-based network inference approach, included in the MetaMIS software v1.02 (Shaw et al., 2016), to infer the underlying interactions of the consortium during crude oil biodegradation. In a nutshell, the software allows the time series data analysis of microbial community profiles. It systematically examines interaction patterns (such as mutualism or competition) and refines the biotic role within microbes, using default parameters.
3 Results
3.1 Taxonomic identification of bacteria used for consortium assembly
To design our bacterial consortium, we used nineteen cultivable bacterial strains isolated from different GoM and MPO samples, twelve from marine sediments and seven from seawater (Table 1). A web map of the sites with latitude/longitude coordinates and depths of the nineteen bacteria described in Table 1 is available in https://www.easymapmaker.com/map/9a9669b0ef586e4e446c1e34bcec22e7. These bacterial isolates could grow in seawater supplemented with light crude oil. Taxonomic identification of two of these strains had been previously performed by genomic analysis. One of them was identified as Paenarthrobacter sp. GOM3 and was found to be a monoaromatic degrader (Rosas-Díaz et al., 2021). The second one, isolated from asphalt marine sediments, was Halopseudomonas aestusnigri GOM5 (Rojas-Vargas et al., 2022). In this study, taxonomic identification of all nineteen strains was done through 16S rRNA sequencing (Table 1), showing seven Pseudomonas isolates. Two of the probable species identified among these were P. aeruginosa and P. stutzeri, both of which have been reported as capable of degrading a wide variety of HCs (Prince et al., 2010). Among other genera identified, we also found Alcanivorax, recognized as a hydrocarbonoclastic genus present in oil spill events (Head et al., 2006), and Rhodococcus, described as a PAHs degrader (Guevara et al., 2019). All genera have been associated with HCs degradation.
3.2 Selection of oil-degrading consortium strains through PB experimental design
Based on the PB design using the 19 bacterial isolates as factors (randomly coded A-T) (Table 1), 20 experiment runs were performed, consisting of 19 consortia of 10 isolates each, plus the abiotic control (Table 2). The crude oil removal percentages for each run are shown in Figure 1A. The response values displayed high variability, with the highest removal obtained for run 5 (60.02 ± 16.65%) and the lowest for run 14 (11.38 ± 4.11%). This last value was similar to the one obtained for the abiotic control (run 20), which was 13.11 ± 3.37%. Additional runs that did not promote HC removal were 9, 10, 11, and 13.
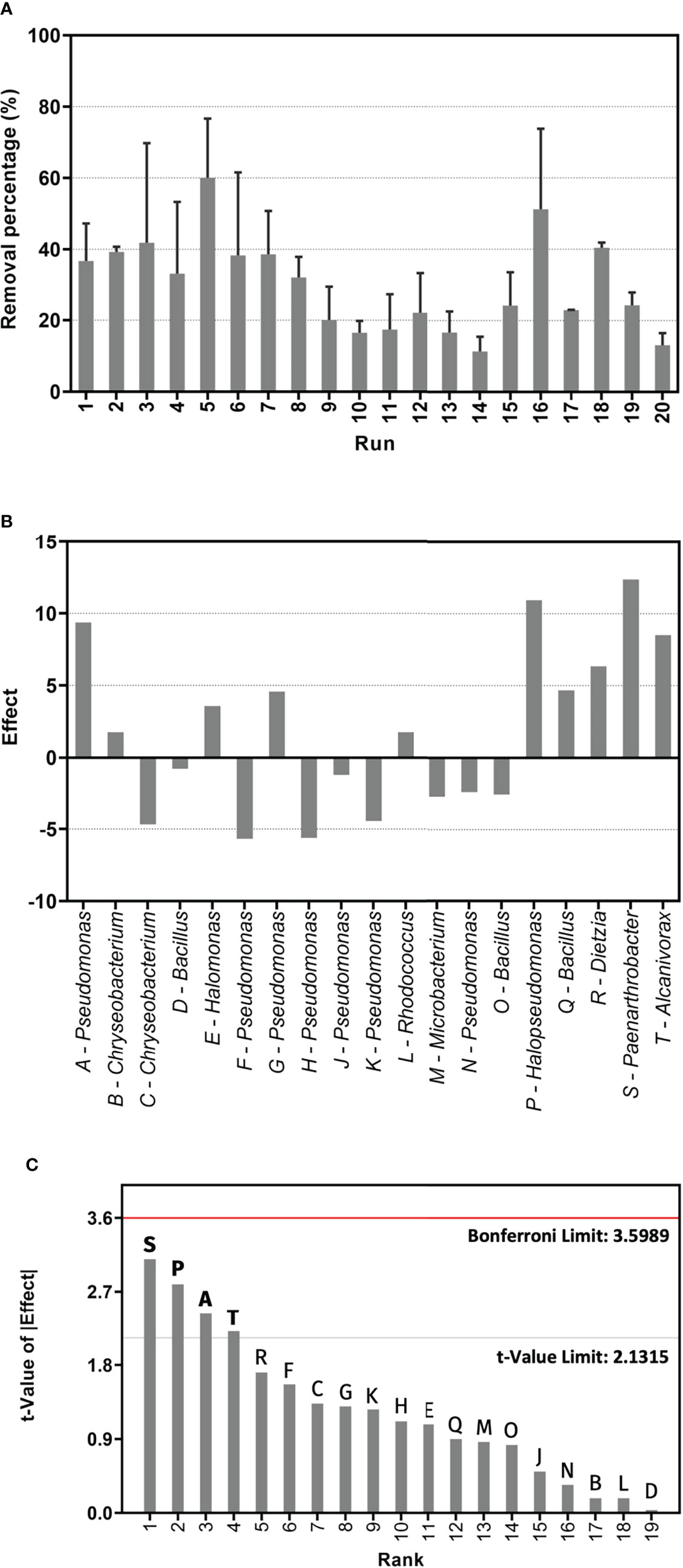
Figure 1 Results of PB experiment for light crude oil removal. (A) Percentage of crude oil removal for each bacterial culture run described in Table 2, measured by gravimetry after a 75-day incubation period. Error bars represent +/-1 standard deviation from the mean, n = 3. Run 20 corresponds to the abiotic control. (B) Standardized effects of PB design factors. (C) Pareto chart for PB design factors. Significant strains are in bold font. Rank (x-axis) corresponds to the factors, arranged in descending order according to the t-value of the absolute value of the effect.
Since the ratio between the maximum and the minimum response was 6.1 (Figure 1A), the ANOVA analysis did not require any transformation and a linear model was used:
Where Y is the measured response (crude oil removal percentage), β0 the intercept, βi the linear coefficients, and Xi the independent factor.
Subsequently, the standardized effect of each factor was determined. The magnitude of the effect indicates the significance of a factor on the response (Hassan et al., 2016). Figure 1B shows these effects, differentiating the positive from the negative ones. By taking the magnitudes of the effects onto a Pareto chart (Figure 1C), we identified which factors were statistically significant in terms of crude oil removal. Any effect found between the two reference limit lines, which were the Bonferroni and t-value, was significant. In this first analysis, we determined that isolates S (Paenarthrobacter sp. GOM3), P (H. aestusnigri GOM5), A (Pseudomonas sp.), and T (Alcanivorax sp.) were significant, with positive effects on the response.
After selecting the significant factors, the best model for the measured response was as follows:
Where [A], [P], [S] and [T] are the 4 significant factors (isolates). The F-value obtained from the ANOVA of this model was 7.00, implying that there was only a 0.18% probability that the estimated model was due to noise. The values obtained for the coefficient of determination were R2 = 0.7143 with adj-R2 = 0.6123. Table 3 summarizes the ANOVA of the model, indicating the p-value by individual factor. A p-value < 0.05 suggested that the factor was significant, while one greater than 0.05 was not significant. Analyzing the best model, the four selected strains had p-value < 0.05 and were selected as part of the final consortium.
3.3 HC removal and biodegradation kinetics of selected strains
To study the HC biodegradation capacity and preferences of the four selected strains, they were cultured individually and in consortium in marine medium using API 35 crude oil as the sole carbon and energy source. Their growth over the course of 75 days was monitored by protein quantification (negative controls without crude oil were used as baseline for calculations), HC removal was measured by gravimetry, and HC biodegradation was determined by GC-MS (the abiotic control was used as baseline for calculation purposes).
