- Department of Integrative Biology, Michigan State University, East Lansing, MI, United States
Quaternary ammonium compounds (QACs) – e.g., betaines – have a chemical structure related to that of the tertiary sulfonium compounds (TSCs) – e.g., dimethylsulfoniopropionate – explaining why these two classes of coral metabolites are often studied and interpreted together. Functionally, both QACs and TSCs play important roles in the photobiology of reef-building corals under stress, according to recent hypotheses. The TSC dimethylsulfoniopropionate (DMSP) is the principal precursor of the gas dimethylsulfide (DMS) which is hypothesized to affect, through influences on cloud formation, the photon and thermal fluxes to which corals are exposed. Simultaneously, QACs – e.g., glycine betaine – in coral tissues are hypothesized to protect the zooxanthellae photosystems against photon and thermal stresses by exerting stabilizing effects on photosystem proteins and by ameliorating reactive-oxygen-species perturbations. This review, which synthesizes the most current available evidence on the relevant actions of QACs, emphasizes the need for enhanced direct study of QAC physiology in corals to ascertain the degree to which coral QACs exert photoprotective effects paralleling their well-established protective effects in plants.
Introduction
In a watershed paper, Rhodes and Hanson (1993) emphasized the logic and value of considering the tertiary sulfonium compounds (TSCs), notably dimethylsulfoniopropionate (DMSP), and the quaternary ammonium compounds (QACs) together because of their similarities in chemical structure and properties. Twenty-five years later, with far more data available, Somero et al. (2017) again affirmed the logic of emphasizing the shared properties of these two sets of small molecules, which they termed the methylsulfonium and methylammonium compounds – terms highlighting the key positions the compounds often occupy in methyl trafficking in cells. In this Special Issue on dimethylsulfide (DMS) in coral reefs, this paper on QACs is included not only because of the shared chemical properties of TSCs and QACs (Figure 1), but also because of important ways in which the two sets of compounds may interact in the ecological physiology of reef-building corals.
Glycine betaine is probably the most thoroughly studied QAC. Mäkelä et al. (2019) document that published research on glycine betaine has more than doubled in each successive decade over the past five decades, with more than 25,000 papers published in 2011-2020. Simultaneously, fewer than 20 papers have been published on glycine betaine or other QACs in reef-building corals over the entire five decades. In this paper, I argue that the near-total neglect of glycine betaine and other QACs in research on corals may represent a serious omission in the effort to conserve coral-reef ecosystems.
The betaines are one of the principal subsets of QACs. Structurally speaking, betaines are amino (or imino) acids fully methylated at the N position; there are in principle as many betaines as there are amino acids. Until a few decades ago, the most common betaine, glycine betaine, was typically called simply “betaine”. However, in this paper – and usually in the current scientific literature – it is called “glycine betaine” to specify the molecule and distinguish it from other betaines (although it is still called “betaine” in nontechnical contexts, such as ingredient lists for consumer products). In addition to glycine betaine, a number of other betaines, such as proline betaine and ß-alanine betaine, are commonly detected in vascular plants and algae when looked for (Rhodes and Hanson, 1993; McNeil et al., 1999). Trimethylamine N-oxide (TMAO) is a chemically related compound, long known in animals but only recently identified in plants (Catalá et al., 2021). All these compounds are zwitterionic, with a permanent positive charge at the N position: a structure that, as emphasized by Rhodes and Hanson (1993), is analogous to that of the TSC dimethylsulfoniopropionate, which is fully methylated at the S position and bears a permanent positive charge at that position (Figure 1).
A principal reason for glycine betaine to be of interest to coral biologists is its potential importance for photosynthesis. Already in 1995, Papageorgiou and Murata (1995) stressed the “unusually strong stabilizing effects of glycine betaine” on photosystem II in vascular plants. Other papers – such as those by Sakamoto and Murata (2002) and Allakhverdiev et al. (2003) – soon reinforced that message, helping to thrust glycine betaine into the center of research on photosynthesis under abiotic stress in vascular plants.
For discussing betaines and other QACs today, it is instructive to recognize the ways in which knowledge of the roles played by these compounds in photosynthetic organisms has expanded over the last several decades. In the 1970s and 1980s, glycine betaine was recognized principally as a compatible solute: an osmolyte that tended not to disturb cellular function (Yancey et al., 1982). As years passed, investigators identified additional roles, including glycine betaine’s stabilization of proteins in photosynthesis already alluded to (and addressed in detail later) and glycine betaine’s favorable effects (Chen and Murata, 2011) on reactive oxygen species (ROS) (Lesser, 2006). In the last few years, investigators have increasingly emphasized evidence that glycine betaine in addition can play signaling roles, as by directly or indirectly controlling gene expression (Figueroa-Soto and Valenzuela-Soto, 2018; Dutta et al., 2019; Hossain et al., 2019; Mäkelä et al., 2019; Valenzuela-Soto and Figueroa-Soto, 2019), and glycine betaine can modulate heat-shock protein expression (Li et al., 2011; Zhang et al., 2020). Today, therefore, when we consider glycine betaine and other QACs, we recognize that they may have broad ranges of action and be multifunctional (Derakhshani et al., 2017).
Betaines in Reef-Building Corals
Only about a decade has passed since publication of the first definitive information on betaines in the tissues of reef-building corals. The very first information seems to have been a report in 1976 by Moore and Huxley (1976) that crown-of-thorns seastars (Acanthaster planci) exhibit aversive behavior when exposed to a compound, tentatively identified as glycine betaine, in the tissues of certain reef-building corals. In 2010, Hill et al. (2010) and Yancey et al. (2010) used rigorous chemical methods [liquid chromatography/mass spectrometry (LC/MS) with internal standards in the case of Hill et al.] to quantify multiple betaines in the tissues of wild-collected, reef-building corals in the Caribbean (Hill et al., 2010) and at Hawaii (Yancey et al., 2010). Later, Hill et al. (2017), working mostly with wild-collected specimens, quantified multiple betaines in coral species in the western Pacific and also in five species of tridacnid clams (genera Tridacna and Hippopus), animals that inhabit Indo-Pacific coral-reef ecosystems and, like corals, live in symbioses with dinoflagellate symbionts. Swan et al. (2017a) also quantified multiple betaines in a set of wild-collected Great Barrier Reef branching corals.
