- 1Chengdu University of Traditional Chinese Medicine, Chengdu, China
- 2Hospital of Chengdu University of Traditional Chinese Medicine, Chengdu, China
Intestinal tuft cells (TCs) are defined as chemosensory cells that can “taste” danger and induce immune responses. They play a critical role in gastrointestinal parasite invasion, inflammatory bowel diseases and high-fat diet-induced obesity. Intestinal IL-25, the unique product of TCs, is a key activator of type 2 immunity, especially to promote group 2 innate lymphoid cells (ILC2s) to secret IL-13. Then the IL-13 mainly promotes intestinal stem cell (ISCs) proliferation into TCs and goblet cells. This pathway formulates the circuit in the intestine. This paper focuses on the potential role of the intestinal TC, ILC2 and their circuit in obesity-induced intestinal damage, and discussion on further study and the potential therapeutic target in obesity.
1 Introduction
Obesity is a worldwide pandemic and a major risk for chronic diseases, including diabetes and cardiovascular disease. The prevalence of obesity has been increasing rapidly worldwide due to changes in lifestyle and diet. Obesity is considered as a typical metabolic syndrome characterized by systemic low-grade chronic inflammation (1). The close interactions between obesity and the gut microbiota and immunity (2) have recently been revealed, but the underlying mechanism has not yet been fully elucidated.
TCs line the epithelial mucosa, such as the intestine, airway and taste bud (3, 4). Although they were discovered more than six decades ago, their exact roles in health remain mysterious. Under homeostatic conditions, a small number of TCs serves as the monitor in the intestines, detecting and responding to various stimuli through the use of succinate (5), sweet and bitter taste receptors. While investigating ulcerative colitis (UC), the researchers noticed a reduction in TCs along with inflammation and tissue damage. However, when they treated UC mice with succinate, they observed an improvement of chronic intestinal inflammation, accompanied by an increase in the number of intestinal TCs (6). TCs are characterized by their apical microvilli and orchestrate the mucosal immune response (7), such as parasite immunity (8–10). Notably, intestinal TCs are unique cells that secrete IL-25 (10), the key activator of ILC2s. In response to IL-25, enteric ILC2s produce type 2 cytokines, including IL-4, IL-5, IL-9 and IL-13, which promote the ISCs differentiation into TCs, thus formulating the circuit (10). The TC-ILC2 circuit is regulated by feed-forward signaling including IL-25, IL-4 and IL-13 (11, 12), which has been shown to regulate intestinal remodeling and homeostasis in response to diet (12). Arora (13) indicated that TCs may be involved in modulating whole-body energy metabolism. ILC2s and their cytokines in the small intestine can also contribute to inflammation and insulin resistance (14). Further understanding of the potential connection between intestinal TC, ILC2 and obesity needs to be conducted. Herein, we review the connection mechanism of the intestinal TC, ILC2 and their circuit in obesity-associated inflammation through intestinal metabolic homeostasis.
2 Intestinal TCs in obesity process
2.1 Distribution, morphology, heterogeneity and origin of intestinal TCs
TCs are mostly present in the columnar epithelium of hollow endoderm-derived organs such as the trachea, thymus, stomach, small intestine, and urethra in mammals (15). Both the small and large intestines contain TCs, with the highest number at the proximal small intestine (16). Cholecystokinin, peptide YY- and glucagon-like peptide-1 (GLP-1) positive endocrine cells are found close to TCs (16). More than 60% of TCs in the small intestine are found in contact with nerve fibers, indicating that TCs are modulators of intestine movements (16) (Figure 1A).
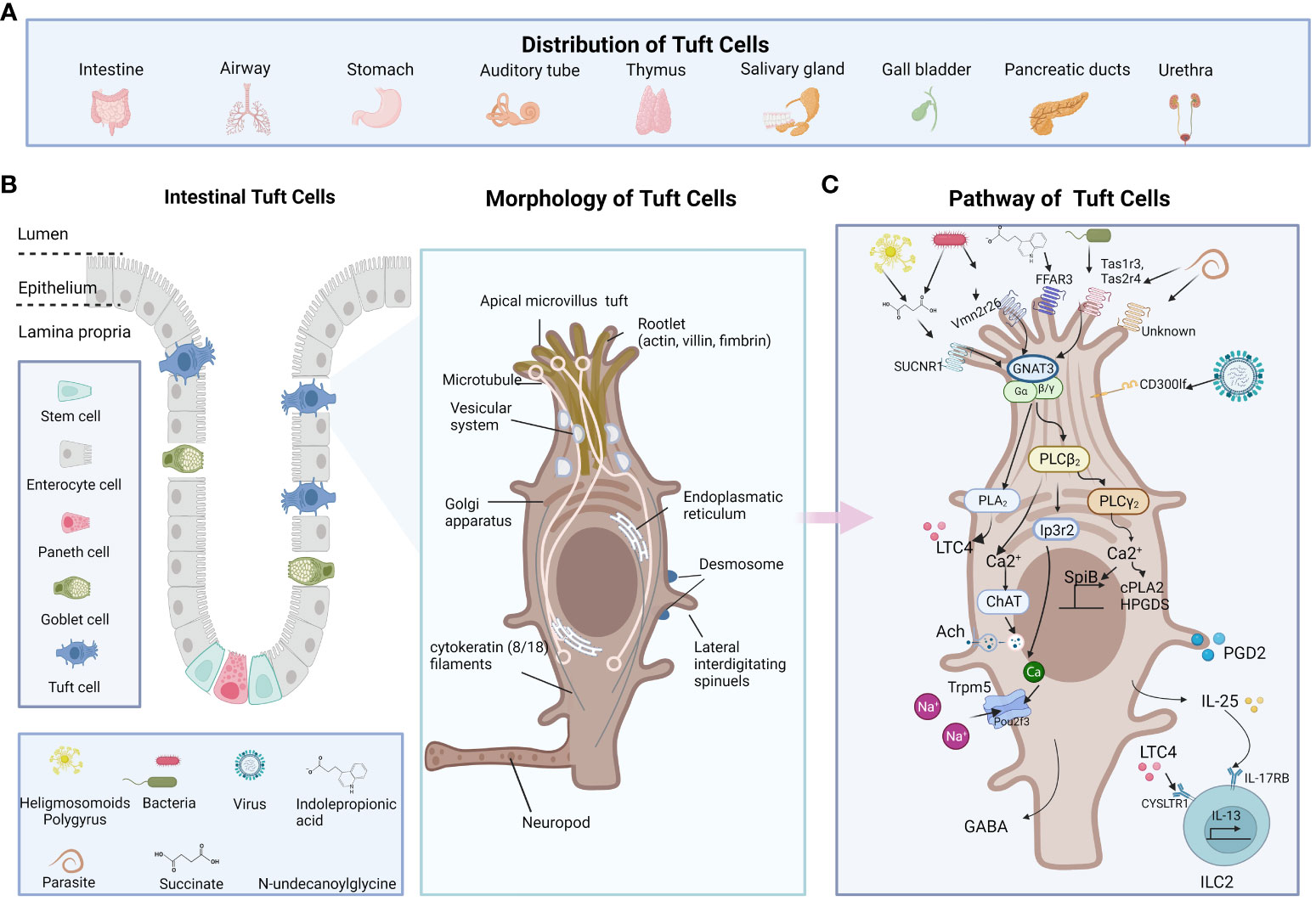
Figure 1 The distribution, morphology, and functions of TCs. (A) TCs present in the columnar epithelium of hollow endoderm-derived organs including trachea, thymus, stomach, small intestine, and urethra in mammals (B) The location and morphology of the TCs are shown in the middle. TC is characterized by its long and compact microvilli, which are connected to a broad network of rootlets that consists of actin, villus, filaments, and microtubules that hold the direction from apical to basal. (C) The receptor of TCs including Tas1Rs/Tas2Rs, SUCNR1, FFAR3, Vmn2r26, CD300lf, and some unknown. The pathway of TCs including GNAT3-Plcβ2-Ip3r2-TRPM5, GPCR-PLCγ2)-Ca(2+) signaling axis and et al. The figure was created by Biorender.
