- 1School of Chemistry and Molecular Biosciences, University of Queensland, St. Lucia, QLD, Australia
- 2Global Virus Network Centre of Excellence, Australian Infectious Diseases Research Centre, Brisbane, QLD, Australia
- 3Inflammation Biology Group, QIMR Berghofer Medical Research Institute, Brisbane, QLD, Australia
Certain insect-specific viruses (ISVs), specifically the mosquito alphaviruses, Eilat and Yada Yada viruses, and orthoflaviviruses, Binjari, Aripo, YN15-283-02 and Chaoyang viruses, have emerged as potential platforms for generation of whole virus vaccines for human and veterinary applications. These ISVs are remarkably tolerant of the substitution of their structural polyproteins with those of alphaviruses and orthoflaviviruses that are pathogenic in humans and/or animals. The resulting ISV-based chimeric vaccines have been evaluated in mouse models and have demonstrated safety and efficacy in non-human primates, crocodiles and pigs. Targets include chikungunya, Venezuelan and eastern equine encephalitis, dengue, Zika, yellow fever, Japanese encephalitis and West Nile viruses. ISV-based chimeric vaccines provide authentically folded tertiary and quaternary whole virion particle structures to the immune system, a key feature for induction of protective antibody responses. These vaccines are manufactured in C6/36 or C7-10 mosquito cell lines, where they grow to high titers, but they do not replicate in vertebrate vaccine recipients. This review discusses the progress of these emerging technologies and addresses challenges related to adjuvanting, safety, and manufacturing.
1 Introduction
The WHO announced the Global Arbovirus Initiative in 2022 in response to the growing concerns over expanding outbreaks of arboviral diseases (1), which are primarily caused by pathogenic viruses in the genus Alphavirus (family Togaviridae) and the genus Orthoflavivirus (family Flaviviridae) (2). Urbanization, globalization, human mobility, and climate change, with the ensuing expansion of mosquito vectors, are all anticipated to increase the global burden of arboviral diseases (1, 3). A key intervention has been the development of vaccines (2) and herein we describe an emerging set of technologies that use mosquito alphaviruses and orthoflaviviruses to generate chimeric arboviral vaccines for human and veterinary applications.
Insect-specific viruses (ISVs) are viruses that replicate only in insects, and are distinct from arboviruses, which can replicate both in arthropod vectors (including insects) and vertebrate animal hosts. A range of factors prevent ISVs from infecting vertebrate cells, with such restriction occurring at various stages in the replication cycles (4–11). ISVs arguably represent the dark matter of virology, with vast numbers of ISVs identified by metagenomics (12–17), but only a few isolated and their behavior studied in vivo and in vitro (18). Studies so far indicate that ISVs are generally transmitted vertically from infected females to their offspring via the eggs (9, 19). Some ISVs appear to exhibit a narrow host range, with, for instance, some mosquito ISVs reported to infect only a limited number of mosquito species (12, 20, 21). ISVs are being explored as potential biological control agents for insects that threaten agricultural crops (22). Of some interest has also been the infection of mosquitoes with certain ISVs in order to inhibit, via various mechanisms, replication of pathogenic arboviruses in those mosquitoes (2, 23–26). However, herein we focus on six ISVs from mosquitoes, specifically, two alphaviruses, Eilat virus (EILV) and Yada Yada virus (YYV) and four orthoflaviviruses, Binjari virus (BinJV), Aripo virus (ARPV), YN15-283-02 virus and Chaoyang virus (CYV). For these ISVs, their structural genes can be exchanged with the structural genes from a range of pathogenic alphaviruses and orthoflaviviruses, respectively. A resulting chimeric virus would thus encode the structural proteins of a pathogenic arbovirus and the non-structural polyproteins of one of the aforementioned ISVs. These chimeric viruses are able to replicate efficiently in mosquito cell lines, but are unable to replicate in vertebrate cells. This has allowed the development of a range of ISV-based chimeric vaccines for a number of arboviral diseases (Figure 1).
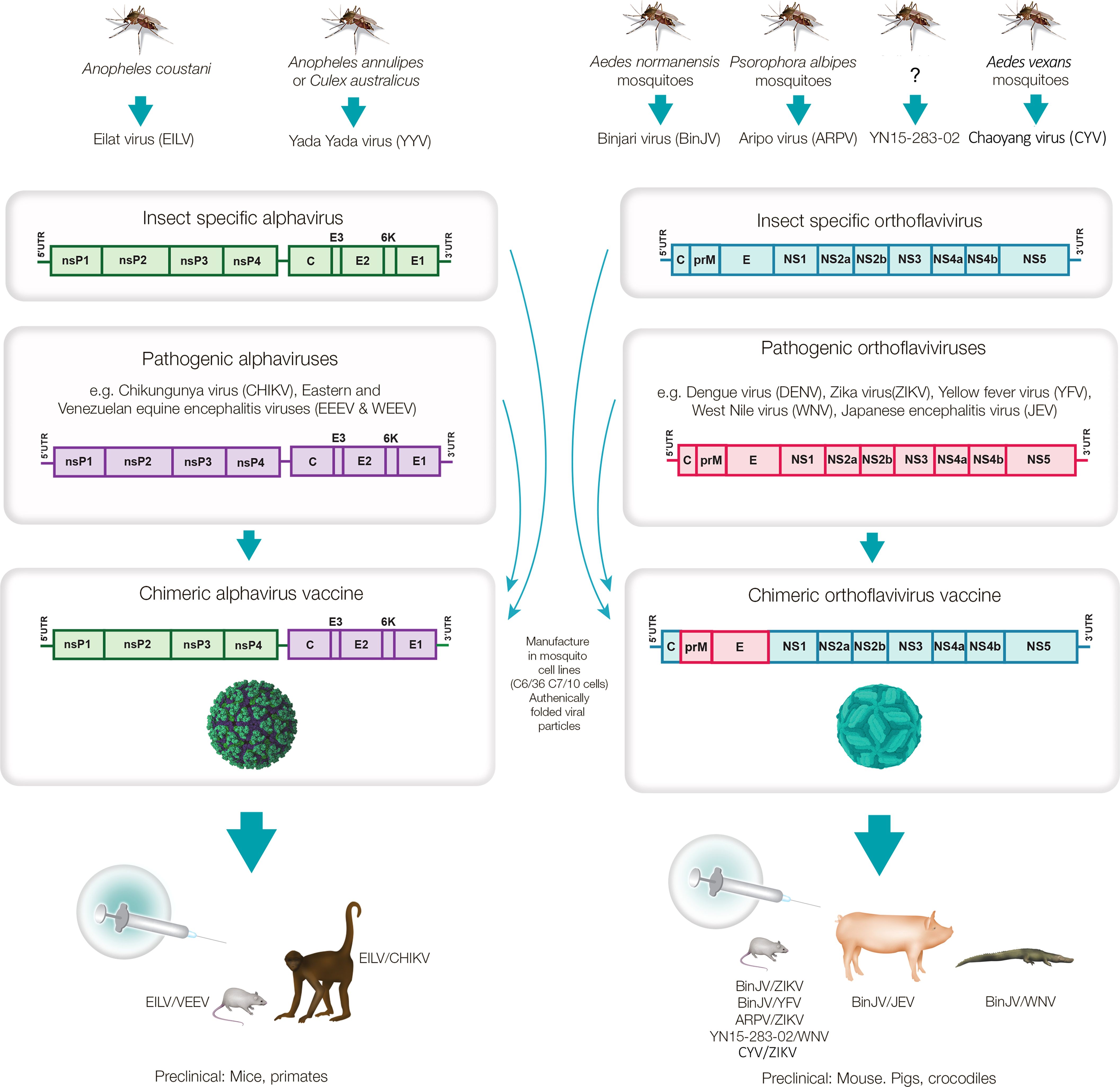
Figure 1. Mosquito-derived ISVs used to generate chimeric virus vaccines against pathogenic alphaviruses and orthoflaviviruses. The mosquito alphaviruses, Eilat virus and Yada Yada virus, and the mosquito orthoflaviruses, Binjari virus, Aripo virus, YN15-283-02 and Chaoyang virus have been used to generate chimeric viruses encoding the structural polyproteins of pathogenic alphaviruses (C-E3-E2-6K-E1) and orthoflaviviruses (CprME), respectively. The chimeric viruses replicate to high titers in mosquito cell lines to produce authentically folded virion particles that can be used as whole-virion vaccines that have provided protective immune responses in a number of animal species. (?) - YN15-283-02 was derived from midge (Culicoides) samples, but the virus was described as a mosquito orthoflavivirus.
A series of chimeric vaccines based on EILV, YYV, BinJV, ARPV, YN15-283-02 and CYV have now been described. They are manufactured in mosquito cell lines and resemble virus-like-particle (VLP) vaccines, as they essentially represent whole-virus, protein-based vaccines that cannot generate viral progeny in vertebrate vaccine recipients (Figure 1). They differ from VLP vaccines (27) in that they contain a fully functioning viral genome that is replication competent in mosquito cells. ISV-chimeric vaccines also differ from licensed live-attenuated orthoflavivirus chimeric virus vaccines such as Imojev, for Japanese encephalitis virus (JEV) and Dengvaxia for dengue virus (DENV), which are replication competent in vaccine recipients. The latter encode the structural proteins of JEV and DENV, but are attenuated as they encode the non-structural proteins of the yellow fever virus (YFV) 17D vaccine strain (2). An experimental chimeric live-attenuated alphavirus vaccine has also been reported for Venezuelan Equine Encephalitis virus (VEEV), and encodes the structural proteins of VEEV (TC-83 vaccine strain) and the non-structural proteins of Sindbis virus (28). Thus, chimeric vaccines for alphaviruses and orthoflaviviruses are well described; however, in contrast to the aforementioned live-attenuated chimeric vaccines, ISV-based chimeric vaccines are naturally replication-defective in vaccine recipients as the ISV RNA replication complex, encoded by the non-structural proteins, is non-functional in vertebrate cells. Like many VLP vaccines [e.g. (29, 30)], ISV-based chimeric vaccines present authentically folded structural proteins of the pathogenic arboviruses to the immune system and thereby promote induction of effective neutralizing antibody responses (2).
Herein we describe the ISV-based chimeric vaccine technologies and focus on the immunological issues associated with these technologies including potential self-adjuvanting activity, formulation with adjuvants, provision of authentic tertiary and quaternary structures, safety issues and manufacturing.
2 The ISV-based chimeric virus vaccine platforms
2.1 The Eilat virus platform for alphavirus vaccine development
Eilat virus (EILV) is an alphavirus isolated from a pool of Anopheles coustani mosquitoes from the Negev desert of Israel (31) and arguably represents the “poster child” for using ISV chimeras for vaccine development (32, 33). Standard cloning methodology, using an infectious cDNA clone of EILV, was used to generate the EILV chimeras with the structural polyprotein (C-E3-E2-6K-E1) of pathogenic alphaviruses replacing those from EILV to generate chimeric viruses (Figure 1) (32, 34). These chimeric viruses are unable to replicate in vertebrate cells even after electroporation of chimeric RNA genomes into vertebrate cell lines. Furthermore, no overt adverse outcomes were seen even after intracranial injections into Ifnar-/- mice (35).
A number of chimeras were generated including, Mayaro virus (EILV/MAYV), o’nyong-nyong virus (EILV/ONNV), Sindbis virus (EILV/SINV) and western equine encephalitis virus (WEEV) (32). In addition, EILV-based chimeric viruses have been evaluated as vaccines for Venezuelan equine encephalitis virus (EILV/VEEV), eastern equine encephalitis virus (EILV/EEEV) (33), and chikungunya (EILV/CHIKV) (35, 36). These vaccines, without adjuvant formulation, provided protection against challenge in mouse and non-human primate (NHP) models after a single immunization (Figure 1, Table 1, Supplementary Table 1).
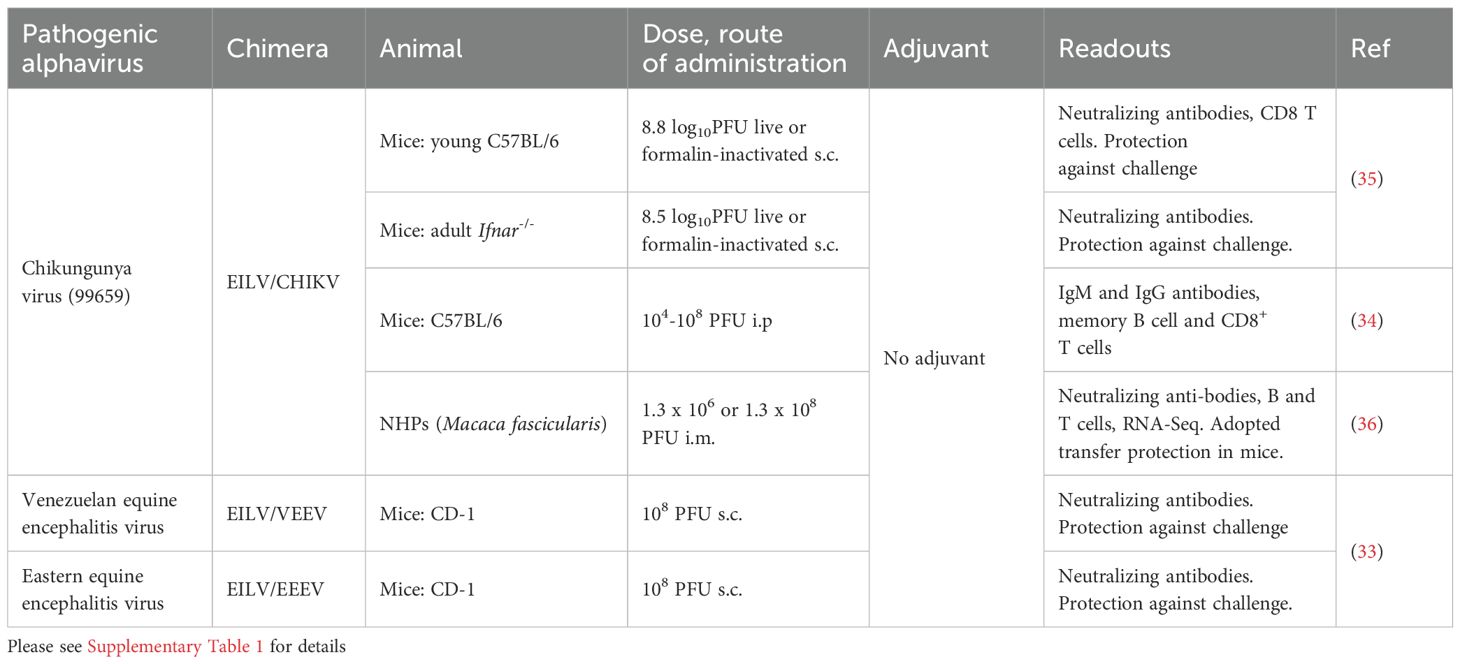
Table 1. Eilat virus (EILV) chimeric vaccines for pathogenic alphaviruses evaluated in mice and NHPs.