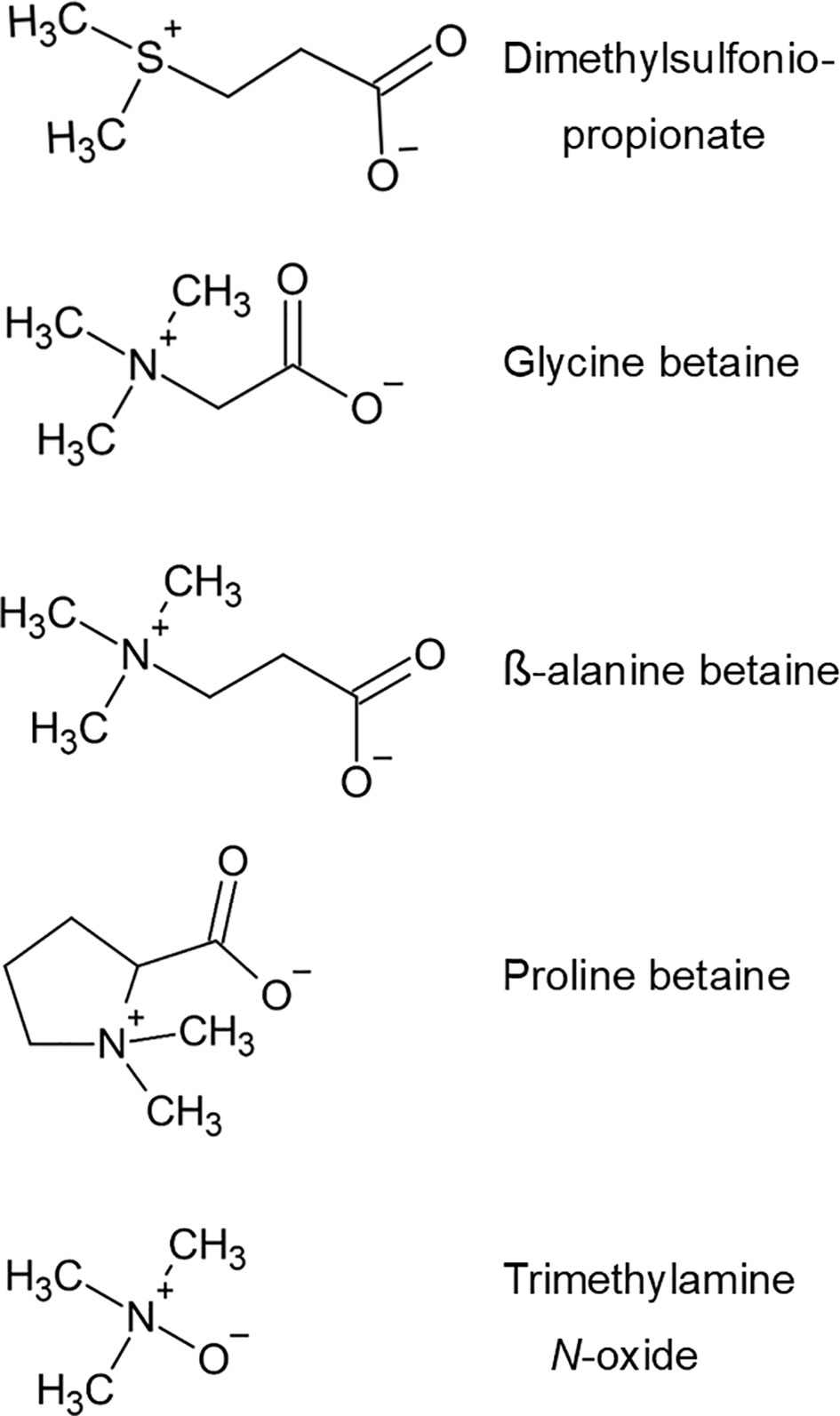
Figure 1 Chemical structures of five compounds that are discussed in this manuscript: dimethylsulfoniopropionate (DMSP), glycine betaine (often called simply “betaine” in the older literature); ß-alanine betaine (trimethylammonium propionate); proline betaine (stachydrine), and trimethylamine N-oxide (TMAO).
The evidence shows that betaines, despite their relative obscurity in corals, are abundant metabolites in the tissues of reef-building corals in the wild. Among the 10 species of Caribbean corals (6 genera) and the 6 species of western Pacific corals (5 genera), collected from the wild, that Hill et al. (2010; 2017) analyzed using LC/MS with internal standards, total betaine concentration was estimated to be 12-204 mmole per liter of living tissue (mean: 60 mM), except in one species, Acropora formosa, in which the total concentration was much lower (1.5 mM). An important point to stress is that these concentrations were calculated assuming a homogeneous distribution of the betaines in tissue. The true, operative concentrations of betaines in living tissue are likely substantially higher because betaines are probably, in fact, more concentrated in some subcellular regions than others. This same consideration applies also to other values reported.
Yancey et al. (2010), using a somewhat less sensitive analytical method than Hill et al. (2010; 2017), measured two betaines, glycine betaine and proline betaine, in 6 wild-collected species (4 genera) of Hawaiian reef-building corals and found total concentrations of about 9-69 mM (mean: 37 mM). Swan et al. (2017a), using a high-sensitivity method, focused on a restricted part of the anatomy, the branch tips, in 6 species of Acropora and one of Stylophora. Most of their estimates of total betaine concentration were 12-52 mM, although in A. valida, their estimate was lower, 6 mM.
Considering all studied coral species as a set, the two most abundant betaines in reef-building corals are glycine betaine and proline betaine. In the three studies that attempted to measure all betaines present in wild-collected corals (Hill et al., 2010; Hill et al., 2017; Swan et al., 2017a), glycine betaine and proline betaine together accounted for ≥90% of all betaines (on a molar basis) in 14 species, 58-88% in 8 species, and <50% in 1 species. Multiple additional betaines (e.g., alanine betaine, ß-alanine betaine, and hydroxyproline betaine) are also typically present in reef-building corals, some at just low concentrations. Different coral taxa sometimes differ substantially in the particular suite of betaines they express.
Ngugi et al. (2020) recently completed a genomic survey of pathways for glycine betaine synthesis, transport, and degradation in a wide sample of invertebrates, including reef-building corals. According to the genomic evidence, corals have pathways for the de novo synthesis of glycine betaine and for transport of glycine betaine into their tissues from the seawater environment. The transporters in particular have a restricted phylogenetic distribution within the invertebrates, suggesting a specialized role in corals and/or coral relatives. Strikingly, Ngugi et al. (2020) calculated that corals worldwide store sufficient glycine betaine to account for 16% of their tissue nitrogen.
Potential Protective Effects of Betaines in Reef-Building Corals: The Message from Physiological Data on Vascular Plants and Ecological Data on Corals
In corals, the biochemical and physiological actions of betaines have yet to be directly studied. However, the situation in vascular plants is quite opposite: By virtue of the massive effort to understand photosynthesis under abiotic stress in crop plants, a great deal is known about protective actions of glycine betaine. By applying the knowledge from vascular plants to reef-building corals, it seems highly reasonable to hypothesize that glycine betaine and other betaines are important photoprotective, protein- and membrane-stabilizing, compounds in corals. Should this hypothesis prove correct, manipulations of betaines (or other QACs) might be useful interventions for protecting reefs against threats of photoinhibition and bleaching (Gorbunov et al., 2001; Lesser, 2011; Frieler et al., 2013; Van Oppen and Lough, 2018), or for aiding recovery. Clearly, the tissue betaine concentrations measured in corals (reviewed in the previous section) are of sufficient magnitude for stabilization of protein and membrane functions, judging by studies in plants (e.g., Prasad and Saradhi, 2004; Shirasawa et al., 2006; Yang et al., 2007; Chen and Murata, 2011).
