- 1Department of Respiratory and Critical Care Medicine, The Affiliated Jiangning Hospital of Nanjing Medical University, Nanjing, China
- 2Shanghai East Clinical Medical College, Nanjing Medical University, Nanjing, China
Liquid-liquid phase separation (LLPS) is integral to various biological processes, facilitating signal transduction by creating a condensed, membrane-less environment that plays crucial roles in diverse physiological and pathological processes. Recent evidence has underscored the significance of LLPS in human health and disease. However, its implications in respiratory diseases remain poorly understood. This review explores current insights into the mechanisms and biological roles of LLPS, focusing particularly on its relevance to respiratory diseases, aiming to deepen our understanding and propose a new paradigm for studying phase separation in this context.
1 Introduction
Liquid-liquid phase separation (LLPS) is a fundamental biophysical phenomenon in cellular biology where biomolecules, such as proteins and RNA, spontaneously aggregate into distinct regions within the cytoplasm or nucleus. These regions exhibit unique physical and chemical properties compared to their surroundings (1, 2). Analogous to the separation of oil and water, LLPS results in the formation of two distinct liquid phases. Facilitated by dynamic and reversible interactions among proteins and nucleic acids, LLPS enables the creation of membrane-less intracellular compartments, giving rise to organelles that lack traditional lipid bilayers (Figure 1A) (3, 4). These structures are crucial for organizing cellular components spatially, thereby regulating diverse cellular functions effectively (5).
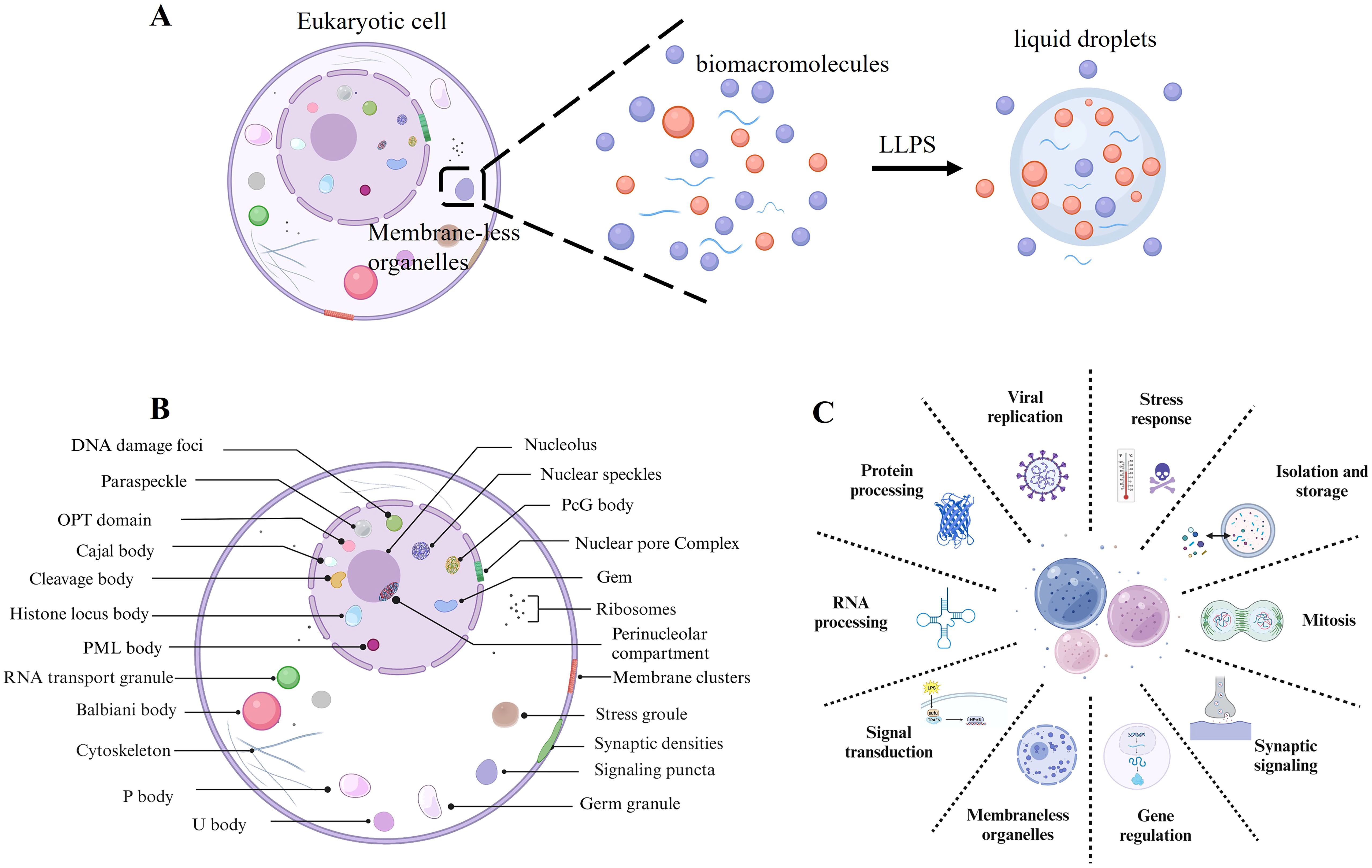
Figure 1. Liquid-liquid phase separation. (A) Biomacromolecules undergo LLPS to form liquid droplets, which contributes to the formation of membrane-less organelles. (B) Examples of membrane-less organelles include those found distributed throughout the nucleus, nuclear membrane, cytoplasm, and plasma membranes of eukaryotic cells. (C) Physiological function of LLPS.
LLPS facilitates the formation of membrane-less organelles such as nucleoli, stress granules (SGs), Cajal bodies, and P-bodies. These structures compartmentalize cellular components without lipid bilayers, thereby establishing specialized biochemical environments (5–7) (Figure 1B). This spatial segregation markedly enhances the efficiency and precision of biochemical reactions within the complex cellular milieu (8–10). Moreover, LLPS is crucial for cellular organization and regulation, affecting a variety of essential cellular processes (Figure 1C). Firstly, LLPS regulates gene expression and RNA processing by forming transcriptional hubs that localize transcription factors and regulatory molecules near specific genes (11, 12). This localization can modulate gene expression, either enhancing or repressing it as needed. In RNA processing and translation, LLPS facilitates the formation and regulation of ribonucleoprotein complexes essential for RNA splicing, transport, stabilization, and translation regulation (13). Similarly, in signal transduction and stress response, LLPS assumes distinctive roles (13). It modulates signal transduction pathways by localizing signaling molecules within phase-separated domains, which enhances or diminishes signal transmission (14). This concentration of signaling molecules enables efficient signal integration and modulation within the cell. During stress conditions, cells utilize LLPS to create SGs that sequester and protect RNA and proteins vital for cellular survival in hostile environments. For instance, in response to heat shock or oxidative stress, cells form SGs via LLPS to shield essential molecular components (15). Furthermore, LLPS is instrumental in protein sequestration and degradation. It aids in creating proteasome-rich compartments for targeted protein degradation and serves as a mechanism for protein storage (16). LLPS has been implicated in controlling cell cycle regulation. Phase-separated compartments concentrate cell cycle regulators, ensuring orderly progression through the cell cycle phases. Beyond these functions, LLPS regulates the spatial and temporal regulation of proteins and RNAs, which are vital for cell differentiation and organ development (17). Lastly, the dynamic nature of LLPS, characterized by the formation and resolution of compartments in response to cellular needs, underscores its essential role in maintaining cellular homeostasis and adapting to environmental changes (18).
Notably, the dysregulation of LLPS is associated with various diseases, such as cancer and neurodegenerative disorders (5, 19–21). Aberrant LLPS in cellular contexts manifests in several forms, including loss, excessive condensation, and abnormal phase transitions, all of which significantly impact cellular organization and function (Figure 2). Beginning with the loss of LLPS, a decrease in this process disrupts the normal formation of membrane-less organelles such as nucleoli, SGs, and P-bodies (22). This deficiency results in the mislocalization of proteins and RNA, undermining essential cellular functions, particularly notable in diseases such as muscular dystrophies and certain cancers, where RNA-binding proteins affected by LLPS loss play a critical role (8, 14, 23). For instance, mutations impairing MeCP2’s LLPS capacity are associated with Rett syndrome, impacting chromosome integrity and transcriptional silence (24–26). Conversely, excessive LLPS leads to the formation of overly stable or large biomolecular condensates (BMCs), trapping essential cellular components and disrupting normal cellular processes. In neurodegenerative diseases such as ALS and frontotemporal dementia, proteins like TDP-43 and FUS undergo excessive LLPS, forming cytotoxic aggregates (27). Similarly, in Ewing sarcoma, the EWS-FLI1 fusion protein utilizes LLPS to form super-enhancers that drive oncogenic gene expression (28–32). Abnormal phase transitions within phase-separated compartments indicate pathological LLPS, transitioning from a dynamic, liquid-like state to a more solid, gel-like consistency (33). These transitions, characterized by irreversible hydrogel formation and involving proteins like FUS and SOD1, are particularly prominent in neurodegenerative disorders such as Alzheimer’s disease (33–37). These changes disrupt cellular functions by impeding molecular mobility, affecting proteostasis, and activating stress responses, thereby highlighting the extensive implications of LLPS dysregulation in disease contexts (37).
