- 1College of Fisheries, Guangdong Ocean University, Zhanjiang, China
- 2Guangxi Key Laboratory of Beibu Gulf Marine Biodiversity Conservation, Beibu Gulf University, Qinzhou, China
Molting behavior in insects is controlled by the ecdysis triggering hormone (ETH), eclosion hormone (EH) and the crustacean cardioactive peptide (CCAP). At present, the regulation of molting behavior in crustaceans remains unclear. Here, we studied the roles of ETH, EH, and CCAP in the molt regulation of the crab, Scylla paramamosain from their expression pattern and in vivo assays. The results showed that transcripts of ETH, EH, and CCAP were mainly localized in thoracic ganglia and fluctuated periodically with the molting cycle. When ETH or CCAP was knockdown at early premolt stage (D0), molting of crabs was interrupted and all animals died at late premolt stage (D2). While the EH gene was knock-down, most crabs were dead before D2. Injection of synthetic peptide for ETH or CCAP rescued ETH- or CCAP-gene knock-down crabs separately. However, none of peptides could rescue dsEH-injected crabs. At D0 stage, knockdown of ETH down-regulated the transcriptions of EH and CCAP; while ETH was up-regulated when EH was knockdown. At D2 stage, ETH transcripts levels were reduced with the injection of dsEH but increased with the same dose of dsETH as crabs at D0 stage. Co-injection of dsETH and dsEH down-regulated ETH at D2 stage. Results showed that ETH, EH, and CCAP play essential but different roles in molt regulation in mud crab. In summary, the result of this study contributes to the discovery of different molecular mechanisms between Insecta and Crustacea and may provide insight to develop fishery drugs that helps aquacultured crustaceans to molt successfully.
Introduction
Insects and crustaceans share a number of similar hormones in the regulation of molting and reproduction. During the molting of insects, many neuropeptides related to molting were regulated by precise change of ecdysteroid titer to prepare for the event (Zitnan and Adams, 2012). These neuropeptides include the ecdysis triggering hormone (ETH), eclosion hormone (EH) and crustacean cardioactive peptide (CCAP), etc. (Song et al., 2017). These hormones are released in large quantities, which in turn initiates the onset of molting behavior (Zieger et al., 2021). However, whether these neuropeptides have similar functions and regulation modes in crustaceans is still unknown. Molting in crustacean is under the tight endocrine control of many hormones. Among those hormones, the steroid hormone ecdysone is the major hormone known to initiate the molting process (Claeys et al., 2006). Therefore, an increase of ecdysteroid titer in the hemolymph is needed for the start of the molting process. A series of ecdysis-related genes are expressed under the influence of cyclical fluctuations of ecdysone, followed by the binding with nuclear receptors to transmit ecdysis signals, so that the crustaceans complete the pre-molting preparations on time and accurately (Zhao, 2020). When molting begin, the initiation of molting behavior occurs (Song et al., 2017). Previous studies have shown that arthropods mainly initiate molting behavior at the premolt stage (D) (Oliphant et al., 2018; Mykles and Chang, 2020). Even the initiation of the molting process has started, there is no guarantee that the animal will molt successfully as the fulfillment of the subsequent events must be straightly followed. In insects, neuropeptides that induce molting behavior include ecdysis-triggering hormone (ETH) (Shi et al., 2019a; Minh Nhut et al., 2020; Shen et al., 2021), eclosion hormone (EH) (Hull et al., 2009; Zhou et al., 2017; Scott et al., 2020), crustacean cardioactive peptide (CCAP) (Veelaert et al., 1997; Arakane et al., 2008; Jackson et al., 2009), etc.
Eclosion hormones (EH) is a neuropeptide that influences several aspects of pupal-adult ecdysis as well as larval-larval ecdysis (Hull et al., 2009; Scott et al., 2020). It was first characterized in the moth Bombyx mori as a brain neuropeptide (Truman and Riddiford, 1970). After its synthesis, EH was transported to the targets via hemolymph. The release of EH from the brain is controlled by a circadian clock in the brain and declining ecdysteroid titers (Truman and Riddiford, 1970). In the targets, signal transduction occurred via membrane receptors and through the action secondary messengers. As a result, the process of metamorphosis was initiated (Morton and Simpson, 2002). Although the crustaceans “EH” (decapod possess two of them, again entirely structurally conserved) has significant similarities to those of insects, homology with respect to function in arthropods is premature. ETH was first discovered in the moth Manduca sexta. It was produced from the secretion of the endocrine gland located in the trachea. ETH can directly stimulate ecdysis (Zitnan et al., 1996) and the larva responds much faster after they were injected as compared to EH. ETH is secreted by Inka cells of the trachea and it shared strong structural and functional conservation among a variety of insects (Adams and Zitnan, 1997; Mesce and Fahrbach, 2002; Park et al., 2002; Zitnan et al., 2003). Most insect ETH genes can express a variety of mature peptides through alternative splicing. The Lepidoptera can produce a pre-ecdysis triggering hormone (PETH) and ETH. PETH triggers early molt regulation, then ETH initiation of late molting regulation and molting behavior (Park et al., 1999, 2002); whereas, most other insects produce two mature peptides, ETH1 and ETH2, both of which are encoded by the same gene of ETH. For example, in Drosophila melanogaster, ETH1 is even more critical in the control of molting behavior (Zitnan et al., 1999). ETH plays a role by binding to its cell membrane receptor ETHR. The gene of ETHR can also encode two receptor subtypes ETHR-A and ETHR-B through alternative splicing and also with functional differentiation. In Bactrocera dorsalis, binding of ETH to the receptor ETHR-A will form a complex which in turn trigger ecdysis behavior. However, when ETH interact with ETHR-B, the complex trigger the process of reproduction regulation (Shi et al., 2017, 2019a). ETH in crustaceans, which was firstly identified as “carcikinin/ETH” in the crab Carcinus maenas (Oliphant et al., 2018), has some similarities with insect ETH, with respect to the C-terminal motif PRI/L-a. Through comparative transcriptomic study of ETH from different crustaceans, it was revealed that two splicing variants of ETH were present in the shrimp Litopenaeus vannamei. However, only one transcript was reported in the fresh water shrimp Macrobrachium rosenbergii, the crabs Eriocheir sinensis and Scylla paramamosain, the crayfish Procambarus clarkii so far. In crayfish P. clarkii, molting was delayed after they were injected with ETH (Minh Nhut et al., 2020), which was different to insects (Arakane et al., 2008; Lenaerts et al., 2017). Also, crustacean express ETH mainly in the nervous system rather than trachea, and its expression pattern increases dramatically in very late premolt (Veenstra, 2016; Oliphant et al., 2018). The situation differs quite markedly in insects where the authentic ETH is only expressed much earlier in the ecdysis program by non-neuronal Inka cells. The amino acid sequences of most decapod “carcikinin/ETH” is identical. To date, there is no published information on the neural architecture of either of these peptides, only expression patterns in the CNS. Also they are produced as secreted circulating neurohormones. Whether the functions of ETH in crustaceans undergo functional differentiation required more research. CCAP is mainly produced in the nervous tissues of both insects and crustaceans (Veenstra, 2016). It has been confirmed that they have different functions on various physiological processes in insects and crustaceans (Gammie and Truman, 1999; Kim et al., 2006; Fort et al., 2007). Whereas what function does CCAP act in the molting of crabs such as S. paramamosain is still unknown. In aquaculture of the crab S. paramamosain, failure of molting was commonly occured that result in an increase in mortality and causes losses to the aquaculture industry. To date, the release patterns, endocrine cascades and titers of the neurohormones such as ETH, EH, CCAP in crustaceans are still unclear. The detailed mechanism that ETH, EH, and CCAP affect molt behavior and the interrelationship of the hormones in the molt of S. paramamosain need further research, which may help to improve the economic benefits of aquaculture.
In this study, we have monitored the expression of several key molting-related genes ETH, EH and CCAP that induce the molting process the crab (Fort et al., 2007; Hull et al., 2009; Shen et al., 2021). Also, we have developed in vivo assays in juvenile crabs during the premolt stage of the molting crab. The irreplaceable role in Scylla molting and the mutual regulatory relationship have further verified that ETH and EH also have different regulatory effects on each other at different stages, which have different roles in different insects.
