- 1Research and Development Centre, Beijing Zhifei Lvzhu Biopharmaceutical Co., Ltd., Beijing, China
- 2Beijing Bacterial Vaccine Engineering Research Centre, Beijing, China
Tuberculosis (TB) remains one of the gravest global health challenges. Mycobacterium tuberculosis (M. tuberculosis), the causative agent, employs sophisticated immune evasion and pathogenesis strategies. Its capability to thrive within immune cells and incite robust inflammatory responses prolongs infection and dissemination. Mycobacterial advanced adaptations facilitate navigation through the human immune system and present a variable antigenic profile throughout different infection stages. Investigating these strategies unfolds targeted approaches to effective vaccine development against TB. This review delves into the most advanced and exhaustive insights into the immune evasion tactics and pathogenic processes of M. tuberculosis across various infection stages. The knowledge distilled from this analysis holds the promise of guiding the creation of innovative TB vaccines and translating theoretical groundwork into practical immunological defenses.
Introduction
In the landscape of global health threats, TB, driven by the virulent M. tuberculosis, firmly stood as a leading cause of infectious mortality, second only to the COVID-19 pandemic in 2022 (1). Despite concerted public health efforts, TB continues to afflict over 10 million individuals annually (1), underscoring the disease’s persistence and reach. The concerning prevalence of latent TB infection, impacting approximately 25% of the global population, exemplifies the insidious nature of M. tuberculosis and the daunting challenge it poses (2). Currently, the Bacillus Calmette-Guérin (BCG) vaccine is the sole prophylactic measure authorized for TB, yet it provides limited protection in the adult population. The discrepancy between the vast scale of infection and the constrained efficacy of existing vaccines and diagnostics highlights a compelling need for rigorous scientific inquiry into novel detection and prevention modalities. There is a paramount need for the development and deployment of innovative, effective strategies that enhance our capacity to accurately identify M. tuberculosis infections and fortify our preventive measures. Embracing novel diagnostic technologies, alongside a fervent pursuit of vaccine research that aims to transcend the constraints of current options, is indispensable.
M. tuberculosis, the etiological agent of TB, manifests a complex array of biological traits that critically impede its prevention and management. Notably, M. tuberculosis is characterized by a slow replication rate, typically requiring 24 hours to divide in artificial culture media or within diseased animal models, which is an attribute that underpins TB’s chronicity and necessitates comprehensive, long-term treatment regimens. This inherent slowness of growth presents a substantial hurdle for researchers and clinicians alike. Furthermore, M. tuberculosis has evolved the capacity for dormancy. Within the infected tissues, it can enter a state of metabolic stasis induced by the host immune response. While this dormancy curtails the progression of active infection, it is insufficient for pathogen eradication. Significantly, this latent reservoir of bacteria can become reactivated and pathogenic once host immunity diminishes due to either the natural aging process or immunosuppression, often culminating in a resurgence of the disease. This dynamic has positioned TB prevention and treatment at the forefront of modern medical challenges. Central to the endeavor of combating TB is the elucidation of the mechanisms underpinning immune recognition and response to M. tuberculosis. A sophisticated comprehension of infection dynamics, immune cell functionality, enhancement of host immune defenses, and the subterfuges M. tuberculosis employs to escape immune surveillance is imperative. This review delineates current knowledge about the immunological landscape of TB, with an emphasis on delineating the complex life cycle of M. tuberculosis, its pathogenic processes, and sophisticated immune evasion strategies. It also concentrates on the imperative of TB vaccine development, spotlighting the identification of immunodominant antigens as a cornerstone strategy for pioneering therapeutics.
Earliest events of M. tuberculosis infection
TB transmission is primarily facilitated by aerosols containing M. tuberculosis, expelled into the air by individuals with active TB during activities such as coughing, sneezing, or speaking (3). The potential for M. tuberculosis to transmit from human to animal has also been documented, with an early account by Hermann Tappeiner in Germany, who in 1878 described the transmission of TB to a dog via inhalation of aerosolized sputum from a TB patient (4). This observation by Tappeiner is possibly the earliest recorded evidence of the transmission route of M. tuberculosis (4). M. tuberculosis is notably highly infectious, with the infectious dose likely being as low as a single organism (5).
Upon inhalation, M. tuberculosis encounters the alveolar lining fluid, a critical component of the lung’s innate defenses. This fluid is rich in soluble factors, including antimicrobial hydrolytic enzymes (6), which can disrupt the M. tuberculosis cell envelope. The presence of both M. tuberculosis and its envelope fragments can enhance the bactericidal activity of macrophages (7). Additionally, these hydrolases can degrade several key components of the cell envelope, such as the carbohydrates arabinose, mannose (Man), and glucose (Glc), among others (6). These carbohydrates play a crucial role in the recognition of M. tuberculosis by macrophages, the regulation of mycobacterial intracellular survival, and the overall pathogenesis of TB (8, 9).
Treatment of M. tuberculosis with alveolar lining fluid has been shown to significantly reduce interactions between the bacterium and macrophages, as well as restrict the intracellular proliferation of the bacteria within macrophages (6, 10). However, this reduced interaction and recognition by macrophages might paradoxically increase the pathogenicity of M. tuberculosis. Intriguingly, the survival capability of M. tuberculosis does not appear to be compromised by the absence of its cell envelope, and M. tuberculosis treated with alveolar lining fluid demonstrates a marginally faster growth rate compared to untreated cells (6).
M. tuberculosis invasion of alveolar epithelial cells
Infection occurs in alveolar epithelial cells within 48 hours. In the early stages of infection, M. tuberculosis demonstrates a specific affinity for invasion and replication within alveolar epithelial type II cells (ATII) typically within 48 hours post-inhalation (11–14). These cells, although non-phagocytic by nature, may inadvertently provide a conducive environment for bacterial proliferation, essentially serving as a sanctuary that facilitates M. tuberculosis growth (15, 16). Moreover, this localization potentially allows M. tuberculosis to gain direct entry into the host’s lymphatic and circulatory systems, bypassing the need for transport by carrier macrophages (12, 17).
The involvement of a heparin-binding hemagglutinin (HBHA) expressed by M. tuberculosis has been proposed to mediate the pathogen’s adherence and subsequent internalization into these nonprofessional phagocytes (16). Investigative work by Pethe et al. demonstrated the functional significance of HBHA; deletion of the hbhA locus in M. tuberculosis did not impair bacillary growth per se but resulted in a significant reduction in the invasion of alveolar epithelial cells, while phagocytic interactions remained unaltered (18). Additionally, though equivalent colonization rates were observed in the lungs of mice infected with wild-type and ΔhbhA M. tuberculosis strains, the latter exhibited a profound deficit in dissemination to distal organs (18).
Post-entry into the alveolar epithelial cells, M. tuberculosis propagation ensues (12, 13, 17, 19). There are four posited mechanisms by which inhaled M. tuberculosis may incite pulmonary infection and orchestrate systemic spread (refer to Figure 1) (16). The first model involves the mycobacteria directly binding and entering alveolar epithelial cells, which then lyse to cause tissue damage and an inflammatory reaction. This process aids in the spread of the bacteria into the bloodstream. The second scenario entails the bacteria attaching and entering into alveolar epithelial cells, transcytosis through the epithelial cells, and being delivered to macrophages or accessing the blood vessels themselves. The third theory proposes that after M. tuberculosis infects alveolar macrophages, the infected macrophages move into the lung interstitium, where they trigger an inflammatory response that draws in more immune cells (16). Additionally, dendritic cells (DCs) have been speculated to play an instrumental role in mycobacterial dispersal. Research by Reljic et al. underscores that DCs migrate into the pulmonary system and phagocytose M. tuberculosis in less than 48 hours (20), thereafter relocating to lung-draining lymph nodes, priming T cells for antigen recognition (21, 22).
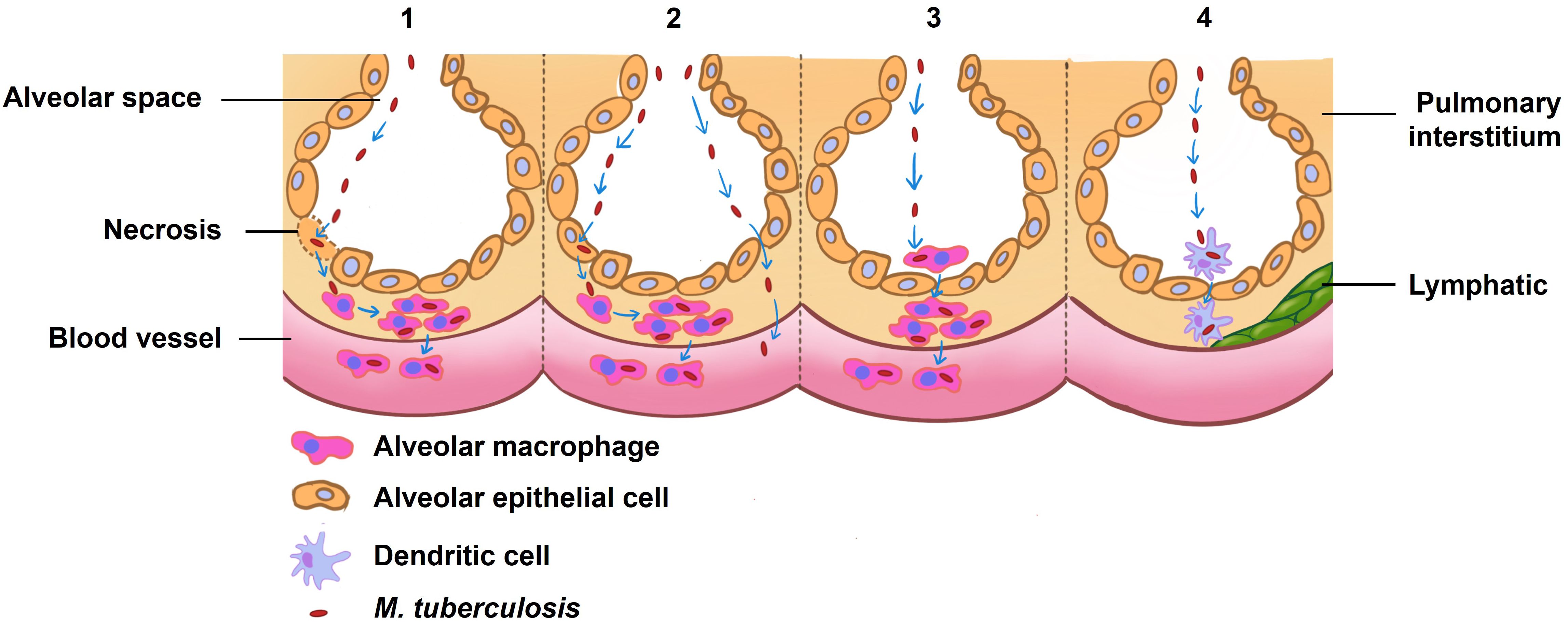
Figure 1 Theories of how inhaled M. tuberculosis might cause an infection in the lung and spread to other areas. The first proposed model delineates a direct binding of M. tuberculosis to alveolar epithelial cells, culminating in cellular lysis, which triggers an inflammatory cascade and consequent tissue damage, thereby paving a pathway for hematogenous spread. In an alternate scenario, M. tuberculosis gains entry into alveolar epithelial cells and, through transcytosis, traverses these cells to either directly invade macrophages or access the vasculature. A third hypothesis posits that alveolar macrophages, upon phagocytosing M. tuberculosis, migrate to the pulmonary interstitium and vascular systems, serving as vectors for the pathogen’s mobility. The final conjecture suggests that dendritic cells resident in the alveoli may capture M. tuberculosis and subsequently transport it to proximal lymph nodes. Collectively, these models underpin our understanding of the initial pulmonary infection dynamics and the potential systemic spread of M. tuberculosis, crucial for informing the development of targeted interventions.
For successful infection establishment, mycobacteria must circumvent anti-bacterial monocyte responses. A genetic variant screen of Mycobacterium marinum identified the cell-surface lipid phthiocerol dimycocerosate (PDIM) as vital for eluding such responses (23). PDIM can infiltrate the alveolar epithelial barrier and recruit permissive monocytes—their movement is orchestrated by the chemokine CCL2, distinct from the Toll-like receptor/myeloid differentiation factor 88 (TLR/Myd88)-dependent monocytes typified by anti-bacterial attributes (24). The unique methyl-branched fatty acids present in PDIM are also thought to increase lipid fluidity within membranes, thereby promoting bacterial dissemination (24).
Alveolar macrophages create an early niche for M. tuberculosis
Fourteen days following aerosol exposure, alveolar macrophages (AMs) emerge as the primary reservoirs of M. tuberculosis within the pulmonary tissue of murine models (25). These macrophages are not mere passive hosts; rather, they are pivotal in shuttling M. tuberculosis from the alveoli to the lung interstitium and subsequently to monocytes, which are amenable to bacillary replication. Research indicates that the eradication of AMs correlates with a marked reduction in mycobacterial burden (26, 27).
The transition of M. tuberculosis-infected AMs from the alveolar space into the lung interstitium is contingent upon two critical elements: MyD88/IL-1 signaling and the ESX-1 secretory system (25). Interleukin-1 (IL-1), a versatile cytokine, is implicated in the modulation of vascular permeability, angiogenesis, and the inflammatory response to infectious agents (28, 29). In the context of TB infection, IL-1 has been identified as having an intricate protective function. MyD88 serves as a universal adaptor molecule that connects the IL-1 receptor and Toll-like receptors (TLRs) to downstream signaling pathways. Activation of MyD88/IL-1 signaling in alveolar epithelial cells contributes to enhanced alveolar permeability, thereby allowing the influx of immune cells such as neutrophils and facilitating the transit of infected AMs across the alveolar epithelium into the lung interstitium (25).
The ESX-1 system, encoded by the region of difference 1 (RD1) locus of M. tuberculosis, has been characterized as a virulence determinant essential for phagosomal escape, enabling the bacteria to gain access to the macrophage cytosol (30). Early secreted Ag of 6 kDa (ESAT-6), one of the components secreted by the ESX-1 system, augments the migration of M. tuberculosis from the phagosome to the cytoplasm (30–33). The cytosolic presence of ESAT-6 is implicated in the activation of the NLRP3 inflammasome complex, including ASC and caspase-1, a process instrumental for the liberation of IL-1 (Figure 2) (32). Concurrently, there is evidence suggesting that the activation of NLRP3 could facilitate further bacterial egress from the phagosome by instigating necrotic cell death, a phenomenon that appears to be TLR2-MyD88 dependent (33–35).
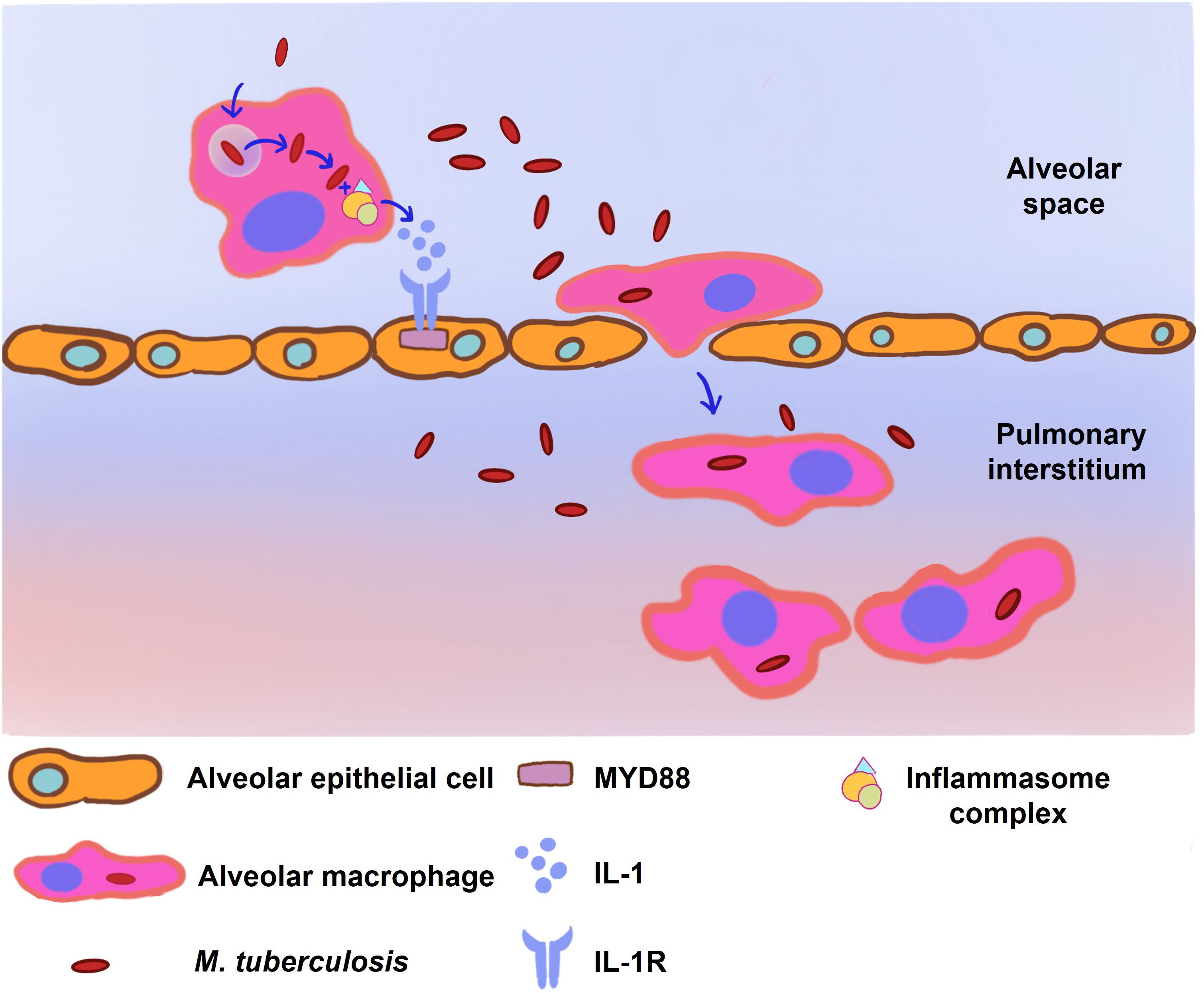
Figure 2 The infected alveolar macrophages (AM) stimulate the MyD88/IL-1 signaling pathway to facilitate their translocation to the pulmonary interstitium. Following M. tuberculosis infection, the ESX-1 secretion system of M. tuberculosis orchestrates the disruption of phagosomal membranes within the AM, a critical event facilitating mycobacterial entry into the macrophage cytoplasm. This invasion triggers the assembly of the NLRP3, ASC, and caspase-1 inflammasome complex, resulting in a surge of cytokine production, notably interleukin-1 (IL-1). IL-1, in turn, activates the MyD88/IL-1 signaling cascade in neighboring alveolar epithelial cells, leading to enhanced alveolar permeability. The culmination of these molecular events facilitates the migration of the infected AMs from the alveolar space into the pulmonary interstitium, underscoring a crucial step in the progression of M. tuberculosis infection within the lung parenchyma.