The structure of TCs was first described in 1956 by Rhodin and Dalhamn (17). The “tuft” or “brush” comes from its unique type of brush border, and the apical microvilli of TCs extend into the gut lumen (18). The TC is characterized by its long and compact microvilli, which are connected to a broad network of rootlets that consists of actin, villus, filaments, and microtubules that hold the direction from apical to basal relative to neighboring cells (19–22). The cytoskeleton is largely composed of intermediate actin filaments (18, 23). Cytoplasmic spinules are a special part of TCs that extend from one lateral of the TC border into the adjacent cells, reaching their nuclei, which could be the route for the transport of molecular cargo wrapped with IL-25 or acetylcholine to the adjacent cells (18, 23, 24). These lateral interdigitating spinules appear on TCs ranging from just below the apical tight and adherent junctional complex to the nuclear level. With no secretory vesicles extending down the basal lamina, the basal area of TCs develops protrusions that resemble neuropods, indicating paracrine communication via neuropods (11) (Figure 1B).
By utilizing the single-cell sequence approach, Haber and his colleagues defined two subtypes of TCs, tuft-1 and tuft-2, in the intestine (25). Montoro also found the two subtypes in the mouse tracheal epithelium (26). Tuft-1 signature genes are correlated with neuronal development, whereas Tuft-2 cells are enriched for immune-related genes. SH2 Domain Containing 6 (Sh2d6) and G protein-coupled receptor (GPCR) vomeronasal type-2 receptor 26 (Vmn2r26) have been proposed as exclusive markers for tuft-2 cells (27), while tubulin polymerization-promoting protein family member 3 (Tppp3) is primarily expressed in tuft-1 cells (27). Besides, Tuft-2 cells express significantly higher levels of the Th2-promoting cytokine, thymic stromal lymphopoietin (TSLP) (25, 28). Markers including Arachidonate 5-lipoxygenase (Alox5), Cytochrome c oxidase 1 (Cox1), Cytochrome c oxidase 2 (Cox2), Hematopoietic Prostaglandin D Synthase (Hpgds), HOP Homeobox (Hopx), and phosphoserine epidermal growth factor receptor (p-Egfr) (29–31) exist in both subtypes. POU class 2 homeobox 3 (Pou2f3) is a crucial transcription factor for TC development, showing a shared ancestry with TCs in the gastrointestinal tract and lingual taste buds (9). In addition, growth factor-independent 1B (Gfi1b) is identified as TC-specific master regulators (32, 33). Both markers are expressed constitutively in all TCs (7). However, tuft-1 and tuft-2 cells both express IL-25 (25). Table 1.
Since the intestinal epithelium has rapid turnover and vigorous proliferation, and ISCs renew every 4-7 days, it is essential canonical Wnt signals combined with TGFβ/BMP pathways proceed unperturbed to maintain the integrity of the intestinal epithelial barrier (44). ISCs identified as leucine rich repeat-containing G protein coupled receptor-5 (Lgr5) positive cells, which are responsible for both renewal and regeneration processes and are located at the base of crypts (45). TCs are relatively rare in the intestine and account for 0.4% to 2.3% of the total epithelial cells in the murine intestinal epithelium (25). Unlike other epithelial cells, differentiated intestinal TCs still express Lgr5 (29).
The origin of intestinal TCs has been a controversial topic for years. Gerbe (30) first demonstrated that mature TCs are derived from Lgr5+ epithelial stem cells and require atonal bHLH transcription factor 1 (Atoh1) to differentiate, identifying them as the secretory lineage. However, some researchers hold a different opinion that doublecortin-like kinase 1 (DCLK1) positive TCs are derived from Lgr5+ stem cells and require neural input for survival in the nonsecretory lineage rather than the columnar lineage (43, 46). Herring (47) used p-Creode analysis of single cells in the intestine with computational and experimental methods to indicate that TCs were possibly Atoh1-independent secretory lineage in the small intestine. while in the colon, TCs arise from an Atoh1-driven alternative developmental program.
2.2 Functional pathways of intestinal TCs
Although TCs were discovered as a unique cell type more than sixty years ago, their function was not fully explored. Sensory and secretory functions were the known main functions of TCs, moreover, they are usually correlated.
TCs exhibit significant similarities with sensory cells. They possess characteristic apical microvilli that are positioned toward the luminal interface, like the tongue’s taste buds. This positioning facilitates access to chemosensory nerve endings. A variety of apical and basolateral receptors allow TCs to detect different parasites, bacteria, viruses or their metabolites (8, 48, 49). TCs taste signal receptors, including Type 1 transduces signals (Tas1Rs) for sweet/umami substances and type 2 for bitter substances (Tas2Rs) (50, 51), succinate receptor 1 (SUCNR1) (5, 12), and free fatty acid receptor 3 (FFAR3) (52) are heterotrimeric guanine nucleotide-binding protein G-coupled receptors (GPCRs). The activation of these distinct receptors triggers a shared signal transduction pathway that involves Gnat3, phospholipase Cβ2 (Plcβ2), inositol triphosphate receptor type 2 (Ip3r2), and Trpm5 a monovalent specific, nonselective cation channel for Na+, K+, and Cs+ ions instead of Ca2+ ions (53). Pou2f3 is the master regulator for the generation of Trpm5-expressing chemosensory TCs (54). These Trpm5-expressing TCs are responsible for helminth infection in the gut (8). However, TCs make use of Ip3r2 to regulate cytosolic calcium and Trpm5 activity in response to Trichinella spiralis infection in a small intestinal organoid, while Trpm5 enhanced the release and hyperplasia of IL-25 (51). The loss of activating transcription factor 5 (Atf5) enhanced the TC-ILC2 circuit by promoting TC differentiation in response to parasitic infections (55). While it is controversial, Heligmosomoides polygyrus seems to block the effects of IL-4 and IL-13 and inhibit the gene expression of TCs both in vitro and in vivo (56). As for the intestinal antimicrobial immunity, tuft-2 senses bacterial metabolite N-undecanoylglycine, then Vmn2r26 engages activated G-protein-coupled receptor-phospholipase C gamma2 (GPCR-PLCγ2)-Ca(2+) signaling axis, which produces prostaglandin D2 (PGD2). PGD2 further enhances antibacterial immunity (27). Moreover, Vmn2r26 signaling also promotes the expression of Spi-B transcription factor, which promotes the expansion of Tuft 2 cells (27). Expressed on the intestinal TCs, CD300lf is identified as a murine norovirus (MNV) receptor, promoting MNV infection in vivo (57). Denatonium, as a bitter substance, is reported to increase intracellular calcium levels in colonic TCs (58). SUCNR1 is a typical receptor that reacts to succinate secreted by symbiotics and helminths (5). FFAR3, another TC receptor, is reported to be sensing indolepropionic acid (IPA), restoring IL-25 function, preventing gut leakage and inhibiting systemic inflammation (52). Interestingly, most reports indicate that multiple taste-associated GPCRs initiate intestinal immunity in TCs (Figure 1C).
TCs are important intestinal secretory cells. The majority of intestinal TCs generate a variety of paracrine and endocrine cytokines, including IL-25 (8), ACh (41), eicosanoids, TSLP and β-endorphins (25). TCs release IL-25 upon helminth infection and activate ILC2s to secrete IL-13, while IL-13 promotes the ISCs differentiation into TCs and goblet cells (10). The IL-25-dependent TC circuit requires macrophage migration inhibitory factor (Mif) (59). TCs express choline acetyltransferase for acetylcholine (ACh) synthesis, leading to the activation of Trpm5 during helminth infection (60). TCs also rapidly synthesize leukotrienes and other eicosanoid derivatives to stimulate competent cells (51, 61). Moreover, TCs are responsible for opioid (62), prostanoid and β-endorphin production (30), which are associated with analgesic effects as well as basic intestinal physiology including intestinal motility, secretion and nutrition absorption (Figure 1C).
2.3 Intestinal TCs in obesity
The intestines play a crucial role in the absorption of nutrients and the elimination of waste materials (63). Excess fat uptake in a Westernized diet is associated with an increased incidence of inflammatory bowel disease, obesity and food allergies (64). High-fat diet (HFD) impairs the intestinal immune system, making it hypersensitive to epithelial damage (65). Besides, it induces the imbalance in the gut microenvironment and active epithelial mucosa cells, including TCs (66).
