- 1Jiangsu Key Laboratory of Marine Bioresources and Environment/Jiangsu Key Laboratory of Marine Biotechnology, Jiangsu Ocean University, Lianyungang, China
- 2Marine Resources Development Institute of Jiangsu, Jiangsu Ocean University, Lianyungang, China
- 3Key Laboratory of Coastal Salt Marsh Ecosystems and Resources Ministry of Natural Resources, Jiangsu Ocean University, Lianyungang, China
- 4Co-Innovation Center of Jiangsu Marine Bio-Industry Technology, Jiangsu Ocean University, Lianyungang, China
Climate changes such as seawater acidification caused by rising atmospheric CO2 and increased ultraviolet radiation (UVR) intensity resulting from shoaling of the upper mixed layer may interact to influence the physiological performance of marine primary producers. But few studies have investigated long-term (>30 days) effects of UVR under seawater acidification conditions, along with less attention on the differential effects of long- and short-wavelength UVA. In the present study, four spectral treatments (>280, >320, >360, and >400 nm) under two pCO2 levels (400 and 1,000 μatm) were set to investigate the interactive effects of seawater acidification and UVR on the bloom-forming diatom Skeletonema costatum. The results showed that UVR decreased growth and effective quantum yield of Photosystem II (PSII) by 9%–16% and 11%–24%, respectively, but it enhanced cell sizes significantly. Long- and short-wavelength UVA showed differential effects on cell volume and the effective quantum yield of PSII, especially at the elevated CO2 level. Generally, seawater acidification depressed the effective quantum yield of PSII and cell volume by 6%–18% and 8%–39%, respectively. Additionally, the contents of key PSII proteins (D1 and D2) decreased at the elevated CO2 level. Elevated CO2 significantly increased the inhibition of UVR on growth in the >280 nm spectral treatment when compared with ambient CO2, while it showed no effects in other spectral treatments. Overall, the results indicate that the effects of seawater acidification on the ubiquitous diatom are light wavelength-dependent.
Introduction
The oceans absorb about one-third of the atmospheric CO2 emitted by anthropogenic activities since the industrial revolution, which leads to ocean acidification (OA) (Sabine et al., 2004). OA is predicted to have significant effects on marine organisms at different trophic levels in the marine ecosystem. Under the OA scenario, seawater pH and concentration decrease, while concentrations of H+, , and aqueous CO2 increase (Hurd et al., 2019). It is supposed that OA, alongside ocean warming and associated shoaling of the upper mixed layer and decline in nutrient availability, would influence the marine ecosystem dramatically (Bopp et al., 2013). For example, calcifying organisms are considered to be more vulnerable to OA, with decreased calcification rate (Gao et al., 2009; Albright et al., 2016; Li et al., 2021), and varied results were found for non-calcifying organisms due to the complexity of ocean dynamics (Gao and Campbell, 2014). High CO2 could increase growth rates of some centric diatom species (Wu et al., 2014), while OA could decrease the growth of the oceanic diatom Thalassiosira oceanica (Li et al., 2016), and no detectable effects of OA on growth and photosynthesis of the coastal diatom Thalassiosira weissflogii were found (Li et al., 2019). In some field studies, enhanced diatom growth was observed in phytoplankton communities when exposed to high CO2 (Tortell et al., 2008; Feng et al., 2009). However, decreased abundance of microplanktonic diatoms under OA scenario was also reported in an Antarctic coastal community (Hancock et al., 2018). The region-specific and species-specific responses of diatoms to OA documented in previous studies may result from the trade-off between the positive effects of elevated CO2 and the negative effects of decreased pH (Gao and Campbell, 2014). Despite the varied results, OA generally decreased the photosynthetic affinity for inorganic carbon and thus led to the downregulation of CO2-concentrating mechanisms (CCMs) in a range of diatoms (Raven and Beardall, 2014). At the projected pCO2 level at the end of this century (800–1,000 ppmv), the downregulation of CCMs is expected to save 3%–6% of energy for carbon fixation in diatoms (Hopkinson et al., 2011).
Phytoplankton cells are exposed to both photosynthetically active radiation (PAR) and ultraviolet radiation (UVR, including UVA and UVB). UVR is generally considered to have adverse effects on the photosynthesis process of phytoplankton (Bais et al., 2018). The influences would continue because of the long existence of ozone-depleting substances in the atmosphere (Williamson et al., 2014) and shoaling of the upper mixed layer (Bopp et al., 2013). Under excessive PAR and UVR, the reaction center of the PSII protein pigment complex in phytoplankton cells would be damaged by reactive oxygen species (ROS) (Salin, 1988). UVR could also damage membrane proteins (Murphy, 1983), which are related to the operation of CCMs and nutrient uptake. Limited studies have focused on the effects of UVR on CCMs of phytoplankton. Beardall et al. (2002) showed that CCM activity of Dunaliella tertiolecta was enhanced by UVB, while UVB depressed the activity of Na+-dependent bicarbonate transporters of the cyanobacterium Microcystis (Song and Qiu, 2007). For nutrient uptake, negative effects of UVB were generally reported, while uptake stimulation was also observed (Aubriot et al., 2004). Phytoplankton have a range of molecular mechanisms to protect themselves from UVR damage. The diatom frustules could attenuate UV radiation and protect cells from UV damage (Ellegaard et al., 2016). Some benthic diatoms were found to increase their antioxidant capacity to detoxify ROS in response to chronic (several days) UV exposure and excessive PAR (Rijstenbil et al., 2000). Some photoprotective pigments accumulated and functioned as sunscreen (Ha et al., 2014), and mycosporyne-like amino acids (MAAs) also accumulated to cope with high light stress (Smith et al., 1992). Although phytoplankton are equipped with these protective mechanisms, significant negative effects caused by UVR have been observed in numerous studies. The UVR tolerance of phytoplankton is different from species to species (De Tommasi et al., 2018), and this may be related to cell size (Wu et al., 2015). Additionally, the UVR effects also depend on the intensity and fluctuation of solar irradiance. Positive effects of UVA on photosynthetic carbon fixation rate of coastal phytoplankton were reported under reduced levels (Gao et al., 2007) or fast-fluctuating solar irradiance (Helbling et al., 2003). UVA has also been shown to be involved in the photorepair process of UVB-induced DNA damage (Buma et al., 2003).
Phytoplankton dwelling in the upper mixed layer are undergoing a series of variations caused by climate changes, such as OA and enhanced UV radiation. As one of the key photosynthetic phytoplankton groups and primary producers in marine ecosystems, diatoms play a vital role in the transfer process of particulate organic material (Tréguer et al., 2018). Independent effects of OA or UVR on marine diatoms have been extensively studied, and some studies have investigated the interactive effects of OA and UV (Beardall et al., 2014). However, most previous studies generally ignore the difference between long- and short-wavelength UVA, which was suggested to have different effects on phytoplankton (Harrison and Smith, 2011). In the present study, we cultured Skeletonema costatum, a bloom-forming diatom species, at two pCO2 levels (400 and 1,000 μatm) to investigate the effects of OA after chronic exposure to four spectral treatments (>280, >320, >360, and >400 nm), with special focus on the differential effects of long- and short-wavelength UVA.
Materials and Methods
Culture Conditions
S. costatum was originally isolated from the coastal water of Gaogong Island, Jiangsu province (34.7074°N, 119.4926°E). S. costatum cells were precultured under natural solar irradiance, and autoclaved natural seawater enriched with f/2 medium (Guillard and Ryther, 1962) was used for cultures. Seawater was collected from coastal regions of Haizhou Bay, and the mean values of in situ nitrate and phosphate concentrations were 14 and 4 μmol l-1, respectively. Precultures were maintained in 500 ml polycarbonate bottles before cells were transferred to quartz tubes in a water tank, and bottles were covered by two layers of neutral-density screens to avoid high light stress and achieve a more realistic light intensity, which was experienced by phytoplankton in the upper mixed layer. Intensities of PAR (400–700 nm), UVA (315–400 nm), and UVB (280–315 nm) were recorded by a broadband solar radiometer (LoggerNet CR3000, Campbell). The culture temperature was controlled at 20°C by a thermostat (HYH-2DR-B, Sunsun).
Experimental Setup
Two pCO2 levels (400 and 1,000 μatm) and four spectral treatments (>280, >320, >360, and >400 nm) were set, and triplicate cultures were used for each treatment. S. costatum was cultured at 20°C in 100-ml quartz tubes and was aerated with sterile ambient air (400 μatm) or CO2-enriched air (1,000 μatm). All the tubes were cultured in a water tank with two layers of neutral-density screens. The high pCO2 levels were achieved by a CO2 plant incubator (HP 1000 G-D, Ruihua). Different light wavelength treatments were realized by using ultraviolet filters that could transmit light with wavelengths above 280, 320, 360, and 400 nm, respectively (Figure 1). Cells were diluted with fresh medium every 3 days, and cell concentrations were controlled at less than 2 × 105 cells ml-1. The pH variation caused by cell metabolism was less than 0.05 units. After acclimation for at least 30 days, the following physiological parameters were sampled and measured.
Growth and Cell Volume Measurements
Triplicate samples of all treatments were collected at 24 and 72 h after dilution and were fixed with Lugol’s solution to estimate cell density and volume. The plankton counting chamber (DSJ-01, Xundeng) and optical microscope (DM500, Leica) were used to count cells. Specific growth rates of cells were calculated as follows: μ = (LnNt-LnN0)/(t-t0), where Nt represents the cell concentration at time t and N0 represents the cell concentration at time t0; in this experiment, t – t0 = 2 d. Cells were photographed with a digital camera (ToupCam, Toup Tek), and the diameter and height of cells were measured by ToupView software, and cell volume was calculated according to the equation for a cylinder.