3.3.1 Bacterial growth and HC removal
All four isolates were able to grow individually using API 35 crude oil as their sole carbon and energy source, as measured by an increase in protein concentration (Figure 2). The protein concentration curves displayed an asymptotic behavior, with overall values from highest to lowest being as follows: Alcanivorax sp., H. aestusnigri GOM5, Pseudomonas sp., and Paenarthrobacter sp. GOM3. A similar growth pattern was observed for the four strains in the consortium. A linear relationship between protein concentration and CFU/mL was previously validated for the four isolates, with R2 values of 0.96 for Alcanivorax sp., 0.91 for H. aestusnigri GOM5 (0.91), 0.85 for Pseudomonas sp., and 0.98 for Paenarthrobacter sp. GOM3.
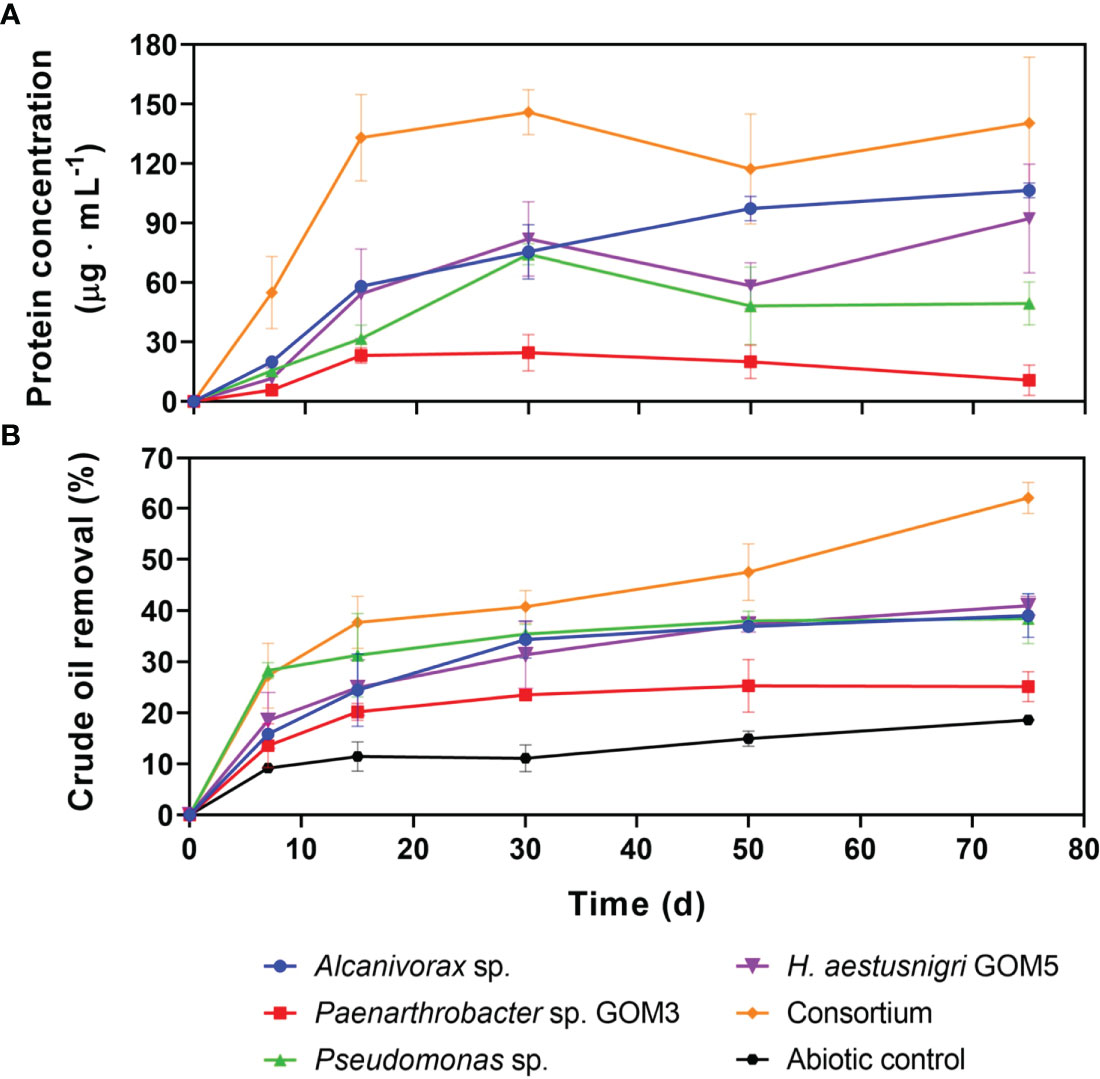
Figure 2 Bacterial growth and HC removal of selected bacterial strains cultured individually and in consortium with light crude oil as the sole carbon and energy source. (A) Bacterial growth was monitored by measuring protein concentration using the Lowry method. The initial concentration of each strain was 1x108 CFU·mL-1. Negative controls without crude oil were used as baseline for protein content calculations. (B) Light crude oil removal by the cultures shown in A, determined by gravimetry. Error bars represent +/-1 standard deviation from the mean, n = 3.
Figure 2 also shows the HC removal percentages for the bacterial isolates individually and in consortium. Pseudomonas sp., Alcanivorax sp. and H. aestusnigri GOM5 reached ~39% HC removal each by day 75. Paenarthrobacter sp. GOM3 had the lowest HC removal values, reaching ~25% by the end of the incubation period. The consortium reached ~62% by day 75. The abiotic control values were not subtracted in the calculation of these HC removal percentages to compare them with those obtained in the PB experiment.
3.3.2 Biodegradation of n-alkanes
The C8-C38 n-alkane biodegradation curves are shown in Figure 3A. When grown individually, the highest biodegradation percentages were reached at 30 days of incubation for all strains, with mean values of 57% for Pseudomonas sp., 53% for Alcanivorax sp., 40% for H. aestusnigri GOM5, and 29% for Paenarthrobacter sp. GOM3. At day 75, the final values were ~48% for Pseudomonas sp., ~40% for Alcanivorax sp. and H. aestusnigri GOM5, and ~13% for Paenarthrobacter sp. GOM3. The consortium reached its maximum of ~60% at day 30 and decreased down to ~45% at day 75. The apparent drop in biodegradation levels observed at longer incubation times was due to the spontaneous degradation of shorter chain n-alkanes (Supplementary Figure S1) (Nicodem et al., 1997; Li et al., 2007).