It is controversial that TCs number varies when the mice are fed with HFD. Widmayer reported that 3 weeks of HFD supply increased the gastral surface mucous cells, mucosal mast cells, and TCs (67), and the expansion TCs demonstrated active leukotriene (LT) secretion. Gastric TCs can quickly sense saturated long-chain fatty acids (LCFAs) through the apical receptors GPR120 (67). Then, it is likely that upon detecting saturated LCFAs, TCs may trigger inflammatory reactions that produce cysteinyl (cys) LTs and activate surface mucous cells and mucosal mast cells, thus regulating the function and integrity of the intestinal mucosa (67). This process could potentially play a role in triggering mucosal inflammation. However, Arora reported that HFD-induced obese mice present an overall decrease of TCs in the small intestine due to the inhibition of IL-25 and TSLP mRNA expression (48). Additionally, Chen (52) and Sun (68) independently observed a reduction in TC population in mice subjected to long-time HFD, which coincided with a decrease in tight junction protein levels. However, TCs were both restored to normal levels following a weight loss intervention. It suggested that restoring the number of TCs in individuals affected by a HFD could potentially promote the restoration of intestinal barrier integrity. Aliluev (69) used single-cell sequencing on small intestinal crypt cells in nutrient-induced obese mice and found a decrease both in TCs and tuft progenitors. Mice fasted for 48 hours presented elevated levels of TCs after refeeding (29). However, a short-term HFD diet for 1, 3, or 7 d decreased the amount of TCs (70). Interestingly, the number of TCs exhibits considerable variation when they are subjected to HFD. It is plausible that the duration of HFD exposure, such as the 3-week HFD regimen employed by Widmayer (67) or the 9-week HFD regimen employed by Arora (48), may contribute to this difference.
Li reported that the succinate-SUCNR1 axis attenuates HFD-induced metabolic disturbance via activating type 2 immune responses and repairing intestinal barrier dysfunction (71). In mice infected with MNV, researchers discovered that intestinal TCs were targeted by MNV to activate mucosal immunity. This activation included various components such as B cell subsets, macrophages, and T cells, which collectively protected against type 1 diabetes in the mice (72).
The listed references provide evidence indicating a connection between disorders in taste cells and metabolic diseases, particularly obesity. Nevertheless, additional research especially the knockout of TCs or the key pathway is needed to further explore the role of TCs in these conditions.
3 ILC2s altered immunity in obesity
3.1 Origin, distribution, and function of ILC2s
ILC2s were recently found to be tissue-resident cells that lack recombination activating gene (RAG)-dependent rearrangement of antigen receptors, despite their particular lymphoid morphology compared to that of T and B cells (73). ILC2s were initially found in early infection of helminths (74). They are defined by biomarkers, including CD127 (IL-7Rα), CD161 (Klrg1) and CD294 (CRTH2) (75) (Table 2), which originate from the common lymphoid progenitor (CLP) in the fetal liver or bone marrow and migrate to peripheral tissues to develop into tissue-resident lymphocytes to combat specific types of infections (88, 89). CLPs are induced by Gata3, Id-2 (90, 91) and the transcription factors Gfi1 and Bcl11b to differentiate into ILC2s (92). ILC2s constitutively express high levels of GATA3 and ROR-α (90, 91), which are required for functional maturation and maintenance. The upstream regulator Bcl11b maintains Gfi1 expression in mature ILC2s (92). ILC2s show the immune function of type 2 immunity, which is stimulated by extracellular parasites, food, microbes and allergens (93–95). IL-25, IL-33, and TSLP activate ILC2s and produce type 2 cytokines IL-4, IL-5, IL-9, IL-13 and amphiregulin, which contribute to pathogen defense, metabolic homeostasis, and tissue remodeling (49, 96–100). ILC2s are divided into two types: natural ILC2s (nILC2s) and inflammatory ILC2s (iILC2s). nILC2s respond to IL-33 and produce IL-9 (93), while iILC2s respond to IL-25 (101) and highly express killer cell lectin-like receptor G1 (KLRG1) (101) and promote their production of IL-13 (102).
3.2 Intestinal ILC2s are involved in obesity
HFD induces systemic low-grade chronic inflammation in animal models and humans. ILC2s work as a bridge linking epithelial cells and the immune system to promote immunity and metabolic homeostasis (103). In adipose tissue, ILC2s induce the type 2 cytokines IL-5 or IL-13 and increase eosinophils numbers, and M2 macrophages stimulate inflammation, which promotes immunity and metabolic homeostasis and curbs obesity (104, 105). In the intestine, Sasaki (14) used Il2rg−/−Rag2−/− mice, which genetically lack all types of ILCs and are resistant to HFD-induced obesity, to reveal that intestinal ILC2s rather than WAT are more important in the development of obesity. The controversial results between the intestine and adipose tissue indicate that the immune function of ILC2s is likely dependent on the tissue/organ microenvironment. Administration of succinate in HFD-induced obese mice could enhance goblet cell production via activating type 2 immune responses in the small intestine (71). The expression of IL-4, IL-13, and IL-5 decreased in HFD-fed mice and was restored by berberine, indicating that ILC2s inhibit obesity-related intestinal inflammation (68). In obese human and mouse models, representative cytokines of ILC2s decreased and were rescued by IPA (52). In vitamin A deficient mice, dramatic expansion of IL-13 to produce ILC2 and resistance to nematode infection revealed that ILC2 might be the primary sensor of dietary stress, and innate type 2 immunity may represent a powerful adaptation of the immune system to promote host survival in the face of ongoing barrier challenges (89). The detailed effect of intestinal ILC2s on obesity still needs to be further investigated.
4 The TC-ILC2 circuit in obesity
4.1 Obesity intestinal microbial metabolites disorders potentially altered the circuit
Obesity and obesity-associated inflammation are closely correlated with dysbiosis in the intestinal microbiota (106). Short-chain fatty acids (SCFAs), including acetate, propionate and butyrate, are mostly produced by the gut microbiota (107). Mammalian cells produce succinate as an intermediate metabolite in the tricarboxylic acid cycle (TCA), yet microbes excrete succinate as a metabolic byproduct (108). Obese people have higher serum levels of succinate, which is associated with worse metabolic and specific intestinal microbiota (109). In a diet-induced obese model, succinate reduced body weight and promoted mitochondrial protein metabolism in brown adipose tissue (110). McKinley (29) noted that fasting and refeeding, as well as the introduction of microbiota to germ-free mice, caused dynamic changes in the quantity, composition and protein expression in TCs. Succinate is sufficient to promote the ISC to proliferate into TCs and goblet cells. However, at homeostasis, low levels of intestinal succinate cannot activate TCs (111). In response to the changes in TCs, intestinal microbial metabolites caused by HFD-induced obesity may affect the circuit, but further compelling evidence is required to support this claim.
4.2 The TC-ILC2 circuit potential function in obesity
The process of the circuit being affected can be explained as follows. The IL-25 receptor is the heterodimer of IL-17RB and IL-17RA. IL-25R is expressed in various respondent cells, including smooth muscle cells, basophils, eosinophils, and ILC2s throughout skin, brain, airway, and intestinal tissues (112–114). While TCs are the only cellular sources of IL-25 in the intestine (10), IL-25 induces ILC2s to produce type 2 immunity, which promotes the early immune response when infected (83). CysLTs and IL-25 collaborate to alert ILC2s to synthesize IL-4, IL-5 and IL-13 (61). IL-13 promotes Lgr5+ epithelial stem cells to differentiate into TCs or goblet cells, possibly by the Notch signaling pathway (115). In addition, IL-13 also promotes TC production and restores immune homeostasis and mucosal barriers (10). In response to dietary, the TC-ILC2 circuit regulates adaptive intestinal remodeling (12). However, this circuit is disrupted and downregulated IL-25 and TSLP marked TCs in obesity (48). Gut-microbiota-derived succinate promotes TC increase and reduces intestinal inflammation (111). Both TCs and IL-25 decreased in obese patients and animal models (52). These decreases could be restored by berberine via the TAS2Rs Gα-gustducin/Gβ1γ13 signaling pathway (68). IL-25 was first identified as a cytokine that induced naive CD4+ T cells into CD4+ Th2 cells (116). It plays a crucial role in regulating Th2-type immunity by modulating actin related gene 1(Act1) (117) and also promoting the secretion of Th2 cytokines (74) (Figure 2). Studies on TCs, ILC2s and the circuit response to obesity are limited and more TCs models including organoids and knockout mice could be applied to investigate the mechanism of obesity barrier damage.
