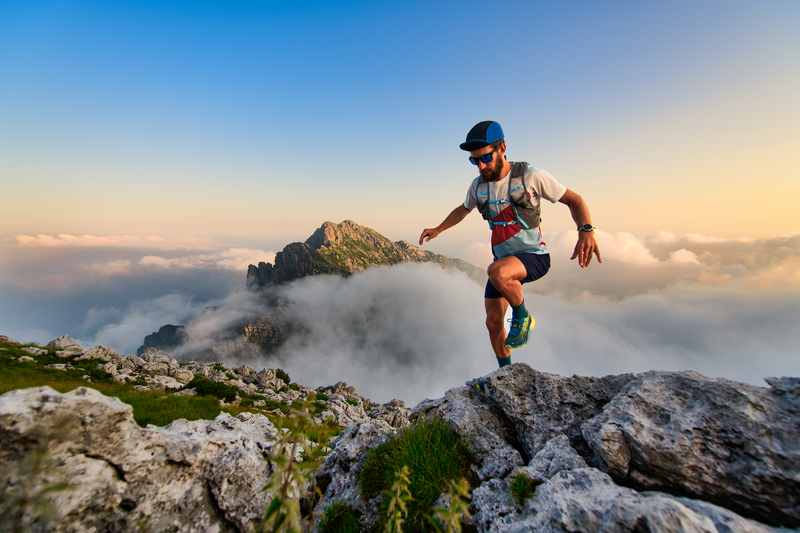
95% of researchers rate our articles as excellent or good
Learn more about the work of our research integrity team to safeguard the quality of each article we publish.
Find out more
REVIEW article
Front. Immunol. , 26 February 2025
Sec. T Cell Biology
Volume 16 - 2025 | https://doi.org/10.3389/fimmu.2025.1550206
This article is part of the Research Topic Formation of Immunological Niches in Tumor Microenvironments: Mechanisms and Therapeutic Potential View all 22 articles
Hypertension, a globally prevalent condition, is closely associated with T cell-mediated inflammatory responses. Studies have shown that T cells, by secreting pro-inflammatory cytokines such as interferon-gamma (IFN-γ), Interleukin-17 (IL-17), and Tumor necrosis factor-alpha (TNF-α), directly lead to vascular dysfunction and elevated blood pressure. The activation of Th1 and Th17 cell subsets, along with the dysfunction of regulatory T cells (Tregs), is a critical mechanism in the onset and progression of hypertension. This review explores the role of T cells in the pathophysiology of hypertension and discusses potential therapeutic strategies targeting T cell regulation, such as immunotherapy and gene-editing technologies. These emerging treatments hold promise for providing personalized therapeutic options for hypertensive patients, reducing inflammatory complications, and improving treatment outcomes.
Hypertension is a globally prevalent cardiovascular disease and one of the leading causes of cardiovascular events and death. As shown in the chart below, the world’s top 25 countries for hypertension mortality in 2021 demonstrate a significant variation in the estimated annual death rates attributed to hypertension (1). This highlights the global burden of the disease and the disparities in hypertension management and control across different regions (Figure 1)
Figure 1. The world’s top 25 countries for hypertension mortality in 2021. Estimated annual death rate attributed to hypertension, also known as high blood pressure, per 100,000 people.
According to the latest data from the World Health Organization (WHO), over 1.1 billion adults worldwide have hypertension, with 78% residing in low- and middle-income countries, contributing to approximately 7.5 million deaths annually, accounting for 12.8% of global mortality. In high-income countries, the control rate of hypertension is 44%, while in low- and middle-income countries, it is only 21% (2, 3).In this context, numerous studies suggest that adopting stricter blood pressure classification standards, such as the new diagnostic threshold of 130/80 mmHg, could increase awareness of early screening and intervention (3). This new standard advances hypertension diagnosis earlier than the previously lenient threshold of 140/90 mmHg, aiming to prevent severe cardiovascular complications later in life through earlier lifestyle modifications and pharmacological interventions. Additionally, hypertension prevalence varies significantly across age, gender, and regions. Among younger adults (20-45 years), men have a higher prevalence of hypertension than women, whereas in the elderly population (65 years and older), the prevalence in women slightly surpasses that in men (4). The higher hypertension control rate in high-income countries contrasts with the lower rate in low- and middle-income countries, reflecting disparities in hypertension management globally (2). Hypertension not only poses a major risk for cardiovascular events but is also closely related to various organ system disorders, including stroke, myocardial infarction, and renal failure (3).
In recent years, growing evidence has highlighted the connection between the immune system and the pathogenesis of hypertension, particularly the crucial role of T cells in hypertension pathophysiology. T cells, traditionally recognized for their role in immune responses, directly participate in hypertension development by influencing vascular endothelial cells and triggering chronic inflammatory responses. Studies indicate that T cells, through the release of multiple cytokines and chemokines, can induce endothelial damage and vascular dysfunction, leading to elevated blood pressure (5–7). RANTES (Regulated on Activation, Normal T Cell Expressed and Secreted), an important chemokine, plays a pivotal role in the inflammatory response and T-cell accumulation associated with hypertension. By promoting T-cell migration to inflammatory sites, RANTES enhances local immune responses and exacerbates vascular dysfunction (8). This discovery provides new possibilities for immune-regulatory targets in hypertension treatment. In summary, modulating T-cell-mediated inflammatory responses and balancing T-cell subtypes could represent potential therapeutic targets for hypertension, promoting the development of personalized and innovative treatment strategies.
Hypertension is a common chronic disease with a complex pathogenesis. Long-term hypertension can lead to severe complications, particularly affecting the cardiovascular, renal, and ocular systems, posing significant threats to patient health and survival. According to WHO statistics, approximately 12.5 million cardiovascular disease-related deaths each year are associated with hypertension, including 3.5 million cases of myocardial infarction and 9.5 million strokes (2). Cardiovascular complications, such as stroke and myocardial infarction, are particularly prominent; about 54% of strokes are associated with hypertension, and myocardial infarction is the leading cause of death in cardiovascular patients, with hypertension increasing its risk by 2-3 times (3). Additionally, the incidence of chronic kidney disease (CKD) is significantly higher in hypertensive patients, with hypertension being the primary cause of 25-30% of end-stage renal disease (ESRD) (9). Hypertension can also cause retinal damage, impacting vision.
Despite the availability of multiple antihypertensive drugs, including ACE inhibitors, beta-blockers, and calcium channel blockers, drug resistance remains a major challenge. Studies indicate that 15-30% of hypertensive patients develop resistance to one or more antihypertensive drugs, leading to suboptimal blood pressure control (10). This resistance may be linked to genetic differences, metabolic abnormalities, and lifestyle factors such as high salt intake and lack of exercise (5). Furthermore, patient compliance is an issue, with about 50% of hypertensive patients failing to consistently adhere to prescribed medications due to side effects, adverse reactions, and insufficient understanding of the disease and treatment. To address this issue, regular blood pressure monitoring, comprehensive treatments combining medication and lifestyle interventions, and research into novel antihypertensive drugs and personalized treatment strategies are crucial. These measures can enhance hypertension management and improve patient quality of life (11).
Hypertension is a chronic condition, and recent research suggests a complex interplay between hypertension and the immune system. Immune cells, which include lymphocytes (such as T cells and B cells), macrophages, and natural killer (NK) cells, are responsible for immune surveillance, defense, and homeostasis, playing key roles in infection defense and tissue repair. During the pathogenesis of hypertension, immune cell activation and functional alterations can trigger vascular inflammation and remodeling.
The interaction between macrophages and T cells is a critical regulatory mechanism. Macrophages contribute significantly to endothelial cell damage and inflammation by secreting pro-inflammatory cytokines like IL-6 and TNF-α, which activate T cells, thereby exacerbating vascular inflammation (12). This interaction depends on the macrophage’s functional phenotype, which ranges from pro-inflammatory M1 to anti-inflammatory M2 (13). This creates a vicious cycle wherein macrophage and T cell activation synergistically enhance inflammation, further damaging the endothelium and worsening hypertension. Activated T cells also release IFN-γ, which amplifies macrophage inflammatory responses, further damaging the endothelium and promoting vascular hardening, ultimately driving hypertension progression (14). Additionally, T cells interact with B cells through CD40 signaling, leading to the production of pro-inflammatory antibodies (especially IgG) by B cells, which enhance macrophage-driven inflammation (15–18). Meanwhile, B cells secrete IL-10 to modulate T cell function and maintain the balance of T cell subsets (19). For instance, B cells help maintain the balance between Th1 and Th17 cells, preventing the differentiation of naïve T cells into these pro-inflammatory subsets, while promoting Treg expansion to suppress pathological Th1/Th17 responses (20, 21). This interplay of immune cells highlights the potential for targeting T cell, B cell, and macrophage functions as novel therapeutic strategies for hypertension. By further understanding these interactions, researchers can explore new therapeutic targets for better managing hypertension and its complications.
T cells, as a subset of lymphocytes, play an essential role in the immune response and have garnered significant attention in the context of hypertension pathophysiology. T-cell-mediated inflammation contributes to the progression of hypertension, with different T cell subsets and their secreted cytokines playing crucial roles in both the onset and progression of the disease.
