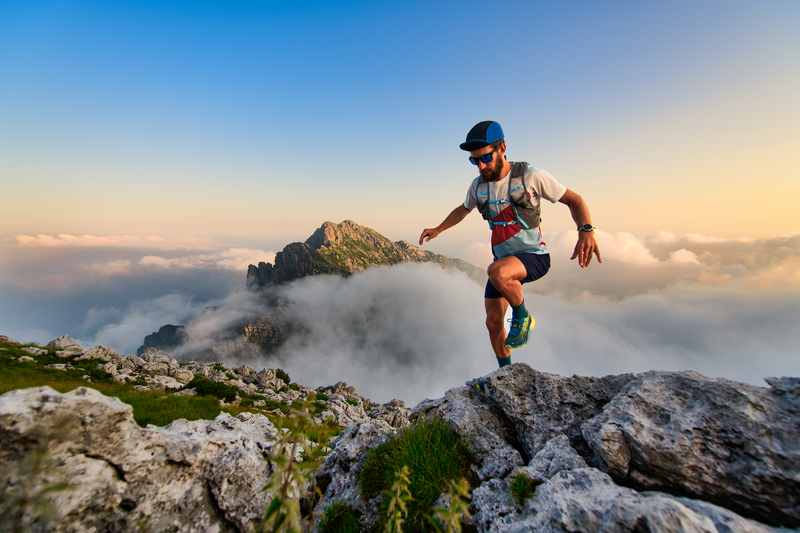
94% of researchers rate our articles as excellent or good
Learn more about the work of our research integrity team to safeguard the quality of each article we publish.
Find out more
REVIEW article
Front. Immunol. , 10 May 2024
Sec. Cancer Immunity and Immunotherapy
Volume 15 - 2024 | https://doi.org/10.3389/fimmu.2024.1370800
This article is part of the Research Topic Advances in Tumor Microenvironment, Immunology and Immunotherapy of Breast Cancer View all 9 articles
Globally, breast cancer stands as the most prevalent form of cancer among women. The tumor microenvironment of breast cancer often exhibits hypoxia. Hypoxia-inducible factor 1-alpha, a transcription factor, is found to be overexpressed and activated in breast cancer, playing a pivotal role in the anoxic microenvironment by mediating a series of reactions. Hypoxia-inducible factor 1-alpha is involved in regulating downstream pathways and target genes, which are crucial in hypoxic conditions, including glycolysis, angiogenesis, and metastasis. These processes significantly contribute to breast cancer progression by managing cancer-related activities linked to tumor invasion, metastasis, immune evasion, and drug resistance, resulting in poor prognosis for patients. Consequently, there is a significant interest in Hypoxia-inducible factor 1-alpha as a potential target for cancer therapy. Presently, research on drugs targeting Hypoxia-inducible factor 1-alpha is predominantly in the preclinical phase, highlighting the need for an in-depth understanding of HIF-1α and its regulatory pathway. It is anticipated that the future will see the introduction of effective HIF-1α inhibitors into clinical trials, offering new hope for breast cancer patients. Therefore, this review focuses on the structure and function of HIF-1α, its role in advancing breast cancer, and strategies to combat HIF-1α-dependent drug resistance, underlining its therapeutic potential.
Graphical Abstract Schematic overview of HIF-1a regulation in breast cancer. Under normoxic conditions, HIF-1α is unstable with a brief half-life. In hypoxic conditions, the depletion of molecular oxygen during mitochondrial oxidative phosphorylation curtails the catalytic functions of PHD and FIH, limiting HIF-1α hydroxylation and degradation, thereby activating the HIF pathway. Subsequently, HIF-1α is involved in angiogenesis, glucose metabolism, cancer cell invasion and metastasis, and immune escape by regulating the expression of target genes.
Breast cancer (BC) is the most common type of malignancy among women and the second leading cause of cancer-related deaths among women after lung cancer (1–3). The treatment of breast cancer mainly includes surgery, endocrine therapy, chemotherapy, radiotherapy, and targeted therapy, depending on the classification of the tumor, with drug therapy playing an important role. In earlier years, the mortality rate of breast cancer patients has declined due to the reduced risk of the disease, improved treatment methods, and the widespread use of early screening (4). However, the emergence of drug resistance during treatment in recent years has posed a severe challenge to the survival of breast cancer patients (5). Hypoxia, caused by an imbalance between oxygen consumption and supply due to rapid tumor growth, is a common feature of the tumor microenvironment in most solid tumors (4, 6). It promotes tumor growth, metastasis, and treatment resistance by regulating the expression of hypoxia-related genes, ultimately leading to more aggressive and fatal cancers. Hypoxia is associated with enhanced invasive behavior and poorer prognosis and has been identified as a poor indicator of patient outcome (7). The hypoxia-inducible factor (HIF) family, which plays a pivotal role in the cellular response to hypoxic stress, consists of transcription factors that are crucial for managing hypoxic stress at the cellular level (8, 9). Research has consistently demonstrated that Hypoxia-inducible factor 1-alpha (HIF-1α) is overexpressed in numerous types of cancer, significantly influencing cancer progression (10–16). HIF-1α is responsible for activating genes associated with angiogenesis, cell growth and survival, invasion and metastasis, glucose metabolism, immune system evasion, and resistance to several cancer therapies. Specifically, HIF-1α is involved in regulating tumor cell metabolism, apoptosis, and autophagy, thereby impacting their survival (8, 17–22). It represents a promising target for anticancer therapy. Therefore, this article provides a detailed description of the structure and function of HIF-1α as well as its mechanism of action in the development of breast cancer. This review also summarizes the reasons for the emergence of HIF-1α-dependent drug resistance and strategies to overcome it, providing systematic information for the development of targeted drugs against HIF-1α.
Hypoxia significantly influences numerous pathophysiological conditions in the human body (1). It is a defining characteristic of the solid tumor microenvironment (TME), resulting from rapid tumor growth and inadequate blood supply. An estimated 50%–60% of tumors exhibit anoxic regions (23). Hypoxia is linked to cancer spread and resistance to conventional therapies like chemotherapy and radiotherapy, indicating a grim prognosis for various cancers, including BC, hepatocellular carcinoma (HCC), and cancers of the pancreas, stomach, and colorectum (24). Therefore, targeting hypoxia is seen as a viable strategy in cancer treatment. Cells have evolved sophisticated mechanisms to adapt to anoxic conditions (25).
Within the hypoxic TME, HIF plays a crucial role in tumor adaptation (26). To date, mammals have been found to express three HIF types. HIF is a heterodimer consisting of an oxygen-sensitive α subunit (HIF-1α, HIF-2α, and HIF-3α) and an oxygen-insensitive β subunit (HIF-1β), crucial for regulating gene expression under hypoxic conditions (27–29). While oxygen levels regulate all three HIF α subunits and they all bind to HIF-1β, research has primarily focused on HIF-1α and HIF-2α (29–34). HIF-1α and HIF-2α share structural similarities, yet their roles differ across tumor and cell types (29, 35, 36). HIF-3α has been less studied due to its multiple variants and complex functionality ( (37, 38). Recent research indicates that specific HIF-1α subtypes are present in several solid tumors, potentially contributing to tumor progression (1, 39).
HIF-1 consists of the HIF-1α subunit, composed of 826 amino acids, and the HIF-1β subunit, which has 782 amino acids (Figure 1) (40, 41). HIF-1α is the most extensively expressed subunit of HIF-1 in mammalian tissues (8). This transcription factor, encoded by the HIF-1α gene on chromosome 14q2124, responds to hypoxic signals (42, 43). It is part of the basic helix–loop–helix (bHLH)/Period Clock Protein (Per)–Aryl Hydrocarbon Receptor Nuclear Translocator (ARNT)–Single-minded Protein (Sim) (bHLH/PAS) family of transcription factors (44). The bHLH and PAS domains, named after the proteins Per, ARNT, and Sim (40) first identified in Drosophila, are essential for DNA binding and the formation of heterodimers between HIF-1α and HIF-1β, respectively (45). HIF-1α includes two transactivation domains (TAD): N-TAD and C-TAD, enriched with acidic and hydrophobic amino acids, linked by an inhibitory domain (ID) (42). C-TAD plays a role in HIF-1α transcription regulation through interaction with the transcriptional coactivator CREB-binding protein (CBP)/p300 in hypoxic conditions, while N-TAD serves as its stable regulator (46, 47). The ID, situated between the two TAD sequences (amino acids 576–785), prevents transcriptional activation by TADs (48). Furthermore, HIF-1α features an oxygen-dependent degradation domain (ODDD) upstream of the N-TAD region, including two hydroxylation sites, Pro-402 and Pro-564, each bearing a conserved LXXLAP motif (49). This ODDD, located centrally in HIF-1α, chiefly mediates the protein’s oxygen-regulated stability and degradation through the ubiquitin-proteasome pathway (50, 51). HIF-1α also possesses two nuclear localization signals (NLS), NLSN (N-terminal, 17–33 amino acids) and NLSC (C-terminal, 718–721 amino acids) (45). HIF-1β, also known as ARNT, is expressed constitutively in all cell types and its expression is not influenced by oxygen levels (52). It includes three domains: bHLH, PAS, and C-TAD, but lacks the ODDD and N-TAD domains (45, 53).
Figure 1 Schematic of hypoxia-inducible factor HIF-1α protein structure and hydroxylation sites at proline and asparagine residues. The basic submotif and the helix-loop-helix domain (bHLH) are located near the N terminus, followed by the Per-ARNT-Sim (PAS) domain. The PAS domain comprises repetitive amino acid sequences PAS-A and PAS-B. The oxygen-dependent degradation domain (ODDD) overlaps with the N-terminal transactivation domain (N-TAD), followed by the C-terminal transactivation domain (C-TAD). Hydroxylation of proline residues within the ODDD and of asparagine residues within the C-TAD of HIF-1α are highlighted. The non-equilibrium hydroxylation by the prolylhydroxylases (PHD) and the asparagine hydroxylase factor inhibiting HIF-1 including substrates and products is depicted exemplarily for two of the three hydroxylation sites of HIF-1α.
Within cells, the regulation of HIF-1α is stringently dependent on oxygen availability, in contrast to HIF-1β, which is constantly expressed irrespective of oxygen tension (40, 51). Under normoxic conditions, HIF-1α is unstable with a brief half-life (Figures 1, 2) (40). Pro-402 and Pro-564 in the ODDD and Asn-803 in the C-TAD are hydroxylated by prolyl hydroxylases (PHD) and factor-inhibiting HIF (FIH) using Fe2+ and 2-oxoglutaric acid as cofactors (40, 51). Hydroxylation at Asn-803 inhibits CBP/p300 binding to HIF-1α, while hydroxylation at proline residues allows the von Hippel-Lindau tumor suppressor protein (pVHL), with E3 ubiquitin ligase activity, to recognize hydroxylated HIF-1α (42, 54). Consequently, HIF-1α is ubiquitinated and swiftly degraded through the pVHL-mediated ubiquitin-proteasome pathway. The second regulation mechanism involves the hydroxylation of asparaginyl residues by FIH (Figures 1, 2) (55), preventing the association of HIF coactivators (CBP/p300) (55, 56). This asparaginyl hydroxylation occurs in the C-TAD domain at N803 in HIF-1α and N851 in HIF-2α (57). Thus, under normoxic conditions, PHD and FIH facilitate HIF degradation through a dual mechanism, suppressing HIF transcriptional activity (58). In hypoxic conditions, the depletion of molecular oxygen during mitochondrial oxidative phosphorylation curtails the catalytic functions of PHD and FIH (Figure 2) (57, 59), limiting HIF-1α hydroxylation and degradation, thereby activating the HIF pathway (60, 61). The stable HIF-1α then moves to the nucleus and forms a transcriptionally active heterodimer with HIF-1β (29). The interaction occurs between the heterodimeric complex of HIF-1α/HIF-1β and CBP/p300, the steroid receptor coactivator-1 family of coactivators, the nuclear redox regulator Ref-1, and the molecular chaperone heat shock protein 90. This interaction facilitates the binding of hypoxia response elements (HRE), leading to increased transcriptional activity of target genes across various signaling pathways and the regulation of cellular adaptive responses to hypoxia (Table 1) (29, 78, 79). However, the degradation of accumulated HIF-1α happens rapidly upon reoxygenation of hypoxic cells, with the rate of degradation being dependent on the duration of hypoxic stress (80).
Figure 2 Schema of regulation of HIF-α degradation and transcriptional activity. Under normoxic conditions, HIF-1α is continuously degraded through the key oxygen sensor PHD, which enables HIF-1α to bind to VHL. Under hypoxic conditions, the hydroxylation of HIF - 1α is inhibited, leading to stabilization of HIF-1α. Next, HIF-1α dimerizes with HIF-1β to form a transcriptional activation complex, which binds to HRE and stimulates the transactivation of target genes.
BC cells necessitate a continual blood supply for oxygen and essential nutrients (81). Initially, during tumor growth, nutrients and oxygen are obtained via diffusion (82). However, as the tumor mass reaches a certain size, diffusion becomes insufficient to sustain growth, prompting the formation of new vasculature to support tumor growth and metastasis (81, 83). Thus, angiogenesis is critical for tumor advancement, proliferation, and metastasis. Tumor blood vessels exhibit high tortuosity, increased vascular permeability, and sluggish blood flow (62)compared to normal vessels, leading to increased local hypoxia, which in turn stabilizes HIF-1α, fostering tumor invasion and metastasis (84–87)
HIF plays a pivotal role in regulating angiogenesis (Figure 3) (88, 89). BC cells exhibit elevated levels of HIF, stimulating gene expression that facilitates proliferation, metastasis, angiogenesis, and invasion (90, 91). HIF-1α is notably expressed in precursor lesions and early stages of BC (92). Suppression of the HIF-1α gene or inhibition of its transcription can hinder tumor cells from secreting vascular endothelial growth factor (VEGF) and impede tumor neovascularization (8, 93, 94).
Figure 3 The mechanisms and pathways of HIF-1α overexpression effects on the induction of angiogenesis genes. HIF-1α translation is amplified by PI3K, RAS, and NF-κβ pathways in the cytoplasm, and then it can pass into the nucleus with coactivator (P300) as a transcription factor and enhances the expression of some essential angiogenesis genes like FLT1, MMP9, VEGF, VEGFR, and PAI-1 in the angiogenesis process.