2.2 The Yada Yada virus platform for alphavirus vaccine development
Yada Yada virus (YYV) was initially identified by metagenomic sequencing of mosquitoes trapped as part of the Victorian Arbovirus Disease Control Program (Australia) in 2016 (37). YYV shows a 75.7% amino acid identity with EILV (37) (GenBank QGR15363.1). Infectious YYV was generated using circular polymerase extension reaction (CPER) methodology, with transfection of C6/36 cells (38). In brief, CPER involves stitching together overlapping synthetic dsDNA fragments covering the complete viral genome, with a circularizing linker fragment containing a promoter for transcribing viral RNA in cells. Replicating virus is then recovered after direct transfection of CPER into mammalian or insect cells capable of supporting replication (8, 39–42). A simplified schematic explaining the CPER methodology is provided in Supplementary Figure 1.
CPER was used to generate YYV chimeric viruses, with the structural polyprotein of YYV replaced with those from pathogenic alphaviruses. The chimeras included CHIKV (YYV/CHIKV), SINV (YYV/SINV), as well as Ross River virus (YYV/RRV) and Barmah Forest virus (YYV/BFV) (Figure 1), with both YYV and the YYV-chimeras unable to replicate in vertebrate cell lines (38). RRV and BFV are Australasian arthritogenic alphaviruses with symptoms similar to, but usually less severe than, CHIKV (43–45). An inactivated whole virus RRV vaccine was previously shown to be well tolerated and immunogenic in a phase 3 human trial (46). The YYV/CHIKV chimera (38) is currently undergoing evaluation as a vaccine to protect against CHIKV in an adult wild-type mouse model (47).
2.3 The Binjari virus platform for orthoflavivirus vaccine development
Binjari virus (BinJV) was isolated in 2016 from a pool of Aedes normanensis mosquitoes trapped at the Bradshaw Field Training Area (Northern Territory, Australia). The sequence (GenBank; MG587038) illustrated that BinJV grouped with the lineage II insect-specific flaviviruses (5, 48). BinJV chimeric virus vaccines were generated using the CPER methodology, with the prME genes of BinJV replaced with the prME genes of pathogenic orthoflaviviruses (Figure 1), with both BinJV and the BinJV-chimeras unable to replicate in vertebrate cell lines (48). BinJV-based chimeric vaccines have now been generated for a range of pathogenic flaviviruses and evaluated in mouse models, crocodiles and pigs (Table 2; Supplementary Table 2).
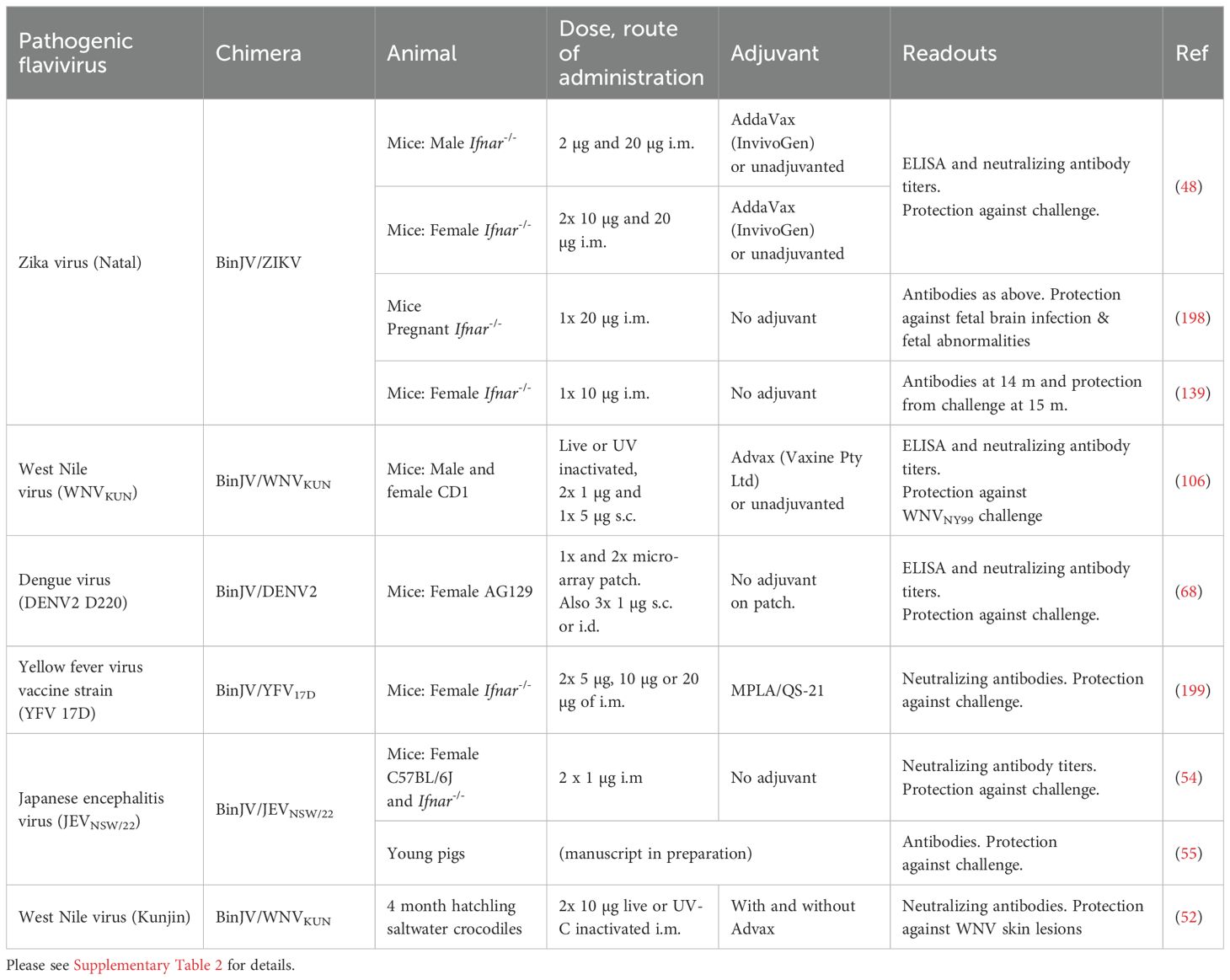
Table 2. Binjari virus (BinJV) chimeric vaccines for pathogenic orthoflaviviruses evaluated in mice, crocodiles and pigs.
Farmed crocodiles in Africa (Crocodylus niloticus) and Australia (C. porosus) and alligators (Alligator mississippiensis) in the USA can be infected by West Nile virus (WNV), with an outbreak of severe neurological disease reported in an alligator farm in Florida in 2002 (49). In Australia, farmed saltwater crocodiles (C. porosus) can develop dark spotted “pix” skin lesions, which results in loss of value or rejection of the hides. These lesions arise from infection with Kunjin virus, the Australian strain of WNV, which shows low virulence or is non-pathogenic in humans (50), but affects horses and crocodiles (51, 52). Two intra-muscular (i.m.) vaccinations of hatchling crocodiles with BinJV/WNVKUN vaccine resulted in no detectable skin lesions after WNVKUN challenge (52) (Table 2; Supplementary Table 2).
An unprecedented outbreak of JEV genotype IV occurred in humans (45 cases, 7 deaths) and pigs (>80 piggeries) in Australia in 2022 (53). Although sera from Imojev (genotype 3) vaccinated humans cross-reacted with a virus isolated from the 2022 outbreak (JEVNSW/22), JEVNSW/22 was neutralized at significantly lower serum dilutions when compared with genotype 3 JEV isolates (53). This prompted the generation of a BinJV/JEVNSW/22 vaccine, which showed efficacy in a mouse model (54), with a media release also reporting a successful trial of this vaccine in pigs (55).
2.4 The Aripo virus platform for orthoflavivirus vaccine development
Aripo virus (ARPV) was isolated from Psorophora albipes mosquitoes collected in Trinidad in 2008 (56) (Figure 1). A chimeric ARPV/ZIKV vaccine was unable to replicate in vertebrate cells (57) and vaccination of C57BL/6J dams resulted in protection of the ~4 week old offspring from ZIKV challenge (58) (Table 3; Supplementary Table 3). A follow-up study suggested that antibodies played the primary role in protection mediated by the ARPV/ZIKV vaccine, with cell mediated responses playing a minor role (59).
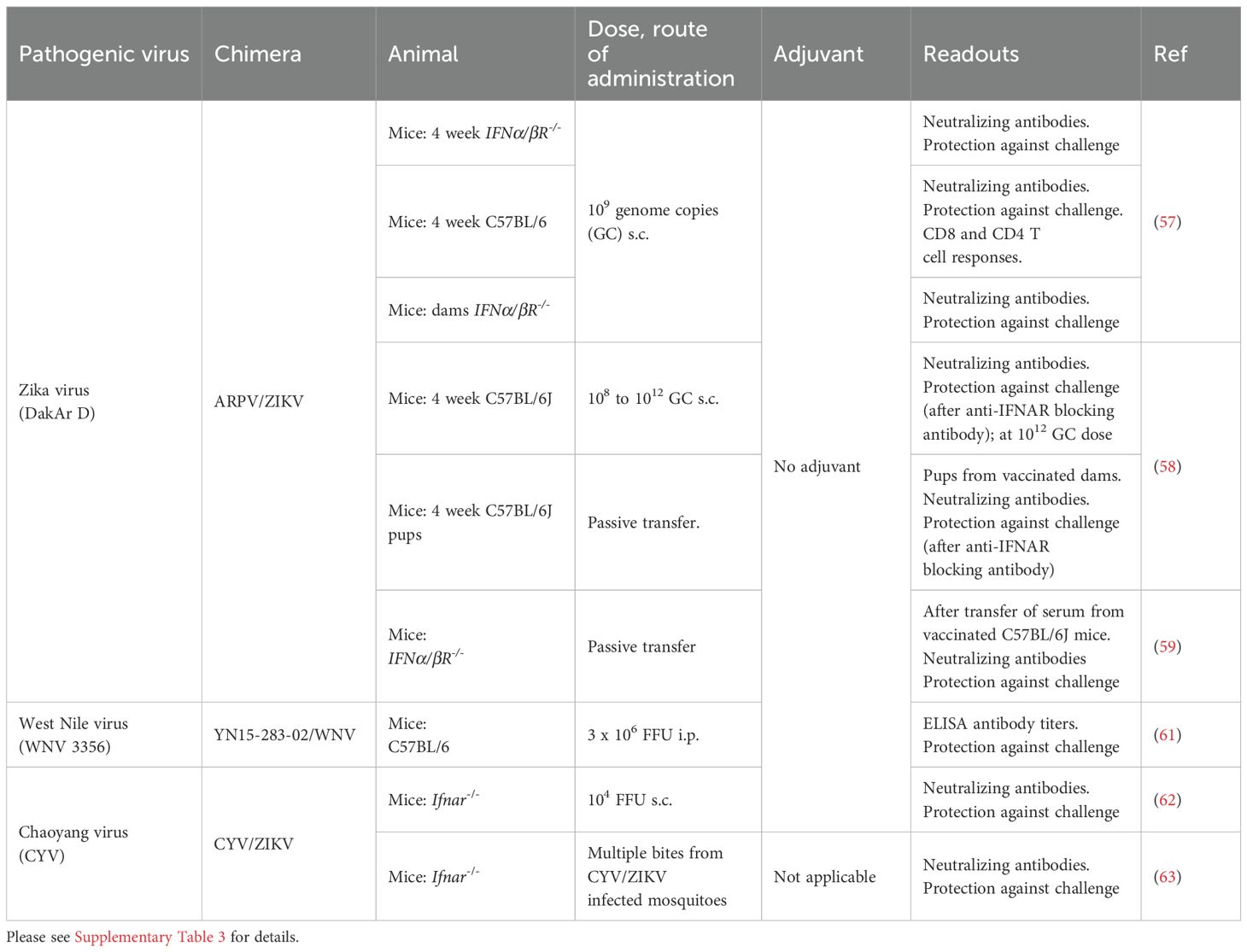
Table 3. Aripo virus (ARPV), YN15-283-02 virus and Chaoyang virus chimeric vaccines for pathogenic orthoflaviviruses evaluated in mice.
2.5 The YN15-283-02 virus platform for orthoflavivirus vaccine development
YN15-283-02 is a mosquito orthoflavivirus that was identified by sequencing the supernatant of C6/36 cells inoculated with a midge homogenate collected in Yunnan, China, with virus recovered using an infectious clone (60). A chimeric YN15-283-02/WNV vaccine was constructed, harvested from the supernatants of infected C6/36 cells, and used to vaccinate C57/BL6 mice. The mice generated Th1 biased antibody responses and were protected from WNV challenge (61) (Table 3; Supplementary Table 3). YN15-283-02/WNV was unable to replicate in vertebrate cells and was also non-pathogenic in Ifnar-/- mice (61).
