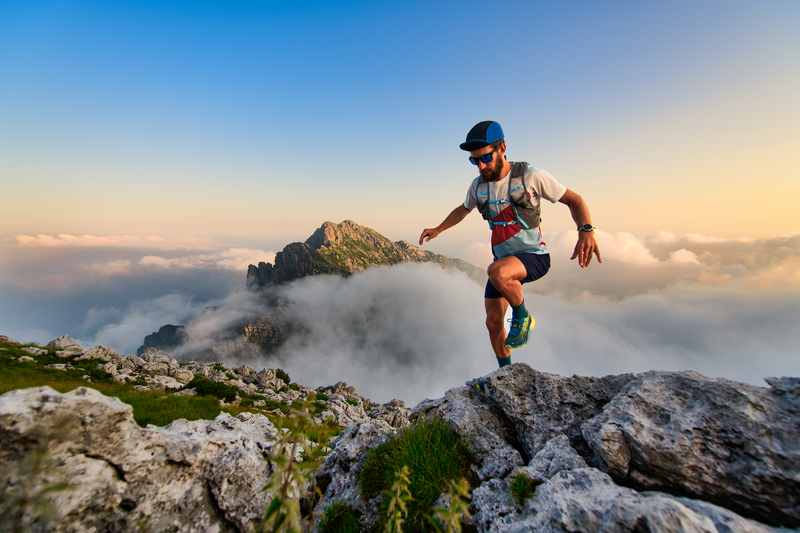
95% of researchers rate our articles as excellent or good
Learn more about the work of our research integrity team to safeguard the quality of each article we publish.
Find out more
REVIEW article
Front. Immunol. , 25 September 2023
Sec. Comparative Immunology
Volume 14 - 2023 | https://doi.org/10.3389/fimmu.2023.905467
This article is part of the Research Topic Insect immunity and its interactions with microorganisms and parasitoids View all 31 articles
Multicellular organisms live in environments containing diverse nutrients and a wide variety of microbial communities. On the one hand, the immune response of organisms can protect from the intrusion of exogenous microorganisms. On the other hand, the dynamic coordination of anabolism and catabolism of organisms is a necessary factor for growth and reproduction. Since the production of an immune response is an energy-intensive process, the activation of immune cells is accompanied by metabolic transformations that enable the rapid production of ATP and new biomolecules. In insects, the coordination of immunity and metabolism is the basis for insects to cope with environmental challenges and ensure normal growth, development and reproduction. During the activation of insect immune tissues by pathogenic microorganisms, not only the utilization of organic resources can be enhanced, but also the activated immune cells can usurp the nutrients of non-immune tissues by generating signals. At the same time, insects also have symbiotic bacteria in their body, which can affect insect physiology through immune-metabolic regulation. This paper reviews the research progress of insect immune-metabolism regulation from the perspective of insect tissues, such as fat body, gut and hemocytes. The effects of microorganisms (pathogenic bacteria/non-pathogenic bacteria) and parasitoids on immune-metabolism were elaborated here, which provide guidance to uncover immunometabolism mechanisms in insects and mammals. This work also provides insights to utilize immune-metabolism for the formulation of pest control strategies.
Microbes and animals have a long-term association that is widely distributed in nature, and plays a vital role in animal adaptation and evolution. Immunity and metabolism are two different biological systems that have traditionally been studied independently, however, recent studies discover that these two processes are interlinked in animal physiology, and has opened up an exciting area of research called immunometabolism (1). More than a century ago, Elie Metchnikoff one of the founders of immunology, observed metabolic changes associated with inflammation phenotypes (2). Further studies have revealed that many metabolic diseases including type 2 diabetes and obesity are partly because of chronic inflammation further demonstrating the importance of immune-metabolic interactions in animal physiology (3–5). The occurrence of an immune response is an energy consuming process. When organisms are infected with pathogens, the immune system is activated, which is closely related to the body’s metabolic switch, including the redistribution of energy resources and increase of glycolysis and glucose consumption by the immune system (6, 7).
Immune-metabolic interactions are an evolutionary conserved phenomenon in all multicellular organisms from vertebrates to invertebrates, and studies have found similar immune-metabolic phenotypes. For example, Drosophila melanogaster infection with parasitic wasps or bacteria results in delayed development and increased carbohydrate mobilization resulting in hyperglycemia-like phenotype, while the energy requirements of immune cells increase from 10% of total glucose consumption to nearly one-third (8, 9). Furthermore, infection induces activation of immune responses leads to a decrease in the overall metabolic rate (10–13), and slow host development and loss of energy reserves during chronic infection (9, 14). Despite the important impact of immune-metabolic interactions on the physiology of the organism, there is currently a large gap in understanding of immune-metabolic interactions. Here, we focus on the metabolic regulations during immune responses in insects, from D. melanogaster a genetically tractable insect model to other important agricultural insects. In view of bacteria and parasitic wasps which are two major groups interacting with insects, this paper reviews the research progress of immunometabolic regulation in their interactions with insects. In addition, some classical studies on the effects of parasites on immunometabolism of insects are also included.
Organisms adapt to changes in external and internal environments through various metabolic regulation. Insulin/IGF signaling (IIS) is a core pathway regulating the balance between anabolic and catabolic processes in the body (15). IIS activity is systematically regulated by insulin-like peptides (ILPs). D. melanogaster contain eight ILPs (DILP1-8), however, there are only two known receptors, dInR (Drosophila insulin receptor) and Lgr3 (leucine-rich G-protein-coupled receptor) for them (16–20). Activited dInR promotes a conserved intracellular signaling cascade transmitted through Chico, a homologue of mammalian insulin receptor substrates (IRS1-4) (21). Together with phosphatidylinositol-3-kinase (PI3K) and protein kinase B (PKB or AKT), IIS affects gene expression through AKT-mediated phosphorylation of FoxO, and subsequent cytoplasmic retention of FoxO, leading to downregulation of its target genes (22–24) (Figure 1). Without immune stimulation, insulin signaling cascade regulates energy and nutrient use for storage, growth and other non-immune processes. The inhibition of IIS has been established as an evolutionary conserved mechanism that promotes diapause in invertebrates and prolong lifespan in both invertebrates and vertebrates in response to environmental challenges (25, 26). The mutation of InR substrate Chico flies with suppressed insulin activity, not only extend longevity when live in normal condition but increase pathogen resistance when infected by Gram-negative bacteria Pseudomonas aeruginosa and gram-positive bacteria Enterococcus faecalis (27). However, when infected with the insect-pathogenic Photorhabdus luminescens and non-pathogenic Escherichia coli, there was no apparent difference in survival between Chico mutant flies and controls, furthermore, after infection Chico mutant flies had lower bacterial loads at most time points (28). In addition, transcriptional sequencing analysis of the insulin-resistant fat body of D. melanogaster also revealed a correlation between IIS and immune response (29).
Figure 1 Classical immune and metabolic signaling pathways in insects. Specific explanation is described in the text. The acronyms in the picture are explained below: dILP, Drosophila insulin like peptide; InR, insulin receptor; Chip, insulin receptor substrate; PI3K, phosphoinositide 3-kinase; AKT, Akt kinase; TOR, target of rapamycin; FoxO, forkhead box O transcription factors; Slif, Amino acid transporter; S6K, phosphorylates S6 Kinase; 4E-BP,eIF4E-binding protein; Spz, Spätzle, ligand for Toll pathway activation; Toll, recepter of Toll pathway; Dif/Dorsal, transcription factor of Toll pathway; PGRP-LC, recepter of IMD pathway; IMD, canonic component of the immune deficiency pathway; Rel, transcription factor of IMD pathway; JNK, c-Jun N-terminal kinase cascade.
Insects occupy a wide range of ecological niches where they inevitably face frequent contact with microorganisms in the surrounding environment, a small number of which can cause diseases. Although, insects lack an adaptive immune system similar to that of vertebrates, they have a variety of ways to fight off infection by pathogens. The first line of defense in insects depends on behavioral adaptations and body barriers; Behavioral adaptations include insect hygienic behaviors, reduced social contacts, and selective foraging; Body barriers include exoskeleton, trachea and intestinal epithelium and intestinal peritrophic matrix (30–32). The innate immune system is able to distinguish between self and non-self. Insects are able to detect different types of microorganisms by recognizing microbe-associated molecular patterns (MAMPs) through pattern recognition receptors (PRRs) (33). Insect innate immune system contain two different types of immune responses, mainly humoral responses including secretion of antimicrobial effectors such as antimicrobial peptides (AMPs) and lysozyme, and cellular responses such as phagocytosis, encapsulation and nodulation (33). Insect cellular response mainly mediated by hemocytes which contains different types of cells responsible for distinct functions. The D. melanogaster larvae contains three major hemocyte cells including plasmatocytes, crystal cells, and lamellocytes that participate in phagocytosis, PO cascade, and encapsulation, respectively (33). While the humoral immunity is mainly regulated by Toll and immunodeficiency (IMD) pathways (33–35). The D. melanogaster Toll and IMD pathways share significant similarities with the mammalian TNF-α pathway and the Toll-like receptor pathway (TLR), respectively (36–38). The IMD pathway activates in immune tissues throughout the body, and recognizes diaminopimelic acid (DAP) type peptidoglycans present on cell walls of most Gram-negative bacteria and some Gram-positive bacteria (39). In contrast, the Toll pathway activates primarily in the fat body or hemolymph or immune cells, and respond to MAMPs from Gram-positive and fungi containing lysine-type peptidoglycans and β-glucans, respectively (40). In response to pathogen infection, the Toll and IMD signaling pathways of D. melanogaster activate the p65-like transcription factor Dif and p105-like transcription factor Relish, respectively; The transcription factor then enters the nucleus and induces the expression of antimicrobial peptide (AMP) by binding to the κB binding site in the AMP gene promoter region (33, 36, 41) (Figure 1).
During non-infectious stage, the immune system remains inactivate, consuming the minimum energy for basic functioning (42). These immune responses during pathogenic infection require timely and precise energy allocation in appropriate cells or tissues for effective self-protection and elimination of pathogens (1). Activation of immune system consumes substantial amount of energy and therefore competes for energy resources needed by other biological processes in the body and this energy competition is well documented in eco-physiological studies of insects (43). Present studies on immunometabolism of insects are mainly in the gut, fat body and hemolymph. This is elaborated below.
The gut is the most important part of the insect digestive system, and the insect gut epithelium is generally considered to be the only way for insects to obtain nutrients (44, 45). On the one hand, gut coordinates defenses against microbial penetration of the intestinal epithelium, on the other hand, it uses digestive enzymes and transporters to transport nutrients to the internal organs (46). Therefore, the link between metabolism and immunity is especially evident in the gut.
In contrast to many multicellular organisms, the intestinal epithelium of insects is simple, consisting of a single cell layer surrounded by a layer of muscle, and in most insects includes only two differentiated cell types: the enterocytes and the endocrine cells (47). The midgut is the main site for food digestion, nutrient absorption, and energy substrate storage (45). The D. melanogaster midgut is composed of four different types of cells, including intestinal stem cells (ISCs) and undifferentiated ISC daughter cells referred as enteroblasts (EBs) (48), which can differentiate into intestinal enterocytes (ECs) or enteroendocrine cells (EEs) (49–51). ISCs usually occur singly or in small groups and are often located in the basal lamina (50, 52). Some midgut cells of other insects also include cells with specialized functions for ion transport, such as goblet cells of Lepidoptera and cuprophilic cells of Diptera, which have the function of maintaining highly alkaline and acidic conditions in the intestinal lumen, respectively (53, 54).
In addition, the insect gut has the peritrophic membrane as its physical barrier, establishing a first line of defense against pathogens, and preventing them from contacting intestinal epithelial cells (55). The peritrophic membrane is a rectangular grid-like structure composed of chitin polymers and proteins such as peritrophin (56, 57). The peritrophic membrane acts like a sieve, limiting the passage of not only pathogens but also toxins and food particles (57). In vertebrates, a mucus layer composed of polysaccharides and proteins (mucins) separates the intestinal epithelium from the outside environment, and the mucus layer keeps bacteria confined to the intestinal lumen (58). The peritrophic membrane of insects acts like a vertebrate mucous layer. In D. melanogaster, the peritrophic membrane is lined up along the epithelial cells of the midgut, just like a mucous layer (59). Annotated information of more than 30 D. melanogaster genomes indicated the presence of genes encoding mucilage layer proteins (60). With the development of mucilage material staining technique (cyclic acid Schiff method), the mucilage layer of D. melanogaster was identified (58), but its function remains to be further studied. Transcriptomic data show that genes for both peritrophic membrane metabolism and mucus production are altered in the midgut of Erwinia carotovora subsp. carotovora 15 (Ecc15) infected flies, suggesting that both barriers are remodeled during infection (61).
Current research on gut immunity mainly focuses on the IMD signaling pathway and the ROS production. The production of reactive oxygen species (ROS) is the first immune response against pathogens in gut, and activated by dioxygenase (DUOX), a member of the nicotinamide adenine dinucleotide phosphate oxidase (NADPH) family (62). Bacterial-derived uracil can induce the formation of calcineurin 99C (Cad99C)-containing endosomes that serve as a phospholipase Cβ (PLCβ)-dependent calcium mobilization platform that is critical for DUOX activation (63). In the midgut, the production of AMPs is regulated by the IMD pathway. The IMD pathway in the gut is activated by two upstream receptors, the cell membrane surface-bound receptor PGRP-LC which acts in the foregut, midgut and hindgut and the intracellular receptor PGRP-LE acts only in the midgut (64, 65). PGRP-LC and PGRP-LE can recognize diaminopimelic acid (DAP) type peptidoglycans present on cell walls of most Gram-negative bacteria and some Gram-positive bacteria (66, 67). When the IMD pathway in the gut is triggered by pathogenic bacteria, intestinal enteroblast ECs release humoral immune factors such as AMPs (47).
The pathogenic bacteria can affect gut homeostasis by directly disrupting epithelial tissue, or indirectly by altering the structure of gut microbial populations (58, 68). Therefore, maintenance of gut homeostasis requires the synergy of antimicrobial and metabolic responses in order to fight pathogenic infection. Recently, the link between the production of ROS and metabolism have been reported by Lee et al. (69). Their study found that the activation of DUOX is depended on tumor necrosis factor (TRAF3). Further, the activation of TRAF3 can inactivate AKT and activate AMPK, and the inactivation of AKT leads to decrease TOR activity then influence the activity of downstream kinases S6K and ATG1 (69). ROS production can lead to S6K inactivation and ATG1 activation, inactivated S6K resists anabolism, and activated ATG1 promotes catabolism, thereby regulating the transition from lipogenesis to lipolysis in the gut epithelia (69). The maintenance of DUOX activity relies on lipolysis to increase NADPH production, thus, the interaction of lipolytic signaling with host immune signaling ensures an effective antimicrobial response (69). When insect-pathogenic bacteria Pseudomonas entomophila infects D. melanogaster, the production of bacterial’s toxins and host’s ROS can inhibit TOR pathway and cause complete termination of protein synthesis, thereby preventing tissue repair and antimicrobial peptide production, ultimately accelerating infection to hosts death (70). Wang et al. showed that Plasmodium falciparum, the deadliest parasite in humans, could induce activation of P38-MAPK in the midgut of its insects vector Anopheles stephensi to weaken the insect’s immune system including inhibition the production of mitochondrial ROS and in turn, enhancing protein synthesis and metabolism (71). Therefore, the triggered p38-MAPK signal in the gut of A. stephensi contributes to both the survival of the parasite and avoids the damage of the host by immune overreaction (71).
