- 1School of Chemistry and Biochemistry, Georgia Institute of Technology, Atlanta, GA, United States
- 2Wallace H. Coulter Department of Biomedical Engineering, Georgia Institute of Technology and Emory University, Atlanta, GA, United States
- 3George W. Woodruff School of Mechanical Engineering, Georgia Institute of Technology, Atlanta, GA, United States
Expressed on the surface of CD8+ T cells, the CD8 co-receptor is a key component of the T cells that contributes to antigen recognition, immune cell maturation, and immune cell signaling. While CD8 is widely recognized as a co-stimulatory molecule for conventional CD8+ αβ T cells, recent reports highlight its multifaceted role in both adaptive and innate immune responses. In this review, we discuss the utility of CD8 in relation to its immunomodulatory properties. We outline the unique structure and function of different CD8 domains (ectodomain, hinge, transmembrane, cytoplasmic tail) in the context of the distinct properties of CD8αα homodimers and CD8αβ heterodimers. We discuss CD8 features commonly used to construct chimeric antigen receptors for immunotherapy. We describe the molecular interactions of CD8 with classical MHC-I, non-classical MHCs, and Lck partners involved in T cell signaling. Engineered and naturally occurring CD8 mutations that alter immune responses are discussed. The applications of anti-CD8 monoclonal antibodies (mABs) that target CD8 are summarized. Finally, we examine the unique structure and function of several CD8/mAB complexes. Collectively, these findings reveal the promising immunomodulatory properties of CD8 and CD8 binding partners, not only to uncover basic immune system function, but to advance efforts towards translational research for targeted immunotherapy.
1 Introduction
The immune system is a complex network of molecular interactions and cellular responses many of which involve and/or depend on the function of cells expressing either CD4 or CD8 co-receptors on their surfaces (1, 2). CD4 expresses primarily on the surface of helper T cells whereas CD8 expresses on the surface of cytotoxic and suppressor T cells (3). CD8+ T cells detect pathogens, cancer, and autoimmunity towards eliminating diseased cells through T cell antigen receptor (TCR) recognition of antigens presented by classical and non-classical major histocompatibility complex class I (MHC-I) molecules on the surface of all nucleated cells (4). CD4 and CD8 are termed co-receptors because they bind the same MHC ligand as the receptor TCR. However, CD4 and CD8 associate with the membrane-proximal domains of the MHC-II and I molecules, respectively, as opposed to the TCR, which binds the membrane-distal domains of the MHC-II and I molecules, respectively (5, 6). CD8 is a dimeric receptor that contains several domains: ectodomain, hinge, transmembrane, and cytoplasmic tail (7). CD8 primarily acts as a co-stimulator, and occasionally as a co-repressor, of immune responses (7, 8). Its mechanism of action is thought to occur through several cooperative events, each differing based on the cell type and the immunoreceptors involved. Firstly, the association of CD8 with MHC-I stabilizes TCR/antigen/MHC-I complexes (9–13). High affinity TCR/antigen/MHC-I interactions (KD < 10 μM) can result in CD8+ T cell activation without the need for CD8 (7, 14, 15). However, in cases of low to medium affinity TCR/antigen/MHC-I interactions (KD > 30 μM), CD8 stabilizes the TCR/antigen/MHC-I complex to enhance recognition stability, sensitivity, and specificity (7, 14, 15). CD8 also participates in immune cell mechanotransduction by promoting dynamic catch bonds that result from the cooperativity between TCR/CD8/antigen/MHC-I interactions (16). It should be noted that several groups have identified non-canonical TCR/MHC docking modes for which some conformations could still accommodate CD8 binding to the MHC (17–20). However, several pieces of evidence suggest some of these non-canonical binding modes (i.e., reverse polarity TCR binding) would not allow for robust CD8 interaction with the MHC (21–23). Secondly, the cytoplasmic tail of CD8 binds and recruits the lymphocyte-specific protein tyrosine kinase, p56Lck (Lck), to the TCR/CD3 complex. Here, Lck helps in initiating TCR signaling by phosphorylating immunoreceptor tyrosine-based activation motifs (ITAMs) located within the cytoplasmic tails of CD3γ, CD3δ, CD3ϵ, and CD3ζ subunits associated with the TCR (24–26). The phosphorylated ITAMs serve as docking sites for another kinase, ZAP-70, which phosphorylates downstream signaling proteins, Linker for activation of T cells (LAT) and SLP-76, ultimately resulting in the release of cytokines, granzymes, and perforin towards the target cell (27, 28). Beyond its classical role in CD8+ cytotoxic T cell signaling, CD8 has also demonstrated contributions to T cell development/maturation, T cell differentiation, immune responses in a wide range of unconventional immune cell subsets, and cross-talk with B cell mediated responses (several of these are discussed in section 2.1).
As new studies elucidate the complexities of immune regulation, there is a need to unravel how the CD8 co-receptor contributes to immune responses, either as a co-stimulator or co-repressor. This exploration naturally extends to the realm of monoclonal antibodies (mABs), potent immunomodulators known for their specificity and affinity, used in treating cancer, pathogen infections, autoimmune diseases, and for organ transplantation (29–31). Several anti-CD8 mABs have been discovered to exhibit interesting effects on immune cell signaling and development, highlighting CD8 and the immunological synapse as a novel strategy for targeted immunomodulation (Tables 1, 2, Figure 1D). In this review, we highlight the structure and function of CD8 through the lens of its use as a target for immunomodulation. We also outline what is known (and what remains poorly understood) regarding the mechanistic understanding of how mABs engage with CD8 to promote or abrogate T cell signaling.
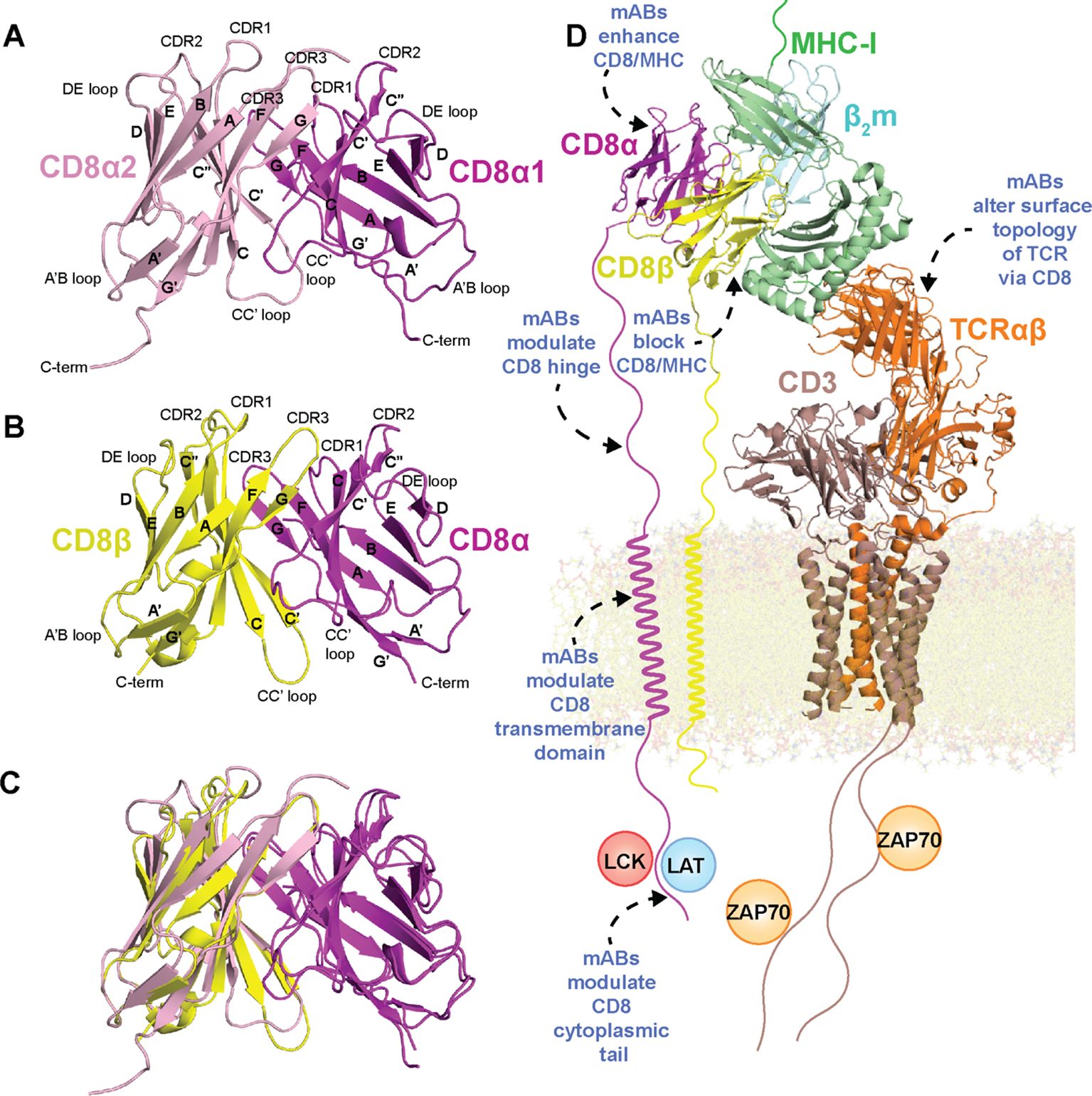
Figure 1. Structure of CD8 isoforms and placement within the immunological synapse with potential strategies for immunomodulation. (A) Crystal structure of mouse CD8αα ectodomain (PDB ID 1BQH). (B) Crystal structure of mouse CD8αβ ectodomain (PDB ID 2ATP). (C) Overlay of mouse CD8αα with mouse CD8αβ ectodomain. (D) Molecular model of the immunological synapse derived from the cryo-EM structure of the MHC-I/TCR/CD3 complex (PDB ID 7PHR) aligned to mouse CD8αα/H-2Kb (PDB ID 1BQH). The complex was adapted from a model presented by Pandey et al. (56). MHC-I heavy chain is colored green, β2m light chain colored cyan, peptide antigen colored salmon, CD8α colored magenta, CD8β colored yellow, TCR chains colored orange, and CD3 chains colored brown. Potential mechanisms for immunomodulation of CD8 structure/interactions by mABs (described in detail in the text) are highlighted with blue text/dotted arrows.
2 CD8 structure and function
2.1 Distinct functional roles for CD8αα and CD8αβ in CD8+ T cell signaling and T cell development
CD8+ T cells play diverse roles in biology, ranging from antigen recognition in adaptive and innate immune responses for combating pathogen infection, cancer, and autoimmune disease, to cross-talking with B cell responses (57–59). Landmark studies revealed that the elimination of T cells expressing CD8α and CD8β chains resulted in a complete loss of immune cell-mediated cytotoxic responses (60–62). CD8 is expressed on CD8+ T cell surfaces in three possible isoforms: a CD8αα homodimer, a CD8ββ homodimer, and a CD8αβ heterodimer (63–65). Most commonly, one of the three isoforms is expressed in the absence of the other two. However, not all T cells express CD8; CD8-/CD4+ T cell and CD8-/CD4- T cell subsets also exist (66). CD8+ T cell subtypes expressing CD8αα, CD8αβ, or CD8ββ are largely distinct (64, 67). CD8+ T cell subsets rarely express both CD8αα and CD8αβ (63, 64). CD8αβ is primarily expressed on the surface of conventional naïve cytotoxic T cells, mature cytotoxic T cells, memory T cells, natural killer T cells, mucosal associated invariant T cells, and γδ T cells (64, 67, 68). CD8αα is primarily expressed on the surface of intraepithelial T cells, thymocytes, conventional cytotoxic T cells, γδ T cells, memory T cells, natural killer T cells, mucosal associated invariant T cells, and dendritic cells (64, 69–72). CD8αα and CD8αβ are structurally similar but functionally distinct (72) (discussed in sections 2.2 through 2.6). The biological relevance of CD8ββ is largely contested since the CD8β chain is retained intracellularly in the absence of CD8α in some species but not others (65). Although the expression of CD8ββ has been demonstrated in some cell types, its structure/function remain poorly understood and are yet to be characterized (73).
Several reports from Sherman, Littman, and Mescher revealed CD8 as a main player in T cell activation where CD8/MHC-I binding serves as a TCR-activated adhesion-signaling system through cooperation with several other adhesion interactions (LFA-1/ICAM, VLA-ECM) (74–76). The adhesion property of CD8 with MHC-I was confirmed through several studies to be mediated by the MHC-I α3 domain (77–80). Purified MHC-I molecules immobilized on plastic were both necessary and sufficient to stimulate cytotoxic T cells in a TCR and CD8 dependent fashion (81, 82). Soluble anti-CD8 antibodies were able to inhibit TCR-activated binding and response to non-antigenic MHC-I (promoted by an anti-TCR antibody), further supporting a direct MHC-I/CD8 interaction contributes to T cell responses via an adhesion model (83). CD8αβ, and potentially CD8ββ, plays a role as a co-stimulatory molecule of CD8+ T cell signaling through its interaction with classical and non-classical MHC-I molecules (84) (discussed in section 2.3). CD8αα also interacts with classical and non-classical MHC-I molecules to function as a co-repressor for killer cell immunoglobulin-like receptors (KIRs) on natural killer (NK) cells (85). Both CD8αα and CD8αβ can associate with Lck via the CD8α cytoplasmic tail to promote CD3 ITAM phosphorylation, although the efficiency of this process seems to vary between CD8 isoforms (discussed in section 2.6). For example, in conventional CD8+ T cell subsets interacting with classical MHC-I molecules, CD8αβ has been observed to be ~100 times stronger of a co‐stimulator than CD8αα due to increased localization in lipid rafts for efficient Lck recruitment (86). Apart from its role as a co-stimulatory molecule of immune cell signaling, CD8αβ is involved in CD8+ T-lineage cell development, including thymocyte selection, maturation, and differentiation into memory and other classical and non-classical subsets (87–89). CD8αα is not a functional homolog of CD8αβ (72). While CD8αα’s function remains somewhat elusive, it has been associated with negative regulation of intestinal intraepithelial T cells that carry out cytotoxic functions in gastrointestinal and reproductive tracts (90). In this context, unlike CD8αβ, CD8αα decreases sensitivity of TCRs towards antigens (8). Additionally, CD8αα plays critical roles in generation of virus-specific memory T cells after infection (91, 92). Altogether, these studies reveal unique functional roles for CD8αα and CD8αβ isoforms in immune cell function and development, highlighting a wide range of responses to target for immunomodulation.
2.2 Evolutionary conservation of CD8α and CD8β chains
Amino acid sequence alignments of CD8α and CD8β chains across species indicate that despite sharing low sequence identity (~20 to 60% between species), several conserved sites are found in each of the ectodomain, hinge, transmembrane, and cytoplasmic tail (35, 40) (Figures 2, 3). There are 24 and 21 fully conserved residues in CD8α and CD8β chains, respectively. The presence of these conserved sites suggests important structural and functional roles. For example, the ectodomain alignment highlights several conserved residues required for the stability of CD8’s immunoglobulin (Ig) fold and the intermolecular interface. The Cys residues in the ectodomain involved in the formation of an intramolecular disulfide bond, which stabilize CD8α and CD8β Ig-like folds, is also conserved. Cys residues in the hinge and transmembrane domains involved in an intermolecular disulfide bond, which stabilize CD8αα and CD8αβ dimers, are also conserved. Additionally, several CD8 residues involved in the interaction with MHC-I (ectodomain) and Lck (cytoplasmic tail) are partially conserved. Finally, several CD8 residues involved in transmembrane domain localization and interactions with lipid membrane are conserved. These conserved sites are attractive targets for immunomodulatory mABs that exhibit cross-species reactivity (discussed in section 3 and 4) (Figure 1D).
