- 1Frontiers Science Center for Deep Ocean Multispheres and Earth System, and Key Laboratory of Marine Chemistry Theory and Technology, Ministry of Education, Ocean University of China, Qingdao, China
- 2College of Chemistry & Chemical Engineering, Ocean University of China, Qingdao, Shandong, China
- 3School of Marine Sciences, Sun Yat-sen University, and Southern Marine Science and Engineering Guangdong Laboratory (Zhuhai), Zhuhai, China
- 4School of Geography Science and Geomatics Engineering, Suzhou University of Science and Technology, Suzhou, China
Sediment nitrogen (N) mineralization and immobilization are two crucial processes driven by microorganisms, which may play significant roles in the regulation of water quality in aquaculture ecosystems. However, limited information is available about the quantitative importance of sedimentary N mineralization and immobilization in coastal aquaculture systems. Here, a combination of incubation experiments with a 15N isotope dilution technique were employed, aiming to quantify N mineralization and immobilization processes in surface sediments (0–5 cm) of three types of aquaculture ecosystems (seabass, white shrimp, and green crab ponds) reclaimed within the western bank of the Pearl River Estuary. Our results showed that no significant difference in sediment N mineralization and immobilization rates, microbial abundances, and organic matter among different aquaculture types on small-scale range. Meanwhile, prolonged pond-drying significant reduced sediment N mineralization and immobilization rates, bacterial abundances, organic matter, moisture content, ferrous ion (Fe2+), Fe2+/Fe3+, and ammonium (NH4+), while not strongly altered sediment percentage of NH4+ mineralized per day (PAM), relative ammonium immobilization (RAI), fungal abundances, TOC/TN, nitrate (NO3−), and δ13Corg. N mineralization and immobilization rates were both significantly related to overlying water NO3−, as well as sediment moisture content, bulk density, organic matter, Fe2+, and microbial abundances. In addition, the total mineralized and immobilized N in aquaculture surface sediments from the Guangdong-Hong Kong-Macao Greater Bay Area were estimated to be approximately 4.55×104 and 3.68×104 t N yr-1, respectively. Higher N mineralization relative to N immobilized fluxes indicated that the sediment serves as an important source of eutrophication in reclaimed aquaculture system of coastal wetlands.
1. Introduction
Since the last century, anthropogenic activities have already become one of the major factors driving the change of global nitrogen (N) cycling and caused more than tripled N-flows higher than those caused by natural processes, and thus resulting in a total globally fixed N of about 413 Tg N y-1 (Zilio et al., 2020). The increasing reactive N load disrupts the N balance in both marine and terrestrial ecosystems. Meanwhile, a series of ecological problems, such as the loss of habitats and biodiversity (Galloway et al., 2008), eutrophication and the expansion of periodic or permanent low oxygen zone (Diaz and Rosenberg, 2008), the outbreak of toxic algae (Li et al., 2014), and the strengthened emission of greenhouse gases (Murray et al., 2015; Mao et al., 2022) were caused in the latest century. Therefore, coastal N pollution is one of the global significant and urgent environmental problem. Studies regarding N biogeochemical processes of estuarine and coastal ecosystems have become a cutting-edge scientific issue, which is also a key topic of many international research programs such as International Geophere-Biosphere Programme/Land-Ocean Interactions in Coastal Zone (IGBP/LOICZ), Integrated Marine Biogeochemistry and Ecosystem Research (IMBER) and Future Earth (Howarth et al., 2011; Buzzelli et al., 2013).
N mineralization and immobilization processes of N biogeochemical cycle in estuarine and coastal sediments. N mineralization is an important N-cycling process by which organic N is converted into inorganic forms available to organisms, which can exacerbate water eutrophication (Mishra et al., 2005). Conversely, microbial N immobilization is an important N-cycling process in which microorganisms convert inorganic N into organic N (e.g., amino acid and proteins) (Zhu et al., 2013), Meanwhile, some previous studies indicated that ammonium (NH4+) immobilization is considered as the major process of anaerobic ammonia consumption, which can effectively transform NH4+ into organic N and maintain the health of ecosystem (Matheson et al., 2003; Huang et al., 2021; Yang et al., 2022). These two important N biogeochemical processes have important ecological and environmental significance for maintaining N balance in estuarine and coastal ecosystems. Previous studies have reported that sediment N mineralization and immobilization are mainly affected by the physicochemical properties of sediment (e.g., soil texture, total carbon and N, C/N, pH, and water content) (Paul et al., 2003; Rutigliano et al., 2009; Huang et al., 2021), environmental climate factors (e.g., temperature, precipitation) (Gao et al., 2014; Lin et al., 2016a), and microorganisms in complex estuarine and coastal ecosystems (Cufrey and Kemp, 1992; Herbert, 1999; Bai et al., 2012; Qi et al., 2019). Thus, it is necessary to fully understand these two processes and their controlling factors for evaluation of N balance and prediction of N dynamics with the ever-changing environmental conditions in estuaries and coasts.
A large area of coastal wetlands has been reclaimed for development over the past few decades, and aquaculture use is one of the major reclamation purposes. In China, more than 1,260 km2 of coastal wetlands was reclaimed for aquaculture every year (Cao et al., 2011; Zuo et al., 2013). In order to meet the unprecedented demand of aquatic products supply, large amount of nitrogen- and phosphorus-rich bait is added into these ecosystems. When the nutrients in the water column exceed the requirment of plankton and aquacultured organisms, several disadvantages including water quality deterioration, frequent disease occurrence, and aquaculture benefits decline appeared (Amano et al., 2011). Coastal aquaculture ecosystem is generally regarded as a major source of N pollutants, and subsequent environemntal problems have attracted widespread concerns nowadays (Wu et al., 2014; Lin and Lin, 2022). Sediment is a vital place where N mineralization and immobilization occurred, representing frequent transformation between inorganic N and organic N, which could aggravate eutrophication to some extent. Coastal wetlands in the Pearl River Estuary are reclamation hotspots for aquaculture and have been significantly affected by anthropogenic activities. Investigation of N mineralization and immobilization around this area could refelct both regional and typical characteristics. In this study, relcaimed brackish aquaculture ecosystems located on the west river bank of the Pearl River Estuary were selected, and a combination of sediment incubation experiment and 15N stable isotope dilution method was employed. The main objectives of this study are to: (1) quantify sediment N mineralization and immobilization rates; (2) compare the effects of different cultured species (seabass, white shrimp, and green crab) on sediment N mineralization and immobilization, and identify the key controlling factors of these two processes; (3) preliminarily estimate sediment N mineralization and immobilization fluxes in the Guangdong-Hong Kong-Macao Greater Bay Area, and provide scientific basis for ecological effect evaluation as well as policy formulation and management in the area.
2. Materials and method
2.1. Study area and sampling
The Pearl River Delta (PRD, 112.9–114.4°E, 21.82–23.25°N) located on southern China covers an area of ~54,754 km2 and is surrounded by several highly populated cities such as Hong Kong, Guangzhou, Macau, and Shenzhen with a total population of over 78.61 million people in 2021 (Li et al., 2000; Liu et al., 2018). It is plain river network area with the slope of 0.1–0.2‰ and the density of river network of ~0.8 km km-2, which is characterized by subtropical humid climate with an annual average temperature of 21.8°C and an annual average rainfall of 1747.4 mm (Wang et al., 2012). A small irregular semidiurnal tide dominates this area with the average of 0.86 to 1.6 m and maximum of 2.29 to 3.36 m (Wang et al., 2012). The shallow aquaculture pools that were created by the removal of original marsh vegetation (mangrove). The PRD is experiencing massive industrialization and urbanization nowadays and makes great contributions to China’s economy. Take the year of 2021 as an example, this area provided ~12.3% of the national gross domestic product ($1406.18 billion) according to the China Statistical Yearbook 2022. Meanwhile, the coastal habitats of the PRD have been highly productive ecosystems, being an extremely important aquaculture area of China (Zhou et al., 2019). Coastal wetlands reclamation for aquaculture pond took the largest proportion of the total conversion from natural wetlands (estuarine water, mangrove forest and salt marsh) towards constructed wetlands in this region. For example, the breeding area of the PRD increased about threefold from 256.01 km2 in 1980 to 826.72 km2 in 2015 (Zhou et al., 2019). With rapid economic growth and urbanization of the PRD, local environment has suffered from serious environmental problems, especially the high N loading, which has great effects on water quality and N cycling processes (Dai et al., 2008; Li et al., 2013; Wu et al., 2020).
Our samples were selected from three separate coastal ponds aquaculturing different economic species on the western bank of the Pearl River Estuary, one for fish (Perca fluviatilis), one for shrimp (Penaeus vannamei), and one for crab (Scylla serrata) (Figure 1). The sediments were collected using a core cylinder, a PVC pipe handle and a one-way valve from these sites in winter (January 19, 2019) and summer (July 12, 2019). At each site, triplicate surface sediments (0–5 cm) were taken from the cores, and sealed with air-tight, acid-cleaned plastic bags. Overlying water samples of each site were also collected in polyethylene bottles and filtered through 0.2 μm filters (Millipore, Bedford, United States). All the samples were transported to the laboratory on ice within 8 h. In the laboratory, filter samples were immediately stored at -20°C. Sediments of each sampling site were mixed thoroughly under a helium condition, and subsequently divided into three parts. One part was stored at 4°C for measuring microbial biomass carbon (MBC) and N-cycling rates, the second part was frozen at -20°C for determination of physicochemical parameters, and the third part was preserved at -80°C for molecular analysis.
