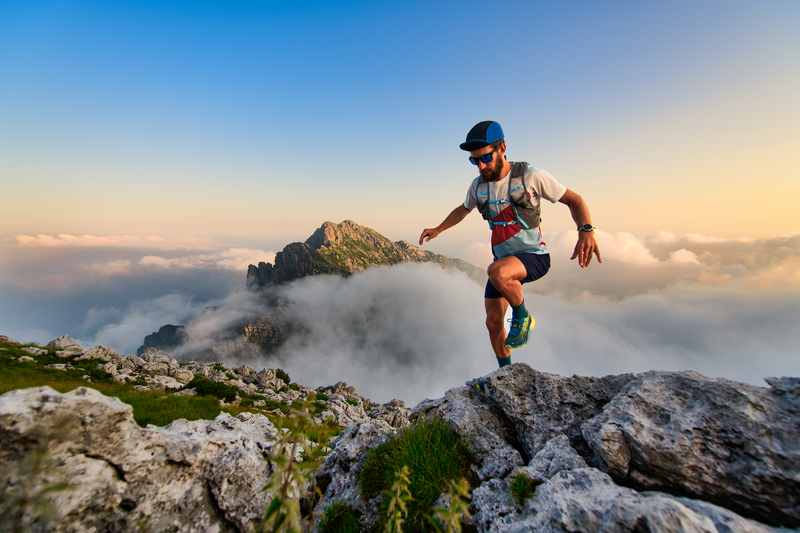
94% of researchers rate our articles as excellent or good
Learn more about the work of our research integrity team to safeguard the quality of each article we publish.
Find out more
REVIEW article
Front. Immunol. , 11 June 2024
Sec. Cancer Immunity and Immunotherapy
Volume 15 - 2024 | https://doi.org/10.3389/fimmu.2024.1404974
This article is part of the Research Topic Metabolism Regulation of T Cell Mediated-Tumor Immunity and Immunotherapy View all 5 articles
Foxp3+ regulatory T cells (Foxp3+ Treg) play a role in regulating various types of tumors, but uncertainty still exists regarding the exact mechanism underlying Foxp3+ Treg activation in gastrointestinal malignancies. As of now, research has shown that Foxp3+ Treg expression, altered glucose metabolism, or a hypoxic tumor microenvironment all affect Foxp3+ Treg function in the bodies of tumor patients. Furthermore, it has been demonstrated that post-translational modifications are essential for mature Foxp3 to function properly. Additionally, a considerable number of non-coding RNAs (ncRNAs) have been implicated in the activation of the Foxp3 signaling pathway. These mechanisms regulating Foxp3 may one day serve as potential therapeutic targets for gastrointestinal malignancies. This review primarily focuses on the properties and capabilities of Foxp3 and Foxp3+Treg. It emphasizes the advancement of research on the regulatory mechanisms of Foxp3 in different malignant tumors of the digestive system, providing new insights for the exploration of anticancer treatments.
Data from the two most populous countries in the world, China and the United States, indicate that in 2020, China accounted for approximately 23.7% of the global cancer incidence and 30.2% of all cancer deaths, with 4,568,754 new cancer cases and 3,002,899 deaths (1); by 2023, the United States anticipates approximately 1958310 new cancer cases and 609820 cancer deaths (2). Thus, Medical professionals have shown that Foxp3+Treg expression is elevated in high-grade digestive malignant tumors, such as liver, pancreatic, and stomach cancers, and is typically linked to a poor prognosis for patients. This discovery has been made when investigating the formation mechanism of diverse malignant tumors (3–6).
This article mainly summarizes the research progress on the expression mechanism and function of Foxp3+Treg and Foxp3 related features and functions in digestive system malignant tumors, which have been publicly published in publications such as Pubmed. We also discuss the application of immunotherapy techniques to inhibit the tumor immunosuppressive effect of Foxp3+Treg based on the regulation of Foxp3+Treg expression in the tumor microenvironment, thereby improving the patient’s own immune and anti-tumor ability, and providing new ideas for personalized treatment of tumor patients.
Foxp3+ Treg cells predominantly secrete Foxp3, a subset of T cells that regulate the body’s immune system by exerting immunosuppressive effects. Foxp3+ Treg can be acquired in two ways: the first is through maturation in the thymus and release into the periphery, at which point Foxp3+ Treg are also known as natural regulatory T cells (nTregs); another method of production is through Transforming Growth Factor-β (TGF-β) Induced regulatory T cells (iTreg). These cells protect their own reactive lymphocytes from immunological reactions, thereby maintaining their immunity and tissue stability (7) (Table 1).
The transcription factor Foxp3 belongs to the multi-domain Fox family of forkhead box proteins. Proline and other amino acids make up the majority of the N-terminal region, which binds to different protein molecules to carry out transcriptional inhibition functions. For example, it can inhibit the production of NF-AT-mediated transcription-activating factor IL-2 (36, 37); the stable C-terminal forked head domain (FKH) can recognize and bind specific DNA sequences. Central zinc finger transcriptional regulation and oligomerization modification with leucine domain (38). Foxp3 is primarily produced by T cells; however, non-Treg cells can also generate some Foxp3. Foxp3, a crucial transcription factor in regulatory T cells (Treg cells), regulates various functions and processes, such as development and maturation. The function of Treg cells may be inhibited by a lack of or low expression. Significantly, human X-linked and autoimmune diseases, including immune system disorders, several endocrine disorders, intestinal diseases, X-linked syndrome (IPEX syndrome), and others, can result from the functional loss or mutation of the Foxp3 gene (39, 40). The primary features include various autoimmune disorders affecting organs, significant allergies, and excessive inflammation. T cell differentiation and function may be influenced by the interactions of Foxp3 with other factors. Through its antagonistic effects on Toll-like receptors (TLRs), Foxp3 contributes to the reprogramming of T cell metabolism, enhancing T cell oxidative phosphorylation (OXPHOS) and fatty acid oxidation capacity. Additionally, Foxp3 collaborates with the original glycolytic pathway to produce energy, ensuring that Treg cells have the essential resources for proliferation and preventing cell apoptosis (41, 42). Conversely, Foxp3 suppresses the glycolysis and Myc signaling pathways when it binds to the oncogene Myc promoter, which impacts T cell metabolism (43).
Additionally, Foxp3 can control the body’s immunity by directly or indirectly influencing downstream components in tumor cells. In their investigation of non-small cell lung cancer cells, Peng et al. (44) discovered that Foxp3 overexpression in the tumor microenvironment can suppress anti-tumor immunity and promote the proliferation of cancer cells. Direct binding of Foxp3 to the LINC00885 promoter can upregulate the production of proteins associated with the epithelial mesenchymal transition (EMT), thereby promoting the growth and invasion of cervical cancer cells (45). Furthermore, numerous clinical investigations have established that overexpression of Foxp3 is associated with a poor prognosis and low survival rate in cancer patients (46, 47). For instance, Foxp3 expression is higher and survival is lower in individuals with oral squamous cell carcinoma (48). On the other hand, patients with specific malignancies have been shown to benefit from elevated Foxp3 expression. According to published research, patients with high levels of VEGF and CD44 expression in breast cancer have a comparatively short survival period. Additionally, there is a negative correlation between Foxp3 expression and VEGF and CD44 expression. On one hand, Foxp3 directly inhibits the activity of the VEGF promoter through a specific forkhead binding motif, which leads to the inhibition of angiogenesis in breast cancer by suppressing the expression of VEGF, consequently downregulating VEGF (49); On the other hand, Foxp3 binds to the promoter of CD44 coding gene to suppress breast cancer metastasis, thereby hindering the progress of breast cancer (50). In addition, Gal-1 regulates the anti-tumor properties of Foxp3 by binding with the FKH domain of Foxp3 in Foxp3-positive breast cancer cells, thereby maintaining the stability of cancer cells (51). According to reports, Foxp3 is a downstream target of p53 mediated cell aging, promoting the aging of epithelial cancer cells by inducing the expression and generation of p21 and ROS (52). It Collaborates with microRNA-155 to regulate the transcription process of Zinc finger E-box binding homology box 2 (ZEB2), inhibiting the expression of ZEB2 in colon cancer. This inhibition leads to the suppression of cancer cell proliferation and metastasis, enhancing anti-tumor immunity (53) (Figure 1). Therefore, due to the widespread expression of Foxp3 in tumor cells, it has a dual role in tumor induced proliferation or inhibition. Studying the regulatory mechanisms of Foxp3+ Treg cells and Foxp3 involvement in malignant tumors is of great significance for understanding and developing treatments for each disease.
Figure 1 Expression of Foxp3 in relation to T cells and cancer cells in the CRC tumor microenvironment. In colon cancer cells, Foxp3 and microRNA-155 work together to synergistically regulate the transcription of Zinc finger E-box binding homology box 2 (ZEB2), which suppresses ZEB2 expression and boosts anti-tumor immunity, reducing cancer cell growth and metastasis; In addition, Overexpression of Foxp3 also promotes MMP9 expression through the SAM cycle; The decrease of TCF-1 leads to an increase in the binding of Foxp3 to downstream gene regulatory elements, thereby promoting the expression of Treg, inhibiting the proliferation of effector T cells, and promoting the progression of CRC. ZEB2,the Zinc finger E-box binding homology box 2; MMP9, Matrix Metallo Protein 9; TCF-1,T Cell Transcription Factor 1.
Expression of Foxp3 in relation to T cells and cancer cells in the CRC tumor microenvironment. In colon cancer cells, Foxp3 and microRNA-155 work together to synergistically regulate the transcription of Zinc finger E-box binding homology box 2 (ZEB2), which suppresses ZEB2 expression and boosts anti-tumor immunity, reducing cancer cell growth and metastasis; In addition, Overexpression of Foxp3 also promotes MMP9 expression through the SAM cycle; The decrease of TCF-1 leads to an increase in the binding of Foxp3 to downstream gene regulatory elements, thereby promoting the expression of Treg, inhibiting the proliferation of effector T cells, and promoting the progression of CRC. ZEB2,the Zinc finger E-box binding homology box 2; MMP9,Matrix Metallo Protein 9; TCF-1,T Cell Transcription Factor 1.
The translated Foxp3 protein precursor is altered by methylation, acetylation and deacetylation, phosphorylation, ubiquitination and deubiquitination, glycosylation, and other modifications in addition to epigenetic modifications that control Foxp3 expression during transcription. Additionally, Foxp3 expression may be impacted directly or indirectly by changes in sugar metabolism or short RNAs (Figure 2).
Figure 2 The regulation of transcription and translation modifications related to Foxp3 in Foxp3+ Treg cells. The transcription of Foxp3 and the activation process of Foxp3 are regulated by binding to non-coding sequences such as c-Rel, ENO1, and Tet2, like CNS0-3, during transcription. In addition, the long non-coding RNA (lncRNA) Fricr is similar to the Foxp3 genome and can act in cis on the transcription process of Foxp3. Foxp3's post-translational regulation process mainly involves the methylation of PRMT1, glycosylation of PARP-1, cooperative ubiquitination of USP44 and USP7, dephosphorylation of PP1, acetylation regulation of SIRT1 and p300, and glucose metabolism process. The interaction of HIF-1α with Foxp3 protein and other factors can influence the expression and function of Foxp3. TGF-β, Transforming Growth Factor β; USP44, Ubiquitin Specific Peptidase 44; USP7, Ubiquitin Specific Peptidase 7; PRMT1, Protein arginine methyltransferase 1; PARP-1, Poly (adp-ribose) polymerase 1; PPI, Phosphoproteinase 1; TNF-α, Tumor necrosis factor-α; GLUT, The glucose transporter; VEGF, Vascular Endothelial Growth Factor; HIF-1α, Hypoxia-inducible factor-1α; Tet2, Tet methylcytosine dioxygenase 2.