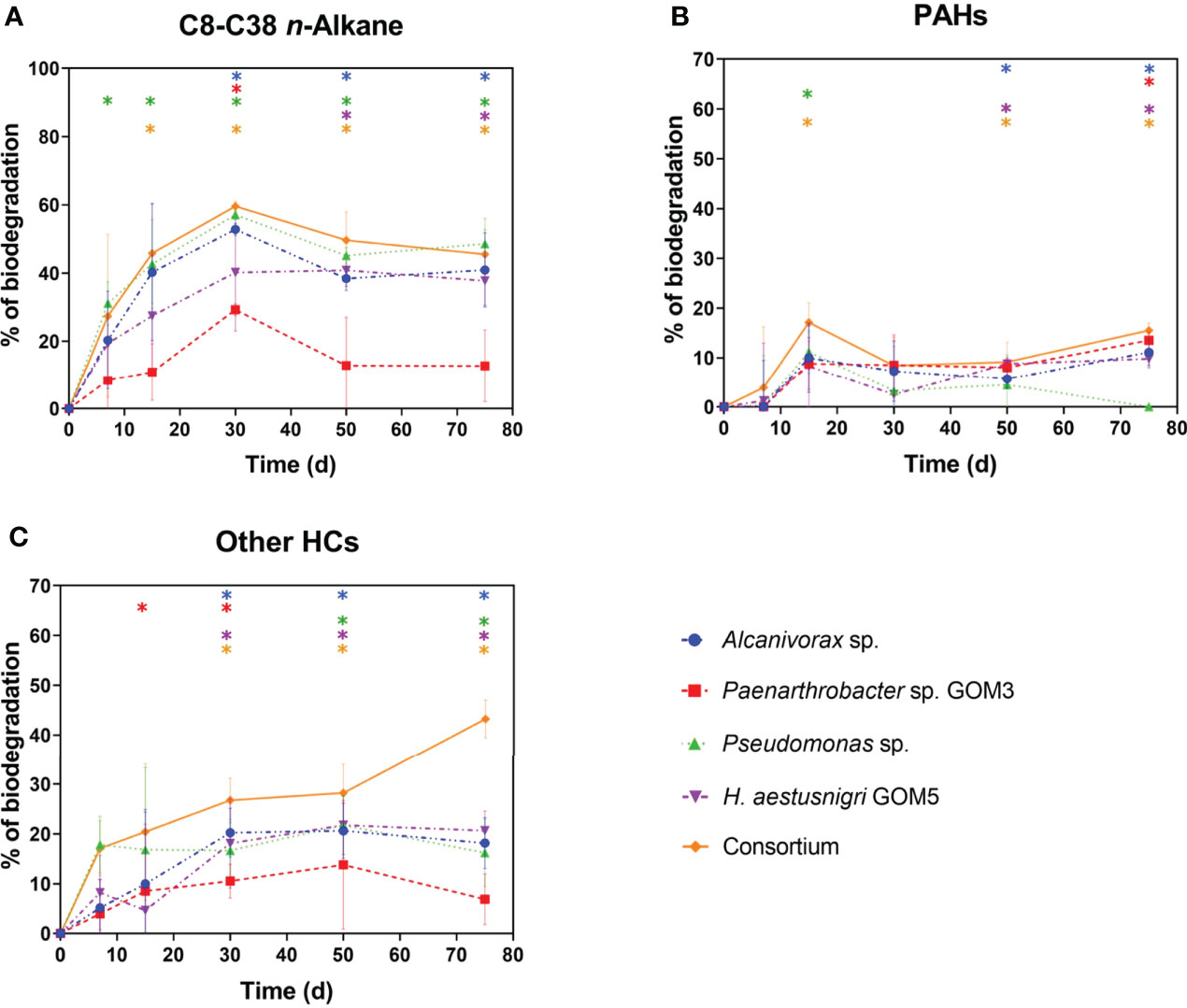
Figure 3 HC biodegradation kinetics by selected bacterial strains cultured individually and in consortium with light crude oil as the sole carbon and energy source. The initial concentration of each strain was 1x108 CFU·mL-1. (A) C8-C38 n-alkanes. (B) PAHs. (C) Other HCs. Data points in (A, B) correspond to the sum of those obtained for the HCs analyzed individually, shown in Figures S2 and S3, respectively. Statistically significant differences (p < 0.05) between each experimental point and the corresponding abiotic control are indicated with color-coordinated asterisks. Error bars are +/-1 standard deviation from the mean, n = 3.
The biodegradation levels of the six n-alkane subgroups described in Materials and Methods are shown in Supplementary Figure S2. The C8-C12 fraction exhibited the lowest biodegradation levels, reaching a maximum of ~13% at day 15 for all strains grown individually and in consortium (Supplementary Figure S2A). However, the volatile nature of this fraction makes it less bioavailable over time (Mapelli et al., 2017) as shown in Supplementary Figure S1F, where the remaining content of this fraction in the abiotic control was ~15% by day 15. The C13-17 fraction also exhibited volatility and natural degradation (Supplementary Figure S1F), reaching by day 30 ~70% of biodegradation for Alcanivorax sp. and Pseudomonas sp., and ~55% and ~40% for H. aestusnigri GOM5 and Paenarthrobacter sp. GOM3, respectively, then dropping drastically by day 50 to ~38% for Pseudomonas sp., ~33% for Alcanivorax sp. and H. aestusnigri GOM5, and ~0% for Paenarthrobacter sp. GOM3 (Supplementary Figure S2B). The remaining content of this fraction in the abiotic control was ~81% by day 30, and ~41% by day 50 (Supplementary Figure S1F).
The highest biodegradation levels were obtained with the C18-C22 fraction, reaching a maximum of ~90% for Pseudomonas sp., ~84% for Alcanivorax sp., ~61% for H. aestusnigri GOM5 and ~32% for Paenarthrobacter sp. GOM3 by day 50 (Supplementary Figure S2C). For the C23-C27 fraction the highest biodegradation levels were reached at day 50, with ~64% for Pseudomonas sp., ~49% for Alcanivorax sp., ~52% for H. aestusnigri GOM5 and ~31% for Paenarthrobacter sp. GOM3 (Figure S2D). The C28-C32 fraction exhibited maximum biodegradation levels at day 50 by Pseudomonas sp., H. aestusnigri GOM5, and Paenarthrobacter sp. GOM3, with values of ~44%, 22%, and ~18%, respectively. In the case of Alcanivorax sp., the maximum of ~35% was reached at day 75 (Figure S2E). The C33-C38 fraction reached maxima of ~59% for Pseudomonas sp., ~45% for Alcanivorax sp., ~44% for H. aestusnigri GOM5 and ~26% for Paenarthrobacter sp. GOM3 by day 50 (Supplementary Figure S2F).
The consortium exhibited its lowest n-alkane biodegradation levels for the C8-C12 fraction, reaching a maximum of ~12% by day 15 (Supplementary Figure S2A). The C13-C17 fraction reached a maximum of ~77% by day 30, then dropped drastically by day 50 to ~35% (Supplementary Figure S2B). Just as the individual strains, the consortium also displayed an optimal biodegradation of the C18-C22 fraction, reaching ~92% by day 50 (Supplementary Figure S2C). Maximal biodegradation by the consortium of the C23-C27, C28-C32, and C33-C38 fractions was observed at day 50, reaching values of ~62% (Figure S2D), ~53% (Figure S2E) and ~62%, (Supplementary Figure S2F), respectively.
These results indicate that both individually and in consortium, the four selected strains could use n-alkanes as their carbon source, showing a preference for specific chain lengths.
3.3.3 Biodegradation of PAHs and other HCs
Individually, the strains exhibited similar PAH biodegradation kinetics (reaching a maximum of ~10-14% on day 75), except for Pseudomonas sp., which peaked to ~11% on day 15, and decreased subsequently down to a negligible value at the end point (Figure 3B). The consortium exhibited a kinetic curve similar to that of Alcanivorax sp., Paenarthrobacter sp. GOM3 and H. aestusnigri GOM5, reaching a maximum of ~16% on day 75. Among the 12 PAHs analyzed, the most susceptible to biodegradation individually and in consortium were both 3-ring PAHs (fluorene, 1-methyl-9H-fluorene), dibenzothiophene (DBT), 2,3,6-trimethylnaphthalene, both methylated phenanthrenes (1-methyl- and 2-methyl-), and pyrene (Figure S3). The other PAHs analyzed were hardly biodegraded.
A mass balance was carried out to determine the biodegradation of all other HCs that were not analyzed by GC-MS (branched and cyclic alkanes, alkenes, monoaromatics, resins, etc.). The biodegradation profile of these HCs, referred to as “other’’, revealed a maximum of around ~20% for H. aestusnigri GOM5, Alcanivorax sp. and Pseudomonas sp., and ~11% for the Paenarthrobacter sp. GOM3, at day 50 (Figure 3C). The consortium reached a maximum of ~46% at day 75 (Figure 3C).
3.4 Population dynamics and inferred microbial interactions
Amplicon analyses were used to study the population dynamics of the selected strains during HC biodegradation in consortium. Amplicon reads obtained with MiSeq Illumina were of good quality for bioinformatic analysis, ~70% of sequences were with ≥Q20 (Table 4). The relative abundances at the genus level are shown in Figure 4A. While the initial inoculum was the same for all four strains in terms of CFUs (1x108 CFU·mL-1 each), their relative abundances at time 0 differed (30% Alcanivorax sp., 32% H. aestusnigri GOM5, 20% Pseudomonas sp., 18% Paenarthrobacter sp. GOM3). This may be since the number of CFUs is not always directly proportional to the actual number of individual cells (Tortora et al., 2007). By day 7, Alcanivorax sp. had largely displaced the Pseudomonas sp. and Paenarthrobacter sp. GOM3 populations, and from then on, the relative abundances of all four strains were basically stable. The relative abundance of H. aestusnigri GOM5 essentially remained unchanged.