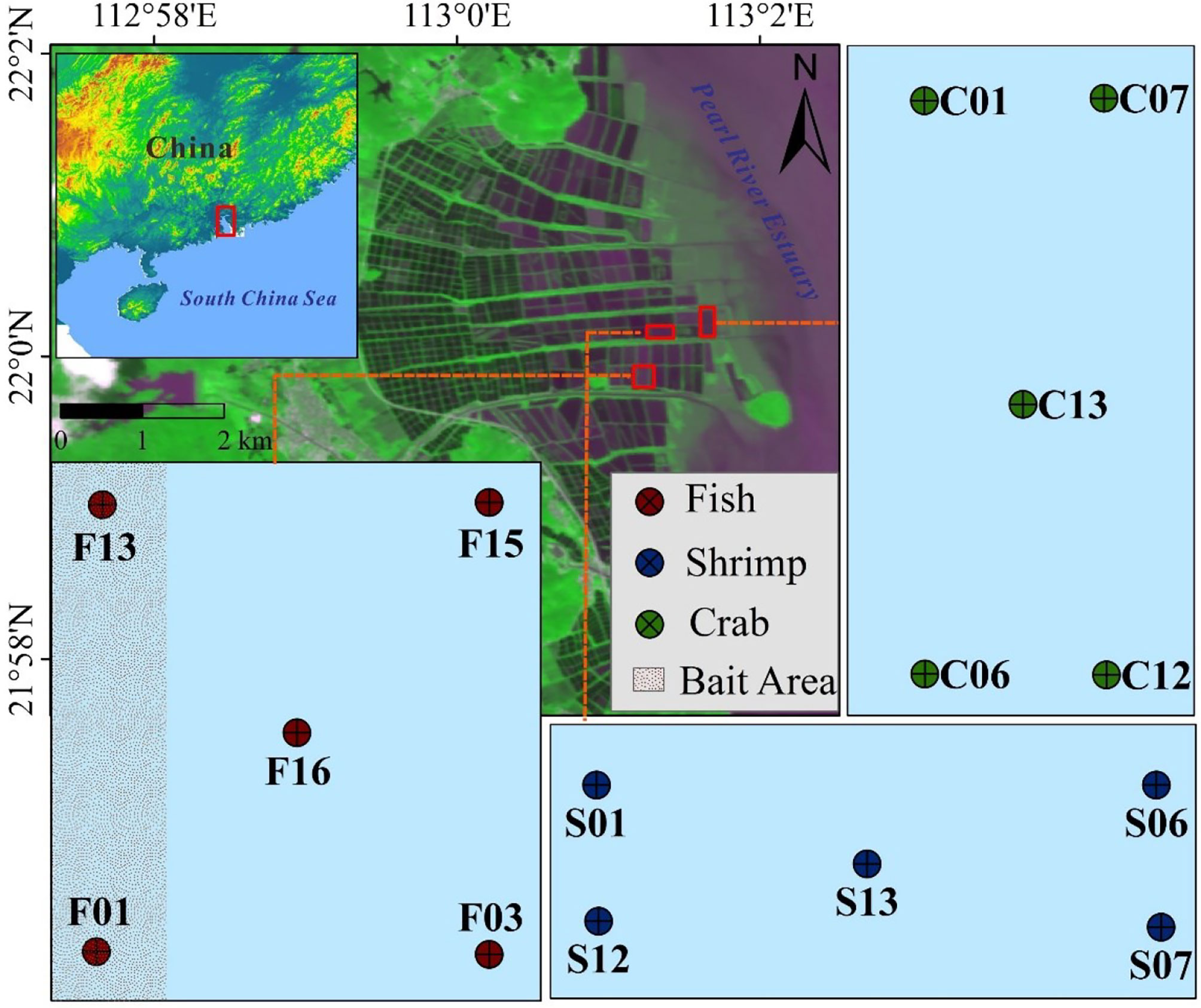
Figure 1 Study area and sampling sites of three types of reclaimed aquaculture ecosystem in the Pearl River Estuary.
2.2. Determination of physicochemical properties
Sediment water content was determined according to the weight loss from fresh sediment after freeze-drying until constant weight. Sediment pH was determined by measuring the pH of the mixture of fresh sediment and Milli-Q (MQ) water (1:2.5 in v/v) (Zhang et al., 2015). Sediment NH4+, NO2−, and NO3− were extracted by 2 M KCl purged with N2 for 30 min and measured using 2 M KCl as standard curve substrate (Hou et al., 2013). Total extractable iron (Fe) and ferrous oxide (Fe2+) were extracted by 0.5 M HCl and 0.25 M hydroxylamine hydrochloride (both purged with N2 for ~15 min) from fresh sediments, and the concentrations were determined using ferrozine-based colorimetric method using the spectrophotometer. The concentrations of ferric iron (Fe3+) were calculated by subtracting Fe2+ from Fe (Lovley and Phillips, 1987; Hu et al., 2022). Sediment texture was determined by laser particle size analyzer LS13 320 (Hou et al., 2013). After effective leaching of carbonates with 1 M HCl, sediment total organic carbon (TOC) and total nitrogen (TN) were measured on an elemental analyzer (Vario EL, Elementar, Germany) (Zhang et al., 2015; Wu et al., 2021). Sediment stable organic carbon isotopic composition (δ13Corg) were analyzed on a Thermo MAT 253Plu isotope ratio mass spectrometer. After oxidation with 333 mmol L–1 KMnO4, sediment easily oxidized organic carbon (EOC) was measured on a spectrophotometer colorimetry (Vieira et al., 2007). Sediment dissolved organic carbon (DOC) was extracted with MQ water and determined with a Shimadzu TOC-TN analyzer (Shimadzu Crop., Kyoto, Japan). Sediment MBC were extracted with K2SO4 and determined through chloroform fumigation-extraction method (Vance et al., 1987; Beck et al., 1997).
2.3. Measurements of N transformation rates
Gross N mineralization (GNM) and NH4+ immobilization (GAI) rates were determined using 15N isotope dilution technique (Kirkham and Bartholomew, 1954). Briefly, twelve centrifuge tubes (50 mL) were prepared for each sample, and 5 g fresh sediment was weighed into each centrifuge tube. For determination of the GNM and GAI rates, six centrifuge tubes with a mixture of sediment and saltwater (1:5 in w/w) were vortexed thoroughly. Tubes were sealed and preincubated for 24 h under in situ temperature in dark on a shaker table (150 rpm). After the preincubation, 15NH4+ (99 atom%, 15NH4Cl) was added into the tubes, the final concentration of 15N was about 2 μg g-1 and the final percentage of 15N was about 10–15%. Triplicate initial samples were terminated by adding 1 mL 50% Zinc chloride (ZnCl2) solution and immediately frozen at -20°C, and triplicates incubation samples were sealed and were put back to the incubator. After 24-hour incubation, final samples were terminated and immediately frozen at -20°C.
Mi and Mf (μg N g-1 dry weight) are total NH4+ concentrations of initial (before incubation) and final (after incubation) samples; Hiand Hf (μg N g-1 dry weight) are 15NH4+ concentrations of initial and final samples; t (d) is the incubation time (24 h).
The relative NH4+ immobilization (RAI) is calculated as the ratio of GAI to GNM rates, and RAI value of ≥ 1 indicates a N-limited sediment, whereas a value of approximately 0.5 indicates N saturation (Aber, 1992). The percentage of NH4+ mineralized per day (PAM%) was referred to as the rates of GNM divided by sediment N contents, which can indicate the sediment available N by the internal N cycle.
2.4. Microbial analysis
Sediment DNA was extracted by the FastDNA spin kit for sediment (MP Biomedical, United States) based on the manufacturer’s instructions. The concentration and purity of extracted DNA were determined by NanoDrop spectrophotometer (NanoDrop 2000C, Thermo Scientific, United States), and the fragments size and quality were evaluated by 1.0% agarose gel electrophoresis. Quantitative PCR (qPCR) assays were used to measure microbial gene abundances on an ABI 7500 Fast real-time qPCR system (Applied Biosystems, United States). For bacterial 16S rRNA gene and fungal ITS gene, primer pairs 341F/519R (Bachar et al., 2010) and SSU081 and 1196R were used (Rousk et al., 2010), respectively. The standard curves for these two genes were created using a 10-fold dilution series (102–109 copies) of the standard plasmids DNA. Each sample was measured in triplicates.
2.5. Calculations and statistical analyses
Statistical analysis was performed using SPSS 19.0 (SPSS Inc., United States). All variables were tested for normality by Shapiro-Wilk test, and the non-normality variable were normalized by using Blom’s formula. Statistically significant difference of N transformation rates and sediment physicochemical properties between three ponds were determined by one-way ANOVA, Turkey test (p<0.05, equal variances assumed) or Dunnett’s test (p<0.05, equal variances not assumed). The correlations were analyzed by and linear regression analyses and Pearson test (two-tailed, p<0.05). The graphs were drawn by ArcGIS 10.2 (ArcMap 10.2, ESRI, United States) and Origin 2019 (OriginLab Corporation, United States).
3. Results
3.1. Physicochemical properties of overlying water
Physicochemical properties of overlying water in the three types of relcaimed aquaculture ponds are shown in Table 1 and Table S1. During winter sampling, crab pond was in the early period of pond-drying, and only a litter overlying water was remaing in the crab ponds, while the shrimp pond was in the late drying period, and the surface sediments were dried and cracked. Therefore, overlying water of the shrimp and crab ponds in winter was not sampled because of that those two ponds were in drying and dreging period. One-way ANOVA showed that overlying water salinity, pH, NH4+, NO3−, and NO2− of the fish pond showed significant seasonal differences (p<0.05), while no significant seasonal difference was found for the dissolved oxygen (DO) (p>0.05). The salinity and pH in winter was higher than those in summer. Influenced by aquaculture activities, NH4+, NO2−, and NO3− were all significantly higher in summer than those in winter (p<0.05). In summer, both DO and salinity showed significantly differences among three ponds (p<0.05 for all). The highest DO was observed in the shrimp ponds (10.30 ± 1.05 mg L-1) followed by the crab ponds (7.90 ± 0.52 mg L-1) and fish ponds (5.11 ± 0.14 mg L-1), which may potentailly result from the most operating waterwheel-type aerators in the shrimp and crab ponds. Consistenly, the highest salinity was measured in the crap ponds (2.85 ± 0.00 ‰) followed by the crab ponds (2.25 ± 0.18 ‰) and fish ponds (0.9 ± 0.04 ‰). The overlying water quality of these ponds were relatively well controlled. Overlying water NH4+ and NO2− of the fish ponds were both significantly higher that those in shrimp and crab ponds (p<0.05).
3.2. Physicochemical properties of sediments
Physicochemical properties of surface sediments in the relcaimed aquaculture ponds are shown in Table 2 and Table S2. Due to geographic proximity, most surface sediment physicochemical properties (excluding NO2−, Fe2+, and TOC/TN) were found no significant spatial variation among those three ponds in summer. In winter, the shrimp and crab ponds were in the drying and dredging period, and the surface sediments of the shrimp ponds were dried and cracked. Thus we found that surface sediment moisture content, Fe2+, EOC, DOC, and MBC of shrimp pond in winter were significantly lower than those in summer (p<0.05 for all), and the sediment bulk density in winter were significantly higher that those in summer (p<0.05). Meanwhile, several sediment physicochemical properties in shrimp pond were significantly higher (including bulk density and δ13Corg) or lower (including moisture content, TOC/TN, DOC, MBC, and bacterial 16S rRNA abundances) that those in other ponds in winter (p<0.05 for all). In crab pond, sediment temperature, NH4+, NO3−, Fe2+, Fe3+, TOC, TN, EOC, and MBC in summer were significantly higher that those in winter (p<0.05 for all). In addition, all the surface sediments in those three ponds were mainly composed of fine silt and clay with low median grain size (7.69−57.77 μm).
