- 1Department of Marine Sciences-Tjärnö, University of Gothenburg, Strömstad, Sweden
- 2Department of Biology and Biological Engineering-Food and Nutrition Science, Chalmers University of Technology, Gothenburg, Sweden
- 3Department of Biology and Biological Engineering-Industrial Biotechnology, Chalmers University of Technology, Gothenburg, Sweden
Sea lettuce (Ulva) aquaculture has increased the last decade due to high productivity, wide environmental tolerance, and interesting functional and nutritional properties of the crop. Research focus has mainly been on adult biomass production, but knowledge of performance and biochemical content of early developmental stages – which are the basis to any large-scale production - is still limited. The life-history of Ulva alternates between a diploid sporophytic life-stage and a haplontic gametophytic life-stage. Whereas the sporophyte give raise to recombinant gametophytes through zoids, gametophytes can give raise to parthenogenetically developing, clonal gametes in absence of a mating partner. This study shows that recombinant gametophytes have a faster ontogenetic development, higher growth rate, as well as higher protein, fatty acid, and pigment contents compared to clonal gametophytes of the crop Ulva fenestrata. Nutrient addition is required for a normal development, but temperature and swarmer density have relatively small effects on the hatchery success, relative growth rate and biochemical profile of the juvenile biomass. Our study reveals that the selection of the life-history-phase in novel sea lettuce crop strains could largely contribute to the emerging seaweed aquaculture sector.
Introduction
Seaweed aquaculture is currently the most rapidly expanding aquaculture sector (Brakel et al., 2021). Because seaweeds are relatively environmentally friendly to produce and contain a large variety of high-value compounds, used in various food and non-food applications, seaweed aquaculture is an obvious part of a future sustainable Blue Economy and has received increased research interest during the last decades (Holdt and Kraan, 2011; FAO et al., 2016; Naylor et al., 2021). The largest part of the seaweed market is composed of brown kelp species (e.g. Laminaria sp., Saccharina sp. and Undaria sp.) as well as red seaweeds (e.g. Eucheuma spp., Gracilaria spp., Kappaphycus alvarezii, and Porphyra spp.) and their products are mostly targeted for the food and phycocolloid industry (alginates, agars, and carrageenans) (FAO et al., 2016, FAO et al., 2018). Although green seaweeds currently contribute less than 0.1% to the total biomass production (Paul et al., 2012), the cosmopolitan genus Ulva is characterized by several traits that make it commercially interesting. For example, Ulva biomass and associated compounds are used in the food industry (Colombo et al., 2006; Abreu et al., 2014; Santos et al., 2015; Trigo et al., 2021), and their application is being evaluated for the pharmaceutical, nutraceutical and cosmetic sector (Coelho et al., 2014; Hafting et al., 2015; Barzkar et al., 2019), as well as feedstock for biofuel production (Coelho et al., 2014; Chen et al., 2015; Abomohra et al., 2018). Furthermore, Ulva species stand out with their rapid proliferation and high growth rates (Lawton et al., 2013; Sebök et al., 2019), can cope and thrive in high stocking densities (Mata et al., 2010; Mata et al., 2016; Al-Hafedh et al., 2014), and are tolerant towards varying environmental factors (e.g. temperature, salinity, eutrophication) (Steinhagen et al., 2019a; Steinhagen et al., 2019b), which offers excellent qualities as crop species for the emerging Blue Economy at a global scale. Cultivation oriented research especially on the foliose shaped sea-lettuce-type of Ulva has increased during the past ten to fifteen years (Califano et al., 2020; Toth et al., 2020; Steinhagen et al., 2021; Steinhagen et al., 2022). Foliose species of Ulva are mostly maintained in free-floating cultures and are cultivated near- or on-shore (Hafting et al., 2015) in tanks (Toth et al., 2020), bioreactors (Sebök et al., 2019), basins (e.g. paddle-wheel basins) (Amosu et al., 2016) or ponds (Califano et al., 2020) and more recently also off-shore (Steinhagen et al., 2021; Steinhagen et al., 2022). Due to the increasing interest of the European seaweed sector in the aquaculture of Ulva species – which is based on the combination of above named compelling traits for cultivation and biomass valorization – upscaling processes, novel large-scale farming techniques, and closed life-cycle crop systems are needed. Another crucial part for a successful economic-scale seaweed aquaculture is the elucidation of optimal cultivation conditions, which strongly depend on the desired biomass trait such as yield and/or biochemical composition (Toth et al., 2020). Previous studies have shown that environmental growth conditions have significant effects on the relative growth rate as well as on the biochemical profile as on e.g. protein, fatty acids, carbohydrate or phenolic contents (Gao et al., 2018a; Gao et al., 2018b; Toth et al., 2020; Olsson et al., 2020a; Olsson et al., 2020b).
In most studies, the origin of cultivated Ulva biomass is either declared as originating from wild harvests or is not clearly defined, and only seldom raised from explicit seedling hatcheries. In contrast to wild harvests and proliferating collected input biomass, artificial seeding and controlled seedling hatcheries allow for biomass stocks of similar age and life-stage. This guarantees independence of natural and/or seasonal fluctuations and thus result in cleaner input stocks (e.g. by exclusion of epiphytic impurities, pests and attached macrozoobenthos incl. eggs). Furthermore, by controlled seedling hatcheries, closed life-cycle aquacultures are enabled, which protects native ecosystems and seaweed stocks (Mac Monagail et al., 2017). Additionally, previous studies stressed that the exploitation of wild seaweed resources (mainly kelp) in Scandinavia (e.g. Norway) could not satisfy the demand of the industry (Stévant et al., 2017), underlining the necessity of sound future seaweed hatcheries. Since most of the produced Ulva biomass originates from opportunistic wild harvests of adult material in close vicinity to the farming site (Fort et al., 2019), an aspect that has been widely neglected is the determination of the life-history stage of the farmed biomass and its possible effects on growth performance and/or biochemical composition. As already mentioned by Carl et al. (2014), key to a reliable, cost-effective, and constant biomass supply of Ulva for commercial purpose is the development and optimization of closed life cycle biomass production.
The life cycle of Ulva is characterized as being isomorphic (gametophyte and sporophyte look the same) and biphasic (Bråten, 1971). The life-history of Ulva alternates between a diploid sporophytic life-stage and a haplontic gametophytic life-stage which are macroscopic. The haploid gametophytes are either recombinant and originate from haploid zoids of sporophytes, or they are clonal and originate from unmated (parthenogenetic) biflagellate gametes, or seldomly from zoids of parthenosporophytes (Løvlie and Bryhni, 1978; Wichard et al., 2015). Gametes originating from gametophytes are characterized as biflagellate. They are mostly positive phototactic (attracted by light) and can sexually reproduce with a gamete of the opposite mating type and in absence of a sexual mating partner they bare the potential of parthenogenesis (Wichard et al., 2015). Zoids (also called zoospores) originate from sporophytes and distinguish as being quadriflagellate and negative phototactic (repelled by light). Zoids of both mating types are released by sporophytes in equal ratios. It should be especially stressed that under rare conditions also quadriflagellate parthenospores have been observed (Bråten, 1971; Wichard et al., 2015). In most distromatic (two cell layers), foliose Ulva species the formation of reproductive cells occurs on the thallus margins (Hiraoka et al., 1998; Wichard and Oertel, 2010). The marginal cells either directly differentiate into gametangia (gametophyte) or sporangia (sporophyte) and cellular changes from vegetative to reproductive tissue are also recognized by the emerging yellowish to brownish color change (Hiraoka et al., 1998). Sporophytes give raise to recombinant gametophytes through zoids and gametophytes give raise to parthenogenetically developing gametes.
Although, many Ulva species have been identified as being isogamous (Bråten, 1971; Kagami et al., 2008), anisogamous traits have been identified in certain species (Melkonian, 1980; Hiraoka et al., 1998). Gametes of northern hemisphere individuals identified as Ulva lactuca Linnaeus [recently identified as Ulva fenestrata Postels & Ruprecht based on molecular observations (Hughey et al., 2019; Steinhagen et al., 2019a)] exhibited significant differences in the colour and thus pigment composition between the two mating types (Melkonian, 1980). Thus, respective differences among the reproductive cell types of different Ulva spp. have been observed in previous studies (Melkonian, 1980; Hiraoka et al., 1998). If differences observed among reproductive stages might also manifest during their early ontogenetic development, or lead to different biochemical properties of the biomass, has not yet been investigated and such vital information for the aquaculture sector is lacking.
U. fenestrata is a putative crop species in the Northern Hemisphere (Steinhagen et al., 2021; Steinhagen et al., 2022) where it is abundantly present in marine water bodies (Hughey et al., 2019; Steinhagen et al., 2019a). Individuals can be found in the sublittoral and are mainly present during Northern Hemisphere spring to summer conditions, whereas especially during summer conditions fertile margins of mature individuals could be observed.
Within the present study we aimed to investigate how changes in cultivation conditions (seeding density [500, 5000, 10 000 swarmers ml-1], temperature [10, 15°C], nutrients [PES, PESx3 (Provasoli Enriched Seawater)]) affect the hatchery behavior and thus the early ontogenetic development of two different reproductive stages – gametes and zoids – of Swedish Ulva fenestrata (formerly identified in the region as Ulva lactuca). Additionally, by further cultivating the hatched seedlings and applying respective manipulated cultivation conditions, we aimed to test, if recombinant and clonal gametophytes show differences in growth and their biochemical profiles (total fatty acid, protein, carbohydrate, pigments). We conducted two consecutive, manipulative experiments where we tested the interactive effects of above named factors on 1) the early ontogenetic development of gametes and zoids and on 2) the biochemical composition of young thalli of U. fenestrata. With above described manipulative experiments we aimed to investigate whether the life-history stage affects the large-scale cultivability and/or biochemical composition of Ulva, which until date, has never been investigated in detail. Furthermore, we aimed to optimize the cultivation conditions of the northern hemisphere U. fenestrata to facilitate a sustainable closed life-cycle biomass production.
Methods and materials
Algal material and preparation of reproductive tissue
For the initial zoid and gamete release, sporophytes and gametophytes of U. fenestrata were taken from long-term indoor tank cultivations located at the Tjärnö Marine Laboratory, University of Gothenburg, Sweden (TML, 58°52’36.4”N 11°6’42.84”E). The molecular identification of the long-term culture of U. fenestrata used in our study is described in detail by Toth et al. (2020) and GenBank accession numbers of tufA sequences are publicly available (MN240309, MN240310, MN240311). The parental seaweed thalli were grown in 90 L cultivation tanks in a green-house under a 16:8 h (L:D) photo regime at an irradiance of 120-140 µmol m-2 s-1 (light source: INDY66 LED 60 W 4000 K 6000 lumen). The seaweeds grew in filtered (5 µm + UV) natural seawater (from 40 m depth) without additional medium or chemicals in a flow-through system (flow = 10-14 L h-1) under permanent aeration. The salinity and temperature fluctuated depending on the prevailing weather and seasonal conditions (SMHI, 2022).
To induce the fertility, discs with a radius of 4 cm were taken from epiphyte free gametophytic and sporophytic vegetative thalli tissue and subsequently transferred into a 14 L aquarium at 12°C. The discs were kept under permanent aeration and a flow of approximately 7 L h-1. After 4-5 days the discs turned darker in color and either sporangia or gametangia started to form. The fertile discs were washed under sterile filtrated seawater and transferred to a beaker filled with approximately 80-100 mL of sterile seawater where they immediately started to release the respective swarmers. The life stage of the swarmers was determined by counting the number of flagella and measuring their size via light microscopy. Both, zoids and gametes used in the following experiment originated from single individuals. Swarmer solutions were concentrated by centrifugation in a chilled centrifuge (12°C) at 5000 rpm for 5 min, immobilized in the dark for 24 hours at 12°C, and the density of the solutions were determined with a hemocytometer.