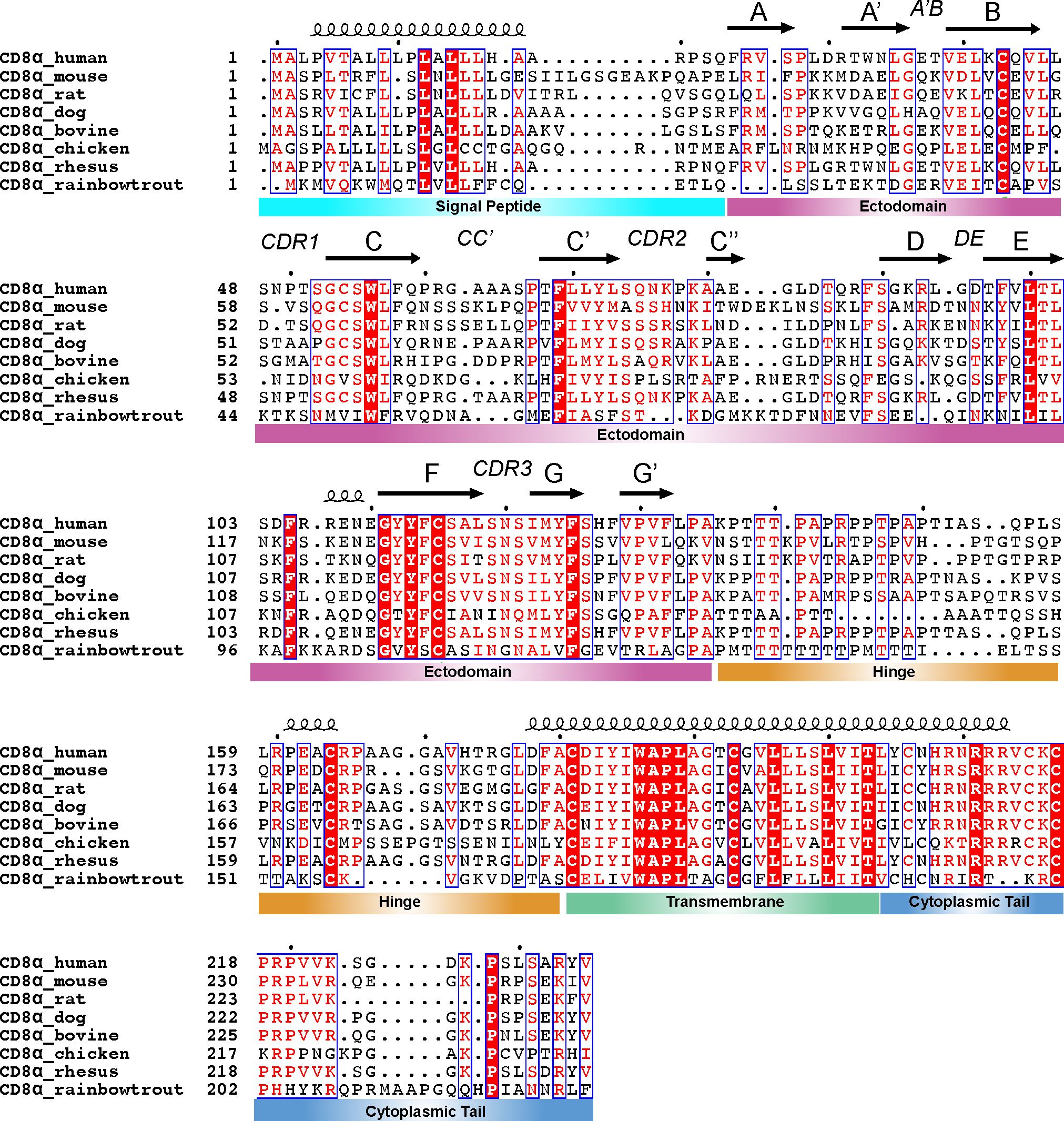
Figure 2. Sequence conservation of CD8α domains across species. Clustal Omega (v1.2.4) alignment (93) of sequences for CD8α from human (UniProt #P01732), mouse (UniProt #P01731), rat (UniProt #P07725), dog (UniProt #P33706), bovine (UniProt #P31783), chicken (UniProt #A0A8V0YYR0), rhesus monkey (UniProt #F7DXK3), and rainbow trout (UniProt #Q9IAL5). The secondary structure of each domain above the sequence is derived from the AlphaFold2 prediction of human CD8α (UniProt AF-P01732-F1). The alignment was processed in ESPript (v3) with domain disambiguation added manually (94). The dots above the sequence highlight the first residue (residue 1) and then every 10 residues after that using the first sequence as a reference.
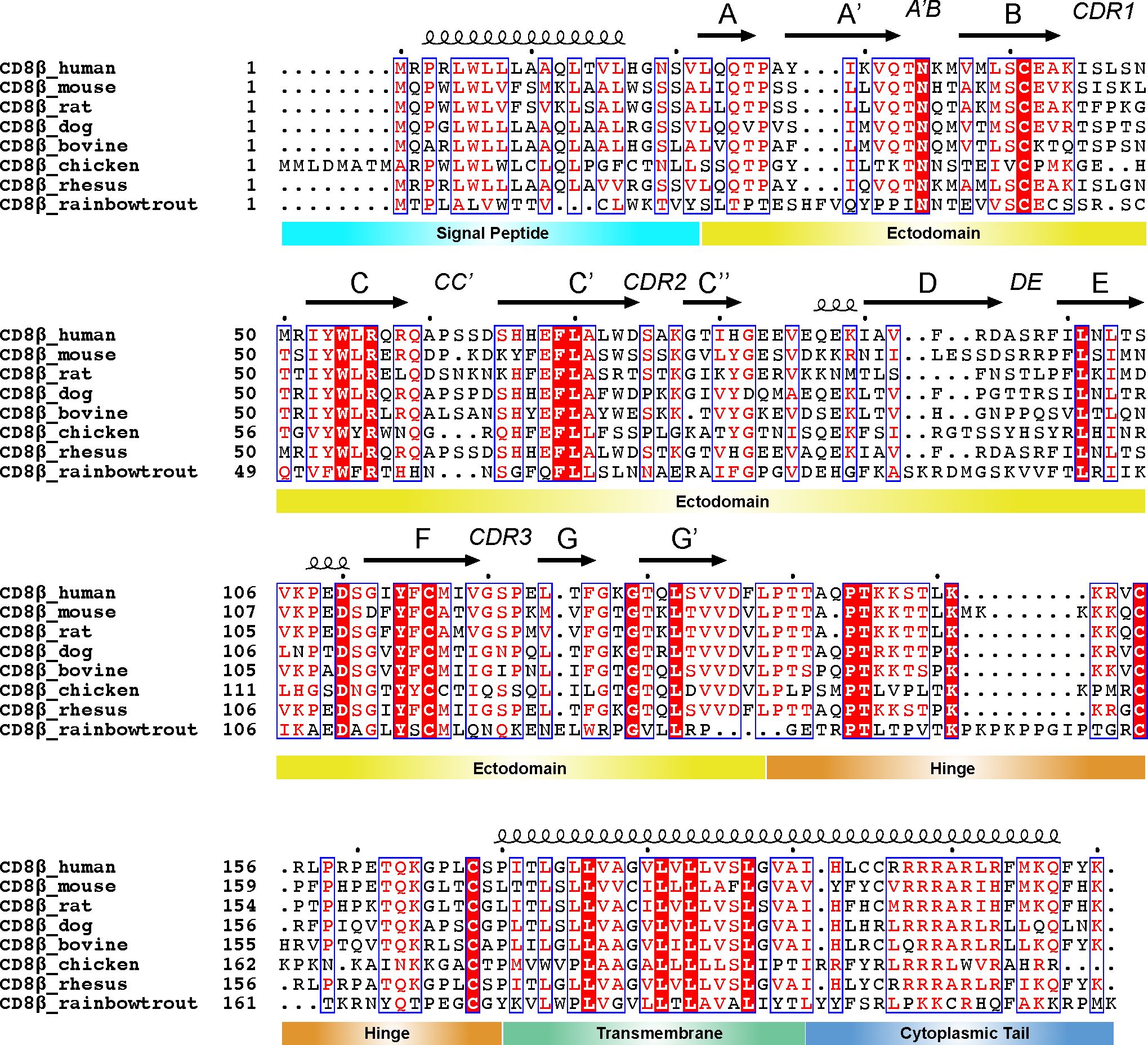
Figure 3. Sequence conservation of CD8β domains across species. Clustal Omega (v1.2.4) alignment (93) of sequences for CD8β from human (UniProt #P10966), mouse (UniProt #P10300), rat (UniProt #P05541), dog (UniProt #A0A8C0MNN1), bovine (UniProt #A7YW30), chicken (UniProt #A0A8V0Z0D5), rhesus monkey (UniProt #F7EXY4), and rainbow trout (UniProt #A0A8C7PPI8). The secondary structure of each domain above the sequence is derived from the AlphaFold2 prediction of human CD8β (UniProt AF-P10966-F1). The alignment was processed in ESPript (v3) with domain disambiguation added manually (94). The dots above the sequence highlight the first residue (residue 1) and then every 10 residues after that using the first sequence as a reference.
2.3 CD8 structure – the ectodomain binds to MHC-I and MHC-I related molecules to promote stability of MHC/TCR complexes
The CD8 ectodomain is an extracellular domain (amino acids 22-135 for CD8α and 22-138 for CD8β in humans) that takes on at least two forms: the CD8αα homodimer or CD8αβ heterodimer (7, 39). CD8ββ may provide a third isoform in some species, although its structure and function is poorly understood (65, 95). Crystal structures of the N-terminal globular domain of CD8αβ and CD8αα ectodomains reveal strikingly similar structural characteristics: an Ig-like fold consisting of eleven β-strands (termed A, A’, B, C, C’, C’’, D, E, F, G, and G’’) and six major loops (termed CDR1, CDR2, CDR3, DE, CC’, and A’B) (32) (Figures 2, 3, 1A, B). The dimer interface surface area differs between CD8αβ and CD8αα (~1914 Å2 versus ~2290 Å2) (32). The Cα RMSD between CD8αβ and CD8αα is ~1.16 Å, highlighting the overall similarity in the orientation of each CD8 chains in the two dimers (32) (Figure 1C).
CD8αα and CD8αβ ectodomains each interact with classical and non-classical MHC-I molecules using mostly similar binding modes (Table 1, Figure 4). While the exact positioning of the CD8 ectodomain varies across the different complexes, overall, the CD8β chain occupies a position equivalent to CD8α1, proximal to the T cell membrane, while the CD8α subunit of CD8αβ is positioned similarly to CD8α2, distal to the T cell membrane (Figures 1D, 4). In crystal structures of mouse CDαβ/H-2Dd and mouse CD8αα/H-2Kb complexes, hydrogen bonds, salt bridges, and hydrophobic interactions stabilize interactions between CD8β and CD8α CDR1, CDR2, and CDR3 loops with a conformationally flexible loop on the MHC-I heavy chain α3 domain (39, 40) (Figure 4). Likewise, in crystal structures of human CDαα/HLA-A∗02:01 and human CD8αα/HLA-A∗24:02 complexes, CD8α contacts not only the MHC-I α3 domain, but also the MHC-I α2 helix and invariant light chain β2-microglobulin (β2m) (37, 38) (Figure 4). The structures of CDαα or CD8αβ with other HLA alleles (i.e., HLA-B, HLA-C, HLA-G, etc.) are not available, but are expected to exhibit similar binding modes. Species-dependent differences in CD8/MHC binding modes have been reported. For example, crystal structures of chicken CDαα with BF2*04:01 and BF2*15:01 reveals two distinct modes: a classical antibody-like binding mode characteristic of most CD8/MHC-I complexes and a “face-to-face” binding mode that tilts the orientation of CD8αα homodimer relative to the MHC-I (41) (Figure 4), although the functional relevance of the second binding mode is unclear. The utility of anti-CD8 mABs for targeting CD8 ectodomain/MHC-I interactions is discussed in detail in section 4.
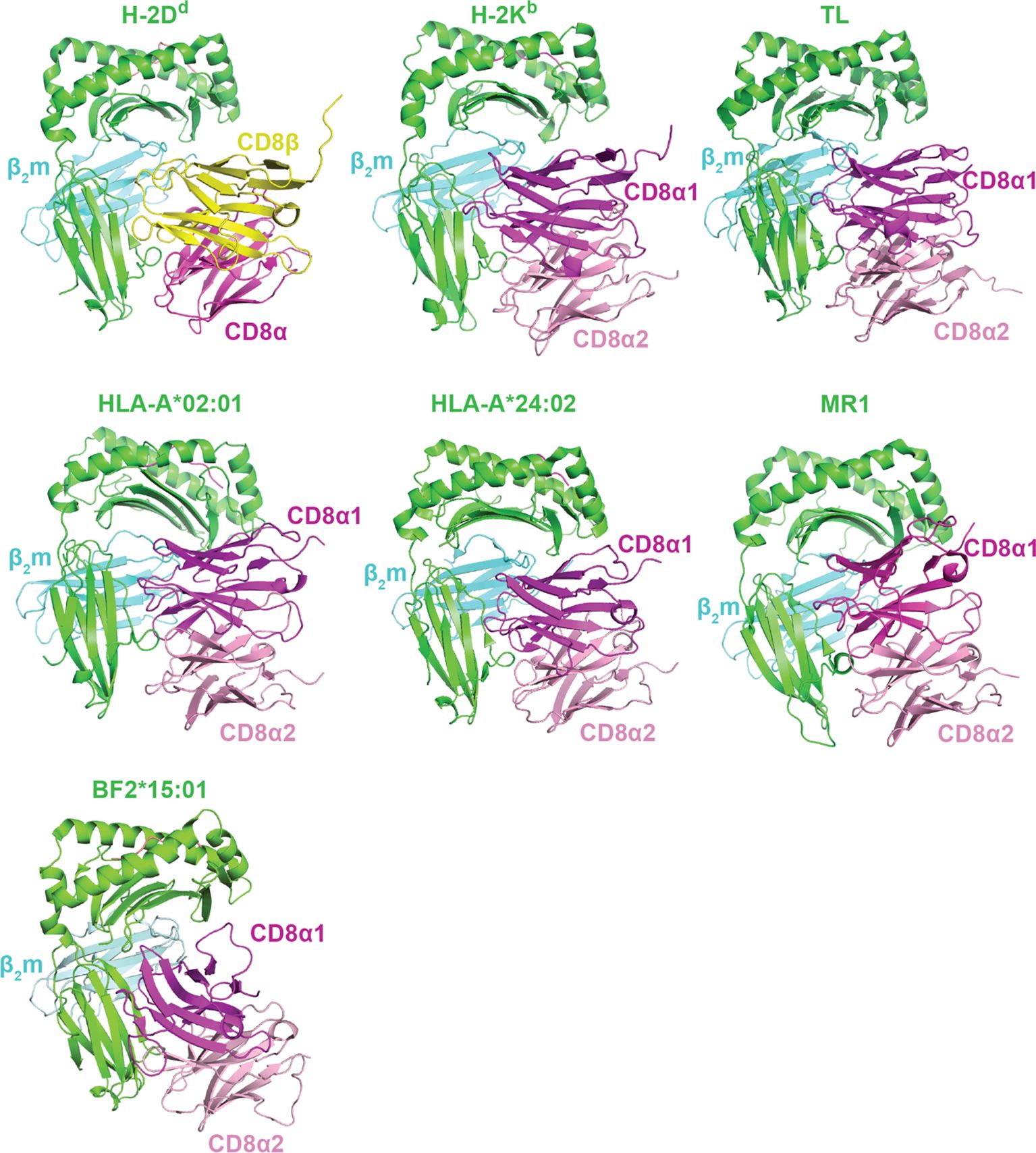
Figure 4. Binding modes of CD8αα and CD8αβ isoforms with classical and non-classical MHC-I molecules. A summary of crystal structures of CD8 ectodomain/MHC-I complexes. From top left to bottom right: mouse CD8αβ/H-2Dd (PDB ID 3DMM), mouse CD8αα/H-2Kb (PDB ID 1BQH), mouse CDαα/TL (PDB ID 1NEZ), human CD8αα/HLA-A*02:01 (PDB ID 1AKJ), human CD8αα/HLA-A*24:02 (PDB ID 3QZW), human CD8αα/MR1 (PDB ID 7UMG), and chicken CD8αα/BF2*15:01 (PDB ID 6LHF). MHC heavy chains are colored green, β2m light chains colored cyan, antigens colored salmon, CD8α chains are colored magenta/light pink, and CD8β chains are colored yellow.
