- Department of Colorectal Surgery, Shanghai Changhai Hospital, Naval Medical University, Shanghai, China
Solid gastrointestinal tumors often respond poorly to immunotherapy for the complex tumor microenvironment (TME), which is exacerbated by immune system alterations. Immunosenescence is the process of increased diversification of immune genes due to aging and other factors, leading to a decrease in the recognition function of the immune system. This process involves immune organs, immune cells, and the senescence-associated secretory phenotype (SASP). The most fundamental change is DNA damage, resulting in TME remodeling. The main manifestations are worsening inflammation, increased immunosuppressive SASP production, decreased immune cell antitumor activity, and the accumulation of tumor-associated fibroblasts and myeloid-derived suppressor cells, making antitumor therapy less effective. Senotherapy strategies to remove senescent cells and block key senescence processes can have synergistic effects with other treatments. This review focuses on immunoenescence and its impact on the solid TME. We characterize the immunosenescent TME and discuss future directions for antitumor therapies targeting senescence.
Background
Health problems caused by population aging are among the great challenges the world is facing today. Nearly half of the global disease burden (92 diseases (including 35 cancers), accounting for 51.3%, 95% uncertainty interval, 48.5–53.9) is considered age-related (1). These include colorectal cancer, a solid tumor of the gastrointestinal tract with the third-highest incidence and second-highest mortality rate globally (2). Cancer morbidity and mortality rates are the highest in individuals over 50 years of age, suggesting that aging may play a significant role in cancer development and progression (3). Studies on the mechanisms of aging and tumor development have shown that some hallmarks of aging (including genomic instability, epigenetic alterations, chronic inflammation, and dysbiosis) promote oncogenesis and progression, whereas others have shown antagonistic (telomere attrition and stem cell exhaustion) or ambivalent effects (disabled macroautophagy and cellular senescence) on tumors (4). Therefore, the effects of aging on tumors need to be specifically explored at the systemic, microenvironmental, and cellular levels.
The immune system constitutes the body’s defensive barrier by monitoring, protecting, and eliminating threats (5). However, the interaction between adaptive and innate immune cells can lead to chronic inflammation and increase the likelihood of cancer development, and different types of infiltrating immune cells can have opposite effects on tumor prognosis (6). In addition, immune system function decreases with age, known as immunosenescence, which increases the risk of cancer and is a key player in cancer development (7). It was Roy Walford who first elucidated the link between immunity and aging and coined the term “immunosenescence,” which refers to increased immunogenetic diversification due to aging, leading to a progressive decrease in the recognition function of the immune system (8, 9). Immune aging is not simply a one-way process that leads to dysfunction and other harmful effects, but a dynamic balance between adaptation and maladaptation (10).
Changes in the immune senescence process will further complicate the immune features of the tumor microenvironment (TME) and may therefore have diverse impacts on tumor development and immunotherapy. The TME is composed of multiple types of immune cells, cancer-associated fibroblasts, endothelial cells, pericytes, various tissue-resident cell types, and extracellular matrix (ECM) (11). The complexity of the TME lies in the fact that immune cells are recruited to and infiltrate the TME through the action of cytokines and chemokines secreted from cancer cells to play an antitumor role, but simultaneously produce additional features of the TME that facilitate immunosuppression and limit antitumor immune responses (12–14). Particularly in colorectal cancer, slight alterations in the TME will trigger complex immunotherapy changes (15). Increasing evidence suggests that both innate and adaptive immune cells in the TME have a facilitative effect on tumor progression, while crosstalk with cancer cells enhances the recruitment of suppressive immune cells, including myeloid-derived suppressor cells (MDSCs) and tumor-associated macrophages (16–18). In addition, a decrease in antitumor immune cell infiltration and function, together with the accumulation of immunosuppressive cells and upregulation of ligands that bind to inhibitory receptors on immune cells, may contribute to immune escape and consequently lead to poor immunotherapy results (19, 20).
Although remarkable research advancements have been made for both immunosenescence and the TME in the past decades, the impact of their interaction on different constituents and tumor progression remains to be further explored. This review focuses on the process of immunosenescence and the role of TME regulation. In addition, we discuss the impact of immunosenescence on tumor progression and immunotherapy. Finally, we describe future directions for limiting tumor progression by intervening in immunosenescence.
The process of immunosenescence
The process of immune aging involves three distinct but interrelated components, i.e., the immune organs, immune cells, and circulating factors (chemokines, cytokines, and other soluble molecules), which change during aging and produce corresponding effects (Figure 1) (21). Immune system aging ultimately results in increased incidence of infectious diseases and mortality, reduced responsiveness to vaccines, accelerated aging of other organs, and increased risk of tumors (22–25) Immunosenescence is a complex and well-integrated process.
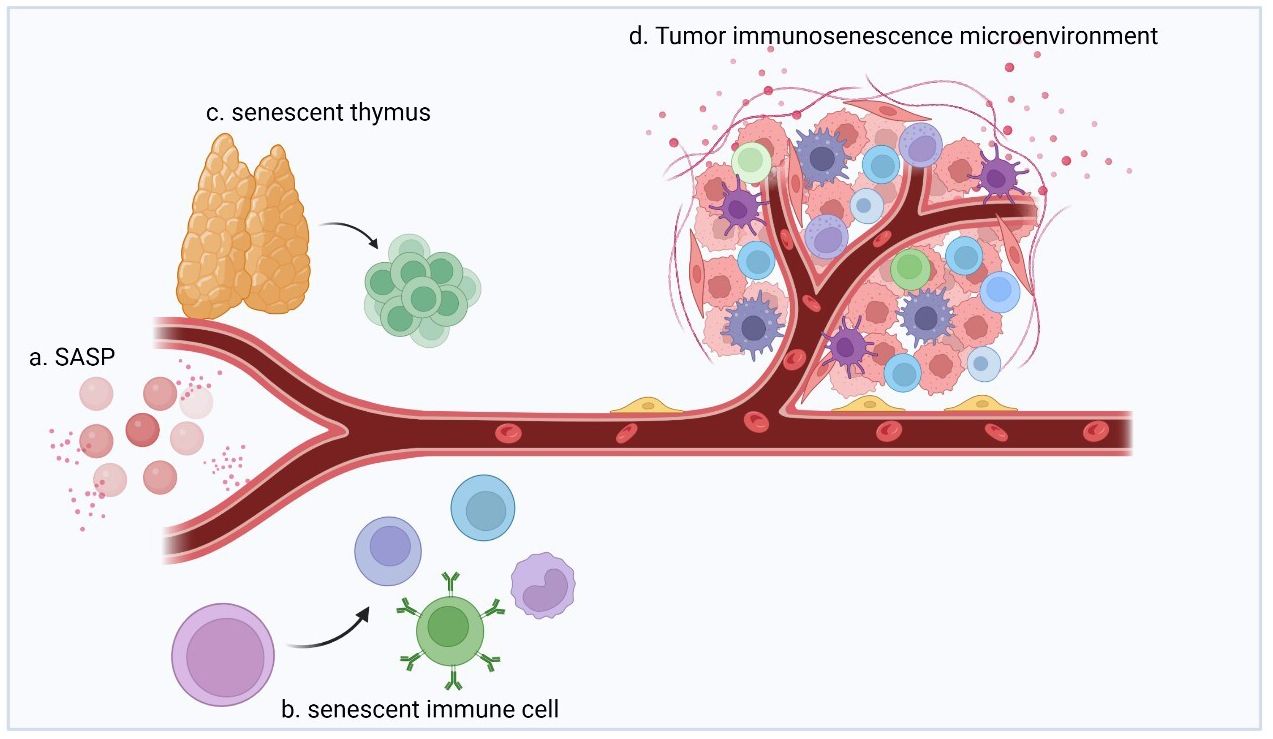
Figure 1 The immunosenescence process involves immune organs, immune cells, and circulating factors (chemokines, cytokines, and other soluble molecules) as three distinct but interrelated components that undergo changes during aging, with corresponding effects. (A) During aging, senescent cells secrete signaling and action molecules such as inflammatory, extracellular modifying, and growth factors, collectively known as SASP, which are key factors in facilitating and mediating immunosenescence. (B) Immune cells produced by senescent hematopoietic stem cells interact with SASPs and are characterized by increased numbers, increased secretion of inflammatory factors, decreased self-renewal capacity, diminished homing effects, and decreased energy metabolism. (C) During aging, thymus cells are gradually replaced by adipocytes, which results in a decrease in the proportion of undifferentiated T cells produced by the thymus (e.g., naïve T cells). (D) Multiple factors act together to shape the immunosenescent TME and exhibit strong immunosuppressive effects.
The aging of immune organs is the most noticeable change. For example, thymus function degenerates in nearly all species. Thymic involution begins in childhood and reaches its peak in adolescence. While excessive energy use is reduced in this process, age-related degeneration is detrimental to the organism (26, 27). During this process, thymus cells are gradually replaced by adipocytes, which results in a decrease in the proportion of undifferentiated T cells produced by the thymus (e.g., naïve T cells) and an increase in that of terminally differentiated cells (e.g., memory or depleted phenotypic T cells) (28). Such changes are also observed in neonates with early thyme resection, suggesting that they are a sign of immune deficiency (29). In conclusion, thymic degeneration is associated with the age-related immune decline and makes one prone to age-related diseases.
The key factors in promoting and mediating immunosenescence are alterations in circulating factors (chemokines, cytokines, and other soluble molecules). Immunosenescence causes the body to gradually enter an age-related pro-inflammatory state, while simultaneously, the body exerts anti-inflammatory effects through low-level, sterile chronic inflammation to adapt and remodel the immune system (30). During this process, senescent cells secrete inflammatory, extracellular modifying, and growth factors as signaling and acting molecules collectively referred to as the senescence-associated secretory phenotype (SASP) (31).
