- 1Department of Ultrasound, Xichong People’s Hospital, Nanchong, China
- 2Department of Internal Medicine, Guang’an Vocational & Technical College, Guang’an, China
- 3Department of Oncology, Chongqing General Hospital, Chongqing University, Chongqing, China
Pancreatic cancer (PC) is a highly aggressive and lethal malignancy characterized by a complex tumor microenvironment (TME) and immunosuppressive features that limit the efficacy of existing treatments. This paper reviews the potential of combining ultrasound with macrophage exhaustion in the treatment of pancreatic cancer. Macrophages, particularly tumor-associated macrophages (TAMs), are crucial in pancreatic cancer progression and immune escape. Prolonged exposure to the immunosuppressive TME leads to macrophage exhaustion, reducing their anti-tumor ability and instead promoting tumor growth. The CSF1/CSF1R signaling pathway is key in macrophage recruitment and functional regulation, making it an effective target for combating macrophage exhaustion. Ultrasound technology not only plays a significant role in diagnosis and staging but also enhances therapeutic efficacy by guiding radiofrequency ablation (RFA) and percutaneous alcohol injection (PEI) in combination with immunomodulators. Additionally, ultrasound imaging can monitor the number and functional status of TAMs in real-time, providing a basis for optimizing treatment strategies. Future studies should further investigate the combined use of ultrasound and immunomodulators to refine treatment regimens, address challenges such as individual variability and long-term effects, and offer new hope for pancreatic cancer patients.
1 Introduction
Pancreatic cancer is a highly aggressive and metastatic malignant tumor that is difficult to diagnose at an early stage and has an extremely poor prognosis (1–4). In recent years, the incidence and mortality of pancreatic cancer have continued to rise. Statistically, the 5-year survival rate for pancreatic cancer for the period 2013-2019 remains only 13%, one of the lowest among all cancers (4, 5). One of the main reasons for the poor prognosis is the lack of symptoms and inaccurate diagnosis in the early stages. About 90% of cases are diagnosed at an advanced stage, by which time more than 50% of patients have developed systemic metastases (5). The treatment of pancreatic cancer usually involves a multidisciplinary approach that includes chemotherapy, radiation, immunotherapy, and sometimes surgical interventions (3). However, the majority of pancreatic cancer patients who undergo radical resection and systemic chemotherapy still experience recurrence with local or systemic metastases (6). Commonly used chemotherapeutic agents such as gemcitabine and FOLFIRINOX have limited their application due to rapid chemoresistance (7–9). On the other hand, although emerging immunotherapies have demonstrated significant efficacy in a variety of cancers, their efficacy in pancreatic cancer and other quiescent tumors such as breast cancer remains limited (10, 11). Therefore, there is an urgent need to discover new therapies for pancreatic cancer patients, especially combination therapy strategies.
The TME of pancreatic cancer is complex and uniquely characterized, contributing to tumor immune escape and therapeutic failure (1). Among its components, TAMs play a crucial role in determining tumor growth, and therapeutic resistance (12). Prolonged exposure to cancer antigens and inhibitory TME significantly impairs macrophage efficacy, leading to their multifaceted functional exhaustion, including the upregulation of immune checkpoint ligands (13). In particular, the CSF1/CSF1R signaling pathway, which modulates TAM functions and behaviors, is vital in regulating the TME. Therefore, targeting the CSF1/CSF1R signaling pathway may represent a promising anti-tumor strategy in the future.
Ultrasound technology also has significant applications in the diagnosis and treatment of pancreatic cancer. Ultrasound-guided fine-needle aspiration (FNA) and coarse-needle biopsy (CNB) can accurately obtain macrophage samples from the TME, providing essential data for studying the role of TAMs in pancreatic cancer (14–16). Additionally, interventional techniques such as ultrasound-guided radiofrequency ablation (RFA) and percutaneous alcohol injection (PEI) can be combined with immunomodulators for precise delivery of targeted drugs, improving treatment efficacy (17, 18). Ultrasound can also monitor changes in the number and functional status of TAMs in real time during the treatment process, optimizing the treatment strategy (19, 20). Therefore, combining ultrasound with macrophage exhaustion and incorporating the unique properties of the TME may bring new breakthroughs and hope for pancreatic cancer patients.
2 Macrophage exhaustion
2.1 Classification and function of TAMs in TME
Pancreatic cancer has a highly reactive TME enriched with immunosuppressive and inflammatory cells capable of specifically silencing anti-tumor immune responses, allowing tumor cells to evade the effects of traditional monotherapies such as chemotherapy, immunotherapy, etc (1, 21). In addition, the TME of pancreatic cancer specifically contains pancreatic stellate cells (PSCs), cancer-associated fibroblasts (CAFs) as well as a variety of non-cellular components such as extracellular matrix (ECM), cytokines, and growth factors (1, 22). Together, these components contribute to an immunosuppressive TME, which ultimately leads to metastasis of tumor cells (Figure 1A).