2.6 The Chaoyang virus platform for orthoflavivirus vaccine development
Chaoyang virus (CYV) was initially isolated from Aedes vexans in China, with a single CYV/ZIKV vaccination providing partial protection from ZIKV challenge (62) (Table 3; Supplementary Table 3). In addition, immunization of mice via bites from mosquitoes infected with CYV/ZIKV, elicited ZIKV-specific immune responses (increasing after 2 and 3 bites) and conferred protection against ZIKV challenge (63) (Table 3; Supplementary Table 3). No virus replication was detected in Ifnar-/- mice bitten by such mosquitoes (63), and no signs of infection were seen in one day old suckling in Ifnar-/- mice inoculated intracranially with CYV/ZIKV (62).
3 Adjuvant issues for ISV-based chimeric virus vaccines
3.1 Self-adjuvanting properties of ISV-based chimeric vaccines?
RNA-Seq studies have suggested that ISV-based chimeric vaccines may mediate self-adjuvanting properties via the induction of type I interferon (IFN) responses, triggered via Toll-like receptors (TLRs) and RIG-I (DDX58) (34, 36, 56, 64). TLR and RIG-I agonists, primarily via induction of type I IFNs, are well known to provide adjuvant activity (65–67). Several reports of effective immune response generation without formulation of ISV-based chimeric vaccines with adjuvants (Tables 1-3; Supplementary Tables 1-3) would appear to support the contention of self-adjuvanting activity. However, although purification processes have been developed (34, 68), to date these vaccines have not been shown to have been purified to Good Laboratory Practice (GLP) or Good Manufacturing Practice (GMP) standards. Given they are isolated from supernatants of infected insect cell lines, contamination with material from infected cells (69) or cell debris is likely, although cytopathic effects in infected insect cell lines may be moderate rather than overt (70, 71). Contaminants with TLR-stimulating activity might include mRNA and genomic/mitochondrial DNA from the insect cell line, as well as unencapsidated chimeric viral genomic single-stranded RNA (ssRNA), subgenomic RNA, and/or double-stranded RNA (dsRNA) replication intermediates. RNA-Seq data and subsequent bioinformatic analyses would be unable convincingly to distinguish between stimulation of type I IFN stimulated genes (ISGs) via TLR-stimulating contaminates versus true self-adjuvanting activity, such as might be mediated by stimulation of cytoplasmic sensors (like RIG-I). The two pathways induce an extensively overlapping set of ISG mRNAs.
Notwithstanding the aforementioned issues, delivery of ISV-based chimeric vaccine ssRNA into the cytoplasm of vertebrate cells would appear likely, although no clear viral RNA replication has been detected in wild-type vertebrate cells (5, 6, 10, 33, 35, 48, 52). Capping of viral RNA substantially reduces RIG-I stimulation (72). However, as many alphavirus virions can contain uncapped ssRNA genomes (73), incoming viral nucleocapsids containing viral RNA with exposed 5′-triphosphates may trigger RIG-I, without the requirement for viral RNA synthesis/replication (74). Whether a significant proportion of incoming orthoflavivirus chimeric vaccine RNA genomes would be uncapped remains unclear. Other sensors like Protein Kinase R (PKR) and ZAP could also detect cytoplasmic vaccine ssRNA. ZAP binds ssRNA rich in CpG dinucleotides (11, 75) and potently promotes RIG-I signaling (76), with CpGs present with relatively high frequencies in ISVs (10, 77). PKR recognizes short (∼15 bp) stem-loop RNA structures containing flanking ssRNA sequences (78), which are present in alphaviral and orthoflavivirus genomes, both in the untranslated (79–82), and likely also in the translated regions of the genomes (83). PKR has a role in NLRP3 inflammasome activation (84, 85), a pathway also stimulated by aluminium-based adjuvants (86). PKR stimulation also stabilizes IFNβ mRNA, thereby promoting type I IFN responses (87). Also conceivable is stimulation of TLR7, although this would require release of encapsidated vaccine ssRNA from the virion (presumably within or into an endosome), RNA processing, and then access of the products to TLR7 in endosomes (88).
In summary, self-adjuvant activity arising from genomic ssRNA delivered by ISV-based chimeric vaccines can be envisaged. However, this has yet to be formally distinguished from immunostimulatory contaminants by illustrating that purified material produced to GLP/GMP standards, retains adequate self-adjuvanting activity.
3.2 Formulation with adjuvants
ISV-based chimeric vaccines provide authentically folded virus particle immunogens to the immune system, much like many established VLP-based vaccines. Most VLP vaccine products currently in the market are adjuvanted (89, 90), and some pre-clinical studies on BinJV-based chimeric vaccines have also used adjuvant formulations (Table 2). For instance, formulation of the BinJV/WNVKUN vaccine with Advax slightly improved neutralizing antibody titers in crocodiles, although this did not reach significance (52). A baculovirus manufactured COVID-19 vaccine formulated with Advax, SpikoGen, recently received marketing authorization in Iran (91).
Adjuvants provide dose sparing (less immunogen thus required per dose), with the cost of immunogen manufacture often relatively high for cell culture-derived VLP-based vaccine products (92). Cost considerations are amplified for livestock vaccines, where, despite their clear benefits (93), commercial cost-benefit calculations usually require livestock vaccines to be considerably cheaper than human vaccines. In addition to standard aluminium-based adjuvants (94), new adjuvants for human use and applied to VLP vaccine products (90) have emerged in recent years and include MF59 (95) and the AS0 series (GSK) (e.g. Cervarix, AS04) (96). New adjuvants for livestock products include (i) ImpranFLEX, a proprietary water-based polymer adjuvant used in Ciroflex (Boehringer Ingelheim), a baculovirus-generated VLP product for porcine circovirus type 2, (ii) light liquid paraffin used in Porcilis PCV (Intervet), a baculovirus-generated VLP vaccine for porcine circovirus type 2 (97), (iii) Emulsigen (MVP adjuvants), a new oil-in-water adjuvant that has USDA approval for use in pigs, and (iv) Montanide adjuvants (SEPPIC) approved in the EU.
4 Inactivation of ISV-based chimeric virus vaccines?
An ISV-based chimeric vaccine would be viewed as a Genetically Modified Organism (GMO) in most regulatory environments. A series of potential ensuing risks can be perceived after release of these organisms into the environment (see below), with a range of varied restrictions applied in different countries (98, 99). To avoid such risks, ISV-based chimeric vaccines have been inactivated using traditional methods such as formalin fixation (35, 55), UV-irradiation (52) or X-ray irradiation (63). The former clearly reduced induction of neutralizing antibody responses to EILV/CHIKV chimeras, with formalin fixation preventing cell entry (35) and reducing immunogenicity (100) by inter alia irreversibly modifying lysine residues [discussed in (101)]. Nevertheless, formalin inactivated ISV-based chimeric vaccines, just like many formalin-inactivated whole virus vaccine products (2), can provide protective immune responses (35, 55). Manufacturing and regulatory processes for formalin inactivation of whole virus vaccines are also well established (102). UV-inactivation of RNA viruses is primarily achieved through uracil and cytosine dimer formation (103). However, irradiation technologies are still primarily in the research and development phase for whole virus vaccines (104, 105) and are not currently used for commercial whole arbovirus vaccine products (2). Delivering the correct irradiation dose safely, evenly and consistently during large scale manufacture (i.e. enough to inactivate, but not too much to damage immunogenicity) represent challenges for these processes. For instance, although a UV-inactivated BinJV/WNVKUN vaccine still afforded protection in mice, neutralizing antibody levels induced by the UV-inactivated vaccine were significantly reduced (106).
Arguably, inactivation of ISV-based chimeric vaccines negates a key advantage of this technology as these vaccines are already intrinsically “inactive”, being unable to replicate in vertebrates. Avoiding the down-stream processes for inactivation and validation of inactivation protocols should represent a key advantage for these ISV-based technologies. Inactivation would remove the GMO classification, and thus the ensuing regulatory hurdles (99), as in most jurisdictions an organism would be defined (in this context) as a replication competent entity. Environmental risks of dissemination and recombination would clearly also be removed by inactivation. However, it should be noted that use of replication competent GMOs as vaccine products is already well established, with a number of licensed live-attenuated arboviral vaccines classed as GMOs e.g. (i) Ixchiq (Valneva), the recently approved CHIKV vaccine that has a 186 nucleotide (62 amino acid) deletion in nsP3, (ii) Imojev (Sanofi), a JEV vaccine, which has the non-structural proteins from YFV 17D, (iii) Dengvaxia (Sanofi), a DENV vaccine, also with a YFV backbone, and (iv) Qdenga (Takeda), where all four serotypes have the non-structural proteins of DENV2. Recombinant virally vectored vaccines are also classified as GMOs with a range of these now also licensed, e.g. (i) Covishield (AstraZeneca) a recombinant adenovirus vaccine for COVID-19, (ii) Raboral (Boehringer Ingelheim), a recombinant vaccinia veterinary vaccine for rabies, and (iii) Trovac-NDV (Boehringer Ingelheim), a recombinant fowlpox livestock vaccine for Newcastle disease (107).
5 The role of authentic tertiary and quaternary structures
The importance of presenting the immune system with authentically folded arboviral vaccine immunogens is widely recognized (92, 108), with protective antibodies often targeting quaternary epitopes (109–111). Currently licensed orthoflavivirus and alphavirus vaccines likely deliver antigens with authentically folded tertiary and quaternary protein structures, facilitated by the robust ability of orthoflavi- and alpha-viral structural polyproteins to self-assemble into virion particles in a range of settings (2).
ISV-based chimeric vaccines are fully functional viruses in insect cells and assemble into authentic virion particle structures (33, 48, 61, 112). For instance, low resolution analysis of BinjV chimeras by cryo EM revealed that the virus particles accurately mimic the virion structure of the wild-type pathogens (112). However, subtle differences may emerge to be important, for instance, ortho flavivirus envelope proteins are able to “breath”, adopting more “bumpy” versus “smooth” configurations at the virion surface. Ensuring that an ISV-based chimeric vaccine has the same structure or conformational dynamics as the contemporary pathogenic orthoflavivirus being targeted by the vaccine, may be important for optimizing vaccine efficacy (113).
Authentically folded immunogens are important for induction of antibodies capable of high-affinity and high-avidity binding to the target arbovirus virions and arbovirus infected cells. The vaccine-induced antibodies can be both neutralizing and non-neutralizing, with the latter also mediating a range of protective activities that are generally difficult to measure in clinical trial settings (2). Indirect evidence for a protective role for non-neutralizing, vaccine-induced, antibodies in humans comes from inter alia a phase III trial of Qdenga, where neutralizing antibody titers against DENV2, DENV3 and DENV4 did not correlate with protection (114).
6 The role of non-structural proteins and CD8 T cell responses
For all the ISV-based chimeric vaccines the non-structural proteins of the pathogenic arboviruses are not present as vaccine antigens. These proteins are nsP1, nsP2, nsP3, nsP4 for alphaviruses, and NS1, NS2A, NS2B, NS3, NS4A, NS4B, NS5 for orthoflaviviruses (Figure 1). The ISV versions of these non-structural proteins are synthesized in the insect cell lines used to manufacture the chimeric vaccines. ISV-based chimeric vaccines also contain ssRNA chimeric genomes, which encode the non-structural proteins of the ISV (but are not translated in vaccine recipients) (Figure 1).
Ixchiq, the recently approved, live-attenuated CHIKV vaccine, would present CHIKV nsPs to vaccine recipients, whereas this would not occur for non-replicating VLP-based or ISV-based chimeric vaccines. Anti-nsP responses likely do not play a significant role in protection against pathogenic alphavirus infections, a contention supported inter alia by successful phase 3 trials recently reported for an aluminum hydroxide adjuvanted, CHIKV VLP vaccine (115). Anti-alphaviral CD8 T cell responses may play a minor role in protection, but they are generally viewed as secondary to antibody responses (116–120). CHIKV appears able to evade surveillance by antiviral CD8 T cells, in part, by nsP2-mediated disruption of MHC-I antigen presentation (121). Nevertheless, induction of CD8 T cells was shown for the EILV/CHIKV vaccine in mice (34) and NHPs (36), and for the ARPV/ZIKV vaccine in mice (59), suggesting that these vaccines can infect and endosome escape, thereby delivering structural protein antigens into the MHC-I processing pathway (122).
In contrast to alphaviruses, protective immune responses directed at orthoflaviviral non-structural proteins, in particular NS1, are well described, with a number of groups pursuing the development of NS1-based vaccines (123–127). Although cross-reactivity of anti-ZIKV NS1 responses with self has been described (128), a causal link to autoimmune disease (129) has yet to be established (130, 131). NS1 proteins of flaviviruses show a degree of sequence homology (132), so live attenuated vaccines such as Dengvaxia (Sanofi) and IMOJEV (Sanofi Pasteur) with a YFV 17D backbone, would likely induce anti-NS1 responses capable of cross-reacting with DENV and JEV NS1 proteins, respectively. Qdenga (Takeda), with a DENV2 backbone, would arguably induce better anti-DENV NS1 responses (2), as sequence homologies between NS1 from DENV2 versus DENV1, 3 and 4 are more substantial (132). However, even if anti-NS1 protein responses can mediate effective protection, a number of licensed orthoflavivirus vaccines achieve adequate protection without inducing such responses, specifically, the inactivated vaccines for (i) JEV, Ixiaro (Valneva), (ii) tick-borne encephalitis virus, Encepur (Bavarian Nordic), and (iii) West Nile virus, Innovator (a horse vaccine from Zoetis). An inactivated virus vaccine for ZIKV was also recently shown to be effective in pregnant marmosets (133).