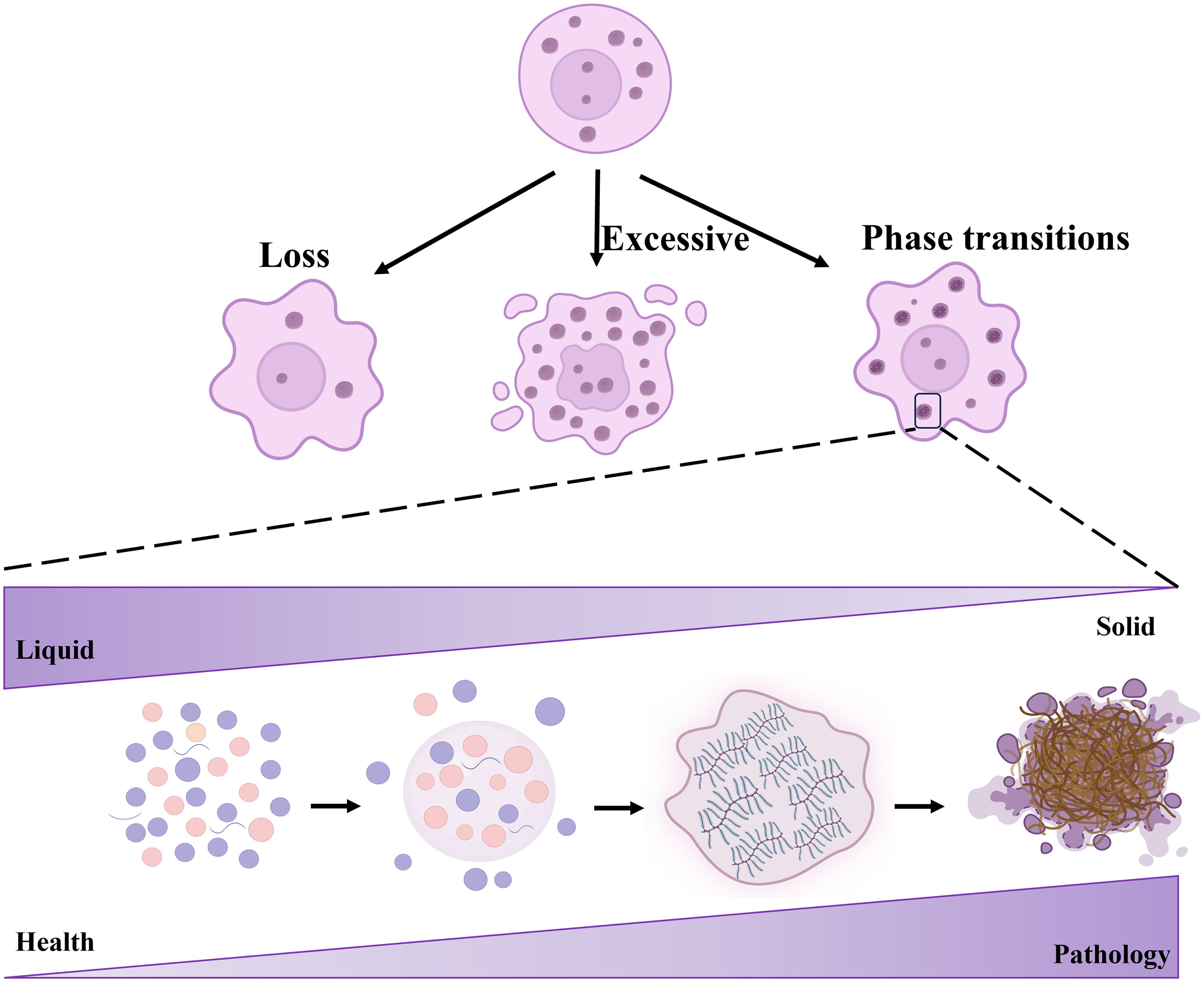
Figure 2. Aberrant LLPS encompasses loss, excessive condensation, and abnormal phase transitions. Biomacromolecules undergo LLPS to form droplets, initially reversible but over time transitioning to more solid-like states such as fibers or hydrogels.
2 Potential roles of LLPS in respiratory diseases
Respiratory diseases cover a wide spectrum of pathologies affecting the airways, lungs, pleura, and related clinical syndromes. Clinical presentations vary widely, ranging from slight symptoms such as cough, chest pain, and mild dyspnea, to severe manifestations including respiratory distress, hypoxia, and potentially fatal respiratory failure (38). These diseases are broadly categorized based on lesion location and characteristics into obstructive, restrictive, pulmonary vascular, infectious lung diseases, and malignancies (38). Factors such as global air pollution, smoking, and aging populations significantly increase the incidence and mortality rates of chronic obstructive pulmonary disease, asthma, lung cancer, interstitial lung fibrosis, and pulmonary infections worldwide (39). Respiratory diseases have emerged as major causes of global morbidity and mortality (40). Despite this, substantial knowledge gaps persist in understanding the mechanisms and treatments of these diseases, highlighting the need for urgent and comprehensive research efforts. LLPS encompasses diverse physiological functions and is associated with a wide range of biological activities. Aberrant LLPS is implicated in multiple diseases, indicating that LLPS-induced aberrant biological activities may contribute to the pathogenesis of respiratory diseases (41). Recent studies have demonstrated that LLPS is involved in various respiratory diseases, including lung cancer, viral or fungal infections, as well as lung injury (42, 43).
2.1 LLPS in lung cancer
Lung cancer, characterized by uncontrolled proliferation and growth of cells, remains the leading cause of cancer-related mortality globally, accounting for approximately 18% of all cancer deaths. The etiology and pathogenesis of lung cancer are complex, involving mutations in oncogenes, inactivation of tumor suppressor proteins, and dysregulation of signaling pathways (44). Recent advancements in elucidating the molecular mechanisms underlying lung cancer have underscored the critical role of LLPS in tumorigenesis (42) (Table 1). Biomolecules undergo phase transitions to form membrane-less organelles or condensates. These condensates influence various cellular processes, and their dysregulation is increasingly recognized as a crucial factor in lung cancer development.
To begin with, lung cancer frequently involves mutations in oncogenic genes that are crucial for both initiating and advancing the disease. Notably, Anaplastic Lymphoma Kinase (ALK) fusions, particularly the EML4-ALK fusion, are integral to the oncogenic landscape of specific non-small cell lung cancer (NSCLC) (56–58). Research by Qin et al. has demonstrated that EML4-ALK variant 1 can form liquid-like condensates in the cytoplasm. The EML4 region of the fusion protein is crucial for this process. The EML4-N fragment alone can induce phase separation, in contrast to the dispersed nature of the ALK-C fragment (45). Mutations in aromatic residues within the EML4 region significantly disrupt phase separation, underscoring the importance of these residues. Similarly, rearranged during transfection (RET) is another important driver gene for NSCLC (59). The Coiled-coil domain containing 6 (CCDC6)-RET fusion gene undergoes LLPS in the cytoplasm independently of ligands or associated proteins (46). Studies of CCDC6-RET LLPS have revealed the simultaneous recruitment of GRB2 and SHC1, thereby establishing a membrane-less signaling microdomain that facilitates Ras/MAPK signaling transduction. Additionally, long non-coding RNAs (lncRNAs) play a crucial role in lung cancer, especially in the formation of macromolecular condensates. The oncogenic lncRNA MELTF-AS1 is upregulated in NSCLC and correlates with advanced TNM stages, larger tumor size, and poorer survival rates (53). MELTF-AS1 directly interacts with YBX1, an RNA-binding protein involved in tumorigenesis, promoting its phase separation and consequently activating ANXA8 transcription, which drives NSCLC progression (Figure 3A). Another lncRNA MNX1 antisense RNA 1 (MNX1-AS1) is also upregulated in NSCLC due to copy-number gain and c-Myc-mediated transcriptional activation, and is associated with poor clinical outcomes. MNX1-AS1 induces phase separation of IGF2BP1, enhancing its interaction with the 3’- untranslated region of c-Myc and E2F1 mRNAs, thereby stabilizing these mRNAs and accelerating cell-cycle progression and proliferation in NSCLC (55).
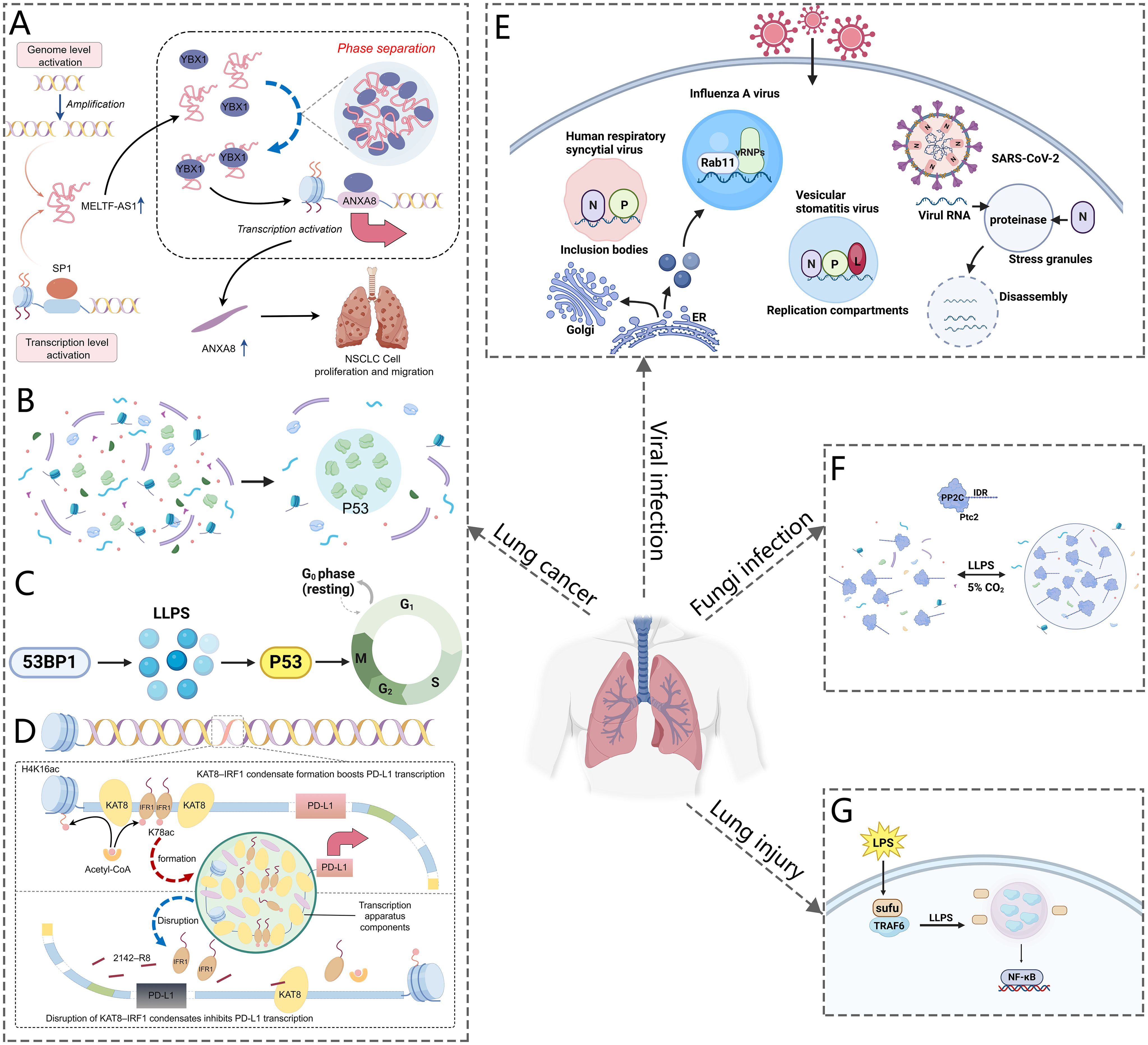
Figure 3. Schematic representation of LLPS in respiratory diseases. (A) MELTF-AS1 directly binds to YBX1, inducing its phase separation, which activates ANXA8 transcription and promotes NSCLC tumorigenesis. (B) P53 forms phase-separated condensates. (C) Phase-separated 53BP1 regulates p53 activity and maintains genomic stability. (D) KAT8 acetylates IRF1, forming condensates with PD-L1 and transcriptional machinery to enhance PD-L1 expression and facilitate immune evasion by cancer cells. (E) The SARS-CoV-2 N protein undergoes phase separation to form droplets. It interacts with G3BP1, leading to stress granule disassembly. Additionally, the virus forms liquid compartments through LLPS, which aids in viral replication and packaging. (F) CO2 induces the formation of phase-separated droplets of PP2Cs in C albicans. (G) Sufu inhibits TRAF6 droplet formation through phase separation, preventing NF-κB activation in response to LPS stimulation.