Th1 cells, for example, release pro-inflammatory cytokines such as IFN-γ, which are implicated in vascular smooth muscle cell (VSMC) proliferation and vascular inflammation. Recent studies have demonstrated that Th1 cells activate the angiotensin II (Ang II) signaling pathway, promoting VSMC proliferation and triggering vascular inflammation, which can elevate blood pressure (14). Th1-mediated inflammation contributes to the hypertensive pathological process through immune responses that promote vascular damage.
Similarly, Th17 cells secrete IL-17, which has been shown to play a significant role in Ang II-induced hypertension. IL-17 exacerbates vascular inflammation, increases vasoconstriction, and promotes endothelial dysfunction, directly driving the pathological processes of hypertension (6). Additionally, RANTES (Regulated upon Activation, Normal T cell Expressed and Secreted), a key chemokine, not only attracts T cells to inflammatory sites but also intensifies vascular inflammation, worsening the progression of hypertension (8).
The imbalance between T-cell subsets is another critical factor contributing to hypertension. A reduction in regulatory T cells (Tregs) weakens their inhibitory effect on pro-inflammatory T cells, such as Th1 and Th17 cells, leading to uncontrolled inflammation that exacerbates hypertension. This imbalance is particularly prominent in vascular stiffening, where a lack of Tregs leads to endothelial dysfunction and inflammatory dysregulation, further aggravating hypertension (22). Moreover, studies suggest that T cells can maintain immune balance in hypertension by regulating Th1 and Th17 cell activity, especially in vascular lesions where the immune balance is disturbed (23).
Recent research has also highlighted the importance of epigenetic modifications in regulating T-cell function, particularly in hypertension. Mechanisms such as DNA methylation and histone modifications alter the activation state of T cells, suggesting that gene regulation may contribute significantly to the role of T cells in hypertension beyond just inflammatory responses (24, 25). This highlights the complex regulatory network that influences T cell activity and its contribution to hypertension pathogenesis.
Pro-inflammatory cytokines, such as TNF-α, IL-6, and MCP-1, also play critical roles in T-cell mediated hypertension (26, 27). TNF-α is linked to chronic inflammation, promoting VSMC proliferation, vascular hardening, and the activation of the NF-κB pathway in T cells, further driving vascular damage (28–30). IL-6 and MCP-1, secreted by T cells, activate inflammatory signaling pathways such as the JAK/STAT pathway, contributing to the chronic inflammation that exacerbates hypertension (27, 31, 32).
Although these preclinical studies highlight the critical role of T cells in hypertension, relevant clinical trials are still ongoing. Further clinical data will help validate these findings and guide clinical treatment strategies.
T cell activation is the cornerstone of immune response, involving the proliferation and differentiation of T cells into effector cells. This process requires two signals: the first is delivered through the T-cell receptor (TCR) and is enhanced by adhesion molecules; the second is a co-stimulatory signal, provided by the interaction between co-stimulatory molecules on antigen-presenting cells (APCs) and receptors on T cells, which amplifies the TCR signal (33, 34). The first signal alone cannot induce a full immune response in T cells. Without the second signal, T cells enter a state of anergy, immune tolerance, or undergo programmed cell death, likely due to limited activation of the major histocompatibility complex (MHC)-peptide-TCR complex and internalization of the TCR-CD3 complex. Co-stimulatory complexes like B7-CD28 can prevent such outcomes (35). The first signal determines the specificity of T cell activation, while the co-stimulatory signal directs the functional outcome (36).
Different T cell subsets and their secreted cytokines regulate inflammation through multiple signaling pathways, which play critical roles in the pathogenesis of hypertension. T cell activation and differentiation not only rely on antigen recognition but also involve intricate signaling cascades, including the TCR, NF-κB, JAK/STAT, mTOR, and Notch pathways.
The TCR signaling pathway is central to T cell activation. Upon TCR binding to its antigen, downstream signals such as NF-κB and MAPK are triggered, promoting T cell proliferation and functional differentiation (33). TCR activation induces specific T cell subsets (e.g., Th1 cells) to secrete large amounts of pro-inflammatory cytokines, including IFN-γ and TNF-α (33, 37). These cytokines exacerbate immune responses in hypertension and, through various signaling pathways, stimulate vascular smooth muscle cell proliferation and vascular remodeling, leading to further elevation in blood pressure.
The NF-κB signaling pathway plays a crucial role in T cell-mediated inflammatory responses. Activated by TCR and cytokine signals, NF-κB regulates the expression of various pro-inflammatory cytokines, such as TNF-α and IL-6 (37). Sustained activation of the NF-κB pathway in chronic inflammation accelerates vascular damage, contributing to endothelial dysfunction and arterial stiffening (38–40). NF-κB signaling pathway, activated via Angiotensin II binding to the Angiotensin II type 1 receptor (AT1R), is involved in pro-inflammatory responses, promoting vascular smooth muscle cell proliferation and vascular remodeling, indirectly leading to elevated blood pressure (40–43). The JAK/STAT signaling pathway is closely related to T cell differentiation, particularly in the differentiation of Th17 cells (44). IL-6 activates the JAK/STAT3 pathway, inducing Th17 differentiation and promoting IL-17 secretion (31, 45–47). IL-17 amplifies vascular inflammation and endothelial dysfunction, exacerbating hypertension. Inhibiting the IL-6/JAK/STAT3 pathway reduces Th17 activity, alleviating vascular inflammation and damage in hypertension (6, 31, 48, 49). The mTOR pathway, which regulates T cell metabolism, influences T cell differentiation as well. In hypertension, excessive mTOR activation enhances the proliferation of pro-inflammatory T cell subsets, such as Th1 and Th17, aggravating inflammatory responses. Studies in animal models have shown that mTOR inhibitors can reduce inflammatory T cell numbers and lower blood pressure (50–52). The Notch signaling pathway also plays a pivotal role in T cell differentiation, particularly in regulating Th1 and Th17 differentiation (53). While Notch signaling is linked to pro-inflammatory T cell activation and its role in vascular inflammation, whether direct inhibition of Notch can significantly lower blood pressure in hypertensive patients remains insufficiently supported. Animal studies suggest that inhibiting Notch signaling can reduce inflammation, but its effect on lowering blood pressure alone has not been conclusively demonstrated.
In summary, T cell activation and differentiation signaling pathways regulate the expression of pro-inflammatory and anti-inflammatory cytokines, playing an important role in the pathophysiology of hypertension. Interventions targeting these signaling pathways offer new avenues for future immunotherapy in hypertension, particularly in reducing inflammation and controlling blood pressure (Figure 2)
Figure 2. Signaling pathways for T cell activation and differentiation. T cell subsets and their secreted cytokines regulate inflammation through signaling pathways such as TCR, NF-kB, JAK/STAT, mTOR, and Notch, playing a role in the pathogenesis of hypertension.
Recent studies have increasingly focused on the role of epigenetic and post-transcriptional modifications in regulating T cell function. These regulatory mechanisms are crucial not only for T cell activation, proliferation, differentiation, and maintenance of function but are also closely linked to the development and progression of inflammatory diseases like hypertension.
DNA methylation is a classic form of epigenetic regulation, catalyzed by DNA methyltransferases (DNMTs), which add methyl groups to CpG islands within genes, thereby modulating gene expression. In T cells, DNA methylation plays a key role in determining their differentiation and functional status (24). For instance, the Foxp3 gene, which is essential for the differentiation of regulatory T cells (Tregs), is influenced by its DNA methylation status (54). Elevated methylation of the Foxp3 gene in hypertensive patients weakens Treg function, exacerbating immune inflammation and vascular damage. Research has shown that reducing the methylation of Foxp3 can restore Treg function, potentially alleviating hypertension-associated immune inflammation (55–57).
Not only Tregs, but pro-inflammatory T cell subsets like Th1 and Th17 also rely on DNA methylation for their differentiation. DNMTs suppress excessive activation of these cells by methylating key genes (58–61). Studies have shown that inhibiting DNMT activity can significantly reduce the proliferation of Th1 and Th17 cells and suppress the secretion of pro-inflammatory cytokines, such as IFN-γ and IL-17, thereby alleviating chronic inflammation in hypertension (61). This mechanism presents new possibilities for controlling pro-inflammatory immune responses through epigenetic regulation.
MicroRNAs (miRNAs), as key post-transcriptional gene regulators, modulate T cell function by binding to target mRNAs, inhibiting their translation or inducing their degradation (62). miRNAs finely regulate T cell activation, differentiation, and cytokine secretion. For instance, miR-21 is upregulated in hypertensive patients and regulates Treg function, mitigating inflammation and improving vascular function (63–65). In contrast, miR-155 is highly expressed in pro-inflammatory T cells (e.g., Th1 and Th17 cells), enhancing their inflammatory activity and promoting the secretion of IFN-γ and IL-17, thereby aggravating vascular inflammation (66, 67). Targeting miR-155 may reduce the secretion of pro-inflammatory cytokines, alleviating hypertension-related vascular dysfunction (67, 68).