7 Induction of long-term protective immune responses
An ideal characteristic for many alphavirus and orthoflavivirus vaccines would be provision of long-term protective immunity, an issue particularly pertinent for human vaccines in resource poor settings, although clearly less of an issue for short-lived livestock. Perhaps a standout is the YFV 17D vaccine, which provides life-long immunity in humans after a single vaccination (134). Non-replicating, protein-based vaccines are often viewed as providing poor long-term protection. However, this is not entirely accurate with, for instance, the whole-virion-based, formalin inactivated, aluminium hydroxide adjuvanted, hepatitis A vaccine providing protection for >20 years, and the VLP-based, aluminium hydroxide adjuvanted, human papilloma virus vaccine (Gardasil), providing protection for up to 14 years (135). The current COVID-19 mRNA vaccines would appear to perform poorly with respect to durability of protective responses, with neutralizing antibody responses waning within 6 months (136). However, this may be related to an inability to generate spike-specific, long-lived plasma cells (137). The durability of protection after vaccination with mRNA vaccines against arboviruses has yet to be evaluated (138). The same goes for ISV-based chimeric vaccines, and may depend on the choice of adjuvant. Perhaps encouraging, a single dose of a BinJV/ZIKV vaccine, with no adjuvant formulation, provided protective immunity in Ifnar-/- mice for 15 months (139).
8 Vaccine manufacture
Manufacture of human and animal vaccines need to adhere to a series of production and quality control standards, with requirements for human vaccines considerably more onerous than for animal vaccines (140, 141). The number of cell lines available for manufacture of human products is limited, but include CHO (142), HEK293 (143) and Vero cells (144), as well as baculovirus systems that use insect cells from Spodoptera frugiperda (armyworm moth) (29, 145). However, to date, no large-scale vaccine production systems have been developed for mosquito cell lines. A desirable property of C6/36 cells is that they do not contain adventitious viruses (146), with such agents having caused problems for human and veterinary vaccine products in the past (147, 148). Another desirable property of ISV-chimeric virus vaccines is that they often grow to higher titers in insect cell lines than their parental viruses (34), with, for instance, the BinJV/ZIKV chimera produced yields of up to ~109.5 CCID50/mL or ~7 mg/liter (48). Production and purification of EILV/CHIKV vaccine in C7-10 cells has been described, with culture in serum free medium for 18 h before harvest, followed by Cellufine sulfate column and then sucrose gradient purification (34). This purified vaccine, delivered once i.m. at up to 1.3 x 108 PFU, showed no overt adverse effects in NHPs (36). Manufacturing and purification processes for BinJV chimeric vaccines have also been developed and involve growth in C6/36 cells and purification by density gradient centrifugation (54, 68). A serum free, suspension culture system was recently described for production of BinJV chimeras in C6/36 cells, representing an important step in the development of a scalable manufacturing system (149).
A number of critical steps remain for development of human ISV-based vaccines. These include (i) regulatory clearance for a master cell bank of a suitable mosquito cell line, (ii) characterization of impurities and development of GLP/GMP vaccine production and purification processes, (iii) identification of suitable excipients and storage protocols that preserve vaccine immunogenicity, and (iv) development of quality control processes to monitor vaccine production. Thereafter, phase I human trials, which primarily determine safety, might be initiated. For veterinary vaccines, depending on the animal species, a priority is often cost-effectiveness, so manufacturing costs will generally need to be low (150).
9 Safety considerations
9.1 Reversion to virulence
Reversion to virulence for ISV-based chimeric vaccines is unlikely as ISV replication is blocked or restricted at various levels in the vertebrate host (4–8). Viral proteins have evolved to interact with various host proteins to promote efficient replication, and to interfere with the hosts anti-viral responses (151–153). These virus-host interactions (or interactomes) are often quite species specific, with, for instance, some ISVs only replicating in a limited number of mosquito species (12, 20, 21). The probability of ISV non-structural proteins undergoing extensive mutations to overcome the multiple restrictions (4–11) and provide an ISV-based chimeric vaccine with the capacity to replicate in vertebrate vaccine recipients with any degree of efficiency, might thus be viewed as extremely low.
9.2 Mosquito cell line derived impurities as potential allergens
Mosquitos are found on every continent of the world except Antarctica, with over half the world’s population at risk from diseases spread by mosquitoes (154). Individuals bitten by mosquitoes are exposed to mosquito allergens that are present in the mosquito saliva and injected into the skin by mosquitoes taking a blood meal. Saliva proteins, once injected, generally stimulate a series of acute immune responses (155–158) and induce a local itch response that usually involves IgE-mediated hypersensitivity (159). Local allergic reactions to mosquito bites are usually clinically mild and self-limiting, but in certain individuals can be more severe. For instance, Skeeter syndrome, arising from IgE and IgG responses against mosquito saliva, can occur in immunocompromised individuals, resulting in large local inflammatory reactions, occasionally accompanied by fever and, more rarely, lymphadenopathy (159, 160). However, few, if any, human deaths have occurred as a result of anaphylactic shock caused by a mosquito bite (161). In addition, salivary gland proteins are being considered as potential vaccines for mosquito borne diseases (to induce IgG rather than IgE responses) (162).
C6/36 and C7-10 cells are derived from Aedes albopictus larvae, and likely share allergens (or contain immunologically cross-reactive allergens) with mosquito saliva proteins, as supernatants from C6/36 cultures injected into human skin can induce hypersensitivity and anaphylactic reactions (163). Unfortunately, no C6/36 allergens doses (i.e. µg of protein per injection) were provided in this study. The EILV/CHIKV vaccine, purified by Cellufine sulfate affinity chromatography column (AMS-BIO) and sucrose gradient centrifugation (34), was shown to cause little or no skin hypersensitivity reactions in mice or guinea pigs sensitized to mosquito bites (34, 36). The level of purification and thus the allergen dose is likely to be a central issue. For instance, pre-existing egg allergy is no longer considered a contra-indication for modern egg-derived influenza vaccines (164). This is not the case for the yellow fever vaccine, which contains higher levels of egg allergens (165). Future research will likely need to characterize the mosquito-derived proteins that co-purify with the ISV-based vaccines, and compare these with allergenic mosquito salivary proteins (166) in order to better understand the potential risks. Nevertheless, predisposition to Skeeter syndrome (and perhaps other anaphylactic conditions) may emerge to be a contra-indication for C6/36 and C7-10-derived vaccines.
9.3 Transmission to mosquitoes
As determined for other live attenuated arboviral vaccines, like the YFV 17D vaccine (167, 168) and the VEEV vaccine (TC-83) (169), the capacity of ISV-based chimeric vaccines to be transmitted to, and by, mosquitoes may need to be characterized. Concerns might arise from (i) introduction of a GMO into the environment and (ii) the potential of the vaccine to interact with the mosquito virome (see below), although both ISV and pathogenic arbovirus genes are already present in the environment. Another potential concern is transmission of a GMO to other humans without their consent; however, given the small doses delivered by mosquito bites and the absence of replication post-delivery, human welfare is unlikely to be impacted.
As ISV-based chimeric vaccines do not replicate in the vaccine recipient, the mosquito would need to be infected by virus from the vaccine inoculum. The mosquito would thus need to take a blood meal at or near the injection site and close to the time of inoculation. The mosquito proboscis penetrates about 2-3 mm into the skin to reach a blood capillary, whereas subcutaneous (and certainly intramuscular) injections are usually deeper, with dissemination into local capillaries likely to be transient. The titers imbibed by the mosquito would also need to be high enough to initiate infection and transmission, with a number of threshold barriers to infection and transmission recognized for mosquitoes. These barriers primarily regulate virus escape from the midgut, entry into the salivary glands, replication in the salivary glands, and release of virus into the saliva (170–172). CYV/ZIKV was able to infect and disseminate to the saliva in Aedes aegypti, and less so in Aedes albopictus, after artificial blood meals containing 108 FFU/mL (63). Once initiated, amplification of ISV-based chimeric vaccines in the mosquito might be expected, given the high titer replication of ISV-based chimeric viruses in insect cell lines (35, 48). Formulation of the ISV-based chimeric vaccines with adjuvants, for instance, by adsorption onto aluminium-based adjuvants or by formulation in emulsion adjuvants, would likely reduce the probability of uptake and infection of mosquitoes.
9.4 Recombination
The ability of alphaviruses to recombine is well described (173, 174), whereas orthoflaviviruses appear to have a much lower propensity to recombine (175, 176). Recombination would require the ISV-based chimeric vaccine and another virus to replicate in the same cell, something which would likely only be possible after infection of mosquitoes. Superinfection exclusion may mitigate against ISV-based chimeric vaccine and another virus infecting the same cell in the mosquito (2, 177). Nevertheless, any potential interactions with complex mosquito viromes might be viewed as unpredictable, given our limited understanding of these complex ecosystems (12, 13, 178). However, ISVs and pathogenic arboviruses have already extensively co-infected mosquitoes, with all the genes in ISV-based chimeric vaccines thus having already been present in mosquito populations over evolutionary time periods.
10 Needle-free delivery opportunities
Needle-free vaccine delivery overcomes needle-phobia, eliminates needle-stick injuries and the necessity for sharps disposal, and may emerge to be attractive and cost-effective in mass vaccination campaigns, especially in resource poor settings (179). Two devices have been licensed for delivery of vaccines, Stratis (PharmaJet) for delivery of inactivated influenza vaccine (Afluria, Seqirus) in the USA, and Tropis (PharmaJet) for delivery of the ZyCoV-D DNA COVID-19 vaccine (Zydus Lifesciences) in India (180) for Restricted Use in Emergency Situations. These devices are applied to the skin and deliver vaccines via a narrow precise fluid stream that penetrates the skin. Similar needle free systems have also been developed for the livestock industry and have the advantage of reducing pain, saving time, avoiding lesions in muscles (meat products), as well as avoiding the aforementioned safety issues associated with needle use (181).
An alternative needle-free vaccine delivery technology involves microarray patches or microprojection arrays (182), which involves dry coating of vaccine onto microprojections arrayed on small patches that are applied to the skin and that deliver antigen to skin antigen presenting cells (183, 184). A number of microarray patches have been evaluated in phase I human trials for inter alia influenza, measles, rubella, and SARS-CoV-2 vaccines. They were generally regarded as safe and well tolerated, inducing similar or increased immune responses when compared with conventional needle-based vaccination (185–188).
The technology developed by Vaxxas (188–190) has been used to deliver a BinJV-based chimeric vaccine for DENV that provided protection in a mouse model (68, 191).
11 Conclusions
A number of mosquito ISV-based chimeric vaccines for human arboviral pathogens have been developed and been shown to generate protective immunity in preclinical studies in mice and NHPs. However, human vaccine applications will require a certified mosquito cell line and GMP manufacturing and purification processes to be established, with evaluation of different adjuvant formulations also likely required. Ultimately, ISV-based chimeric vaccines may have to compete with mRNA vaccine technologies (192, 193) on inter alia cost of goods, durability of responses, and/or safety. Manufacture of ISV-based vaccines is at an early stage and data is currently limited. Conceivably, the manufacturing performance of mosquito cell line systems might emerge to be comparable with baculovirus systems (145); however, cell line-derived, whole-virus vaccines that need inactivation and adjuvanting generally have a relatively high cost of goods (194). Future developments in mRNA vaccine technology may also reduce the costs of this new technology (195). Specific adjuvants may be required to promote responses and durability of protection of ISV-based chimeric vaccines in humans (2, 135). New developments may also lead to improvements in the durability of responses after vaccination with mRNA vaccines (196). The safety profile of mRNA vaccines is now well established with billions of doses delivered globally (197). The safety profile for ISV-based vaccines has yet to be formally established and must await phase I human trials.
Livestock applications have been illustrated for BinJV/WNVKUN for crocodiles and BinJV/JEV for pigs. Although unable to replicate in vaccine recipients, inactivation (e.g. formalin) of ISV-based chimeric vaccines removes the regulatory hurdles associated with release of GMOs and avoids any risk of transmission to mosquitoes. Whether such vaccines would emerge to be cost-effective enough for livestock markets has yet to be determined.
Author contributions
RH: Writing – review & editing. WN: Writing – review & editing. AK: Writing – review & editing. AS: Writing – original draft, Writing – review & editing.
Funding
The author(s) declare that financial support was received for the research and/or publication of this article. WN was funded by a philanthropic donation from the Brazil Family Foundation for JEV research at QIMR Berghofer MRI. AS was supported by an Investigator grant awarded to AS from the National Health and Medical Research Council of Australia (APP1173880).
Acknowledgments
The authors would like to thank Dr J Hobson-Peters for her extensive input, Madeleine Flynn for graphic design, and Dr Viviana Lutzky for proof reading.
Conflict of interest
RH and AK are inventors on a patent WO/2018/176075, which covers the BinJV technology.
The remaining authors declare that the research was conducted in the absence of any commercial or financial relationships that could be construed as a potential conflict of interest.
Generative AI statement
The author(s) declare that no Generative AI was used in the creation of this manuscript.
Publisher’s note
All claims expressed in this article are solely those of the authors and do not necessarily represent those of their affiliated organizations, or those of the publisher, the editors and the reviewers. Any product that may be evaluated in this article, or claim that may be made by its manufacturer, is not guaranteed or endorsed by the publisher.