Chlorophyll a and Biogenic Silica Measurements
Each sample of 80 ml for chlorophyll a measurement was filtered onto a GF/F filter, and then 4 ml of methanol was added. Chlorophyll a was extracted at 4°C overnight in darkness. Then, the absorption values of supernatants at 632, 665, and 750 nm were detected by an ultraviolet spectrophotometer (Ultrospect 3300 pro, Amersham Bioscience). The chlorophyll a concentration of S. costatum was calculated by the equation of Ritchie (2006).
Each sample of 80 ml for biogenic silica (BSi) measurement was filtered onto a polycarbonate filter (25 mm, Merck Millipore). Filters were then dried at 80°C overnight. BSi on each filter was digested by NaOH neutralized with HCl as previously described (Li et al., 2021). After adding molybdate soln and reducing agent into each tube, the absorption was measured at 810 nm by an ultraviolet spectrophotometer (Ultrospect 3300 pro, Amersham Bioscience) according to Brzezinski and Nelson (1995).
Chlorophyll Fluorescence Measurement
The effective quantum yield of PSII (Fv’/Fm’) and rapid light curves (RLCs) were measured by a handheld chlorophyll fluorometer (AquaPen-C, AP-P 100). Cells were concentrated by gentle vacuum filtration, and RLCs were measured at eight light intensities (0, 10, 20, 50, 100, 200, 500, and 1,000 μmol photons m-2 s-1) lasting for 10 s each. Samples were acclimated to culture light condition before RLC measurements to avoid an induction effect. Relative electron transport rates (rETR) were estimated as follows: rETR = PAR × Fv’/Fm’ × 0.5, where PAR represents the actinic light intensity (μmol photons m-2 s-1); Fv’/Fm’ represents the effective quantum yield of PSII; 0.5 is based on the assumption that PSII receives half of all absorbed quanta. RLCs were fitted as follows: rETR = PAR/(a × PAR2 + b × PAR + c), where a, b, and c are model parameters. Then, three parameters were used to calculate the maximum relative electron transport rate (rETRmax), apparent photon transfer efficiency (α), and light saturation point (Ik) according to Eilers and Peeters (1988).
Photosynthesis and Respiration Measurements
Photosynthesis vs. light intensity (P-I) curves were measured at 20°C by a Clark-type oxygen electrode (Oxygraph+, Hansatech). Cells were gently concentrated onto cellulose acetate membranes (47 mm, Xinya) and then were resuspended in 5 ml Tris-buffered medium. Respiration rates in darkness and net photosynthetic oxygen evolution rates at eight light intensities (0, 10, 20, 50, 100, 200, 400, 600, and 1,000 μmol photons m-2 s-1) were identified. A halogen lamp (QVF135, Philips) was used as the light source, and the increasing light intensities were realized by adjusting the distance between the lamp and the electrode. P-I curves were fitted according to Henley (1993): P = Pm × tanh (α×PAR/Pm) + Rd, where P represents photosynthetic rate, Pm represents light-saturated photosynthetic rate, α is apparent photon transfer efficiency, PAR is light intensity, and Rd is dark respiration rate. Ik (light saturation point) and Ic (light compensation point) were calculated as follows:
Protein Measurement
The abundance of PsbA (D1), PsbD (D2), PsbB (CP47), and RbcL proteins of cells in different treatments was quantified as previously described (Li et al., 2021). Briefly, protein samples were separated by sodium dodecyl sulfate polyacrylamide gel electrophoresis (SDS-PAGE) (12%) and were transferred onto polyvinylidene difluoride (PVDF) membranes that were then immersed in blocking solution with antibodies (D1, D2, CP47, Rubisco L; Agrisera) for 1 h, and then goat anti-rabbit secondary antibodies were used. Blots were developed by using an enhanced chemiluminescence (ECL) reagent and were quantified with a chemiluminescence detection system (Tanon 5500, Tanon).
Data Analyses
Data were presented as mean ± SD (standard deviation). Data of specific growth rate were analyzed by a repeated-measures ANOVA. One-way ANOVA was used to detect differences in other parameters among solar spectral irradiance treatments. Tukey test was used for post-hoc analysis when P values were <0.05. The independent-samples t-test was applied to compare differences between two pCO2 levels. The general linear model was used to assess the interactive effects of CO2 level and spectral treatment on parameters.
Results
Solar Irradiance
The experiment was conducted in summer time and lasted for about 1 month. During the experimental period, the average solar irradiance at noon in a day could reach 238.41 W m-2 (Figure 2A), and the maximal total daily doses were found in day 6 (PAR: 10.61 MJ m-2, UVA: 1.17 MJ m-2, UVB: 0.05 MJ m-2), while the minimum values were observed in day 4 (PAR: 1.77 MJ m-2, UVA: 0. 20 MJ m-2, UVB: 0.01 MJ m-2; Figure 2B). Two layers of neutral-density screens filtered out about 60% of solar irradiance.
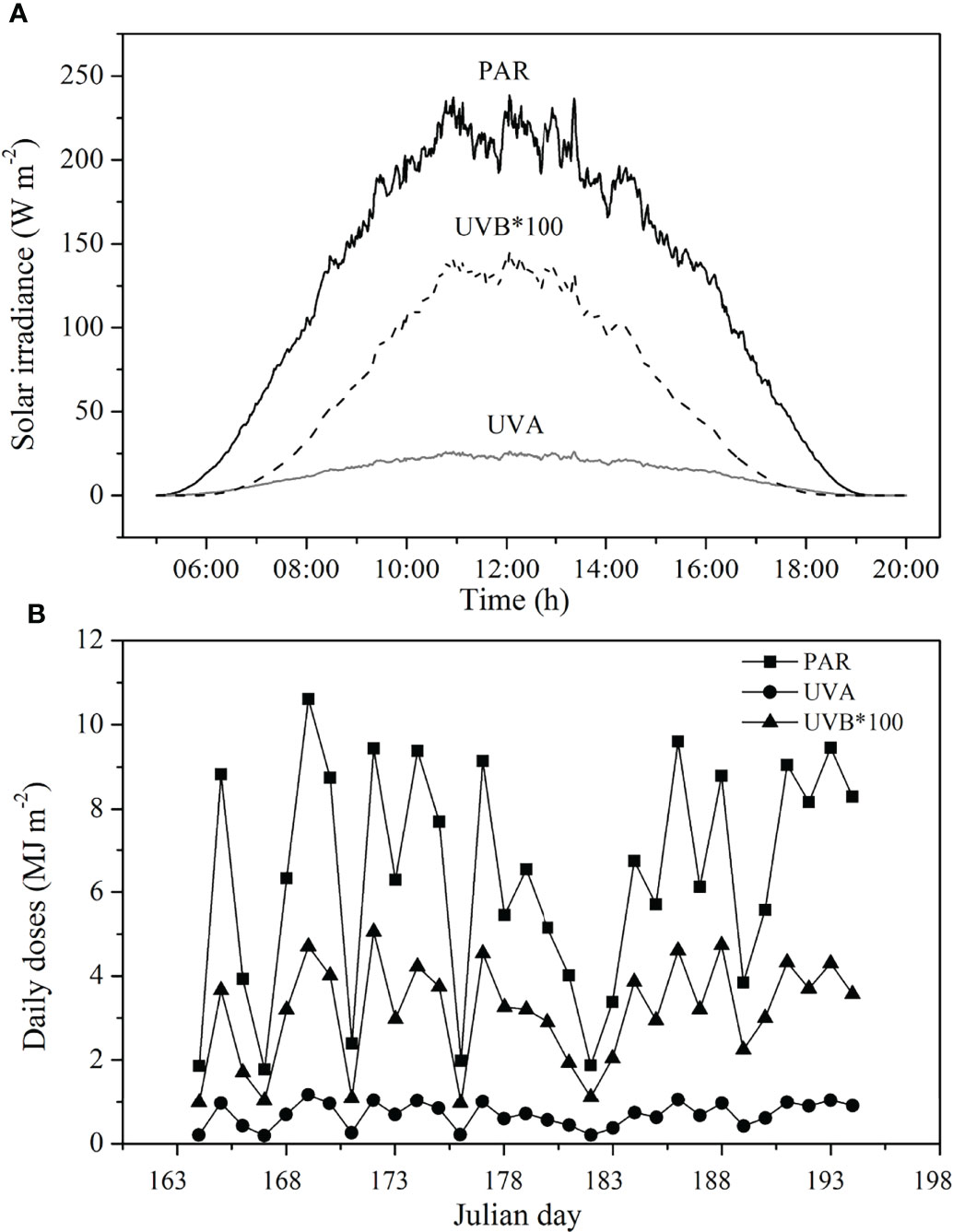
Figure 2 Average irradiance levels (A) and daily doses of (B) of PAR, UVA, and UVB during the experiment period. PAR, photosynthetically active radiation; UVA, ultraviolet A; UVB, ultraviolet B.
Growth Rate and Cell Volume
The specific growth rates in different treatments ranged from 1.50 ± 0.12 to 1.94 ± 0.10 d-1. The growth rate of cells in the PAR treatment (>400 nm) was significantly higher than that in other treatments regardless of CO2 treatments, except for no significant difference between >360 and >400 nm spectral treatments at the elevated CO2 level (Figure 3). Compared with cells in the >400 nm spectral treatment, cells exposed to UVR showed 9%–16% lower growth rate. In the same spectral treatment, high CO2 always showed no effects on growth of S. costatum cells. However, HC cells in the >280 nm spectral treatment showed 12% lower growth rate than LC ones (P = 0.007).