Experimental set up phase I: seedling hatchery
The first experiment on the hatchery performance of gametes and zoids of U. fenestrata was performed in 5 mL six-well-plates (Sarstedt, Nümbrecht, Germany) in thermostatic culture cabinets (LIEBHERR, Kirchdorf, Germany) where temperature (10°C and 15°C), swarmer density (500, 5000, 10 000 swarmers mL-1) and the concentration of Provasoli Enriched Seawater (Seawater [SW], 1x PES, 3x PES) (Provasoli, 1986) were manipulated in a fully crossed design (n = 6, corresponding to 108 wells). The swarmers in respective treatments grew under a 16:8 h (L:D) photo regime at an irradiance of 80 µmol m-2 s-1 (light source: LED white light). To prevent diatom growth 1 mg L-1 GeO2 was added was added to each well. Seedlings were cultivated under the respective conditions for eight weeks with a weekly media refreshment and a weekly photographic documentation by the help of an inverted microscope (Olympus IX71, Tokio, Japan).
Experimental set up phase II: nursery of juveniles
After eight weeks of growth in six-well-plates under above-described conditions, the biomass from three wells of each treatment was pooled and transferred to 750 ml cultivation flasks (Sarstedt, Nümbrecht, Germany) (n = 2, a total of 36 flasks). Individuals were cautiously removed from the wells by usage of Sarstedt´s cell scrapers without damaging the single individuals and keeping rhizoidal zones intact. The applied treatments continued, and aeration was applied to keep the thalli suspended in the water column. Weekly media refreshments and growth measurements continued as described above. After two weeks, the juvenile thalli were harvested (fresh weight was not determined) and transferred into larger cultivation devices (14 L). Because the treatments without nutrient addition showed no significant growth (thalli < 500 µm), they were excluded from the further cultivation experiments (n = 2, i.e. a total of 24 aquaria). The 14 L aquaria were filled with sterile filtrated seawater and located in a 10°C chilled climate chamber. To maintain before mentioned temperature elevations, the water temperature of the respective treatments was manipulated using submersed heaters (Aquarium Heaters EHEIM, Deizisau, Germany) and adjusted to 15 (± 1.5) °C. Culture medium (PES) was added in the respective above mentioned concentrations. The seaweeds were cultivated under a 16:8 h (L:D) photo regime for two weeks with a weekly media change. After two weeks of cultivation in phase II the experiments were ended (after a total growth period of 12 weeks). The seaweeds were harvested from the aquaria and immediately frozen at -80°C and dried by lyophilization. Until further processing the samples were stored at -80°C.
Growth rate and observation of ontogenetic phase transitions
Throughout the experiment, the growth of seedlings (Phase I) and juvenile thalli (Phase II) was monitored weekly by microscopy and documented via photographs (Figure 1). The photographs were used to quantify the length and width of the thalli per treatment and replicated at ten individuals respectively. Subsequent measurements were carried out with the help of ImageJ (Schneider et al., 2012). The early development of Ulva was categorized as five different phases a seedling has to undergo to proceed from a swarmer (either gamete or zoid) until becoming a juvenile thallus: I) attached, germinating cell, II) germinating cell with an apparent germination tube, III) beginning of distal cell division IV), beginning of lateral cell division, V) juvenile thallus with clear growth by distal and lateral cell divisions (Figure 1).
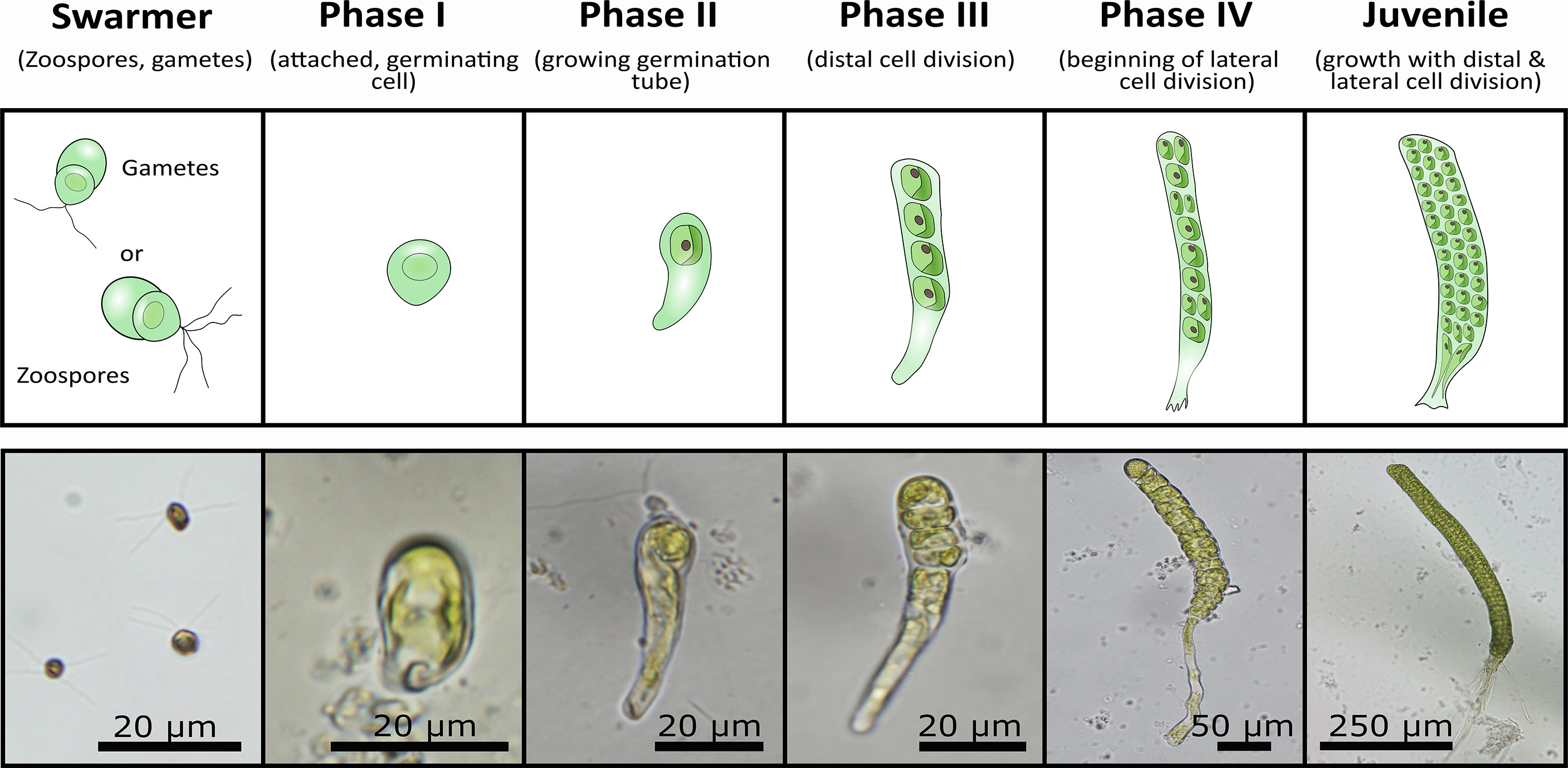
Figure 1 Overview about the four different early ontogenetic development phases from swarmer until juvenile stage in Ulva fenestrata. A schematic overview (upper row) is connected to original microscopic images (lower row), which show the single ontogenetic developmental stages that are similar in clonal (originating form gametophytes) and recombinant gametophytes (originating from sporophytes). This universal system for ontogenetic development of all juvenile life-history-phases in U. fenestrata was used to determine the development rate and success of the single gametophytic individuals.
Biochemical profiling
Fatty acid content and composition
Assessments of the fatty acid content and composition of lyophilized U. fenestrata juveniles followed a direct transesterification method which was successfully applied in previous studies on Ulva biomass (see also Toth et al., 2020; Steinhagen et al., 2021; Steinhagen et al., 2022) and was described in detail by Harrysson et al. (2018). We used C17:0 as internal standard for quantification and identification of fatty acids was done using GLC-463 Reference standards (Nu-Check Prep, Inc.). Using the MS-library, C16:1n9, C16:2n6, C16:3n3, C16:4n3, C18:4n3 and C20:4n3 were identified. The total fatty acid content was calculated by adding all measured fatty acids for each sample.
Crude protein content
Total nitrogen of the juvenile U. fenestrata biomass was determined by combustion using a LECO Trumac nitrogen analyser, EDTA Calibration Sample (LECO Cooperation) was used as standard. Thereafter, the total protein content was calculated using a nitrogen to protein conversion factor of 5 (Angell et al., 2016).
Carbohydrate content and composition
The analysis of the monosaccharide composition in juvenile biomass of U. fenestrata followed the description of Bikker et al. (2016) and has previously been successfully implemented to quantify monosaccharides in Ulva biomass (Olsson et al., 2020a; Olsson et al., 2020b). The method is based upon an initial two-step sulfuric acid hydrolysis which was performed on duplicate samples with 6 mg of lyophilized biomass. The released monosaccharides were analyzed by high-performance anion exchange chromatography. Either of Thermo ScientificTM DionexTM ICS-3000 or ICS-500 system (Dionex, Sunnyvale, CA, USA) with a pulsed amperometric detector was used with a Dionex CarbopacTM PA1 4 mm × 250 mm column and a 4 mm × 50 mm guard. The total carbohydrate content was calculated by adding the released monosaccharides for each sample.
Pigment content
Total chlorophyll a,b and carotenoids contents of U. fenestrata were determined via an acetone extraction followed by spectrophotometric assessment. Absorbance was measured on a spectrophotometer at four different wavelengths: 647 nm, 664 nm, 510 nm and 480 nm. The total contents of chlorophyll a and b as well as carotenoids were calculated using the respective equations for higher plants and green algae (Jeffrey and Humphrey, 1975; Parsons et al., 1984). The detailed process was described by Steinhagen et al. (2021).
Statistical analysis
Data on the thallus length and width and biochemical composition of juvenile U. fenestrata after 12 weeks in the hatchery were statistically analysed in JMP (JMP®, Version 15, SAS Institute Inc., Cary, NC, USA) using orthogonal 4-way analysis of variance (ANOVA, Tables 1, 2) with gametophyte type (clonal and recombinant), temperature (10 and 15°C), nutrients (N1=PES and N2=PESx3) and density (D1 = 10000, D2 = 5000, and D3 = 500 swarmers ml-1) as fixed 2- or 3-level factors. Significant differences between means were compared using Student-Newman-Keul´s (SNK) procedure (Underwood, 2004).