The SASP is expressed upon exposure to excessive stresses, such as repetitive cell division, oxidative stress, mitochondrial degradation, oncogene expression, and other stresses that cause DNA damage (Figure 2) (32). As an inflammatory response, the regulation of SASP is strongly associated with nuclear factor kappa-light-chain-enhancer of activated B cells (NF-κB) activation. As a classical DNA damage response pathway, the p38 MAPK pathway is activated by oxidative stress and DNA damage and regulates NF-κB through the p16INK4A, p53, and DNA damage checkpoint kinase CHK1/CHK2 mechanisms, which in turn produce the SASP (33–37). Another DNA damage response-related pathway, the ATM/ATR pathway, is thought to mediate NF-κB action via the key molecule GATA4 to produce the SASP (38). In addition, the downregulation of DNase (DNase2/TREX1) expression in senescent cells leads to the accumulation of DNA in the cytoplasm, which in turn leads to abnormal cGAS-STING pathway activation and SASP production through IFN-mediated NF-κB activation (39). Another pathway validated to produce SASP via NF activation is regulated by IL-1α, which phosphorylates IRAK1 via IRAK4 after binding to the IL-1 receptor and eventually activates NF-κB (40). Another signaling molecule that can regulate SASP is NOTCH1, which acts synergistically with NF-κB by activating the NOTCH-JAG1 pathway to produce TGF-β to induce aging while inhibiting C/EBPβ (41). In recent years, JAK/STAT pathway activation by signaling molecules including phospholipase A2 receptor 1 (PLA2R1), tumor necrosis factor (TNF)-α, and interferon (IFN)-γ has been shown to also induce SASP production (42, 43). The SASP generated through multiple pathways will profoundly impact immune cell function and ultimately restructure the TME.
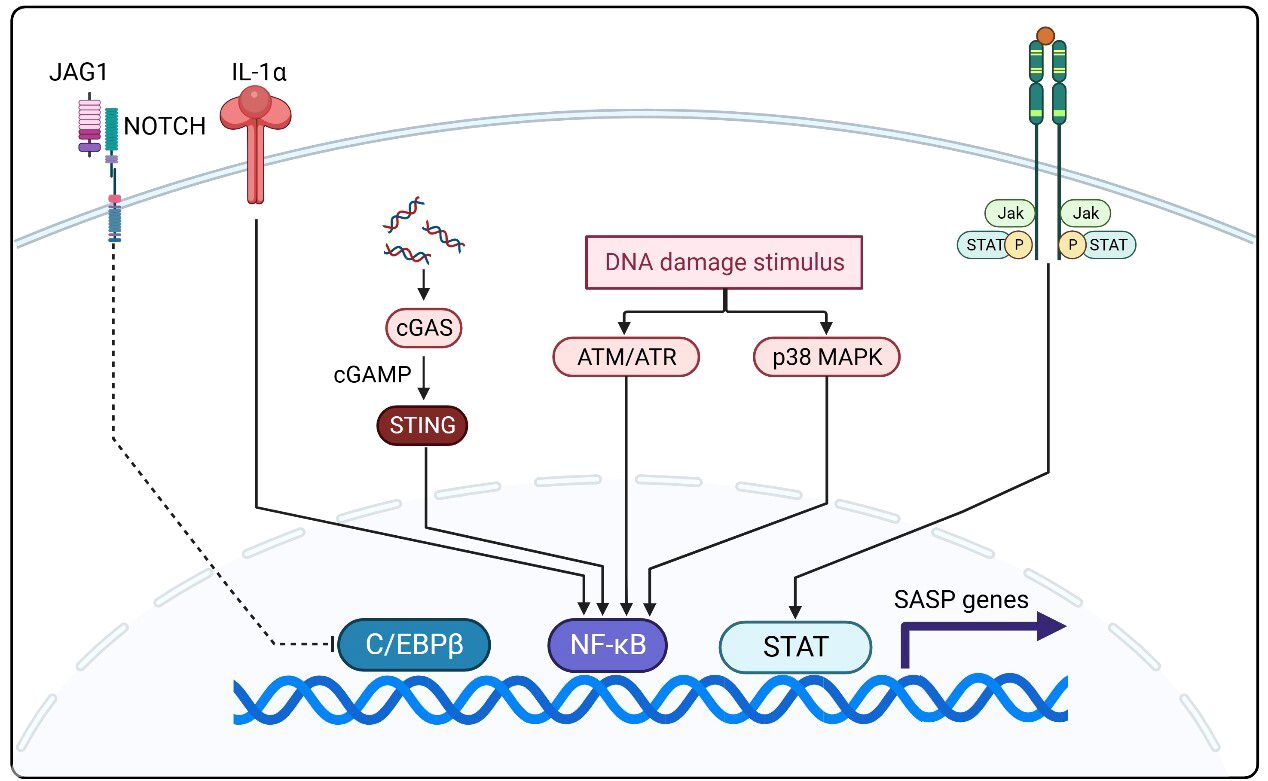
Figure 2 NF-κB activation is closely related to SASP regulation and activation. The p38 MAPK, ATM/ATR, and cGAS-STING pathways and aberrant IL-1α activation mediate SASP production by NF-κB. The NOTCH-JAG1 pathway can synergize with NF-κB to activate SASP production by inhibiting C/EBPβ. In recent years, JAK/STAT pathway activation by signaling molecules including phospholipase A2 receptor 1 (PLA2R1), TNF-production by iN-γ has been shown to also induce SASP production.
Alterations in immune cells are the most complex part of immune aging and produce direct effects. Such alterations are mainly due to two aspects: on the one hand, as mentioned above, the SASP plays a regulatory role in immune cell senescence, and on the other hand, hematopoietic stem cell (HSC) senescence is considered to be the basis of immunosenescence (44). Inflammation is a major factor in HSC aging, as inflammatory factors such as IL-1, IFNα/γ, and TNF-α drive HSC aging (45–47). Aging HSCs and immune cells differentiated from HSCs are increased in numbers and show increased inflammatory factor secretion, reduced self-renewal capacity, diminished homing effects, and reduced energy metabolism (44). Aging immune cells interact with soluble factors, including the SASP, in the TME to influence tumor progression and therapeutic efficacy, reflecting the impact of immunosenescence on cancer.
Impact of immunosenescence on TME alterations and tumor progression
The TME consists of various components that can be classified into a non-cancerous cellular fraction, including fibroblasts, neurons, adipocytes, and immune cells (adaptive and innate), and a non-cellular fraction, including ECM, chemokines, growth factors, cytokines, and vesicles (48). According to the characteristics of each component, the TME can also be subdivided into tumor immune microenvironment, tumor biophysical microenvironment, tumor microbe microenvironment, etc. (49–51) We propose the term “immunosenescence microenvironment” as a new TME component to reflect the impact of senescent immune-related cells and signaling molecules in the TME on tumor development (Table 1). By analyzing the individual components of the immunosenescent TME, we can more clearly the delineate the role of immunosenescence on tumor development.
SASP
A large number of SASP signaling molecules originate from the ECM, which plays a microenvironmental regulatory role in the immunosenescent TME, and these molecules determine the overall state of the TME (52). The SASP, derived from senescent cells, plays an important regulatory role in antitumor immunity in the ECM. Its effects are generally mediated by the paracrine way and have both positive and negative effects on tumor progression (53).
Various factors of the interleukin (IL) family involved in the SASP, such as IL-6, IL-8, and IL1α/β, function in microenvironment regulation. The IL-6/JAK/STAT3 signaling pathway drives tumor cell proliferation, invasion and metastasis and suppresses anti-tumor immune responses by reducing tumor antigen expression and decreasing responses to genotoxicity (54–57, 103). IL-6 as well as IL-8 can enhance tumor metastasis by promoting neoangiogenesis (58–61). IL-1β mediates immunosuppression by NLRP3 by inducing the expansion of MDSCs, leading to a decrease in the activity of natural killer (NK) cells and CD8+ T cells and an increase in the number of inhibitory antitumor immune cells, such as regulatory T (Treg) cells and M2 macrophages, in the TME (62, 63). Similarly, IL-1α/β secreted by tumor cells also induces fibroblasts to release pro-tumorigenic chemokines including CXCL9 and CXCL10 (65).
Chemokines involved in the SASP are another important type of regulatory molecules in the TME. CCL5, CXCL1, CXCL2, CXCL5, and CXCL12 are chemokines produced by senescent cells that have opposite effects on tumor development (66). Chemokines such as CCL5 recruit antitumor immune cells to enhance antitumor immunity while recruiting immunosuppressive lymphocytes such as Treg cells, leading to tumor immune escape (64, 67, 68). On the contrary, CXCL5, CXCL1 and CXCL2 have a tumor-promoting effect because they recruit MDSCs, which can play an immunosuppressive role by suppressing the immune function of lymphocytes through the secretion of Arg-1 and iNOS, and CXCL1 and CXCL2 also reduce the number of CD8+ T cells (69–72, 75, 76). CXCL12 attenuates T-cell infiltration and tumor cell-killing ability and increases tumor angiogenesis and immune resistance via CXCR4/CXCL12 (73, 74, 77).
Other important modulations of tumor progression by the SASP include TNF-α-mediated cell death, vascular endothelial growth factor-promoted tumor angiogenesis, and granulocyte macrophage colony-stimulating factor-induced immune-cell depletion, which inhibits antitumor immunity and promotes tumor progression (78, 79, 104).
T cells
Tumor cells and Treg cells are thought to induce T-cell senescence directly, and some senescent cells secrete SASPs that may have a consistent effect. Tumor-derived cyclic adenosine monophosphate can cause DNA damage and senescence in both CD4+ T cells and CD8+ T cells and immunoglobulin-like transcript 4 and its derivative PIR-B induce T cell senescence by increasing the fatty acid synthesis and lipid accumulation in tumor cells via MAPK ERK1/2 signaling (80). In CD4+ T cells, AMPK can trigger p38 phosphorylation via the scaffolding protein TAB1, which in turn activates the MAPK signaling pathway to induce senescence (81). While in CD8+ T cells, activation of the p38 MAPK pathway leads to the secretion of SASP (82).