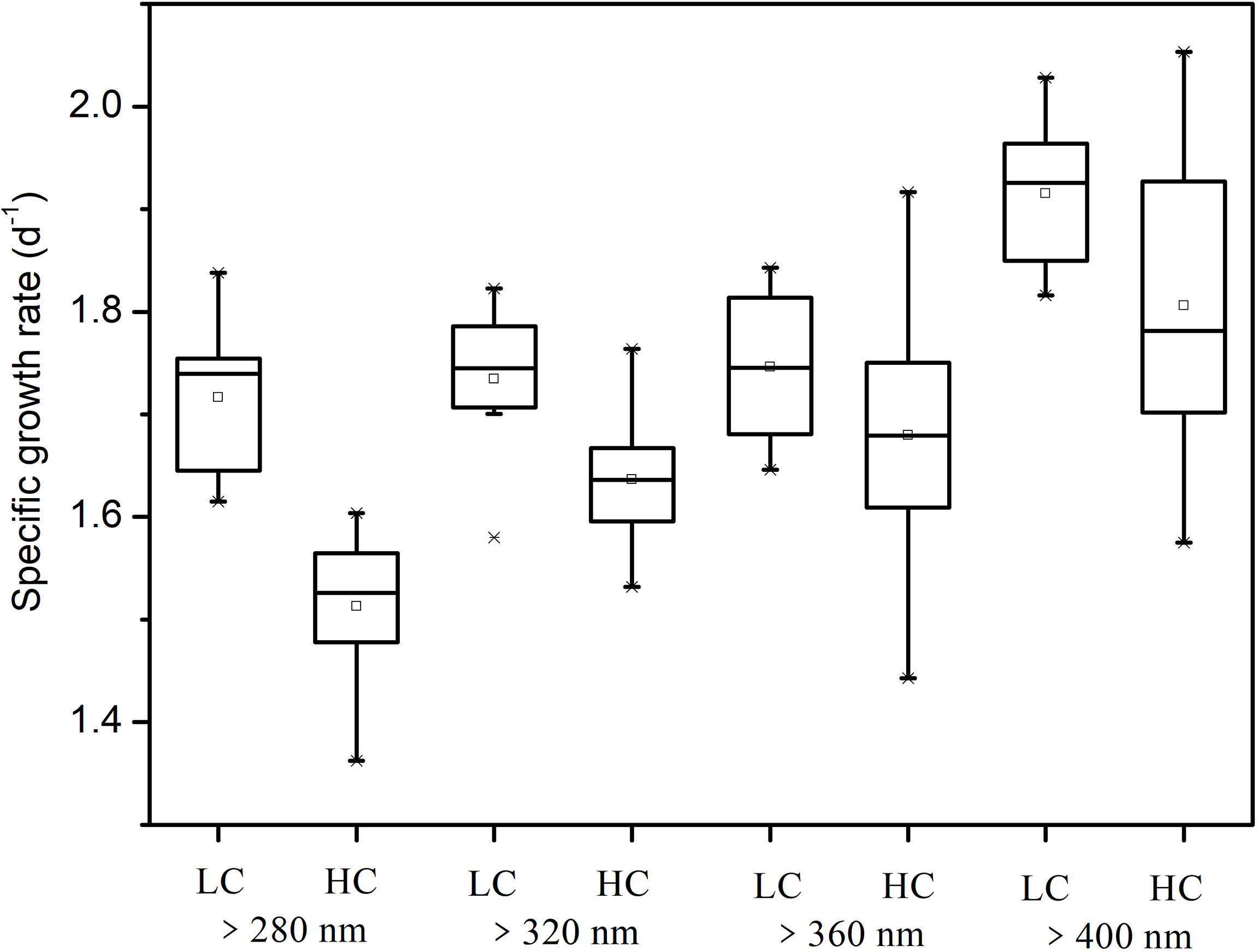
Figure 3 Specific growth rate of S. costatum acclimated to ambient (LC) and elevated CO2 (HC) in different spectral treatments.
UVR significantly increased the cell volume of S. costatum at both CO2 levels (Figure 4). At ambient CO2 levels, cells in the >360 nm spectral treatment had much smaller volumes than those of the >320- and >280 nm spectral treatments (P < 0.05), and cells in the PAR treatment had the smallest volume. Cells at the high CO2 level showed a similar trend as those at ambient CO2 levels, except for no significant difference between >360 and >400 nm spectral treatments. Elevated CO2 decreased cell sizes in all treatments except for the >400 nm spectral treatment (P = 0.001, P = 0.002, P = 0.009 for the >280, >320, and >360 nm spectral treatments, respectively). CO2 level and spectral treatment had a notable interaction on cell volume (P = 0.002).
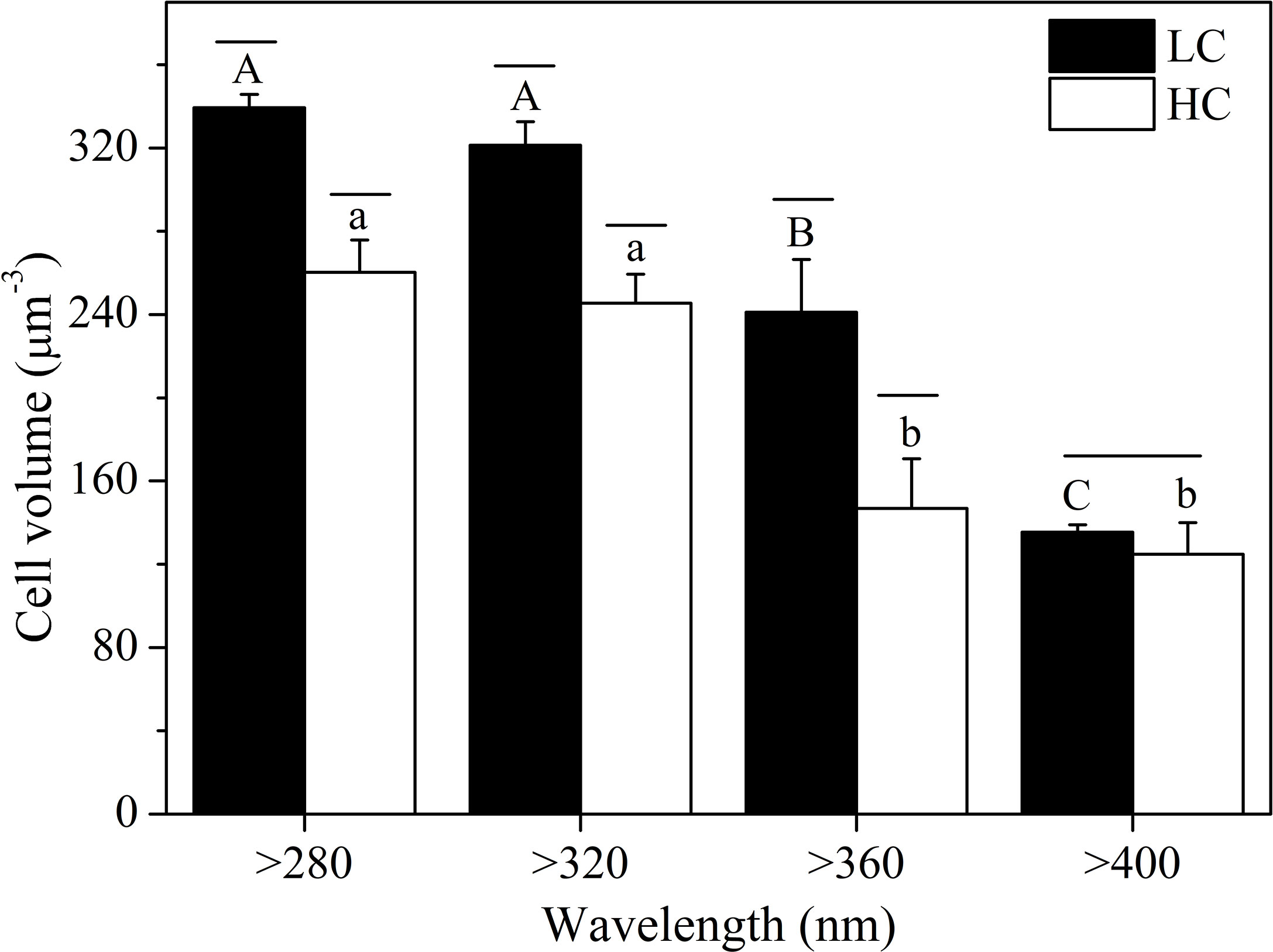
Figure 4 Cell volume of S. costatum acclimated to ambient (LC, black bars) and elevated CO2 (HC, white bars) in different spectral treatments. The different uppercase and lowercase letters represent significant differences between different spectral treatments under LC and HC conditions, respectively.
Chlorophyll a and Biogenic Silica Contents
At ambient CO2 levels, chl a content of cells in the >280 nm spectral treatment was significantly lower than that in other treatments (P < 0.05), while there were no significant differences among the four spectral treatments at the elevated CO2 level (Figure 5A). Elevated CO2 resulted in higher chl a content in all spectral treatments (P < 0.05). The CO2 level and spectral treatment had a notable interaction on chl a content (P = 0.025).