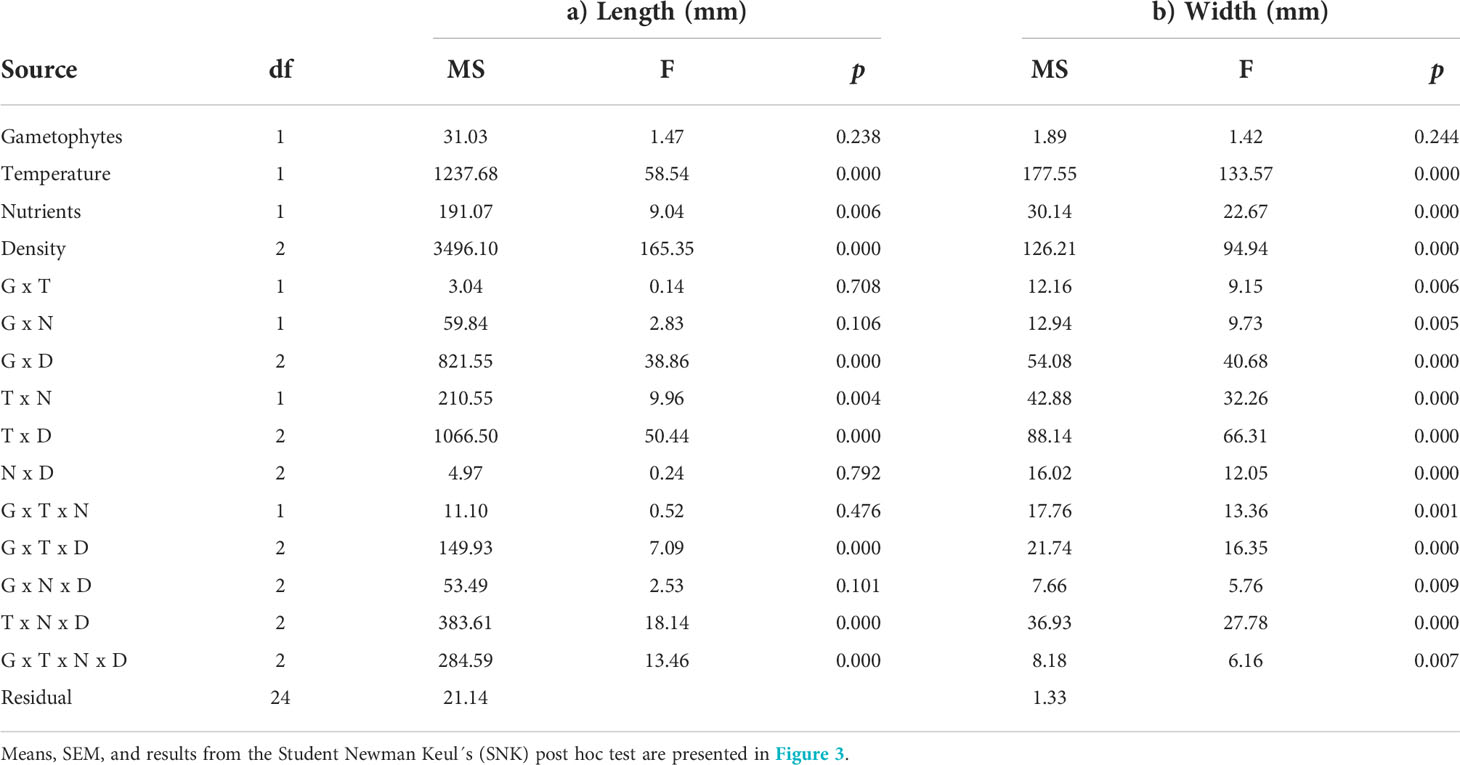
Table 1 Analysis of variance of a) length (mm), and b) width (mm) of clonal and recombinant gametophytes (G) exposed to different temperature (T), nutrient concentrations (N) and densities (D) in a 12 week hatchery experiment.
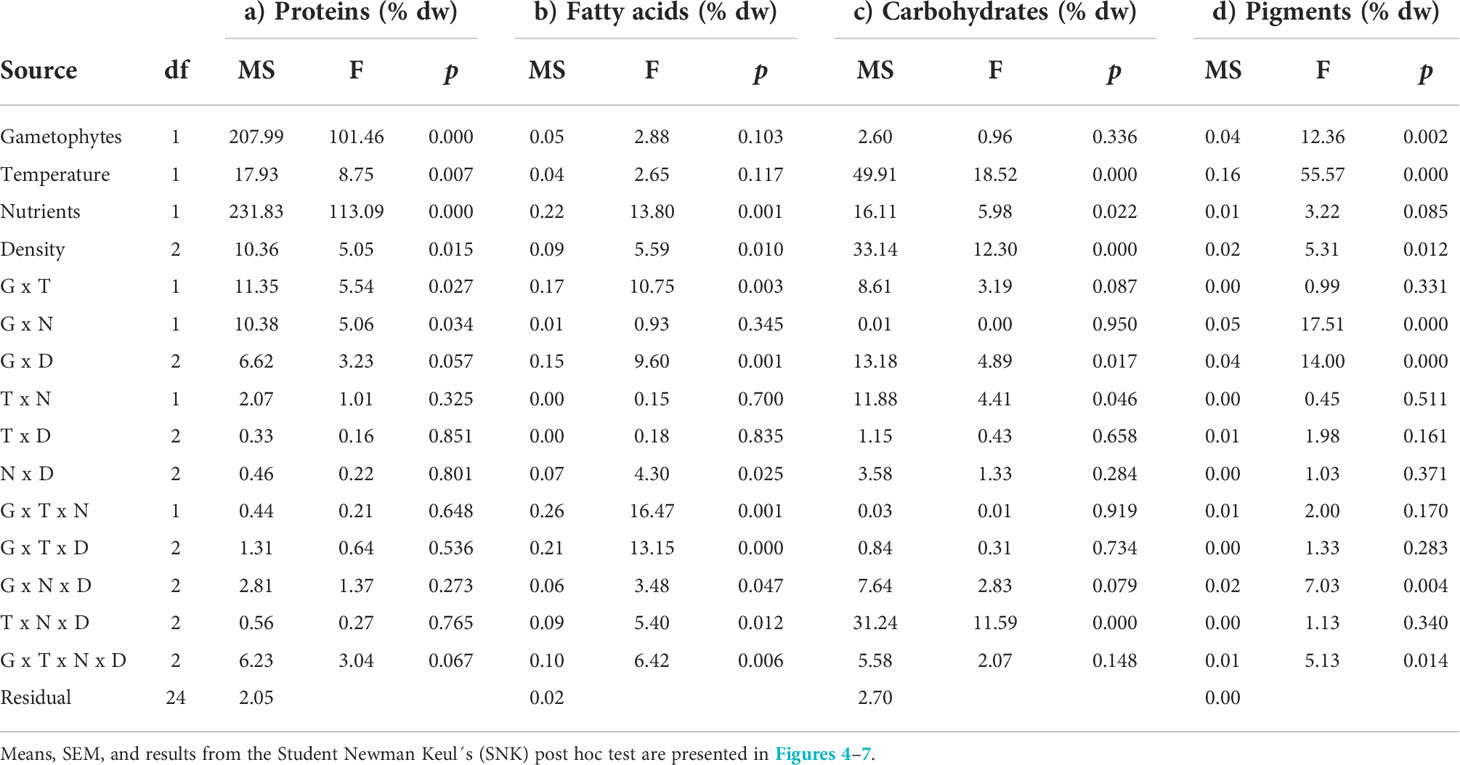
Table 2 Analysis of variance of a) total protein (% dw), b) fatty acid (% dw), c) carbohydrate (% dw), and d) pigment content in clonal and recombinant gametophytes (G) exposed to different temperature (T), nutrient concentrations (N) and densities (D) in a 12 week hatchery experiment.
Results
Ontogenetic development
The developed classification scheme, which separated the ontogenetic development of Ulva in a total of four different phases until a swarmer develops into juvenile state, was found to successfully determine the rate of ontogenetic development – equally good in both, clonal and recombinant gametophytes. Zoids and gametes showed no differences in attachment and followed similar general developmental patters (in terms of determined phase transitions, see Figures 1, 2), but notable differences in the rate of ontogenetic development among clonal and recombinant gametophytes were found. Recombinant seedlings developed faster in terms of transitioning through the ontogenetic phases and generally reached the stage of juvenile thalli earlier than clonal gametophytes (Figure 2, Supplementary Figure S1). High temperatures (15°C) and nutrient addition (PES and PESx3) favored a rapid seedling development in both clonal and recombinant gametophytes (Figure 2, Supplementary Figure S1). Notably, all seawater treatments showed a worse performance in comparison to treatments with enriched nutrients, both in recombinant and clonal gametophytes. None of the individuals treated with only seawater reached the juvenile stage after 4 weeks of hatchery (Figure 2, Supplementary Figure S1). Whereas > 80% of recombinant gametophytes reached the developmental stage of juvenile thalli in the first week at above named treatments, 100% of the individuals were recorded being at a juvenile developmental stage in the second week (Figure 2, Supplementary Figure S1). Fastest developmental patterns in clonal gametophytes were observed in treatments with high temperature and nutrient addition as well, however the first thalli reaching juvenile stage (> 80% of individuals) were only observed at week 2. At week 3, 100% of the clonal gametophytic individuals were evaluated as being juvenile in afore mentioned treatments (Figure 2, Supplementary Figure S1). Due to very limited biomass availability, seawater treatments were excluded from the second part of the experiment.
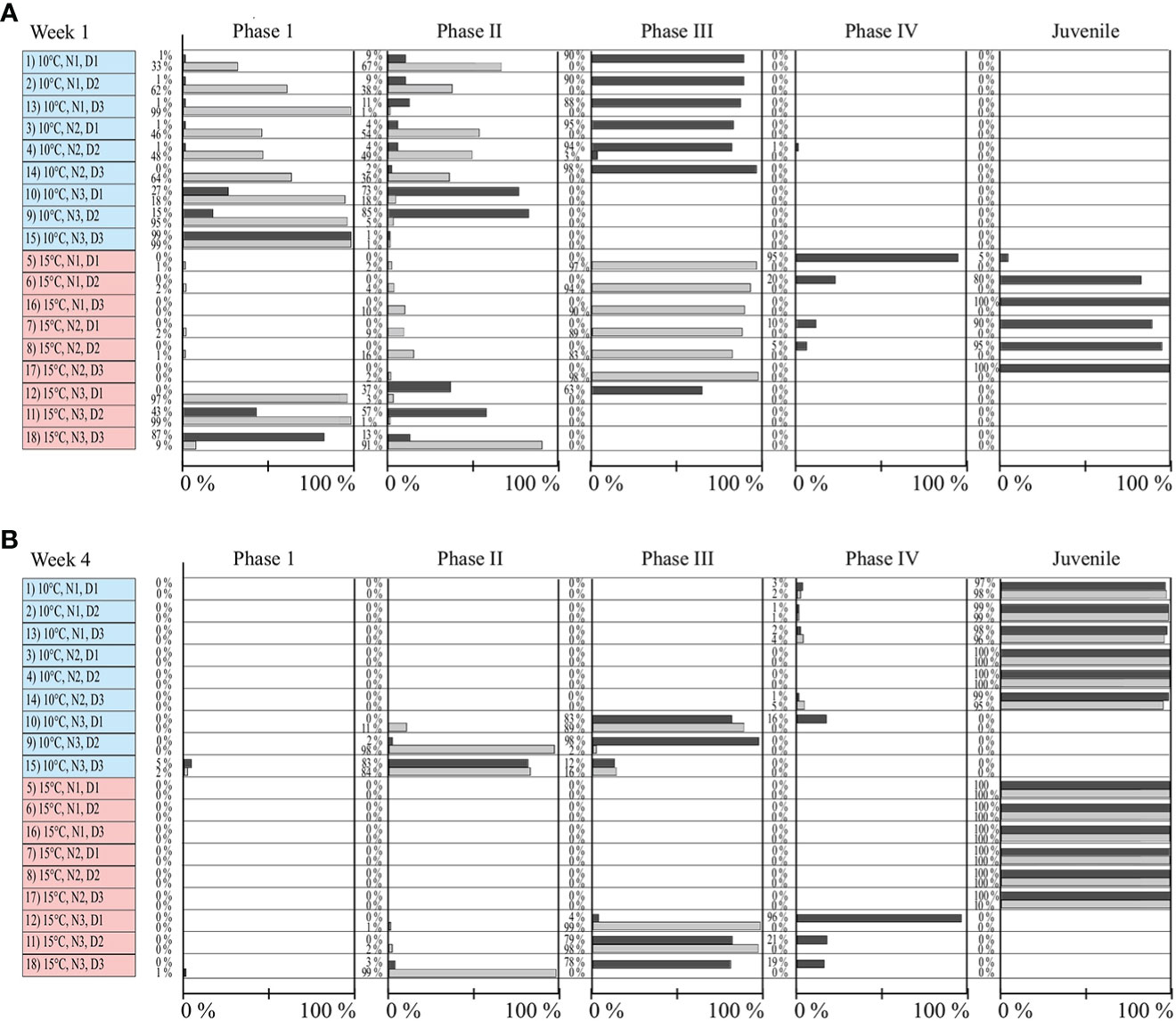
Figure 2 Development rate of early ontogenetic stages of clonal (light grey) and recombinant (dark grey) gametophyes of Ulva fenestarata under different environmental conditions (10°C and 15°C, N1 = PES, N2 = 3xPES, N3 = SW, D1 = 10 000 swarmers ml-1, D2 = 5000 swarmers ml-1 and D3 = 500 swarmers ml-1, for further explanations see text). Given is the amount (in %) of individuals in the respective ontogenetic development phase after (A) 1 week and (B) 4 weeks (for full data see also Supplementary Figure S1).