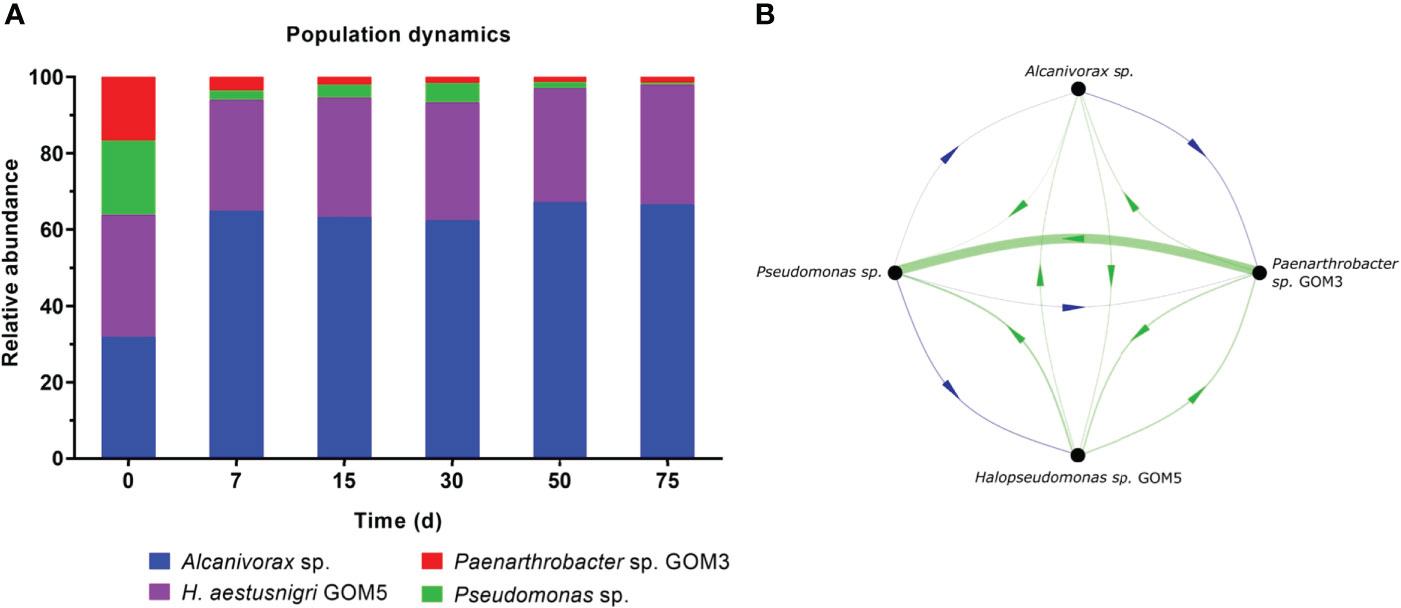
Figure 4 Population dynamics and inferred microbial interactions within the bacterial consortium when cultured with light crude oil as the sole carbon and energy source. The initial concentration of each strain was 1x108 CFU·mL-1. (A) Normalized relative abundance of the four bacterial isolates in the consortium, measured through amplicon analysis of the 16S rRNA V3-V4 regions. (B) Microbial interactions predicted by the MetaMIS software. Green lines indicate positive interactions, and blue lines indicate negative ones. Line thickness indicates the weight of each interaction, and the arrows indicate the direction of influence.
The population dynamics were subsequently analyzed using the MetaMIS tool to infer a network of interactions with a consensus direction (Figure 4B). According to this simulation, there were eight positive and four negative interactions among strains, indicating that the relationships were more cooperative than competitive overall. The strongest interaction was a positive one, from Paenarthrobacter sp. GOM3 to Pseudomonas sp. Pseudomonas sp. had a negative influence on all other consortium members; the remaining negative interaction was from Alcanivorax sp. to Paenarthrobacter sp. GOM3.
4 Discussion
Biodegradation has emerged as an eco-friendly strategy to remove HCs from the environment. It is preferred over any physical or chemical processes because it directly degrades contaminants, rather than just transforming them (Ravindra et al., 2019). Therefore, any effort that contributes to new knowledge in this field is valuable. All aerobic organisms seem to have some ability to metabolize HCs, but some use them as their major source of carbon for growth (Prince et al., 2010). While some HC-degraders are limited to certain HCs, others can be more versatile (Brzeszcz and Kaszycki, 2018).
Existing research on the bacterial degradation of HCs includes various approaches, such as focusing on the capacity of a single species to consume one or more HCs as the sole carbon source, or the use of mixed cultures consisting of 1, 2 or more species (Jia et al., 2016). The first approach may provide information about the metabolic pathways and the gene machinery involved. Notably, crude oil is a mixture of over 20,000 chemical compounds (Prince et al., 2010), and these studies demonstrate that one single bacterial species cannot degrade all of them. The second approach explores possible cooperative interactions among species. For instance, metabolic complementarity was observed during alkane degradation by a synthetic consortium consisting of Pseudomonas and Acinetobacter (Chen et al., 2014). Other studies have individually selected bacterial strains to assemble a consortium with improved degradation capacity, demonstrating that a synthetic bacterial community may be more efficient for bioremediation than a single species, especially in the case of complex pollutant mixtures (Malik, 2012; Ebadi et al., 2017). There are many additional reports where natural microbial communities have been tested for oil degradation, validating their potential use in bioremediation (Marietou et al., 2018; Xu et al., 2018). While some of these studies resulted in the development of patents employing bacterial consortia, patents targeting oil-contaminated seawater are currently very limited, despite the high daily risks of oil spills worldwide (Villela et al., 2019).
Bacterial communities can offer a greater variety of genes involved in HC biodegradation: while some bacteria may secrete HC-degrading enzymes and growth factors, others may produce biosurfactants that improve the solubility of HCs for better community access (Patowary et al., 2016). Nevertheless, not every combination of microorganisms can improve HC biodegradation, and selecting the bacteria to assemble a synergic consortium may be challenging. Indeed, our PB factorial design results (Figure 1A) showed that some combinations of bacteria reached a light oil removal of up to ~60%, while others had values close to that of the abiotic control. These results indicate that HC removal was favored in some consortia, but also suggested that competitive interactions may be occurring with certain species combinations as have been reported in other studies (Freilich et al., 2011). Of the 19 bacterial combinations tested, run 5 was the one with the highest HC removal. This consortium consisted of seven of the ten original genera, most of them being strains of the genus Pseudomonas, which is well-known for its ability to degrade a wide range of HCs (Prince et al., 2010).
According to our PB statistical analysis, the four strains with the highest positive effects on HC removal (Figure 1B) were also the most statistically significant in terms of light crude oil removal, and thus were selected to assemble our final consortium. This consortium achieved an HC removal of ~62%, a result comparable to the one obtained with the best PB combination consisting of ten bacterial strains (run 5). Such reduction in the number of combinations tested and of strains selected based on a PB design, represents a biotechnological advantage, as it reduces the amount of time and resources invested on consortium development.
The isolates selected for our consortium, belonging to the genera Pseudomonas, Halopseudomonas, Paenarthrobacter and Alcanivorax, removed light crude oil individually, reaching 25-39% of removal by gravimetry after 75 days (Figure 2B). As one species lacks the entire gene machinery to degrade alone the diverse array of hydrocarbons present in crude oil, we believe that any bacteria with crude oil biodegradation ability represents a potential resource to be used in bioremediation strategies. This is confirmed by numerous reports of individual bacteria with total crude oil removal percentages of 12-34% (Sugiura et al., 1997), 44% (Hara et al., 2003), 10-60% (Bilen Ozyurek and Seyis Bilkay, 2017), and 35-41% (Titah et al., 2021), among others.