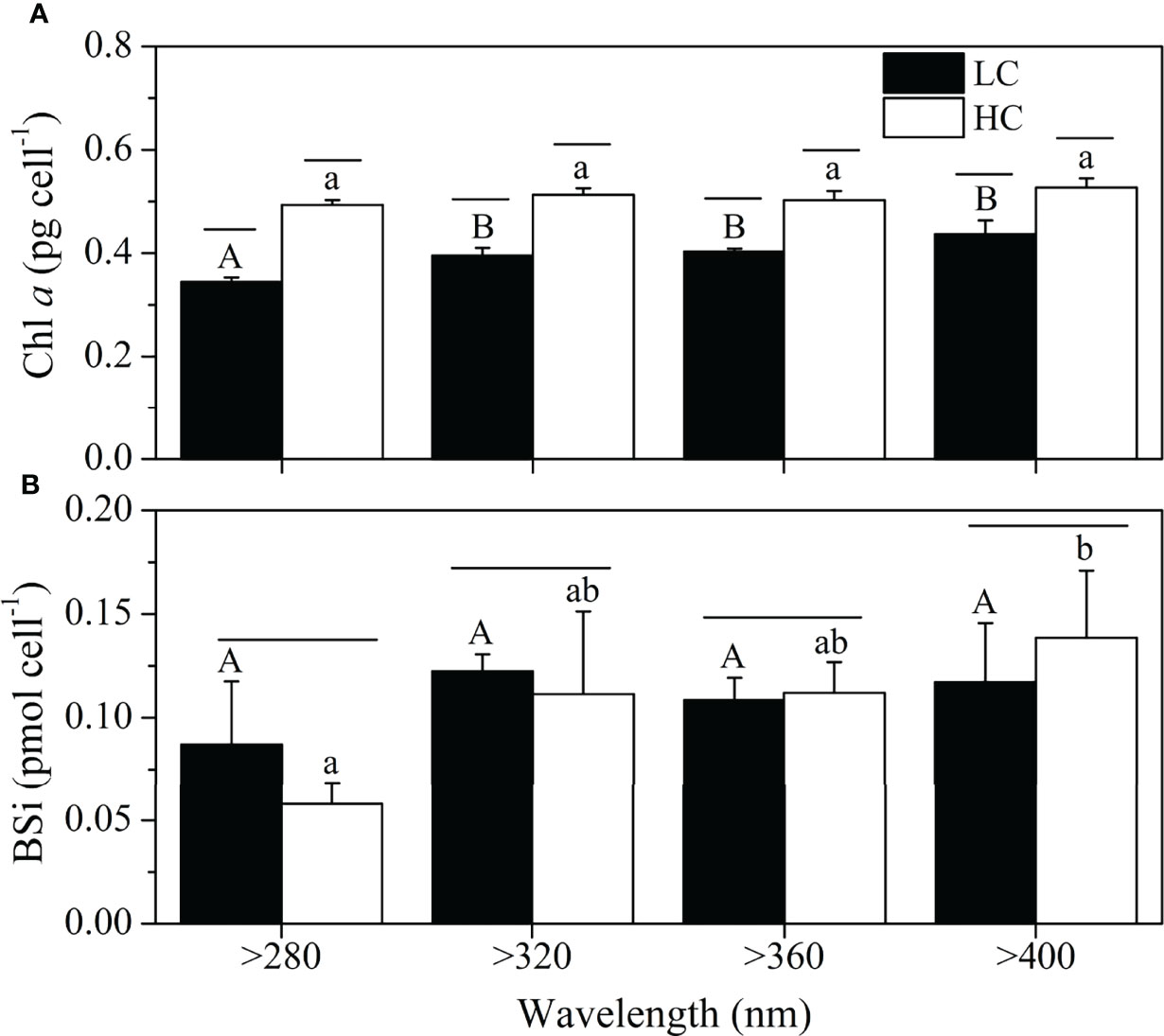
Figure 5 Chla (A) and BSi contents (B) of S. costatum acclimated to ambient (LC, blackbars) and elevated CO2 (HC, whitebars) in different spectral treatments. The different uppercase and lowercase letters represent significant differences between different spectral treatments under LC and HC conditions, respectively.
The BSi contents were unaltered by the spectral treatments, except for the lower content in cells treated by the >280 nm spectral treatment than that of >400 nm spectral treatment at the elevated CO2 level (Figure 5B). Elevated CO2 had no significant effect on BSi contents for the same spectral treatment.
Chlorophyll Fluorescence
The effective quantum yield of PSII (Fv’/Fm’) significantly increased when short wavelengths were cut off (Figure 6) at both CO2 levels, and the highest value was observed in the >400 nm spectral treatment (LC = 0.70 ± 0.01, HC = 0.66 ± 0.01). For the same spectral treatment, high CO2 significantly reduced Fv’/Fm’ by 6%–18% (P < 0.001). The CO2 level and spectral treatment had a notable interaction on Fv’/Fm’ (P < 0.001).
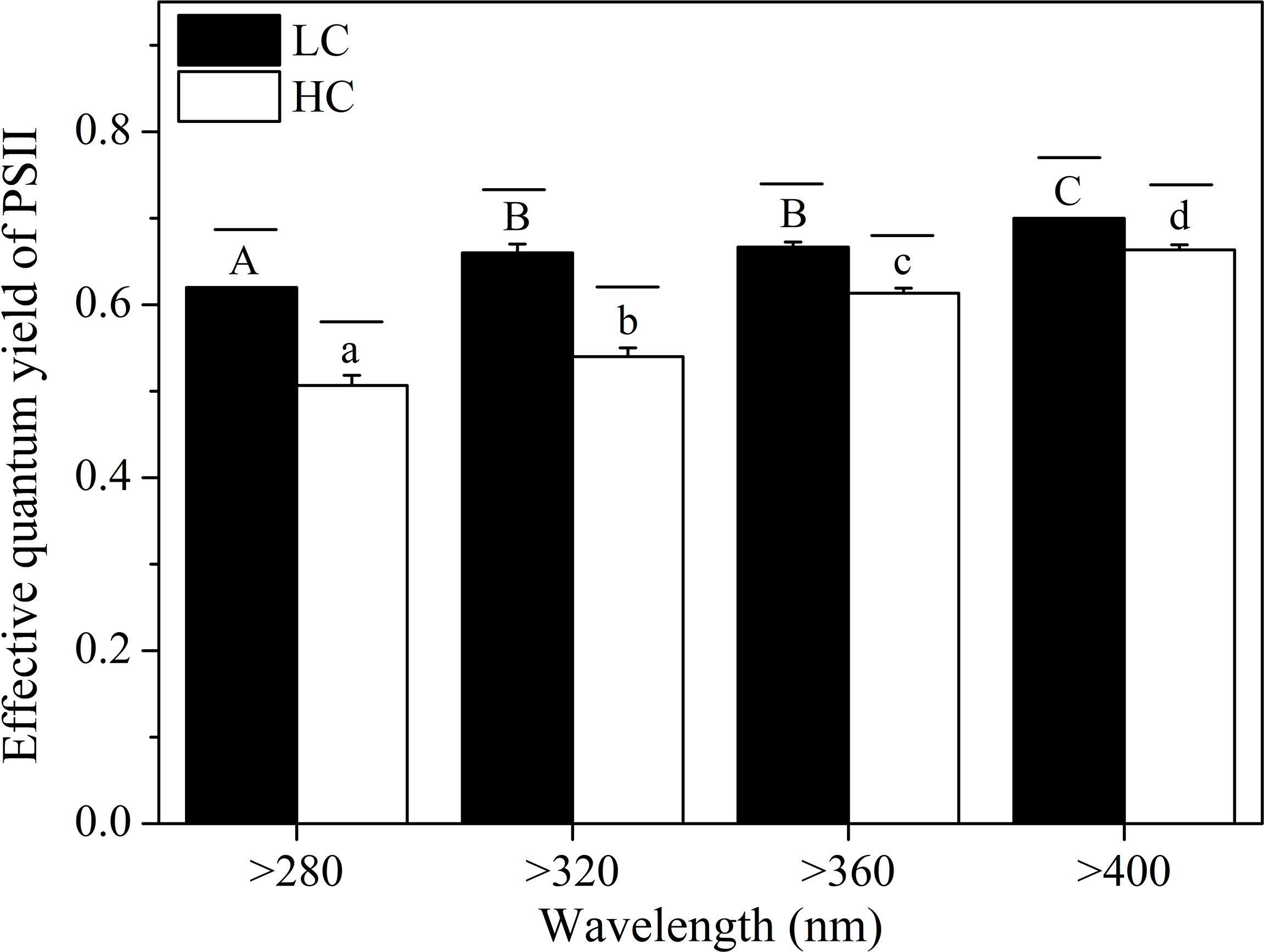
Figure 6 The effective quantum yield of PSII (Fv’/Fm’) of S. costatum acclimated to ambient (LC, black bars) and elevated CO2 (HC, white bars) in different spectral treatments. The different uppercase and lowercase letters represent significant differences between different spectral treatments under LC and HC conditions, respectively.
Different spectral treatments had no significant effects on Ik (light saturation point), α (apparent photon transfer efficiency), and rETRmax (the maximum relative electron rate) at both CO2 levels (Figure 7 and Table 1), except for the lower Ik in the >400 nm spectral treatment compared with the >320 nm spectral treatment at the high CO2 level. High CO2 significantly decreased the α value in the >320 nm spectral treatment (P = 0.04) and decreased rETRmax and Ik in the >400 nm treatment (P = 0.020, P = 0.023, respectively).