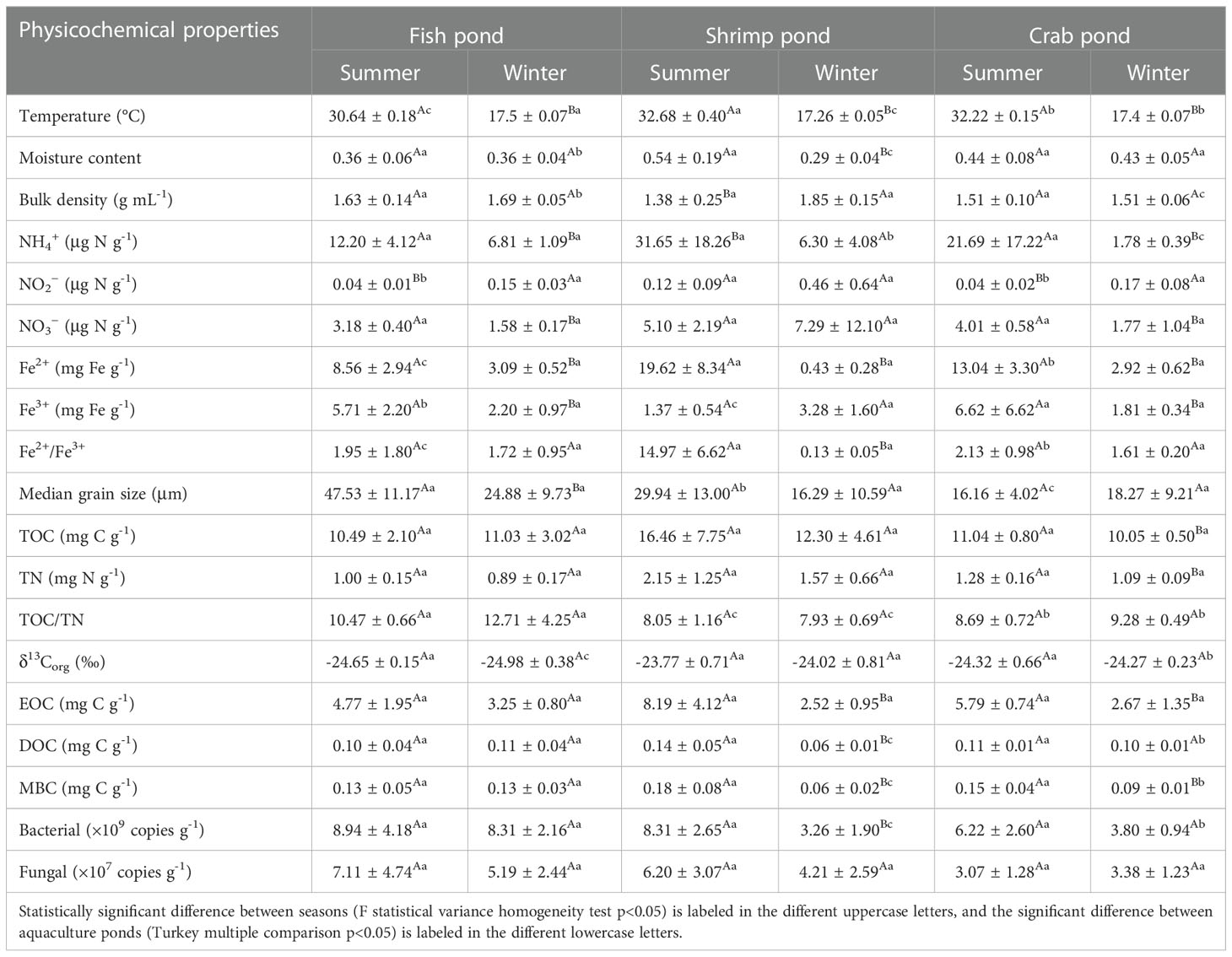
Table 2 Spatiotemporal variations of sediment physicochemical properties in different reclaimed aquaculture ponds.
3.3. Spatiotemporal variations of N transformation rates and microbial abundances
The spatiotemporal distributions of sediment GNM, GAI, RAI, PAM, and microbial abundances in three reclaimed aquaculture ponds were shown in Figure 2. GNM rates varied from 5.77 to 14.58 μg N g-1 d-1 with an average value of 9.64 ± 3.31 μg N g-1 d-1 in summer and from 1.75 to 6.45 μg N g-1 d-1 with an average value of 3.60 ± 1.19 μg N g-1 d-1 in winter. Significant seasonal variations in GNM rates were observed in those three ponds, with significantly higher values in summer than in winter (p<0.05 for all, Figure 2A). No significant difference in GNM rates occurred among three ponds in summer (p>0.05 for all, Figure 2A). In comparison, in summer, the GNM rates in crab pond were significantly lower than those in the other two ponds (p<0.05 for both, Figure 2A).
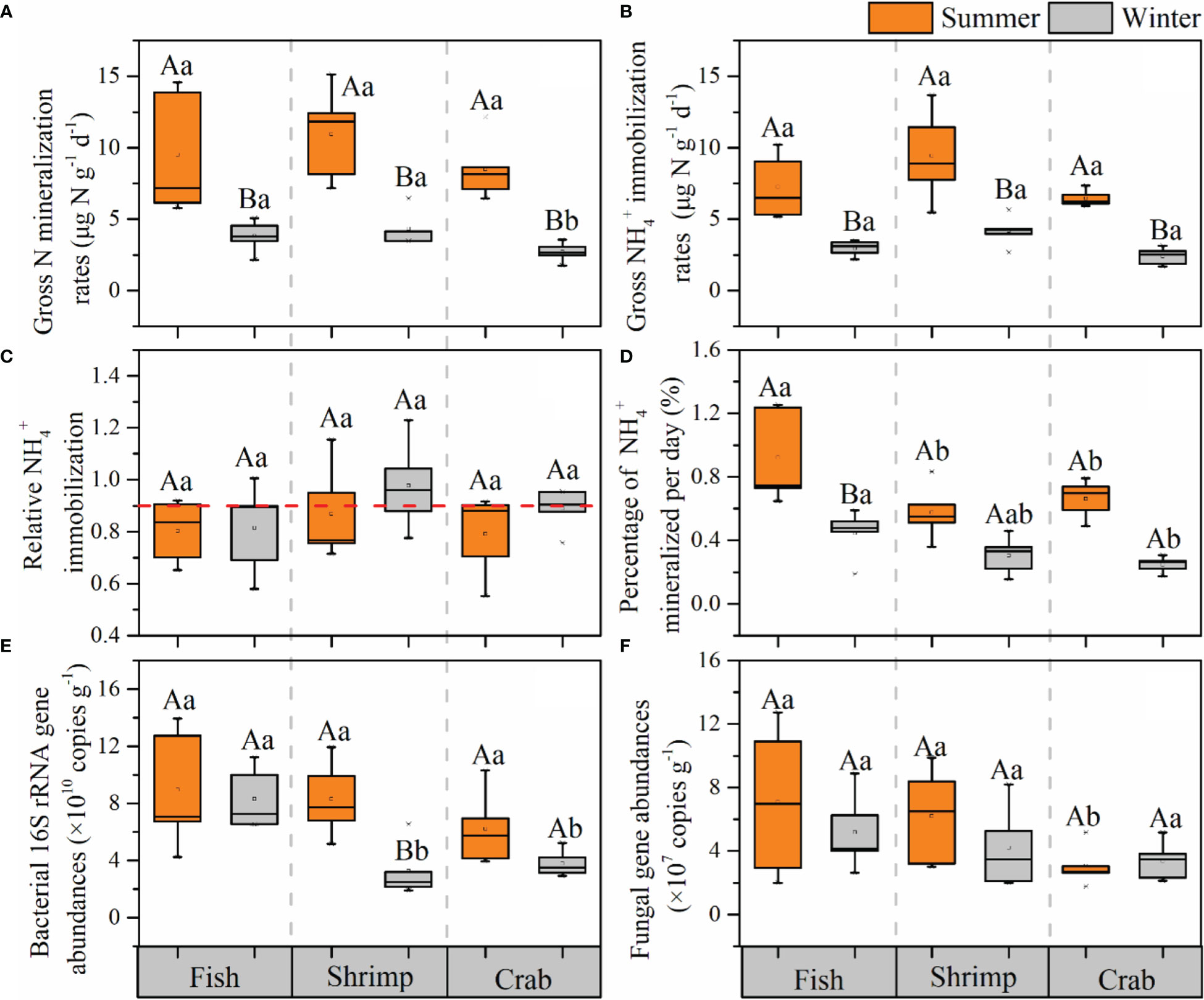
Figure 2 Spatiotemporal variations of gross N mineralization rates (A), gross NH4+ immobilization rates (B), relative NH4+ immobilization (C), percentage of NH4+ mineralized per day (D), and microbial abundances (E, F) in the surface sediments of different reclaimed aquaculture ponds in summer and winter. Statistically significant difference between seasons is labeled in the different uppercase letters, and the significant difference between aquaculture ponds is labeled in the different lowercase letters.
The GAI rates ranged from 5.15 to 13.68 μg N g-1 d-1 with an average of 7.71 ± 2.49 μg N g-1 d-1 in summer and from 1.67 to 5.66 μg N g-1 d-1 with an average of 3.17 ± 1.06 μg N g-1 d-1 in winter. Similarly, GAI rates were higher in summer than in winter with a remarkable seasonal difference within these three ponds (p<0.05 for all, Figure 2B). Spatially, no significant spatial difference in GAI rates occurred among three ponds in both summer and winter (p>0.05 for all, Figure 2B). In addition, GNM rates were significantly positively correlated with GAI rates in the whole study area in both summer and winter (p<0.05 for both, Figure S1).
The relative NH4+ immobilization (RAI) values ranged between 0.55 and 1.15 in summer and between 0.60 and 1.23 in winter (Figure 2C). There was no significant seasonal variation in RAI in those three ponds, and no significant spatial difference among three ponds (p > 0.05 for all; Figure 2C). A previous study has confirmed that RAI ≤ 0.5 and RAI > 0.9 indicated N-saturated environment and N-limited environment, respectively (Aber, 1992). Here, the RAI values in most sampling sites were between 0.5 and 0.9, indicating these reclaimed aquaculture ecosystems were able to maintain N balance.
PAM values ranged from 0.36% to 1.25% in summer and from 0.15% to 0.59% in winter (Figure 2D). Spatially, PAM in fish pond were significantly higher than those in other ponds (p< 0.05 for all; Figure 2D). The PAM in summer (0.92 ± 0.30%) was significantly higher than that in winter (0.45 ± 0.15%) in fish pond (p< 0.05; Figure 2D). In shrimp and crab ponds, PAM in summer were also higher than those in winter, but with no significant seasonal difference (p > 0.05; Figure 2D).