Before going further to explore these ideas, a few comments are appropriate regarding the fact that, at this time, although most research on the functions of betaines in photosynthetic organisms has been carried on one chemical species (glycine betaine), in the literature betaines are often discussed as a group: as a set of chemical species that, to a first approximation, have similar actions in protecting proteins and membranes. At the present time, there are two reasons for considering betaines as a group with common properties. First, several betaines in addition to glycine betaine, including ß-alanine betaine and proline betaine (Figure 1), have been demonstrated in at least limited ways to act as compatible solutes (Rhodes and Hanson, 1993; Bashir et al., 2014). Second, existing theories of betaine action emphasize molecular properties that are shared by betaines as a group rather than being highly specific to certain chemical species. Specifically, the protein- and membrane-stabilizing effects of betaines are attributed for the most part to influences that betaines noncovalently exert – by virtue of their fundamental chemical nature – on the structure of water in the immediate vicinity of protein molecules, enhancing thereby the extent to which native protein states are more favorable thermodynamically than nonnative states (McNeil et al., 1999; Bennion and Daggett, 2004; Street et al., 2006; Auton et al., 2011; Guinn et al., 2011; Bruździak et al., 2013; Roychoudhury et al., 2013). At the present state of knowledge, it seems parsimonious and reasonable to hypothesize that (1) all the principal betaines in corals exert, to a significant extent, similar protective actions in corals and (2) as a corollary, the total betaine concentration in tissues is likely a useful index of betaine functional significance. Of course, looking forward, a full functional understanding of betaines in corals will ultimately require direct empirical study of each specific chemical species.
For discussing betaines as potential protective compounds for photosynthesis in reef-building corals, two interacting lines of investigation on photosynthesis in vascular plants deserve particular attention: first, studies of photosystem II (PSII) and, second, studies of reactive oxygen species (ROS). Regarding PSII, abundant evidence exists that when corals are exposed to heat and photon stress sufficient to cause bleaching or photoinhibition, damaging effects are directly or indirectly exerted on PSII and/or the pathways of electron flow downstream from PSII in the algal symbionts of the corals (Warner et al., 1999; Fitt et al., 2001; Gorbunov et al., 2001; Jones and Hoegh-Guldberg, 2001; Hill et al., 2004; Lesser and Farrell, 2004; Weis, 2008; Warner and Suggett, 2016). Possibly the D1 protein (PsbA) in the PSII reaction center in coral symbionts – sometimes termed the Achilles heel of PSII (Weis, 2008) – is particularly affected (Warner et al., 1999; Hill et al., 2011; Warner and Suggett, 2016). As already emphasized, potential protective actions of glycine betaine or other QACs have not yet been directly studied in corals. However, in vascular plants and free-living algae that have been studied, glycine betaine has been demonstrated by many studies to protect PSII against a number of abiotic stresses, including photon stress and heat stress (Papageorgiou and Murata, 1995; Yang et al., 1996; Schiller and Dau, 2000; Sakamoto and Murata, 2002; Allakhverdiev et al., 2003; Klimov et al., 2003; Prasad and Saradhi, 2004; Hema et al., 2007; Yang et al., 2007; Allakhverdiev et al., 2008; Chen and Murata, 2011; Li et al., 2014; Wang et al., 2014; Kurepin et al., 2015; Huang et al., 2020; Li et al., 2021).
Regarding ROS, when corals are exposed to heat and photon stress sufficient to cause bleaching or photoinhibition, abundant evidence points to accumulation in the coral tissues of ROS, which are generally judged to be principal agents of photodamage (Lesser, 2006; Weis, 2008; Lesser, 2011; Oakley and Davy, 2018). Again, potential protective actions of glycine betaine or other QACs have not yet been directly studied in corals. Glycine betaine, however, has repeatedly been demonstrated to increase photosystem defenses against ROS in vascular plants (Prasad and Saradhi, 2004; Yang et al., 2007; Chen and Murata, 2011; Fan et al., 2012; Li et al., 2014; Gómez et al., 2019; Zhang et al., 2020; Li et al., 2021).
The research on glycine betaine in vascular plants has often had a very practical goal: to enhance the productivity of crop plants by capitalizing on glycine betaine’s beneficial properties. As part of this effort, glycine betaine has long occupied a focal position in the overall effort to employ genetic modification, or other deliberate manipulation, to offset the negative impacts on crop plants of a variety of abiotic stresses, including photon stress and heat stress (Alia et al., 1998; McNeil et al., 1999; Sakamoto and Murata, 2002; Prasad and Saradhi, 2004; Yang et al., 2007; Chen and Murata, 2011; Giri, 2011; Li et al., 2011; Li et al., 2014; Kurepin et al., 2015; Castiglioni et al., 2018; Annunziata et al., 2019; Dutta et al., 2019; Hasanuzzaman et al., 2019; Kido et al., 2019; Zhang et al., 2020; Niazian et al., 2021). Recent review papers provide overviews on the biology and manipulation of glycine betaine from this perspective (Kurepin et al., 2015; Annunziata et al., 2019; Hasanuzzaman et al., 2019; Huang et al., 2020; Zulfiqar et al., 2020).
Ecological studies on coral reefs bolster the hypothesis that glycine betaine and other betaines are important photoprotective compounds in reef-building corals. The ecological studies represent a second major line of investigation pointing in this direction, in addition to the extrapolations from physiological studies of vascular plants already discussed.
Hill et al. (2010) hypothesized that within a population of a species of reef-building corals subject to potential photon stress, if tissue betaines are in fact protective agents for algal symbiont function [by providing either direct protection to the algal cells or indirect protection (e.g., stabilization of the symbiosome membrane)], betaine concentrations would be expected to vary among individual coral colonies in direct relation to solar irradiance experienced. For testing this hypothesis, Hill et al. (2010) selected Madracis species in Curaçao reefs because the light relations of those species had previously been studied in exceptional detail (Vermeij and Bak, 2002). M. mirabilis occurs over a wide range of depths and almost always occupies fully exposed (unshaded) locations regardless of depth (Vermeij and Bak, 2002). With this information, Hill et al. (2010) hypothesized a priori that betaine concentrations in colonies of M. mirabilis vary inversely with depth of residence. In fact, when measured, the concentrations of glycine betaine and proline betaine (Figure 2) – and also alanine betaine and hydroxyproline betaine – were found to vary significantly with depth, being 37-94% higher in coral colonies at 5 m depth than ones at 20 m (Hill et al., 2010). Glycine betaine and proline betaine are the most abundant betaines in M. mirabilis. Another Madracis species, M. pharensis, occupies both fully exposed and highly shaded locations at most depths where it occurs (Vermeij and Bak, 2002). Hill et al. (2010) hypothesized a priori that, at any particular depth, betaine concentrations are higher in exposed colonies than in shaded ones. In fact, when M. pharensis colonies at a single depth (10 m) were studied (Hill et al., 2010), glycine betaine, proline betaine, and alanine betaine were found to be significantly higher in concentration (by 30-44%) in exposed colonies than in shaded ones. Glycine betaine and proline betaine are by far the most abundant betaines in M. pharensis.