Materials and Methods
Animals
Crabs were collected from coastal area of Zhanjiang, Guangdong Province, China. Individuals (i.e., 2.1–3.4 g) were selected and stocked in 1 m3 concrete ponds. They were cultured at 24–27°C, salinity at 20‰ and fed daily with oyster. After a week, healthy crabs at D0 (i.e., 2.4–2.9 g) were selected for molting experiment, healthy crabs at D0 and D2 stages (Ong, 1966) were selected for dsRNA-injected experiments.
Tissue Collection, RNA Preparation and cDNA Synthesis
Crabs were chilled on ice before dissection for tissues. For every tissue that was analyzed, samples were collected in three different pools of five dissected animals each and stored at −80°C until further processing. Total RNA preparation was prepared using a column-based TransZol Up Plus RNA kit (TransGen Biotech, China). After elution, the concentration of total RNA was determined using the Thermo Fisher Scientific the NanoDrop 2000 (Waltham, MA, United States) and the quality of total RNA was tested by 1% agarose gel. For synthesis of first strand cDNA, 5 × All-In-One RT cDNA synthesis kit (ABM Inc., Richmond, BC, Canada) was used. The cDNAs were diluted tenfold for later analyses.
qT-PCR Determination of Transcript Contents
The open reading frame of ETH (SRR3086589, SRR3086590), EH (GeneBank accession No: KR078366.1) and CCAP (GeneBank accession No: MN923209.1) were obtained from NCBI Database (Supplementary Figures 1–3). Primers were designed using the Software Primer 5.0 (Supplementary Table 1) and 18s rRNA and β-actin were used as internal control genes (Chung and Lin, 2006; Liu et al., 2020). Each qPCR reaction contained 10 μL of SYBR qPCR Master Mix (Vazyme, Nanjing, China), 2 μL of cDNA, 7 μL of ultrapure water and 0.5 μL of each Forward and Reverse primer (10 μM). PCR was performed with the Real Time PCR machines (Bio-Rad CFX Connect PCR, Bio-Rad, United States). The following thermal cy-cling profile was applied: 95°C for 2 min, followed by 39 cycles of 95°C for 5 s and 55°C for 30 s, and then a melt curve analysis was performed to verify the specificity of the qRT-PCR reactions. The relative mRNA abundance of each gene was calculated by ΔΔCt method, then normalized by that of 18S and β-actin for each sample. The reaction was performed in triplicated for each sample. All primers used in the present study were given in Supplementary Table 1.
RNAi and Rescue Experiments
Firstly, primers flanked by the T7 promoter sequence were designed and used to synthesize the dsRNA for EH, ETH, CCAP and control gene (GFP). Secondly, the dsRNA template for each gene were amplified (PCR amplification procedure: 95°C for 5 min; followed by 30 cycles of 95°C for 30 s, 57°C for 30 s and 72°C for 30 s; then 72°C for 10 min) with above primers and purified by means of FastPure Gel DNA Extraction Mini Kit (Vazyme, Nanjing, China). After the concentration detection of DNA tem-plates (NanoDrop 2000, Thermo Fisher Scientific, Inc., Waltham, MA, United States). dsRNA was produced with DNA templates under the method of T7 RiboMAX™ Express RNAi Synthesis kit (Promega, Beijing, China). The final dsRNA was diluted to appropriate concentration (1 μg/μL) with water. Synthetic peptides of ETH and CCAP (Oliphant et al., 2018) were synthesized by GenScript (Nanjing, China) and dissolved in water to 1 μg/μL.
RNA interference experiments (n = 50) were done with the injection of dsEH, dsETH, dsCCAP, and dsGFP, respectively. Each crab was injected in dsRNA at a dose of 5 μg/g. For the synthetic peptide compensation experiments, each of dsEH, dsETH and dsCCAP were co-injected with ETH and CCAP synthetic peptides, respectively, and each co-injected combination named a group (n = 50). Both dsRNA and synthetic peptide were injected at a dose of 5 μg/g, which was an over-added level of synthetic peptides in case of inadequate compensation to the negative effects of gene knockdown. Control group was injected with dsGFP following the same injected dose. Boost injections were given every 5 days to ensure a lasting affection of gene mRNA levels. The number of deaths and molts in each group were counted daily, also the stage of any deaths were observed by microscope. Crabs injected with dsGFP were served as control.
Crabs (n = 20) at D0 and D2 stages were collected and separately injected with dsEH and dsETH at a dose of 5 μg/g at each stage. Also, crabs (n = 20) at D2 stage were selected for co-injection of dsEH and dsETH at a dose of 5 μg/g for each dsRNA. After 48 h, the high-expression tissues of nerves were sampled and analyzed. Crabs injected with dsGFP were served as control.
Statistical Analysis
The relative transcriptional levels of ETH, EH, and CCAP were calculated using 2–ΔΔCt method. All data were expressed as means ± standard deviation (SD) and analyzed by GraphPad Prism8.0.1 (GraphPad Software Inc., San Diego, CA, United States). Statistical analysis was performed using one-way ANOVA and followed by Tukey’s analysis method with significant difference (P < 0.05). The differences on transcriptional level of genes between treatments and control groups were analyzed by Student’s t test (P < 0.05). For the analyses of molting rates, the log-rank (Mantel-Cox) test was performed and followed by Student’s t-test with Welch’s correction (P < 0.05).
Results
Expression Patterns of Ecdysis Triggering Hormone, Eclosion Hormone, and Crustacean Cardioactive Peptide in Juvenile Crab
Quantitative real-time PCR (qRT-PCR) results indicated that ETH expression level was the highest in the thoracic ganglion, followed by that in the eyestalks and brain of juvenile crabs (Figure 1A). EH transcripts were mainly localized in the neuronal tissues, and the level was the highest in the thoracic ganglia, followed by the brain and the eyestalks (Figure 1B). CCAP transcript level was the highest in the thoracic ganglia (Figure 1C). A progressive decrease of ETH transcript level was observed from post-molt stages (i.e., A and B) toward the early premolt stage (D0). However, a 100-folds increase in ETH transcript level occurred at the late premolt stage (D2) (Figure 2A). The transcript levels of EH and CCAP at A, B, C, and D0 stages have little changes until the sharp increase at D2 and reach the maximum level at the ecdysis stage (E) (Figures 2B,C).
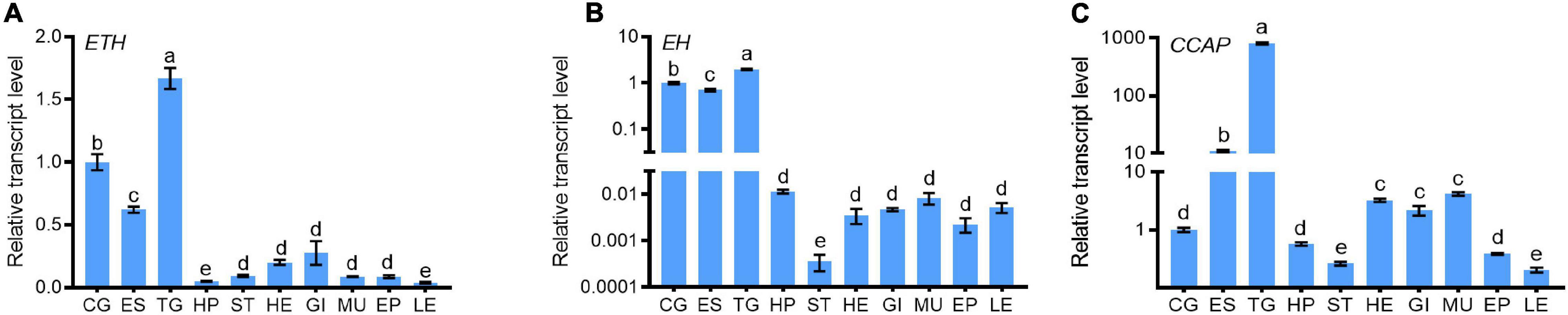
Figure 1. Tissue expression patterns of ETH (A), EH (B), and CCAP (C) in S. paramamosain. Tissues include cerebral ganglion (CG), eyestalk (ES), thoracic ganglia (TG), hepatopancreas (HP), stomach (ST), heart (HE), gill (GI), muscle (MU), epidermis (EP), and leg (LE) of crabs at the intermolt stage. For each sample, five individuals were measured in triplicate using real-time quantitative PCR (qRT-PCR) analysis. The values were calculated using the 2–ΔΔCT method. The relative transcript levels of CG were set as 1. The bars represented averages relative transcript levels and standard deviation (SD). Different letters indicated significant differences at P < 0.05 analyzed by ANOVA and the Tukey-Kramer test.