Notably, epigenetic and post-transcriptional modifications are not isolated processes; they interact to co-regulate T cell fate and function. For example, miRNAs can influence DNA methylation by regulating DNMT expression, while also modulating other epigenetic factors, thus extending the regulatory network of T cell functions (69). This interaction increases the complexity of T cell regulation and plays a key role in inflammatory diseases like hypertension.
In conclusion, epigenetic modifications (such as DNA methylation) and post-transcriptional modifications (such as miRNAs) regulate T cell differentiation and function through multiple layers and pathways, driving the immune pathology of hypertension. Future research can further explore these regulatory mechanisms as potential therapeutic targets in hypertension, with broad clinical applications in reducing vascular inflammation and improving vascular function.
Recent research has revealed that T cells and their secreted pro-inflammatory cytokines play a central role in the pathogenesis of hypertension. Th1 and Th17 cells, along with their products, such as IFN-γ, TNF-α, and IL-17, significantly contribute to vascular inflammation and dysfunction (70). For example, IL-17 induces local inflammatory responses by activating endothelial cells, eventually leading to vascular remodeling and hypertension. Clinical data have shown that IL-17 expression in hypertensive patients correlates positively with blood pressure levels and cardiovascular risk (6, 71). IFN-γ, by activating the Angiotensin II signaling pathway, promotes vascular smooth muscle cell proliferation and exacerbates inflammation, worsening the condition (72). TNF-α is closely linked to chronic inflammation and vascular stiffening, activating the NF-kB signaling pathway, which enhances T cell activation and the release of pro-inflammatory cytokines, accelerating vascular damage and remodeling associated with hypertension (73–75).
Targeted interventions against these molecular markers have become a focus in immunotherapy research for hypertension. For instance, IL-17 inhibitors have demonstrated significant therapeutic effects in animal models, not only reducing blood pressure but also mitigating vascular inflammatory damage (76, 77). Similarly, blocking IFN-γ and TNF-α signaling pathways can reduce vascular inflammation and remodeling, thereby lowering cardiovascular risk.
Furthermore, epigenetic regulation, such as the methylation level of the Foxp3 gene, directly affects the anti-inflammatory function of regulatory T cells (Tregs). Studies have shown that modulating the methylation state of the Foxp3 gene can enhance Tregs’ anti-inflammatory activity, potentially alleviating hypertension-related immune inflammation (78). These findings underscore the critical role of T cells in hypertension and provide promising directions for immunotherapy targeting T cell-related molecular markers, advancing personalized treatment strategies.
Current hypertension medications influence T cell function through various mechanisms, thus modulating immune responses related to inflammation. Common antihypertensive drugs (such as ACE inhibitors [ACEI] and angiotensin receptor blockers [ARBs]) inhibit Th1 and Th17 cell activity, reducing the secretion of pro-inflammatory cytokines like IFN-γ and IL-17, which significantly lowers vascular inflammation and improves blood pressure control (79–82). Calcium channel blockers (CCBs) and diuretics reduce Angiotensin II levels, indirectly inhibiting T cell activation and pro-inflammatory cytokine release, thereby preserving vascular function (83–85). Beta-blockers not only modulate regulatory T cell (Treg) function through metabolic and epigenetic pathways but also promote Treg activation, modulating immune responses and alleviating chronic inflammation in hypertension (86–88). Additionally, Tocilizumab, an anti-inflammatory drug targeting IL-6, is primarily used to treat immune-mediated inflammatory diseases like rheumatoid arthritis. While its mechanism of inhibiting Th17 cell activation and reducing inflammatory cytokines through the IL-6/JAK/STAT pathway has been validated in other diseases, its application in hypertension remains in the exploratory phase. Current research has yet to establish the widespread clinical use of Tocilizumab in managing immune inflammation in hypertension (89–92). Therefore, Tocilizumab’s potential role in hypertension warrants further investigation, though its immunomodulatory mechanism offers new possibilities for personalized hypertension treatment.
These studies highlight the potential of improving hypertension treatment outcomes by regulating T cell function, suggesting that immune modulation not only effectively controls blood pressure but also reduces hypertension-related inflammation and vascular damage.
Lifestyle changes also play a significant role in regulating T cell-mediated inflammatory responses in non-pharmacological treatments. Research has shown that the Mediterranean diet, rich in polyunsaturated fatty acids and antioxidants, effectively inhibits pro-inflammatory T cell subsets (e.g., Th1, Th17), while increasing the proportion of regulatory T cells (Tregs), potentially reducing immune inflammation in hypertensive patients (93, 94). A clinical study demonstrated that the Mediterranean diet significantly lowered IL-6 and TNF-α levels in the blood, reducing the risk of developing hypertension (94–101). Additionally, low-sodium diets and regular aerobic exercise reduce Th17 cell activation and increase Treg proportions, significantly lowering blood pressure and reducing vascular inflammation (94, 102). Exercise interventions also have important benefits for improving T cell function. Regular aerobic exercise not only inhibits pro-inflammatory T cell activation and reduces IL-17 secretion but also promotes the recovery of Treg function, alleviating vascular inflammation and immune imbalance (103–108). Exercise also improves obesity-related metabolic disorders, indirectly modulating T cell activity, further enhancing vascular function and lowering blood pressure (109, 110).
Thus, lifestyle interventions provide an effective non-pharmacological treatment strategy for correcting T cell dysfunction, offering long-term vascular protection for hypertensive patients, and providing essential evidence for the development of personalized management plans.
Recent advancements in gene therapy technology have made significant progress in research on immune regulation related to hypertension. T cells, particularly regulatory T cells (Tregs), play a crucial role in the chronic inflammation associated with hypertension. Thus, restoring and enhancing Treg function has emerged as a promising new strategy in hypertension treatment. CRISPR/Cas9 gene-editing technology has shown great potential for modulating Treg function, particularly by targeting the expression of the Foxp3 gene to restore its immunosuppressive function (111, 112). Foxp3 acts as the central regulator of Treg function, and hypermethylation of Foxp3 is closely associated with Treg dysfunction (113, 114). By precisely regulating Foxp3 expression using CRISPR/Cas9 technology, it is possible to restore the anti-inflammatory properties of Tregs while also reducing the excessive activation of pro-inflammatory T cells, such as Th1 and Th17 cells, thus mitigating endothelial damage (111, 115, 116). Recent studies have further demonstrated that modulating the methylation status of the Foxp3 gene can enhance Treg-mediated immunoregulation, which in turn helps alleviate chronic inflammation in hypertension (114, 117–121).
Despite these promising findings in animal models, the clinical application of gene therapy for hypertension in humans still faces significant challenges. These include concerns over the long-term safety, specificity, and potential off-target effects of gene editing. Therefore, future research must focus on optimizing the precision of gene-editing tools like CRISPR/Cas9 and exploring their individualized application in hypertensive patients to ensure efficacy and safety under specific pathological conditions.
Cell therapy, particularly Treg (regulatory T cell)-based therapy, has shown great potential in the treatment of hypertension. Studies have demonstrated that the number and function of Tregs are significantly reduced in hypertensive patients, while the proportion of pro-inflammatory T cells (e.g., Th17 cells) is elevated, exacerbating inflammatory responses and subsequently increasing blood pressure (22, 122–124). Tregs play a key role in immune regulation by suppressing the release of pro-inflammatory cytokines such as IL-17 and IFN-γ, effectively alleviating hypertension-related chronic inflammation (125, 126). Therefore, expanding and reinfusing autologous Tregs to restore their immunosuppressive abilities has emerged as a promising strategy for improving hypertension treatment (122, 127).
Moreover, researchers are exploring the genetic modification of Tregs to further enhance their immunosuppressive function. For example, using CRISPR/Cas9 technology to modify key regulatory genes in Tregs, such as Foxp3, can significantly improve Treg function and stability. Studies have found that genetically modified Tregs can maintain a longer-lasting anti-inflammatory effect in vivo, enhancing their ability to control chronic inflammation caused by hypertension (113, 124).
As technology advances, the application of Treg cell therapy in the immunoregulatory treatment of hypertension holds even greater promise. Future research will focus on improving the stability and safety of Treg cell therapies, particularly in terms of clinical operability. With further basic research and clinical trials, Treg cell therapy could become an important therapeutic approach, offering personalized and effective treatment options for hypertensive patients.
Research on T cell regulation in hypertension faces several significant limitations. A major issue is the small sample sizes in many studies, which reduce the statistical power of the results and hinder a comprehensive understanding of hypertension and its immune mechanisms. Additionally, existing studies tend to focus on isolated T cell subsets or specific pro-inflammatory factors, often overlooking the broader, systemic mechanisms that contribute to the pathogenesis of hypertension. This narrow focus impedes a holistic understanding of how T cells influence hypertension at a molecular and physiological level.