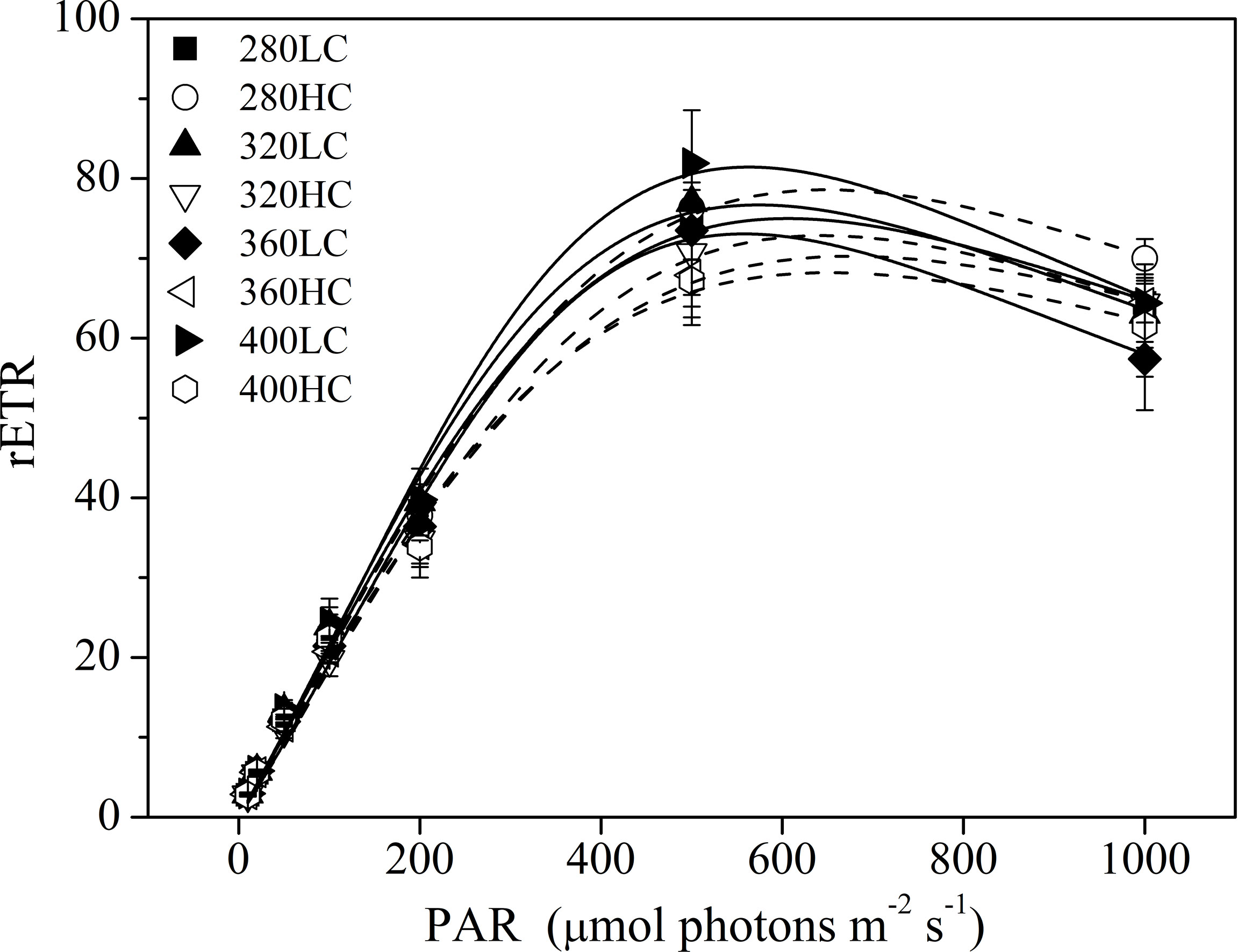
Figure 7 The RLCs of S. costatum acclimated to ambient (LC, black bars) and elevated CO2 (HC, white bars) in different spectral treatments.
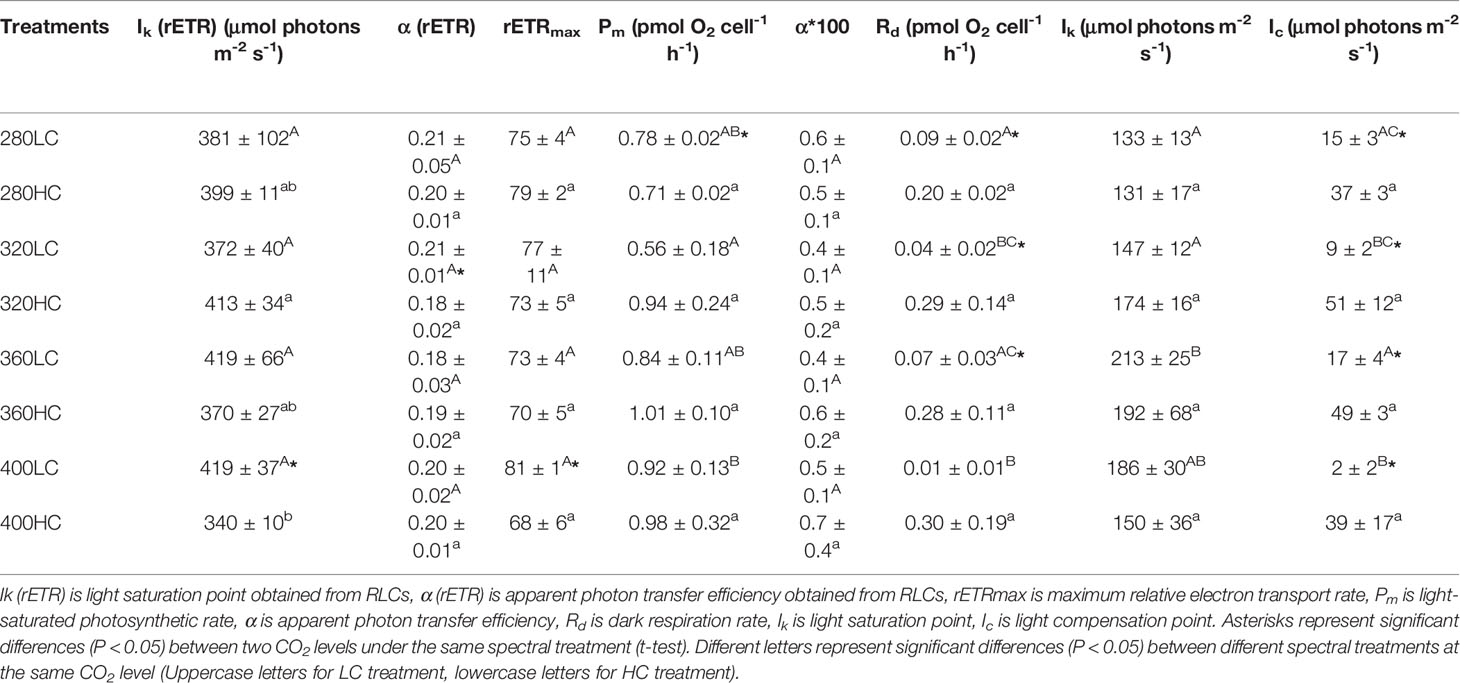
Table 1 Photosynthetic parameters of P-I and rapid light curves for S. costatum acclimated to ambient and elevated CO2 in different spectral treatments.
Photosynthesis and Respiration
According to P-I curves, the maximum photosynthetic rate (Pm), dark respiration rate (Rd), and other parameters were calculated (Figure 8). At ambient CO2 levels, the highest Pm was observed in cells treated by the >400 nm spectral treatment and was significantly higher than that in the >320 nm spectral treatment (P < 0.05, Table 1). The lowest Rd was also observed in cells treated by the >400 nm spectral treatment, which was significantly lower than that of >280 and >360 nm spectral treatments (P < 0.05). The highest values of Ik and Ic were both detected in the >360 nm spectral treatment. At elevated CO2 levels, there were no significant differences in all of these parameters among the four spectral treatments. Elevated CO2 significantly decreased Pm by 9% in the >280 nm spectral treatment (P = 0.01). Elevated CO2 significantly enhanced Rd in all spectral treatments except for the >400 nm spectral treatment. Similarly, the Ic was also enhanced in all spectral treatments (P < 0.05).
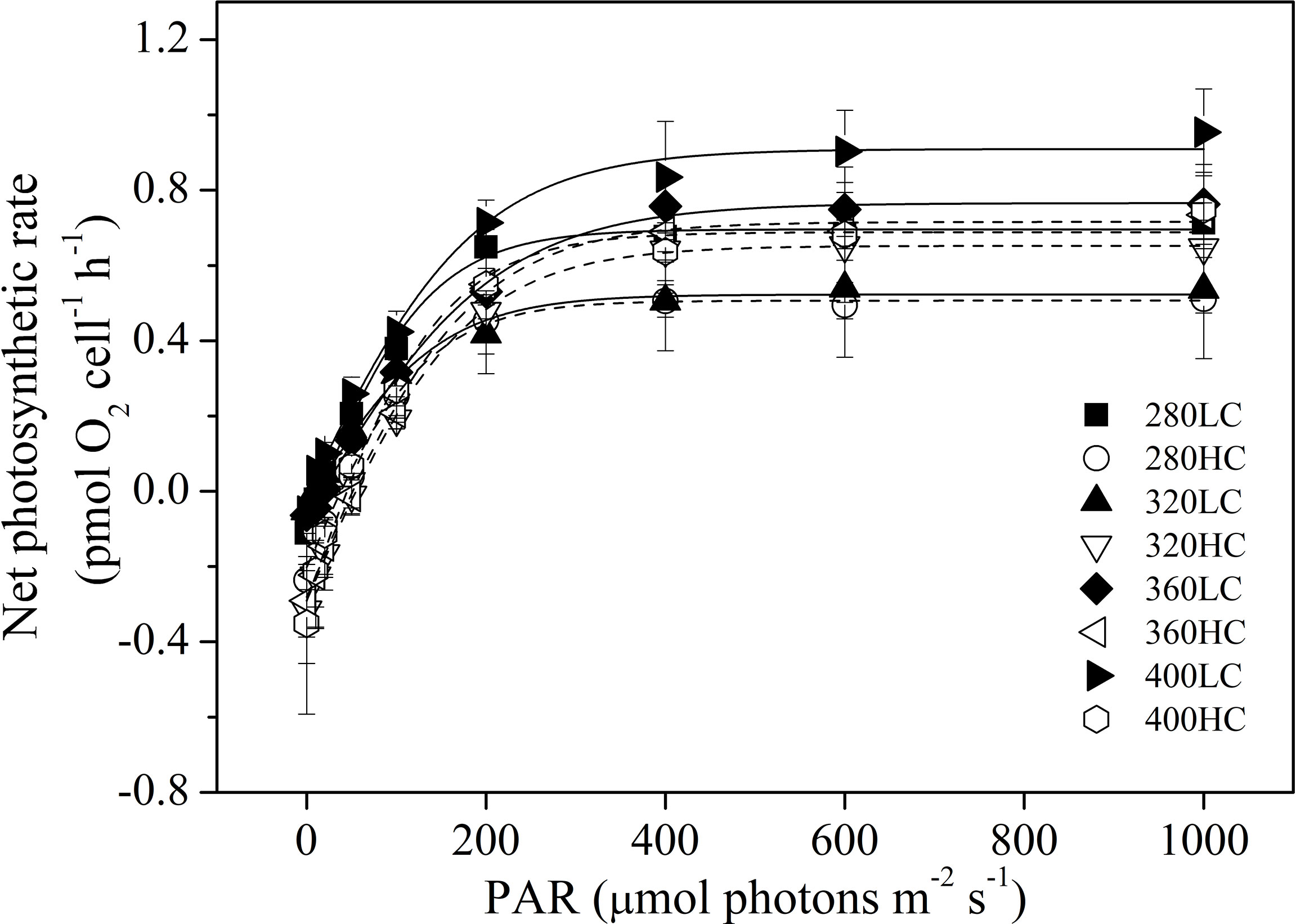
Figure 8 Photosynthesis-light curves (P-I curves) of S. costatum acclimated to ambient (LC, black bars) and elevated CO2 (HC, white bars) in different spectral treatments.