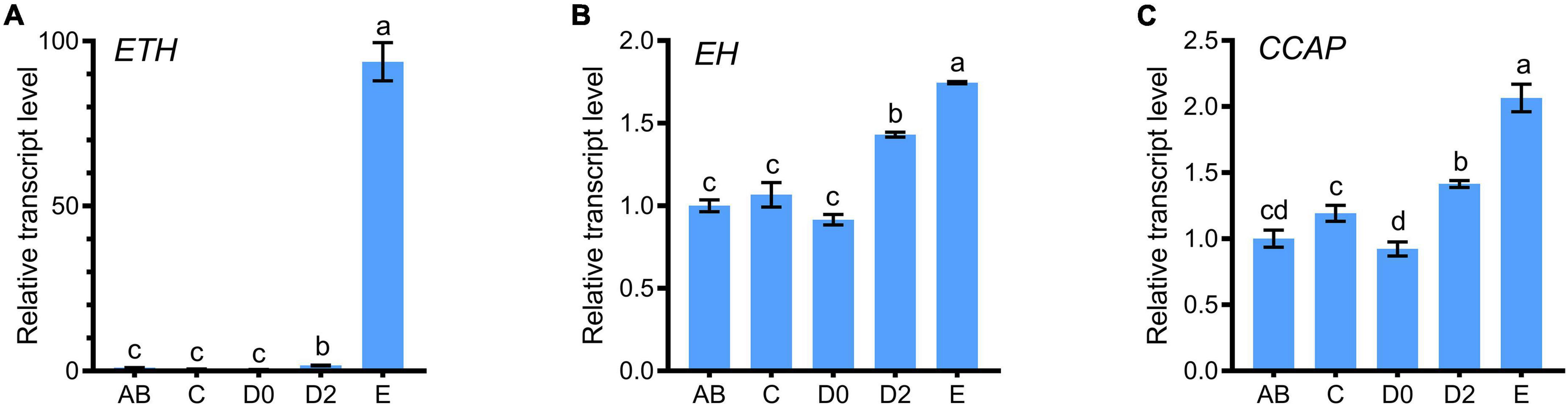
Figure 2. Temporal expression patterns of ETH (A), EH (B), and CCAP (C) in S. paramamosain. The cDNA templates were derived from the post-molt stage (AB), intermolt stage (C), the early premolt stage (D0), the late premolt stage (D2) and the ecdysis stage (E). For each sample, three independent pools of five individuals were measured in triplicate using real-time quantitative PCR (qRT-PCR). The values were calculated using the 2–ΔΔCT method. The relative transcript levels of AB were set as 1. The bars represented averages with vertical lines indicating standard deviation (i.e., SD). Different letters indicate significant differences at P < 0.05 computed with ANOVA and the Tukey-Kramer test.
Knockdown of dsETH, dsEH or dsCCAP in Crabs at D0 Stage Caused Molt Failure and Can Be Rescued by Synthetic Peptides Except dsEH Group
When juvenile crabs at the D0 stage were injected with dsETH, dsEH or dsCCAP (knockdown condition), the percent of crabs that molt normally was seriously affected compared to the dsGFP-injected group. For dsETH and dsCCAP groups, apolysis occurred in those crabs and most crabs could advance to the late premolt stage. Also, the old exoskeleton and the new epidermis for which could be separated from the new cuticle artificially (Figures 3A,B). However, more than half of the crabs failed to initiate the molting process and eventually death occurred at D2 stage in those crabs (Figure 3C). As a comparison, 58% of the crabs injected with dsEH died before D2 stage, which was significantly higher than that in other groups (Figure 3C).
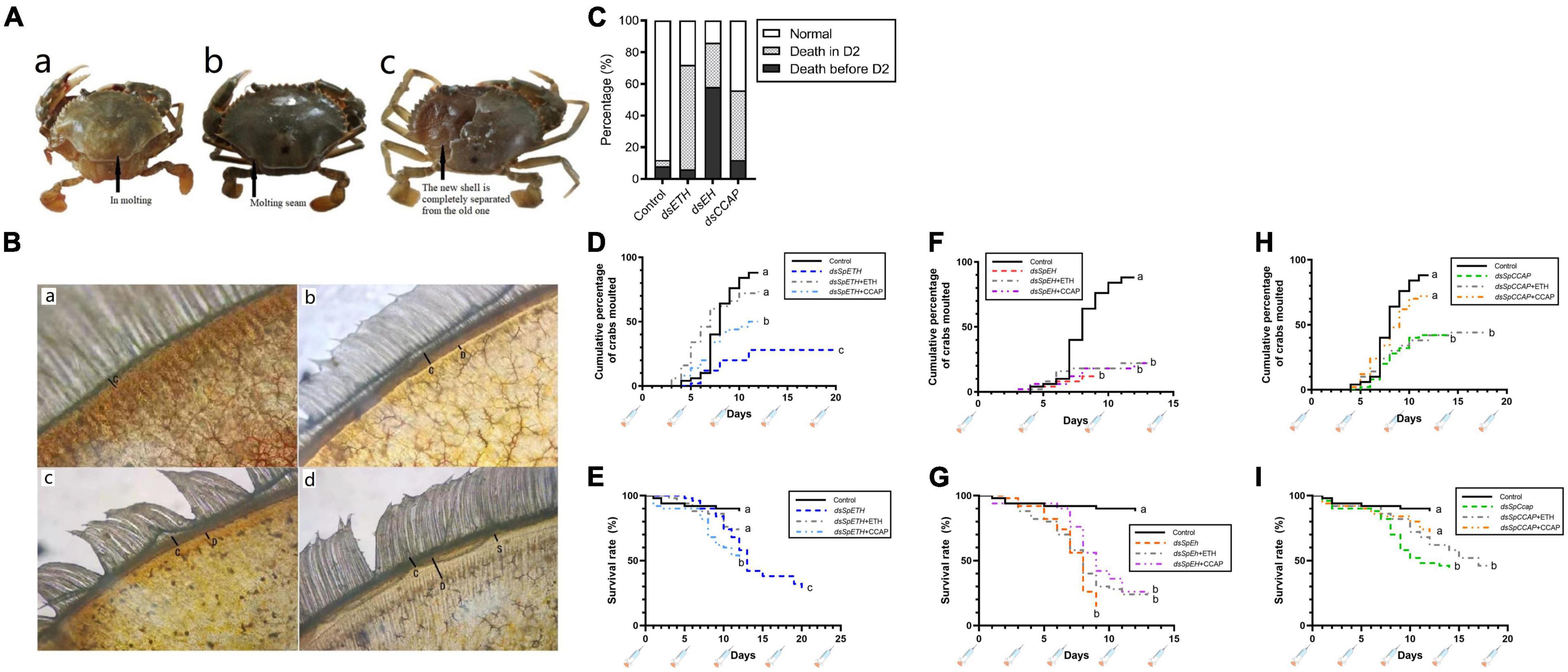
Figure 3. Effect of in vivo dsRNA injection on crabs molting (A,a) Crab that started molting normally; (A,b) crab that unable to initiate molting and died; (A,c) crab died at D2 stage and the exoskeleton of could be peeled off easily. (B) Microscopic examination of a portion of the crab swimming legs at C (B,a), D0 (B,b), D1 (B,c), and D2 (B,d). The letter C in figures represented the old epidermis, letter D represented the new epidermis, letter S represented the ecdysial suture. (C) Death records of crabs at different stages for the four groups injected with dsGFP, dsETH, dsEH, and dsCCAP, respectively (n = 50). (D) The cumulative percentage of groups injected with dsGFP, dsETH, dsETH + ETH and dsETH + CCAP, respectively, at D0 stage. (E) The survival rate of groups injected with dsGFP, dsETH, dsETH + ETH and dsETH + CCAP respectively at D0 stage. (F) The cumulative percentage of groups injected with dsGFP, dsEH, dsEH + ETH and dsEH + CCAP respectively at D0 stage. (G) The survival rate of groups injected with dsGFP, dsEH, dsEH + ETH and dsEH + CCAP respectively at D0 stage. (H) The cumulative percentage of groups injected with dsGFP, dsCCAP, dsCCAP + ETH and dsCCAP + CCAP respectively at D0 stage. (I) The survival rate of groups injected with dsGFP, dsCCAP, dsCCAP + ETH and dsCCAP + CCAP respectively at D0 stage. Statistically significant differences (P) between the control and any knockdown conditions were found via a log-rank (Mantel-Cox) test and are indicated by different letters.