Recent advancements in genetics and gene editing technologies, particularly CRISPR/Cas9, present promising opportunities to address these challenges (128). One notable development is the identification of phosphodiesterase 3A (PDE3A) gene mutations that enhance enzyme activity and are associated with hypertension with brachydactyly (HTNB) (129, 130). This discovery opens new avenues for targeted therapeutic interventions, emphasizing the critical role of genetics in understanding hypertension. However, it also highlights several hurdles. A primary challenge is the need for more in vivo models to confirm the involvement of mutated PDE3A in hypertension development. Existing animal models often fail to replicate the immune responses observed in humans, which limits their utility in fully understanding the genetic-immune interactions that underlie hypertension (128, 130).
A significant barrier in hypertension research lies in the inadequacy of current animal models to faithfully simulate human hypertension, particularly in terms of immune system involvement. While advanced technologies like CRISPR/Cas9 have been used to generate PDE3A-mutant animal models, these models do not yet fully capture the immune dynamics of human hypertension (129, 130). For example, while overexpression of PDE3A in smooth muscle cells leads to increased vascular resistance and hypertension, the interaction between these genetic alterations and immune cells—especially T cell subsets—remains poorly understood. Further investigation into this aspect is needed to explore how these mutations impact immune cell function and contribute to hypertension pathogenesis.
To address these challenges, future research should focus on developing more refined animal models that better simulate human immune dynamics, with particular emphasis on T cell regulation. The interaction between PDE3A mutations and immune cells such as T cells and macrophages is a critical area of research. Understanding how PDE3A mutations influence T cell activation and proliferation could reveal novel gene-targeted therapies for hypertension. These insights may also help identify new pathways for modulating immune responses to reduce vascular damage and improve blood pressure regulation (131).
While these findings provide a solid foundation for future therapeutic strategies, it is important to explore how T cell-related discoveries can be translated into clinical applications. For instance, identifying specific T cell subtypes and their functional alterations in hypertensive patients may guide patient stratification, risk prediction, and individualized treatment strategies, such as the use of immunomodulatory agents. Furthermore, integrating immune and genomic data may lead to more precise treatments, improving patient outcomes and reducing complications. By bridging basic research to clinical practice, we anticipate that personalized treatment strategies will become more precise and tailored, offering new insights into individualized therapy.
Collaboration between genetics, immunology, and cardiology is essential for advancing this field. By integrating gene editing technologies with immune modulation strategies, researchers can create more accurate models of human hypertension, which will provide a clearer picture of the disease’s underlying mechanisms. Such integrated research approaches could uncover novel signaling pathways and mechanisms through which PDE3A mutations influence vascular changes and contribute to hypertension (131).
In the long term, gene therapy and immunotherapy could provide exciting new treatment options for hypertension. Future research should aim to translate findings from animal models into clinical applications. This includes using gene editing technologies to precisely target PDE3A mutations or modulate immune responses to alleviate hypertension (129–131). Overcoming challenges related to the safety, efficiency, and long-term effects of gene therapy will be key to bringing these novel approaches into clinical practice.
In conclusion, while PDE3A mutations represent a promising therapeutic target for hypertension, much work remains to be done. The integration of genetic research and immune regulation, coupled with the development of more precise animal models, will be crucial for advancing our understanding of hypertension and improving treatment strategies.
In the future, precision medicine and personalized treatment will become increasingly important in the management of hypertension. With the rapid development of genomics and immunology, scientists are expected to gain deeper insights into the role of T cells in hypertension, enabling tailored treatment plans for individual patients. Specifically, genomics research can identify genetic variations related to hypertension, particularly those affecting T cell function. This will provide a crucial basis for developing more precise treatment strategies. Moreover, immune phenotyping can reveal changes in T cell subsets within patients, helping clinicians select appropriate immunomodulatory drugs and adjust dosages to optimize therapeutic outcomes.
In this context, interdisciplinary collaboration and technological innovation will be key drivers of progress. By integrating knowledge from fields such as cardiology, immunology, and genomics, researchers can develop novel immunomodulatory drugs and gene therapies aimed at improving the prognosis of hypertensive patients. Overall, future research will continue to explore the role of T cells in hypertension, promote the development of new immunotherapies, and strive for significant progress in improving patients’ quality of life.
In conclusion, hypertension remains a global health crisis with significant mortality rates (102, 132, 133).Recent studies highlight the critical role of T cells in hypertension pathogenesis, particularly in immune regulation and inflammatory responses. Dysregulation of Tregs and the imbalance with pro-inflammatory T cells are central to hypertension-induced inflammation (134). Targeted T cell regulation through immunomodulatory drugs, gene therapy, and cell therapy offers new therapeutic possibilities.
Future research should focus on the interactions between T cells and other immune cells, exploring new regulatory molecules and refining animal models. Integrating immune and genomic data could lead to more precise, personalized treatments, improving patient outcomes and reducing complications. T cell regulation holds great potential for advancing hypertension therapy, offering new insights into individualized treatment strategies.
MF: Writing – original draft. ML: Writing – original draft. JG: Writing – original draft. AM: Writing – review & editing. HQ: Writing – review & editing. HY: Writing – review & editing. WW: Writing – review & editing. ZL: Writing – review & editing. JZ: Writing – review & editing. YW: Writing – review & editing. XM: Writing – review & editing. HW: Writing – review & editing. JC: Writing – original draft, Writing – review & editing.
The author(s) declare that financial support was received for the research, authorship, and/or publication of this article. This work was supported by Hubei Provincial Natural Science Foundation (2022CFB453), Foundation of Health Commission of Hubei (WJ2021M061), Natural Science Foundation of the Bureau of Science and Technology of Shiyan City (grant no. 21Y71), Faculty Development Grants from Hubei University of Medicine (2018QDJZR04), Hubei Key Laboratory of Wudang Local Chinese Medicine Research (Hubei University of Medicine) (Grant No. WDCM2024020, WDCM2024023), and Advantages Discipline Group (Medicine) Project in Higher Education of Hubei Province (2021-2025) (Grant No. 2024XKQT41).
The authors declare that the research was conducted in the absence of any commercial or financial relationships that could be construed as a potential conflict of interest.
The author(s) declare that no Generative AI was used in the creation of this manuscript.
All claims expressed in this article are solely those of the authors and do not necessarily represent those of their affiliated organizations, or those of the publisher, the editors and the reviewers. Any product that may be evaluated in this article, or claim that may be made by its manufacturer, is not guaranteed or endorsed by the publisher.
1. IHME. Global Burden of Disease (2024) – with minor processing by Our World in Data. “Child deaths linked to child and maternal malnutrition” [dataset]. IHME, Global Burden of Disease, “Global Burden of Disease - Risk Factors” [original data]. (2024). Retrieved February 19, 2025 from https://ourworldindata.org/grapher/number-child-deaths-malnutrition.
2. Rukunuzzaman M, Khatun N, Nessa A, Wahed F, Sharmin A, Meherubin I, et al. Study on body mass index, serum total cholesterol and serum triglycerides in adult male hypertensive patients. Mymensingh Med Journal: Mmj. (2023) 32(1):44–8.