The regulation of transcription and translation modifications related to Foxp3 in Foxp3+ Treg cells. The transcription of Foxp3 and the activation process of Foxp3 are regulated by binding to non-coding sequences such as c-Rel, ENO1, and Tet2, like CNS0–3, during transcription. In addition, the long non-coding RNA (lncRNA) Fricr is similar to the Foxp3 genome and can act in cis on the transcription process of Foxp3. Foxp3’s post-translational regulation process mainly involves the methylation of PRMT1, glycosylation of PARP-1, cooperative ubiquitination of USP44 and USP7, dephosphorylation of PP1, acetylation regulation of SIRT1 and p300, and glucose metabolism process. The interaction of HIF-1α with Foxp3 protein and other factors can influence the expression and function of Foxp3. TGF-β, Transforming Growth Factor β; USP44, Ubiquitin Specific Peptidase 44; USP7, Ubiquitin Specific Peptidase 7; PRMT1, Protein arginine methyltransferase 1; PARP-1, Poly (adp-ribose) polymerase 1; PPI,Phosphoproteinase 1; TNF-α, Tumor necrosis factor-α; GLUT, The glucose transporter; VEGF, Vascular Endothelial Growth Factor; HIF-1α, Hypoxia-inducible factor-1α; Tet2, Tet methylcytosine dioxygenase 2.
It has been discovered that the conserved non-coding sequence CNS0–3 includes the Cage1 site, which is situated 1500 bp upstream of the core promoter, and the Cage2 site, which is situated 2000 bp upstream, as well as other required regulatory regions for Foxp3 gene expression (54). The TCR on T cells surface activates Foxp3 transcription negatively by binding to NF-κB. The conserved CNS3 regulatory region of Foxp3 is then joined with the c-Rel of the family. Conversely, acetylated c-Rel on the site inhibits Foxp3 production by adversely regulating the activation of the Foxp3 promoter (8). Alternatively, Foxp3 expression can be inhibited by the specific recruitment of enolase-1 in the glycolytic pathway to the Foxp3 promoter and its CNS2 regulatory region; however, this study also has shown that TCR activation leads to an increase in the cell’s ability to uptake glucose and glycolysis, which, in turn, leads to an increase in the expression of Foxp3 (9). Reports state that long non-coding RNA (lncRNA) fricr is comparable to the Foxp3 genome and has the ability to act cis on Foxp3’s transcription process, decreasing its stability (12); additionally, miR-125b has been shown in thyroid cancer research to target Foxp3 and suppress its mRNA and protein production while increasing cancer cells’ susceptibility to cisplatin treatments (14). Reduced Foxp3 expression is advantageous for enhancing the therapeutic benefit of patients on anti-cancer medications, as miR-4281 directly binds to the Foxp3 promoter, upregulates the expression of Foxp3 protein, and promotes the immunosuppressive role of Foxp3+Treg (15). To better guide anti-cancer treatment, it is imperative to have a thorough understanding of the mechanisms behind the activity of non-coding RNAs (ncRNAs), as they are also crucial in regulating the Foxp3 process.
The TCR signaling pathway has a distinct regulatory mechanism that manifests a twofold regulatory effect throughout the transcriptional activation process of Foxp3. Deoxyribonucleoside triphosphate triphosphate hydrolase (SAMHD1) co-localizes with ODNps25 in the cytoplasm and interacts directly with Foxp3 through phosphorylation of T592 in the TCR/CDK2/SAMHD1 pathway, which increases the stability of Foxp3 mRNA by inhibiting the activity of the dNTPase (10). The DNA demethylating Tet2 enzyme (a member of the TETase family) is increased by high-intensity TCR signaling stimulation (55) or TGF–induced phosphorylation of Uhrf1, which enables it to act on the CpG island of Foxp3 CNS2 and preserves the stability of the Foxp3+ Treg (11). However, Stephan Sauer et al. (13) has shown that stimulation of the PI3K/AKT/mTOR pathway reduces Foxp3 production and persistent TCR signaling prevents naive CD4+T cells from differentiating into Foxp3+CD4+ T cells. In summary, further research is necessary to fully understand the effects of Foxp3 involvement on cancer cells and Foxp3+Treg function in particular tumor microenvironments, as well as to demonstrate the advantageous role of Foxp3 therapeutic blockade in future immunotherapy strategies, which will offer fresh concepts for clinical targeted therapy. This is even though we have clarified the specific regulatory mechanisms of some Foxp3 transcription processes in different tumor types.
Foxp3+Treg’s inhibitory activity is improved by protein arginine methyltransferase 1 (PRMT 1), according to research by Yuki Kagoya and colleagues (16). The adp-ribosylation enzyme Poly (adp-ribose) polymerase 1 (PARP-1) enhances the multimerization (adp-ribosylation) of Foxp3 in contrast. Foxp3 is made unstable by PARP-1 through Stub1-mediated degradation and polyubiquitination, which inhibits Foxp3+Treg’s inhibitory effectand strengthens the immune system (17).
Foxp3+Treg regulation depends on ubiquitination and deubiquitination. It is discovered that activating or up-regulating the deubiquitinating enzyme USP7 in Treg cells could decrease Foxp3 ubiquitination, stabilize Foxp3 protein expression, and enhance Foxp3+Treg’s immunosuppressive effect. Conversely, inhibitors that prevent USP7 from being deubiquitinated can reduce Foxp3+Treg’s ability to suppress the immune system, which can allow tumor cells to evade the immune system (18). Furthermore, TGF-β, as The Smad signaling pathway, activates ubiquitin specific peptidase 44 (USP44), which can stabilize Foxp3 protein and eliminate ubiquitination modifications of K3 linked ubiquitin chain connections; the combined expression of USP44 and USP7 stabilizes Foxp3 expression, and the absence of USP44 in Treg cells can promote anti-tumor immunity and impede tumor growth (19).
The expression of associated proteins like S-phase kinase associated protein 2 (Skp2), matrix metallo protein 9 (MMP9), and vascular endothelial growth factor-A (VEGF-A) is inhibited by the tyrosine kinase LCK-dependent phosphorylation of Foxp3, particularly at tyrosine residue Tyr-191 (20). Additionally, it has been demonstrated that the Ser418 phosphorylation site, which is located at the C-terminus of Foxp3, is phosphorylated in autoimmune diseases. This phosphorylation site can promote the expression of Foxp3 proteins and preserves the inhibitory function of Foxp3+ Treg cells. In contrast, when the Ser418 site is specifically dephosphorylated by TNF-alpha-activated phosphoproteinase 1 (PP1), it can inhibit the function of Foxp3+ Treg cells (21). UBC9 is the only E2 enzyme for Small Ubiquitin-like Modifier (SUMO), which can also target the UBC9 promoter region to up-regulate SUMOization to regulate Foxp3+ Treg cell function after phosphorylation modification of the Y342F site of Foxp3 in human breast cancer cells (56).
Foxp3 protein acetylation, a mechanism that prevents proteasomal degradation, also regulates the level of Foxp3 expression. Loosdregt (22) reported for the first time that acetylation directly controled the level of Foxp3 protein. High levels of Foxp3 acetylation may prevent the protein from being polyubiquitylated, which raises the level of Foxp3 protein and aids in the development of T cells into Foxp3+ Treg. The acetylation modification of Foxp3 by histone acetyltransferases (HAT) p300 can compete with the ubiquitination modification co-acting on lysine residues and restrict Foxp3 degradation; on the other hand, the deacetylation modification of Foxp3 by histone acetyltransferase SIRT1 can obstruct Foxp3 synthesis. Additionally, the Foxp3 protein can be stabilized and acetylated by the TIP-p300-Foxp3 complex, which is formed when TIP60 and p300 work together (23). Additionally, Hye-Sook Koon (24) recently have discovered that SIRT1 modifies Foxp3’s lysine acetylation sites (K31, K262, and K267) by deacetylation in order to adversely control Foxp3 stability.
According to the study’s findings, Foxp3+Treg expression increased in the majority of tumors, and while the tumor microenvironment was anaerobic, HIF-1 aggregation was present (57). In this situation, HIF-1can bind to the Foxp3 protein and can inhibit HIF-1 degradation, and at the same time, Foxp3 can increase HIF-1’s downstream target genes, including VEGF and the glucose transporter protein (GLUT) (58). Lung cancer cell growth is aided by the MIF/NF-ƘB/HIF-1 pathway of macrophage migration inhibitory factor, which keeps the Warburg effect-related factors stable (25). In conditions with high lactate and low sugar, oxidizing lactate to pyruvate facilitates nicotinamide adenine dinucleotide (NAD) regeneration and oxidative phosphorylation. Foxp3+Treg benefits from changes in tumor glycolysis because it strengthens its immunosuppressive capacity in the tumor microenvironment and encourages tumor cell survival (59). Nonetheless, other researchers have also noted that Foxp3 protein’s ubiquitination is disrupted when HIF-1 α binds to it, which in turn impairs T cell development and, ultimately, the production and functionality of Foxp3+Treg (60).
In conclusion, changes in glucose metabolism enable tumor cells to maintain their energy source, promote growth, and adapt better to hypoxic environments. Still, there are additional elements in the glucose metabolism pathway that are regulated by Foxp3 and HIF-1α. We must conduct more research to better understand the connection between Foxp3 and its function.
Esophageal Carcinoma (ESCC) is a cancerous tumor that primarily affects the esophageal epithelium. According to research, the upstream regulatory factor p53 of miR-149–3p (61) is inhibited from being ubiquitinated when miR-5b-1p binds to the molecular sponge rcRUNX3 (62) or lncRNA MEG3. This helps to upregulate Foxp3 expression in ESCC. By increasing Foxp3 expression through STAT3 modification of Foxp3+Treg transcription process, activating the STAT3/Foxp3 signaling pathway within ESCC tissue hinders macrophage phagocytosis and increases Foxp3+Treg immunosuppressive activity, which helps ESCC evade the body’s immune response (26). Furthermore, upon recognition of its receptor ST2, IL-33 attracts a significant number of Foxp3+Tregs to congregate in the stroma of ESCC, thereby initiating the IL-33/ST2/Foxp3+Treg/COX-2/PGs regulatory pathway, encouraging Foxp3+Treg secretion of cyclooxygenase-2 (COX-2) and enabling the conversion and production of prostaglandins (PGs). These actions work in concert to preserve the immune system’s stability and suppress the tumor microenvironment’s effect, encouraging the proliferation and growth of ESCC cells (27).
The most prevalent type of pancreatic cancer is pancreatic ductal adenocarcinoma (PDAC), and an increase in Foxp3 in the tumor microenvironment of pancreatic cancer affects immune cells (DCs), which are antigen-presenting cells, impairing the body’s ability to mount an immune defense (63). It has been shown that Foxp3 works synergistically to control the recruitment of chemokine CCL5 to Treg cells in the tumor microenvironment to promote the immune escape of PDAC. Foxp3 also directly interacts with the promoter region of programmed cell death-ligand (PD-L1) to promote PD-L1 inhibition of effector T cell activation (3, 28, 64). Foxp3 also confers a suppressive phenotype on Tregs in pancreatic cancer cells by repressing the transcriptional activation of several T cell-stimulated target genes, such as Interleukin-2 (IL-2) (65). It is hypothesized that CTLA-4 could be linked to Foxp3+ T cell suppression of effector T cell function and mediate PDAC immune escape because it is another cytotoxic T lymphocyte-associated antigen with immunosuppressive effects that is expressed more frequently (66). The tissues of pancreatic tumors have elevated expression of the Ceramide synthase (CerSs) isoform CERS6. AKT1-mediated phosphorylation of Foxp3 at the S418 site aids in maintaining the production of CERS6, and elevated levels of CERS6 mRNA predict a worse prognosis for PDAC patients, according to ex vivo research. In addition, CERS6 promotes the p53 mutation that causes PDAC. As a result, inhibiting the AKT1/FOXP3/CERS6 axis can be a possible tactic to prevent the growth of pancreatic tumors (29).