In addition to mutations in oncogenic genes, the pathogenesis of lung cancer is intricately associated with defects in tumor suppressors. p53 is a pivotal tumor suppressor essential for inhibiting cancer development. Mutations in p53 can result in the loss of its tumor-suppressive function, thereby promoting cell proliferation and inhibiting apoptosis in cancer cells (60). In lung cancer tissues, mutant p53 protein is significantly overexpressed, which correlates with a poorer prognosis in patients harboring p53 mutations (61). Recent reports indicate that p53 can also perform its functions by participating in the formation of liquid-like condensates. Firstly, p53 contributes to the formation of PML and Cajal bodies under stress response conditions (62, 63). Furthermore, p53 undergoes LLPS in response to DNA damage, forming liquid droplets that recruit and activate downstream effectors of the DNA damage response (Figure 3B). However, mutations in p53 can disrupt the formation of these droplets, resulting in impaired DNA repair and increased genomic instability (64). Studies have also identified the amyloid formation of p53 protein in human cancer tissues. Cancer-associated mutations in p53 accelerate protein aggregation and amyloid formation by disrupting the folding of the p53 core domain (50). Mutant p53 tends to form aggregates with amyloid properties in H1299 cells, especially amyloid oligomers inside the nucleus, which are believed to cause oncogenic gain-of-function. The pathway of mutant p53 from liquid droplets to gel-like and solid-like (amyloid) species may be a suitable target for anticancer therapy (65). Additionally, p53-binding protein 1 (53BP1), a crucial mediator of the DNA damage response and an interactor with p53, recruits other DNA repair proteins, drives transcription of p53 target genes, and affects cellular oncogenicity. Research has shown that 53BP1 undergoes phase separation to form droplet-like compartments near DNA breaks (Figure 3C) (52, 66). The phase-separated 53BP1 serves as a scaffold for recruiting p53 molecules and activating factors, thereby amplifying signaling and maintaining genomic integrity. Disruption of 53BP1 phase separation impairs p53 enrichment and compromises genomic stability.
Last but not least, abnormal activation of signaling pathways in lung cancer promotes the proliferation, survival, and metastasis of cancer cells. As previously discussed, LLPS serves as a pivotal hub in regulating signal transduction and modulating cellular activities. Aberrant LLPS, which disrupts signaling pathways can drive tumorigenesis and cancer progression. Dysregulation of the Hippo signaling pathway, particularly through the activation of its downstream effectors, Yes-associated protein (YAP) and transcriptional coactivator with PDZ-binding motif (TAZ), is a critical factor in the development and progression of NSCLC (67). Recent studies have shown that both YAP and TAZ undergo LLPS, forming condensates that enhance gene transcription by interacting with super enhancers (48, 49). The nuclear factor NONO promotes TAZ LLPS and facilitates its role in oncogenic transcription. Additionally, YAP undergoes LLPS to form nuclear condensates under hyperosmotic stress, which compartmentalize factors like TEAD1 and TAZ, thereby enhancing YAP-specific gene transcription (68). The small nucleolar RNA host gene 9 (SNHG9) drives LATS1 LLPS and inhibits YAP phosphorylation by LATS1 (69–71). This complex interplay highlights the potential of targeting YAP/TAZ in NSCLC, although research in this area remains ongoing. Furthermore, enhancer of zeste homolog 2 (EZH2) binds to and methylates STAT3, leading to STAT3 signaling activity by increased tyrosine phosphorylation of STAT3, and is a significant target for anticancer therapies. Recent studies have shown that myristoylation enables EZH2 to form phase-separated droplets in vitro and liquid-like nuclear puncta in lung cancer cells. STAT3 is observed to co-localize with EZH2 in these puncta. The interaction between EZH2 and STAT3 is further amplified by EZH2 myristoylation, leading to the activation of STAT3 signaling and the promotion of lung cancer cell growth (54). These findings provide a rationale for targeting EZH2 myristoylation, disrupting EZH2-STAT3 interactions within the condensates, or modulating the properties of EZH2 condensates. In addition to Hippo and STAT3 signaling pathway, the programmed death-1 (PD-1)/PD-ligand (L) 1 axis is also closely related to lung cancer development. Activation of the PD-1/PD-L1 pathway contributes to tumor immune escape (72). Wu et al. (47) reported that exposure to interferon-γ (IFNγ) induces phase separation of KAT8 and IRF1 in the A549 lung cancer cells, resulting in the formation of BMCs that enhance PD-L1 expression (Figure 3D). By exploiting the mechanism of KAT8–IRF1 condensate formation, the study identified the 2142–R8 blocking peptide, which disrupts the assembly of these condensates, thereby inhibiting PD-L1 expression and enhancing antitumor immune responses both in vitro and in vivo. These findings underscore the crucial role of KAT8–IRF1 condensates in regulating PD-L1 and propose a competitive peptide strategy to improve antitumor immunity.
Lung cancer is characterized by significant heterogeneity and complexity. Its etiology is diverse, encompassing mutations in oncogenes such as ALK and RET, inactivation of the tumor suppressor protein p53, and dysregulation of various signaling pathways. LLPS plays a crucial role in lung cancer by influencing multiple molecules and signaling pathways. Recent advancements in understanding the biophysical properties of the cancer cell microenvironment and the mechanisms of LLPS in lung cancer have created new opportunities for innovative therapeutic strategies. LLPS-based therapies involve manipulating RNA or protein interactions that govern the formation of liquid droplets within cells, including YAP, TAZ, p53, and lncRNAs. Targeting these interactions can disrupt the formation of liquid droplets that are essential for cancer cell survival. Moreover, targeting oncogenic signaling pathways, including Hippo, STAT3, and PD-L1, has been a key focus of LLPS-based therapies. Although LLPS-based therapies are still in the early stages of development, their potential for personalized cancer treatment is considerable. With ongoing research, LLPS-based targeted therapies could emerge as a cornerstone of lung cancer treatment.
2.2 LLPS in viral infection
Recent research has highlighted a significant link between LLPS and various non-communicable diseases, with this connection now extended to infectious diseases, particularly viral infections (73). LLPS plays a crucial role at multiple stages of the viral lifecycle, including protein synthesis, genome assembly, virus assembly, and the processes of budding and release (Table 2) (74). Notably, LLPS can function as both a host defense mechanism against pathogens and a tool for pathogens to enhance their invasiveness in viral infections. This dual role illustrates its complex involvement in the dynamics of viral diseases (43).
The nucleocapsid (N) protein plays a crucial role in viral replication, the packaging of viral genomic RNA into new virions, and the modulation of the host cell’s response to infection. The emergence of SARS-CoV-2 has led to a global health crisis known as COVID-19, presenting significant challenges to public health systems worldwide (90). Studies have indicated that the nucleocapsid (N) protein of SARS-CoV-2 undergoes LLPS, forming condensates that are rich in RNA and polymerase (Figure 3E) (43, 91). The N protein comprises two globular domains: the RNA-binding domain and the C-terminal dimerization domain, flanked by intrinsically disordered regions (IDRs) (92). This structure facilitates LLPS in the presence of RNA, with the process being modulated by the concentrations of RNA and proteins (93). Phase separation generates spherical droplets that are observable under fluorescence microscopy. These droplets undergo material transformations over time, potentially indicating the initial stages of nucleocapsid assembly. Moreover, the N protein is associated with SGs, cytoplasmic structures formed through LLPS, and plays a crucial role in viral replication efficiency. In cells, the N protein colocalizes with G3BP1, a marker protein for SGs. Fluorescence recovery after photobleaching (FRAP) analysis reveals distinct populations of the N protein within SGs, suggesting diverse sub-structural localizations and functions. Researchers have identified that the N protein can be recruited into SGs, where it interferes with the interaction between G3BP1 and other core SGs components, leading to SGs disassembly (Figure 3E) (94). Guseva et al. (82) demonstrated that purified N protein and phosphoprotein (P) from measles virus (MeV) formed liquid-like, membrane-less organelles upon in vitro mixing. They identified weak interactions involving the intrinsically disordered domains of the N and P proteins, which are essential for phase separation. RNA colocalized with droplets, initiating the assembly of N protein protomers into nucleocapsid-like particles that encapsulated the RNA. The rate of encapsidation within droplets was enhanced compared to the dilute phase, revealing a significant role of LLPS in MeV replication.
During viral infection, the replication and assembly of numerous viruses take place within specialized intracellular compartments. These structures are known as viral factories, replication compartments (RCs), inclusion bodies (IBs), SGs, Negri bodies (NBs), and cytoplasmic virion assembly compartments (cVACs). These compartments concentrate viral proteins, nucleic acids, and cellular factors, facilitating the essential steps of viral replication and concurrently shielding the viral genome from cellular defenses. LLPS may contribute to the formation and maintenance of these compartments. Influenza A virus (IAV) infections represent a significant threat to human health, resulting in annual epidemics and occasional pandemics (95). Alenquer et al. demonstrated that during viral assembly, IAV forms cytosolic inclusions comprising viral ribonucleoproteins. Their study revealed that these viral inclusions possess characteristics akin to liquid organelles, segregating from the cytosol without a defining membrane, dynamically exchanging material, and rapidly adapting to environmental changes (77). Evidence was provided that viral inclusions localize near endoplasmic reticulum (ER) exit sites, rely on continuous ER-Golgi vesicular cycling, and do not provoke an interferon response (Figure 3E). Additionally, the study proposes that viral inclusions isolate ribonucleoproteins from the cytosol and enable specific RNA-RNA interactions within a liquid environment. The RCs of vesicular stomatitis virus (VSV) also display liquid-like properties resulting from LLPS. Analysis of the expression of individual viral components involved in replication has revealed that three key viral proteins, namely the N, P protein and the multifunctional large protein (L), are essential for replication and can induce LLPS within the cytoplasm (Figure 3E) (84). Human adenoviruses (HAdV) are prevalent pathogens responsible for acute respiratory tract infections and have recently shown an increased prevalence, often leading to pneumonia (96, 97). Hidalgo et al. (86) investigated the biophysical properties of intranuclear RCs formed during HAdV infection. They identified the viral DNA-binding protein (DBP) as a major component of RCs, which contains intrinsically disordered and proline-rich regions—features also found in proteins involved in phase transitions. Through FRAP and time-lapse studies in living HAdV-infected cells, they observed that DBP-positive RCs display characteristics similar to liquid BMCs, including fusion and division, eventually forming an intranuclear mesh with diminished fluid-like properties. Additionally, the transient expression of DBP replicates the assembly and liquid-like properties of RCs in HAdV-infected cells. These findings indicate that DBP may act as a scaffold protein in the assembly of HAdV-RCs, thereby guiding future research into the role of BMCs in virus-host interactions. Respiratory syncytial virus (RSV), a major cause of acute respiratory infections in young children and a significant concern for the elderly and immunocompromised, has also been studied in this context (98). Research has shown that cytoplasmic IBs, which are essential for viral replication and transcription, depend on LLPS mediated by interactions between the N and P proteins (Figure 3E) (74, 79, 98). In addition to RSV, Boggs et al. (89) showed that Human metapneumovirus (HMPV) IBs in infected or transfected cells display properties characteristic of liquid organelles, including fusion and fission. Purified HMPV P protein was found to form liquid droplets independently, differentiating it from IBs in other viral systems. In vitro experiments demonstrated that the HMPV P protein recruits monomeric N (N0-P) and N-RNA to form droplets. This observation suggests that the P protein functions as a scaffold, facilitating multivalent interactions with both monomeric and oligomeric N protein and RNA, thereby promoting phase separation of IBs. Moreover, recent studies provided evidence that the endoribonuclease nsp15 of Infectious Bronchitis Virus (IBV) interferes with the formation of host antiviral SGs by regulating the accumulation of viral dsRNA and by antagonizing the activation of protein kinase R, eventually ensuring productive virus replication.