The two-component system of Vibrio cholerae contains carboxylic acid regulator and sensor CrbRS which activates transcription of gene acs1 encoding Acetyl-CoA synthase (ACS-1) to deplete acetate in the gut (72, 73). Oral infection with Vibrio cholerae induces intestinal acetate depletion in D. melanogaster, systemically inhibits the insulin pathway, promotes fat accumulation in intestinal ECs cells, and accelerates infection-induced host death (73). IMD mutant flies have significantly improved survival after oral infection with V. cholerae compared to wild-type, and this phenotype is dependent on the interaction of V. cholerae’s type 6 secretion system (T6SS) and the gut commensal bacteria Acetobacter pasteurianus to cause immune hyperactivity of wild-type hosts (74). The systemic infection by non-pathogenic bacteria Escherichia coli, the extracellular bacterial pathogen Photorhabdus luminescens, and the facultative intracellular pathogen Photorhabdus asymbiotica cause accumulation of lipid droplets in the midgut of D. melanogaster (75). The specific mechanism is that systemic infection leads to mediate the midgut IMD activation through the receptor PGRP-LC, which in turn leads to a decrease in the synthesis of tachykinin TK, which ultimately leads to an increase in midgut fat anabolism; Deliberately reducing TK synthesis in gut can increase the survival time of host under P. asymbiotica infection, but make host more sensitive to P. luminescens infection (75). In addition, oral infection with V. cholerae can also cause lipid metabolism disorders in the host gut and accumulation of triacylglycerols (TAGs) and sterols in intestinal epithelial cells of enterocytes (44).
In Anopheles gambiae, phenylalanine hydroxylase (PAH) which is involved in the conversion of phenylalanine to tyrosine, is a key pathway in amino acid metabolism, and it has been found that silencing of PAH destroys phenylalanine metabolism, resulting in a decrease in tyrosine content, leading to a marked impairment of the gut melanin-dependent encapsulation response to the rodent malaria parasite Plasmodium berghei (76, 77). These studies indicate that when pathogenic microorganisms invade the gut, the energy demand for immune responses increases to resist microbial invasion by coordinating with the IIS and TOR pathways.
The animal gut plays vital role to interact with the microenvironment. Gut-microbe interactions can have important effects on the host, both through microbial-associated molecular patterns (MAMPs) on microbial surfaces and through the metabolites they produce (78, 79). Although members of the microbiota are often referred to as commensals, symbiosis between the microbiota and its host encompasses various forms of relationship, including mutualistic, parasitic, or commensal (80). The presence of gut commensal microbes is essential for establishment and maintenance of immune system of multicellular organisms (80–82). Moreover, gut microbes also have important effects on the metabolic processes of their host animals, such as directly providing the host with essential nutrients (such as vitamins B and K) or indirectly fermenting indigestible carbohydrates, as well as enhancing the activity of host metabolic pathways (78, 79, 83, 84). The study showed that germ-free mouse epithelial cells expressed fewer metabolic key enzymes than normal mice, and significantly down-regulated nicotinamide adenine dinucleotide (NADH)/NAD+ and ATP levels illustrating gut microbes affect epithelial metabolism (85). Several studies using 16S rRNA analysis to determine the common bacterial diversity of D. melanogaster showed that both wild and laboratory-raised populations exhibited low bacterial diversity (1-30 species) in the gut (48, 86–88). Furthermore, these bacteria are not essential for host development or survival, as germ-free D. melanogaster can be maintained for generations under adequate dietary conditions in the laboratory (89).
The recognition of gut commensal bacterium Lactobacillus plantarum (WJL) by the IMD pathway induces intestinal peptidase expression, and elevated levels of intestinal peptidase promote the body to digest proteins, increase the host’s amino acid levels, and promote the TOR pathway in D. melanogaster larvae (90, 91). In line with this, transcriptional studies on germ-free, IMD mutants and enteric IMD-constitutively activated D. melanogaster confirmed the effects of IMD on intestinal metabolic processes, including the expression of digestive peptidase (92–95). In addition, L. plantarum can promote host growth by secreting N-acetyl-glutamine as a byproduct, and hosts in turn improve the environmental adaptability of their symbionts (96, 97). The studies found that the intestinal innate immune NF-kB/Relish transcription factor is a key point for coupling nutrition-immunity-metabolism, Relish regulates the association between diet and host-Lactobacillus by restricting 4E-BP/Thor, adjusting the species composition of gut microbes (96–98). Acetobacter pomorum, another major gut commensal bacterium of D. melanogaster, could promote the growth of larval and adult by increasing the insulin signaling (IIS) pathway (99). Specifically, A. pomorum produces acetic acid via alcohol dehydrogenase (PQQ-ADH), a bacterial-derived short-chain fatty acid that affects health and homeostasis in many biological models (100–104). The increase in intestinal acetate levels activate signaling through the host IIS cascade, affecting host growth, energy metabolism, and intestinal stem cell activity (99). Additionally, microbial-derived acetate can activate the IMD pathway of enteroendocrine cells, and the enhancement of IMD signaling can promote the expression of tachykinin (TK) gene to increase the content of TK (105). TK plays an important role in larval growth and development, lipid metabolism and insulin signal transduction (105–107). Sannino et al. showed that the essential vitamin thiamine provided by A. Pomorum is essential for the growth and development of D. melanogaster (108). Previous work showed that germ-free D. melanogaster store more triglyceride than conventionally reared flies and this phenotype can be rescued by recolonization of Acetobacter fabarum or Lactobacillus brevis (109, 110). The population structure of symbionts in wild Drosophila species are further complicated by differences in geographical populations and food sources (88, 111–114). A study showed that Acetobacter bacteria isolated from wild D. melanogaster can stably colonize in the gut of lab stock and promote larval growth (115). Therefore, the gut commensal microbes, as an additional source of systemic or local immune signals, may be directly involved in regulating the energy homeostasis and growth metabolism of the organism.
Microbe-associated molecular patterns (MAMPs) also exist in commensal microorganisms, and can be recognized by the host immune system (116). The cell walls of both Acetobacter and Lactobacillus, which dominate the gut flora of D. melanogaster, contain DAP-type peptidoglycan, therefore, can be recognized by the gut immune responses (86). Moreover, it was found that the expression AMPs was significantly reduced in germ-free D. melanogaster gut, suggesting that commensal microbiota can induce the IMD pathway (68, 86). PGRP-SC2 is a negative regulator of the D. melanogaster IMD pathway and is homologous to the vertebrate anti-inflammatory proteins PGLYRP1-4 (117, 118). A study reveal that decreased expression of PGRP-SC2 leads to activation of the IMD transcription factor Relish, resulting in altered commensal microbial community composition and increased division of ISCs (119). These studies demonstrate the importance of IMD pathway activity for the composition of gut commensal microbiota.
Duox is a member of the NADPH oxidase family whose activity can be partially activated in the gut by commensal microorganisms and food-derived yeasts, respectively (62, 120). In the presence of gut commensal microbiota, the activity of p38-MAPKs is down-regulated by MAPK phosphatase 3 (Mkp3), thus attenuating Duox activity and maintaining it at a low-level (121). Interestingly, the intestinal epithelial cells of Drosophila contain a second NADPH oxidase, the nitrogen oxidase (Nox), which can be activated by gut commensal bacteria Lactobacillus to produce ROS as a local signaling molecule, which had no deleterious effects on both bacteria and intestinal epithelium cells (122).
Although commensal and pathogenic microorganisms involve in activation of similar gut immune mechanisms, the level of immune response activated by the intestinal commensal microbiota and the degree of damage to intestinal epithelial cells were much lower than that of pathogenic microorganisms (61). Gut commensal microbiota can induce innate immune signals in intestinal epithelial cells, while negative feedback mechanisms limit the expression of immune factors such as AMPs and ROS that regulate bacterial composition in the gut lumen (86, 121, 123). Honeybee (Apis mellifera) exposure to a certain concentration of neonicotinoid insecticide (nitenpyram) causes imbalance of gut microbiota that leads to the alternation of metabolism and immune-related genes in the gut (124). These findings reveal that the insect gut immune system not only eliminate pathogenic microbes but also maintain commensal microbes to regulate metabolism. Therefore, the gut commensal microbes properly coordinate with the host’s immunity and metabolism establishing a long microbiota-insect evolutionary interaction.
Insect fat body is the major organ that provide energy for metabolism and induce humoral immune responses. On the one hand, it is responsible for storing energy including glycogen, triglycerides etc., on the other hand, it provides energy essential for all life activities including immune responses (33, 125). Under the influence of a pathogenic infection, the fat body initiates humoral immune responses including production of AMPs and other immune molecules, restricting energy flow to other processes including anabolism (126).
The fat body’s function of D. melanogaster is analogous to mammalian liver, adipose tissues and immune organs (127, 128), it provides a simple and accessible system for studying molecular integration of immune and metabolic pathways in the presence of microorganisms. In D. melanogaster, metabolism and growth is regulated by insulin-like peptides that bind to insulin receptors on the fat body cells, activate AKT and TOR, and promote protein synthesis and storage of sugar as triglycerides and glycogen in the fat body (125). Fat body responds to systemic infection of different pathogens through Toll and IMD pathways, driving expression of AMPs to eliminate pathogens (34, 35). Additionally, Eiger/TNF-α, JNK and JAK-STAT are also important immune signals in the fat body in response to various immune stimuli (129). These immune signaling pathways are activated upon infection and regulate a wide range of immune response as well as affect metabolism at various levels. Therefore, they can be considered as key components of immune-metabolism integration. Keeping in view the significance of fat body in immunometabolism, several key pathways are discussed below.
IMD pathway is an important NF-κB signaling pathway in the fat body immune regulation. Over-expression of the IMD transcription factor Relish in the fat body does not affect insulin activity (130). However, over-expression of the active form of IMD protein in the fat body suppresses systemic IIS activity and exhibits many phenotypes similar to insulin loss-of-function (131). MEF2 (Myocyte enhancer factor 2) plays a bidirectional regulatory role in fat body anabolism and catabolism through phosphorylation and dephosphorylation (132). MEF2 mediates a metabolic switch under conditions of Gram-negative bacterial infection, shifting the fat body from anabolism to activation of IMD signaling (131). Under normal condition, phosphorylated MEF2 can activate the expression of genes involved in anabolic processes, however, infection leads to dephosphorylation of MEF2, which reduces anabolic processes, while dephosphorylated MEF2 can activate the expression of AMPs (131).
DiAngelo et al. performed selective activation of the Toll signaling pathway in the fat body using genetics and infection to induce immunity and energy redistribution in D. melanogaster (130). The study revealed that activation of Toll signaling in the fat body not only decrease the levels of AKT phosphorylation and attenuated insulin signaling in the fat body, but also suppresses insulin signaling throughout the body resulting in decreased nutrient storage and growth retardation (130). Overexpressing the antimicrobial peptide Drosomycin that responds to the Toll pathway reduced glycogen and triglyceride storage of D. melanogaster (133), and this suggests that inducing the expression of AMPs may indeed cause the body’s energy burden. Activation of Toll in the fat body reduces triglyceride storage and has systemic effects on body growth, suggesting that immune activation in the fat body can lead to a systemic metabolic switch by reducing insulin signaling (129, 132). Roth et al. further showed that growth inhibition induced by overexpression of Toll in the fat body could be rescued by the expression of phosphorylated AKT (134). Activation of Toll signaling in the fat body of D. melanogaster larvae can cause a shift in lipid metabolism, shifting fatty acids from neutral lipid storage to phospholipid synthesis, a shift that supports immune responses in the short term but increases host mortality in the long term (135). Furthermore, the activation of fat body Toll signaling by genetic or bacterial stimulation results in not only tissue-autonomous reductions in triglyceride storage, but the membrane phospholipid synthesis is induced, as manifested by increased levels of phosphatidylcholine and phosphatidylethanolamine in the fat body, this shift facilitates the synthesis and secretion of AMPs (134). Additionally, Toll activation has also been shown to block S6K-mediated phosphorylation of MEF2, leading to a switch from anabolism to immunity in the fat body (132).
From Drosophila to mammals, Hippo signaling pathway plays a vital role in regulating tissue growth, organ size and homeostasis (136–138). In mammals, the Hippo signaling pathway is involved in the regulation of islet cells. Inactivation of Hippo in islet cells can damage islet cells and promote their apoptosis, thereby affecting insulin secretion, causing metabolic disorders and dysfunction, and leading to the occurrence of diabetes (139, 140). Liu et al. demonstrated that the Hippo signaling pathway has an important role in response to Gram-positive bacterial infection in D. melanogaster and that Hippo activation in the fat body can promote the expression of AMPs (141). Activation of the hippo-Yorkie signaling pathway through the Toll-Myd88-Pelle cascade can induce the degradation of the subunit Cka of the Hpo-inhibitory complex, and then Warts-mediated Yorkie inactivation increases Dif activity by limiting Cactus levels and enhances AMPs gene expression (141). Aforementioned results manifest a strong relationship between the Toll signaling pathway and the growth metabolism pathway: IIS and hippo pathway in the immune metabolism of the fat body.
JNK (c-Jun N-terminal kinase) signaling can affect metabolism and immunity in many different ways (43). As a stress and inflammatory signaling pathway, JNK signaling can systemically antagonize IIS by activating FoxO to down-regulate the expression of Dilp2 in IPCs (insulin-producing cells), promoting stress tolerance and prolonging lifespan (71). Moderate JNK signaling activity facilitates the management of energy resources under stressful conditions, but excessive JNK activity in vertebrate adipose tissue has been found to cause type II diabetes (142–144). Therefore, tight regulation of the interaction between the stress signal JNK and the insulin signal (IIS) is necessary for the body to ensure its own homeostasis to adapt environmental challenges. Activation of JNK signaling in the fat body can compensate for the cytoplasmic retention of FoxO caused by IIS overexpression, allowing FoxO to enter the nucleus to regulate target gene expression and antagonize IIS-mediated cell overgrowth (145). Drosophila NLaz is a secreted protein homologous to vertebrate apolipoprotein D (ApoD) and retinol binding protein 4 (RBP4) (146). Oxidative stress or activation of JNK can induce the transcription of NLaz gene. NLaz mediates the antagonism between JNK and Insulin signaling to negatively regulate insulin signaling, thereby improving hunger tolerance and regulating metabolism and growth, enabling the body to respond to environmental challenges (147). The study found that NLaz is not important for immune resistance to gram-positive E. faecalis infection, but another lipocalin, Karl, released from blood cells, helps the host resist E. faecalis infection (147).