3. Whelton PK, Carey RM, Aronow WS, Casey DE Jr, Collins KJ, Dennison Himmelfarb C, et al. 2017 ACC/AHA/AAPA/ABC/ACPM/AGS/APHA/ASH/ASPC/NMA/PCNA guideline for the prevention, detection, evaluation, and management of high blood pressure in adults: A report of the american college of cardiology/american heart association task force on clinical practice guidelines. Circulation. (2018) 138(17):e484–e594. doi: 10.1016/j.jacc.2017.11.006
4. Amini M, Moradinazar M, Rajati F, Soofi M, Sepanlou SG, Poustchi H, et al. Socioeconomic inequalities in prevalence, awareness, treatment and control of hypertension: evidence from the persian cohort study. BMC Public Health. (2022) 22(1):1401. doi: 10.1186/s12889-022-13444-x
5. Harrison DG, Guzik TJ, Lob HE, Madhur MS, Marvar PJ, Thabet SR, et al. Inflammation, immunity, and hypertension. Hypertension (Dallas Tex: 1979). (2011) 57(2):132–40. doi: 10.1161/HYPERTENSIONAHA.110.163576
6. Madhur MS, Lob HE, Mccann LA, Iwakura Y, Blinder Y, Guzik TJ, et al. Interleukin 17 promotes angiotensin ii-induced hypertension and vascular dysfunction. Hypertension (Dallas Tex: 1979). (2010) 55(2):500–7. doi: 10.1161/HYPERTENSIONAHA.109.145094
7. Wu J, Thabet SR, Kirabo A, Trott DW, Saleh MA, Xiao L, et al. Inflammation and mechanical stretch promote aortic stiffening in hypertension through activation of P38 mitogen-activated protein kinase. Circ Res. (2014) 114(4):616–25. doi: 10.1161/CIRCRESAHA.114.302157
8. Marino AP, Da Silva A, Dos Santos P, Pinto LM, Gazzinelli RT, Teixeira MM, et al. Regulated on activation, normal T cell expressed and secreted (Rantes) antagonist (Met-rantes) controls the early phase of trypanosoma cruzi-elicited myocarditis. Circulation. (2004) 110(11):1443–9. doi: 10.1161/01.CIR.0000141561.15939.EC
9. Johansen KL, Chertow GM, Gilbertson DT, Ishani A, Israni A, Ku E, et al. Us renal data system 2022 annual data report: epidemiology of kidney disease in the United States. Am J Of Kidney Diseases: Off J Of Natl Kidney Foundation. (2023) 81(3 Suppl1):A8–A11. doi: 10.1053/j.ajkd.2022.12.001
10. Burnier M, Egan BM. Adherence in hypertension. Circ Res. (2019) 124:1124–40. doi: 10.1161/CIRCRESAHA.118.313220
11. Vrijens B, Vincze G, Kristanto P, Urquhart J, Burnier M. Adherence to prescribed antihypertensive drug treatments: longitudinal study of electronically compiled dosing histories. BMJ (Clinical Res Ed). (2008) 336(7653):1114–7. doi: 10.1136/bmj.39553.670231.25
12. Chen S, Saeed A, Liu Q, Jiang Q, Xu H, Xiao GG, et al. Macrophages in immunoregulation and therapeutics. Signal Transduction And Targeted Ther. (2023) 8(1):207. doi: 10.1038/s41392-023-01452-1
13. Jackaman C, Tomay F, Duong L, Abdol Razak NB, Pixley FJ, Metharom P, et al. Aging and cancer: the role of macrophages and neutrophils. Ageing Res Rev. (2017) 36:105–16. doi: 10.1016/j.arr.2017.03.008
14. Guzik TJ, Hoch NE, Brown KA, McCann LA, Rahman A, Dikalov S, et al. Role of the T cell in the genesis of angiotensin ii induced hypertension and vascular dysfunction. J Of Exp Med. (2007) 204(10):2449–60. doi: 10.1084/jem.20070657
15. Owens T. T-cell costimulation: T cells themselves call the shots. Curr Biol. (1996) 6:32–5. doi: 10.1016/S0960-9822(02)00415-3
16. Yamano T, Steinert M, Klein L. Thymic B cells and central T cell tolerance. Front In Immunol. (2015) 6:376. doi: 10.3389/fimmu.2015.00376
17. Dustin ML. Help to go: T cells transfer cd40l to antigen-presenting B cells. Eur J Of Immunol. (2017) 47:31–4. doi: 10.1002/eji.201646786
18. Elgueta R, Benson MJ, De Vries VC, Wasiuk A, Guo Y, Noelle RJ. Molecular mechanism and function of cd40/cd40l engagement in the immune system. Immunol Rev. (2009) 229(1):152–72. doi: 10.1111/j.1600-065X.2009.00782.x
19. Radomir L, Kramer MP, Perpinial M, Schottlender N, Rabani S, David K, et al. The survival and function of IL-10-producing regulatory B cells are negatively controlled by slamf5. Nat Commun. (2021) 12(1):1893. doi: 10.1038/s41467-021-22230-z
20. Ray A, Basu S, Williams CB, Salzman NH, Dittel BN. A novel IL-10-independent regulatory role for B cells in suppressing autoimmunity by maintenance of regulatory T cells via gitr ligand. J Of Immunol (Baltimore Md: 1950). (2012) 188(7):3188–98. doi: 10.4049/jimmunol.1103354
21. Flores-Borja F, Bosma A, Ng D, Reddy V, Ehrenstein MR, Isenberg DA, et al. CD19+CD24HICD38HI B cells maintain regulatory T cells while limiting th1 and th17 differentiation. Sci Trans Med. (2013) 5(173):173ra23. doi: 10.1126/scitranslmed.3005407
22. Barhoumi T, Kasal DA, Li MW, Shbat L, Laurant P, Neves MF, et al. T regulatory lymphocytes prevent angiotensin II-induced hypertension and vascular injury. Hypertension (Dallas Tex: 1979). (2011) 57(3):469–76. doi: 10.1161/HYPERTENSIONAHA.110.162941
23. Matrougui K, Abd Elmageed Z, Kassan M, Choi S, Nair D, Gonzalez-Villalobos RA, et al. Natural regulatory T cells control coronary arteriolar endothelial dysfunction in hypertensive mice. Am J Of Pathol. (2011) 178(9):434–41. doi: 10.1016/j.ajpath.2010.11.034
24. Bai L, Hao X, Keith J, Feng Y. Dna methylation in regulatory T cell differentiation and function: challenges and opportunities. Biomolecules. (2022) 12(9):1282. doi: 10.3390/biom12091282
25. Bellanti JA, Li D. Treg cells and epigenetic regulation. Adv In Exp Med And Biol. (2021) 1278:95–114. doi: 10.1007/978-981-15-6407-9_6
26. Ridker PM, Rane M. Interleukin-6 signaling and anti-interleukin-6 therapeutics in cardiovascular disease. Circ Res. (2021) 128:1728–46. doi: 10.1161/CIRCRESAHA.121.319077
27. Tanaka T, Narazaki M, Kishimoto T. IL-6 in inflammation, immunity, and disease. Cold Spring Harbor Perspect In Biol. (2014) 6:A016295. doi: 10.1101/cshperspect.a016295
28. Selzman CH, Shames BD, Reznikov LL, Miller SA, Meng X, Barton HA, et al. Liposomal delivery of purified inhibitory-kappabalpha inhibits tumor necrosis factor-alpha-induced human vascular smooth muscle proliferation. Circ Res. (1999) 84(8):867–75. doi: 10.1161/01.RES.84.8.867
29. Rastogi S, Rizwani W, Joshi B, Kunigal S, Chellappan SP. TNF-α Response of vascular endothelial and vascular smooth muscle cells involve differential utilization of ask1 kinase and P73. Cell Death And Differentiation. (2012) 19:274–83. doi: 10.1038/cdd.2011.93
30. Jang DI, Lee AH, Shin HY, Song HR, Park JH, Kang TB, et al. The role of tumor necrosis factor alpha (TNF-α) in autoimmune disease and current TNF-α Inhibitors in therapeutics. Int J Mol Sci. (2021) 22(5):2719. doi: 10.3390/ijms22052719
31. Yao X, Huang J, Zhong H, Shen N, Faggioni R, Fung M, et al. Targeting interleukin-6 in inflammatory autoimmune diseases and cancers. Pharmacol Ther. (2014) 141(2):125–39. doi: 10.1016/j.pharmthera.2013.09.004
32. Singh S, Anshita D, Ravichandiran V. Mcp-1: function, regulation, and involvement in disease. Int Immunopharmacol. (2021) 101:107598. doi: 10.1016/j.intimp.2021.107598
33. Shah K, Al-Haidari A, Sun J, Kazi JU. T cell receptor (TCR) signaling in health and disease. Signal Transduction And Targeted Ther. (2021) 6(1):412. doi: 10.1038/s41392-021-00823-w
34. Wilfahrt D, Delgoffe GM. Metabolic waypoints during T cell differentiation. Nat Immunol. (2024) 25:206–17. doi: 10.1038/s41590-023-01733-5
35. Szeto C, Zareie P, Wirasinha RC, Zhang JB, Nguyen AT, Riboldi-Tunnicliffe A, et al. Covalent tcr-peptide-mhc interactions induce T cell activation and redirect T cell fate in the thymus. Nat Commun. (2022) 13(1):4951. doi: 10.1038/s41467-022-32692-4
36. Hwang JR, Byeon Y, Kim D, Park SG. Recent insights of T cell receptor-mediated signaling pathways for T cell activation and development. Exp Mol Med. (2020) 52:750–61. doi: 10.1038/s12276-020-0435-8
37. Castro-Sanchez P, Teagle AR, Prade S, Zamoyska R. Modulation of tcr signaling by tyrosine phosphatases: from autoimmunity to immunotherapy. Front In Cell And Dev Biol. (2020) 8:608747. doi: 10.3389/fcell.2020.608747