LLPS is also critical for herpesvirus replication and transcription. For example, the herpes simplex virus type 1 (HSV-1) immediate-early protein ICP4, which is intrinsically disordered, induces the formation of nuclear condensates via LLPS (73). The role of ICP4 in viral replication, along with its localization within replication compartments, suggests that LLPS may contribute to the formation of these compartments (88, 99). Additionally, HSV-1 tegument protein UL11, which contains IDRs, also undergoes LLPS in vitro (87, 100). The presence of IDRs in several HSV-1 tegument proteins suggests a potential role in tegument assembly through LLPS (87).
The significance of LLPS in viral processes associated with respiratory infections, such as adsorption, replication, assembly, and release, has become increasingly clear (73, 74). LLPS promotes the formation of BMCs, which concentrate viral replication machinery, enhance viral gene transcription and expression, and modulate innate immune responses by sequestering host sensors within IBs (43). This mechanistic insight into phase separation provides a deeper understanding of the viral life cycle within host cells and suggests potential strategies for developing targeted treatments for viral infections (101).
2.3 LLPS in fungi infection
Candida albicans, commonly known as a yeast species, typically has a symbiotic relationship with the human body under normal conditions, but in cases of weakened immune systems, it can proliferate uncontrollably, leading to candidiasis pneumonia (102). This condition is often characterized by respiratory distress, coughing, chest pain, and fever (103). Zhang et al. (104) discovered that elevated CO2 levels induce the formation of IDR-containing PP2Cs in Candida albicans. The Ser/Thr-rich sequences within the IDRs are essential for driving the phase transitions of PP2Cs under high CO2 conditions. Their findings suggest that interactions between Ser/Thr residues and CO2 may enhance the cohesive forces required for phase separation. This phase separation activates the phosphatase activity of PP2Cs, thereby initiating various CO2-responsive biological processes (Figure 3F). Their data demonstrated that the functionally conserved, yet sequence-diverse, disordered regions of PP2C phosphatases can act as CO2 sensors.
2.4 LLPS in lung injury
Sepsis is marked by severe organ dysfunction, especially in the lungs, due to a dysregulated immune response to infections (103). Recent studies have shown decreased expression of Suppressor of Fused (Sufu) during the early stages of lipopolysaccharide (LPS)-induced acute inflammation in murine lung and peritoneal macrophages. The absence of Sufu worsens lung injury and increases mortality in mice subjected to LPS and cecal ligation and puncture (CLP) challenges (105). Moreover, Sufu deficiency amplifies LPS-induced proinflammatory gene expression in cultured macrophages (105). This research underscores Sufu’s critical role as a negative regulator in the Toll-Like Receptor (TLR)-mediated inflammatory response. Sufu directly interacts with TNF Receptor Associated Factor 6 (TRAF6), inhibiting its oligomerization and autoubiquitination. During LPS-induced inflammation, TRAF6 undergoes phase separation, a crucial step in the activation of ubiquitination and NF-κB signaling (Figure 3G). Sufu effectively prevents the formation of phase-separated TRAF6 droplets, thereby suppressing NF-κB activation in response to LPS. In a septic shock model, TRAF6 depletion alleviated the exacerbated inflammatory phenotype in mice with myeloid cell-specific Sufu deletion.
3 LLPS as a novel therapeutic mechanism
The significance of LLPS in respiratory diseases highlights its potential as a therapeutic target. Indeed, various drugs or molecules can modify the formation rate, composition, stability, and physical properties of LLPS, thus presenting significant opportunities for drug discovery (Figure 4). As previously mentioned, LLPS plays a crucial role in viral infections, indicating that targeting the phase separation process could pave the way for new antiviral drug development. he SARS-CoV-2 N protein interacts with SGs proteins G3BP1/2 and CSNK2B/CSNK2A2, which are subunits of casein kinase 2 (CK2), leading to the disassembly or inhibition of SGs formation. Consequently, CK2 inhibitors such as GO289 and silmitasertib, which disrupt protein-protein interactions between the N protein and SG-associated proteins, could serve as potential chemical probes or antivirals targeting SGs (Figure 4A) (106, 107). Additionally, NF-κB represents a potential therapeutic target for infectious diseases. The compound 1,6-hexanediol, known to inhibit LLPS, suppresses N protein phase separation, thus limiting its role in NF-κB activation (Figure 4A) (108, 109). However, 1,6-hexanediol exhibits high cytotoxicity and inhibits phosphatase and kinase activities. Propylene glycol, in contrast, offers a non-toxic alternative for disrupting viral replication (110) (–).-Gallocatechin gallate (GCG), a green tea polyphenol, disrupts N protein LLPS and inhibits SARS-CoV-2 replication, potentially treating COVID-19 by targeting N-RNA condensation (Figure 4A) (91). Recent studies have shown that the aminoglycoside kanamycin disrupts LLPS in SARS-CoV-2-infected mammalian cells (93). Kanamycin binds to nucleic acids through electrostatic interactions, thereby preventing RNA-protein interactions. The addition of kanamycin to droplets resulted in a reduction in condensate size, a decreased protein/RNA ratio in assays, and the relocalization of the N protein to the nucleus (93). Small-molecule modulators of host kinases or phosphatases could regulate LLPS and act as antiviral agents. Activators of SR protein kinase 1 (SRPK1) are potential antivirals, as they phosphorylate the N protein SR region to attenuate RNA-induced LLPS and viral RNA transcription (111). RSV replication occurs within IBs. The steroidal alkaloid cyclopamine and its chemical analogue A3E inhibit RSV replication by disrupting and solidifying IB condensates (Figures 4A, C) (98). Furthermore, cyclopamine and A3E demonstrate dose-dependent inhibition of RSV replication in mouse models, highlighting their therapeutic potential.
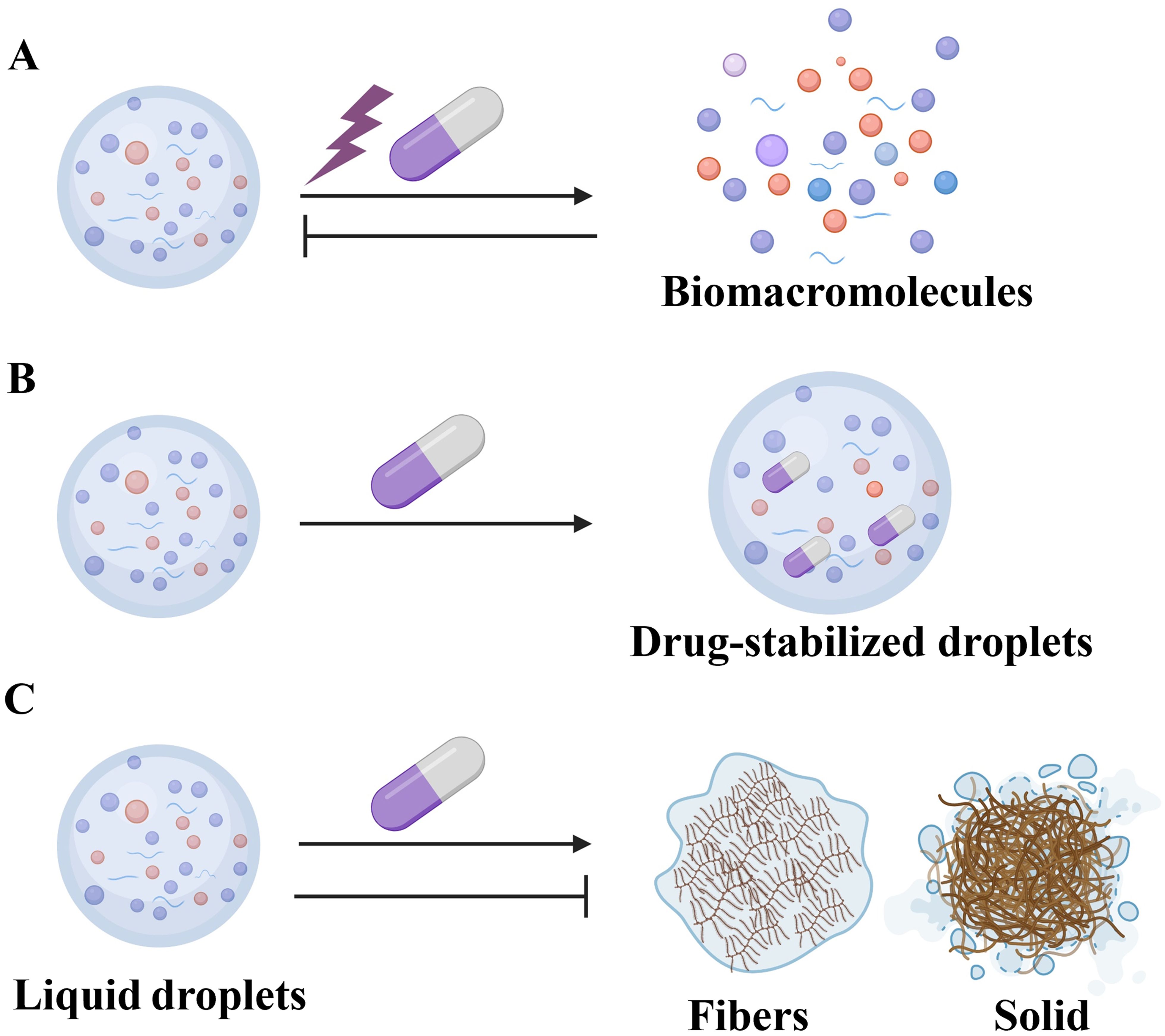
Figure 4. Therapies Targeting LLPS. (A) Drugs such as GO289, 1,6-hexanediol, GCG, A3E, and EVG, as well as environmental adjustments, can disrupt or prevent the formation of specific biomacromolecules into droplets. (B) Drugs, including cisplatin, can accumulate within condensates. (C) The steroidal alkaloid cyclopamine and A3E can solidify condensates. Conversely, other drugs may stabilize these liquid droplets, thus preventing their dissolution or transition into fibrous or solid phases.