Eventually persistent infection develops in the pulmonary interstitium
M. tuberculosis invasion of macrophages
As mycobacterial infection progresses to the pulmonary interstitium, a pivotal phase in the establishment of a persistent infection unfolds through its invasion of macrophages. The infiltration of AMs into the lung interstitium results in the subsequent recruitment and infection of additional macrophage populations. To counteract M. tuberculosis invasion, macrophages deploy a repertoire of defense strategies, including phagosome-lysosome fusion to degrade pathogens, recruitment of hydrolytic lysosomal enzymes, production of reactive oxygen species (ROS) and reactive nitrogen species (RNS), antigen presentation, upregulation and mobilization of major histocompatibility complex class II (MHC class II) molecules, autophagy, and induction of apoptosis (36). Studies have shown that selective ablation of distinct macrophage subtypes escalates the pulmonary M. tuberculosis load in murine models, underscoring the pivotal role of monocyte-derived macrophages in containing M. tuberculosis (26).
While AMs are often the initial phagocytes to encounter M. tuberculosis, their ability to impede intracellular M. tuberculosis proliferation seems compromised compared to interstitial macrophages (IMs), which exhibit a more robust bacteriostatic effect (26, 27). M. tuberculosis exhibits a higher replication rate and a reduced stress response within AMs. Bacterial burden is decreased when AM is depleted, whereas it is elevated when IM is depleted, highlighting macrophages’ differential roles and reiterating the importance of IMs in infection control (26). Notably, IMs possess more enduring stability and a shorter lifespan than AMs (37).
Divergent metabolic pathways are implicated in the variations observed in the bactericidal capabilities of different macrophage populations. Transcriptomic analyses reveal that AMs exhibit augmented fatty acid uptake and β-oxidation, whereas IMs are predominantly glycolytic (26). Given that M. tuberculosis intracellularly harvests cholesterol and fatty acids from the host cell, it’s plausible that the metabolic pathways active in AMs confer a nutritive edge to M. tuberculosis (38–41). Additionally, the interplay between macrophages and CD4+ T cells is critical for the containment of M. tuberculosis. It is suggested that macrophages exerting a restrictive impact on M. tuberculosis are those that have engaged effectively with CD4+ T cells, emphasizing the reliance of macrophage-mediated control of M. tuberculosis on this cellular interaction.
Strategies of M. tuberculosis for immune evasion
M. tuberculosis employs a suite of sophisticated strategies to evade the host immune system, enabling the pathogen to sustain a chronic infection despite the robust inflammatory response mounted by IMs (refer to Figure 3 and Table 1 for an overview of evasion mechanisms). Upon phagocytosis, M. tuberculosis faces an oxidative onslaught, as macrophages initiate a respiratory burst aiming to obliterate the bacilli. Remarkably, M. tuberculosis demonstrates resilience against DNA damage induced by oxidative stress, succumbing only under conditions of extreme oxidative stress (91). An alternative sigma factor, SigH, plays a pivotal role in enabling M. tuberculosis to re-establish oxidative equilibrium following exposure to oxidative stress (79). During the macrophage respiratory burst, reactive oxygen species (ROS) and nitrogen intermediates are generated. The robust cell wall of M. tuberculosis efficiently mitigates ROS damage (97), and the enzymes katG and trxB2—whose expression is significantly upregulated in response to H2O2 and NO—contribute to mycobacterial resistance against oxidative reactions (91, 98).
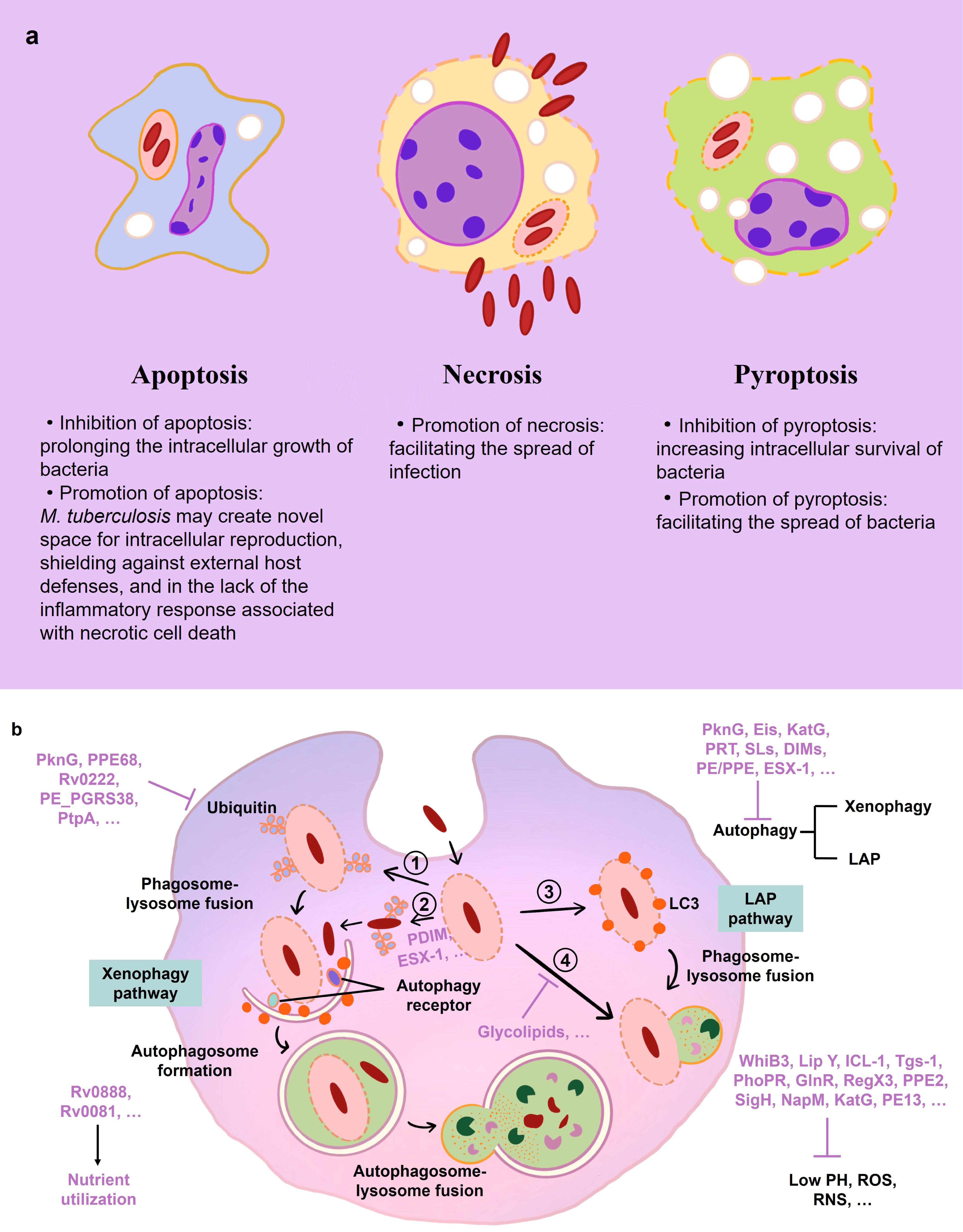
Figure 3 Immune events in macrophage during M. tuberculosis infection. (A) Mechanisms of host cell death during M. tuberculosis infection. (B) Mechanisms of mycobacterial immune evasion in macrophage. M. tuberculosis is absorbed in phagosomes. WhiB3, Lip Y, ICL-1, and other M. tuberculosis factors (purple) aid in the bacilli’s resistance to low pH, ROS, and RNS. PDIM and ESX-1 encourage phagosomal damage. (1, 2) Ubiquitin (Ub) ligases bind the phagosome and M. tuberculosis to attract autophagy receptors, which is blocked by PknG, PPE68 and other factors. The phagophore membrane interaction of LC3 and its subsequent fusion with the lysosome directs M. tuberculosis toward xenophagy. (3) The LC3-associated phagocytosis (LAP) pathways are involved in the macrophage autophagy-mediated elimination of M. tuberculosis. (4) Mycobacteria-containing phagosomes fuse to lysosomes, which is inhibited by the glycolipids of M. tuberculosis. Rv0888 and Rv0081 help M. tuberculosis with the utilization of nutrients in macrophage.
The disruption of phagolysosomal fusion, a seminal strategy utilized by M. tuberculosis, was documented as early as 1971 (99). M. tuberculosis disrupts cellular compartment integrity, including macrophage membranes, phagosomes, and phagolysosomes, through mechanisms dependent on ESX-1 and PDIM (42, 86, 87, 100). ESX-1, part of the M. tuberculosis-specific type VII secretion system (encompassing ESX-1–5), plays a crucial role in this process (87, 101). The type VII secretion system’s impairment is associated with the attenuation observed in the Mycobacterium bovis BCG strain (30, 101, 102). Membrane perforation is facilitated by the primary effector of the ESX-1 system, ESAT-6 (ESX-A), and its substrate, CFP-10 (ESX-B), while ESX system components ESX-3, ESX-H, and ESX-G prevent phagolysosome maturation (103–107).
Furthermore, M. tuberculosis manipulates autophagy and cell death pathways to evade innate immune detection (108–114). Autophagy, the process by which cytoplasmic components are degraded or recycled, is inhibited by members of the ESX-1 system, permitting mycobacterial escape into the cytoplasm (88, 115). ESX-A (ESAT-6) is implicated in inducing necrosis in infected host cells, a mechanism that facilitates bacterial dissemination and hinders macrophage containment of the infection (89, 103, 116). Consequently, M. tuberculosis has evolved multiple evasion tactics, including oxidative stress resistance, inhibition of phagolysosome maturation, escape into the cytosol, and manipulation of apoptosis or necrosis pathways, to transcend innate immune defenses and foster an environment conducive to its latent propagation.
Neutrophils-mediated immunoreaction during M. tuberculosis infection
During M. tuberculosis infection, the host’s immune system mobilizes different myeloid lineage cells to combat the pathogen, including neutrophils, DCs, and various macrophage subsets, aside from AM. Neutrophils, known for their short lifespan, stand at the forefront of the host’s innate immune defenses as professional phagocytes (117). Although neutrophils are activated by M. tuberculosis and employ a range of antimicrobial effector mechanisms, these efforts fall short in controlling the infection. Instead, the interaction between neutrophils and M. tuberculosis frequently culminates in necrotic cell death of the neutrophils. Subsequently, these necrotic cells are phagocytosed by macrophages, a process which paradoxically fosters the proliferation of M. tuberculosis and precipitates the recruitment of additional neutrophils to the site of infection (118, 119).
Adaptive immunity to M. tuberculosis
Following the initial implantation of bacteria into the pulmonary system, the priming of adaptive immune responses in the lung-draining lymph nodes typically commences within a two-week period. This pivotal process is subsequently followed by the infiltration of adaptive immune cells into lung tissue, which becomes actively engaged in combating the infection over the course of an additional one to two weeks. The initiation and orchestration of adaptive immune responses are fundamentally dependent on the early intervention of the innate immune system, underscoring its critical role in the host’s defense mechanism against M. tuberculosis infection.
Cellular immunity in M. tuberculosis infection
In the investigation of cellular immunity against M. tuberculosis infection, Wolf et al. illuminated the critical role of DCs after exposing mice to GFP-expressing M. tuberculosis. They found that DCs, which are professional antigen-presenting cells (APCs), were frequently infected in the lungs and migrated to the draining lymph nodes (120). These cells are instrumental in eliciting adaptive immunity by presenting M. tuberculosis antigens via MHC-II and co-stimulatory molecules, thus initiating antigen-specific T cell responses.
The essential role of MHC-II-restricted CD4+ T cells in mounting a defense against tuberculosis becomes evident in individuals with HIV-related CD4+ T cell impairments, who exhibit a higher susceptibility to the disease (121). This significance is further underscored by studies utilizing CD4+ T cell depletion in non-human primates (NHP) and MHC-II deletion in mice (122, 123). While CD8+ T cells are vital for the immunological response to M. tuberculosis, their presence cannot compensate for the deficiency of CD4+ T cells (122). Mice deficient in the Th1-polarizing cytokine interleukin-12 (IL-12) or the transcription factor T-bet, responsible for specifying the Th1 lineage, succumb shortly after M. tuberculosis exposure, highlighting the dependence of host resistance to mycobacterial infection on Th1-oriented CD4+ T cells (124, 125). The arrest in bacterial growth is achieved when T cells, attracted to the lungs, interact with infected cells, recognize the antigens, and activate myeloid cells to halt the replication of ingested bacteria (125–127).
The study of individuals afflicted by diseases caused by less virulent mycobacteria reveals that genetic mutations impairing the IL12/IFN-γ pathway predispose individuals to mycobacterial infections (128). The pivotal effector mechanism of cell-mediated immunity is believed to be activated macrophages by type-1 Interferon γ (IFN-γ). This cytokine, primarily produced by natural killer (NK) and Th1 cells, is regulated by IL-12, secreted by dendritic cells and macrophages. IFN-γ, in concert with tumor necrosis factor α (TNF-α), triggers the microbicidal activities of macrophages essential for eliminating the intracellular pathogen.
CD8+ T cells also play a crucial protective role against mycobacterial infections. Mice lacking either TAP-1 (transporter associated with antigen processing 1) or β2 microglobulin, crucial components for MHC-I antigen presentation, display impaired CD8+ T cell responses and succumb more rapidly post-M. tuberculosis infection compared to wild-type controls (129, 130). Mott et al. have shown that high intracellular levels of M. tuberculosis induce macrophage death (131). This allows other macrophages to scavenge the resulting cellular debris and indirectly present the TB10.4 antigen to CD8+ T cells. CD8+ T cells’ ability to produce cytolytic effector molecules, such as perforin alongside IFN-γ and TNF-α, to lyse M. tuberculosis-infected macrophages, is vital for curbing bacterial proliferation (130). Moreover, CD8+ T cells’ capacity to release granulysin for direct bacilli destruction within macrophages underscores their importance in the immune response to M. tuberculosis (130, 132). Assistance from CD4+ T cells mitigate CD8+ T cell exhaustion and enhances their functional activities. The collaborative action of CD4+ and CD8+ T cells improve the survival outcomes of infected mice (133).
Strategies of M. tuberculosis for cellular immune evasion
M. tuberculosis employs a myriad of strategies to circumvent cellular immune defense mechanisms, resulting in substantial bacterial loads in the lungs even in the presence of robust adaptive immune responses. This evasion of cellular immunity is intricately illustrated in Figure 4 and Table 2. The transport of M. tuberculosis from the pulmonary site to proximal draining lymph nodes is facilitated by DCs, which play a pivotal role in T cell activation during the infection (154). Instead of adhering to the canonical MHC-II antigen presentation pathway, infected DCs exploit a kinesin-2 dependent mechanism for antigen conveyance, thereby impairing the efficacy of CD4+ T cell stimulation (134). M. tuberculosis further undermines immune defenses by producing effector molecules that derail DC phagosome maturation and integrity, significantly hampering T cell priming.