Classical MHC-I molecules are encoded by one of the most polymorphic genes described to date, with thousands of alleles that vary from person to person (96). In contrast, the gene encoding CD8 in humans is invariant and non-polymorphic, typically showing little variations between individuals, although limited allelic variation of CD8 is found in some species (97). Like CD8, non-classical MHC-I molecules generally exhibit minimal genetic diversity and hence have the potential to be therapeutic targets that are more universal compared to classical MHC-I (98). Mutations and polymorphisms in the MHC-I α3 domain have been shown to either enhance or disrupt CD8/MHC-I binding (80, 99). Mutations at conserved residues within the MHC-I α3 domain (i.e., residues 222, 223, 227, 228, 229, 245) abrogate binding to CD8 and reduces T cell activation despite maintaining TCR binding (11, 37, 78, 100–103). For example, the well described MHC-I α3 domain D227K/T228A mutant disrupts association with CD8 by eliminating a hydrogen bond between D227 (on MHC-I) and Y51 (on CD8α) as well as contacts between T228A (on MHC-I) and T30/N99 (on CD8α) (11, 37, 40).
Surface plasmon resonance (SPR) and micropipette adhesion frequency assay have been used to quantify differences between CD8αα and CD8αβ ectodomains interactions with classical or non-classical MHC-I molecules in solution (i.e., three-dimensional, or 3D, binding) and on T cell membrane (i.e., two-dimensional, or 2D, binding). The formation of CD8/MHC-I complexes doesn’t seem to be largely dependent on the identity of the bound peptide antigen for most cases (104, 105). CD8αα and CD8αβ usually exhibit similar affinities to the same MHC-I molecule (40, 105), which is interesting considering functional differences between the two CD8 isoforms. In general, CD8αα and CD8αβ interact with classical and non-classical MHC-I molecules with moderate to weak affinities in the range of KD ~10 to 500 µM in 3D (40, 42, 43, 103, 106) and AcKA ~10-6 μm4 in 2D (12, 16). Most mABs have a 3D KD ~ nM and 2D AcKA ~10-1 μm4 for their specific antigens (107), which should have no problem blocking CD8/MHC-I interactions. Some alleles, such as HLA-A*68:01, HLA-B*48:01, and HLA-E bind CD8αα extremely weakly (KD ≥ 1 mM), which is biologically defined as non-binding (40, 42, 43, 103, 106). The weaker affinities of CD8 for the latter group of MHC-I molecules result from either i) polymorphisms of residues within the MHC-I α3 domain directly influences interactions with CD8α and CD8β, or ii) alternations in the conformation of the α3 domain (103). CD8 also plays a crucial role in modulating CD8+ T cell activation through non-classical MHC-I molecules, such as MHC-Ib molecules (TL, H2-Q10, H2-T22, Qa-1b), small molecule metabolite antigen presenting MR1, and lipid antigen presenting CD1 (42, 43, 108–110). CD8 seems to also function as a co-stimulatory molecule for conventional CD8+ T cells, γδ T cells, natural killer cells, and MAIT cells via interactions with MR1 (43). However, as shown for classical MHC-I/TCR interactions, high affinity small molecule antigen/MR1/TCR interactions do not strictly require CD8 engagement, while immune cell responses to low affinity MR1/TCR interactions are reduced or abrogated in the absence of CD8 (43). CD8αα homodimers and CD8αβ heterodimers bind MR1 in a manner similar to classical MHC-I (43) (Figure 4). Unlike with most MHC-I molecules, TL shows a stronger affinity to CD8αα compared to CD8αβ (KD = 12 μM for CD8αα versus > 90 μM for CD8αβ) (111). This disparity is not a result of a different binding mode relative to classical MHC-I; instead, the enhanced CD8αα affinity is attributed to the formation of additional hydrogen bonds (111). Overall, these structural and biophysical data shed light on the specific molecular interactions governing the binding of CD8αα or CD8αβ to classical and non-classical MHC-I molecules, elucidating unique features of their complex formation, stability, and cellular function.
Dynamics and conformational heterogeneity is also an intrinsic feature of the MHC-I that regulates its function (112, 113). All-atom molecular dynamics simulations have hinted at the MHC-I α3 domain’s ability to sample a wide range of conformations, especially in the absence of bound peptide (114–118). A global analysis of B-factors, an implicit metric to identify the flexibility of atoms, for X-ray crystal structures of human MHC complexes reveals the MHC-I α3 domain as a region with significant dynamic properties (112). The MHC-I α1 and α2 domains (i.e., antigen binding groove) also display conformational plasticity, which may be allosterically coupled to the α3 domain (112). Gao et al. have suggested that differences in affinities of classical and non-classical class I MHC molecules for CD8 can be attributed to conformational changes in the α3 domain (residues 223-229) (103). Solution NMR (119–121) and hydrogen/deuteration exchange mass spectrometry (122, 123) measurements of MHC-I molecules have also experimentally confirmed the α1, α2, and α3 domains are conformationally labile in both empty and peptide-bound states in an allele dependent manner. Since peptides modulate stability and conformational plasticity of the MHC-I (116, 121, 123), MHC-I/CD8 interactions might display a peptide-dependence. Evidence against this comes from biophysical measurements of HLAs with different peptides, which reveals very similar affinities with CD8 (11). However, this hypothesis is supported by findings that empty HLA-B molecules, which are known to be conformationally diverse in structure, show stronger CD8 binding affinity than those loaded with specific peptides, potentially due to an enhanced ability to sample optimal α3 domain conformations for binding (114, 119, 124). Together, these results suggest that MHC-I/CD8 affinities are similar for high affinity peptides that stabilize the MHC-I, while CD8 could have enhanced affinity for empty MHC-I and MHC-I bound to low affinity peptides that do not stabilize the MHC-I. Finally, mutations/polymorphisms in the MHC-I α3 domain likely alter its conformation landscape to influence, either positivity or negatively, interaction with CD8 to influence immunological outcomes (42, 80, 103, 116, 117, 120, 125, 126).
2.4 CD8 structure – the hinge contributes to the co-regulator function and relay of signaling
The CD8 hinge region (amino acids 136-182 for CD8α and 139-170 for CD8β in humans) connects the N-terminal globular domain with the membrane-embedded transmembrane domain. The CD8 hinge (also called the “stalk”) is thought to play key roles in communication between the transmembrane and ectodomains, ultimately influencing CD8/MHC-I binding and relaying of signals to CD3 ITAMS via Lck (53, 54, 127). Both CD8α and CD8β hinge sequences are rich in proline, threonine, and serine amino acids, but differ in primary sequence, physical length, and glycosylation patterns (53, 128) (Figures 2, 3). Several CD8/MHC-I crystal structures contained CD8 hinge regions in the expression construct, but electron density for those atoms are missing, suggesting the hinge is unstructured and/or conformationally dynamic (40). In agreement with this, solution NMR experiments suggest that the CD8α hinge lacks a well-ordered structure, is intrinsically flexible, and can undergo cis-trans proline isomerization to sample different functionally relevant states (127). The CD8β hinge contains fewer prolines residues, which likely restricts its conformational landscape relative to CD8α hinge. The CD8 hinge undergoes O-linked glycosylation with sialic acid, which likely influences its structure and function (128–131). For example, immature CD8+ thymocytes exhibit different levels of CD8 hinge O-linked glycosylation relative to mature CD8+ T cells, altering the affinity of CD8 for MHC-I for different CD8+ immune cell subsets (129, 132). Modulating CD8 hinge glycosylation could influence association or orientation of CD8αα or CD8αβ ectodomains with classical and non-classical MHC-I molecules by controlling the hinge’s contributions to “cis” or “trans” binding modes (133). One possible strategy for immunomodulation here is altering the glycosylation pattern of the CD8 hinge using sialidase enzymes, a strategy that has been shown to modulate immune cell activation (134).
2.5 CD8 structure – the transmembrane domain traffics CD8 to the cell surface and promotes CD8 dimerization
The CD8 transmembrane domain (amino acids 183-203 for CD8α and 171-191 for CD8β in humans) plays key roles in intracellular trafficking to the cell surface and assembly of CD8 homo- and heterodimers (135, 136). The CD8α and CD8β transmembrane domains each contain a membrane-proximal Cys residue that forms an intermolecular disulfide bond to stabilize CD8αα homodimers and CD8αβ heterodimers (135). While no atomic structures are currently available for the CD8α and CD8β transmembrane domains, they are predicted by as type I single-pass integral membrane sequences with α-helical secondary structures (5).
2.6 CD8 structure – the cytoplasmic tail mediates localization into signaling lipid rafts and recruits Lck to promote TCR signaling
The CD8 cytoplasmic tail (amino acids 204-235 for CD8α and 192-210 for CD8β in humans) plays several essential roles in CD8’s function as a co-regulator: i) it helps localize CD8 to lipids rafts containing membrane and ii) it recruits Lck and/or LAT to the immunological synapse to promote TCR signaling. As a result of these important features, the CD8 cytoplasmic tail also contributes to thymic selection and immune cell maturation (16, 137–140). Both CD8α and CD8β are palmitoylated (covalent addition of palmitic acid at Cys residues) in the cytoplasmic tail, which promotes the incorporation of CD8 into lipid rafts which are enriched in the immunological synapse (141–144). CD8β contains more palmitoylation sites than CD8α, potentially contributing to differences in the localization of CD8αα homodimers and CD8αβ heterodimers in lipid rafts to alter signaling efficiency (95, 143) (Figure 5A).
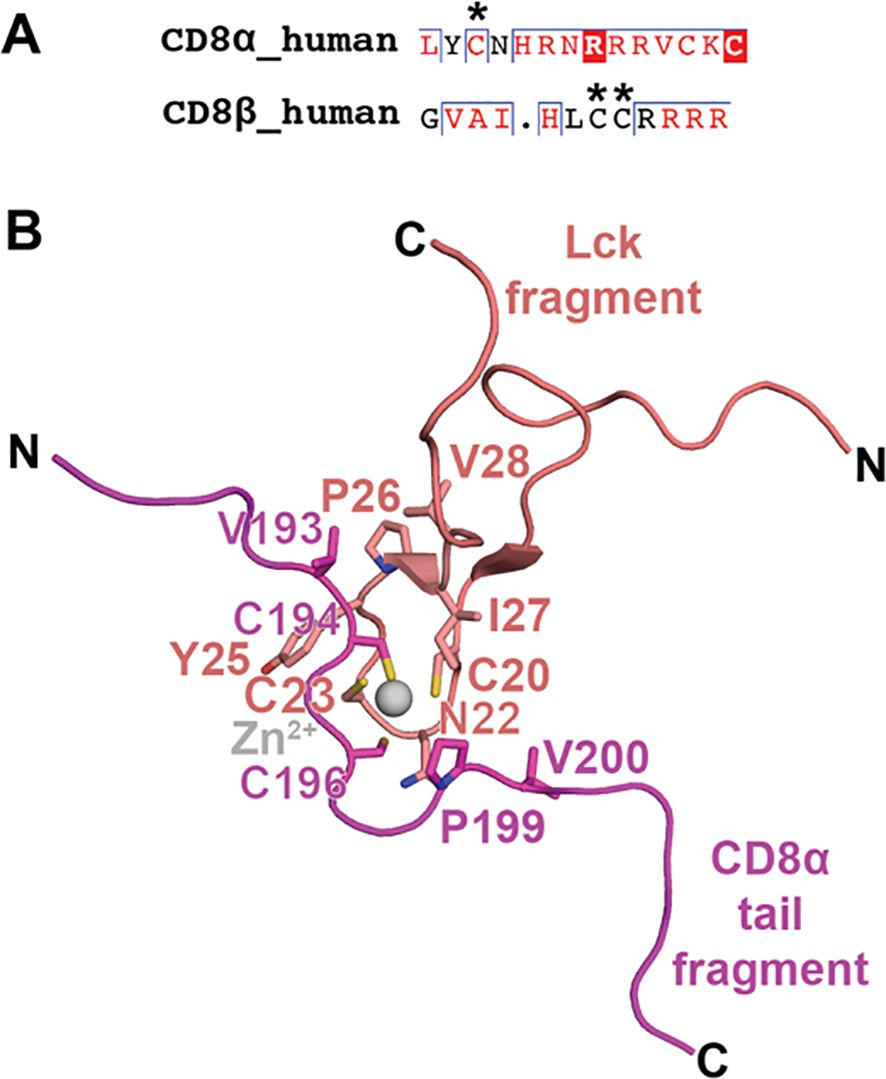
Figure 5. CD8α and CD8β cytoplasmic tails: palmitoylation and binding mode to Lck. (A) A sequence alignment of human CD8α and human CD8β cytoplasmic tails is shown with palmitoylated residues indicated with an asterisk. (B) NMR structure of the interaction between a fragment of the cytoplasmic tail from CD8α with a fragment from Lck (PDB ID 1Q69). The interaction is coordination by a Zn2+ ion and Cys residues on CD8α and Lck. Other residues participating in the interaction are also shown as sticks. CD8α is colored magenta, Lck is colored salmon, and Zn2+ is colored gray.
The cytoplasmic tail of CD8α, but not CD8β, contains a membrane-proximal CxC motif required for Lck binding (24, 145, 146). Thus, in principle, CD8αα and CD8αβ can each associate with two and one Lck molecules, respectively. While CD8β doesn’t contain a Lck binding site, CD8β seems to be required for association and activity of Lck with CD8α, potentially due to the role of its palmitoylation site and recruitment to the cell membrane (147, 148). A partial structure of the CD8α/Lck complex was determined by solution NMR (25) (Figure 5B). The structure suggests that the CD8α/Lck complex is stabilized by two mechanisms. First, a zinc ion is coordinated by the CxC motif of the CD8α cytoplasmic tail and Cys residues on Lck that adopt a zinc-hairpin structure (25, 149). Second, CD8α establishes hydrophobic interactions with Lck, notably through residues V193, P199, and V200, which further stabilize the complex by engaging with the Lck hairpin (25). The dissociation of Lck from the CD8α cytoplasmic tail is a complex regulatory event orchestrated by inhibitory receptors, such as LAG3 (150). Despite the absence of a CxC motif, the CD8β chain seems to be critical for CD8αβ co-receptor function (147, 148, 151, 152).
Whether CD8αα associates in an appreciable way with Lck is a contentious point in the field. It is clear that the co-receptor function of CD8αα and CD8αβ is quite different, which could be related to differences in interactions with MHC, Lck, or LAT (8, 72). However, a question remains on whether these differences are tied to changes in affinities of CD8αα and CD8αβ for Lck, or due to other contributing factors (i.e., ability of CD8β to recruit proteins into kinase-rich lipid rafts for enhanced signaling (95, 143)). Several studies in hybridomas and thymocytes imply that Lck association with CD8α chain is reduced in cell lines expressing CD8αα compared with CD8αβ, resulting in abrogated T cell activation (147, 148, 151, 152). However, bimolecular fluorescence complementation assays suggest Lck has the ability to associate with both CD8αα and CD8αβ, which are each efficiently recruited to the immunological synapse through interactions with the MHC-I (86). The authors suggest CD8αβ’s ability to act as a more robust co-receptor than CD8αα is tied to the CD8β chain’s action in recruiting Lck in the appropriate lift raft environment rather than stark differences in intrinsic Lck affinity, MHC-I binding, or recruitment to the immunological synapse (86). Further study in cell lines associated with CD8αα expression and function could provide additional insights (90). The cytoplasmic tail of CD8α also binds to LAT (153). While structure insights into CD8/LAT interactions are lacking, the complex seems to be mediated by an overlapping epitope with Lck since Lck and LAT binding to CD8 is mutually exclusive (153).
Ultimately, TCR signaling may involve direct competition of Lck and LAT for the cytoplasmic tails of CD8 and CD4 co-receptors, which differ in their affinity for and occupancy of Lck (2, 153). Some reports suggest that only a small percentage of CD4 and CD8 coreceptors engage with Lck (6.8% CD4 vs 0.6% CD8αβ), such that TCRs are required to scan multiple coreceptor molecules to identify a Lck-coupled state for signaling (140). Other studies have measured a much higher Lck occupancy where CD4 is also reported to be higher than CD8αβ (~100% CD4 vs 60% CD8αβ) (154). The stark differences between measurements has been attributed to sample preparation, processing, or experimental conditions (154), although consistency is observed in increase of Lck occupancy by CD4 relative to CD8αβ. CD4/Lck and CD8/Lck occupancy may also vary depending on specific cell type or signaling conditions (154, 155). It has been suggested that the increased occupancy of Lck by CD4 relative to CD8αβ compensates for low affinity of CD4/MHC-II assemblies (154).