In addition to direct interventions targeting LLPS formation, modifying environmental conditions can regulate the LLPS states of target proteins. SGs are particularly sensitive to oxidative, osmotic, and heat shock stresses. Temperature variations may thus affect translation mechanisms within SGs under stress (112). Furthermore, SARS-CoV-2 N protein condenses with specific RNA genomic elements under physiological buffer conditions and condensation is enhanced at human body temperatures (33°C and 37°C) and reduced at room temperature (22°C) (113). Roden et al. also observed that N protein can condense in the absence of RNA at high temperatures, but addition of RNA lowers the temperature at which droplets emerge (114). These findings highlight the potential of environmental modulation, such as temperature adjustment, as a strategy to disrupt LLPS and inhibit viral replication, suggesting it could be a promising adjunctive systemic therapy (Figure 4A).
As our understanding of the role of LLPS in various biological processes advances, its potential to modulate tumor-associated proteins and their associated upstream and downstream signaling pathways is increasingly recognized, thereby improving therapeutic strategies for lung cancer. Although anti-PD-1/PD-L1 therapy has shown efficacy in lung cancer, drug resistance remains a significant challenge. Recent studies have shown that YAP interacts with histone acetyltransferase EP300, transcription factor TEAD4, and mediator 1 to form phase-separated transcriptional condensates, which enhance the transcription of target genes (115). Disrupting YAP’s LLPS capacity inhibits cancer cell growth, enhances the immune response, and increases the sensitivity of tumor cells to anti-PD-1 therapy. Another study found that SRC-1 co-expresses with YAP in NSCLC and is essential for lung cancer growth. SRC-1 interacts with YAP/TEAD to enhance YAP transcriptional activity through the formation of compartmentalized SRC-1/YAP/TEAD condensates. The anti-HIV drug elvitegravir (EVG) specifically disrupts SRC-1 condensate formation in cells, thereby efficiently inhibiting YAP oncogenic transcriptional activity and constraining YAP-dependent cancer cell growth (Figure 4A) (116).
Recent studies indicate that antitumor drugs accumulate in specific condensates within cancer cells, with their distribution profoundly affecting drug efficacy (Figure 4B). Furthermore, modifications to the properties of phase-separation condensates can further influence drug concentrations and effectiveness (Figure 4C). Cisplatin is a potent chemotherapeutic agent used to treat lung cancer through its ability to induce DNA damage and cell apoptosis. Studies have demonstrated that, in vitro, reconstituted condensates can concentrate various anticancer drugs, including cisplatin, mitoxantrone, tamoxifen, THZ1, and JQ1, all of which interact with DNA (117). These drugs showed high enrichment within the condensates of the transcription regulator MED1, which are formed by super-enhancers and large enhancer clusters associated with oncogenesis and gene activation (117, 118). Co-incubation of DNA with cisplatin in reconstituted MED1 condensates led to substantial DNA modification through platination (119). In cancer cells, plastinated DNA frequently co-localizes with MED1 condensates, suggesting that the partitioning of cisplatin into these condensates enhances drug activity (117, 119). Notably, reconstituted MED1 condensates gradually dissolved in the presence of cisplatin, and prolonged cisplatin exposure led to MED1 depletion from super-enhancers in cancer cells (117, 119). These findings suggest that the selective partitioning and concentration of antineoplastic drugs within condensates play a critical role in drug pharmacodynamics. Further investigation of this phenomenon could advance therapeutic approaches for diseases.
Modulating the assembly and dynamics of LLPS condensates enables precise control over cellular processes. This approach offers a unique opportunity to restore normal cellular functions and mitigate disease progression. LLPS-based therapies have shown considerable promise in treating respiratory diseases. However, several challenges must be addressed before these therapies can be transitioned into clinical practice. A major obstacle is the limited understanding of the specific mechanisms of LLPS in respiratory diseases and the complex signaling pathways involved. Another significant challenge involves developing efficient and precise LLPS inhibitors, as well as evaluating their safety and toxicity. Moreover, most studies have been conducted from a monocellular perspective, limiting observations to narrow contexts and overlooking complex intercellular interactions and the intricate body system. Transitioning from in vitro to in vivo models, as well as from cellular to animal models, is crucial for elucidating the role of phase separation in biochemical reactions. This advancement could significantly enhance drug targeting strategies that focus on phase separation. Addressing these challenges requires future research to further unravel the mechanisms of LLPS in respiratory diseases and identify novel LLPS regulators and therapeutic targets.
4 Perspectives
LLPS orchestrates the formation of various biomacromolecular condensates, which are crucial for numerous cellular processes, such as subcellular compartmentalization, cell cycle regulation, signal transduction, gene expression modulation, and protein quality control. Dysregulated phase separation, resulting from mutations in phase-separated proteins, compromised quality control systems, or environmental changes, has been implicated in the pathogenesis of various respiratory diseases. Consequently, identifying molecules that modulate phase separation represents a promising strategy for drug development and therapeutic interventions targeting diseases associated with abnormal LLPS.
Interest in the role of LLPS in respiratory diseases, particularly lung cancer and respiratory viral infections, is growing, as abnormal LLPS emerges as a crucial pathophysiological factor. Research into therapeutic strategies and medications targeting LLPS in these diseases is also expanding. Nevertheless, research connecting LLPS to lung injury and fungal infections remains limited and warrants further investigation. Although direct evidence linking LLPS to other respiratory diseases, such as chronic obstructive pulmonary disease (COPD), pulmonary fibrosis, and asthma, is scarce, emerging data suggest plausible associations. For instance, lncRNAs, functioning as architectural RNAs, recruit proteins that influence disease progression in the respiratory system. Studies have confirmed that certain lncRNAs are involved in regulating LLPS (120, 121). Future research should explore how LLPS interacts with these lncRNAs during the progression of respiratory disease. Aberrant protein expression, misfolding, and functional dysregulation are implicated in the initiation and progression of respiratory diseases. Recent studies have identified many proteins capable of LLPS, including N protein and p53, which are involved in disease pathogenesis (37, 122). Databases such as DrLLPS and LLPSDB compile extensive datasets of over 400,000 known and potential LLPS-related proteins, thereby providing crucial support for advancing LLPS research (123, 124). Investigating the ultrastructure and functional consequences of previously unknown proteins through these databases is of significant value. Further research will elucidate the precise roles of additional abnormal LLPS proteins in the pathogenesis of respiratory diseases. Additionally, SGs are membrane-less organelles formed through LLPS in response to various stress stimuli, have been identified in diseases such as asthma, COPD, and lung cancer (125).
Future research should prioritize the development and optimization of LLPS-modulating agents, focusing on their safety and efficacy in clinical settings. Exploring the broader implications of LLPS in various respiratory diseases, as well as combinatorial therapies involving LLPS modulators, may lead to innovative treatments. A thorough understanding of LLPS mechanisms in pathogenesis will be essential for designing targeted interventions. Additionally, modulating the environment, particularly through temperature control, shows promise for disrupting LLPS and potentially inhibiting viral replication, thereby complementing other therapeutic strategies. In oncology, targeting LLPS mechanisms in cancer cells, particularly those involving tumor-associated proteins and signaling pathways, may enhance therapeutic strategies, address drug resistance, and improve outcomes for patients with lung cancer. The accumulation and partitioning of antitumor drugs within specific cellular condensates offer a promising avenue for refining cancer therapy. Advancing our knowledge in these areas will enable the development of more effective treatment strategies and the optimization of therapeutic outcomes. Addressing the challenges related to LLPS mechanisms and translating findings from monocellular models to in vivo systems will be crucial for advancing LLPS-based therapies. Continued research into LLPS holds the potential to significantly impact the treatment of both respiratory diseases and lung cancer.
Overall, research into the relationship between respiratory diseases and liquid-phase separation is still in its early stages but is beginning to demonstrate significant potential. As technology and research advance, this field is anticipated to offer valuable insights and methods for the early diagnosis and personalized treatment of respiratory diseases.
Author contributions
LW: Conceptualization, Data curation, Investigation, Methodology, Writing – original draft. YW: Conceptualization, Data curation, Supervision, Writing – original draft. ZK: Formal analysis, Funding acquisition, Writing – original draft. ZW: Software, Supervision, Writing – original draft. YG: Formal analysis, Investigation, Writing – original draft. YZ: Methodology, Software, Supervision, Writing – original draft. XZ: Funding acquisition, Project administration, Writing – review & editing. ZG: Funding acquisition, Supervision, Writing – review & editing. BW: Conceptualization, Data curation, Formal analysis, Project administration, Writing – review & editing.
Funding
The author(s) declare financial support was received for the research, authorship, and/or publication of this article. This work was supported by grants from the following sources: the National Natural Science Foundation of China (81971726), the Natural Science Foundation of Jiangsu Province (BK2019131), Nanjing Science and Technology Project (YKK22220), Science Foundation of Kangda College of Nanjing Medical University (KD2023KYJJ220), and supported by the Affiliated Jiangning Hospital of Nanjing Medical University Youth Innovation Scientific Research Project (JNYYZXKY202305, JNYYZXKY202205).
Acknowledgments
Figures 3A, D were generated using Figdraw, with each image authorized and assigned a legal ID (AYSIA0b85b; PIIPI6c6c0). All other figures were created with Biorender.com.
Conflict of interest
The authors declare that the research was conducted in the absence of any commercial or financial relationships that could be construed as a potential conflict of interest.
Publisher’s note
All claims expressed in this article are solely those of the authors and do not necessarily represent those of their affiliated organizations, or those of the publisher, the editors and the reviewers. Any product that may be evaluated in this article, or claim that may be made by its manufacturer, is not guaranteed or endorsed by the publisher.