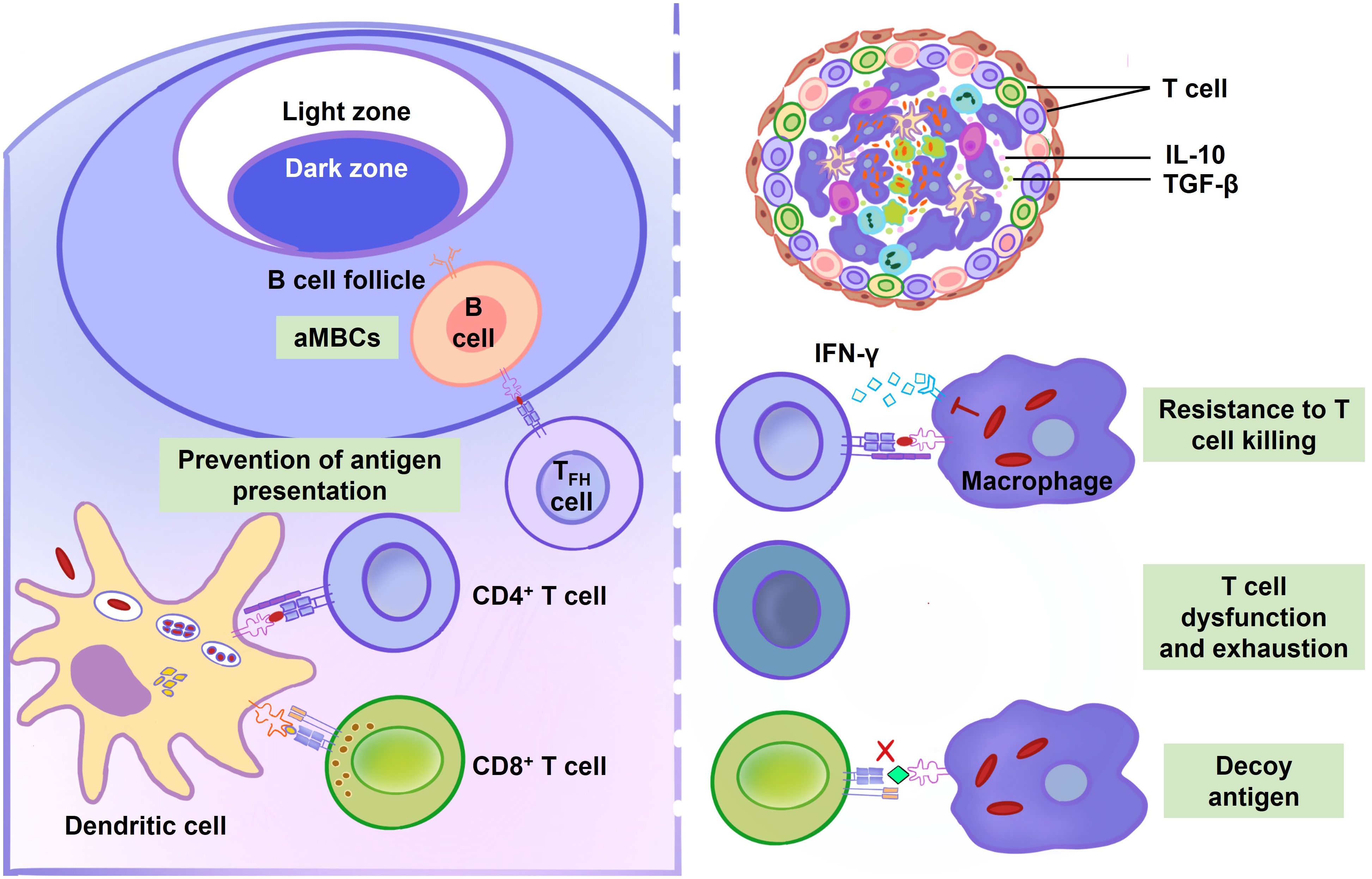
Figure 4 Adaptive immune response during M. tuberculosis infection. Dendritic cells (DCs) exhibit a delayed migration and antigen presentation to lymph nodes after M. tuberculosis infection. Compared to uninfected DCs, infected DCs are less efficient in stimulating T cells. GroEL2, PDIM and MPT64 suppress the maturation and activation of DCs. By preventing antigen presentation, EsxH, PE_PGRS47, 19-kDa lipoprotein, TDM, and NuoG postpone T cell activation. B cell function is also compromised during M. tuberculosis infection. Atypical memory B cells (aMBCs) express a variety of inhibitory receptors and resist antigen stimulation, making it difficult to stimulate them to replicate or release cytokines or antibodies. The proliferation of aMBCs obstructs the establishment of TB immunity. Additionally, it is difficult for T cells killing in granulomas because they are located far from infected center. IL-10 and transforming growth factor-β (TGF-β) play a role in the granuloma’s localized T cell suppression. Tryptophan can be produced by M. tuberculosis in order to resist T cells killing. Mycobacterial lipoglycans, mycolic acids, MPT70 and ESAT-6 cause T cells dysfunction and exhaustion. M. tuberculosis selectively elicits T cell responses to decoy antigens, which is poorly presented by infected cells.
Saini et al., through genome-wide analysis, spotlight the mycobacterial PE_PGRS47 protein as an autophagy inhibitor that constrains the delivery of MHC-II-restricted antigen by M. tuberculosis-infected dendritic cells (139). Additional hurdles, such as the pathogen’s slow replication rate, its inhibition of host cell apoptosis, and the sluggish activation and migration of DCs, collectively contribute to the delayed initiation of adaptive immunity. These elements synergistically facilitate the establishment and persistence of M. tuberculosis infection within the lungs.
Despite prompt lung infiltration by antigen-specific T cells, their attempts to clear the infection are thwarted by M. tuberculosis through multiple mechanisms. Effective suppression of intracellular M. tuberculosis necessitates the direct recognition of infected cells by CD4+ T cells (155). Yet, the physical separation between T cells and their infected targets often presents a significant obstacle. Furthermore, membrane vesicles containing M. tuberculosis cell envelope lipoglycans, released by infected macrophages, can impede CD4+ T cell activation, as evidenced by diminished interleukin-2 (IL-2) secretion and impaired T cell proliferation (145).
Another challenge arises when CD4+ T cells encounter consistently expressed antigens like ESAT-6, leading to their proliferation within the lung parenchyma and subsequent functional exhaustion or progression towards terminal differentiation, diminishing their defensive capabilities (144). M. tuberculosis strategically redirects T cell focus towards immunodominant, yet nonprotective antigens that are ineffectively presented by infected cells (148).
In summation, the adaptive immune response against M. tuberculosis is significantly delayed and inadequate, characterized by an orchestrated evasion strategy that leverages both the manipulation of antigen presentation and the impairment of T cell function, thereby enabling the pathogen’s sustained survival within the host.
Humoral immunity in M. tuberculosis infection
An increasing body of research suggests that antibodies and B cells may confer protection against M. tuberculosis infection (156–162). The role of humoral immunity in the context of M. tuberculosis infection has been substantiated by a plethora of serological studies, which reveal that despite being an intracellular bacterium, M. tuberculosis elicits a comprehensive humoral response against a diverse array of mycobacterial antigens in humans (163). Animal models deficient in humoral immunity and B cell function exhibit increased susceptibility to tuberculosis infection, underscoring the importance of these immune components (161, 164–167). Additionally, the passive transfer of monoclonal antibodies targeting M. tuberculosis has been shown to ameliorate infections in mice, further supporting their protective role (168–180). In humans, elevated titers of certain IgG are typically observed in tuberculosis patients. However, the absence of galactose in the carbohydrates linked to the Fc region of IgG impairs the protective function of these antibodies, as demonstrated by Olivares et al., where mice treated with deglycosylated IgG did not exhibit protection (181). This finding indicates that IgG glycosylation is necessary for immunoglobulin to confer a protective effect against TB in mice.
Antibody-mediated opsonization is believed to confer protective benefits at the onset of infection. This is predominantly achieved through the enhancement of mycobacterial antigen uptake by phagocytic cells (160). The process is facilitated via Fc receptor (FcR)-mediated phagocytosis, which subsequently leads to an increase in intracellular pathogen elimination (182).
In addition to providing direct assistance with phagocytosis, antibodies can modulate the complement system, thereby catalyzing inflammation and further enhancing phagocytic activity (183). During complement receptor-mediated phagocytosis of M. tuberculosis, altered Ca2+ signaling has been observed. The suppression of this Ca2+ signaling pathway by M. tuberculosis indicates that such alterations in macrophage activation significantly contribute to the inhibition of phagosome-lysosome fusion, consequently promoting the intracellular survival of the mycobacteria (184).
Furthermore, antibodies targeting the cell-surface lipopolysaccharide of M. tuberculosis may expedite pathogen clearance, thereby attenuating the possibility of detrimental interference with immune responses. The mycobacterial surface material lipoarabinomannan (LAM) has been associated with several detrimental effects on immune system function. In mice, LAM-binding antibodies significantly reduced the amount of LAM deposited in the spleen, suggesting that these antibodies may play a role in altering the course of mycobacterial infection (171, 185).
Antibodies carry with them the dual capability to either dampen or amplify inflammatory responses due to their constituents of pro- and anti-inflammatory molecules (186). This multifaceted functionality permits antibodies to regulate the intensity of the immune response, potentially mitigating the tissue-damaging consequences of uncontrolled granuloma—a process intricately described in subsequent sections. Pro-inflammatory antibodies can assist hosts with insufficient inflammatory responses, while anti-inflammatory antibodies may benefit hosts experiencing excessive inflammation that leads to tissue damage (156).
In summary, the humoral immune response against M. tuberculosis is marked by a multilayered mechanism involving phagocytic enhancement, complement activation, pathogen clearance, and modulation of the inflammatory milieu. This intricate interplay between various antibody-mediated activities plays an essential role in shaping the course and outcome of M. tuberculosis infection.
Strategies of M. tuberculosis for humoral immune evasion
Recent investigations have unveiled novel strategies by which M. tuberculosis subverts humoral immunity, particularly impacting the functional capacity of certain B lymphocytes—a phenomenon comprehensively illustrated in Figure 4 and Table 2. These B cells manifest a compromised state evidenced by their diminished cytokine secretion, immunoglobulin production, and proliferative ability (152). This dysfunctional B cell phenotype, frequently termed ‘atypical’, has been observed in the milieu of various infectious diseases, notably chronic or recurrent infections such as malaria, and infections caused by hepatitis B and C viruses, as well as HIV (153, 187, 188).
The role of atypical B cells within the immune landscape remains an enigma, yet they are hypothesized to exert detrimental effects on pathogen-specific immune responses. The concerns pivot around the observed elevated concentrations of these cells during infection, which may signify a potential hindrance to the efficacy of the humoral response (153). As such, mycobacterial ability to induce and sustain the proliferation of atypical B cells represents another facet of its immune evasion repertoire, possibly allowing the pathogen to persist by attenuating the protective functions typically mediated by conventional B lymphocytes.
Formation of the granuloma
The formation of the granuloma constitutes a hallmark histological feature of tuberculosis infection. Granulomas are structured aggregates predominantly comprised of both infected and uninfected macrophages at various stages of differentiation (as depicted in Figure 5) (189–191). Within these complex formations, macrophages undergo epithelioid transformation, become lipid-laden foamy cells, or coalesce into multinucleated giant cells. This central macrophage hub is encircled by a heterogeneous population of immune cells including neutrophils, DCs, T cells, B cells, and fibroblasts. Gradual development of hypoxia within the granuloma culminates in necrotic death of cells, leading to the formation of a necrotic cell-free zone known as the caseum (192).
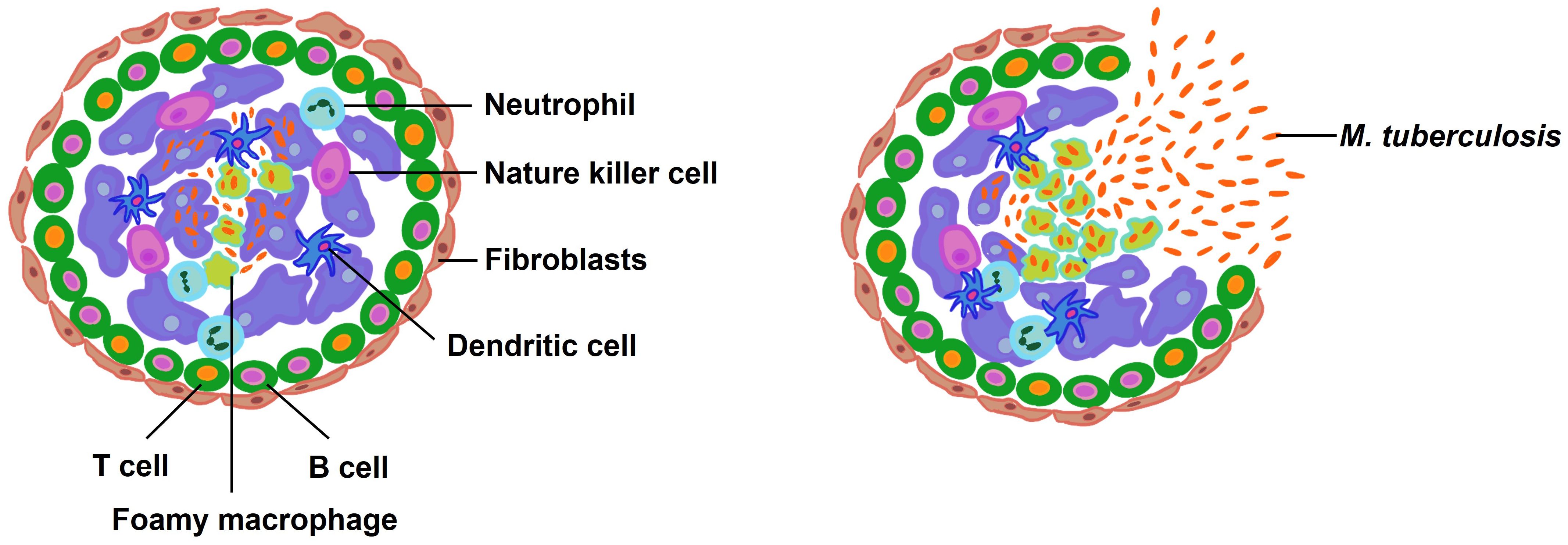
Figure 5 The composition and rupture of granuloma. Macrophages consume the bacilli when they enter the lung. In order to sterilize the infected macrophages, more immune cells are enlisted, which causes the granuloma formation. Latent infection persists in healthy individuals but reactivation is possible. When M. tuberculosis is reactivated for some reason, the bacteria multiply and the bacterial load rises to an unmanageable level. At this point, the granuloma bursts, releasing the bacteria into the airways.
Granulomas serve a dual role; they are essential host defense structures that contain the bacterial spread and also provide a niche wherein M. tuberculosis can reside and potentially persist in a dormant state until conditions favor reactivation and proliferation. While the immune response helps to eradicate bacteria from some granulomas, in others, the bacteria can survive and proliferate. Early granulomas with high bacterial loads are influenced by mast cells, type 2 immunity, and tissue remodeling. In contrast, low-burden, late-forming granulomas are associated with a response involving cytotoxic T cells and type 1-type 17 immunity (193).
Immunologically, granulomas are characterized by an inhibitory milieu, with the immune-regulatory cytokine IL-10 implicated in compromising T cell activity within this environment (149, 194, 195). Moreover, T cell suppression within the granuloma is further enhanced by transforming growth factor-β (TGF-β). Disruption of TGF-β signaling in T cells has been shown to augment IFN-γ production and enhances T cell accumulation within granulomas (150). Notably, CD4+ T cells are predominantly localized at the periphery of granulomas, maintaining a distance from the centrally located infected macrophages, potentially limiting their effectiveness in controlling the infection (150, 151).
Mycobacterial lipids play a pivotal role in the pathogenesis of granuloma formation. They not only impair the antimicrobial capabilities of macrophages but also promote the recruitment of additional macrophages, ultimately facilitating the dissemination of M. tuberculosis (196). Granulomas harboring M. tuberculosis can remain stable for extended periods, often spanning decades (197). However, factors such as the organism’s capacity for immunosuppression can jeopardize the granuloma’s integrity, potentially leading to its rupture and consequent dissemination of a substantial number of viable bacilli.
Pulmonary and extrapulmonary tuberculosis
The clinical manifestations of TB are contingent upon the anatomical site of the M. tuberculosis proliferation within a host. As an obligate aerobe, M. tuberculosis exhibits a predilection for the pulmonary system, whereby pulmonary TB can manifest as persistent cough, often accompanied by hemoptysis or sputum production, chest pain, and a protracted cough persisting beyond three weeks. General systemic symptoms attributable to TB may include malaise, anorexia, significant weight loss, febrile episodes, chills, and nocturnal hyperhidrosis. Other organs afflicted by M. tuberculosis may present with localized symptoms reflective of the pathogen’s dissemination.
Pulmonary immune responses elicited by mycobacterial infection are characterized by the activation of AMs, DCs, and inflammatory mediators, which in turn recruit and activate monocytes, neutrophils, T cells, and B cells. These inflammatory events culminate in granuloma formation. However, the persistent inflammatory response associated with M. tuberculosis infection provokes tissue necrosis, chronic lung inflammation, airway remodeling, and fibrotic alterations (198–204). The ensuing chronic obstructive pulmonary pathology ultimately culminates in irreversible airflow obstruction, potentially escalating to chronic respiratory failure and mortality (205, 206).
Beyond the pulmonary confines, M. tuberculosis can travel through the blood or lymphatic system from the primary lung lesions to disparate organ systems—this process characterizes extrapulmonary TB, accounting for approximately 15% of TB cases (207). Following the initial aerosolized infection and lung invasion, M. tuberculosis colonizes adjacent lymph nodes before gaining access to the bloodstream via the lymphatic network (illustrated in Figure 6) (208). Both immunocompetent and immunocompromised individuals are susceptible to extrapulmonary TB, although individuals living with HIV bear a heightened risk for extrapulmonary manifestations, particularly within the lymphatic system, with widespread dissemination, and affecting the central nervous system (CNS) (209). This complex pathology underscores the necessity for robust diagnostic and treatment strategies to mitigate the extensive spectrum of TB disease.