Supplementary material
The Supplementary Material for this article can be found online at: https://www.frontiersin.org/articles/10.3389/fimmu.2025.1521104/full#supplementary-material
References
1. Wallau GL, Abanda NN, Abbud A, Abdella S, Abera A, Ahuka-Mundeke S, et al. Arbovirus researchers unite: expanding genomic surveillance for an urgent global need. Lancet Global Health. (2023) 11:e1501–e2. doi: 10.1016/S2214-109X(23)00325-X
2. Rawle DJ, Hugo LE, Cox AL, Devine GJ, Suhrbier A. Generating prophylactic immunity against arboviruses in vertebrates and invertebrates. Nat Rev Immunol. (2024) 24:621–36. doi: 10.1038/s41577-024-01016-6
3. Naddaf M. Mosquito-borne diseases are surging in Europe - how worried are scientists? Nature. (2024) 633:749. doi: 10.1038/d41586-024-03031-y
4. Elrefaey AM, Abdelnabi R, Rosales Rosas AL, Wang L, Basu S, Delang L. Understanding the mechanisms underlying host restriction of insect-specific viruses. Viruses. (2020) 12:964. doi: 10.3390/v12090964
5. Harrison JJ, Hobson-Peters J, Colmant AMG, Koh J, Newton ND, Warrilow D, et al. Antigenic characterization of new lineage II insect-specific flaviviruses in Australian mosquitoes and identification of host restriction factors. mSphere. (2020) 5:e00095–20. doi: 10.1128/msphere.00095-20
6. Nasar F, Gorchakov RV, Tesh RB, Weaver SC. Eilat virus host range restriction is present at multiple levels of the virus life cycle. J Virol. (2015) 89:1404–18. doi: 10.1128/JVI.01856-14
7. Tangudu CS, Hargett AM, Blitvich BJ. Evidence that untranslated genomic sequences are key determinants of insect-specific flavivirus host restriction. Virology. (2022) 574:102–14. doi: 10.1016/j.virol.2022.07.013
8. Piyasena TBH, Newton ND, Hobson-Peters J, Vet LJ, Setoh YX, Bielefeldt-Ohmann H, et al. Chimeric viruses of the insect-specific flavivirus Palm Creek with structural proteins of vertebrate-infecting flaviviruses identify barriers to replication of insect-specific flaviviruses in vertebrate cells. J Gen Virol. (2019) 100:1580–6. doi: 10.1099/jgv.0.001326
9. Peterson AJ, Hall RA, Harrison JJ, Hobson-Peters J, Hugo LE. Unleashing nature’s allies: comparing the vertical transmission dynamics of insect-specific and vertebrate-infecting flaviviruses in mosquitoes. Viruses. (2024) 16:1499. doi: 10.3390/v16091499
10. Colmant AMG, Hobson-Peters J, Slijkerman TAP, Harrison JJ, Pijlman GP, van Oers MM, et al. Insect-specific flavivirus replication in mammalian cells is inhibited by physiological temperature and the zinc-finger antiviral protein. Viruses. (2021) 13:573. doi: 10.3390/v13040573
11. Fros JJ, Visser I, Tang B, Yan K, Nakayama E, Visser TM, et al. The dinucleotide composition of the Zika virus genome is shaped by conflicting evolutionary pressures in mammalian hosts and mosquito vectors. PloS Biol. (2021) 19:e3001201. doi: 10.1371/journal.pbio.3001201
12. Pan Y-F, Zhao H, Gou Q-Y, Shi P-B, Tian J-H, Feng Y, et al. Metagenomic analysis of individual mosquito viromes reveals the geographical patterns and drivers of viral diversity. Nat Ecol Evol. (2024) 8:947–59. doi: 10.1038/s41559-024-02365-0
13. Zakrzewski M, Rasic G, Darbro J, Krause L, Poo YS, Filipovic I, et al. Mapping the virome in wild-caught Aedes aEgypti from Cairns and Bangkok. Sci Rep. (2018) 8:4690. doi: 10.1038/s41598-018-22945-y
14. Ramírez Ana L, Colmant Agathe MG, Warrilow D, Huang B, Pyke Alyssa T, McMahon Jamie L, et al. Metagenomic analysis of the virome of mosquito excreta. mSphere. (2020) 5:e00587–20. doi: 10.1128/msphere.00587-20
15. Moonen JP, Schinkel M, van der Most T, Miesen P, van Rij RP. Composition and global distribution of the mosquito virome - A comprehensive database of insect-specific viruses. One Health. (2023) 16:100490. doi: 10.1016/j.onehlt.2023.100490
16. Qi Y-H, Ye Z-X, Zhang C-X, Chen J-P, Li J-M. Diversity of RNA viruses in agricultural insects. Comput Struct Biotechnol J. (2023) 21:4312–21. doi: 10.1016/j.csbj.2023.08.036
17. Hollingsworth BD, Grubaugh ND, Lazzaro BP, Murdock CC. Leveraging insect-specific viruses to elucidate mosquito population structure and dynamics. PloS Pathog. (2023) 19:e1011588. doi: 10.1371/journal.ppat.1011588
18. Carvalho VL, Long MT. Insect-Specific Viruses: An overview and their relationship to arboviruses of concern to humans and animals. Virology. (2021) 557:34–43. doi: 10.1016/j.virol.2021.01.007
19. Nag DK, Efner KJ. Transovarial transmission of cell-fusing agent virus in naturally infected aedes aEgypti mosquitoes. Viruses. (2024) 16:1116. doi: 10.3390/v16071116
20. Nasar F, Haddow AD, Tesh RB, Weaver SC. Eilat virus displays a narrow mosquito vector range. Parasit Vectors. (2014) 7:595. doi: 10.1186/s13071-014-0595-2
21. McLean BJ, Hobson-Peters J, Webb CE, Watterson D, Prow NA, Nguyen HD, et al. A novel insect-specific flavivirus replicates only in Aedes-derived cells and persists at high prevalence in wild Aedes vigilax populations in Sydney, Australia. Virology. (2015) 486:272–83. doi: 10.1016/j.virol.2015.07.021
22. Sun K, Fu K, Hu T, Shentu X, Yu X. Leveraging insect viruses and genetic manipulation for sustainable agricultural pest control. Pest Manage Sci. (2024) 80:2515–27. doi: 10.1002/ps.7878
23. de Faria IJS, de Almeida JPP, Marques JT. Impact of symbiotic insect-specific viruses on mosquito vector competence for arboviruses. Curr Opin Insect Sci. (2024) 63:101194. doi: 10.1016/j.cois.2024.101194
24. Nasar F, Erasmus JH, Haddow AD, Tesh RB, Weaver SC. Eilat virus induces both homologous and heterologous interference. Virology. (2015) 484:51–8. doi: 10.1016/j.virol.2015.05.009
25. Joseph RE, Bozic J, Werling KL, Krizek RS, Urakova N, Rasgon JL. Eilat virus (EILV) causes superinfection exclusion against West Nile virus (WNV) in a strain-specific manner in Culex tarsalis mosquitoes. J Gen Virol. (2024) 105:002017. doi: 10.1099/jgv.0.002017
26. Chen J, Deng S, Peng H. Insect-specific viruses used in biocontrol of mosquito-borne diseases. Interdiscip Med. (2023) 1:e20220001. doi: 10.1002/INMD.20220001
27. Kheirvari M, Liu H, Tumban E. Virus-like particle vaccines and platforms for vaccine development. Viruses. (2023) 15:1109. doi: 10.3390/v15051109
28. Paessler S, Fayzulin Rafik Z, Anishchenko M, Greene Ivorlyne P, Weaver Scott C, Frolov I. Recombinant sindbis/Venezuelan equine encephalitis virus is highly attenuated and immunogenic. J Virol. (2003) 77:9278–86. doi: 10.1128/jvi.77.17.9278-9286.2003
29. Miao Q, Nguyen W, Zhu J, Liu G, van Oers MM, Tang B, et al. A getah virus-like-particle vaccine provides complete protection from viremia and arthritis in wild-type mice. Vaccine. (2024) 42:126136. doi: 10.1016/j.vaccine.2024.07.037
30. Raji AA, Dastjerdi PZ, Omar AR. Virus-like particles in poultry disease: an approach to effective and safe vaccination. Front Veterinary Sci. (2024) 11:1405605. doi: 10.3389/fvets.2024.1405605
31. Nasar F, Palacios G, Gorchakov RV, Guzman H, Da Rosa AP, Savji N, et al. Eilat virus, a unique alphavirus with host range restricted to insects by RNA replication. Proc Natl Acad Sci U.S.A. (2012) 109:14622–7. doi: 10.1073/pnas.1204787109
32. Tan L, Zhang Y, Wang X, Kim DY. A productive expression platform derived from host-restricted eilat virus: its extensive validation and novel strategy. Viruses. (2021) 13:660. doi: 10.3390/v13040660
33. Erasmus JH, Seymour RL, Kaelber JT, Kim DY, Leal G, Sherman MB, et al. Novel insect-specific eilat virus-based chimeric vaccine candidates provide durable, mono- and multivalent, single-dose protection against lethal alphavirus challenge. J Virol. (2018) 92:10–1128. doi: 10.1128/JVI.01274-17
34. Adam A, Luo H, Osman SR, Wang B, Roundy CM, Auguste AJ, et al. Optimized production and immunogenicity of an insect virus-based chikungunya virus candidate vaccine in cell culture and animal models. Emerg Microbes Infect. (2021) 10:305–16. doi: 10.1080/22221751.2021.1886598
35. Erasmus JH, Auguste AJ, Kaelber JT, Luo H, Rossi SL, Fenton K, et al. A chikungunya fever vaccine utilizing an insect-specific virus platform. Nat Med. (2017) 23:192–9. doi: 10.1038/nm.4253
36. Adam A, Woolsey C, Lu H, Plante K, Wallace SM, Rodriguez L, et al. A safe insect-based Chikungunya fever vaccine affords rapid and durable protection in cynomolgus macaques. NPJ Vaccines. (2024) 9:251. doi: 10.1101/2024.05.21.595029
37. Batovska J, Buchmann Jan P, Holmes Edward C, Lynch Stacey E. Coding-complete genome sequence of yada yada virus, a novel alphavirus detected in Australian mosquitoes. Microbiol Resource Announcements. (2020) 9:10–1128. doi: 10.1128/mra.01476-19
38. Bell MG, Parry RH, Lee TSE, Habarugira G, McMahon IE, Thompson MA, et al. Synthetic recovery of Yada Yada virus expands insect-specific alphavirus knowledge and facilitates production of chimeric viruses. NPJ Viruses. (2024) 2:45. doi: 10.1038/s44298-024-00052-2
39. Edmonds J, van Grinsven E, Prow N, Bosco-Lauth A, Brault Aaron C, Bowen Richard A, et al. A novel bacterium-free method for generation of flavivirus infectious DNA by circular polymerase extension reaction allows accurate recapitulation of viral heterogeneity. J Virol. (2013) 87:2367–72. doi: 10.1128/jvi.03162-12
40. Amarilla AA, Sng JDJ, Parry R, Deerain JM, Potter JR, Setoh YX, et al. A versatile reverse genetics platform for SARS-CoV-2 and other positive-strand RNA viruses. Nat Commun. (2021) 12:3431. doi: 10.1038/s41467-021-23779-5
41. Setoh YX, Prow NA, Peng N, Hugo LE, Devine G, Hazlewood JE, et al. De novo generation and characterization of new zika virus isolate using sequence data from a microcephaly case. mSphere. (2017) 2:e00190–17. doi: 10.1128/mspheredirect.00190-17
42. Piyasena TBH, Setoh YX, Hobson-Peters J, Newton ND, Bielefeldt-Ohmann H, McLean BJ, et al. Infectious DNAs derived from insect-specific flavivirus genomes enable identification of pre- and post-entry host restrictions in vertebrate cells. Sci Rep. (2017) 7:2940. doi: 10.1038/s41598-017-03120-1
43. Suhrbier A, Jaffar-Bandjee MC, Gasque P. Arthritogenic alphaviruses–an overview. Nat Rev Rheumatol. (2012) 8:420–9. doi: 10.1038/nrrheum.2012.64
44. Zaid A, Burt FJ, Liu X, Poo YS, Zandi K, Suhrbier A, et al. Arthritogenic alphaviruses: epidemiological and clinical perspective on emerging arboviruses. Lancet Infect Dis. (2021) 21:e123–e33. doi: 10.1016/S1473-3099(20)30491-6
45. Mylonas AD, Brown AM, Carthew TL, McGrath B, Purdie DM, Pandeya N, et al. Natural history of Ross River virus-induced epidemic polyarthritis. Med J Aust. (2002) 177:356–60. doi: 10.5694/j.1326-5377.2002.tb04837.x
46. Wressnigg N, van der Velden Maikel VW, Portsmouth D, Draxler W, O’Rourke M, Richmond P, et al. An inactivated ross river virus vaccine is well tolerated and immunogenic in an adult population in a randomized phase 3 trial. Clin Vaccine Immunol. (2015) 22:267–73. doi: 10.1128/CVI.00546-14
47. Prow NA, Liu L, Nakayama E, Cooper TH, Yan K, Eldi P, et al. A vaccinia-based single vector construct multi-pathogen vaccine protects against both Zika and chikungunya viruses. Nat Commun. (2018) 9:1230. doi: 10.1038/s41467-018-03662-6
48. Hobson-Peters J, Harrison JJ, Watterson D, Hazlewood JE, Vet LJ, Newton ND, et al. A recombinant platform for flavivirus vaccines and diagnostics using chimeras of a new insect-specific virus. Sci Transl Med. (2019) 11:eaax7888. doi: 10.1126/scitranslmed.aax7888
49. Simulundu E, Ndashe K, Chambaro HM, Squarre D, Reilly PM, Chitanga S, et al. West nile virus in farmed crocodiles, Zambia, 2019. Emerg Infect Dis. (2020) 26:811–4. doi: 10.3201/eid2604.190954
50. Gray TJ, Burrow JN, Markey PG, Whelan PI, Jackson J, Smith DW, et al. West nile virus (Kunjin subtype) disease in the northern territory of Australia–a case of encephalitis and review of all reported cases. Am J Trop Med Hyg. (2011) 85:952–6. doi: 10.4269/ajtmh.2011.11-0165
51. Frost MJ, Zhang J, Edmonds JH, Prow NA, Gu X, Davis R, et al. Characterization of virulent West Nile virus Kunjin strain, Australia, 2011. Emerg Infect Dis. (2012) 18:792–800. doi: 10.3201/eid1805.111720
52. Habarugira G, Harrison JJ, Moran J, Suen WW, Colmant AMG, Hobson-Peters J, et al. A chimeric vaccine protects farmed saltwater crocodiles from West Nile virus-induced skin lesions. NPJ Vaccines. (2023) 8:93. doi: 10.1038/s41541-023-00688-w
53. Nguyen W, Gyawali N, Stewart R, Tang B, Cox AL, Yan K, et al. Characterisation of a Japanese Encephalitis virus genotype 4 isolate from the 2022 Australian outbreak. NPJ Viruses. (2024) 2:15. doi: 10.1038/s44298-024-00025-5
54. Harrison JJ, Nguyen W, Morgan MS, Tang B, Habarugira G, de Malmanche H, et al. A chimeric vaccine derived from Australian genotype IV Japanese encephalitis virus protects mice from lethal challenge. NPJ Vaccines. (2024) 9:134. doi: 10.1038/s41541-024-00903-2
55. UQ_News. Vaccine to protect pigs from Japanese encephalitis virus. Available online at: https://www.uq.edu.au/news/article/2023/02/vaccine-protect-pigs-Japanese-encephalitis-virus (Accessed Oct 2024).