In consortium, the selected isolates presumably removed light crude oil through metabolic complementarity and/or cooperative interactions. Pseudomonas, related to Halopseudomonas (Rudra and Gupta, 2021) and Arthrobacter, closely related to Paenarthrobacter (Busse, 2016) are two of the genera most frequently employed in patented bioremediation strategies, as they are versatile bacteria, capable of degrading recalcitrant compounds and different types of HCs (Villela et al., 2019). There are many reports describing HC degradation by the ubiquitous Pseudomonas. Our biodegradation kinetics study of the individually cultured strains, helpful to identify the hydrocarbon preferences of each isolate, showed that Pseudomonas sp. is capable of degrading a wide range of n-alkanes, with a preference for the C13-C17, the C18-C22 and the C33-C38 fractions (Supplementary Figure S2), and some PAHs (Supplementary Figures S3, S4), albeit having lower aromatic biodegradation levels than those of the other strains (Figure 3B).
Rosas-Díaz et al. (2021) reported the monoaromatic degradation capacity of the Paenarthrobacter sp. GOM3 strain and suggested its potential use in a bacterial consortium for oil removal. Our results show that Paenarthrobacter sp. GOM3 degrades some n-alkanes (preferring the C13-C17 and the C18-C22 fractions, Supplementary Figure S2) and PAHs (Supplementary Figures S3, S4). On the other hand, high efficiencies of HC degradation had been reported with the Alcanivorax genus (Santisi et al., 2015; Liu et al., 2019). This hydrocarbonoclastic taxon can use alkanes as a source of carbon, and some strains can even degrade PAHs (Brito et al., 2006), just as observed with our strain (Supplementary Figures S2, S3). Our literature search found no reports describing HC degradation by H. aestusnigri, even though we are aware of at least two strains of this species having been recovered from oil contaminated sources (Sánchez et al., 2014; Rojas-Vargas et al., 2022). Our H. aestusnigri GOM5 was recovered from an asphalt marine sediment (Rojas-Vargas et al., 2022), degrades some n-alkanes (preferring the C18-C22 fraction, Supplementary Figure S2) and PAHs (Supplementary Figures S3, S4).
The diverse HC compounds analyzed by GC-MS presented individual biodegradation patterns among the isolates, although some curves can be comparable (Supplementary Figures S2 and S3). We consider this was helpful to predict the role of each isolate. We hypothesize that our Pseudomonas sp. and Alcanivorax sp. isolates had a key role in alkane degradation by the consortium, given that they exhibited a biodegradation curve pattern similar to that of the consortium (Figure 3B). Presumably in consortium, Pseudomonas sp. consumes faster the C23-C27, C28-C32 and C33-C38 fractions within the first seven days, and both strains consume the C13-C17 and C18-C22 fractions faster than Paenarthrobacter sp. GOM3 and H. aestusnigri GOM5 (Supplementary Figure S2). Thus, explaining their competitive relationships (negative interactions) as predicted by MetaMIS simulation. Many reports have described the genes and the pathways involved in oil biodegradation by different species of these two genera (Xu et al., 2018; Moreno and Rojo, 2019). They are known to have the basic membrane alkane monooxygenase system (alkB, rubB, rdxR) and other complementary genes that encode for a flavin-binding monooxygenase (almA) or a cytochrome P450. The drop in the relative abundance of Pseudomonas sp. after day 7 (Figure 4A) could be due to the depletion of some hydrocarbons that stimulated its rapid growth (Supplementary Figure S1), and to its poor aromatic degradation performance compared to the other isolates (Figure 4B). This observation leads us to infer that, in the 7-day initial period of adaptation of the populations, the isolates might be defining their roles in degradation, precisely when the highest concentration drop of HCs occurred, as evidenced by gravimetry (Figure 3) and by GC-MS analysis (Supplementary Figures S1, S4). On the other hand, the relative abundance of Alcanivorax sp. almost doubled by day 7 and remained constant thereafter, perhaps due to its good performance in degrading aromatics (Figure 3B) and other HCs (Figure 3C). The relative abundance of H. aestusnigri GOM5 was stable during the entire incubation period, indicating a good adaptation within the consortium. This is consistent with the fact that the MetaMIS simulation showed positive interactions from this strain to all others in the consortium (Figure 4B).
While our biodegradation analysis did not include monoaromatic compounds, it is likely that these were present in the light crude oil used, and we thus infer that Paenarthrobacter sp. GOM3 degraded some of them, in turn improving the bioavailability of other HCs and/or producing some metabolites during their degradation. These events could conceivably benefit the Pseudomonas isolate and could explain the strong interaction from Paenarthrobacter sp. GOM3 to Pseudomonas sp. inferred by the MetaMIS simulation (Figure 4B). These kinds of relationships have been reported in many natural and artificial bacterial communities (Freilich et al., 2011). Besides, Paenarthrobacter sp. GOM3 had the highest positive effect in PBD among the 19 isolates (Figure 1B) and was statistically significant for light crude oil removal (Table 3). By this analysis, its presence in the consortium is important. Also, it’s been reported that rare populations in a community may play a key role for the equilibrium of it (Jain et al., 2014).
H. aestusnigri GOM5 had positive interactions with the rest of the strains, indicating it promoted their growth. Upon examination of its genome (GenBank accession number GCA_021184005.1), we found genes that encode for the synthesis of biosurfactants, namely 1-acylglycerol-3-phosphate O-acyltransferase gene (plsC), phosphatidate cytidylyltransferase gene (cdsA), CDP-diacylglycerol glycerol-3-phosphate 3-phosphatidyltransferase gene (pgsA), glycerol-3-phosphate acyltransferase gene (plsY). Biosurfactants provide for a less toxic HC-containing environment because they emulsify and solubilize them, readily promoting their bioavailability and biodegradation (Abe et al., 1995).
The inferred interaction network predicted positive relationships from Alcanivorax sp. to H. aestusnigri GOM5 and Pseudomonas sp., but negative to Paenarthrobacter sp. GOM3, which may be associated with a competitive relationship (Figure 4B). Alcanivorax sp. and Paenarthrobacter sp. GOM3 exhibited similar biodegradation patterns for some PAHs like 1-methyl-9H-fluorene, fluorene and 2,6-dimethylnaphthalene, during the first 15 days (Supplementary Figure S3), and for the C23-C27 and the C33-C38 alkane fractions during the first seven days (Supplementary Figure S2). The other three negative interactions were from Pseudomonas sp. to all other genera. This genus exhibited the highest biodegradation levels of n-alkanes individually, suggesting probable competitive interactions for the same resources.
Another relevant phenomenon to consider in our study is the possible occurrence of cometabolism events during light crude oil biodegradation. These take place when non-growth-substrates are consumed together with growth-promoting-substrates, and they may be expected when there is more than one carbon source. Cometabolism has been reported for fluorene, pyrene, phenanthrene, chlorinated compounds and many other HCs (Nzila, 2013). The Paenarthrobacter sp. GOM3 strain was isolated with phenanthrene as the sole carbon source but exhibited higher growth rates with monoaromatics. This strain was reported as a monoaromatic degrader (Rosas-Díaz et al., 2021), but it can degrade other complex PAHs present in light crude oil (Supplementary Figure S3). This is consistent with the presence of cytochrome P450 monooxygenase (PF00067) in its genome (GenBank accession number GCA_018215265.1), which has been related to the degradation of alkanes and of pyrene and phenanthrene compounds (Zhou et al., 2020).
The potential advantages of using bacterial consortia include improved biodegradation levels, distribution of the metabolic load among bacterial species, and achieving stable dynamic interactions (Jia et al., 2016). Limitations include the extended time required for biodegradation, compared to physicochemical methods, as well as the difficulty of achieving comparable results during in situ applications, where countless and uncontrollable biotic and abiotic factors are at play (Ravindra et al., 2019). Further genomic analyses and gene expression studies of the consortium will be needed to gain a better understanding of its HCs biodegradation dynamics.
5 Conclusion
The PB experimental design was useful in the identification of four significant bacterial strains for the removal of light crude oil in seawater. When these strains were grown in consortium, they achieved a HC removal level comparable to that of the best PB consortium made up of 10 strains. Such reduction in the number of consortium members represents a biotechnological advantage for potential implementation as a bioremediation strategy. The observed stable population dynamics during the degradation kinetics demonstrate presumably synergistic interactions among isolates. Additionally, the inferred interaction network for the consortium, derived from the 16S amplicon analysis, showed eight out of 12 (67%) positive relationships among the isolates. This is consistent with the observed persistence of the four bacterial strains throughout the entire study period (75 days), suggesting possible cooperative relationships among them.