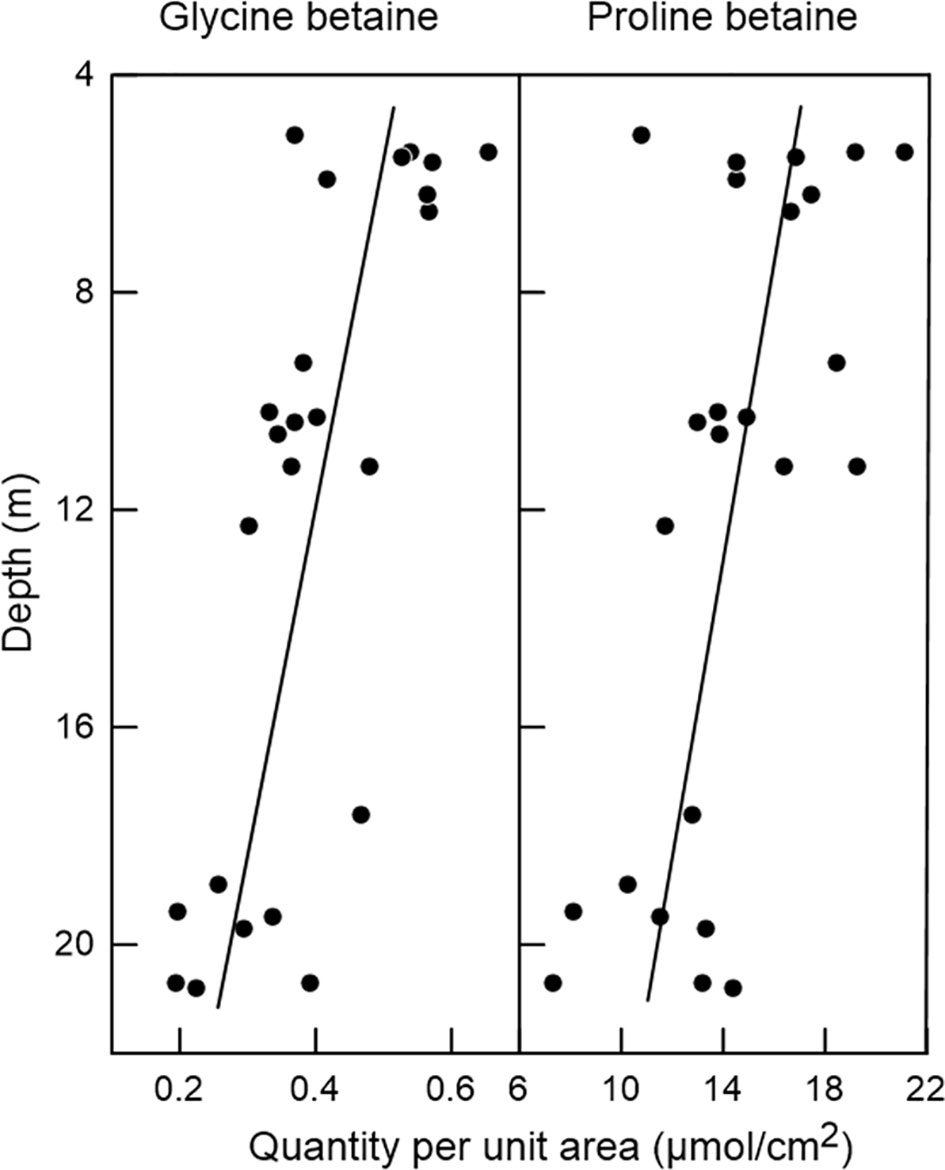
Figure 2 Abundance of glycine betaine and proline betaine in the tissues of Madracis mirabilis as a function of depth of residence. Each symbol is for a single, separate coral colony. All colonies grew in fully exposed, unshaded locations. Lines were fitted by least-squares regression. Based on ANOVA, differences among depths are statistically significant (p < 0.05). Betaine abundance is expressed as a ratio of the coral surface area sampled. (Data from Hill et al., 2010).
Reinforcing the ecological evidence for a protective role for glycine betaine in Madracis, Swan et al. (2017a) studied osmolyte concentrations in the branch tips of three Acropora species (A. aspera, A. millepora, and A. valida) during summer (February) and winter (August) in the Great Barrier Reef. They observed a 3.7- to 7.8-fold increase in average glycine betaine concentration in summer, compared with winter, in all three species: the pattern predicted if glycine betaine is employed in photoprotection. Although proline betaine was also abundant in these species, it exhibited just relatively small, inconsistent differences in concentration between the summer and winter samplings.
In summary, existing physiological and ecological evidence justifies a hypothesis that betaines play interacting photoprotective and ROS-protective roles in reef-building corals. In my view, recognizing the dire threats now faced by corals (Frieler et al., 2013), it would be logical to place a high priority on carrying out direct tests of this hypothesis in the near future (Hill et al., 2010; Hill et al., 2017). Lesser (2011) and others have emphasized that throughout the history of studies on photosynthesis in reef-building corals, investigators have made great progress by using prior studies on vascular plants and free-living algae as guides. Today, based on a large literature (Mäkelä et al., 2019), glycine betaine is well known in the study of vascular plants as a metabolite that exerts multiple effects – notably protein-stabilizing, membrane-stabilizing, and ROS-ameliorating effects – that help defend plants stressed by high irradiance, unusual temperatures, and other agents. Because this knowledge has been sought principally with the practical objective of defending crop plants against abiotic stress, many published attempts have been made – often with successful results – to manipulate glycine betaine to advantage, either by exogenous application or by genetic manipulation of endogenous synthesis (Alia et al., 1998; McNeil et al., 1999; Sakamoto and Murata, 2002; Prasad and Saradhi, 2004; Yang et al., 2007; Chen and Murata, 2011; Giri, 2011; Li et al., 2011; Li et al., 2014; Kurepin et al., 2015; Castiglioni et al., 2018; Annunziata et al., 2019; Dutta et al., 2019; Hasanuzzaman et al., 2019; Hossain et al., 2019; Huang et al., 2020; Zhang et al., 2020; Niazian et al., 2021). Perhaps this background of experience will prove useful for the protection of coral reefs confronted with photosystem and ROS stress (Van Oppen and Lough, 2018).
Just recently, trimethylamine N-oxide (TMAO; Figure 1) has been discovered to be widespread in vascular plants, and evidence from Arabidopsis and tomato indicates that TMAO, like betaines, plays roles in protein stabilization and tolerance of abiotic stress (Catalá et al., 2021). If TMAO proves to be present in reef-building corals, it also should be investigated along with betaines for actions that have potential to aid coral survival.
Physiological and Ecological Interactions Between the TSC System and the QAC System
If we assume for the moment that glycine betaine and other QACs exert protective effects in reef-building corals – paralleling their demonstrated protective effects in vascular plants – important potential interactions between QACs and TSCs in corals become apparent. These interactions could be manifest at an ecosystem level and/or within the tissues of particular coral polyps.