HIF-1α shows increased expression particularly in triple-negative BC (TNBC). The nuclear factor kappa B (NF-κB) signaling pathway is activated in TNBC, promoting tumor cell proliferation, angiogenesis, and evasion of apoptosis (Figure 3) (95). Tumor necrosis factor-alpha (TNF-α) or interleukin (IL)-1 activates inhibitory kappa B kinase (IKKB), leading to NF-Kb activation (96). Additionally, under chronic hypoxia in TNBC cell lines, reactive oxygen species (ROS) further activate NF-κB by degrading inhibitor of κB-α (IκBα) (97). The NF-κB pathway boosts HIF-1α mRNA expression by enhancing its transcription (98, 99). TNF-α may elevate HIF-1α expression by activating NF-κB signaling in TNBC cells and could be influenced by IL-17 (100).
In BC, HIF-1α primarily induces angiogenesis by regulating the expression of VEGF, hepatocyte growth factor (HGF), vascular cell adhesion molecule 1 (VCAM1), and VEGF receptor (VEGFR) (Figure 3) (62, 101). VEGF, a critical downstream target of the HIF pathway, drives angiogenesis by influencing endothelial cell migration, proliferation, permeability, and survival (102–105). Under hypoxic conditions, HIF-1α binds to the VEGF promoter, significantly increasing VEGF mRNA levels in TNBC compared to other BC subtypes (106). HIF-1α also upregulates breast tumor kinase mRNA and protein expression, which stimulates angiogenesis via hepatocyte growth factor (107). Furthermore, HIF-1α induces overexpression of C1q binding protein (C1QBP), indirectly stimulating the NF-κB signaling cascade to upregulate VCAM1 expression and promote TNBC angiogenesis (74). Short-term hyperoxia induces ROS formation, leading to increased brain-derived neurotrophic factor expression and VEGFR receptor upregulation through HIF-1α, promoting angiogenesis (108).
Although HIF-1α predominates in BC, the HIF-2α isoform is equally crucial as a key regulator of pathophysiological angiogenesis (109). RAB11B-AS1, a long noncoding RNA (lncRNA), enhances VEGFA and angiopoietin-like 4 (ANGPTL4) expression in hypoxic BC cells in a HIF-2α-dependent manner, promoting tumor angiogenesis and metastasis (110). In conclusion, angiogenesis is a multifaceted process, and HIF-1α significantly contributes to angiogenesis in BC (62, 111).
Enhanced glucose metabolism plays a vital role in the growth and division of cancer cells, which require significant amounts of biomass and alternate energy sources to offset the diminished oxidative phosphorylation. The activity of HIF is believed to be intimately associated with glucose metabolism. Genes activated by HIF transcription include crucial components of glucose metabolism, such as glucose transporters (GLUT1 and GLUT3), enzymes involved in the glycolytic pathway (hexokinase, phosphofructokinase, aldolase, glyceraldehyde 3-phosphate dehydrogenase, phosphoglycerate kinase, enolase, pyruvate kinase, and lactate dehydrogenase [LDH]), and pyruvate dehydrogenase (PDH) kinase (PDK) (Figure 4) (28, 55, 112, 113). Du et al. investigated the potential roles and mechanisms of the hypoxic MIR210HG axis, finding that MIR210HG increases HIF-1α protein levels by directly interacting with the 5’-UTR of HIF1α mRNA. This elevation in HIF-1α protein enhances the expression of enzymes related to glycolysis (pyruvate kinase M2, LDHA) and GLUT1 (114). In hypoxic conditions, HIF-1α facilitates the shift of tumor cells from oxidative to glycolytic metabolism by triggering genes that encode glucose transporters and glycolytic enzymes (115). In particular, the overexpression of HIF-1α in glucose augments glucose absorption in tumor cells through the elevation of glucose transporter levels. The modification of proteins by O-linked β-N-acetylglucosamine (O-GlcNAc) (O-GlcNAcylation) influences glycolysis in BC cells via the HIF-1α/GLUT1 signaling pathway (81, 116). Glucose is processed through the glycolytic pathway in tumor cells, generating substantial amounts of pyruvate (117). Hexokinase 2 (HK2) serves as both the initial and rate-limiting enzyme in the glycolysis pathway (81). CircRNF20 is found to accelerate tumor progression by targeting miR-487a/HIF-1α/HK2 in BC (73). HIF-1α enhances PDK activity and blocks the transformation of pyruvate to acetyl-CoA by inhibiting PDH; thus, reducing the entry of pyruvate into the tricarboxylic acid cycle (55, 118). LDHA converts pyruvate into lactic acid, which is then transported out of the cell by the monocarboxylate transporter (MCT) (62). As cancer cell metabolism shifts to aerobic glycolysis, lactate supplants pyruvate and is expelled into the tumor microenvironment (TME), thereby fostering an immunosuppressive milieu that supports tumor cell proliferation, metastasis, and invasion (119, 120).
Figure 4 The involved procedure of HIF-1α in cancer glucose metabolism. HIF-1 enhances the expression of glucose transporters GLUT1 and GLUT3 and activates glycolytic enzymes, including hexokinase 2 (HK2), phosphofructokinase (PFK-L), pyruvate kinase isozymes M2 (PKM2) to generate an increasing amount of pyruvate. After this process, pyruvate is largely converted to lactate by lactate dehydrogenase A (LDHA) and removed from the cancer cell by monocarboxylate transporter (MCT). HIF-1 also inhibits the TCA cycle and oxidative phosphorylation process by activating the expression of HIF-1-dependent pyruvate dehydrogenase kinase (PDK), resulting in the decrease of mitochondrial activities and the oxygen consumption in hypoxia.
Glycolysis can lead to an increase in HIF-1α levels, which in turn raises VEGF expression (121). Aerobic glycolysis stimulates angiogenesis by producing lactate, which acidifies the extracellular environment and enhances VEGF expression (122). Additionally, the end products of glycolysis, lactate and pyruvate, influence VEGF expression through the augmentation of HIF-1α levels (123–125). Hence, it is postulated that the transition to glycolysis precedes angiogenesis. In summary, HIF-1α plays a crucial role in regulating glucose metabolism in BC.
Invasion and metastasis involve the spread of cancer cells from the primary tumor site to distant organs, leading to the formation of secondary tumors (81). Early estimates indicate that nearly two-thirds of cancer-related deaths and three-quarters of BC-related deaths result from metastasis (126). EMT, a process in which epithelial cells convert into mesenchymal cells through specific mechanisms, is a key aspect of tumor metastasis (127, 128). Cancer cells that undergo EMT exhibit enhanced invasive capabilities and resistance to apoptosis. Often, EMT is induced by hypoxia, with HIF-1α overexpression linked to various molecules and pathways (Figure 5) (76, 129–132). Research has shown that hypoxia-induced HIF-1α expression leads to the activation of major transcription factors such as TWIST, Snail, Slug, SIP1, STAT3, and ZEB. This activation results in E-cadherin suppression and vimentin induction in BC, with HIF-1α inhibition markedly increasing E-cadherin levels (133). While E-cadherin encourages collective migration of mixed E/M phenotypes by inhibiting TGF-β, TGF-β activation promotes single-cell migration (134). CSF-1, a regulator of EMT, is influenced by hypoxia. HIF-1α induces a mixed E/M phenotype via its target gene CSF-1, facilitating collective migration (135). Additionally, hypoxia has been shown to increase Slug and Snail expression and decrease E-cadherin levels during HIF1-induced EMT through the Notch pathway (136). HIF-1α activates matrix metalloproteinases 1, 2, 9, and 14, aiding in the breakdown of extracellular matrix components and basement membrane degradation, thereby easing cancer cell migration and spread (75, 113, 130, 137, 138). Chemokine receptors 4 (CXCR4) and 3 (CXCR3), associated with invasion, angiogenesis, metastasis, and prognosis, are upregulated by HIF-1-dependent expression, enhancing cell migration and survival during cycling (139, 140). HIF signaling impacts cell extravasation by modulating genes encoding L1 cell adhesion molecules and ANGPTL4, which reduces endothelial cell adhesion (141). He et al. (2020) showed that hypoxia-induced HIF-1α regulates BC cell migration and EMT through the MiR3383p/ZEB2 axis (142). Moon et al. identified MRPL52 as a transcriptional target of HIF-1α, with MRPL52 promoting EMT, migration, and invasion in hypoxic BC cells via the ROS-Notch1-Snail pathway (143).
Figure 5 Target genes regulated by HIF-1α. HIF-1α can regulate the expression level of lncRNA and form a mutual activation pathway with lncRNA, thereby promoting the production of EMT-TFs and promoting the process of tumor EMT. HIF-1α plays a key role in inducing the transcription of genes involved in invasion, metastasis, apoptosis, autophagy, and immune escape.
Recent research indicates that HIF-dependent lncRNAs may contribute to the metastatic phenotype of BC cells. Under hypoxia, lncRNA BCRT1, regulated by HIF-1α transcriptionally, promotes EMT (144). Liu et al. identified HIF-1α as a potential transcription factor for lncRNA HLA complex group 18 (HCG18), with a positive correlation between HCG18 and HIF-1α expression in BC tissue. Knockdown of HIF-1α reduced HCG18 levels in BC cells, and HIF-1α binding to specific HREs in the HCG18 promoter stimulates HCG18 expression. In vivo assays showed that decreasing HCG18 expression in MDA-MB-231 cells curbed tumor growth and lung metastasis in xenograft mouse models, highlighting HIF1α’s role as a critical regulator of hypoxia-induced EMT and metastasis (145). Metastasis poses a significant prognostic challenge in BC, and targeting HIF-1α to inhibit BC metastasis presents a viable strategy.
Apoptosis, a genetically controlled form of cell death, is crucial for normal cellular regulation. Cancer cells, however, often evade apoptosis, contributing to chemotherapy resistance or tumor relapse. This evasion involves a complex interplay of proteins and cytokines. Research indicates that HIF-1α exhibits a dual role in apoptosis, capable of both inducing and counteracting it (1). HIF-1α can induce and antagonize apoptosis (Figure 5). The proapoptotic effects of HIF-1α involve the regulation of genes such as BNIP, Bid, Bax, Bak, Bad, BNIP3, NIX, and NOXA (146), whereas its antiapoptotic effects are seen in the modulation of Bcl-2, Bcl-xL, and myeloid cell leukemia (Mcl-1) expression (1). In MDA-MB-231 cells treated with paclitaxel, a HIF-1α-dependent alteration in the expression of various pro- and antiapoptotic genes was observed. Under hypoxic conditions, compared to normoxic conditions with paclitaxel, a reduction in proapoptotic gene expression (BAK1, CASP3, CASP8, CASP10, and TNFRSF10A) was noted (147).
HIFs also play a significant role in autophagy, another programmed cell death mechanism (Figure 5). In MCF7 cells subjected to radiation, HIF-1α induces autophagy by inhibiting the PI3K/AKT/mTOR/p70 pathway and increases the expression of Mcl-1 and BNIP-3 (148). Mcl-1 participates in the neutralization of proapoptotic proteins, inhibiting cytochrome c release from mitochondria (149), while BNIP-3, a mitochondrial protein in the Bcl-2 family, triggers selective mitophagy by releasing Beclin-1 to initiate autophagy (150, 151). The interplay between autophagy and apoptosis is crucial, especially for inducing cell death in antiapoptotic BC cell lines (152).
Cancer-associated fibroblasts (CAFs) interact with tumor cells to promote tumor cell growth and metastasis. CAFs, mainly normal interstitial fibroblasts (NFs), are the most abundant cell type in the stroma of breast cancer (153, 154). In breast cancer tumors, only a small fraction of fibroblasts are quiescent; these fibroblasts are responsible for the structural integrity of the extracellular matrix (ECM) and its nutrient supply and contribute to wound healing (155). However, most fibroblasts exhibit an activated phenotype characterized by producing various extracellular matrix components and paracrine mediators (156). Among the many mechanisms involved in transforming NFs to CAFs, local hypoxia has been proven to drive the differentiation of NFs into activated myofibroblasts by triggering the formation of reactive oxygen species (ROS) (157, 158). Additionally, CAF activation is reversible: chronic hypoxia inactivates CAFs, leading to the loss of contractility, reduction of surrounding extracellular matrix remodeling, and ultimately damage CAF-mediated cancer cell invasion (159). Studies have shown that hypoxia-dependent deletion of PHD2 suppresses tumor growth and reduces the metastatic activity of CAFs (160, 161). Hypoxia inhibited prolyl hydroxylase domain protein 2 (PHD2), resulting in the stabilization of HIF-1α, reduced expression of αSMA and periostin, and decreased myosin II activity. Treatment with the PHD inhibitor DMOG in an orthotopic breast cancer model significantly reduced spontaneous metastasis to the lungs and liver, which correlated with decreased tumor stiffness and fibroblast activation (159). Another study revealed that the loss of PHD2 is associated with normalization of the vasculature, reduced CAF activation, and decreased intravascular invasion of metastases (161). These findings suggest that blocking PHD2 in CAFs may be a novel strategy for inhibiting prometastatic signals in the breast cancer tumor microenvironment.
In fact, CAFs have been shown to regulate metabolic interdependency between cancer cells and their surrounding microenvironment through the action of HIF (162). HIF-1α is involved in regulating biventricular metabolic symbiosis between synthetic metabolic cancer cells and catabolic stromal fibroblasts (163). The enhanced glycolytic rate shown by CAFs is partially dependent on HIF signaling (164). Furthermore, the high-energy metabolic byproducts produced by catabolic CAFs are taken up by tumor cells to support their high anabolic demands. The increased metabolic flux in cancer cells generates ROS, which then propagate throughout the tumor microenvironment and through CAFs to promote HIF-dependent metabolic reprogramming (165).
Tumor immune escape, where cancer cells avoid detection and destruction by the host immune system (166), is facilitated under hypoxic conditions through HIF-1α overexpression(Figure 5) (167). HIFs increase CD47 immunoglobulin expression and hinder T cell proliferation and activation by attracting myeloid-derived suppressor cells (28). Changes in the expression of CD47, CD73, and PDL1 in TNBC cells treated with chemotherapy agents like carboplatin, doxorubicin, gemcitabine, or paclitaxel enhance cancer cells’ ability to evade both innate and adaptive immune responses (168). Increased PDL1 mRNA expression in human TNBC cell lines was linked to elevated HIF-1α expression due to endoplasmic reticulum oxidoreductase 1-α (ERO1-α), with ERO1-α knockdown significantly reducing PDL1-mediated T-cell apoptosis, suggesting avenues for therapeutic intervention in hypoxia-mediated immune resistance (169).