The bacterial 16S rRNA gene abundances were in a range of 3.93–13.94×109 copies g-1 with an average of 7.82 ± 3.22×109 copies g-1 in summer. In winter, the range and average value decreased to 1.91–11.22×109 copies g-1 and 5.12 ± 2.85×109 copies g-1. The bacterial 16S rRNA abundances in summer were significantly higher than those in winter in shrimp pond (p< 0.05; Figure 2E).The values in sediment of fish pond were significantly higher than those in other two ponds in winter (p<0.05 for both, Figure 2E). The fungal ITS gene abundances were in a range of 1.76–12.73×107 copies g-1 with an average of 5.46 ± 3.57×107 copies g-1 in summer and in a range of 2.00–8.88×107 copies g-1 with an average of 4.26 ± 2.15×107 copies g-1 in winter, showing no significant temporal differences in those three ponds and no spatial differences among three ponds (p< 0.05 for all; Figure 2F).
3.4. Effects of physicochemical properties on N-cycling processes
Considering all sampling sites, GNM and GAI rates showed significant positive correlations with sediment temperature, organic matter (TOC, TN, EOC, DOC, and MBC), microbial abundances (bacterial 16S rRNA and fungal abundances), Fe2+, NH4+, moisture content, as well as overlying water DO and NO3−, while they were correlated negatively with sediment bulk density (p<0.05; Table 3). PAM were significantly positively correlated with sediment MBC, microbial abundances, Fe2+, Fe3+, as well as overlying water DIN (p<0.05; Table 3). RAI were positively correlated with sediment δ13Corg and correlated negatively with sediment microbial abundances, MBC, DOC, and TOC/TN (p<0.05; Table 3).
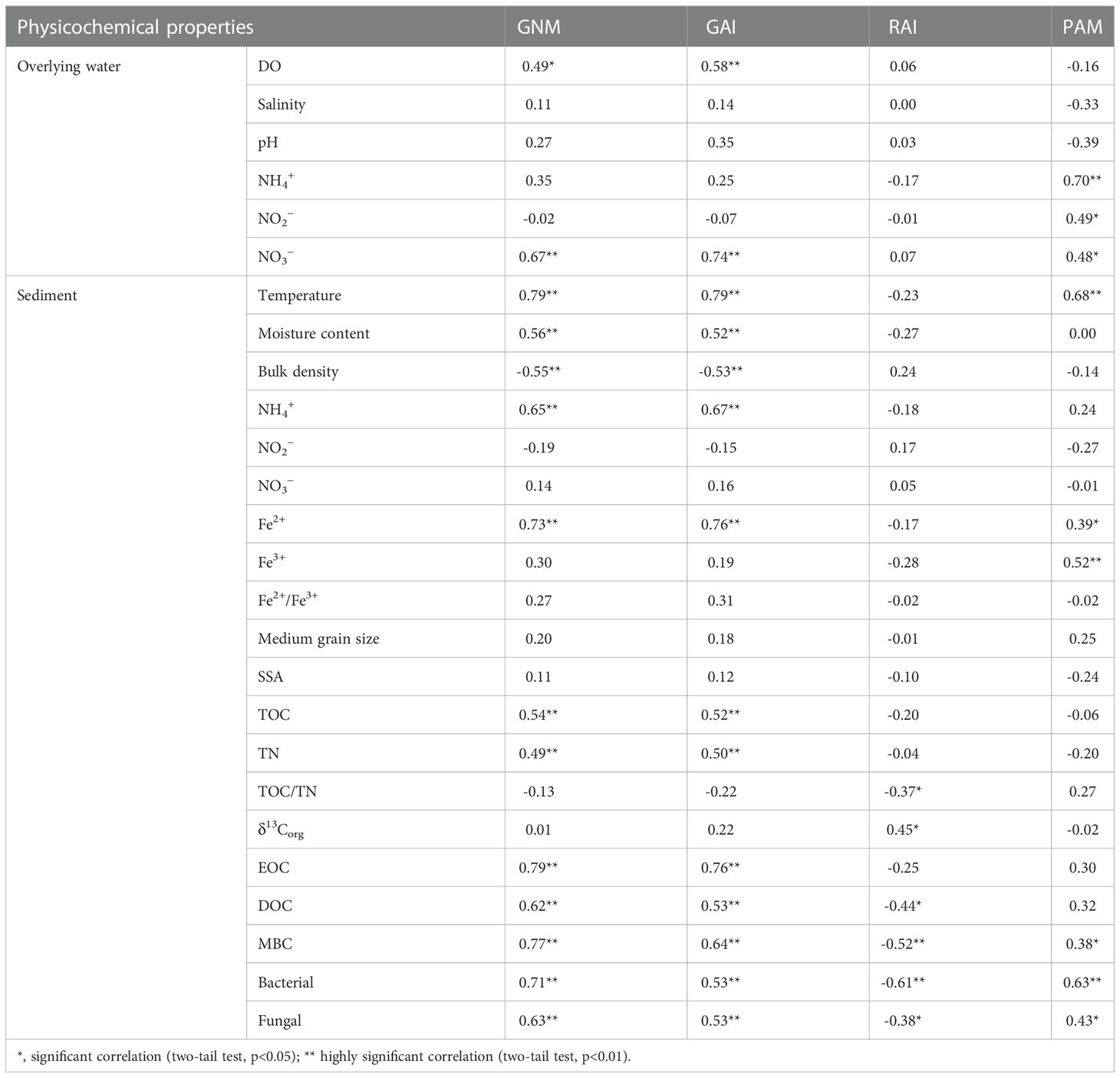
Table 3 Correlations between physicochemical properties and N mineralization and immobilization rates.
4. Discussion
4.1. Effects of physicochemical properties on sediment N mineralization and immobilization
Temperature could have directly impacts on microbial physiological activities through the regulation of enzyme activities and is considered as a crucial factor of N mineralization and immobilization (Yang et al., 2010). In this study, GNM and GAI rates were both significantly higher in summer than in winter among those three ponds (p<0.05 for all). The seasonal variations are mainly due to temperature differences between summer (31.81 ± 0.86°C) and winter (17.54 ± 0.05°C). Also, the ratios of N-cycling rates in summer to rates in winter were used to characterize the temperature sensitivity of N mineralization and immobilization rates. A previous study found that total N immobilization rate is very sensitive to temperature while N mineralization rate shows relatively less sensitivity under low temperature condition (5–15°C) (Andersen and Jensen, 2001). However, we found that no significant difference between the temperature sensitivity of GNM and GAI (Figure 3A). The major reson is that the winter temperature in our study is over 15°C, and the temperature sensitivity of N mineralization has been found to decrease with increasing temperature (Kirschbaum, 1995). In addition, no significant spatial difference in temperature sensitivity of N mineralization and immobilization rates among those three ponds (Figure 3A). This is mainly due to similar physicochemical properties (including sediment grain size, organic matter, DIN, and TOC : TN) among those three ponds, and previous studies have found that temperature sensitivity of N mineralization and immobilization rates mainly depended on the above physicochemical properties in agricultural ecosystems (Liu et al., 2017; Miller and Geisseler, 2018).
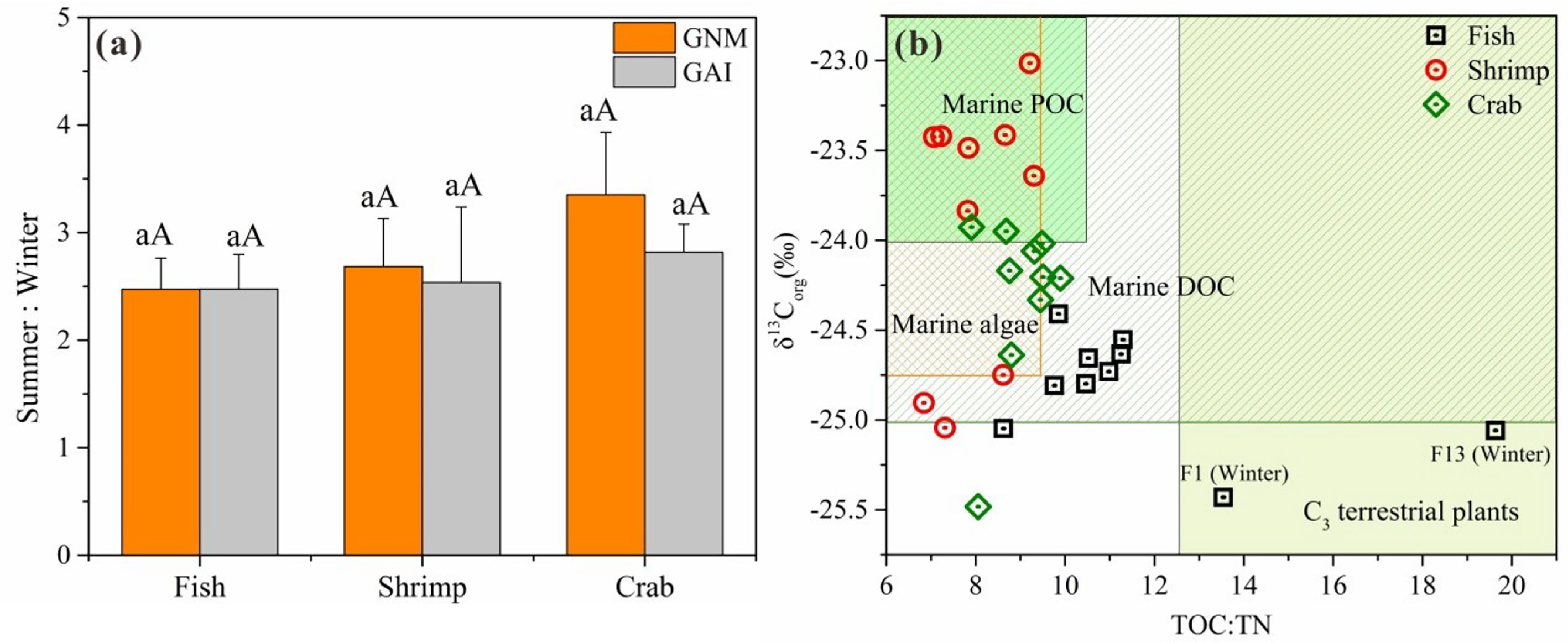
Figure 3 The temperature sensitivity of sediment GNM and GAI rates to seasonal change in those three ponds (A); Determination of organic matter sources from autochthonous or allochthonous (B). The backgrond ranges of organic matter difference sources were obtained by Lamb et al. (2006). Statistically significant difference between seasons is labeled in the different uppercase letters, and the significant difference between aquaculture ponds is labeled in the different lowercase letters. The error bar represents the standard error.