Juvenile growth
When growth at the afore mentioned hatchery conditions - measured as the length and width of the thalli - of gametophytic U. fenestrata thalli was statistically analysed after 12 weeks, we found a significant interaction among all investigated factors (Tables 1a, b). The significantly (SNK, p < 0.05) longest and broadest thalli were observed in high temperature (15°C), high nutrients (PESx3), and very low swarmer density (500 swarmers per ml) for both clonal and recombinant gametophytes (Figures 3a, b; Supplementary Figure S2). Furthermore, under these conditions recombinant gametophytes were significantly (SNK, p < 0.05) larger compared to clonal gametophytes (Figures 3a, b; Supplementary Figure S2; Supplementary Figure S2). Apart from this result, there was no clear pattern between significant differences in average length and width among juvenile gametophytes exposed to different hatchery conditions (Figures 3a, b; Supplementary Figure S2).
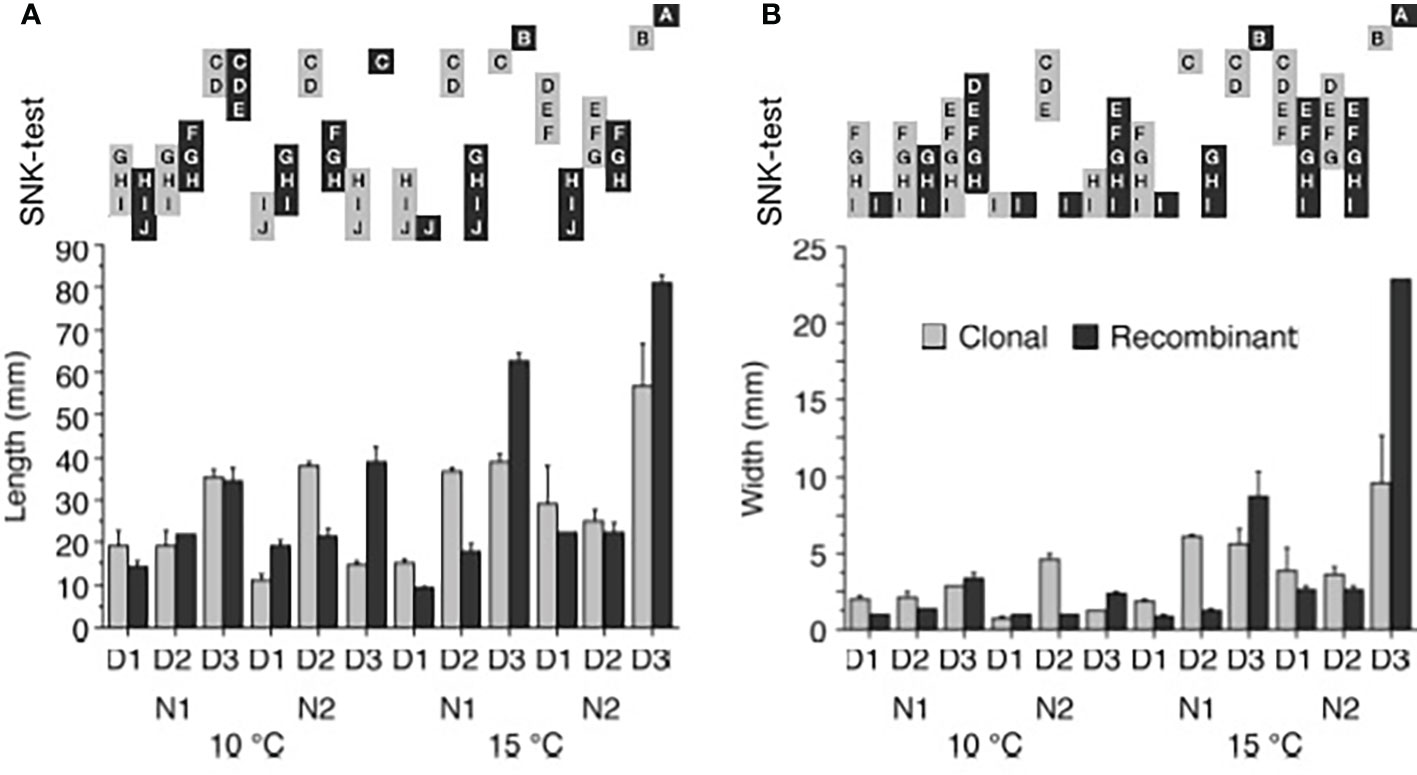
Figure 3 Ulva growth. (A) Length (mm), and (B) width (mm) of Ulva fenestrata clonal and recombinant gametophytes after 12 weeks culture under different environmental conditions (10°C and 15°C, N1 = PES and N2 = 3xPES, D1 = 10 000 swarmers ml-1, D2 = 5000 swarmers ml-1 and D3 = 500 swarmers ml-1, for further explanations see text). Letters above bars denote significant differences based on the Student Newman Keul´s (SNK) post hoc test. Treatments with non-overlapping letters are significantly different (SNK, p < 0.05). Error bars show + SEM (n = 2).
Biochemical composition
The total protein content of the juvenile U. fenestrata gametophyte thalli varied between 14.9 to 29.0% dw. There was a significant interaction between gametophyte type and temperature, as well as between gametophyte type and nutrient concentration of the cultivation medium (Table 2a; for an overview see also Supplementary Figure S2). Recombinant gametophytes had significantly (SNK, p < 0.05) higher protein content compared to clonal gametophytes, and the difference was higher when the juveniles were grown under high temperature and high nutrient conditions (Figures 4a, b). Furthermore, clonal gametophytes also had a higher protein content when grown in 3xPES compared to normal PES medium (Figure 4b). We also found a significant difference in protein content of juvenile U. fenestrata that had grown at different swarmer densities (Table 2a). Juveniles grown at the highest density had significantly (SNK, p < 0.05) lower protein content (19.7 ± 0.86% dw, means ± SEM) compared to juveniles grown at low (21.0 ± 0.99% dw, means ± SEM) and very low (21.1 ± 0.81% dw, means ± SEM) density.
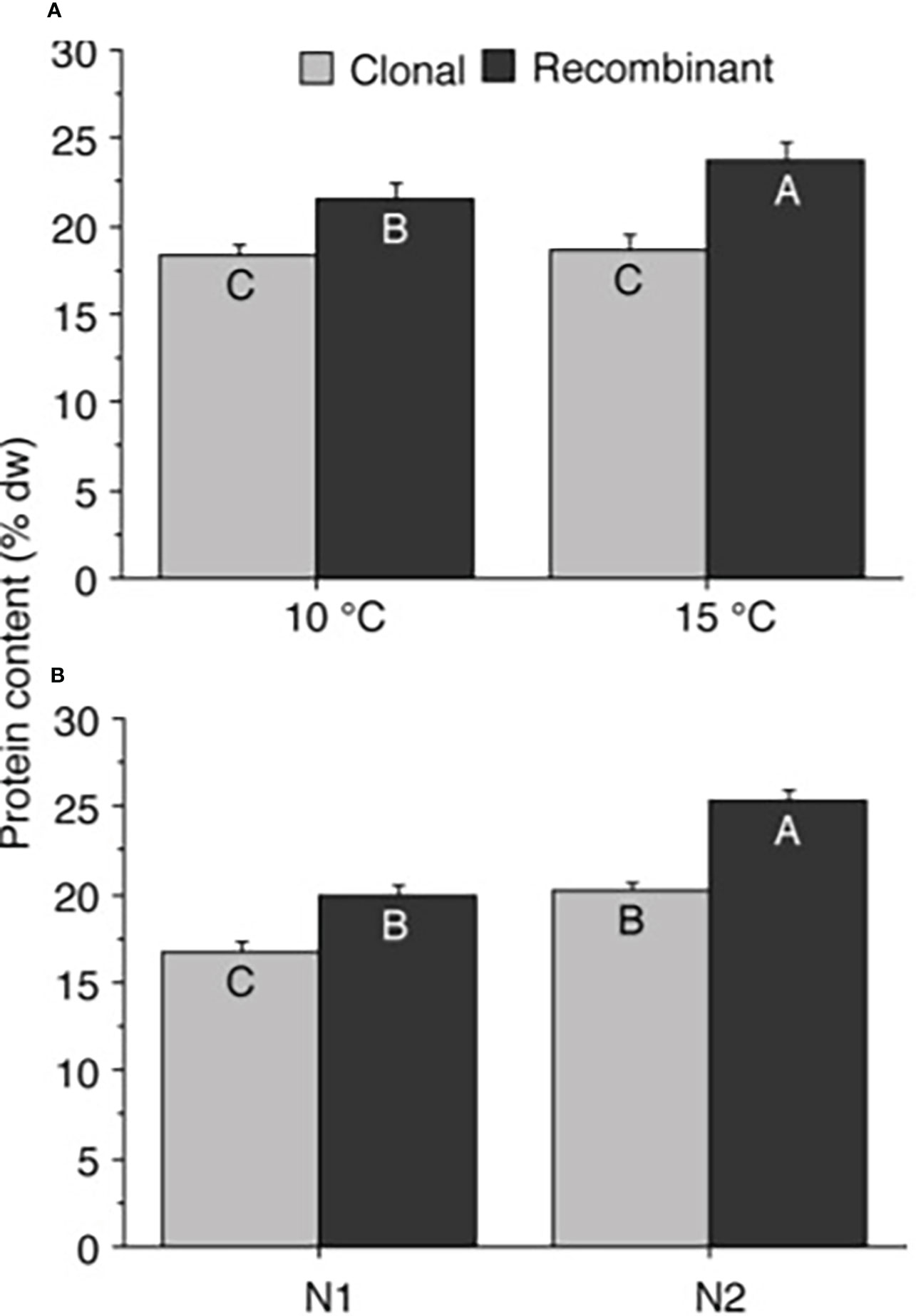
Figure 4 Ulva protein content. Protein content (% dw) in Ulva fenestrata clonal and recombinant gametophytes after 12 weeks culture under different (A) temperature (10 and 15°C) and (B) nutrient conditions (N1 = PES, N2 = 3xPES, for further explanations see text). Letters within bars denote significant differences based on the Student Newman Keul´s (SNK) post hoc test. Treatments with non-overlapping letters are significantly different (SNK, p < 0.05). Error bars show SEM (n = 12).
The total fatty acid content of the juvenile U. fenestrata gametophyte thalli varied between 1.7-2.6% dw (Figure 5a). When data were statistically analysed with ANOVA, we found a significant interaction between all investigated factors (Table 2b for an overview see also Supplementary Figure S2). Recombinant gametophytes had significantly (SNK, p < 0.05) higher total fatty acid content compared to clonal gametophytes in most treatment combinations, but otherwise no clear pattern between different hatchery conditions could be detected (Figure 5a). The fatty acids of juvenile Ulva gametophytes mostly belonged to the PUFA class (58-68% of total fatty acids), followed by SFA (20-25% of total fatty acids), and MUFA (11-19% of total fatty acids) (Figure 5b). It should be mentioned that the amount of health-beneficial long-chain n-3 polyunsaturated fatty acids (LC n-3 PUFA), including docosapentaenoic acid (C22:5n3) and eicosapentaenoic acid (C20:5n3), was significantly higher in recombinant gametophytes compared to clonal material.