To further verify the function of molt regulation between ETH, EH, and CCAP, synthetic peptides of ETH and CCAP were co-injected with these three dsRNAs separately. Injection of ETH increased the number of successful molts and survival rate in the ETH-knockdown group from 28 to 72%. However, this has no effect on the EH-knockdown and CCAP-knockdown groups (Figure 3). Injection of CCAP increased the number of successful molts and survival rate in the CCAP-knockdown group from 42 to 72%. However, it has no effect to the EH-knockdown group. However, injection of CCAP the number of successful molts and survival rate in ETH-knockdown group from 28 to 50% (Figure 3).
Transcript Levels of Molt-Related Genes in Injected Crabs at D0 Stage
To further elucidate the relationship between EH and ETH at different molting stages, transcriptional levels of genes in dsETH- and dsEH-injected crabs at D0 and D2 stages were analyzed. For crabs at the D0 stage, injection of dsETH caused a significant reduction of ETH transcript level (−30.82%). The transcript levels of EH and CCAP also decreased significantly (Figures 4A–C). Injection of dsEH to juvenile crabs at stage D0 silenced the transcription of EH to a level of 61.92% (Figure 4D). However, the transcripts level of ETH increased sharply (+360%) (Figure 4E), but there was no change in the transcript level of CCAP (Figure 4F).
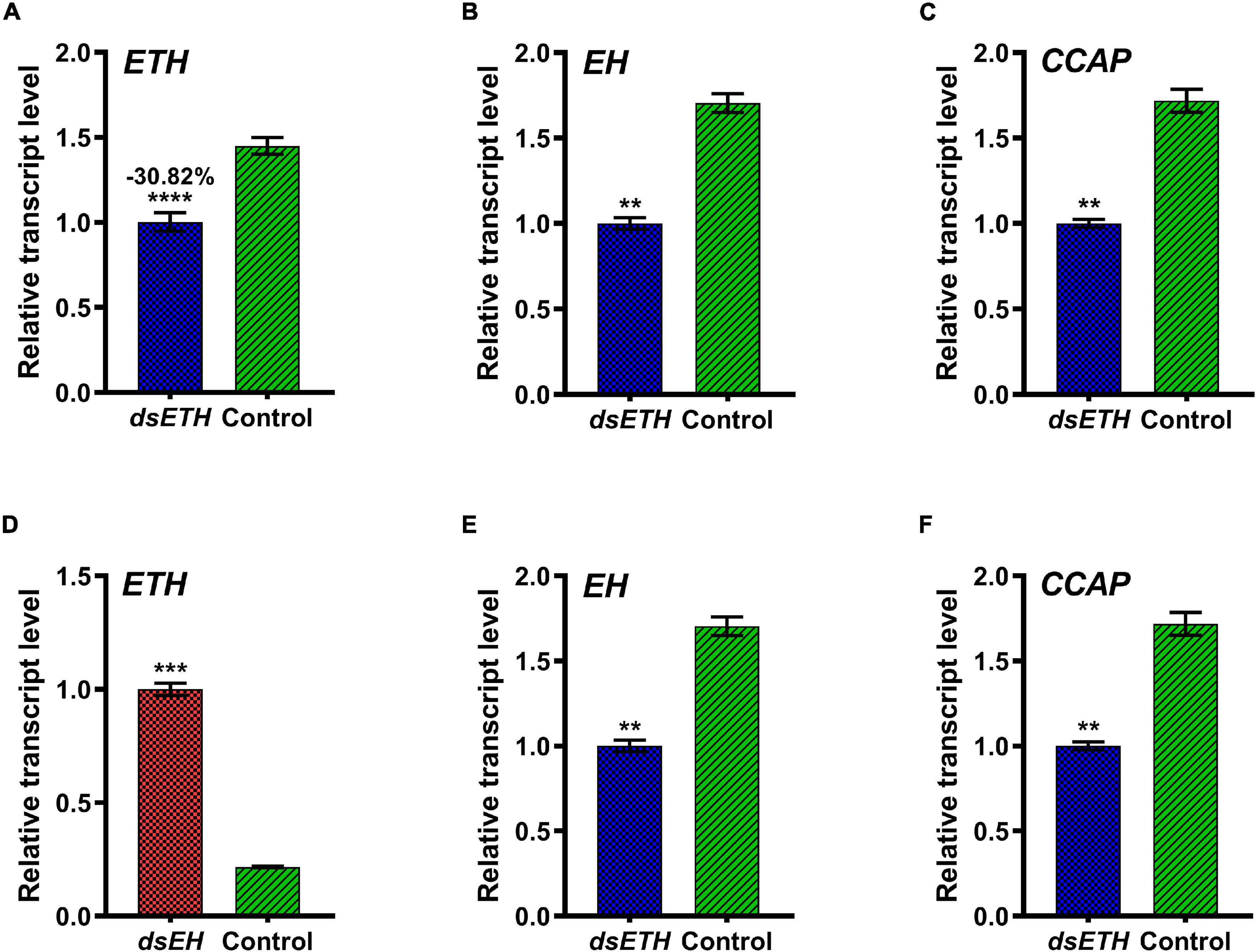
Figure 4. Relative transcript levels of ETH (A), EH (B), and CCAP (C) in thoracic ganglia of dsGFP and dsETH-injected crabs at D0 stage were measured. Also, relative transcript levels of ETH (D), EH (E), and CCAP (F) in the thoracic ganglia of dsGFP and dsEH-injected crabs at the D0 stage were measured. These data represented the mean ± SD. For each sample, three independent pools of five individuals were measured in technical triplicate using real-time quantitative PCR (qRT-PCR). Statistically significant differences between the measurements were found via a t-test with Welch’s correction and are indicated by asterisks (**P < 0.01; ***P < 0.001; ****P < 0.0001).
Transcriptional Levels of Genes in Injected Crabs at D2 Stage
When crabs at D2 stage were injected with the same amount of dsETH as at D0 stage, the transcripts level of ETH had a significant increase conversely and CCAP transcripts level was decreased significantly compared to control but the transcripts level of EH had no change (Figures 5A–C). When juvenile crabs at D2 stage were injected with the same amount of dsEH as at D0 stage, the transcripts level of EH was decreased to 23.22% compared to that of the controls (Figure 5D). In addition to the injection of dsETH, knock-down of EH could also reduce the transcripts level of ETH significantly (Figure 5E), in which the transcripts level of CCAP decreased significantly too (Figure 5F).