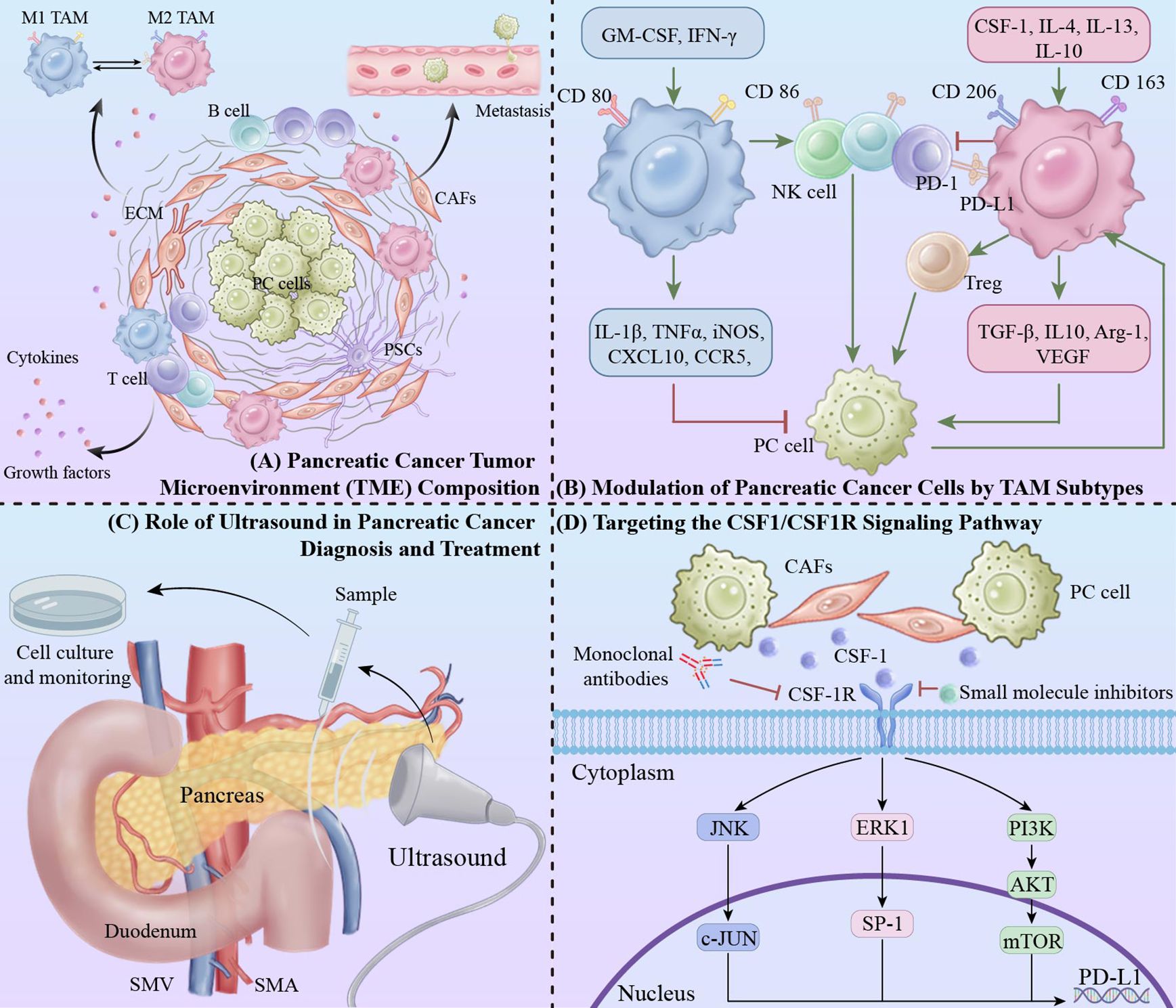
Figure 1. Integrative approaches in pancreatic cancer: targeting tumor microenvironment and macrophage modulation via ultrasound and CSF1/CSF1R pathway inhibition. (A) Pancreatic cancer TME composition. (B) Modulation of pancreatic cancer cells by TAM subtypes: The red “T” symbol indicates inhibitory effects, while the green arrow represents promotive actions within the signaling pathways. (C) Role of ultrasound in pancreatic cancer diagnosis and treatment. (D) Targeting the CSF1/CSF1R signaling pathway.
Macrophages, as an important component of the innate and adaptive immune system, are widely studied immune cells in TME. The majority of macrophages originate from circulating monocytes. Based on their morphology, phenotype, and function, TAMs can be divided into 2 main classes: “anti-tumor” classically activated M1 TAMs and “pro-tumor” selectively activated M2 TAMs (Figure 1B) (12, 13). In the typical classification, pro-inflammatory macrophages acquire the M1 phenotype through activation of granulocyte-macrophage colony-stimulating factor (GM-CSF), lipopolysaccharide, and interferon gamma (IFN-γ), and anti-inflammatory macrophages acquire the M2 phenotype through induction of Colony-stimulating factor 1 (CSF-1), interleukin 4 (IL-4), IL-13, and IL-10 (23, 24). In the M2 TAM, there are characteristic biomarkers, including CDC, CDSF, and IL-10, that are associated with the M2 phenotype. characteristic biomarkers, including CD206 and CD163, among others. Biomarkers of M1 TAM polarization include CD80 and CD86. These molecules form the basis for the identification of TAMs (25).
2.2 Impact of macrophage exhaustion on pancreatic cancer development
Immune cell depletion is a dysfunctional state characterized by the fact that immune cells that play a key role in the antitumor immune response, such as T cells, natural killer (NK) cells, B cells, and macrophages together, exhibit reduced effector function. This depletion is not only a depletion of cell populations, but also manifests itself in altered expression of inhibitory receptors (e.g., PD-1, CTLA-4), metabolic dysregulation, and transcriptional changes leading to reduced cytokine production and proliferative capacity. TME plays a crucial role in this process. More research findings have been found for immune depletion targeting T cells, but it is still to be explored for the other immune cell depletion. Targeted macrophage exhaustion will perhaps be critical for the development of next-generation immunotherapies (26–28).
Prolonged exposure to immunosuppressive TME (characterized by low oxygen levels, high lactate levels, inflammation, and oxidative stress) significantly impairs the anti-inflammatory efficacy of macrophages, leading to macrophage exhaustion (29, 30). At this point, macrophages tend to adopt an M2 phenotype characterized by the secretion of immunosuppressive cytokines, such as IL-10, TGF-β, and other cytokines, in order to promote angiogenesis generation, lymphangiogenesis, immunosuppression, and tumor progression (13). macrophage exhaustion is also characterized by increased recruitment of M2 macrophages and upregulation of immune checkpoint ligands (e.g., PD-1L). Together, these changes promote immunosuppressive TME formation in pancreatic cancer patients, further leading to a vicious cycle.
In addition, data suggest that TAMs in immunosuppressive TME can also lead to depletion of other immune cells, particularly NK cells and T lymphocytes. TAMs can not only promote T cell depletion by overexpression of PD-1L, but also block cytotoxicity by expression of TAM-associated molecules, such as collagen structural macrophage receptor (MARCO) and CD163 T cell and natural killer cell activation (31). Deficiency of MARCO was found to significantly inhibit tumor progression and metastasis in a mouse model of pancreatic cancer, and correlation of clinical data showed a strong trend toward poorer survival in patients with high CD163 and MARCO macrophage infiltration (32). Notably, targeting these molecules with antibodies or knocking down these molecules may repolarize the TAM, thereby restoring the ability of T cells and anti-tumor capacity of natural killer cells, as well as down-regulate the activity of regulatory T cells (Treg) (33, 34). Considering that TAM induces immunosuppressive TME, reprogramming TAM to modulate the anti-tumor immune response has been suggested as a novel therapeutic approach for pancreatic cancer treatment.
3 CSF1/CSF1R signaling pathway
CSF-1, derived from fibroblasts, tumor cells, etc., is produced in membrane-bound form, secreted glycoproteins and proteoglycans. Currently, CSF-1R is considered to be the sole receptor for CSF-1. These cells regulate macrophage growth, differentiation and function by secreting CSF1. Colony-stimulating factor receptor (CSF1R), a type I single-transmembrane protein, is ubiquitously expressed in myeloid cells such as monocytes, macrophages, neuroglia, and osteoblasts. CSF1R induces receptor homodimerization by binding to either CSF-1 or IL-34, followed by activation of receptor signaling and activation of extracellular pro-cell-survival kinase cascades, including PI3K, ERK1/2, and JNK (35–38).