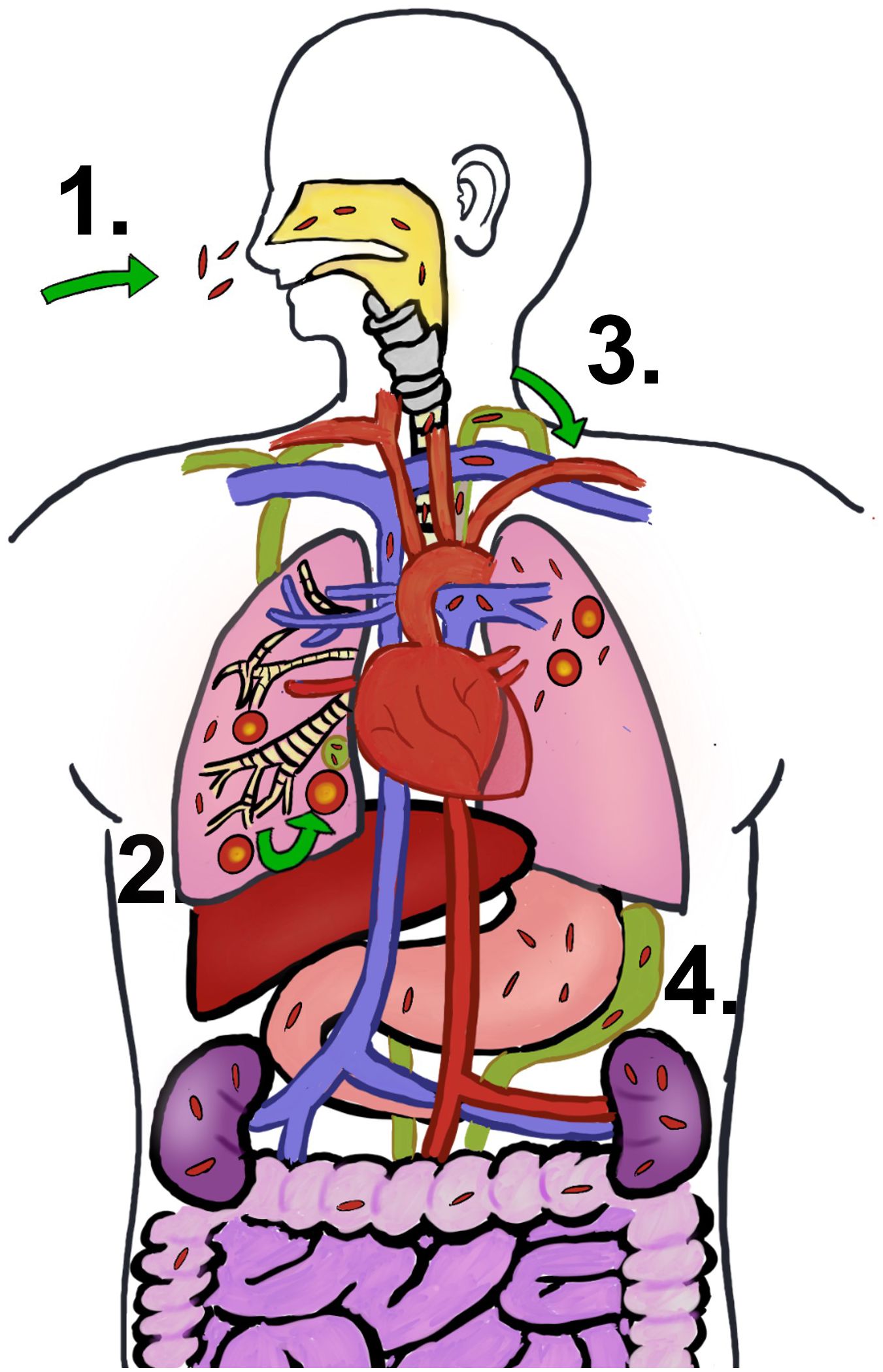
Figure 6 M. tuberculosis infection progression. (1) People get TB by breathing in the bacteria’s air. (2) Initial infection is developed in lung and go on to become granuloma. The early infection site impacts the surrounding lymph nodes as well. (3) Once the bacteria leave the lung and enter the lymphatic system, they most likely find their way into the circulatory system via the thoracic duct’s entrance into the subclavian vein. (4) Extrapulmonary TB is caused by the hematogenous spread of M. tuberculosis.
Designing tuberculosis vaccines based on pathogenesis
The global tuberculosis vaccine pipeline currently includes 17 candidates undergoing clinical trials, which we have organized and evaluated in Table 3. Although many of these candidates have successfully entered Phase I clinical trials, only a limited number have progressed to large-scale studies (227). A significant factor contributing to this limited advancement is the diminished efficacy of the vaccines.
To effectively counter TB, vaccine development must be intricately informed by a nuanced understanding of the pathogenesis of M. tuberculosis. An optimized vaccine would preemptively hinder M. tuberculosis from entering host cells, amplify both innate and adaptive immune responses, and surmount the bacterium’s immune evasion tactics. Nearly all of the antigens known to be involved in the pathogenic process of M. tuberculosis are summarized in Tables 1, 2. The development of new tuberculosis vaccines can consider combining these appropriate antigens.
The interplay of surface adhesion molecules is crucial for the invasion of host cells by mycobacteria, facilitating their association with and entry through host cellular receptors (228, 229). Various adhesion molecules, such as HBHA and fibronectin-binding proteins (FnBPs), significantly contribute to bacterial colonization by promoting the internalization of M. tuberculosis into host cells (230). The application of anti-HBHA antibodies to wild-type mycobacteria significantly inhibits their ability to disseminate following intranasal infection (231). These interactions with host cells not only aid in attachment and invasion but also trigger a series of signaling cascades, including the activation of the mitogen-activated protein kinases (MAPKs) pathway and the IFN-γ response, which can elicit pro-inflammatory and/or anti-inflammatory events (229). Moreover, adhesion molecules interfere with host signaling pathways and modify intracellular mechanisms, thereby modulating the immune response. Consequently, targeting these molecules is pivotal for the development of innovative tuberculosis vaccines and therapeutics.
Augmenting the bactericidal activity of primary mycobacterial targets—innate immune cells—stands as a critical strategy for curtailing M. tuberculosis proliferation. Trained immunity refers to a heightened state of innate immunological readiness that confers prolonged protection (232). Evidence suggests this heightened readiness can preserve its protective efficacy for months, if not years, following exposure to live vaccines (233, 234). Epigenetic and metabolic reprogramming of monocytes and macrophages constitute the mechanistic underpinnings of trained immunity, bolstering mycobacterial eradication and fostering expeditious clearance (235). β-glucan, renowned for immune potentiation in cancer therapy, exemplifies an agent that has shown promise in clinical trials for enhancing resistance to M. tuberculosis infection by stimulating myeloid progenitor proliferation (236–240). Similarly, the BCG vaccine can induce preventive innate immunity through the reprogramming of hematopoietic stem cells towards myelopoiesis in the bone marrow (241). The exploration of vaccine candidates that amplify innate immunity and elicit trained immunity could revolutionize TB vaccine efficacy.
DCs serve as a linchpin in the host’s defensive apparatus as primary antigen-presenting cells. Bolstering DCs through vaccines and immunotherapies could potentiate TB mitigation efforts. Enhancement of glutathione levels within DCs kickstarts the NF-κB signaling pathway, augmenting DC functionality in their dual roles of mycobacterial containment and antigen presentation (242). Exogeneous CD40 stimulation in infected DCs has been observed to amplify DC efficacy and boost CD4+ T cell responses, which aids in managing pulmonary bacterial loads (243). The introduction of antigen-loaded DCs has demonstrated the potential to enhance the efficacy of BCG vaccines, accentuating the imperative role of DCs in vaccine success (244).
Immunological research has demonstrated the critical importance of CD4+ Th1 cells and cytokines such as IFN-γ, TNF-α, and IL-2 in orchestrating host immunity (245). By elucidating the pathogenesis of M. tuberculosis, researchers can more accurately identify antigens that instigate robust T cell responses, necessitating continued exploration for novel mycobacterial antigens through bioinformatics and genomics. In the field of immunoinformatics, computational techniques are employed to address immunological complexities, enabling the discovery of immunogenic T-cell peptides from M. tuberculosis, specifically MPT64, PPE68, CFP21, and Ag85B (246–250). These peptides have shown promising immunogenicity in laboratory studies and represent candidates for potential inclusion in future tuberculosis vaccines. Furthermore, unraveling the mechanisms behind the generation and maintenance of memory T cells is crucial for the development of effective T cell-targeted vaccines (251).
Recent studies have posited a reinforcing role for antibodies in the defensive cohort against M. tuberculosis, with antibodies against capsular polysaccharides conferring protection in various microbial infections (252). The capsule constituent arabinomannan (AM) plays a critical role in TB pathophysiology (253), and vaccinations using AM conjugates have conferred heightened resistance in murine models (254, 255). HBHA also plays a critical role in the dissemination of mycobacteria. Studies have shown that coating BCG with anti-HBHA antibodies significantly reduces spleen colonization, suggesting that targeting HBHA can be an effective strategy to limit the spread of mycobacterial infections (231). In mycobacterium-infected mice, intranasal administration of an IgA monoclonal antibody targeting the α-crystallin antigen of M. tuberculosis resulted in a significant tenfold reduction in bacterial counts within the lungs (174). Furthermore, BCG and several other antigen-based vaccines elicit humoral responses that enhance TB prognoses (156).
Confronting mycobacterial adept immune evasion strategies is another key to vaccine advancement. M. tuberculosis employs mechanisms to dampen immune responses, devastate immune cells, and diminish metabolic visibility for persistent cellular habitation (Tables 1, 2). Targeted disruption of these mycobacterial evasion pathways will substantially amplify vaccine potency. For instance, the ESX-1 type VII secretion system is implicated in facilitating mycobacterial cellular escape and dissemination, and its incorporation into the BCG genome—yielding BCG::ESX-1—has shown enhanced protective capacity against TB (256). The major secretory antigen, the antigen 85 (Ag85) complex, comprising Ag85A, Ag85B, and Ag85C, plays a crucial role in the pathogenicity of M. tuberculosis. This complex impedes the formation of phagolysosomes, thereby enabling the bacteria to overcome the host immune response and persist within host cells. Given its significant impact on the infection process, Ag85 molecules are being utilized in diagnostic procedures and the development of novel vaccines (257). ESAT-6 reduces T cell activation without altering upstream T cell receptor (TCR) signaling processes, thereby significantly inhibiting T cell production of IFN-γ in response to M. tuberculosis or TCR activation (258). ESAT-6 is currently utilized in tuberculosis-related vaccines, including AEC/BC02 and GamTBvac. The antigen TB10.4 is expressed by both M. tuberculosis and BCG. Upon activation, CD8+ T cells specific to the TB10.4 3–11epitope are recruited to the site of infection following M. tuberculosis exposure. These cells produce TNF-α and IFN-γ, and exhibit upregulated expression of Fas ligand (FasL) and lysosomal-associated membrane proteins 1 and 2 (LAMP-1/2, also known as CD107A/B) (259). The TB-related vaccine TB/FLU-05E, currently in Phase I clinical trials, employs TB10.4 as one of its antigens.
Collectively, these insights point towards a multifaceted approach to TB vaccine development, encompassing the targeting of adhesion molecules, fortification of innate immune responses, enhancement of DC functionality, discovery of novel antigens, and interruption of M. tuberculosis immune evasion mechanisms. Such a strategy presages advancements in durable and effective vaccines against TB.
Conclusions
This review sheds light on the mechanisms by which M. tuberculosis enters host cells, disseminates infection, and evades the immune system. The vaccines for the future will need to overcome myeloid cell malfunction and the inability of the innate and adaptive immune systems caused by M. tuberculosis in order to eradicate the bacilli. The knowledge of pathogenesis should enable novel methods to produce effective vaccines, despite the numerous gaps that still need to be filled.
Author contributions
HY: Writing – original draft, Writing – review & editing. XL: Writing – review & editing. SC: Writing – review & editing. GS: Writing – review & editing. LD: Writing – review & editing, Supervision.
Funding
The author(s) declare that no financial support was received for the research, authorship, and/or publication of this article.
Conflict of interest
All authors were employed by company Beijing Zhifei Lvzhu Biopharmaceutical Co., Ltd.
Publisher’s note
All claims expressed in this article are solely those of the authors and do not necessarily represent those of their affiliated organizations, or those of the publisher, the editors and the reviewers. Any product that may be evaluated in this article, or claim that may be made by its manufacturer, is not guaranteed or endorsed by the publisher.
References
2. Houben RM, Dodd PJ. The global burden of latent tuberculosis infection: A re-estimation using mathematical modelling. PLoS Med. (2016) 13:e1002152. doi: 10.1371/journal.pmed.1002152
3. Leung AN. Pulmonary tuberculosis: the essentials. Radiology. (1999) 210:307–22. doi: 10.1148/radiology.210.2.r99ja34307
4. Tappeiner. Tappeiner Neue experimentelle Beiträge zur Inhalationstuberculose der Hunde. Archiv f. pathol. Anat. (1880) 82:353–9. doi: 10.1007/BF01928915
5. Donald PR, Diacon AH, Lange C, Demers AM, von Groote-Bidlingmaier F, Nardell E. Droplets, dust and Guinea pigs: an historical review of tuberculosis transmission research, 1878-1940. Int J Tuberc Lung Dis. (2018) 22:972–82. doi: 10.5588/ijtld.18.0173
6. Arcos J, Sasindran SJ, Moliva JI, Scordo JM, Sidiki S, Guo H, et al. Mycobacterium tuberculosis cell wall released fragments by the action of the human lung mucosa modulate macrophages to control infection in an IL-10-dependent manner. Mucosal Immunol. (2017) 10(5):1248–58. doi: 10.1038/mi.2016.115
7. Arcos J, Sasindran SJ, Fujiwara N, Turner J, Schlesinger LS, Torrelles JB. Human lung hydrolases delineate Mycobacterium tuberculosis-macrophage interactions and the capacity to control infection. J Immunol. (2011) 187(1):372–81. doi: 10.4049/jimmunol.1100823
8. Ryll R, Kumazawa Y, Yano I. Immunological properties of trehalose dimycolate (cord factor) and other mycolic acid-containing glycolipids–a review. Microbiol Immunol. (2001) 45:801–11. doi: 10.1111/j.1348-0421.2001.tb01319.x
9. Hunter RL, Olsen MR, Jagannath C, Actor JK. Multiple roles of cord factor in the pathogenesis of primary, secondary, and cavitary tuberculosis, including a revised description of the pathology of secondary disease. Ann Clin Lab Sci. (2006) 36(4):371–86.