2.7 CD8 features used for CAR T cell engineering
Unlike conventional CD8+ T cells, chimeric antigen receptor (CAR) T cells contain CARs engineered to recognize target cell surface antigens, usually independently of MHC-I based antigen presentation (156). However, CARs are still designed with structural components to contain many properties important for conventional T cell signaling: a target recognition domain (typically an antibody, nanobody, or other ligand), a hinge region as a spacer, a transmembrane domain, a co-stimulatory domain (typically derived from CD28), and a cytoplasmic tail that serves as a signaling motif (typically derived from CD3) (156). CARs are often engineered to include CD8α’s hinge region, which regulates CAR T cell receptor flexibility, antigen recognition, and signaling (127, 157–159). Further engineering of CAR length and sequence composition can enhance the properties of the hinge even further. For example, removal of glycine residues in the CD8α hinge reduces the flexibility of second generation CARs, preventing overactivation of CAR T cells by altering steric hindrance and spatial accessibility CAR recognition domain for target antigens (158). Flanking the CD8 hinge, the CD8α transmembrane is also often incorporated into engineered CARs where it dictates receptor surface expression level and signaling activity (157, 160). Interestingly, CAR T cells engineered with CD8α hinge and transmembrane domains are less susceptible to activation-induced cell death compared to those derived from other receptors, such as CD28 (161). It would be interesting to evaluate functional properties of CD8 hinge and transmembrane domains (i.e., cis-proline isomerization, palmitoylation, homodimerization) with respect to CAR T cell function.
3 Immunomodulatory CD8 mutations
Mutations in CD8, obtained through genetic mutation, naturally occurring polymorphisms, or experimental engineering, can lead to downregulation or upregulation in T cell signaling (44, 162–164). For example, familial missense mutations in CD8α cause CD8 deficiency due to protein misfolding (135, 138). The lack of CD8α expression in patients results in high percentages of CD8– T cells and dysregulated immune responses (49, 162). In contrast, engineered CD8 mutations have been used to improve antigen sensitivity for low-affinity MHC-I/TCR complexes (163). Examples of engineered enhancing variants include S53N, S53G, and C33A/S53N for CD8α (located on the C strand and CDR2 loop) and S53L and L58R for CD8β (located on the CDR2 loop) (44, 162–164). The X-ray structure of the engineered human CDαα C33A/S53N mutant suggests that the enhanced affinity is due to a new electrostatic interaction between N53 of CD8α1 and D223 on the MHC-I α3 domain (44). Engineered CD8 molecules could be used enhance the sensitivity of antigen recognition for low-affinity TCRs (163).
4 CD8 interactions with monoclonal antibodies
Anti-CD8 mABs have seen widespread use in immunology research, immunotherapy, and modern medicine. They are common reagents used for flow cytometry to evaluate/isolate T cell subsets, in T cell stimulation assays, to elucidate TCR/CAR signaling mechanisms, and to characterize disease-specific antigen responses (50, 57, 165). Beyond their use in basic immunology research, anti-CD8 mABs show great promise as immunomodulatory therapeutics in medicine. For example, in situ and in vivo studies have suggested anti-CD8 mABs have the potential to block detrimental activity of autoreactive CD8+ T cells in autoimmune diseases, such as glomerulonephritis (166), type 1 diabetes (167, 168), and rheumatoid arthritis (169). Anti-CD8 antibody therapy also could play a role in mitigating transplant rejection related to HLA mismatch between patients (31, 170). In a completely separate application, treatment of CD8+ T cells with agonistic anti-CD8 mABs has been shown to enhance tumor targeting and killing by cytotoxic T cells (171). However, in some cases, mAB binding to CD8 is “neutral” and does not influence T cell activity (50). While these findings are very promising, there are caveats associated with the use of anti-CD8 mABS since it has been suggested that they alters the phenotype and behavior of CD8+ T cells, which have positive or negative effects to therapy outcome (172). The full utility of anti-CD8 mABs in therapy remains to be characterized, and requires further studies evaluating a panel of different mABs with different disease types.
Ultimately, the CD8/mAB binding sites and modes relative to the cell membranes dictate the mechanisms governing their immunomodulatory properties (Figure 1D). Given their wide range of binding modes and functional outcomes, it is essential to characterize how anti-CD8 mABs structurally engage with CD8αα and/or CD8αβ isoforms. Characterizing atomic structures of CD8/mAB complexes not only uncovers mechanistic insights into mAB function, but also provides a molecular blueprint to improve affinity, activity, and specificity. Several structures of CD8/mAB complexes have been reported in the literature (Table 1), however structural insights into many important CD8/mAB interactions remain lacking (Table 2). Antibodies targeted to the CD8α chain have the potential to interact with both CD8αα and CD8αβ isoforms, depending on the recognized epitope, while antibodies against the CD8β chain are specific for CD8αβ or CD8ββ (if present). Thus, defining binding epitopes and structures of CD8/mAB complexes and quantifying CD8/mAB binding affinities reveals important information on the CD8+ T cell subtypes with potential for immunomodulation. Finally, structural elucidation informs whether anti-CD8 mABs are expected to be restricted to certain species (i.e., the mAB recognizes a non-conserved CD8 epitope) or exhibit cross-reactivity against many species (i.e., the mAB recognizes a conserved CD8 epitope). The information known about how mABs bind to CD8 to carry out their function is discussed below.
4.1 YTS 105.18 antibody blocks CD8+ T cell signaling by two distinct mechanisms
YTS 105.18, a rat IgG2a anti-CD8α monoclonal antibody, like most anti-CD8 mABs identified to date is widely used for the blocking of CD8+ T cell activation (173). YTS 105.18 can induce tolerance to xenograft transplantation and reduce insulin-dependent diabetes mellitus by downregulating CD8+ T cell activity (174, 175). YTS 105.18 has also been used to show that CD8+ T cell blockade plays a role in promoting the development of CD4+ regulatory T cells (176). Insights into the blocking mechanism of YTS 105.18 came from an X-ray structure of the mouse CD8αα/YTS 105.18 complex (45, 46) (Figures 6, 7A, B). YTS 105.18 recognizes an epitope spanning the A, A′ and B strands of CD8α, suggesting it can recognize both CD8αα homodimers and CD8αβ heterodimers with distinct mechanisms. In a model where YTS 105.18 binds to CD8αβ, the blocking activity of YTS 105.18 is likely not mediated by sterically hindering CD8αβ/MHC interactions (Figure 7A), and could be due to disruptions of the topology of the interfacial membranes between the T cell and the MHC-expressing cell (e.g., immunological synapse) (45, 46) (Figure 1D). In contrast, for T cells expressing CD8αα on the surface, YTS 105.18 would have the potential to sterically hinder CD8αα/MHC-I interactions (Figure 7B).
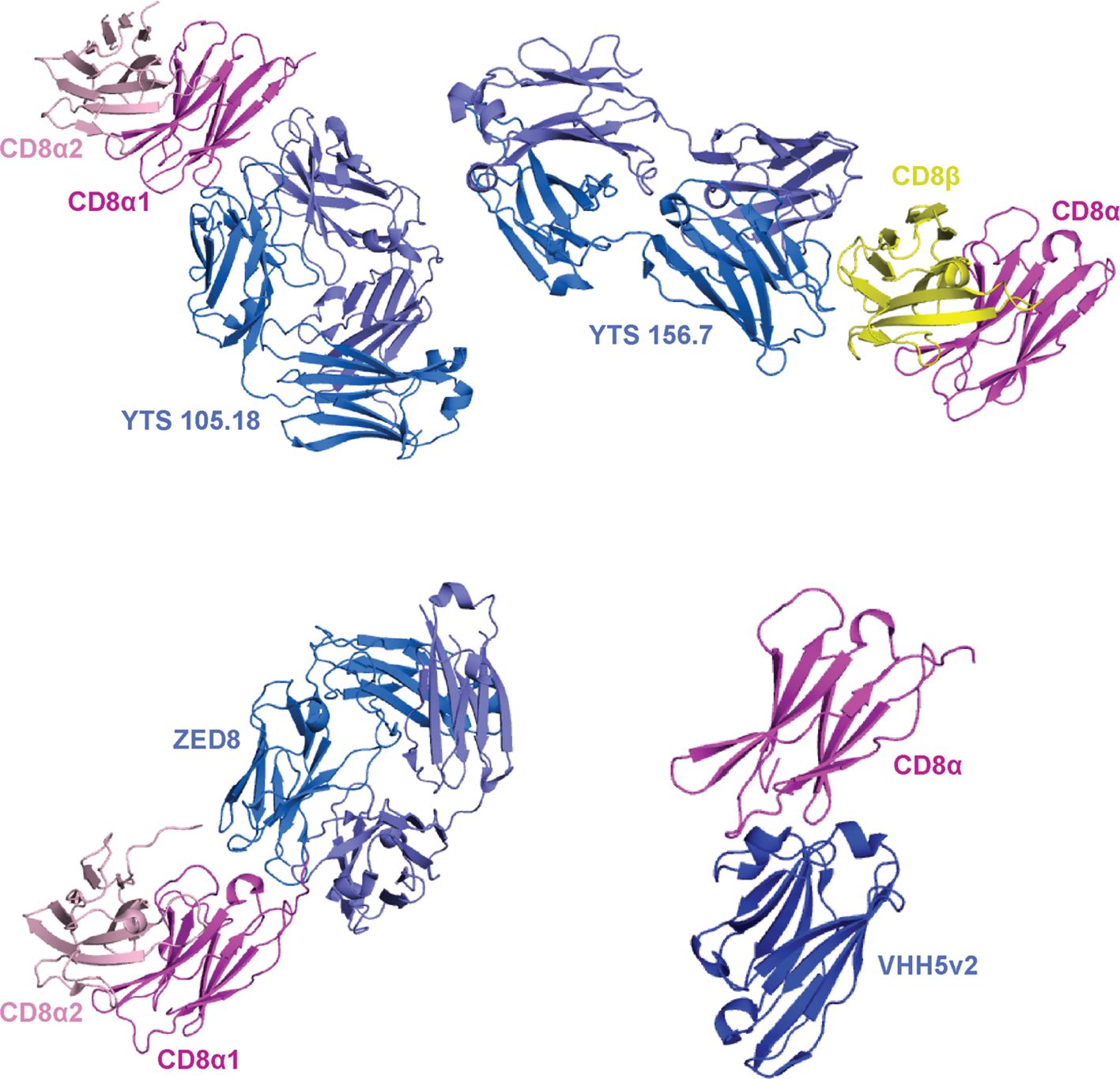
Figure 6. Binding modes of mABs to CD8αα and CD8αβ. A summary of crystal structures of CD8 ectodomain/mAB complexes. From top left to bottom right: mouse CDαα/YTS 105.18 (PDB ID 2ARJ), mouse CD8αβ/YTS 156.7 (PDB ID 3B9K), human CD8αα/ZED8 (PDB ID 7UVF), and human CD8α/VHH5v2 (PDB ID 8EW6). mABs are colored blue/light blue, CD8α chains are colored magenta/light pink, and CD8β chains are colored yellow. The orientation of the magenta CD8α is maintained throughout for comparison.
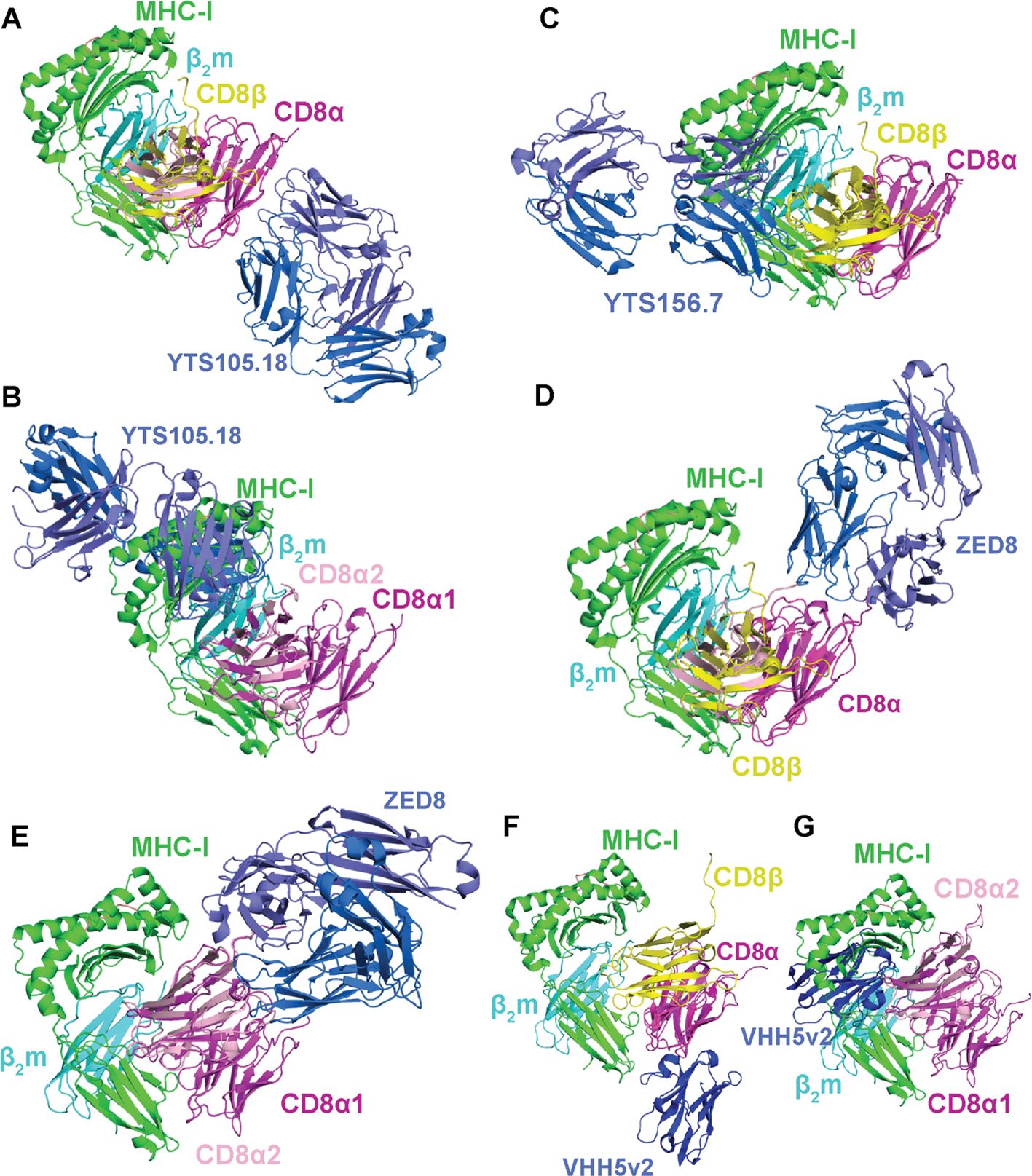
Figure 7. Potential for different mABs to block or enhance CD8αα and CD8αβ interactions with classical and non-classical MHC-I molecules. (A) Overlay of mouse CDαα/YTS 105.18 (PDB ID 2ARJ) with mouse CD8αβ/H-2Dd (PDB ID 3DMM). In this binding mode, no steric hindrance of CD8/MHC is seen. (B) Overlay of mouse CDαα/YTS 105.18 (PDB ID 2ARJ) with mouse CD8αα/H-2Kb (PDB ID 1BQH). In this binding mode, steric hindrance of CD8/MHC is seen. (C) Overlay of mouse CD8αβ/YTS 156.7 (PDB ID 3B9K) with mouse CD8αβ/H-2Dd (PDB ID 3DMM). In this binding mode, steric hindrance of CD8/MHC is seen. (D) Overlay of human CD8αα/ZED8 (PDB ID 7UVF) with mouse CD8αβ/H-2Dd (PDB ID 3DMM). In this binding mode, no steric hindrance of CD8/MHC is seen. (E) Overlay of human CD8αα/ZED8 (PDB ID 7UVF) with mouse CD8αα/H-2Kb (PDB ID 1BQH). In this binding mode, no steric hindrance of CD8/MHC is seen. (F) Overlay of human CD8α/VHH5v2 (PDB ID 8EW6) with mouse CD8αβ/H-2Dd (PDB ID 3DMM). In this binding mode, no steric hindrance of CD8/MHC is seen. (G) Overlay of human CD8α/VHH5v2 (PDB ID 8EW6) with mouse CD8αα/H-2Kb (PDB ID 1BQH). In this binding mode, steric hindrance of CD8/MHC is seen. In all panels, the orientation of the magenta CD8α1 is maintained throughout for comparison. MHC-I heavy chains are colored green, β2m light chains colored cyan, antigens colored salmon, CD8α chains colored magenta/light pink, CD8β chains colored yellow, and mABs colored blue/light blue.