3.1 Role of CSF1/CSF1R in TME
The CSF1/CSF1R-mediated signaling pathway is critical for the differentiation and recruitment of the mononuclear phagocyte system, particularly macrophages. In TME, activation of the CSF1/CSF1R signaling pathway plays a critical role by promoting the transformation of macrophages to an immunosuppressive phenotype (i.e., M2-type macrophages) (39). These M2-type macrophages inhibit anti-tumor immune responses and promote tumor growth and metastasis by secreting a variety of immunosuppressive factors (e.g., IL-10, TGF-β) and factors that promote tumor angiogenesis (e.g., VEGF). In addition, the CSF1/CSF1R signaling pathway maintains the immunosuppressive state of the TME by regulating the recruitment and survival of TAMs (35).
CAFs are present in tumors at all stages, are heterogeneous, and their primary function is to synthesize, deposit, and remodel the ECM. However, CAFs also secrete cytokines, chemokines, growth factors, and angiogenic factors. In the TME, CAFs and TAMs can interact via the CSF1-CSF1R axis (40). For example, CSF1 expression was positively correlated with the abundance of CSF1R+ CD163+ macrophages in skin cancer patients, which is consistent with a role for CSF1 in mediating macrophage survival (41). In addition to fibroblasts, tumor cells can also secrete CSF1, suggesting that it may play a pro-tumorigenic role. Consistent with this, in metastatic PDAC, tumor cell-derived CSF1 induces macrophages to produce granulin, a secreted glycoprotein that promotes fibroblast activation and stimulates tumor growth (42).
Since the presence of CSF1R+ macrophages within tumors correlates with poor survival in various tumor types, targeting CSF1/CSF1R signaling pathway transduction that promotes tumor growth is an attractive strategy to eliminate TAMs, reduce M2 macrophage recruitment, or repolarize them (43).
3.2 Relationship between CSF1/CSF1R and macrophage exhaustion
The CSF1/CSF1R signaling pathway plays an important role in macrophage exhaustion, and its activation induces macrophage exhaustion, causing them to lose their anti-tumor function and instead support tumor growth and immune escape (44). Studies have shown that macrophage polarization in the TME is highly dependent on the presence of cytokines originating from the tumor cells, from other stromal cells (e.g., immune cells or fibroblasts), and from the local cytokine environment of the macrophages themselves. M2 TAM is the result of the persistence of growth factors such as CSF1 and cytokines such as IL-4 and IL-10 (45–47). M2 TAM, in addition to its direct tumor growth-promoting ability, suppresses immune effector cell function, thereby contributing to the elimination of tumor cells. This silencing of immune effector cells is achieved through the production of cytokines and enzymes that inhibit effector cells either directly or indirectly through other immune cell types such as intratumoral dendritic cells (DCs), Treg cells, and type 2 helper T cells (48–50).
In addition, over-activation of the CSF1/CSF1R signaling pathway not only leads to increased expression of macrophage surface inhibitory receptors, which further inhibits the function of immune cells, such as macrophages and T-cells, but also induces metabolic changes in macrophages, such as a decrease in oxidative phosphorylation and an increase in glycolysis, which can lead to their functional depletion (51–53).
4 Combination therapeutic strategies targeting macrophage exhaustion
4.1 Therapies targeting macrophage exhaustion
Therapeutic strategies targeting macrophage exhaustion focus on improving the suppressive TME and enhancing anti-tumor immune responses by inhibiting macrophage recruitment, reprogramming TAMs, and directly depleting TAMs. These strategies have shown promising potential in preclinical studies and are expected to play an important role in future cancer therapy.
The CSF1/CSF1R signaling pathway plays a key role in the recruitment and maintenance of TAMs. By inhibiting this signaling pathway, the recruitment of macrophages to the TME can be effectively reduced, decreasing their number and immunosuppressive function. CSF1R inhibitors such as Pexidartinib (PLX3397) have shown promising anti-tumor effects in preclinical and clinical studies (54).
TAMs in the TME usually behave as M2-type macrophages, which have immunosuppressive and tumor growth-promoting functions. Reprogramming TAMs to reverse-polarize them to M1-type macrophages can enhance their anti-tumor functions. Methods include the use of TLR agonists, IFN-γ and CSF1R inhibitors, etc. (55–59), which promote the production of pro-inflammatory cytokines and anti-tumor factors by altering the polarization state of macrophages to enhance the overall anti-tumor immune response.
Depletion of TAMs is another effective therapeutic strategy that can significantly improve the anti-tumor immune environment by directly targeting and removing these immunosuppressive cells. Targeted drugs commonly used today, such as anti-CSF1R antibodies, can induce apoptosis or functional inhibition of TAMs by blocking the CSF1/CSF1R signaling pathway (60). Cytotoxic drugs, such as Clodronate Liposomes, also deplete TAMs by inducing apoptosis (61).
4.2 Interventions targeting the CSF1/CSF1R signaling pathway
Interventions targeting the CSF1/CSF1R signaling pathway reduce the number and function of macrophages, improve the immunosuppressive state in the TME, and reverse immune depletion through multiple mechanisms (Table 1) (Figure 1C). In combination with other immunotherapies, it is expected to further improve the therapeutic efficacy and provide a new treatment strategy for pancreatic cancer patients (43, 62, 63).
CSF1R inhibitors inhibit the activation of downstream signaling pathways by blocking the binding of CSF1 to CSF1R, thereby reducing macrophage survival, proliferation and recruitment to the TME. Currently, several CSF1R inhibitors have shown promising anti-tumor effects in clinical trials. For example, Pexidartinib (PLX3397) significantly reduced the number of TAMs and enhanced T-cell-mediated anti-tumor immune responses in multiple tumor models (82).
Monoclonal antibody targeting CSF1R is also an effective strategy.CSF1R antibodies kill CSF1R-expressing macrophages by blocking CSF1/CSF1R signaling, inducing receptor internalization and degradation, and by antibody-dependent cell-mediated cellular cytotoxicity (ADCC) effects. For example, Emactuzumab, a monoclonal antibody targeting the CSF1R, has demonstrated potential therapeutic efficacy in a variety of solid tumors (76, 77, 83). Bispecific antibodies are an emerging strategy for targeting both CSF1Rs and other tumor-associated antigens to enhance anti-tumor effects. Several studies are developing bispecific antibodies targeting CSF1R and PD-L1 to enhance therapeutic efficacy by simultaneously inhibiting immunosuppressive signaling and activating immune effects.