Regulatory T cells (Tregs), which suppress immune responses through cytokines and metabolites, play a role in tumor development (170–173). In TNBC, HIF-1α modulates Tregs’ immunosuppressive functions and aggregation by regulating forkhead box P3 (FoxP3) and C-X-C motif CXCR4. HIF-1α enhances FoxP3 expression by binding to HREs and indirectly increases CXCR4 expression; thus, supporting immunosuppression (174). Moreover, M2 macrophages, known for their immunosuppressive capabilities through IL-10 and TGF-β release, are influenced by HIF-1α (175). This factor drives the polarization of tumor-associated macrophages (TAMs) towards an M2 phenotype, creating an immunosuppressive microenvironment. It has been shown that TAMs in TNBC are more likely to adopt an M2 phenotype compared to other BC subtypes (176, 177). HIF-1α, present in BRCA1-IRIS overexpressing TNBC cells, secretes high levels of granulocyte-macrophage colony-stimulating factor (GM-CSF), recruiting and polarizing macrophages towards an M2 phenotype; thus, facilitating immune escape (178, 179). HIF-1α’s ability to polarize TAM to M2 by regulating GM-CSF and macrophage CSF-1 in TNBC underscores its role in promoting immune evasion. HIF can also lead to extracellular acidification by regulating the expression of MCT4, which not only diminishes immune response efficiency but also impacts the efficacy of anticancer drugs (180).
Based on their genomic locations, long noncoding RNAs (lncRNAs) are categorized into five types: antisense, sense, intergenic, intronic, or bidirectional. The location of lncRNAs can be specific to the nucleus, the cytoplasm, or both (25, 181). The expression of lncRNAs is regulated similarly to that of protein-coding RNAs, through mechanisms such as epigenetic modification, gene transcription, and post-transcriptional regulation (182). In tumors, lncRNAs are often abnormally expressed and play roles in the regulation of tumor proliferation, invasion, metastasis, metabolism, angiogenesis, and survival (183, 184). Certain noncoding RNAs can influence tumor-related biological processes by regulating HIF-1α (Figure 6) (25, 185, 186). For instance, LINC00649 enhances the stability of HIF-1α mRNA and protein expression by interacting with the nuclear factor 90 (NF90)/NF45 complex (187). MIR210HG specifically impacts triple-negative breast cancer (TNBC) by regulating HIF-1α at the translation level, thereby increasing HIF-1α protein expression and influencing the expression of glycolysis genes (114). MicroRNAs (miRNAs) exert a dual regulatory effect on HIF-1α, both promoting and inhibiting its expression. Saatci et al. identified eight common miRNAs that target HIF-1α, lysyl oxidase (LOX), and ITGA5, with miR-142–3p and miR-128–3p showing negative correlation with the expression of HIF-1α, LOX, and ITGA5. Hypoxia can inhibit miR-142–3p, leading to increased HIF-1α mRNA and protein expression, activating the FAK/Src signaling pathway, and triggering chemotherapy resistance in TNBC (188). However, the regulatory mechanism of miR-142–3p on HIF-1α remains unclear. Overexpression of miR-101 decreases VHL levels, stabilizing HIF-1α and inducing VEGFA expression, ultimately enhancing TNBC invasiveness (189). Thus, noncoding RNA regulates HIF-1α expression in TNBC by maintaining the stability of the HIF-1α protein and regulating the stability and translation level of HIF-1α mRNA.
In BC treatment options vary by subtype and include surgery, endocrine therapy, chemotherapy, radiotherapy, and targeted therapy, with drug therapy playing a crucial role depending on the tumor classification. However, drug resistance presents a significant challenge in BC treatment (190–192), often due to inherent or acquired resistance over time (193). Hypoxia is a common characteristic of both primary and metastatic BC (194), with HIF-1α expression in tumor tissues associated with poor prognosis and drug resistance (Figure 7) (132). Research indicates that HIF-1α may contribute to resistance against conventional therapies via various signaling pathways, including drug efflux, tumor stem cell enrichment, autophagy, and apoptosis (192, 195), necessitating further investigation into HIF-1α-induced drug resistance mechanisms in BC.
Figure 7 HIF-1α-mediated stemness and drug resistance. On the one hand, HIF-1α can induce drug resistance by regulating stem cell surface markers. On the other hand, HIF-1α promotes chemotherapy resistance through drug resistance-related proteins.
Drug efflux transporters such as MDR1, multidrug resistance-associated protein 1 (MRP1), and breast cancer resistance protein (BCRP) are directly regulated by HIF-1α (Figure 7), encoded by the ATP-binding cassette (ABC) transporters ABCB1, ABCC1, and ABCG2, respectively. Their promoters contain HREs sensitive to HIF-1α transcriptional regulation (196). The link between these transporters and drug resistance in BC has been extensively studied (197–199), with all three proteins inducing drug resistance by facilitating drug efflux from tumor cells. Studies have shown that hypoxia-induced MDR1 expression can be significantly reduced by inhibiting HIF-1 expression with antisense oligonucleotides (200).In a separate investigation, 41% of BC tumors exhibited increased levels of MDR1, leading to a threefold higher likelihood of chemotherapy failure (201). Taxanes and anthracyclines represent the primary chemotherapy agents for BC treatment. The upregulation of MDR1, also referred to as P-glycoprotein or Pgp, contributes to resistance against taxanes and anthracyclines (202). The activation of HIF-1α enhances the resistance of BC cells to these drugs (147, 203, 204). The genes ABCC1 and ABCG2, which encode MRP1 and BCRP, respectively, possess hypoxia response elements (HREs) upstream of their coding sequences. The deletion of these elements can prevent hypoxia-induced activation (196, 205). MRP1 is elevated in cells with activated HIF-1α, and this effect can be reversed by HIF-1α siRNA, indicating that ABCC1 is a direct target of HIF-1α. Another investigation discovered that HIF-1α binds to the HRE region of the BCRP promoter in LTLTCa cells, with significantly increased binding observed in the presence of CoCl2 (206). The expression of BCRP correlated with the degree of drug resistance to irinotecan and topotecan (207). Hence, it is proposed that the expression and stability of HIF-1α can enhance the mRNA and protein levels of MDR1, MRP1, and BRCP, thereby contributing to HIF-1α-mediated drug resistance.
Autophagy has been shown to increase resistance to endocrine and cytotoxic drugs in BC (208–210). HIF-1α can activate various molecular mechanisms, such as inducing PTP-PEST expression and activating AMPK, which in turn increases BNIP3 expression. This disrupts the interaction between Beclin-1 and Bcl-2 and induces autophagy, thereby increasing BC cells’ resistance to drugs like adriamycin (211, 212). Additionally, endocrine therapies, including tamoxifen and flurvist, have been found to increase autophagy markers in drug-resistant BC cells (213, 214). Thus, HIF-1α plays a role in inducing BC resistance to both endocrine and cytotoxic drugs by upregulating autophagy.
Apoptosis, leading to cell death, is a crucial outcome of most cancer treatments. Cancer cells, however, have developed mechanisms to evade apoptosis, contributing to resistance to chemotherapy or recurrence of tumors (215). This evasion involves various proteins and cytokines, including the Bcl-2 family, apoptosis inhibitor proteins, and the caspase family, along with cytochrome c and proteases (146). HIF-1α plays a direct role in the regulation of apoptosis, exhibiting both proapoptotic and antiapoptotic effects (146). The proapoptotic actions of HIF-1α include the downregulation of proapoptotic members of the Bcl-2 family, such as BNIP3, NIX, and NOXA, while its antiapoptotic effects involve increasing the levels of antiapoptotic proteins like Bcl-2, Bcl-xL, and Mcl-1, and decreasing the levels of proapoptotic proteins such as Bid, Bax, and Bak (216–218). In breast cancer (BC) cells, alterations in apoptotic activity following HIF-1α activation are associated with increased drug resistance (147, 219, 220), though the precise mechanisms warrant further investigation.
Stem cells play a significant role in BC, driving tumor progression and metastasis, and displaying inherent resistance to chemotherapy and radiotherapy (221, 222). These cells can enhance drug efflux, increase drug metabolism in the tumor, activate survival pathways like Notch and Hedgehog, and dampen apoptosis signaling, all contributing to chemoresistance (223). HIF-1α directly upregulates genes such as NANOG, SOX2, KLF4, and OCT4, which inhibit differentiation and maintain a stem cell-like phenotype (203, 224). Furthermore, HIF-1α supports the survival of breast cancer stem cells (BCSC) by inducing ROS-dependent expression of HIF-1α and HIF-2α, leading to HIF-mediated expression of IL-6, IL-8, and MDR1. Exposure of MDA-MB-231, SUM-149, and SUM-159 cells to paclitaxel increased the percentage of ALDH+ cells, indicative of stem cell characteristics, by twelvefold in vitro and in vivo (203). Therefore, HIF-1α activation promotes the proliferation and enrichment of tumor stem cells, contributing to drug resistance.
To overcome HIF-1α-dependent drug resistance, strategies involve directly or indirectly targeting HIF-1α with inhibitors (225). Direct inhibitors of HIF-1α target protein-protein/DNA interactions, impacting DNA binding, transcriptional activity of HIF-1α, heterodimerization with HIF-1β, and interactions with transcriptional coactivators (226–230). Indirect inhibitors aim to downregulate HIF-1α transcription or translation, reduce HIF-1α stability, or prevent its degradation, offering potential pathways to mitigate drug resistance in cancer treatment.
Numerous studies have highlighted the potential of HIF-1α inhibitors and compounds targeting the HIF-1α pathway, demonstrating their effectiveness in vitro (62, 81)(Table 2). For instance, treatment of MDA-MB-231 cells with the PHD inhibitor molidustat, which stabilizes HIF, resulted in reduced viability, growth, clone formation, cell cycle arrest, and increased chemosensitivity, indicating potential anticancer activity of HIF (249) Among 68 newly synthesized arylformamide compounds, compound 30 m showed the most potent inhibitory activity against HIF-1α with minimal cytotoxicity, effectively reducing hypoxia-induced HIF-1α protein accumulation (81, 250). However, clinical trials have failed to show the effect of HIF-1α inhibitors in BC treatment (81, 251)(Table 3). Preliminary data published in 2013 showed some clinical efficacy of digoxin in patients with stages I–III BC. However, the treatment window of the drug is narrow; serum levels exceeding 1–2 nM produce significant side effects. So far, no follow-up data are available (192, 252). The differential expression of HIF-1α and HIF-1α inhibitor monotherapy may be the factors limiting the efficacy of anti-HIF-1α therapy (1). Yu et al. discussed the lack of clinical effectiveness of HIF-1α inhibitors (230). First, although both HIF-1α and HIF-2α are involved in cancer progression, most inhibitors have targeted only HIF-1α. Therefore, the role of HIF-2α in drug resistance needs to be understood. Second, patient selection contributes to the success of clinical trials. Accuracy in the measurement of tumor HIF-1α may help to better distinguish responders from non-responders. Finally, although hypoxia varies between and within different BC subtypes, evidence suggests that the hypoxia of the patient’s tumor itself is different (253). This heterogeneity may be the main determinant of the overall drug response. Considering the limitations of HIF-1α inhibitors, a combination of HIF-1α inhibitors with chemotherapeutic drugs or other agents may improve outcomes. A preclinical study indicated that digoxin enhances the sensitivity of triple-negative breast cancer cell lines to paclitaxel and gemcitabine in vivo (203). Interestingly, molidustat has been found to enhance the cytotoxicity of gemcitabine in MDA-MB-231 cells (249). However, currently, the related combination therapy of BC is in the preclinical stage, and thus, a clear conclusion cannot be drawn. The latest HIF-1α drug delivery system is based on nanocarriers that can improve targeting specificity, overcome solubility problems, reduce drug toxicity, and maintain safe drug concentrations. Furthermore, the mode of drug administration also affects the efficacy of HIF-1α-related drugs and should be investigated in future research (81). In conclusion, the results on BC cell lines show that aspects, such as comparison of HIF-1α and HIF-2α inhibition, double vs. single isomer inhibition, different statuses of hormone receptors, metastasis, and other unexplored issues, should be considered. Thus, we need to understand the role of HIFs in BC before targeting them for clinical application (55).
HIF-1α, an important transcription factor under hypoxic conditions, plays a crucial role in the growth and development of the body as well as various physiological and pathological processes. HIF-1α induces the expression of numerous genes related to angiogenesis, growth and survival, invasion and metastasis, glucose metabolism, epithelial–mesenchymal transition (EMT), immune evasion, and resistance to various cancer treatments. The overexpression of hypoxia-inducible factor-1α (HIF-1α) is also closely related to drug resistance and prognosis in breast cancer patients. Therefore, regulating the activity of HIF-1α may be a breakthrough in treating many diseases. Upregulating the activity of HIF-1α can increase cell survival under hypoxic conditions and enhance angiogenesis in hypoxic tissues. Conversely, HIF-1α inhibitors can block angiogenesis and reduce the survival rate of hypoxic or inflammatory tissues. This article elaborates on the structure and function of HIF-1α, its mechanism of action in developing breast cancer, and drug resistance mechanisms. This review also summarizes strategies to overcome HIF-1α-dependent drug resistance and the current status of targeted HIF-1α therapy for breast cancer.
However, the complex interactions among multiple pathways involving HIF-1α pose greater challenges for its clinical application as an inhibitor. A deeper understanding of the intricate interactions between oxygen tension, the microenvironment, receptor expression, and HIF-1α expression is needed. This not only facilitates the development of new drug combinations but also aids in the discovery of novel drug targets for breast cancer treatment. Currently, drugs targeting HIF-1α are mainly focused on preclinical research, and their actual clinical effects, patient tolerance, dosing regimens, and other factors require further evaluation and validation. Additionally, drug delivery and efficacy are limited by factors such as tumor acidosis, hypoxic microenvironments, and elevated interstitial fluid pressure within tumors. Therefore, there is a need to develop more suitable drug carriers and delivery systems to enhance therapeutic outcomes. In conclusion, more comprehensive and in-depth research is required on HIF-1α and the pathways it mediates. Although current clinical trials have not yet demonstrated satisfactory results for HIF-1α inhibitors as monotherapy in breast cancer treatment, with continuous and in-depth research on the role of HIF-1α in cancer development, it is believed that targeted HIF-1α therapeutics will bring new hope to breast cancer patients in the near future.