4.2 YTS 156.7 antibody blocks CD8+ T cell signaling by inhibiting CD8/MHC-I binding
YTS 156.7 is a rat IgG2b anti-CD8β monoclonal antibody which, like YTS 105.18, blocks CD8+ T cell activation. YTS 156.7 has been used to deplete CD8+ T cells in vivo to enable immunomodulatory applications similar to YTS 105.18 . Insights into the mechanism of YTS 156.7 were revealed by an X-ray structure of the mouse CD8αβ/YTS 156.7 complex (46) (Figures 6, 7C). YTS 156.7 recognizes an epitope spanning the CDR1, C-C′ and D–E loops of CD8β (46), which is distinct from the CD8α epitope recognized by YTS 105.18. The blocking activity of YTS 156.7 for T cells expressing CD8αβ is most likely mediated by steric hinderance of CD8αβ/MHC-I interactions (46) (Figure 7C). Since YTS 156.7 is specific for the CD8β chain, it would likely not influence CD8+ T cells expressing CD8αα.
4.3 ZED8 and VHH5v2 antibodies used in immunoPET imaging of CD8
Immuno-positron emission tomography (immunoPET) is a technique used for molecular imaging of proteins by combining PET imaging with radioisotope-labeled mABs targeted to a protein of interest (177). ImmunoPET protocols utilizing anti-CD8α mABs, such as 89Zr-labeled ZED8 and 18F-labeled VHH5v2, have enabled imaging of CD8+ T cells in solid tumors and xenografts over time (47, 48). ImmunoPET anti-CD8 mABs may also have immunomodulating properties depending on their binding mechanisms. ZED8 antibody recognizes an epitope on CD8α spanning the A’B loop, the α-helix flanking the F strand, and the ectodomain/hinge transition, and does not appear to alter CD8+ T cell activation (47, 178). In line with this, the X-ray structure of human CD8αα with ZED8 antibody suggests it does not sterically hinder either CD8αβ/MHC-I or CD8αα/MHC-I interactions (47) (Figures 7D, E). The VHH5v2 antibody recognizes an epitope on CD8α spanning the CDR1 and DE loops with affinity of ~500 pM. The immunomodulatory properties of VHH5v2 have not been examined. However, the X-ray structure of human CD8α with VHH5v2 suggests it likely does not sterically hinder CD8αβ/MHC-I interactions but could disrupt CD8αα/MHC-I complex formation (48) (Figures 7F, G).
4.4 Immunomodulation of CD8 by other monoclonal antibodies
Several anti-CD8 mABs block CD8+ T cell activation through epitopes targeted on CD8α (i.e., SK1, DK25, YTS 105.18, H59.101, OX-8, CT-CD8a, KT15, YTS 169.4) or CD8β (i.e., YTS 156.7, 53.5.8, 2ST8.5H7) (10, 51, 173, 179) (Table 2). Some mABs block CD8+ T cell activation and maturation by mechanisms independent of the MHC-I/CD8 interaction. Interestingly, mAB binding with CD8 does not always abrogate CD8+ T cell signaling. For example, anti-CD8α mABs (53.6.7, OKT8) and anti-CD8β mAb (KT112, CT-CD8b) enhance CD8+ T cell signaling (10, 50, 52, 180) (Table 2). As for YTS 105.18 and YTS 156.7, most blocking anti-CD8 mABs appear to work by one of two mechanisms: i) sterically hindering CD8/MHC-I interactions, or ii) altering the topology of the immunological synapse relative to the T cell or antigen presenting cell membranes (10, 51, 173, 179). While structures of many of these CD8/mAB complexes are lacking, the general location of the epitopes recognized by some of these mABs has been mapped by site-directed mutagenesis to the CD8 ectodomain (52). Other mABs, such as the anti-CD8α MRC OX-8 antibody, have shown an ability to recognize the CD8 hinge region to carry out their function (54). Binding of blocking mABs KT15 and CT-CD8a was decreased by mutating CD8α residue K52 located on the CDR2 loop at the interface with the MHC-I, suggesting they sterically hinder CD8αβ/MHC-I interactions (Figure 8). Binding of blocking mAB H59.101 to CD8α was decreased by mutating CD8α residues R8, K12, and K13 located on the A strand, suggesting it does not sterically hinder CD8αβ/MHC-I interactions (Figure 8).
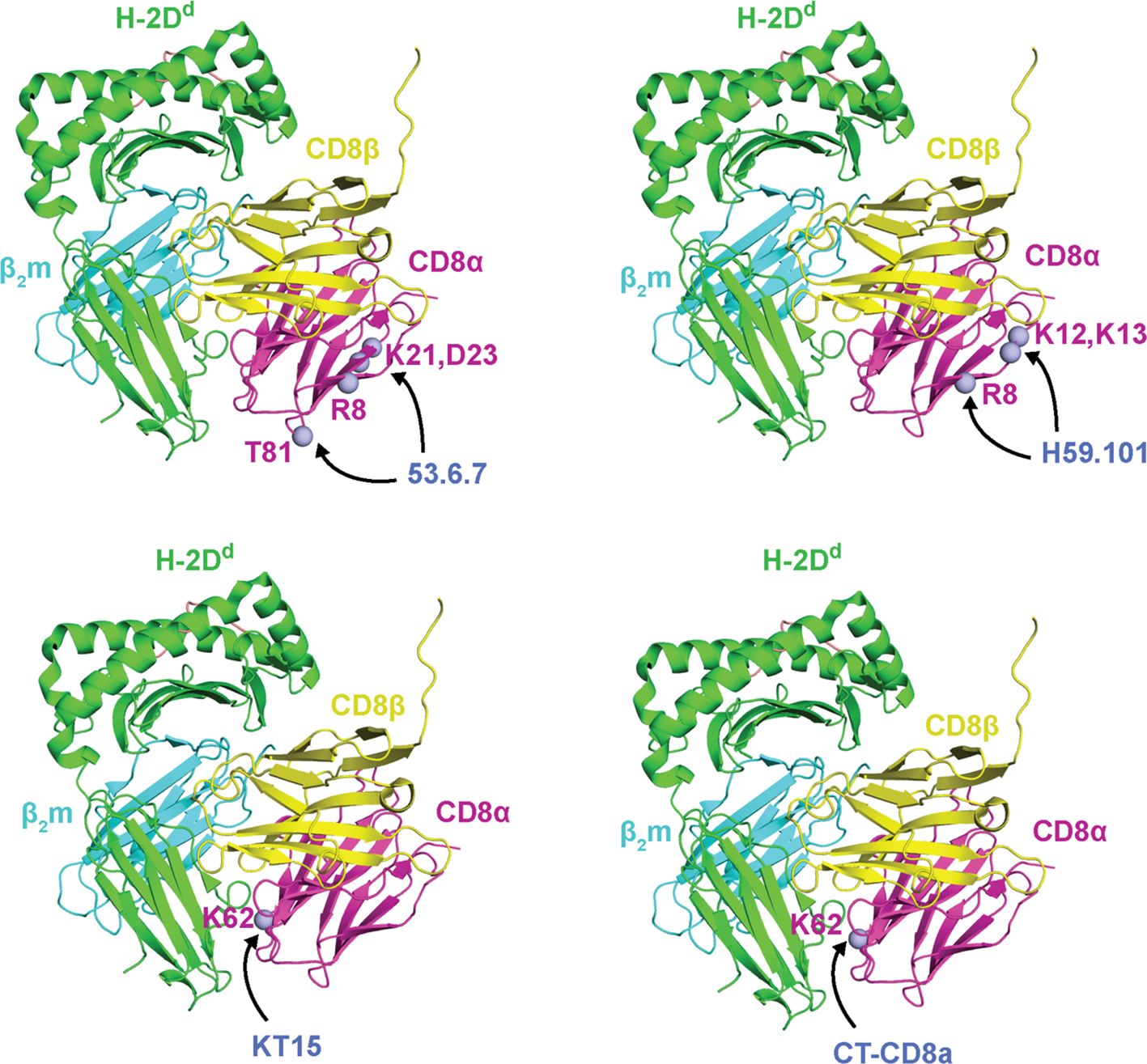
Figure 8. Site-directed mutagenesis identified CD8α epitopes recognized by anti-CD8 mAbs. CDα mutations that influence mAB binding are shown as light blue spheres. From top left to bottom right: mouse CDα mutations at residues R8, K21, D23, and T81 (ectodomain numbering) reduce binding to 53.6.7 antibody, mouse CDα mutations at residues R8, K12, and K13 (ectodomain numbering) reduce binding to H59.101 antibody, and mouse CDα mutations at residues K62 (ectodomain numbering) reduce binding to KT15 and CT-CD8a antibodies. As a reference, mutations are plotted onto CDα from mouse CD8αβ/H-2Dd (PDB ID 3DMM). MHC-I heavy chains are colored green, β2m light chains are colored cyan, peptide antigens are colored salmon, CD8α chains are colored magenta, CD8β chains are colored yellow, and the mABs names are colored blue. Data were derived from Devine et al. (52).
Less is known about the mechanisms driving anti-CD8 mAB enhancement of CD8+ T cell responses. However, they seem to enhance CD8αβ/MHC-I or CD8αα/MHC-I binding, through either stabilizing CD8/MHC-I complexes or influence signal transduction from the ectodomain to the cytoplasmic tail. For example, the inhibition of Lck reduces the enhancing effect of 53.6.7 antibody, suggesting that it functions by modulating signal transduction of CD8αβ ectodomain to the cytoplasmic tail (13, 52). The binding of enhancing mAB 53.6.7 to CD8α was decreased by mutating CD8α residues R8, K21, D23, and T81 located on the A, B, and D strands (52) (Figure 8). Another CD8+ T cell enhancing antibody, the anti-CD8α mAB OKT8, can induce cytokine release from several CD8+ T cells in the absence of specific MHC-I/TCR engagement, possibly through rearrangements in the immunological synapse topology (50). No structural information is available for the CD8αβ/OKT8 complex. Future studies should elucidate the atomic structure of CD8αβ and CD8αα with the monoclonal antibodies to provide mechanistic insights towards their application in immunomodulatory therapeutics.
5 Discussion
Several future directions are needed to move the utility of CD8 for immunomodulation into new horizons. First, relative to CD8αβ, the exact biological functions of CD8αα, and potentially CD8ββ, remain to be fully understood, especially in the context of non-conventional immune cell subsets. For example, in what cases does CD8 function as a co-stimulatory receptor versus a co-repressor? How do the differences in the structure of CD8α and CD8β regulate their function? Second, structural insights into important features of CD8 remain to be determined (i.e, hinge domain, transmembrane domain, cytoplasmic tails). Third, the binding affinities and structures of CD8αα and CD8αβ with a wider range of classical MHC-I alleles across different species, and non-classical MHC-I alleles (i.e., CD1, T22, M10.5, MILL, HFE, FcRn), is required (181). This new structural and biophysical information will inform the potential for immunomodulation of for the broader class of MHC-like molecules by anti-CD8 mABs. Fourth, further details concerning the features of CD8 hinge and transmembrane domains that contribute to efficacy of CAR T cell signaling should be examined. Fifth, many anti-CD8 antibodies still require biophysical and structural characterization with CD8αα or CD8αβ to determine their binding epitopes and mechanisms of action (Tables 1, 2). Finally, it is also likely that computational antibody design can be applied for the rational design of stable, tunable anti-CD8 mABs that target desired functional sites of CD8α or CD8β (182, 183) (Figure 1D). In all of the above cases, care must be taken since mABs can exhibit off-target effects or immunotoxicity, resulting in mAbs-induced adverse effects related to immunosuppression and hypersensitivity (184). Ultimately, we expect that in the coming years the CD8 co-receptor will emerge as one of the prime targets for immunomodulation.
Author contributions
SS: Conceptualization, Visualization, Writing – original draft, Writing – review & editing. CZ: Writing – original draft, Writing – review & editing. AM: Conceptualization, Formal analysis, Project administration, Visualization, Writing – original draft, Writing – review & editing.
Funding
The author(s) declare financial support was received for the research, authorship, and/or publication of this article. AM acknowledges start-up funding from the Georgia Institute of Technology. This work was also supported by NIH grants U01CA250040, R01CA243486, and R01CA284604 (to CZ).
Conflict of interest
The authors declare that the research was conducted in the absence of any commercial or financial relationships that could be construed as a potential conflict of interest.
The author(s) declared that they were an editorial board member of Frontiers, at the time of submission. This had no impact on the peer review process and the final decision.
Publisher’s note
All claims expressed in this article are solely those of the authors and do not necessarily represent those of their affiliated organizations, or those of the publisher, the editors and the reviewers. Any product that may be evaluated in this article, or claim that may be made by its manufacturer, is not guaranteed or endorsed by the publisher.