References
1. Banani SF, Rice AM, Peeples WB, Lin Y, Jain S, Parker R, et al. Compositional control of phase-separated cellular bodies. Cell. (2016) 166:651–63. doi: 10.1016/j.cell.2016.06.010
2. Xiao Q, McAtee CK, Su X. Phase separation in immune signalling. Nat Rev Immunol. (2022) 22:188–99. doi: 10.1038/s41577-021-00572-5
3. Zhou S, Fu Z, Zhang Z, Jia X, Xu G, Sun L, et al. Liquid-liquid phase separation mediates the formation of herpesvirus assembly compartments. J Cell Biol. (2023) 222:e202201088. doi: 10.1083/jcb.202201088
4. Gao Y, Li X, Li P, Lin Y. A brief guideline for studies of phase-separated biomolecular condensates. Nat Chem Biol. (2022) 18:1307–18. doi: 10.1038/s41589-022-01204-2
5. Boeynaems S, Chong S, Gsponer J, Holt L, Milovanovic D, Mitrea DM, et al. Phase separation in biology and disease; current perspectives and open questions. J Mol Biol. (2023) 435:167971. doi: 10.1016/j.jmb.2023.167971
6. Gallego LD, Schneider M, Mittal C, Romanauska A, Gudino CR, Schubert T, et al. Phase separation directs ubiquitination of gene-body nucleosomes. Nature. (2020) 579:592–97. doi: 10.1038/s41586-020-2097-z
7. Laflamme G, Mekhail K. Biomolecular condensates as arbiters of biochemical reactions inside the nucleus. Commun Biol. (2020) 3:773. doi: 10.1038/s42003-020-01517-9
8. Alberti S, Gladfelter A, Mittag T. Considerations and challenges in studying liquid-liquid phase separation and biomolecular condensates. Cell. (2019) 176:419–34. doi: 10.1016/j.cell.2018.12.035
9. Zhang H, Ji X, Li P, Liu C, Lou J, Wang Z, et al. Liquid-liquid phase separation in biology: mechanisms, physiological functions and human diseases. Sci China Life Sci. (2020) 63:953–85. doi: 10.1007/s11427-020-1702-x
10. Tiwary AK, Zheng Y. Protein phase separation in mitosis. Curr Opin Cell Biol. (2019) 60:92–8. doi: 10.1016/j.ceb.2019.04.011
11. Zhang H, Elbaum-Garfinkle S, Langdon EM, Taylor N, Occhipinti P, Bridges AA, et al. RNA controls polyQ protein phase transitions. Mol Cell. (2015) 60:220–30. doi: 10.1016/j.molcel.2015.09.017
12. Maharana S, Wang J, Papadopoulos DK, Richter D, Pozniakovsky A, Poser I, et al. RNA buffers the phase separation behavior of prion-like RNA binding proteins. Science. (2018) 360:918–21. doi: 10.1126/science.aar7366
13. Lu J, Qian J, Xu Z, Yin S, Zhou L, Zheng S, et al. Emerging roles of liquid-liquid phase separation in cancer: from protein aggregation to immune-associated signaling. Front Cell Dev Biol. (2021) 9:631486. doi: 10.3389/fcell.2021.631486
14. Mehta S, Zhang J. Liquid-liquid phase separation drives cellular function and dysfunction in cancer. Nat Rev Cancer. (2022) 22:239–52. doi: 10.1038/s41568-022-00444-7
15. Millar SR, Huang JQ, Schreiber KJ, Tsai YC, Won J, Zhang J, et al. A new phase of networking: the molecular composition and regulatory dynamics of mammalian stress granules. Chem Rev. (2023) 123:9036–64. doi: 10.1021/acs.chemrev.2c00608
16. Duan Y, Du A, Gu J, Duan G, Wang C, Gui X, et al. PARylation regulates stress granule dynamics, phase separation, and neurotoxicity of disease-related RNA-binding proteins. Cell Res. (2019) 29:233–47. doi: 10.1038/s41422-019-0141-z
17. Ahn JH, Davis ES, Daugird TA, Zhao S, Quiroga IY, Uryu H, et al. Phase separation drives aberrant chromatin looping and cancer development. Nature. (2021) 595:591–95. doi: 10.1038/s41586-021-03662-5
18. TaNiue K, Akimitsu N. Aberrant phase separation and cancer. FEBS J. (2022) 289:17–39. doi: 10.1111/febs.15765
19. Cable J, Brangwynne C, Seydoux G, Cowburn D, Pappu RV, Castaneda CA, et al. Phase separation in biology and disease-a symposium report. Ann N Y Acad Sci. (2019) 1452:3–11. doi: 10.1111/nyas.14126
20. Shin Y, Brangwynne CP. Liquid phase condensation in cell physiology and disease. Science. (2017) 357:eaaf4382. doi: 10.1126/science.aaf4382
21. Tong X, Tang R, Xu J, Wang W, Zhao Y, Yu X, et al. Liquid-liquid phase separation in tumor biology. Signal Transduct Target Ther. (2022) 7:221. doi: 10.1038/s41392-022-01076-x
22. Banani SF, Lee HO, Hyman AA, Rosen MK. Biomolecular condensates: organizers of cellular biochemistry. Nat Rev Mol Cell Biol. (2017) 18:285–98. doi: 10.1038/nrm.2017.7
23. Sun M, Jia M, Ren H, Yang B, Chi W, Xin G, et al. NuMA regulates mitotic spindle assembly, structural dynamics and function via phase separation. Nat Commun. (2021) 12:7157. doi: 10.1038/s41467-021-27528-6
24. Janssen A, Colmenares SU, Karpen GH. Heterochromatin: guardian of the genome. Annu Rev Cell Dev Biol. (2018) 34:265–88. doi: 10.1146/annurev-cellbio-100617-062653
25. Li CH, Coffey EL, Dall’Agnese A, Hannett NM, Tang X, Henninger JE, et al. MeCP2 links heterochromatin condensates and neurodevelopmental disease. Nature. (2020) 586:440–44. doi: 10.1038/s41586-020-2574-4
26. Collins BE, Neul JL. Rett syndrome and MECP2 duplication syndrome: disorders of meCP2 dosage. Neuropsychiatr Dis Treat. (2022) 18:2813–35. doi: 10.2147/NDT.S371483
27. Song J. Molecular mechanisms of phase separation and amyloidosis of ALS/FTD-linked FUS and TDP-43. Aging Dis. (2023) 15:2084–112. doi: 10.14336/AD.2023.1118
28. Arvand A, Denny CT. Biology of EWS/ETS fusions in Ewing’s family tumors. Oncogene. (2001) 20:5747–54. doi: 10.1038/sj.onc.1204598
29. Delattre O, Zucman J, Plougastel B, Desmaze C, Melot T, Peter M, et al. Gene fusion with an ETS DNA-binding domain caused by chromosome translocation in human tumours. Nature. (1992) 359:162–65. doi: 10.1038/359162a0
30. Johnson KM, Mahler NR, Saund RS, Theisen ER, Taslim C, Callender NW, et al. Role for the EWS domain of EWS/FLI in binding GGAA-microsatellites required for Ewing sarcoma anchorage independent growth. Proc Natl Acad Sci U.S.A. (2017) 114:9870–75. doi: 10.1073/pnas.1701872114
31. Boulay G, Sandoval GJ, Riggi N, Iyer S, Buisson R, Naigles B, et al. Cancer-specific retargeting of BAF complexes by a prion-like domain. Cell. (2017) 171:163–78. doi: 10.1016/j.cell.2017.07.036
32. Riggi N, Knoechel B, Gillespie SM, Rheinbay E, Boulay G, Suva ML, et al. EWS-FLI1 utilizes divergent chromatin remodeling mechanisms to directly activate or repress enhancer elements in Ewing sarcoma. Cancer Cell. (2014) 26:668–81. doi: 10.1016/j.ccell.2014.10.004
33. Boeynaems S, Alberti S, Fawzi NL, Mittag T, Polymenidou M, Rousseau F, et al. Protein phase separation: A new phase in cell biology. Trends Cell Biol. (2018) 28:420–35. doi: 10.1016/j.tcb.2018.02.004
34. Wang W, Chen Y, Xu A, Cai M, Cao J, Zhu H, et al. Protein phase separation: A novel therapy for cancer? Br J Pharmacol. (2020) 177:5008–30. doi: 10.1111/bph.15242
35. Murray DT, Kato M, Lin Y, Thurber KR, Hung I, McKnight SL, et al. Structure of FUS protein fibrils and its relevance to self-assembly and phase separation of low-complexity domains. Cell. (2017) 171:615–27. doi: 10.1016/j.cell.2017.08.048
36. Patel A, Lee HO, Jawerth L, Maharana S, Jahnel M, Hein MY, et al. A liquid-to-solid phase transition of the ALS protein FUS accelerated by disease mutation. Cell. (2015) 162:1066–77. doi: 10.1016/j.cell.2015.07.047
37. Mateju D, Franzmann TM, Patel A, Kopach A, Boczek EE, Maharana S, et al. An aberrant phase transition of stress granules triggered by misfolded protein and prevented by chaperone function. EMBO J. (2017) 36:1669–87. doi: 10.15252/embj.201695957
38. Gould GS, Hurst JR, Trofor A, Alison JA, Fox G, Kulkarni MM, et al. Recognising the importance of chronic lung disease: a consensus statement from the Global Alliance for Chronic Diseases (Lung Diseases group). Respir Res. (2023) 24:15. doi: 10.1186/s12931-022-02297-y
39. Labaki WW, Han MK. Chronic respiratory diseases: a global view. Lancet Respir Med. (2020) 8:531–33. doi: 10.1016/S2213-2600(20)30157-0
40. Soriano J, Kendrick P, Paulson K, Gupta V, Abrams E, Adedoyin R, et al. Prevalence and attributable health burden of chronic respiratory diseases, 1990-2017: a systematic analysis for the Global Burden of Disease Study 2017. Lancet Respir Med. (2020) 8:585–96. doi: 10.1016/S2213-2600(20)30105-3
41. Zhang X, Yuan L, Zhang W, Zhang Y, Wu Q, Li C, et al. Liquid-liquid phase separation in diseases. Medcomm (2020). (2024) 5:e640. doi: 10.1002/mco2.640
42. Zou Y, Zheng H, Ning Y, Yang Y, Wen Q, Fan S. New insights into the important roles of phase seperation in the targeted therapy of lung cancer. Cell Biosci. (2023) 13:150. doi: 10.1186/s13578-023-01101-8
43. Zhang X, Zheng R, Li Z, Ma J. Liquid-liquid phase separation in viral function. J Mol Biol. (2023) 435:167955. doi: 10.1016/j.jmb.2023.167955
44. Relli V, Trerotola M, Guerra E, Alberti S. Abandoning the notion of non-small cell lung cancer. Trends Mol Med. (2019) 25:585–94. doi: 10.1016/j.molmed.2019.04.012
45. Qin Z, Sun H, Yue M, Pan X, Chen L, Feng X, et al. Phase separation of EML4-ALK in firing downstream signaling and promoting lung tumorigenesis. Cell Discovery. (2021) 7:33. doi: 10.1038/s41421-021-00270-5
46. Qiu T, Kong Y, Wei G, Sun K, Wang R, Wang Y, et al. CCDC6-RET fusion protein regulates Ras/MAPK signaling through the fusion- GRB2-SHC1 signal niche. Proc Natl Acad Sci U.S.A. (2024) 121:e1972608175. doi: 10.1073/pnas.2322359121