Eiger is the homolog of tumor necrosis factor (TNF) in D. melanogaster, and members of the TNF family are important pleiotropic cytokines that play important roles in regulating infection, inflammation, autoimmune disease, and tissue homeostasis (148, 149). Evidence show that Eiger affect immune responses and metabolism and is a link between immune activation and systemic metabolism (128, 150, 151). Under low-protein diet conditions, Eiger is released from the fat body into the hemolymph and then into the brain, where it inhibits Dilp2 and Dilp3 expression by activating JNK signaling in IPCs by binding to the tumor necrosis factor receptor (128). Under immune stimulation, Eiger can be expressed in both blood cells and fat bodies, and influence the immune response under infection. The release of Eiger from the fat body under the condition of low protein diet can inhibit the expression of Dilp2-3 in IPCs (128), and the release of Eiger possible has the same effect under the condition of infection, which can change the body from anabolism to anti-infection by inhibiting IIS. Eiger expression in the fat body has a positive effect on host survival against extracellular pathogen infection, but loss of Eiger improves host survival when infected with intracellular pathogens, and this difference may stem from Eiger being required for phagocytosis (152, 153). Tang et al. showed that infected housefly Musca domestica by Escherichia coli or Staphylococcus aureus increased Eiger like gene (Mdeiger) expression resulting in decreased mortality, and knockdown of Mdeiger expression by RNAi downregulate expression of JNK and Dorsal, but upregulate the expression of Relish (154). These studies demonstrate that Eiger can enhance Toll-mediated immune responses, but in contrast to the IMD-Relish-mediated immune response, Eiger inhibits Relish activity by activating JNK, a result that is consistent with the Eiger mutant producing more IMD-regulated AMPs than wild-type (153, 154).
Cytokine Unpaire-dependent (Upd) activation of JAK-STAT signaling in Drosophila is similar to JAK-STAT signaling activated by type 1 cytokines such as IL-6 in mammalian systems (155, 156). In D. melanogaster adults, a high-fat diet stimulated blood cells to secrete Upd3, which in turn activated JAK-STAT signaling in muscle and gut and caused decreased insulin sensitivity (156). Deletion or specific silencing of the Upd3 gene in blood cells reduces JAK-STAT activation and increases insulin sensitivity and lifespan of D. melanogaster under high-fat diet conditions, while fat storage is not affected (156). The release of Upd from blood cells on a high-fat diet can cause decreased insulin sensitivity in peripheral tissues, similar to the role of its mammalian homolog, the proinflammatory cytokine IL-6, in insulin resistance (157, 158). Previous research showed that activation of JAK-STAT in muscle by cytokines Upd2 and Upd3 released from blood cells helps D. melanogaster to resist parasitic wasp infection (159). At the same time, this parasitic wasp infection can cause decreased insulin signaling in the muscle and fat body (160). However, the relationship between JAK-STAT and IIS is not well understood. The study on high-fat diet seems to provide insights into the relationship between Upd-JAK-IIS under infection condition (156), that is, under infection of parasitic wasps, Upd3 released by blood cells may reduce insulin sensitivity of the muscle and fat body by activating JAK/STAT, thus reducing the anabolism of the muscle and fat body and saving energy for blood cell’s cellular immunity to against parasitic wasp infestation. Agaisse et al. found that bacterial infection can also trigger blood cell-specific expression of Upd3, which in turn activates TotA-mediated fat body immune responses via JAK-STAT-Rrelish (161). However, specific downregulation of the JAK-STAT pathway transcription factor Stat92E in the fat body did not affect the body’s triglyceride storage, suggesting that JAK-STAT does not autonomously regulate energy metabolism in the fat body (162). The roles of Upd cytokines and JAK-STAT on insulin signaling and their relationship with metabolism in Drosophila immune response remain to be further elucidated.
As the main component of insect blood sugar, trehalose is synthesized by trehalose-6-phosphate synthase (TPS) and trehalose phosphatase in insect fat body. Studies in houseflies Musca domestica show that trehalose-6-phosphate synthase (TPS) is involved in immune defense against pathogens by regulating the synthesis of trehalose (163). Studies have shown that systemic infection of pathogenic bacteria E. coli or Staphylococcus aureus can cause the increase of TPS transcription and trehalose content in the host, and the reduction of TPS content by RNA interference can lead to the increase of mortality of the host under infection condition, and this phenotype can be partially compensated by feeding trehalose (163).
Female parasitic wasps introduce various virulence factors into host insects during oviposition and realize parasitism by influencing host immunity and physiology. Recent studies have shown that female parasitic wasps Pachycrepoideus vindemiae use a novel venom protein, glucose-6-phosphate dehydrogenase (PvG6PDH), which affects carbohydrate metabolism by inhibiting the activity of glucose-6-phosphate (G6P) in host Drosophila to help achieve parasitism (164). However, the effect of PvG6PDH on the immune response of host insects during parasitism needs further study.
The fat body is an important site for energy reserves in insects, and infection with Mycobacterium marinum leads to progressive depletion of fat and glycogen in Drosophila, in part due to systemic AKT inactivation and FoxO dysregulation (14). Metabolic depletion phenotype similar to that described above after infection with Listeria monocytogenes (11). These metabolic phenotypes imply that the occurrence of systemic immunity will have an important impact on the body’s energy metabolism. These studies of core immune pathways demonstrate a direct link between metabolism and immune responses in the fat body energy redistribution at the molecular level (Figure 2).
Figure 2 Systematic molecular regulation of immune and metabolic pathways in Drosophila. The red line represents metabolic regulation under normal conditions; The black lines represent signal regulation in the state of immune activation. Within pathways activity regulation, the arrow represents facilitation and the Bar represents inhibition roles. Refer to the text for the interaction of each pathway.
In contrast to mammals which contain the closed circulatory system, the body cavity of insects is an open space filled with hemolymph (165). Insect hemolymph contains hemocytes and plasma, hemocytes are mainly macrophage-like plasmatocytes, which are involved in the cellular immune response (166). Insect hemocytes can be classified according to functional characteristics. In D. melanogaster hemocytes can be divided into three types: (1) plasmatocytes, the most abundant cell type in hemolymph, have phagocytosis and further differentiate into two other subtypes; (2) crystal cells that produce phenoloxidase (PO) and are involved in melanization; (3) Lamellocytes, flat adherent cells responsible for encapsulation of large foreign particles (16, 167–170). In some insects, granulocytes have the ability to adhere to foreign surfaces, similar to the Lamellocytes of D. melanogaster; in addition, spherulocytes have been found to transport components of the stratum corneum (171).
In D. melanogaster larvae, about 10% of the body’s glucose is needed to maintain quiescent hemocytes, and when cellular immunity is triggered, such as phagocytosis, the hemocytes expends more energy (8, 172). Immunometabolism mechanisms in vertebrate blood cells is not the focus of this review, and have been extensively discussed elsewhere (173–175). It is well understood that activated immune cells often need to switch from fatty acid oxidation to glycolysis, a phenomenon known as the Warburg effect, providing energy for rapid cellular proliferation and synthesis of antimicrobial effectors (173, 174). Once the physical barrier such as insect skin or intestinal membrane is compromised, and pathogens enter into insect body cavity, blood cells eliminate the invading pathogens through encapsulation, phagocytosis, nodulation, and melanization (176).
To gain a clear understanding of cellular immunity in insects, it is necessary to understand the metabolic reorganization of mammalian immune cells. In mammals, when immune cells are activated, it results in a metabolic switch within immune cells that is dependent on the supply of large amounts of glucose and glutamine (177). When immune cells are inactivated, they use glycolysis to produce pyruvate which enters the mitochondria and converted to aceytyl-CoA. Acetyl-CoA is also produced by β-oxidation of fatty acids used in the tricarboxylic acid (TCA) cycle linked to oxidative phosphorylation (OXPHOS) to generate ATP in the most efficient manner. Thus, glycolysis and β-oxidation synergies with oxidative phosphorylation (OXPHOS) to produce ATP for energy supply to quiescent immune cells (178). While this is a very metabolically efficient form of ATP production, the rate at which is too slow to meet cellular energy demands in an immune-activated state. Upon immune stimulation, immune cells switch from a state of low nutrient uptake to an optimized metabolic state, rapidly producing ATP and synthesizing a large number of new molecules, ATP production is mainly through glycolysis, albeit not very efficiently (only 2 ATP per glucose molecule), but produces ATP much faster than the glycolysis-TCA-OXPHOS (43). Glucose carbons which produced through glycolysis are used to a greater extent by activated immune cells to produce new immune-effector macromolecules, and are less lost as carbon dioxide (CO2) through the oxidative phosphorylation pathway (OXPHOS). The pentose phosphate pathway (PPP) is a branch of glycolysis that generates ribose for nucleotide synthesis and NADPH for ROS (179–181). But mitochondrial metabolism is significantly altered in activated immune cells, pyruvate is diverted from the TCA cycle, which is disrupted in the citrate step, and glutamine is metabolized by the interstitium in mitochondria, replenishing TCA intermediates, thus become another important metabolite of activated immune cells (181, 182). In short, massively increased glycolysis (supplying PPP, lipid synthesis, and leading to lactate production) following immune cell activation is associated with rewired mitochondrial metabolism (breaking the TCA cycle and inhibiting OXPHOS), which makes activated immune cells dependent on high-dose glucose and glutamine supply (177).
Metabolic reprogramming of activated immune cells has been studied primarily in mammalian systems, and although the bactericidal function of insect macrophages (hemocytes) is highly conserved with mammals, the metabolic remodeling of insect macrophages remains poorly understood. Nevertheless, the Warburg effect has also been found in insect cancer cells as well as in activated immune cells (183–186). Myc is an important regulator of immune cell metabolic remodeling, and in mammals, the metabolic reorganization of proliferating T lymphocytes is associated with Myc activation (187, 188). In insects, Myc is strongly expressed in blood cells of the highly proliferating Hop lethal mutant line (hop tuml) (189). Hypoxia-inducible factor 1α (HIF-1α) was originally found to regulate cellular metabolism by participating in mitochondrial oxidative phosphorylation under hypoxia (190). In mammals, activation of Toll-like receptors and NF-κB signaling leads to increased expression and enhanced stability of HIF-1α (191, 192). Krejčová et al. used adult D. melanogaster to study the metabolic changes of macrophages during the acute and remission phases of Streptococcus-induced sepsis and found that macrophage activation, bactericidal and fighting infection depends on the help of HIF-1α and lactate dehydrogenase homologues (193). This demonstrate the existence of a cellular metabolic mechanism in insects that is conserved with mammals, namely that macrophages induce systemic metabolic changes through aerobic glycolysis (193). Metabolic pathway studies during D. melanogaster plasmatocytes activation revealed that peroxisomes, an organelle related to lipid metabolism and oxidation reactions in plasmatocytes, are required for phagosome formation and antimicrobial peptide production, and this role conserved with mouse macrophages (194). Wasps such as Leptopilina boulardi parasitize D. melanogaster larvae by laying eggs that are too large to be phagocytosed (195). The D. melanogaster kill the invading parasite’s eggs by encapsulation them with lamellocytes, a type of differentiated hemocytes (196). During parasitoid infestation of Drosophila larvae, lamellocytes proliferation and differentiation are associated with increased blood cell-specific glycolytic gene expression, accompanied by increased glucose consumption and lactate production (8). In addition, similar findings have been made in other insects. For example, activation of phagocytes in Blaberus giganteus produces metabolic changes similar to the Warburg effect (172). The transcriptome analysis of immune-activated hemocytes in mosquitoes and Spodoptera exigua also revealed associations with glycolytic and LDH genes (150, 197–200). At present, studies based on a variety of insects have found that activated immune cells can significantly increase glucose consumption, glycolysis and lactic acid production, but the relationship between insect immunity and metabolism is still not as clear as that of mammals, and further investigation is needed.
During the immune response, metabolic regulation ensures that sufficient energy is available for an effective immune response, but since energy is finite, so immune responses must be properly regulated to accommodate the energy demands of other physiological characteristics. Like many immune responses, activation of lamellocyte requires reallocation of resources to fuel the differentiation and activation of blood cells (7). To this end, blood cells secrete extracellular adenosine (e-Ado), which enables other tissues to release stored glucose to provide energy for the activation of lamellocyte (8). However, the release of e-Ado must be regulated because the increased energy (glucose) of the blood body cavity is also available to pathogens. Later in infection, immune cells express the adenosine deaminase (ADGF-A) to regulate e-Ado levels and prevent pathogens from exploiting host resources (9). Interestingly, high levels of e-Ado were detected in human sepsis, suggesting that e-Ado has a similar effect in mammals (201, 202). Lin et al. found that adenosine receptor signaling (AdoR) is involved in the regulation of metabolic remodeling after budding virus (AcMNPV) infection in silkworm Bombyx mori, which can promote virus clearance and antiviral protein gloverin production (203). The study on another lepidopteran insect model Spodoptera frugiperda Sf-21 cells proved that in the metabolic regulation of Sf-21 cells after infection with baculovirus, adenosine signal transduction activates the host’s energy synthesis, supporting the innate immune response against infection, showing that glycolysis regulated by adenosine signaling pathway is a conserved mechanism (203). Another study on the regulation of adenosine metabolism showed that the symbiotic virus (SmBV) of the parasitic wasp Snellenius manilae was able to downregulate the extracellular adenosine (e-Ado) of the host Spodoptera litura, thereby inhibiting host metabolic switching and attenuating its immune response (204). This study provides us with a new perspective that the parasitoid symbiotic virus regulates the host’s adenosine pathway, allowing the eggs and larvae of S. manilae to evade the immune response of the host Spodoptera litura (Figure 2).
PGRP-SA and Spätzle, produced by hemocytes, are critical for the activation of Toll in fat, and their expression increased in response to pathogen infection (129, 205, 206). Because Toll activation leads not only to the expression of AMPs but also to the inhibition of insulin signaling, it implies that blood cells play a role in the activation of humoral immunity associated with metabolic switches through the expression of PGRP-SA and Spätzle. Cytokines released by blood cells have also been implicated in the control of metabolic homeostasis in D. melanogaster raised on a high-fat diet increased the expression of the Upd3 cytokine in blood cells, leading to systemic activation of JAK/STAT and decreased sensitivity to insulin (207). Under infection conditions, blood cells can not only participate in immune regulation but also regulate body metabolism by releasing cellular factors such as Eiger and Upd (150, 159).