Treg cells also play an essential role in inducing T-cell senescence. Treg cells have a selective metabolic profile that accelerates glucose depletion compared to effector T cells and suppresses responding T cells and induces senescence through cross-talk (83). This is because metabolic competition controls DNA damage in effector T cells through ERK1/2 and p38 signaling in cooperation with STAT1 and STAT3, leading to senescence and functional changes that are molecularly distinct from energy and exhaustion (105). Some genotoxins from pathogenic bacteria can also induce CD4+ T-cell senescence through DNA damage, suggesting that the gastrointestinal microbiota may complicate the tumor immunosenescence microenvironment (84).
CD8+ T cells are key immune cells that exert tumor-cell killing; therefore, their senescence significantly affects antitumor capacity. Changes in surface costimulatory molecules such as CD27, CD28, and CD57 reduce the tumor-associated antigen recognition ability of CD8+ T cells, resulting in decreased antitumor activity of CD8+ T cells (85, 86, 106). Further, decreased perforin production by senescent CD8+ T cells reduces cytolysis and decreases their tumor cell-killing function (107). However, a recent study came to the opposite conclusion, suggesting that the effect of aging on the ability of CD8+ T cells to kill tumor cells needs to be further explored (108). Research on T-cell senescence is limited, but some hallmarks of T-cell senescence have been identified (109). The DNA damage produced during the process is mainly regulated by the MAPK and STAT pathways (81, 87, 110). TNFα and proteases are the main SASP components secreted by senescent T cells (82). Changes in T-cell surface proteins, including the downregulation of CD27 and CD28 and the upregulation of CD57, are one of the hallmarks (88, 89). Further, senescent T cells enter cell-cycle arrest after T-cell receptor stimulation (83, 90, 91, 111).
B cells
B cells in TME can produce antibodies that bind to tumor-associated antigens and exert antitumor effects of antigen presentation (92). Their senescence arises predominantly from a decrease in B-cell differentiation and maturation in the bone marrow due to HSC senescence, as well as the reorganization of peripheral B-cell subsets (112, 113).
The impact of senescent B cells on TME is reflected not only in a decrease in antigen-presenting capacity but also in the pro-inflammatory effects derived from a class of B cells known as “age-associated B cells”, which are thought to be associated with TNFα secretion (93, 94, 114). IgD CD27 double-negative B cells, which play an immunosuppressive role, are another type of B cell that expands in aging populations (95). These cells are more likely to aggregate in areas of chronic inflammation due to surface expression of CCR6 and CCR7 after senescence and exacerbate the inflammatory microenvironment through pro-inflammatory factor production, worsening the immunosuppressive function of the TME (96, 97).
NK cells
NK cells are a key innate immune component of the TME that exerts antitumor immunity. These cells are more environmentally sensitive, as evidenced by the fact that passive transfer to environments of different age states can have a significant effect on cytotoxicity (115).
In senescent NK cells, the proportion of CD56dim increases, whereas that of CD56bright declines, which in turn leads to diminished immunocyte function (116). Senescent NK cells appear to enter a silent phase, as cytotoxicity after senescence is attenuated by reduced perforin production and decreased degranulation, and even the production of cytokines such as IFN-γ, MIP-1α, and IL-8 after stimulation is lower than that in nonsenescent NK cells (117–120).
Other cells
Dendritic cells are important antigen-presenting cells that play an important coordinating role in the immune response (121). However, as a result of immune senescence, the endocytosis and presentation of antigens by dendritic cells are diminished, while more pro-inflammatory cytokines are secreted (98). In addition, the ability of dendritic cells to activate T cells is reduced by senescence (99, 100).
MDSCs are a class of immunosuppressive cells recruited by the chronic inflammatory tumor immunosenescence microenvironment (101). MDSCs can inhibit the anti-tumor function of T cells and NK cells by expressing immune checkpoint molecules such as PD-L1 (102, 122, 123). In addition, MDSCs can affect the normal amino acid metabolism of T cells by depriving them of cysteine. This process affects the utilization of tryptophan by T cells and produces the immunosuppressive metabolite l-kynurenine, which ultimately induces T cell loss of function and promotes Treg cell differentiation (124, 125).
MDSCs drive immunosenescence and structure the immunosuppressive microenvironment, which are correlated with their multiple immunosuppressive functions (126). Upon the activation of MDSC amplification due to chronic inflammation caused by tumors and aging, certain chemokines, such as CCL2, CXCL1, and CXCR2, can recruit them to the TME, and this process can be enhanced by the complex gastrointestinal bacterial environment (127–132). Inflammatory molecules in the TME activate the immunosuppressive function of MDSCs mainly via JAK-STAT and NF-κB signaling, causing MDSCs to produce inflammatory molecules such as IL-10 and TGF-β that have antigen presentation-inhibitory or immunosuppressive effects (101, 133, 134). In addition, the immunosuppressive effects of MDSCs are reflected in the enhanced oxidative stress state in the microenvironment via the active generation of reactive oxygen species and the inhibition of immune checkpoint protein-mediated contact between T cells and tumor cells (135–137).
Other cells such as macrophages and neutrophils also exhibit immunosuppressive effects in the immunosenescent TMEby exacerbating chronic inflammation and increasing pro-tumorigenic M2-type macrophages (138). These molecular and cellular changes in the TME in the context of immune senescence promote tumor progression to a certain extent, and more importantly, influence antitumor therapy.
Immunosenescence and tumor therapy
The relationship between immunosenescence and antitumor therapy reflects the fact that there always are two sides to the same coin. On the one hand, for gastrointestinal solid tumors such as colorectal tumors, radiotherapy, chemotherapy, and immunotherapy are important antitumor treatments besides surgery; however, these treatments induce immune senescence, termed “treatment-induced immune senescence.”
Radiotherapy and chemotherapy can cause cancer-associated fibroblasts to expand in the TME and exacerbate the inflammatory state, leading to immune senescence (139). In addition, radiotherapy and chemotherapy can accelerate cellular senescence by directly causing DNA damage (140–142). Immunotherapies such as immune checkpoint inhibitors can also induce senescence in TME components by inducing increased production of senescence-related cytokines (143).
Senotherapy strategies targeting immunosenescence
On the other hand, senescence can be exploited as a new target in antitumor therapies (Table 2) (144, 145). This is explained by the fact that senescent cells continue to secrete SASP and lead to the presence of a pro-tumoral tumor microenvironment. Thus, senotherapy refers to the rational use of treatments that target senescent cells to fight tumors (146).
One senotherapeutic strategy is the removal of senescent cells by using anti-aging cell drugs that complement other antitumor therapies by mitigating the negative effects of treatment-induced senescence. Some drugs are used after senescence-inducing cancer therapies to target senescent tumor cells for clearance (147). BCL family inhibitors (e.g., ABT-737 and ABT-263) are representative of this class of drugs; they scavenge senescent cells by inhibiting the anti-apoptotic BCL protein family (148, 149). The tyrosine kinase inhibitor dasatinib combined with quercetin selectively kills senescent cells by inhibiting the pro-survival network that is upregulated in senescent cells (150). In addition, there are immunotherapeutic drugs and antibody-drug combinations that can similarly remove senescent cells and synergize with senescence-inducing antitumor treatments (151, 152). Engineered CAR-T cells targeting urokinase-type plasminogen activator receptor, a characteristic protein on the surface of senescent cells, can be used as a therapeutic modality to remove senescent cancer cells (153). Another senotherapeutic strategy is to induce senescence of tumor cells and then target them for elimination. The key to this strategy is to find corresponding drugs that can be targeted to induce tumor-cell senescence. For example, the DNA replication kinase CDC7 can selectively induce senescence in TP53-mutated hepatocellular carcinoma cells, which can subsequently be killed by mTOR signaling inhibitors (154). Mitigating the negative effects of the SASP is another senotherapeutic strategy. This approach is mainly based on the inhibition of SASP-producing pathways, such as the above-mentioned p38 MAPK, NF-κB, and JAK/STAT pathways (155, 156).
Other therapeutic approaches, such as the use of engineered tumor-targeting TCR-T cells or of photochemotherapy to increase immune cell infiltration, have been successful in countering the immunosenescent TME by enhancing antitumor immune efficacy (157, 158).These diverse therapeutic approaches combined can exert a more significant antitumor effect by targeting the immunosenescent environment from different angles.
Emerging biomarkers of immunosenescence in gastrointestinal tumors
Although the molecular biology of immunosenescence has been explored and therapeutic strategies for tumors have been optimized based on its action mechanisms, the timely identification of immunosenescent phenotypes is more clinically relevant, which provides an opportunity for early intervention (159, 160).
Since aging occurs in the immune system, accordingly, the type of biomarker that most readily comes to mind is the senescent phenotype of immune cells. Of these, both CD8+ TEMRA (CD45RA+CCR7-CD28-CD27-) and CD4+ TEMRA are markers of immunosenescence, with increased proportions and absolute numbers in colorectal cancer patients, reflecting low value-added potential and anti-apoptotic properties (161, 162). However, these markers are cell surface receptors and must be assayed using flow cytometry, which requires fresh blood samples. In contrast, the soluble form of immunosenescence markers are more stable and can be measured from stored serum, making them promising candidates as soluble markers of immunosenescence. However, these markers are less specific, as they can also be detected during acute inflammation. The soluble markers sCD163, sCD28 and sCTLA-4 have great potential for application as biomarkers of immunosenescence (163–165). These soluble markers can be detected using ELISA methods, but further studies are needed to compare the diagnostic performance of these markers with the gold standard (cell surface receptor) assay. In addition, some mutations in genes associated with immunosenescence such as PIK3CA, TP53, NF-κB, AMPK, mTOR, and P53 may also serve as biomarkers of the aging process (166–169).