47. Wu Y, Zhou L, Zou Y, Zhang Y, Zhang M, Xu L, et al. Disrupting the phase separation of KAT8-IRF1 diminishes PD-L1 expression and promotes antitumor immunity. Nat Cancer. (2023) 4:382–400. doi: 10.1038/s43018-023-00522-1
48. Cai D, Feliciano D, Dong P, Flores E, Gruebele M, Porat-Shliom N, et al. Phase separation of YAP reorganizes genome topology for long-term YAP target gene expression. Nat Cell Biol. (2019) 21:1578–89. doi: 10.1038/s41556-019-0433-z
49. Lu Y, Wu T, Gutman O, Lu H, Zhou Q, Henis YI, et al. Phase separation of TAZ compartmentalizes the transcription machinery to promote gene expression. Nat Cell Biol. (2020) 22:453–64. doi: 10.1038/s41556-020-0485-0
50. Lemos C, Schulze L, Weiske J, Meyer H, Braeuer N, Barak N, et al. Identification of small molecules that modulate mutant p53 condensation. Iscience. (2020) 23:101517. doi: 10.1016/j.isci.2020.101517
51. Alexander KA, Cote A, Nguyen SC, Zhang L, Gholamalamdari O, Agudelo-Garcia P, et al. p53 mediates target gene association with nuclear speckles for amplified RNA expression. Mol Cell. (2021) 81:1666–81. doi: 10.1016/j.molcel.2021.03.006
52. Kilic S, Lezaja A, Gatti M, Bianco E, Michelena J, Imhof R, et al. Phase separation of 53BP1 determines liquid-like behavior of DNA repair compartments. EMBO J. (2019) 38:e101379. doi: 10.15252/embj.2018101379
53. Lu X, Wang J, Wang W, Lu C, Qu T, He X, et al. Copy number amplification and SP1-activated lncRNA MELTF-AS1 regulates tumorigenesis by driving phase separation of YBX1 to activate ANXA8 in non-small cell lung cancer. Oncogene. (2022) 41:3222–38. doi: 10.1038/s41388-022-02292-z
54. Zhang J, Zeng Y, Xing Y, Li X, Zhou L, Hu L, et al. Myristoylation-mediated phase separation of EZH2 compartmentalizes STAT3 to promote lung cancer growth. Cancer Lett. (2021) 516:84–98. doi: 10.1016/j.canlet.2021.05.035
55. Zhu Q, Zhang C, Qu T, Lu X, He X, Li W, et al. MNX1-AS1 promotes phase separation of IGF2BP1 to drive c-myc-mediated cell-cycle progression and proliferation in lung cancer. Cancer Res. (2022) 82:4340–58. doi: 10.1158/0008-5472.CAN-22-1289
56. Reck M, Carbone DP, Garassino M, Barlesi F. Targeting KRAS in non-small-cell lung cancer: recent progress and new approaches. Ann Oncol. (2021) 32:1101–10. doi: 10.1016/j.annonc.2021.06.001
57. Ma S, Tang T, Probst G, Konradi A, Jin C, Li F, et al. Transcriptional repression of estrogen receptor alpha by YAP reveals the Hippo pathway as therapeutic target for ER(+) breast cancer. Nat Commun. (2022) 13:1061. doi: 10.1038/s41467-022-28691-0
58. Sabir SR, Yeoh S, Jackson G, Bayliss R. EML4-ALK variants: biological and molecular properties, and the implications for patients. Cancers (Basel). (2017) 9:118. doi: 10.3390/cancers9090118
59. Ibanez CF. Structure and physiology of the RET receptor tyrosine kinase. Cold Spring Harb Perspect Biol. (2013) 5:a009134. doi: 10.1101/cshperspect.a009134
60. Hassin O, Oren M. Drugging p53 in cancer: one protein, many targets. Nat Rev Drug Discovery. (2023) 22:127–44. doi: 10.1038/s41573-022-00571-8
61. Bian C, Li Z, Xu Y, Wang J, Xu L, Shen H. Clinical significance of mutant p53 protein expression in lung adenocarcinoma. Zhongguo Fei Ai Za Zhi. (2015) 18:23–8. doi: 10.3779/j.issn.1009-3419.2015.01.04
62. Guo A, Salomoni P, Luo J, Shih A, Zhong S, Gu W, et al. The function of PML in p53-dependent apoptosis. Nat Cell Biol. (2000) 2:730–36. doi: 10.1038/35036365
63. Cioce M, Lamond AI. Cajal bodies: a long history of discovery. Annu Rev Cell Dev Biol. (2005) 21:105–31. doi: 10.1146/annurev.cellbio.20.010403.103738
64. Kamagata K, Kanbayashi S, Honda M, Itoh Y, Takahashi H, Kameda T, et al. Liquid-like droplet formation by tumor suppressor p53 induced by multivalent electrostatic interactions between two disordered domains. Sci Rep. (2020) 10:580. doi: 10.1038/s41598-020-57521-w
65. Petronilho EC, Pedrote MM, Marques MA, Passos YM, Mota MF, Jakobus B, et al. Phase separation of p53 precedes aggregation and is affected by oncogenic mutations and ligands. Chem Sci. (2021) 12:7334–49. doi: 10.1039/d1sc01739j
66. Huang N, Dong H, Shao B. Phase separation in immune regulation and immune-related diseases. J Mol Med (Berl). (2022) 100:1427–40. doi: 10.1007/s00109-022-02253-9
67. Liang H, Xu Y, Zhao J, Chen M, Wang M. Hippo pathway in non-small cell lung cancer: mechanisms, potential targets, and biomarkers. Cancer Gene Ther. (2024) 31:652–66. doi: 10.1038/s41417-024-00761-z
68. Pobbati AV, Hong W. A combat with the YAP/TAZ-TEAD oncoproteins for cancer therapy. Theranostics. (2020) 10:3622–35. doi: 10.7150/thno.40889
69. Franklin JM, Wu Z, Guan KL. Insights into recent findings and clinical application of YAP and TAZ in cancer. Nat Rev Cancer. (2023) 23:512–25. doi: 10.1038/s41568-023-00579-1
70. Mokhtari RB, Ashayeri N, Baghaie L, Sambi M, Satari K, Baluch N, et al. The hippo pathway effectors YAP/TAZ-TEAD oncoproteins as emerging therapeutic targets in the tumor microenvironment. Cancers (Basel). (2023) 15:3468. doi: 10.3390/cancers15133468
71. Wei Y, Hui V, Chen Y, Han R, Han X, Guo Y. YAP/TAZ: Molecular pathway and disease therapy. Medcomm (2020). (2023) 4:e340. doi: 10.1002/mco2.340
72. Konen JM, Wu H, Gibbons DL. Immune checkpoint blockade resistance in lung cancer: emerging mechanisms and therapeutic opportunities. Trends Pharmacol Sci. (2024) 45:520–36. doi: 10.1016/j.tips.2024.04.006
73. Li H, Ernst C, Kolonko-Adamska M, Greb-Markiewicz B, Man J, Parissi V, et al. Phase separation in viral infections. Trends Microbiol. (2022) 30:1217–31. doi: 10.1016/j.tim.2022.06.005
74. Wei W, Bai L, Yan B, Meng W, Wang H, Zhai J, et al. When liquid-liquid phase separation meets viral infections. Front Immunol. (2022) 13:985622. doi: 10.3389/fimmu.2022.985622
75. Zhao D, Xu W, Zhang X, Wang X, Ge Y, Yuan E, et al. Understanding the phase separation characteristics of nucleocapsid protein provides a new therapeutic opportunity against SARS-CoV-2. Protein Cell. (2021) 12:734–40. doi: 10.1007/s13238-021-00832-z
76. Savastano A, Ibanez DOA, Rankovic M, Zweckstetter M. Nucleocapsid protein of SARS-CoV-2 phase separates into RNA-rich polymerase-containing condensates. Nat Commun. (2020) 11:6041. doi: 10.1038/s41467-020-19843-1
77. Alenquer M, Vale-Costa S, Etibor TA, Ferreira F, Sousa AL, Amorim MJ. Influenza A virus ribonucleoproteins form liquid organelles at endoplasmic reticulum exit sites. Nat Commun. (2019) 10:1629. doi: 10.1038/s41467-019-09549-4
78. Yamauchi Y. Influenza A virus uncoating. Adv Virus Res. (2020) 106:1–38. doi: 10.1016/bs.aivir.2020.01.001
79. Galloux M, Risso-Ballester J, Richard CA, Fix J, Rameix-Welti MA, Eleouet JF. Minimal elements required for the formation of respiratory syncytial virus cytoplasmic inclusion bodies in vivo and in vitro. Mbio. (2020) 11:e01202–20. doi: 10.1128/mBio.01202-20
80. Selvaraj M, Yegambaram K, Todd E, Richard CA, Dods RL, Pangratiou GM, et al. The structure of the human respiratory syncytial virus M2-1 protein bound to the interaction domain of the phosphoprotein P defines the orientation of the complex. Mbio. (2018) 9:e01554-18. doi: 10.1128/mBio.01554-18
81. Richard CA, Rincheval V, Lassoued S, Fix J, Cardone C, Esneau C, et al. RSV hijacks cellular protein phosphatase 1 to regulate M2-1 phosphorylation and viral transcription. PloS Pathog. (2018) 14:e1006920. doi: 10.1371/journal.ppat.1006920
82. Guseva S, Milles S, Jensen MR, Salvi N, Kleman JP, Maurin D, et al. Measles virus nucleo- and phosphoproteins form liquid-like phase-separated compartments that promote nucleocapsid assembly. Sci Adv. (2020) 6:eaaz7095. doi: 10.1126/sciadv.aaz7095
83. Zhou Y, Su JM, Samuel CE, Ma D. Measles virus forms inclusion bodies with properties of liquid organelles. J Virol. (2019) 93:e00948-19. doi: 10.1128/JVI.00948-19
84. Heinrich BS, Maliga Z, Stein DA, Hyman AA, Whelan S. Phase transitions drive the formation of vesicular stomatitis virus replication compartments. Mbio. (2018) 9:e02290-17. doi: 10.1128/mBio.02290-17
85. Gao B, Gong X, Fang S, Weng W, Wang H, Chu H, et al. Inhibition of anti-viral stress granule formation by coronavirus endoribonuclease nsp15 ensures efficient virus replication. PloS Pathog. (2021) 17:e1008690. doi: 10.1371/journal.ppat.1008690
86. Hidalgo P, Pimentel A, Mojica-Santamaria D, von Stromberg K, Hofmann-Sieber H, Lona-Arrona C, et al. Evidence that the adenovirus single-stranded DNA binding protein mediates the assembly of biomolecular condensates to form viral replication compartments. Viruses. (2021) 13:1778. doi: 10.3390/v13091778
87. Metrick CM, Koenigsberg AL, Heldwein EE. Conserved outer tegument component UL11 from herpes simplex virus 1 is an intrinsically disordered, RNA-binding protein. Mbio. (2020) 11:e00810-20. doi: 10.1128/mBio.00810-20
88. Seyffert M, Georgi F, Tobler K, Bourqui L, Anfossi M, Michaelsen K, et al. The HSV-1 transcription factor ICP4 confers liquid-like properties to viral replication compartments. Int J Mol Sci. (2021) 22:4447. doi: 10.3390/ijms22094447