Genetic variations and epigenetic changes are linked to an increased risk of gastric cancer (GC) (67). In GC tumor tissues, Foxp3 protein is expressed more intracellularly (68). When compared to GC tumor tissues, the CD8/Foxp3 ratio in GC paracancerous tissues is much greater, and the elevated expression of PD-L1 could signal a bad prognosis for GC patients (69). The number of Foxp3 + Treg cells is significantly higher than that of paraneoplastic tissues, and the release of COX-2 by Foxp3 + Treg leads to the induction of Prostaglandin E2 (PGE2) expression. These factors together inhibits the anti-cancer effects of effector T cells (6), allowing GC to avoid immune attack and promoting its immune escape and proliferation. Additionally, using COX-2 inhibitors to treat GC offers the chance to reduce Foxp3+ Treg activity. Foxp3 interacts to the PSMD7 promoter and stimulates PSMD7 expression, both of which are up-regulated in gastric cancer tissues, boosting GC cell proliferation and blocking apoptosis (70). With extensive research, Li et al. (30) discovered that FoxM1, along with an increase in the number of Foxp3+Treg, increased significantly in gastric cancer tissues, that overexpression of FoxM1 and Foxp3+Treg favored GC infiltration and invasion, and that inhibition of the regulatory pathways of FoxM1 and Foxp3 could block GC proliferation, implying that the combination of FoxM1 and Foxp3+Treg could be used as a biomarker for diagnosis and prognostic survival of clinical gastric cancer patients. Foxp3+Treg cells share the functional characteristics of Foxp3+Treg cells and RORt-expressing TH17 cells, respectively, for having enhanced immunosuppressive and pro-inflammatory effects, while inhibiting the production of anticancer factors, such as IFN-γ, granzyme B, and other anticancer factors by effector T cells, resulting in an imbalance in the body’s immunity to achieve anticancer immune escape, which is detrimental to the prognosis of patients (71).
Furthermore, investigations have demonstrated that Foxp3 plays an oncogenic function in GC. Foxp3 promotes p21 protein expression by binding to the p21 promoter region, which inhibits cancer cell proliferation; however, in the presence of a large amount of inflammatory infiltration in the GC, Foxp3 has an enhanced interaction with p65, which reduces binding to the p21 promoter, thus contributing to cancer cell proliferation (72); S-phase kinase-associated protein 2 (SKP2) is associated with a double negative feedback loop of p21 and p27, which can lead to the hypothesis that overexpression of Foxp3 in GC inhibits the oncogenic effects of SKP2 (73); the MKL1/miR34a/Foxp3 pathway inhibits the expression of Foxp3 and promotes the proliferation of GC (31). Foxp3 inhibits GC cell proliferation by activating the apoptotic signaling pathway, and increasing Foxp3 expression can increase the expression of pro-apoptotic genes such as PARP, caspase-3, and Casp9, effectively inducing apoptosis in GC cells, and vice versa (74). Foxp3 suppression or binding to miR-133a-3p can limit Foxp3 expression, which stimulates GC cell proliferation and autophagy, helping to deplete and remove damaged cells and maintain cancer cell homeostasis (32). Meanwhile, Foxp3 reduces COX2 expression and cell metastasis as a negative regulator of NF-ƘB activity, presenting new therapeutic and diagnostic alternatives for gastric cancer (75, 76).
The study of CRC markers and their associated signaling pathways may offer suggestions for targeted therapy treatment of colon cancer because colorectal cancer (CRC) is a common cancer of the digestive system, the incidence rate is rising annually in China, and the survival rate of patients is poor (77). Treg cells have different subtypes, and Treg accumulates at the tumor site. On the one hand, it may be due to the co expression of chemokines and other products such as Foxp3 and Helios produced by tumor cells and stroma in the TME of CRC, which can enhance the inhibitory characteristics of Treg more strongly than other molecules acting alone. In addition, The expression levels of PD-1/CTLA-4 and PD-1/CD39 in the subgroup of Treg are elevated. They have a synergistic effect in inhibiting T cell activation and function, as well as inhibiting tumor specific immune responses, thereby helping tumor cells evade T cell immune attacks and promoting cancer cell progression (33). On the other hand, in the presence of TGF - β, it can also drive the expression of tumor infiltrating Treg cells. In addition, The Treg density of FOXP3 in tumor tissue is higher than that in normal colon mucosa, and its increased expression is associated with poor CRC survival rate. Overexpression of Foxp3 promotes MMP9 expression through the SAM cycle, promoting liver metastasis in CRC (78–82) (Figure 1). TCF-1 and Foxp3 can bind to regulate the same genes. TCF-1 can control T cell development in the thymus (34). As TCF-1 levels drop in CRC, more Foxp3 can bind to downstream gene regulatory elements, promoting the development of Treg, inhibiting the growth of effector T cells, and advancing CRC (83) (Figure 1). While Foxp3+Treg is not positively correlated with Foxp3+ cancer cells, and purely high expression of Foxp3+Treg has no correlation with CRC prognosis, some researchers have discovered, contrary to the majority of findings, that high expression of Foxp3 in Foxp3+ cancer cells is correlated with poor prognosis (84). Additionally, Liu et al.’s research (85) demonstrated that Foxp3 expression was markedly down-regulated in colon cancer stem cells, that activating the Foxp3/NF-κB/COX2 pathway could prevent COX2’s transcriptional activation and impede the proliferation of colon cancer stem cells, and that Foxp3 was able to inhibit the growth of cancer cells. In summary, the research on Foxp3 expression in CRC is valuable and has predictive implications, but further investigation is required to comprehend its role in different cell types. This knowledge will be beneficial for improving CRC treatment and prevention strategies.
Hepatocellular carcinoma (HCC) refers to malignant tumors of the liver, including primary and metastatic hepatocellular carcinoma, and HCC patients are often detected in peripheral mononuclear lymphocytes with a significant increase in the expression of the factors Foxp3 and RORt, which are closely related to the development of HCC, compared to normal cells (86, 87). Yong Huang (88) has discovered that in HBV-infected people, IL-17-producing Th17 cells congregate with Foxp3+ Treg cells, facilitating HCC progression. MMP12 might promote Foxp3+Treg infiltration in tumor tissue; TLR4 might obliquely attract Foxp3+Treg to the tumor location by interacting with TGF-β and macrophages (89, 90). Through promoting Treg cell polarization, it facilitates HCC immunological escape. High basal levels of lnc-EGFR specifically bind to EGFR in HCC patients, blocking its interaction and ubiquitination with c-CBL, stabilizing it and enhancing its own and downstream activation of the AP-1/NF-AT1 axis. This prolongs the lifespan of EGFR, drives Treg differentiation, stifles CTL activity, and encourages the growth of HCC (91). Furthermore, it was discovered that TLR ligands, particularly polyIC (a synthetic double-stranded RNA polyinosinic polycytidylic acid), stimulated an increased capacity of hepatocellular carcinoma cell line (CCL-9.1) in mice to release Hepatoma Derived Growth Factor (HDGF), as well as Foxp3+ Treg cells to proliferate, which together inhibit the release of perforin and granzyme B from effector CD8+ T cells into the tumor microenvironment for the purpose of assisting cancer cell immune escape (35). The binding of the lncRNA NEAT1 to the Foxp3 binding site results in high expression of its downstream target gene, pyruvate kinase PKM2, and over-expression of NEAT1/Foxp3 promotes PKM2 transcriptional activation (92), which promotes HCC proliferation by enhancing the aerobic glycolysis pathway.
Furthermore, Foxp3 are found to have an oncogenic influence in both HCC and CRC. Mutations in the FKH structural domain of Foxp3 has been found at the transcriptional level in HCC tumor tissues, affecting the function of controlling the expression of target genes (93). Foxp3 expression is down-regulated in HCC tissues, but P62 expression is up-regulated. Low Foxp3 expression and P62 over-expression are found to be closely connected to a decrease in overall survival in HCC patients (94). Liu et al. (95) has discovered that the Foxp3 promoter and CpG region are hypermethylated by NA (cytosine-5)-methyl transferase 1 (DNMT1), which can limit Foxp3+Treg function and negatively controls HCC progression. Moreover, Gong et al. (96) also can confirme that Foxp3 exhibits oncogenic effects in HCC through in vitro and in vivo experiments, and Foxp3 regulates the TGF-β/smad3/4 pathway to recognize and directly or indirectly act on the Myc promoter region of oncogenes to inhibit oncogene expression; at the same time, the over-expression of Foxp3 could promote the increase of apoptotic marker Bax and expression of apoptosis inhibitor p53, which promote cancer cell apoptosis (97, 98).
Regulating T cell infiltration is a major obstacle to immunotherapy in TME and is often associated with poor prognosis. We found that the accumulation of Foxp3+Treg cells in lung cancer is higher than that in normal tissues, and the increased infiltration of regulatory T cells into the core tumor area may be an independent predictor of poor overall survival in non-small cell lung cancer (NSCLC) patients (99). We found in vitro culture that over-expression of Foxp3 in Treg cells enhances the activity and invasiveness of related immune cells, indicating that an increase in Foxp3 levels in the tumor microenvironment may promote tumor cell growth (44); And the correlation between TGF - β and Foxp3 was also shown in the lungs of non-small cell lung cancer patients; Compared with patients with limited period NSCLC, the incidence of circulating CD4+CD25+Foxp3+Treg cells in advanced NSCLC patients is significantly increased, and the frequency of circulating CD4+CD25+Foxp3+Treg cells is negatively correlated with interleukin (IL) -17 and positively correlated with serum IL-10 levels (100). Therefore, an increase in circulating CD4+CD25+Foxp3+Treg cells may be involved in the pathogenesis of NSCLC. In addition, analysis of 70 cervical cancer patients found that, The expression of Foxp3 and VISTA is associated with clinical staging, The group with double positive expression of Foxp3 and VISTA had the worst prognosis, The positive expression of Foxp3 and VISTA may serve as independent prognostic factors for cervical cancer, providing strong evidence for immunotherapy of cervical cancer (101). However, in breast cancer patients, the expression of chemokine receptor CCR4 in tumor infiltrating Tregs is higher than that in peripheral Tregs, The expression of FOXP3 in breast cancer tissue is higher than that in normal tissue, and overexpression of FOXP3 is associated with better prognosis. Knockdown FOXP3 with siRNA in vitro can promote the migration and invasion of human breast cancer MCF-7 cells; In addition, CCL22 and CCL17 released by tumor cells and tumor associated macrophages can attract CCR4+Tregs to the tumor site, and FOXP3 and HAT1 can together epigenetically alter the promoter on CCR4+Tregs, providing space for FOXP3 binding and CCR4 gene activation. Overexpression of FOXP3 increases the infiltration of CCR4+Tregs cells, leading to reduced anti-tumor immune response and tumor progression. These findings indicate that, as a transcriptional activator of CCR4 and a regulator of Treg invasion, FOXP3 overexpression is related to the good prognosis of breast cancer and plays an important role in the tumor microenvironment of breast cancer (102–104).
Based on the above research, we found that Foxp3+Treg cells are widely involved in the immune regulation of different types of tumor cells, but their roles vary in different types of tumors, with both positive and negative effects. Therefore, we need to further explore and analyze the specific functions of Foxp3+Treg cells in a certain tumor, in order to provide ideas for the treatment of tumors.