ShiZ: Validation, Writing – original draft. CC: Validation, Writing – review & editing. HH: Visualization, Writing – review & editing. ZZ: Supervision, Writing – review & editing. FZ: Conceptualization, Funding acquisition, Writing – review & editing. ShuZ: Conceptualization, Funding acquisition, Writing – review & editing.
The author(s) declare financial support was received for the research, authorship, and/or publication of this article. This research was supported by the Sichuan Science and Technology Program (2022YFS0623) and the Natural Science Foundation of Southwest Medical University (2022QN079).
The authors declare that the research was conducted in the absence of any commercial or financial relationships that could be construed as a potential conflict of interest.
All claims expressed in this article are solely those of the authors and do not necessarily represent those of their affiliated organizations, or those of the publisher, the editors and the reviewers. Any product that may be evaluated in this article, or claim that may be made by its manufacturer, is not guaranteed or endorsed by the publisher.
1. Yong L, Tang S, Yu H, Zhang H, Zhang Y, Wan Y, et al. The role of hypoxia-inducible factor-1 alpha in multidrug-resistant breast cancer. Front Oncol. (2022) 12:964934. doi: 10.3389/fonc.2022.964934
2. Siegel RL, Miller KD, Fuchs HE, Jemal A. Cancer statistics, 2021. CA Cancer J Clin. (2021) 71:7–33. doi: 10.3322/caac.21654
3. Shamis SAK, McMillan DC, Edwards J. The relationship between hypoxia-inducible factor 1α (Hif-1α) and patient survival in breast cancer: systematic review and meta-analysis. Crit Rev Oncol Hematol. (2021) 159:103231. doi: 10.1016/j.critrevonc.2021.103231
4. Munoz D, Near AM, van Ravesteyn NT, Lee SJ, Schechter CB, Alagoz O, et al. Effects of screening and systemic adjuvant therapy on er-specific us breast cancer mortality. J Natl Cancer Inst. (2014) 106:1–9. doi: 10.1093/jnci/dju289
5. Kaminska K, Akrap N, Staaf J, Alves CL, Ehinger A, Ebbesson A, et al. Distinct mechanisms of resistance to fulvestrant treatment dictate level of er independence and selective response to cdk inhibitors in metastatic breast cancer. Breast Cancer Res. (2021) 23:26. doi: 10.1186/s13058–021-01402–1
6. Vaupel P, Schlenger K, Knoop C, Höckel M. Oxygenation of human tumors: evaluation of tissue oxygen distribution in breast cancers by computerized O2 tension measurements. Cancer Res. (1991) 51:3316–22.
7. Wang W, He YF, Sun QK, Wang Y, Han XH, Peng DF, et al. Hypoxia-inducible factor 1α in breast cancer prognosis. Clin Chim Acta. (2014) 428:32–7. doi: 10.1016/j.cca.2013.10.018
8. Li RL, He LY, Zhang Q, Liu J, Lu F, Duan HX, et al. Hif-1α Is a potential molecular target for herbal medicine to treat diseases. Drug Des Devel Ther. (2020) 14:4915–49. doi: 10.2147/dddt.S274980
9. Semenza GL, Wang GL. A nuclear factor induced by hypoxia via de novo protein synthesis binds to the human erythropoietin gene enhancer at a site required for transcriptional activation. Mol Cell Biol. (1992) 12:5447–54. doi: 10.1128/mcb.12.12.5447–5454.1992
10. Rashid M, Zadeh LR, Baradaran B, Molavi O, Ghesmati Z, Sabzichi M, et al. Up-down regulation of hif-1α in cancer progression. Gene. (2021) 798:145796. doi: 10.1016/j.gene.2021.145796
11. LeCouter J, Kowalski J, Foster J, Hass P, Zhang Z, Dillard-Telm L, et al. Identification of an angiogenic mitogen selective for endocrine gland endothelium. Nature. (2001) 412:877–84. doi: 10.1038/35091000
12. Chen C, Pore N, Behrooz A, Ismail-Beigi F, Maity A. Regulation of glut1 mrna by hypoxia-inducible factor-1. Interaction between H-ras and hypoxia. J Biol Chem. (2001) 276:9519–25. doi: 10.1074/jbc.M010144200
13. Ben-Yosef Y, Lahat N, Shapiro S, Bitterman H, Miller A. Regulation of endothelial matrix metalloproteinase-2 by hypoxia/reoxygenation. Circ Res. (2002) 90:784–91. doi: 10.1161/01.RES.0000015588.70132.DC
14. Krishnamachary B, Berg-Dixon S, Kelly B, Agani F, Feldser D, Ferreira G, et al. Regulation of colon carcinoma cell invasion by hypoxia-inducible factor 1. Cancer Res. (2003) 63:1138–43.
15. Balamurugan K. Hif-1 at the crossroads of hypoxia, inflammation, and cancer. Int J Cancer. (2016) 138:1058–66. doi: 10.1002/ijc.29519
16. Semenza GL. Defining the role of hypoxia-inducible factor 1 in cancer biology and therapeutics. Oncogene. (2010) 29:625–34. doi: 10.1038/onc.2009.441
17. Chen X, Liu J, He B, Li Y, Liu S, Wu B, et al. Vascular endothelial growth factor (Vegf) regulation by hypoxia inducible factor-1 alpha (Hif1a) starts and peaks during endometrial breakdown, not repair, in a mouse menstrual-like model. Hum Reprod. (2015) 30:2160–70. doi: 10.1093/humrep/dev156
18. Guru SK, Pathania AS, Kumar S, Ramesh D, Kumar M, Rana S, et al. Secalonic acid-D represses hif1α/vegf-mediated angiogenesis by regulating the akt/mtor/P70s6k signaling cascade. Cancer Res. (2015) 75:2886–96. doi: 10.1158/0008–5472.Can-14–2312
19. Ren QZ, Qian ZH, Jia SH, Xu ZZ. Vascular endothelial growth factor expression up-regulated by endometrial ischemia in secretory phase plays an important role in endometriosis. Fertil Steril. (2011) 95:2687–9. doi: 10.1016/j.fertnstert.2011.05.001
20. Jing SW, Wang YD, Kuroda M, Su JW, Sun GG, Liu Q, et al. Hif-1α Contributes to hypoxia-induced invasion and metastasis of esophageal carcinoma via inhibiting E-cadherin and promoting mmp-2 expression. Acta Med Okayama. (2012) 66:399–407. doi: 10.18926/amo/48964
21. Liu H, Zhang Z, Xiong W, Zhang L, Xiong Y, Li N, et al. Hypoxia-inducible factor-1α Promotes endometrial stromal cells migration and invasion by upregulating autophagy in endometriosis. Reproduction. (2017) 153:809–20. doi: 10.1530/rep-16–0643
22. Xiong Y, Liu Y, Xiong W, Zhang L, Liu H, Du Y, et al. Hypoxia-inducible factor 1α-induced epithelial-mesenchymal transition of endometrial epithelial cells may contribute to the development of endometriosis. Hum Reprod. (2016) 31:1327–38. doi: 10.1093/humrep/dew081
23. Rankin EB, Giaccia AJ. Hypoxic control of metastasis. Science. (2016) 352:175–80. doi: 10.1126/science.aaf4405
24. Bertout JA, Patel SA, Simon MC. The impact of O2 availability on human cancer. Nat Rev Cancer. (2008) 8:967–75. doi: 10.1038/nrc2540
25. Wang X, Zhao D, Xie H, Hu Y. Interplay of long non-coding rnas and hif-1α: A new dimension to understanding hypoxia-regulated tumor growth and metastasis. Cancer Lett. (2021) 499:49–59. doi: 10.1016/j.canlet.2020.11.007
26. Huang Y, Lin D, Taniguchi CM. Hypoxia inducible factor (Hif) in the tumor microenvironment: friend or foe? Sci China Life Sci. (2017) 60:1114–24. doi: 10.1007/s11427-017-9178-y
27. Prabhakar NR, Semenza GL. Adaptive and maladaptive cardiorespiratory responses to continuous and intermittent hypoxia mediated by hypoxia-inducible factors 1 and 2. Physiol Rev. (2012) 92:967–1003. doi: 10.1152/physrev.00030.2011
28. Schito L, Semenza GL. Hypoxia-inducible factors: master regulators of cancer progression. Trends Cancer. (2016) 2:758–70. doi: 10.1016/j.trecan.2016.10.016
29. Kheshtchin N, Hadjati J. Targeting hypoxia and hypoxia-inducible factor-1 in the tumor microenvironment for optimal cancer immunotherapy. J Cell Physiol. (2022) 237:1285–98. doi: 10.1002/jcp.30643
30. Wang V, Davis DA, Haque M, Huang LE, Yarchoan R. Differential gene up-regulation by hypoxia-inducible factor-1alpha and hypoxia-inducible factor-2alpha in hek293t cells. Cancer Res. (2005) 65:3299–306. doi: 10.1158/0008–5472.Can-04–4130
31. Zhang J, Qin Y, Martinez M, Flores-Bellver M, Rodrigues M, Dinabandhu A, et al. Hif-1α and hif-2α Redundantly promote retinal neovascularization in patients with ischemic retinal disease. J Clin Invest. (2021) 131:1–19. doi: 10.1172/jci139202
32. Koh MY, Lemos R Jr., Liu X, Powis G. The hypoxia-associated factor switches cells from hif-1α- to hif-2α-dependent signaling promoting stem cell characteristics, aggressive tumor growth and invasion. Cancer Res. (2011) 71:4015–27. doi: 10.1158/0008–5472.Can-10–4142
33. Downes NL, Laham-Karam N, Kaikkonen MU, Ylä-Herttuala S. Differential but complementary hif1α and hif2α Transcriptional regulation. Mol Ther. (2018) 26:1735–45. doi: 10.1016/j.ymthe.2018.05.004
34. Lin Q, Cong X, Yun Z. Differential hypoxic regulation of hypoxia-inducible factors 1alpha and 2alpha. Mol Cancer Res. (2011) 9:757–65. doi: 10.1158/1541–7786.Mcr-11–0053
35. Keith B, Johnson RS, Simon MC. Hif1α and hif2α: sibling rivalry in hypoxic tumour growth and progression. Nat Rev Cancer. (2011) 12:9–22. doi: 10.1038/nrc3183
36. Koh MY, Powis G. Passing the baton: the hif switch. Trends Biochem Sci. (2012) 37:364–72. doi: 10.1016/j.tibs.2012.06.004
37. Hara S, Hamada J, Kobayashi C, Kondo Y, Imura N. Expression and characterization of hypoxia-inducible factor (Hif)-3alpha in human kidney: suppression of hif-mediated gene expression by hif-3alpha. Biochem Biophys Res Commun. (2001) 287:808–13. doi: 10.1006/bbrc.2001.5659
38. Duan C. Hypoxia-inducible factor 3 biology: complexities and emerging themes. Am J Physiol Cell Physiol. (2016) 310:C260–9. doi: 10.1152/ajpcell.00315.2015
39. Cowman SJ, Koh MY. Revisiting the hif switch in the tumor and its immune microenvironment. Trends Cancer. (2022) 8:28–42. doi: 10.1016/j.trecan.2021.10.004
40. Ghosh R, Samanta P, Sarkar R, Biswas S, Saha P, Hajra S, et al. Targeting hif-1α by natural and synthetic compounds: A promising approach for anti-cancer therapeutics development. Molecules. (2022) 27:1–37. doi: 10.3390/molecules27165192
41. Wang GL, Semenza GL. Purification and characterization of hypoxia-inducible factor 1. J Biol Chem. (1995) 270:1230–7. doi: 10.1074/jbc.270.3.1230
42. Zeng CY, Wang XF, Hua FZ. Hif-1α in osteoarthritis: from pathogenesis to therapeutic implications. Front Pharmacol. (2022) 13:927126. doi: 10.3389/fphar.2022.927126
43. Fernández-Torres J, Martínez-Nava GA, Gutiérrez-Ruíz MC, Gómez-Quiroz LE, Gutiérrez M. Role of hif-1α Signaling pathway in osteoarthritis: A systematic review. Rev Bras Reumatol Engl Ed. (2017) 57:162–73. doi: 10.1016/j.rbre.2016.07.008
44. Kewley RJ, Whitelaw ML, Chapman-Smith A. The mammalian basic helix-loop-helix/pas family of transcriptional regulators. Int J Biochem Cell Biol. (2004) 36:189–204. doi: 10.1016/s1357–2725(03)00211–5