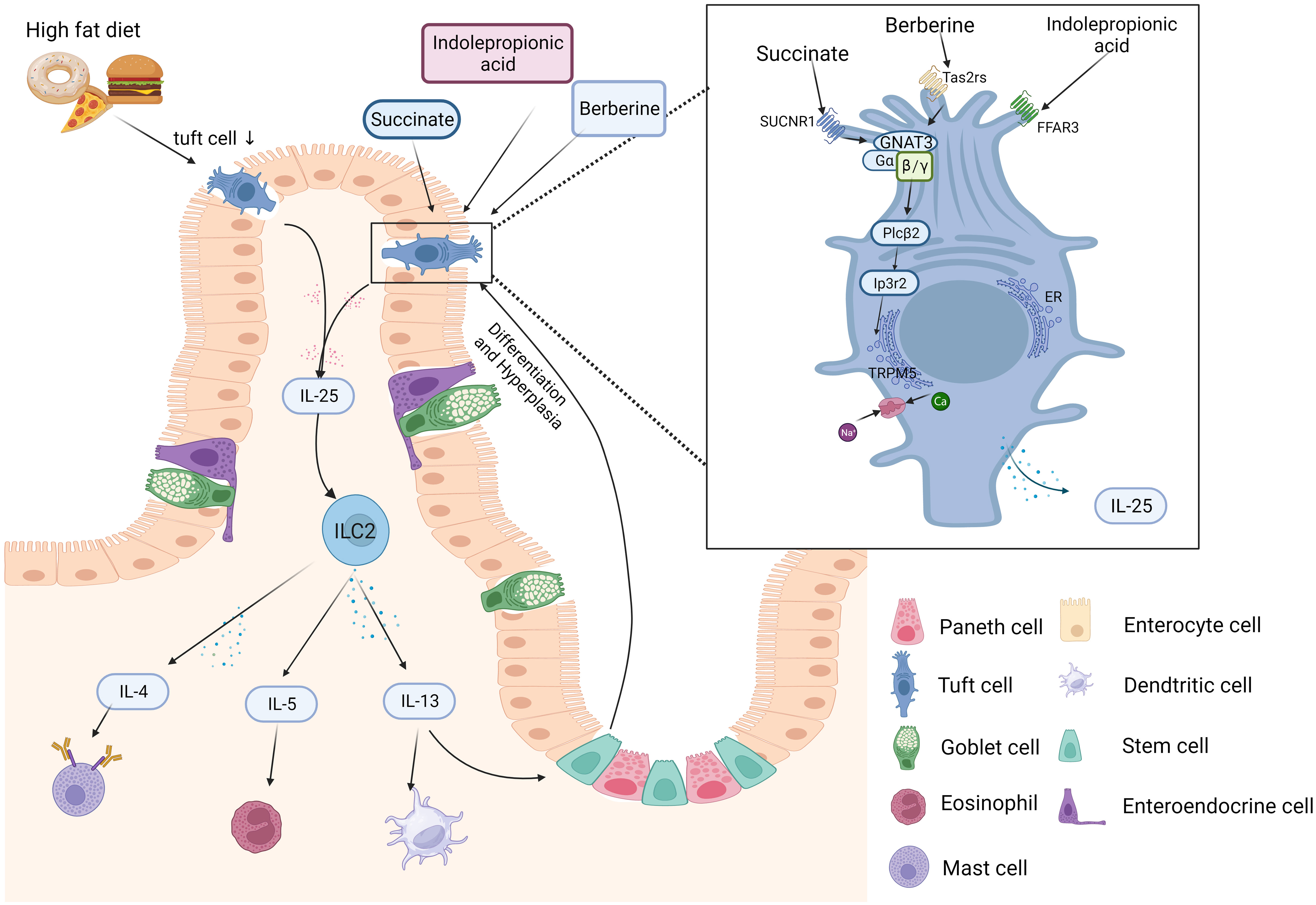
Figure 2 Schematic diagram of the intestinal TC-ILC2 circuit in obesity. Bacteria, metabolic byproducts (e.g., IPA and succinate), and helminths can be sensed by receptors on the surface of tuft cells (TCs) in the intestinal lumen. TC-derived IL-25 can induce Th2- and ILC2-secreted cytokines. Th2 cells mainly secrete IL-4, IL-5 and IL-13. ILC2s produce type 2 cytokines including IL-13 and IL-5. IL-13 can promote stem cell differentiation into TCs. IL-25 is the main molecule by which TCs “taste” metabolites (e.g., succinate) and activate signaling downstream pathways, including GNAT3 and TRPM5. The figure was created by Biorender.
5 The TC-ILC2 circuit may be a potential intervention target in obesity
Obese mice induced by HFD were administered IL-25 for 21 days, their body weight, serum glucose and intraperitoneal glucose tolerance were remarkably reversed (118). Despite the circuit, Feng reported that IL-25 promotes M2 macrophage polarization and thereby stimulates lipolysis and mitochondrial activity against obesity (119). IPA supplementation prevents the development of HFD-induced obesity (52), and the dosage of IPA is negatively correlated with obesity-induced inflammation (120). IPA holds potential as a reagent for obesity treatment due to its ability to activate TCs and promote IL-25 production. Furthermore, it exhibits interaction with FFAR3, further highlighting its potential as a valuable tool in combating obesity (52). A recent study by Sun (68) discovered that berberine, when sensed by TAS2Rs in TCs, stimulates the synthesis of IL-25. This feedback in TCs may turn out to be a novel mechanism by which berberine ameliorates obesity. Supplementation of drinking water with succinate robustly suppressed weight gain and improved glucose tolerance in HFD mice, resulting in an elevation in global energy expenditure (121). Thus, intervention targets on TCs, ILC2s or the circuit including IL-25, IPA, berberine and succinate, dramatically reduced the obesity status.
6 Conclusion and prospects
TCs use apical microvilli to taste danger signals, particularly metabolites derived from worms, viruses and bacteria. In response, TCs trigger downstream pathways to produce cytokines such as IL-25 to promote ILC2 activity, leading to Lgr5+ stem cell differentiation into TCs in a positive feedforward loop. In obese subjects, the TCs, ILC2s and the circuit are disrupted. IL-25, IPA and berberine have been reported to rescue the altered TC-ILC2 circuit in obese subjects, yet the underlying mechanisms remain to be fully elucidated. TC knockout models (27) and organoids (68, 122) could be better tools for further revealing the mechanism of TCs and ILC2s in obesity.
Author contributions
HY headed the team writing and editing. Y-XH, P-YX, J-QL, J-LC and XL, Y-JG contributed to writing and editing. W-JD reviewed and edited the draft. All authors contributed to the article and approved the submitted version.
Funding
The author(s) declare financial support was received for the research, authorship, and/or publication of this article. This research was funded by the Strengthening the Foundation Plan of Chengdu University of TCM (No. 242022007), “Xinglin Scholars” Discipline Talent Research Enhancement Program of Chengdu University of TCM (No. MPRC2022023), Chengdu Health Commission Research Project (No. 202317033303). and National Natural Science Foundation of China (NO. 82074151).
Conflict of interest
The authors declare that the research was conducted in the absence of any commercial or financial relationships that could be construed as a potential conflict of interest.
Publisher’s note
All claims expressed in this article are solely those of the authors and do not necessarily represent those of their affiliated organizations, or those of the publisher, the editors and the reviewers. Any product that may be evaluated in this article, or claim that may be made by its manufacturer, is not guaranteed or endorsed by the publisher.
References
1. Munir M, Zakaria ZA, Nisar H, Ahmed Z, Korma SA, Esatbeyoglu T. Global human obesity and global social index: relationship and clustering. Front Nutr (2023) 10:1150403. doi: 10.3389/fnut.2023.1150403
2. Liu BN, Liu XT, Liang ZH, Wang JH. Gut microbiota in obesity. World J Gastroenterol (2021) 27(25):3837–50. doi: 10.3748/wjg.v27.i25.3837
3. Montoro DT, Haber AL, Rood JE, Regev A, Rajagopal J. A synthesis concerning conservation and divergence of cell types across epithelia. Cold Spring Harb Perspect Biol (2020) 12(11):a035733. doi: 10.1101/cshperspect.a035733
4. Höfer D, Püschel B, Drenckhahn D. Taste receptor-like cells in the rat gut identified by expression of alpha-gustducin. Proc Natl Acad Sci (1996) 93(13):6631–4. doi: 10.1073/pnas.93.13.6631
5. Nadjsombati MS, McGinty JW, Lyons-Cohen MR, Jaffe JB, DiPeso L, Schneider C, et al. Detection of succinate by intestinal tuft cells triggers a type 2 innate immune circuit. Immunity (2018) 49(1):33–41.e7. doi: 10.1016/j.immuni.2018.06.016
6. Banerjee A, Herring CA, Chen B, Kim H, Simmons AJ, Southard-Smith AN, et al. Succinate produced by intestinal microbes promotes specification of tuft cells to suppress ileal inflammation. Gastroenterology (2020) 159(6):2101–15.e5. doi: 10.1053/j.gastro.2020.08.029
7. Gerbe F, Jay P. Intestinal tuft cells: epithelial sentinels linking luminal cues to the immune system. Mucosal Immunol (2016) 9(6):1353–9. doi: 10.1038/mi.2016.68
8. Howitt MR, Lavoie S, Michaud M, Blum AM, Tran SV, Weinstock JV, et al. Tuft cells, taste-chemosensory cells, orchestrate parasite type 2 immunity in the gut. Science (2016) 351(6279):1329–33. doi: 10.1126/science.aaf1648
9. Gerbe F, Sidot E, Smyth DJ, Ohmoto M, Matsumoto I, Dardalhon V, et al. Intestinal epithelial tuft cells initiate type 2 mucosal immunity to helminth parasites. Nature (2016) 529(7585):226–30. doi: 10.1038/nature16527
10. von Moltke J, Ji M, Liang H-E, Locksley RM. Tuft-cell-derived il-25 regulates an intestinal ilc2–epithelial response circuit. Nature (2015) 529(7585):221–5. doi: 10.1038/nature16161
11. von Moltke J. Intestinal Tuft Cells. In: Said HM, editor. Physiology of the Gastrointestinal Tract (Sixth Edition). London: Elsevier Inc. (2018). p. 721–33.