Tumor immunotherapy is progressively being used in clinical practice to treat a variety of malignant tumors, in addition to conventional surgical resection, radiation, and chemotherapy (105–107). This has a significant positive impact on the clinical outcomes of various cancers and gives cancer patients hope. Tumor immunotherapy is the use of novel medications to actively or passively interact with intracellular signaling pathways and receptors linked to the transformation and progression of cancer. This results in an immune response specific to the tumor, inhibits the growth and survival of cancer cells, and kills or inhibits tumors (108). To enhance the body’s anti-tumor response and increase the effectiveness of cancer patients’ treatments, tumor immunotherapy mostly consists of immune checkpoint inhibitors (ICIs), adoptive cell transfer therapy (ACT), anti-tumor monoclonal antibodies (mAbs), tumor vaccines, small molecule antibodies, etc (109–111).
Patients with various cancers showed an increase in Foxp3+Treg cells in their tumor microenvironment. These cells can help tumor cells evade the immune system by blocking anti-tumor immunity. This is typically linked to a patient’s clinical prognosis and poor tumor development (3, 112–115). Since Foxp3 is the primary distinctive marker of Foxp3+Treg cells (3), Foxp3-targeting medications include enzyme inhibitors, both synthetic and natural; Foxp3’s research and development typically entail changing process-related enzymes, downstream small molecules, or Foxp3 itself in order to interfere with its transcription and translation processes, limit its output and lowers the expression of the immunosuppressive subgroup Foxp3+Treg of CD4+T cells as well as their ability to fight tumors (116). Foxp3-microRNA or Foxp3-shRNA generated by R&D design can be delivered to tumor cells in an experimental study to decrease Foxp3 expression and Foxp3+ Treg function using Ultrasound-Targeted Microbubble Destruction (UTMD) for gene delivery (117). The synthetic peptide can inhibit Foxp3 protein-protein (PPI) interactions, and as a result, it has the potential to be employed as a novel medication to disrupt Foxp3+Treg function. Hawley et al. (118) investigated that hydrocarbon-anastomosing α-helix (SAH) peptide could target the Foxp3 homodimer protein region, blocking interactions between Foxps, disrupting the signaling pathway, and decreasing Foxp3-mediated immunosuppression. However, the peptide cannot have membrane-penetrating properties and needs to be transfected into the cells, but the specific mechanism of action is still unclear and needs to be explored in depth. In light of the fact that Foxp3 also occurs in the organism as a complex and that Foxp3 collaborates with Rcor1/2 to create the COREST complex, inhibitors of the COREST complex block Rcor1 endoenzyme activity, which in turn prevents Foxp3+Treg activity (119). Methotrexate (MTX), a medication used to treat psoriasis, controls immunological homeostasis by boosting Foxp3 mRNA expression and Treg cell expression (120). To enhance the body’s ability to fight tumors, it is crucial to thoroughly evaluate strategies for blocking the Foxp3 regulatory pathway, considering both the cancer-promoting and cancer-fighting effects of Foxp3 in tumors.
Moreover, a group of antibodies called immune checkpoint inhibitors (ICIs) work by blocking inhibitory receptors on immune cells. In medical settings, these immunotherapeutic drugs target immune cells to some extent and show significant anti-cancer effects in cancer patients. Currently, immune checkpoint blockers (ICBs) can directly target Foxp3+Treg by focusing on co-inhibitory receptors like anti-CTLA4, anti-PD-1, and anti-PD-ligand 1 (PD-L1). This approach can enhance anti-tumor immune responses by hindering the immunosuppressive function of Foxp3+Treg. However, despite the considerable success, a considerable number of patients have not shown positive responses (121). However, the loss of Treg homeostasis can also result in potentially fatal autoimmune adverse events (irAEs) because Tregs play a critical role in immunological tolerance maintenance and the prevention of autoimmune disorders (122) (Table 2). The most frequent and early adverse event (irAE) is skin toxicity, and immune regulatory effects triggered by ICIs and targeted therapy are required for anti-tumor effectiveness. But because cancerous cells and healthy skin mucosal tissue share a signaling route, immunotherapy-induced stimulation may also impact healthy skin tissue, which can result in the development of autoimmune skin disorders (123–127). According to preliminary observations, the therapy of ICIs was also observed to have an impact on the cardiovascular system, including conditions such as myocarditis, fulminant myocarditis, arrhythmia, venous thromboembolic illness, acceleration of atherosclerosis, atherosclerosis, and other associated cardiovascular issues (128, 129). Treatment with inhibitors of inflammatory response modifiers (ICIs) has been linked to an increase in cardiovascular events not just during the initial few weeks of treatment but even months or years after treatment commencement; However, the risks of cardiac toxicity vary throughout combination immunotherapy techniques and ICI treatment regimens. When using a single ICI treatment, CTLA-4 may be more cardiacally toxic than PD-1 or PD-L1. When using dual therapy, there is a greater chance of cardiac toxicity with dual ICI therapy than with single chemotherapy or single targeted therapy (130). Moreover, a lot of people have grown resistant to medications over time (131–134). The resistance mechanisms of sporadic MSI-H endoplasmic reticulum cells were revealed to be antigen processing, presentation flaws, and induction disorders in interferon response in a prospective phase 2 pilot study based on pembrolizumab in patients with recurrent MSI-H endometrial cancer. Patients with high microsatellite instability (MSI-H)/mismatch repair defects (dMMR) appear to be able to slow their progression with surgical resection or local therapy, in addition to ongoing pembrolizumab discontinuation studies (145). Furthermore, ICIs and targeted therapy can cause rheumatoid arthritis clinical symptoms, nail toxicity, oral mucosal toxicity, and hair loss (135–140, 146–148). In addition, ICI treatment can also lead to hepatitis (141–144). Eleonora et al. evaluated the features of liver injury using ICIs and discovered that liver biopsy is useful in determining the diagnosis and degree of liver injury in patients with metastatic cancer undergoing immune-mediated hepatitis. According to this study, patient-centered care is crucial and could eventually prevent the need for needless systemic corticosteroid therapy (149). Patients receiving targeted and immune checkpoint inhibitor (ICI) therapy for multiple health conditions need to carefully follow the Society for Immunotherapy of Cancer’s (SITC) Clinical Practice Guidelines for Adverse Events Related to Immune Checkpoint Inhibitors and the National Comprehensive Cancer Network’s (NCCN) updated guideline for managing immune-related adverse events (IRAEs). It is crucial for patients to work closely with dermatologists and other specialists, and make informed decisions about their medications based on scientific guidance (150–152). This approach helps minimize the impact of treatment side effects on patients’ well-being and encourages their commitment to and enthusiasm for the therapy.
Table 2 The loss of Treg homeostasis can also lead to life-threatening autoimmune adverse events (irAEs).
Maintaining a balanced immune response is a significant challenge when targeting and suppressing Tregs within the tumor microenvironment (TME) of cancer patients, without affecting overall self-tolerance. To address this, modifying nanobiomaterials to create intelligent nanocarriers for drug delivery systems (DDS) can be helpful. By delivering ICI to specific targets such as Foxp3+Treg, the immunosuppressive TME can be altered to boost anti-tumor immunity, while minimizing side effects and improving the effectiveness of ICI treatment. This approach leads to safe and efficient cancer immunotherapy, with these specially designed nanoparticles offering advantages over traditional delivery methods (153). Thus, more study is needed in the direction of targeting tumor-specific Tregs without compromising Treg homeostasis overall and preventing the development of irAEs. Furthermore, because of the comprehensive study of tumor immunology theory and the ongoing advancements in technology, ICIs in conjunction with other anti-cancer therapeutic modalities have been approved for use in a variety of cancer types (154). For example, research on triple negative breast cancer (TNBC) suggests that combining immunotherapy with cancer vaccines and immune checkpoint inhibitors may be beneficial for non-immunogenic tumors. In a study by Liu et al., nanoparticles (NPs) were created to deliver mRNA vaccines encoding the tumor antigen MUC1 to dendritic cells (DCs) in lymph nodes, aiming to activate tumor-specific T lymphocytes. By combining the mRNA vaccine with an anti-CTLA-4 monoclonal antibody, the anti-tumor effects can be maximized. NP-based mRNA vaccines that target mannose receptors on DCs have shown in vivo efficacy in expressing tumor antigens and inducing a strong cytotoxic T lymphocyte response in TNBC 4T1 cells. Furthermore, combining vaccines with anti-CTLA-4 monoclonal antibodies has been found to significantly enhance the anti-tumor immune response compared to using either treatment alone. These results highlight the potential synergistic benefits of NP-based mRNA and CTLA-4 inhibitors in treating TNBC, with NPs serving as a carrier for mRNA vaccine delivery (155). Thus, it is anticipated that the combined use of other tumor treatment techniques will lead to new advancements in cancer eradication.
Based on the above discussion, we should now have a better understanding of the function of Foxp3+Treg in tumor immunity by reviewing a wide range of prior studies. Particularly, the investigation of the mechanism regulating the expression of its key characteristic transcription factor Foxp3 in digestive malignancies offers a broad array of supportive therapeutic targets and early diagnostic markers with predictive value for tumor diagnosis and treatment. Nevertheless, little is known about how Foxp3, a crucial regulatory component in tumor immunology, affects the immune response that relies on Foxp3+Treg cells in the tumor microenvironment. From the standpoint of Foxp3, we still do not fully understand how Foxp3 is expressed during the post-translational modification stage or the precise mechanisms underlying its function in various cell types. Thus, more studies on Foxp3 are required to enhance our understanding of Foxp3+Treg cells and to guide the development of new immunotherapy approaches. Furthermore, specific pharmacological technologies should be developed for treatment because the Foxp3 pathway is currently underutilized in the development of anti-tumor therapies, as indicated by available experimental evidence. By disrupting signaling pathways, regulating the immune balance of the tumor system, mediating Foxp3 expression and the immunosuppressive effect of Foxp3+ Treg cells, and employing a variety of treatment modalities simultaneously to enhance anti-cancer immunity, we aim to achieve the goal of curative treatment for tumors.
WW: Writing – review & editing, Writing – original draft, Visualization, Investigation. MD: Writing – review & editing, Supervision, Investigation. QW: Writing – review & editing, Investigation. YS: Writing – review & editing, Investigation. KH: Writing – review & editing, Investigation. XC: Writing – review & editing, Investigation. ZX: Writing – review & editing, Investigation. LL: Writing – review & editing, Supervision, Resources, Funding acquisition.
The author(s) declare financial support was received for the research, authorship, and/or publication of this article. This research was supported by Jointly Guided Project of Heilongjiang Provincial Natural Fund(SS2021H001) and Climbing Project of Basic Scientific Research Operating Expenses of Heilongjiang Provincial Department of Education (2020-kyywf-0741).
The authors declare that the research was conducted in the absence of any commercial or financial relationships that could be construed as a potential conflict of interest.
All claims expressed in this article are solely those of the authors and do not necessarily represent those of their affiliated organizations, or those of the publisher, the editors and the reviewers. Any product that may be evaluated in this article, or claim that may be made by its manufacturer, is not guaranteed or endorsed by the publisher.