The microbiota in insects is controlled by the host, i.e. the abundance, composition and location of microorganisms must all be within a certain range (208). For microorganisms, hemolymph is rich in nutrients, has a balanced ionic composition, and has a PH value close to neutral, which is very beneficial for their survival (208). However, the hemolymph is protected by the immune system, including blood cells and a variety of soluble effectors (antibacterial peptides, reactive oxygen species, phenoloxidases, etc.) that can kill, phagocytose, and encapsulate invading microorganisms to varying degrees (33). For a long time, research believed that in healthy insects, the hemolymph had little or no microorganisms (33). But recent studies have shown that various non-pathogenic microorganisms can stably or transiently inhabit within the hemolymph of a wide variety of insects (209). The most widely reported hemolymph microorganisms in insects are spiroplasmas of firmicutes, and these bacteria are mostly distributed in Hymenoptera, Hemiptera, Lepidoptera, Coleoptera and Diptera (209–211). Spiroplasmas are mainly found in the hemolymph or intestinal lumen, but can also be present in the fat body and ovary to cause intracellular infections (212). For example, the bacteria of spiroplasma in the hemolymph of D. melanogaster combine with yolk granules released from adipocytes and are transformed into developing oocytes through hemolymph to achieve vertical transmission (213). Moreover, a large number of bacterial members of the enterobacteriaceae (gamma-proteobacteria) are present in the hemolymph of insects, especially the three symbiotic bacteria found in aphids: Serratia Symbiotica and Hamiltonella defensa and Regiella insecticola, also known as secondary symbiotic bacteria (209). In some insects, the hemolymph microbiome also includes obligate intracellular symbiotic bacterial groups, such as Wolbachia and Rickettsia of the order Rickettsiales (alpha-proteobacteria), which are very common in insects (214, 215).
MAMPs of bacterial cell wall are target molecules recognized by insect immune receptors, and the interaction between hemolymph symbionts and the immune system depends on the extent of bacterial genome alterations. Genome sequencing of insect symbionts revealed that they suffer deletions in their gene sequences including virulence genes and bacterial cell wall element genes (216–219). Gene expression profiling of D. melanogaster with and without spiroplasma found no significant differences in the expression of various immune-related genes, including those expressing antimicrobial peptides (212, 220, 221). The reason behind this could be that Spiroplasma lacks the peptidoglycan cell wall of most bacteria and thus is not recognized by the host’s pattern recognition receptors to activate the insect’s immune response (33). Analysis of the hemolymph of D. melanogaster colonized with and without Spiroplasma showed increased amino acid concentrations and decreased lipid titers (triacylglycerol TAG and Diacylglycerol DAG), however, sugar and sterol levels and storage of the main carbohydrate glycogen were not significantly altered (213). This study further demonstrated that these host metabolic differences are mediated by Spiroplasma consumption of DAG, and reduced the production of insect lipoproteins (lipoproteins that transport DAG in hemolymph) by RNAi can significantly reduce the amount of DAG and Spiroplasma populations in insect hemolymph; Furthermore, in mutant flies that block fat mobilization from the fat body (specifically double mutations in the Brummer lipase and adipokinetic hormone receptor genes), Spiroplasma titers are not affected (213). These results suggest that DAG utilized by Spiroplasma are truncated dietary-derived lipids rather than stored lipids mobilized from the fat body and other organs. Spiroplasma has been shown to protect Drosophila melanogaster and Drosophila hydei from parasitic wasps (222, 223). Additionally, Spiroplasmas also protects Drosophila neotestacea from parasitic wasps and nematodes (Howardula aeronymphium), protecting the pea aphid (Acyrthosiphon pisum Harris) from pathogenic fungi (222–225). Among them, the protective effect of Spiroplasmas on host from parasitism was attributed to the influence of host metabolism, especially the competitive utilization of DAG by spiroplasmas in D. melanogaster (226).
To understand the metabolic effects of symbionts in host hemolymph of pea aphid, a comparative metabolomic analysis was conducted between aphid with secondary symbionts (Serratia symbiotica, Hamiltonella, Regiella) and without them (227). The results showed that there were significant differences in metabolites between the aphid with and without symbiosis, but the metabolomics characteristics of the aphid with different species of secondary symbiosis were highly similar, and the levels of amino acids in aphid samples containing secondary symbiotic bacteria increased compared to controls, while the levels of sugars and sugar alcohols decreased (227). These results suggest that the hemolymph symbiotic bacteria can affect host metabolism of aphid. Research on the effects of secondary symbiotic bacteria on the immune system of aphid mainly focused on cellular immune response. Laughton et al. used Serratia symbiotica, Hamiltonella defensa and Regiella insecticola to colonize aphid respectively, demonstrating increased hemocytes density in hemolymph samples from aphid colonized with Hamiltonella defensa and enhanced hemocytes response of encapsulation to Sephadex beads from aphid hemolymph colonized with Regiella insecticola, suggesting that the innate immune system of host insects can recognize these hemolymph symbiosis bacteria (228, 229). These findings suggest that the symbiotic bacteria in aphid hemolymph can affect the metabolism and immunity of the host, but whether there is a direct link between immune-metabolism remains to be further investigated.
Wolbachia were first identified in the mosquito’s germline over a century ago, and as an endosymbiont, they are surprisingly diverse and can co-exist with up to 66% of insect species (230). It can be stably inherited in the host population and influence the evolution, immunity, physiology and development of the host (231). Three major surface proteins have been identified in Wolbachia: wsp and its two analogs, wspA and wspB, which are associated with important pathogenic bacteria such as Ehrlichia and Neisseria membrane proteins with antigenic function are homologous (232–234). Studies in vertebrates have shown that after infection with Wolbachia-containing Filarial nematodes, the host can elicit an immune response by recognizing the Wolbachia membrane protein wsp (235), suggesting that Wolbachia may play a role in redirecting immune responses in vertebrates. In D. melanogaster, Wolbachia infection is widespread. Research has compared the differences in IIS-related phenotypes in the presence and absence of Wolbachia in IIS mutant flies. They show the absence of Wolbachia in IIS mutant flies further reduces IIS activity and indicating the role of Wolbachia in normal could increase host IIS activity and promote grow of host flies (236). In conclusion, the presence of the endosymbiotic Wolbachia can improve the IIS activity of the host, contrary to the influence of the pathogenic pathogen on the host IIS activity (236). Meanwhile, studies on the Wolbachia surface protein Wsp in vertebrates also showed that the Wolbachia can induce the host immune response (235).
Muscle is a major energy consuming organ, Zhao et al. found that feeding Pe (Pseudomonas entomophila) bacteria can induce differential expression of NF-κB signal in epidermal muscle tissue between individual D. melanogaster populations (237). D. melanogaster with restricted expression of NF-κB/IMD signal not only exhibited longer life span, but also showed increased defecation phenotype. In these individual’s muscle tissue, glutamate dehydrogenase (Gdh) expression is significantly upregulated in NF-κB signaling was mildly activated, leading to an increase in circulating glutamate content; glutamate can participate in vitamin metabolism mediated by sodium-dependent multivitamin transporter (svmt) after transported by glutamate transporter (dmGlut) into adipose tissue, ultimately leading to enhanced lipid mobilization and intestinal defecation response (237). These findings suggest that modest activation of NF-κB signaling in muscle tissue contributes to improved host survival under oral infection by mobilizing lipolysis in the fat body. Zhao et al. showed that oral bacterial infection can remotely regulate immune and metabolic reactions in the muscle and fat body to help the host eliminate bacteria (237), suggesting that such communication between organs is crucial to host homeostasis regulation under infection. Yang et al. also found multi-organ communication under oral bacterial infection, the IMD response of D. melanogaster is activated sequentially from the gut to the fat body under the mediating of the polyol pathway of sugar alcohols (238). The gut IMD activation leads to an increase in sorbitol concentration in the hemolymph, which in turn activates metalloproteinase 2 (Mmp2), and the activated Mmp2 cleaves PGRP-LC to activate the IMD response in the fat body (238).
Insects go through different developmental stages such as eggs, larvae, pupa, and adults throughout their lives. The interaction between microorganisms and insects exists in different developmental stages. Therefore, the coordinated regulation of immunity and metabolism is essential for the normal growth, development, and survival of insects. Toll, Imd, Eiger/TNF-α, JNK and JAK-STAT are the most important signals in the immune response, and they are activated by various immune stimuli. In addition to being critical for inducing immune responses, they also affect metabolism at various levels. Although immune-metabolism research in other invertebrates have not been extensively and comprehensively carried out, some existing examples are listed here (Table 1). At the same time, we summarized the molecular regulatory networks of immunity and metabolism in existing studies (Table 2).
Although current research has clarified the relationship between immunity and metabolism, the specific physiological functions of the interaction between the two, the differences in the immune-metabolism interaction between different sexes of insects, the effect of the brain on vertebrates and invertebrates between different organs and signal coordination remains to be further explored (69). The brain has extensive regulation of the body’s eating behavior and metabolism (251, 252), and studies have also confirmed the coordination of the brain’s immune response (253). Therefore, the regulation of the brain’s immunity and metabolism cannot be ignored. The advancement of technology makes personalized medicine possible; the above research issues should be paid more attention in future research. Insect, as an ancient and simple research model, especially D. melanogaster is a convenient means of genetic manipulation, so that we can use insect model research, not only to provide guidance for the study of immunometabolic diseases in vertebrates including humans, but also to provide strategies from the perspective of immunometabolism for the control of agricultural insect pests. The more hypotheses and proposals for future are shown as the Table 3.
SL, JW and XT were responsible for the study concept, design, and writing of the first draft. XT designed and drew the Figure and Table. ST provided literatures review and responsible for modify the content of the article. WH reviewed, proofread, and revised the draft. All authors contributed to the article and approved the submitted version.
This work was supported by the National Natural Science Foundation of China (32070507,32150410346, 32070495, 32372617), Open Fund Project of State Key Laboratory Of Silkworm Genome Biology (SKLSGB-ORP202114) and the Science and Technology Foundation of the Educational Commission of Jiangxi Province of China (GJJ211740), Self selected project of Shangrao Normal University (202137) and Science and Technology Commission of Shanghai Municipality (19ZR1466500). The funders had no role in study design, data collection and analysis, decision to publish, or preparation of the manuscript.
The authors declare that the research was conducted in the absence of any commercial or financial relationships that could be construed as a potential conflict of interest.
All claims expressed in this article are solely those of the authors and do not necessarily represent those of their affiliated organizations, or those of the publisher, the editors and the reviewers. Any product that may be evaluated in this article, or claim that may be made by its manufacturer, is not guaranteed or endorsed by the publisher.
1. Hotamisligil GS. Inflammation, metaflammation and immunometabolic disorders. Nature (2017) 542(7640):177–85. doi: 10.1038/nature21363
2. Tauber AI. Metchnikoff and the phagocytosis theory. Nat Rev Mol Cell Biol (2003) 4(11):897–901. doi: 10.1038/nrm1244
3. Esser N, Legrand-Poels S, Piette J, Scheen AJ, Paquot N. Inflammation as a link between obesity, metabolic syndrome and type 2 diabetes. Diabetes Res Clin Pract (2014) 105(2):141–50. doi: 10.1016/j.diabres.2014.04.006
4. Gregor MF, Hotamisligil GS. Inflammatory mechanisms in obesity. Annu Rev Immunol (2011) 29:415–45. doi: 10.1146/annurev-immunol-031210-101322
5. Hotamisligil GS, Erbay E. Nutrient sensing and inflammation in metabolic diseases. Nat Rev Immunol (2008) 8(12):923–34. doi: 10.1038/nri2449