38. Taniguchi K, Karin M. NF-κB, inflammation, immunity and cancer: coming of age. Nat Rev Immunol. (2018) 18:309–24. doi: 10.1038/nri.2017.142
39. Yu H, Lin L, Zhang Z, Zhang H, Hu H. Targeting NF-κB pathway for the therapy of diseases: mechanism and clinical study. Signal Transduction And Targeted Ther. (2020) 5(1):209. doi: 10.1038/s41392-020-00312-6
40. Lawrence T. The nuclear factor nf-kappab pathway in inflammation. Cold Spring Harbor Perspect In Biol. (2009) 1:A001651. doi: 10.1007/978-981-15-6407-9_6
41. Barnabei L, Laplantine E, Mbongo W, Rieux-Laucat F, Weil R. NF-κB: at the borders of autoimmunity and inflammation. Front In Immunol. (2021) 12:716469. doi: 10.3389/fimmu.2021.716469
42. Wolf G, Wenzel U, Burns KD, Harris RC, Stahl RA, Thaiss F. Angiotensin ii activates nuclear transcription factor-kappab through at1 and at2 receptors. Kidney Int. (2002) 61(6):1986–95. doi: 10.1046/j.1523-1755.2002.00365.x
43. Esteban V, Ruperez M, Sánchez-López E, Rodríguez-Vita J, Lorenzo O, Demaegdt H, et al. Angiotensin iv activates the nuclear transcription factor-kappab and related proinflammatory genes in vascular smooth muscle cells. Circ Res. (2005) 96(9):965–73. doi: 10.1161/01.RES.0000166326.91395.74
44. Fortelny N, Farlik M, Fife V, Gorki AD, Lassnig C, Maurer B, et al. Jak-stat signaling maintains homeostasis in T cells and macrophages. Nat Immunol. (2024) 25(5):847–59. doi: 10.1038/s41590-024-01804-1
45. Fujimoto M, Serada S, Mihara M, Uchiyama Y, Yoshida H, Koike N, et al. Interleukin-6 blockade suppresses autoimmune arthritis in mice by the inhibition of inflammatory th17 responses. Arthritis And Rheumatism. (2008) 58(12):3710–9. doi: 10.1002/art.v58:12
46. Yu H, Lee H, Herrmann A, Buettner R, Jove R. Revisiting stat3 signalling in cancer: new and unexpected biological functions. Nat Rev Cancer. (2014) 14(11):736–46. doi: 10.1038/nrc3818
47. Wang SW, Sun YM. The IL-6/jak/stat3 pathway: potential therapeutic strategies in treating colorectal cancer (Review). Int J Of Oncol. (2014) 44:1032–40. doi: 10.3892/ijo.2014.2259
48. Gabay C. Interleukin-6 and chronic inflammation. Arthritis Res Ther. (2006) 8 Suppl 2:S3. doi: 10.1186/ar1917
49. Johnson DE, O'keefe RA, Grandis JR. Targeting the IL-6/jak/stat3 signalling axis in cancer. Nat Rev Clin Oncol. (2018) 15:234–48. doi: 10.1038/nrclinonc.2018.8
50. Huang H, Long L, Zhou P, Chapman NM, Chi H. mTOR signaling at the crossroads of environmental signals and T-cell fate decisions. Immunol Rev. (2020) 295(1):15–38. doi: 10.1111/imr.v295.1
51. Zeng H, Chi H. Mtor signaling in the differentiation and function of regulatory and effector T cells. Curr Opin In Immunol. (2017) 46:103–11. doi: 10.1016/j.coi.2017.04.005
52. Zeng H, Yang K, Cloer C, Neale G, Vogel P, Chi H. mTORC1 couples immune signals and metabolic programming to establish T(Reg)-cell function. Nature. (2013) 499(7459):485–90. doi: 10.1038/nature12297
53. Amsen D, Helbig C, Backer RA. Notch in T cell differentiation: all things considered. Trends In Immunol. (2015) 36(10):802–14. doi: 10.1016/j.it.2015.10.007
54. Huehn J, Polansky JK, Hamann A. Epigenetic control of FOXP3 expression: the key to A stable regulatory T-cell lineage? Nat Rev Immunol. (2009) 9:83–9. doi: 10.1038/nri2474
55. Sakaguchi S, Wing K, Onishi Y, Prieto-Martin P, Yamaguchi T. Regulatory T cells: how do they suppress immune responses? Int Immunol. (2009) 21:1105–11. doi: 10.1093/intimm/dxp095
56. Sumida TS, Cheru NT, Hafler DA. The regulation and differentiation of regulatory T cells and their dysfunction in autoimmune diseases. Nat Rev Immunol. (2024) 24:503–17. doi: 10.1038/s41577-024-00994-x
57. Floess S, Freyer J, Siewert C, Baron U, Olek S, Polansky J, et al. Epigenetic control of the FOXP3 locus in regulatory T cells. PloS Biol. (2007) 5(2):E38. doi: 10.1371/journal.pbio.0050038
58. Xia Y, Yang J, Wang G, Li C, Li Q. Age-related changes in dna methylation associated with shifting th1/th2 balance. Inflammation. (2016) 39(6):1892–903. doi: 10.1007/s10753-016-0425-0
59. Brand S, Kesper DA, Teich R, Kilic-Niebergall E, Pinkenburg O, Bothur E, et al. Dna methylation of th1/th2 cytokine genes affects sensitization and progress of experimental asthma. J Of Allergy And Clin Immunol. (2012) 129(6):1602–10.E6. doi: 10.1016/j.jaci.2011.12.963
60. Guan H, Nagarkatti PS, Nagarkatti M. Cd44 reciprocally regulates the differentiation of encephalitogenic th1/th17 and th2/regulatory T cells through epigenetic modulation involving dna methylation of cytokine gene promoters, thereby controlling the development of experimental autoimmune encephalomyelitis. J Of Immunol (Baltimore Md: 1950). (2011) 186:6955–64. doi: 10.4049/jimmunol.1004043
61. Qiu Y, Zhu Y, Yu H, Zhou C, Kijlstra A, Yang P. Dynamic dna methylation changes of tbx21 and rorc during experimental autoimmune uveitis in mice. Mediators Of Inflammation. (2018) 2018:9129163. doi: 10.1155/2018/9129163
62. Lu TX, Rothenberg ME. MicroRNA. J Of Allergy And Clin Immunol. (2018) 141:1202–7. doi: 10.1016/j.jaci.2017.08.034
63. Lu Q, Wu R, Zhao M, Garcia-Gomez A, Ballestar E. Mirnas as therapeutic targets in inflammatory disease. Trends In Pharmacol Sci. (2019) 40(11):853–65. doi: 10.1016/j.tips.2019.09.007
64. Li X, Wei Y, Wang Z. MicroRNA-21 and hypertension. Hypertension Research: Off J Of Japanese Soc Of Hypertension. (2018) 41:649–61. doi: 10.1038/s41440-018-0071-z
65. Sekar D, Shilpa BR, Das AJ. Relevance of microRNA 21 in different types of hypertension. Curr Hypertension Rep. (2017) 19:57. doi: 10.1007/s11906-017-0752-z
66. Hu J, Huang S, Liu X, Zhang Y, Wei S, Hu X. Mir-155: an important role in inflammation response. J Of Immunol Res. (2022) 2022:7437281. doi: 10.1155/2022/7437281
67. Zhang A, Wang K, Zhou C, Gan Z, Ma D, Ye P, et al. Knockout of microRNA-155 ameliorates the th1/th17 immune response and tissue injury in chronic rejection. J Of Heart And Lung Transplantation: Off Publ Of Int Soc For Heart Transplant. (2017) 36(2):175–84. doi: 10.1016/j.healun.2016.04.018
68. Eshraghi R, Rafiei M, Hadian Jazi Z, Shafie D, Raisi A, Mirzaei H. MicroRNA-155 and exosomal microRNA-155: small pieces in the cardiovascular diseases puzzle. Pathology Res Pract. (2024) 257:155274. doi: 10.1016/j.prp.2024.155274
69. Sato F, Tsuchiya S, Meltzer SJ, Shimizu K. MicroRNAs and epigenetics. FEBS J. (2011) 278(10):1598–609. doi: 10.1111/j.1742-4658.2011.08089.x
70. Navaneethabalakrishnan S, Smith HL, Arenaz CM, Goodlett BL, McDermott JG, Mitchell BM. Update on immune mechanisms in hypertension. Am J Of Hypertension. (2022) 35(10):842–51. doi: 10.1093/ajh/hpac077
71. Liu XH, Ji QW, Huang Y, Zeng QT. Th17 response promotes angiotensin ii-induced atherosclerosis. Med Hypotheses. (2011) 76(4):593–5. doi: 10.1016/j.mehy.2011.01.008
72. Small HY, Migliarino S, Czesnikiewicz-Guzik M, Guzik TJ. Hypertension: focus on autoimmunity and oxidative stress. Free Radical Biol Med. (2018) 125:104–15. doi: 10.1016/j.freeradbiomed.2018.05.085
73. Gwon WG, Joung EJ, Kwon MS, Lim SJ, Utsuki T, Kim HR. Sargachromenol protects against vascular inflammation by preventing TNF-α-induced monocyte adhesion to primary endothelial cells via inhibition of NF-κB activation. Int Immunopharmacol. (2017) 42:81–9. doi: 10.1016/j.intimp.2016.11.014
74. Nallasamy P, Si H, Babu PV, Pan D, Fu Y, Brooke EA, et al. Sulforaphane reduces vascular inflammation in mice and prevents TNF-α-induced monocyte adhesion to primary endothelial cells through interfering with the NF-κB pathway. J Of Nutr Biochem. (2014) 25(8):824–33. doi: 10.1016/j.jnutbio.2014.03.011
75. Jia Z, Nallasamy P, Liu D, Shah H, Li JZ, Chitrakar R, et al. Luteolin protects against vascular inflammation in mice and TNF-alpha-induced monocyte adhesion to endothelial cells via suppressing IKBA/NF-κB signaling pathway. J Of Nutr Biochem. (2015) 26(3):293–302. doi: 10.1016/j.jnutbio.2014.11.008