1. Zhouyi Y, Mengyuan W, Weicheng Y, Kaifeng P, Wenqing L. Interpretation of the 2022 US cancer statistics report and comparison of cancer epidemic in China and the Q21 United State. J Multidiscip Cancer Management. (2022) 8(02):54–63. doi: 10.12151/JMCM.2022.02-05
2. Sinicrope FA, Rego RL, Ansell SM, Knutson KL, Foster NR, Sargent DJ. Intraepithelial effector (CD3+)/regulatory (FoxP3+) T-cell ratio predicts a clinical outcome of human colon carcinoma. Gastroenterology. (2009) 137:1270–9. doi: 10.1053/j.gastro.2009.06.053
3. Wang X, Lang M, Zhao T, Feng X, Zheng C, Huang C, et al. Cancer-FOXP3 directly activated CCL5 to recruit FOXP3(+)Treg cells in pancreatic ductal adenocarcinoma. Oncogene. (2017) 36:3048–58. doi: 10.1038/onc.2016.458
4. Shen Z, Zhou S, Wang Y, Li RL, Zhong C, Liang C, et al. Higher intratumoral infiltrated Foxp3+ Treg numbers and Foxp3+/CD8+ ratio are associated with adverse prognosis in resectable gastric cancer. J Cancer Res Clin Oncol. (2010) 136:1585–95. doi: 10.1007/s00432–010-0816–9
5. Wang Y, Liu T, Tang W, Deng B, Chen Y, Zhu J, et al. Hepatocellular carcinoma cells induce regulatory T cells and lead to poor prognosis via production of transforming growth factor-β1. Cell Physiol Biochem. (2016) 38:306–18. doi: 10.1159/000438631
6. Yuan XL, Chen L, Li MX, Dong P, Xue J, Wang J, et al. Elevated expression of Foxp3 in tumor-infiltrating Treg cells suppresses T-cell proliferation and contributes to gastric cancer progression in a COX-2-dependent manner. Clin Immunol (Orlando Fla). (2010) 134:277–88. doi: 10.1016/j.clim.2009.10.005
7. Deng G, Song X, Fujimoto S, Piccirillo CA, Nagai Y, Greene MI. Foxp3 post-translational modifications and Treg suppressive activity. Front Immunol. (2019) 10:2486. doi: 10.3389/fimmu.2019.02486
8. de Jesus TJ, Tomalka JA, Centore JT, Staback Rodriguez FD, Agarwal RA, Liu AR, et al. Negative regulation of FOXP3 expression by c-Rel O-GlcNAcylation. Glycobiology. (2021) 31:812–26. doi: 10.1093/glycob/cwab001
9. De Rosa V, Galgani M, Porcellini A, Colamatteo A, Santopaolo M, Zuchegna C, et al. Glycolysis controls the induction of human regulatory T cells by modulating the expression of FOXP3 exon 2 splicing variants. Nat Immunol. (2015) 16:1174–84. doi: 10.1038/ni.3269
10. Kim YC, Kim KK, Yoon J, Scott DW, Shevach EM. SAMHD1 posttranscriptionally controls the expression of Foxp3 and Helios in human T regulatory cells. J Immunol (Baltimore Md: 1950). (2018) 201:1671–80. doi: 10.4049/jimmunol.1800613
11. Sun X, Cui Y, Feng H, Liu H, Liu X. TGF-β signaling controls Foxp3 methylation and T reg cell differentiation by modulating Uhrf1 activity. J Exp Med. (2019) 216:2819–37. doi: 10.1084/jem.20190550
12. Zemmour D, Pratama A, Loughhead SM, Mathis D, Benoist C. Flicr, a long noncoding RNA, modulates Foxp3 expression and autoimmunity. Proc Natl Acad Sci U States A. (2017) 114:E3472–e80. doi: 10.1073/pnas.1700946114
13. Sauer S, Bruno L, Hertweck A, Finlay D, Leleu M, Spivakov M, et al. T cell receptor signaling controls Foxp3 expression via PI3K, Akt, and mTOR. Proc Natl Acad Sci U States A. (2008) 105:7797–802. doi: 10.1073/pnas.0800928105
14. Wang S, Wu J, Ren J, Vlantis AC, Li MY, Liu SYW, et al. MicroRNA-125b interacts with Foxp3 to induce autophagy in thyroid cancer. Mol Ther. (2018) 26:2295–303. doi: 10.1016/j.ymthe.2018.06.015
15. Zhang Y, Liu W, Chen Y, Liu J, Wu K, Su L, et al. A cellular microRNA facilitates regulatory T lymphocyte development by targeting the FOXP3 promoter TATA-box motif. J Immunol (Baltimore Md: 1950). (2018) 200:1053–63. doi: 10.4049/jimmunol.1700196
16. Kagoya Y, Saijo H, Matsunaga Y, Guo T, Saso K, Anczurowski M, et al. Arginine methylation of FOXP3 is crucial for the suppressive function of regulatory T cells. J autoimmunity. (2019) 97:10–21. doi: 10.1016/j.jaut.2018.09.011
17. Luo X, Nie J, Wang S, Chen Z, Chen W, Li D, et al. Poly(ADP-ribosyl)ation of FOXP3 protein mediated by PARP-1 regulates the function of regulatory T cells. J Biol Chem. (2016) 291:1201. doi: 10.1074/jbc.A115.661611
18. Wang F, Wang L, Wu J, Sokirniy I, Nguyen P, Bregnard T, et al. Active site-targeted covalent irreversible inhibitors of USP7 impair the functions of Foxp3+ T-regulatory cells by promoting ubiquitination of Tip60. PLoS One. (2017) 12:e0189744. doi: 10.1371/journal.pone.0189744
19. Khosravi M, Bidmeshkipour A, Cohen JL, Moravej A, Hojjat-Assari S, Naserian S, et al. Induction of CD4(+)CD25(+)FOXP3(+) regulatory T cells by mesenchymal stem cells is associated with modulation of ubiquitination factors and TSDR demethylation. Stem Cell Res Ther. (2018) 9:273. doi: 10.1186/s13287–018-0991–1
20. Nakahira K, Morita A, Kim NS, Yanagihara I. Phosphorylation of FOXP3 by LCK downregulates MMP9 expression and represses cell invasion. PLoS One. (2013) 8:e77099. doi: 10.1371/journal.pone.0077099
21. Nie H, Zheng Y, Li R, Guo TB, He D, Fang L, et al. Phosphorylation of FOXP3 controls regulatory T cell function and is inhibited by TNF-α in rheumatoid arthritis. Nat Med. (2013) 19:322–8. doi: 10.1038/nm.3085
22. van Loosdregt J, Vercoulen Y, Guichelaar T, Gent YY, Beekman JM, van Beekum O, et al. Regulation of Treg functionality by acetylation-mediated Foxp3 protein stabilization. Blood. (2010) 115:965–74. doi: 10.1182/blood-2009–02-207118
23. Bin Dhuban K, d'Hennezel E, Nagai Y, Xiao Y, Shao S, Istomine R, et al. Suppression by human FOXP3(+) regulatory T cells requires FOXP3-TIP60 interactions. Sci Immunol. (2017) 2(12):eaai9297. doi: 10.1126/sciimmunol.aai9297
24. Kwon HS, Lim HW, Wu J, Schnölzer M, Verdin E, Ott M. Three novel acetylation sites in the Foxp3 transcription factor regulate the suppressive activity of regulatory T cells. J Immunol (Baltimore Md: 1950). (2012) 188:2712–21. doi: 10.4049/jimmunol.1100903
25. Li J, Zhang J, Xie F, Peng J, Wu X. Macrophage migration inhibitory factor promotes Warburg effect via activation of the NF−κB/HIF−1α pathway in lung cancer. Int J Mol Med. (2018) 41:1062–8. doi: 10.3892/ijmm.2017.3277
26. Yang L, Zhao Q, Wang X, Pilapong C, Li Y, Zou J, et al. Investigation on the regulatory T cells signature and relevant Foxp3/STAT3 axis in esophageal cancer. Cancer Med. (2023) 12:4993–5008. doi: 10.1002/cam4.5194
27. Cui G, Li Z, Ren J, Yuan A. IL-33 in the tumor microenvironment is associated with the accumulation of FoxP3-positive regulatory T cells in human esophageal carcinomas. Virchows Archiv: an Int J pathol. (2019) 475:579–86. doi: 10.1007/s00428–019-02579–9
28. Wang X, Li X, Wei X, Jiang H, Lan C, Yang S, et al. PD-L1 is a direct target of cancer-FOXP3 in pancreatic ductal adenocarcinoma (PDAC), and combined immunotherapy with antibodies against PD-L1 and CCL5 is effective in the treatment of PDAC. Signal transduction targeted Ther. (2020) 5:38. doi: 10.1038/s41392–020-0144–8
29. Qi D, Song X, Xue C, Yao W, Shen P, Yu H, et al. AKT1/FOXP3 axis-mediated expression of CerS6 promotes p53 mutant pancreatic tumorigenesis. Cancer Lett. (2021) 522:105–18. doi: 10.1016/j.canlet.2021.06.024
30. Li X, Ma K, Song S, Shen F, Kuang T, Zhu Y, et al. Tight correlation between FoxM1 and FoxP3+ Tregs in gastric cancer and their clinical significance. Clin Exp Med. (2018) 18:413–20. doi: 10.1007/s10238–018-0505–6
31. Li JP, Liao XH, Xiang Y, Yao A, Fan LJ, Li H, et al. MKL1/miR34a/FOXP3 axis regulates cell proliferation in gastric cancer. J Cell Biochem. (2019) 120:7814–24. doi: 10.1002/jcb.28056
32. Li JP, Zhang HM, Liu MJ, Xiang Y, Li H, Huang F, et al. miR-133a-3p/FOXP3 axis regulates cell proliferation and autophagy in gastric cancer. J Cell Biochem. (2020) 121:3392–405. doi: 10.1002/jcb.29613
33. Syed Khaja AS, Toor SM, El Salhat H, Ali BR, Elkord E. Intratumoral FoxP3(+)Helios(+) regulatory T cells upregulating immunosuppressive molecules are expanded in human colorectal cancer. Front Immunol. (2017) 8:619. doi: 10.3389/fimmu.2017.00619
34. Gounari F, Khazaie K. TCF-1: a maverick in T cell development and function. Nat Immunol. (2022) 23:671–8. doi: 10.1038/s41590–022-01194–2
35. Sun AM, Li CG, Zhang YQ, Lin SM, Niu HR, Shi YS. Hepatocarcinoma cell-derived hepatoma-derived growth factor (HDGF) induces regulatory T cells. Cytokine. (2015) 72:31–5. doi: 10.1016/j.cyto.2014.12.001
36. Lu L, Barbi J, Pan F. The regulation of immune tolerance by FOXP3. Nat Rev Immunol. (2017) 17:703–17. doi: 10.1038/nri.2017.75
37. Lopes JE, Torgerson TR, Schubert LA, Anover SD, Ocheltree EL, Ochs HD, et al. Analysis of FOXP3 reveals multiple domains required for its function as a transcriptional repressor. J Immunol (Baltimore Md: 1950). (2006) 177:3133–42. doi: 10.4049/jimmunol.177.5.3133
38. Koh KP, Sundrud MS, Rao A. Domain requirements and sequence specificity of DNA binding for the forkhead transcription factor FOXP3. PLoS One. (2009) 4:e8109. doi: 10.1371/journal.pone.0008109
39. Zhan H, Sinclair J, Adams S, Cale CM, Murch S, Perroni L, et al. Immune reconstitution and recovery of FOXP3 (forkhead box P3)-expressing T cells after transplantation for IPEX (immune dysregulation, polyendocrinopathy, enteropathy, X-linked) syndrome. Pediatrics. (2008) 121:e998–1002. doi: 10.1542/peds.2007–1863
40. De Benedetti F, Insalaco A, Diamanti A, Cortis E, Muratori F, Lamioni A, et al. Mechanistic associations of a mild phenotype of immunodysregulation, polyendocrinopathy, enteropathy, x-linked syndrome. Clin Gastroenterol Hepatol. (2006) 4:653–9. doi: 10.1016/j.cgh.2005.12.014