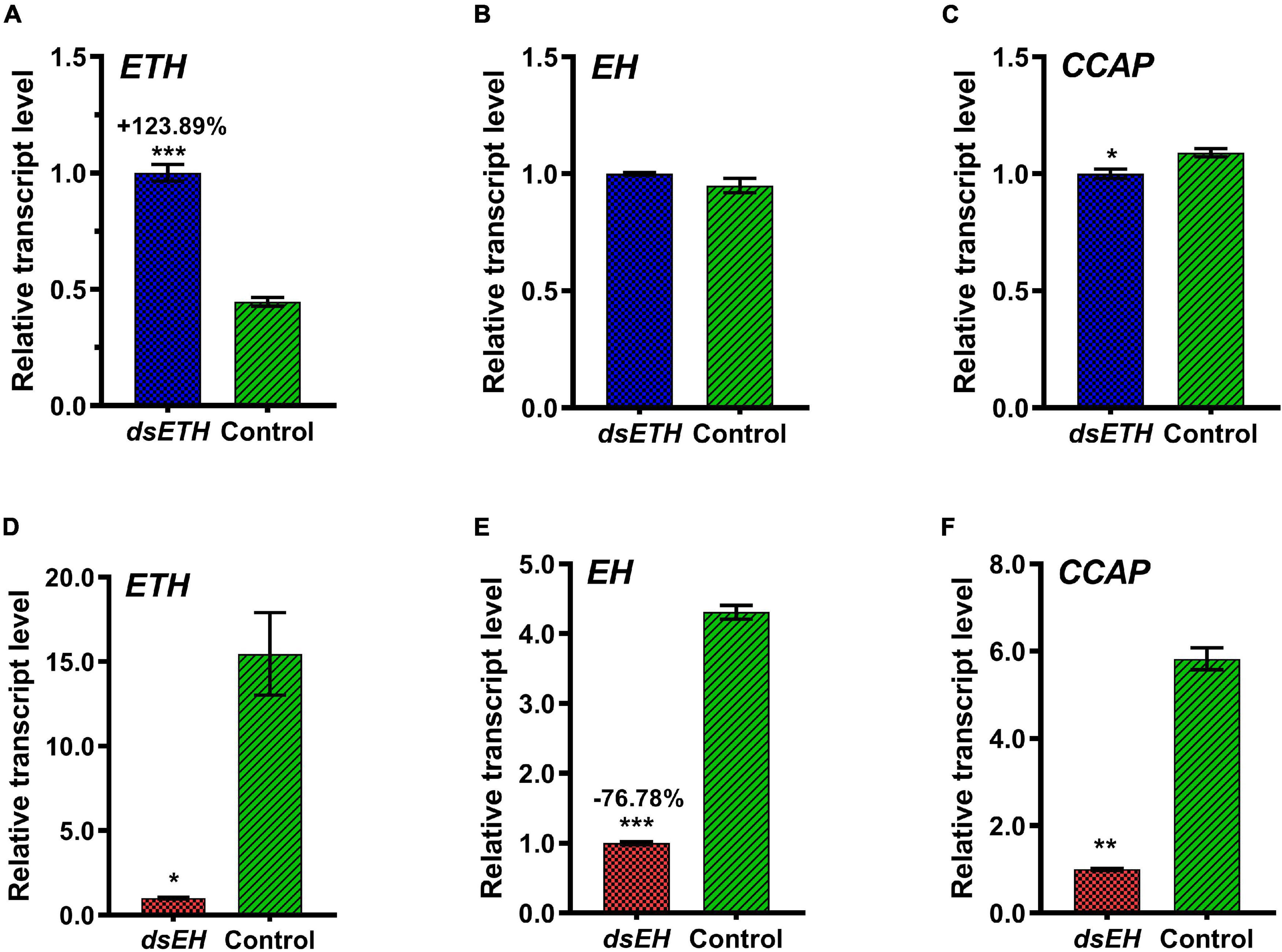
Figure 5. Relative transcript levels of ETH (A), EH (B), and CCAP (C) in thoracic ganglia of dsGFP and dsETH-injected crabs at D2 stage were measured. Also, relative transcript levels of ETH (D), EH (E), and CCAP (F) in the thoracic ganglia of dsGFP and dsEH-injected crabs at D2 stage were measured. These data represented the mean ± SD. For each sample, three independent pools of five individuals were measured in technical triplicate using real-time quantitative PCR (qRT-PCR). Statistically significant differences between the measurements were found via a t-test with Welch’s correction and are indicated by asterisks (*P < 0.05; **P < 0.01; ***P < 0.001).
Co-injection of dsETH and dsEH to Crabs at D2 Stage
To verify the regulatory relationship between ETH and EH, co-injection of dsETH and dsEH were conducted to crabs at D2 stage. Results show that ETH expression was knock-down as compared to the controls, with a decrease of 68.06% (Figure 6A), and the transcriptional level of EH decreased by 77.19% (Figure 6B). Similarly, the transcriptional level of CCAP was significantly reduced than that of the control group (Figure 6C).
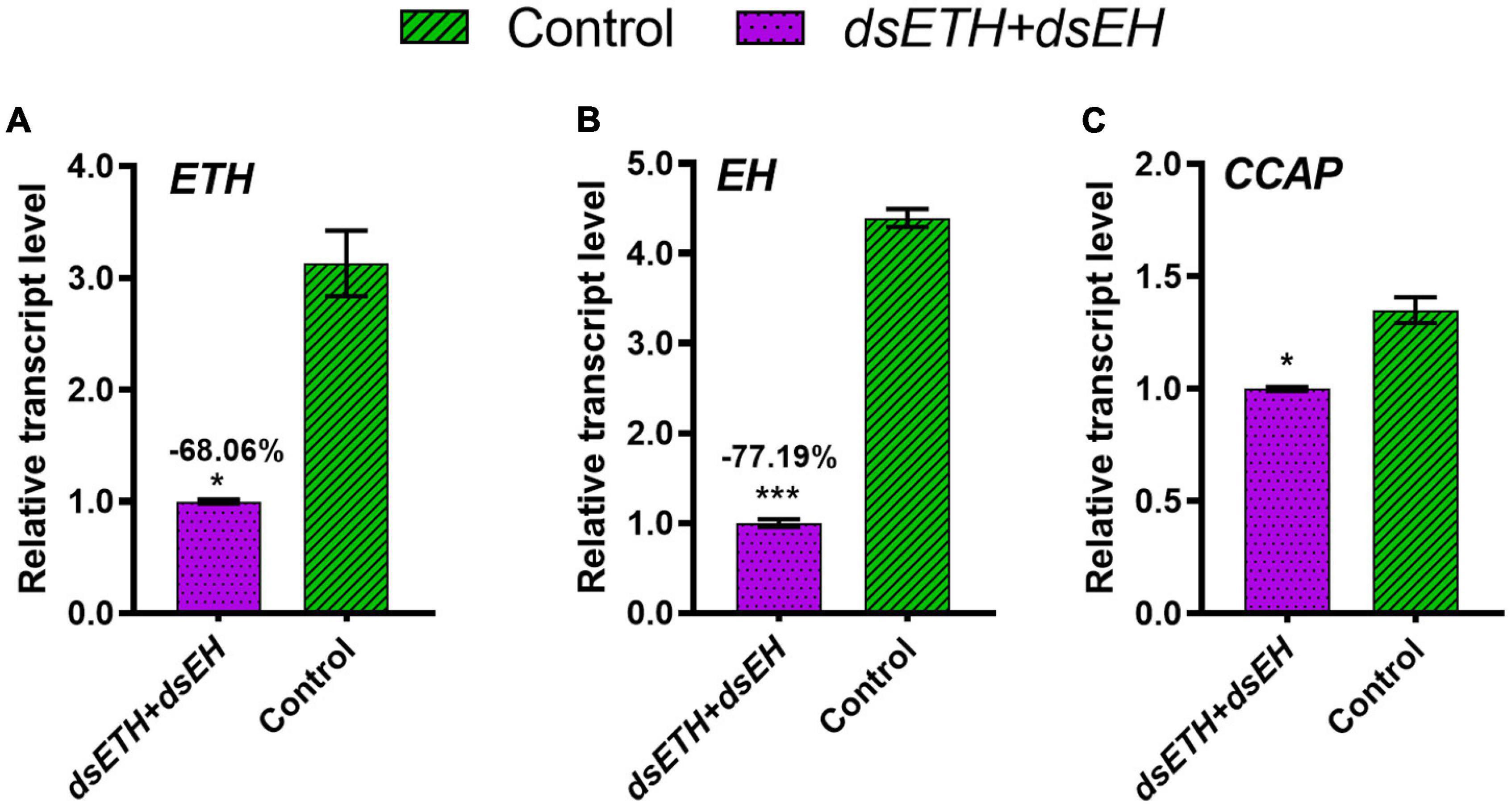
Figure 6. Relative transcript levels of ETH (A), EH (B), and CCAP (C) in thoracic ganglia of dsGFP and co-injected crabs at D2 stage were measured. These data represented the mean ± SD. For each sample, three independent pools of five individuals were measured in technical triplicate using real-time quantitative PCR (qRT-PCR). Statistically significant differences between the measurements were found via a t-test with Welch’s correction and are indicated by asterisks (*P < 0.05; ***P < 0.001).
Discussion
From post-larva onward, mud crab has to molt 18–20 times in its lifetime (Kim et al., 2006). The early larval molts were characterized by metamorphic transformation. From the juvenile stage onward, molting was characterized by size increment only. In the present study, ETH, EH, and CCAP were selected based on the knowledge that these genes are involved in insects molting (Fort et al., 2007; Hull et al., 2009; Jackson et al., 2009), and experiments were conducted under in vivo assays with juvenile crabs.