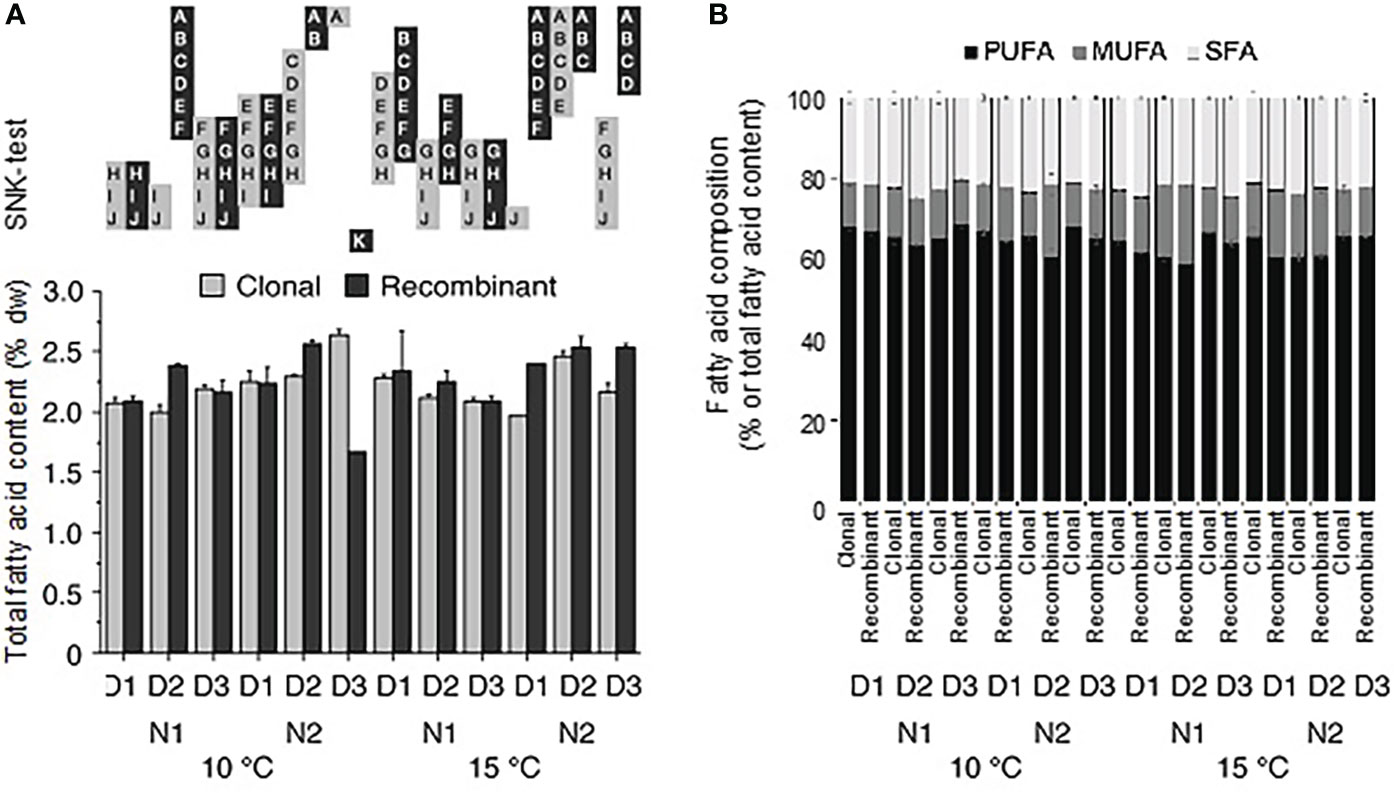
Figure 5 Ulva fatty acid content and composition. (A) Total fatty acid content (% dw) and (B) the distribution of different fatty acid classes (PUFA, MUFA, and SFA) in Ulva fenestrata clonal and recombinant gametophytes after 12 weeks culture under different environmental conditions (10°C and 15°C, N1 = PES and N2 = 3xPES, D1 = 10 000 swarmers ml-1, D2 = 5000 swarmers ml-1 and D3 = 500 swarmers ml-1, for further explanations see text). Letters above bars denote significant differences based on the Student Newman Keul´s (SNK) post hoc test. Treatments with non-overlapping letters are significantly different (SNK, p < 0.05). Error bars show + SEM (n = 2).
The total carbohydrate content of the juvenile U. fenestrata gametophyte thalli exposed to different hatchery conditions varied between 18.2-28.2% dw. There was a significant difference (SNK, p < 0.05) between clonal and recombinant gametophytes cultured under different density conditions (Table 2c; for an overview see also Supplementary Figure S2). The total carbohydrate content of clonal gametophytes decreased with decreasing density, while the carbohydrate content of recombinant gametophytes did not change significantly (Figure 6a). Furthermore, there was a significant interaction between the factor temperature, nutrients, and density (Table 2c). In general, the total carbohydrate content in Ulva fenestrata juveniles decreased with increasing temperature and density, especially under high nutrient conditions (Figure 6b). Glucose was the monosaccharide present in highest amounts (32-57% of total carbohydrates) in all treatment combinations, followed by rhamnose (18-31% of total carbohydrates) (Figure 6c). Iduronic acid (8-14% of total carbohydrates), xylose (7-15% of total carbohydrates) and glucuronic acid (7-13% of total carbohydrates) were present in similar amounts, and galactose was the monosaccharide with lowest percentage (2-3% of total carbohydrates) in the juvenile U. fenestrata (Figure 6c).
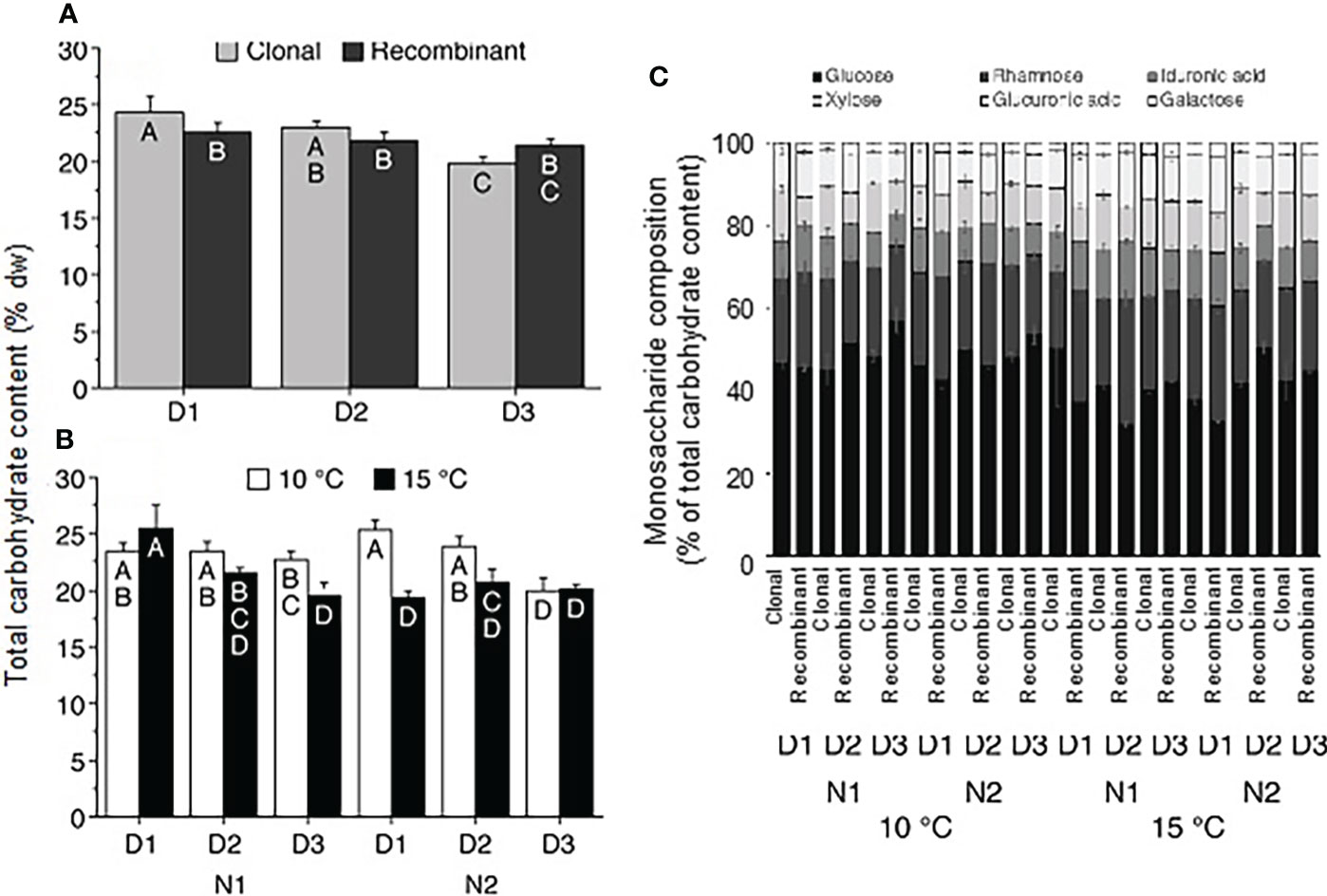
Figure 6 Ulva carbohydrate content and composition. Total carbohydrate content (% dw) in Ulva fenestrata (A) clonal and recombinant gametophytes after 12 weeks culture under different densities (D1 = 10 000 swarmers ml-1, D2 = 5000 swarmers ml-1 and D3 = 500 swarmers ml-1, for further explanations see text) and (B) under different temperature (10 and 15°C) and nutrient conditions (N1 = PES, N2 = 3xPES, for further explanations see text). Letters within bars denote significant differences based on the Student Newman Keul´s (SNK) post hoc test. Error bars show SEM (n = 8 in a and n = 4 in b). (C) Distribution of different carbohydrate classes in U. fenestrata clonal and recombinantrecombinand gametophytes exposed to the above conditions. Error bars show SEM (n = 2).
The total pigment content of juvenile U. fenestrata gametophytes varied between 0.13-0.58%. When data were statistically analysed, we found significant interactions for all tested factors (Table 2d, Supplementary Table S1). Although no clear pattern among differences between different factors could be identified when means were compared in the SNK-test, temperature tended to have a positive effect on the pigment content in U. fenestrata juveniles (Figure 7a). Furthermore, recombinant gametophytes tended to have higher Chl a content, especially under high nutrient and density conditions (Figure 7b), while clonal gametophytes had high Chl b content especially under high temperature and low nutrient conditions (Figure 7b). The highest pigment content was found in recombinant gametophytes grown in high temperature, high nutrient-, and low-density conditions (Figure 7a).