12. Schneider C, O’Leary CE, von Moltke J, Liang H-E, Ang QY, Turnbaugh PJ, et al. A metabolite-triggered tuft cell-ilc2 circuit drives small intestinal remodeling. Cell (2018) 174(2):271–84.e14. doi: 10.1016/j.cell.2018.05.014
13. Chen L, Li L, Ma L, Zhao Z, Luo S, Gong B, et al. Il-25–induced shifts in macrophage polarization promote development of beige fat and improve metabolic homeostasis in mice. PloS Biol (2021) 19(8):e3001348. doi: 10.1371/journal.pbio.3001348
14. Sasaki T, Moro K, Kubota T, Kubota N, Kato T, Ohno H, et al. Innate lymphoid cells in the induction of obesity. Cell Rep (2019) 28(1):202–17.e7. doi: 10.1016/j.celrep.2019.06.016
15. Hendel SK, Kellermann L, Hausmann A, Bindslev N, Jensen KB, Nielsen OH. Tuft cells and their role in intestinal diseases. Front Immunol (2022) 13:822867. doi: 10.3389/fimmu.2022.822867
16. Cheng X, Voss U, Ekblad E. Tuft cells: distribution and connections with nerves and endocrine cells in mouse intestine. Exp Cell Res (2018) 369(1):105–11. doi: 10.1016/j.yexcr.2018.05.011
17. Rhodin J, Dalhamn T. Electron microscopy of the tracheal ciliated mucosa in rat. Z für Zellforschung und Mikroskopische Anatomie (1956) 44(4):345–412. doi: 10.1007/BF00345847
18. Hoover B, Baena V, Kaelberer MM, Getaneh F, Chinchilla S, Bohórquez DV. The intestinal tuft cell nanostructure in 3d. Sci Rep (2017) 7(1):1652. doi: 10.1038/s41598-017-01520-x
19. Höfer D, Drenckhahn D. Identification of the taste cell G-protein, A-gustducin, in brush cells of the rat pancreatic duct system. Histochem Cell Biol (1998) 110(3):303–9. doi: 10.1007/s004180050292
20. Morroni M, Cangiotti AM, Cinti S. Brush cells in the human duodenojejunal junction: an ultrastructural study. J Anat (2007) 211(1):125–31. doi: 10.1111/j.1469-7580.2007.00738.x
21. Sato A, Hisanaga Y, Inoue Y, Nagato T, Toh H. Three-dimensional structure of apical vesicles of tuft cells in the main excretory duct of the rat submandibular gland. Eur J Morphol (2002) 40(4):235–9. doi: 10.1076/ejom.40.4.235.16690
22. Trier JS, Allan CH, Marcial MA, Madara JL. Structural features of the apical and tubulovesicular membranes of rodent small intestinal tuft cells. Anatomical Rec (2005) 219(1):69–77. doi: 10.1002/ar.1092190112
23. Höfer D, Drenckhahn D. Cytoskeletal markers allowing discrimination between brush cells and other epithelial cells of the gut including enteroendocrine cells. Histochem Cell Biol (1996) 105(5):405–12. doi: 10.1007/BF01463662
24. Kozono T, Tamura-Nakano M, Kawamura YI, Tonozuka T, Nishikawa A. Novel protocol to observe the intestinal tuft cell using transmission electron microscopy. Biol Open (2022) 11(2):bio059007. doi: 10.1242/bio.059007
25. Haber AL, Biton M, Rogel N, Herbst RH, Shekhar K, Smillie C, et al. A single-cell survey of the small intestinal epithelium. Nature (2017) 551(7680):333–9. doi: 10.1038/nature24489
26. Montoro DT, Haber AL, Biton M, Vinarsky V, Lin B, Birket SE, et al. A revised airway epithelial hierarchy includes cftr-expressing ionocytes. Nature (2018) 560(7718):319–24. doi: 10.1038/s41586-018-0393-7
27. Xiong Z, Zhu X, Geng J, Xu Y, Wu R, Li C, et al. Intestinal tuft-2 cells exert antimicrobial immunity via sensing bacterial metabolite N-undecanoylglycine. Immunity (2022) 55(4):686–700.e7. doi: 10.1016/j.immuni.2022.03.001
28. Biton M, Levin A, Slyper M, Alkalay I, Horwitz E, Mor H, et al. Epithelial micrornas regulate gut mucosal immunity via epithelium–T cell crosstalk. Nat Immunol (2011) 12(3):239–46. doi: 10.1038/ni.1994
29. McKinley ET, Sui Y, Al-Kofahi Y, Millis BA, Tyska MJ, Roland JT, et al. Optimized multiplex immunofluorescence single-cell analysis reveals tuft cell heterogeneity. JCI Insight (2017) 2(11):e93487. doi: 10.1172/jci.insight.93487
30. Gerbe F, van Es JH, Makrini L, Brulin B, Mellitzer G, Robine S, et al. Distinct atoh1 and neurog3 requirements define tuft cells as a new secretory cell type in the intestinal epithelium. J Cell Biol (2011) 192(5):767–80. doi: 10.1083/jcb.201010127
31. O’Leary CE, Schneider C, Locksley RM. Tuft cells—Systemically dispersed sensory epithelia integrating immune and neural circuitry. Annu Rev Immunol (2019) 37(1):47–72. doi: 10.1146/annurev-immunol-042718-041505
32. Huang Y-H, Klingbeil O, He X-Y, Wu XS, Arun G, Lu B, et al. Pou2f3 is a master regulator of a tuft cell-like variant of small cell lung cancer. Genes Dev (2018) 32(13-14):915–28. doi: 10.1101/gad.314815.118
33. O’Leary CE, Ma Z, Culpepper T, Novak SW, DelGiorno KE. New insights into tuft cell formation: implications for structure–function relationships. Curr Opin Cell Biol (2022) 76:102082. doi: 10.1016/j.ceb.2022.102082
34. Bomidi C, Robertson M, Coarfa C, Estes MK, Blutt SE. Single-cell sequencing of rotavirus-infected intestinal epithelium reveals cell-type specific epithelial repair and tuft cell infection. Proc Natl Acad Sci (2021) 118(45):e2112814118. doi: 10.1073/pnas.2112814118
36. Hoffman MT, Kemp SB, Salas-Escabillas DJ, Zhang Y, Steele NG, The S, et al. The gustatory sensory G-protein gnat3 suppresses pancreatic cancer progression in mice. Cell Mol Gastroenterol Hepatol (2021) 11(2):349–69. doi: 10.1016/j.jcmgh.2020.08.011
37. Pan J, Zhang L, Shao X, Huang J. Acetylcholine from tuft cells: the updated insights beyond its immune and chemosensory functions. Front Cell Dev Biol (2020) 8:606. doi: 10.3389/fcell.2020.00606
38. Yi J, Bergstrom K, Fu J, Shan X, McDaniel JM, McGee S, et al. Dclk1 in tuft cells promotes inflammation-driven epithelial restitution and mitigates chronic colitis. Cell Death Differentiation (2018) 26(9):1656–69. doi: 10.1038/s41418-018-0237-x
39. Kjærgaard S, Jensen TSR, Feddersen UR, Bindslev N, Grunddal KV, Poulsen SS, et al. Decreased number of colonic tuft cells in quiescent ulcerative colitis patients. Eur J Gastroenterol Hepatol (2020) 33(6):817–24. doi: 10.1097/meg.0000000000001959
40. Steele SP, Melchor SJ, Petri WA. Tuft cells: new players in colitis. Trends Mol Med (2016) 22(11):921–4. doi: 10.1016/j.molmed.2016.09.005
41. Schütz B, Ruppert A-L, Strobel O, Lazarus M, Urade Y, Büchler MW, et al. Distribution pattern and molecular signature of cholinergic tuft cells in human gastro-intestinal and pancreatic-biliary tract. Sci Rep (2019) 9(1):17466. doi: 10.1038/s41598-019-53997-3
42. Banerjee A, McKinley ET, von Moltke J, Coffey RJ, Lau KS. Interpreting heterogeneity in intestinal tuft cell structure and function. J Clin Invest (2018) 128(5):1711–9. doi: 10.1172/jci120330
43. Bjerknes M, Khandanpour C, Möröy T, Fujiyama T, Hoshino M, Klisch TJ, et al. Origin of the brush cell lineage in the mouse intestinal epithelium. Dev Biol (2012) 362(2):194–218. doi: 10.1016/j.ydbio.2011.12.009