10. Scordo JM, Olmo-Fontánez AM, Kelley HV, Sidiki S, Arcos J, Akhter A, et al. The human lung mucosa drives differential Mycobacterium tuberculosis infection outcome in the alveolar epithelium. Mucosal Immunol. (2019) 12:795–804. doi: 10.1038/s41385-019-0156-2
11. Bermudez LE, Sangari FJ, Kolonoski P, Petrofsky M, Goodman J. The efficiency of the translocation of Mycobacterium tuberculosis across a bilayer of epithelial and endothelial cells as a model of the alveolar wall is a consequence of transport within mononuclear phagocytes and invasion of alveolar epithelial cells. Infect Immun. (2002) 70:140–6. doi: 10.1128/IAI.70.1.140-146.2002
12. McDonough KA, Kress Y. Cytotoxicity for lung epithelial cells is a virulence-associated phenotype of Mycobacterium tuberculosis. Infect Immun. (1995) 63:4802–11. doi: 10.1128/iai.63.12.4802-4811.1995
13. Castro-Garza J, King CH, Swords WE, Quinn FD. Demonstration of spread by Mycobacterium tuberculosis bacilli in A549 epithelial cell monolayers. FEMS Microbiol Lett. (2002) 212:145–9. doi: 10.1111/fml.2002.212.issue-2
14. Dobos KM, Spotts EA, Quinn FD, King CH. Necrosis of lung epithelial cells during infection with Mycobacterium tuberculosis is preceded by cell permeation. Infect Immun. (2000) 68:6300–10. doi: 10.1128/IAI.68.11.6300-6310.2000
15. Scordo JM, Knoell DL, Torrelles JB. Alveolar epithelial cells in mycobacterium tuberculosis infection: Active players or innocent bystanders? J Innate Immun. (2016) 8:3–14. doi: 10.1159/000439275
17. Zhang M, Kim KJ, Iyer D, Lin Y, Belisle J, McEnery K, et al. Effects of Mycobacterium tuberculosis on the bioelectric properties of the alveolar epithelium. Infect Immun. (1997) 65:692–8. doi: 10.1128/iai.65.2.692-698.1997
18. Pethe K, Aumercier M, Fort E, Gatot C, Locht C, Menozzi FD. Characterization of the heparin-binding site of the mycobacterial heparin-binding hemagglutinin adhesin. J Biol Chem. (2000) 275:14273–80. doi: 10.1074/jbc.275.19.14273
19. Mehta PK, King CH, White EH, Murtagh JJ Jr, Quinn FD. Comparison of in vitro models for the study of Mycobacterium tuberculosis invasion and intracellular replication. Infect Immun. (1996) 64:2673–9. doi: 10.1128/iai.64.7.2673-2679.1996
20. Reljic R, Di Sano C, Crawford C, Dieli F, Challacombe S, Ivanyi J. Time course of mycobacterial infection of dendritic cells in the lungs of intranasally infected mice. Tuberculosis (Edinb). (2005) 85:81–8. doi: 10.1016/j.tube.2004.09.006
21. Marino S, Kirschner DE. A multi-compartment hybrid computational model predicts key roles for dendritic cells in tuberculosis infection. Comput (Basel). (2016) 4. doi: 10.3390/computation4040039
22. Moule MG, Cirillo JD. Mycobacterium tuberculosis dissemination plays a critical role in pathogenesis. Front Cell Infect Microbiol. (2020) 10:65. doi: 10.3389/fcimb.2020.00065
23. Cambier CJ, Takaki KK, Larson RP, Hernandez RE, Tobin DM, Urdahl KB, et al. Mycobacteria manipulate macrophage recruitment through coordinated use of membrane lipids. Nature. (2014) 505:218–22. doi: 10.1038/nature12799
24. Cambier CJ, Banik SM, Buonomo JA, Bertozzi CR. Spreading of a mycobacterial cell-surface lipid into host epithelial membranes promotes infectivity. Elife. (2020) 9:e60648. doi: 10.7554/eLife.60648
25. Cohen SB, Gern BH, Delahaye JL, Adams KN, Plumlee CR, Winkler JK, et al. Alveolar macrophages provide an early mycobacterium tuberculosis niche and initiate dissemination. Cell Host Microbe. (2018) 24:439–46.e4. doi: 10.1016/j.chom.2018.08.001
26. Huang L, Nazarova EV, Tan S, Liu Y, Russell DG. Growth of Mycobacterium tuberculosis in vivo segregates with host macrophage metabolism and ontogeny. J Exp Med. (2018) 215:1135–52. doi: 10.1084/jem.20172020
27. Leemans JC, Juffermans NP, Florquin S, van Rooijen N, Vervoordeldonk MJ, Verbon A, et al. Depletion of alveolar macrophages exerts protective effects in pulmonary tuberculosis in mice. J Immunol. (2001) 166:4604–11. doi: 10.4049/jimmunol.166.7.4604
28. Mantovani A, Dinarello CA, Molgora M, Garlanda C. Interleukin-1 and related cytokines in the regulation of inflammation and immunity. Immunity. (2019) 50:778–95. doi: 10.1016/j.immuni.2019.03.012
29. Fahey E, Doyle SL. IL-1 family cytokine regulation of vascular permeability and angiogenesis. Front Immunol. (2019) 10:1426. doi: 10.3389/fimmu.2019.01426
30. Gröschel MI, Sayes F, Simeone R, Majlessi L, Brosch R. ESX secretion systems: mycobacterial evolution to counter host immunity. Nat Rev Microbiol. (2016) 14:677–91. doi: 10.1038/nrmicro.2016.131
31. Silvério D, Gonçalves R, Appelberg R, Saraiva M. Advances on the role and applications of interleukin-1 in tuberculosis. mBio. (2021) 12:e0313421. doi: 10.1128/mBio.03134-21
32. Mishra BB, Moura-Alves P, Sonawane A, Hacohen N, Griffiths G, Moita LF, et al. Mycobacterium tuberculosis protein ESAT-6 is a potent activator of the NLRP3/ASC inflammasome. Cell Microbiol. (2010) 12:1046–63. doi: 10.1111/j.1462-5822.2010.01450.x
33. Rahman A, Sobia P, Gupta N, Kaer LV, Das G. Mycobacterium tuberculosis subverts the TLR-2-MyD88 pathway to facilitate its translocation into the cytosol. PLoS One. (2014) 9:e86886. doi: 10.1371/journal.pone.0086886
34. Wong KW, Jacobs WR Jr. Critical role for NLRP3 in necrotic death triggered by Mycobacterium tuberculosis. Cell Microbiol. (2011) 13:1371–84. doi: 10.1111/j.1462-5822.2011.01625.x
35. Pathak SK, Basu S, Basu KK, Banerjee A, Pathak S, Bhattacharyya A, et al. Direct extracellular interaction between the early secreted antigen ESAT-6 of Mycobacterium tuberculosis and TLR2 inhibits TLR signaling in macrophages. Nat Immunol. (2007) 8:610–8. doi: 10.1038/ni1468
36. Hmama Z, Peña-Díaz S, Joseph S, Av-Gay Y. Immunoevasion and immunosuppression of the macrophage by Mycobacterium tuberculosis. Immunol Rev. (2015) 264:220–32. doi: 10.1111/imr.12268
37. Guilliams M, Svedberg FR. Does tissue imprinting restrict macrophage plasticity? Nat Immunol. (2021) 22:118–27. doi: 10.1038/s41590-020-00849-2
38. Lee W, VanderVen BC, Fahey RJ, Russell DG. Intracellular Mycobacterium tuberculosis exploits host-derived fatty acids to limit metabolic stress. J Biol Chem. (2013) 288:6788–800. doi: 10.1074/jbc.M112.445056
39. Podinovskaia M, Lee W, Caldwell S, Russell DG. Infection of macrophages with Mycobacterium tuberculosis induces global modifications to phagosomal function. Cell Microbiol. (2013) 15:843–59. doi: 10.1111/cmi.2013.15.issue-6
40. VanderVen BC, Fahey RJ, Lee W, Liu Y, Abramovitch RB, Memmott C, et al. Novel inhibitors of cholesterol degradation in Mycobacterium tuberculosis reveal how the bacterium's metabolism is constrained by the intracellular environment. PLoS Pathog. (2015) 11:e1004679. doi: 10.1371/journal.ppat.1004679
41. Nazarova EV, Montague CR, La T, Wilburn KM, Sukumar N, Lee W, et al. Rv3723/LucA coordinates fatty acid and cholesterol uptake in Mycobacterium tuberculosis. Elife. (2017) 6:e26969. doi: 10.7554/eLife.26969
42. Augenstreich J, Arbues A, Simeone R, Haanappel E, Wegener A, Sayes F, et al. ESX-1 and phthiocerol dimycocerosates of Mycobacterium tuberculosis act in concert to cause phagosomal rupture and host cell apoptosis. Cell Microbiol. (2017) 19(7). doi: 10.1111/cmi.12726
43. Wang J, Ge P, Lei Z, Lu Z, Qiang L, Chai Q, et al. Mycobacterium tuberculosis protein kinase G acts as an unusual ubiquitinating enzyme to impair host immunity. EMBO Rep. (2021) 22:e52175. doi: 10.15252/embr.202052175
44. Dou Y, Xie Y, Zhang L, Liu S, Xu D, Wei Y, et al. Host MKRN1-mediated mycobacterial PPE protein ubiquitination suppresses innate immune response. Front Immunol. (2022) 13:880315. doi: 10.3389/fimmu.2022.880315
45. Kim JS, Kim HK, Cho E, Mun SJ, Jang S, Jang J, et al. PE_PGRS38 interaction with HAUSP downregulates antimycobacterial host defense via TRAF6. Front Immunol. (2022) 13:862628. doi: 10.3389/fimmu.2022.862628
46. Wang L, Wu J, Li J, Yang H, Tang T, Liang H, et al. Host-mediated ubiquitination of a mycobacterial protein suppresses immunity. Nature. (2020) 577:682–8. doi: 10.1038/s41586-019-1915-7
47. Ge P, Lei Z, Yu Y, Lu Z, Qiang L, Chai Q, et al. M. tuberculosis PknG manipulates host autophagy flux to promote pathogen intracellular survival. Autophagy. (2022) 18:576–94. doi: 10.1080/15548627.2021.1938912
48. Shin DM, Jeon BY, Lee HM, Jin HS, Yuk JM, Song CH, et al. Mycobacterium tuberculosis eis regulates autophagy, inflammation, and cell death through redox-dependent signaling. PLoS Pathog. (2010) 6:e1001230. doi: 10.1371/journal.ppat.1001230
49. Duan L, Yi M, Chen J, Li S, Chen W. Mycobacterium tuberculosis EIS gene inhibits macrophage autophagy through up-regulation of IL-10 by increasing the acetylation of histone H3. Biochem Biophys Res Commun. (2016) 473:1229–34. doi: 10.1016/j.bbrc.2016.04.045
50. Sengupta S, Nayak B, Meuli M, Sander P, Mishra S, Sonawane A. Mycobacterium tuberculosis phosphoribosyltransferase promotes bacterial survival in macrophages by inducing histone hypermethylation in autophagy-related genes. Front Cell Infect Microbiol. (2021) 11:676456. doi: 10.3389/fcimb.2021.676456
51. Bah A, Sanicas M, Nigou J, Guilhot C, Astarie-Dequeker C, Vergne I. The Lipid Virulence Factors of Mycobacterium tuberculosis Exert Multilayered Control over Autophagy-Related Pathways in Infected Human Macrophages. Cells. (2020) 9(3):666. doi: 10.3390/cells9030666
52. Strong EJ, Jurcic Smith KL, Saini NK, Ng TW, Porcelli SA, Lee S. Identification of autophagy-inhibiting factors of mycobacterium tuberculosis by high-throughput loss-of-function screening. Infect Immun. (2020) 88(12):e00269–20. doi: 10.1128/IAI.00269-20
53. Strong EJ, Ng TW, Porcelli SA, Lee S. Mycobacterium tuberculosis PE_PGRS20 and PE_PGRS47 proteins inhibit autophagy by interaction with rab1A. mSphere. (2021) 6:e0054921. doi: 10.1128/mSphere.00549-21
54. Strong EJ, Wang J, Ng TW, Porcelli SA, Lee S. Mycobacterium tuberculosis PPE51 inhibits autophagy by suppressing toll-like receptor 2-dependent signaling. mBio. (2022) 13:e0297421. doi: 10.1128/mbio.02974-21
55. Jayakumar D, Jacobs WR Jr, Narayanan S. Protein kinase E of Mycobacterium tuberculosis has a role in the nitric oxide stress response and apoptosis in a human macrophage model of infection. Cell Microbiol. (2008) 10:365–74. doi: 10.1111/j.1462-5822.2007.01049.x
56. Dutta NK, Mehra S, Martinez AN, Alvarez X, Renner NA, Morici LA, et al. The stress-response factor SigH modulates the interaction between Mycobacterium tuberculosis and host phagocytes. PLoS One. (2012) 7:e28958. doi: 10.1371/journal.pone.0028958
57. Halder P, Kumar R, Jana K, Chakraborty S, Ghosh Z, Kundu M, et al. Gene expression profiling of Mycobacterium tuberculosis Lipoarabinomannan-treated macrophages: A role of the Bcl-2 family member A1 in inhibition of apoptosis in mycobacteria-infected macrophages. IUBMB Life. (2015) 67:726–36. doi: 10.1002/iub.1430
58. Joseph S, Yuen A, Singh V, Hmama Z. Mycobacterium tuberculosis Cpn60.2 (GroEL2) blocks macrophage apoptosis via interaction with mitochondrial mortalin. Biol Open. (2017) 6:481–8. doi: 10.1242/bio.023119
59. Yang W, Deng W, Zeng J, Ren S, Ali MK, Gu Y, et al. Mycobacterium tuberculosis PE_PGRS18 enhances the intracellular survival of M. smegmatis via altering host macrophage cytokine profiling and attenuating the cell apoptosis. Apoptosis. (2017) 22:502–9. doi: 10.1007/s10495-016-1336-0
60. Long Q, Xiang X, Yin Q, Li S, Yang W, Sun H, et al. PE_PGRS62 promotes the survival of Mycobacterium smegmatis within macrophages via disrupting ER stress-mediated apoptosis. J Cell Physiol. (2019) 234:19774–84. doi: 10.1002/jcp.28577
61. Ali MK, Zhen G, Nzungize L, Stojkoska A, Duan X, Li C, et al. Mycobacterium tuberculosis PE31 (Rv3477) Attenuates Host Cell Apoptosis and Promotes Recombinant M. smegmatis Intracellular Survival via Up-regulating GTPase Guanylate Binding Protein-1. Front Cell Infect Microbiol. (2020) 10:40. doi: 10.3389/fcimb.2020.00040
62. Asaad M, Kaisar Ali M, Abo-Kadoum MA, Lambert N, Gong Z, Wang H, et al. Mycobacterium tuberculosis PPE10 (Rv0442c) alters host cell apoptosis and cytokine profile via linear ubiquitin chain assembly complex HOIP-NF-κB signaling axis. Int Immunopharmacol. (2021) 94:107363. doi: 10.1016/j.intimp.2020.107363
63. Zhang W, Lu Q, Dong Y, Yue Y, Xiong S. Rv3033, as an emerging anti-apoptosis factor, facilitates mycobacteria survival via inhibiting macrophage intrinsic apoptosis. Front Immunol. (2018) 9:2136. doi: 10.3389/fimmu.2018.02136
64. Aguilo JI, Alonso H, Uranga S, Marinova D, Arbués A, de Martino A, et al. ESX-1-induced apoptosis is involved in cell-to-cell spread of Mycobacterium tuberculosis. Cell Microbiol. (2013) 15:1994–2005. doi: 10.1111/cmi.12169
65. Deng W, Yang W, Zeng J, Abdalla AE, Xie J. Mycobacterium tuberculosis PPE32 promotes cytokines production and host cell apoptosis through caspase cascade accompanying with enhanced ER stress response. Oncotarget. (2016) 7:67347–59. doi: 10.18632/oncotarget.v7i41
66. Zhang J, Jiang R, Takayama H, Tanaka Y. Survival of virulent Mycobacterium tuberculosis involves preventing apoptosis induced by Bcl-2 upregulation and release resulting from necrosis in J774 macrophages. Microbiol Immunol. (2005) 49:845–52. doi: 10.1111/j.1348-0421.2005.tb03673.x
67. Zhao X, Khan N, Gan H, Tzelepis F, Nishimura T, Park SY, et al. Bcl-x(L) mediates RIPK3-dependent necrosis in M. tuberculosis-infected macrophages. Mucosal Immunol. (2017) 10:1553–68. doi: 10.1038/mi.2017.12
68. Rastogi S, Ellinwood S, Augenstreich J, Mayer-Barber KD, Briken V. Mycobacterium tuberculosis inhibits the NLRP3 inflammasome activation via its phosphokinase PknF. PLoS Pathog. (2021) 17:e1009712. doi: 10.1371/journal.ppat.1009712
69. Danelishvili L, Everman JL, McNamara MJ, Bermudez LE. Inhibition of the plasma-membrane-associated serine protease cathepsin G by mycobacterium tuberculosis rv3364c suppresses caspase-1 and pyroptosis in macrophages. Front Microbiol. (2011) 2:281. doi: 10.3389/fmicb.2011.00281
70. Beckwith KS, Beckwith MS, Ullmann S, Sætra RS, Kim H, Marstad A, et al. Plasma membrane damage causes NLRP3 activation and pyroptosis during Mycobacterium tuberculosis infection. Nat Commun. (2020) 11:2270. doi: 10.1038/s41467-020-16143-6
71. Deng W, Long Q, Zeng J, Li P, Yang W, Chen X, et al. Mycobacterium tuberculosis PE_PGRS41 Enhances the Intracellular Survival of M. smegmatis within Macrophages Via Blocking Innate Immunity and Inhibition of Host Defense. Sci Rep. (2017) 7:46716. doi: 10.1038/srep46716
72. Wang J, Li BX, Ge PP, Li J, Wang Q, Gao GF, et al. Mycobacterium tuberculosis suppresses innate immunity by coopting the host ubiquitin system. Nat Immunol. (2015) 16:237–45. doi: 10.1038/ni.3096