Organic matter of aquaculture sediments originates from both autochthonous sources (microalgae, sediment and particulate organic matter) and bait inputs (Chen et al., 2016). According to the values of TOC/N ratio and δ13Corg, we found the surface sediment organic matter were mainly from marine algae, marine DOC, and marine POC (Figure 3B). This is caused mainly by the fact that the aquaculture ponds were located on the estuary, where a significant proportion of overlying water is derived from marine. More importantly, the main ingredients (~60%) of bait are fish meal and fish oil (Park et al., 2021), and they were made from marine fish (e.g. Engraulis japonicus).
As we all know, organic matter acting as energy source of microorganisms and substrates of N mineralization, which plays an important role in microbial-mediated N-cycling processes. To clarify how labile C impacts on N mineralization and immobilization in the reclaimed aquaculture sediments, several labile organic carbons including sediment EOC, DOC, and MBC, as well as potential N mineralization and immobilization rates, were measured. When considering all sites, the correlation strengths between labile organic carbons (EOC, DOC, and MBC) and N mineralization and immobilization rates were much higher than those between TOC and the rates. The order of above correlations was MBC>EOC>DOC>TOC (Table 3). When considering seasonal effects, we found that this regularity and trend was only found in summer (Figure 4), and the order of above correlations was changed slightly (MBC>DOC>EOC>TOC). This finding agreed well with several previous studies, which found that high availability of organic matter is favorable for soil/sediment N mineralization process in coastal wetland (Li et al., 2020; Yang et al., 2022), estuary (Huang et al., 2022) and forest (Jones and Kielland, 2012). Thus, our results suggest that organic quality rather than quantity was more crucial to the control of N mineralization and immobilization in aquaculture sediments.
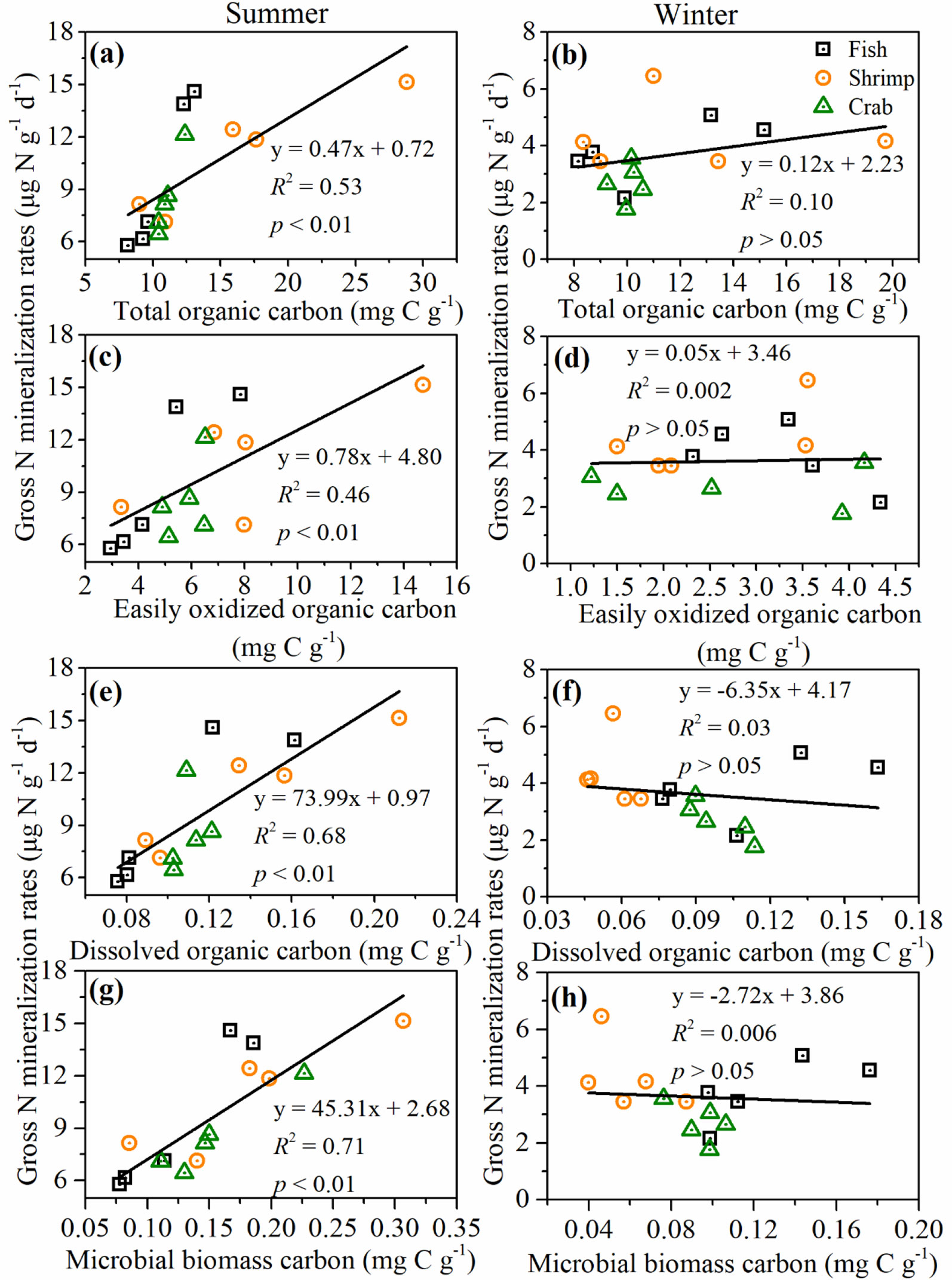
Figure 4 Correlations between different organic matter compounds and gross N mineralization rates in the surface sediments of different reclaimed aquaculture ponds. (A, B) Total organic carbon; (C, D) easily oxidized organic carbon; (E, F) dissolved organic carbon; and (G, H) microbial biomass carbon.
No significant correlations existed between organic matter and N mineralization and immobilization rates in winter, this may be probably due to the profound effect of human activities (pond-drying) on these different aquaculture ponds. As mentioned in the preceding text, fish ponds are still being farmed, and shrimp and crab ponds are in a resting state in winter. Namely, shrimp and crab ponds were in the drying and dredging period, and surface sediment of the shrimp ponds was dried and cracked. Firstly, pond-drying can significantly reduce the sediment moisture content in shrimp ponds in winter, previous studies have found that N mineralization increased with the increasing soil/sediment moisture (Jia et al., 2019; Li et al., 2020; Huang et al., 2022). Sediment moisture is involved in the metabolism of microbes and is also the irreplaceable medium linking substrates supply and microbial activity (Greaver et al., 2016). Elevated sediment moisture has also been reported to increase the decomposition and leaching of organic matter and extracellular enzyme activities, promoting soil N transformations (Mooshammer et al., 2014; Greaver et al., 2016; Jia et al., 2017). Also, increased soil moisture could limit oxygen to penetrate soils and further lead to more strongly anoxic and reducing environments, thereby facilitating denitrification and N mineralization in coastal wetlands (Jia et al., 2019). Secondly, our measured sediment labile organic matter contents (including EOC, DOC, and MBC) in shrimp pond were significantly less in winter compared with those in summer (Table 2, p<0.05 for all). Thus, pond-drying can accelerate the decomposition of surface sediment organic matter, resulting in decreased the supply of energy source and substrate for microbial growth and mineralization. In addition, pond-drying can significantly reduce microbial biomass (MBC) and bacterial abundances in shrimp pond (Table 2, p<0.05 for both), thereby regulating the N mineralization and immobilization. Therefore, most physicochemical properties were significantly changed with aquaculture management activities (pond-drying), resulting in the profound reduction of sediment N mineralization and immobilization in aquaculture ecosystems.
No significant seasonal and spatial difference in microbial abundances was observed in our study excluding those in shrimp ponds in winter (Table 2), this is largely due to small summer-winter temperature differences and low spatial heterogeneity in sediment physicochemical properties. When the surface sediment was dried and cracked after long-term pond-drying, this aquaculture management activity can significantly reduce the microbial biomass and bacterial abundances, but not for fungal abundances (Table 2). This is mainly due to fungi can be remarkably drought tolerant, and they can remain active and even grow under extremely dry conditions (Treseder et al., 2010; Yuste et al., 2011). However, our study lack of data about microbial community structure and diversity. A previous study showed that pond-drying can change the sediment microbial community structure, and the abundance of Proteobacteria, Nitrospirae and Bacteroidetes were increased, while the pernicious microbe such as Cyanobacteria was decreased after pond-drying in aquaculture ecosystem (Wang et al., 2020). In addition, natural sunlight has effect on the abundance and microbial community structure in the sediments during pond-drying. Thus, we boldly speculated that pond-drying can alter microbial community composition from anaerobic, fast-growing, and copiotrophic to aerobic, slow-growing, and oligotrophic, thus promote the ecosystem reparation effectively.
Many previous studies have reported that N mineralization and immobilization rates were affected by the microbial biomass and activities, and thus they were generally closely related to the microbial respiration rates, enzymatic activities (including extracellular and intracellular enzymes), and ATP content (Bengtsson et al., 2003; Silva et al., 2005). N mineralization and immobilization rates were also controlled by the microbial biomass (MBC) and abundances (including bacterial 16S rRNA and fungal abundances) in this study (Table 4), which is consistent with previous sudies in many estuarine and coastal zone (Lin et al., 2016a; Li et al., 2020; Huang et al., 2022; Yang et al., 2022). Thus, microbial abundances could be considered as important indicators of N mineralization and immobilization rates in aquaculture sediments.