At an ecosystem level, long-term monitoring has revealed that the atmospheric concentration of DMS varies significantly in coral-reef ecosystems on hourly, daily, and seasonal time scales; and as the atmospheric DMS concentration varies, the atmospheric concentration of aerosols and the solar irradiance at sea level sometimes vary in correlated ways (Jackson et al., 2020a). At times when the atmospheric DMS concentration falls and the irradiance at sea level accordingly increases, corals could offset the increased risk of photosystem stress by upregulating glycine betaine or other QACs in their tissues. To assess the likelihood of this type of response, more research will be required on the time scales on which corals are able to modulate betaine expression.
At the level of an individual coral polyp, tissue QACs and TSCs seem likely interact in ameliorating ROS damage, including ROS-mediated damage to photosystem components such as PSII. As earlier noted, tissue glycine betaine has repeatedly been demonstrated to enhance defenses against ROS in vascular plants and free-living algae (Prasad and Saradhi, 2004; Hema et al., 2007; Yang et al., 2007; Chen and Murata, 2011; Fan et al., 2012; Gómez et al., 2019; Li et al., 2021). Similarly, DMSP and DMS are recognized as ROS scavengers in corals (Sunda et al., 2002; Lesser, 2006; Deschaseaux et al., 2014; Jones and King, 2015; Oakley and Davy, 2018; Jackson et al., 2020b). Thus both tissue QACs and TSCs likely function in the amelioration of ROS damage in corals, and they may be coordinated in various ways. To illustrate, corals have been observed to outgas DMS during air exposure or other stresses of low tide, when exposed or nearly exposed corals are subject to relatively high solar irradiance (Swan et al., 2017b). The corals might upregulate tissue betaines to compensate for any reduction in tissue DMS.
These briefly described hypothetical scenarios help to articulate the potential ways in which the QAC and TSC systems interact in the lives of reef corals and other photosynthetic reef inhabitants such as the tridacnid clams (Hill et al., 2017).
Conclusion
In the study of photosynthesis, of all the focal topics receiving frequent attention in vascular plants, the actions of betaines and other QACs are among the most neglected in reef-building corals. This neglect in corals is regrettable because corals are existentially threatened by abiotic stresses, and betaines attract interest in the study of crop plants precisely because of evidence that they can reduce the impact of abiotic stresses.
Recent research demonstrates that betaines (particularly glycine betaine and proline betaine) are abundant metabolites in the tissues of reef-building corals. Their functions in corals have not yet been directly studied. However, two lines of investigation – physiological and ecological – point to a hypothesis that betaines are photoprotective in corals. The physiological evidence focuses on the fact that when corals are subjected to heat and photon stresses sufficient to cause bleaching or photoinhibition, they experience damage to photosystems (e.g., PSII) and they accumulate photodamaging ROS: exigencies known to be ameliorated by glycine betaine in vascular plants. The ecological evidence is that when free-living corals have been examined to detect correlations between betaine abundance and solar irradiance, glycine betaine and (in some cases) other betaines have been observed to be significantly more concentrated in the tissues of coral colonies exposed to high irradiance than in conspecific colonies exposed to lower irradiance.
Betaines are not simple to study, in part because of the complexity of technologies available for quantification (e.g., LC/MS) and the challenges of calibration (Hill et al., 2010; Swan et al., 2017a). Nonetheless, a large body of evidence exists on their manipulation (e.g., exogenous application and genetic manipulation) in crop plants to defend against abiotic stresses, and with this background of experience, it is reasonable to hypothesize that they could be manipulated to aid the survival of corals exposed to heat and photon stresses.
Author Contributions
RH composed this paper in its entirety and prepared the figures.
Funding
No external funding was sought or provided for the preparation of this publication.
Conflict of Interest
The author declares that the research was conducted in the absence of any commercial or financial relationships that could be construed as a potential conflict of interest.
Publisher’s Note
All claims expressed in this article are solely those of the authors and do not necessarily represent those of their affiliated organizations, or those of the publisher, the editors and the reviewers. Any product that may be evaluated in this article, or claim that may be made by its manufacturer, is not guaranteed or endorsed by the publisher.
Acknowledgments
This paper is dedicated to the memory of Ron Kiene in recognition of his personal encouragement and his prescient contributions to the science of QACs and TSCs. John Dacey, Douglas Gage, and Wayne Hicks provided insights without which I would never have become interested in the betaines. Special thanks to the following colleagues who have played important roles in my direct studies of coral betaines: Eric Armstrong, Rolf Bak, Ahser Edward, Aaron Florn, Pedro Frade, Dan Jones, Chao Li, and Mark Vermeij. Mark Hahn and his lab at Woods Hole Oceanographic Institution continue to provide much-appreciated intellectual support. Thanks to ACD/Labs for their generosity in providing the freeware version of ChemSketch.
References
Alia, Hayashi H., Sakamoto A., Murata N. (1998). Enhancement of the Tolerance of Arabidopsis to High Temperatures By Genetic Engineering of the Synthesis of Glycinebetaine. Plant J. 16, 155–161. doi: 10.1046/j.1365-313x.1998.00284.x
Allakhverdiev S. I., Hayashi H., Nishiyama Y., Ivanov A. G., Aliev J. A., Klimov V. V., et al. (2003). Glycinebetaine Protects the D1/D2/Cytb559 Complex of Photosystem II Against Photo-Induced and Heat-Induced Inactivation. J. Plant Physiol. 160, 41–49. doi: 10.1078/0176-1617-00845
Allakhverdiev S. I., Kreslavski V. D., Klimov V. V., Los D. A., Carpentier R., Mohanty P. (2008). Heat Stress: An Overview of Molecular Responses in Photosynthesis. Photosynth. Res. 98, 541–550. doi: 10.1007/s11120-008-9331-0
Annunziata M. G., Ciarmiello L. F., Woodrow P., Dell’Aversana E., Carillo P. (2019). Spatial and Temporal Profile of Glycine Betaine Accumulation in Plants Under Abiotic Stress. Front. Plant Sci. 10, 230. doi: 10.3389/fpls.2019.00230
Auton M., Rösgen J., Sinev M., Holthauzen L. M. F., Bolen D. W. (2011). Osmolyte Effects on Protein Stability and Solubility: A Balancing Act Between Backbone and Side-Chains. Biophys. Chem. 159, 90–99. doi: 10.1016/j.bpc.2011.05.012
Bashir A., Hoffmann T., Kempf B., Xie X., Smits S. H. J., Bremer E. (2014). Plant-Derived Compatible Solutes Proline Betaine and Betonicine Confer Enhanced Osmotic and Temperature Stress Tolerance to Bacillus subtilis. Microbiology 160, 2283–2294. doi: 10.1099/mic.0.079665-0
Bennion B. J., Daggett V. (2004). Counteraction of Urea-Induced Protein Denaturation By Trimethylamine N-oxide: A Chemical Chaperone at Atomic Resolution. Proc. Natl. Acad. Sci. U. S. A. 101, 6433–6438. doi: 10.1073/pnas.0308633101
Bruździak P., Panuszko A., Stangret J. (2013). Influence of Osmolytes on Protein and Water Structure: A Step to Understanding the Mechanism of Protein Stabilization. J. Phys. Chem. B 117, 11502–11508. doi: 10.1021/jp404780c
Castiglioni P., Bell E., Lund A., Rosenberg A. F., Galligan M., Hinchey B. S., et al. (2018). Identification of GB1, A Gene Whose Constitutive Overexpression Increases Glycinebetaine Content in Maize and Soybean. Plant Direct 2018, 1–7. doi: 10.1002/pld3.40
Catalá R., López-Cobollo R., Berbis M. A., Jiménez-Barbero J., Salinas J. (2021). Trimethylamine N-oxide Is a New Plant Molecule That Promotes Abiotic Stress Tolerance. Sci. Adv. 7, eabd9296. doi: 10.1126/sciadv.abd9296
Chen T. H. H., Murata N. (2011). Glycinebetaine Protects Plants Against Abiotic Stress: Mechanisms and Biotechnological Applications. Plant Cell Environ. 34, 1–20. doi: 10.1111/j.1365-3040.2010.02232.x
Derakhshani Z., Malherbe F., Bhave M. (2017). Multifunctional Biomolecules With Roles in Abiotic Stress Tolerance as Well as Nutraceutical Potential. J. Plant Biochem. Biotechnol. 26, 121–131. doi: 10.1007/s13562-016-0372-8
Deschaseaux E. S. M., Jones G. B., Deseo M. A., Shepherd K. M., Kiene R. P., Swan H. B., et al. (2014). Effects of Environmental Factors on Dimethylated Sulfur Compounds and Their Potential Role in the Antioxidant System of the Coral Holobiont. Limnol. Oceanogr. 59, 758–768. doi: 10.4319/lo.2014.59.3.0758
Dutta T., Neelapu N. R. R., Wani S. H., Surekha C. (2019). “Role and Regulation of Osmolytes as Signaling Molecules to Abiotic Stress Tolerance,” in Plant Signaling Molecules. Role and Regulation Under Stressful Environments. Eds. Khan M. I. R., Reddy P. S., Ferrante A., Khan N. A. (Duxford: Elsevier), p. 459–477.