Protein Concentrations
Generally, spectral treatment showed no significant effect on the abundance of these key proteins. Elevated CO2 decreased the D1 and D2 protein contents, while it did not change the contents of CP47 and RbcL, with the exception that cells grown at elevated CO2 levels had lower CP47 contents than those at ambient CO2 levels for the >360 nm spectral treatment (Figure 9).
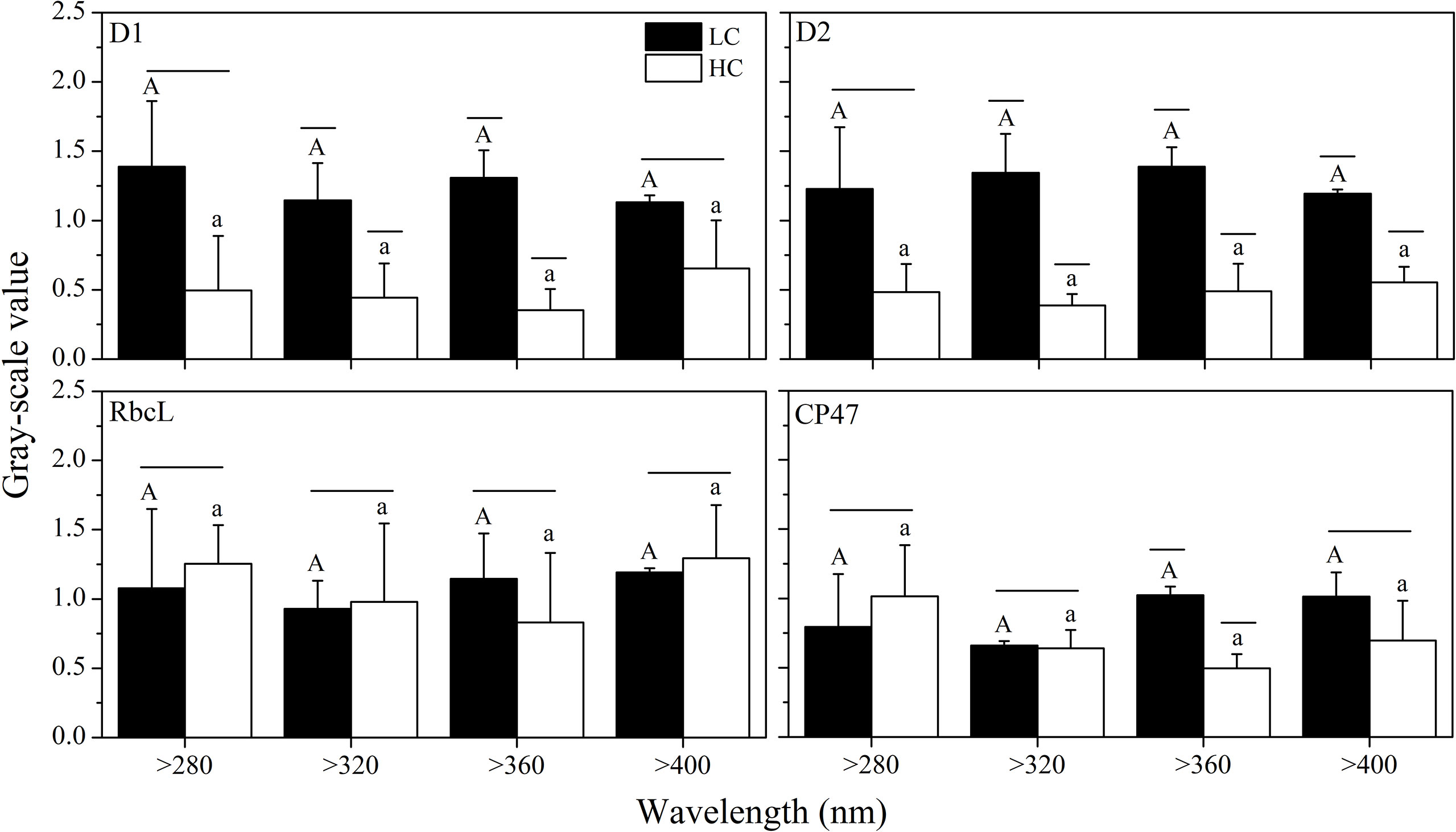
Figure 9 Quantitative analysis of proteins of S. costatum acclimated to ambient (LC, black bars) and elevated CO2 (HC, white bars) in different spectral treatments. The different uppercase and lowercase letters represent significant differences between different spectral treatments under LC and HC conditions, respectively.
Discussion
Effects of UVR on Growth and Photosynthesis of S. costatum
In the present study, UVR decreased the specific growth rate of S. costatum by 9%–16%. At ambient CO2 levels, no significant differences were observed among the spectral treatments with wavelengths shorter than 400 nm. It means that UVB did not exacerbate the negative effects of short- and long-wavelength UVA at ambient CO2 levels. This may be caused by the low UVB dose in the present study, as we added two layers of neutral-density screens. Effects of UVR (specially UVA) are supposed to be dose-dependent (Gao et al., 2007). For coastal phytoplankton assemblages of the South China Sea, the irradiance thresholds at which the effects of UVR shift from positive to negative are reported in the ranges of 37.2–50.1 W m-2 and 1.76–2.33 W m-2 for UVA and UVB, respectively (Gao et al., 2007). In the present study, the mean UVA (6.4 W m-2) and UVB (0.28 W m-2) irradiances were under the lower limited values. However, no positive effects of UVR were observed in the present study. This may be related to the species-specific tolerance to high light (Key et al., 2010) and UVR (Wu et al., 2015). The reduced growth rate was accompanied by the increased cell volume in cells exposed to UVR treatments. Nahon et al. (2010) also found that the S. costatum cells exposed to UVR were about twice the biovolume of the control cells. This means that, in the presence of UVR, the thickness of silicified cell wall became thinner given the unaltered BSi content per cell and markedly increased cell volume.
The effective quantum yield of PSII of S. costatum cells was depressed by UVR by 11%. However, this did not indicate the irreversible damage of PSII system by UVR, as shown by the unaltered rETRmax and D1 and D2 protein abundance. Similarly, there were no differences in the light-saturated photosynthetic rate (determined under PAR) between cells exposed to >280 and >400 nm spectral treatments. As mentioned above, this may be partly related to the low UVB used during the experimental period. Additionally, the stimulated activity of extracellular carbonic anhydrase of S. costatum cells may also play a part (Wu and Gao, 2009).
Long- and short-wavelength UVA showed differential effects on cell volume and the effective quantum yield of PSII of S. costatum, especially at elevated CO2 levels. Cells treated by short-wavelength UVA had lower effective quantum yield and larger cell sizes than cells in the long-wavelength UVA treatment. The differential effects of long- and short-wavelength UVA were also reported in both marine (Mengelt and Prézelin, 2005) and freshwater phytoplankton communities (Harrison and Smith, 2011). It is reasonable to assume that the difference in inhibition effects between long- and short-wavelength UVA will be enlarged with the UVR irradiance increases. As the short-wavelength UVR attenuates more rapidly with depth in most water bodies, and dissolved organic matter influences the attenuation coefficient of UVR (Kirk, 1994), the effects of UVR could be depth-dependent and be related to the concentration of organic matter in water columns. Thus, it is important to investigate the effects of long-wavelength UVR and consider the distinct light environment at different water depths in further studies.
Interactive Effects of Ocean Acidification and Spectral Exposure
Cells grown in HC treatment always showed lower growth rates than those of LC population, with increasing respiration but smaller cell size. This might be related to the high energy cost for cells to maintain intracellular acid–base homeostasis (Raven, 2011). In all spectral treatments, elevated CO2 significantly decreased the contents of D1 and D2 protein, which includes both photochemically active PSII and PSII that is inactivated but retains D1 and D2 subunits. This was in line with the lower effective quantum yield of PSII at higher CO2. Along with the faster removal of PsbA from a pool of photoinactivated PSII centers (Gao et al., 2018), elevated CO2 could alter the dynamic balance of photoinactivation and repair of PSII.