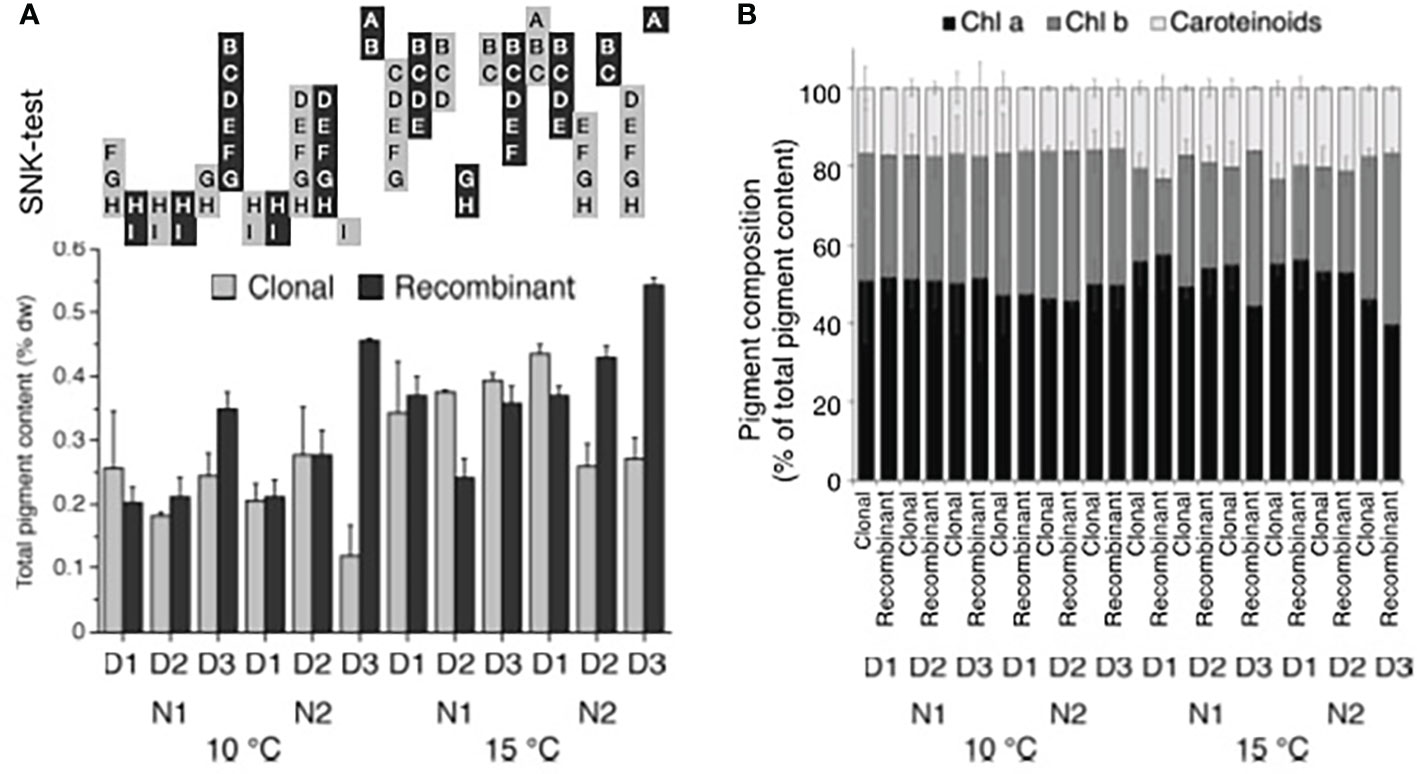
Figure 7 Ulva pigment content and composition. (A) Total pigment content (% dw) and (B) the distribution of chl a, chl b, and carotenoids in Ulva fenestrata clonal and recombinant gametophytes after 12 weeks culture under different environmental conditions (10°C and 15°C, N1 = PES and N2 = 3xPES, D1 = 10 000 swarmers ml-1, D2 = 5000 swarmers ml-1 and D3 = 500 swarmers ml-1, for further explanations see text). Letters above bars denote significant differences based on the Student Newman Keul´s (SNK) post hoc test. Treatments with non-overlapping letters are significantly different (SNK, p < 0.05). Error bars show + SEM (n = 2).
Discussion
Our results demonstrate that the prevailing life-history-phase strongly defines the ontogenetic development rate, thallus growth, and biochemical composition of individuals of the crop Ulva fenestrata, which are all crucial factors for a successful closed life-cycle seaweed aquaculture. We support this conclusion with three main lines of evidence including (1) increased rate of ontogenetic development in recombinant gametophytes, (2) the growth of recombinant gametophytes significantly increased under certain cultivation conditions compared to clonal gametophytes, and (3) significant increases in certain biochemicals were favored by chosen cultivation conditions and differed between clonal and recombinant gametophytes. These novel results strongly emphasize the importance of recognizing the life-history-phases in Ulva aquaculture to facilitate a closed life-cycle-aquaculture, optimize biomass production schedules, and adapt cultivation parameters for optimal yield and desired biochemical profiles of the biomass.
Ontogenetic development and juvenile growth
Our study shows that seedlings derived from different life-history-forms (gametophytes or sporophytes) of U. fenestrata – here clonal and recombinant gametophytes – show considerable differences in their ontogenetic development. It was generally evident that the rate of ontogenetic development, measured by the time an individual needed to transition from a swarmer to juvenile phase, was faster in recombinant compared to clonal gametophytes. These results were confirmed under varying cultivation conditions, and although the general effects of the respective factors on the seedlings were similar, we can show that under most of the tested abiotic factors, recombinant gametophytes performed better.
Crucial for a sustainable seaweed aquaculture is the production of biomass from seedlings to both, protect natural seaweed stands and being independent of external factors, such as biomass availability, weather events, or pests (Titlyanov and Titlyanova, 2010; Steinhagen et al., 2021). Therefore, the early ontogenetic development forms a corner stone in cultivated crop strains, e.g. of Ulva fenestrata. Most aquaculture studies on Ulva however use opportunistically sampled source material (Fort et al., 2019) and therefore studies on the hatchery of Ulva are scarce and limited to lab-strains (Stratmann et al., 1996; Wichard and Oertel, 2010). Our study demonstrates that U. fenestrata can be easily cultivated and permanently propagated under laboratory conditions.
The here described classification system to determine the status of early ontogenetic development in Ulva has proven applicable among seedlings of all life-history-stages (development data of sporophytes not shown) of U. fenestrata. Since the early development is relatively stable within the genus Ulva (Carter, 1926; Løvlie and Bryhni, 1978; Wichard et al., 2015) the displayed system could not only constitute a basis for the comparison of the ontogenetic development of different life-history-forms, but also to assess differences among different Ulva species, strains, and cultivars.
That e.g. temperature, nutrient availability, and seedling density are important factors to consider in Ulva hatcheries has been demonstrated previously (Carl et al., 2014; Steinhagen et al., 2021) and was also found to be important for the ontogenetic development of U. fenestrata in the present study. Our study shows that fastest developmental rates of both seedling types were recorded at high temperatures and elevated nutrient conditions which agreed with previous studies on the same species (Steinhagen et al., 2021). Our results show furthermore that an artificial nutrient source is beneficial during the hatchery phase of U. fenestrata and that a weekly water change alone is not sufficient for large-scale seedling development.
Similarly, as observed for the early ontogenetic development, the optimal growth conditions for juveniles were found to be at high temperature, high nutrients, and additionally included very low swarmer density for both clonal and recombinant gametophytes. Although, both seedling types performed best at afore mentioned conditions, recombinant gametophytes were significantly larger than clonal individuals.
That seeding density is a key factor in Ulva aquaculture and related to a steady growth rate and sufficient biomass production has been demonstrated before (Carl et al., 2014; Steinhagen et al., 2021). Whereas a high seedling density was favorable in off-shore cultivation of Ulva (Carl et al., 2014; Steinhagen et al., 2021), seedlings of the recent lab-based study performed best at lower densities. As suggested for other seaweed species (Steen and Scrosati, 2004), this might be due to intraspecific competition for nutrients and shading effects of densely growing seedlings in laboratory settings, whereas these factors could be excluded in off-shore settings (Carl et al., 2014; Steinhagen et al., 2021). Therefore, optimal cultivation conditions during Ulva hatcheries are strongly depending on the later applied cultivation methodology.
Although, our study shows a substantially faster development and growth of recombinant gametophytes, it should be recognized that from an aquaculture perspective a clonal gametophytic strain, which makes use of the parthogenetic nature of unmated gametes, is generally easier to handle as it does not afford mating partners for propagation.
Biochemical composition
The necessity of the provision of renewable and novel materials and nutritious food sources – especially vegetarian and vegan protein – was emphasized by the United Nations Sustainable Development Goals (SDGs) (UN General Assembly, 2015). Ulva biomass has recently gained attention in several economic sectors due to its multipurpose use in commodity products which is favored by their different high-value biochemical compounds (Holdt and Kraan, 2011; Toth et al., 2020; Olsson et al., 2020b). It has been shown by several previous studies that varying abiotic factors in cultivation settings influence the biochemical content of adult Ulva biomass (Holdt and Kraan, 2011; Toth et al., 2020; Olsson et al., 2020b; Steinhagen et al., 2022), but knowledge on the effect on early life-history-stages is until date lacking. The protein content (14.9-29% dw) measured in the juvenile biomass of U. fenestrata was in an average range compared with previous studies on Ulva spp. (4–44% dw) (Holdt and Kraan, 2011; Toth et al., 2020; Steinhagen et al., 2021). Our study however revealed that recombinant gametophytes had a significantly higher protein content in comparison to clonal gametophytes, which was most striking under high temperature and high nutrient conditions. The consumption of alternative protein sources is forecasted to grow by an annual rate of 9% until 2054 (Probst et al., 2015). Therefore, there is a growing need for new protein rich crop strains - and likewise protein enriched life-history-stages of crop species could play an important role in pursuing the ongoing dietary protein shift. Unlike terrestrial crops, seaweeds do not compete for arable soil or freshwater supply and are considered a future oriented food source (Holdt and Kraan, 2011; FAO et al., 2018). That the market share of micro- and macroalgae is expected to increase by 16% between 2024 and 2054 (Probst et al., 2015) underlines not only the necessity of suitable seaweed protein extraction methodologies (Holdt and Kraan, 2011; Trigo et al., 2021; Juul et al., 2021) but also supports the need of the optimization of protein yields of crop strains such as U. fenestrata which also includes considering life-history-traits.
The total fatty acid content (1.7-2.6% dw) of juvenile U. fenestrata was higher in our study when compared to other Ulva spp. (ca. 1.6% dw, (Holdt and Kraan, 2011)), but lower than compared to offshore cultivated biomass of the same species used in the present study (3.2-3.55% dw, (Steinhagen et al., 2021)). The fatty acid content of recombinant gametophytes was found to be significantly higher than compared with that of clonal gametophytes. The consumers trend for healthy, nutritious foods with health-promoting functions is driving a decent part of the food industry to produce functional foods (Chan and Matanjun, 2017). Their valuable physiochemical composition (Ortiz et al., 2006; Holdt and Kraan, 2011) and relatively high amount of PUFAs makes Ulva biomass very attractive from an economical perspective. Especially the presences of health-beneficial LC n-3 PUFA in both, clonal and recombinant gametophytes, makes the biomass interesting for the functional food market. However, as detected for the general PUFA amount also the amount of docosapentaenoic acid and eicosapentaenoic acid was distinctively higher in recombinant gametophytes, which once more points out that also life-history-traits should be considered in order to maximize e.g. the content of fatty acids in the biomass.
The total amount of carbohydrates (18.2-28.2%) of juvenile U. fenestrata gametophytes was in a similar range as observed previously for U. fenestrata (25.95–29.69% dw, Steinhagen et al., 2021) and in the middle to lower range when compared to other Ulva spp. (Holdt and Kraan, 2011; Olsson et al., 2020b). We observed significant different effects of culture conditions on the carbohydrate content of clonal and recombinant gametophytes. Whereas the carbohydrate content of recombinant gametophytes did not vary significantly at decreasing densities, the decreasing carbohydrate content of clonal gametophytes correlated with decreasing density. Furthermore, contradictory to previous studies, which emphasized an increase in carbohydrates at elevated temperature (Holdt and Kraan, 2011; Olsson et al., 2020a; Olsson et al., 2020b; Steinhagen et al., 2022), the total carbohydrate content in U. fenestrata juveniles decreased with increasing temperature and density, especially under high nutrient conditions. However, the percentage of different monosaccharides was similar in both, clonal and recombinant gametophytes at all cultivation conditions, with glucose having the highest amounts, followed by rhamnose, whereas iduronic acid, xylose, and glucuronic acid were recorded in similar amounts. Besides dietary fibers, which are not taken up by the human body but improve the intestinal environment (Holdt and Kraan, 2011), carbohydrates extracted from Ulva biomass have a market application in innovative materials (Wahlström et al., 2020a; Wahlström et al., 2020b) or in the pharmaceutical sector (Holdt and Kraan, 2011; Olsson et al., 2020a; Olsson et al., 2020b). It has been shown in previous studies that hatchery conditions affect the biochemical composition of off-shore cultivated U. fenestrata biomass (Steinhagen et al., 2021) and we can here conclude that both, applied hatchery conditions as well as the life-history-stage of seedlings play an important role in the carbohydrate content of juvenile U. fenestrata biomass.