Fan W., Zhang M., Zhang H., Zhang P. (2012). Improved Tolerance to Various Abiotic Stresses in Transgenic Sweet Potato (Ipomoea batatas) Expressing Spinach Betaine Aldehyde Dehydrogenase. PloS One 7, e37344. doi: 10.1371/journal.pone.0037344
Figueroa-Soto C. G., Valenzuela-Soto E. M. (2018). Glycine Betaine Rather Than Acting Only as an Osmolyte Also Plays a Role as Regulator in Cellular Metabolism. Biochimie 147, 89–97. doi: 10.1016/j.biochi.2018.01.002
Fitt W. K., Brown B. E., Warner M. E., Dunne R. P. (2001). Coral Bleaching: Interpretation of Thermal Tolerance Limits and Thermal Thresholds in Tropical Corals. Coral Reefs 20, 51–65. doi: 10.1007/s003380100146
Frieler K., Meinschausen M., Golly A., Mengel M., Lebek K., Donner S. D., et al. (2013). Limiting Global Warming to 2°C Is Unlikely to Save Most Coral Reefs. Nat. Climate Change 3, 165–170. doi: 10.1038/nclimate1674
Giri J. (2011). Glycinebetaine and Abiotic Stress Tolerance in Plants. Plant Signal. Behav. 6, 1746–1751. doi: 10.4161/psb.6.11.17801
Gómez R., Vicino P., Carrillo N., Lodeyro A. F. (2019). Manipulation of Oxidative Stress Responses as a Strategy to Generate Stress-Tolerant Crops. From Damage to Signaling to Tolerance. Crit. Rev. Biotechnol. 39, 693–708. doi: 10.1080/07388551.2019.1597829
Gorbunov M. Y., Kolber Z. S., Lesser M. P., Falkowski P. G. (2001). Photosynthesis and Photoprotection in Symbiotic Corals. Limnol. Oceanogr. 46, 75–85. doi: 10.4319/lo.2001.46.1.0075
Guinn E. J., Pegram L. M., Capp M. W., Pollock M. N., Record M. T., Jr. (2011). Quantifying Why Urea Is a Protein Denaturant, Whereas Glycine Betaine Is a Protein Stabilizer. Proc. Nat. Acad. Sci. U. S. A. 108, 16932–16937. doi: 10.1073/pnas.1109372108
Hasanuzzaman M., Banerjee A., Bhuyan M. H. M. B., Roychoudhury A., Al Mahmud J., Fujita M. (2019). Targeting Glycinebetaine for Abiotioc Stress Tolerance in Crop Plants: Physiological Mechanism, Molecular Interaction and Signaling. Phyton. Intl. J. Exp. Bot. 88, 185–221. doi: 10.32604/phyton.2019.07559
Hema R., Senthil-Kumar M., Shivakumar S., Reddy P. C., Udayakumar M. (2007). Chlamydomonas reinhardtii, a Model System for Functional Validation of Abiotic Stress Responsive Genes. Planta 226, 655–670. doi: 10.1007/s00425-007-0514-2
Hill R. W., Armstrong E. J., Florn A. M., Li C., Walquist R. W., Edward A. (2017). Abundant Betaines in Giant Clams (Tridacnidae) and Western Pacific Reef Corals, Including Study of Coral Betaine Acclimatization. Mar. Ecol. Progr. Ser. 576, 27–41. doi: 10.3354/meps12181
Hill R., Brown C. M., DeZeeuw K., Campbell D. A., Ralph P. J. (2011). Increased Rate of D1 Repair in Coral Symbionts During Bleaching Is Insufficient to Counter Accelerated Photo-Inactivation. Limnol. Oceanogr. 56, 139–146. doi: 10.4319/lo.2011.56.1.0139
Hill R., Larkum A. W. D., Frankart C., Kühl M., Ralph P. J. (2004). Loss of Functional Photosystem II Reaction Centres in Zooxanthellae of Corals Exposed to Bleaching Conditions: Using Fluorescence Rise Kinetics. Photosynth. Res. 82, 59–72. doi: 10.1023/B:PRES.0000040444.41179.09
Hill R. W., Li C., Jones A. D., Gunn J. P., Frade P. R. (2010). Abundant Betaines in Reef-Building Corals and Ecological Indicators of a Photoprotective Role. Coral Reefs 29, 869–880. doi: 10.1007/s00338-010-0662-x
Hossain M. A., Kumar V., Burritt D. J., Fujita M., Mäkelä P. S. A. Eds. (2019). Osmoprotectant-Mediated Abiotic Stress Tolerance in Plants. Recent Advances and Future Perspectives. Recent advances and Future Perspectives (Cham: Springer Nature Switzerland).