Transcripts of Ecdysis Triggering Hormone, Eclosion Hormone, and Crustacean Cardioactive Peptide Are Mainly Localized in Thoracic Ganglia and Fluctuate Periodically With Molt in Scylla paramamosain
In insects such as Leptinotarsa decemlineata and Bactrocera dorsalis, ETH is mainly produced by Inka epithelial cells of the endotracheal gland in the trachea. The ETH expression level is the highest at the late premolt stage (Shi et al., 2017; Shen et al., 2021). In Drosophila, EH is expressed in the neuronal tissues and trachea, which participate in the regulation of molting behaviors (Scott et al., 2020). Although the crustaceans did not evolve a tracheal system, Both the Insecta and Crustacea shared a large number of homologous neuropeptides that are highly conserved in structure and functions (Veenstra, 2016; Oliphant et al., 2018). In this study, ETH, EH and CCAP are mainly expressed in thoracic ganglion in S. paramamosain (Figures 1A–C), which indicates that crab nerve tissue assumes part of the similar endocrine regulation function as the insect tracheal tissue. In S. paramamosain, the transcriptional level of ETH fluctuated dramatically with the ecdysis cycle, which indicated that ETH might be the major gene initiating molting behavior (Figure 2A). Unlike ETH, the transcripts of EH and CCAP at D2 and E stages increased gradually (Figures 2B,C). At the initial premolt stage of insects such as Manduca sexta, ETH is at a low level and could regulate the initiation of the entire molting behavior (Gammie and Truman, 1999). In the crab, the transcription level of ETH is also the lowest at D0 stage, it’s interesting to know if crab has a similar ETH regulatory mode.
Ecdysis Triggering Hormone, Eclosion Hormone, and Crustacean Cardioactive Peptide Play Different but Critical Roles to Molt in Scylla paramamosain
Results show that ETH, EH, and CCAP are essential for the regulation of molting behavior in mud crabs. In insects, mortality occurred when ETH, EH or CCAP was interrupted. For example, EH null mutant Drosophila and EH-interrupted Tribolium, pre-ecdysis behaviors were disrupted and mass mortality occurred before molting (Arakane et al., 2008; Kruger et al., 2015). Similarly in the red flour beetles Tribolium, the dsETH- or dsCCAP-injected animals have completed material accumulation, water re-absorption, but eventually died instead of shedding the old exoskeleton (Arakane et al., 2008). Interestingly, for dsEH-injected crabs, pre-ecdysis behaviors were disrupted and crabs were unable to advance to D2 stage and died (Figures 3C,F,G). Both ETH and CCAP synthetic peptides could rescue the molting of crabs when their genes were silenced, which suggests they play key roles in crab molting. Compared to ETH and CCAP, EH appears to have a specific function on the formation of new epidermis at premolt stage as synthetic peptides for ETH and CCAP can’t offset the gene knock-down effect of EH (Figures 3D–I). Also, our results show that dsETH- or dsCCAP-injected crabs developed to D2 stage smoothly but failed to molt, at which crabs had completed the formation of new epidermis (Figure 3A). The results were similar to that of the red flour beetles. Remarkably, for the dsETH-injected crabs, injection of CCAP rescued half of them from failed molt, whereas same result didn’t see in the dsCCAP-injected crabs under the rescue of ETH synthetic peptide (Figures 3D,E,H,I). Considering that CCAP may be a downstream gene of ETH and can replace part of the role of ETH in ecdysis behaviors.
The Transcriptional Level of Eclosion Hormone and Crustacean Cardioactive Peptide Were Effected by Ecdysis Triggering Hormone at D0 Stage
In Manduca sexta, 20-E level at early premolt stage is high, which can activate the low-level expression of ETH in the body (Zitnan et al., 2007). The release of ETH then activates the synthesis and release of EH in the brain neurons by binding to its receptor ETHR-A (Gammie and Truman, 1999; Kim et al., 2006). Similarly, the ETH and EH transcriptional levels of S. paramamosain at D0 stage were low (Figures 2A,B), the transcriptional levels of EH and CCAP were increased when ETH was knockdown (Figure 4). In Drosophila, CCAP null mutants did not affect the normal development and molting of the worms (Clark et al., 2004). Whereas the knockdown of CCAP caused failure of molt and eventually died in Tribolium (Arakane et al., 2008). Studies found that CCAP was regulated by 20E in Bactrocera dorsalis (Shi et al., 2019b), which means CCAP is involved in molting-related regulation in B. dorsalis. The above reports indicate that CCAP has functional differentiation in some species such as Drosophila in Insecta. In the present study, the strong dependence of the transcript levels of EH and CCAP on ETH indicated that EH and CCAP have positive correlations with ETH in S. paramamosain at D0 stage.
Increase of Ecdysis Triggering Hormone Transcripts Was Affected by Eclosion Hormone and an Unknown Factor at D2 Stage
At late premolt stage of Tribolium castaneum (Arakane et al., 2008), ETH acts on the ventral neurons to release EH into hemolymph, and EH then acts on the ETH storage cells to stimulate large quantities release of ETH. The positive feedback regulation further activates a series of molting-related neuronal genes such as CCAP and bursicon, and initiates molting. However, in Drosophila, the release of ETH has no correlation with EH (Clark et al., 2004), which indicates that the positive feedback regulation mode between ETH and EH is not conserved in Insecta. Our study shows that when crabs developed to D2 stage, ETH and EH transcriptional levels rose rapidly (Figures 2A,B). A strong positive of ETH may exist at D2 stage to ensure its continuous and massive expression. The obvious change in dsETH-injected crabs didn’t cause a fluctuation of EH (Figures 5A,B), which suggests that the gene that directly regulates the retaliatory increase of ETH is not EH. The expression of ETH and ecdysis of crabs were significantly inhibited with the knockdown of EH at D2 stage (Figures 5D,E), which suggesting that although not a direct regulatory gene to ETH at D2 stage, EH plays an important role in the massive expression of ETH. To further verify the relationship between ETH and EH at D2 stage, co-injection of dsETH and dsEH to crabs at D2 stage were down (Figures 6A–C). The results indicated that when EH was disturbed, the unknown factor required for ETH’s surge was also inhibited, and EH may indirectly regulate the increase of ETH at this stage by regulating the unknown factor. Also, the transcription levels of ETH, EH, and CCAP are highest at E stage, whereas the higher levels of mRNA for EH and CCAP should not to be coincident with the highest levels of ETH because stage E lasts just a few minutes in crabs, and it would be very difficult to envisage how an increased levels of ETH or EH might influence transcription/translation of CCAP or ETH in such a short time frame. Therefore, the correlation of these three genes might not be a direct regulation but an inter-relationships with each other.
Conclusion
Following with recent climate anomalies, environmental water pollution and increased using of pesticide, failure of molt has become more frequent in wild and farmed crustaceans (Verslycke et al., 2004; Soin and Smagghe, 2007; Zitnan et al., 2007). In this study, ETH is the upstream regulatory gene of CCAP, and ETH, EH, and CCAP had different but essential roles in crab’s molt. Also, ETH and EH have different interactional relationships at D0 and D2 stages. As a result, we proposed a model for the molting behavior regulation of Scylla paramamosain (Figure 7). The complicated molting regulation mode of arthropods is highly susceptible to fluctuations by external interference, chemical disturbances, and habitat deterioration. The study of molting process may reveal novel molecular targets for the discovery of different molecular mechanisms between Insecta and Crustacea, and may contribute to develop strategy to reduce molting failure in aquaculture crabs.