6. Fong YM, Marano MA, Moldawer LL, Wei H, Calvano SE, Kenney JS, et al. The acute splanchnic and peripheral tissue metabolic response to endotoxin in humans. J Clin Invest (1990) 85(6):1896–904. doi: 10.1172/JCI114651
7. Straub RH, Cutolo M, Buttgereit F, Pongratz G. Energy regulation and neuroendocrine-immune control in chronic inflammatory diseases. J Intern Med (2010) 267(6):543–60. doi: 10.1111/j.1365-2796.2010.02218.x
8. Bajgar A, Kucerova K, Jonatova L, Tomcala A, Schneedorferova I, Okrouhlik J, et al. Extracellular adenosine mediates a systemic metabolic switch during immune response. PloS Biol (2015) 13(4):e1002135. doi: 10.1371/journal.pbio.1002135
9. Bajgar A, Dolezal T. Extracellular adenosine modulates host-pathogen interactions through regulation of systemic metabolism during immune response in Drosophila. PloS Pathog (2018) 14(4):e1007022. doi: 10.1371/journal.ppat.1007022
10. Arnold PA, Johnson KN, White CR. Physiological and metabolic consequences of viral infection in Drosophila melanogaster. J Exp Biol (2013) 216(Pt 17):3350–7. doi: 10.1242/jeb.088138
11. Chambers MC, Song KH, Schneider DS. Listeria monocytogenes infection causes metabolic shifts in Drosophila melanogaster. PloS One (2012) 7(12):e50679. doi: 10.1371/journal.pone.0050679
12. Gray EM, Bradley TJ. Malarial infection in Aedes aEgypti: effects on feeding, fecundity and metabolic rate. Parasitology (2006) 132(Pt 2):169–76. doi: 10.1017/S0031182005008966
13. Ibrahim E, Dobes P, Kunc M, Hyrsl P, Kodrik D. Adipokinetic hormone and adenosine interfere with nematobacterial infection and locomotion in Drosophila melanogaster. J Insect Physiol (2018) 107:167–74. doi: 10.1016/j.jinsphys.2018.04.002
14. Dionne MS, Pham LN, Shirasu-Hiza M, Schneider DS. AKT and FOXO dysregulation contribute to infection-induced wasting in Drosophila. Curr Biol (2006) 16(20):1977–85. doi: 10.1016/j.cub.2006.08.052
15. Schwartsburd PM. Catabolic and anabolic faces of insulin resistance and their disorders: a new insight into circadian control of metabolic disorders leading to diabetes. Future Sci OA (2017) 3(3):FSO201. doi: 10.4155/fsoa-2017-0015
16. Fernandez R, Tabarini D, Azpiazu N, Frasch M, Schlessinger J. The Drosophila insulin receptor homolog: a gene essential for embryonic development encodes two receptor isoforms with different signaling potential. EMBO J (1995) 14(14):3373–84. doi: 10.1002/j.1460-2075.1995.tb07343.x
17. Brogiolo W, Stocker H, Ikeya T, Rintelen F, Fernandez R, Hafen E. An evolutionarily conserved function of the Drosophila insulin receptor and insulin-like peptides in growth control. Curr Biol (2001) 11(4):213–21. doi: 10.1016/s0960-9822(01)00068-9
18. Grönke S, Clarke D-F, Broughton S, Andrews TD, Partridge L. Molecular evolution and functional characterization of Drosophila insulin-like peptides. PloS Genet (2010) 6(2):e1000857. doi: 10.1371/journal.pgen.1000857
19. Colombani J, Andersen DS, Léopold P. Secreted peptide Dilp8 coordinates Drosophila tissue growth with developmental timing. Science (2012) 336:582–5. doi: 10.1126/science.1216689
20. Semaniuk U, Strilbytska O, Malinovska K, Storey KB, Vaiserman A, Lushchak V, et al. Factors that regulate expression patterns of insulin-like peptides and their association with physiological and metabolic traits in Drosophila. Insect Biochem Mol Biol (2021) 135:103609. doi: 10.1016/j.ibmb.2021.103609
21. Böhni R, Riesgo-Escovar J, Oldham S, Brogiolo W, Stocker H, Andruss BF, et al. Autonomous control of cell and organ size by CHICO, a Drosophila homolog of vertebrate IRS1-4. Cell (1999) 97(7):865–75. doi: 10.1016/s0092-8674(00)80799-0
22. Accili D, Arden KC. FoxOs at the crossroads of cellular metabolism, differentiation, and transformation. Cell (2004) 117(4):421–6. doi: 10.1016/s0092-8674(04)00452-0
23. Hekimi S, Guarente L. Genetics and the specificity of the aging process. Science (2003) 299(5611):1352–4. doi: 10.1126/science.1082358
24. Kenyon C. A conserved regulatory system for aging. Cell (2001) 105(2):165–8. doi: 10.1016/s0092-8674(01)00306-3
25. Karpac J, Jasper H. Insulin and JNK: optimizing metabolic homeostasis and lifespan. Trends Endocrinol Metab (2009) 20(3):100–6. doi: 10.1016/j.tem.2008.11.004
26. Tatar M, Bartke A, Antebi A. The endocrine regulation of aging by insulin-like signals. Science (2003) 299(5611):1346–51. doi: 10.1126/science.1081447
27. Libert S, Chao Y, Zwiener J, Pletcher SD. Realized immune response is enhanced in long-lived puc and chico mutants but is unaffected by dietary restriction. Mol Immunol (2008) 45(3):810–7. doi: 10.1016/j.molimm.2007.06.353
28. McCormack S, Yadav S, Shokal U, Kenney E, Cooper D, Eleftherianos I. The insulin receptor substrate Chico regulates antibacterial immune function in Drosophila. Immun Ageing (2016) 13:15. doi: 10.1186/s12979-016-0072-1
29. Musselman LP, Fink JL, Grant AR, Gatto JA, Tuthill BF 2nd, Baranski TJ. A complex relationship between immunity and metabolism in Drosophila diet-induced insulin resistance. Mol Cell Biol (2018) 38(2):e00259–17. doi: 10.1128/MCB.00259-17
30. de Roode JC, Lefèvre T. Behavioral immunity in insects. Insects (2012) 3(3):789–820. doi: 10.3390/insects3030789
31. Davis MM, Engström Y. Immune response in the barrier epithelia: lessons from the fruit fly Drosophila melanogaster. J Innate Immun (2012) 4(3):273–83. doi: 10.1159/000332947
32. Erlandson MA, Toprak U, Hegedus DD. Role of the peritrophic matrix in insect-pathogen interactions. J Insect Physiol (2019) 117:103894. doi: 10.1016/j.jinsphys.2019.103894
33. Lemaitre B, Hoffmann J. The host defense of Drosophila melanogaster. Annu Rev Immunol (2007) 25:697–743. doi: 10.1146/annurev.immunol.25.022106.141615
34. Lemaitre B, Reichhart JM, Hoffmann JA. Drosophila host defense: Differential induction of antimicrobial peptide genes after infection by various classes of microorganisms. Proc Natl Acad Sci USA (1997) 94(26):14614–9. doi: 10.1073/pnas.94.26.14614
35. Lemaitre B, Kromer-Metzger E, Michaut L, Nicolas E, Meister M, Georgel P, et al. A recessive mutation, immune deficiency (imd), defines two distinct control pathways in the Drosophila host defense. Proc Natl Acad Sci USA (1995) 92(21):9465–9. doi: 10.1073/pnas.92.21.9465
36. Buchon N, Silverman N, Cherry S. Immunity in Drosophila melanogaster–from microbial recognition to whole-organism physiology. Nat Rev Immunol (2014) 14(12):796–810. doi: 10.1038/nri3763
37. Myllymäki H, Valanne S, Rämet M. The Drosophila imd signaling pathway. J Immunol (2014) 192(8):3455–62. doi: 10.4049/jimmunol.1303309
38. Valanne S, Wang J-H, Rämet M. The Drosophila Toll signaling pathway. J Immunol (2011) 186(2):649–56. doi: 10.4049/jimmunol.1002302
39. Kaneko T, Golenbock D, Silverman N. Peptidoglycan recognition by the Drosophila Imd pathway. J Endotoxin Res (2005) 11(6):383–9. doi: 10.1179/096805105X76823
40. El Chamy L, Leclerc V, Caldelari I, Reichhart JM. Sensing of ‘danger signals’ and pathogen-associated molecular patterns defines binary signaling pathways ‘upstream’ of Toll. Nat Immunol (2008) 9(10):1165–70. doi: 10.1038/ni.1643
41. Hultmark D. Drosophila immunity: paths and patterns. Curr Opin Immunol (2003) 15(1):12–9. doi: 10.1016/s0952-7915(02)00005-5
42. McKean KA, Yourth CP, Lazzaro BP, Clark AG. The evolutionary costs of immunological maintenance and deployment. BMC Evol Biol (2008) 8:76. doi: 10.1186/1471-2148-8-76
43. Dolezal T, Krejcova G, Bajgar A, Nedbalova P, Strasser P. Molecular regulations of metabolism during immune response in insects. Insect Biochem Mol Biol (2019) 109:31–42. doi: 10.1016/j.ibmb.2019.04.005
44. Huang JH, Jing X, Douglas AE. The multi-tasking gut epithelium of insects. Insect Biochem Mol Biol (2015) 67:15–20. doi: 10.1016/j.ibmb.2015.05.004
45. Miguel-Aliaga I, Jasper H, Lemaitre B. Anatomy and physiology of the digestive tract of Drosophila melanogaster. Genetics (2018) 210(2):357–96. doi: 10.1534/genetics.118.300224
46. Chng WA, Hietakangas V, Lemaitre B. Physiological adaptations to sugar intake: new paradigms from Drosophila melanogaster. Trends Endocrinol Metab (2017) 28(2):131–42. doi: 10.1016/j.tem.2016.11.003
47. Lemaitre B, Miguel-Aliaga I. The digestive tract of Drosophila melanogaster. Annu Rev Genet (2013) 47:377–404. doi: 10.1146/annurev-genet-111212-133343
48. Ren C, Webster P, Finkel SE, Tower J. Increased internal and external bacterial load during Drosophila aging without life-span trade-off. Cell Metab (2007) 6(2):144–52. doi: 10.1016/j.cmet.2007.06.006
49. Ankrah NYD, Wilkes RA, Zhang FQ, Zhu D, Kaweesi T, Aristilde L, et al. Syntrophic splitting of central carbon metabolism in host cells bearing functionally different symbiotic bacteria. ISME J (2020) 14(8):1982–93. doi: 10.1038/s41396-020-0661-z
50. Micchelli CA, Perrimon N. Evidence that stem cells reside in the adult Drosophila midgut epithelium. Nature (2006) 439(7075):475–9. doi: 10.1038/nature04371
51. Ohlstein B, Spradling A. Multipotent Drosophila intestinal stem cells specify daughter cell fates by differential Notch signaling. Science (2007) 315(5814):988–92. doi: 10.1126/science.1136606
52. Ohlstein B, Spradling A. The adult Drosophila posterior midgut is maintained by pluripotent stem cells. Nature (2006) 439(7075):470–4. doi: 10.1038/nature04333
53. Gomes FM, Carvalho DB, MaChado EA, Miranda K. Ultrastructural and functional analysis of secretory goblet cells in the midgut of the lepidopteran Anticarsia gemmatalis. Cell Tissue Res (2013) 352(2):313–26. doi: 10.1007/s00441-013-1563-4
54. Shanbhag S, Tripathi S. Epithelial ultrastructure and cellular mechanisms of acid and base transport in the Drosophila midgut. J Exp Biol (2009) 212(Pt 11):1731–44. doi: 10.1242/jeb.029306
55. Wu K, Yang B, Huang W, Dobens L, Song H, Ling E. Gut immunity in Lepidopteran insects. Dev Comp Immunol (2016) 64:65–74. doi: 10.1016/j.dci.2016.02.010
56. Lehane MJ. Peritrophic matrix structure and function. Annu Rev Entomol (1997) 42:520–50. doi: 10.1146/annurev.ento.42.1.525
57. Hegedus D, Erlandson M, Gillott C, Toprak U. New insights into peritrophic matrix synthesis, architecture, and function. Annu Rev Entomol (2009) 54:285–302. doi: 10.1146/annurev.ento.54.110807.090559
58. Buchon N, Broderick NA, Lemaitre B. Gut homeostasis in a microbial world: insights from Drosophila melanogaster. Nat Rev Microbiol (2013) 11(9):615–26. doi: 10.1038/nrmicro3074
59. Vodovar N, Vinals M, Liehl P, Basset A, Degrouard J, Spellman P, et al. Drosophila host defense after oral infection by an entomopathogenic Pseudomonas species. Proc Natl Acad Sci USA (2005) 102(32):11414–9. doi: 10.1073/pnas.0502240102
60. Syed ZA, Härd T, Uv A, van Dijk-Härd IF. A potential role for Drosophila mucins in development and physiology. PloS One (2008) 3(8):e3041. doi: 10.1371/journal.pone.0003041
61. Buchon N, Broderick NA, Poidevin M, Pradervand S, Lemaitre B. Drosophila intestinal response to bacterial infection: activation of host defense and stem cell proliferation. Cell Host Microbe (2009) 5(2):200–11. doi: 10.1016/j.chom.2009.01.003
62. Lee KA, Kim SH, Kim EK, Ha EM, You H, Kim B, et al. Bacterial-derived uracil as a modulator of mucosal immunity and gut-microbe homeostasis in Drosophila. Cell (2013) 153(4):797–811. doi: 10.1016/j.cell.2013.04.009
63. Lee KA, Kim B, Bhin J, Kim DH, You H, Kim EK, et al. Bacterial uracil modulates Drosophila DUOX-dependent gut immunity via Hedgehog-induced signaling endosomes. Cell Host Microbe (2015) 17(2):191–204. doi: 10.1016/j.chom.2014.12.012
64. Bosco-Drayon V, Poidevin M, Boneca IG, Narbonne-Reveau K, Royet J, Charroux B. Peptidoglycan sensing by the receptor PGRP-LE in the Drosophila gut induces immune responses to infectious bacteria and tolerance to microbiota. Cell Host Microbe (2012) 12(2):153–65. doi: 10.1016/j.chom.2012.06.002
65. Neyen C, Poidevin M, Roussel A, Lemaitre B. Tissue- and ligand-specific sensing of gram-negative infection in Drosophila by PGRP-LC isoforms and PGRP-LE. J Immunol (2012) 189(4):1886–97. doi: 10.4049/jimmunol.1201022
66. Kaneko T, Goldman WE, Mellroth P, Steiner H, Fukase K, Kusumoto S, et al. Monomeric and polymeric gram-negative peptidoglycan but not purified LPS stimulate the Drosophila IMD pathway. Immunity (2004) 20(5):637–49. doi: 10.1016/s1074-7613(04)00104-9
67. Leulier F, Parquet C, Pili-Floury S, Ryu JH, Caroff M, Lee WJ, et al. The Drosophila immune system detects bacteria through specific peptidoglycan recognition. Nat Immunol (2003) 4(5):478–84. doi: 10.1038/ni922
68. Buchon N, Broderick NA, Chakrabarti S, Lemaitre B. Invasive and indigenous microbiota impact intestinal stem cell activity through multiple pathways in Drosophila. Genes Dev (2009) 23(19):2333–44. doi: 10.1101/gad.1827009
69. Lee KA, Lee WJ. Immune-metabolic interactions during systemic and enteric infection in Drosophila. Curr Opin Insect Sci (2018) 29:21–6. doi: 10.1016/j.cois.2018.05.014
70. Chakrabarti S, Liehl P, Buchon N, Lemaitre B. Infection-induced host translational blockage inhibits immune responses and epithelial renewal in the Drosophila gut. Cell Host Microbe (2012) 12(1):60–70. doi: 10.1016/j.chom.2012.06.001
71. Wang B, Pakpour N, Napoli E, Drexler A, Glennon EK, Surachetpong W, et al. Anopheles stephensi p38 MAPK signaling regulates innate immunity and bioenergetics during Plasmodium falciparum infection. Parasit Vectors (2015) 8:424. doi: 10.1186/s13071-015-1016-x
72. Wolfe AJ. The acetate switch. Microbiol Mol Biol Rev (2005) 69(1):12–50. doi: 10.1128/MMBR.69.1.12-50.2005
73. Hang S, Purdy AE, Robins WP, Wang Z, Mandal M, Chang S, et al. The acetate switch of an intestinal pathogen disrupts host insulin signaling and lipid metabolism. Cell Host Microbe (2014) 16(5):592–604. doi: 10.1016/j.chom.2014.10.006