Data availability statement
The amplicon raw sequences presented in the study are deposited at NCBI under the BioProject accession number PRJNA845943 (https://www.ncbi.nlm.nih.gov/bioproject/PRJNA845943/), and Sequence Read Archive (SRA) accession numbers (https://www.ncbi.nlm.nih.gov/sra/PRJNA845943): SRX15604802 (day 0), SRX15604803 (7), SRX15604804 (15), SRX15604805 (30), SRX15604806 (50), and SRX15604807 (75).
Author contributions
JR-V and LP-L contributed to the design and implementation of the research. JR-V, and AS-F performed the computational and bioinformatic analyses. JR-V, LA, HS-J, AL-N and AG performed the experiments and analyzed the data. LP-L coordinated the group, managed the resources, and guaranteed their availability to perform the experiments and analysis. All authors contributed to the writing of the manuscript and approved the final version.
Funding
JR-V is a doctoral student from Programa de Doctorado en Ciencias Bioquímicas, Universidad Nacional Autónoma de México (UNAM), and received fellowship 965003 from the Consejo Nacional de Ciencia y Tecnología (CONACYT). This research was funded by the CONACYT – Mexican Ministry of Energy- Hydrocarbon Trust, project 201441. This is a contribution of the Consorcio de Investigación del Golfo de México (CIGoM). LP-L received financial support from PASPA DGAPA-UNAM for a sabbatical year.
Acknowledgments
We thank the technical support in the IBt’s Pilot Plant of PhD Leobardo Serrano Carreón, Eng. Veronica Albiter Hernandez, and M.B. Jose Raunel Tinoco Valencia, and in the laboratory of Fisicoquimica of ICML of Héctor M. Alexander Valdés. We are grateful to Luz Patricia Ortega Tenorio in hydrocarbon analysis, and to MSc. Arturo Aguilar Aguila for the PB method guidance. We thank Shirley Elizabeth Ainsworth Gore for bibliography assistance.
Conflict of interest
The authors declare that the research was conducted in the absence of any commercial or financial relationships that could be construed as a potential conflict of interest.
Publisher’s note
All claims expressed in this article are solely those of the authors and do not necessarily represent those of their affiliated organizations, or those of the publisher, the editors and the reviewers. Any product that may be evaluated in this article, or claim that may be made by its manufacturer, is not guaranteed or endorsed by the publisher.
Supplementary material
The Supplementary Material for this article can be found online at: https://www.frontiersin.org/articles/10.3389/fmars.2022.962071/full#supplementary-material
References
Abe A., Inoue A., Usami R., Moriya K., Horikoshi K.. (1995). Properties of newly isolated marine bacterium that can degrade polyaromatic hydrocarbons in the presence of organic solvents. J. Mar. Biotechnol. 2 (4), 182–186.
Antony J. (2014). Chapter 5 - Screening designs,” in design of experiments for engineers and scientists (Elsevier, NL) 51–62.
Bertani G. (2004). Lysogeny at mid-twentieth century: P1, P2, and other experimental systems. J. Bacteriol. 186 (3), 595–600. doi: 10.1128/JB.186.3.595-600.2004
Bilen Ozyurek S., Seyis Bilkay I. (2017). Determination of petroleum biodegradation by bacteria isolated from drilling fluid, waste mud pit and crude oil. Turk Biyokimya dergisi [Turkish J. Biochem] 42 (6), 1–8. doi: 10.1515/tjb-2017-0087
Box G. E. P., Stuart Hunter J., Hunter W. G. (2005). “Chapter 7 - Additional Fractionals and Analysis”, in Statistics for experimenters: Design, innovation, and discovery. Second edition. Wiley (US) 281–313.
Brito E. M. S., Guyoneaud R., Goñi-Urriza M., Ranchou-Peyruse A., Verbaere A., Crapez M. A., et al. (2006). Characterization of hydrocarbonoclastic bacterial communities from mangrove sediments in guanabara bay, Brazil. Res. Microbiol. 157 (8), 752–762. doi: 10.1016/j.resmic.2006.03.005
Brzeszcz J., Kaszycki P. (2018). Aerobic bacteria degrading both n-alkanes and aromatic hydrocarbons: An undervalued strategy for metabolic diversity and flexibility. Biodegradation 29 (4), 359–407. doi: 10.1007/s10532-018-9837-x
Busse H.-J. (2016). ‘Review of the taxonomy of the genus arthrobacter, emendation of the genus Arthrobacter sensu lato, proposal to reclassify selected species of the genus Arthrobacter in the novel genera Glutamicibacter gen. nov., Paeniglutamicibacter gen. nov., Pseudoglutamicibacter gen. nov., Paenarthrobacter gen. nov. and Pseudarthrobacter gen. nov., and emended description of Arthrobacter roseus. Int. J. Systematic Evol. Microbiol. 66 (1), 9–37. doi: 10.1099/ijsem.0.000702
Cappello S., Denaro R., Genovese M., Giuliano L., Yakimov M. M.. (2007). Predominant growth of Alcanivorax during experiments on “oil spill bioremediation” in mesocosms. Microbiological Res. 162 (2), 185–190. doi: 10.1016/j.micres.2006.05.010
Chen Y., Li C., Zhou Z., Wen J., You X., Mao Y., et al. (2014). Enhanced biodegradation of alkane hydrocarbons and crude oil by mixed strains and bacterial community analysis. Appl. Biochem. Biotechnol. 172 (7), 3433–3447. doi: 10.1007/s12010-014-0777-6
Chen Y., Ye W., Zhang Y., Xu Y.. (2015). High speed BLASTN: An accelerated MegaBLAST search tool. Nucleic Acids Res. 43 (16), 7762–7768. doi: 10.1093/nar/gkv784
Committee on the Effects of the Deepwater Horizon Mississippi Canyon-252 Oil Spill on Ecosystem Services in the Gulf of Mexico, Ocean Studies Board, Division on Earth and Life Studies, National Research Council. (2013). “Chapter 4 - Oil spill response technologies”, in An Ecosystem Services Approach to Assessing the Impacts of the Deepwater Horizon Oil Spill in the Gulf of Mexico. National Academies Press (US) 71–102. Available from: https://www.ncbi.nlm.nih.gov/books/NBK201632/
Dash H. R., Mangwani N., Chakraborty J., Kumari S., Das S.. (2013). Marine bacteria: Potential candidates for enhanced bioremediation. Appl. Microbiol. Biotechnol. 97 (2), 561–571. doi: 10.1007/s00253-012-4584-0
Ebadi A., Khoshkholgh Sima N. A., Olamaee M., Hashemi M., Ghorbani Nasrabadi R.. (2017). Effective bioremediation of a petroleum-polluted saline soil by a surfactant-producing Pseudomonas aeruginosa consortium. J. Advertising Res. 8 (6), 627–633. doi: 10.1016/j.jare.2017.06.008
Freilich S., Zarecki R., Eilam O., Segal E.S., Henry C.S., Kupiec M., et al. (2011). Competitive and cooperative metabolic interactions in bacterial communities. Nat. Commun. 2, 589. doi: 10.1038/ncomms1597
Ghanem K., Al-Zahrani M. (2016). Bioremediation of diesel fuel by fungal consortium using statistical experimental designs. Polish J. Environ. Stud. 25 (1), 97–106. doi: 10.15244/pjoes/42493
Godoy-Lozano E. E., Escobar-Zepeda A., Raggi L., Merino E., Gutierrez-Rios R.M., Juarez K., et al. (2018). Bacterial diversity and the geochemical landscape in the southwestern gulf of Mexico. Front. Microbiol. 9, 2528. doi: 10.3389/fmicb.2018.02528
Grosskopf T., Soyer O. S. (2014). Synthetic microbial communities. Curr. Opin. Microbiol. 18, 72–77. doi: 10.1016/j.mib.2014.02.002
Guevara G., Castillo Lopez M., Alonso S., Perera J., Navarro-Llorens J.M.. (2019). New insights into the genome of Rhodococcus ruber strain chol-4. BMC Genomics 20 (1), 332. doi: 10.1186/s12864-019-5677-2
Hall T. A. (1999). BioEdit: a user-friendly biological sequence alignment editor and analysis program for windows 95/98/NT’. Nucleic Acids Symposium Ser. c1979-c2000, 95–98. Others.