Huang S., Zuo T., Ni W. (2020). Important Roles of Glycinebetaine in Stabilizing the Structure and Function of the Photosystem II Complex Under Abiotic Stresses. Planta 251, 36. doi: 10.1007/s00425-019-0330-z
Jackson R. L., Gabric A. J., Woodhouse M. T., Swan H. B., Jones G. B., Cropp R., et al. (2020a). Coral Reef Emissions of Atmospheric Dimethylsulfide and the Influence on Marine Aerosols in the Southern Great Barrier Reef, Australia. J. Geophys. Res. Atmos. 125, e2019JD031837. doi: 10.1029/2019JD031837
Jackson R. L., Gabric A. J., Cropp R., Woodhouse M. T. (2020b). Dimethylsulfide (DMS), Marine Biogenic Aerosols and the Ecophysiology of Coral Reefs. Biogeosciences 17, 2181–2204. doi: 10.5194/bg-17-2181-2020
Jones R. J., Hoegh-Guldberg O. (2001). Diurnal Changes in the Photochemical Efficiency of the Symbiotic Dinoflagellates (Dinophyceae) of Corals: Photoprotection, Photoinactivation and the Relationship to Coral Bleaching. Plant Cell Environ. 24, 89–99. doi: 10.1046/j.1365-3040.2001.00648.x
Jones G. B., King S. (2015). Dimethylsulphoniopropionate (DMSP) as an Indicator of Bleaching Tolerance in Scleractinian Corals. J. Mar. Sci. Eng. 3, 444–465. doi: 10.3390/jmse3020444
Kido E. A., Ferreira-Neto J. R. C., da Silva M. D., Santos V. E. P., Filho J. L. B. S., Benko-Iseppon A. M. (2019). “Osmoprotectant-Related Genes in Plants Under Abiotic Stress: Expression Dynamics, in Silico Genome Mapping, and Biotechnology,” in Osmoprotectant-Mediated Abiotic Stress Tolerance in Plants. Eds. Hossain M. A., Kumar V., Burritt D. J., Fujita M., Mäkelä P. S. A. (Cham: Springer Nature Switzerland), p. 1–40.
Klimov V. V., Allakhverdiev S. I., Nishiyama Y., Khorobrykh A. A., Murata N. (2003). Stabilization of the Oxygen-Evolving Complex of Photosystem II By Bicarbonate and Glycinebetaine in Thylakoid and Subthylakoid Preparations. Funct. Plant Biol. 30, 797–803. doi: 10.1071/FP03068
Kurepin L. V., Ivanov A. G., Zaman M., Pharis R. P., Allakhverdiev S. I., Hurry V., et al. (2015). Stress-Related Hormones and Glycinebetaine Interplay in Protection of Photosynthesis Under Abiotic Stress Conditions. Photosyn. Res. 126, 221–235. doi: 10.1007/s11120-015-0125-x
Lesser M. P. (2006). Oxidative Stress in Marine Environments: Biochemistry and Physiological Ecology. Annu. Rev. Physiol. 68, 253–278. doi: 10.1146/annurev.physiol.68.040104.110001
Lesser M. P. (2011). “Coral Bleaching: Causes and Mechanisms,” in Coral Reefs: An Ecosystem in Transition. Eds. Dubinsky Z., Stambler N. (New York: Springer), 405–419.
Lesser M. P., Farrell J. H. (2004). Exposure to Solar Radiation Increases Damage to Both Host Tissues and Algal Symbionts of Corals During Thermal Stress. Coral Reefs 23, 367–377. doi: 10.1007/s00338-004-0392-z
Li M., Li Z., Li S., Guo S., Meng Q., Li G., et al. (2014). Genetic Engineering of Glycine Betaine Biosynthesis Reduces Heat-Enhanced Photoinhibition By Enhancing Antioxidative Defense and Alleviating Lipid Peroxidation in Tomato. Plant Mol. Biol. Rep. 32, 42–51. doi: 10.1007/s11105-013-0594-z
Li S., Li F., Wang J., Zhang W., Meng Q., Chen T. H. H., et al. (2011). Glycinebetaine Enhances the Tolerance of Tomato Plants to High Temperature During Germination of Seeds and Growth of Seedlings. Plant Cell Environ. 34, 1931–1943. doi: 10.1111/j.1365-3040.2011.02389.x
Li D., Wang M., Zhang T., Chen X., Li C., Liu Y., et al. (2021). Glycinebetaine Mitigated the Photoinhibition of Photosystem II at High Temperature in Transgenic Tomato Plants. Photosyn. Res. 147, 301–315. doi: 10.1007/s11120-020-00810-2
Mäkelä P. S. A., Jokinen K., Himanen K. (2019). “Roles of Endogenous Glycinebetaine in Plant Abiotic Stress Responses”, in Osmoprotectant-Mediated Abiotic Stress Tolerance in Plants. Eds. Hossain M. A., Kumar V., Burritt D. J., Fujita M., Mäkelä P. S. A. (Cham: Springer Nature Switzerland), 153–173.
McNeil S. D., Nuccio M. L., Hanson A. D. (1999). Betaines and Related Osmoprotectants. Targets for Metabolic Engineering of Stress Resistance. Plant Physiol. 120, 945–949. doi: 10.1104/pp.120.4.945
Moore R. J., Huxley C. J. (1976). Aversive Behaviour of Crown-of-Thorns Starfish to Coral Evoked By Food-Related Chemicals. Nature 263, 407–409. doi: 10.1038/263407a0
Ngugi D. K., Ziegler M., Duarte C. M., Voolstra C. R. (2020). Genomic Blueprint of Glycine Betaine Metabolism in Coral Metaorganisms and Their Contribution to Reef Nitrogen Budgets. iScience 23, 101120. doi: 10.1016/j.isci.2020.101120
Niazian M., Sadat-Noori S. A., Tohidfar M., Mortazavian S. M. M., Sabbatini P. (2021). Betaine Aldehyde Dehydrogenase (BADH) vs. flavodoxin (Fld): Two Important Genes for Enhancing Plants Stress Tolerance and Productivity. Front. Plant Sci. 12, 650215. doi: 10.3389/fpls.2021.650215
Oakley C. A., Davy S. K. (2018). “Cell Biology of Coral Bleaching,” in Coral Bleaching. Patterns, Processes, Causes and Consequences, 2nd ed. Eds. Van Oppen M. J. H., Lough J. M. (Cham: Springer International Publishing), 189–211.