Conclusions
Immunosenescence, as a feature of this stage, increases the risk of infectious diseases and tumors while decreasing antitumor immune functions. This is because immune senescence results in changes in immune organs, immune cells, and the SASP, which all interact with each other. Such changes significantly impact solid tumors of the gastrointestinal tract, such as colorectal cancer, which have a complex TME, and ultimately lead to the formation of an immunosuppressive tumor immunosenescence microenvironment. In such an environment, immunosuppression is manifested in multiple aspects, including immunosuppressive cell recruitment, increased secretion of inhibitory cytokines, and diminished antitumor immune cell function. These changes allow the tumor to develop and deteriorate, and increase its tendency to invade, and affect antitumor therapy, which in itself induces immune senescence and induces immunosuppressive changes. Therefore, senotherapy, a new therapy targeting immune senescence, has been developed on the basis of various antitumor therapies to remove senescent tumor cells and restore the antitumor capacity of immune cells from a different perspective. However, more in-depth studies on the tumor immune senescence microenvironment need to be conducted to paint a more complete picture of immunosuppression and explore the mechanisms by which immunosenescence attenuates antitumor immunity. This will enable the development of antitumor drugs and different therapeutic strategies for aging characteristics. However, the therapeutic effects remain to be verified in long-term experiments.
Author contributions
TZ: Conceptualization, Investigation, Writing – original draft. RW: Conceptualization, Investigation, Methodology, Writing – original draft. HF: Conceptualization, Investigation, Writing – original draft. YY: Conceptualization, Formal Analysis, Writing – original draft. HJ: Data curation, Writing – original draft. ZP: Data curation, Writing – original draft. LZ: Investigation, Supervision, Writing – review & editing. GY: Funding acquisition, Supervision, Writing – review & editing. WZ: Funding acquisition, Project administration, Supervision, Writing – review & editing.
Funding
The author(s) declare financial support was received for the research, authorship, and/or publication of this article. National Natural Science Foundation of China (82072750, 82203137), Shanghai Sailing Program (21YF1459300), Shanghai Municipal Health Commission Health Industry Clinical Research Project (20224Y0348).
Conflict of interest
The authors declare that the research was conducted in the absence of any commercial or financial relationships that could be construed as a potential conflict of interest.
Publisher’s note
All claims expressed in this article are solely those of the authors and do not necessarily represent those of their affiliated organizations, or those of the publisher, the editors and the reviewers. Any product that may be evaluated in this article, or claim that may be made by its manufacturer, is not guaranteed or endorsed by the publisher.
References
1. Chang AY, Skirbekk VF, Tyrovolas S, Kassebaum NJ, Dieleman JL. Measuring population ageing: an analysis of the Global Burden of Disease Study 2017. Lancet Public Health. (2019) 4:e159–67. doi: 10.1016/S2468-2667(19)30019-2
2. Sung H, Ferlay J, Siegel RL, Laversanne M, Soerjomataram I, Jemal A, et al. Global cancer statistics 2020: GLOBOCAN estimates of incidence and mortality worldwide for 36 cancers in 185 countries. CA Cancer J Clin. (2021) 71:209–49. doi: 10.3322/caac.21660
3. Lin L, Li Z, Yan L, Liu Y, Yang H, Li H. Global, regional, and national cancer incidence and death for 29 cancer groups in 2019 and trends analysis of the global cancer burden, 1990-2019. J Hematol Oncol. (2021) 14:197. doi: 10.1186/s13045-021-01213-z
4. López-Otín C, Pietrocola F, Roiz-Valle D, Galluzzi L, Kroemer G. Meta-hallmarks of aging and cancer. Cell Metab. (2023) 35:12–35. doi: 10.1016/j.cmet.2022.11.001
5. Parkin J, Cohen B. An overview of the immune system. Lancet. (2001) 357:1777–89. doi: 10.1016/S0140-6736(00)04904-7
6. de Visser KE, Eichten A, Coussens LM. Paradoxical roles of the immune system during cancer development. Nat Rev Cancer. (2006) 6:24–37. doi: 10.1038/nrc1782
7. Lian J, Yue Y, Yu W, Zhang Y. Immunosenescence: a key player in cancer development. J Hematol Oncol. (2020) 13:151. doi: 10.1186/s13045-020-00986-z
8. RL WALFORD. THE IMMUNOLOGIC THEORY OF AGING. Gerontologist. (1964) 4:195–7. doi: 10.1093/geront/4.4.195
9. Effros RB. Roy Walford and the immunologic theory of aging. Immun Ageing. (2005) 2:7. doi: 10.1186/1742-4933-2-7
10. Fulop T, Larbi A, Hirokawa K, Cohen AA, Witkowski JM. Immunosenescence is both functional/adaptive and dysfunctional/maladaptive. Semin Immunopathol. (2020) 42:521–36. doi: 10.1007/s00281-020-00818-9
11. Chen J, Zhu H, Yin Y, Jia S, Luo X. Colorectal cancer: Metabolic interactions reshape the tumor microenvironment. Biochim Biophys Acta Rev Cancer. (2022) 1877:188797. doi: 10.1016/j.bbcan.2022.188797
12. DePeaux K, Delgoffe GM. Metabolic barriers to cancer immunotherapy. Nat Rev Immunol. (2021) 21:785–97. doi: 10.1038/s41577-021-00541-y
13. Weulersse M, Asrir A, Pichler AC, Lemaitre L, Braun M, Carrié N, et al. Eomes-dependent loss of the co-activating receptor CD226 restrains CD8(+) T cell anti-tumor functions and limits the efficacy of cancer immunotherapy. Immunity. (2020) 53:824–39.e10. doi: 10.1016/j.immuni.2020.09.006
14. Vignali P, DePeaux K, Watson MJ, Ye C, Ford BR, Lontos K, et al. Hypoxia drives CD39-dependent suppressor function in exhausted T cells to limit antitumor immunity. Nat Immunol. (2023) 24:267–79. doi: 10.1038/s41590-022-01379-9
15. Ganesh K. Optimizing immunotherapy for colorectal cancer. Nat Rev Gastroenterol Hepatol. (2022) 19:93–4. doi: 10.1038/s41575-021-00569-4
16. Hinshaw DC, Shevde LA. The tumor microenvironment innately modulates cancer progression. Cancer Res. (2019) 79:4557–66. doi: 10.1158/0008-5472.CAN-18-3962
17. Jou E, Rodriguez-Rodriguez N, Ferreira AF, Jolin HE, Clark PA, Sawmynaden K, et al. An innate IL-25-ILC2-MDSC axis creates a cancer-permissive microenvironment for Apc mutation-driven intestinal tumorigenesis. Sci Immunol. (2022) 7:eabn0175. doi: 10.1126/sciimmunol.abn0175
18. Huang C, Wang X, Wang Y, Feng Y, Wang X, Chen S, et al. Sirpα on tumor-associated myeloid cells restrains antitumor immunity in colorectal cancer independent of its interaction with CD47. Nat Cancer. (2024) 5:500–16. doi: 10.1038/s43018-023-00691-z
19. DeBerardinis RJ. Tumor microenvironment, metabolism, and immunotherapy. N Engl J Med. (2020) 382:869–71. doi: 10.1056/NEJMcibr1914890
20. Yang L, Li A, Lei Q, Zhang Y. Tumor-intrinsic signaling pathways: key roles in the regulation of the immunosuppressive tumor microenvironment. J Hematol Oncol. (2019) 12:125. doi: 10.1186/s13045-019-0804-8
21. Nikolich-Žugich J. The twilight of immunity: emerging concepts in aging of the immune system. Nat Immunol. (2018) 19:10–9. doi: 10.1038/s41590-017-0006-x
22. Yousefzadeh MJ, Flores RR, Zhu Y, Schmiechen ZC, Brooks RW, Trussoni CE, et al. An aged immune system drives senescence and ageing of solid organs. Nature. (2021) 594:100–5. doi: 10.1038/s41586-021-03547-7
23. Müller L, Andrée M, Moskorz W, Drexler I, Walotka L, Grothmann R, et al. Age-dependent immune response to the biontech/pfizer BNT162b2 coronavirus disease 2019 vaccination. Clin Infect Dis. (2021) 73:2065–72. doi: 10.1093/cid/ciab381
24. Akbar AN, Gilroy DW. Aging immunity may exacerbate COVID-19. Science. (2020) 369:256–7. doi: 10.1126/science.abb0762
25. Bottazzi B, Riboli E, Mantovani A. Aging, inflammation and cancer. Semin Immunol. (2018) 40:74–82. doi: 10.1016/j.smim.2018.10.011
26. Elyahu Y, Monsonego A. Thymus involution sets the clock of the aging T-cell landscape: Implications for declined immunity and tissue repair. Ageing Res Rev. (2021) 65:101231. doi: 10.1016/j.arr.2020.101231
27. Xu L, Wei C, Chen Y, Wu Y, Shou X, Chen W, et al. IL-33 induces thymic involution-associated naive T cell aging and impairs host control of severe infection. Nat Commun. (2022) 13:6881. doi: 10.1038/s41467-022-34660-4