45. Xu R, Wang F, Yang H, Wang Z. Action sites and clinical application of hif-1α Inhibitors. Molecules. (2022) 27:1–14. doi: 10.3390/molecules27113426
46. Schmid T, Zhou J, Brüne B. Hif-1 and P53: communication of transcription factors under hypoxia. J Cell Mol Med. (2004) 8:423–31. doi: 10.1111/j.1582-4934.2004.tb00467.x
47. Li ZL, Wang B, Lv LL, Tang TT, Wen Y, Cao JY, et al. Fih-1-modulated hif-1α C-tad promotes acute kidney injury to chronic kidney disease progression via regulating klf5 signaling. Acta Pharmacol Sin. (2021) 42:2106–19. doi: 10.1038/s41401–021-00617–4
48. Jiang BH, Zheng JZ, Leung SW, Roe R, Semenza GL. Transactivation and inhibitory domains of hypoxia-inducible factor 1alpha. Modulation of transcriptional activity by oxygen tension. J Biol Chem. (1997) 272:19253–60. doi: 10.1074/jbc.272.31.19253
49. Ke Q, Costa M. Hypoxia-inducible factor-1 (Hif-1). Mol Pharmacol. (2006) 70:1469–80. doi: 10.1124/mol.106.027029
50. Bruick RK, McKnight SL. A conserved family of prolyl-4-hydroxylases that modify hif. Science. (2001) 294:1337–40. doi: 10.1126/science.1066373
51. Huang LE, Gu J, Schau M, Bunn HF. Regulation of hypoxia-inducible factor 1alpha is mediated by an O2-dependent degradation domain via the ubiquitin-proteasome pathway. Proc Natl Acad Sci U.S.A. (1998) 95:7987–92. doi: 10.1073/pnas.95.14.7987
52. Rocha S. Gene regulation under low oxygen: holding your breath for transcription. Trends Biochem Sci. (2007) 32:389–97. doi: 10.1016/j.tibs.2007.06.005
53. Infantino V, Santarsiero A, Convertini P, Todisco S, Iacobazzi V. Cancer cell metabolism in hypoxia: role of hif-1 as key regulator and therapeutic target. Int J Mol Sci. (2021) 22:1–20. doi: 10.3390/ijms22115703
54. Lee JW, Bae SH, Jeong JW, Kim SH, Kim KW. Hypoxia-inducible factor (Hif-1)Alpha: its protein stability and biological functions. Exp Mol Med. (2004) 36:1–12. doi: 10.1038/emm.2004.1
55. Kozal K, Krześlak A. The role of hypoxia-inducible factor isoforms in breast cancer and perspectives on their inhibition in therapy. Cancers (Basel). (2022) 14:1–20. doi: 10.3390/cancers14184518
56. Malkov MI, Lee CT, Taylor CT. Regulation of the hypoxia-inducible factor (Hif) by pro-inflammatory cytokines. Cells. (2021) 10:1–15. doi: 10.3390/cells10092340
57. Keeley TP, Mann GE. Defining physiological normoxia for improved translation of cell physiology to animal models and humans. Physiol Rev. (2019) 99:161–234. doi: 10.1152/physrev.00041.2017
58. Rani S, Roy S, Singh M, Kaithwas G. Regulation of transactivation at C-tad domain of hif-1α by factor-inhibiting hif-1α (Fih-1): A potential target for therapeutic intervention in cancer. Oxid Med Cell Longev. (2022) 2022:2407223. doi: 10.1155/2022/2407223
59. Yang H, Huang J, Mao Y, Wang L, Li R, Ha C. Vitexin alleviates interleukin-1β-induced inflammatory responses in chondrocytes from osteoarthritis patients: involvement of hif-1α Pathway. Scand J Immunol. (2019) 90:e12773. doi: 10.1111/sji.12773
60. Hagen T, Taylor CT, Lam F, Moncada S. Redistribution of intracellular oxygen in hypoxia by nitric oxide: effect on hif1alpha. Science. (2003) 302:1975–8. doi: 10.1126/science.1088805
61. Singh M, Devi U, Roy S, Gupta PS, Saraf SA, Kaithwas G. Prolyl hydroxylase mediated inhibition of fatty acid synthase to combat tumor growth in mammary gland carcinoma. Breast Cancer. (2016) 23:820–9. doi: 10.1007/s12282–016-0683–6
62. Liu Q, Guan C, Liu C, Li H, Wu J, Sun C. Targeting hypoxia-inducible factor-1alpha: A new strategy for triple-negative breast cancer therapy. BioMed Pharmacother. (2022) 156:113861. doi: 10.1016/j.biopha.2022.113861
63. Ko YS, Rugira T, Jin H, Joo YN, Kim HJ. Radiotherapy-resistant breast cancer cells enhance tumor progression by enhancing premetastatic niche formation through the hif-1α-lox axis. Int J Mol Sci. (2020) 21:1–14. doi: 10.3390/ijms21218027
64. Crowder SW, Balikov DA, Hwang YS, Sung HJ. Cancer stem cells under hypoxia as a chemoresistance factor in breast and brain. Curr Pathobiol Rep. (2014) 2:33–40. doi: 10.1007/s40139–013-0035–6
65. Lan J, Lu H, Samanta D, Salman S, Lu Y, Semenza GL. Hypoxia-inducible factor 1-dependent expression of adenosine receptor 2b promotes breast cancer stem cell enrichment. Proc Natl Acad Sci U.S.A. (2018) 115:E9640–e8. doi: 10.1073/pnas.1809695115
66. Krock BL, Skuli N, Simon MC. Hypoxia-induced angiogenesis: good and evil. Genes Cancer. (2011) 2:1117–33. doi: 10.1177/1947601911423654
67. Ozretic P, Alvir I, Sarcevic B, Vujaskovic Z, Rendic-Miocevic Z, Roguljic A, et al. Apoptosis regulator bcl-2 is an independent prognostic marker for worse overall survival in triple-negative breast cancer patients. Int J Biol Markers. (2018) 33:109–15. doi: 10.5301/ijbm.5000291
68. Lee SH, Golinska M, Griffiths JR. Hif-1-independent mechanisms regulating metabolic adaptation in hypoxic cancer cells. Cells. (2021) 10:1–25. doi: 10.3390/cells10092371
69. Lock FE, McDonald PC, Lou Y, Serrano I, Chafe SC, Ostlund C, et al. Targeting carbonic anhydrase ix depletes breast cancer stem cells within the hypoxic niche. Oncogene. (2013) 32:5210–9. doi: 10.1038/onc.2012.550
70. Jin W. Role of jak/stat3 signaling in the regulation of metastasis, the transition of cancer stem cells, and chemoresistance of cancer by epithelial-mesenchymal transition. Cells. (2020) 9:1–23. doi: 10.3390/cells9010217
71. Srivastava N, Usmani SS, Subbarayan R, Saini R, Pandey PK. Hypoxia: syndicating triple negative breast cancer against various therapeutic regimens. Front Oncol. (2023) 13:1199105. doi: 10.3389/fonc.2023.1199105
72. Ma S, Zhao Y, Lee WC, Ong LT, Lee PL, Jiang Z, et al. Hypoxia induces hif1α-dependent epigenetic vulnerability in triple negative breast cancer to confer immune effector dysfunction and resistance to anti-pd-1 immunotherapy. Nat Commun. (2022) 13:4118. doi: 10.1038/s41467–022-31764–9
73. Cao L, Wang M, Dong Y, Xu B, Chen J, Ding Y, et al. Circular rna circrnf20 promotes breast cancer tumorigenesis and warburg effect through mir-487a/hif-1α/hk2. Cell Death Dis. (2020) 11:145. doi: 10.1038/s41419–020-2336–0
74. Wu H, Chu Y, Sun S, Li G, Xu S, Zhang X, et al. Hypoxia-mediated complement 1q binding protein regulates metastasis and chemoresistance in triple-negative breast cancer and modulates the pkc-nf-Kb-vcam-1 signaling pathway. Front Cell Dev Biol. (2021) 9:607142. doi: 10.3389/fcell.2021.607142
75. Goggins E, Kakkad S, Mironchik Y, Jacob D, Wildes F, Krishnamachary B, et al. Hypoxia inducible factors modify collagen I fibers in mda-mb-231 triple negative breast cancer xenografts. Neoplasia. (2018) 20:131–9. doi: 10.1016/j.neo.2017.11.010
76. Storci G, Sansone P, Mari S, D’Uva G, Tavolari S, Guarnieri T, et al. Tnfalpha up-regulates slug via the nf-kappab/hif1alpha axis, which imparts breast cancer cells with a stem cell-like phenotype. J Cell Physiol. (2010) 225:682–91. doi: 10.1002/jcp.22264
77. Yang MH, Wu MZ, Chiou SH, Chen PM, Chang SY, Liu CJ, et al. Direct regulation of twist by hif-1alpha promotes metastasis. Nat Cell Biol. (2008) 10:295–305. doi: 10.1038/ncb1691
78. Weidemann A, Johnson RS. Biology of hif-1alpha. Cell Death Differ. (2008) 15:621–7. doi: 10.1038/cdd.2008.12
79. Semenza GL. Oxygen sensing, hypoxia-inducible factors, and disease pathophysiology. Annu Rev Pathol. (2014) 9:47–71. doi: 10.1146/annurev-pathol-012513–104720
80. Berra E, Richard DE, Gothié E, Pouysségur J. Hif-1-dependent transcriptional activity is required for oxygen-mediated hif-1alpha degradation. FEBS Lett. (2001) 491:85–90. doi: 10.1016/s0014–5793(01)02159–7
81. Luo S, Jiang Y, Anfu Z, Zhao Y, Wu X, Li M, et al. Targeting hypoxia-inducible factors for breast cancer therapy: A narrative review. Front Pharmacol. (2022) 13:1064661. doi: 10.3389/fphar.2022.1064661
82. Hanahan D, Weinberg RA. The hallmarks of cancer. Cell. (2000) 100:57–70. doi: 10.1016/s0092–8674(00)81683–9
83. Hanahan D, Folkman J. Patterns and emerging mechanisms of the angiogenic switch during tumorigenesis. Cell. (1996) 86:353–64. doi: 10.1016/s0092–8674(00)80108–7
84. Potente M, Gerhardt H, Carmeliet P. Basic and therapeutic aspects of angiogenesis. Cell. (2011) 146:873–87. doi: 10.1016/j.cell.2011.08.039
85. Rey S, Schito L, Wouters BG, Eliasof S, Kerbel RS. Targeting hypoxia-inducible factors for antiangiogenic cancer therapy. Trends Cancer. (2017) 3:529–41. doi: 10.1016/j.trecan.2017.05.002
86. Rapisarda A, Melillo G. Overcoming disappointing results with antiangiogenic therapy by targeting hypoxia. Nat Rev Clin Oncol. (2012) 9:378–90. doi: 10.1038/nrclinonc.2012.64
87. Viallard C, Larrivée B. Tumor angiogenesis and vascular normalization: alternative therapeutic targets. Angiogenesis. (2017) 20:409–26. doi: 10.1007/s10456–017-9562–9
88. Hashimoto T, Shibasaki F. Hypoxia-inducible factor as an angiogenic master switch. Front Pediatr. (2015) 3:33. doi: 10.3389/fped.2015.00033
89. Olejarz W, Kubiak-Tomaszewska G, Chrzanowska A, Lorenc T. Exosomes in angiogenesis and anti-angiogenic therapy in cancers. Int J Mol Sci. (2020) 21:1–25. doi: 10.3390/ijms21165840
90. Badodekar N, Sharma A, Patil V, Telang G, Sharma R, Patil S, et al. Angiogenesis induction in breast cancer: A paracrine paradigm. Cell Biochem Funct. (2021) 39:860–73. doi: 10.1002/cbf.3663
91. Bos R, Zhong H, Hanrahan CF, Mommers EC, Semenza GL, Pinedo HM, et al. Levels of hypoxia-inducible factor-1 alpha during breast carcinogenesis. J Natl Cancer Inst. (2001) 93:309–14. doi: 10.1093/jnci/93.4.309
92. Maxwell PH, Dachs GU, Gleadle JM, Nicholls LG, Harris AL, Stratford IJ, et al. Hypoxia-inducible factor-1 modulates gene expression in solid tumors and influences both angiogenesis and tumor growth. Proc Natl Acad Sci U.S.A. (1997) 94:8104–9. doi: 10.1073/pnas.94.15.8104
93. Baldewijns MM, Thijssen VL, Van den Eynden GG, Van Laere SJ, Bluekens AM, Roskams T, et al. High-grade clear cell renal cell carcinoma has a higher angiogenic activity than low-grade renal cell carcinoma based on histomorphological quantification and qrt-pcr mrna expression profile. Br J Cancer. (2007) 96:1888–95. doi: 10.1038/sj.bjc.6603796
94. Ryan HE, Poloni M, McNulty W, Elson D, Gassmann M, Arbeit JM, et al. Hypoxia-inducible factor-1alpha is a positive factor in solid tumor growth. Cancer Res. (2000) 60:4010–5.