73. Wang J, Teng JL, Zhao D, Ge P, Li B, Woo PC, et al. The ubiquitin ligase TRIM27 functions as a host restriction factor antagonized by Mycobacterium tuberculosis PtpA during mycobacterial infection. Sci Rep. (2016) 6:34827. doi: 10.1038/srep34827
74. Saini V, Farhana A, Steyn AJ. Mycobacterium tuberculosis WhiB3: a novel iron-sulfur cluster protein that regulates redox homeostasis and virulence. Antioxid Redox Signal. (2012) 16:687–97. doi: 10.1089/ars.2011.4341
75. Feng L, Chen S, Hu Y. PhoPR Positively Regulates whiB3 Expression in Response to Low pH in Pathogenic Mycobacteria. J Bacteriol. (2018) 200(8):e00766–17. doi: 10.1128/JB.00766-17
76. You D, Xu Y, Yin BC, Ye BC. Nitrogen Regulator GlnR Controls Redox Sensing and Lipids Anabolism by Directly Activating the whiB3 in Mycobacterium smegmatis. Front Microbiol. (2019) 10:74. doi: 10.3389/fmicb.2019.00074
77. Mahatha AC, Mal S, Majumder D, Saha S, Ghosh A, Basu J, et al. RegX3 Activates whiB3 Under Acid Stress and Subverts Lysosomal Trafficking of Mycobacterium tuberculosis in a WhiB3-Dependent Manner. Front Microbiol. (2020) 11:572433. doi: 10.3389/fmicb.2020.572433
78. Srivastava S, Battu MB, Khan MZ, Nandicoori VK, Mukhopadhyay S. Mycobacterium tuberculosis PPE2 Protein Interacts with p67(phox) and Inhibits Reactive Oxygen Species Production. J Immunol. (2019) 203:1218–29. doi: 10.4049/jimmunol.1801143
79. Sharp JD, Singh AK, Park ST, Lyubetskaya A, Peterson MW, Gomes AL, et al. Comprehensive definition of the sigH regulon of mycobacterium tuberculosis reveals transcriptional control of diverse stress responses. PLoS One. (2016) 11:e0152145. doi: 10.1371/journal.pone.0152145
80. Liu Y, Xie Z, Zhou X, Li W, Zhang H, He ZG. NapM enhances the survival of Mycobacterium tuberculosis under stress and in macrophages. Commun Biol. (2019) 2:65. doi: 10.1038/s42003-019-0314-9
81. Speer A, Sun J, Danilchanka O, Meikle V, Rowland JL, Walter K, et al. Surface hydrolysis of sphingomyelin by the outer membrane protein Rv0888 supports replication of Mycobacterium tuberculosis in macrophages. Mol Microbiol. (2015) 97:881–97. doi: 10.1111/mmi.13073
82. Lata S, Mahatha AC, Mal S, Gupta UD, Kundu M, Basu J. Unraveling novel roles of the Mycobacterium tuberculosis transcription factor Rv0081 in regulation of the nucleoid-associated proteins Lsr2 and EspR, cholesterol utilization, and subversion of lysosomal trafficking in macrophages. Mol Microbiol. (2022) 117:1104–20. doi: 10.1111/mmi.14895
83. Dubey N, Khan MZ, Kumar S, Sharma A, Das L, Bhaduri A, et al. Mycobacterium tuberculosis peptidyl prolyl isomerase A interacts with host integrin receptor to exacerbate disease progression. J Infect Dis. (2021) 224:1383–93. doi: 10.1093/infdis/jiab081
84. Master SS, Rampini SK, Davis AS, Keller C, Ehlers S, Springer B, et al. Mycobacterium tuberculosis prevents inflammasome activation. Cell Host Microbe. (2008) 3:224–32. doi: 10.1016/j.chom.2008.03.003
85. Dabla A, Liang YC, Rajabalee N, Irwin C, Moonen CGJ, Willis JV, et al. TREM2 promotes immune evasion by mycobacterium tuberculosis in human macrophages. mBio. (2022) 13:e0145622. doi: 10.1128/mbio.01456-22
86. Ates LS, Houben ENG, Bitter W. Type VII secretion: A highly versatile secretion system. Microbiol Spectr. (2016) 4(1). doi: 10.1128/microbiolspec.VMBF-0011-2015
87. Tiwari S, Casey R, Goulding CW, Hingley-Wilson S, Jacobs WR Jr. Infect and inject: How mycobacterium tuberculosis exploits its major virulence-associated type VII secretion system, ESX-1. Microbiol Spectr. (2019) 7(3):10.1128/microbiolspec.BAI-0024-2019. doi: 10.1128/microbiolspec.BAI-0024-2019
88. Wong KW. The role of ESX-1 in mycobacterium tuberculosis pathogenesis. Microbiol Spectr. (2017) 5(3). doi: 10.1128/microbiolspec.TBTB2-0001-2015
89. Behar SM, Divangahi M, Remold HG. Evasion of innate immunity by Mycobacterium tuberculosis: is death an exit strategy? Nat Rev Microbiol. (2010) 8(9):668–74. doi: 10.1038/nrmicro2387
90. Siregar TAP, Prombutara P, Kanjanasirirat P, Kunkaew N, Tubsuwan A, Boonmee A, et al. The autophagy-resistant Mycobacterium tuberculosis Beijing strain upregulates KatG to evade starvation-induced autophagic restriction. Pathog Dis. (2022) 80(1):ftac004. doi: 10.1093/femspd/ftac004
91. Voskuil MI, Bartek IL, Visconti K, Schoolnik GK. The response of mycobacterium tuberculosis to reactive oxygen and nitrogen species. Front Microbiol. (2011) 2:105. doi: 10.3389/fmicb.2011.00105
92. Gautam US, Mehra S, Kumari P, Alvarez X, Niu T, Tyagi JS, et al. Mycobacterium tuberculosis sensor kinase DosS modulates the autophagosome in a DosR-independent manner. Commun Biol. (2019) 2:349. doi: 10.1038/s42003-019-0594-0
93. Yang W, Liu M, Yu X, Huang Y, Zeng J, Dai Y, et al. Mycobacterium tuberculosis Rv1515c antigen enhances survival of M. smegmatis within macrophages by disrupting the host defence. Microb Pathog. (2021) 153:104778. doi: 10.1016/j.micpath.2021.104778
94. Rani A, Alam A, Ahmad F, P M, Saurabh A, Zarin S, et al. Mycobacterium tuberculosis methyltransferase rv1515c can suppress host defense mechanisms by modulating immune functions utilizing a multipronged mechanism. Front Mol Biosci. (2022) 9:906387. doi: 10.3389/fmolb.2022.906387
95. Blanc L, Gilleron M, Prandi J, Song OR, Jang MS, Gicquel B, et al. Mycobacterium tuberculosis inhibits human innate immune responses via the production of TLR2 antagonist glycolipids. Proc Natl Acad Sci U.S.A. (2017) 114:11205–10. doi: 10.1073/pnas.1707840114
96. Li H, Li Q, Yu Z, Zhou M, Xie J. Mycobacterium tuberculosis PE13 (Rv1195) manipulates the host cell fate via p38-ERK-NF-κB axis and apoptosis. Apoptosis. (2016) 21:795–808. doi: 10.1007/s10495-016-1249-y
97. Zhai W, Wu F, Zhang Y, Fu Y, Liu Z. The immune escape mechanisms of mycobacterium tuberculosis. Int J Mol Sci. (2019) 20(2):340. doi: 10.3390/ijms20020340
98. Lamichhane G. Mycobacterium tuberculosis response to stress from reactive oxygen and nitrogen species. Front Microbiol. (2011) 2:176. doi: 10.3389/fmicb.2011.00176
99. Armstrong JA, Hart PD. Response of cultured macrophages to Mycobacterium tuberculosis, with observations on fusion of lysosomes with phagosomes. J Exp Med. (1971) 134:713–40. doi: 10.1084/jem.134.3.713
100. Målen H, Berven FS, Fladmark KE, Wiker HG. Comprehensive analysis of exported proteins from Mycobacterium tuberculosis H37Rv. Proteomics. (2007) 7:1702–18. doi: 10.1002/pmic.200600853
101. Tran HR, Grebenc DW, Klein TA, Whitney JC. Bacterial type VII secretion: An important player in host-microbe and microbe-microbe interactions. Mol Microbiol. (2021) 115:478–89. doi: 10.1111/mmi.14680
102. van Pinxteren LA, Ravn P, Agger EM, Pollock J, Andersen P. Diagnosis of tuberculosis based on the two specific antigens ESAT-6 and CFP10. Clin Diagn Lab Immunol. (2000) 7:155–60. doi: 10.1128/CDLI.7.2.155-160.2000
103. Simeone R, Bobard A, Lippmann J, Bitter W, Majlessi L, Brosch R, et al. Phagosomal rupture by Mycobacterium tuberculosis results in toxicity and host cell death. PLoS Pathog. (2012) 8:e1002507. doi: 10.1371/journal.ppat.1002507
104. de Jonge MI, Pehau-Arnaudet G, Fretz MM, Romain F, Bottai D, Brodin P, et al. ESAT-6 from Mycobacterium tuberculosis dissociates from its putative chaperone CFP-10 under acidic conditions and exhibits membrane-lysing activity. J Bacteriol. (2007) 189:6028–34. doi: 10.1128/JB.00469-07
105. Conrad WH, Osman MM, Shanahan JK, Chu F, Takaki KK, Cameron J, et al. Mycobacterial ESX-1 secretion system mediates host cell lysis through bacterium contact-dependent gross membrane disruptions. Proc Natl Acad Sci U.S.A. (2017) 114(6):1371–6. doi: 10.1073/pnas.1620133114
106. Gao LY, Guo S, McLaughlin B, Morisaki H, Engel JN, Brown EJ. A mycobacterial virulence gene cluster extending RD1 is required for cytolysis, bacterial spreading and ESAT-6 secretion. Mol Microbiol. (2004) 53:1677–93. doi: 10.1111/j.1365-2958.2004.04261.x
107. Philips JA. Mycobacterial manipulation of vacuolar sorting. Cell Microbiol. (2008) 10:2408–15. doi: 10.1111/cmi.2008.10.issue-12
108. Divangahi M, Behar SM, Remold H. Dying to live: how the death modality of the infected macrophage affects immunity to tuberculosis. Adv Exp Med Biol. (2013) 783:103–20. doi: 10.1007/978-1-4614-6111-1
109. Keane J, Remold HG, Kornfeld H. Virulent Mycobacterium tuberculosis strains evade apoptosis of infected alveolar macrophages. J Immunol. (2000) 164:2016–20. doi: 10.4049/jimmunol.164.4.2016
110. Velmurugan K, Chen B, Miller JL, Azogue S, Gurses S, Hsu T, et al. Mycobacterium tuberculosis nuoG is a virulence gene that inhibits apoptosis of infected host cells. PLoS Pathog. (2007) 3:e110. doi: 10.1371/journal.ppat.0030110
111. Upadhyay S, Mittal E, Philips JA. Tuberculosis and the art of macrophage manipulation. Pathog Dis. (2018) 76(4):fty037. doi: 10.1093/femspd/fty037
112. Dey B, Bishai WR. Crosstalk between Mycobacterium tuberculosis and the host cell. Semin Immunol. (2014) 26:486–96. doi: 10.1016/j.smim.2014.09.002
113. Zulauf KE, Sullivan JT, Braunstein M. The SecA2 pathway of Mycobacterium tuberculosis exports effectors that work in concert to arrest phagosome and autophagosome maturation. PLoS Pathog. (2018) 14:e1007011. doi: 10.1371/journal.ppat.1007011
114. Shariq M, Quadir N, Sheikh JA, Singh AK, Bishai WR, Ehtesham NZ, et al. Post translational modifications in tuberculosis: ubiquitination paradox. Autophagy. (2021) 17:814–7. doi: 10.1080/15548627.2020.1850009
115. Behura A, Mishra A, Chugh S, Mawatwal S, Kumar A, Manna D, et al. ESAT-6 modulates Calcimycin-induced autophagy through microRNA-30a in mycobacteria infected macrophages. J Infect. (2019) 79:139–52. doi: 10.1016/j.jinf.2019.06.001
116. Mahamed D, Boulle M, Ganga Y, Mc Arthur C, Skroch S, Oom L, et al. Intracellular growth of Mycobacterium tuberculosis after macrophage cell death leads to serial killing of host cells. Elife. (2017) 6:e28205. doi: 10.7554/eLife.28205
117. Nathan C. Neutrophils and immunity: challenges and opportunities. Nat Rev Immunol. (2006) 6:173–82. doi: 10.1038/nri1785
118. Repasy T, Martinez N, Lee J, West K, Li W, Kornfeld H. Bacillary replication and macrophage necrosis are determinants of neutrophil recruitment in tuberculosis. Microbes Infect. (2015) 17:564–74. doi: 10.1016/j.micinf.2015.03.013
119. Dallenga T, Repnik U, Corleis B, Eich J, Reimer R, Griffiths GW, et al. M. tuberculosis-induced necrosis of infected neutrophils promotes bacterial growth following phagocytosis by macrophages. Cell Host Microbe. (2017) 22:519–30.e3. doi: 10.1016/j.chom.2017.09.003
120. Wolf AJ, Linas B, Trevejo-Nuñez GJ, Kincaid E, Tamura T, Takatsu K, et al. Mycobacterium tuberculosis infects dendritic cells with high frequency and impairs their function in vivo. J Immunol. (2007) 179:2509–19. doi: 10.4049/jimmunol.179.4.2509
121. Bell LCK, Noursadeghi M. Pathogenesis of HIV-1 and Mycobacterium tuberculosis co-infection. Nat Rev Microbiol. (2018) 16:80–90. doi: 10.1038/nrmicro.2017.128
122. Mogues T, Goodrich ME, Ryan L, LaCourse R, North RJ. The relative importance of T cell subsets in immunity and immunopathology of airborne Mycobacterium tuberculosis infection in mice. J Exp Med. (2001) 193:271–80. doi: 10.1084/jem.193.3.271
123. Caruso AM, Serbina N, Klein E, Triebold K, Bloom BR, Flynn JL. Mice deficient in CD4 T cells have only transiently diminished levels of IFN-gamma, yet succumb to tuberculosis. J Immunol. (1999) 162:5407–16. doi: 10.4049/jimmunol.162.9.5407
124. Cooper AM, Magram J, Ferrante J, Orme IM. Interleukin 12 (IL-12) is crucial to the development of protective immunity in mice intravenously infected with mycobacterium tuberculosis. J Exp Med. (1997) 186:39–45. doi: 10.1084/jem.186.1.39
125. Sullivan BM, Jobe O, Lazarevic V, Vasquez K, Bronson R, Glimcher LH, et al. Increased susceptibility of mice lacking T-bet to infection with Mycobacterium tuberculosis correlates with increased IL-10 and decreased IFN-gamma production. J Immunol. (2005) 175:4593–602. doi: 10.4049/jimmunol.175.7.4593
126. Chackerian AA, Alt JM, Perera TV, Dascher CC, Behar SM. Dissemination of Mycobacterium tuberculosis is influenced by host factors and precedes the initiation of T-cell immunity. Infect Immun. (2002) 70:4501–9. doi: 10.1128/IAI.70.8.4501-4509.2002
127. Chackerian AA, Perera TV, Behar SM. Gamma interferon-producing CD4+ T lymphocytes in the lung correlate with resistance to infection with Mycobacterium tuberculosis. Infect Immun. (2001) 69:2666–74. doi: 10.1128/IAI.69.4.2666-2674.2001
128. Ottenhoff TH, Kumararatne D, Casanova JL. Novel human immunodeficiencies reveal the essential role of type-I cytokines in immunity to intracellular bacteria. Immunol Today. (1998) 19:491–4. doi: 10.1016/S0167-5699(98)01321-8
129. Sousa AO, Mazzaccaro RJ, Russell RG, Lee FK, Turner OC, Hong S, et al. Relative contributions of distinct MHC class I-dependent cell populations in protection to tuberculosis infection in mice. Proc Natl Acad Sci U.S.A. (2000) 97(8):4204–8. doi: 10.1073/pnas.97.8.4204
130. Behar SM, Dascher CC, Grusby MJ, Wang CR, Brenner MB. Susceptibility of mice deficient in CD1D or TAP1 to infection with Mycobacterium tuberculosis. J Exp Med. (1999) 189:1973–80. doi: 10.1084/jem.189.12.1973
131. Mott D, Yang J, Baer C, Papavinasasundaram K, Sassetti CM, Behar SM. High bacillary burden and the ESX-1 type VII secretion system promote MHC class I presentation by mycobacterium tuberculosis-infected macrophages to CD8 T cells. J Immunol. (2023) 210:1531–42. doi: 10.4049/jimmunol.2300001
132. Ernst WA, Thoma-Uszynski S, Teitelbaum R, Ko C, Hanson DA, Clayberger C, et al. Granulysin, a T cell product, kills bacteria by altering membrane permeability. J Immunol. (2000) 165:7102–8. doi: 10.4049/jimmunol.165.12.7102
133. Lu YJ, Barreira-Silva P, Boyce S, Powers J, Cavallo K, Behar SM. CD4 T cell help prevents CD8 T cell exhaustion and promotes control of Mycobacterium tuberculosis infection. Cell Rep. (2021) 36:109696. doi: 10.1016/j.celrep.2021.109696
134. Srivastava S, Grace PS, Ernst JD. Antigen export reduces antigen presentation and limits T cell control of M. tuberculosis. Cell Host Microbe. (2016) 19:44–54. doi: 10.1016/j.chom.2015.12.003
135. Singh S, Maurya SK, Aqdas M, Bashir H, Arora A, Bhalla V, et al. Mycobacterium tuberculosis exploits MPT64 to generate myeloid-derived suppressor cells to evade the immune system. Cell Mol Life Sci. (2022) 79:567. doi: 10.1007/s00018-022-04596-5
136. Pinto R, Nambiar JK, Leotta L, Counoupas C, Britton WJ, Triccas JA. Influence of phthiocerol dimycocerosate on CD4(+) T cell priming and persistence during Mycobacterium tuberculosis infection. Tuberculosis (Edinb). (2016) 99:25–30. doi: 10.1016/j.tube.2016.04.001