74. Fast D, Kostiuk B, Foley E, Pukatzki S. Commensal pathogen competition impacts host viability. Proc Natl Acad Sci USA (2018) 115(27):7099–104. doi: 10.1073/pnas.1802165115
75. Harsh S, Heryanto C, Eleftherianos I. Intestinal lipid droplets as novel mediators of host-pathogen interaction in Drosophila. Biol Open (2019) 8(7):bio039040. doi: 10.1242/bio.039040
76. Kapahi P, Zid BM, Harper T, Koslover D, Sapin V, Benzer S. Regulation of lifespan in Drosophila by modulation of genes in the TOR signaling pathway. Curr Biol (2004) 14(10):885–90. doi: 10.1016/j.cub.2004.03.059
77. Fuchs S, Behrends V, Bundy JG, Crisanti A, Nolan T. Phenylalanine metabolism regulates reproduction and parasite melanization in the malaria mosquito. PloS One (2014) 9(1):e84865. doi: 10.1371/journal.pone.0084865
78. Nicholson JK, Holmes E, Kinross J, Burcelin R, Gibson G, Jia W, et al. Host-gut microbiota metabolic interactions. Science (2012) 336(6086):1262–7. doi: 10.1126/science.1223813
79. Tremaroli V, Bäckhed F. Functional interactions between the gut microbiota and host metabolism. Nature (2012) 489(7415):242–9. doi: 10.1038/nature11552
80. Belkaid Y, Hand TW. Role of the microbiota in immunity and inflammation. Cell (2014) 157(1):121–41. doi: 10.1016/j.cell.2014.03.011
81. Nash MJ, Frank DN, Friedman JE. Early microbes modify immune system development and metabolic homeostasis-the “Restaurant” Hypothesis revisited. Front Endocrinol (Lausanne) (2017) 8:349. doi: 10.3389/fendo.2017.00349
82. Postler TS, Ghosh S. Understanding the holobiont: how microbial metabolites affect human health and shape the immune system. Cell Metab (2017) 26(1):110–30. doi: 10.1016/j.cmet.2017.05.008
83. Flint HJ, Scott KP, Duncan SH, Louis P, Forano E. Microbial degradation of complex carbohydrates in the gut. Gut Microbes (2012) 3(4):289–306. doi: 10.4161/gmic.19897
84. LeBlanc JG, Milani C, de Giori GS, Sesma F, van Sinderen D, Ventura M. Bacteria as vitamin suppliers to their host: a gut microbiota perspective. Curr Opin Biotechnol (2013) 24(2):160–8. doi: 10.1016/j.copbio.2012.08.005
85. Donohoe DR, Garge N, Zhang X, Sun W, O’Connell TM, Bunger MK, et al. The microbiome and butyrate regulate energy metabolism and autophagy in the mammalian colon. Cell Metab (2011) 13(5):517–26. doi: 10.1016/j.cmet.2011.02.018
86. Ryu J-H, Kim S-H, Lee H-Y, Bai JY, Nam Y-D, Bae J-W, et al. Innate immune homeostasis by the homeobox gene caudal and commensal-gut mutualism in Drosophila. Science (2008) 319(5864):777–82. doi: 10.1126/science.1149357
87. Cox CR, Gilmore MS. Native microbial colonization of Drosophila melanogaster and its use as a model of Enterococcus faecalis pathogenesis. Infect Immun (2007) 75(4):1565–76. doi: 10.1128/IAI.01496-06
88. Chandler JA, Lang JM, Bhatnagar S, Eisen JA, Kopp A. Bacterial communities of diverse Drosophila species: ecological context of a host-microbe model system. PloS Genet (2011) 7(9):e1002272. doi: 10.1371/journal.pgen.1002272
89. Bakula M. The persistence of a microbial flora during postembryogenesis of Drosophila melanogaster. J Invertebr Pathol (1996) 14(3):365–74. doi: 10.1016/0022-2011(69)90163-3
90. Storelli G, Defaye A, Erkosar B, Hols P, Royet J, Leulier F. Lactobacillus plantarum promotes Drosophila systemic growth by modulating hormonal signals through TOR-dependent nutrient sensing. Cell Metab (2011) 14(3):403–14. doi: 10.1016/j.cmet.2011.07.012
91. Erkosar B, Storelli G, Mitchell M, Bozonnet L, Bozonnet N, Leulier F. Pathogen virulence impedes mutualist-mediated enhancement of host juvenile growth via inhibition of protein digestion. Cell Host Microbe (2015) 18(4):445–55. doi: 10.1016/j.chom.2015.09.001
92. Broderick NA, Buchon N, Lemaitre B. Microbiota-induced changes in Drosophila melanogaster host gene expression and gut morphology. mBio (2014) 5(3):e01117–14. doi: 10.1128/mBio.01117-14
93. Dobson AJ, Chaston JM, Douglas AE. The Drosophila transcriptional network is structured by microbiota. BMC Genomics (2016) 17(1):975. doi: 10.1186/s12864-016-3307-9
94. Erkosar B, Defaye A, Bozonnet N, Puthier D, Royet J, Leulier F. Drosophila microbiota modulates host metabolic gene expression via IMD/NF-κB signaling. PloS One (2014) 9(4):e94729. doi: 10.1371/journal.pone.0094729
95. Petkau K, Ferguson M, Guntermann S, Foley E. Constitutive immune activity promotes tumorigenesis in Drosophila intestinal progenitor cells. Cell Rep (2017) 20(8):1784–93. doi: 10.1016/j.celrep.2017.07.078
96. Martino ME, Joncour P, Leenay R, Gervais H, Shah M, Hughes S, et al. Bacterial adaptation to the host’s diet is a key evolutionary force shaping Drosophila-lactobacillus symbiosis. Cell Host Microbe (2018) 24(1):109–19 e6. doi: 10.1016/j.chom.2018.06.001
97. Storelli G, Strigini M, Grenier T, Bozonnet L, Schwarzer M, Daniel C, et al. Drosophila perpetuates nutritional mutualism by promoting the fitness of its intestinal symbiont Lactobacillus plantarum. Cell Metab (2018) 27(2):362–77 e8. doi: 10.1016/j.cmet.2017.11.011
98. Vandehoef C, Molaei M, Karpac J. Dietary adaptation of microbiota in Drosophila requires NF-κB-dependent control of the translational regulator 4E-BP. Cell Rep (2020) 31(10):107736. doi: 10.1016/j.celrep.2020.107736
99. Shin SC, Kim S-H, You H, Kim B, Kim AC, Lee K-A, et al. Drosophila microbiome modulates host developmental and metabolic homeostasis via insulin signaling. Science (2011) 334(6056):670–4. doi: 10.1126/science.1212782
100. Corrêa-Oliveira R, Fachi JL, Vieira A, Sato FT, Vinolo MAR. Regulation of immune cell function by short-chain fatty acids. Clin Transl Immunol (2016) 5(4):e73. doi: 10.1038/cti.2016.17
101. Kim Y, Ahmed S, Stanley D, An C. Eicosanoid-mediated immunity in insects. Dev Comp Immunol (2018) 83:130–43. doi: 10.1016/j.dci.2017.12.005
102. Lee WJ, Hase K. Gut microbiota-generated metabolites in animal health and disease. Nat Chem Biol (2014) 10(6):416–24. doi: 10.1038/nchembio.1535
103. Morrison DJ, Preston T. Formation of short chain fatty acids by the gut microbiota and their impact on human metabolism. Gut Microbes (2016) 7(3):189–200. doi: 10.1080/19490976.2015.1134082
104. Ríos-Covián D, Ruas-Madiedo P, Margolles A, Gueimonde M, de Los Reyes-Gavilán CG, Salazar N. Intestinal short chain fatty acids and their link with diet and human health. Front Microbiol (2016) 7:185. doi: 10.3389/fmicb.2016.00185
105. Kamareddine L, Robins WP, Berkey CD, Mekalanos JJ, Watnick PI. The Drosophila immune deficiency pathway modulates enteroendocrine function and host metabolism. Cell Metab (2018) 28(3):449–62 e5. doi: 10.1016/j.cmet.2018.05.026
106. Amcheslavsky A, Song W, Li Q, Nie Y, Bragatto I, Ferrandon D, et al. Enteroendocrine cells support intestinal stem-cell-mediated homeostasis in Drosophila. Cell Rep (2014) 9(1):32–9. doi: 10.1016/j.celrep.2014.08.052
107. Song W, Veenstra JA, Perrimon N. Control of lipid metabolism by tachykinin in Drosophila. Cell Rep (2014) 9(1):40–7. doi: 10.1016/j.celrep.2014.08.060
108. Sannino DR, Dobson AJ, Edwards K, Angert ER, Buchon N. The Drosophila melanogaster gut microbiota provisions thiamine to its host. mBio (2018) 9(2):e00155–18. doi: 10.1128/mBio.00155-18
109. Wong AC, Dobson AJ, Douglas AE. Gut microbiota dictates the metabolic response of Drosophila to diet. J Exp Biol (2014) 217(Pt 11):1894–901. doi: 10.1242/jeb.101725
110. Sommer AJ, Newell PD. Metabolic basis for mutualism between gut bacteria and its impact on the Drosophila melanogaster host. Appl Environ Microbiol (2019) 85(2):e01882–18. doi: 10.1128/AEM.01882-18
111. Adair KL, Wilson M, Bost A, Douglas AE. Microbial community assembly in wild populations of the fruit fly Drosophila melanogaster. ISME J (2018) 12(4):959–72. doi: 10.1038/s41396-017-0020-x
112. Corby-Harris V, Pontaroli AC, Shimkets LJ, Bennetzen JL, Habel KE, Promislow DE. Geographical distribution and diversity of bacteria associated with natural populations of Drosophila melanogaster. Appl Environ Microbiol (2007) 73(11):3470–9. doi: 10.1128/AEM.02120-06
113. Staubach F, Baines JF, Kunzel S, Bik EM, Petrov DA. Host species and environmental effects on bacterial communities associated with Drosophila in the laboratory and in the natural environment. PloS One (2013) 8(8):e70749. doi: 10.1371/journal.pone.0070749
114. Wong AC, Chaston JM, Douglas AE. The inconstant gut microbiota of Drosophila species revealed by 16S rRNA gene analysis. ISME J (2013) 7(10):1922–32. doi: 10.1038/ismej.2013.86
115. Pais IS, Valente RS, Sporniak M, Teixeira L. Drosophila melanogaster establishes a species-specific mutualistic interaction with stable gut-colonizing bacteria. PloS Biol (2018) 16(7):e2005710. doi: 10.1371/journal.pbio.2005710
116. Chu H, Mazmanian SK. Innate immune recognition of the microbiota promotes host-microbial symbiosis. Nat Immunol (2013) 14(7):668–75. doi: 10.1038/ni.2635
117. Bischoff V, Vignal C, Duvic B, Boneca IG, Hoffmann JA, Royet J. Downregulation of the Drosophila immune response by peptidoglycan-recognition proteins SC1 and SC2. PloS Pathog (2006) 2(2):e14. doi: 10.1371/journal.ppat.0020014
118. Dziarski R, Gupta D. The peptidoglycan recognition proteins (PGRPs). Genome Biol (2006) 7(8):232. doi: 10.1186/gb-2006-7-8-232
119. Guo L, Karpac J, Tran Susan L, Jasper H. PGRP-SC2 promotes gut immune homeostasis to limit commensal dysbiosis and extend lifespan. Cell (2014) 156(1-2):109–22. doi: 10.1016/j.cell.2013.12.018
120. Lee KA, Cho KC, Kim B, Jang IH, Nam K, Kwon YE, et al. Inflammation-modulated metabolic reprogramming is required for DUOX-dependent gut immunity in Drosophila. Cell Host Microbe (2018) 23(3):338–52 e5. doi: 10.1016/j.chom.2018.01.011
121. Ha EM, Lee KA, Seo YY, Kim SH, Lim JH, Oh BH, et al. Coordination of multiple dual oxidase-regulatory pathways in responses to commensal and infectious microbes in Drosophila gut. Nat Immunol (2009) 10(9):949–57. doi: 10.1038/ni.1765
122. Jones RM, Luo L, Ardita CS, Richardson AN, Kwon YM, Mercante JW, et al. Symbiotic lactobacilli stimulate gut epithelial proliferation via Nox-mediated generation of reactive oxygen species. EMBO J (2013) 32(23):3017–28. doi: 10.1038/emboj.2013.224
123. Lhocine N, Ribeiro PS, Buchon N, Wepf A, Wilson R, Tenev T, et al. PIMS modulates immune tolerance by negatively regulating Drosophila innate immune signaling. Cell Host Microbe (2008) 4(2):147–58. doi: 10.1016/j.chom.2008.07.004
124. Zhu L, Qi S, Xue X, Niu X, Wu L. Nitenpyram disturbs gut microbiota and influences metabolic homeostasis and immunity in honey bee (Apis mellifera L.). Environ pollut (2020) 258:113671. doi: 10.1016/j.envpol.2019.113671
125. Lehmann M. Endocrine and physiological regulation of neutral fat storage in Drosophila. Mol Cell Endocrinol (2018) 461:165–77. doi: 10.1016/j.mce.2017.09.008
126. Wagner A. Energy constraints on the evolution of gene expression. Mol Biol Evol (2005) 22(6):1365–74. doi: 10.1093/molbev/msi126
127. Hotamisligil GS. Inflammation and metabolic disorders. Nature (2006) 444(7121):860–7. doi: 10.1038/nature05485
128. Agrawal N, Delanoue R, Mauri A, Basco D, Pasco M, Thorens B, et al. The Drosophila TNF eiger is an adipokine that acts on insulin-producing cells to mediate nutrient response. Cell Metab (2016) 23(4):675–84. doi: 10.1016/j.cmet.2016.03.003
129. Boutros M, Agaisse H, Perrimon N. Sequential activation of signaling pathways during innate immune responses in Drosophila. Dev Cell (2002) 3(5):711–22. doi: 10.1016/s1534-5807(02)00325-8
130. DiAngelo JR, Bland ML, Bambina S, Cherry S, Birnbaum MJ. The immune response attenuates growth and nutrient storage in Drosophila by reducing insulin signaling. Proc Natl Acad Sci USA (2009) 106(49):20853–8. doi: 10.1073/pnas.0906749106
131. Davoodi S, Galenza A, Panteluk A, Deshpande R, Ferguson M, Grewal S, et al. The immune deficiency pathway regulates metabolic homeostasis in Drosophila. J Immunol (2019) 202(9):2747–59. doi: 10.4049/jimmunol.1801632
132. Clark RI, Tan SW, Pean CB, Roostalu U, Vivancos V, Bronda K, et al. MEF2 is an in vivo immune-metabolic switch. Cell (2013) 155(2):435–47. doi: 10.1016/j.cell.2013.09.007
133. Rera M, Clark RI, Walker DW. Intestinal barrier dysfunction links metabolic and inflammatory markers of aging to death in Drosophila. Proc Natl Acad Sci USA (2012) 109(52):21528–33. doi: 10.1073/pnas.1215849110
134. Roth SW, Bitterman MD, Birnbaum MJ, Bland ML. Innate immune signaling in Drosophila blocks insulin signaling by uncoupling PI(3,4,5)P3 production and AKT activation. Cell Rep (2018) 22(10):2550–6. doi: 10.1016/j.celrep.2018.02.033
135. Martinez BA, Hoyle RG, Yeudall S, Granade ME, Harris TE, Castle JD, et al. Innate immune signaling in Drosophila shifts anabolic lipid metabolism from triglyceride storage to phospholipid synthesis to support immune function. PloS Genet (2020) 16(11):e1009192. doi: 10.1371/journal.pgen.1009192
136. Harvey K, Tapon N. The Salvador-Warts-Hippo pathway - an emerging tumour-suppressor network. Nat Rev Cancer (2007) 7(3):182–91. doi: 10.1038/nrc2070
137. Johnson R, Halder G. The two faces of Hippo: targeting the Hippo pathway for regenerative medicine and cancer treatment. Nat Rev Drug Discovery (2014) 13(1):63–79. doi: 10.1038/nrd4161
138. Pan D. The hippo signaling pathway in development and cancer. Dev Cell (2010) 19(4):491–505. doi: 10.1016/j.devcel.2010.09.011
139. Sharma A, Yerra VG, Kumar A. Emerging role of Hippo signalling in pancreatic biology: YAP re-expression and plausible link to islet cell apoptosis and replication. Biochimie (2017) 133:56–65. doi: 10.1016/j.biochi.2016.12.009
140. Wang Y, Yu H, He J. Involvement of the hippo pathway in the development of diabetes. Discov Med (2021) 31(162):37–44.