28. Park JE, Botting RA, Domínguez Conde C, Popescu DM, Lavaert M, Kunz DJ, et al. A cell atlas of human thymic development defines T cell repertoire formation. Science. (2020) 367:eaay3224. doi: 10.1126/science.aay3224
29. Deya-Martinez A, Flinn AM, Gennery AR. Neonatal thymectomy in children-accelerating the immunologic clock. J Allergy Clin Immunol. (2020) 146:236–43. doi: 10.1016/j.jaci.2020.02.028
30. Santoro A, Bientinesi E, Monti D. Immunosenescence and inflammaging in the aging process: age-related diseases or longevity. Ageing Res Rev. (2021) 71:101422. doi: 10.1016/j.arr.2021.101422
31. Faget DV, Ren Q, Stewart SA. Unmasking senescence: context-dependent effects of SASP in cancer. Nat Rev Cancer. (2019) 19:439–53. doi: 10.1038/s41568-019-0156-2
32. Coppé JP, Desprez PY, Krtolica A, Campisi J. The senescence-associated secretory phenotype: the dark side of tumor suppression. Annu Rev Pathol. (2010) 5:99–118. doi: 10.1146/annurev-pathol-121808-102144
33. Xu Y, Li N, Xiang R, Sun P. Emerging roles of the p38 MAPK and PI3K/AKT/mTOR pathways in oncogene-induced senescence. Trends Biochem Sci. (2014) 39:268–76. doi: 10.1016/j.tibs.2014.04.004
34. Freund A, Patil CK, Campisi J. p38MAPK is a novel DNA damage response-independent regulator of the senescence-associated secretory phenotype. EMBO J. (2011) 30:1536–48. doi: 10.1038/emboj.2011.69
35. Xinxing Z, Beijia H, Fengting Z, Jie L, Lixiang H, Yangxia Z, et al. p38-mediated FOXN3 phosphorylation modulates lung inflammation and injury through the NF-κB signaling pathway. Nucleic Acids Res. (2023) 51:2195–214. doi: 10.1093/nar/gkad057
36. Hao M, Ding C, Peng X, Chen H, Dong L, Zhang Y, et al. Ginseng under forest exerts stronger anti-aging effects compared to garden ginseng probably via regulating PI3K/AKT/mTOR pathway, SIRT1/NF-κB pathway and intestinal flora. Phytomedicine. (2022) 105:154365. doi: 10.1016/j.phymed.2022.154365
37. Sayegh S, Fantecelle CH, Laphanuwat P, Subramanian P, Rustin M, Gomes D, et al. Vitamin D(3) inhibits p38 MAPK and senescence-associated inflammatory mediator secretion by senescent fibroblasts that impacts immune responses during ageing. Aging Cell. (2024) 23:e14093. doi: 10.1111/acel.14093
38. Kang C, Xu Q, Martin TD, Li MZ, Demaria M, Aron L, et al. The DNA damage response induces inflammation and senescence by inhibiting autophagy of GATA4. Science. (2015) 349:aaa5612. doi: 10.1126/science.aaa5612
39. Takahashi A, Loo TM, Okada R, Kamachi F, Watanabe Y, Wakita M, et al. Downregulation of cytoplasmic DNases is implicated in cytoplasmic DNA accumulation and SASP in senescent cells. Nat Commun. (2018) 9:1249. doi: 10.1038/s41467-018-03555-8
40. Orjalo AV, Bhaumik D, Gengler BK, Scott GK, Campisi J. Cell surface-bound IL-1alpha is an upstream regulator of the senescence-associated IL-6/IL-8 cytokine network. Proc Natl Acad Sci U.S.A. (2009) 106:17031–6. doi: 10.1073/pnas.0905299106
41. Hoare M, Ito Y, Kang TW, Weekes MP, Matheson NJ, Patten DA, et al. NOTCH1 mediates a switch between two distinct secretomes during senescence. Nat Cell Biol. (2016) 18:979–92. doi: 10.1038/ncb3397
42. Beaulieu D, Attwe A, Breau M, Lipskaia L, Marcos E, Born E, et al. Phospholipase A2 receptor 1 promotes lung cell senescence and emphysema in obstructive lung disease. Eur Respir J. (2021) 58:2000752. doi: 10.1183/13993003.00752-2020
43. Renuka K, Xiaomeng Y, Tamar T, Martin George M, Kirkland James L, Junko O. TNF-α/IFN-γ synergy amplifies senescence-associated inflammation and SARS-CoV-2 receptor expression via hyper-activated JAK/STAT1. Aging Cell. (2022) 21:e13646. doi: 10.1111/acel.13646
44. Li X, Li C, Zhang W, Wang Y, Qian P, Huang H. Inflammation and aging: signaling pathways and intervention therapies. Signal Transduct Target Ther. (2023) 8:239. doi: 10.1038/s41392-023-01502-8
45. Mitchell CA, Verovskaya EV, Calero-Nieto FJ, Olson OC, Swann JW, Wang X, et al. Stromal niche inflammation mediated by IL-1 signalling is a targetable driver of haematopoietic ageing. Nat Cell Biol. (2023) 25:30–41. doi: 10.1038/s41556-022-01053-0
46. Pioli PD, Casero D, Montecino-Rodriguez E, Morrison SL, Dorshkind K. Plasma cells are obligate effectors of enhanced myelopoiesis in aging bone marrow. Immunity. (2019) 51:351–66.e6. doi: 10.1016/j.immuni.2019.06.006
47. Chen J, Feng X, Desierto MJ, Keyvanfar K, Young NS. IFN-γ-mediated hematopoietic cell destruction in murine models of immune-mediated bone marrow failure. Blood. (2015) 126:2621–31. doi: 10.1182/blood-2015-06-652453
48. Xiao Y, Yu D. Tumor microenvironment as a therapeutic target in cancer. Pharmacol Ther. (2021) 221:107753. doi: 10.1016/j.pharmthera.2020.107753
49. Ma J, Huang L, Hu D, Zeng S, Han Y, Shen H. The role of the tumor microbe microenvironment in the tumor immune microenvironment: bystander, activator, or inhibitor. J Exp Clin Cancer Res. (2021) 40:327. doi: 10.1186/s13046-021-02128-w
50. Fu T, Dai LJ, Wu SY, Xiao Y, Ma D, Jiang YZ, et al. Spatial architecture of the immune microenvironment orchestrates tumor immunity and therapeutic response. J Hematol Oncol. (2021) 14:98. doi: 10.1186/s13045-021-01103-4
51. Zhang T, Jia Y, Yu Y, Zhang B, Xu F, Guo H. Targeting the tumor biophysical microenvironment to reduce resistance to immunotherapy. Adv Drug Delivery Rev. (2022) 186:114319. doi: 10.1016/j.addr.2022.114319
52. Huang J, Zhang L, Wan D, Zhou L, Zheng S, Lin S, et al. Extracellular matrix and its therapeutic potential for cancer treatment. Signal Transduct Target Ther. (2021) 6:153. doi: 10.1038/s41392-021-00544-0
53. D'Ambrosio M, Gil J. Reshaping of the tumor microenvironment by cellular senescence: An opportunity for senotherapies. Dev Cell. (2023) 58:1007–21. doi: 10.1016/j.devcel.2023.05.010
54. Johnson DE, O'Keefe RA, Grandis JR. Targeting the IL-6/JAK/STAT3 signalling axis in cancer. Nat Rev Clin Oncol. (2018) 15:234–48. doi: 10.1038/nrclinonc.2018.8
55. Meraviglia-Crivelli D, Villanueva H, Zheleva A, Villalba-Esparza M, Moreno B, Menon AP, et al. IL-6/STAT3 signaling in tumor cells restricts the expression of frameshift-derived neoantigens by SMG1 induction. Mol Cancer. (2022) 21:211. doi: 10.1186/s12943-022-01679-6
56. Bent EH, Millán-Barea LR, Zhuang I, Goulet DR, Fröse J, Hemann MT. Microenvironmental IL-6 inhibits anti-cancer immune responses generated by cytotoxic chemotherapy. Nat Commun. (2021) 12:6218. doi: 10.1038/s41467-021-26407-4
57. Heichler C, Scheibe K, Schmied A, Geppert CI, Schmid B, Wirtz S, et al. STAT3 activation through IL-6/IL-11 in cancer-associated fibroblasts promotes colorectal tumour development and correlates with poor prognosis. Gut. (2020) 69:1269–82. doi: 10.1136/gutjnl-2019-319200
58. Chen RY, Yen CJ, Liu YW, Guo CG, Weng CY, Lai CH, et al. CPAP promotes angiogenesis and metastasis by enhancing STAT3 activity. Cell Death Differ. (2020) 27:1259–73. doi: 10.1038/s41418-019-0413-7
59. Sharma I, Singh A, Siraj F, Saxena S. IL-8/CXCR1/2 signalling promotes tumor cell proliferation, invasion and vascular mimicry in glioblastoma. J BioMed Sci. (2018) 25:62. doi: 10.1186/s12929-018-0464-y
60. Rolny C, Capparuccia L, Casazza A, Mazzone M, Vallario A, Cignetti A, et al. The tumor suppressor semaphorin 3B triggers a prometastatic program mediated by interleukin 8 and the tumor microenvironment. J Exp Med. (2008) 205:1155–71. doi: 10.1084/jem.20072509
61. Fousek K, Horn LA, Palena C. Interleukin-8: A chemokine at the intersection of cancer plasticity, angiogenesis, and immune suppression. Pharmacol Ther. (2021) 219:107692. doi: 10.1016/j.pharmthera.2020.107692
62. Tengesdal IW, Dinarello A, Powers NE, Burchill MA, Joosten L, Marchetti C, et al. Tumor NLRP3-derived IL-1β Drives the IL-6/STAT3 axis resulting in sustained MDSC-mediated immunosuppression. Front Immunol. (2021) 12:661323. doi: 10.3389/fimmu.2021.661323
63. Tengesdal IW, Menon DR, Osborne DG, Neff CP, Powers NE, Gamboni F, et al. Targeting tumor-derived NLRP3 reduces melanoma progression by limiting MDSCs expansion. Proc Natl Acad Sci U.S.A. (2021) 118:e2000915118. doi: 10.1073/pnas.2000915118
64. Korbecki J, Grochans S, Gutowska I, Barczak K, Baranowska-Bosiacka I. CC chemokines in a tumor: A review of pro-cancer and anti-cancer properties of receptors CCR5, CCR6, CCR7, CCR8, CCR9, and CCR10 ligands. Int J Mol Sci. (2020) 21:7619. doi: 10.3390/ijms21207619