56. Auguste AJ, Langsjoen RM, Porier DL, Erasmus JH, Bergren NA, Bolling BG, et al. Isolation of a novel insect-specific flavivirus with immunomodulatory effects in vertebrate systems. Virology. (2021) 562:50–62. doi: 10.1016/j.virol.2021.07.004
57. Porier DL, Wilson SN, Auguste DI, Leber A, Coutermarsh-Ott S, Allen IC, et al. Enemy of my enemy: a novel insect-specific flavivirus offers a promising platform for a Zika virus vaccine. Vaccines. (2021) 9:1142. doi: 10.3390/vaccines9101142
58. Tanelus M, López K, Smith S, Muller JA, Porier DL, Auguste DI, et al. Exploring the immunogenicity of an insect-specific virus vectored Zika vaccine candidate. Sci Rep. (2023) 13:19948. doi: 10.1038/s41598-023-47086-9
59. Porier DL, Adam A, Kang L, Michalak P, Tupik J, Santos MA, et al. Humoral and T-cell-mediated responses to an insect-specific flavivirus-based Zika virus vaccine candidate. PloS Pathog. (2024) 20:e1012566. doi: 10.1371/journal.ppat.1012566
60. Huang Y, Zhang H, Li X, Zhao L, Cai D, Wang S, et al. In vitro and in vivo characterization of a new strain of mosquito flavivirus derived from culicoides. Viruses. (2022) 14:1298. doi: 10.3390/v14061298
61. Zhang HQ, Li N, Zhang ZR, Deng CL, Xia H, Ye HQ, et al. A chimeric classical insect-specific flavivirus provides complete protection against West Nile virus lethal challenge in mice. J Infect Dis. (2024) 229:43–53. doi: 10.1093/infdis/jiad238
62. Dong H-L, Chen Z-L, He M-J, Cui J-Z, Cheng H, Wang Q-Y, et al. The chimeric chaoyang-zika vaccine candidate is safe and protective in mice. Vaccines. (2024) 12:215. doi: 10.3390/vaccines12020215
63. Wen D, Ding LS, Zhang Y, Li X, Zhang X, Yuan F, et al. Suppression of flavivirus transmission from animal hosts to mosquitoes with a mosquito-delivered vaccine. Nat Commun. (2022) 13:7780. doi: 10.1038/s41467-022-35407-x
64. Hazlewood JE. Zika virus: developing and exploiting mouse models for preclinical evaluation of new vaccines. Brisbane, Australia: The University of Queensland (2022). Bioproject PRJNA1170411.
65. Kayesh MEH, Kohara M, Tsukiyama-Kohara K. TLR agonists as vaccine adjuvants in the prevention of viral infections: an overview. Front Microbiol. (2023) 14:1249718. doi: 10.3389/fmicb.2023.1249718
66. Martínez-Gil L, Goff PH, Hai R, García-Sastre A, Shaw ML, Palese P. A sendai virus-derived RNA agonist of RIG-I as a virus vaccine adjuvant. J Virol. (2013) 87:1290–300. doi: 10.1128/jvi.02338-12
67. Beljanski V, Chiang C, Kirchenbaum Greg A, Olagnier D, Bloom Chalise E, Wong T, et al. Enhanced influenza virus-like particle vaccination with a structurally optimized RIG-I agonist as adjuvant. J Virol. (2015) 89:10612–24. doi: 10.1128/jvi.01526-15
68. Choo JJY, Vet LJ, McMillan CLD, Harrison JJ, Scott CAP, Depelsenaire ACI, et al. A chimeric dengue virus vaccine candidate delivered by high density microarray patches protects against infection in mice. NPJ Vaccines. (2021) 6:66. doi: 10.1038/s41541-021-00328-1
69. Martínez-Rojas PP, Quiroz-García E, Monroy-Martínez V, Agredano-Moreno LT, Jiménez-García LF, Ruiz-Ordaz BH. Participation of extracellular vesicles from zika-virus-infected mosquito cells in the modification of naïve cells’ Behavior by mediating cell-to-cell transmission of viral elements. Cells. (2020) 9:123. doi: 10.3390/cells9010123
70. Rivera JA, Rengifo AC, Sarmiento L, Diaz T, Laiton-Donato K, Gracia M, et al. Nuclei ultrastructural changes of C6/36 cells infected with virus dengue type 2. Biomedica. (2018) 38:135–43. doi: 10.7705/biomedica.v38i0.3997
71. Wang H, Blair CD, Olson KE, Clem RJ. Effects of inducing or inhibiting apoptosis on Sindbis virus replication in mosquito cells. J Gen Virol. (2008) 89:2651–61. doi: 10.1099/vir.0.2008/005314-0
72. Schweibenz BD, Solotchi M, Hanpude P, Devarkar Swapnil C, Patel Smita S. RIG-I recognizes metabolite-capped RNAs as signaling ligands. Nucleic Acids Res. (2023) 51:8102–14. doi: 10.1093/nar/gkad518
73. Sokoloski KJ, Haist KC, Morrison TE, Mukhopadhyay S, Hardy RW. Noncapped alphavirus genomic RNAs and their role during infection. J Virol. (2015) 89:6080–92. doi: 10.1128/jvi.00553-15
74. Weber M, Gawanbacht A, Habjan M, Rang A, Borner C, Schmidt Anna M, et al. Incoming RNA virus nucleocapsids containing a 5'-triphosphorylated genome activate RIG-I and antiviral signaling. Cell Host Microbe. (2013) 13:336–46. doi: 10.1016/j.chom.2013.01.012
75. Shao R, Visser I, Fros JJ, Yin X. Versatility of the zinc-finger antiviral protein (ZAP) as a modulator of viral infections. Int J Biol Sci. (2024) 20:4585–600. doi: 10.7150/ijbs.98029
76. Hayakawa S, Shiratori S, Yamato H, Kameyama T, Kitatsuji C, Kashigi F, et al. ZAPS is a potent stimulator of signaling mediated by the RNA helicase RIG-I during antiviral responses. Nat Immunol. (2011) 12:37–44. doi: 10.1038/ni.1963
77. Ventoso I, Berlanga JJ, Toribio R, Díaz-López I. Translational control of alphavirus-host interactions: implications in viral evolution, tropism and antiviral response. Viruses. (2024) 16:205. doi: 10.3390/v16020205
78. Mayo CB, Wong CJ, Lopez PE, Lary JW, Cole JL. Activation of PKR by short stem-loop RNAs containing single-stranded arms. RNA. (2016) 22:1065–75. doi: 10.1261/rna.053348.115
79. Ortega Granda O, Valle C, Shannon A, Decroly E, Canard B, Coutard B, et al. Structure and sequence requirements for RNA capping at the Venezuelan equine encephalitis virus RNA 5′ End. J Virol. (2021) 95:e00777–21. doi: 10.1128/jvi.00777-21
80. Lee E, Bujalowski PJ, Teramoto T, Gottipati K, Scott SD, Padmanabhan R, et al. Structures of flavivirus RNA promoters suggest two binding modes with NS5 polymerase. Nat Commun. (2021) 12:2530. doi: 10.1038/s41467-021-22846-1
81. Slonchak A, Parry R, Pullinger B, Sng JDJ, Wang X, Buck TF, et al. Structural analysis of 3’UTRs in insect flaviviruses reveals novel determinants of sfRNA biogenesis and provides new insights into flavivirus evolution. Nat Commun. (2022) 13:1279. doi: 10.1038/s41467-022-28977-3
82. Pallarés Horacio M, González López Ledesma María M, Oviedo-Rouco S, Castellano Luciana A, Costa Navarro Guadalupe S, Fernández-Alvarez-Ana J, et al. Zika virus non-coding RNAs antagonize antiviral responses by PKR-mediated translational arrest. Nucleic Acids Res. (2024) 52:11128–47. doi: 10.1093/nar/gkae507
83. Boerneke MA, Gokhale NS, Horner SM, Weeks KM. Structure-first identification of RNA elements that regulate dengue virus genome architecture and replication. Proc Natl Acad Sci. (2023) 120:e2217053120. doi: 10.1073/pnas.2217053120
84. Chukwurah E, Farabaugh KT, Guan B-J, Ramakrishnan P, Hatzoglou M. A tale of two proteins: PACT and PKR and their roles in inflammation. FEBS J. (2021) 288:6365–91. doi: 10.1111/febs.15691
85. Lu B, Nakamura T, Inouye K, Li J, Tang Y, Lundbäck P, et al. Novel role of PKR in inflammasome activation and HMGB1 release. Nature. (2012) 488:670–4. doi: 10.1038/nature11290
86. Eisenbarth SC, Colegio OR, O’Connor W, Sutterwala FS, Flavell RA. Crucial role for the Nalp3 inflammasome in the immunostimulatory properties of aluminium adjuvants. Nature. (2008) 453:1122–6. doi: 10.1038/nature06939
87. Schulz O, Pichlmair A, Rehwinkel J, Rogers NC, Scheuner D, Kato H, et al. Protein kinase R contributes to immunity against specific viruses by regulating interferon mRNA integrity. Cell Host Microbe. (2010) 7:354–61. doi: 10.1016/j.chom.2010.04.007
88. Bérouti M, Lammens K, Heiss M, Hansbauer L, Bauernfried S, Stöckl J, et al. Lysosomal endonuclease RNase T2 and PLD exonucleases cooperatively generate RNA ligands for TLR7 activation. Immunity. (2024) 57:1482–96.e8. doi: 10.1016/j.immuni.2024.04.010
89. Facciolà A, Visalli G, Laganà A, Di Pietro A. An overview of vaccine adjuvants: current evidence and future perspectives. Vaccines. (2022) 10:819. doi: 10.3390/vaccines10050819
90. Mohsen MO, Augusto G, Bachmann MF. The 3Ds in virus-like particle based-vaccines: “Design, Delivery and Dynamics. Immunol Rev. (2020) 296:155–68. doi: 10.1111/imr.12863
91. Petrovsky N. Clinical development of SpikoGen(R), an Advax-CpG55.2 adjuvanted recombinant spike protein vaccine. Hum Vaccin Immunother. (2024) 20:2363016. doi: 10.1080/21645515.2024.2363016
92. Gupta R, Arora K, Roy SS, Joseph A, Rastogi R, Arora NM, et al. Platforms, advances, and technical challenges in virus-like particles-based vaccines. Front Immunol. (2023) 14:1123805. doi: 10.3389/fimmu.2023.1123805
93. Laxminarayan R, Gleason A, Sheen J, Saad-Roy CM, Metcalf CJ, Palmer GH, et al. Unlock the potential of vaccines in food-producing animals. Science. (2024) 384:1409–11. doi: 10.1126/science.adj5918
94. Bennett SR, McCarty JM, Ramanathan R, Mendy J, Richardson JS, Smith J, et al. Safety and immunogenicity of PXVX0317, an aluminium hydroxide-adjuvanted chikungunya virus-like particle vaccine: a randomised, double-blind, parallel-group, phase 2 trial. Lancet Infect Dis. (2022) 22:1343–55. doi: 10.1016/S1473-3099(22)00226-2
95. Smit MJ, Sander AF, Ariaans MBPA, Fougeroux C, Heinzel C, Fendel R, et al. First-in-human use of a modular capsid virus-like vaccine platform: an open-label, non-randomised, phase 1 clinical trial of the SARS-CoV-2 vaccine ABNCoV2. Lancet Microbe. (2023) 4:e140–e8. doi: 10.1016/S2666-5247(22)00337-8
96. Brai A, Poggialini F, Pasqualini C, Trivisani CI, Vagaggini C, Dreassi E. Progress towards adjuvant development: focus on antiviral therapy. Int J Mol Sci. (2023) 24:9225. doi: 10.3390/ijms24119225
97. Crisci E, Bárcena J, Montoya M. Virus-like particle-based vaccines for animal viral infections. Inmunologia. (2013) 32:102–16. doi: 10.1016/j.inmuno.2012.08.002
98. Kauffmann F, Van Damme P, Leroux-Roels G, Vandermeulen C, Berthels N, Beuneu C, et al. Clinical trials with GMO-containing vaccines in Europe: Status and regulatory framework. Vaccine. (2019) 37:6144–53. doi: 10.1016/j.vaccine.2019.08.018
99. Eckerstorfer MF, Dolezel M, Miklau M, Greiter A, Heissenberger A, Engelhard M. Scanning the horizon for environmental applications of genetically modified viruses reveals challenges for their environmental risk assessment. Int J Mol Sci. (2024) 25:1507. doi: 10.3390/ijms25031507
100. Fan Y-C, Chiu H-C, Chen L-K, Chang G-JJ, Chiou S-S. Formalin inactivation of Japanese encephalitis virus vaccine alters the antigenicity and immunogenicity of a neutralization epitope in envelope protein domain III. PloS Negl Trop Dis. (2015) 9:e0004167. doi: 10.1371/journal.pntd.0004167
101. Nguyen W, Nakayama E, Yan K, Tang B, Le TT, Liu L, et al. Arthritogenic alphavirus vaccines: serogrouping versus cross-protection in mouse models. Vaccines (Basel). (2020) 8:209. doi: 10.3390/vaccines8020209
102. Shi H, Ross TM. Inactivated recombinant influenza vaccine: the promising direction for the next generation of influenza vaccine. Expert Rev Vaccines. (2024) 23:409–18. doi: 10.1080/14760584.2024.2333338
103. Heßling M, Hönes K, Vatter P, Lingenfelder C. Ultraviolet irradiation doses for coronavirus inactivation - review and analysis of coronavirus photoinactivation studies. GMS Hyg Infect Control. (2020) 15:Doc08. doi: 10.3205/dgkh000343
104. Wijewardana V, Ulbert S, Cattoli G. Irradiation technologies for vaccine development. Front Immunol. (2023) 13:1075335. doi: 10.3389/fimmu.2022.1075335
105. Vrablikova A, Fojtikova M, Hezova R, Simeckova P, Brezani V, Strakova N, et al. UV-C irradiation as an effective tool for sterilization of porcine chimeric VP1-PCV2bCap recombinant vaccine. Sci Rep. (2023) 13:19337. doi: 10.1038/s41598-023-46791-9
106. Vet LJ, Setoh YX, Amarilla AA, Habarugira G, Suen WW, Newton ND, et al. Protective efficacy of a chimeric insect-specific flavivirus vaccine against west Nile virus. Vaccines. (2020) 8:258. doi: 10.3390/vaccines8020258
107. Wang H, Tian J, Zhao J, Zhao Y, Yang H, Zhang G. Current status of poultry recombinant virus vector vaccine development. Vaccines. (2024) 12:630. doi: 10.3390/vaccines12060630
108. Castilho LR, Mattos NR, Abreu WS, Gutarra MLE. Virus-like particles (VLPs) as important tools for flavivirus vaccine development. Biologics. (2022) 2:226–42. doi: 10.3390/biologics2040018
109. Collins MH, Tu HA, Gimblet-Ochieng C, Liou GA, Jadi RS, Metz SW, et al. Human antibody response to Zika targets type-specific quaternary structure epitopes. JCI Insight. (2019) 4:e124588. doi: 10.1172/jci.insight.124588
110. Kudlacek ST, Metz S, Thiono D, Payne AM, Phan TTN, Tian S, et al. Designed, highly expressing, thermostable dengue virus 2 envelope protein dimers elicit quaternary epitope antibodies. Sci Adv. (2021) 7:eabg4084. doi: 10.1126/sciadv.abg4084
111. Ormundo LF, Barreto CT, Tsuruta LR. Development of therapeutic monoclonal antibodies for emerging arbovirus infections. Viruses. (2023) 15:2177. doi: 10.3390/v15112177
112. Hardy JM, Newton ND, Modhiran N, Scott CAP, Venugopal H, Vet LJ, et al. A unified route for flavivirus structures uncovers essential pocket factors conserved across pathogenic viruses. Nat Commun. (2021) 12:3266. doi: 10.1038/s41467-021-22773-1
113. Kuhn RJ, Barrett ADT, Desilva AM, Harris E, Kramer LD, Montgomery RR, et al. A prototype-pathogen approach for the development of flavivirus countermeasures. J Infect Dis. (2023) 228:S398–413. doi: 10.1093/infdis/jiad193
114. European Medicines Agency. Assessment Report: Qdenga. Available online at: https://www.ema.europa.eu/en/documents/assessment-report/qdenga-epar-public-assessment-report_en.pdf (Accessed Oct 2022).