89. Boggs KB, Edmonds K, Cifuentes-Munoz N, El NF, Ossandon C, Roe M, et al. Human metapneumovirus phosphoprotein independently drives phase separation and recruits nucleoprotein to liquid-like bodies. Mbio. (2022) 13:e109922. doi: 10.1128/mbio.01099-22
90. Wu W, Cheng Y, Zhou H, Sun C, Zhang S. The SARS-CoV-2 nucleocapsid protein: its role in the viral life cycle, structure and functions, and use as a potential target in the development of vaccines and diagnostics. Virol J. (2023) 20:6. doi: 10.1186/s12985-023-01968-6
91. Zhao M, Yu Y, Sun LM, Xing JQ, Li T, Zhu Y, et al. GCG inhibits SARS-CoV-2 replication by disrupting the liquid phase condensation of its nucleocapsid protein. Nat Commun. (2021) 12:2114. doi: 10.1038/s41467-021-22297-8
92. Caruso IP, Dos SAV, Do AM, de Andrade GC, de Araujo GR, de Araujo TS, et al. Insights into the specificity for the interaction of the promiscuous SARS-CoV-2 nucleocapsid protein N-terminal domain with deoxyribonucleic acids. Int J Biol Macromol. (2022) 203:466–80. doi: 10.1016/j.ijbiomac.2022.01.121
93. Iserman C, Roden C, Boerneke M, Sealfon R, McLaughlin G, Jungreis I, et al. Specific viral RNA drives the SARS CoV-2 nucleocapsid to phase separate. Biorxiv. (2020) 12:2020.06.11.147199. doi: 10.1101/2020.06.11.147199
94. Luo L, Li Z, Zhao T, Ju X, Ma P, Jin B, et al. SARS-CoV-2 nucleocapsid protein phase separates with G3BPs to disassemble stress granules and facilitate viral production. Sci Bull (Beijing). (2021) 66:1194–204. doi: 10.1016/j.scib.2021.01.013
95. Lee N, Le Sage V, Nanni AV, Snyder DJ, Cooper VS, Lakdawala SS. Genome-wide analysis of influenza viral RNA and nucleoprotein association. Nucleic Acids Res. (2017) 45:8968–77. doi: 10.1093/nar/gkx584
96. Jung J, Seo E, Yoo RN, Sung H, Lee J. Clinical significance of viral-bacterial codetection among young children with respiratory tract infections: Findings of RSV, influenza, adenoviral infections. Med (Baltimore). (2020) 99:e18504. doi: 10.1097/MD.0000000000018504
97. Probst V, Datyner EK, Haddadin Z, Rankin DA, Hamdan L, Rahman HK, et al. Human adenovirus species in children with acute respiratory illnesses. J Clin Virol. (2021) 134:104716. doi: 10.1016/j.jcv.2020.104716
98. Risso-Ballester J, Galloux M, Cao J, Le Goffic R, Hontonnou F, Jobart-Malfait A, et al. A condensate-hardening drug blocks RSV replication in vivo. Nat. (2021) 595:596–99. doi: 10.1038/s41586-021-03703-z
99. Wagner LM, Lester JT, Sivrich FL, DeLuca NA. The N terminus and C terminus of herpes simplex virus 1 ICP4 cooperate to activate viral gene expression. J Virol. (2012) 86:6862–74. doi: 10.1128/JVI.00651-12
100. Tunnicliffe RB, Lockhart-Cairns MP, Levy C, Mould AP, Jowitt TA, Sito H, et al. The herpes viral transcription factor ICP4 forms a novel DNA recognition complex. Nucleic Acids Res. (2017) 45:8064–78. doi: 10.1093/nar/gkx419
101. Chau BA, Chen V, Cochrane AW, Parent LJ, Mouland AJ. Liquid-liquid phase separation of nucleocapsid proteins during SARS-CoV-2 and HIV-1 replication. Cell Rep. (2023) 42:111968. doi: 10.1016/j.celrep.2022.111968
102. Pappas PG, Lionakis MS, Arendrup MC, Ostrosky-Zeichner L, Kullberg BJ. Invasive candidiasis. Nat Rev Dis Primers. (2018) 4:18026. doi: 10.1038/nrdp.2018.26
103. Deutschman CS, Tracey KJ. Sepsis: current dogma and new perspectives. Immunity. (2014) 40:463–75. doi: 10.1016/j.immuni.2014.04.001
104. Zhang M, Zhu C, Duan Y, Liu T, Liu H, Su C, et al. The intrinsically disordered region from PP2C phosphatases functions as a conserved CO(2) sensor. Nat Cell Biol. (2022) 24:1029–37. doi: 10.1038/s41556-022-00936-6
105. Li Y, Peng J, Xia Y, Pan C, Li Y, Gu W, et al. Sufu limits sepsis-induced lung inflammation via regulating phase separation of TRAF6. Theranostics. (2023) 13:3761–80. doi: 10.7150/thno.83676
106. Gordon DE, Jang GM, Bouhaddou M, Xu J, Obernier K, White KM, et al. A SARS-CoV-2 protein interaction map reveals targets for drug repurposing. Nature. (2020) 583:459–68. doi: 10.1038/s41586-020-2286-9
107. Bouhaddou M, Memon D, Meyer B, White KM, Rezelj VV, Correa MM, et al. The global phosphorylation landscape of SARS-coV-2 infection. Cell. (2020) 182:685–712. doi: 10.1016/j.cell.2020.06.034
108. Duster R, Kaltheuner IH, Schmitz M, Geyer M. 1,6-Hexanediol, commonly used to dissolve liquid-liquid phase separated condensates, directly impairs kinase and phosphatase activities. J Biol Chem. (2021) 296:100260. doi: 10.1016/j.jbc.2021.100260
109. Wu Y, Ma L, Cai S, Zhuang Z, Zhao Z, Jin S, et al. RNA-induced liquid phase separation of SARS-CoV-2 nucleocapsid protein facilitates NF-kappaB hyper-activation and inflammation. Signal Transduct Target Ther. (2021) 6:167. doi: 10.1038/s41392-021-00575-7
110. Geiger F, Acker J, Papa G, Wang X, Arter WE, Saar KL, et al. Liquid-liquid phase separation underpins the formation of replication factories in rotaviruses. EMBO J. (2021) 40:e107711. doi: 10.15252/embj.2021107711
111. Yaron TM, Heaton BE, Levy TM, Johnson JL, Jordan TX, Cohen BM, et al. Host protein kinases required for SARS-CoV-2 nucleocapsid phosphorylation and viral replication. Sci Signal. (2022) 15:eabm808. doi: 10.1126/scisignal.abm0808
112. Alberti S, Hyman AA. Biomolecular condensates at the nexus of cellular stress, protein aggregation disease and ageing. Nat Rev Mol Cell Biol. (2021) 22:196–213. doi: 10.1038/s41580-020-00326-6
113. Iserman C, Roden CA, Boerneke MA, Sealfon R, McLaughlin GA, Jungreis I, et al. Genomic RNA elements drive phase separation of the SARS-coV-2 nucleocapsid. Mol Cell. (2020) 80:1078–91. doi: 10.1016/j.molcel.2020.11.041
114. Roden CA, Dai Y, Giannetti CA, Seim I, Lee M, Sealfon R, et al. Double-stranded RNA drives SARS-CoV-2 nucleocapsid protein to undergo phase separation at specific temperatures. Nucleic Acids Res. (2022) 50:8168–92. doi: 10.1093/nar/gkac596
115. Yu M, Peng Z, Qin M, Liu Y, Wang J, Zhang C, et al. Interferon-gamma induces tumor resistance to anti-PD-1 immunotherapy by promoting YAP phase separation. Mol Cell. (2021) 81:1216–30. doi: 10.1016/j.molcel.2021.01.010
116. Zhu G, Xie J, Fu Z, Wang M, Zhang Q, He H, et al. Pharmacological inhibition of SRC-1 phase separation suppresses YAP oncogenic transcription activity. Cell Res. (2021) 31:1028–31. doi: 10.1038/s41422-021-00504-x
117. Klein IA, Boija A, Afeyan LK, Hawken SW, Fan M, Dall’Agnese A, et al. Partitioning of cancer therapeutics in nuclear condensates. Science. (2020) 368:1386–92. doi: 10.1126/science.aaz4427
118. Sabari BR, Dall’Agnese A, Boija A, Klein IA, Coffey EL, Shrinivas K, et al. Coactivator condensation at super-enhancers links phase separation and gene control. Science. (2018) 361:eaar3958. doi: 10.1126/science.aar3958
119. Strzyz P. Drugs enter a liquid phase. Nat Rev Mol Cell Biol. (2020) 21:419. doi: 10.1038/s41580-020-0268-2
120. Fox AH, Nakagawa S, Hirose T, Bond CS. Paraspeckles: where long noncoding RNA meets phase separation. Trends Biochem Sci. (2018) 43:124–35. doi: 10.1016/j.tibs.2017.12.001
121. Guo Q, Shi X, Wang X. RNA and liquid-liquid phase separation. Noncoding RNA Res. (2021) 6:92–9. doi: 10.1016/j.ncrna.2021.04.003
122. Mukherjee S, Poudyal M, Dave K, Kadu P, Maji SK. Protein misfolding and amyloid nucleation through liquid-liquid phase separation. Chem Soc Rev. (2024) 53:4976–5013. doi: 10.1039/d3cs01065a
123. Wang X, Zhou X, Yan Q, Liao S, Tang W, Xu P, et al. LLPSDB v2.0: an updated database of proteins undergoing liquid-liquid phase separation in vitro. Bioinf. (2022) 38:2010–14. doi: 10.1093/bioinformatics/btac026
124. Ning W, Guo Y, Lin S, Mei B, Wu Y, Jiang P, et al. DrLLPS: a data resource of liquid-liquid phase separation in eukaryotes. Nucleic Acids Res. (2020) 48:D288–95. doi: 10.1093/nar/gkz1027
Keywords: liquid-liquid phase separation, membrane-less organelle, biomolecular condensates, respiratory diseases, lung
Citation: Wang L, Wang Y, Ke Z, Wang Z, Guo Y, Zhang Y, Zhang X, Guo Z and Wan B (2024) Liquid-liquid phase separation: a new perspective on respiratory diseases. Front. Immunol. 15:1444253. doi: 10.3389/fimmu.2024.1444253
Received: 05 June 2024; Accepted: 09 September 2024;
Published: 26 September 2024.
Edited by:
Fusheng Si, Shanghai Academy of Agricultural Sciences, ChinaReviewed by:
Chunyi Hu, National University of Singapore, SingaporeHao Zhang, Sun Yat-sen University, China
Copyright © 2024 Wang, Wang, Ke, Wang, Guo, Zhang, Zhang, Guo and Wan. This is an open-access article distributed under the terms of the Creative Commons Attribution License (CC BY). The use, distribution or reproduction in other forums is permitted, provided the original author(s) and the copyright owner(s) are credited and that the original publication in this journal is cited, in accordance with accepted academic practice. No use, distribution or reproduction is permitted which does not comply with these terms.
*Correspondence: Bing Wan, YmluZ3dhbjc2QDE2My5jb20=; Zhongliang Guo, ZHJndW96aGxAMTYzLmNvbQ==
†These authors have contributed equally to this work