65. Pein M, Insua-Rodríguez J, Hongu T, Riedel A, Meier J, Wiedmann L, et al. Metastasis-initiating cells induce and exploit a fibroblast niche to fuel Malignant colonization of the lungs. Nat Commun. (2020) 11:1494. doi: 10.1038/s41467-020-15188-x
66. Takasugi M, Yoshida Y, Ohtani N. Cellular senescence and the tumour microenvironment. Mol Oncol. (2022) 16:3333–51. doi: 10.1002/1878-0261.13268
67. Chang LY, Lin YC, Mahalingam J, Huang CT, Chen TW, Kang CW, et al. Tumor-derived chemokine CCL5 enhances TGF-β-mediated killing of CD8(+) T cells in colon cancer by T-regulatory cells. Cancer Res. (2012) 72:1092–102. doi: 10.1158/0008-5472.CAN-11-2493
68. Böttcher JP, Bonavita E, Chakravarty P, Blees H, Cabeza-Cabrerizo M, Sammicheli S, et al. NK Cells Stimulate Recruitment of cDC1 into the Tumor Microenvironment Promoting Cancer Immune Control. Cell. (2018) 172:1022–37.e14. doi: 10.1016/j.cell.2018.01.004
69. Taki M, Abiko K, Baba T, Hamanishi J, Yamaguchi K, Murakami R, et al. Snail promotes ovarian cancer progression by recruiting myeloid-derived suppressor cells via CXCR2 ligand upregulation. Nat Commun. (2018) 9:1685. doi: 10.1038/s41467-018-03966-7
70. Hu J, Zhao Q, Kong LY, Wang J, Yan J, Xia X, et al. Regulation of tumor immune suppression and cancer cell survival by CXCL1/2 elevation in glioblastoma multiforme. Sci Adv. (2021) 7:eabc2511. doi: 10.1126/sciadv.abc2511
71. Chen Y, Kim J, Yang S, Wang H, Wu CJ, Sugimoto H, et al. Type I collagen deletion in αSMA(+) myofibroblasts augments immune suppression and accelerates progression of pancreatic cancer. Cancer Cell. (2021) 39:548–565.e6. doi: 10.1016/j.ccell.2021.02.007
72. Sorrentino C, D'Antonio L, Ciummo SL, Fieni C, Landuzzi L, Ruzzi F, et al. CRISPR/Cas9-mediated deletion of Interleukin-30 suppresses IGF1 and CXCL5 and boosts SOCS3 reducing prostate cancer growth and mortality. J Hematol Oncol. (2022) 15:145. doi: 10.1186/s13045-022-01357-6
73. Garg B, Giri B, Modi S, Sethi V, Castro I, Umland O, et al. NFκB in pancreatic stellate cells reduces infiltration of tumors by cytotoxic T cells and killing of cancer cells, via up-regulation of CXCL12. Gastroenterology. (2018) 155:880–891.e8. doi: 10.1053/j.gastro.2018.05.051
74. Heidegger I, Fotakis G, Offermann A, Goveia J, Daum S, Salcher S, et al. Comprehensive characterization of the prostate tumor microenvironment identifies CXCR4/CXCL12 crosstalk as a novel antiangiogenic therapeutic target in prostate cancer. Mol Cancer. (2022) 21:132. doi: 10.1186/s12943-022-01597-7
75. Akiyama T, Yasuda T, Uchihara T, Yasuda-Yoshihara N, Tan B, Yonemura A, et al. Stromal reprogramming through dual PDGFRα/β Blockade boosts the efficacy of anti-PD-1 immunotherapy in fibrotic tumors. Cancer Res. (2023) 83:753–70. doi: 10.1158/0008-5472.CAN-22-1890
76. Ding D, Zhong H, Liang R, Lan T, Zhu X, Huang S, et al. Multifunctional nanodrug mediates synergistic photodynamic therapy and MDSCs-targeting immunotherapy of colon cancer. Adv Sci (Weinh). (2021) 8:e2100712. doi: 10.1002/advs.202100712
77. Daniel SK, Seo YD, Pillarisetty VG. The CXCL12-CXCR4/CXCR7 axis as a mechanism of immune resistance in gastrointestinal Malignancies. Semin Cancer Biol. (2020) 65:176–88. doi: 10.1016/j.semcancer.2019.12.007
78. Cui Y, Liu H, Wang Z, Zhang H, Tian J, Wang Z, et al. Fructose promotes angiogenesis by improving vascular endothelial cell function and upregulating VEGF expression in cancer cells. J Exp Clin Cancer Res. (2023) 42:184. doi: 10.1186/s13046-023-02765-3
79. Arnold IC, Artola-Boran M, Gurtner A, Bertram K, Bauer M, Frangez Z, et al. The GM-CSF-IRF5 signaling axis in eosinophils promotes antitumor immunity through activation of type 1 T cell responses. J Exp Med. (2020) 217:e20190706. doi: 10.1084/jem.20190706
80. Gao A, Liu X, Lin W, Wang J, Wang S, Si F, et al. Tumor-derived ILT4 induces T cell senescence and suppresses tumor immunity. J Immunother Cancer. (2021) 9:e001536. doi: 10.1136/jitc-2020-001536
81. Lanna A, Henson SM, Escors D, Akbar AN. The kinase p38 activated by the metabolic regulator AMPK and scaffold TAB1 drives the senescence of human T cells. Nat Immunol. (2014) 15:965–72. doi: 10.1038/ni.2981
82. Callender LA, Carroll EC, Beal R, Chambers ES, Nourshargh S, Akbar AN, et al. Human CD8(+) EMRA T cells display a senescence-associated secretory phenotype regulated by p38 MAPK. Aging Cell. (2018) 17:e12675. doi: 10.1111/acel.12675
83. Ye J, Ma C, Hsueh EC, Dou J, Mo W, Liu S, et al. TLR8 signaling enhances tumor immunity by preventing tumor-induced T-cell senescence. EMBO Mol Med. (2014) 6:1294–311. doi: 10.15252/emmm.201403918
84. Mathiasen SL, Gall-Mas L, Pateras IS, Theodorou S, Namini M, Hansen MB, et al. Bacterial genotoxins induce T cell senescence. Cell Rep. (2021) 35:109220. doi: 10.1016/j.celrep.2021.109220
85. Chen X, Liu Q, Xiang AP. CD8+CD28- T cells: not only age-related cells but a subset of regulatory T cells. Cell Mol Immunol. (2018) 15:734–6. doi: 10.1038/cmi.2017.153
86. Hendriks J, Gravestein LA, Tesselaar K, van Lier RA, Schumacher TN, Borst J. CD27 is required for generation and long-term maintenance of T cell immunity. Nat Immunol. (2000) 1:433–40. doi: 10.1038/80877
87. Lanna A, Gomes DC, Muller-Durovic B, McDonnell T, Escors D, Gilroy DW, et al. A sestrin-dependent Erk-Jnk-p38 MAPK activation complex inhibits immunity during aging. Nat Immunol. (2017) 18:354–63. doi: 10.1038/ni.3665
88. Daniel L, Tassery M, Lateur C, Thierry A, Herbelin A, Gombert JM, et al. Allotransplantation is associated with exacerbation of CD8 T-cell senescence: the particular place of the innate CD8 T-cell component. Front Immunol. (2021) 12:674016. doi: 10.3389/fimmu.2021.674016
89. Fernandes JR, Pinto T, Arruda LB, da Silva C, de Carvalho C, Pinto R, et al. Age-associated phenotypic imbalance in TCD4 and TCD8 cell subsets: comparison between healthy aged, smokers, COPD patients and young adults. Immun Ageing. (2022) 19:9. doi: 10.1186/s12979-022-00267-y
90. Liu X, Hoft DF, Peng G. Senescent T cells within suppressive tumor microenvironments: emerging target for tumor immunotherapy. J Clin Invest. (2020) 130:1073–83. doi: 10.1172/JCI133679
91. Ye J, Ma C, Hsueh EC, Eickhoff CS, Zhang Y, Varvares MA, et al. Tumor-derived γδ regulatory T cells suppress innate and adaptive immunity through the induction of immunosenescence. J Immunol. (2013) 190:2403–14. doi: 10.4049/jimmunol.1202369
92. Laumont CM, Nelson BH. B cells in the tumor microenvironment: Multi-faceted organizers, regulators, and effectors of anti-tumor immunity. Cancer Cell. (2023) 41:466–89. doi: 10.1016/j.ccell.2023.02.017
93. Ratliff M, Alter S, Frasca D, Blomberg BB, Riley RL. In senescence, age-associated B cells secrete TNFα and inhibit survival of B-cell precursors. Aging Cell. (2013) 12:303–11. doi: 10.1111/acel.12055
94. Qin Y, Cai ML, Jin HZ, Huang W, Zhu C, Bozec A, et al. Age-associated B cells contribute to the pathogenesis of rheumatoid arthritis by inducing activation of fibroblast-like synoviocytes via TNF-α-mediated ERK1/2 and JAK-STAT1 pathways. Ann Rheum Dis. (2022) 81:1504–14. doi: 10.1136/ard-2022-222605
95. Chung M, Gong L, Kwong DL, Lee VH, Lee AW, Guan XY, et al. Functions of double-negative B cells in autoimmune diseases, infections, and cancers. EMBO Mol Med. (2023) 15:e17341. doi: 10.15252/emmm.202217341
96. Bulati M, Caruso C, Colonna-Romano G. From lymphopoiesis to plasma cells differentiation, the age-related modifications of B cell compartment are influenced by "inflamm-ageing". Ageing Res Rev. (2017) 36:125–36. doi: 10.1016/j.arr.2017.04.001
97. Bulati M, Buffa S, Martorana A, Gervasi F, Camarda C, Azzarello DM, et al. Double negative (IgG+IgD-CD27-) B cells are increased in a cohort of moderate-severe Alzheimer's disease patients and show a pro-inflammatory trafficking receptor phenotype. J Alzheimers Dis. (2015) 44:1241–51. doi: 10.3233/JAD-142412
98. Agrawal A, Gupta S. Impact of aging on dendritic cell functions in humans. Ageing Res Rev. (2011) 10:336–45. doi: 10.1016/j.arr.2010.06.004
99. Grolleau-Julius A, Harning EK, Abernathy LM, Yung RL. Impaired dendritic cell function in aging leads to defective antitumor immunity. Cancer Res. (2008) 68:6341–9. doi: 10.1158/0008-5472.CAN-07-5769