115. Richardson JS, Anderson D, Mendy J, Muhammad S, Tindale L, Loreth T, et al. 2888. Safety and immunogenicity of an adjuvanted chikungunya virus (CHIKV) virus-like particle (VLP) based vaccine in two pivotal phase 3 trials, ≥12 years of age. Open Forum Infect Dis. (2023) 10:ofad500.2471. doi: 10.1093/ofid/ofad500.2471
116. Linn ML, Mateo L, Gardner J, Suhrbier A. Alphavirus-specific cytotoxic T lymphocytes recognize a cross-reactive epitope from the capsid protein and can eliminate virus from persistently infected macrophages. J Virol. (1998) 72:5146–53. doi: 10.1128/jvi.72.6.5146-5153.1998
117. Teo T-H, Lum F-M, Claser C, Lulla V, Lulla A, Merits A, et al. A pathogenic role for CD4+ T cells during chikungunya virus infection in mice. J Immunol. (2013) 190:259–69. doi: 10.4049/jimmunol.1202177
118. Dias CNDS, Gois BM, Lima VS, Guerra-Gomes IC, Araújo JMG, Gomes JDAS, et al. Human CD8 T-cell activation in acute and chronic chikungunya infection. Immunology. (2018) 155:499–504. doi: 10.1111/imm.12992
119. Poo YS, Rudd PA, Gardner J, Wilson JAC, Larcher T, Colle M-A, et al. Multiple immune factors are involved in controlling acute and chronic chikungunya virus infection. PloS Negl Trop Dis. (2014) 8:e3354. doi: 10.1371/journal.pntd.0003354
120. Burrack KS, Montgomery SA, Homann D, Morrison TE. CD8+ T cells control ross river virus infection in musculoskeletal tissues of infected mice. J Immunol. (2015) 194:678–89. doi: 10.4049/jimmunol.1401833
121. Ware BC, Parks MG, da Silva MOL, Morrison TE. Chikungunya virus infection disrupts MHC-I antigen presentation via nonstructural protein 2. PloS Pathog. (2024) 20:e1011794. doi: 10.1371/journal.ppat.1011794
122. Baljon JJ, Wilson JT. Bioinspired vaccines to enhance MHC class-I antigen cross-presentation. Curr Opin Immunol. (2022) 77:102215. doi: 10.1016/j.coi.2022.102215
123. Grubor-Bauk B, Wijesundara D, Masavuli M, Abbink P, Peterson R, Prow N, et al. NS1 DNA vaccination protects against Zika infection through T cell–mediated immunity in immunocompetent mice. Sci Adv. (2019) 5:eaax2388. doi: 10.1126/sciadv.aax2388
124. Bailey MJ, Broecker F, Duehr J, Arumemi F, Krammer F, Palese P, et al. Antibodies elicited by an NS1-based vaccine protect mice against Zika virus. mBio. (2019) 10:e02861–18. doi: 10.1128/mbio.02861-18
125. Modhiran N, Song H, Liu L, Bletchly C, Brillault L, Amarilla AA, et al. A broadly protective antibody that targets the flavivirus NS1 protein. Science. (2021) 371:190–4. doi: 10.1126/science.abb9425
126. Kurup D, Wirblich C, Lambert R, Diba LZ, Leiby BE, Schnell MJ. Measles-based Zika vaccine induces long-term immunity and requires NS1 antibodies to protect the female reproductive tract. NPJ Vaccines. (2022) 7:43. doi: 10.1038/s41541-022-00464-2
127. Guirakhoo F, Domi A, McCurley NP. Methods for generating a Zikv immune response utilizing a recombinant modified vaccina Ankara vector encoding the NS1 protein. (2024). US patent US11638750B2.
128. Cavazzoni CB, Bozza VB, Lucas TC, Conde L, Maia B, Mesin L, et al. The immunodominant antibody response to Zika virus NS1 protein is characterized by cross-reactivity to self. J Exp Med. (2021) 218:e20210580. doi: 10.1084/jem.20210580
129. Filgueiras IS, Torrentes de Carvalho A, Cunha DP, Mathias da Fonseca DL, El Khawanky N, Freire PP, et al. The clinical spectrum and immunopathological mechanisms underlying ZIKV-induced neurological manifestations. PloS Negl Trop Dis. (2021) 15:e0009575. doi: 10.1371/journal.pntd.0009575
130. Trier NH, Houen G. Antibody cross-reactivity in auto-immune diseases. Int J Mol Sci. (2023) 24:13609. doi: 10.3390/ijms241713609
131. Mustelin T, Andrade F. Autoimmunity: the neoantigen hypothesis. Front Immunol. (2024) 15:1432985. doi: 10.3389/fimmu.2024.1432985
132. Perera DR, Ranadeva ND, Sirisena K, Wijesinghe KJ. Roles of NS1 protein in flavivirus pathogenesis. ACS Infect Dis. (2024) 10:20–56. doi: 10.1021/acsinfecdis.3c00566
133. Kim I-J, Gonzalez O, Tighe MP, Lanthier PA, Clark MJ, Travis KL, et al. Protective efficacy of a Zika purified inactivated virus vaccine candidate during pregnancy in marmosets. NPJ Vaccines. (2024) 9:35. doi: 10.1038/s41541-024-00824-0
134. Wieten RW, Jonker EF, van Leeuwen EM, Remmerswaal EB, Ten Berge IJ, de Visser AW, et al. A single 17D yellow fever vaccination provides lifelong immunity; characterization of yellow-fever-specific neutralizing antibody and T-cell responses after vaccination. PloS One. (2016) 11:e0149871. doi: 10.1371/journal.pone.0149871
135. Vashishtha VM, Kumar P. The durability of vaccine-induced protection: an overview. Expert Rev Vaccines. (2024) 23:389–408. doi: 10.1080/14760584.2024.2331065
136. Evans JP, Zeng C, Carlin C, Lozanski G, Saif LJ, Oltz EM, et al. Neutralizing antibody responses elicited by SARS-CoV-2 mRNA vaccination wane over time and are boosted by breakthrough infection. Sci Transl Med. (2022) 14:eabn8057. doi: 10.1126/scitranslmed.abn8057
137. Tehrani ZR, Habibzadeh P, Flinko R, Chen H, Abbasi A, Yared JA, et al. Deficient generation of spike-specific long-lived plasma cells in the bone marrow after severe acute respiratory syndrome coronavirus 2 infection. J Infect Dis. (2024) 230:e30–e3. doi: 10.1093/infdis/jiad603
138. Buitrago-Pabón AL, Ruiz-Sáenz S, Jiménez-Alberto A, Aparicio-Ozores G, Castelán-Vega JA, Ribas-Aparicio RM. An update on Zika virus vaccine development and new research approaches. Microbiol Res. (2024) 15:667–92. doi: 10.3390/microbiolres15020044
139. Hazlewood JE, Tang B, Yan K, Rawle DJ, Harrison JJ, Hall RA, et al. The chimeric Binjari-Zika vaccine provides long-term protection against ZIKA virus challenge. Vaccines (Basel). (2022) 10:85. doi: 10.3390/vaccines10010085
140. Covarrubias CE, Rivera TA, Soto CA, Deeks T, Kalergis AM. Current GMP standards for the production of vaccines and antibodies: An overview. Front Public Health. (2022) 10:1021905. doi: 10.3389/fpubh.2022.1021905
141. Technical standards for manufacturing and quality control of veterinary vaccines. Available online at: https://www.woah.org/en/produit/technical-standards-for-manufacturing-and-quality-control-of-veterinary-vaccines/ (Accessed Oct 2024).