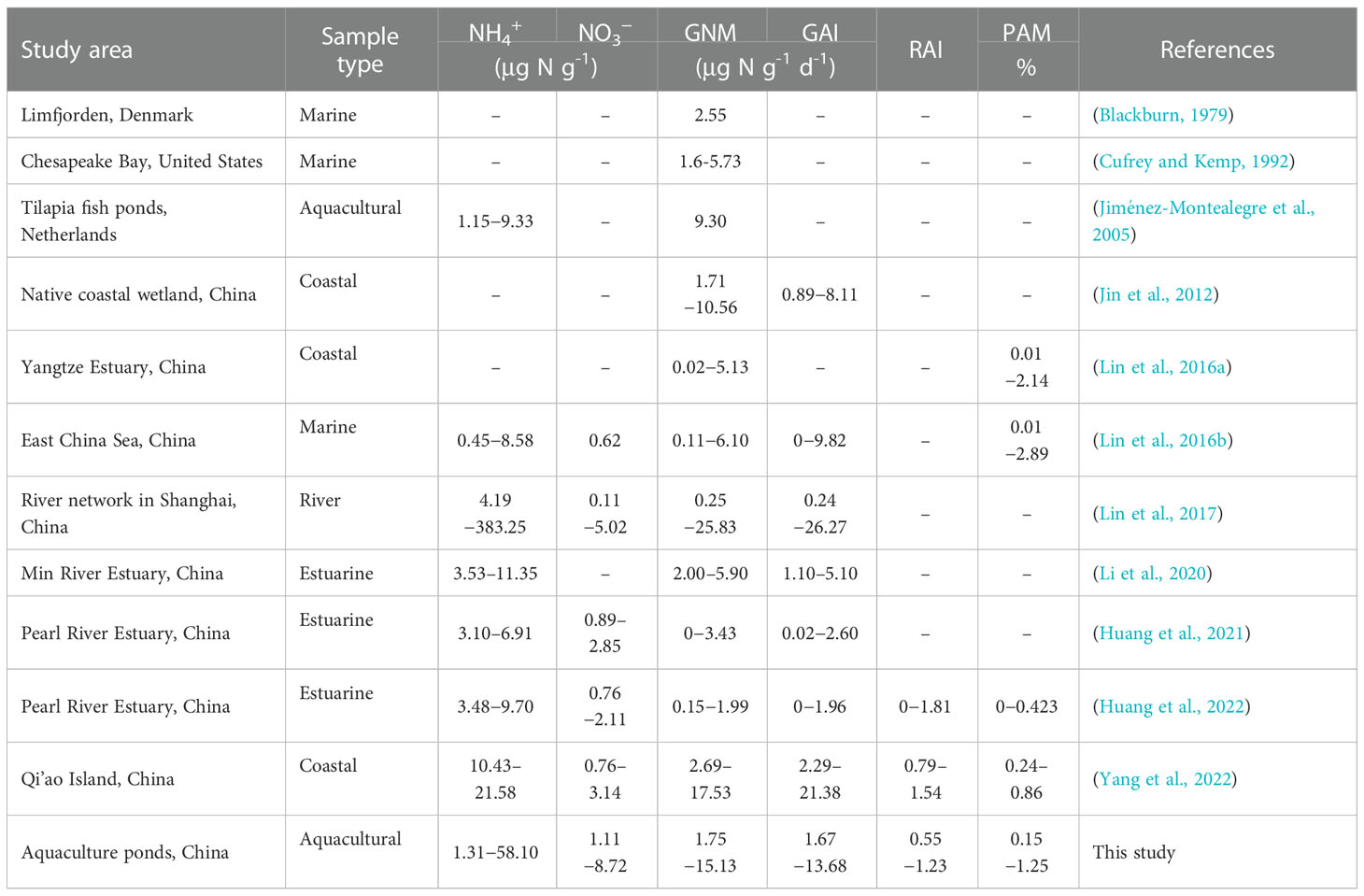
Table 4 Comparison of N mineralization and immobilization in coastal, estuarine, and aquacultural ecosystems and our study area.
In addition, GNM and GAI rates were also positively correlated with overlying water NO3− (Table 3, p<0.05). Previous studies have revealed significant influence of environmental effective electron acceptors on N mineralization. For example, NO3−, as an electron acceptor of oxidized organic matter, could promote N mineralization (equation 3) and make high contribution (15−35%) to the mineralizaiont of organic matter especially under anoxic conditions (Khalil et al., 2018). Under anaerobic conditions, NO3−, Fe3+, SO42− as well as iron and manganese ions often work as active electron acceptors for coupling redox reactions with sediment organic matter (equation 4 and 5) (Pena et al., 2010). As sediment is flooded for long time and the vertical penetration ability of oxygen from overlying water into sediment is relatively weak, anaerobic conditions for the aforementioned reactions could be created in aquaculture ecosystem. Additional work regarding aerobic N mineralization can be done to compare the mechanisms between anoxic environments and aerobic environments.
4.2. Environmental implications for N mineralization and immobilization in reclaimed aquaculture ecosystems
The average N mineralization and immobilization rates of reclaimed aquaculture ponds in this study area were lower than the rates of other aquaculture, urban river network, and mangrove wentland sediments, and higher than the rates of estuarine and coastal sediments (Table 4). The daily mineralization percentage of NH4+ was 0.55−1.23%, which as comparable with the value in coastal wetlands (Table 4). In addition, the value of RAI in this study (average: 0.86 ± 0.15; range: 0.58−1.23) implied that the ecosystem might be located between N limitation condition and N saturation condition (Aber, 1992). Significantly positive correlations between GNM and GAI identified in this study showed consistency with the observations in offshore seawater (Lin et al., 2016), wetlands (Jin et al., 2012), grassland (Corre et al., 2002) and forests (Bengtsson et al., 2003). It’s also noteworthy that a combination of sediment incubation experiment and 15N stable isotope dilution method was adopted in this study. As described in Lin et al. (2017), the added 15NH4+ may stimulate microbial responses, along with the dilution ratio of 1:5 (fresh sediment: water) and aerobic incubation conditions, the measured GNM and GAI might not reflect in situ activities. Nevertheless, it can still imply potential activities of N mineralization and immobilization in aquaculture surface sediments.
Sediment N mineralization and immobilization are important N-cycling processes in coastal wetland reclamation and aquaculture ecosystems, which plays vital roles in the reactive N balance. However, very few systematic studies investigated environmental properties, N mineralization and immobilization, and their relationships, especially in reclaimed aquaculture ecosystems. Here, based on GNM and GNI measured in this study, annual surface sediment (0−5 cm) N mineralization and immoblization rates in reclaimed aquaculture ecosystems of the Great Bay Area were calculated (equation 6).
In which, F (t N yr-1) is annual N mineralization or immoblization flux; mi and mj (μg N g-1 d-1) is GNM or GAI of surface sediment (0−5 cm) in summer and winter, respectively; di and dj (g cm-3) is bulk density of surface sediment (0−5 cm) in summer and winter, respectively; a is a unit conversion factor; s (m2) is the area of reclaimed aquaculture ecosystems in the Great Bay Area (~395.57 km2 according to remote sensing data in 2018 followed by the calclution using ArcGIS 10.2) (Figure S2); h (cm) is sampling depth (5 cm); t (d) is time (365 days).
In total, annual sediment N mineralization and immoblization rates in reclaimed aquaculture ecosystems are approximately 4.55×104 t N yr-1 and 3.68×104 t N yr-1, respectively in the Guangdong-Hong Kong-Macao Greater Bay Area. GNM flux shows higher level than GAI flux, indicating that sediment is a nonnegligible DIN source in the aquaculture ecosystem, which plays a non-negligible role in the exacerbation of eutrophication in the estuarine and coastal ecosystems.
5. Conclusions
This study investigated N mineralization and immobilization rates in surface sediments of the reclaimed aquaculture ecosystems. GNM and GAI rates ranged from 1.75 to 15.13 μg N g-1 d-1 and from 1.67 to 13.68 μg N g-1 d-1, respectively. Both GNM and GAI rates were significantly higher in summer than their counterparts in winter (p<0.05). No significant differences among three types of aquaculture ecosystems were observed (p>0.05). N mineralization and immobilization rates were significantly correlated with overlying water NO3−, as well as sediment moisture content, bulk density, organic matter, Fe2+, and microbial abundances. Furthermore, this study estimated the total mineralized and immobilized N in aquaculture surface sediments from the Guangdong-Hong Kong-Macao Greater Bay Area, with the estimation of approximately 4.55×104 t N yr-1 and 3.68×104 t N yr-1, respectively. GNM flux shows higher level than GAI flux, indicating that sediment is a crucial DIN source in the aquaculture ecosystem, which plays a non-negligible role in the exacerbation of eutrophication. Overall, this study improves the understanding of sediment N transformation processes and relevant regulation mechanisms in the reclaimed aquaculture ecosystems.
Data availability statement
The original contributions presented in the study are included in the article/Supplementary Material. Further inquiries can be directed to the corresponding author.
Author contributions
XL: Methodology, Validation, Formal analysis, Investigation, Data curation, Writing-original draft, Writing-review & editing, Funding acquisition. GL: Writing-original draft, Formal analysis, Writing-review & editing. YZ: Writing-original draft, Formal analysis, Visualization, Funding acquisition. WL: Investigation, Data curation, Writing - review & editing. PG: Data curation, Formal analysis, Writing - review & editing. SF: Writing-original draft, Formal analysis. TK: Data curation, Formal analysis. DT: Formal analysis, Data curation, Supervision. DS: Investigation, Data curation. ZS: Writing-original draft, Writing - review & editing, Funding acquisition. All authors contributed to the article and approved the submitted version.
Funding
This work was supported by the Natural Science Foundation of China (grant numbers: 31970486, 42001088), Fundamental Research Funds for the Central Universities (202262007), Innovation Group Project of Southern Marine Science and Engineering Guangdong Laboratory (Zhuhai) (311021004), the Guangdong Basic and Applied Basic Research Foundation (2020A1515010908), the Science and Technology Program of Guangzhou (No. 202002030453) and the Special fund for scientific innovation strategy-construction of high-level Academy of Agriculture Science (R2020YJ-YB3006).
Acknowledgments
We thank Prof. Jun Gong, Prof. Kedong Yin, Prof. Lijun Hou, Prof. Min Liu, and Prof. Qiangtai Huang for providing the test platform. We thank Zexin Li, Bin Wang, Rixuan Gao, Yijing Liu, Yijing Shen, Yongyi Peng, and Shuai Li for data collection. We also thank Prof. Bin Ai, Prof. Jun Zhao and Ke Huang for the help in the data about Figure S1. Thanks are given to the editor and reviewers for valuable comments on this manuscript.
Conflict of interest
The authors declare that the research was conducted in the absence of any commercial or financial relationships that could be construed as a potential conflict of interest.
Publisher’s note
All claims expressed in this article are solely those of the authors and do not necessarily represent those of their affiliated organizations, or those of the publisher, the editors and the reviewers. Any product that may be evaluated in this article, or claim that may be made by its manufacturer, is not guaranteed or endorsed by the publisher.