The impacts of elevated CO2 on S. costatum depended on light wavelengths, as shown by the changes in specific growth rate. Elevated CO2 significantly exacerbated the inhibition of UVR on growth in the >280 nm spectral treatment when compared with ambient CO2, while it showed no effects in other spectral treatments. This indicates that elevated CO2 exacerbates the negative effects of UVB on the growth of S. costatum. Similarly, the diatom Thalassiosira pseudonana was more sensitive to UVR (UVA: 6.91 W m-2; UVB: 0.44 W m-2) after being acclimated to elevated CO2 for 7–10 days (Sobrino et al., 2008). However, increased CO2 partly counteracted the UVB (1.2 W m-2)-induced damage on Phaeodactylum tricornutum (Li et al., 2012). The inconsistency seems to be related to the UVR dose used and CCM efficiency of species tested in studies. UVB was shown to have species-specific effects on CCMs of different phytoplankton (Beardall et al., 2002; Song and Qiu, 2007), which could influence the energy budget of cells.
The negative effects of increased CO2 on growth of S. costatum were found when light intensity was higher than 178 μmol photons m-2 s-1 (Gao et al., 2012). In the present study, the effective quantum yield of PSII was 6% lower at elevated CO2 levels compared with ambient CO2 levels, while no effects of CO2 on growth were detected in this spectral treatment due to the large variations. Dynamic light could impose extra stress on the photosynthetic apparatus, resulting in decreased growth and carbon fixation rates compared with constant light (Hoppe et al., 2015). Thus, it should be worth noting that the results obtained under constant light condition cannot be simply extrapolated to real oceans. Additionally, the effects of elevated CO2 were shown to be modulated by other factors, such as nutrient availability (Hong et al., 2017), temperature (Taucher et al., 2015), competition with other photoautotrophs (Gao et al., 2019), and combination of temperature and photoperiod (Li et al., 2021). It is crucial to clarify the interactions of the main environmental factors to make accurate predictions about the effects of climate changes on marine primary producers.
Data Availability Statement
The raw data supporting the conclusions of this article will be made available by the authors without undue reservation.
Author Contributions
FL and JX contributed to conception and design of the study. FL, HL, and TX performed the experiments. SL and JX analyzed the data. FL, HL, and JX wrote the first draft of the article. All authors contributed to article revision and read and approved the submitted version.
Funding
This work was supported by the “521project” of Lianyungang City (LYG06521202129), China Postdoctoral Science Foundation (2019M661766), Jiangsu Planned Projects for Postdoctoral Research Funds (2021K181B), Lianyungang Postdoctoral Science Foundation Innovation, Jiangsu Institute of Marine Resources Development Program (JSIMR202008), and MEL Visiting Fellowship (MELRS2104), Priority Academic Program Development of Jiangsu Higher Education Institutions.
Conflict of Interest
The authors declare that the research was conducted in the absence of any commercial or financial relationships that could be construed as a potential conflict of interest.
Publisher’s Note
All claims expressed in this article are solely those of the authors and do not necessarily represent those of their affiliated organizations, or those of the publisher, the editors and the reviewers. Any product that may be evaluated in this article, or claim that may be made by its manufacturer, is not guaranteed or endorsed by the publisher.
Acknowledgments
The authors thank all of the members of N301 for their support for the study.
References
Albright R., Caldeira L., Hosfelt J., Kwiatkowski L., Maclaren J. K., Mason B. M., et al. (2016). Reversal of Ocean Acidification Enhances Net Coral Reef Calcification. Nature 531, 362–365. doi: 10.1038/nature17155
Aubriot L., Conde D., Bonilla S., Sommaruga R. (2004). Phosphate Uptake Behavior of Natural Phytoplankton During Exposure to Solar Ultraviolet Radiation in a Shallow Coastal Lagoon. Mar. Biol. 144, 623–631. doi: 10.1007/s00227-003-1229-y
Bais A. F., Lucas R. M., Bornman J. F., Williamson C. E., Sulzberger B., Austin A. T., et al. (2018). Environmental Effects of Ozone Depletion, UV Radiation and Interactions With Climate Change: UNEP Environmental Effects Assessment Panel, Update 2017. Photochem. Photobiol. Sci. 17, 127–179. doi: 10.1039/C7PP90043K
Beardall J., Heraud P., Roberts S., Shelly K., Stojkovic S. (2002). Effects of UVB Radiation on Inorganic Carbon Acquisition by the Marine Microalga Dunaliella Tertiolecta (Chlorophyceae). Phycologia 41, 268–272. doi: 10.2216/i0031-8884-41-3-268.1
Beardall J., Stojkovic S., Gao K. (2014). Interactive Effects of Nutrient Supply and Other Environmental Factors on the Sensitivity of Marine Primary Producers to Ultraviolet Radiation: Implications for the Impacts of Global Change. Aquat. Biol., 22, 5–23. doi: 10.3354/ab00582
Bopp L., Resplandy L., Orr J. C., Doney S. C., Dunne J. P., Gehlen M., et al. (2013). Multiple Stressors of Ocean Ecosystems in the 21st Century: Projections With CMIP5 Models. Biogeosciences 10, 6225–6245. doi: 10.5194/bg-10-6225-2013
Brzezinski M. A., Nelson D. M. (1995). The Annual Silica Cycle in the Sargasso Sea Near Bermuda. Deep. Sea. Res. Part I.: Oceanogr. Res. Pap. 42, 1215–1237. doi: 10.1016/0967-0637(95)93592-3
Buma A. G., Boelen P., Jeffrey W. H. (2003). UVR-Induced DNA Damage in Aquatic Organisms, in UV Effects in Aquatic Organisms and Ecosystems. R. Soc. Chem. Cambridge, UK: Royal Society of Chemistry 1, 291–327.
De Tommasi E., Congestri R., Dardano P., De Luca A. C., Managò S., Rea I., et al. (2018). UV-Shielding and Wavelength Conversion by Centric Diatom Nanopatterned Frustules. Sci. Rep. 8, 16285. doi: 10.1038/s41598-018-34651-w
Eilers P., Peeters J. (1988). A Model for the Relationship Between Light Intensity and the Rate of Photosynthesis in Phytoplankton. Ecol. Modell. 42, 199–215. doi: 10.1016/0304-3800(88)90057-9
Ellegaard M., Lenau T., Lundholm N., Maibohm C., Friis S. M. M., Rottwitt K., et al. (2016). The Fascinating Diatom Frustule—Can it Play a Role for Attenuation of UV Radiation? J. Appl. Phycol. 28, 3295–3306. doi: 10.1007/s10811-016-0893-5
Feng Y., Hare C. E., Leblanc K., Rose J. M., Zhang Y., DiTullio G. R., et al. (2009). The Effects of Increased Pco2 and Temperature on the North Atlantic Spring Bloom: I. The Phytoplankton Community and Biogeochemical Response. Mar. Ecol. Prog. Ser. 388, 13–25. doi: 10.3354/meps08133
Gao K., Campbell D. A. (2014). Photophysiological Responses of Marine Diatoms to Elevated CO2 and Decreased Ph: A Review. Funct. Plant Biol. 41, 449–459. doi: 10.1071/FP13247
Gao G., Fu Q., Beardall J., Wu M., Xu J. (2019). Combination of Ocean Acidification and Warming Enhances the Competitive Advantage of Skeletonema Costatum Over a Green Tide Alga, Ulva Linza. Harmful Algae 85, 101698. doi: 10.1016/j.hal.2019.101698
Gao K., Ruan Z., Villafane V. E., Gattuso J.-P., Helbling E. W. (2009). Ocean Acidification Exacerbates the Effect of UV Radiation on the Calcifying Phytoplankter Emiliania Huxleyi. Limnol. Oceanogr. 54, 1855–1862. doi: 10.4319/lo.2009.54.6.1855
Gao G., Shi Q., Xu Z., Xu J., Campbell D. A., Wu H. (2018). Global Warming Interacts With Ocean Acidification to Alter PSII Function and Protection in the Diatom Thalassiosira Weissflogii. Environ. Exp. Bot. 147, 95–103. doi: 10.1016/j.envexpbot.2017.11.014
Gao K., Wu Y., Li G., Wu H., Villafane V. E., Helbling E. W. (2007). Solar UV Radiation Drives CO2 Fixation in Marine Phytoplankton: A Double-Edged Sword. Plant Physiol. 144, 54–59. doi: 10.1104/pp.107.098491
Gao K., Xu J., Gao G., Li Y., Hutchins D. A., Huang B., et al. (2012). Rising CO2 and Increased Light Exposure Synergistically Reduce Marine Primary Productivity. Nat. Climate Change 2, 519–523. doi: 10.1038/nclimate1507
Guillard R. R. L., Ryther J. H. (1962). tudies of Marine Planktonic Diatoms: I. Cyclotella Nana Hustedt, and Detonula Confervacea (Cleve) Gran. Can. J. Microbiol. 8, 229–239. doi: 10.1139/m62-029
Ha S.-Y., La H. S., Min J.-O., Chung K.-H., Kang S.-H., Shin K.-H. (2014). Photoprotective Function of Mycosporine-Like Amino Acids in a Bipolar Diatom (Porosira Glacialis): Evidence From Ultraviolet Radiation and Stable Isotope Probing. Diatom. Res. 29, 399–409. doi: 10.1080/0269249X.2014.894945
Hancock A. M., Davidson A. T., McKinlay J., McMinn A., Schulz K. G., Enden R. L. (2018). Ocean Acidification Changes the Structure of an Antarctic Coastal Protistan Community. Biogeosciences 15, 2393–2410. doi: 10.5194/bg-15-2393-2018
Harrison J. W., Smith R. E. (2011). The Spectral Sensitivity of Phytoplankton Communities to Ultraviolet Radiation-Induced Photoinhibition Differs Among Clear and Humic Temperate Lakes. Limnol. Oceanogr. 56, 2115–2126. doi: 10.4319/lo.2011.56.6.2115
Helbling E. W., Gao K., Gonçalves R. J., Wu H., Villafañe V. E. (2003). Utilization of Solar UV Radiation by Coastal Phytoplankton Assemblages Off SE China When Exposed to Fast Mixing. Mar. Ecol. Prog. Ser. 259, 59–66. doi: 10.3354/meps259059
Henley W. J. (1993). Measurement and Interpretation of Photosynthetic Light-Response Curves in Algae in the Context of Photoinhibition and Diel Changes. J. Phycol. 29, 729–739. doi: 10.1111/j.0022-3646.1993.00729.x
Hong H., Li D., Lin W., Li W., Shi D. (2017). Nitrogen Nutritional Condition Affects the Response of Energy Metabolism in Diatoms to Elevated Carbon Dioxide. Mar. Ecol. Prog. Ser. 567, 41–56. doi: 10.3354/meps12033
Hopkinson B. M., Dupont C. L., Allen A. E., Morel F. M. (2011). Efficiency of the CO2-Concentrating Mechanism of Diatoms. Proc. Natl. Acad. Sci. 108, 3830–3837. doi: 10.1073/pnas.1018062108
Hoppe C. J., Holtz L. M., Trimborn S., Rost B. (2015). Ocean Acidification Decreases the Light-Use Efficiency in an Antarctic Diatom Under Dynamic But Not Constant Light. New Phytol. 207, 159–171. doi: 10.1111/nph.13334
Hurd C. L., Beardall J., Comeau S., Cornwall C. E., Havenhand J. N., Munday P. L., et al. (2019). Ocean Acidification as a Multiple Driver: How Interactions Between Changing Seawater Carbonate Parameters Affect Marine Life. Mar. Freshw. Res. 71, 263–274. doi: 10.1071/MF19267
Key T., McCarthy A., Campbell D. A., Six C., Roy S., Finkel Z. V. (2010). Cell Size Trade-Offs Govern Light Exploitation Strategies in Marine Phytoplankton. Environ. Microbiol. 12, 95–104. doi: 10.1111/j.1462-2920.2009.02046.x
Kirk J. T. O. (1994). Light and Photosynthesis in Aquatic Ecosystems (Cambridge, UK: Cambridge University Press).