137. Georgieva M, Sia JK, Bizzell E, Madan-Lala R, Rengarajan J. Mycobacterium tuberculosis groEL2 modulates dendritic cell responses. Infect Immun. (2018) 86(2):e00387–17. doi: 10.1128/IAI.00387-17
138. Portal-Celhay C, Tufariello JM, Srivastava S, Zahra A, Klevorn T, Grace PS, et al. Mycobacterium tuberculosis EsxH inhibits ESCRT-dependent CD4(+) T-cell activation. Nat Microbiol. (2016) 2:16232. doi: 10.1038/nmicrobiol.2016.232
139. Saini NK, Baena A, Ng TW, Venkataswamy MM, Kennedy SC, Kunnath-Velayudhan S, et al. Suppression of autophagy and antigen presentation by Mycobacterium tuberculosis PE_PGRS47. Nat Microbiol. (2016) 1:16133. doi: 10.1038/nmicrobiol.2016.133
140. Pai RK, Convery M, Hamilton TA, Boom WH, Harding CV.. Inhibition of IFN-gamma-induced class II transactivator expression by a 19-kDa lipoprotein from Mycobacterium tuberculosis: a potential mechanism for immune evasion. J Immunol. (2003) 171:175–84. doi: 10.4049/jimmunol.171.1.175
141. Kan-Sutton C, Jagannath C, Hunter RL Jr. Trehalose 6,6'-dimycolate on the surface of Mycobacterium tuberculosis modulates surface marker expression for antigen presentation and costimulation in murine macrophages. Microbes Infect. (2009) 11:40–8. doi: 10.1016/j.micinf.2008.10.006
142. Blomgran R, Desvignes L, Briken V, Ernst JD. Mycobacterium tuberculosis inhibits neutrophil apoptosis, leading to delayed activation of naive CD4 T cells. Cell Host Microbe. (2012) 11:81–90. doi: 10.1016/j.chom.2011.11.012
143. Zhang YJ, Reddy MC, Ioerger TR, Rothchild AC, Dartois V, Schuster BM, et al. Tryptophan biosynthesis protects mycobacteria from CD4 T-cell-mediated killing. Cell. (2013) 155:1296–308. doi: 10.1016/j.cell.2013.10.045
144. Moguche AO, Musvosvi M, Penn-Nicholson A, Plumlee CR, Mearns H, Geldenhuys H, et al. Antigen availability shapes T cell differentiation and function during tuberculosis. Cell Host Microbe. (2017) 21:695–706.e5. doi: 10.1016/j.chom.2017.05.012
145. Athman JJ, Sande OJ, Groft SG, Reba SM, Nagy N, Wearsch PA, et al. Mycobacterium tuberculosis membrane vesicles inhibit T cell activation. J Immunol. (2017) 198:2028–37. doi: 10.4049/jimmunol.1601199
146. Nishimura N, Tomiyasu N, Torigoe S, Mizuno S, Fukano H, Ishikawa E, et al. Mycobacterial mycolic acids trigger inhibitory receptor Clec12A to suppress host immune responses. Tuberculosis (Edinb). (2023) 138:102294. doi: 10.1016/j.tube.2022.102294
147. Clemmensen HS, Dube JY, McIntosh F, Rosenkrands I, Jungersen G, Aagaard C, et al. In Vivo Antigen Expression Regulates CD4 T Cell Differentiation and Vaccine Efficacy against Mycobacterium tuberculosis Infection. mBio. (2021) 12(2):e00226–21. doi: 10.1128/mBio.00226-21
148. Sutiwisesak R, Hicks ND, Boyce S, Murphy KC, Papavinasasundaram K, Carpenter SM, et al. A natural polymorphism of Mycobacterium tuberculosis in the esxH gene disrupts immunodomination by the TB10.4-specific CD8 T cell response. PLoS Pathog. (2020) 16:e1009000. doi: 10.1371/journal.ppat.1009000
149. Carow B, Hauling T, Qian X, Kramnik I, Nilsson M, Rottenberg ME. Spatial and temporal localization of immune transcripts defines hallmarks and diversity in the tuberculosis granuloma. Nat Commun. (2019) 10:1823. doi: 10.1038/s41467-019-09816-4
150. Gern BH, Adams KN, Plumlee CR, Stoltzfus CR, Shehata L, Moguche AO, et al. TGFβ restricts expansion, survival, and function of T cells within the tuberculous granuloma. Cell Host Microbe. (2021) 29:594–606.e6. doi: 10.1016/j.chom.2021.02.005
151. Gautam US, Foreman TW, Bucsan AN, Veatch AV, Alvarez X, Adekambi T, et al. In vivo inhibition of tryptophan catabolism reorganizes the tuberculoma and augments immune-mediated control of Mycobacterium tuberculosis. Proc Natl Acad Sci U.S.A. (2018) 115:E62–e71. doi: 10.1073/pnas.1711373114
152. Joosten SA, van Meijgaarden KE, Del Nonno F, Baiocchini A, Petrone L, Vanini V, et al. Patients with tuberculosis have a dysfunctional circulating B-cell compartment, which normalizes following successful treatment. PLoS Pathog. (2016) 12:e1005687. doi: 10.1371/journal.ppat.1005687
153. Portugal S, Obeng-Adjei N, Moir S, Crompton PD, Pierce SK. Atypical memory B cells in human chronic infectious diseases: An interim report. Cell Immunol. (2017) 321:18–25. doi: 10.1016/j.cellimm.2017.07.003
154. Srivastava S, Ernst JD. Cell-to-cell transfer of M. tuberculosis antigens optimizes CD4 T cell priming. Cell Host Microbe. (2014) 15:741–52. doi: 10.1016/j.chom.2014.05.007
155. Srivastava S, Ernst JD. Cutting edge: Direct recognition of infected cells by CD4 T cells is required for control of intracellular Mycobacterium tuberculosis in vivo. J Immunol. (2013) 191:1016–20. doi: 10.4049/jimmunol.1301236
156. Achkar JM, Casadevall A. Antibody-mediated immunity against tuberculosis: implications for vaccine development. Cell Host Microbe. (2013) 13:250–62. doi: 10.1016/j.chom.2013.02.009
157. Achkar JM, Chan J, Casadevall A. Role of B cells and antibodies in acquired immunity against Mycobacterium tuberculosis. Cold Spring Harb Perspect Med. (2014) 5(3):a018432. doi: 10.1101/cshperspect.a018432
158. Chan J, Mehta S, Bharrhan S, Chen Y, Achkar JM, Casadevall A, et al. The role of B cells and humoral immunity in Mycobacterium tuberculosis infection. Semin Immunol. (2014) 26:588–600. doi: 10.1016/j.smim.2014.10.005
159. Kozakiewicz L, Phuah J, Flynn J, Chan J. The role of B cells and humoral immunity in Mycobacterium tuberculosis infection. Adv Exp Med Biol. (2013) 783:225–50. doi: 10.1007/978-1-4614-6111-1_12
160. Achkar JM, Chan J, Casadevall A. B cells and antibodies in the defense against Mycobacterium tuberculosis infection. Immunol Rev. (2015) 264:167–81. doi: 10.1111/imr.12276
161. Vordermeier HM, Venkataprasad N, Harris DP, Ivanyi J. Increase of tuberculous infection in the organs of B cell-deficient mice. Clin Exp Immunol. (1996) 106:312–6. doi: 10.1046/j.1365-2249.1996.d01-845.x
162. Glatman-Freedman A, Casadevall A. Serum therapy for tuberculosis revisited: reappraisal of the role of antibody-mediated immunity against Mycobacterium tuberculosis. Clin Microbiol Rev. (1998) 11:514–32. doi: 10.1128/CMR.11.3.514
163. Steingart KR, Dendukuri N, Henry M, Schiller I, Nahid P, Hopewell PC, et al. Performance of purified antigens for serodiagnosis of pulmonary tuberculosis: a meta-analysis. Clin Vaccine Immunol. (2009) 16:260–76. doi: 10.1128/CVI.00355-08
164. Maglione PJ, Xu J, Casadevall A, Chan J. Fc gamma receptors regulate immune activation and susceptibility during Mycobacterium tuberculosis infection. J Immunol. (2008) 180(5):3329–38. doi: 10.4049/jimmunol.180.5.3329
165. Maglione PJ, Xu J, Chan J. B cells moderate inflammatory progression and enhance bacterial containment upon pulmonary challenge with Mycobacterium tuberculosis. J Immunol. (2007) 178:7222–34. doi: 10.4049/jimmunol.178.11.7222
166. Rodríguez A, Tjärnlund A, Ivanji J, Singh M, García I, Williams A, et al. Role of IgA in the defense against respiratory infections IgA deficient mice exhibited increased susceptibility to intranasal infection with Mycobacterium bovis BCG. Vaccine. (2005) 23:2565–72. doi: 10.1016/j.vaccine.2004.11.032
167. Tjärnlund A, Rodríguez A, Cardona PJ, Guirado E, Ivanyi J, Singh M, et al. Polymeric IgR knockout mice are more susceptible to mycobacterial infections in the respiratory tract than wild-type mice. Int Immunol. (2006) 18:807–16. doi: 10.1093/intimm/dxl017
168. Li H, Wang XX, Wang B, Fu L, Liu G, Lu Y, et al. Latently and uninfected healthcare workers exposed to TB make protective antibodies against Mycobacterium tuberculosis. Proc Natl Acad Sci U.S.A. (2017) 114(19):5023–8. doi: 10.1073/pnas.1611776114
169. Balu S, Reljic R, Lewis MJ, Pleass RJ, McIntosh R, van Kooten C, et al. A novel human IgA monoclonal antibody protects against tuberculosis. J Immunol. (2011) 186:3113–9. doi: 10.4049/jimmunol.1003189
170. Chambers MA, Gavier-Widén D, Hewinson RG. Antibody bound to the surface antigen MPB83 of Mycobacterium bovis enhances survival against high dose and low dose challenge. FEMS Immunol Med Microbiol. (2004) 41:93–100. doi: 10.1016/j.femsim.2004.01.004
171. Hamasur B, Haile M, Pawlowski A, Schroder U, Kallenius G, Svenson SB. A mycobacterial lipoarabinomannan specific monoclonal antibody and its F(ab') fragment prolong survival of mice infected with Mycobacterium tuberculosis. Clin Exp Immunol. (2004) 138:30–8. doi: 10.1111/j.1365-2249.2004.02593.x
172. López Y, Yero D, Falero-Diaz G, Olivares N, Sarmiento ME, Sifontes S, et al. Induction of a protective response with an IgA monoclonal antibody against Mycobacterium tuberculosis 16kDa protein in a model of progressive pulmonary infection. Int J Med Microbiol. (2009) 299:447–52. doi: 10.1016/j.ijmm.2008.10.007
173. Teitelbaum R, Glatman-Freedman A, Chen B, Robbins JB, Unanue E, Casadevall A, et al. A mAb recognizing a surface antigen of Mycobacterium tuberculosis enhances host survival. Proc Natl Acad Sci U.S.A. (1998) 95:15688–93. doi: 10.1073/pnas.95.26.15688
174. Williams A, Reljic R, Naylor I, Clark SO, Falero-Diaz G, Singh M, et al. Passive protection with immunoglobulin A antibodies against tuberculous early infection of the lungs. Immunology. (2004) 111:328–33. doi: 10.1111/j.1365-2567.2004.01809.x
175. Buccheri S, Reljic R, Caccamo N, Meraviglia S, Ivanyi J, Salerno A, et al. Prevention of the post-chemotherapy relapse of tuberculous infection by combined immunotherapy. Tuberculosis (Edinb). (2009) 89:91–4. doi: 10.1016/j.tube.2008.09.001
176. Guirado E, Amat I, Gil O, Díaz J, Arcos V, Caceres N, et al. Passive serum therapy with polyclonal antibodies against Mycobacterium tuberculosis protects against post-chemotherapy relapse of tuberculosis infection in SCID mice. Microbes Infect. (2006) 8:1252–9. doi: 10.1016/j.micinf.2005.12.004
177. Olivares N, León A, López Y, Puig A, Cádiz A, Falero G, et al. The effect of the administration of human gamma globulins in a model of BCG infection in mice. Tuberculosis (Edinb). (2006) 86:268–72. doi: 10.1016/j.tube.2006.01.006
178. Roy E, Stavropoulos E, Brennan J, Coade S, Grigorieva E, Walker B, et al. Therapeutic efficacy of high-dose intravenous immunoglobulin in Mycobacterium tuberculosis infection in mice. Infect Immun. (2005) 73:6101–9. doi: 10.1128/IAI.73.9.6101-6109.2005
179. Olivares N, Puig A, Aguilar D, Moya A, Cádiz A, Otero O, et al. Prophylactic effect of administration of human gamma globulins in a mouse model of tuberculosis. Tuberculosis (Edinb). (2009) 89:218–20. doi: 10.1016/j.tube.2009.02.003
180. Reljic R, Ivanyi J. A case for passive immunoprophylaxis against tuberculosis. Lancet Infect Dis. (2006) 6:813–8. doi: 10.1016/S1473-3099(06)70658-2
181. Olivares N, Marquina B, Mata-Espinoza D, Zatarain-Barron ZL, Pinzón CE, Estrada I, et al. The protective effect of immunoglobulin in murine tuberculosis is dependent on IgG glycosylation. Pathog Dis. (2013) 69:176–83. doi: 10.1111/fim.2013.69.issue-3
182. Joller N, Weber SS, Müller AJ, Spörri R, Selchow P, Sander P, et al. Antibodies protect against intracellular bacteria by Fc receptor-mediated lysosomal targeting. Proc Natl Acad Sci U.S.A. (2010) 107(47):20441–6. doi: 10.1073/pnas.1013827107
183. Manivannan S, Rao NV, Ramanathan VD. Role of complement activation and antibody in the interaction between Mycobacterium tuberculosis and human macrophages. Indian J Exp Biol. (2012) 50(8):542–50.
184. Malik ZA, Denning GM, Kusner DJ. Inhibition of Ca(2+) signaling by Mycobacterium tuberculosis is associated with reduced phagosome-lysosome fusion and increased survival within human macrophages. J Exp Med. (2000) 191:287–302. doi: 10.1084/jem.191.2.287
185. Glatman-Freedman A, Mednick AJ, Lendvai N, Casadevall A. Clearance and organ distribution of Mycobacterium tuberculosis lipoarabinomannan (LAM) in the presence and absence of LAM-binding immunoglobulin M. Infect Immun. (2000) 68:335–41. doi: 10.1128/IAI.68.1.335-341.2000
186. Lux A, Aschermann S, Biburger M, Nimmerjahn F. The pro and anti-inflammatory activities of immunoglobulin G. Ann Rheum Dis. (2010) 69 Suppl 1:i92–6. doi: 10.1136/ard.2009.117101
187. Burton AR, Pallett LJ, McCoy LE, Suveizdyte K, Amin OE, Swadling L, et al. Circulating and intrahepatic antiviral B cells are defective in hepatitis B. J Clin Invest. (2018) 128:4588–603. doi: 10.1172/JCI121960
188. Sundling C, Rönnberg C, Yman V, Asghar M, Jahnmatz P, Lakshmikanth T, et al. B cell profiling in malaria reveals expansion and remodelling of CD11c+ B cell subsets. JCI Insight. (2019) 5(9):e126492. doi: 10.1172/jci.insight.126492
189. Ramakrishnan L. Revisiting the role of the granuloma in tuberculosis. Nat Rev Immunol. (2012) 12:352–66. doi: 10.1038/nri3211
190. Cadena AM, Fortune SM, Flynn JL. Heterogeneity in tuberculosis. Nat Rev Immunol. (2017) 17:691–702. doi: 10.1038/nri.2017.69
191. Russell DG, Cardona PJ, Kim MJ, Allain S, Altare F. Foamy macrophages and the progression of the human tuberculosis granuloma. Nat Immunol. (2009) 10:943–8. doi: 10.1038/ni.1781
192. Via LE, Lin PL, Ray SM, Carrillo J, Allen SS, Eum SY, et al. Tuberculous granulomas are hypoxic in Guinea pigs, rabbits, and nonhuman primates. Infect Immun. (2008) 76:2333–40. doi: 10.1128/IAI.01515-07
193. Gideon HP, Hughes TK, Tzouanas CN, Wadsworth MH 2nd, Tu AA, Gierahn TM, et al. Multimodal profiling of lung granulomas in macaques reveals cellular correlates of tuberculosis control. Immunity. (2022) 55:827–46.e10. doi: 10.1016/j.immuni.2022.04.004
194. Moreira-Teixeira L, Redford PS, Stavropoulos E, Ghilardi N, Maynard CL, Weaver CT, et al. T cell-derived IL-10 impairs host resistance to mycobacterium tuberculosis infection. J Immunol. (2017) 199:613–23. doi: 10.4049/jimmunol.1601340
195. de la Barrera S, Aleman M, Musella R, Schierloh P, Pasquinelli V, Garcia V, et al. IL-10 down-regulates costimulatory molecules on Mycobacterium tuberculosis-pulsed macrophages and impairs the lytic activity of CD4 and CD8 CTL in tuberculosis patients. Clin Exp Immunol. (2004) 138:128–38. doi: 10.1111/j.1365-2249.2004.02577.x
196. Kim H, Shin SJ. Revolutionizing control strategies against Mycobacterium tuberculosis infection through selected targeting of lipid metabolism. Cell Mol Life Sci. (2023) 80:291. doi: 10.1007/s00018-023-04914-5
197. Kaufmann SHE. The tuberculosis vaccine development pipeline: Present and future priorities and challenges for research and innovation. In: Migliori G. B., Raviglione M. C. eds. Essential Tuberculosis. Cham: Springer (2021). doi: 10.1007/978-3-030-66703-0_43
198. Radovic M, Ristic L, Stankovic I, Pejcic T, Rancic M, Ciric Z, et al. Chronic airflow obstruction syndrome due to pulmonary tuberculosis treated with directly observed therapy–a serious changes in lung function. Med Arh. (2011) 65:265–9. doi: 10.5455/medarh.