Papageorgiou G. C., Murata N. (1995). The Unusually Strong Stabilizing Effects of Glycine Betaine on the Structure and Function of the Oxygen-Evolving Photosystem II Complex. Photosynth. Res. 44, 243–252. doi: 10.1007/BF00048597
Prasad K. V. S. K., Saradhi P. P. (2004). Enhanced Tolerance to Photoinhibition in Transgenic Plants Through Targeting of Glycinebetaine Biosynthesis Into the Chloroplasts. Plant Sci. 166, 1197–1212. doi: 10.1016/j.plantsci.2003.12.031
Rhodes D., Hanson A. D. (1993). Quaternary Ammonium and Tertiary Sulfonium Compounds in Higher Plants. Annu. Rev. Plant Physiol. Plant Mol. Biol. 44, 357–384. doi: 10.1146/annurev.pp.44.060193.002041
Roychoudhury A., Bieker A., Häussinger D., Oesterhelt F. (2013). Membrane Protein Stability Depends on the Concentration of Compatible Solutes –A Single Molecule Force Spectroscopic Study. Biol. Chem. 394, 1465–1474. doi: 10.1515/hsz-2013-0173
Sakamoto A., Murata N. (2002). The Role of Glycine Betaine in the Protection of Plants From Stress: Clues From Transgenic Plants. Plant Cell Environ. 25, 163–171. doi: 10.1046/j.0016-8025.2001.00790.x
Schiller H., Dau H. (2000). Preparation Protocols for High-Activity Photosystem II Membrane Particles of Green Algae and Higher Plants, pH Dependence of Oxygen Evolution and Comparison of the S2-State Multiline Signal By X-Band EPR Spectroscopy. J. Photochem. Photobiol. B Biol. 55, 138–144. doi: 10.1016/S1011-1344(00)00036-1
Shirasawa K., Takabe T., Takabe T., Kishitani S. (2006). Accumulation of Glycinebetaine in Rice Plants That Overexpress Choline Monooxygenase From Spinach and Evaluation of Their Tolerance to Abiotic Stress. Ann. Bot. 98, 565–571. doi: 10.1093/aob/mcl126
Somero G. N., Lockwood B. L., Tomanek L. (2017). Biochemical Adaptation. Response to Environmental Challenges From Life’s Origins to the Anthropocene (Sunderland, MA: Sinauer Associates).
Street T. O., Bolen D. W., Rose G. D. (2006). A Molecular Mechanism for Osmolyte-Induced Protein Stability. Proc. Natl. Acad. Sci. USA 17064, 13997–14002. doi: 10.1073/pnas.0606236103
Sunda W., Kieber D. J., Kiene R. P., Huntsman S. (2002). An Antioxidant Function for DMSP and DMS in Marine Algae. Nature 418, 317–320. doi: 10.1038/nature00851
Swan H. B., Deschaseaux E. S. M., Jones G. B., Eyre B. D. (2017a). The Relative Abundance of Dimethylsulfoniopropionate (DMSP) Among Other Zwitterions in Branching Coral at Heron Island, Southern Great Barrier Reef. Anal. Bioanal. Chem. 409, 4409–4423. doi: 10.1007/s00216-017-0385-8
Swan H. B., Jones G. B., Deschaseaux E. S. M., Eyre B. D. (2017b). Coral Reef Origins of Atmospheric Dimethylsulfide at Heron Island, Southern Great Barrier Reef, Australia. Biogeosciences 14, 229–239. doi: 10.5194/bg-14-229-2017
Valenzuela-Soto E. M., Figueroa-Soto C. G. (2019). “Biosynthesis and Degradation of Glycine Betaine and Its Potential to Control Plant Growth and Development,” in Osmoprotectant-Mediated Abiotic Stress Tolerance in Plants. Eds. Hossain M. A., Kumar V., Burritt D. J., Fujita M., Mäkelä P. S. A. (Cham: Springer Nature Switzerland), 123–140.
Van Oppen M. J. H., Lough J. M. Eds (2018). Coral Bleaching. Patterns, Processes, Causes and Consequences. 2nd ed (Cham: Springer International Publishing).
Vermeij M. J. A., Bak R. P. M. (2002). How Are Coral Populations Structured By Light? Marine Light Regimes and the Distribution of Madracis. Mar. Ecol. Prog. Ser. 233, 105–116. doi: 10.3354/meps233105
Wang Y., Liu S., Zhang H., Zhao Y., Zhao H., Liu H. (2014). Glycine Betaine Application in Grain Filling Wheat Plants Alleviates Heat and High Light-Induced Photoinhibition By Enhancing the psbA Transcription and Stomatal Conductance. Acta Physiol. Plant 36, 2195–2202. doi: 10.1007/s11738-014-1596-7
Warner M. E., Fitt W. K., Schmidt G. W. (1999). Damage to Photosystem II in Symbiotic Dinoflagellates: A Determinant of Coral Bleaching. Proc. Natl. Acad. Sci. U. S. A. 96, 8007–8012. doi: 10.1073/pnas.96.14.8007
Warner M. E., Suggett D. J. (2016). “The Photobiology of Symbiodinium spp.: Linking Physiological Diversity to the Implications of Stress and Resilience,” in The Cnidaria, Past, Present and Future. Eds. Goffredo S., Dubinsky Z. (Cham: Springer Nature Switzerland), 489–509.
Weis V. M. (2008). Cellular Mechanisms of Cnidarian Bleaching: Stress Causes the Collapse of Symbiosis. J. Exp. Biol. 211, 3059–3066. doi: 10.1242/jeb.009597
Yancey P. H., Clark M. E., Hand S. C., Bowlus R. D., Somero G. N. (1982). Living With Water Stress: Evolution of Osmolyte Systems. Science 217, 1214–1222. doi: 10.1126/science.7112124
Yancey P. H., Heppenstall M., Ly S., Andrell R. M., Gates R. D., Carter V. L., et al. (2010). Betaines and Dimethysulfoniopropionate as Major Osmolytes in Cnidaria With Endosymbiotic Dinoflagellates. Physiol. Biochem. Zool. 83, 167–173. doi: 10.1086/644625
Yang G., Rhodes D., Joly R. J. (1996). Effects of High Temperature on Membrane Stability and Chlorophyll Fluorescence in Glycinebetaine-Deficient and Glycinebetaine-Containing Maize Lines. Aust. J. Plant Physiol. 23, 437–443. doi: 10.1071/PP9960437
Yang X., Wen X., Gong H., Lu Q., Yang Z., Tang Y., et al. (2007). Genetic Engineering of the Biosynthesis of Glycinebetaine Enhances Thermotolerance of Photosystem II in Tobacco Plants. Planta 225, 719–733. doi: 10.1007/s00425-006-0380-3
Zhang T., Li Z., Li D., Li C., Wei D., Li S., et al. (2020). Comparative Effects of Glycinebetaine on the Thermotolerance in codA- and BADH-Transgenic Tomato Plants Under High Temperature Stress. Plant Cell Rep. 39, 1525–1538. doi: 10.1007/s00299-020-02581-5
Keywords: abiotic stress, betaines, bleaching, glycine betaine, osmolytes, photon stress, photosynthesis, Tridacnidae
Citation: Hill RW (2022) Quaternary Ammonium Compounds as Candidate Photoprotective Compounds in Reef-Building Corals. Front. Mar. Sci. 9:869739. doi: 10.3389/fmars.2022.869739
Received: 04 February 2022; Accepted: 30 March 2022;
Published: 24 May 2022.
Edited by:
Graham Barry Jones, Southern Cross University, AustraliaCopyright © 2022 Hill. This is an open-access article distributed under the terms of the Creative Commons Attribution License (CC BY). The use, distribution or reproduction in other forums is permitted, provided the original author(s) and the copyright owner(s) are credited and that the original publication in this journal is cited, in accordance with accepted academic practice. No use, distribution or reproduction is permitted which does not comply with these terms.
*Correspondence: Richard W. Hill, aGlsbHJAbXN1LmVkdQ==