Small molecule inhibitors are also effective tools for targeting the CSF1R signaling pathway. These inhibitors block downstream signaling by competing with the ATP-binding site of CSF1R and inhibiting its kinase activity. For example, Sotuletinib(BLZ945), a potent small molecule inhibitor of CSF1R, has shown the ability to enhance immune responses in a variety of cancer models.
Combining inhibitors of the CSF1/CSF1R signaling pathway with other immunotherapies may further enhance therapeutic effects (84, 85). For example, in patients with advanced pancreatic cancer, CSF1R inhibitors in combination with PD-1/PD-L1 inhibitors may simultaneously deregulate immune checkpoint inhibition and reduce the number of immunosuppressive macrophages, thereby enhancing the anti-tumor response of T cells (86). In addition, CSF1R inhibitors may also be able to be used in combination with chemotherapy, radiotherapy, or other targeted therapies to enhance the tumor cell killing effect.
4.3 Combined ultrasound and macrophage exhaustion in an integrated treatment program
At the diagnostic and staging level, FNA and CNB are precise methods used for the diagnosis and staging of pancreatic cancer. These techniques accurately localize the tumor and the associated microenvironment through real-time imaging, obtaining cell and tissue samples from the tumor and its surrounding tissues (15, 16). These samples can be further used to analyze the status of macrophages in the TME, to understand their depletion and immunosuppressive properties, and to provide a basis for subsequent treatment.
On the other hand, at the therapeutic level targeting the TME, RFA and PEI performed under ultrasound guidance can effectively ablate tumor tissues while reducing immunosuppressive macrophages in the TME (17, 18). RFA ablates the tumor cells by thermal effects, while PEI by injecting ethanol causes cell dehydration and necrosis. Used in combination with immunomodulators, such as CSF1R inhibitors and anti-PD-1 antibodies, the macrophage exhaustion state can be further suppressed and the anti-tumor immune response enhanced.
In addition, ultrasonography can dynamically assess tumor blood flow and tissue properties, indirectly reflecting macrophage activity (19). Specifically, ultrasonography combined with nanotechnology for real-time in situ imaging of macrophages can directly and dynamically monitor changes in the number of TAMs in the TME (20). Through ultrasound monitoring, treatment plans can be promptly adjusted to effectively alleviate macrophage exhaustion, thereby enhancing overall treatment efficacy (Figure 1D).
5 Discussion
Although the therapeutic strategy of ultrasound combined with macrophage exhaustion has demonstrated significant potential in preclinical and clinical studies, some limitations remain, such as individual variability of treatment and unclear long-term effects on the TME in pancreatic cancer (87–89). Exploring different immunomodulator combinations with ultrasound is crucial to overcome patient variability. Optimizing dosage, managing side effects, and creating standardized protocols are essential for long-term treatment success (21).
Applying ultrasound combined with macrophage exhaustion in clinical settings presents challenges, such as determining optimal dosage, managing side effects, and establishing standardized protocols. Overcoming these challenges is crucial for clinical translation and improving treatment efficacy for pancreatic cancer patients. Evidence from other clinical applications, like ultrasound-guided hormone injections, shows enhanced treatment outcomes and supports the potential effectiveness of combining ultrasound with immunomodulatory therapies.
Macrophage exhaustion plays a critical role in tumor progression and immune suppression. Targeting and reversing macrophage exhaustion with ultrasound therapy can improve the TME and enhance anti-tumor immune responses (90). Future research should focus on increasing the specificity and efficacy of this strategy to address the complexity of pancreatic cancer. Overall, optimizing these protocols will improve patient outcomes and survival rates (91–93).
Author contributions
QW: Writing – original draft, Writing – review & editing. JW: Writing – original draft, Writing – review & editing. KX: Writing – original draft, Writing – review & editing. ZL: Writing – original draft, Writing – review & editing.
Funding
The author(s) declare that no financial support was received for the research, authorship, and/or publication of this article.
Conflict of interest
The authors declare that the research was conducted in the absence of any commercial or financial relationships that could be construed as a potential conflict of interest.
Publisher’s note
All claims expressed in this article are solely those of the authors and do not necessarily represent those of their affiliated organizations, or those of the publisher, the editors and the reviewers. Any product that may be evaluated in this article, or claim that may be made by its manufacturer, is not guaranteed or endorsed by the publisher.
References
1. Hessmann E, Buchholz SM, Demir IE, Singh SK, Gress TM, Ellenrieder V, et al. Microenvironmental determinants of pancreatic cancer. Physiol Rev. (2020) 100:1707–51. doi: 10.1152/physrev.00042.2019
2. Del Chiaro M, Sugawara T, Karam SD, Messersmith WA. Advances in the management of pancreatic cancer. BMJ (Clinical Res ed.). (2023) 383:e073995. doi: 10.1136/bmj-2022-073995
3. Hu ZI, O'Reilly EM. Therapeutic developments in pancreatic cancer. Nat Rev Gastroenterol Hepatol. (2024) 21:7–24. doi: 10.1038/s41575-023-00840-w
4. Chi H, Su L, Yan Y, Gu X, Su K, Li H, et al. Illuminating the immunological landscape: mitochondrial gene defects in pancreatic cancer through a multiomics lens. Front Immunol. (2024) 15:1375143. doi: 10.3389/fimmu.2024.1375143
5. Siegel RL, Giaquinto AN, Jemal A. Cancer statistics, 2024. CA: Cancer J Clin. (2024) 74:12–49. doi: 10.3322/caac.21820