References
1. Gascoigne NRJ, Zal T, Yachi PP, Hoerter JAH. Co-receptors and recognition of self at the immunological synapse. Curr Top Microbiol Immunol. (2010) 340:171–89. doi: 10.1007/978-3-642-03858-7_9
2. Mørch AM, Bálint Š, Santos AM, Davis SJ, Dustin ML. Coreceptors and TCR signaling - the strong and the weak of it. Front Cell Dev Biol. (2020) 8:597627. doi: 10.3389/fcell.2020.597627
3. Vukmanovic-Stejic M, Thomas MJ, Noble A, Kemeny DM. Specificity, restriction and effector mechanisms of immunoregulatory CD8 T cells. Immunology. (2001) 102:115–22. doi: 10.1046/j.1365-2567.2001.01193.x
4. Rudolph MG, Stanfield RL, Wilson IA. How TCRs bind MHCs, peptides, and coreceptors. Annu Rev Immunol. (2006) 24:419–66. doi: 10.1146/annurev.immunol.23.021704.115658
5. Sušac L, Vuong MT, Thomas C, von Bülow S, O’Brien-Ball C, Santos AM, et al. Structure of a fully assembled tumor-specific T cell receptor ligated by pMHC. Cell. (2022) 185:3201–3213.e19. doi: 10.1016/j.cell.2022.07.010
6. Yin Y, Wang XX, Mariuzza RA. Crystal structure of a complete ternary complex of T-cell receptor, peptide-MHC, and CD4. Proc Natl Acad Sci U.S.A. (2012) 109:5405–10. doi: 10.1073/pnas.1118801109
7. Cole DK, Laugel B, Clement M, Price DA, Wooldridge L, Sewell AK. The molecular determinants of CD8 co-receptor function. Immunology. (2012) 137:139–48. doi: 10.1111/j.1365-2567.2012.03625.x
8. Cheroutre H, Lambolez F. Doubting the TCR coreceptor function of CD8alphaalpha. Immunity. (2008) 28:149–59. doi: 10.1016/j.immuni.2008.01.005
9. König R. Interactions between MHC molecules and co-receptors of the TCR. Curr Opin Immunol. (2002) 14:75–83. doi: 10.1016/s0952-7915(01)00300-4
10. Daniels MA, Jameson SC. Critical role for cd8 in T cell receptor binding and activation by peptide/major histocompatibility complex multimers. J Exp Med. (2000) 191:335–46. doi: 10.1084/jem.191.2.335
11. Wooldridge L, van den Berg HA, Glick M, Gostick E, Laugel B, Hutchinson SL, et al. Interaction between the CD8 coreceptor and major histocompatibility complex class I stabilizes T cell receptor-antigen complexes at the cell surface. J Biol Chem. (2005) 280:27491–501. doi: 10.1074/jbc.M500555200
12. Liu B, Zhong S, Malecek K, Johnson LA, Rosenberg SA, Zhu C, et al. 2D TCR-pMHC-CD8 kinetics determines T-cell responses in a self-antigen-specific TCR system. Eur J Immunol. (2014) 44:239–50. doi: 10.1002/eji.201343774
13. Jiang N, Huang J, Edwards LJ, Liu B, Zhang Y, Beal CD, et al. Two-stage cooperative T cell receptor-peptide major histocompatibility complex-CD8 trimolecular interactions amplify antigen discrimination. Immunity. (2011) 34:13–23. doi: 10.1016/j.immuni.2010.12.017
14. Laugel B, van den Berg HA, Gostick E, Cole DK, Wooldridge L, Boulter J, et al. Different T cell receptor affinity thresholds and CD8 coreceptor dependence govern cytotoxic T lymphocyte activation and tetramer binding properties. J Biol Chem. (2007) 282:23799–810. doi: 10.1074/jbc.M700976200
15. Holler PD, Kranz DM. Quantitative analysis of the contribution of TCR/pepMHC affinity and CD8 to T cell activation. Immunity. (2003) 18:255–64. doi: 10.1016/s1074-7613(03)00019-0
16. Hong J, Ge C, Jothikumar P, Yuan Z, Liu B, Bai K, et al. A TCR mechanotransduction signaling loop induces negative selection in the thymus. Nat Immunol. (2018) 19:1379–90. doi: 10.1038/s41590-018-0259-z
17. Rossjohn J, Gras S, Miles JJ, Turner SJ, Godfrey DI, McCluskey J. T cell antigen receptor recognition of antigen-presenting molecules. Annu Rev Immunol. (2015) 33:169–200. doi: 10.1146/annurev-immunol-032414-112334
18. Singh NK, Abualrous ET, Ayres CM, Noé F, Gowthaman R, Pierce BG, et al. Geometrical characterization of T cell receptor binding modes reveals class-specific binding to maximize access to antigen. Proteins. (2020) 88:503–13. doi: 10.1002/prot.25829
19. Szeto C, Lobos CA, Nguyen AT, Gras S. TCR recognition of peptide–MHC-I: rule makers and breakers. Int J Mol Sci. (2020) 22:68. doi: 10.3390/ijms22010068
20. Wegrecki M, Ocampo TA, Gunasinghe SD, von Borstel A, Tin SY, Reijneveld JF, et al. Atypical sideways recognition of CD1a by autoreactive γδ T cell receptors. Nat Commun. (2022) 13:3872. doi: 10.1038/s41467-022-31443-9
21. Zareie P, Szeto C, Farenc C, Gunasinghe SD, Kolawole EM, Nguyen A, et al. Canonical T cell receptor docking on peptide-MHC is essential for T cell signaling. Science. (2021) 372:eabe9124. doi: 10.1126/science.abe9124
22. Buslepp J, Wang H, Biddison WE, Appella E, Collins EJ. A correlation between TCR Valpha docking on MHC and CD8 dependence: implications for T cell selection. Immunity. (2003) 19:595–606. doi: 10.1016/s1074-7613(03)00269-3
23. Gras S, Chadderton J, Del Campo CM, Farenc C, Wiede F, Josephs TM, et al. Reversed T cell receptor docking on a major histocompatibility class I complex limits involvement in the immune response. Immunity. (2016) 45:749–60. doi: 10.1016/j.immuni.2016.09.007
24. Turner JM, Brodsky MH, Irving BA, Levin SD, Perlmutter RM, Littman DR. Interaction of the unique N-terminal region of tyrosine kinase p56lck with cytoplasmic domains of CD4 and CD8 is mediated by cysteine motifs. Cell. (1990) 60:755–65. doi: 10.1016/0092-8674(90)90090-2
25. Kim PW, Sun Z-YJ, Blacklow SC, Wagner G, Eck MJ. A zinc clasp structure tethers Lck to T cell coreceptors CD4 and CD8. Science. (2003) 301:1725–8. doi: 10.1126/science.1085643
27. Bubeck Wardenburg J, Fu C, Jackman JK, Flotow H, Wilkinson SE, Williams DH, et al. Phosphorylation of SLP-76 by the ZAP-70 protein-tyrosine kinase is required for T-cell receptor function. J Biol Chem. (1996) 271:19641–4. doi: 10.1074/jbc.271.33.19641
28. Wang H, Kadlecek TA, Au-Yeung BB, Goodfellow HES, Hsu L-Y, Freedman TS, et al. ZAP-70: an essential kinase in T-cell signaling. Cold Spring Harb Perspect Biol. (2010) 2:a002279. doi: 10.1101/cshperspect.a002279
29. Melero I, Hervas-Stubbs S, Glennie M, Pardoll DM, Chen L. Immunostimulatory monoclonal antibodies for cancer therapy. Nat Rev Cancer. (2007) 7:95–106. doi: 10.1038/nrc2051
30. Hafeez U, Gan HK, Scott AM. Monoclonal antibodies as immunomodulatory therapy against cancer and autoimmune diseases. Curr Opin Pharmacol. (2018) 41:114–21. doi: 10.1016/j.coph.2018.05.010
31. Mahmud N, Klipa D, Ahsan N. Antibody immunosuppressive therapy in solid-organ transplant. mAbs. (2010) 2:148–56. doi: 10.4161/mabs.2.2.11159
32. Chang H-C, Tan K, Ouyang J, Parisini E, Liu J, Le Y, et al. Structural and mutational analyses of a CD8alphabeta heterodimer and comparison with the CD8alphaalpha homodimer. Immunity. (2005) 23:661–71. doi: 10.1016/j.immuni.2005.11.002
33. Leahy DJ, Axel R, Hendrickson WA. Crystal structure of a soluble form of the human T cell coreceptor CD8 at 2.6 A resolution. Cell. (1992) 68:1145–62. doi: 10.1016/0092-8674(92)90085-q
34. Zong L, Chen Y, Peng H, Gao F, Iwamoto A, Gao GF. Rhesus macaque: a tight homodimeric CD8alphaalpha. Proteins. (2009) 75:241–4. doi: 10.1002/prot.22331
35. Liu Y, Li X, Qi J, Zhang N, Xia C. The structural basis of chicken, swine and bovine CD8αα dimers provides insight into the co-evolution with MHC I in endotherm species. Sci Rep. (2016) 6:24788. doi: 10.1038/srep24788
36. Jia Z, Feng J, Dooley H, Zou J, Wang J. The first crystal structure of CD8αα from a cartilaginous fish. Front Immunol. (2023) 14:1156219. doi: 10.3389/fimmu.2023.1156219
37. Gao GF, Tormo J, Gerth UC, Wyer JR, McMichael AJ, Stuart DI, et al. Crystal structure of the complex between human CD8alpha(alpha) and HLA-A2. Nature. (1997) 387:630–4. doi: 10.1038/42523
38. Shi Y, Qi J, Iwamoto A, Gao GF. Plasticity of human CD8αα binding to peptide-HLA-A*2402. Mol Immunol. (2011) 48:2198–202. doi: 10.1016/j.molimm.2011.05.009
39. Kern PS, Teng MK, Smolyar A, Liu JH, Liu J, Hussey RE, et al. Structural basis of CD8 coreceptor function revealed by crystallographic analysis of a murine CD8alphaalpha ectodomain fragment in complex with H-2Kb. Immunity. (1998) 9:519–30. doi: 10.1016/s1074-7613(00)80635-4
40. Wang R, Natarajan K, Margulies DH. Structural basis of the CD8αβ/MHCI interaction: focused recognition orients CD8β to a T cell proximal position. J Immunol Baltim Md 1950. (2009) 183:2554–64. doi: 10.4049/jimmunol.0901276
41. Liu Y, Chen R, Liang R, Sun B, Wu Y, Zhang L, et al. The combination of CD8αα and peptide-MHC-I in a face-to-face mode promotes chicken γδT cells response. Front Immunol. (2020) 11:605085. doi: 10.3389/fimmu.2020.605085
42. Liu Y, Xiong Y, Naidenko OV, Liu J, Zhang R, Joachimiak A, et al. The crystal structure of a TL/CD8alphaalpha complex at 2.1 A resolution: implications for modulation of T cell activation and memory. Immunity. (2003) 18:205–15. doi: 10.1016/s1074-7613(03)00027-x
43. Souter MNT, Awad W, Li S, Pediongco TJ, Meehan BS, Meehan LJ, et al. CD8 coreceptor engagement of MR1 enhances antigen responsiveness by human MAIT and other MR1-reactive T cells. J Exp Med. (2022) 219:e20210828. doi: 10.1084/jem.20210828
44. Cole DK, Rizkallah PJ, Boulter JM, Sami M, Vuidepot A, Glick M, et al. Computational design and crystal structure of an enhanced affinity mutant human CD8 alphaalpha coreceptor. Proteins. (2007) 67:65–74. doi: 10.1002/prot.21176
45. Shore DA, Teyton L, Dwek RA, Rudd PM, Wilson IA. Crystal structure of the TCR co-receptor CD8alphaalpha in complex with monoclonal antibody YTS 105.18 Fab fragment at 2.88 A resolution. J Mol Biol. (2006) 358:347–54. doi: 10.1016/j.jmb.2006.02.016
46. Shore DA, Issafras H, Landais E, Teyton L, Wilson IA. The crystal structure of CD8 in complex with YTS156.7.7 Fab and interaction with other CD8 antibodies define the binding mode of CD8 alphabeta to MHC class I. J Mol Biol. (2008) 384:1190–202. doi: 10.1016/j.jmb.2008.09.069
47. Ogasawara A, Kiefer JR, Gill H, Chiang E, Sriraman S, Ferl GZ, et al. Preclinical development of ZED8, an 89Zr immuno-PET reagent for monitoring tumor CD8 status in patients undergoing cancer immunotherapy. Eur J Nucl Med Mol Imaging. (2023) 50:287–301. doi: 10.1007/s00259-022-05968-6
48. Sriraman SK, Davies CW, Gill H, Kiefer JR, Yin J, Ogasawara A, et al. Development of an 18F-labeled anti-human CD8 VHH for same-day immunoPET imaging. Eur J Nucl Med Mol Imaging. (2023) 50:679–91. doi: 10.1007/s00259-022-05998-0
49. Mancebo E, Moreno-Pelayo MA, Mencía A, de la Calle-Martín O, Allende LM, Sivadorai P, et al. Gly111Ser mutation in CD8A gene causing CD8 immunodeficiency is found in Spanish Gypsies. Mol Immunol. (2008) 45:479–84. doi: 10.1016/j.molimm.2007.05.022
50. Clement M, Ladell K, Ekeruche-Makinde J, Miles JJ, Edwards ESJ, Dolton G, et al. Anti-CD8 antibodies can trigger CD8+ T-cell effector function in the absence of TCR engagement and improve pMHCI tetramer staining. J Immunol Baltim Md 1950. (2011) 187:654–63. doi: 10.4049/jimmunol.1003941
51. Wooldridge L, Hutchinson SL, Choi EM, Lissina A, Jones E, Mirza F, et al. Anti-CD8 antibodies can inhibit or enhance peptide-MHC class I (pMHCI) multimer binding: this is paralleled by their effects on CTL activation and occurs in the absence of an interaction between pMHCI and CD8 on the cell surface. J Immunol Baltim Md 1950. (2003) 171:6650–60. doi: 10.4049/jimmunol.171.12.6650
52. Devine L, Hodsdon ME, Daniels MA, Jameson SC, Kavathas PB. Location of the epitope for an anti-CD8alpha antibody 53.6.7 which enhances CD8alpha-MHC class I interaction indicates antibody stabilization of a higher affinity CD8 conformation. Immunol Lett. (2004) 93:123–30. doi: 10.1016/j.imlet.2004.02.002
53. Wong JS, Wang X, Witte T, Nie L, Carvou N, Kern P, et al. Stalk region of beta-chain enhances the coreceptor function of CD8. J Immunol Baltim Md 1950. (2003) 171:867–74. doi: 10.4049/jimmunol.171.2.867
54. Classon BJ, Brown MH, Garnett D, Somoza C, Barclay AN, Willis AC, et al. The hinge region of the CD8 alpha chain: structure, antigenicity, and utility in expression of immunoglobulin superfamily domains. Int Immunol. (1992) 4:215–25. doi: 10.1093/intimm/4.2.215
55. Tomonari K, Spencer S. Epitope-specific binding of CD8 regulates activation of T cells and induction of cytotoxicity. Int Immunol. (1990) 2:1189–94. doi: 10.1093/intimm/2.12.1189
56. Pandey PR, Różycki B, Lipowsky R, Weikl TR. Structural variability and concerted motions of the T cell receptor - CD3 complex. eLife. (2021) 10:e67195. doi: 10.7554/eLife.67195
57. Koh C-H, Lee S, Kwak M, Kim B-S, Chung Y. CD8 T-cell subsets: heterogeneity, functions, and therapeutic potential. Exp Mol Med. (2023) 55:2287–99. doi: 10.1038/s12276-023-01105-x
58. Berg RE, Forman J. The role of CD8 T cells in innate immunity and in antigen non-specific protection. Curr Opin Immunol. (2006) 18:338–43. doi: 10.1016/j.coi.2006.03.010
59. Valentine KM, Davini D, Lawrence TJ, Mullins GN, Manansala M, Al-Kuhlani M, et al. CD8 follicular T cells promote B cell antibody class-switch in autoimmune disease. J Immunol Baltim Md 1950. (2018) 201:31–40. doi: 10.4049/jimmunol.1701079
60. Cantor H, Boyse EA. Functional subclasses of T-lymphocytes bearing different Ly antigens. I. The generation of functionally distinct T-cell subclasses is a differentiative process independent of antigen. J Exp Med. (1975) 141:1376–89. doi: 10.1084/jem.141.6.1376
61. Shiku H, Kisielow P, Bean MA, Takahashi T, Boyse EA, Oettgen HF, et al. Expression of T-cell differentiation antigens on effector cells in cell-mediated cytotoxicity in vitro. Evidence for functional heterogeneity related to the surface phenotype of T cells. J Exp Med. (1975) 141:227–41. doi: 10.1084/jem.141.1.227
62. Kisielow P, Hirst JA, Shiku H, Beverley PC, Hoffman MK, Boyse EA, et al. Ly antigens as markers for functionally distinct subpopulations of thymus-derived lymphocytes of the mouse. Nature. (1975) 253:219–20. doi: 10.1038/253219a0
63. Terry LA, DiSanto JP, Small TN, Flomenberg N. Differential expression and regulation of the human CD8 alpha and CD8 beta chains. Tissue Antigens. (1990) 35:82–91. doi: 10.1111/j.1399-0039.1990.tb01761.x