41. Gerriets VA, Kishton RJ, Johnson MO, Cohen S, Siska PJ, Nichols AG, et al. Foxp3 and Toll-like receptor signaling balance T(reg) cell anabolic metabolism for suppression. Nat Immunol. (2016) 17:1459–66. doi: 10.1038/ni.3577
42. Howie D, Cobbold SP, Adams E, Ten Bokum A, Necula AS, Zhang W, et al. Foxp3 drives oxidative phosphorylation and protection from lipotoxicity. JCI Insight. (2017) 2:e89160. doi: 10.1172/jci.insight.89160
43. Angelin A, Gil-de-Gómez L, Dahiya S, Jiao J, Guo L, Levine MH, et al. Foxp3 reprograms T cell metabolism to function in low-glucose, high-lactate environments. Cell Metab. (2017) 25:1282–93.e7. doi: 10.1016/j.cmet.2016.12.018
44. Peng J, Yu Z, Xue L, Wang J, Li J, Liu D, et al. The effect of foxp3-overexpressing Treg cells on non-small cell lung cancer cells. Mol Med Rep. (2018) 17:5860–8. doi: 10.3892/mmr.2018.8606
45. Liu Y, Tu H, Zhang L, Xiong J, Li L. FOXP3−induced LINC00885 promotes the proliferation and invasion of cervical cancer cells. Mol Med Rep. (2021) 23(6):458. doi: 10.3892/mmr.2021.12097
46. Huang C, Zhou L, Chang X, Pang X, Zhang H, Zhang S. B7-H3, B7-H4, Foxp3 and IL-2 expression in cervical cancer: Associations with patient outcome and clinical significance. Oncol Rep. (2016) 35:2183–90. doi: 10.3892/or.2016.4607
47. Yang S, Liu Y, Li MY, Ng CSH, Yang SL, Wang S, et al. FOXP3 promotes tumor growth and metastasis by activating Wnt/β-catenin signaling pathway and EMT in non-small cell lung cancer. Mol cancer. (2017) 16:124. doi: 10.1186/s12943–017-0700–1
48. Song JJ, Zhao SJ, Fang J, Ma D, Liu XQ, Chen XB, et al. Foxp3 overexpression in tumor cells predicts poor survival in oral squamous cell carcinoma. BMC cancer. (2016) 16:530. doi: 10.1186/s12885–016-2419–6
49. Li X, Gao Y, Li J, Zhang K, Han J, Li W, et al. FOXP3 inhibits angiogenesis by downregulating VEGF in breast cancer. Cell Death disease. (2018) 9:744. doi: 10.1038/s41419–018-0790–8
50. Zhang C, Xu Y, Hao Q, Wang S, Li H, Li J, et al. FOXP3 suppresses breast cancer metastasis through downregulation of CD44. Int J cancer. (2015) 137:1279–90. doi: 10.1002/ijc.29482
51. Gao Y, Li X, Shu Z, Zhang K, Xue X, Li W, et al. Nuclear galectin-1-FOXP3 interaction dampens the tumor-suppressive properties of FOXP3 in breast cancer. Cell Death disease. (2018) 9:416. doi: 10.1038/s41419–018-0448–6
52. Kim JE, Shin JS, Moon JH, Hong SW, Jung DJ, Kim JH, et al. Foxp3 is a key downstream regulator of p53-mediated cellular senescence. Oncogene. (2017) 36:219–30. doi: 10.1038/onc.2016.193
53. Yang LP, Lin Q, Mu XL. MicroRNA-155 and FOXP3 jointly inhibit the migration and invasion of colorectal cancer cells by regulating ZEB2 expression. Eur Rev Med Pharmacol Sci. (2019) 23:6131–8. doi: 10.26355/eurrev_201907_18426
54. Zheng Y, Josefowicz S, Chaudhry A, Peng XP, Forbush K, Rudensky AY. Role of conserved non-coding DNA elements in the Foxp3 gene in regulatory T-cell fate. Nature. (2010) 463:808–12. doi: 10.1038/nature08750
55. Wakamatsu E, Omori H, Kawano A, Ogawa S, Abe R. Strong TCR stimulation promotes the stabilization of Foxp3 expression in regulatory T cells induced in vitro through increasing the demethylation of Foxp3 CNS2. Biochem Biophys Res Commun. (2018) 503:2597–602. doi: 10.1016/j.bbrc.2018.07.021
56. Wang CM, Yang WH, Liu R, Wang L, Yang WH. FOXP3 activates SUMO-conjugating UBC9 gene in MCF7 breast cancer cells. Int J Mol Sci. (2018) 19(7):2036. doi: 10.3390/ijms19072036
57. Yanli S, Fei C, Wu C. Hypoxia inducible factor-1 α The expression and clinical significance of Foxp3 in colorectal cancer tissue Chinese Experimental Diagnosis. Chin J Anal Lab. (2019) 23(05):770–3. doi: 10.3969/j.issn.1007-4287.2019.05.003
58. Clambey ET, McNamee EN, Westrich JA, Glover LE, Campbell EL, Jedlicka P, et al. Hypoxia-inducible factor-1 alpha-dependent induction of FoxP3 drives regulatory T-cell abundance and function during inflammatory hypoxia of the mucosa. Proc Natl Acad Sci USA. (2012) 109:E2784–93. doi: 10.1073/pnas.1202366109
59. Patwardhan RS, Singh B, Pal D, Checker R, Bandekar M, Sharma D, et al. Redox regulation of regulatory T-cell differentiation and functions. Free Radical Res. (2020) 54:947–60. doi: 10.1080/10715762.2020.1745202
60. Hsu TS, Lai MZ. Hypoxia-inducible factor 1α plays a predominantly negative role in regulatory T cell functions. J leukocyte Biol. (2018) 104:911–8. doi: 10.1002/JLB.MR1217-481R
61. Xu QR, Tang J, Liao HY, Yu BT, He XY, Zheng YZ, et al. Long non-coding RNA MEG3 mediates the miR-149–3p/FOXP3 axis by reducing p53 ubiquitination to exert a suppressive effect on regulatory T cell differentiation and immune escape in esophageal cancer. J Trans Med. (2021) 19:264. doi: 10.1186/s12967–021-02907–1
62. Wang C, Zhou M, Zhu P, Ju C, Sheng J, Du D, et al. IGF2BP2-induced circRUNX1 facilitates the growth and metastasis of esophageal squamous cell carcinoma through miR-449b-5p/FOXP3 axis. J Exp Clin Cancer Res: CR. (2022) 41:347. doi: 10.1186/s13046–022-02550–8
63. Xuejia L. Inhibition of FOXP3 expression on DCs activation and immune function in pancreatic cancer. J Nanjing Univ. (2015).
64. Ke B. The effects and clinical significance of PD-1, PDL-1, and Foxp3 proteins on pancreatic ductal adenocarcinoma. J Nanjing Univ. (2018).
65. Hinz S, Pagerols-Raluy L, Oberg HH, Ammerpohl O, Grüssel S, Sipos B, et al. Foxp3 expression in pancreatic carcinoma cells as a novel mechanism of immune evasion in cancer. Cancer Res. (2007) 67:8344–50. doi: 10.1158/0008–5472.Can-06–3304
66. Lang C, Wang J, Chen L. CD25-expressing Th17 cells mediate CD8(+) T cell suppression in CTLA-4 dependent mechanisms in pancreatic ductal adenocarcinoma. Exp Cell Res. (2017) 360:384–9. doi: 10.1016/j.yexcr.2017.09.030
67. Jinling J, Chenfei Z, Chao W, Liqin Z, Junwei W, Jun Z. New progress in research and diagnosis and treatment of gastric cancer in 2022. Chin J Cancer. (2023) 33:303–14. doi: 10.19401/j.cnki.1007–3639.2023.04.001
68. Zhang L, Xu J, Zhang X, Zhang Y, Wang L, Huang X, et al. The role of tumoral FOXP3 on cell proliferation, migration, and invasion in gastric cancer. Cell Physiol Biochem. (2017) 42:1739–54. doi: 10.1159/000479442
69. Ying L, Yan F, Meng Q, Yu L, Yuan X, Gantier MP, et al. PD-L1 expression is a prognostic factor in subgroups of gastric cancer patients stratified according to their levels of CD8 and FOXP3 immune markers. Oncoimmunology. (2018) 7:e1433520. doi: 10.1080/2162402x.2018.1433520
70. Xu Y, Wang D, Zhao G. Transcriptional activation of Proteasome 26S non-ATPase subunit 7 by forkhead box P3 participates in gastric cancer cell proliferation and apoptosis. Bioengineered. (2022) 13:2525–36. doi: 10.1080/21655979.2021.2018097
71. Fei Y, Cao Y, Gu Y, Fang H, Chen Y, Wang J, et al. Intratumoral Foxp3(+)RORγt(+) T cell infiltration determines poor prognosis and immunoevasive contexture in gastric cancer patients. Cancer Immunol Immunother: CII. (2022) 71:1–11. doi: 10.1007/s00262–021-02950–3
72. Hao Q, Li W, Zhang C, Qin X, Xue X, Li M, et al. TNFα induced FOXP3-NFκB interaction dampens the tumor suppressor role of FOXP3 in gastric cancer cells. Biochem Biophys Res Commun. (2013) 430:436–41. doi: 10.1016/j.bbrc.2012.11.039
73. Kim HK, Won KY, Han SA. The antioncogenic effect of Beclin-1 and FOXP3 is associated with SKP2 expression in gastric adenocarcinoma. Medicine. (2021) 100:e26951. doi: 10.1097/md.0000000000026951
74. Ma GF, Chen SY, Sun ZR, Miao Q, Liu YM, Zeng XQ, et al. FoxP3 inhibits proliferation and induces apoptosis of gastric cancer cells by activating the apoptotic signaling pathway. Biochem Biophys Res Commun. (2013) 430:804–9. doi: 10.1016/j.bbrc.2012.11.065
75. Hao Q, Zhang C, Gao Y, Wang S, Li J, Li M, et al. FOXP3 inhibits NF-κB activity and hence COX2 expression in gastric cancer cells. Cell signalling. (2014) 26:564–9. doi: 10.1016/j.cellsig.2013.11.030
76. Pan DY, Zeng XQ, Ma GF, Gao J, Li N, Miao Q, et al. Label-free quantitative proteomic analysis identifies CTNNB1 as a direct target of FOXP3 in gastric cancer cells. Oncol letters. (2018) 15:7655–60. doi: 10.3892/ol.2018.8277
77. Ziyi Z, Quanren L, Chunyu Y, Yan J. Research progress on stem cell markers and signaling pathways in colon cancer life sciences. China Bull Life Sci. (2019) 31(08):802–11. doi: 10.13376/j.cbls/2019098
78. Zhuo C, Li Z, Xu Y, Wang Y, Li Q, Peng J, et al. Higher FOXP3-TSDR demethylation rates in adjacent normal tissues in patients with colon cancer were associated with worse survival. Mol cancer. (2014) 13:153. doi: 10.1186/1476–4598-13–153
79. Wang Z, Zhang J. FOXP3 promotes colorectal carcinoma liver metastases by evaluating MMP9 expression via regulating S-adenosylmethionine metabolism. Ann Trans Med. (2020) 8:592. doi: 10.21037/atm-20–3287
80. Cui K, Yao S, Zhang H, Zhou M, Liu B, Cao Y, et al. Identification of an immune overdrive high-risk subpopulation with aberrant expression of FOXP3 and CTLA4 in colorectal cancer. Oncogene. (2021) 40:2130–45. doi: 10.1038/s41388-021-01677-w
81. Ay EN, Demirkol Ş, Hakan MT, Horozoğlu C, Arıkan S, Doğan MB, et al. Investigation of possible associations between tryptophan/kynurenine status and FOXP3 expression in colorectal cancer. Scandinavian J Clin Lab Invest. (2022) 82:185–91. doi: 10.1080/00365513.2022.2040050