Li F., Fan J., Hu L., Beardall J., Xu J. (2019). Physiological and Biochemical Responses of Thalassiosira Weissflogii (Diatom) to Seawater Acidification and Alkalization. ICES J. Mar. Sci. 76, 1850–1859. doi: 10.1093/icesjms/fsz028
Li Y., Gao K., Villafañe V. E., Helbling E. W. (2012). Ocean Acidification Mediates Photosynthetic Response to UV Radiation and Temperature Increase in the Diatom Phaeodactylum Tricornutum. Biogeosciences 9, 3931–3942. doi: 10.5194/bg-9-3931-2012
Li F., Wu Y., Hutchins D. A., Fu F., Gao K. (2016). Physiological Responses of Coastal and Oceanic Diatoms to Diurnal Fluctuations in Seawater Carbonate Chemistry Under Two CO2 Concentrations. Biogeosciences 13, 6247–6259. doi: 10.5194/bg-13-6247-2016
Li F., Xu J., Beardall J., Gao K. (2021). Diurnally Fluctuating Pco2 Enhances Growth of a Coastal Strain of Emiliania Huxleyi Under Future-Projected Ocean Acidification Conditions. ICES J. Mar. Sci. 78, 1301–1310. doi: 10.1093/icesjms/fsab036
Li H., Xu T., Ma J., Li F., Xu J. (2021). Physiological Responses of Skeletonema Costatum to the Interactions of Seawater Acidification and the Combination of Photoperiod and Temperature. Biogeosciences 18, 1439–1449. doi: 10.5194/bg-18-1439-2021
Mengelt C., Prézelin B. B. (2005). UVA Enhancement of Carbon Fixation and Resilience to UV Inhibition in the Genus Pseudo-Nitzschia may Provide a Competitive Advantage in High UV Surface Waters. Mar. Ecol. Prog. Ser. 301, 81–93. doi: 10.3354/meps301081
Murphy T. M. (1983). Membranes as Targets of Ultraviolet Radiation. Physiol. Plantar. 58, 381–388. doi: 10.1111/j.1399-3054.1983.tb04198.x
Nahon S., Charles F., Lantoine F., Vétion G., Escoubeyrou K., Desmalades M., et al. (2010). Ultraviolet Radiation Negatively Affects Growth and Food Quality of the Pelagic Diatom Skeletonema Costatum. J. Exp. Mar. Biol. Ecol. 383, 164–170. doi: 10.1016/j.jembe.2009.12.006
Raven J. (2011). Effects on Marine Algae of Changed Seawater Chemistry With Increasing Atmospheric CO2. Biol. Envi.: Proc. R. Irish. Acad. 111, 1–17. doi: 10.3318/BIOE.2011.01
Raven J. A., Beardall J. (2014). CO2 Concentrating Mechanisms and Environmental Change. Aquat. Bot. 118, 24–37. doi: 10.1016/j.aquabot.2014.05.008
Rijstenbil J., Coelho S., Eijsackers M. (2000). A Method for the Assessment of Light-Induced Oxidative Stress in Embryos of Fucoid Algae via Confocal Laserscan Microscopy. Mar. Biol. 137, 763–774. doi: 10.1007/s002270000443
Ritchie R. J. (2006). Consistent Sets of Spectrophotometric Chlorophyll Equations for Acetone, Methanol and Ethanol Solvents. Photosynt. Res. 89, 27–41. doi: 10.1007/s11120-006-9065-9
Sabine C. L., Feely R. A., Gruber N., Key R. M., Lee K., Bullister J. L., et al. (2004). The Oceanic Sink for Anthropogenic CO2. Science 305, 367–371. doi: 10.1126/science.1097403
Salin M. L. (1988). Toxic Oxygen Species and Protective Systems of the Chloroplast. Physiol. Plantar. 72, 681–689. doi: 10.1111/j.1399-3054.1988.tb09182.x
Smith R. C., Prezelin B. B., Baker K. S., Bidigare R. R., Boucher N. P., Coley T., et al. (1992). Ozone Depletion: Ultraviolet Radiation and Phytoplankton Biology in Antarctic Waters. Science 255, 952–959. doi: 10.1126/science.1546292
Sobrino C., Ward M. L., Neale P. J. (2008). Acclimation to Elevated Carbon Dioxide and Ultraviolet Radiation in the Diatom Thalassiosira Pseudonana: Effects on Growth, Photosynthesis, and Spectral Sensitivity of Photoinhibition. Limnol. Oceanogr. 53, 494–505. doi: 10.4319/lo.2008.53.2.0494
Song Y., Qiu B. (2007). The CO2 Concentrating Mechanism in the Bloom-Forming Cyanobacterium Microcystis Aeruginosa (Cyanophyceae) and Effects of UVB Radiation on its Operation. J. Phycol. 43, 957–964. doi: 10.1111/j.1529-8817.2007.00391.x
Taucher J., Jones J., James A., Brzezinski M. A., Carlson C. A., Riebesell U., et al. (2015). Combined Effects of CO2 and Temperature on Carbon Uptake and Partitioning by the Marine Diatoms Thalassiosira Weissflogii and Dactyliosolen Fragilissimus. Limnol. Oceanogr. 60, 901–919. doi: 10.1002/lno.10063
Tortell P. D., Payne C. D., Li Y., Trimborn S., Rost B., Smith W. O., et al. (2008). CO2 Sensitivity of Southern Ocean Phytoplankton. Geophys. Res. Lett. 35, L04605. doi: 10.1029/2007GL032583
Tréguer P., Bowler C., Moriceau B., Dutkiewicz S., Gehlen M., Aumont O., et al. (2018). Influence of Diatom Diversity on the Ocean Biological Carbon Pump. Nat. Geosci. 11, 27–37. doi: 10.1038/s41561-017-0028-x
Williamson C. E., Zepp R. G., Lucas R. M., Madronich S., Austin A. T., Ballaré C. L., et al. (2014). Solar Ultraviolet Radiation in a Changing Climate. Nat. Climate Change 4, 434–441. doi: 10.1038/nclimate2225
Wu Y., Campbell D. A., Irwin A. J., Suggett D. J., Finkel Z. V. (2014). Ocean Acidification Enhances the Growth Rate of Larger Diatoms. Limnol. Oceanogr. 59, 1027–1034. doi: 10.4319/lo.2014.59.3.1027
Wu H., Gao K. (2009). Ultraviolet Radiation Stimulated Activity of Extracellular Carbonic Anhydrase in the Marine Diatom Skeletonema Costatum. Funct. Plant Biol. 36, 137–143. doi: 10.1071/FP08172
Keywords: growth, photosynthetic performances, seawater acidification, Skeletonema costatum, UV radiation
Citation: Li F, Li H, Xu T, Li S and Xu J (2022) Seawater Acidification Exacerbates the Negative Effects of UVR on the Growth of the Bloom-Forming Diatom Skeletonema costatum. Front. Mar. Sci. 9:905255. doi: 10.3389/fmars.2022.905255
Received: 26 March 2022; Accepted: 06 May 2022;
Published: 02 June 2022.
Edited by:
Yuanyuan Feng, Shanghai Jiao Tong University, ChinaReviewed by:
Zou Dinghui, South China University of Technology, ChinaZhiguang Xu, Ludong University, China
Copyright © 2022 Li, Li, Xu, Li and Xu. This is an open-access article distributed under the terms of the Creative Commons Attribution License (CC BY). The use, distribution or reproduction in other forums is permitted, provided the original author(s) and the copyright owner(s) are credited and that the original publication in this journal is cited, in accordance with accepted academic practice. No use, distribution or reproduction is permitted which does not comply with these terms.
*Correspondence: Juntian Xu, anR4dUBqb3UuZWR1LmNu
†These authors have contributed equally to this work and share first authorship