142. Joubert S, Stuible M, Lord-Dufour S, Lamoureux L, Vaillancourt F, Perret S, et al. A CHO stable pool production platform for rapid clinical development of trimeric SARS-CoV-2 spike subunit vaccine antigens. Biotechnol Bioengineering. (2023) 120:1746–61. doi: 10.1002/bit.28387
143. Silva CAT, Kamen AA, Henry O. Intensified influenza virus production in suspension HEK293SF cell cultures operated in fed-batch or perfusion with continuous harvest. Vaccines. (2023) 11:1819. doi: 10.3390/vaccines11121819
144. Pérez Rubio A, Eiros JM. Cell culture-derived flu vaccine: Present and future. Hum Vaccin Immunother. (2018) 14:1874–82. doi: 10.1080/21645515.2018.1460297
145. Pidre ML, Arrías PN, Amorós Morales LC, Romanowski V. The magic staff: A comprehensive overview of baculovirus-based technologies applied to human and animal health. Viruses. (2023) 15:80. doi: 10.3390/v15010080
146. Weger-Lucarelli J, Ruckert C, Grubaugh ND, Misencik MJ, Armstrong PM, Stenglein MD, et al. Adventitious viruses persistently infect three commonly used mosquito cell lines. Virology. (2018) 521:175–80. doi: 10.1016/j.virol.2018.06.007
147. Zhou F, Wang A, Chen L, Wang X, Cui D, Chang H, et al. Isolation and phylogenetic analysis of Getah virus from a commercial modified live vaccine against porcine reproductive and respiratory syndrome virus. Mol Cell Probes. (2020) 53:101650. doi: 10.1016/j.mcp.2020.101650
148. Petricciani J, Sheets R, Griffiths E, Knezevic I. Adventitious agents in viral vaccines: Lessons learned from 4 case studies. Biologicals. (2014) 42:223–36. doi: 10.1016/j.biologicals.2014.07.003
149. Dawurung JS, Harrison JJ, Modhiran N, Hobson-Peters J, Hall RA, de Malmanche H. Serum-free suspension culture of the Aedes albopictus C6/36 cell line for chimeric orthoflavivirus vaccine production. Viruses. (2025) 17:250. doi: 10.3390/v17020250
150. Barbosa RDM, Silva AM, Silva CFD, Cardoso JC, Severino P, Meirelles LMA, et al. Production technologies, regulatory parameters, and quality control of vaccine vectors for veterinary use. Technologies. (2022) 10:109. doi: 10.3390/technologies10050109
151. Yin P, Jian X, Liu Y, Liu Y, Lv L, Cui H, et al. Elucidating cellular interactome of chikungunya virus identifies host dependency factors. Virologica Sin. (2023) 38:497–507. doi: 10.1016/j.virs.2023.05.007
152. Westcott CE, Isom CM, Karki D, Sokoloski KJ. Dancing with the devil: A review of the importance of host RNA-binding proteins to alphaviral RNAs during infection. Viruses. (2023) 15:164. doi: 10.3390/v15010164
153. Ventoso I, Berlanga JJ, Toribio R, Díaz-López I. Translational control of alphavirus–host interactions: implications in viral evolution, tropism and antiviral response. Viruses. (2024) 16:205. doi: 10.3390/v16020205
154. Colón-González FJ, Sewe MO, Tompkins AM, Sjödin H, Casallas A, Rocklöv J, et al. Projecting the risk of mosquito-borne diseases in a warmer and more populated world: a multi-model, multi-scenario intercomparison modelling study. Lancet Planetary Health. (2021) 5:e404–e14. doi: 10.1016/S2542-5196(21)00132-7
155. Vogt MB, Lahon A, Arya RP, Kneubehl AR, Spencer Clinton JL, Paust S, et al. Mosquito saliva alone has profound effects on the human immune system. PloS Negl Trop Dis. (2018) 12:e0006439. doi: 10.1371/journal.pntd.0006439
156. Guerrero D, Vo HTM, Lon C, Bohl JA, Nhik S, Chea S, et al. Evaluation of cutaneous immune response in a controlled human in vivo model of mosquito bites. Nat Commun. (2022) 13:7036. doi: 10.1038/s41467-022-34534-9
157. Spencer Clinton JL, Vogt MB, Kneubehl AR, Hibl BM, Paust S, Rico-Hesse R. Sialokinin in mosquito saliva shifts human immune responses towards intracellular pathogens. PloS Negl Trop Dis. (2023) 17:e0011095. doi: 10.1371/journal.pntd.0011095
158. Lefteri DA, Bryden SR, Pingen M, Terry S, McCafferty A, Beswick EF, et al. Mosquito saliva enhances virus infection through sialokinin-dependent vascular leakage. Proc Natl Acad Sci. (2022) 119:e2114309119. doi: 10.1073/pnas.2114309119
159. Vander Does A, Labib A, Yosipovitch G. Update on mosquito bite reaction: Itch and hypersensitivity, pathophysiology, prevention, and treatment. Front Immunol. (2022) 13:1024559. doi: 10.3389/fimmu.2022.1024559
160. Opasawatchai A, Yolwong W, Thuncharoen W, Inrueangsri N, Itsaradisaikul S, Sasisakulporn C, et al. Novel salivary gland allergens from tropical mosquito species and IgE reactivity in allergic patients. World Allergy Organ J. (2020) 13:100099. doi: 10.1016/j.waojou.2020.100099
161. Prudêncio M. In fairness to mosquitoes. Trends Parasitol. (2020) 36:876–7. doi: 10.1016/j.pt.2020.08.003
162. Marín-López A, Raduwan H, Chen T-Y, Utrilla-Trigo S, Wolfhard DP, Fikrig E. Mosquito salivary proteins and arbovirus infection: from viral enhancers to potential targets for vaccines. Pathogens. (2023) 12:371. doi: 10.3390/pathogens12030371
163. Scott RM, Shelton AL, Eckels KH, Bancroft WH, Summers RJ, Russell PK. Human hypersensitivity to a sham vaccine prepared from mosquito-cell culture fluids. J Allergy Clin Immunol. (1984) 74:808–11. doi: 10.1016/0091-6749(84)90183-0
164. Grohskopf LA, Ferdinands JM, Blanton LH, Broder KR, Loehr J. Prevention and control of seasonal influenza with vaccines: recommendations of the advisory committee on immunization practices - United States, 2024-25 influenza season. MMWR Recomm Rep. (2024) 73:1–25. doi: 10.15585/mmwr.rr7305a1
165. Cançado BLB, Aranda CS, Mallozi MC, Weckx LY, Solé D. Egg allergy and yellow fever vaccination. J Pediatr (Rio J). (2024) 100:60–6. doi: 10.1016/j.jped.2023.07.004
166. Guo J, He X, Tao J, Sun H, Yang J. Unraveling the molecular mechanisms of mosquito salivary proteins: new frontiers in disease transmission and control. Biomolecules. (2025) 15:82. doi: 10.3390/biom15010082
167. Danet L, Beauclair G, Berthet M, Moratorio G, Gracias S, Tangy F, et al. Midgut barriers prevent the replication and dissemination of the yellow fever vaccine in Aedes aEgypti. PloS Negl Trop Dis. (2019) 13:e0007299. doi: 10.1371/journal.pntd.0007299
168. de Miranda RM, Fernandes RS, da-Silva-Fernandes AT, Ferreira-de-Brito A, Moreira SB, Pereira RC, et al. Neotropical sylvatic mosquitoes and aedes aEgypti are not competent to transmit 17DD attenuated yellow fever virus from vaccinated viremic new world non-human primates. Viruses. (2022) 14:2231. doi: 10.3390/v14102231
169. Pedersen CEJ, Robinson DM, Cole FEJ. Isolation of the vaccine strain of Venezuelan equine encephalomyelitis virus from mosquitoes in Louisiana. Am J Epidemiol. (1972) 95:490–6. doi: 10.1093/oxfordjournals.aje.a121416
170. Veronesi E, Paslaru A, Ettlin J, Ravasi D, Flacio E, Tanadini M, et al. Estimating the impact of consecutive blood meals on vector competence of aedes albopictus for chikungunya virus. Pathogens. (2023) 12:849. doi: 10.3390/pathogens12060849
171. Chouin-Carneiro T, David MR, de Bruycker Nogueira F, dos Santos FB, Lourenço-de-Oliveira R. Zika virus transmission by Brazilian Aedes aEgypti and Aedes albopictus is virus dose and temperature-dependent. PloS Negl Trop Dis. (2020) 14:e0008527. doi: 10.1371/journal.pntd.0008527
172. Hugo LE, Prow NA, Tang B, Devine G, Suhrbier A. Chikungunya virus transmission between Aedes albopictus and laboratory mice. Parasit Vectors. (2016) 9:555. doi: 10.1186/s13071-016-1838-1
173. Hick TAH, Geertsema C, Nguyen W, Bishop CR, van Oosten L, Abbo SR, et al. Safety concern of recombination between self-amplifying mRNA vaccines and viruses is mitigated. vivo. Mol Ther. (2024) 32:2519–34. doi: 10.1016/j.ymthe.2024.06.019
174. Kamrud KI, Alterson K, Custer M, Dudek J, Goodman C, Owens G, et al. Development and characterization of promoterless helper RNAs for the production of alphavirus replicon particle. J Gen Virol. (2010) 91:1723–7. doi: 10.1099/vir.0.020081-0
175. Taucher C, Berger A, Mandl CW. A trans-complementing recombination trap demonstrates a low propensity of flaviviruses for intermolecular recombination. J Virol. (2010) 84:599–611. doi: 10.1128/jvi.01063-09
176. Pijlman GP, Suhrbier A, Khromykh AA. Kunjin virus replicons: an RNA-based, non-cytopathic viral vector system for protein production, vaccine and gene therapy applications. Expert Opin Biol Ther. (2006) 6:135–45. doi: 10.1517/14712598.6.2.135
177. Laureti M, Paradkar PN, Fazakerley JK, Rodriguez-Andres J. Superinfection exclusion in mosquitoes and its potential as an arbovirus control strategy. Viruses. (2020) 12:1259. doi: 10.3390/v12111259
178. Gómez M, Martinez D, Muñoz M, Ramírez JD. Aedes aEgypti and Ae. albopictus microbiome/virome: new strategies for controlling arboviral transmission? Parasites Vectors. (2022) 15:287. doi: 10.1186/s13071-022-05401-9
179. Ledesma-Feliciano C, Chapman R, Hooper JW, Elma K, Zehrung D, Brennan MB, et al. Improved DNA vaccine delivery with needle-free injection systems. Vaccines. (2023) 11:280. doi: 10.3390/vaccines11020280
180. Khobragade A, Bhate S, Ramaiah V, Deshpande S, Giri K, Phophle H, et al. Efficacy, safety, and immunogenicity of the DNA SARS-CoV-2 vaccine (ZyCoV-D): the interim efficacy results of a phase 3, randomised, double-blind, placebo-controlled study in India. Lancet. (2022) 399:1313–21. doi: 10.1016/S0140-6736(22)00151-9
181. Lee S, Mattoo SU, Jeong C-G, Kim S-C, Nazki S, Lee G, et al. Intradermal inoculation of inactivated foot-and-mouth disease vaccine induced effective immune responses comparable to conventional intramuscular injection in pigs. Vaccines. (2024) 12:190. doi: 10.3390/vaccines12020190
182. Scarnà T, Menozzi-Arnaud M, Friede M, DeMarco K, Plopper G, Hamer M, et al. Accelerating the development of vaccine microarray patches for epidemic response and equitable immunization coverage requires investment in microarray patch manufacturing facilities. Expert Opin Drug Delivery. (2023) 20:315–22. doi: 10.1080/17425247.2023.2168641
183. Prow TW, Chen X, Prow NA, Fernando GJP, Tan CSE, Raphael AP, et al. Nanopatch-targeted skin vaccination against west nile virus and chikungunya virus in mice. Small. (2010) 6:1776–84. doi: 10.1002/smll.201000331
184. Choo JJY, McMillan CLD, Young PR, Muller DA. Microarray patches: scratching the surface of vaccine delivery. Expert Rev Vaccines. (2023) 22:937–55. doi: 10.1080/14760584.2023.2270598
185. Berger MN, Mowbray ES, Farag MWA, Mathieu E, Davies C, Thomas C, et al. Immunogenicity, safety, usability and acceptability of microarray patches for vaccination: a systematic review and meta-analysis. BMJ Glob Health. (2023) 8:e012247. doi: 10.1136/bmjgh-2023-012247
186. Adigweme I, Yisa M, Ooko M, Akpalu E, Bruce A, Donkor S, et al. A measles and rubella vaccine microneedle patch in The Gambia: a phase 1/2, double-blind, double-dummy, randomised, active-controlled, age de-escalation trial. Lancet. (2024) 403:1879–92. doi: 10.1016/S0140-6736(24)00532-4
187. Hooper JW, Kwilas SA, Josleyn M, Norris S, Hutter JN, Hamer M, et al. Phase 1 clinical trial of Hantaan and Puumala virus DNA vaccines delivered by needle-free injection. NPJ Vaccines. (2024) 9:221. doi: 10.1038/s41541-024-00998-7
188. Baker B, Bermingham IM, Leelasena I, Hickling J, Young PR, Muller DA, et al. Safety, tolerability, and immunogenicity of measles and rubella vaccine delivered with a high-density microarray patch: results from a randomized, partially double-blinded, placebo-controlled phase I clinical trial. Vaccines. (2023) 11:1725. doi: 10.3390/vaccines11111725
189. Baker B, Hacker E, Siller G, Lee M, Mursaliyev N, Forster A. Evaluation of the self-administration potential of high-density microarray patches to human skin: A preliminary study. Hum Vaccines Immunotherapeutics. (2023) 19:2189409. doi: 10.1080/21645515.2023.2189409
190. McMillan CLD, Wijesundara DK, Choo JJY, Amarilla AA, Modhiran N, Fernando GJP, et al. Enhancement of cellular immunity following needle-free vaccination of mice with SARS-CoV-2 spike protein. J Gen Virol. (2024) 105:1947. doi: 10.1099/jgv.0.001947
191. Choo JJ, McMillan CL, Fernando GJ, Hall RA, Young PR, Hobson-Peters J, et al. Developing a stabilizing formulation of a live chimeric dengue virus vaccine dry coated on a high-density microarray patch. Vaccines. (2021) 9:1301. doi: 10.3390/vaccines9111301
192. Essink B, Chu L, Seger W, Barranco E, Le Cam N, Bennett H, et al. The safety and immunogenicity of two Zika virus mRNA vaccine candidates in healthy flavivirus baseline seropositive and seronegative adults: the results of two randomised, placebo-controlled, dose-ranging, phase 1 clinical trials. Lancet Infect Dis. (2023) 23:621–33. doi: 10.1016/S1473-3099(22)00764-2
193. Liu X, Li Z, Li X, Wu W, Jiang H, Zheng Y, et al. A single-dose circular RNA vaccine prevents Zika virus infection without enhancing dengue severity in mice. Nat Commun. (2024) 15:8932. doi: 10.1038/s41467-024-53242-0
194. Cid R, Bolívar J. Platforms for production of protein-based vaccines: from classical to next-generation strategies. Biomolecules. (2021) 11:1072. doi: 10.3390/biom11081072
195. Pardi N, Krammer F. mRNA vaccines for infectious diseases — advances, challenges and opportunities. Nat Rev Drug Discovery. (2024) 23:838–61. doi: 10.1038/s41573-024-01042-y
196. Maine CJ, Miyake-Stoner SJ, Spasova DS, Picarda G, Chou AC, Brand ED, et al. Safety and immunogenicity of an optimized self-replicating RNA platform for low dose or single dose vaccine applications: a randomized, open label Phase I study in healthy volunteers. Nat Commun. (2025) 16:456. doi: 10.1038/s41467-025-55843-9
197. Iqbal SM, Rosen AM, Edwards D, Bolio A, Larson HJ, Servin M, et al. Opportunities and challenges to implementing mRNA-based vaccines and medicines: lessons from COVID-19. Front Public Health. (2024) 12:1429265. doi: 10.3389/fpubh.2024.1429265
198. Hazlewood JE, Rawle DJ, Tang B, Yan K, Vet LJ, Nakayama E, et al. A zika vaccine generated using the chimeric insect-specific binjari virus platform protects against fetal brain infection in pregnant mice. Vaccines (Basel). (2020) 8:496. doi: 10.3390/vaccines8030496
Keywords: vaccine, arbovirus, Eilat virus, Binjari virus, Yada Yada virus, Aripo virus, YN15-283-02 virus, Chaoyang virus
Citation: Hall RA, Nguyen W, Khromykh AA and Suhrbier A (2025) Insect-specific virus platforms for arbovirus vaccine development. Front. Immunol. 16:1521104. doi: 10.3389/fimmu.2025.1521104
Received: 01 November 2024; Accepted: 28 February 2025;
Published: 14 March 2025.
Edited by:
Eva Calvo Pinilla, National Institute for Agricultural and Food Research and Technology, SpainReviewed by:
Rupsa Basu, Humane Genomics, United StatesArley Calle-Tobon, Tulane University, United States
Copyright © 2025 Hall, Nguyen, Khromykh and Suhrbier. This is an open-access article distributed under the terms of the Creative Commons Attribution License (CC BY). The use, distribution or reproduction in other forums is permitted, provided the original author(s) and the copyright owner(s) are credited and that the original publication in this journal is cited, in accordance with accepted academic practice. No use, distribution or reproduction is permitted which does not comply with these terms.
*Correspondence: Roy A. Hall, cm95LmhhbGxAdXEuZWR1LmF1; Andreas Suhrbier, QW5kcmVhcy5TdWhyYmllckBxaW1yYmVyZ2hvZmVyLmVkdS5hdQ==
†These authors share last authorship