44. Reynolds A, Wharton N, Parris A, Mitchell E, Sobolewski A, Kam C, et al. Canonical wnt signals combined with suppressed tgfβ/bmp pathways promote renewal of the native human colonic epithelium. Gut (2014) 63(4):610–21. doi: 10.1136/gutjnl-2012-304067
45. Liu Y, Chen Y-G. Intestinal epithelial plasticity and regeneration via cell dedifferentiation. Cell Regeneration (2020) 9(1):14. doi: 10.1186/s13619-020-00053-5
46. Westphalen CB, Asfaha S, Hayakawa Y, Takemoto Y, Lukin DJ, Nuber AH, et al. Long-lived intestinal tuft cells serve as colon cancer–initiating cells. J Clin Invest (2014) 124(3):1283–95. doi: 10.1172/jci73434
47. Herring CA, Banerjee A, McKinley ET, Simmons AJ, Ping J, Roland JT, et al. Unsupervised trajectory analysis of single-cell rna-seq and imaging data reveals alternative tuft cell origins in the gut. Cell Syst (2018) 6(1):37–51.e9. doi: 10.1016/j.cels.2017.10.012
48. Arora P, Andersen D, Moll JM, Danneskiold-Samsøe NB, Xu L, Zhou B, et al. Small intestinal tuft cell activity associates with energy metabolism in diet-induced obesity. Front Immunol (2021) 12:629391. doi: 10.3389/fimmu.2021.629391
49. Roach SN, Fiege JK, Shepherd FK, Wiggen TD, Hunter RC, Langlois RA, et al. Respiratory influenza virus infection causes dynamic tuft cell and innate lymphoid cell changes in the small intestine. J Virol (2022) 96(9):e0035222. doi: 10.1128/jvi.00352-22
50. Howitt MR, Cao YG, Gologorsky MB, Li JA, Haber AL, Biton M, et al. The taste receptor tas1r3 regulates small intestinal tuft cell homeostasis. ImmunoHorizons (2020) 4(1):23–32. doi: 10.4049/immunohorizons.1900099
51. Luo X-C, Chen Z-H, Xue J-B, Zhao D-X, Lu C, Li Y-H, et al. Infection by the parasitic helminthtrichinella spiralisactivates a tas2r-mediated signaling pathway in intestinal tuft cells. Proc Natl Acad Sci (2019) 116(12):5564–9. doi: 10.1073/pnas.1812901116
52. Chen L, Yang Y, Sun S, Xie Y, Pan C, Li M, et al. Indolepropionic acid reduces obesity-induced metabolic dysfunction through colonic barrier restoration mediated via tuft cell-derived il-25. FEBS J (2022) 289(19):5985–6004. doi: 10.1111/febs.16470
53. Gaetani S, Larsson MH, Håkansson P, Jansen FP, Magnell K, Brodin P. Ablation of trpm5 in mice results in reduced body weight gain and improved glucose tolerance and protects from excessive consumption of sweet palatable food when fed high caloric diets. PloS One (2015) 10(9):e0138373. doi: 10.1371/journal.pone.0138373
54. Ishimaru Y, Yamashita J, Ohmoto M, Yamaguchi T, Matsumoto I, Hirota J. Skn-1a/pou2f3 functions as a master regulator to generate trpm5-expressing chemosensory cells in mice. PloS One (2017) 12(12):e0189340. doi: 10.1371/journal.pone.0189340
55. Nakano H, Hata A, Ishimura U, Kosugi R, Miyamoto E, Nakamura K, et al. Activating transcription factor 5 (Atf5) controls intestinal tuft and goblet cell expansion upon succinate-induced type 2 immune responses in mice. Cell Tissue Res (2023) 393(2):343–55. doi: 10.1007/s00441-023-03781-7
56. Drurey C, Lindholm HT, Coakley G, Poveda MC, Löser S, Doolan R, et al. Intestinal epithelial tuft cell induction is negated by a murine helminth and its secreted products. J Exp Med (2022) 219(1):e20211140. doi: 10.1084/jem.20211140
57. Wilen CB, Lee S, Hsieh LL, Orchard RC, Desai C, Hykes BL, et al. Tropism for tuft cells determines immune promotion of norovirus pathogenesis. Science (2018) 360(6385):204–8. doi: 10.1126/science.aar3799
58. Schütz B JI, Bader S, Ringer C, von Engelhardt J, Chubanov V, Gudermann T, et al. Chemical coding and chemosensory properties of cholinergic brush cells in the mouse gastrointestinal and biliary tract. Front Physiol (2015) 6:87. doi: 10.3389/fphys.2015.00087
59. Varyani F, Löser S, Filbey KJ, Harcus Y, Drurey C, Poveda MC, et al. The il-25-dependent tuft cell circuit driven by intestinal helminths requires macrophage migration inhibitory factor (Mif). Mucosal Immunol (2022) 15(6):1243–56. doi: 10.1038/s41385-022-00496-w
60. Billipp TE, Fung C, Webeck LM, Sargent DB, Gologorsky MB, McDaniel MM, et al. Tuft cell-derived acetylcholine regulates epithelial fluid secretion. bioRxiv (2023) 2023-03. doi: 10.1101/2023.03.17.533208
61. McGinty JW, Ting H-A, Billipp TE, Nadjsombati MS, Khan DM, Barrett NA, et al. Tuft-cell-derived leukotrienes drive rapid anti-helminth immunity in the small intestine but are dispensable for anti-protist immunity. Immunity (2020) 52(3):528–41.e7. doi: 10.1016/j.immuni.2020.02.005
62. Kokrashvili Z, Rodriguez D, Yevshayeva V, Zhou H, Margolskee RF, Mosinger B. Release of endogenous opioids from duodenal enteroendocrine cells requires trpm5. Gastroenterology (2009) 137(2):598–606.e2. doi: 10.1053/j.gastro.2009.02.070
63. Weiss GA, Hennet T. Mechanisms and consequences of intestinal dysbiosis. Cell Mol Life Sci (2017) 74(16):2959–77. doi: 10.1007/s00018-017-2509-x
64. Chiba M, Morita N, Nakamura A, Tsuji K, Harashima E. Increased incidence of inflammatory bowel disease in association with dietary transition (Westernization) in Japan. JMA J (2021) 4(4):347–57. doi: 10.31662/jmaj.2021-0038
65. Tanaka S, Nemoto Y, Takei Y, Morikawa R, Oshima S, Nagaishi T, et al. High-fat diet-derived free fatty acids impair the intestinal immune system and increase sensitivity to intestinal epithelial damage. Biochem Biophys Res Commun (2020) 522(4):971–7. doi: 10.1016/j.bbrc.2019.11.158
66. Okumura R, Takeda K. Roles of intestinal epithelial cells in the maintenance of gut homeostasis. Exp Mol Med (2017) 49(5):e338–e. doi: 10.1038/emm.2017.20
67. Widmayer P, Pregitzer P, Breer H. Short-term high fat feeding induces inflammatory responses of tuft cells and mucosal barrier cells in the murine stomach. Histol Histopathol (2023) 38(3):273–86. doi: 10.14670/hh-18-503
68. Sun S, Yang Y, Xiong R, Ni Y, Ma X, Hou M, et al. Oral berberine ameliorates high-fat diet-induced obesity by activating tas2rs in tuft and endocrine cells in the gut. Life Sci (2022) 311:121141. doi: 10.1016/j.lfs.2022.121141
69. Aliluev A, Tritschler S, Sterr M, Oppenländer L, Hinterdobler J, Greisle T, et al. Diet-induced alteration of intestinal stem cell function underlies obesity and prediabetes in mice. Nat Metab (2021) 3(9):1202–16. doi: 10.1038/s42255-021-00458-9
70. Enriquez JR, McCauley HA, Zhang KX, Sanchez JG, Kalin GT, Lang RA, et al. A dietary change to a high-fat diet initiates a rapid adaptation of the intestine. Cell Rep (2022) 41(7):111641. doi: 10.1016/j.celrep.2022.111641