64. Moebius U, Kober G, Griscelli AL, Hercend T, Meuer SC. Expression of different CD8 isoforms on distinct human lymphocyte subpopulations. Eur J Immunol. (1991) 21:1793–800. doi: 10.1002/eji.1830210803
65. Devine L, Kieffer LJ, Aitken V, Kavathas PB. Human CD8 beta, but not mouse CD8 beta, can be expressed in the absence of CD8 alpha as a beta beta homodimer. J Immunol Baltim Md 1950. (2000) 164:833–8. doi: 10.4049/jimmunol.164.2.833
66. Crispe IN. CD4/CD8-negative T cells with alpha beta antigen receptors. Curr Opin Immunol. (1994) 6:438–41. doi: 10.1016/0952-7915(94)90124-4
67. Kadivar M, Petersson J, Svensson L, Marsal J. CD8αβ+ γδ T cells: A novel T cell subset with a potential role in inflammatory bowel disease. J Immunol Baltim Md 1950. (2016) 197:4584–92. doi: 10.4049/jimmunol.1601146
68. Gherardin NA, Souter MN, Koay H-F, Mangas KM, Seemann T, Stinear TP, et al. Human blood MAIT cell subsets defined using MR1 tetramers. Immunol Cell Biol. (2018) 96:507–25. doi: 10.1111/imcb.12021
69. Walker LJ, Kang Y-H, Smith MO, Tharmalingham H, Ramamurthy N, Fleming VM, et al. Human MAIT and CD8αα cells develop from a pool of type-17 precommitted CD8+ T cells. Blood. (2012) 119:422–33. doi: 10.1182/blood-2011-05-353789
70. Shortman K, Heath WR. The CD8+ dendritic cell subset. Immunol Rev. (2010) 234:18–31. doi: 10.1111/j.0105-2896.2009.00870.x
71. Addison EG, North J, Bakhsh I, Marden C, Haq S, Al-Sarraj S, et al. Ligation of CD8alpha on human natural killer cells prevents activation-induced apoptosis and enhances cytolytic activity. Immunology. (2005) 116:354–61. doi: 10.1111/j.1365-2567.2005.02235.x
72. Gangadharan D, Cheroutre H. The CD8 isoform CD8alphaalpha is not a functional homologue of the TCR co-receptor CD8alphabeta. Curr Opin Immunol. (2004) 16:264–70. doi: 10.1016/j.coi.2004.03.015
73. Fonseca S, Pereira V, Lau C, Teixeira MDA, Bini-Antunes M, Lima M. Human peripheral blood gamma delta T cells: Report on a series of healthy caucasian portuguese adults and comprehensive review of the literature. Cells. (2020) 9:729. doi: 10.3390/cells9030729
74. O’Rourke AM, Mescher MF. T-cell receptor-activated adhesion systems. Curr Opin Cell Biol. (1990) 2:888–93. doi: 10.1016/0955-0674(90)90088-v
75. Norment AM, Salter RD, Parham P, Engelhard VH, Littman DR. Cell-cell adhesion mediated by CD8 and MHC class I molecules. Nature. (1988) 336:79–81. doi: 10.1038/336079a0
76. Rosenstein Y, Ratnofsky S, Burakoff SJ, Herrmann SH. Direct evidence for binding of CD8 to HLA class I antigens. J Exp Med. (1989) 169:149–60. doi: 10.1084/jem.169.1.149
77. Irwin MJ, Heath WR, Sherman LA. Species-restricted interactions between CD8 and the alpha 3 domain of class I influence the magnitude of the xenogeneic response. J Exp Med. (1989) 170:1091–101. doi: 10.1084/jem.170.4.1091
78. Potter TA, Rajan TV, Dick RF, Bluestone JA. Substitution at residue 227 of H-2 class I molecules abrogates recognition by CD8-dependent, but not CD8-independent, cytotoxic T lymphocytes. Nature. (1989) 337:73–5. doi: 10.1038/337073a0
79. Connolly JM, Potter TA, Wormstall EM, Hansen TH. The Lyt-2 molecule recognizes residues in the class I alpha 3 domain in allogeneic cytotoxic T cell responses. J Exp Med. (1988) 168:325–41. doi: 10.1084/jem.168.1.325
80. Salter RD, Norment AM, Chen BP, Clayberger C, Krensky AM, Littman DR, et al. Polymorphism in the alpha 3 domain of HLA-A molecules affects binding to CD8. Nature. (1989) 338:345–7. doi: 10.1038/338345a0
81. Kane KP, Sherman LA, Mescher MF. Molecular interactions required for triggering alloantigen-specific cytolytic T lymphocytes. J Immunol Baltim Md 1950. (1989) 142:4153–60. doi: 10.4049/jimmunol.142.12.4153
82. Kane KP, Mescher MF. Activation of CD8-dependent cytotoxic T lymphocyte adhesion and degranulation by peptide class I antigen complexes. J Immunol Baltim Md 1950. (1993) 150:4788–97. doi: 10.4049/jimmunol.150.11.4788
83. O’Rourke AM, Rogers J, Mescher MF. Activated CD8 binding to class I protein mediated by the T-cell receptor results in signalling. Nature. (1990) 346:187–9. doi: 10.1038/346187a0
84. Sun J, Kavathas PB. Comparison of the roles of CD8 alpha alpha and CD8 alpha beta in interaction with MHC class I. J Immunol Baltim Md 1950. (1997) 159:6077–82. doi: 10.4049/jimmunol.159.12.6077
85. Geng J, Raghavan M. CD8αα homodimers function as a coreceptor for KIR3DL1. Proc Natl Acad Sci U.S.A. (2019) 116:17951–6. doi: 10.1073/pnas.1905943116
86. Rybakin V, Clamme J-P, Ampudia J, Yachi PP, Gascoigne NRJ. CD8αα and -αβ isotypes are equally recruited to the immunological synapse through their ability to bind to MHC class I. EMBO Rep. (2011) 12:1251–6. doi: 10.1038/embor.2011.209
87. Nakayama K, Nakayama K, Negishi I, Kuida K, Louie MC, Kanagawa O, et al. Requirement for CD8 beta chain in positive selection of CD8-lineage T cells. Science. (1994) 263:1131–3. doi: 10.1126/science.8108731
88. Huang Y, Park Y, Wang-Zhu Y, Larange A, Arens R, Bernardo I, et al. Mucosal CD8 Memory T Cells are selected in the periphery by an MHC Class I Molecule. Nat Immunol. (2011) 12:1086–95. doi: 10.1038/ni.2106
89. LaFace DM, Vestberg M, Yang Y, Srivastava R, DiSanto J, Flomenberg N, et al. Human CD8 transgene regulation of HLA recognition by murine T cells. J Exp Med. (1995) 182:1315–25. doi: 10.1084/jem.182.5.1315
90. Gui Y, Cheng H, Zhou J, Xu H, Han J, Zhang D. Development and function of natural TCR+ CD8αα+ intraepithelial lymphocytes. Front Immunol. (2022) 13:1059042. doi: 10.3389/fimmu.2022.1059042
91. Madakamutil LT, Christen U, Lena CJ, Wang-Zhu Y, Attinger A, Sundarrajan M, et al. CD8alphaalpha-mediated survival and differentiation of CD8 memory T cell precursors. Science. (2004) 304:590–3. doi: 10.1126/science.1092316
92. Zhong W, Reinherz EL. CD8αα homodimer expression and role in CD8 T cell memory generation during influenza virus A infection in mice. Eur J Immunol. (2005) 35:3103–10. doi: 10.1002/eji.200535162
93. Sievers F, Wilm A, Dineen D, Gibson TJ, Karplus K, Li W, et al. Fast, scalable generation of high-quality protein multiple sequence alignments using Clustal Omega. Mol Syst Biol. (2011) 7:539. doi: 10.1038/msb.2011.75
94. Robert X, Gouet P. Deciphering key features in protein structures with the new ENDscript server. Nucleic Acids Res. (2014) 42:W320–324. doi: 10.1093/nar/gku316
95. Pang DJ, Hayday AC, Bijlmakers M-J. CD8 Raft localization is induced by its assembly into CD8alpha beta heterodimers, Not CD8alpha alpha homodimers. J Biol Chem. (2007) 282:13884–94. doi: 10.1074/jbc.M701027200
96. Gonzalez-Galarza FF, Christmas S, Middleton D, Jones AR. Allele frequency net: a database and online repository for immune gene frequencies in worldwide populations. Nucleic Acids Res. (2011) 39:D913–919. doi: 10.1093/nar/gkq1128
97. Luhtala M, Tregaskes CA, Young JR, Vainio O. Polymorphism of chicken CD8-alpha, but not CD8-beta. Immunogenetics. (1997) 46:396–401. doi: 10.1007/s002510050293
98. Rozemuller E, Eckle SBG, McLaughlin I, Penning M, Mulder W, de Bruin H, et al. MR1 encompasses at least six allele groups with coding region alterations. HLA. (2021) 98:509–16. doi: 10.1111/tan.14390
99. Wooldridge L, Lissina A, Vernazza J, Gostick E, Laugel B, Hutchinson SL, et al. Enhanced immunogenicity of CTL antigens through mutation of the CD8 binding MHC class I invariant region. Eur J Immunol. (2007) 37:1323. doi: 10.1002/eji.200636765
100. Connolly JM, Hansen TH, Ingold AL, Potter TA. Recognition by CD8 on cytotoxic T lymphocytes is ablated by several substitutions in the class I alpha 3 domain: CD8 and the T-cell receptor recognize the same class I molecule. Proc Natl Acad Sci U.S.A. (1990) 87:2137–41. doi: 10.1073/pnas.87.6.2137
101. Purbhoo MA, Boulter JM, Price DA, Vuidepot AL, Hourigan CS, Dunbar PR, et al. The human CD8 coreceptor effects cytotoxic T cell activation and antigen sensitivity primarily by mediating complete phosphorylation of the T cell receptor zeta chain. J Biol Chem. (2001) 276:32786–92. doi: 10.1074/jbc.M102498200
102. Hutchinson SL, Wooldridge L, Tafuro S, Laugel B, Glick M, Boulter JM, et al. The CD8 T cell coreceptor exhibits disproportionate biological activity at extremely low binding affinities. J Biol Chem. (2003) 278:24285–93. doi: 10.1074/jbc.M300633200
103. Gao GF, Willcox BE, Wyer JR, Boulter JM, O’Callaghan CA, Maenaka K, et al. Classical and nonclassical class I major histocompatibility complex molecules exhibit subtle conformational differences that affect binding to CD8alphaalpha. J Biol Chem. (2000) 275:15232–8. doi: 10.1074/jbc.275.20.15232
104. Wyer JR, Willcox BE, Gao GF, Gerth UC, Davis SJ, Bell JI, et al. T cell receptor and coreceptor CD8 alphaalpha bind peptide-MHC independently and with distinct kinetics. Immunity. (1999) 10:219–25. doi: 10.1016/s1074-7613(00)80022-9
105. Huang J, Edwards LJ, Evavold BD, Zhu C. Kinetics of MHC-CD8 interaction at the T cell membrane. J Immunol Baltim Md 1950. (2007) 179:7653–62. doi: 10.4049/jimmunol.179.11.7653
106. Cole DK, Dunn SM, Sami M, Boulter JM, Jakobsen BK, Sewell AK. T cell receptor engagement of peptide-major histocompatibility complex class I does not modify CD8 binding. Mol Immunol. (2008) 45:2700–9. doi: 10.1016/j.molimm.2007.12.009
107. Zhang F, Marcus WD, Goyal NH, Selvaraj P, Springer TA, Zhu C. Two-dimensional kinetics regulation of αLβ2-ICAM-1 interaction by conformational changes of the αL-inserted domain. J Biol Chem. (2005) 280:42207. doi: 10.1074/jbc.M510407200
108. James CA, Xu Y, Aguilar MS, Jing L, Layton ED, Gilleron M, et al. CD4 and CD8 co-receptors modulate functional avidity of CD1b-restricted T cells. Nat Commun. (2022) 13:78. doi: 10.1038/s41467-021-27764-w
109. Goodall KJ, Nguyen A, McKenzie C, Eckle SBG, Sullivan LC, Andrews DM. The murine CD94/NKG2 ligand, Qa-1b, is a high-affinity, functional ligand for the CD8αα homodimer. J Biol Chem. (2020) 295:3239–46. doi: 10.1074/jbc.RA119.010509
110. Teitell M, Mescher MF, Olson CA, Littman DR, Kronenberg M. The thymus leukemia antigen binds human and mouse CD8. J Exp Med. (1991) 174:1131–8. doi: 10.1084/jem.174.5.1131
111. Leishman AJ, Naidenko OV, Attinger A, Koning F, Lena CJ, Xiong Y, et al. T cell responses modulated through interaction between CD8alphaalpha and the nonclassical MHC class I molecule, TL. Science. (2001) 294:1936–9. doi: 10.1126/science.1063564
112. Wieczorek M, Abualrous ET, Sticht J, Álvaro-Benito M, Stolzenberg S, Noé F, et al. Major histocompatibility complex (MHC) class I and MHC class II proteins: conformational plasticity in antigen presentation. Front Immunol. (2017) 8:292. doi: 10.3389/fimmu.2017.00292
113. van Hateren A, Bailey A, Elliott T. Recent advances in Major Histocompatibility Complex (MHC) class I antigen presentation: Plastic MHC molecules and TAPBPR-mediated quality control. F1000Research. (2017) 6:158. doi: 10.12688/f1000research.10474.1
114. Zacharias M, Springer S. Conformational flexibility of the MHC class I α1-α2 domain in peptide bound and free states: A molecular dynamics simulation study. Biophys J. (2004) 87:2203–14. doi: 10.1529/biophysj.104.044743
115. Abualrous ET, Saini SK, Ramnarayan VR, Ilca FT, Zacharias M, Springer S. The carboxy terminus of the ligand peptide determines the stability of the MHC class I molecule H-2Kb: A combined molecular dynamics and experimental study. PLoS One. (2015) 10(8):e0135421. doi: 10.1371/journal.pone.0135421
116. Ayres CM, Abualrous ET, Bailey A, Abraham C, Hellman LM, Corcelli SA, et al. Dynamically driven allostery in MHC proteins: peptide-dependent tuning of class I MHC global flexibility. Front Immunol. (2019) 10:966. doi: 10.3389/fimmu.2019.00966
117. Ayres CM, Corcelli SA, Baker BM. Peptide and peptide-dependent motions in MHC proteins: immunological implications and biophysical underpinnings. Front Immunol. (2017) 8:935. doi: 10.3389/fimmu.2017.00935
118. Sieker F, Springer S, Zacharias M. Comparative molecular dynamics analysis of tapasin-dependent and -independent MHC class I alleles. Protein Sci Publ Protein Soc. (2007) 16:299–308. doi: 10.1110/ps.062568407
119. McShan AC, Natarajan K, Kumirov VK, Flores-Solis D, Jiang J, Badstübner M, et al. Peptide exchange on MHC-I by TAPBPR is driven by a negative allostery release cycle. Nat Chem Biol. (2018) 14:811–20. doi: 10.1038/s41589-018-0096-2
120. McShan AC, Devlin CA, Overall SA, Park J, Toor JS, Moschidi D, et al. Molecular determinants of chaperone interactions on MHC-I for folding and antigen repertoire selection. Proc Natl Acad Sci U.S.A. (2019) 116:25602–13. doi: 10.1073/pnas.1915562116
121. Yanaka S, Ueno T, Shi Y, Qi J, Gao GF, Tsumoto K, et al. Peptide-dependent conformational fluctuation determines the stability of the human leukocyte antigen class I complex. J Biol Chem. (2014) 289:24680–90. doi: 10.1074/jbc.M114.566174
122. van Hateren A, Anderson M, Bailey A, Werner JM, Skipp PJ, Elliott T. Direct evidence for conformational dynamics in Major Histocompatibility Complex class I molecules. J Biol Chem. (2017) 292(49):20255–69. doi: 10.1074/jbc.M117.809624
123. McShan AC, Flores-Solis D, Sun Y, Garfinkle SE, Toor JS, Young MC, et al. Conformational plasticity of RAS Q61 family of neoepitopes results in distinct features for targeted recognition. Nat Commun. (2023) 14:8204. doi: 10.1038/s41467-023-43654-9
124. Geng J, Altman JD, Krishnakumar S, Raghavan M. Empty conformers of HLA-B preferentially bind CD8 and regulate CD8+ T cell function. eLife. (2018) 7:e36341. doi: 10.7554/eLife.36341
125. Bailey A, Dalchau N, Carter R, Emmott S, Phillips A, Werner JM, et al. Selector function of MHC I molecules is determined by protein plasticity. Sci Rep. (2015) 5:srep14928. doi: 10.1038/srep14928
126. Schreiner W, Karch R, Ribarics R, Cibena M, Ilieva N. Relative movements of domains in large molecules of the immune system. J Immunol Res. (2015) 2015:210675. doi: 10.1155/2015/210675