100. Gardner JK, Mamotte C, Jackaman C, Nelson DJ. Modulation of dendritic cell and T cell cross-talk during aging: The potential role of checkpoint inhibitory molecules. Ageing Res Rev. (2017) 38:40–51. doi: 10.1016/j.arr.2017.07.002
101. Veglia F, Sanseviero E, Gabrilovich DI. Myeloid-derived suppressor cells in the era of increasing myeloid cell diversity. Nat Rev Immunol. (2021) 21:485–98. doi: 10.1038/s41577-020-00490-y
102. Hsu J, Hodgins JJ, Marathe M, Nicolai CJ, Bourgeois-Daigneault MC, Trevino TN, et al. Contribution of NK cells to immunotherapy mediated by PD-1/PD-L1 blockade. J Clin Invest. (2018) 128:4654–68. doi: 10.1172/JCI99317
103. Jin Y, Kang Y, Wang M, Wu B, Su B, Yin H, et al. Targeting polarized phenotype of microglia via IL6/JAK2/STAT3 signaling to reduce NSCLC brain metastasis. Signal Transduct Target Ther. (2022) 7:52. doi: 10.1038/s41392-022-00872-9
104. Habtetsion T, Ding ZC, Pi W, Li T, Lu C, Chen T, et al. Alteration of tumor metabolism by CD4+ T cells leads to TNF-α-dependent intensification of oxidative stress and tumor cell death. Cell Metab. (2018) 28:228–42.e6. doi: 10.1016/j.cmet.2018.05.012
105. Liu X, Mo W, Ye J, Li L, Zhang Y, Hsueh EC, et al. Regulatory T cells trigger effector T cell DNA damage and senescence caused by metabolic competition. Nat Commun. (2018) 9:249. doi: 10.1038/s41467-017-02689-5
106. Song DG, Ye Q, Poussin M, Harms GM, Figini M, Powell DJ Jr.CD27 costimulation augments the survival and antitumor activity of redirected human T cells in vivo. Blood. (2012) 119:696–706. doi: 10.1182/blood-2011-03-344275
107. Gong Z, Jia Q, Chen J, Diao X, Gao J, Wang X, et al. Impaired cytolytic activity and loss of clonal neoantigens in elderly patients with lung adenocarcinoma. J Thorac Oncol. (2019) 14:857–66. doi: 10.1016/j.jtho.2019.01.024
108. Zöphel D, Angenendt A, Kaschek L, Ravichandran K, Hof C, Janku S, et al. Faster cytotoxicity with age: Increased perforin and granzyme levels in cytotoxic CD8(+) T cells boost cancer cell elimination. Aging Cell. (2022) 21:e13668. doi: 10.1111/acel.13668
109. Mittelbrunn M, Kroemer G. Hallmarks of T cell aging. Nat Immunol. (2021) 22:687–98. doi: 10.1038/s41590-021-00927-z
110. Wang X, Guo S, Zhou H, Sun Y, Gan J, Zhang Y, et al. Pan-cancer transcriptomic analysis identified six classes of immunosenescence genes revealed molecular links between aging, immune system and cancer. Genes Immun. (2023) 24:81–91. doi: 10.1038/s41435-023-00197-9
111. Ye J, Huang X, Hsueh EC, Zhang Q, Ma C, Zhang Y, et al. Human regulatory T cells induce T-lymphocyte senescence. Blood. (2012) 120:2021–31. doi: 10.1182/blood-2012-03-416040
112. Frasca D, Diaz A, Romero M, Garcia D, Blomberg BB. B cell immunosenescence. Annu Rev Cell Dev Biol. (2020) 36:551–74. doi: 10.1146/annurev-cellbio-011620-034148
113. Yu K, Ravoor A, Malats N, Pineda S, Sirota M. A pan-cancer analysis of tumor-infiltrating B cell repertoires. Front Immunol. (2021) 12:790119. doi: 10.3389/fimmu.2021.790119
114. Nickerson KM, Smita S, Hoehn KB, Marinov AD, Thomas KB, Kos JT, et al. Age-associated B cells are heterogeneous and dynamic drivers of autoimmunity in mice. J Exp Med. (2023) 220:e20221346. doi: 10.1084/jem.20221346
115. Chiu BC, Martin BE, Stolberg VR, Chensue SW. The host environment is responsible for aging-related functional NK cell deficiency. J Immunol. (2013) 191:4688–98. doi: 10.4049/jimmunol.1301625
116. Hazeldine J, Lord JM. The impact of ageing on natural killer cell function and potential consequences for health in older adults. Ageing Res Rev. (2013) 12:1069–78. doi: 10.1016/j.arr.2013.04.003
117. Hazeldine J, Hampson P, Lord JM. Reduced release and binding of perforin at the immunological synapse underlies the age-related decline in natural killer cell cytotoxicity. Aging Cell. (2012) 11:751–9. doi: 10.1111/j.1474-9726.2012.00839.x
118. Shehata HM, Hoebe K, Chougnet CA. The aged nonhematopoietic environment impairs natural killer cell maturation and function. Aging Cell. (2015) 14:191–9. doi: 10.1111/acel.12303
119. Mariani E, Pulsatelli L, Meneghetti A, Dolzani P, Mazzetti I, Neri S, et al. Different IL-8 production by T and NK lymphocytes in elderly subjects. Mech Ageing Dev. (2001) 122:1383–95. doi: 10.1016/S0047-6374(01)00270-6
120. Mariani E, Meneghetti A, Neri S, Ravaglia G, Forti P, Cattini L, et al. Chemokine production by natural killer cells from nonagenarians. Eur J Immunol. (2002) 32:1524–9. doi: 10.1002/1521-4141(200206)32:6<1524::AID-IMMU1524>3.0.CO;2-E
121. Wang Y, Xiang Y, Xin VW, Wang XW, Peng XC, Liu XQ, et al. Dendritic cell biology and its role in tumor immunotherapy. J Hematol Oncol. (2020) 13:107. doi: 10.1186/s13045-020-00939-6
122. Sun R, Xiong Y, Liu H, Gao C, Su L, Weng J, et al. Tumor-associated neutrophils suppress antitumor immunity of NK cells through the PD-L1/PD-1 axis. Transl Oncol. (2020) 13:100825. doi: 10.1016/j.tranon.2020.100825
123. Fleming V, Hu X, Weller C, Weber R, Groth C, Riester Z, et al. Melanoma extracellular vesicles generate immunosuppressive myeloid cells by upregulating PD-L1 via TLR4 signaling. Cancer Res. (2019) 79:4715–28. doi: 10.1158/0008-5472.CAN-19-0053
124. Mezrich JD, Fechner JH, Zhang X, Johnson BP, Burlingham WJ, Bradfield CA. An interaction between kynurenine and the aryl hydrocarbon receptor can generate regulatory T cells. J Immunol. (2010) 185:3190–8. doi: 10.4049/jimmunol.0903670
125. Frumento G, Rotondo R, Tonetti M, Damonte G, Benatti U, Ferrara GB. Tryptophan-derived catabolites are responsible for inhibition of T and natural killer cell proliferation induced by indoleamine 2,3-dioxygenase. J Exp Med. (2002) 196:459–68. doi: 10.1084/jem.20020121
126. Salminen A, Kaarniranta K, Kauppinen A. Immunosenescence: the potential role of myeloid-derived suppressor cells (MDSC) in age-related immune deficiency. Cell Mol Life Sci. (2019) 76:1901–18. doi: 10.1007/s00018-019-03048-x
127. Liao W, Overman MJ, Boutin AT, Shang X, Zhao D, Dey P, et al. KRAS-IRF2 axis drives immune suppression and immune therapy resistance in colorectal cancer. Cancer Cell. (2019) 35:559–572.e7. doi: 10.1016/j.ccell.2019.02.008
128. Chen H, Pan Y, Zhou Q, Liang C, Wong CC, Zhou Y, et al. METTL3 inhibits antitumor immunity by targeting m(6)A-BHLHE41-CXCL1/CXCR2 axis to promote colorectal cancer. Gastroenterology. (2022) 163:891–907. doi: 10.1053/j.gastro.2022.06.024
129. Wu P, Wu D, Ni C, Ye J, Chen W, Hu G, et al. γδT17 cells promote the accumulation and expansion of myeloid-derived suppressor cells in human colorectal cancer. Immunity. (2014) 40:785–800. doi: 10.1016/j.immuni.2014.03.013
130. Chun E, Lavoie S, Michaud M, Gallini CA, Kim J, Soucy G, et al. CCL2 promotes colorectal carcinogenesis by enhancing polymorphonuclear myeloid-derived suppressor cell population and function. Cell Rep. (2015) 12:244–57. doi: 10.1016/j.celrep.2015.06.024
131. Schneider KM, Mohs A, Gui W, Galvez E, Candels LS, Hoenicke L, et al. Imbalanced gut microbiota fuels hepatocellular carcinoma development by shaping the hepatic inflammatory microenvironment. Nat Commun. (2022) 13:3964. doi: 10.1038/s41467-022-31312-5
132. Zhang Z, Zheng Y, Chen Y, Yin Y, Chen Y, Chen Q, et al. Gut fungi enhances immunosuppressive function of myeloid-derived suppressor cells by activating PKM2-dependent glycolysis to promote colorectal tumorigenesis. Exp Hematol Oncol. (2022) 11:88. doi: 10.1186/s40164-022-00334-6
133. Ibrahim ML, Klement JD, Lu C, Redd PS, Xiao W, Yang D, et al. Myeloid-derived suppressor cells produce IL-10 to elicit DNMT3b-dependent IRF8 silencing to promote colitis-associated colon tumorigenesis. Cell Rep. (2018) 25:3036–3046.e6. doi: 10.1016/j.celrep.2018.11.050
134. Condamine T, Gabrilovich DI. Molecular mechanisms regulating myeloid-derived suppressor cell differentiation and function. Trends Immunol. (2011) 32:19–25. doi: 10.1016/j.it.2010.10.002
135. Redd PS, Ibrahim ML, Klement JD, Sharman SK, Paschall AV, Yang D, et al. SETD1B activates iNOS expression in myeloid-derived suppressor cells. Cancer Res. (2017) 77:2834–43. doi: 10.1158/0008-5472.CAN-16-2238
136. Conche C, Finkelmeier F, Pešić M, Nicolas AM, Böttger TW, Kennel KB, et al. Combining ferroptosis induction with MDSC blockade renders primary tumours and metastases in liver sensitive to immune checkpoint blockade. Gut. (2023) 72:1774–1782. doi: 10.1136/gutjnl-2022-327909