Supplementary material
The Supplementary Material for this article can be found online at: https://www.frontiersin.org/articles/10.3389/fmars.2022.1093279/full#supplementary-material
References
Aber J. D. (1992). Nitrogen cycling and nitrogen saturation in temperate forest ecosystems. Trends Ecol. Evol. 7 (7), 220–224. doi: 10.1016/0169-5347(92)90048-G
Amano T., Yoshinaga I., Yamagishi T., Van Thuoc C., Thu P., Ueda S., et al. (2011). Contribution of anammox bacteria to benthic nitrogen cycling in a mangrove forest and shrimp ponds, haiphong, Vietnam. Microbes Environ. 26 (1), 1–6. doi: 10.1264/jsme2.ME10150
Andersen M. K., Jensen L. S. (2001). Low soil temperature effects on short-term gross n mineralisation-immobilisation turnover after incorporation of a green manure. Soil Biol. Biochem. 33 (4-5), 511–521. doi: 10.1016/S0038-0717(00)00192-9
Bachar A., Al-Ashhab A., Soares M. I. M., Sklarz M. Y., Angel R., Ungar E. D., et al. (2010). Soil microbial abundance and diversity along a low precipitation gradient. Microbial Ecol. 60, 453–461. doi: 10.1007/s00248-010-9727-1
Bai J., Gao H., Xiao R., Wang J., Huang C. (2012). A review of soil nitrogen mineralization as affected by water and salt in coastal wetlands: issues and methods. CLEAN-Soil Air Water 40 (10), 1099–1105. doi: 10.1002/clen.201200055
Beck T., Joergensen R. G., Kandeler E., Makeschin F., Nuss E., Oberholzer H. R., et al. (1997). An inter-laboratory comparison of ten different ways of measuring soil microbial biomass c. Soil Biol. Biochem. 29 (7), 1023–1032. doi: 10.1016/S0038-0717(97)00030-8
Bengtsson G., Bengtson P., Månsson K. F. (2003). Gross nitrogen mineralization-, immobilization-, and nitrification rates as a function of soil C/N ratio and microbial activity. Soil Biol. Biochem. 35 (1), 143–154. doi: 10.1016/S0038-0717(02)00248-1
Blackburn T. H. (1979). Method for measuring rates of NH4+ turnover in anoxic marine sediments, using a 15N-NH4+ dilution technique. Appl. Environ. Microbiol. 37 (4), 760–765. doi: 10.1128/aem.37.4.760-765.1979
Buzzelli C., Wan Y., Doering P. H., Boyer J. N. (2013). Seasonal dissolved inorganic nitrogen and phosphorus budgets for two sub-tropical estuaries in south Florida, USA. Biogeosciences 10 (10), 6721–6736. doi: 10.5194/bg-10-6721-2013
Cao L., Diana J. S., Keoleian G. A., Lai Q. (2011). Life cycle assessment of Chinese shrimp farming systems targeted for export and domestic sales. Environ. Sci. Technol. 45 (15), 6531–6538. doi: 10.1021/es104058z
Chen Y., Dong S., Wang F., Gao Q., Tian X. (2016). Carbon dioxide and methane fluxes from feeding and no-feeding mariculture ponds. Environ. pollut. 212, 489–497. doi: 10.1016/j.envpol.2016.02.039
Corre M. D., Schnabel R. R., Stout W. L. (2002). Spatial and seasonal variation of gross nitrogen transformations and microbial biomass in a northeastern US grassland. Soil Biol. Biochem. 34 (4), 445–457. doi: 10.1016/S0038-0717(01)00198-5
Cufrey J. M., Kemp W. M. (1992). Influence of the submersed plant, potamogeton perfoliatus, on nitrogen cycling in estuarine sediments. Limnology Oceanography 37 (7), 1483–1495. doi: 10.4319/lo.1992.37.7.1483
Dai M., Wang L., Guo X., Zhai W., Li Q., He B., et al. (2008). Nitrification and inorganic nitrogen distribution in a large perturbed river/estuarine system: The pearl river estuary, China. Biogeosciences 5 (5), 1227–1244. doi: 10.5194/bg-5-1227-2008
Diaz R. J., Rosenberg R. (2008). Spreading dead zones and consequences for marine ecosystems. Science 321 (5891), 926–929. doi: 10.1126/science.1156401
Galloway J. N., Townsend A. R., Erisman J. W., Bekunda M., Cai Z., Freney J. R., et al. (2008). Transformation of the nitrogen cycle: recent trends, questions, and potential solutions. Science 320 (5878), 889–892. doi: 10.1126/science.1136674
Gao H., Bai J., He X., Zhao Q., Lu Q., Wang J. (2014). High temperature and salinity enhance soil nitrogen mineralization in a tidal freshwater marsh. PloS One 9 (4), e95011. doi: 10.1371/journal.pone.0095011
Greaver T. L., Clark C. M., Compton J. E., Vallano D., Talhelm A. F., Weaver C. P., et al. (2016). Key ecological responses to nitrogen are altered by climate change. Nat. Climate Change 6 (9), 836e843. doi: 10.1038/nclimate3088
Herbert R. A. (1999). Nitrogen cycling in coastal marine ecosystems. FEMS Microbiol. Rev. 23 (5), 563–590. doi: 10.1111/j.1574-6976.1999.tb00414.x
Hou L., Zheng Y., Liu M., Gong J., Zhang X., Yin G., et al. (2013). Anaerobic ammonium oxidation (anammox) bacterial diversity, abundance, and activity in marsh sediments of the Yangtze estuary. J. Geophysical Research: Biogeosciences 118 (3), 1237–1246. doi: 10.1002/jgrg.20108
Howarth R., Chan F., Conley D. J., Garnier J., Doney S. C., Marino R., et al. (2011). Coupled biogeochemical cycles: eutrophication and hypoxia in temperate estuaries and coastal marine ecosystems. Front. Ecol. Environ. 9 (1), 18–26. doi: 10.1890/100008
Huang F., Lin X., Hu W., Zeng F., He L., Yin K. (2021). Nitrogen cycling processes in sediments of the pearl river estuary: Spatial variations, controlling factors, and environmental implications. Catena 206, 105545. doi: 10.1016/j.catena.2021.105545
Huang F., Lin X., Yin K. (2022). Effects of algal-derived organic matter on sediment nitrogen mineralization and immobilization in a eutrophic estuary. Ecol. Indic. 138, 108813. doi: 10.1016/j.ecolind.2022.108813
Hu M., Sardans J., Le Y., Yan R., Zhong Y., Huang J., et al. (2022). Biogeochemical behavior of p in the soil and porewater of a low-salinity estuarine wetland: Availability, diffusion kinetics, and mobilization mechanism. Water Res. 219, 118617. doi: 10.1016/j.watres.2022.118617
Jia J., Bai J. H., Gao H. F., Wang W., Yin S., Wang D. W., et al. (2019). Effects of salinity and moisture on sediment net nitrogen mineralization in salt marshes of a Chinese estuary. Chemosphere 228, 174–182. doi: 10.1016/j.chemosphere.2019.04.006
Jia J., Bai J. H., Gao H. F., Wen X. J., Zhang G. L., Cui B. S., et al. (2017). In situ soil net nitrogen mineralization in coastal salt marshes (Suaeda salsa) with different flooding periods in a Chinese estuary. Ecol. Indic. 73, 559e565. doi: 10.1016/j.ecolind.2016.10.012
Jiménez-Montealegre R., Verdegem M. C., Van Dam A. A., Verreth J. A. (2005). Effect of organic nitrogen and carbon mineralization on sediment organic matter accumulation in fish ponds. Aquaculture Res. 36 (10), 1001–1014. doi: 10.1111/j.1365-2109.2005.01307.x
Jin X., Huang J., Zhou Y. (2012). Impact of coastal wetland cultivation on microbial biomass, ammonia-oxidizing bacteria, gross n transformation and N2O and NO potential production. Biol. Fertility Soils 48 (4), 363–369. doi: 10.1007/s00374-011-0631-8
Jones D. L., Kielland K. (2012). Amino acid, peptide and protein mineralization dynamics in a taiga forest soil. Soil Biol. Biochem. 55, 60e69. doi: 10.1016/j.soilbio.2012.06.005
Khalil K., Laverman A. M., Raimonet M., Rabouille C. (2018). Importance of nitrate reduction in benthic carbon mineralization in two eutrophic estuaries: Modeling, observations and laboratory experiments. Mar. Chem. 199, 24–36. doi: 10.1016/j.marchem.2018.01.004
Kirkham D. O. N., Bartholomew W. V. (1954). Equations for following nutrient transformations in soil, utilizing tracer data. Soil Sci. Soc. America J. 18 (1), 33–34. doi: 10.2136/sssaj1954.03615995001800010009x
Kirschbaum M. U. (1995). The temperature dependence of soil organic matter decomposition, and the effect of global warming on soil organic c storage. Soil Biol. Biochem. 27 (6), 753–760. doi: 10.1016/0038-0717(94)00242-S
Lamb A. L., Wilson G. P., Leng M. J. (2006). A review of coastal palaeoclimate and relative sea-level reconstructions using δ13C and C/N ratios in organic material. Earth-Science Rev. 75 (1-4), 29–57. doi: 10.1016/j.earscirev.2005.10.003
Li M., Cao H., Hong Y., Gu J. D. (2013). Using the variation of anammox bacteria community structures as a bio-indicator for anthropogenic/terrestrial nitrogen inputs in the pearl river delta (PRD). Appl. Microbiol. Biotechnol. 97 (22), 9875–9883. doi: 10.1007/s00253-013-4990-y
Li X., Hou L., Liu M., Tong C. (2020). Biogeochemical controls on nitrogen transformations in subtropical estuarine wetlands. Environ. pollut. 263, 114379. doi: 10.1016/j.envpol.2020.114379
Lin X., Hou L., Liu M., Li X., Yin G., Zheng Y., et al. (2016a). Gross nitrogen mineralization in surface sediments of the Yangtze estuary. PloS One 11 (3), e0151930. doi: 10.1371/journal.pone.0151930
Lin X., Hou L., Liu M., Li X., Zheng Y., Yin G., et al. (2016b). Nitrogen mineralization and immobilization in sediments of the East China Sea: Spatiotemporal variations and environmental implications. J. Geophysical Research: Biogeosciences 121 (11), 2842–2855. doi: 10.1002/2016JG003499
Lin X., Li X., Gao D., Liu M., Cheng L. (2017). Ammonium production and removal in the sediments of shanghai river networks: Spatiotemporal variations, controlling factors, and environmental implications. J. Geophysical Research: Biogeosciences 122 (10), 2461–2478. doi: 10.1002/2017JG003769
Lin G., Lin X. (2022). Bait input altered microbial community structure and increased greenhouse gases production in coastal wetland sediment. Water Res. 218, 118520. doi: 10.1016/j.watres.2022.118520