71. Li X, Huang G, Zhang Y, Ren Y, Zhang R, Zhu W, et al. Succinate signaling attenuates high-fat diet-induced metabolic disturbance and intestinal barrier dysfunction. Pharmacol Res (2023) 194:106865. doi: 10.1016/j.phrs.2023.106865
72. Pearson JA, Tai N, Ekanayake-Alper DK, Peng J, Hu Y, Hager K, et al. Norovirus changes susceptibility to type 1 diabetes by altering intestinal microbiota and immune cell functions. Front Immunol (2019) 10:2654. doi: 10.3389/fimmu.2019.02654
73. Vivier E, Artis D, Colonna M, Diefenbach A, Di Santo JP, Eberl G, et al. Innate lymphoid cells: 10 years on. Cell (2018) 174(5):1054–66. doi: 10.1016/j.cell.2018.07.017
74. Fallon PG, Ballantyne SJ, Mangan NE, Barlow JL, Dasvarma A, Hewett DR, et al. Identification of an interleukin (Il)-25–dependent cell population that provides il-4, il-5, and il-13 at the onset of helminth expulsion. J Exp Med (2006) 203(4):1105–16. doi: 10.1084/jem.20051615
75. Mjösberg JM, Trifari S, Crellin NK, Peters CP, van Drunen CM, Piet B, et al. Human il-25- and il-33-responsive type 2 innate lymphoid cells are defined by expression of crth2 and cd161. Nat Immunol (2011) 12(11):1055–62. doi: 10.1038/ni.2104
76. Ghaedi M, Shen ZY, Orangi M, Martinez-Gonzalez I, Wei L, Lu X, et al. Single-cell analysis of rorα Tracer mouse lung reveals ilc progenitors and effector ilc2 subsets. J Exp Med (2020) 217(3):e20182293. doi: 10.1084/jem.20182293
77. Gonzalez-Polo V, Pucci-Molineris M, Cervera V, Gambaro S, Yantorno SE, Descalzi V, et al. Group 2 innate lymphoid cells exhibit progressively higher levels of activation during worsening of liver fibrosis. Ann Hepatol (2019) 18(2):366–72. doi: 10.1016/j.aohep.2018.12.001
78. Rajput C, Cui T, Han M, Lei J, Hinde JL, Wu Q, et al. Rorα-dependent type 2 innate lymphoid cells are required and sufficient for mucous metaplasia in immature mice. Am J Physiology-Lung Cell Mol Physiol (2017) 312(6):L983–L93. doi: 10.1152/ajplung.00368.2016
79. Kyoizumi S, Kubo Y, Kajimura J, Yoshida K, Hayashi T, Nakachi K, et al. Fate decision between group 3 innate lymphoid and conventional nk cell lineages by notch signaling in human circulating hematopoietic progenitors. J Immunol (2017) 199(8):2777–93. doi: 10.4049/jimmunol.1601711
80. Kadel S, Ainsua-Enrich E, Hatipoglu I, Turner S, Singh S, Khan S, et al. A major population of functional klrg1– ilc2s in female lungs contributes to a sex bias in ilc2 numbers. ImmunoHorizons (2018) 2(2):74–86. doi: 10.4049/immunohorizons.1800008
81. Oyesola OO, Duque C, Huang LC, Larson EM, Früh SP, Webb LM, et al. The prostaglandin D2 receptor crth2 promotes il-33–induced ilc2 accumulation in the lung. J Immunol (2020) 204(4):1001–11. doi: 10.4049/jimmunol.1900745
82. Kanaoka Y, Boyce JA. Cysteinyl leukotrienes and their receptors; emerging concepts. Allergy Asthma Immunol Res (2014) 6(4):288–95. doi: 10.4168/aair.2014.6.4.288
83. Fritz JH, Angkasekwinai P, Sodthawon W, Jeerawattanawart S, Hansakon A, Pattanapanyasat K, et al. Ilc2s activated by il-25 promote antigen-specific th2 and th9 functions that contribute to the control of trichinella spiralis infection. PloS One (2017) 12(9):e0184684. doi: 10.1371/journal.pone.0184684
84. Hoyler T, Klose Christoph SN, Souabni A, Turqueti-Neves A, Pfeifer D, Rawlins Emma L, et al. The transcription factor gata-3 controls cell fate and maintenance of type 2 innate lymphoid cells. Immunity (2012) 37(4):634–48. doi: 10.1016/j.immuni.2012.06.020
85. Paclik D, Stehle C, Lahmann A, Hutloff A, Romagnani C. Icos regulates the pool of group 2 innate lymphoid cells under homeostatic and inflammatory conditions in mice. Eur J Immunol (2015) 45(10):2766–72. doi: 10.1002/eji.201545635
86. Cardoso V, Chesné J, Ribeiro H, García-Cassani B, Carvalho T, Bouchery T, et al. Neuronal regulation of type 2 innate lymphoid cells via neuromedin U. Nature (2017) 549(7671):277–81. doi: 10.1038/nature23469
87. Topczewska PM, Rompe ZA, Jakob MO, Stamm A, Leclère PS, Preußer A, et al. Ilc2 require cell-intrinsic st2 signals to promote type 2 immune responses. Front Immunol (2023) 14:1130933. doi: 10.3389/fimmu.2023.1130933
88. Nagasawa M, Spits H, Ros XR. Innate lymphoid cells (Ilcs): cytokine hubs regulating immunity and tissue homeostasis. Cold Spring Harbor Perspect Biol (2018) 10(12):a030304. doi: 10.1101/cshperspect.a030304
89. Spencer SP, Wilhelm C, Yang Q, Hall JA, Bouladoux N, Boyd A, et al. Adaptation of innate lymphoid cells to a micronutrient deficiency promotes type 2 barrier immunity. Science (2014) 343(6169):432–7. doi: 10.1126/science.1247606
90. Serafini N, Klein Wolterink RGJ, Satoh-Takayama N, Xu W, Vosshenrich CAJ, Hendriks RW, et al. Gata3 drives development of rorγt+ Group 3 innate lymphoid cells. J Exp Med (2014) 211(2):199–208. doi: 10.1084/jem.20131038
91. Yagi R, Zhong C, Northrup Daniel L, Yu F, Bouladoux N, Spencer S, et al. The transcription factor gata3 is critical for the development of all il-7rα-expressing innate lymphoid cells. Immunity (2014) 40(3):378–88. doi: 10.1016/j.immuni.2014.01.012
92. Walker JA, Oliphant CJ, Englezakis A, Yu Y, Clare S, Rodewald H-R, et al. Bcl11b is essential for group 2 innate lymphoid cell development. J Exp Med (2015) 212(6):875–82. doi: 10.1084/jem.20142224
93. Turner J-E, Morrison PJ, Wilhelm C, Wilson M, Ahlfors H, Renauld J-C, et al. Il-9–mediated survival of type 2 innate lymphoid cells promotes damage control in helminth-induced lung inflammation. J Exp Med (2013) 210(13):2951–65. doi: 10.1084/jem.20130071
94. Lee M, Ko H-J, Hong S-W, Park J, Ham S, Kim M, et al. Dietary antigens suppress the proliferation of type 2 innate lymphoid cells by restraining homeostatic il-25 production. Sci Rep (2022) 12(1):7443. doi: 10.1038/s41598-022-11466-4
95. Gury-BenAri M, Thaiss CA, Serafini N, Winter DR, Giladi A, Lara-Astiaso D, et al. The spectrum and regulatory landscape of intestinal innate lymphoid cells are shaped by the microbiome. Cell (2016) 166(5):1231–46.e13. doi: 10.1016/j.cell.2016.07.043
96. Neill DR, Wong SH, Bellosi A, Flynn RJ, Daly M, Langford TKA, et al. Nuocytes represent a new innate effector leukocyte that mediates type-2 immunity. Nature (2010) 464(7293):1367–70. doi: 10.1038/nature08900
97. Monticelli LA, Osborne LC, Noti M, Tran SV, Zaiss DMW, Artis D. Il-33 promotes an innate immune pathway of intestinal tissue protection dependent on amphiregulin–egfr interactions. Proc Natl Acad Sci (2015) 112(34):10762–7. doi: 10.1073/pnas.1509070112
98. Monticelli LA, Sonnenberg GF, Abt MC, Alenghat T, Ziegler CG, Doering TA, et al. Innate lymphoid cells promote lung-tissue homeostasis after infection with influenza virus. Nat Immunol (2011) 12(11):1045–54. doi: 10.1031/ni.2131