137. Ohl K, Tenbrock K. Reactive oxygen species as regulators of MDSC-mediated immune suppression. Front Immunol. (2018) 9:2499. doi: 10.3389/fimmu.2018.02499
138. Jackaman C, Tomay F, Duong L, Abdol Razak NB, Pixley FJ, Metharom P, et al. Aging and cancer: The role of macrophages and neutrophils. Ageing Res Rev. (2017) 36:105–16. doi: 10.1016/j.arr.2017.03.008
139. Nicolas AM, Pesic M, Engel E, Ziegler PK, Diefenhardt M, Kennel KB, et al. Inflammatory fibroblasts mediate resistance to neoadjuvant therapy in rectal cancer. Cancer Cell. (2022) 40:168–184.e13. doi: 10.1016/j.ccell.2022.01.004
140. Zhao Y, Tyshkovskiy A, Muñoz-Espín D, Tian X, Serrano M, de Magalhaes JP, et al. Naked mole rats can undergo developmental, oncogene-induced and DNA damage-induced cellular senescence. Proc Natl Acad Sci U.S.A. (2018) 115:1801–6. doi: 10.1073/pnas.1721160115
141. Han Z, Wei W, Dunaway S, Darnowski JW, Calabresi P, Sedivy J, et al. Role of p21 in apoptosis and senescence of human colon cancer cells treated with camptothecin. J Biol Chem. (2002) 277:17154–60. doi: 10.1074/jbc.M112401200
142. Jaiswal AS, Panda H, Law BK, Sharma J, Jani J, Hromas R, et al. NSC666715 and its analogs inhibit strand-displacement activity of DNA polymerase β and potentiate temozolomide-induced DNA damage, senescence and apoptosis in colorectal cancer cells. PloS One. (2015) 10:e0123808. doi: 10.1371/journal.pone.0123808
143. Ahmetlic F, Fauser J, Riedel T, Bauer V, Flessner C, Hömberg N, et al. Therapy of lymphoma by immune checkpoint inhibitors: the role of T cells, NK cells and cytokine-induced tumor senescence. J Immunother Cancer. (2021) 9:e001660. doi: 10.1136/jitc-2020-001660
144. Prasanna PG, Citrin DE, Hildesheim J, Ahmed MM, Venkatachalam S, Riscuta G, et al. Therapy-induced senescence: opportunities to improve anticancer therapy. J Natl Cancer Inst. (2021) 113:1285–98. doi: 10.1093/jnci/djab064
145. Wang B, Kohli J, Demaria M. Senescent cells in cancer therapy: friends or foes. Trends Cancer. (2020) 6:838–57. doi: 10.1016/j.trecan.2020.05.004
146. Sikora E, Bielak-Zmijewska A, Mosieniak G. Targeting normal and cancer senescent cells as a strategy of senotherapy. Ageing Res Rev. (2019) 55:100941. doi: 10.1016/j.arr.2019.100941
147. Wang L, Lankhorst L, Bernards R. Exploiting senescence for the treatment of cancer. Nat Rev Cancer. (2022) 22:340–55. doi: 10.1038/s41568-022-00450-9
148. Yosef R, Pilpel N, Tokarsky-Amiel R, Biran A, Ovadya Y, Cohen S, et al. Directed elimination of senescent cells by inhibition of BCL-W and BCL-XL. Nat Commun. (2016) 7:11190. doi: 10.1038/ncomms11190
149. Chang J, Wang Y, Shao L, Laberge RM, Demaria M, Campisi J, et al. Clearance of senescent cells by ABT263 rejuvenates aged hematopoietic stem cells in mice. Nat Med. (2016) 22:78–83. doi: 10.1038/nm.4010
150. Zhu Y, Tchkonia T, Pirtskhalava T, Gower AC, Ding H, Giorgadze N, et al. The Achilles' heel of senescent cells: from transcriptome to senolytic drugs. Aging Cell. (2015) 14:644–58. doi: 10.1111/acel.12344
151. Duro-Sánchez S, Nadal-Serrano M, Lalinde-Gutiérrez M, Arenas EJ, Bernadó Morales C, Morancho B, et al. Therapy-induced senescence enhances the efficacy of HER2-targeted antibody-drug conjugates in breast cancer. Cancer Res. (2022) 82:4670–9. doi: 10.1158/0008-5472.CAN-22-0787
152. Chaturvedi P, George V, Shrestha N, Wang M, Dee MJ, Zhu X, et al. Immunotherapeutic HCW9218 augments anti-tumor activity of chemotherapy via NK cell-mediated reduction of therapy-induced senescent cells. Mol Ther. (2022) 30:1171–87. doi: 10.1016/j.ymthe.2022.01.025
153. Amor C, Feucht J, Leibold J, Ho YJ, Zhu C, Alonso-Curbelo D, et al. Senolytic CAR T cells reverse senescence-associated pathologies. Nature. (2020) 583:127–32. doi: 10.1038/s41586-020-2403-9
154. Wang C, Vegna S, Jin H, Benedict B, Lieftink C, Ramirez C, et al. Inducing and exploiting vulnerabilities for the treatment of liver cancer. Nature. (2019) 574:268–72. doi: 10.1038/s41586-019-1607-3
155. Acosta JC, O'Loghlen A, Banito A, Guijarro MV, Augert A, Raguz S, et al. Chemokine signaling via the CXCR2 receptor reinforces senescence. Cell. (2008) 133:1006–18. doi: 10.1016/j.cell.2008.03.038
156. Toso A, Revandkar A, Di Mitri D, Guccini I, Proietti M, Sarti M, et al. Enhancing chemotherapy efficacy in Pten-deficient prostate tumors by activating the senescence-associated antitumor immunity. Cell Rep. (2014) 9:75–89. doi: 10.1016/j.celrep.2014.08.044
157. Sui D, Li C, Tang X, Meng X, Ding J, Yang Q, et al. Sialic acid-mediated photochemotherapy enhances infiltration of CD8(+) T cells from tumor-draining lymph nodes into tumors of immunosenescent mice. Acta Pharm Sin B. (2023) 13:425–39. doi: 10.1016/j.apsb.2022.06.005
158. Legscha KJ, Antunes Ferreira E, Chamoun A, Lang A, Awwad M, Ton G, et al. Δ133p53α enhances metabolic and cellular fitness of TCR-engineered T cells and promotes superior antitumor immunity. J Immunother Cancer. (2021) 9:e001846. doi: 10.1136/jitc-2020-001846
159. Pawelec G. The human immunosenescence phenotype: does it exist. Semin Immunopathol. (2020) 42:537–44. doi: 10.1007/s00281-020-00810-3
160. Bao H, Cao J, Chen M, Chen M, Chen W, Chen X, et al. Biomarkers of aging. Sci China Life Sci. (2023) 66:893–1066. doi: 10.1007/s11427-023-2305-0
161. Salumets A, Tserel L, Rumm AP, Türk L, Kingo K, Saks K, et al. Epigenetic quantification of immunosenescent CD8(+) TEMRA cells in human blood. Aging Cell. (2022) 21:e13607. doi: 10.1111/acel.13607
162. Zhang L, Chen X, Zu S, Lu Y. Characteristics of circulating adaptive immune cells in patients with colorectal cancer. Sci Rep. (2022) 12:18166. doi: 10.1038/s41598-022-23190-0
163. Aprilia A, Handono K, Sujuti H, Sabarudin A, Winaris N. sCD163, sCD28, sCD80, and sCTLA-4 as soluble marker candidates for detecting immunosenescence. Immun Ageing. (2024) 21:9. doi: 10.1186/s12979-023-00405-0
164. Arikan S, Gümüş A, Küçükhüseyin Ö, Coşkun C, Turan S, Cacina C, et al. The effect of CTLA-4 and CD28 gene variants and circulating protein levels in patients with gastric cancer. Turk J Biochem. (2017) 42(5):551–8. doi: 10.1515/tjb-2017-0024
165. Ding D, Song Y, Yao Y, Zhang S. Preoperative serum macrophage activated biomarkers soluble mannose receptor (sMR) and soluble haemoglobin scavenger receptor (sCD163), as novel markers for the diagnosis and prognosis of gastric cancer. Oncol Lett. (2017) 14:2982–90. doi: 10.3892/ol.2017.6547
166. Al-Danakh A, Safi M, Jian Y, Yang L, Zhu X, Chen Q, et al. Aging-related biomarker discovery in the era of immune checkpoint inhibitors for cancer patients. Front Immunol. (2024) 15:1348189. doi: 10.3389/fimmu.2024.1348189
167. Shah Y, Verma A, Marderstein AR, White J, Bhinder B, Garcia Medina JS, et al. Pan-cancer analysis reveals molecular patterns associated with age. Cell Rep. (2021) 37:110100. doi: 10.1016/j.celrep.2021.110100
168. Lee W, Wang Z, Saffern M, Jun T, Huang KL. Genomic and molecular features distinguish young adult cancer from later-onset cancer. Cell Rep. (2021) 37:110005. doi: 10.1016/j.celrep.2021.110005
Keywords: gastrointestinal tumors, colorectal cancer, immunosenescence, tumor microenvironment, immunotherapy
Citation: Zhang T, Wen R, Fan H, Yu Y, Jia H, Peng Z, Zhou L, Yu G and Zhang W (2024) Impact and potential value of immunosenescence on solid gastrointestinal tumors. Front. Immunol. 15:1375730. doi: 10.3389/fimmu.2024.1375730
Received: 24 January 2024; Accepted: 17 June 2024;
Published: 28 June 2024.
Edited by:
Shahid Ali, University of Swat, PakistanCopyright © 2024 Zhang, Wen, Fan, Yu, Jia, Peng, Zhou, Yu and Zhang. This is an open-access article distributed under the terms of the Creative Commons Attribution License (CC BY). The use, distribution or reproduction in other forums is permitted, provided the original author(s) and the copyright owner(s) are credited and that the original publication in this journal is cited, in accordance with accepted academic practice. No use, distribution or reproduction is permitted which does not comply with these terms.
*Correspondence: Wei Zhang, d2VpemhhbmcyMDAwY25AMTYzLmNvbQ==; Guanyu Yu, eXVndWFueXUwNDUxQDE2My5jb20=; Leqi Zhou, cmljaGFyZDEyQDEyNi5jb20=
†These authors have contributed equally to this work