127. Chen X, Mirazee JM, Skorupka KA, Matsuo H, Youkharibache P, Taylor N, et al. The CD8α hinge is intrinsically disordered with a dynamic exchange that includes proline cis-trans isomerization. J Magn Reson San Diego Calif 1997. (2022) 340:107234. doi: 10.1016/j.jmr.2022.107234
128. Merry AH, Gilbert RJC, Shore DA, Royle L, Miroshnychenko O, Vuong M, et al. O-glycan sialylation and the structure of the stalk-like region of the T cell co-receptor CD8. J Biol Chem. (2003) 278:27119–28. doi: 10.1074/jbc.M213056200
129. Moody AM, Chui D, Reche PA, Priatel JJ, Marth JD, Reinherz EL. Developmentally regulated glycosylation of the CD8alphabeta coreceptor stalk modulates ligand binding. Cell. (2001) 107:501–12. doi: 10.1016/s0092-8674(01)00577-3
130. Shore DA, Wilson IA, Dwek RA, Rudd PM. Glycosylation and the function of the T cell co-receptor CD8. Adv Exp Med Biol. (2005) 564:71–84. doi: 10.1007/0-387-25515-X_12
131. Pascale MC, Malagolini N, Serafini-Cessi F, Migliaccio G, Leone A, Bonatti S. Biosynthesis and oligosaccharide structure of human CD8 glycoprotein expressed in a rat epithelial cell line. J Biol Chem. (1992) 267:9940–7. doi: 10.1016/S0021-9258(19)50183-1
132. Daniels MA, Devine L, Miller JD, Moser JM, Lukacher AE, Altman JD, et al. CD8 binding to MHC class I molecules is influenced by T cell maturation and glycosylation. Immunity. (2001) 15:1051–61. doi: 10.1016/s1074-7613(01)00252-7
133. Liu Y, Cuendet MA, Goffin L, Šachl R, Cebecauer M, Cariolato L, et al. CD8 Binding of MHC-Peptide Complexes in cis or trans Regulates CD8+ T-cell Responses. J Mol Biol. (2019) 431:4941–58. doi: 10.1016/j.jmb.2019.10.019
134. Sadighi Akha AA, Berger SB, Miller RA. Enhancement of CD8 T-cell function through modifying surface glycoproteins in young and old mice. Immunology. (2006) 119:187–94. doi: 10.1111/j.1365-2567.2006.02420.x
135. Hennecke S, Cosson P. Role of transmembrane domains in assembly and intracellular transport of the CD8 molecule. J Biol Chem. (1993) 268:26607–12. doi: 10.1016/S0021-9258(19)74355-5
136. Briant K, Johnson N, Swanton E. Transmembrane domain quality control systems operate at the endoplasmic reticulum and Golgi apparatus. PLoS One. (2017) 12:e0173924. doi: 10.1371/journal.pone.0173924
137. Itano A, Cado D, Chan FK, Robey E. A role for the cytoplasmic tail of the beta chain of CD8 in thymic selection. Immunity. (1994) 1:287–90. doi: 10.1016/1074-7613(94)90080-9
138. Bosselut R, Feigenbaum L, Sharrow SO, Singer A. Strength of signaling by CD4 and CD8 coreceptor tails determines the number but not the lineage direction of positively selected thymocytes. Immunity. (2001) 14:483–94. doi: 10.1016/s1074-7613(01)00128-5
139. Fung-Leung WP, Louie MC, Limmer A, Ohashi PS, Ngo K, Chen L, et al. The lack of CD8 alpha cytoplasmic domain resulted in a dramatic decrease in efficiency in thymic maturation but only a moderate reduction in cytotoxic function of CD8+ T lymphocytes. Eur J Immunol. (1993) 23:2834–40. doi: 10.1002/eji.1830231117
140. Stepanek O, Prabhakar AS, Osswald C, King CG, Bulek A, Naeher D, et al. Coreceptor scanning by the T cell receptor provides a mechanism for T cell tolerance. Cell. (2014) 159:333–45. doi: 10.1016/j.cell.2014.08.042
141. Hoeveler A, Malissen B. The cysteine residues in the cytoplasmic tail of CD8 alpha are required for its coreceptor function. Mol Immunol. (1993) 30:755–64. doi: 10.1016/0161-5890(93)90147-4
142. Arcaro A, Grégoire C, Boucheron N, Stotz S, Palmer E, Malissen B, et al. Essential role of CD8 palmitoylation in CD8 coreceptor function. J Immunol Baltim Md 1950. (2000) 165:2068–76. doi: 10.4049/jimmunol.165.4.2068
143. Arcaro A, Grégoire C, Bakker TR, Baldi L, Jordan M, Goffin L, et al. CD8beta endows CD8 with efficient coreceptor function by coupling T cell receptor/CD3 to raft-associated CD8/p56(lck) complexes. J Exp Med. (2001) 194:1485–95. doi: 10.1084/jem.194.10.1485
144. Kabouridis PS. Lipid rafts in T cell receptor signalling. Mol Membr Biol. (2006) 23:49–57. doi: 10.1080/09687860500453673
145. Veillette A, Bookman MA, Horak EM, Bolen JB. The CD4 and CD8 T cell surface antigens are associated with the internal membrane tyrosine-protein kinase p56lck. Cell. (1988) 55:301–8. doi: 10.1016/0092-8674(88)90053-0
146. Zamoyska R, Derham P, Gorman SD, von Hoegen P, Bolen JB, Veillette A, et al. Inability of CD8 alpha’ polypeptides to associate with p56lck correlates with impaired function in vitro and lack of expression in vivo. Nature. (1989) 342:278–81. doi: 10.1038/342278a0
147. Bosselut R, Kubo S, Guinter T, Kopacz JL, Altman JD, Feigenbaum L, et al. Role of CD8beta domains in CD8 coreceptor function: importance for MHC I binding, signaling, and positive selection of CD8+ T cells in the thymus. Immunity. (2000) 12:409–18. doi: 10.1016/s1074-7613(00)80193-4
148. Irie HY, Mong MS, Itano A, Crooks ME, Littman DR, Burakoff SJ, et al. The cytoplasmic domain of CD8 beta regulates Lck kinase activation and CD8 T cell development. J Immunol Baltim Md 1950. (1998) 161:183–91. doi: 10.4049/jimmunol.161.1.183
149. Lin RS, Rodriguez C, Veillette A, Lodish HF. Zinc is essential for binding of p56(lck) to CD4 and CD8alpha. J Biol Chem. (1998) 273:32878–82. doi: 10.1074/jbc.273.49.32878
150. Guy C, Mitrea DM, Chou P-C, Temirov J, Vignali KM, Liu X, et al. LAG3 associates with TCR-CD3 complexes and suppresses signaling by driving co-receptor-Lck dissociation. Nat Immunol. (2022) 23:757–67. doi: 10.1038/s41590-022-01176-4
151. Renard V, Romero P, Vivier E, Malissen B, Luescher IF. CD8 beta increases CD8 coreceptor function and participation in TCR-ligand binding. J Exp Med. (1996) 184:2439–44. doi: 10.1084/jem.184.6.2439
152. Karaki S, Tanabe M, Nakauchi H, Takiguchi M. Beta-chain broadens range of CD8 recognition for MHC class I molecule. J Immunol Baltim Md 1950. (1992) 149:1613–8. doi: 10.4049/jimmunol.149.5.1613
153. Bosselut R, Zhang W, Ashe JM, Kopacz JL, Samelson LE, Singer A. Association of the adaptor molecule LAT with CD4 and CD8 coreceptors identifies a new coreceptor function in T cell receptor signal transduction. J Exp Med. (1999) 190:1517–26. doi: 10.1084/jem.190.10.1517
154. Mørch AM, Schneider F, Jenkins E, Santos AM, Fraser SE, Davis SJ, et al. The kinase occupancy of T cell coreceptors reconsidered. Proc Natl Acad Sci U.S.A. (2022) 119:e2213538119. doi: 10.1073/pnas.2213538119
155. Carmo AM, Mason DW, Beyers AD. Physical association of the cytoplasmic domain of CD2 with the tyrosine kinases p56lck and p59fyn. Eur J Immunol. (1993) 23:2196–201. doi: 10.1002/eji.1830230922
156. Rafiq S, Hackett CS, Brentjens RJ. Engineering strategies to overcome the current roadblocks in CAR T cell therapy. Nat Rev Clin Oncol. (2020) 17:147–67. doi: 10.1038/s41571-019-0297-y
157. Fujiwara K, Tsunei A, Kusabuka H, Ogaki E, Tachibana M, Okada N. Hinge and transmembrane domains of chimeric antigen receptor regulate receptor expression and signaling threshold. Cells. (2020) 9:1182. doi: 10.3390/cells9051182
158. Zhang A, Sun Y, Du J, Dong Y, Pang H, Ma L, et al. Reducing hinge flexibility of CAR-T cells prolongs survival in vivo with low cytokines release. Front Immunol. (2021) 12:724211. doi: 10.3389/fimmu.2021.724211
159. Mazinani M, Rahbarizadeh F. CAR-T cell potency: from structural elements to vector backbone components. biomark Res. (2022) 10:70. doi: 10.1186/s40364-022-00417-w
160. Zhang H, Zhao P, Huang H. Engineering better chimeric antigen receptor T cells. Exp Hematol Oncol. (2020) 9:34. doi: 10.1186/s40164-020-00190-2
161. Alabanza L, Pegues M, Geldres C, Shi V, Wiltzius JJW, Sievers SA, et al. Function of novel anti-CD19 chimeric antigen receptors with human variable regions is affected by hinge and transmembrane domains. Mol Ther J Am Soc Gene Ther. (2017) 25:2452–65. doi: 10.1016/j.ymthe.2017.07.013
162. de la Calle-Martin O, Hernandez M, Ordi J, Casamitjana N, Arostegui JI, Caragol I, et al. Familial CD8 deficiency due to a mutation in the CD8α gene. J Clin Invest. (2001) 108:117. doi: 10.1172/JCI10993
163. Knezevic L, Wachsmann TLA, Francis O, Dockree T, Bridgeman JS, Wouters A, et al. High-affinity CD8 variants enhance the sensitivity of pMHCI antigen recognition via low-affinity TCRs. J Biol Chem. (2023) 299:104981. doi: 10.1016/j.jbc.2023.104981
164. Devine L, Thakral D, Nag S, Dobbins J, Hodsdon ME, Kavathas PB. Mapping the binding site on CD8 beta for MHC class I reveals mutants with enhanced binding. J Immunol Baltim Md 1950. (2006) 177:3930–8. doi: 10.4049/jimmunol.177.6.3930
165. Flynn J, Gorry P. Flow cytometry analysis to identify human CD8+ T cells. Methods Mol Biol Clifton NJ. (2019) 2048:1–13. doi: 10.1007/978-1-4939-9728-2_1
166. Reynolds J, Norgan VA, Bhambra U, Smith J, Cook HT, Pusey CD. Anti-CD8 monoclonal antibody therapy is effective in the prevention and treatment of experimental autoimmune glomerulonephritis. J Am Soc Nephrol JASN. (2002) 13:359–69. doi: 10.1681/ASN.V132359
167. Clement M, Pearson JA, Gras S, van den Berg HA, Lissina A, Llewellyn-Lacey S, et al. Targeted suppression of autoreactive CD8+ T-cell activation using blocking anti-CD8 antibodies. Sci Rep. (2016) 6:35332. doi: 10.1038/srep35332
168. Parish N, Cooke A. Characterisation of CD8 monoclonal antibody-induced protection from diabetes in NOD mice. Autoimmunity. (2005) 38:597–604. doi: 10.1080/08916930500438241
169. Raposo BR, Rodrigues-Santos P, Carvalheiro H, Agua-Doce AM, Carvalho L, Pereira da Silva JA, et al. Monoclonal anti-CD8 therapy induces disease amelioration in the K/BxN mouse model of spontaneous chronic polyarthritis. Arthritis Rheum. (2010) 62:2953–62. doi: 10.1002/art.27729
170. Allan JS, Choo JK, Vesga L, Arn JS, Pins MR, Sachs DH, et al. Cardiac allograft vasculopathy is abrogated by anti-CD8 monoclonal antibody therapy. Ann Thorac Surg. (1997) 64:1019–25. doi: 10.1016/s0003-4975(97)00796-0
171. Madi A, Weisshaar N, Buettner M, Poschet G, Ma S, Wu J, et al. CD8 agonism functionally activates memory T cells and enhances antitumor immunity. Int J Cancer. (2022) 151:797–808. doi: 10.1002/ijc.34059
172. Cross EW, Blain TJ, Mathew D, Kedl RM. Anti-CD8 monoclonal antibody-mediated depletion alters the phenotype and behavior of surviving CD8+ T cells. PLoS One. (2019) 14:e0211446. doi: 10.1371/journal.pone.0211446
173. Qin SX, Wise M, Cobbold SP, Leong L, Kong YC, Parnes JR, et al. Induction of tolerance in peripheral T cells with monoclonal antibodies. Eur J Immunol. (1990) 20:2737–45. doi: 10.1002/eji.1830201231
174. Parish NM, Bowie L, Zusman Harach S, Phillips JM, Cooke A. Thymus-dependent monoclonal antibody-induced protection from transferred diabetes. Eur J Immunol. (1998) 28:4362–73. doi: 10.1002/(ISSN)1521-4141
175. Phillips JM, Parish NM, Raine T, Bland C, Sawyer Y, de la Peña H, et al. Type 1 diabetes development requires both CD4+ and CD8+ T cells and can be reversed by non-depleting antibodies targeting both T cell populations. Rev Diabetes Stud RDS. (2009) 6:97–103. doi: 10.1900/RDS.2009.6.97
176. Wang Z, Davies JD. CD8 blockade promotes the expansion of antigen-specific CD4+ FOXP3+ regulatory T cells in vivo. Int Immunopharmacol. (2007) 7:249–65. doi: 10.1016/j.intimp.2006.10.012
177. Manafi-Farid R, Ataeinia B, Ranjbar S, Jamshidi Araghi Z, Moradi MM, Pirich C, et al. ImmunoPET: antibody-based PET imaging in solid tumors. Front Med. (2022) 9:916693. doi: 10.3389/fmed.2022.916693
178. Gill H, Seipert R, Carroll VM, Gouasmat A, Yin J, Ogasawara A, et al. The production, quality control, and characterization of ZED8, a CD8-specific 89Zr-labeled immuno-PET clinical imaging agent. AAPS J. (2020) 22:22. doi: 10.1208/s12248-019-0392-0
179. Chen Z, Cobbold S, Metcalfe S, Waldmann H. Tolerance in the mouse to major histocompatibility complex-mismatched heart allografts, and to rat heart xenografts, using monoclonal antibodies to CD4 and CD8. Eur J Immunol. (1992) 22:805–10. doi: 10.1002/eji.1830220326
180. Luescher IF, Vivier E, Layer A, Mahiou J, Godeau F, Malissen B, et al. CD8 modulation of T-cell antigen receptor-ligand interactions on living cytotoxic T lymphocytes. Nature. (1995) 373:353–6. doi: 10.1038/373353a0
181. Adams EJ, Luoma AM. The adaptable major histocompatibility complex (MHC) fold: structure and function of nonclassical and MHC class I-like molecules. Annu Rev Immunol. (2013) 31:529–61. doi: 10.1146/annurev-immunol-032712-095912
182. Adolf-Bryfogle J, Kalyuzhniy O, Kubitz M, Weitzner BD, Hu X, Adachi Y, et al. RosettaAntibodyDesign (RAbD): A general framework for computational antibody design. PLoS Comput Biol. (2018) 14:e1006112. doi: 10.1371/journal.pcbi.1006112
183. Watson JL, Juergens D, Bennett NR, Trippe BL, Yim J, Eisenach HE, et al. De novo design of protein structure and function with RFdiffusion. Nature. (2023) 620:1089–100. doi: 10.1038/s41586-023-06415-8
Keywords: CD8 co-receptor, immunomodulation, T cell signaling, T cell receptor, major histocompatibility complex, monoclonal antibodies, chimeric antigen receptor
Citation: Srinivasan S, Zhu C and McShan AC (2024) Structure, function, and immunomodulation of the CD8 co-receptor. Front. Immunol. 15:1412513. doi: 10.3389/fimmu.2024.1412513
Received: 05 April 2024; Accepted: 05 August 2024;
Published: 26 August 2024.
Edited by:
Jonathan S. Duke-Cohan, Dana–Farber Cancer Institute, United StatesReviewed by:
Lance Hellman, Nevada State College, United StatesMichael Loran Dustin, University of Oxford, United Kingdom
Copyright © 2024 Srinivasan, Zhu and McShan. This is an open-access article distributed under the terms of the Creative Commons Attribution License (CC BY). The use, distribution or reproduction in other forums is permitted, provided the original author(s) and the copyright owner(s) are credited and that the original publication in this journal is cited, in accordance with accepted academic practice. No use, distribution or reproduction is permitted which does not comply with these terms.
*Correspondence: Andrew C. McShan, YW5kcmV3Lm1jc2hhbkBjaGVtaXN0cnkuZ2F0ZWNoLmVkdQ==