Pigments such as chlorophyll a,b and carotenoids are phytochemicals which add important value to Ulva biomass by their scavenge activity of malign free radicals which are known to cause oxidative stress (Abd El-Baky et al., 2009). They have been described to significantly benefiting human health by their antioxidant, anti-inflammatory and anti-tumoral activities (Egner et al., 2003; Lanfer-Marquez et al., 2005; Eismann et al., 2020) and are therefore considered positive ingredients in functional foods and nutraceuticals (Holdt and Kraan, 2011). Thus, the pigment profile of U. fenestrata is highly interesting for several economic markets. The pigment content measured in the present study was in the average range of what was previously reported for the same species (Toth et al., 2020; Steinhagen et al., 2021) and in the upper range compared to other Ulva species (Holdt and Kraan, 2011).
Although, no clear patterns among the different applied factors could be identified, temperature tended to have a positive effect on the pigment content in both, clonal and recombinant gametophytes of U. fenestrata. Similar to our recent study, a positive temperature effect on the pigment content of Ulva was emphasized by Eismann et al. (2020) under laboratory conditions. However, a study on U. fenestrata cultivated at offshore conditions revealed a negative effect of higher temperatures applied during hatchery conditions on the pigment content (Steinhagen et al., 2021). Thus, it depends on the later cultivation method used for biomass production which hatchery conditions are favourable for high pigment yields.
Although, the main focus of the current work was the performance assessment of clonal and recombinant gametophytes for the aquaculture industry it should be mentioned, that our findings probably have implications for natural populations as well. In general, the better performance of recombinant gametophytes could be seen as an evolutionary advantage, as recombination and therefore higher genetic variation is generally regarded as more advantageous in comparison to clonal and genetic poorer gene pools (literature). This hypothesis should be however evaluated under natural conditions and requires e.g. the development of life-history-stage specific molecular markers.
Conclusions
To conclude, in most of the investigated parameters did clonal and recombinant gametophytes of the crop U. fenestrata perform significantly different. This study yields important information about the differences of their ontogenetic development, thallus growth, and biochemical composition. Generally, recombinant gametophytes stood out with a faster ontogenetic development, faster growth rates, and higher amounts of commercially attractive biochemical compounds (e.g. proteins and fatty acids). These novel results could not only support the growing Blue Economy but also contribute an easily replicable development assessment system to select best performing source material for attractive commercial seaweed aquaculture, thereby supporting a shift towards a sustainable, closed life-cycle seaweed aquaculture system.
Data availability statement
The original contributions presented in the study are included in the article/Supplementary Material. Further inquiries can be directed to the corresponding author.
Author contributions
SST: conceptualization of the study, implementation of experiment, investigation, data analyses, visualization, and original draft; KL: data analyses and refining of draft; JO: data analyses and refining of draft; EA: data analyses and refining of draft; IU: funding acquisition, data analyses, and refining of draft; HP: funding acquisition and refining of draft; GT: funding acquisition and data analyses, original draft. All authors contributed to the article and approved the submitted version.
Acknowledgments
The authors thank the Swedish Foundation for Strategic Research (SSF), project number RBP14-0045, as well as the Formas-funded projects ‘CirkAlg’, project number 2018-01839, and BlueGreen, project number 2021-02340, for financial support. We want to thank Samanta Hoffmann for her valuable comments on this study and provision of graphic support. Further, we thank Annelous Oerbekke for technical support during seedling hatcheries.
Conflict of interest
The authors declare that the research was conducted in the absence of any commercial or financial relationships that could be construed as a potential conflict of interest.
Publisher’s note
All claims expressed in this article are solely those of the authors and do not necessarily represent those of their affiliated organizations, or those of the publisher, the editors and the reviewers. Any product that may be evaluated in this article, or claim that may be made by its manufacturer, is not guaranteed or endorsed by the publisher.
Supplementary material
The Supplementary Material for this article can be found online at: https://www.frontiersin.org/articles/10.3389/fmars.2022.942679/full#supplementary-material
Supplementary Figure 1 | Development rate of early ontogenetic stages of clonal (dark grey) and recombinant (light grey) gametophyes of Ulva fenestarata under different environmental conditions (10°C and 15°C, N1 = PES, N2 = 3xPES, N3 = SW, D1 = 10 000 swarmers ml-1, D2 = 5000 swarmers ml-1 and D3 = 500 swarmers ml-1, for further explanations see text). Given is the amount (in %) of individuals in the respective ontogenetic development phase after 1, 2, 3, and 4 weeks.
Supplementary Figure 2 | Mean thallus length (mm), width (mm), total fatty acid (% dw), protein (% dw), and carbohydrate (% dw) content in recombinant and clonal Ulva fenestrata individuals after exposure to different environmental conditions (10°C and 15°C, N1 = PES, N2 = 3xPES, N3 = SW, D1 = 10 000 swarmers ml-1, D2 = 5000 swarmers ml-1 and D3 = 500 swarmers ml-1, for further explanations see text). Error bars show SEM (n = 2).
References
Abd El-Baky H. H., El-Baz F. K., El-Baroty G. S. (2009). Natural preservative ingredient from marine alga Ulva lactuca l. Int. J. Food Sci. Technol. 44, 1688–1695. doi: 10.1111/j.1365-2621.2009.01926.x
Abomohra A.--., El-Naggar A. H., Baeshen A. A. (2018). Potential of macroalgae for biodiesel production: screening and evaluation studies. J. Biosci. Bioeng. 125, 231–237. doi: 10.1016/j.jbiosc.2017.08.020
Abreu M. H., Peeira R., Sassi J. F. (2014). “Marine algae and the global food industry,” in Marine algae, biodiversity, taxonomy, environmental assessment, and biotechnology. Eds. Pereira L., Magalhaes J. (Boca Raton, FL: CRC Press), 300–319.
Al-Hafedh Y. S., Alam A., Buschmann A. H. (2014). Bioremediation potential, growth and biomass yield of the green seaweed, Ulva lactuca in an integrated marine aquaculture system at the red Sea coast of Saudi Arabia at different stocking densities and effluent flow rates. Rev. Aquac. 7, 161–171. doi: 10.1111/raq.12060
Amosu A. O., Robertson-Andersson D. V., Kean E., Maneveldt G. W., Cyster L. (2016). Biofiltering and uptake of dissolved nutrients by Ulva armoricana (Chlorophyta) in a land-based aquaculture system. Int. J. Agric. Biol. 18 (2), 298–304. doi: 10.17957/IJAB/15.0086
Angell A. R., Mata L., de Nys R., Paul N. A. (2016). The protein content of seaweeds: a universal nitrogen-to-protein conversion factor of five. J. Appl. Phycol. 28, 511–524. doi: 10.1007/s10811-015-0650-1
Barzkar N., Tamadoni Jahromi S. T., Poorsaheli H. B., Vianello F. (2019). Metabolites from marine microorganisms, micro, and macroalgae: immense scope for pharmacology. Mar. Drugs 17, 464. doi: 10.3390/md17080464
Bikker P., van Krimpen M. M., van Wikselaar P., Houweling-Tan B., Scaccia N., van Hal J. W., et al. (2016). Biorefinery of the green seaweed Ulva lactuca to produce animal feed, chemicals and biofuels. J. Appl. Phycol. 28, 3511–3525. doi: 10.1007/s10811-016-0842-3
Brakel J., Sibonga R. C., Dumilag R. V., Montalescot V., Campbell J., Cottier-Cook E. J., et al. (2021). Exploring, harnessing and conserving marine genetic resources towards a sustainable seaweed aquaculture. Plants People Planet 3 (4), 337–349. doi: 10.1002/ppp3.10190
Bråten T. (1971). The ultrastructure of fertilization and zygote formation in the green alga Ulva mutabilis føyn. J. Cell Sci. 9 (3), 621–635. doi: 10.1242/jcs.9.3.621
Califano G., Kwantes M., Abreu M. H., Costa R., Wichard T. (2020). Cultivating the macroalgal holobiont: Effects of integrated multi-trophic aquaculture on the microbiome of Ulva rigida (Chlorophyta). Front. Mar. Sci. 7, 52. doi: 10.3389/fmars.2020.00052
Carl C., de Nys R., Lawton R. J., Paul N. A. (2014). Methods for the induction of reproduction in a tropical species of filamentous Ulva. PloS One 9 (5), e97396. doi: 10.1371/journal.pone.0097396
Carter N. (1926). An investigation into the cytology and biology of the ulvaceae. Ann. Bot. 40 (159), 665–689. doi: 10.1093/oxfordjournals.aob.a090043
Chan P. T., Matanjun P. (2017). Chemical composition and physicochemical properties of tropical seaweed, Gracilaria changii. Food Chem. 221, 302–310. doi: 10.1016/j.foodchem.2016.10.066
Chen H., Zhou D., Luo G., Zhang S., Chen J. (2015). Macroalgae for biofuels production: progress and perspectives. Renewable Sustain. Energy Rev. 47, 427–437. doi: 10.1016/j.rser.2015.03.086
Coelho M. S., Barbosa F. G., de Souza M. D. A. Z. (2014). The scientometric research on macroalgal biomass as a source of biofuel feedstock. Algal Res. 6, 132–138. doi: 10.1016/j.algal.2014.11.001
Colombo M. L., Risè P., Giavarini F., Angelis D. E. L., Galli C., Bolis C. L. (2006). Marine macroalgae as sources of polyunsaturated fatty acids. Plant Foods Hum. Nutr. 61, 67–72. doi: 10.1007/s11130-006-0015-7
Egner P. A., Muñoz A., Kensler T. W. (2003). Chemoprevention with chlorophyllin in individuals exposed to dietary aflatoxin. Mutat. Research/Fundam. Mol. Mech. Mutagenesis 523, 209–216. doi: 10.1016/S0027-5107(02)00337-8
Eismann A. I., Reis R. P., da Silva A. F., Cavalcanti D. N. (2020). Ulva spp. carotenoids: Responses to environmental conditions. Algal Res. 48, 101916. doi: 10.1016/j.algal.2020.101916
FAO, IFAD, UNICEF, WFP, WHO (2016). “The state of food and aquaculture 2016,” in Climate change, agriculture and food security (Rome: FAO).
FAO, IFAD, UNICEF, WFP, WHO (2018). “The state of food security and nutrition in the world 2018,” in Building climate resilience for food security and nutrition (Rome: FAO).