82. Anz D, Mueller W, Golic M, Kunz WG, Rapp M, Koelzer VH, et al. CD103 is a hallmark of tumor-infiltrating regulatory T cells. Int J cancer. (2011) 129:2417–26. doi: 10.1002/ijc.25902
83. Osman A, Yan B, Li Y, Pavelko KD, Quandt J, Saadalla A, et al. TCF-1 controls T(reg) cell functions that regulate inflammation, CD8(+) T cell cytotoxicity and severity of colon cancer. Nat Immunol. (2021) 22:1152–62. doi: 10.1038/s41590–021-00987–1
84. Kim M, Grimmig T, Grimm M, Lazariotou M, Meier E, Rosenwald A, et al. Expression of Foxp3 in colorectal cancer but not in Treg cells correlates with disease progression in patients with colorectal cancer. PLoS One. (2013) 8:e53630. doi: 10.1371/journal.pone.0053630
85. Liu S, Zhang C, Zhang K, Gao Y, Wang Z, Li X, et al. FOXP3 inhibits cancer stem cell self-renewal via transcriptional repression of COX2 in colorectal cancer cells. Oncotarget. (2017) 8:44694–704. doi: 10.18632/oncotarget.17974
86. Qian Y, Yuan W. Analysis of the current situation and trend of liver cancer prevalence. Chin Oncol. (2020) 29:185–91. doi: 10.11735/j.issn.1004-0242.2020.03.A005
87. Lin ZW, Wu LX, Xie Y, Ou X, Tian PK, Liu XP, et al. The expression levels of transcription factors T-bet, GATA-3, RORγt and FOXP3 in peripheral blood lymphocyte (PBL) of patients with liver cancer and their significance. Int J Med Sci. (2015) 12:7–16. doi: 10.7150/ijms.8352
88. Huang Y, Wang F, Wang Y, Zhu Z, Gao Y, Ma Z, et al. Intrahepatic interleukin-17+ T cells and FoxP3+ regulatory T cells cooperate to promote development and affect the prognosis of hepatocellular carcinoma. J Gastroenterol hepatol. (2014) 29:851–9. doi: 10.1111/jgh.12418
89. He MK, Le Y, Zhang YF, Ouyang HY, Jian PE, Yu ZS, et al. Matrix metalloproteinase 12 expression is associated with tumor FOXP3(+) regulatory T cell infiltration and poor prognosis in hepatocellular carcinoma. Oncol letters. (2018) 16:475–82. doi: 10.3892/ol.2018.8642
90. Yang J, Zhang JX, Wang H, Wang GL, Hu QG, Zheng QC. Hepatocellular carcinoma and macrophage interaction induced tumor immunosuppression via Treg requires TLR4 signaling. World J Gastroenterol. (2012) 18:2938–47. doi: 10.3748/wjg.v18.i23.2938
91. Jiang R, Tang J, Chen Y, Deng L, Ji J, Xie Y, et al. The long noncoding RNA lnc-EGFR stimulates T-regulatory cells differentiation thus promoting hepatocellular carcinoma immune evasion. Nat Commun. (2017) 8:15129. doi: 10.1038/ncomms15129
92. Pan J, Hu Y, Yuan C, Wu Y, Zhu X. lncRNA NEAT1 promotes the proliferation and metastasis of hepatocellular carcinoma by regulating the FOXP3/PKM2 axis. Front Oncol. (2022) 12:928022. doi: 10.3389/fonc.2022.928022
93. Ren J, Liu Y, Wang S, Wang Y, Li W, Chen S, et al. The FKH domain in FOXP3 mRNA frequently contains mutations in hepatocellular carcinoma that influence the subcellular localization and functions of FOXP3. J Biol Chem. (2020) 295:5484–95. doi: 10.1074/jbc.RA120.012518
94. Liu S, Zhang H, Yan J, Zhu J, Bai Z, Li X. FOXP3 and SQSTM1/P62 correlate with prognosis and immune infiltration in hepatocellular carcinoma. Pathol Res practice. (2023) 242:154292. doi: 10.1016/j.prp.2022.154292
95. Liu Q, Du F, Huang W, Ding X, Wang Z, Yan F, et al. Epigenetic control of Foxp3 in intratumoral T-cells regulates growth of hepatocellular carcinoma. Aging. (2019) 11:2343–51. doi: 10.18632/aging.101918
96. Gong Z, Jia H, Yu J, Liu Y, Ren J, Yang S, et al. Nuclear FOXP3 inhibits tumor growth and induced apoptosis in hepatocellular carcinoma by targeting c-Myc. Oncogenesis. (2020) 9:97. doi: 10.1038/s41389-020-00283-x
97. Shi JY, Ma LJ, Zhang JW, Duan M, Ding ZB, Yang LX, et al. FOXP3 Is a HCC suppressor gene and Acts through regulating the TGF-β/Smad2/3 signaling pathway. BMC cancer. (2017) 17:648. doi: 10.1186/s12885–017-3633–6
98. Duan SF, Zhang MM, Dong Q, Yang B, Liu W, Zhang X, et al. A water-soluble hydrogen sulfide donor suppresses the growth of hepatocellular carcinoma via inhibiting the AKT/GSK-3β/β-catenin and TGF-β/smad2/3 signaling pathways. J Oncol. (2023) 2023:8456852. doi: 10.1155/2023/8456852
99. Barua S, Fang P, Sharma A, Fujimoto J, Wistuba I, Rao AUK, et al. Spatial interaction of tumor cells and regulatory T cells correlates with survival in non-small cell lung cancer. Lung Cancer. (2018) 117:73–9. doi: 10.1016/j.lungcan.2018.01.022
100. Kachler K, Holzinger C, Trufa DI, Sirbu H, Finotto S. The role of Foxp3 and Tbet co-expressing Treg cells in lung carcinoma. Oncoimmunology. (2018) 7(8):e1456612. doi: 10.1080/2162402x.2018.1456612
101. Li L, Xu XT, Wang LL, Qin SB, Zhou JY. Expression and clinicopathological significance of Foxp3 and VISTA in cervical cancer. Am J Transl Res. (2021) 13:10428–38. doi: 10.11817/j.issn.1672-7347.2018.08.003
102. Sarkar T, Dhar S, Chakraborty D, Pati S, Bose S, Panda AK, et al. FOXP3/HAT1 axis controls Treg infiltration in the tumor microenvironment by inducing CCR4 expression in breast cancer. Front Immunol. (2022) 13:740588. doi: 10.3389/fimmu.2022.740588
103. Li J, Zhang X, Liu B, Shi C, Ma X, Ren S, et al. The expression landscape of FOXP3 and its prognostic value in breast cancer. Ann Trans Med. (2022) 10:801–. doi: 10.21037/atm-22–3080
104. Kim S, Lee A, Lim W, Park S, Cho MS, Koo H, et al. Zonal difference and prognostic significance of Foxp3 regulatory T cell infiltration in breast cancer. J Breast Cancer. (2014) 17(1):8–17. doi: 10.4048/jbc.2014.17.1.8
105. Chen C, Chen D, Zhang Y, Chen Z, Zhu W, Zhang B, et al. Changes of CD4+CD25+FOXP3+ and CD8+CD28- regulatory T cells in non-small cell lung cancer patients undergoing surgery. Int immunopharmacol. (2014) 18:255–61. doi: 10.1016/j.intimp.2013.12.004
106. Romesser PB, Sherman EJ, Whiting K, Ho ML, Shaha AR, Sabra MM, et al. Intensity-modulated radiation therapy and doxorubicin in thyroid cancer: A prospective phase 2 trial. Cancer. (2021) 127:4161–70. doi: 10.1002/cncr.33804
107. McEachron J, Chen YJ, Zhou N, Kao J, Gorelick C, Kanis MJ, et al. Improved survival with combination chemotherapy and external beam radiation therapy in uterine carcinosarcoma. Int J Gynecol Cancer. (2022) 32:1402–9. doi: 10.1136/ijgc-2022–003717
108. Rui R, Zhou L, He S. Cancer immunotherapies: advances and bottlenecks. Front Immunol. (2023) 14:1212476. doi: 10.3389/fimmu.2023.1212476
109. Nishikawa H, Sakaguchi S. Regulatory T cells in cancer immunotherapy. Curr Opin Immunol. (2014) 27:1–7. doi: 10.1016/j.coi.2013.12.005
110. Wada M, Tsuchikawa T, Kyogoku N, Abiko T, Miyauchi K, Takeuchi S, et al. Clinical implications of CD4(+)CD25(+)Foxp3(+)Regulatory T cell frequencies after CHP-MAGE-A4 cancer vaccination. Anticancer Res. (2018) 38:1435–44. doi: 10.21873/anticanres.12368
111. Zhang Y, Zhang Z. The history and advances in cancer immunotherapy: understanding the characteristics of tumor-infiltrating immune cells and their therapeutic implications. Cell Mol Immunol. (2020) 17:807–21. doi: 10.1038/s41423–020-0488–6
112. Gong Z, Jia H, Xue L, Li D, Zeng X, Wei M, et al. The emerging role of transcription factor FOXP3 in thyroid cancer. Rev endocrine Metab Disord. (2022) 23:421–9. doi: 10.1007/s11154–021-09684–8
113. Hu L, Zhu M, Shen Y, Zhong Z, Wu B. The prognostic value of intratumoral and peritumoral tumor-infiltrating FoxP3+Treg cells in of pancreatic adenocarcinoma: a meta-analysis. World J Surg Oncol. (2021) 19:300. doi: 10.1186/s12957–021-02420–1
114. French JD, Weber ZJ, Fretwell DL, Said S, Klopper JP, Haugen BR. Tumor-associated lymphocytes and increased FoxP3+ regulatory T cell frequency correlate with more aggressive papillary thyroid cancer. J Clin Endocrinol Metab. (2010) 95:2325–33. doi: 10.1210/jc.2009–2564
115. Calais G, Alfonsi M, Bardet E, Sire C, Germain T, Bergerot P, et al. Randomized trial of radiation therapy versus concomitant chemotherapy and radiation therapy for advanced-stage oropharynx carcinoma. J Natl Cancer Institute. (1999) 91:2081–6. doi: 10.1093/jnci/91.24.2081
116. Huang J, Wang S, Jia Y, Zhang Y, Dai X, Li B. Targeting FOXP3 complex ensemble in drug discovery. Adv Protein Chem Struct Biol. (2020) 121:143–68. doi: 10.1016/bs.apcsb.2019.11.010
117. Shi C, Zhang Y, Yang H, Dong T, Chen Y, Xu Y, et al. Ultrasound-targeted microbubble destruction-mediated Foxp3 knockdown may suppress the tumor growth of HCC mice by relieving immunosuppressive Tregs function. Exp Ther Med. (2018) 15:31–8. doi: 10.3892/etm.2017.5421
118. Hawley KM, Eclov RJ, Schnorenberg MR, Tian Y, Shah RN, Thomas-Toth AT, et al. Inhibition of FOXP3 by stapled alpha-helical peptides dampens regulatory T cell function. Proc Natl Acad Sci USA. (2022) 119:e2209044119. doi: 10.1073/pnas.2209044119
119. Xiong Y, Wang L, Di Giorgio E, Akimova T, Beier UH, Han R, et al. Inhibiting the coregulator CoREST impairs Foxp3+ Treg function and promotes antitumor immunity. J Clin Invest. (2020) 130:1830–42. doi: 10.1172/jci131375
120. Yehong K, Heng Z, Wu Z, Lisa W, Wangqing C, Yan L, et al. The role of methotrexate in regulating the number of regulatory T cells and Foxp3 expression in the treatment of psoriasis. J Cent South Univ (Medical Edition). (2018) 43:835–42. doi: 10.11817/j.issn.1672-7347.2018.08.003