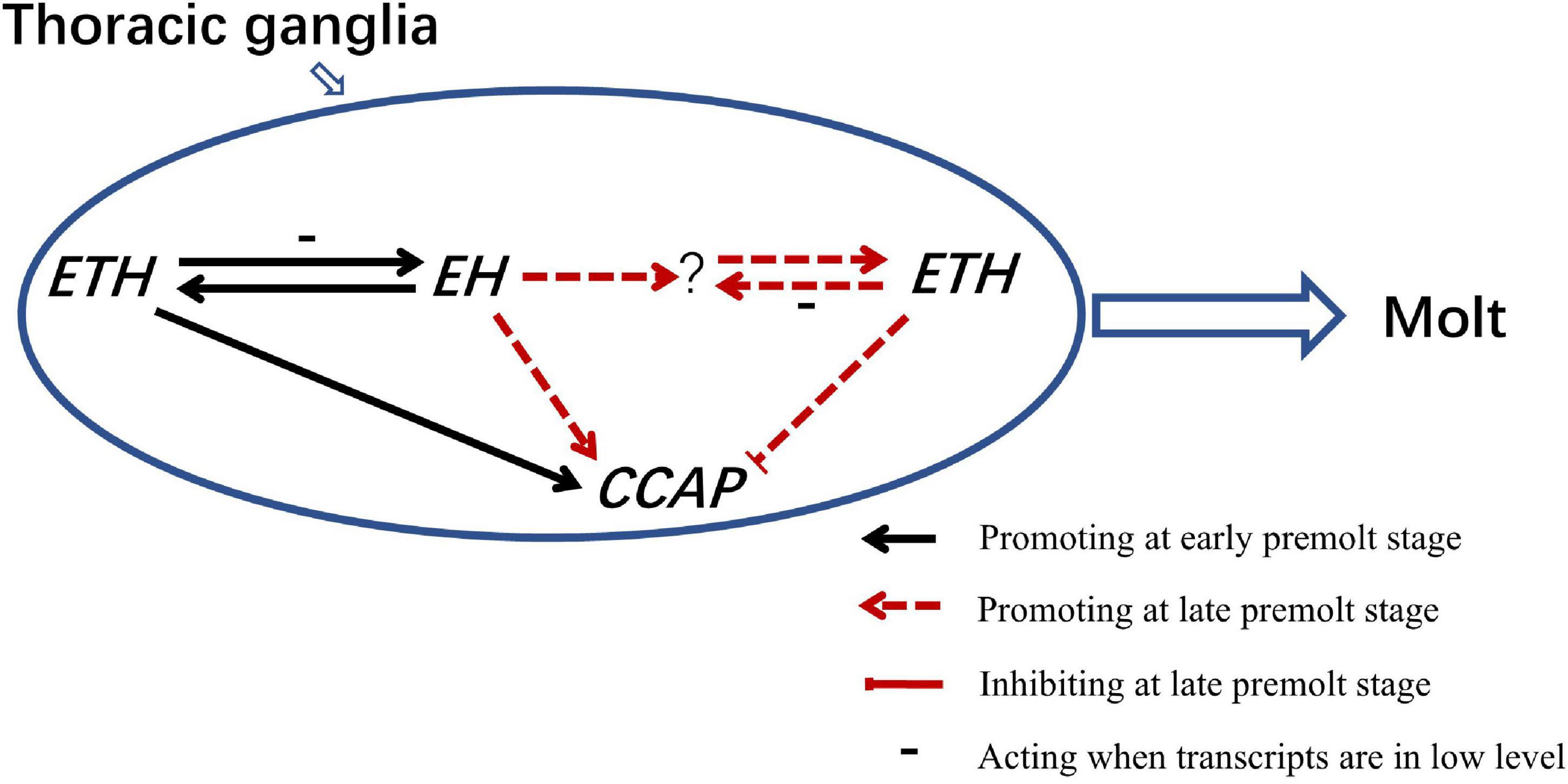
Figure 7. A model of ETH, EH, and CCAP genes in the regulation of molting behavior in Scylla paramamosain.
Data Availability Statement
The original contributions presented in the study are included in the article/Supplementary Material, further inquiries can be directed to the corresponding authors.
Author Contributions
S-FC and Y-FZ: conceptualization, writing, review, and editing. S-FC, Y-FZ, and C-GW: methodology. Y-FZ and Q-QW: software and data curation. C-MA, Q-QW, and WW: validation. Y-FZ and WW: formal analysis. S-FC, Y-FZ, and WW: investigation. C-GW: resources. Y-FZ: writing – original draft preparation. C-MA and L-LS: visualization. S-FC: supervision, project administration, and funding acquisition. All authors have read and agreed to the published version of the manuscript.
Funding
This research was funded by the Guangdong Provisional Research Grant (#2014B020202014) and Guangxi Key Laboratory of Beibu Gulf Marine Biodiversity Conservation Fund (2019KB06).
Conflict of Interest
The authors declare that the research was conducted in the absence of any commercial or financial relationships that could be construed as a potential conflict of interest.
Publisher’s Note
All claims expressed in this article are solely those of the authors and do not necessarily represent those of their affiliated organizations, or those of the publisher, the editors and the reviewers. Any product that may be evaluated in this article, or claim that may be made by its manufacturer, is not guaranteed or endorsed by the publisher.
Supplementary Material
The Supplementary Material for this article can be found online at: https://www.frontiersin.org/articles/10.3389/fmars.2022.855391/full#supplementary-material
References
Adams, M. E., and Zitnan, D. (1997). Identification of ecdysis-triggering hormone in the silkworm Bombyx mori. Biochem. Biophys. Res. Commun. 230, 188–191. doi: 10.1006/bbrc.1996.5915
Arakane, Y., Li, B., Muthukrishnan, S., Beeman, R. W., Kramer, K. J., and Park, Y. (2008). Functional analysis of four neuropeptides, EH, ETH, CCAP and bursicon, and their receptors in adult ecdysis behavior of the red flour beetle, Tribolium castaneum. Mech. Dev. 125, 984–995. doi: 10.1016/j.mod.2008.09.002
Chung, K.-F., and Lin, H.-C. (2006). Osmoregulation and Na, K-ATPase expression in osmoregulatory organs of Scylla paramamosain. comparative biochemistry and physiology. Part A Mol. Integr. Physiol. 144, 48–57. doi: 10.1016/j.cbpa.2006.02.003
Claeys, I., Breugelmans, B., Simonet, G., Franssens, V., Van Soest, S., and Vanden Broeck, I. (2006). Regulation of Schistocerca greparia neuroparsin transcript levels by juvenile hormone and 20-hydroxyecdysone. Arch. Insect Biochem. Physiol. 62, 107–115. doi: 10.1002/arch.20127
Clark, A. C., del Campo, M. L., and Ewer, J. (2004). Neuroendocrine control of larval ecdysis behavior in Drosophila: complex regulation by partially redundant neuropeptides. J. Neurosci. 24, 4283–4292. doi: 10.1523/JNEUROSCI.4938-03.2004
Fort, T. J., Garcia-Crescioni, K., Agricola, H. J., Brezina, V., and Miller, M. W. (2007). Regulation of the crab heartbeat by crustacean cardioactive peptide (CCAP): central and peripheral actions. J. Neurophysiol. 97, 3407–3420. doi: 10.1152/jn.00939.2006
Gammie, S., and Truman, J. (1999). Eclosion hormone provides a link between ecdysis-triggering hormone and crustacean cardioactive peptide in the neuroendocrine cascade that controls ecdysis behavior. J. Exp. Biol. 202, 343–352.
Hull, J. J., Copley, K. S., Schegg, K. M., Quilici, D. R., Schooley, D. A., and Welch, W. H. (2009). De novo molecular modeling and biophysical characterization of Manduca sexta eclosion hormone. Biochemistry 48, 9047–9060. doi: 10.1021/bi901078y
Jackson, G. E., Mabula, A. N., Stone, S. R., Gade, G., Kover, K. E., Szilagyi, L., et al. (2009). Solution conformations of an insect neuropeptide: crustacean cardioactive peptide (CCAP). Peptides 30, 557–564. doi: 10.1016/j.peptides.2008.11.014
Kim, Y. J., Zitnan, D., Cho, K. H., Schooley, D. A., Mizoguchi, A., and Adams, M. E. (2006). Central peptidergic ensembles associated with organization of an innate behavior. Proc. Natl. Acad. Sci. U.S.A. 103, 14211–14216. doi: 10.1073/pnas.0603459103
Kruger, E., Mena, W., Lahr, E. C., Johnson, E. C., and Ewer, J. (2015). Genetic analysis of eclosion hormone action during Drosophila larval ecdysis. Development 142, 4279–4287. doi: 10.1242/dev.126995
Lenaerts, C., Cools, D., Verdonck, R., Verbakel, L., Vanden Broeck, J., and Marchal, E. (2017). The ecdysis triggering hormone system is essential for successful moulting of a major hemimetabolous pest insect, Schistocerca gregaria. Sci. Rep. 7:46502. doi: 10.1038/srep46502
Liu, J., Liu, A., Liu, F., Huang, H. Y., and Ye, H. H. (2020). Role of neuroparsin 1 in vitellogenesis in the mud crab, Scylla paramamosain. Gen. Comp. Endocrinol. 285:113248. doi: 10.1016/j.ygcen.2019.113248
Mesce, K. A., and Fahrbach, S. E. (2002). Integration of endocrine signals that regulate insect ecdysis. Front. Neuroendocrinol. 23, 179–199. doi: 10.1006/frne.2002.0228
Minh Nhut, T., Mykles, D. L., Elizur, A., and Ventura, T. (2020). Ecdysis triggering hormone modulates molt behaviour in the redclaw crayfish Cherax quadricarinatus, providing a mechanistic evidence for conserved function in molt regulation across Pancrustacea. Gen. Comp. Endocrinol. 298:113556. doi: 10.1016/j.ygcen.2020.113556
Morton, D., and Simpson, P. (2002). Cellular signaling in eclosion hormone action. J. Insect Physiol. 48, 1–13. doi: 10.1016/S0022-1910(01)00157-3
Mykles, D. L., and Chang, E. S. (2020). Hormonal control of the crustacean molting gland: insights from transcriptomics and proteomics. Gen. Comp. Endocrinol. 294:113493. doi: 10.1016/j.ygcen.2020.113493
Oliphant, A., Alexander, J. L., Swain, M. T., Webster, S. G., and Wilcockson, D. C. (2018). Transcriptomic analysis of crustacean neuropeptide signaling during the moult cycle in the green shore crab, Carcinus maenas. BMC Genomics 19:711. doi: 10.1186/s12864-018-5057-3
Ong, K. S. (1966). Observations on the post larval life history of Scylla serrata Forsk氓l, reared in the laboratory. Malay. Agric. J. 45, 429–443.