Fort A., Lebrault M., Allaire M., Esteves-Ferreira A. A., McHale M., Lopez F., et al. (2019). Extensive variations in diurnal growth patterns and metabolism among ulva spp. strains. Plant Physiol. 180 (1), 109–123. doi: 10.1104/pp.18.01513
Gao G., Clare A. S., Chatzidimitriou E., Rose C., Caldwell G. (2018a). Effects of ocean warming and acidification, combined with nutrient enrichment, on chemical composition and functional properties of Ulva rigida. Food Chem. 258, 71–78. doi: 10.1016/j.foodchem.2018.03.040
Gao G., Clare A. S., Rose C., Caldwell G. S. (2018b). Ulva rigida in the future ocean: potential for carbon capture, bioremediation and biomethane production. GBC Bioenergy 10, 39–51. doi: 10.1111/gcbb.12465
Hafting J. T., Craigie J. S., Stengel D. B., Loureiro R. R., Buschmann A. H., Yarish C., et al. (2015). Prospects and challenges for industrial production of seaweed bioactivities. J. Phycol. 51, 821–837. doi: 10.1111/jpy.12326
Harrysson H., Hayes M., Eimer F., Carlsson N.-G., Toth G. B., Undeland I. (2018). Production of protein extracts from Swedish red, green, and brown seaweeds, Porphyra umbilicalis kützing, Ulva lactuca Linnaeus, and Saccharina latissima (Linnaeus) j. v. lamouroux using three different methods. J. Appl. Phycol. 30, 3565–3580. doi: 10.1007/s10811-018-1481-7
Hiraoka M., Obata S., Ohno M. (1998). Pigment content of the reproductive cells of Ulva pertusa (Ulvales, ulvophyceae): evidence of anisogamy. Phycologia 37 (3), 222–226. doi: 10.2216/i0031-8884-37-3-222.1
Holdt S. L., Kraan S. (2011). Bioactive compounds in seaweed: functional food applications and legislation. J. Appl. Phycol. 23 (2011), 543–597. doi: 10.1007/s10811-010-9632-5
Hughey J. R., Maggs C. A., Mineur F., Jarvis C., Miller K. A., Hamdy Shabaka S., et al. (2019). Genetic analysis of the linnaean Ulva lactuca (Ulvales, chlorophyta) holotype and related type specimens reveals name misapplications, unexpected origins, and new synonymies. J. Phycol. 55, 503–508. doi: 10.1111/jpy.12860
Jeffrey S., Humphrey G. (1975). New spectrophotometric equations for determining a, b, c1 and c2 in higher plants, algae and natural phytoplankton. Biochemie und Physiol. der Pflanzen 167, 191–194. doi: 10.1016/S0015-3796(17)30778-3
Juul L., Danielsen M., Nebel C., Steinhagen S., Bruhn A., Jensen S. K., et al. (2021). Ulva fenestrata protein–comparison of three extraction methods with respect to protein yield and protein quality. Algal Res. 60, 102496. doi: 10.1016/j.algal.2021.102496
Kagami Y., Mogi Y., Arai T., Yamamoto M., Kuwano K., Kawano S. (2008). Sexuality and uniparental inheritance of chloroplast DNA in the isogamous green alga Ulva compressa (Ulvophyceae). J. Phycol. 44 (3), 691–702. doi: 10.1111/j.1529-8817.2008.00527.x
Løvlie A., Bryhni E. (1978). On the relation between sexual and parthenogenetic reproduction in haplo-diplontic algae. Botanica Marina 21 (3), 155–164. doi: 10.1515/botm.1978.21.3.155
Lanfer-Marquez U. M., Barros R. M., Sinnecker P. (2005). Antioxidant activity of chlorophylls and their derivatives. Food Res. Int. 38 (8-9), 885–891. doi: 10.1016/j.foodres.2005.02.012
Lawton R. J., Mata L., de Nys R., Paul N. A. (2013). Algal bioremediation of waste waters from land-based aquaculture using Ulva: Selecting target species and strains. PloS One 15 (3). doi: 10.1371/journal.pone.0077344
Mac Monagail M., Cornish L., Morrison L., Araújo R., Critchley A. T. (2017). Sustainable harvesting of wild seaweed resources. Eur. J. Phycol. 52 (4), 371–390. doi: 10.1080/09670262.2017.1365273
Mata L., Schuenhoff A., Santos R. A. (2010). A direct comparison of the performance of the seaweed biofilters, Asparagopsis armata and Ulva rigida. J. Appl. Phycol. 22, 639–644. doi: 10.1007/s10811-010-9504-z
Mata L., Magnusson M., Paul N. A., de Nys R. (2016). The intensive land-based production of the green seaweeds Derbesia tenuissima and Ulva ohnoi: biomass and bioproducts. J. Appl. Phycol. 28(1), 365–375.
Melkonian M. (1980). Flagellar roots, mating structure and gametic fusion in the green alga Ulva lactuca (Ulvales). J. Cell Sci. 46 (1), 149–169. doi: 10.1242/jcs.46.1.149
Naylor R. L., Hardy R. W., Buschmann A. H., Bush S. R., Cao L., Klinger D. H., et al. (2021). A 20-year retrospective review of global aquaculture. Nature 591, 551–563. doi: 10.1038/s41586-021-03308-6
Olsson J., Raikova S., Mayers J. J., Steinhagen S., Chuck C. J., Nylund G. M., et al. (2020a). Effects of geographical location on potentially valuable components in Ulva intestinalis sampled along the Swedish coast. Appl. Phycol. 1 (1), 80–92. doi: 10.1080/26388081.2020.1827454
Olsson J., Toth G. B., Oerbekke A., Cvijetinovic S., Wahlström N., Harrysson H., et al. (2020b). Cultivation conditions affect the monosaccharide composition in Ulva fenestrata. J. Appl. Phycol. 32, 3255–3263. doi: 10.1007/s10811-020-02138-9
Ortiz J., Romero N., Robert P., Araya J., Lopez-Hernández J., Bozzo C., et al. (2006). Dietary fiber, amino acid, fatty acid, and tocopherol contents of the edible seaweeds Ulva lactuca and Durvillaea antarctica. Food Chem. 99, 98–104. doi: 10.1016/j.foodchem.2005.07.027
Parsons T. R., Maita Y., Lalli C. M. (1984). A manual for chemical and biological methods for seawater analysis (Oxford, UK: Pergamon Press).
Paul N. A., Tseng C. K., Borowitzka M. (2012). “Seaweed and microalgae,” in Aquaculture: Farming aquatic animals and plants. Eds. Lucas J. S., Southgate P. C. (Malaysia: Blackwell Publishing), 268–284.
Probst L., Frideres L., Pedersen B., Amato F. (2015). Sustainable safe, and nutritious food: New nutrient sources (Luxembourg: Publications Office of the European Union), 11.
Provasoli L. (1968). “Media and prospects for the cultivation of marine algae,” in Cultures and collections of algae, proceedings of the US-Japan conference, hakone, Japan (Tokyo, Japan: Society of Plant Physiology), 12–15.
Santos S. A. O., Vilela C., Freire C. S. R., Abreu M. H., Rocha S. M., Silvestre A. J. D. (2015). Chlorophyta and rhodophyta macroalgae: a source of health promoting phytochemicals. Food Chem. 183, 122–128. doi: 10.1016/j.foodchem.2015.03.006
Schneider C. A., Rasband W. S., Eliceiri K. W. (2012). NIH Image to ImageJ: 25 years of image analysis. Nat. Methods 9 (7), 671–675. doi: 10.1038/nmeth.2089
Sebök S., Herppich W. B., Hanelt D. (2019). Outdoor cultivation of Ulva lactuca in a recently developed ring-shaped photobioreactor: effects of elevated CO2 concentration on growth and photosynthetic performance. Botanica Marina 62, 179–190. doi: 10.1515/bot-2018-0016
SMHI (2022) Marina Miljöövervakningsdata (Marine environmental data). Available at: https://www.smhi.se/ (Accessed 27 June 2022).
Steen H., Scrosati R. (2004). Intraspecific competition in Fucus serratus and F. evanescens (Phaeophyceae: Fucales) germlings: effects of settlement density, nutrient concentration, and temperature. Mar. Biol. 144 (1), 61–70. doi: 10.1007/s00227-003-1175-8
Steinhagen S., Enge S., Larsson K., Olsson J., Nylund G. M., Albers E., et al. (2021). Sustainable large-scale aquaculture of the northern hemisphere Sea lettuce, Ulva fenestrata, in an off-shore seafarm. J. Mar. Sci. Eng. 9, 615. doi: 10.3390/jmse9060615
Steinhagen S., Enge S., Cervin G., Larsson K., Edlund U., Schmidt A. E.M., et al. (2022). Harvest time affects the optimal yield and quality of sea lettuce (Ulva fenestrata) in a sustainable sea-based cultivation. Front. Mar. Sci. 9. doi: 10.3389/fmars.2022.816890
Steinhagen S., Karez R., Weinberger F. (2019a). Cryptic, alien and lost species: molecular diversity of Ulva sensu lato along the German coasts of the north and Baltic seas. Eur. J. Phycol. 54 (3), 466–483. doi: 10.1080/09670262.2019.1597925
Steinhagen S., Karez R., Weinberger F. (2019b). Surveying seaweeds from the ulvales and fucales in the world’s most frequently used artificial waterway, the Kiel canal 1), 51–61. doi: 10.1515/bot-2018-0020
Stévant P., Rebours C., Chapman A. (2017). Seaweed aquaculture in Norway: recent industrial developments and future perspectives. Aquac. Int. 25, 1373–1390. doi: 10.1007/s10499-017-0120-7
Stratmann J., Paputsoglu G., Oertel W. (1996). Differentiation of Ulva mutabilis (Chlorophyta) gametangia and gamete release are controlled by extracellular inhibitors. J. Phycol. 32 (6), 1009–1102. doi: 10.1111/j.0022-3646.1996.01009.x
Titlyanov E. A., Titlyanova T. V. (2010). Seaweed cultivation: Methods and problems. Russian J. Mar. Biol. 36, 227–242. doi: 10.1134/S1063074010040012
Toth G. B., Harrysson H., Wahlström N., Olsson J., Oerbekke A., Steinhagen S., et al. (2020). Effects of irradiance, temperature, nutrients, and pCO2 on the growth and biochemical composition of cultivated Ulva fenestrata. J. Appl. Phycol. 32, 3243–3254. doi: 10.1007/s10811-020-02155-8
Trigo J. P., Engström N., Steinhagen S., Juul L., Harrysson H., Toth G. B. (2021). In vitro digestibility and caco-2 cell bioavailability of sea lettuce (Ulva fenestrata) proteins extracted using pH-shift processing. Food Chem. 356, 129683. doi: 10.1016/j.foodchem.2021.129683
UN General Assembly (2015). “Sustainable development goals,” in SDGs transform our world, 2030. United Nations, New York
Wahlström N., Steinhagen S., Toth G., Pavia H., Edlund U. (2020a). Ulvan dialdehyde-gelatin hydrogels for removal of heavy metals and methylene blue from aqueous solution. Carbohydr. Polymers 249, 116841. doi: 10.1016/j.carbpol.2020.116841
Wahlström N., Edlund U., Pavia H., Toth G., Jaworski A., Pell A. J., et al. (2020b). Cellulose from the green macroalgae Ulva lactuca: isolation, characterization, optotracing, and production of cellulose nanofibrils. Cellulose 27 (7), 3707–3725. doi: 10.1007/s10570-020-03029-5
Wichard T., Charrier B., Mineur F., Bothwell J. H., Clerck O. D., Coates J. C. (2015). The green seaweed Ulva: a model system to study morphogenesis. Front. Plant Sci. 6, 72. doi: 10.3389/fpls.2015.00072
Keywords: Ulva fenestrata, Ulva lactuca, aquaculture, biochemical composition, protein, sea lettuce
Citation: Steinhagen S, Larsson K, Olsson J, Albers E, Undeland I, Pavia H and Toth GB (2022) Closed life-cycle aquaculture of sea lettuce (Ulva fenestrata): performance and biochemical profile differ in early developmental stages. Front. Mar. Sci. 9:942679. doi: 10.3389/fmars.2022.942679
Received: 12 May 2022; Accepted: 04 July 2022;
Published: 25 July 2022.
Edited by:
Yunyan Deng, Institute of Oceanology (CAS), ChinaReviewed by:
Fuli Liu, Ocean University of China, ChinaAlejandro H. Buschmann, University of Los Lagos, Chile
Mingyang Ma, Institute of Hydrobiology (CAS), China
Copyright © 2022 Steinhagen, Larsson, Olsson, Albers, Undeland, Pavia and Toth. This is an open-access article distributed under the terms of the Creative Commons Attribution License (CC BY). The use, distribution or reproduction in other forums is permitted, provided the original author(s) and the copyright owner(s) are credited and that the original publication in this journal is cited, in accordance with accepted academic practice. No use, distribution or reproduction is permitted which does not comply with these terms.
*Correspondence: Sophie Steinhagen, sophie.steinhagen@gu.se
†ORCID: Sophie Steinhagen, orcid.org/0000-0001-8410-9932
Karin Larsson, orcid.org/0000-0002-4265-9044
Eva Albers, orcid.org/0000-0002-1921-3415
Ingrid Undeland, orcid.org/0000-0002-9732-3644
Henrik Pavia, orcid.org/0000-0001-7834-6026
Gunilla B. Toth, orcid.org/0000-0002-1225-7773