99. Lai D-M, Shu Q, Fan J. The origin and role of innate lymphoid cells in the lung. Military Med Res (2016) 3(1):25. doi: 10.1186/s40779-016-0093-2
100. Sonnenberg GF, Artis D. Innate lymphoid cells in the initiation, regulation and resolution of inflammation. Nat Med (2015) 21(7):698–708. doi: 10.1038/nm.3892
101. Huang Y, Guo L, Qiu J, Chen X, Hu-Li J, Siebenlist U, et al. Il-25-responsive, lineage-negative klrg1hi cells are multipotential ‘Inflammatory’ Type 2 innate lymphoid cells. Nat Immunol (2014) 16(2):161–9. doi: 10.1038/ni.3078
102. Leyva-Castillo JM, Galand C, Mashiko S, Bissonnette R, McGurk A, Ziegler SF, et al. Ilc2 activation by keratinocyte-derived il-25 drives il-13 production at sites of allergic skin inflammation. J Allergy Clin Immunol (2020) 145(6):1606–14.e4. doi: 10.1016/j.jaci.2020.02.026
103. Zhen Y, Shu W, Hou X, Wang Y. Innate immune system orchestrates metabolic homeostasis and dysfunction in visceral adipose tissue during obesity. Front Immunol (2021) 12:702835. doi: 10.3389/fimmu.2021.702835
104. Molofsky AB, Nussbaum JC, Liang H-E, Van Dyken SJ, Cheng LE, Mohapatra A, et al. Innate lymphoid type 2 cells sustain visceral adipose tissue eosinophils and alternatively activated macrophages. J Exp Med (2013) 210(3):535–49. doi: 10.1084/jem.20121964
105. Brestoff JR, Kim BS, Saenz SA, Stine RR, Monticelli LA, Sonnenberg GF, et al. Group 2 innate lymphoid cells promote beiging of white adipose tissue and limit obesity. Nature (2014) 519(7542):242–6. doi: 10.1038/nature14115
106. Cheng Z, Zhang L, Yang L, Chu H. The critical role of gut microbiota in obesity. Front Endocrinol (2022) 13:1025706. doi: 10.3389/fendo.2022.1025706
107. Kim CH. Control of lymphocyte functions by gut microbiota-derived short-chain fatty acids. Cell Mol Immunol (2021) 18(5):1161–71. doi: 10.1038/s41423-020-00625-0
108. Fernández-Veledo S, Vendrell J. Gut microbiota-derived succinate: friend or foe in human metabolic diseases? Rev Endocrine Metab Disord (2019) 20(4):439–47. doi: 10.1007/s11154-019-09513-z
109. Serena C, Ceperuelo-Mallafré V, Keiran N, Queipo-Ortuño MI, Bernal R, Gomez-Huelgas R, et al. Elevated circulating levels of succinate in human obesity are linked to specific gut microbiota. ISME J (2018) 12(7):1642–57. doi: 10.1038/s41396-018-0068-2
110. Gaspar RS, Delafiori J, Zuccoli G, Carregari VC, Prado TP, Morari J, et al. Exogenous succinate impacts mouse brown adipose tissue mitochondrial proteome and potentiates body mass reduction induced by liraglutide. Am J Physiology-Endocrinol Metab (2023) 324(3):E226–E40. doi: 10.1152/ajpendo.00231.2022
111. Lei W, Ren W, Ohmoto M, Urban JF, Matsumoto I, Margolskee RF, et al. Activation of intestinal tuft cell-expressed sucnr1 triggers type 2 immunity in the mouse small intestine. Proc Natl Acad Sci (2018) 115(21):5552–7. doi: 10.1073/pnas.1720758115
112. Arumugam P, Carroll KL, Berceli SA, Barnhill S, Wrenshall LE. Expression of a functional il-2 receptor in vascular smooth muscle cells. J Immunol (2019) 202(3):694–703. doi: 10.4049/jimmunol.1701151
113. Tang W, Smith SG, Beaudin S, Dua B, Howie K, Gauvreau G, et al. Il-25 and il-25 receptor expression on eosinophils from subjects with allergic asthma. Int Arch Allergy Immunol (2014) 163(1):5–10. doi: 10.1159/000355331
114. Bellocchia M, Boita M, Solidoro P, Coni F, Bardessono M, Mercante L, et al. Il-25 receptor expression on basophil membrane is related to phenotype and severity of asthma. Eur Respir Soc (2015) 46:PA1093. doi: 10.1183/13993003.congress-2015.PA1093
115. Basak O, Beumer J, Wiebrands K, Seno H, van Oudenaarden A, Clevers H. Induced quiescence of lgr5+ Stem cells in intestinal organoids enables differentiation of hormone-producing enteroendocrine cells. Cell Stem Cell (2017) 20(2):177–90.e4. doi: 10.1016/j.stem.2016.11.001
116. Fort MM, Cheung J, Yen D, Li J, Zurawski SM, Lo S, et al. Il-25 induces il-4, il-5, and il-13 and th2-associated pathologies in vivo. Immunity (2001) 15(6):985–95. doi: 10.1016/s1074-7613(01)00243-6
117. Claudio E, Sønder SU, Saret S, Carvalho G, Ramalingam TR, Wynn TA, et al. The adaptor protein ciks/act1 is essential for il-25-mediated allergic airway inflammation. J Immunol (2009) 182(3):1617–30. doi: 10.4049/jimmunol.182.3.1617
118. Smith AD, Fan A, Qin B, Desai N, Zhao A, Shea-Donohue T. Il-25 treatment improves metabolic syndrome in high-fat diet and genetic models of obesity. Diabetes Metab Syndrome Obesity: Targets Ther (2021) 14:4875–87. doi: 10.2147/dmso.S335761
119. Feng J, Li L, Ou Z, Li Q, Gong B, Zhao Z, et al. Il-25 stimulates M2 macrophage polarization and thereby promotes mitochondrial respiratory capacity and lipolysis in adipose tissues against obesity. Cell Mol Immunol (2017) 15(5):493–505. doi: 10.1038/cmi.2016.71
120. Tuomainen M, Lindström J, Lehtonen M, Auriola S, Pihlajamäki J, Peltonen M, et al. Associations of serum indolepropionic acid, a gut microbiota metabolite, with type 2 diabetes and low-grade inflammation in high-risk individuals. Nutr Diabetes (2018) 8(1):35. doi: 10.1038/s41387-018-0046-9
121. Mills EL, Pierce KA, Jedrychowski MP, Garrity R, Winther S, Vidoni S, et al. Accumulation of succinate controls activation of adipose tissue thermogenesis. Nature (2018) 560(7716):102–6. doi: 10.1038/s41586-018-0353-2
Keywords: obesity, tuft cell (TC), group 2 innate lymphoid cell (ILC2), TC-ILC2 circuit, intestinal remodeling, immune homeostasis
Citation: Yang H, Huang Y-X, Xiong P-Y, Li J-Q, Chen J-L, Liu X, Gong Y-J and Ding W-J (2024) Possible connection between intestinal tuft cells, ILC2s and obesity. Front. Immunol. 14:1266667. doi: 10.3389/fimmu.2023.1266667
Received: 26 July 2023; Accepted: 21 December 2023;
Published: 12 January 2024.
Edited by:
Kathrin S. Michelsen, Cedars Sinai Medical Center, United StatesReviewed by:
Vini John, Washington University in St. Louis, United StatesMichael Buchert, Olivia Newton-John Cancer Research Institute, Australia
Copyright © 2024 Yang, Huang, Xiong, Li, Chen, Liu, Gong and Ding. This is an open-access article distributed under the terms of the Creative Commons Attribution License (CC BY). The use, distribution or reproduction in other forums is permitted, provided the original author(s) and the copyright owner(s) are credited and that the original publication in this journal is cited, in accordance with accepted academic practice. No use, distribution or reproduction is permitted which does not comply with these terms.
*Correspondence: Wei-Jun Ding, RGluZ3dlaWp1bkBjZHV0Y20uZWR1LmNu
†These authors have contributed equally to this work and share first authorship