Park, Y., Filippov, V., Gill, S. S., and Adams, M. E. (2002). Deletion of the ecdysis-triggering hormone gene leads to lethal ecdysis deficiency. Development 129, 493–503. doi: 10.1242/dev.129.2.493
Park, Y., Zitnan, D., Gill, S. S., and Adams, M. E. (1999). Molecular cloning and biological activity of ecdysis-triggering hormones in Drosophila melanogaster. FEBS Lett 463, 133–138. doi: 10.1016/s0014-5793(99)01622-1
Scott, R. L., Diao, F., Silva, V., Park, S., Luan, H., Ewer, J., et al. (2020). Non-canonical eclosion hormone-expressing cells regulate Drosophila ecdysis. iScience 23:101108. doi: 10.1016/j.isci.2020.101108
Shen, C. H., Xu, Q. Y., Fu, K. Y., Guo, W. C., Jin, L., and Li, G. Q. (2021). Ecdysis triggering hormone is essential for larva-pupa-adult transformation in Leptinotarsa decemlineata. Insect Mol. Biol. 30, 241–252. doi: 10.1111/imb.12691
Shi, Y., Jiang, H. B., Gui, S. H., Liu, X. Q., Pei, Y. X., Xu, L., et al. (2017). Ecdysis triggering hormone signaling (ETH/ETHR-A) is required for the Larva-Larva Ecdysis in Bactrocera dorsalis (Diptera: Tephritidae). Front. Physiol. 8:587. doi: 10.3389/fphys.2017.00587
Shi, Y., Liu, T. Y., Jiang, H. B., Liu, X. Q., Dou, W., Park, Y., et al. (2019a). The Ecdysis triggering hormone system, via ETH/ETHR-B, is essential for successful reproduction of a major pest insect, Bactrocera dorsalis (Hendel). Front. Physiol. 10:151. doi: 10.3389/fphys.2019.00151
Shi, Y., Liu, T. Y., Pei, Y. X., Jiang, H. B., Dou, W., Smagghe, G., et al. (2019b). Crustacean cardioactive peptide (CCAP) of the oriental fruit fly, Bactrocera dorsalis (Diptera: Tephritidae): molecular characterization, distribution and its potential roles in larva-pupa ecdysis. Peptides 122:169929. doi: 10.1016/j.peptides.2018.02.007
Soin, T., and Smagghe, G. (2007). Endocrine disruption in aquatic insects: a review. Ecotoxicology 16, 83–93. doi: 10.1007/s10646-006-0118-9
Song, Y., Villeneuve, D., Toyota, K., and Tollefsen, K. E. (2017). Ecdysone receptor agonism leading to lethal molting disruption in arthropods: review and adverse outcome pathway development. Environ. Sci. Technol. 51, 4142–4157. doi: 10.1021/acs.est.7b00480
Truman, J. W., and Riddiford, L. M. (1970). Neuroendocrine control of ecdysis in silkmoths. Science 167, 1624–1626. doi: 10.1126/science.167.3925.1624
Veelaert, D., Passier, P., Devreese, B., Vanden Broeck, J., Van Beeumen, J., Vullings, H. G., et al. (1997). Isolation and characterization of an adipokinetic hormone release-inducing factor in locusts: the crustacean cardioactive peptide. Endocrinology 138, 138–142. doi: 10.1210/endo.138.1.4855
Veenstra, J. A. (2016). Similarities between decapod and insect neuropeptidomes. PeerJ 4:e2043. doi: 10.7717/peerj.2043
Verslycke, T. A., Fockedey, N., McKenney, C. L., Roast, S. D., Jones, M. B., Mees, J., et al. (2004). Mysid crustaceans as potential test organisms for the evaluation of environmental endocrine disruption: a review. Environ. Toxicol. Chem. 23, 1219–1234. doi: 10.1897/03-332
Zhao, X. F. (2020). G protein-coupled receptors function as cell membrane receptors for the steroid hormone 20-hydroxyecdysone. Cell Commun. Signal. 18:146. doi: 10.1186/s12964-020-00620-y
Zhou, L. H., Li, S. H., Wang, Z. W., Li, F. H., and Xiang, J. H. (2017). An eclosion hormone-like gene participates in the molting process of palaemonid shrimp exopalaemon carinicauda. Dev. Genes. Evol. 227, 189–199. doi: 10.1007/s00427-017-0580-9
Zieger, E., Robert, N. S. M., Calcino, A., and Wanninger, A. (2021). Ancestral role of ecdysis-related neuropeptides in animal life cycle transitions. Curr. Biol. 31:207. doi: 10.1016/j.cub.2020.10.004
Zitnan, D., and Adams, M. (2012). Neuroendocrine regulation of ecdysis. Insect Endocrinol. 3, 253–309. doi: 10.1016/B978-0-12-384749-2.10007-X
Zitnan, D., Kim, Y. J., Zitnanova, I., Roller, L., and Adams, M. E. (2007). Complex steroid-peptide-receptor cascade controls insect ecdysis. Gen. Comp. Endocrinol. 153, 88–96. doi: 10.1016/j.ygcen.2007.04.002
Zitnan, D., Kingan, T. G., Hermesman, J. L., and Adams, M. E. (1996). Identification of ecdysis-triggering hormone from an epitracheal endocrine system. Science 271, 88–91. doi: 10.1126/science.271.5245.88
Zitnan, D., Ross, L. S., Zitnanova, I., Hermesman, J. L., Gill, S. S., and Adams, M. E. (1999). Steroid induction of a peptide hormone gene leads to orchestration of a defined behavioral sequence. Neuron 23, 523–535. doi: 10.1016/s0896-6273(00)80805-3
Keywords: Scylla paramamosain, Crustacea, ecdysis triggering hormone, eclosion hormone, crustacean cardioactive peptide, RNA interference, molt
Citation: Zhao Y-F, Wen Q-Q, Ao C-M, Wang W, Shi L-L, Wang C-G and Chan S-F (2022) Ecdysis Triggering Hormone, Eclosion Hormone, and Crustacean Cardioactive Peptide Play Essential but Different Roles in the Molting Process of Mud Crab, Scylla paramamosain. Front. Mar. Sci. 9:855391. doi: 10.3389/fmars.2022.855391
Received: 15 January 2022; Accepted: 08 February 2022;
Published: 28 February 2022.
Edited by:
Shengming Sun, Shanghai Ocean University, ChinaReviewed by:
Simon G. Webster, Bangor University, United KingdomJie Gong, Nantong University, China
Copyright © 2022 Zhao, Wen, Ao, Wang, Shi, Wang and Chan. This is an open-access article distributed under the terms of the Creative Commons Attribution License (CC BY). The use, distribution or reproduction in other forums is permitted, provided the original author(s) and the copyright owner(s) are credited and that the original publication in this journal is cited, in accordance with accepted academic practice. No use, distribution or reproduction is permitted which does not comply with these terms.
*Correspondence: Cheng-Gui Wang, bG9uZ3Nob3JlQDE2My5jb20=; Siuming-Francis Chan, c2l1bWluZzU3M0BzaW5hLmNvbQ==