121. Wang J, Yang T, Xu J. Therapeutic development of immune checkpoint inhibitors. Adv Exp Med Biol. (2020) 1248:619–49. doi: 10.1007/978–981-15–3266-5_23
122. Okiyama N, Tanaka R. Immune-related adverse events in various organs caused by immune checkpoint inhibitors. Allergology Int. (2022) 71:169–78. doi: 10.1016/j.alit.2022.01.001
123. Deutsch A, Leboeuf NR, Lacouture ME, McLellan BN. Dermatologic adverse events of systemic anticancer therapies: cytotoxic chemotherapy, targeted therapy, and immunotherapy. Am Soc Clin Oncol Educ book Am Soc Clin Oncol Annu Meeting. (2020) 40:485–500. doi: 10.1200/edbk_289911
124. Belum VR, Benhuri B, Postow MA, Hellmann MD, Lesokhin AM, Segal NH, et al. Characterisation and management of dermatologic adverse events to agents targeting the PD-1 receptor. Eur J Cancer (Oxford England: 1990). (2016) 60:12–25. doi: 10.1016/j.ejca.2016.02.010
125. Sibaud V, Meyer N, Lamant L, Vigarios E, Mazieres J, Delord JP. Dermatologic complications of anti-PD-1/PD-L1 immune checkpoint antibodies. Curr Opin Oncol. (2016) 28:254–63. doi: 10.1097/cco.0000000000000290
126. Voudouri D, Nikolaou V, Laschos K, Charpidou A, Soupos N, Triantafyllopoulou I, et al. Anti-PD1/PDL1 induced psoriasis. Curr Probl Cancer. (2017) 41:407–12. doi: 10.1016/j.currproblcancer.2017.10.003
127. Cutroneo P, Ingrasciotta Y, Isgrò V, Rullo EV, Berretta M, Fiorica F, et al. Psoriasis and psoriasiform reactions secondary to immune checkpoint inhibitors. Dermatol Ther. (2021) 34:e14830. doi: 10.1111/dth.14830
128. Thuny F, Naidoo J, Neilan TG. Cardiovascular complications of immune checkpoint inhibitors for cancer. Eur Heart J. (2022) 43:4458–68. doi: 10.1093/eurheartj/ehac456
129. Zhang C, Wei F, Ma W, Zhang J. Immune-related cardiovascular toxicities of PD-1/PD-L1 inhibitors in solid tumors: an updated systematic review and meta-analysis. Front Immunol. (2024) 15:1255825. doi: 10.3389/fimmu.2024.1255825
130. Liu M, Cheng X, Ni R, Zheng B, Huang S, Yang J. Cardiotoxicity of immune checkpoint inhibitors: A frequency network meta-analysis. Front Immunol. (2022) 13:1006860. doi: 10.3389/fimmu.2022.1006860
131. Kelderman S, Schumacher TN, Haanen JB. Acquired and intrinsic resistance in cancer immunotherapy. Mol Oncol. (2014) 8:1132–9. doi: 10.1016/j.molonc.2014.07.011
132. Shan J, Han D, Shen C, Lei Q, Zhang Y. Mechanism and strategies of immunotherapy resistance in colorectal cancer. Front Immunol. (2022) 13:1016646. doi: 10.3389/fimmu.2022.1016646
133. Marei HE, Hasan A, Pozzoli G, Cenciarelli C. Cancer immunotherapy with immune checkpoint inhibitors (ICIs): potential, mechanisms of resistance, and strategies for reinvigorating T cell responsiveness when resistance is acquired. Cancer Cell Int. (2023) 23:64. doi: 10.1186/s12935–023-02902–0
134. Aldea M, Andre F, Marabelle A, Dogan S, Barlesi F, Soria JC. Overcoming resistance to tumor-targeted and immune-targeted therapies. Cancer Discov. (2021) 11:874–99. doi: 10.1158/2159–8290.Cd-20–1638
135. Lacouture M, Sibaud V. Toxic side effects of targeted therapies and immunotherapies affecting the skin, oral mucosa, hair, and nails. Am J Clin Dermatol. (2018) 19:31–9. doi: 10.1007/s40257–018-0384–3
136. Villa A, Kuten-Shorrer M. Pathogenesis of oral toxicities associated with targeted therapy and immunotherapy. Int J Mol Sci. (2023) 24(9):8188. doi: 10.3390/ijms24098188
137. Vigarios E, Epstein JB, Sibaud V. Oral mucosal changes induced by anticancer targeted therapies and immune checkpoint inhibitors. Support Care Cancer. (2017) 25:1713–39. doi: 10.1007/s00520–017-3629–4
138. Srivastava A, Al-Zubidi N, Appelbaum E, Gombos DS, Nader ME, Gidley PW, et al. Immune-related oral, otologic, and ocular adverse events. Adv Exp Med Biol. (2020) 1244:295–307. doi: 10.1007/978–3-030–41008-7_17
139. Wetzel ML, Rubin AI, Hanania H, Patel AB. Treatment recommendations for nail unit toxicities secondary to targeted cancer therapy based on collective experience and evidence-based literature review. J Am Acad Dermatol. (2022) 87:180–3. doi: 10.1016/j.jaad.2021.07.022
140. van Damme C, Sibaud V, André J, Richert B, Berlingin E. Anti-programmed cell death protein 1-induced lichenoid changes of the nail unit: Histopathologic description. JAAD Case Rep. (2021) 10:110–2. doi: 10.1016/j.jdcr.2021.02.016
141. Zen Y, Yeh MM. Hepatotoxicity of immune checkpoint inhibitors: a histology study of seven cases in comparison with autoimmune hepatitis and idiosyncratic drug-induced liver injury. Mod Pathol. (2018) 31:965–73. doi: 10.1038/s41379-018-0013-y
142. Yamamoto A, Yano Y, Ueda Y, Yasutomi E, Hatazawa Y, Hayashi H, et al. Clinical features of immune-mediated hepatotoxicity induced by immune checkpoint inhibitors in patients with cancers. J Cancer Res Clin Oncol. (2021) 147:1747–56. doi: 10.1007/s00432–020-03448–8
143. Personeni N, Pressiani T, D'Alessio A, Prete MG, Bozzarelli S, Terracciano L, et al. Hepatotoxicity in patients with hepatocellular carcinoma on treatment with immune checkpoint inhibitors. Cancers (Basel). (2021) 13(22):5665. doi: 10.3390/cancers13225665
144. Zheng C, Huang S, Lin M, Hong B, Ni R, Dai H, et al. Hepatotoxicity of immune checkpoint inhibitors: What is Currently Known. Hepatol Commun. (2023) 7:e0063. doi: 10.1097/hc9.0000000000000063
145. Bellone S, Roque DM, Siegel ER, Buza N, Hui P, Bonazzoli E, et al. A phase 2 evaluation of pembrolizumab for recurrent Lynch-like versus sporadic endometrial cancers with microsatellite instability. Cancer. (2022) 128:1206–18. doi: 10.1002/cncr.34025
146. Cano-Cruz LG, Barrera-Vargas A, Mateos-Soria A, Soto-Perez-de-Celis E, Merayo-Chalico J. Rheumatological adverse events of cancer therapy with immune checkpoint inhibitors. Arch Med Res. (2022) 53:113–21. doi: 10.1016/j.arcmed.2021.09.004
147. Zarbo A, Belum VR, Sibaud V, Oudard S, Postow MA, Hsieh JJ, et al. Immune-related alopecia (areata and universalis) in cancer patients receiving immune checkpoint inhibitors. Br J Dermatol. (2017) 176:1649–52. doi: 10.1111/bjd.15237
148. Antoury L, Maloney NJ, Bach DQ, Goh C, Cheng K. Alopecia areata as an immune-related adverse event of immune checkpoint inhibitors: A review. Dermatol Ther. (2020) 33:e14171. doi: 10.1111/dth.14171
149. De Martin E, Michot JM, Papouin B, Champiat S, Mateus C, Lambotte O, et al. Characterization of liver injury induced by cancer immunotherapy using immune checkpoint inhibitors. J hepatol. (2018) 68:1181–90. doi: 10.1016/j.jhep.2018.01.033
150. Brahmer JR, Abu-Sbeih H, Ascierto PA, Brufsky J, Cappelli LC, Cortazar FB, et al. Society for Immunotherapy of Cancer (SITC) clinical practice guideline on immune checkpoint inhibitor-related adverse events. J Immunother Cancer. (2021) 9(6):e002435. doi: 10.1136/jitc-2021–002435
151. Thompson JA, Schneider BJ, Brahmer J, Achufusi A, Armand P, Berkenstock MK, et al. Management of immunotherapy-related toxicities, version 1.2022, NCCN clinical practice guidelines in oncology. J Natl Compr Canc Netw. (2022) 20:387–405. doi: 10.6004/jnccn.2022.0020
152. Thompson JA, Schneider BJ, Brahmer J, Andrews S, Armand P, Bhatia S, et al. Management of immunotherapy-related toxicities, version 1.2019. J Natl Compr Canc Netw. (2019) 17:255–89. doi: 10.6004/jnccn.2019.0013
153. Yang M, Li J, Gu P, Fan X. The application of nanoparticles in cancer immunotherapy: Targeting tumor microenvironment. Bioactive Materials. (2021) 6:1973–87. doi: 10.1016/j.bioactmat.2020.12.010
154. Yan Y, Kumar AB, Finnes H, Markovic SN, Park S, Dronca RS, et al. Combining immune checkpoint inhibitors with conventional cancer therapy. Front Immunol. (2018) 9:1739. doi: 10.3389/fimmu.2018.01739
Keywords: Foxp3, FOXP3+ regulatory T cells, Foxp3 transcriptional and post-translational modifications, digestive system malignancies, immunotherapy targeting Foxp3+Treg
Citation: Wang W, Ding M, Wang Q, Song Y, Huo K, Chen X, Xiang Z and Liu L (2024) Advances in Foxp3+ regulatory T cells (Foxp3+ Treg) and key factors in digestive malignancies. Front. Immunol. 15:1404974. doi: 10.3389/fimmu.2024.1404974
Received: 22 March 2024; Accepted: 24 May 2024;
Published: 11 June 2024.
Edited by:
Xiong Li, Huazhong University of Science and Technology, ChinaReviewed by:
Sweta Ghosh, University of Louisville, United StatesCopyright © 2024 Wang, Ding, Wang, Song, Huo, Chen, Xiang and Liu. This is an open-access article distributed under the terms of the Creative Commons Attribution License (CC BY). The use, distribution or reproduction in other forums is permitted, provided the original author(s) and the copyright owner(s) are credited and that the original publication in this journal is cited, in accordance with accepted academic practice. No use, distribution or reproduction is permitted which does not comply with these terms.
*Correspondence: Lantao Liu, bGl1bGFudGFvQG1kam11LmVkdS5jbg==
Disclaimer: All claims expressed in this article are solely those of the authors and do not necessarily represent those of their affiliated organizations, or those of the publisher, the editors and the reviewers. Any product that may be evaluated in this article or claim that may be made by its manufacturer is not guaranteed or endorsed by the publisher.
Research integrity at Frontiers
Learn more about the work of our research integrity team to safeguard the quality of each article we publish.