76. Santisteban MM, Schaeffer S, Anfray A, Faraco G, Brea D, Wang G, et al. Meningeal interleukin-17-producing T cells mediate cognitive impairment in A mouse model of salt-sensitive hypertension. Nat Neurosci. (2024) 27(1):63–77. doi: 10.1038/s41593-023-01497-z
77. Guzik TJ, Nosalski R, Maffia P, Drummond GR. Immune and inflammatory mechanisms in hypertension. Nat Rev Cardiol. (2024) 21(6):396–416. doi: 10.1038/s41569-023-00964-1
78. Chen CN, Hajji N, Yeh FC, Rahman S, Ali S, Wharton J, et al. Restoration of FOXP3(+) regulatory T cells by hdac-dependent epigenetic modulation plays A pivotal role in resolving pulmonary arterial hypertension pathology. Am J Of Respir And Crit Care Med. (2023) 208(8):879–95. doi: 10.1164/rccm.202301-0181OC
79. Phoksawat W, Jumnainsong A, Sornkayasit K, Srisak K, Komanasin N, Leelayuwat C. IL-17 and IFN-Γ Productions by CD4+ T cells and T cell subsets expressing nkg2d associated with the number of risk factors for cardiovascular diseases. Mol Immunol. (2020) 122:193–9. doi: 10.1016/j.molimm.2020.04.003
80. Toussirot E, Gallais-Sérézal I, Aubin F. The cardiometabolic conditions of psoriatic disease. Front In Immunol. (2022) 13:970371. doi: 10.3389/fimmu.2022.970371
81. Nosalski R, Guzik TJ. Perivascular adipose tissue inflammation in vascular disease. Br J Of Pharmacol. (2017) 174:3496–513. doi: 10.1111/bph.v174.20
82. Guzik TJ, Mohiddin SA, Dimarco A, Patel V, Savvatis K, Marelli-Berg FM, et al. Covid-19 and the cardiovascular system: implications for risk assessment, diagnosis, and treatment options. Cardiovasc Res. (2020) 116(10):1666–87. doi: 10.1093/cvr/cvaa106
83. Yeh JL, Hsu JH, Liang JC, Chen IJ, Liou SF. Lercanidipine and labedipinedilol–A attenuate lipopolysaccharide/interferon-Γ-induced inflammation in rat vascular smooth muscle cells through inhibition of hmgb1 release and mmp-2, 9 activities. Atherosclerosis. (2013) 226(2):364–72. doi: 10.1016/j.atherosclerosis.2012.12.005
84. Qi J, Zheng JB, Ai WT, Yao XW, Liang L, Cheng G, et al. Felodipine inhibits ox-LDL-induced reactive oxygen species production and inflammation in human umbilical vein endothelial cells. Mol Med Rep. (2017) 16(4):4871–8. doi: 10.3892/mmr.2017.7181
85. Jukema JW, van der Hoorn JW. Amlodipine and atorvastatin in atherosclerosis: A review of the potential of combination therapy. Expert Opin On Pharmacotherapy. (2004) 5:459–68. doi: 10.1517/14656566.5.2.459
86. Fumagalli C, Maurizi N, Marchionni N, Fornasari D. β-blockers: their new life from hypertension to cancer and migraine. Pharmacol Res. (2020) 151:104587. doi: 10.1016/j.phrs.2019.104587
87. Argulian E, Bangalore S, Messerli FH. Misconceptions and facts about beta-blockers. Am J Of Med. (2019) 132:816–9. doi: 10.1016/j.amjmed.2019.01.039
88. Ogrodowczyk M, Dettlaff K, Jelinska A. Beta-blockers: current state of knowledge and perspectives. Mini Rev In Medicinal Chem. (2016) 16:40–54. doi: 10.2174/1389557515666151016125948
89. Liu X, Yang J, Deng W. The inflammatory cytokine IL-22 promotes murine gliomas via proliferation. Exp And Ther Med. (2017) 13:1087–92. doi: 10.3892/etm.2017.4059
90. Guevara M, Kollipara CS. Recent advances in giant cell arteritis. Curr Rheumatol Rep. (2018) 20:25. doi: 10.1007/s11926-018-0737-1
91. Zhang SX, Chen HR, Wang J, Shao HF, Cheng T, Pei RM, et al. The efficacy and safety of short-term and low-dose IL-2 combined with tocilizumab to treat rheumatoid arthritis. Front In Immunol. (2024) 15:1359041. doi: 10.3389/fimmu.2024.1359041
92. Fang Y, Chen M, Li G, Yang Y, He P, Chen J, et al. Cancer-associated fibroblast-like fibroblasts in vocal fold leukoplakia suppress cd8(+)T cell functions by inducing IL-6 autocrine loop and interacting with th17 cells. Cancer Lett. (2022) 546:215839. doi: 10.1016/j.canlet.2022.215839
93. Whelton PK, Carey RM, Aronow WS, Casey DE Jr, Collins KJ, Dennison Himmelfarb C, et al. 2017 aACC/AHA/AAPA/ABC/ACPM/AGS/APhA/ASH/ASPC/NMA/PCNA guideline for the prevention, detection, evaluation, and management of high blood pressure in adults: A report of the american college of cardiology/american heart association task force on clinical practice guidelines. Circulation. (2018) 138(17):e484–594. doi: 10.1161/CIR.0000000000000596
94. Luo T, Ji WJ, Yuan F, Guo ZZ, Li YX, Dong Y, et al. Th17/treg imbalance induced by dietary salt variation indicates inflammation of target organs in humans. Sci Rep. (2016) 6:26767. doi: 10.1038/srep26767
95. Andreo-López MC, Contreras-Bolívar V, Muñoz-Torres M, García-Fontana B, García-Fontana C. Influence of the mediterranean diet on healthy aging. Int J Of Mol Sci. (2023) 24(5):4491. doi: 10.3390/ijms24054491
96. Mentella MC, Scaldaferri F, Ricci C, Gasbarrini A, Miggiano GAD. Cancer and mediterranean diet: A review. Nutrients. (2019) 11(9):2059. doi: 10.3390/nu11092059
97. Ros E, Martínez-González MA, Estruch R, Salas-Salvadó J, Fitó M, Martínez JA, et al. Mediterranean diet and cardiovascular health: teachings of the predimed study. Adv Nutr (Bethesda Md). (2014) 5(3):330s–6s. doi: 10.3945/an.113.005389
98. Panagiotakis SH, Simos P, Basta M, Zaganas I, Perysinaki GS, Akoumianakis I, et al. Interactions of mediterranean diet, obesity, polypharmacy, depression and systemic inflammation with frailty status. Maedica. (2022) 17(1):20–7. doi: 10.26574/maedica.2022.17.1.20
99. Di Giosia P, Stamerra CA, Giorgini P, Jamialahamdi T, Butler AE, Sahebkar A. The role of nutrition in inflammaging. Ageing Res Rev. (2022) 77:101596. doi: 10.1016/j.arr.2022.101596
100. Kenđel Jovanović G, Mrakovcic-Sutic I, Pavičić Žeželj S, Šuša B, Rahelić D, Klobučar Majanović S. The efficacy of an energy-restricted anti-inflammatory diet for the management of obesity in younger adults. Nutrients. (2020) 12(11):3583. doi: 10.3390/nu12113583
101. Nani A, Murtaza B, Sayed Khan A, Khan NA, Hichami A. Antioxidant and anti-inflammatory potential of polyphenols contained in mediterranean diet in obesity: molecular mechanisms. Molecules. (2021) 26(4):985. doi: 10.3390/molecules26040985
102. Neal B, Wu Y, Feng X, Zhang R, Zhang Y, Shi J, et al. Effect of salt substitution on cardiovascular events and death. New Engl J Of Med. (2021) 385(12):1067–77. doi: 10.1056/NEJMoa2105675
103. Sugama K, Suzuki K, Yoshitani K, Shiraishi K, Kometani T. IL-17, neutrophil activation and muscle damage following endurance exercise. Exercise Immunol Rev. (2012) 18:116–27.
104. MaChado OAS, Diniz VLS, Passos MEP, de Oliveira HH, Santos-Oliveira LC, Alecrim AL, et al. Physical exercise increases global and gene-specific (Interleukin-17 and interferon-Γ) dna methylation in lymphocytes from aged women. Exp Physiol. (2021) 106(9):1878–85. doi: 10.1113/eph.v106.9
105. Golzari Z, Shabkhiz F, Soudi S, Kordi MR, Hashemi SM. Combined exercise training reduces IFN-Γ And IL-17 levels in the plasma and the supernatant of peripheral blood mononuclear cells in women with multiple sclerosis. Int Immunopharmacol. (2010) 10(11):1415–9. doi: 10.1016/j.intimp.2010.08.008
106. Jin F, Li Y, Gao X, Yang X, Li T, Liu S, et al. Exercise training inhibits macrophage-derived IL-17a-cxcl5-cxcr2 inflammatory axis to attenuate pulmonary fibrosis in mice exposed to silica. Sci Of Total Environ. (2023) 902:166443. doi: 10.1016/j.scitotenv.2023.166443
107. Chen Z, Yan W, Mao Y, Ni Y, Zhou L, Song H, et al. Effect of aerobic exercise on treg and th17 of rats with ischemic cardiomyopathy. J Of Cardiovasc Trans Res. (2018) 11(3):230–5. doi: 10.1007/s12265-018-9794-0
108. Duzova H, Karakoc Y, Emre MH, Dogan ZY, Kilinc E. Effects of acute moderate and strenuous exercise bouts on IL-17 production and inflammatory response in trained rats. J Of Sports Sci Med. (2009) 8(2):219–24.