95. Pires BRB, Binato R, Ferreira GM, Cecchini R, Panis C, Abdelhay E. Nf-kappab regulates redox status in breast cancer subtypes. Genes (Basel). (2018) 9:1–21. doi: 10.3390/genes9070320
96. Lawrence T. The nuclear factor nf-kappab pathway in inflammation. Cold Spring Harb Perspect Biol. (2009) 1:a001651. doi: 10.1101/cshperspect.a001651
97. Culver C, Sundqvist A, Mudie S, Melvin A, Xirodimas D, Rocha S. Mechanism of hypoxia-induced nf-kappab. Mol Cell Biol. (2010) 30:4901–21. doi: 10.1128/mcb.00409–10
98. Liang S, Chen Z, Jiang G, Zhou Y, Liu Q, Su Q, et al. Activation of gper suppresses migration and angiogenesis of triple negative breast cancer via inhibition of nf-Kb/il-6 signals. Cancer Lett. (2017) 386:12–23. doi: 10.1016/j.canlet.2016.11.003
99. Jin Y, Huynh DTN, Myung CS, Heo KS. Ginsenoside rh1 prevents migration and invasion through mitochondrial ros-mediated inhibition of stat3/nf-Kb signaling in mda-mb-231 cells. Int J Mol Sci. (2021) 22:1–16. doi: 10.3390/ijms221910458
100. Changchun K, Pengchao H, Ke S, Ying W, Lei W. Interleukin-17 augments tumor necrosis factor A-mediated increase of hypoxia-inducible factor-1α and inhibits vasodilator-stimulated phosphoprotein expression to reduce the adhesion of breast cancer cells. Oncol Lett. (2017) 13:3253–60. doi: 10.3892/ol.2017.5825
101. Darbeheshti F, Mahdiannasser M, Noroozi Z, Firoozi Z, Mansoori B, Daraei A, et al. Circular rna-associated cerna network involved in hif-1 signalling in triple-negative breast cancer: circ_0047303 as a potential key regulator. J Cell Mol Med. (2021) 25:11322–32. doi: 10.1111/jcmm.17066
102. Semenza GL. Hif-1: using two hands to flip the angiogenic switch. Cancer Metastasis Rev. (2000) 19:59–65. doi: 10.1023/a:1026544214667
103. Schoppmann SF, Fenzl A, Schindl M, Bachleitner-Hofmann T, Nagy K, Gnant M, et al. Hypoxia inducible factor-1alpha correlates with vegf-C expression and lymphangiogenesis in breast cancer. Breast Cancer Res Treat. (2006) 99:135–41. doi: 10.1007/s10549–006-9190–3
104. Saponaro C, Malfettone A, Ranieri G, Danza K, Simone G, Paradiso A, et al. Vegf, hif-1α Expression and mvd as an angiogenic network in familial breast cancer. PloS One. (2013) 8:e53070. doi: 10.1371/journal.pone.0053070
105. Ahluwalia A, Tarnawski AS. Critical role of hypoxia sensor–hif-1α in vegf gene activation. Implications for angiogenesis and tissue injury healing. Curr Med Chem. (2012) 19:90–7. doi: 10.2174/092986712803413944
106. Hait NC, Maiti A, Xu P, Qi Q, Kawaguchi T, Okano M, et al. Regulation of hypoxia-inducible factor functions in the nucleus by sphingosine-1-phosphate. FASEB J. (2020) 34:4293–310. doi: 10.1096/fj.201901734RR
107. Regan Anderson TM, Ma SH, Raj GV, Cidlowski JA, Helle TM, Knutson TP, et al. Breast tumor kinase (Brk/ptk6) is induced by hif, glucocorticoid receptor, and pelp1-mediated stress signaling in triple-negative breast cancer. Cancer Res. (2016) 76:1653–63. doi: 10.1158/0008–5472.Can-15–2510
108. Tiron A, Ristescu I, Postu PA, Tiron CE, Zugun-Eloae F, Grigoras I. Long-term deleterious effects of short-term hyperoxia on cancer progression-is brain-derived neurotrophic factor an important mediator? An experimental study. Cancers (Basel). (2020) 12:1–18. doi: 10.3390/cancers12030688
109. de Heer EC, Jalving M, Harris AL. Hifs, angiogenesis, and metabolism: elusive enemies in breast cancer. J Clin Invest. (2020) 130:5074–87. doi: 10.1172/jci137552
110. Niu Y, Bao L, Chen Y, Wang C, Luo M, Zhang B, et al. Hif2-induced long noncoding rna rab11b-as1 promotes hypoxia-mediated angiogenesis and breast cancer metastasis. Cancer Res. (2020) 80:964–75. doi: 10.1158/0008–5472.Can-19–1532
111. Pang RW, Poon RT. Clinical implications of angiogenesis in cancers. Vasc Health Risk Manag. (2006) 2:97–108. doi: 10.2147/vhrm.2006.2.2.97
112. Wang L, Fan J, Yan CY, Ling R, Yun J. Activation of hypoxia-inducible factor-1α by prolonged in vivo hyperinsulinemia treatment potentiates cancerous progression in estrogen receptor-positive breast cancer cells. Biochem Biophys Res Commun. (2017) 491:545–51. doi: 10.1016/j.bbrc.2017.03.128
113. Muñoz-Nájar UM, Neurath KM, Vumbaca F, Claffey KP. Hypoxia stimulates breast carcinoma cell invasion through mt1-mmp and mmp-2 activation. Oncogene. (2006) 25:2379–92. doi: 10.1038/sj.onc.1209273
114. Du Y, Wei N, Ma R, Jiang SH, Song D. Long noncoding rna mir210hg promotes the warburg effect and tumor growth by enhancing hif-1α Translation in triple-negative breast cancer. Front Oncol. (2020) 10:580176. doi: 10.3389/fonc.2020.580176
115. Loftus RM, Finlay DK. Immunometabolism: cellular metabolism turns immune regulator. J Biol Chem. (2016) 291:1–10. doi: 10.1074/jbc.R115.693903
116. Ferrer CM, Lynch TP, Sodi VL, Falcone JN, Schwab LP, Peacock DL, et al. O-glcnacylation regulates cancer metabolism and survival stress signaling via regulation of the hif-1 pathway. Mol Cell. (2014) 54:820–31. doi: 10.1016/j.molcel.2014.04.026
117. Kozal K, Jóźwiak P, Krześlak A. Contemporary perspectives on the warburg effect inhibition in cancer therapy. Cancer Control. (2021) 28:10732748211041243. doi: 10.1177/10732748211041243
118. Lee P, Chandel NS, Simon MC. Cellular adaptation to hypoxia through hypoxia inducible factors and beyond. Nat Rev Mol Cell Biol. (2020) 21:268–83. doi: 10.1038/s41580-020-0227-y
119. Liberti MV, Locasale JW. The warburg effect: how does it benefit cancer cells? Trends Biochem Sci. (2016) 41:211–8. doi: 10.1016/j.tibs.2015.12.001
120. El-Sahli S, Wang L. Cancer stem cell-associated pathways in the metabolic reprogramming of breast cancer. Int J Mol Sci. (2020) 21:1–23. doi: 10.3390/ijms21239125
121. Zare ME, Kansestani AN, Hemmati S, Mansouri K, Vaisi-Raygani A. The rate of aerobic glycolysis is a pivotal regulator of tumor progression. J Diabetes Metab Disord. (2021) 20:523–31. doi: 10.1007/s40200–021-00774–7
122. Rastogi S, Mishra SS, Arora MK, Kaithwas G, Banerjee S, Ravichandiran V, et al. Lactate acidosis and simultaneous recruitment of tgf-B Leads to alter plasticity of hypoxic cancer cells in tumor microenvironment. Pharmacol Ther. (2023) 250:108519. doi: 10.1016/j.pharmthera.2023.108519
123. Shi Q, Le X, Wang B, Abbruzzese JL, Xiong Q, He Y, et al. Regulation of vascular endothelial growth factor expression by acidosis in human cancer cells. Oncogene. (2001) 20:3751–6. doi: 10.1038/sj.onc.1204500
124. Lu H, Forbes RA, Verma A. Hypoxia-inducible factor 1 activation by aerobic glycolysis implicates the warburg effect in carcinogenesis. J Biol Chem. (2002) 277:23111–5. doi: 10.1074/jbc.M202487200
125. Jung SY, Song HS, Park SY, Chung SH, Kim YJ. Pyruvate promotes tumor angiogenesis through hif-1-dependent pai-1 expression. Int J Oncol. (2011) 38:571–6. doi: 10.3892/ijo.2010.859
126. Dillekås H, Rogers MS, Straume O. Are 90% of deaths from cancer caused by metastases? Cancer Med. (2019) 8:5574–6. doi: 10.1002/cam4.2474
127. Lee JM, Dedhar S, Kalluri R, Thompson EW. The epithelial-mesenchymal transition: new insights in signaling, development, and disease. J Cell Biol. (2006) 172:973–81. doi: 10.1083/jcb.200601018
128. Lee K, Gjorevski N, Boghaert E, Radisky DC, Nelson CM. Snail1, snail2, and E47 promote mammary epithelial branching morphogenesis. EMBO J. (2011) 30:2662–74. doi: 10.1038/emboj.2011.159
129. Bao B, Azmi AS, Ali S, Ahmad A, Li Y, Banerjee S, et al. The biological kinship of hypoxia with csc and emt and their relationship with deregulated expression of mirnas and tumor aggressiveness. Biochim Biophys Acta. (2012) 1826:272–96. doi: 10.1016/j.bbcan.2012.04.008
130. Yang H, Geng YH, Wang P, Zhou YT, Yang H, Huo YF, et al. Extracellular atp promotes breast cancer invasion and epithelial-mesenchymal transition via hypoxia-inducible factor 2α Signaling. Cancer Sci. (2019) 110:2456–70. doi: 10.1111/cas.14086
131. Bao X, Zhang J, Huang G, Yan J, Xu C, Dou Z, et al. The crosstalk between hifs and mitochondrial dysfunctions in cancer development. Cell Death Dis. (2021) 12:215. doi: 10.1038/s41419–021-03505–1
132. Gilkes DM, Semenza GL. Role of hypoxia-inducible factors in breast cancer metastasis. Future Oncol. (2013) 9:1623–36. doi: 10.2217/fon.13.92
133. Zhou Z, Wang S, Song C, Hu Z. Paeoniflorin prevents hypoxia-induced epithelial-mesenchymal transition in human breast cancer cells. Onco Targets Ther. (2016) 9:2511–8. doi: 10.2147/ott.S102422
134. Cheng W, Xiao X, Liao Y, Cao Q, Wang C, Li X, et al. Conducive target range of breast cancer: hypoxic tumor microenvironment. Front Oncol. (2022) 12:978276. doi: 10.3389/fonc.2022.978276
135. Kai K, Iwamoto T, Zhang D, Shen L, Takahashi Y, Rao A, et al. Csf-1/csf-1r axis is associated with epithelial/mesenchymal hybrid phenotype in epithelial-like inflammatory breast cancer. Sci Rep. (2018) 8:9427. doi: 10.1038/s41598-018-27409-x
136. Chen J, Imanaka N, Chen J, Griffin JD. Hypoxia potentiates notch signaling in breast cancer leading to decreased E-cadherin expression and increased cell migration and invasion. Br J Cancer. (2010) 102:351–60. doi: 10.1038/sj.bjc.6605486
137. Hamad HA, Enezei HH, Alrawas A, Zakuan NM, Abdullah NA, Cheah YK, et al. Identification of potential chemical substrates as fuel for hypoxic tumors that may be linked to invadopodium formation in hypoxia-induced mda-mb-231 breast-cancer cell line. Molecules. (2020) 25:1–17. doi: 10.3390/molecules25173876
138. Choi JY, Jang YS, Min SY, Song JY. Overexpression of mmp-9 and hif-1α in breast cancer cells under hypoxic conditions. J Breast Cancer. (2011) 14:88–95. doi: 10.4048/jbc.2011.14.2.88
139. Lin Q, Fang X, Liang G, Luo Q, Cen Y, Shi Y, et al. Silencing ctnnd1 mediates triple-negative breast cancer bone metastasis via upregulating cxcr4/cxcl12 axis and neutrophils infiltration in bone. Cancers (Basel). (2021) 13:1–21. doi: 10.3390/cancers13225703
140. Todd VM, Vecchi LA 3rd, Clements ME, Snow KP, Ontko CD, Himmel L, et al. Hypoxia inducible factor signaling in breast tumors controls spontaneous tumor dissemination in a site-specific manner. Commun Biol. (2021) 4:1122. doi: 10.1038/s42003–021-02648–3
141. Zhang H, Wong CCL, Wei H, Gilkes DM, Korangath P, Chaturvedi P, et al. Correction: hif-1-dependent expression of angiopoietin-like 4 and L1cam mediates vascular metastasis of hypoxic breast cancer cells to the lungs. Oncogene. (2021) 40:1552–3. doi: 10.1038/s41388-020-01618-z
142. He J, Wang J, Li S, Li T, Chen K, Zhang S. Hypoxia-inhibited mir-338–3p suppresses breast cancer progression by directly targeting zeb2. Cancer Sci. (2020) 111:3550–63. doi: 10.1111/cas.14589
143. Moon EJ, Mello SS, Li CG, Chi JT, Thakkar K, Kirkland JG, et al. The hif target maff promotes tumor invasion and metastasis through il11 and stat3 signaling. Nat Commun. (2021) 12:4308. doi: 10.1038/s41467–021-24631–6
144. Liang Y, Song X, Li Y, Chen B, Zhao W, Wang L, et al. Lncrna bcrt1 promotes breast cancer progression by targeting mir-1303/ptbp3 axis. Mol Cancer. (2020) 19:85. doi: 10.1186/s12943–020-01206–5
145. Liu X, Qiao K, Zhu K, Li X, Zhao C, Li J, et al. Long noncoding rna hcg18 promotes Malignant phenotypes of breast cancer cells via the hcg18/mir-103a-3p/ube2o/mtorc1/hif-1α-positive feedback loop. Front Cell Dev Biol. (2021) 9:675082. doi: 10.3389/fcell.2021.675082
146. Sendoel A, Hengartner MO. Apoptotic cell death under hypoxia. Physiol (Bethesda). (2014) 29:168–76. doi: 10.1152/physiol.00016.2013
147. Flamant L, Notte A, Ninane N, Raes M, Michiels C. Anti-apoptotic role of hif-1 and ap-1 in paclitaxel exposed breast cancer cells under hypoxia. Mol Cancer. (2010) 9:191. doi: 10.1186/1476–4598-9–191