Hara A., Syutsubo K., Harayama S. (2003). Alcanivorax which prevails in oil-contaminated seawater exhibits broad substrate specificity for alkane degradation. Environ. Microbiol. 5 (9), 746–753. doi: 10.1046/j.1468-2920.2003.00468.x
Hassan M., Essam T., Yassin A. S., Salama A. (2016). Optimization of rhamnolipid production by biodegrading bacterial isolates using plackett-burman design. Int. J. Biol. Macromolecules 82, 573–579. doi: 10.1016/j.ijbiomac.2015.09.057
Hazen T. C., Dubinsky E. A., DeSantis T. Z., Andersen G. L., Piceno Y. M., Singh N., et al. (2010). Deep-sea oil plume enriches indigenous oil-degrading bacteria. Science 330 (6001), 204–208. doi: 10.1126/science.1195979
Head I. M., Jones D. M., Röling W. F. M. (2006). ‘Marine microorganisms make a meal of oil’, nature reviews. Microbiology 4 (3), 173–182. doi: 10.1038/nrmicro1348
Herlemann D. P., Labrenz M., Jürgens K., Bertilsson S., Waniek J. J., Andersson A. F. (2011). Transitions in bacterial communities along the 2000 km salinity gradient of the Baltic Sea. ISME J. 5 (10), 1571–1579. doi: 10.1038/ismej.2011.41
Jain M., Flynn D. F., Prager C. M., Hart G. M., Devan C. M., Ahrestani F. S., et al. (2014). The importance of rare species: A trait-based assessment of rare species contributions to functional diversity and possible ecosystem function in tall-grass prairies. Ecol. Evol. 4 (1), 104–112. doi: 10.1002/ece3.915
Jia X., Liu C., Song H., Ding M., Du J., Ma Q., et al. (2016). Design, analysis and application of synthetic microbial consortia. Synthetic Syst. Biotechnol. 1 (2), 109–117. doi: 10.1016/j.synbio.2016.02.001
Jiang R., Sun L. W., Zhao Y., Feng K., Chen S. Y.. (2013). ‘Optimization of culture parameters for expression of recombinant human basic fibroblast growth factor in pichia pastoris using response surface methodology combining with plackett-burman design’. Biomaterial Bioeng. 647, 456–461. doi: 10.4028/www.scientific.net/AMR.647.456
Karkaria B. D., Fedorec A. J. H., Barnes C. P. (2021). Automated design of synthetic microbial communities. Nat. Commun. 12 (1), 672. doi: 10.1038/s41467-020-20756-2
Kong W., Meldgin D. R., Collins J. J., Lu T.. (2018). Designing microbial consortia with defined social interactions. Nat. Chem. Biol. 14 (8), 821–829. doi: 10.1038/s41589-018-0091-7
Lane D. J. (1991)16S/23S rRNA sequencing. In: Nucleic acid techniques in bacterial systematic (New York: John Wiley and Sons). Available at: https://www.scirp.org/(S(lz5mqp453edsnp55rrgjct55))/reference/ReferencesPapers.aspx?ReferenceID=1870033 (Accessed 2 February 2022).
Li Z.-D., Zhao Y. Z., Quan X., Chen S.. (2007). Photochemical degradation of petroleum on soil surfaces under simulated visible light. Huan jing ke xue= Huanjing kexue/[bian ji Zhongguo ke xue yuan huan jing ke xue wei yuan hui ‘Huan jing ke xue’ bian ji wei yuan hui 28 (9), 2052–2057.
Li W., Godzik A. (2006). Cd-hit: a fast program for clustering and comparing large sets of protein or nucleotide sequences. Bioinformatics 22 (13), 1658–1659. doi: 10.1093/bioinformatics/btl158
Liu J., Zheng Y., Lin H., Wang X., Li M., Liu Y., et al. (2019). Proliferation of hydrocarbon-degrading microbes at the bottom of the Mariana trench. Microbiome 7 (1), 47. doi: 10.1186/s40168-019-0652-3
Lowry O. H., Rosebrough N. J., Farr A. L., Randall R. J.. (1951). Protein measurement with the folin phenol reagent. J. Biol. Chem. 193 (1), 265–275. doi: 10.1016/S0021-9258(19)52451-6
Lv Y., Chen Y., Sun S., Hu Y.. (2014). Interaction among multiple microorganisms and effects of nitrogen and carbon supplementations on lignin degradation. Bioresource Technol. 155, 144–151. doi: 10.1016/j.biortech.2013.12.012
Magoč T., Salzberg S. L. (2011). FLASH: Fast length adjustment of short reads to improve genome assemblies. Bioinformatics 27 (21), 2957–2963. doi: 10.1093/bioinformatics/btr507
Malik Z. A., Ahmed S. (2012). Degradation of petroleum hydrocarbons by oil field isolated bacterial consortium. Afr. J. Biotechnol. 11 (3), 650–658. doi: 10.5897/ajb11.036
Mapelli F., Scoma A., Michoud G., Aulenta F., Boon N., Borin S., et al. (2017). Biotechnologies for marine oil spill cleanup: Indissoluble ties with microorganisms. Trends Biotechnol. 35 (9), 860–870. doi: 10.1016/j.tibtech.2017.04.003
Marietou A., Chastain R., Beulig F., Scoma A., Hazen T. C., Bartlett D. H.. (2018). The effect of hydrostatic pressure on enrichments of hydrocarbon degrading microbes from the gulf of Mexico following the deepwater horizon oil spill. Front. Microbiol. 9, 808. doi: 10.3389/fmicb.2018.00808
Mazzucotelli C. A., Durruty I., Kotlar C. E., Moreira M. R., Ponce A. G., Roura S. I. (2014). Development of a microbial consortium for dairy wastewater treatment. Biotechnol. Bioprocess Eng: BBE 19 (2), 221–230. doi: 10.1007/s12257-013-0517-8
McGinnis S., Madden T. L. (2004). BLAST: At the core of a powerful and diverse set of sequence analysis tools. Nucleic Acids Res. 32 (Web Server issue), W20–W25. doi: 10.1093/nar/gkh435
Migahed F., Abdelrazak A., Fawzy G. (2017). Batch and continuous removal of heavy metals from industrial effluents using microbial consortia. Int. J. Environ. Sci. Technol. 14 (6), 1169–1180. doi: 10.1007/s13762-016-1229-3
Monciardini P., Sosio M., Cavaletti L., Chiocchini C., Donadio S.. (2002). New PCR primers for the selective amplification of 16S rDNA from different groups of actinomycetes. FEMS Microbiol. Ecol. 42 (3), 419–429. doi: 10.1016/S0168-6496(02)00353-7
Moreno R., Rojo F. (2019). “Enzymes for aerobic degradation of alkanes in bacteria,” in Aerobic utilization of hydrocarbons, oils, and lipids. Ed. Rojo F. (Cham: Springer International Publishing), 117–142.
Muriel-Millán L. F., Rodríguez-Mejía J. L., Godoy-Lozano E. E., Rivera-Gómez N., Gutierrez-Rios R. M., Morales-Guzmán D., et al. (2019). Functional and genomic characterization of a Pseudomonas aeruginosa strain isolated from the southwestern gulf of Mexico reveals an enhanced adaptation for long-chain alkane degradation. Front. Mar. Sci. 6. doi: 10.3389/fmars.2019.00572
Muriel-Millán L. F., Millán-López S., Pardo-López L. (2021). Biotechnological applications of marine bacteria in bioremediation of environments polluted with hydrocarbons and plastics. Appl. Microbiol. Biotechnol. 105 (19), 7171–7185. doi: 10.1007/s00253-021-11569-4
Nicodem D. E., Guedes C. L., Correa R. J.. (1997). Photochemical processes and the environmental impact of petroleum spills. Biogeochemistry 39 (2), 121–138. doi: 10.1023/A:1005802027380
Nzila A. (2013). Update on the cometabolism of organic pollutants by bacteria. Environ. pollut. 178, 474–482. doi: 10.1016/j.envpol.2013.03.042
Patowary K., Patowary R., Kalita M. C., Deka S.. (2016). Development of an efficient bacterial consortium for the potential remediation of hydrocarbons from contaminated sites. Front. Microbiol. 7, 1092. doi: 10.3389/fmicb.2016.01092
Prajapati V. S., Soni N., Trivedi U. B., Patel K. C.. (2014). An enhancement of red pigment production by submerged culture of Monascus purpureus MTCC 410 employing statistical methodology. Biocatalysis Agric. Biotechnol. 3 (2), 140–145. doi: 10.1016/j.bcab.2013.08.008
Prince R. C., Gramain A., McGenity T. J. (2010). “Prokaryotic hydrocarbon degraders,” in Handbook of hydrocarbon and lipid microbiology. Ed. Timmis K. N. (Berlin, Heidelberg: Springer Berlin Heidelberg), 1669–1692.