109. Russo A, Bartolini D, Mensà E, Torquato P, Albertini MC, Olivieri F, et al. Physical activity modulates the overexpression of the inflammatory mir-146a-5p in obese patients. IUBMB Life. (2018) 70(10):1012–22. doi: 10.1002/iub.v70.10
110. Villareal DT, Aguirre L, Gurney AB, Waters DL, Sinacore DR, Colombo E, et al. Aerobic or resistance exercise, or both, in dieting obese older adults. New Engl J Of Med. (2017) 376(20):1943–55. doi: 10.1056/NEJMoa1616338
111. Henschel P, Landwehr-Kenzel S, Engels N, Schienke A, Kremer J, Riet T, et al. Supraphysiological FOXP3 expression in human car-tregs results in improved stability, efficacy, and safety of car-treg products for clinical application. J Of Autoimmun. (2023) 138:103057. doi: 10.1016/j.jaut.2023.103057
112. Nguyen DN, Roth TL, Li PJ, Chen PA, Apathy R, Mamedov MR, et al. Polymer-stabilized cas9 nanoparticles and modified repair templates increase genome editing efficiency. Nat Biotechnol. (2020) 38(1):44–9. doi: 10.1038/s41587-019-0325-6
113. Alvarez Salazar EK, Cortés-Hernández A, Alemán-Muench GR, Alberú J, Rodríguez-Aguilera JR, Recillas-Targa F, et al. Methylation of FOXP3 tsdr underlies the impaired suppressive function of tregs from long-term belatacept-treated kidney transplant patients. Front In Immunol. (2017) 8:219. doi: 10.3389/fimmu.2017.00219
114. Piotrowska M, Gliwiński M, Trzonkowski P, Iwaszkiewicz-Grzes D. Regulatory T cells-related genes are under dna methylation influence. Int J Of Mol Sci. (2021) 22(13):7144. doi: 10.3390/ijms22137144
115. Revenko A, Carnevalli LS, Sinclair C, Johnson B, Peter A, Taylor M, et al. Direct targeting of FOXP3 in tregs with AZD8701, A novel antisense oligonucleotide to relieve immunosuppression in cancer. J For Immunotherapy Of Cancer. (2022) 10(4):e003892. doi: 10.1136/jitc-2021-003892
116. Cortez JT, Montauti E, Shifrut E, Gatchalian J, Zhang Y, Shaked O, et al. CRISPR screen in regulatory T cells reveals modulators of FOXP3. Nature. (2020) 582(7812):416–20. doi: 10.1038/s41586-020-2246-4
117. Bézie S, Freuchet A, Sérazin C, Salama A, Vimond N, Anegon I, et al. IL-34 actions on FOXP3(+) tregs and CD14(+) monocytes control human graft rejection. Front In Immunol. (2020) 11:1496. doi: 10.3389/fimmu.2020.01496
118. Zhao H, Liao X, Kang Y. Tregs: where we are and what comes next? Front In Immunol. (2017) 8:1578. doi: 10.3389/fimmu.2017.01578
119. Wang J, Gong R, Zhao C, Lei K, Sun X, Ren H. Human FOXP3 and tumour microenvironment. Immunology. (2023) 168(2):248–55. doi: 10.1111/imm.v168.2
120. Borna Š, Lee E, Nideffer J, Ramachandran A, Wang B, Baker J, et al. Identification of unstable regulatory and autoreactive effector T cells that are expanded in patients with FOXP3 mutations. Sci Trans Med. (2023) 15(727):eadg6822. doi: 10.1126/scitranslmed.adg6822
121. Piotrowska M, Gliwiński M, Trzonkowski P, Iwaszkiewicz-Grzes D. Regulatory T cells-related genes are under dna methylation influence. Int J Of Mol Sci. (2021) 22(13):7144. doi: 10.3390/ijms22137144
122. Wang G, Yan Y, Xu N, Yin D, Hui Y. Treatment of type 1 diabetes by regulatory T-cell infusion via regulating the expression of inflammatory cytokines. J Of Cell Biochem. (2019) 120(12):19338–44. doi: 10.1002/jcb.v120.12
123. Dall'era M, Pauli ML, Remedios K, Taravati K, Sandova PM, Putnam AL, et al. Adoptive treg cell therapy in A patient with systemic lupus erythematosus. Arthritis Rheumatol (Hoboken NJ). (2019) 71(3):431–40. doi: 10.1002/art.2019.71.issue-3
124. Kvakan H, Kleinewietfeld M, Qadri F, Park JK, Fischer R, Schwarz I, et al. Regulatory T cells ameliorate angiotensin ii-induced cardiac damage. Circulation. (2009) 119(22):2904–12. doi: 10.1161/CIRCULATIONAHA.108.832782
125. Wilck N, Matus MG, Kearney SM, Olesen SW, Forslund K, Bartolomaeus H, et al. Salt-responsive gut commensal modulates T(H)17 axis and disease. Nature. (2017) 551(7682):585–9. doi: 10.1038/nature24628
126. Bartolomaeus H, Balogh A, Yakoub M, Homann S, Markó L, Höges S, et al. Short-chain fatty acid propionate protects from hypertensive cardiovascular damage. Circulation. (2019) 139(11):1407–21. doi: 10.1161/CIRCULATIONAHA.118.036652
127. Singh TP, Farias Amorim C, Lovins VM, Bradley CW, Carvalho LP, Carvalho EM, et al. Regulatory T cells control staphylococcus aureus and disease severity of cutaneous leishmaniasis. J Of Exp Med. (2023) 220(12):e20230558. doi: 10.1084/jem.20230558
128. Li ZH, Wang J, Xu JP, Wang J, Yang X. Recent advances in CRISPR-based genome editing technology and its applications in cardiovascular research. Military Med Res. (2023) 10(1):12. doi: 10.1186/s40779-023-00447-x
129. Ercu M, Markó L, Schächterle C, Tsvetkov D, Cui Y, Maghsodi S, et al. Phosphodiesterase 3A and arterial hypertension. Circulation. (2020) 142(2):133–49. doi: 10.1161/CIRCULATIONAHA.119.043061
130. Ercu M, Walter S, Klussmann E. Mutations in phosphodiesterase 3A (PDE3A) cause hypertension without cardiac damage. Hypertension (Dallas Tex: 1979). (2023) 80:1171–9. doi: 10.1161/HYPERTENSIONAHA.122.19433
131. Ercu M, Mücke MB, Pallien T, Markó L, Sholokh A, Schächterle C, et al. Mutant phosphodiesterase 3A protects from hypertension-induced cardiac damage. Circulation. (2022) 146(23):1758–78. doi: 10.1161/CIRCULATIONAHA.122.060210
132. Burnier M, Damianaki A. Hypertension as cardiovascular risk factor in chronic kidney disease. Circ Res. (2023) 132(23):1050–63. doi: 10.1161/CIRCRESAHA.122.321762
133. Wang C, Yuan Y, Zheng M, Pan A, Wang M, Zhao M, et al. Association of age of onset of hypertension with cardiovascular diseases and mortality. J Of Am Coll Of Cardiol. (2020) 75(23):2921–30. doi: 10.1016/j.jacc.2020.04.038
Keywords: hypertension, regulatory T cells, inflammation, immunotherapy, gene editing
Citation: Fu M, Lv M, Guo J, Mei A, Qian H, Yang H, Wu W, Liu Z, Zhong J, Wei Y, Min X, Wu H and Chen J (2025) The clinical significance of T-cell regulation in hypertension treatment. Front. Immunol. 16:1550206. doi: 10.3389/fimmu.2025.1550206
Received: 09 January 2025; Accepted: 10 February 2025;
Published: 26 February 2025.
Edited by:
Qingyu Luo, Dana–Farber Cancer Institute, United StatesReviewed by:
Kezhi Yan, Cystic Fibrosis Foundation, United StatesCopyright © 2025 Fu, Lv, Guo, Mei, Qian, Yang, Wu, Liu, Zhong, Wei, Min, Wu and Chen. This is an open-access article distributed under the terms of the Creative Commons Attribution License (CC BY). The use, distribution or reproduction in other forums is permitted, provided the original author(s) and the copyright owner(s) are credited and that the original publication in this journal is cited, in accordance with accepted academic practice. No use, distribution or reproduction is permitted which does not comply with these terms.
*Correspondence: Jun Chen, Y2hlbmp1bjAxMjFAMTI2LmNvbQ==; Haiyan Wu, a2VraTAyN0BzaW5hLmNvbQ==; Xinwen Min, bWlueGlud2VuQDE2My5jb20=
†These authors have contributed equally to this work
Disclaimer: All claims expressed in this article are solely those of the authors and do not necessarily represent those of their affiliated organizations, or those of the publisher, the editors and the reviewers. Any product that may be evaluated in this article or claim that may be made by its manufacturer is not guaranteed or endorsed by the publisher.
Research integrity at Frontiers
Learn more about the work of our research integrity team to safeguard the quality of each article we publish.