148. Zhong R, Xu H, Chen G, Zhao G, Gao Y, Liu X, et al. The role of hypoxia-inducible factor-1α in radiation-induced autophagic cell death in breast cancer cells. Tumour Biol. (2015) 36:7077–83. doi: 10.1007/s13277-015-3425-z
149. Michels J, Johnson PW, Packham G. Mcl-1. Int J Biochem Cell Biol. (2005) 37:267–71. doi: 10.1016/j.biocel.2004.04.007
150. Zhang H, Bosch-Marce M, Shimoda LA, Tan YS, Baek JH, Wesley JB, et al. Mitochondrial autophagy is an hif-1-dependent adaptive metabolic response to hypoxia. J Biol Chem. (2008) 283:10892–903. doi: 10.1074/jbc.M800102200
151. Wigerup C, Påhlman S, Bexell D. Therapeutic targeting of hypoxia and hypoxia-inducible factors in cancer. Pharmacol Ther. (2016) 164:152–69. doi: 10.1016/j.pharmthera.2016.04.009
152. Romero MA, Bayraktar Ekmekcigil O, Bagca BG, Avci CB, Sabitaliyevich UY, Zhenisovna TG, et al. Role of autophagy in breast cancer development and progression: opposite sides of the same coin. Adv Exp Med Biol. (2019) 1152:65–73. doi: 10.1007/978–3-030–20301-6_5
153. Polyak K, Weinberg RA. Transitions between epithelial and mesenchymal states: acquisition of Malignant and stem cell traits. Nat Rev Cancer. (2009) 9:265–73. doi: 10.1038/nrc2620
154. Zeisberg EM, Potenta S, Xie L, Zeisberg M, Kalluri R. Discovery of endothelial to mesenchymal transition as a source for carcinoma-associated fibroblasts. Cancer Res. (2007) 67:10123–8. doi: 10.1158/0008–5472.Can-07–3127
155. Aghakhani S, Silva-Saffar SE, Soliman S, Niarakis A. Hybrid computational modeling highlights reverse warburg effect in breast cancer-associated fibroblasts. Comput Struct Biotechnol J. (2023) 21:4196–206. doi: 10.1016/j.csbj.2023.08.015
156. Qiao A, Gu F, Guo X, Zhang X, Fu L. Breast cancer-associated fibroblasts: their roles in tumor initiation, progression and clinical applications. Front Med. (2016) 10:33–40. doi: 10.1007/s11684–016-0431–5
157. Comito G, Giannoni E, Di Gennaro P, Segura CP, Gerlini G, Chiarugi P. Stromal fibroblasts synergize with hypoxic oxidative stress to enhance melanoma aggressiveness. Cancer Lett. (2012) 324:31–41. doi: 10.1016/j.canlet.2012.04.025
158. Toullec A, Gerald D, Despouy G, Bourachot B, Cardon M, Lefort S, et al. Oxidative stress promotes myofibroblast differentiation and tumour spreading. EMBO Mol Med. (2010) 2:211–30. doi: 10.1002/emmm.201000073
159. Madsen CD, Pedersen JT, Venning FA, Singh LB, Moeendarbary E, Charras G, et al. Hypoxia and loss of phd2 inactivate stromal fibroblasts to decrease tumour stiffness and metastasis. EMBO Rep. (2015) 16:1394–408. doi: 10.15252/embr.201540107
160. Wottawa M, Leisering P, Ahlen M, Schnelle M, Vogel S, Malz C, et al. Knockdown of prolyl-4-hydroxylase domain 2 inhibits tumor growth of human breast cancer mda-mb-231 cells by affecting tgf-B1 processing. Int J Cancer. (2013) 132:2787–98. doi: 10.1002/ijc.27982
161. Kuchnio A, Dewerchin M, Carmeliet P. The phd2 oxygen sensor paves the way to metastasis. Oncotarget. (2015) 6:35149–50. doi: 10.18632/oncotarget.6216
162. Ju JA, Godet I, Ye IC, Byun J, Jayatilaka H, Lee SJ, et al. Hypoxia selectively enhances integrin A(5)B(1) receptor expression in breast cancer to promote metastasis. Mol Cancer Res. (2017) 15:723–34. doi: 10.1158/1541–7786.Mcr-16–0338
163. Chiavarina B, Martinez-Outschoorn UE, Whitaker-Menezes D, Howell A, Tanowitz HB, Pestell RG, et al. Metabolic reprogramming and two-compartment tumor metabolism: opposing role(S) of hif1α and hif2α in tumor-associated fibroblasts and human breast cancer cells. Cell Cycle. (2012) 11:3280–9. doi: 10.4161/cc.21643
164. Migneco G, Whitaker-Menezes D, Chiavarina B, Castello-Cros R, Pavlides S, Pestell RG, et al. Glycolytic cancer associated fibroblasts promote breast cancer tumor growth, without a measurable increase in angiogenesis: evidence for stromal-epithelial metabolic coupling. Cell Cycle. (2010) 9:2412–22. doi: 10.4161/cc.9.12.11989
165. Costa A, Scholer-Dahirel A, Mechta-Grigoriou F. The role of reactive oxygen species and metabolism on cancer cells and their microenvironment. Semin Cancer Biol. (2014) 25:23–32. doi: 10.1016/j.semcancer.2013.12.007
166. Prendergast GC, Metz R, Muller AJ. Towards a genetic definition of cancer-associated inflammation: role of the ido pathway. Am J Pathol. (2010) 176:2082–7. doi: 10.2353/ajpath.2010.091173
167. Wu Q, You L, Nepovimova E, Heger Z, Wu W, Kuca K, et al. Hypoxia-inducible factors: master regulators of hypoxic tumor immune escape. J Hematol Oncol. (2022) 15:77. doi: 10.1186/s13045–022-01292–6
168. Samanta D, Park Y, Ni X, Li H, Zahnow CA, Gabrielson E, et al. Chemotherapy induces enrichment of cd47(+)/cd73(+)/pdl1(+) immune evasive triple-negative breast cancer cells. Proc Natl Acad Sci U.S.A. (2018) 115:E1239–e48. doi: 10.1073/pnas.1718197115
169. Tanaka T, Kutomi G, Kajiwara T, Kukita K, Kochin V, Kanaseki T, et al. Cancer-Associated Oxidoreductase Ero1-A Promotes Immune Escape through up-Regulation of Pd-L1 in Human Breast Cancer. Oncotarget. (2017) 8:24706–18. doi: 10.18632/oncotarget.14960
170. Li C, Jiang P, Wei S, Xu X, Wang J. Regulatory T cells in tumor microenvironment: new mechanisms, potential therapeutic strategies and future prospects. Mol Cancer. (2020) 19:116. doi: 10.1186/s12943–020-01234–1
171. Vignali DA, Collison LW, Workman CJ. How regulatory T cells work. Nat Rev Immunol. (2008) 8:523–32. doi: 10.1038/nri2343
172. Oshi M, Asaoka M, Tokumaru Y, Angarita FA, Yan L, Matsuyama R, et al. Abundance of regulatory T cell (Treg) as a predictive biomarker for neoadjuvant chemotherapy in triple-negative breast cancer. Cancers (Basel). (2020) 12:1–16. doi: 10.3390/cancers12103038
173. Ohue Y, Nishikawa H. Regulatory T (Treg) cells in cancer: can treg cells be a new therapeutic target? Cancer Sci. (2019) 110:2080–9. doi: 10.1111/cas.14069
174. Yan M, Jene N, Byrne D, Millar EK, O’Toole SA, McNeil CM, et al. Recruitment of regulatory T cells is correlated with hypoxia-induced cxcr4 expression, and is associated with poor prognosis in basal-like breast cancers. Breast Cancer Res. (2011) 13:R47. doi: 10.1186/bcr2869
175. Zhang P, Liu G, Hu J, Chen S, Wang B, Peng P, et al. Tenascin-C can serve as an indicator for the immunosuppressive microenvironment of diffuse low-grade gliomas. Front Immunol. (2022) 13:824586. doi: 10.3389/fimmu.2022.824586
176. Reggiani F, Bertolini F. Gm-csf promotes a supportive adipose and lung microenvironment in metastatic breast cancer. Oncoscience. (2017) 4:126–7. doi: 10.18632/oncoscience.371
177. Ryan D, Sinha A, Bogan D, Davies J, Koziol J, ElShamy WM. A niche that triggers aggressiveness within brca1-iris overexpressing triple negative tumors is supported by reciprocal interactions with the microenvironment. Oncotarget. (2017) 8:103182–206. doi: 10.18632/oncotarget.20892
178. Chaturvedi P, Gilkes DM, Takano N, Semenza GL. Hypoxia-inducible factor-dependent signaling between triple-negative breast cancer cells and mesenchymal stem cells promotes macrophage recruitment. Proc Natl Acad Sci U.S.A. (2014) 111:E2120–9. doi: 10.1073/pnas.1406655111
179. Sami E, Paul BT, Koziol JA, ElShamy WM. The immunosuppressive microenvironment in brca1-iris-overexpressing tnbc tumors is induced by bidirectional interaction with tumor-associated macrophages. Cancer Res. (2020) 80:1102–17. doi: 10.1158/0008–5472.Can-19–2374
180. Mimeault M, Batra SK. Hypoxia-inducing factors as master regulators of stemness properties and altered metabolism of cancer- and metastasis-initiating cells. J Cell Mol Med. (2013) 17:30–54. doi: 10.1111/jcmm.12004
181. Kopp F, Mendell JT. Functional classification and experimental dissection of long noncoding rnas. Cell. (2018) 172:393–407. doi: 10.1016/j.cell.2018.01.011
182. Ransohoff JD, Wei Y, Khavari PA. The functions and unique features of long intergenic non-coding rna. Nat Rev Mol Cell Biol. (2018) 19:143–57. doi: 10.1038/nrm.2017.104
183. Slack FJ, Chinnaiyan AM. The role of non-coding rnas in oncology. Cell. (2019) 179:1033–55. doi: 10.1016/j.cell.2019.10.017
184. Lin HC, Yeh CC, Chao LY, Tsai MH, Chen HH, Chuang EY, et al. The hypoxia-responsive lncrna ndrg-ot1 promotes ndrg1 degradation via ubiquitin-mediated proteolysis in breast cancer cells. Oncotarget. (2018) 9:10470–82. doi: 10.18632/oncotarget.23732
185. Geismann C, Arlt A. Coming in the air: hypoxia meets epigenetics in pancreatic cancer. Cells. (2020) 9:1–13. doi: 10.3390/cells9112353
186. Liu J, Zhang X, Chen K, Cheng Y, Liu S, Xia M, et al. Ccr7 chemokine receptor-inducible lnc-dpf3 restrains dendritic cell migration by inhibiting hif-1α-mediated glycolysis. Immunity. (2019) 50:600–15.e15. doi: 10.1016/j.immuni.2019.01.021
187. Zhang J, Du C, Zhang L, Wang Y, Zhang Y, Li J. Lncrna linc00649 promotes the growth and metastasis of triple-negative breast cancer by maintaining the stability of hif-1α through the nf90/nf45 complex. Cell Cycle. (2022) 21:1034–47. doi: 10.1080/15384101.2022.2040283
188. Saatci O, Kaymak A, Raza U, Ersan PG, Akbulut O, Banister CE, et al. Targeting lysyl oxidase (Lox) overcomes chemotherapy resistance in triple negative breast cancer. Nat Commun. (2020) 11:2416. doi: 10.1038/s41467–020-16199–4
189. Liu N, Xia WY, Liu SS, Chen HY, Sun L, Liu MY, et al. Microrna-101 targets von hippel-lindau tumor suppressor (Vhl) to induce hif1α Mediated apoptosis and cell cycle arrest in normoxia condition. Sci Rep. (2016) 6:20489. doi: 10.1038/srep20489
190. McDonald ES, Clark AS, Tchou J, Zhang P, Freedman GM. Clinical diagnosis and management of breast cancer. J Nucl Med. (2016) 57 Suppl 1:9s–16s. doi: 10.2967/jnumed.115.157834
191. Nie C, Lv H, Bie L, Hou H, Chen X. Hypoxia-inducible factor 1-alpha expression correlates with response to neoadjuvant chemotherapy in women with breast cancer. Med (Baltimore). (2018) 97:e13551. doi: 10.1097/md.0000000000013551
192. McAleese CE, Choudhury C, Butcher NJ, Minchin RF. Hypoxia-mediated drug resistance in breast cancers. Cancer Lett. (2021) 502:189–99. doi: 10.1016/j.canlet.2020.11.045
193. Wang X, Zhang H, Chen X. Resistance CDJ癌. Drug Resistance Combating Drug Resistance Cancer. (2019) 2:20. doi: 10.20517/cdr
194. Vaupel P, Thews O, Hoeckel M. Treatment resistance of solid tumors: role of hypoxia and anemia. Med Oncol. (2001) 18:243–59. doi: 10.1385/mo:18:4:243
195. Jing X, Yang F, Shao C, Wei K, Xie M, Shen H, et al. Role of hypoxia in cancer therapy by regulating the tumor microenvironment. Mol Cancer. (2019) 18:157. doi: 10.1186/s12943–019-1089–9
196. Lv Y, Zhao S, Han J, Zheng L, Yang Z, Zhao L. Hypoxia-inducible factor-1α Induces multidrug resistance protein in colon cancer. Onco Targets Ther. (2015) 8:1941–8. doi: 10.2147/ott.S82835
197. Yan M, Wang J, Ren Y, Li L, He W, Zhang Y, et al. Over-expression of fsip1 promotes breast cancer progression and confers resistance to docetaxel via mrp1 stabilization. Cell Death Dis. (2019) 10:204. doi: 10.1038/s41419–018-1248–8
198. Jendželovský R, Jendželovská Z, Kuchárová B, Fedoročko P. Breast cancer resistance protein is the enemy of hypericin accumulation and toxicity of hypericin-mediated photodynamic therapy. BioMed Pharmacother. (2019) 109:2173–81. doi: 10.1016/j.biopha.2018.11.084
199. Yang X, Wang Y, Chen S, Zhang S, Cui C. Cetuximab-modified human serum albumin nanoparticles co-loaded with doxorubicin and mdr1 sirna for the treatment of drug-resistant breast tumors. Int J Nanomedicine. (2021) 16:7051–69. doi: 10.2147/ijn.S332830
200. Comerford KM, Wallace TJ, Karhausen J, Louis NA, Montalto MC, Colgan SP. Hypoxia-inducible factor-1-dependent regulation of the multidrug resistance (Mdr1) gene. Cancer Res. (2002) 62:3387–94.