141. Liu B, Zheng Y, Yin F, Yu J, Silverman N, Pan D. Toll receptor-mediated hippo signaling controls innate immunity in Drosophila. Cell (2016) 164(3):406–19. doi: 10.1016/j.cell.2015.12.029
142. Hirosumi J, Tuncman G, Chang L, Görgün CZ, Uysal KT, Maeda K, et al. A central role for JNK in obesity and insulin resistance. Nature (2002) 420(6913):333–6. doi: 10.1038/nature01137
143. Yazıcı D, Sezer H. Insulin resistance, obesity and lipotoxicity. Adv Exp Med Biol (2017) 960:277–304. doi: 10.1007/978-3-319-48382-5_12
144. Solinas G, Becattini B. JNK at the crossroad of obesity, insulin resistance, and cell stress response. Mol Metab (2016) 6(2):174–84. doi: 10.1016/j.molmet.2016.12.001
145. Wang MC, Bohmann D, Jasper H. JNK extends life span and limits growth by antagonizing cellular and organism-wide responses to insulin signaling. Cell (2005) 121(1):115–25. doi: 10.1016/j.cell.2005.02.030
146. Muffat J, Walker DW, Benzer S. Human ApoD, an apolipoprotein up-regulated in neurodegenerative diseases, extends lifespan and increases stress resistance in Drosophila. Proc Natl Acad Sci USA (2008) 105(19):7088–93. doi: 10.1073/pnas.0800896105
147. Hull-Thompson J, Muffat J, Sanchez D, Walker DW, Benzer S, Ganfornina MD, et al. Control of metabolic homeostasis by stress signaling is mediated by the lipocalin NLaz. PloS Genet (2009) 5(4):e1000460. doi: 10.1371/journal.pgen.1000460
148. Locksley RM, Killeen N, Lenardo MJ. The TNF and TNF receptor superfamilies: integrating mammalian biology. Cell (2001) 104(4):487–501. doi: 10.1016/s0092-8674(01)00237-9
149. Igaki T, Kanda H, Yamamoto-Goto Y, Kanuka H, Kuranaga E, Aigaki T, et al. Eiger, a TNF superfamily ligand that triggers the Drosophila JNK pathway. EMBO J (2002) 21(12):3009–18. doi: 10.1093/emboj/cdf306
150. Johansson KC, Metzendorf C, Söderhäll K. Microarray analysis of immune challenged Drosophila hemocytes. Exp Cell Res (2005) 305(1):145–55. doi: 10.1016/j.yexcr.2004.12.018
151. Mabery EM, Schneider DS. The Drosophila TNF ortholog eiger is required in the fat body for a robust immune response. J Innate Immun (2010) 2(4):371–8. doi: 10.1159/000315050
152. Brandt SM, Dionne MS, Khush RS, Pham LN, Vigdal TJ, Schneider DS. Secreted bacterial effectors and host-produced eiger/TNF drive death in aSalmonella-infected fruit fly. PloS Biol (2004) 2(12):e418. doi: 10.1371/journal.pbio.0020418
153. Schneider DS, Ayres JS, Brandt SM, Costa A, Dionne MS, Gordon MD, et al. Drosophila eiger mutants are sensitive to extracellular pathogens. PloS Pathog (2007) 3(3):e41. doi: 10.1371/journal.ppat.0030041
154. Tang T, Li W, Wang X, Wu Y, Liu F. A house fly TNF ortholog Eiger regulates immune defense via cooperating with Toll and Imd pathways. Dev Comp Immunol (2019) 90:21–8. doi: 10.1016/j.dci.2018.08.016
155. Vanha-Aho LM, Valanne S, Ramet M. Cytokines in Drosophila immunity. Immunol Lett (2016) 170:42–51. doi: 10.1016/j.imlet.2015.12.005
156. Woodcock KJ, Kierdorf K, Pouchelon CA, Vivancos V, Dionne MS, Geissmann F. Macrophage-derived upd3 cytokine causes impaired glucose homeostasis and reduced lifespan in Drosophila fed a lipid-rich diet. Immunity (2015) 42(1):133–44. doi: 10.1016/j.immuni.2014.12.023
157. Kim TH, Choi SE, Ha ES, Jung JG, Han SJ, Kim HJ, et al. IL-6 induction of TLR-4 gene expression via STAT3 has an effect on insulin resistance in human skeletal muscle. Acta Diabetol (2013) 50(2):189–200. doi: 10.1007/s00592-011-0259-z
158. Straub RH. Insulin resistance, selfish brain, and selfish immune system: an evolutionarily positively selected program used in chronic inflammatory diseases. Arthritis Res Ther (2014) 16 Suppl 2(Suppl 2):S4. doi: 10.1186/ar4688
159. Yang H, Kronhamn J, Ekstrom JO, Korkut GG, Hultmark D. JAK/STAT signaling in Drosophila muscles controls the cellular immune response against parasitoid infection. EMBO Rep (2015) 16(12):1664–72. doi: 10.15252/embr.201540277
160. Yang H, Hultmark D. Drosophila muscles regulate the immune response against wasp infection via carbohydrate metabolism. Sci Rep (2017) 7(1):15713. doi: 10.1038/s41598-017-15940-2
161. Agaisse H, Petersen U-M, Boutros M, Mathey-Prevot B, Perrimon N. Signaling role of hemocytes in Drosophila JAK/STAT-dependent response to septic injury. Dev Cell (2003) 5(3):441–50. doi: 10.1016/s1534-5807(03)00244-2
162. Rajan A, Perrimon N. Drosophila cytokine unpaired 2 regulates physiological homeostasis by remotely controlling insulin secretion. Cell (2012) 151(1):123–37. doi: 10.1016/j.cell.2012.08.019
163. Zhang Y, Wang F, Feng Q, Wang H, Tang T, Huang D, et al. Involvement of trehalose-6-phosphate synthase in innate immunity of Musca domestica. Dev Comp Immunol (2019) 91:85–92. doi: 10.1016/j.dci.2018.10.010
164. Yang L, Qiu L, Fang Q, Wu S, Ye G. A venom protein of ectoparasitoid Pachycrepoideus vindemiae, PvG6PDH, contributes to parasitism by inhibiting host glucose-6-phosphate metabolism. Insect Sci (2021) 29:399–410. doi: 10.1111/1744-7917.12935
165. Rotstein B, Paululat A. On the morphology of the Drosophila heart. J Cardiovasc Dev Dis (2016) 3(2):15. doi: 10.3390/jcdd3020015
166. Parsons B, Foley E. Cellular immune defenses of Drosophila melanogaster. Dev Comp Immunol (2016) 58:95–101. doi: 10.1016/j.dci.2015.12.019
167. Pasquier LD. The immune system of invertebrates and vertebrates. Comp Biochem Physiol B Biochem Mol Biol (2001) 129(1):1–15. doi: 10.1016/s1096-4959(01)00306-2
168. Leitão AB, Sucena E. Drosophila sessile hemocyte clusters are true hematopoietic tissues that regulate larval blood cell differentiation. Elife (2015) 4:e06166. doi: 10.7554/eLife.06166
169. Cevik D, Acker M, Michalski C, Jacobs JR. Pericardin, a Drosophila collagen, facilitates accumulation of hemocytes at the heart. Dev Biol (2019) 454(1):52–65. doi: 10.1016/j.ydbio.2019.06.006
170. Lanot R, Zachary D, Holder F, Meister M. Postembryonic hematopoiesis in Drosophila. Dev Biol (2001) 230(2):243–57. doi: 10.1006/dbio.2000.0123
171. Lavine MD, Strand MR. Insect hemocytes and their role in immunity. Insect Biochem Mol Biol (2002) 32(10):1295–309. doi: 10.1016/s0965-1748(02)00092-9
172. Anderson RS, Holmes B, Good RA. Comparative biochemistry of phagocytizing insect hemocytes. Comp Biochem Physiol B (1973) 46(3):595–602. doi: 10.1016/0305-0491(73)90099-0
173. Buck MD, Sowell RT, Kaech SM, Pearce EL. Metabolic instruction of immunity. Cell (2017) 169(4):570–86. doi: 10.1016/j.cell.2017.04.004
174. Ganeshan K, Chawla A. Metabolic regulation of immune responses. Annu Rev Immunol (2014) 32:609–34. doi: 10.1146/annurev-immunol-032713-120236
175. Lackey DE, Olefsky JM. Regulation of metabolism by the innate immune system. Nat Rev Endocrinol (2016) 12(1):15–28. doi: 10.1038/nrendo.2015.189
176. Satyavathi VV, Minz A, Nagaraju J. Nodulation: an unexplored cellular defense mechanism in insects. Cell Signal (2014) 26(8):1753–63. doi: 10.1016/j.cellsig.2014.02.024
177. Van den Bossche J, O’Neill LA, Menon D. Macrophage immunometabolism: where are we (Going)? Trends Immunol (2017) 38(6):395–406. doi: 10.1016/j.it.2017.03.001
178. Palsson-McDermott EM, O’Neill LA. The Warburg effect then and now: from cancer to inflammatory diseases. Bioessays (2013) 35(11):965–73. doi: 10.1002/bies.201300084
179. Bergin D, Reeves EP, Renwick J, Wientjes FB, Kavanagh K. Superoxide production in Galleria mellonella hemocytes: identification of proteins homologous to the NADPH oxidase complex of human neutrophils. Infect Immun (2005) 73(7):4161–70. doi: 10.1128/IAI.73.7.4161-4170.2005
180. Rybicka JM, Balce DR, Khan MF, Krohn RM, Yates RM. NADPH oxidase activity controls phagosomal proteolysis in macrophages through modulation of the lumenal redox environment of phagosomes. Proc Natl Acad Sci USA (2010) 107(23):10496–501. doi: 10.1073/pnas.0914867107
181. Arnold PK, Jackson BT, Paras KI, Brunner JS, Hart ML, Newsom OJ, et al. A non-canonical tricarboxylic acid cycle underlies cellular identity. Nature (2022) 603(7901):477–81. doi: 10.1038/s41586-022-04475-w
182. Jha AK, Huang SC, Sergushichev A, Lampropoulou V, Ivanova Y, Loginicheva E, et al. Network integration of parallel metabolic and transcriptional data reveals metabolic modules that regulate macrophage polarization. Immunity (2015) 42(3):419–30. doi: 10.1016/j.immuni.2015.02.005
183. Herranz H, Cohen SM. Drosophila as a model to study the link between metabolism and cancer. J Dev Biol (2017) 5(4):15. doi: 10.3390/jdb5040015
184. Slaninova V, Krafcikova M, Perez-Gomez R, Steffal P, Trantirek L, Bray SJ, et al. Notch stimulates growth by direct regulation of genes involved in the control of glycolysis and the tricarboxylic acid cycle. Open Biol (2016) 6(2):150155. doi: 10.1098/rsob.150155
185. Tennessen JM, Bertagnolli NM, Evans J, Sieber MH, Cox J, Thummel CS. Coordinated metabolic transitions during Drosophila embryogenesis and the onset of aerobic glycolysis. G3 (Bethesda) (2014) 4(5):839–50. doi: 10.1534/g3.114.010652
186. Wang CW, Purkayastha A, Jones KT, Thaker SK, Banerjee U. In vivo genetic dissection of tumor growth and the Warburg effect. Elife (2016) 5:e18126. doi: 10.7554/eLife.18126
187. Gnanaprakasam JN, Wang R. MYC in regulating immunity: metabolism and beyond. Genes (Basel) (2017) 8(3):88. doi: 10.3390/genes8030088
188. Wang R, Dillon CP, Shi LZ, Milasta S, Carter R, Finkelstein D, et al. The transcription factor Myc controls metabolic reprogramming upon T lymphocyte activation. Immunity (2011) 35(6):871–82. doi: 10.1016/j.immuni.2011.09.021
189. Anderson AM, Bailetti AA, Rodkin E, De A, Bach EA. A genetic screen reveals an unexpected role for yorkie signaling in JAK/STAT-dependent hematopoietic Malignancies in Drosophila melanogaster. G3 (Bethesda) (2017) 7(8):2427–38. doi: 10.1534/g3.117.044172
190. Wenger RH, Stiehl DP, Camenisch G. Integration of oxygen signaling at the consensus HRE. Science’s StKe (2005) 2005(306):e12. doi: 10.1126/stke.3062005re12
191. Jung Y, Isaacs JS, Lee S, Trepel J, Liu Z-G, Neckers L. Hypoxia-inducible factor induction by tumour necrosis factor in normoxic cells requires receptor-interacting protein-dependent nuclear factor kappa B activation. Biochem J (2003) 370(Pt 3):1011–7. doi: 10.1042/BJ20021279
192. Siegert I, Schödel J, Nairz M, Schatz V, Dettmer K, Dick C, et al. Ferritin-mediated iron sequestration stabilizes hypoxia-inducible factor-1alpha upon LPS activation in the presence of ample oxygen. Cell Rep (2015) 13(10):2048–55. doi: 10.1016/j.celrep.2015.11.005
193. Krejčová G, Danielová A, Nedbalová P, Kazek M, Strych L, Chawla G, et al. Drosophila macrophages switch to aerobic glycolysis to mount effective antibacterial defense. Elife (2019) 8:e50414. doi: 10.7554/eLife.50414
194. Cara FD, Sheshachalam A, Braverman NE, Rachubinski RA, Simmonds AJ. Peroxisome-mediated metabolism is required for immune response to microbial infection. Immunity (2017) 47(1):93–106 e7. doi: 10.1016/j.immuni.2017.06.016
195. Keebaugh ES, Schlenke TA. Insights from natural host-parasite interactions: the Drosophila model. Dev Comp Immunol (2014) 42(1):111–23. doi: 10.1016/j.dci.2013.06.001
196. Carton Y, Poirié M, Nappi AJ. Insect immune resistance to parasitoids. Insect Sci (2008) 15(1):67–87. doi: 10.1111/j.1744-7917.2008.00188.x
197. Bartholomay LC, Cho WL, Rocheleau TA, Boyle JP, Beck ET, Fuchs JF, et al. Description of the transcriptomes of immune response-activated hemocytes from the mosquito vectors Aedes aEgypti and Armigeres subalbatus. Infect Immun (2004) 72(7):4114–26. doi: 10.1128/IAI.72.7.4114-4126.2004
198. Choi YJ, Fuchs JF, Mayhew GF, Yu HE, Christensen BM. Tissue-enriched expression profiles in Aedes aEgypti identify hemocyte-specific transcriptome responses to infection. Insect Biochem Mol Biol (2012) 42(10):729–38. doi: 10.1016/j.ibmb.2012.06.005
199. Pinto SB, Lombardo F, Koutsos AC, Waterhouse RM, McKay K, An C, et al. Discovery of Plasmodium modulators by genome-wide analysis of circulating hemocytes in Anopheles Gambiae. Proc Natl Acad Sci USA (2009) 106(50):21270–5. doi: 10.1073/pnas.0909463106
200. Shelby KS, Popham HJ. RNA-seq study of microbially induced hemocyte transcripts from larval Heliothis virescens (Lepidoptera: noctuidae). Insects (2012) 3(3):743–62. doi: 10.3390/insects3030743
201. Bours MJ, Swennen EL, Di Virgilio F, Cronstein BN, Dagnelie PC. Adenosine 5’-triphosphate and adenosine as endogenous signaling molecules in immunity and inflammation. Pharmacol Ther (2006) 112(2):358–404. doi: 10.1016/j.pharmthera.2005.04.013
202. Martin C, Leone M, Viviand X, Ayem ML, Guieu R. High adenosine plasma concentration as a prognostic index for outcome in patients with septic shock. Crit Care Med (2000) 28(9):3198–202. doi: 10.1097/00003246-200009000-00014