6. Zang L, Zhang B, Zhou Y, Zhang F, Tian X, Tian Z, et al. Machine learning algorithm integrates bulk and single-cell transcriptome sequencing to reveal immune-related personalized therapy prediction features for pancreatic cancer. Aging (Albany NY). (2023) 15:14109–40. doi: 10.18632/aging.205293
7. O'Reilly EM, Ko AH, Friedberg JW. Flashback foreword: gemcitabine for advanced pancreatic cancer. J Clin oncology: Off J Am Soc Clin Oncol. (2023) 41:5479–80. doi: 10.1200/JCO.23.01895
8. Conroy T, Hammel P, Hebbar M, Ben Abdelghani M, Wei AC, Raoul J-L, et al. FOLFIRINOX or gemcitabine as adjuvant therapy for pancreatic cancer. N Engl J Med. (2018) 379:2395–406. doi: 10.1056/NEJMoa1809775
9. Labori KJ, Bratlie SO, Andersson B, Angelsen JH, Biörserud C, Björnsson B, et al. Neoadjuvant FOLFIRINOX versus upfront surgery for resectable pancreatic head cancer (NORPACT-1): a multicentre, randomised, phase 2 trial. Lancet Gastroenterol Hepatol. (2024) 9:205–17. doi: 10.1016/S2468-1253(23)00405-3
10. Bear AS, Vonderheide RH, O'Hara MH. Challenges and opportunities for pancreatic cancer immunotherapy. Cancer Cell. (2020) 38:788–802. doi: 10.1016/j.ccell.2020.08.004
11. Hilmi M, Delaye M, Muzzolini M, Nicolle R, Cros J, Hammel P, et al. The immunological landscape in pancreatic ductal adenocarcinoma and overcoming resistance to immunotherapy. Lancet Gastroenterol Hepatol. (2023) 8:1129–42. doi: 10.1016/S2468-1253(23)00207-8
12. Kloosterman DJ, Akkari L. Macrophages at the interface of the co-evolving cancer ecosystem. Cell. (2023) 186:1627–51. doi: 10.1016/j.cell.2023.02.020
13. Locati M, Curtale G, Mantovani A. Diversity, mechanisms, and significance of macrophage plasticity. Annu Rev Pathol. (2020) 15:123–47. doi: 10.1146/annurev-pathmechdis-012418-012718
14. Kitano M, Minaga K, Hatamaru K, Ashida R. Clinical dilemma of endoscopic ultrasound-guided fine needle aspiration for resectable pancreatic body and tail cancer. Digestive endoscopy: Off J Japan Gastroenterological Endoscopy Soc. (2022) 34:307–16. doi: 10.1111/den.14120
15. Moutinho-Ribeiro P, Iglesias-Garcia J, Gaspar R, Macedo G. Early pancreatic cancer - The role of endoscopic ultrasound with or without tissue acquisition in diagnosis and staging. Digestive liver disease: Off J Ital Soc Gastroenterol Ital Assoc Study Liver. (2019) 51:4–9. doi: 10.1016/j.dld.2018.09.027
16. King D, Kamran U, Dosanjh A, Coupland B, Mytton J, Leeds JS, et al. Rate of pancreatic cancer following a negative endoscopic ultrasound and associated factors. Endoscopy. (2022) 54:1053–61. doi: 10.1055/a-1784-1661
17. Crinò SF, Napoleon B, Facciorusso A, Lakhtakia S, Borbath I, Caillol F, et al. Endoscopic ultrasound-guided radiofrequency ablation versus surgical resection for treatment of pancreatic insulinoma. Clin Gastroenterol hepatology: Off Clin Pract J Am Gastroenterological Assoc. (2023) 21:2834–43. doi: 10.1016/j.cgh.2023.02.022
18. Crinò SF, Partelli S, Napoleon B, Bellocchi MCC, Facciorusso A, Salvia R, et al. Study protocol for a multicenter randomized controlled trial to compare radiofrequency ablation with surgical resection for treatment of pancreatic insulinoma. Digestive liver disease: Off J Ital Soc Gastroenterol Ital Assoc Study Liver. (2023) 55:1187–93. doi: 10.1016/j.dld.2023.06.021
19. Buxbaum J, Ko C, Varghese N, Lee A, Sahakian A, King K, et al. Qualitative and quantitative contrast-enhanced endoscopic ultrasound improves evaluation of focal pancreatic lesions. Clin Gastroenterol hepatology: Off Clin Pract J Am Gastroenterological Assoc. (2020) 18:917–25.e4. doi: 10.1016/j.cgh.2019.08.054
20. Kim I, Elliott JC, Lawanprasert A, Wood GM, Simon JC, Medina SH. Real-time, in situ imaging of macrophages via phase-change peptide nanoemulsions. Small (Weinheim an der Bergstrasse Germany). (2023) 19:e2301673. doi: 10.1002/smll.202301673
21. Ho WJ, Jaffee EM, Zheng L. The tumour microenvironment in pancreatic cancer - clinical challenges and opportunities. Nat Rev Clin Oncol. (2020) 17:527–40. doi: 10.1038/s41571-020-0363-5
22. Huber M, Brehm CU, Gress TM, Buchholz M, Alashkar Alhamwe B, von Strandmann EP, et al. The immune microenvironment in pancreatic cancer. Int J Mol Sci. (2020) 21:7307. doi: 10.3390/ijms21197307
23. Murray PJ, Wynn TA. Protective and pathogenic functions of macrophage subsets. Nat Rev Immunol. (2011) 11:723–37. doi: 10.1038/nri3073
24. Lundahl MLE, Mitermite M, Ryan DG, Case S, Williams NC, Yang M, et al. Macrophage innate training induced by IL-4 and IL-13 activation enhances OXPHOS driven anti-mycobacterial responses. Elife. (2022) 11:e74690. doi: 10.7554/eLife.74690.sa2
25. Yenyuwadee S, Aliazis K, Wang Q, Christofides A, Shah R, Patsoukis N, et al. Immune cellular components and signaling pathways in the tumor microenvironment. Semin Cancer Biol. (2022) 86:187–201. doi: 10.1016/j.semcancer.2022.08.004
26. Zhang H, Liu L, Liu J, Dang P, Hu S, Yuan W, et al. Roles of tumor-associated macrophages in anti-PD-1/PD-L1 immunotherapy for solid cancers. Mol Cancer. (2023) 22:58. doi: 10.1186/s12943-023-01725-x
27. Chow A, Perica K, Klebanoff CA, Wolchok JD. Clinical implications of T cell exhaustion for cancer immunotherapy. Nat Rev Clin Oncol. (2022) 19:775–90. doi: 10.1038/s41571-022-00689-z
28. Baessler A, Vignali DAA. T cell exhaustion. Annu Rev Immunol. (2024) 42:179–206. doi: 10.1146/annurev-immunol-090222-110914
29. Colegio OR, Chu N-Q, Szabo AL, Chu T, Rhebergen AM, Jairam V, et al. Functional polarization of tumour-associated macrophages by tumour-derived lactic acid. Nature. (2014) 513:559–63. doi: 10.1038/nature13490
30. Ippolito L, Morandi A, Giannoni E, Chiarugi P. Lactate: A metabolic driver in the tumour landscape. Trends Biochem Sci. (2019) 44:153–66. doi: 10.1016/j.tibs.2018.10.011
31. Jeremiasen M, Borg D, Hedner C, Svensson M, Nodin B, Leandersson K, et al. Tumor-associated CD68(+), CD163(+), and MARCO(+) macrophages as prognostic biomarkers in patients with treatment-naïve gastroesophageal adenocarcinoma. Front Oncol. (2020) 10:534761. doi: 10.3389/fonc.2020.534761