201. Trock BJ, Leonessa F, Clarke R. Multidrug resistance in breast cancer: A meta-analysis of mdr1/gp170 expression and its possible functional significance. J Natl Cancer Inst. (1997) 89:917–31. doi: 10.1093/jnci/89.13.917
202. Robey RW, Pluchino KM, Hall MD, Fojo AT, Bates SE, Gottesman MM. Revisiting the role of abc transporters in multidrug-resistant cancer. Nat Rev Cancer. (2018) 18:452–64. doi: 10.1038/s41568–018-0005–8
203. Samanta D, Gilkes DM, Chaturvedi P, Xiang L, Semenza GL. Hypoxia-inducible factors are required for chemotherapy resistance of breast cancer stem cells. Proc Natl Acad Sci U.S.A. (2014) 111:E5429–38. doi: 10.1073/pnas.1421438111
204. Doublier S, Belisario DC, Polimeni M, Annaratone L, Riganti C, Allia E, et al. Hif-1 activation induces doxorubicin resistance in mcf7 3-D spheroids via P-glycoprotein expression: A potential model of the chemo-resistance of invasive micropapillary carcinoma of the breast. BMC Cancer. (2012) 12:4. doi: 10.1186/1471–2407-12–4
205. He X, Wang J, Wei W, Shi M, Xin B, Zhang T, et al. Hypoxia regulates abcg2 activity through the activivation of erk1/2/hif-1α and contributes to chemoresistance in pancreatic cancer cells. Cancer Biol Ther. (2016) 17:188–98. doi: 10.1080/15384047.2016.1139228
206. Kazi AA, Gilani RA, Schech AJ, Chumsri S, Sabnis G, Shah P, et al. Nonhypoxic regulation and role of hypoxia-inducible factor 1 in aromatase inhibitor resistant breast cancer. Breast Cancer Res. (2014) 16:R15. doi: 10.1186/bcr3609
207. Lee HJ, Choi CH. Characterization of sn38-resistant T47d breast cancer cell sublines overexpressing bcrp, mrp1, mrp2, mrp3, and mrp4. BMC Cancer. (2022) 22:446. doi: 10.1186/s12885-022-09446-y
208. Smith AG, Macleod KF. Autophagy, cancer stem cells and drug resistance. J Pathol. (2019) 247:708–18. doi: 10.1002/path.5222
209. Li X, Zhou Y, Li Y, Yang L, Ma Y, Peng X, et al. Autophagy: A novel mechanism of chemoresistance in cancers. BioMed Pharmacother. (2019) 119:109415. doi: 10.1016/j.biopha.2019.109415
210. Wu M, Zhang P. Egfr-mediated autophagy in tumourigenesis and therapeutic resistance. Cancer Lett. (2020) 469:207–16. doi: 10.1016/j.canlet.2019.10.030
211. Yu L, Shi Q, Jin Y, Liu Z, Li J, Sun W. Blockage of ampk-ulk1 pathway mediated autophagy promotes cell apoptosis to increase doxorubicin sensitivity in breast cancer (Bc) cells: an in vitro study. BMC Cancer. (2021) 21:195. doi: 10.1186/s12885-021-07901-w
212. Pan Y, Shao D, Zhao Y, Zhang F, Zheng X, Tan Y, et al. Berberine reverses hypoxia-induced chemoresistance in breast cancer through the inhibition of ampk- hif-1α. Int J Biol Sci. (2017) 13:794–803. doi: 10.7150/ijbs.18969
213. Cook KL, Shajahan AN, Clarke R. Autophagy and endocrine resistance in breast cancer. Expert Rev Anticancer Ther. (2011) 11:1283–94. doi: 10.1586/era.11.111
214. Actis C, Muzio G, Autelli R. Autophagy triggers tamoxifen resistance in human breast cancer cells by preventing drug-induced lysosomal damage. Cancers (Basel). (2021) 13:1–23. doi: 10.3390/cancers13061252
215. Marzo I, Naval J. Bcl-2 family members as molecular targets in cancer therapy. Biochem Pharmacol. (2008) 76:939–46. doi: 10.1016/j.bcp.2008.06.009
216. Bashari MH, Fan F, Vallet S, Sattler M, Arn M, Luckner-Minden C, et al. Mcl-1 confers protection of her2-positive breast cancer cells to hypoxia: therapeutic implications. Breast Cancer Res. (2016) 18:26. doi: 10.1186/s13058–016-0686–4
217. Sasabe E, Tatemoto Y, Li D, Yamamoto T, Osaki T. Mechanism of hif-1alpha-dependent suppression of hypoxia-induced apoptosis in squamous cell carcinoma cells. Cancer Sci. (2005) 96:394–402. doi: 10.1111/j.1349-7006.2005.00065.x
218. Chen N, Chen X, Huang R, Zeng H, Gong J, Meng W, et al. Bcl-xl is a target gene regulated by hypoxia-inducible factor-1{Alpha}. J Biol Chem. (2009) 284:10004–12. doi: 10.1074/jbc.M805997200
219. Li J, Shi M, Cao Y, Yuan W, Pang T, Li B, et al. Knockdown of hypoxia-inducible factor-1alpha in breast carcinoma mcf-7 cells results in reduced tumor growth and increased sensitivity to methotrexate. Biochem Biophys Res Commun. (2006) 342:1341–51. doi: 10.1016/j.bbrc.2006.02.094
220. Flamant L, Roegiers E, Pierre M, Hayez A, Sterpin C, De Backer O, et al. Tmem45a is essential for hypoxia-induced chemoresistance in breast and liver cancer cells. BMC Cancer. (2012) 12:391. doi: 10.1186/1471–2407-12–391
221. Batlle E, Clevers H. Cancer stem cells revisited. Nat Med. (2017) 23:1124–34. doi: 10.1038/nm.4409
222. Raman D, Tiwari AK, Tiriveedhi V, Rhoades Sterling JA. Editorial: the role of breast cancer stem cells in clinical outcomes. Front Oncol. (2020) 10:299. doi: 10.3389/fonc.2020.00299
223. Zhao J. Cancer stem cells and chemoresistance: the smartest survives the raid. Pharmacol Ther. (2016) 160:145–58. doi: 10.1016/j.pharmthera.2016.02.008
224. Mathieu J, Zhang Z, Zhou W, Wang AJ, Heddleston JM, Pinna CM, et al. Hif induces human embryonic stem cell markers in cancer cells. Cancer Res. (2011) 71:4640–52. doi: 10.1158/0008–5472.Can-10–3320
225. Singh L, Roy S, Kumar A, Rastogi S, Kumar D, Ansari MN, et al. Repurposing combination therapy of voacamine with vincristine for downregulation of hypoxia-inducible factor-1α/fatty acid synthase co-axis and prolyl hydroxylase-2 activation in er+ Mammary neoplasia. Front Cell Dev Biol. (2021) 9:736910. doi: 10.3389/fcell.2021.736910
226. Lee K, Zhang H, Qian DZ, Rey S, Liu JO, Semenza GL. Acriflavine inhibits hif-1 dimerization, tumor growth, and vascularization. Proc Natl Acad Sci U.S.A. (2009) 106:17910–5. doi: 10.1073/pnas.0909353106
227. Li SH, Shin DH, Chun YS, Lee MK, Kim MS, Park JW. A novel mode of action of yc-1 in hif inhibition: stimulation of fih-dependent P300 dissociation from hif-1{Alpha}. Mol Cancer Ther. (2008) 7:3729–38. doi: 10.1158/1535–7163.Mct-08–0074
228. Shin DH, Chun YS, Lee DS, Huang LE, Park JW. Bortezomib inhibits tumor adaptation to hypoxia by stimulating the fih-mediated repression of hypoxia-inducible factor-1. Blood. (2008) 111:3131–6. doi: 10.1182/blood-2007–11-120576
229. Li Z, You Q, Zhang X. Small-molecule modulators of the hypoxia-inducible factor pathway: development and therapeutic applications. J Med Chem. (2019) 62:5725–49. doi: 10.1021/acs.jmedchem.8b01596
230. Yu T, Tang B, Sun X. Development of inhibitors targeting hypoxia-inducible factor 1 and 2 for cancer therapy. Yonsei Med J. (2017) 58:489–96. doi: 10.3349/ymj.2017.58.3.489
231. Narita T, Yin S, Gelin CF, Moreno CS, Yepes M, Nicolaou KC, et al. Identification of a novel small molecule hif-1alpha translation inhibitor. Clin Cancer Res. (2009) 15:6128–36. doi: 10.1158/1078–0432.Ccr-08–3180
232. Lee DH, Lee YJ. Quercetin suppresses hypoxia-induced accumulation of hypoxia-inducible factor-1alpha (Hif-1alpha) through inhibiting protein synthesis. J Cell Biochem. (2008) 105:546–53. doi: 10.1002/jcb.21851
233. Lang L, Liu X, Li Y, Zhou Q, Xie P, Yan C, et al. A synthetic manassantin a derivative inhibits hypoxia-inducible factor 1 and tumor growth. PloS One. (2014) 9:e99584. doi: 10.1371/journal.pone.0099584
234. Terzuoli E, Puppo M, Rapisarda A, Uranchimeg B, Cao L, Burger AM, et al. Aminoflavone, a ligand of the aryl hydrocarbon receptor, inhibits hif-1alpha expression in an ahr-independent fashion. Cancer Res. (2010) 70:6837–48. doi: 10.1158/0008–5472.Can-10–1075
235. Li G, Shao Y, Pan Y, Li Y, Wang Y, Wang L, et al. Total synthesis and biological evaluation of 7-hydroxyneolamellarin a as hypoxia-inducible factor-1α Inhibitor for cancer therapy. Bioorg Med Chem Lett. (2021) 50:128338. doi: 10.1016/j.bmcl.2021.128338
236. Liu Y, Veena CK, Morgan JB, Mohammed KA, Jekabsons MB, Nagle DG, et al. Methylalpinumisoflavone inhibits hypoxia-inducible factor-1 (Hif-1) activation by simultaneously targeting multiple pathways. J Biol Chem. (2009) 284:5859–68. doi: 10.1074/jbc.M806744200
237. Parhira S, Zhu GY, Chen M, Bai LP, Jiang ZH. Cardenolides from calotropis gigantea as potent inhibitors of hypoxia-inducible factor-1 transcriptional activity. J Ethnopharmacol. (2016) 194:930–6. doi: 10.1016/j.jep.2016.10.070
238. Welsh S, Williams R, Kirkpatrick L, Paine-Murrieta G, Powis G. Antitumor activity and pharmacodynamic properties of px-478, an inhibitor of hypoxia-inducible factor-1alpha. Mol Cancer Ther. (2004) 3:233–44. doi: 10.1158/1535-7163.233.3.3
239. Jones DT, Harris AL. Identification of novel small-molecule inhibitors of hypoxia-inducible factor-1 transactivation and DNA binding. Mol Cancer Ther. (2006) 5:2193–202. doi: 10.1158/1535–7163.Mct-05–0443
240. Wang KL, Hsia SM, Chan CJ, Chang FY, Huang CY, Bau DT, et al. Inhibitory effects of isoliquiritigenin on the migration and invasion of human breast cancer cells. Expert Opin Ther Targets. (2013) 17:337–49. doi: 10.1517/14728222.2013.756869
241. Jin J, Qiu S, Wang P, Liang X, Huang F, Wu H, et al. Cardamonin inhibits breast cancer growth by repressing hif-1α-dependent metabolic reprogramming. J Exp Clin Cancer Res. (2019) 38:377. doi: 10.1186/s13046–019-1351–4
242. Bailey CM, Liu Y, Peng G, Zhang H, He M, Sun D, et al. Liposomal formulation of hif-1α Inhibitor echinomycin eliminates established metastases of triple-negative breast cancer. Nanomedicine. (2020) 29:102278. doi: 10.1016/j.nano.2020.102278
243. Mir Hassani Z, Nabiuni M, Parivar K, Abdirad S, Karimzadeh L. Melittin inhibits the expression of key genes involved in tumor microenvironment formation by suppressing hif-1α Signaling in breast cancer cells. Med Oncol. (2021) 38:77. doi: 10.1007/s12032–021-01526–6
244. Wang T, Meng J, Wang C, Wen T, Jia M, Ge Y, et al. Inhibition of murine breast cancer metastases by hydrophilic as(4)S(4) nanoparticles is associated with decreased ros and hif-1α Downregulation. Front Oncol. (2019) 9:333. doi: 10.3389/fonc.2019.00333
245. Su Q, Wang J, Wu Q, Ullah A, Ghauri MA, Sarwar A, et al. Sanguinarine combats hypoxia-induced activation of ephb4 and hif-1α Pathways in breast cancer. Phytomedicine. (2021) 84:153503. doi: 10.1016/j.phymed.2021.153503
246. Xiang L, Gilkes DM, Chaturvedi P, Luo W, Hu H, Takano N, et al. Ganetespib blocks hif-1 activity and inhibits tumor growth, vascularization, stem cell maintenance, invasion, and metastasis in orthotopic mouse models of triple-negative breast cancer. J Mol Med (Berl). (2014) 92:151–64. doi: 10.1007/s00109–013-1102–5
247. Wong CC, Zhang H, Gilkes DM, Chen J, Wei H, Chaturvedi P, et al. Inhibitors of hypoxia-inducible factor 1 block breast cancer metastatic niche formation and lung metastasis. J Mol Med (Berl). (2012) 90:803–15. doi: 10.1007/s00109-011-0855-y
248. Wei Z, Shan Y, Tao L, Liu Y, Zhu Z, Liu Z, et al. Diallyl trisulfides, a natural histone deacetylase inhibitor, attenuate hif-1α Synthesis, and decreases breast cancer metastasis. Mol Carcinog. (2017) 56:2317–31. doi: 10.1002/mc.22686
249. Kachamakova-Trojanowska N, Podkalicka P, Bogacz T, Barwacz S, Józkowicz A, Dulak J, et al. Hif-1 stabilization exerts anticancer effects in breast cancer cells in vitro and in vivo. Biochem Pharmacol. (2020) 175:113922. doi: 10.1016/j.bcp.2020.113922
250. Liu M, Liang Y, Zhu Z, Wang J, Cheng X, Cheng J, et al. Discovery of novel aryl carboxamide derivatives as hypoxia-inducible factor 1α Signaling inhibitors with potent activities of anticancer metastasis. J Med Chem. (2019) 62:9299–314. doi: 10.1021/acs.jmedchem.9b01313
251. Holmquist-Mengelbier L, Fredlund E, Löfstedt T, Noguera R, Navarro S, Nilsson H, et al. Recruitment of hif-1alpha and hif-2alpha to common target genes is differentially regulated in neuroblastoma: hif-2alpha promotes an aggressive phenotype. Cancer Cell. (2006) 10:413–23. doi: 10.1016/j.ccr.2006.08.026
252. Bardia A, Santa-Maria CA, Jacobs LK, Cimino-Mathews A. Digoxin as an inhibitor of global hypoxia inducible factor-1α (Hif1α) expression and downstream targets in breast cancer: dig-hif1 pharmacodynamic trial. (2013) 31(15_suppl):TPS1144–TPS. Stearns VJJoCO. J Clin Oncol. doi: 10.1200/jco.2013.31.15_suppl.tps1144
Keywords: breast cancer, hypoxia-inducible factor, angiogenesis, invasion and metastasis, apoptosis and autophagy, drug resistance
Citation: Zhi S, Chen C, Huang H, Zhang Z, Zeng F and Zhang S (2024) Hypoxia-inducible factor in breast cancer: role and target for breast cancer treatment. Front. Immunol. 15:1370800. doi: 10.3389/fimmu.2024.1370800
Received: 15 January 2024; Accepted: 26 April 2024;
Published: 10 May 2024.
Edited by:
Ying Lin, Sun Yat-Sen University, ChinaReviewed by:
Daniele Vergara, University of Salento, ItalyCopyright © 2024 Zhi, Chen, Huang, Zhang, Zeng and Zhang. This is an open-access article distributed under the terms of the Creative Commons Attribution License (CC BY). The use, distribution or reproduction in other forums is permitted, provided the original author(s) and the copyright owner(s) are credited and that the original publication in this journal is cited, in accordance with accepted academic practice. No use, distribution or reproduction is permitted which does not comply with these terms.
*Correspondence: Shujun Zhang, emhhbmdzaHVqdW5Ac3dtdS5lZHUuY24=; Fancai Zeng, emZjYWlAc3dtdS5lZHUuY24=
Disclaimer: All claims expressed in this article are solely those of the authors and do not necessarily represent those of their affiliated organizations, or those of the publisher, the editors and the reviewers. Any product that may be evaluated in this article or claim that may be made by its manufacturer is not guaranteed or endorsed by the publisher.
Research integrity at Frontiers
Learn more about the work of our research integrity team to safeguard the quality of each article we publish.