203. Lin YH, Tai CC, Broz V, Tang CK, Chen P, Wu CP, et al. Adenosine Receptor Modulates Permissiveness of Baculovirus (Budded Virus) Infection via Regulation of Energy Metabolism in Bombyx mori. Front Immunol (2020) 11:763. doi: 10.3389/fimmu.2020.00763
204. Chang Y, Tang CK, Lin YH, Tsai CH, Lu YH, Wu YL. Snellenius manilae bracovirus suppresses the host immune system by regulating extracellular adenosine levels in Spodoptera litura. Sci Rep (2020) 10(1):2096. doi: 10.1038/s41598-020-58375-y
205. Irving P, Ubeda JM, Doucet D, Troxler L, Lagueux M, Zachary D, et al. New insights into Drosophila larval haemocyte functions through genome-wide analysis. Cell Microbiol (2005) 7(3):335–50. doi: 10.1111/j.1462-5822.2004.00462.x
206. Shia AK, Glittenberg M, Thompson G, Weber AN, Reichhart JM, Ligoxygakis P. Toll-dependent antimicrobial responses in Drosophila larval fat body require Spätzle secreted by haemocytes. J Cell Sci (2009) 122(Pt 24):4505–15. doi: 10.1242/jcs.049155
207. Watkins PA, Maiguel D, Jia Z, Pevsner J. Evidence for 26 distinct acyl-coenzyme A synthetase genes in the human genome. J Lipid Res (2007) 48(1):2736–50. doi: 10.1194/jlr.M700378-JLR200
208. Douglas AE. The molecular basis of bacterial-insect symbiosis. J Mol Biol (2014) 426(23):3830–7. doi: 10.1016/j.jmb.2014.04.005
209. Blow F, Douglas AE. The hemolymph microbiome of insects. J Insect Physiol (2019) 115:33–9. doi: 10.1016/j.jinsphys.2019.04.002
210. Gasparich GE. Spiroplasmas: evolution, adaptation and diversity. Front Biosci (2002) 7:d619–d40. doi: 10.2741/A799
211. Clark TB. Spiroplasmas: diversity of arthropod reservoirs and host-parasite relationships. Science (1982) 217(4554):57–9. doi: 10.1126/science.217.4554.57
212. Anbutsu H, Fukatsu T. Evasion, suppression and tolerance of Drosophila innate immunity by a male-killing Spiroplasma endosymbiont. Insect Mol Biol (2010) 19(4):481–8. doi: 10.1111/j.1365-2583.2010.01008.x
213. Herren JK, Paredes JC, Schupfer F, Arafah K, Bulet P, Lemaitre B. Insect endosymbiont proliferation is limited by lipid availability. Elife (2014) 3:e02964. doi: 10.7554/eLife.02964
214. Perlman SJ, Hunter MS, Zchori-Fein E. The emerging diversity of Rickettsia. Proc Biol Sci (2006) 273(1598):2097–106. doi: 10.1098/rspb.2006.3541
215. Zug R, Hammerstein P. Still a host of hosts for Wolbachia: analysis of recent data suggests that 40% of terrestrial arthropod species are infected. PloS One (2012) 7(6):e38544. doi: 10.1371/journal.pone.0038544
216. Gil R, Silva FJ, Zientz E, Delmotte F, Gonzalez-Candelas F, Latorre A, et al. The genome sequence of Blochmannia floridanus: comparative analysis of reduced genomes. Proc Natl Acad Sci USA (2003) 100(16):9388–93. doi: 10.1073/pnas.1533499100
217. López-Madrigal S, Latorre A, Porcar M, Moya A, Gil R. Complete genome sequence of “Candidatus Tremblaya princeps” strain PCVAL, an intriguing translational machine below the living-cell status. J Bacteriol (2011) 193(19):5587–8. doi: 10.1128/JB.05749-11
218. Nakabachi A, Yamashita A, Toh H, Ishikawa H, Dunbar HE, Moran NA, et al. The 160-kilobase genome of the bacterial endosymbiont Carsonella. Science (2006) 314(5797):267. doi: 10.1126/science.1134196
219. Shigenobu S, Watanabe H, Hattori M, Sakaki Y, Ishikawa H. Genome sequence of the endocellular bacterial symbiont of aphids Buchnera sp. APS. Nature (2000) 407(6800):81–6. doi: 10.1038/35024074
220. Hurst GDD, Anbutsu H, Kutsukake M, Fukatsu T. Hidden from the host: Spiroplasma bacteria infecting Drosophila do not cause an immune response, but are suppressed by ectopic immune activation. Insect Mol Biol (2003) 12(1):93–7. doi: 10.1046/j.1365-2583.2003.00380.x
221. Hutchence KJ, Fischer B, Paterson S, Hurst GD. How do insects react to novel inherited symbionts? A microarray analysis of Drosophila melanogaster response to the presence of natural and introduced Spiroplasma. Mol Ecol (2011) 20(5):950–8. doi: 10.1111/j.1365-294X.2010.04974.x
222. Xie J, Butler S, Sanchez G, Mateos M. Male killing Spiroplasma protects Drosophila melanogaster against two parasitoid wasps. Heredity (Edinb) (2014) 112(4):399–408. doi: 10.1038/hdy.2013.118
223. Xie J, Vilchez I, Mateos M. Spiroplasma bacteria enhance survival of Drosophila hydei attacked by the parasitic wasp Leptopilina heterotoma. PloS One (2010) 5(8):e12149. doi: 10.1371/journal.pone.0012149
224. Łukasik P, Mv A, Guo H, Ferrari J, Godfray HCJ. Unrelated facultative endosymbionts protect aphids against a fungal pathogen. Ecol Lett (2013) 16(2):214–8. doi: 10.1111/ele.12031
225. Jaenike J, Unckless R, Cockburn SN, Boelio LM, Perlman SJ. Adaptation via symbiosis: recent spread of a Drosophila defensive symbiont. Science (2010) 329(5988):212–5. doi: 10.1126/science.1188235
226. Paredes JC, Herren JK, Schupfer F, Lemaitre B. The role of lipid competition for endosymbiont-mediated protection against parasitoid wasps in Drosophila. mBio (2016) 7(4):e01006–16. doi: 10.1128/mBio.01006-16
227. Burke G, Fiehn O, Moran N. Effects of facultative symbionts and heat stress on the metabolome of pea aphids. ISME J (2010) 4(2):242–52. doi: 10.1038/ismej.2009.114
228. Laughton AM, Garcia JR, Gerardo NM. Condition-dependent alteration of cellular immunity by secondary symbionts in the pea aphid, Acyrthosiphon pisum. J Insect Physiol (2016) 86:17–24. doi: 10.1016/j.jinsphys.2015.12.005
229. Laughton AM, Fan MH, Gerardo NM. The combined effects of bacterial symbionts and aging on life history traits in the pea aphid, Acyrthosiphon pisum. Appl Environ Microbiol (2014) 80(2):470–7. doi: 10.1128/AEM.02657-13
230. Hilgenboecker K, Hammerstein P, Schlattmann P, Telschow A, Werren JH. How many species are infected with Wolbachia?-A statistical analysis of current data. FEMS Microbiol Lett (2008) 281(2):215–20. doi: 10.1111/j.1574-6968.2008.01110.x
231. Werren JH, Baldo L, Clark ME. Wolbachia: master manipulators of invertebrate biology. Nat Rev Microbiol (2008) 6(10):741–51. doi: 10.1038/nrmicro1969
232. Melnikow E, Xu S, Liu J, Li L, Oksov Y, Ghedin E, et al. Interaction of a Wolbachia WSP-like protein with a nuclear-encoded protein of Brugia malayi. Int J Parasitol (2011) 41(10):1053–61. doi: 10.1016/j.ijpara.2011.05.008
233. Heukelbach J, Bonow I, Witt L, Feldmeier H, Fischer P. High infection rate of Wolbachia endobacteria in the sand flea Tunga penetrans from Brazil. Acta Trop (2004) 92(3):225–30. doi: 10.1016/j.actatropica.2004.08.005
234. Sanogo YO, Dobson SL, Bordenstein SR, Novak RJ. Disruption of the Wolbachia surface protein gene wspB by a transposable element in mosquitoes of the Culex pipiens complex (Diptera, Culicidae). Insect Mol Biol (2007) 16(2):143–54. doi: 10.1111/j.1365-2583.2006.00707.x
235. Kramer LH, Tamarozzi F, Morchon R, Lopez-Belmonte J, Marcos-Atxutegi C, Martin-Pacho R, et al. Immune response to and tissue localization of the Wolbachia surface protein (WSP) in dogs with natural heartworm (Dirofilaria immitis) infection. Vet Immunol Immunopathol (2005) 106(3-4):303–8. doi: 10.1016/j.vetimm.2005.03.011
236. Ikeya T, Broughton S, Alic N, Grandison R, Partridge L. The endosymbiont Wolbachia increases insulin/IGF-like signalling in Drosophila. . Proc Biol Sci (2009) 276(1674):3799–807. doi: 10.1098/rspb.2009.0778
237. Zhao X, Karpac J. Glutamate metabolism directs energetic trade-offs to shape host-pathogen susceptibility in Drosophila. Cell Metab (2021) 33(12):2428–44 e8. doi: 10.1016/j.cmet.2021.10.003
238. Yang S, Zhao Y, Yu J, Fan Z, Gong ST, Tang H, et al. Sugar alcohols of polyol pathway serve as alarmins to mediate local-systemic innate immune communication in Drosophila. Cell Host Microbe (2019) 26(2):240–51.e8. doi: 10.1016/j.chom.2019.07.001
239. Login FH, Heddi A. Insect immune system maintains long-term resident bacteria through a local response. J Insect Physiol (2013) 59(2):232–9. doi: 10.1016/j.jinsphys.2012.06.015
240. Login FH, Balmand S, Vallier A, Vincent-Monégat C, Vigneron A, Weiss-Gayet M, et al. Antimicrobial peptides keep insect endosymbionts under control. Science (2011) 334(6054):362–5. doi: 10.1126/science.1209728
241. Gao X, Xue H, Luo J, Ji J, Zhang L, Niu L, et al. Molecular evidence that Lysiphlebia japonica regulates the development and physiological metabolism of Aphis gossypii. Int J Mol Sci (2020) 21(13):4610. doi: 10.3390/ijms21134610
242. Lin Z, Wang RJ, Cheng Y, Du J, Volovych O, Han LB, et al. Insights into the venom protein components of Microplitis mediator, an endoparasitoid wasp. Insect Biochem Mol Biol (2019) 105:33–42. doi: 10.1016/j.ibmb.2018.12.013
243. Wang B, Ren C, Yang L, Fang Q, Song Q, Ye G. Venom α-amylase of the endoparasitic wasp Pteromalus puparum influences host metabolism. Pest Manage Sci (2020) 76(6):2180–9. doi: 10.1002/ps.5755
244. Kang X, Wang Y, Liang W, Tang X, Zhang Y, Wang L, et al. Bombyx mori nucleopolyhedrovirus downregulates transcription factor BmFoxO to elevate virus infection. Dev Comp Immunol (2021) 116:103904. doi: 10.1016/j.dci.2020.103904
245. Ma M, Tu C, Luo J, Lu M, Zhang S, Xu L. Metabolic and immunological effects of gut microbiota in leaf beetles at the local and systemic levels. Integr Zool (2021) 16(3):313–23. doi: 10.1111/1749-4877.12528
246. Ho PN, Klanrit P, Hanboonsong Y, Yordpratum U, Suksawat M, Kulthawatsiri T, et al. Bacterial challenge-associated metabolic phenotypes in Hermetia illucens defining nutritional and functional benefits. Sci Rep (2021) 11(1):23316. doi: 10.1038/s41598-021-02752-8
247. Liu K, He J, Guan Z, Zhong M, Pang R, Han Q. Transcriptomic and metabolomic analyses of Diaphorina citri Kuwayama infected and non-infected with Candidatus Liberibacter Asiaticus. Front Physiol (2021) 11:63003. doi: 10.3389/fphys.2020.63003
248. Yu H, Li N, Zeng X, Song J, Yu X, Su H, et al. Transcriptome analyses of Diaphorina citri midgut responses to Candidatus Liberibacter Asiaticus infection. Insects (2020) 11(3):171. doi: 10.3390/insects11030171
249. Son JH, Weiss BL, Schneider DI, Dera KM, Gstöttenmayer F, Opiro R, et al. Infection with endosymbiotic Spiroplasma disrupts tsetse (Glossina fuscipes fuscipes) metabolic and reproductive homeostasis. PloS Pathog (2021) 17(9):e1009539. doi: 10.1371/journal.ppat.1009539
250. Nascimento da Silva J, Calixto Conceição C, Cristina Ramos de Brito G, Costa Santos D, Martins da Silva R, Arcanjo A, et al. Wolbachia pipientis modulates metabolism and immunity during Aedes fluviatilis oogenesis. Insect Biochem Mol Biol (2022) 146:103776. doi: 10.1016/j.ibmb.2022.103776
251. Itskov PM, Ribeiro C. The dilemmas of the gourmet fly: the molecular and neuronal mechanisms of feeding and nutrient decision making in Drosophila. Front Neurosci (2013) 7:12. doi: 10.3389/fnins.2013.00012
252. Murray S, Tulloch A, Gold MS, Avena NM. Hormonal and neural mechanisms of food reward, eating behaviour and obesity. Nat Rev Endocrinol (2014) 10(9):540–52. doi: 10.1038/nrendo.2014.91
Keywords: insects, Drosophila, immunometabolism, pathogenic, symbiotic bacteria, insulin signaling, IMD/Toll
Citation: Li S, Wang J, Tian X, Toufeeq S and Huang W (2023) Immunometabolic regulation during the presence of microorganisms and parasitoids in insects. Front. Immunol. 14:905467. doi: 10.3389/fimmu.2023.905467
Received: 27 March 2022; Accepted: 04 September 2023;
Published: 25 September 2023.
Edited by:
Yonggyun Kim, Andong National University, Republic of KoreaReviewed by:
Jorge Contreras-Garduño, National Autonomous University of Mexico, MexicoCopyright © 2023 Li, Wang, Tian, Toufeeq and Huang. This is an open-access article distributed under the terms of the Creative Commons Attribution License (CC BY). The use, distribution or reproduction in other forums is permitted, provided the original author(s) and the copyright owner(s) are credited and that the original publication in this journal is cited, in accordance with accepted academic practice. No use, distribution or reproduction is permitted which does not comply with these terms.
*Correspondence: Shahzad Toufeeq, dG91ZmVlcUBjZW1wcy5hYy5jbg==; Wuren Huang, aHVhbmd3dXJlbjEwQDE2My5jb20=
†These authors have contributed equally to this work and share first authorship
Disclaimer: All claims expressed in this article are solely those of the authors and do not necessarily represent those of their affiliated organizations, or those of the publisher, the editors and the reviewers. Any product that may be evaluated in this article or claim that may be made by its manufacturer is not guaranteed or endorsed by the publisher.
Research integrity at Frontiers
Learn more about the work of our research integrity team to safeguard the quality of each article we publish.