Li H. M., Tang H. J., Shi X. Y., Zhang C. S., Wang X. L. (2014). Increased nutrient loads from the changjiang (Yangtze) river have led to increased harmful algal blooms. Harmful Algae 39, 92–101. doi: 10.1016/j.hal.2014.07.002
Liu B., Peng S., Liao Y., Long W. (2018). The causes and impacts of water resources crises in the pearl river delta. J. Cleaner Production 177, 413–425. doi: 10.1016/j.jclepro.2017.12.203
Liu Y., Wang C., He N., Wen X., Gao Y., Li S., et al. (2017). A global synthesis of the rate and temperature sensitivity of soil nitrogen mineralization: latitudinal patterns and mechanisms. Global Change Biol. 23 (1), 455–464. doi: 10.1111/gcb.13372
Li X., Wai O. W., Li Y. S., Coles B. J., Ramsey M. H., Thornton I. (2000). Heavy metal distribution in sediment profiles of the pearl river estuary, south China. Appl. Geochemistry 15 (5), 567–581. doi: 10.1016/S0883-2927(99)00072-4
Lovley D. R., Phillips E. J. (1987). Rapid assay for microbially reducible ferric iron in aquatic sediments. Appl. Environ. Microbiol. 53 (7), 1536–1540. doi: 10.1128/aem.53.7.1536-1540.1987
Mao S. H., Zhang H. H., Zhuang G. C., Li X. J., Liu Q., Zhou Z., et al. (2022). Aerobic oxidation of methane significantly reduces global diffusive methane emissions from shallow marine waters. Nat. Commun. 13, 7309. doi: 10.1038/s41467-022-35082-y
Matheson F. E., Nguyen M. L., Cooper A. B., Burt T. P. (2003). Short-term nitrogen transformation rates in riparian wetland soil determined with nitrogen-15. Biol. Fertility Soils 38 (3), 129–136. doi: 10.1007/s00374-003-0640-3
Miller K. S., Geisseler D. (2018). Temperature sensitivity of nitrogen mineralization in agricultural soils. Biol. Fertility Soils 54 (7), 853–860. doi: 10.1007/s00374-018-1309-2
Mishra S., Di H. J., Cameron K. C., Monaghan R., Carran A. (2005). Gross nitrogen mineralization rates in pastural soils and their relationships with organic nitrogen fractions, microbial biomass and protease activity under glasshouse conditions. Biol. Fertility soils 42 (1), 45–53. doi: 10.1007/s00374-005-0863-6
Mooshammer M., Wanek W., Hämmerle I., Fuchslueger L., Hofhansl F., Knoltsch A., et al. (2014). Adjustment of microbial nitrogen use efficiency to carbon: nitrogen imbalances regulates soil nitrogen cycling. Nat. Communication 5, 3694. doi: 10.1038/ncomms4694
Murray R. H., Erler D. V., Eyre B. D. (2015). Nitrous oxide fluxes in estuarine environments: Response to global change. Global Change Biol. 21 (9), 3219–3245. doi: 10.1111/gcb.12923
Park S. J., Seo B. S., Park H. S., Lee B. J., Hur S. W., Nam T. J., et al. (2021). Effect of fishmeal content in the diet on the growth and sexual maturation of olive flounder (Paralichthys olivaceus) at a typical fish farm. Animals 11 (7), 2055. doi: 10.3390/ani11072055
Paul K. I., Polglase P. J., O'connell A. M., Carlyle J. C., Smethurst P. J., Khanna P. K. (2003). Defining the relation between soil water content and net nitrogen mineralization. Eur. J. Soil Sci. 54 (1), 39–48. doi: 10.1046/j.1365-2389.2003.00502.x
Pena M. A., Katsev S., Oguz T., Gilbert D. (2010). Modeling dissolved oxygen dynamics and hypoxia. Biogeosciences 7 (3), 933–957. doi: 10.5194/bg-7-933-2010
Qi X., Liu H., Lin Z., Liu X., Gong H. (2019). Impacts of age and expansion direction of invasive spartina alterniflora on soil organic carbon dynamics in coastal salt marshes along eastern China. Estuaries Coasts 42 (7), 1858–1867. doi: 10.1007/s12237-019-00611-4
Rousk J., Bååth E., Brookes P. C., Lauber C. L., Lozupone C., Caporaso J. G, et al (2010). Soil bacterial and fungal communities across a pH gradient in an arable soil. ISME J. 4 (10), 1340–1351.
Rutigliano F. A., Castaldi S., D’ascoli R., Papa S., Carfora A., Marzaioli R., et al. (2009). Soil activities related to nitrogen cycle under three plant cover types in Mediterranean environment. Appl. Soil Ecol. 43 (1), 40–46. doi: 10.1016/j.apsoil.2009.05.010
Silva R. G., Jorgensen E. E., Holub S. M., Gonsoulin M. E. (2005). Relationships between culturable soil microbial populations and gross nitrogen transformation processes in a clay loam soil across ecosystems. Nutrient Cycling Agroecosystems 71 (3), 259–270. doi: 10.1007/s10705-004-6378-y
Treseder K. K., Schimel J. P., Garcia M. O., Whiteside M. D. (2010). Slow turnover and production of fungal hyphae during a Californian dry season. Soil Bology Biochem. 42 (9), 1657–1660. doi: 10.1016/j.soilbio.2010.06.005
Vance E. D., Brookes P. C., Jenkinson D. S. (1987). An extraction method for measuring soil microbial biomass c. Soil Bology Biochem. 19 (6), 703–707. doi: 10.1016/0038-0717(87)90052-6
Vieira F. C. B., Bayer C., Zanatta J. A., Dieckow J., Mielniczuk J., He Z. L. (2007). Carbon management index based on physical fractionation of soil organic matter in an acrisol under long-term no-till cropping systems. Soil Tillage Res. 96 (1-2), 195–204. doi: 10.1016/j.still.2007.06.007
Wang R. N., Wang M., Huang Q. B., Yi M. M., Li Z. H., Li Q. Y., et al. (2020). Analysis of differences in microbial community structure of eel (Anguilla japonica) pond before and after pond-drying based on high-throughput sequencing. J. Agric. Biotechnol. 28 (7), 1250–1259. doi: 10.3969/j.issn.1674-7968.2020.07.011
Wang S., Zhu G., Peng Y., Jetten M. S., Yin C. (2012). Anammox bacterial abundance, activity, and contribution in riparian sediments of the pearl river estuary. Environ. Sci. Technol. 46 (16), 8834–8842. doi: 10.1021/es3017446
Wu J. P., Hong Y. G., Liu X. H., Hu Y. H. (2021). Variations in nitrogen removalrates and microbial communities over sediment depth in daya bay, China. Environ. pollut. 286, 117267. doi: 10.1016/j.envpol.2021.117267
Wu J. P., Hong Y. G., Wen X. M., Li Y. B., Wang Y., Chang X. Y. (2020). Activity,abundance and community composition of anaerobic ammonia-oxidizing (anammox) bacteria in sediment cores of the pearl river estuary. Estuaries Coasts 43, 73–85. doi: 10.1007/s12237-019-00668-1
Wu H., Peng R., Yang Y., He L., Wang W., Zheng T., et al. (2014). Mariculture pond influence on mangrove areas in south China: Significantly larger nitrogen and phosphorus loadings from sediment wash-out than from tidal water exchange. Aquaculture 426, 204–212. doi: 10.1016/j.aquaculture.2014.02.009
Yang X., Hu C., Wang B., Lin H., Xu Y., Guo H., et al. (2022). Sediment nitrogen mineralization and immobilization affected by non-native Sonneratia apetala plantation in an intertidal wetland of south China. Environ. pollut. 305, 119289. doi: 10.1016/j.envpol.2022.119289
Yang C., Wang S., Jin X., Wu F. (2010). Nitrogen and phosphorus mineralization in sediments of taihu lake after the removal of light fraction organic matter. Environ. Earth Sci. 59 (7), 1437–1446. doi: 10.1007/s12665-009-0130-5
Yuste J. C., Penuelas J., Estiarte M., Garcia-Mas J., Mattana S., Ogaya R., et al. (2011). Drought-resistant fungi control soil organic matter decomposition and its response to temperature. Global Change Biol. 17 (3), 1475–1486. doi: 10.1111/j.1365-2486.2010.02300.x
Zhang W. L., Zeng C. S., Tong C., Zhai S. J., Lin X., Gao D. Z., et al. (2015). Spatial distribution of phosphorus speciation in marsh sediments along a hydrologic gradient in a subtropical estuarine wetland, China. Estuarine, Coastal and Shelf Science 154 (3), 30–38.
Zhou H. H., Du J., Nan Y., Song K. S., Zhao B. Y., Xiang X. Y. (2019). Landscape patterns of coastal wetlands in pearl river delta and their changes for 5 periods since 1980. Wetland Sci. 17, 559–566. doi: 10.13248/j.cnki.wetlandsci.2019.05.009
Zhu T., Meng T., Zhang J., Yin Y., Cai Z., Yang W., et al. (2013). Nitrogen mineralization, immobilization turnover, heterotrophic nitrification, and microbial groups in acid forest soils of subtropical China. Biol. Fertility Soils 49 (3), 323–331. doi: 10.1007/s00374-012-0725-y
Zilio M., Motta S., Tambone F., Scaglia B., Boccasile G., Squartini A., et al. (2020). The distribution of functional n-cycle related genes and ammonia and nitrate nitrogen in soil profiles fertilized with mineral and organic n fertilizer. PloS One 15 (6), e0228364. doi: 10.1371/journal.pone.0228364
Keywords: N mineralization and immobilization, available organic carbon fractions, sediment, reclaimed aquaculture ecosystem, 15N isotope dilution technique
Citation: Lin X, Lin G, Zheng Y, Li W, Guo P, Fan S, Kong T, Tian D, Sun D and Shen Z (2023) Nitrogen mineralization and immobilization in surface sediments of coastal reclaimed aquaculture ecosystems. Front. Mar. Sci. 9:1093279. doi: 10.3389/fmars.2022.1093279
Received: 08 November 2022; Accepted: 12 December 2022;
Published: 09 January 2023.
Edited by:
Liyang Yang, Fuzhou University, ChinaReviewed by:
Xiaofei Li, East China Normal University, ChinaJunhong Bai, Beijing Normal University, China
Copyright © 2023 Lin, Lin, Zheng, Li, Guo, Fan, Kong, Tian, Sun and Shen. This is an open-access article distributed under the terms of the Creative Commons Attribution License (CC BY). The use, distribution or reproduction in other forums is permitted, provided the original author(s) and the copyright owner(s) are credited and that the original publication in this journal is cited, in accordance with accepted academic practice. No use, distribution or reproduction is permitted which does not comply with these terms.
*Correspondence: Zhuo Shen, enNoZW4yMThAMTI2LmNvbQ==
†These authors have contributed equally to this work