32. Shi B, Chu J, Huang T, Wang X, Li Q, Gao Q, et al. The scavenger receptor MARCO expressed by tumor-associated macrophages are highly associated with poor pancreatic cancer prognosis. Front Oncol. (2021) 11:771488. doi: 10.3389/fonc.2021.771488
33. Eisinger S, Sarhan D, Boura VF, Ibarlucea-Benitez I, Tyystjärvi S, Oliynyk G, et al. Targeting a scavenger receptor on tumor-associated macrophages activates tumor cell killing by natural killer cells. Proc Natl Acad Sci USA. (2020) 117:32005–16. doi: 10.1073/pnas.2015343117
34. Sarhan D, Eisinger S, He F, Bergsland M, Pelicano C, Driescher C, et al. Targeting myeloid suppressive cells revives cytotoxic anti-tumor responses in pancreatic cancer. iScience. (2022) 25:105317. doi: 10.1016/j.isci.2022.105317
35. Wen C-Y, Chen P-H, Hsieh F-R, Chang R, Wu M-Y, Yong S-B, et al. CSF-1R: A promising therapeutic target for various diseases. Pharmacol Res. (2024) 204:107196. doi: 10.1016/j.phrs.2024.107196
36. Jiang J, Bai J, Qin T, Wang Z, Han L. NGF from pancreatic stellate cells induces pancreatic cancer proliferation and invasion by PI3K/AKT/GSK signal pathway. J Cell Mol Med. (2020) 24:5901–10. doi: 10.1111/jcmm.v24.10
37. Hobbs GA, Baker NM, Miermont AM, Thurman RD, Pierobon M, Tran TH, et al. Atypical KRAS(G12R) mutant is impaired in PI3K signaling and macropinocytosis in pancreatic cancer. Cancer Discov. (2020) 10:104–23. doi: 10.1158/2159-8290.CD-19-1006
38. Jiao J, Ruan L, Cheng CS, Wang F, Yang P, Chen Z. Paired protein kinases PRKCI-RIPK2 promote pancreatic cancer growth and metastasis via enhancing NF-κB/JNK/ERK phosphorylation. Mol Med. (2023) 29:47. doi: 10.1186/s10020-023-00648-z
39. Chen BQ, Zhao Y, Zhang Y, Pan YJ, Xia HY, Kankala RK, et al. Immune-regulating camouflaged nanoplatforms: A promising strategy to improve cancer nano-immunotherapy. Bioact Mater. (2023) 21:1–19. doi: 10.1016/j.bioactmat.2022.07.023
40. Timperi E, Romano E. Stromal circuits involving tumor-associated macrophages and cancer-associated fibroblasts. Front Immunol. (2023) 14:1194642. doi: 10.3389/fimmu.2023.1194642
41. Neubert NJ, Schmittnaegel M, Bordry N, Nassiri S, Wald N, Martignier C, et al. T cell-induced CSF1 promotes melanoma resistance to PD1 blockade. Sci Trans Med. (2018) 10:eaan3311. doi: 10.1126/scitranslmed.aan3311
42. Quaranta V, Rainer C, Nielsen SR, Raymant ML, Ahmed MS, Engle DD, et al. Macrophage-derived granulin drives resistance to immune checkpoint inhibition in metastatic pancreatic cancer. Cancer Res. (2018) 78:4253–69. doi: 10.1158/0008-5472.CAN-17-3876
43. Li M, Li M, Yang Y, Liu Y, Xie H, Yu Q, et al. Remodeling tumor immune microenvironment via targeted blockade of PI3K-γ and CSF-1/CSF-1R pathways in tumor associated macrophages for pancreatic cancer therapy. J Controlled release: Off J Controlled Release Soc. (2020) 321:23–35. doi: 10.1016/j.jconrel.2020.02.011
44. Jin X, Zhang J, Zhang Y, He J, Wang M, Hei Y, et al. Different origin-derived exosomes and their clinical advantages in cancer therapy. Front Immunol. (2024) 15:1401852. doi: 10.3389/fimmu.2024.1401852
45. Cannarile MA, Weisser M, Jacob W, Jegg AM, Ries CH, Rüttinger D. Colony-stimulating factor 1 receptor (CSF1R) inhibitors in cancer therapy. J Immunother Cancer. (2017) 5:53. doi: 10.1186/s40425-017-0257-y
46. Ishida K, Nagatake T, Saika A, Kawai S, Node E, Hosomi K, et al. Induction of unique macrophage subset by simultaneous stimulation with LPS and IL-4. Front Immunol. (2023) 14:1111729. doi: 10.3389/fimmu.2023.1111729
47. Chen S, Wang M, Lu T, Liu Y, Hong W, He X, et al. JMJD6 in tumor-associated macrophage regulates macrophage polarization and cancer progression via STAT3/IL-10 axis. Oncogene. (2023) 42:2737–50. doi: 10.1038/s41388-023-02781-9
48. Ruffell B, Chang-Strachan D, Chan V, Rosenbusch A, Ho CMT, Pryer N, et al. Macrophage IL-10 blocks CD8+ T cell-dependent responses to chemotherapy by suppressing IL-12 expression in intratumoral dendritic cells. Cancer Cell. (2014) 26:623–37. doi: 10.1016/j.ccell.2014.09.006
49. Wang N, Wang S, Wang X, Zheng Y, Yang B, Zhang J, et al. Research trends in pharmacological modulation of tumor-associated macrophages. Clin Trans Med. (2021) 11:e288. doi: 10.1002/ctm2.v11.1
50. Zong Y, Deng K, Chong WP. Regulation of Treg cells by cytokine signaling and co-stimulatory molecules. Front Immunol. (2024) 15:1387975. doi: 10.3389/fimmu.2024.1387975
51. Zhang Z, Liu X, Chen D, Yu J. Radiotherapy combined with immunotherapy: the dawn of cancer treatment. Signal Transduct Target Ther. (2022) 7:258. doi: 10.1038/s41392-022-01102-y
52. Rojas LA, Sethna Z, Soares KC, Olcese C, Pang N, Patterson E, et al. Personalized RNA neoantigen vaccines stimulate T cells in pancreatic cancer. Nature. (2023) 618:144–50. doi: 10.1038/s41586-023-06063-y
53. Wang Y, Cho J-W, Kastrunes G, Buck A, Razimbaud C, Culhane AC, et al. Immune-restoring CAR-T cells display antitumor activity and reverse immunosuppressive TME in a humanized ccRCC mouse model. iScience. (2024) 27:108879. doi: 10.1016/j.isci.2024.108879
54. Lamb YN. Correction to: pexidartinib: first approval. Drugs. (2020) 80:447. doi: 10.1007/s40265-020-01280-5
55. Rodell CB, Arlauckas SP, Cuccarese MF, Garris CS, Li R, Ahmed MS, et al. TLR7/8-agonist-loaded nanoparticles promote the polarization of tumour-associated macrophages to enhance cancer immunotherapy. Nat Biomed Eng. (2018) 2:578–88. doi: 10.1038/s41551-018-0236-8
56. Shang T, Yu X, Gu Y, Du R, Cai Y, Li Y, et al. Supermolecular nanovehicles co-delivering TLR7/8-agonist and anti-CD47 siRNA for enhanced tumor immunotherapy. Int J Biol Macromol. (2023) 251:126539. doi: 10.1016/j.ijbiomac.2023.126539
57. Varesio L, Blasi E, Thurman GB, Talmadge JE, Wiltrout RH, Herberman RB. Potent activation of mouse macrophages by recombinant interferon-gamma. Cancer Res. (1984) 44:4465–9.