199. Cao J, Zhang L, Li D, Xu F, Huang S, Xiang Y, et al. IL-27 is elevated in patients with COPD and patients with pulmonary TB and induces human bronchial epithelial cells to produce CXCL10. Chest. (2012) 141:121–30. doi: 10.1378/chest.10-3297
200. Sarkar M, Srinivasa, Madabhavi I, Kumar K. Tuberculosis associated chronic obstructive pulmonary disease. Clin Respir J. (2017) 11:285–95. doi: 10.1111/crj.12621
201. Stek C, Allwood B, Walker NF, Wilkinson RJ, Lynen L, Meintjes G. The immune mechanisms of lung parenchymal damage in tuberculosis and the role of host-directed therapy. Front Microbiol. (2018) 9:2603. doi: 10.3389/fmicb.2018.02603
202. Allwood BW, Rigby J, Griffith-Richards S, Kanarek D, du Preez L, Mathot B, et al. Histologically confirmed tuberculosis-associated obstructive pulmonary disease. Int J Tuberc Lung Dis. (2019) 23:552–4. doi: 10.5588/ijtld.18.0722
203. Chin AT, Rylance J, Makumbirofa S, Meffert S, Vu T, Clayton J, et al. Chronic lung disease in adult recurrent tuberculosis survivors in Zimbabwe: a cohort study. Int J Tuberc Lung Dis. (2019) 23:203–11. doi: 10.5588/ijtld.18.0313
204. Singh S, Allwood BW, Chiyaka TL, Kleyhans L, Naidoo CC, Moodley S, et al. Immunologic and imaging signatures in post tuberculosis lung disease. Tuberculosis (Edinb). (2022) 136:102244. doi: 10.1016/j.tube.2022.102244
205. Salvi S. The silent epidemic of COPD in Africa. Lancet Glob Health. (2015) 3:e6–7. doi: 10.1016/S2214-109X(14)70359-6
206. Venkatesan P. GOLD report: 2022 update. Lancet Respir Med. (2022) 10:e20. doi: 10.1016/S2213-2600(21)00561-0
207. Rodriguez-Takeuchi SY, Renjifo ME, Medina FJ. Extrapulmonary tuberculosis: pathophysiology and imaging findings. Radiographics. (2019) 39:2023–37. doi: 10.1148/rg.2019190109
208. Margaret A, Macpherson C. Primary Tuberculosis of the Lung and some of its Consequences. Postgrad Med J. (1942) 18:139–41. doi: 10.1136/pgmj.18.201.139
209. Leeds IL, Magee MJ, Kurbatova EV, del Rio C, Blumberg HM, Leonard MK, et al. Site of extrapulmonary tuberculosis is associated with HIV infection. Clin Infect Dis. (2012) 55:75–81. doi: 10.1093/cid/cis303
210. Stop TB Partnership. Available online at: https://newtbvaccines.org/tb-vaccine-pipeline/clinical-phase/.
211. Nell AS, D'lom E, Bouic P, Sabaté M, Bosser R, Picas J, et al. Safety, tolerability, and immunogenicity of the novel antituberculous vaccine RUTI: randomized, placebo-controlled phase II clinical trial in patients with latent tuberculosis infection. PLoS One. (2014) 9:e89612. doi: 10.1371/journal.pone.0089612
212. Munseri P, Said J, Amour M, Magohe A, Matee M, Rees CA, et al. DAR-901 vaccine for the prevention of infection with Mycobacterium tuberculosis among BCG-immunized adolescents in Tanzania: A randomized controlled, double-blind phase 2b trial. Vaccine. (2020) 38:7239–45. doi: 10.1016/j.vaccine.2020.09.055
213. von Reyn CF, Lahey T, Arbeit RD, Landry B, Kailani L, Adams LV, et al. Safety and immunogenicity of an inactivated whole cell tuberculosis vaccine booster in adults primed with BCG: A randomized, controlled trial of DAR-901. PLoS One. (2017) 12(5):e0175215. doi: 10.1371/journal.pone.0175215
214. Lahey T, Laddy D, Hill K, Schaeffer J, Hogg A, Keeble J, et al. Immunogenicity and protective efficacy of the DAR-901 booster vaccine in a murine model of tuberculosis. PLoS One. (2016) 11:e0168521. doi: 10.1371/journal.pone.0168521
215. Tameris M, Mearns H, Penn-Nicholson A, Gregg Y, Bilek N, Mabwe S, et al. Live-attenuated Mycobacterium tuberculosis vaccine MTBVAC versus BCG in adults and neonates: a randomised controlled, double-blind dose-escalation trial. Lancet Respir Med. (2019) 7:757–70. doi: 10.1016/S2213-2600(19)30251-6
216. Nemes E, Geldenhuys H, Rozot V, Rutkowski KT, Ratangee F, Bilek N, et al. Prevention of M. tuberculosis infection with H4:IC31 vaccine or BCG revaccination. N Engl J Med. (2018) 379:138–49. doi: 10.1056/NEJMoa1714021
217. Cotton MF, Madhi SA, Luabeya AK, Tameris M, Hesseling AC, Shenje J, et al. Safety and immunogenicity of VPM1002 versus BCG in South African newborn babies: a randomised, phase 2 non-inferiority double-blind controlled trial. Lancet Infect Dis. (2022) 22:1472–83. doi: 10.1016/S1473-3099(22)00222-5
218. Tait DR, Hatherill M, Van Der Meeren O, Ginsberg AM, Van Brakel E, Salaun B, et al. Final analysis of a trial of M72/AS01(E) vaccine to prevent tuberculosis. N Engl J Med. (2019) 381:2429–39. doi: 10.1056/NEJMoa1909953
219. Tkachuk AP, Bykonia EN, Popova LI, Kleymenov DA, Semashko MA, Chulanov VP, et al. Safety and immunogenicity of the gamTBvac, the recombinant subunit tuberculosis vaccine candidate: A phase II, multi-center, double-blind, randomized, placebo-controlled study. Vaccines (Basel). (2020) 8(4):652. doi: 10.3390/vaccines8040652
220. Choi YH, Kang YA, Park KJ, Choi JC, Cho KG, Ko DY, et al. Safety and immunogenicity of the ID93 + GLA-SE tuberculosis vaccine in BCG-vaccinated healthy adults: A randomized, double-blind, placebo-controlled phase 2 trial. Infect Dis Ther. (2023) 12:1605–24. doi: 10.1007/s40121-023-00806-0
221. Lu JB, Chen BW, Wang GZ, Fu LL, Shen XB, Su C, et al. Recombinant tuberculosis vaccine AEC/BC02 induces antigen-specific cellular responses in mice and protects Guinea pigs in a model of latent infection. J Microbiol Immunol Infect. (2015) 48:597–603. doi: 10.1016/j.jmii.2014.03.005
222. Dijkman K, Lindenstrøm T, Rosenkrands I, Søe R, Woodworth JS, Lindestam Arlehamn CS, et al. A protective, single-visit TB vaccination regimen by co-administration of a subunit vaccine with BCG. NPJ Vaccines. (2023) 8:66. doi: 10.1038/s41541-023-00666-2
223. Jeyanathan M, Fritz DK, Afkhami S, Aguirre E, Howie KJ, Zganiacz A, et al. Aerosol delivery, but not intramuscular injection, of adenovirus-vectored tuberculosis vaccine induces respiratory-mucosal immunity in humans. JCI Insight. (2022) 7(3):e155655. doi: 10.1172/jci.insight.155655
224. Vasilyev K, Shurygina AP, Zabolotnykh N, Sergeeva M, Romanovskaya-Romanko E, Pulkina A, et al. Enhancement of the Local CD8(+) T-Cellular Immune Response to Mycobacterium tuberculosis in BCG-Primed Mice after Intranasal Administration of Influenza Vector Vaccine Carrying TB10.4 and HspX Antigens. Vaccines (Basel). (2021) 9(11):1273. doi: 10.3390/vaccines9111273
225. Sergeeva M, Romanovskaya-Romanko E, Zabolotnyh N, Pulkina A, Vasilyev K, Shurigina AP, et al. Mucosal Influenza Vector Vaccine Carrying TB10.4 and HspX Antigens Provides Protection against Mycobacterium tuberculosis in Mice and Guinea Pigs. Vaccines (Basel). (2021) 9(4):394. doi: 10.3390/vaccines9040394
226. Wilkie M, Satti I, Minhinnick A, Harris S, Riste M, Ramon RL, et al. A phase I trial evaluating the safety and immunogenicity of a candidate tuberculosis vaccination regimen, ChAdOx1 85A prime - MVA85A boost in healthy UK adults. Vaccine. (2020) 38:779–89. doi: 10.1016/j.vaccine.2019.10.102
227. Lai R, Ogunsola AF, Rakib T, Behar SM. Key advances in vaccine development for tuberculosis-success and challenges. NPJ Vaccines. (2023) 8:158. doi: 10.1038/s41541-023-00750-7
228. Kline KA, Fälker S, Dahlberg S, Normark S, Henriques-Normark B. Bacterial adhesins in host-microbe interactions. Cell Host Microbe. (2009) 5:580–92. doi: 10.1016/j.chom.2009.05.011
229. Govender VS, Ramsugit S, Pillay M. Mycobacterium tuberculosis adhesins: potential biomarkers as anti-tuberculosis therapeutic and diagnostic targets. Microbiol (Reading). (2014) 160:1821–31. doi: 10.1099/mic.0.082206-0
230. García B, Merayo-Lloves J, Martin C, Alcalde I, Quirós LM, Vazquez F. Surface proteoglycans as mediators in bacterial pathogens infections. Front Microbiol. (2016) 7:220. doi: 10.3389/fmicb.2016.00220
231. Pethe K, Alonso S, Biet F, Delogu G, Brennan MJ, Locht C, et al. The heparin-binding haemagglutinin of M. tuberculosis is required for extrapulmonary dissemination. Nature. (2001) 412:190–4. doi: 10.1038/35084083
232. Divangahi M, Aaby P, Khader SA, Barreiro LB, Bekkering S, Chavakis T, et al. Trained immunity, tolerance, priming and differentiation: distinct immunological processes. Nat Immunol. (2021) 22:2–6. doi: 10.1038/s41590-020-00845-6
233. Nankabirwa V, Tumwine JK, Mugaba PM, Tylleskär T, Sommerfelt H, PROMISE- EBF Study Group. Child survival and BCG vaccination: a community based prospective cohort study in Uganda. BMC Public Health. (2015) 15:175. doi: 10.1186/s12889-015-1497-8
234. Netea MG, Domínguez-Andrés J, Barreiro LB, Chavakis T, Divangahi M, Fuchs E, et al. Defining trained immunity and its role in health and disease. Nat Rev Immunol. (2020) 20:375–88. doi: 10.1038/s41577-020-0285-6
235. Koeken VACM, Verrall AJ, Netea MG, Hill PC, van Crevel R. Trained innate immunity and resistance to Mycobacterium tuberculosis infection. Clin Microbiol Infect. (2019) 25:1468–72. doi: 10.1016/j.cmi.2019.02.015
236. Ostadrahimi A, Ahmadikhatir S, Amirazad H, EivaziZiaei J, AsghariJafarabadi M, Barzeghari A, et al. Effect of beta glucan on white blood cell counts and serum levels of IL-4 and IL-12 in women with breast cancer undergoing chemotherapy: a randomized double-blind placebo-controlled clinical trial. Asian Pac J Cancer Prev. (2014) 15:5733–9. doi: 10.7314/APJCP.2014.15.14.5733
237. Bashir KMI, Choi JS. Clinical and physiological perspectives of β-glucans: The past, present, and future. Int J Mol Sci. (2017) 18(9):1906. doi: 10.3390/ijms18091906
238. Vetvicka V, Vannucci L, Sima P, Richter J. Beta glucan: Supplement or drug? From laboratory to clinical trials. Molecules. (2019) 24(7):1251. doi: 10.3390/molecules24071251
239. Moorlag SJCFM, Khan N, Novakovic B, Kaufmann E, Jansen T, van Crevel R, et al. β-Glucan Induces Protective Trained Immunity against Mycobacterium tuberculosis Infection: A Key Role for IL-1. Cell Rep. (2020) 31:107634. doi: 10.1016/j.celrep.2020.107634
240. Mitroulis I, Ruppova K, Wang B, Chen LS, Grzybek M, Grinenko T, et al. Modulation of myelopoiesis progenitors is an integral component of trained immunity. Cell. (2018) 172:147–61.e12. doi: 10.1016/j.cell.2017.11.034
241. Kaufmann E, Sanz J, Dunn JL, Khan N, Mendonça LE, Pacis A, et al. BCG educates hematopoietic stem cells to generate protective innate immunity against tuberculosis. Cell. (2018) 172:176–190.e19. doi: 10.1016/j.cell.2017.12.031
242. Morris D, Gonzalez B, Khurasany M, Kassissa C, Luong J, Kasko S, et al. Characterization of dendritic cell and regulatory T cell functions against Mycobacterium tuberculosis infection. BioMed Res Int. (2013) 2013:402827. doi: 10.1155/2013/402827
243. Sia JK, Bizzell E, Madan-Lala R, Rengarajan J. Engaging the CD40-CD40L pathway augments T-helper cell responses and improves control of Mycobacterium tuberculosis infection. PLoS Pathog. (2017) 13:e1006530. doi: 10.1371/journal.ppat.1006530
244. Griffiths KL, Ahmed M, Das S, Gopal R, Horne W, Connell TD, et al. Targeting dendritic cells to accelerate T-cell activation overcomes a bottleneck in tuberculosis vaccine efficacy. Nat Commun. (2016) 7:13894. doi: 10.1038/ncomms13894
245. Zhuang L, Ye Z, Li L, Yang L, Gong W. Next-generation TB vaccines: progress, challenges, and prospects. Vaccines (Basel). (2023) 11(8):1304. doi: 10.3390/vaccines11081304
246. Backert L, Kohlbacher O. Immunoinformatics and epitope prediction in the age of genomic medicine. Genome Med. (2015) 7:119. doi: 10.1186/s13073-015-0245-0
247. Reche P, Flower DR, Fridkis-Hareli M, Hoshino Y. Peptide-based immunotherapeutics and vaccines 2017. J Immunol Res. (2018) 2018:4568239. doi: 10.1155/2018/4568239
248. Li W, Joshi MD, Singhania S, Ramsey KH, Murthy AK. Peptide vaccine: progress and challenges. Vaccines (Basel). (2014) 2:515–36. doi: 10.3390/vaccines2030515
249. Malonis RJ, Lai JR, Vergnolle O. Peptide-based vaccines: Current progress and future challenges. Chem Rev. (2020) 120:3210–29. doi: 10.1021/acs.chemrev.9b00472
250. Ortega-Tirado D, Arvizu-Flores AA, Velazquez C, Garibay-Escobar A. The role of immunoinformatics in the development of T-cell peptide-based vaccines against Mycobacterium tuberculosis. Expert Rev Vaccines. (2020) 19:831–41. doi: 10.1080/14760584.2020.1825950
251. Liu X, Li H, Li S, Yuan J, Pang Y. Maintenance and recall of memory T cell populations against tuberculosis: Implications for vaccine design. Front Immunol. (2023) 14:1100741. doi: 10.3389/fimmu.2023.1100741
252. Casadevall A, Pirofski LA. A reappraisal of humoral immunity based on mechanisms of antibody-mediated protection against intracellular pathogens. Adv Immunol. (2006) 91:1–44. doi: 10.1016/S0065-2776(06)91001-3
253. Angala SK, Belardinelli JM, Huc-Claustre E, Wheat WH, Jackson M. The cell envelope glycoconjugates of Mycobacterium tuberculosis. Crit Rev Biochem Mol Biol. (2014) 49:361–99. doi: 10.3109/10409238.2014.925420
254. Glatman-Freedman A, Casadevall A, Dai Z, Jacobs WR Jr, Li A, Morris SL, et al. Antigenic evidence of prevalence and diversity of Mycobacterium tuberculosis arabinomannan. J Clin Microbiol. (2004) 42(7):3225–31. doi: 10.1128/JCM.42.7.3225-3231.2004
255. Hamasur B, Haile M, Pawlowski A, Schröder U, Williams A, Hatch G, et al. Mycobacterium tuberculosis arabinomannan-protein conjugates protect against tuberculosis. Vaccine. (2003) 21:4081–93. doi: 10.1016/S0264-410X(03)00274-3
256. Bottai D, Frigui W, Clark S, Rayner E, Zelmer A, Andreu N, et al. Increased protective efficacy of recombinant BCG strains expressing virulence-neutral proteins of the ESX-1 secretion system. Vaccine. (2015) 33:2710–8. doi: 10.1016/j.vaccine.2015.03.083
257. Karbalaei Zadeh Babaki M, Soleimanpour S, Rezaee SA. Antigen 85 complex as a powerful Mycobacterium tuberculosis immunogene: Biology, immune-pathogenicity, applications in diagnosis, and vaccine design. Microb Pathog. (2017) 112:20–9. doi: 10.1016/j.micpath.2017.08.040
258. Wang X, Barnes PF, Dobos-Elder KM, Townsend JC, Chung YT, Shams H, et al. ESAT-6 inhibits production of IFN-gamma by Mycobacterium tuberculosis-responsive human T cells. J Immunol. (2009) 182:3668–77. doi: 10.4049/jimmunol.0803579
Keywords: tuberculosis, M. tuberculosis, pathogenesis, immune evasion, vaccine
Citation: Yang H, Lei X, Chai S, Su G and Du L (2024) From pathogenesis to antigens: the key to shaping the future of TB vaccines. Front. Immunol. 15:1440935. doi: 10.3389/fimmu.2024.1440935
Received: 30 May 2024; Accepted: 04 July 2024;
Published: 23 July 2024.
Edited by:
Juraj Ivanyi, King’s College London, United KingdomReviewed by:
Clara Espitia, National Autonomous University of Mexico, MexicoUtpal Sengupta, The Leprosy Mission Trust India, India
Copyright © 2024 Yang, Lei, Chai, Su and Du. This is an open-access article distributed under the terms of the Creative Commons Attribution License (CC BY). The use, distribution or reproduction in other forums is permitted, provided the original author(s) and the copyright owner(s) are credited and that the original publication in this journal is cited, in accordance with accepted academic practice. No use, distribution or reproduction is permitted which does not comply with these terms.
*Correspondence: Guimin Su, c3VndWltaW5AemhpZmVpc2hlbmd3dS5jb20=; Lin Du, ZHVsaW5AemhpZmVpc2hlbmd3dS5jb20=