Raggi L., García-Guevara F., Godoy-Lozano E. E., Martínez-Santana A., Escobar-Zepeda A., Gutierrez-Rios R. M., et al. (2020). Metagenomic profiling and microbial metabolic potential of perdido fold belt (NW) and campeche knolls (SE) in the gulf of mexico’. Front. Microbiol. 11, 1825. doi: 10.3389/fmicb.2020.01825
Ravindra, Arya P., Haq S. A. (2019). “Chapter 4 - effects of xenobiotics and their biodegradation in marine life,” in Smart bioremediation technologies. Ed. Bhatt P. First edition. Academic Press (UK) 63–81. doi: 10.1016/B978-0-12-818307-6.00004-4
Richter W. (1997). Primary methods of measurement in chemical analysis, Accreditation and quality assurance. 2 (8), 354–359. doi: 10.1007/s007690050165
Rodríguez-Salazar J., Loza A., Ornelas-Ocampo K., Gutierrez-Rios R. M., Pardo-López L., et al. (2021). Bacteria from the southern gulf of Mexico: Baseline, diversity, hydrocarbon-degrading potential and future applications. Front. Mar. Sci. 8. doi: 10.3389/fmars.2021.625477
Rojas-Vargas J., González-Sánchez R., Sánchez-Flores A., Licea-Navarro A. F., Pardo-López L.. (2022). Complete genome sequence of Halopseudomonas aestusnigri strain GOM5, isolated from asphalt marine sediments of the gulf of Mexico. Microbiol. Resource Announcements 11 (4), e0122221. doi: 10.1128/mra.01222-21
Rosas-Díaz J., Escobar-Zepeda A., Adaya L., Rojas-Vargas J., Cuervo-Amaya D. H., Sánchez-Reyes A., et al. (2021). Paenarthrobacter sp. GOM3 is a novel marine species with monoaromatic degradation relevance. Front. Microbiol. 12, 713702. doi: 10.3389/fmicb.2021.713702
Rudra B., Gupta R. S. (2021). Phylogenomic and comparative genomic analyses of species of the family pseudomonadaceae: Proposals for the genera Halopseudomonas gen. nov. and Atopomonas gen. nov., merger of the genus Oblitimonas with the genus Thiopseudomonas, and transfer of some misclassified species of the genus Pseudomonas into other genera. Int. J. Systematic Evol. Microbiol. 71 (9), 005011. doi: 10.1099/ijsem.0.005011
Sánchez D., Mulet M., Rodríguez A. C., David Z., Lalucat J., García-Valdés E.. (2014). Pseudomonas aestusnigri sp. nov., isolated from crude oil-contaminated intertidal sand samples after the prestige oil spill. Systematic Appl. Microbiol. 37 (2), 89–94. doi: 10.1016/j.syapm.2013.09.004
Santisi S., Cappello S., Catalfamo M., Mancini G., Hassanshahian M., Genovese L., et al. (2015). Biodegradation of crude oil by individual bacterial strains and a mixed bacterial consortium. Braz. J. Microbiol. 46 (2), 377–387. doi: 10.1590/S1517-838246120131276
Shaw G. T.-W., Pao Y.-Y., Wang D. (2016). MetaMIS: A metagenomic microbial interaction simulator based on microbial community profiles. BMC Bioinf. 17 (1), 488. doi: 10.1186/s12859-016-1359-0
Silva-Jiménez H., Araujo-Palomares C. L., Macías-Zamora J. V., Ramírez-Álvarez N., García-Lara B., et al. (2018). Identification by MALDI-TOF MS of environmental bacteria with high potential to degrade pyrene. J. Mexican Chem. Soc. 62 (2), 214–225. doi: 10.29356/jmcs.v62i2.411
Stoddard S. F., Smith B. J., Hein R., Roller B. R., Schmidt T. M.. (2015). rrnDB: improved tools for interpreting rRNA gene abundance in bacteria and archaea and a new foundation for future development. Nucleic Acids Res. 43 (Database issue), D593–D598. doi: 10.1093/nar/gku1201
Sugiura K., Ishihara M., Shimauchi T., Harayama S.. (1997). Physicochemical properties and biodegradability of crude oil. Environ. Sci. Technol. 31 (1), 45–51. American Chemical Society (ACS). doi: 10.1021/es950961r
Titah H. S., Pratikno H., Purwanti I. F., Wardhani W. K.. (2021). Biodegradation of crude oil spill using Bacillus subtilis and Pseudomonas putida in sequencing method. Inżynieria Ekologiczna 22 (11), 157–167. Wydawnictwo Naukowe Gabriel Borowski (WNGB). doi: 10.12911/22998993/142913
Tortora G. J., Funke B. R., Case C. L. (2007). Microbiology: An Introduction. Ninth edition. Pearson Benjamin Cummings (US).
Vatsal A., Potdar C., Zinjarde S. S., Ravi Kumar V., Kulkarni B. D., RaviKumar A.. (2016). Role of aliasing and interacting factors in the enhanced production of dehalogenase from Yarrowia lipolytica for degradation of brominated compounds. J. Ind. Eng. Chem. 41, 114–121. doi: 10.1016/j.jiec.2016.07.015
Villela H. D. M., Peixoto R. S., Soriano A. U., Carmo F. L.. (2019). Microbial bioremediation of oil contaminated seawater: A survey of patent deposits and the characterization of the top genera applied. Sci. Total Environ. 666, 743–758. doi: 10.1016/j.scitotenv.2019.02.153
Xu X., Liu W., Tian S., Wang W., Qi Q., Jiang P., et al. (2018). Petroleum hydrocarbon-degrading bacteria for the remediation of oil pollution under aerobic conditions: A perspective analysis. Front. Microbiol. 9, 2885. doi: 10.3389/fmicb.2018.02885
Keywords: marine bacteria consortium, hydrocarbon-degrading bacteria, Gulf of Mexico, Plackett-Burman, oil-contaminated seawater, marine bioremediation
Citation: Rojas-Vargas J, Adaya L, Silva-Jiménez H, Licea-Navarro AF, Sanchez-Flores A, Gracia A and Pardo-López L (2022) Oil-degrading bacterial consortium from Gulf of Mexico designed by a factorial method, reveals stable population dynamics. Front. Mar. Sci. 9:962071. doi: 10.3389/fmars.2022.962071
Received: 05 June 2022; Accepted: 05 September 2022;
Published: 23 September 2022.
Edited by:
M. Leopoldina Aguirre-Macedo, Center for Research and Advanced Studies-Mérida Unit, MexicoReviewed by:
Piyush Pandey, Assam University, IndiaRafael Bargiela, Bangor University, United Kingdom
Copyright © 2022 Rojas-Vargas, Adaya, Silva-Jiménez, Licea-Navarro, Sanchez-Flores, Gracia and Pardo-López. This is an open-access article distributed under the terms of the Creative Commons Attribution License (CC BY). The use, distribution or reproduction in other forums is permitted, provided the original author(s) and the copyright owner(s) are credited and that the original publication in this journal is cited, in accordance with accepted academic practice. No use, distribution or reproduction is permitted which does not comply with these terms.
*Correspondence: Liliana Pardo-López, bGlsaWFuYS5wYXJkb0BpYnQudW5hbS5teA==