58. Pyonteck SM, Akkari L, Schuhmacher AJ, Bowman RL, Sevenich L, Quail DF, et al. CSF-1R inhibition alters macrophage polarization and blocks glioma progression. Nat Med. (2013) 19:1264–72. doi: 10.1038/nm.3337
59. Hume DA, MacDonald KPA. Therapeutic applications of macrophage colony-stimulating factor-1 (CSF-1) and antagonists of CSF-1 receptor (CSF-1R) signaling. Blood. (2012) 119:1810–20. doi: 10.1182/blood-2011-09-379214
60. Ries CH, Cannarile MA, Hoves S, Benz J, Wartha K, Runza V, et al. Targeting tumor-associated macrophages with anti-CSF-1R antibody reveals a strategy for cancer therapy. Cancer Cell. (2014) 25:846–59. doi: 10.1016/j.ccr.2014.05.016
61. Maldonado MDM, Schlom J, Hamilton DH. Blockade of tumor-derived colony-stimulating factor 1 (CSF1) promotes an immune-permissive tumor microenvironment. Cancer Immunol Immunother: CII. (2023) 72:3349–62. doi: 10.1007/s00262-023-03496-2
62. Yu M, Wu Y, Li Q, Hong W, Yang Y, Hu X, et al. Colony-stimulating factor-1 receptor inhibition combined with paclitaxel exerts effective antitumor effects in the treatment of ovarian cancer. Genes Dis. (2024) 11:100989. doi: 10.1016/j.gendis.2023.04.023
63. Li H-W, Tang S-L. Colony stimulating factor-1 and its receptor in gastrointestinal Malignant tumors. J Cancer. (2021) 12:7111–9. doi: 10.7150/jca.60379
64. Tap WD, Gelderblom H, Palmerini E, Desai J, Bauer S, Blay J-Y, et al. Pexidartinib versus placebo for advanced tenosynovial giant cell tumour (ENLIVEN): a randomised phase 3 trial. Lancet (London England). (2019) 394:478–87. doi: 10.1016/S0140-6736(19)30764-0
65. Healey JH, Tap WD, Gelhorn HL, Ye X, Speck RM, Palmerini E, et al. Pexidartinib provides modest pain relief in patients with tenosynovial giant cell tumor: results from ENLIVEN. Clin Orthop Relat Res. (2023) 481:107–16. doi: 10.1097/CORR.0000000000002335
66. Thongchot S, Duangkaew S, Yotchai W, Maungsomboon S, Phimolsarnti R, Asavamongkolkul A, et al. Novel CSF1R-positive tenosynovial giant cell tumor cell lines and their pexidartinib (PLX3397) and sotuletinib (BLZ945)-induced apoptosis. Hum Cell. (2023) 36:456–67. doi: 10.1007/s13577-022-00823-0
67. Strachan DC, Ruffell B, Oei Y, Bissell MJ, Coussens LM, Pryer N, et al. CSF1R inhibition delays cervical and mammary tumor growth in murine models by attenuating the turnover of tumor-associated macrophages and enhancing infiltration by CD8+ T cells. Oncoimmunology. (2013) 2:e26968. doi: 10.4161/onci.26968
68. Johnson M, Dudek AZ, Sukari A, Call J, Kunk PR, Lewis K, et al. ARRY-382 in combination with pembrolizumab in patients with advanced solid tumors: results from a phase 1b/2 study. Clin Cancer research: an Off J Am Assoc Cancer Res. (2022) 28:2517–26. doi: 10.1158/1078-0432.CCR-21-3009
69. Mancuso R, Fryatt G, Cleal M, Obst J, Pipi E, Monzón-Sandoval J, et al. CSF1R inhibitor JNJ-40346527 attenuates microglial proliferation and neurodegeneration in P301S mice. Brain: J Neurol. (2019) 142:3243–64. doi: 10.1093/brain/awz241
70. von Tresckow B, Morschhauser F, Ribrag V, Topp MS, Chien C, Seetharam S, et al. Multicenter, phase I/II study of JNJ-40346527, a CSF-1R inhibitor, in patients with relapsed or refractory hodgkin lymphoma. Clin Cancer research: an Off J Am Assoc Cancer Res. (2015) 21:1843–50. doi: 10.1158/1078-0432.CCR-14-1845
71. Smith BD, Kaufman MD, Wise SC, Ahn YM, Caldwell TM, Leary CB, et al. Vimseltinib: A precision CSF1R therapy for tenosynovial giant cell tumors and diseases promoted by macrophages. Mol Cancer Ther. (2021) 20:2098–109. doi: 10.1158/1535-7163.MCT-21-0361
72. Tap WD, Sharma MG, Vallee M, Smith BD, Sherman ML, Ruiz-Soto R, et al. The MOTION study: a randomized, phase III study of vimseltinib for the treatment of tenosynovial giant cell tumor. Future Oncol (London England). (2024) 20:593–601. doi: 10.2217/fon-2023-0238
73. Xu J, Shen L, Zhou Z, Li J, Bai C, Chi Y, et al. Surufatinib in advanced extrapancreatic neuroendocrine tumours (SANET-ep): a randomised, double-blind, placebo-controlled, phase 3 study. Lancet Oncol. (2020) 21:1500–12. doi: 10.1016/S1470-2045(20)30496-4
75. Pan C, Yu T, Han L, Hao D, Yang M, Li L, et al. Surufatinib combined camrelizumab as a valuable third-line rescue therapy for a patient with extensive-stage for small-cell lung cancer: a case report and literature review. Anticancer Drugs. (2024) 35:271–6. doi: 10.1097/CAD.0000000000001552
76. Gomez-Roca CA, Italiano A, Le Tourneau C, Cassier PA, Toulmonde M, D'Angelo SP, et al. Phase I study of emactuzumab single agent or in combination with paclitaxel in patients with advanced/metastatic solid tumors reveals depletion of immunosuppressive M2-like macrophages. Ann oncology: Off J Eur Soc Med Oncol. (2019) 30:1381–92. doi: 10.1093/annonc/mdz163
77. Gomez-Roca C, Cassier P, Zamarin D, Machiels J-P, Perez Gracia JL, Stephen Hodi F, et al. Anti-CSF-1R emactuzumab in combination with anti-PD-L1 atezolizumab in advanced solid tumor patients naïve or experienced for immune checkpoint blockade. J Immunother Cancer. (2022) 10:e004076. doi: 10.1136/jitc-2021-004076
78. Razak AR, Cleary JM, Moreno V, Boyer M, Calvo Aller E, Edenfield W, et al. Safety and efficacy of AMG 820, an anti-colony-stimulating factor 1 receptor antibody, in combination with pembrolizumab in adults with advanced solid tumors. J Immunother Cancer. (2020) 8:e001006. doi: 10.1136/jitc-2020-001006
79. Weiss SA, Djureinovic D, Jessel S, Krykbaeva I, Zhang L, Jilaveanu L, et al. A phase I study of APX005M and cabiralizumab with or without nivolumab in patients with melanoma, kidney cancer, or non-small cell lung cancer resistant to anti-PD-1/PD-L1. Clin Cancer Res. (2021) 27:4757–67. doi: 10.1158/1078-0432.CCR-21-0903
80. Autio KA, Klebanoff CA, Schaer D, Kauh JSW, Slovin SF, Adamow M, et al. Immunomodulatory activity of a colony-stimulating factor-1 receptor inhibitor in patients with advanced refractory breast or prostate cancer: A phase I study. Clin Cancer research: an Off J Am Assoc Cancer Res. (2020) 26:5609–20. doi: 10.1158/1078-0432.CCR-20-0855
81. Kitko CL, Arora M, DeFilipp Z, Zaid MA, Di Stasi A, Radojcic V, et al. Axatilimab for chronic graft-versus-host disease after failure of at least two prior systemic therapies: results of a phase I/II study. J Clin Oncol. (2023) 41:1864–75. doi: 10.1200/JCO.22.00958
82. Fujiwara T, Yakoub MA, Chandler A, Christ AB, Yang G, Ouerfelli O, et al. CSF1/CSF1R signaling inhibitor pexidartinib (PLX3397) reprograms tumor-associated macrophages and stimulates T-cell infiltration in the sarcoma microenvironment. Mol Cancer Ther. (2021) 20:1388–99. doi: 10.1158/1535-7163.MCT-20-0591
83. Machiels J-P, Gomez-Roca C, Michot J-M, Zamarin D, Mitchell T, Catala G, et al. Phase Ib study of anti-CSF-1R antibody emactuzumab in combination with CD40 agonist selicrelumab in advanced solid tumor patients. J Immunother Cancer. (2020) 8:e001153. doi: 10.1136/jitc-2020-001153
84. Shimizu D, Yuge R, Kitadai Y, Ariyoshi M, Miyamoto R, Hiyama Y, et al. Pexidartinib and immune checkpoint inhibitors combine to activate tumor immunity in a murine colorectal cancer model by depleting M2 macrophages differentiated by cancer-associated fibroblasts. Int J Mol Sci. (2024) 25:7001. doi: 10.3390/ijms25137001
85. Li Z, Ding Y, Liu J, Wang J, Mo F, Wang Y, et al. Depletion of tumor associated macrophages enhances local and systemic platelet-mediated anti-PD-1 delivery for post-surgery tumor recurrence treatment. Nat Commun. (2022) 13:1845. doi: 10.1038/s41467-022-29388-0
86. Voissière A, Gomez-Roca C, Chabaud S, Rodriguez C, Nkodia A, Berthet J, et al. The CSF-1R inhibitor pexidartinib affects FLT3-dependent DC differentiation and may antagonize durvalumab effect in patients with advanced cancers. Sci Trans Med. (2024) 16:eadd1834. doi: 10.1126/scitranslmed.add1834
87. Shao C, Zhang J, Guo J, Zhang L, Zhang Y, Ma L, et al. A radiomics nomogram model for predicting prognosis of pancreatic ductal adenocarcinoma after high-intensity focused ultrasound surgery. Int J hyperthermia: Off J Eur Soc Hyperthermic Oncology North Am Hyperthermia Group. (2023) 40:2184397. doi: 10.1080/02656736.2023.2184397
88. Chi H, Peng G, Wang R, Yang F, Xie X, Zhang J, et al. Cuprotosis programmed-cell-death-related lncRNA signature predicts prognosis and immune landscape in PAAD patients. Cells. (2022) 11:3436. doi: 10.3390/cells11213436
89. Huang X, Chi H, Gou S, Guo X, Li L, Peng G, et al. An aggrephagy-related lncRNA signature for the prognosis of pancreatic adenocarcinoma. Genes (Basel). (2023) 14:124. doi: 10.3390/genes14010124
90. Chen YJ, Li GN, Li XJ, Wei LX, Fu MJ, Cheng ZL, et al. Targeting IRG1 reverses the immunosuppressive function of tumor-associated macrophages and enhances cancer immunotherapy. Sci Adv. (2023) 9:eadg0654. doi: 10.1126/sciadv.adg0654
91. Mantovani A, Allavena P, Marchesi F, Garlanda C. Macrophages as tools and targets in cancer therapy. Nat Rev Drug Discov. (2022) 21:799–820. doi: 10.1038/s41573-022-00520-5
92. Chi H, Chen H, Wang R, Zhang J, Jiang L, Zhang S, et al. Proposing new early detection indicators for pancreatic cancer: Combining machine learning and neural networks for serum miRNA-based diagnostic model. Front Oncol. (2023) 13:1244578. doi: 10.3389/fonc.2023.1244578
Keywords: TAMs, immune exhaustion, TME, CSF1/CSF1R, ultrasound, pancreatic cancer
Citation: Wang Q, Wang J, Xu K and Luo Z (2024) Targeting the CSF1/CSF1R signaling pathway: an innovative strategy for ultrasound combined with macrophage exhaustion in pancreatic cancer therapy. Front. Immunol. 15:1481247. doi: 10.3389/fimmu.2024.1481247
Received: 15 August 2024; Accepted: 11 September 2024;
Published: 02 October 2024.
Edited by:
Miguel Pereira-Silva, University of Coimbra, PortugalReviewed by:
Qihang Yuan, Dalian Medical University, ChinaCopyright © 2024 Wang, Wang, Xu and Luo. This is an open-access article distributed under the terms of the Creative Commons Attribution License (CC BY). The use, distribution or reproduction in other forums is permitted, provided the original author(s) and the copyright owner(s) are credited and that the original publication in this journal is cited, in accordance with accepted academic practice. No use, distribution or reproduction is permitted which does not comply with these terms.
*Correspondence: Zhibin Luo, bHVvemhpYmluemxrQDEyNi5jb20=; Ke Xu, Y3FnaHh1a2VAY3F1LmVkdS5jbg==