- 1Department of Pharmacy, Zhuhai People’s Hospital (Zhuhai Hospital Affiliated with Jinan University), Zhuhai, China
- 2College of Pharmacy, Jinan University, Guangzhou, China
Dendritic cells (DCs), a class of professional antigen-presenting cells, are considered key factors in the initiation and maintenance of anti-tumor immunity due to their powerful ability to present antigen and stimulate T-cell responses. The important role of DCs in controlling tumor growth and mediating potent anti-tumor immunity has been demonstrated in various cancer models. Accordingly, the infiltration of stimulatory DCs positively correlates with the prognosis and response to immunotherapy in a variety of solid tumors. However, accumulating evidence indicates that DCs exhibit a significantly dysfunctional state, ultimately leading to an impaired anti-tumor immune response due to the effects of the immunosuppressive tumor microenvironment (TME). Currently, numerous preclinical and clinical studies are exploring immunotherapeutic strategies to better control tumors by restoring or enhancing the activity of DCs in tumors, such as the popular DC-based vaccines. In this review, an overview of the role of DCs in controlling tumor progression is provided, followed by a summary of the current advances in understanding the mechanisms by which the TME affects the normal function of DCs, and concluding with a brief discussion of current strategies for DC-based tumor immunotherapy.
1 Introduction
Dendritic cells (DCs), first discovered by Steinman and Cohn in 1973 (1), serve as a bridge between innate and adaptive immunity in the host immune response. Based on differences in the expression of cell surface markers, DCs can be divided into two main subgroups: conventional DCs (cDCs) and plasmacytoid DCs (pDCs), each with a unique function in immune activity (2). cDCs have powerful antigen capture and presentation capacities and are one of the mainstays of T-cell activation in the body. In contrast, pDCs can present antigens to T-cells, although not as efficiently as cDCs. The main characteristic of pDCs is that they can direct the immune response by secreting high levels of type I interferons (IFN-I) (3, 4). Furthermore, DCs have been extensively studied, and their central role in initiating and maintaining anti-tumor immune responses to hinder tumor progression has been well established. However, the tumor microenvironment (TME) shows characteristics that are different from those of normal tissues, including the infiltration of a large population of immunosuppressive cells and a unique environment of hypoxia and lactate accumulation (5–7), rendering DCs incompetent by impairing their maturation, limiting their antigen capture, and downregulating the expression of costimulatory molecules in a variety of ways (8, 9). In this review, the essential role of DCs in tumor immunosurveillance is discussed, and the mechanisms by which the TME affects the function of DCs in tumors are summarized. Finally, we evaluated the improvement in DC-based tumor immunotherapy strategies, particularly DC-based vaccines.
2 The role of dendritic cells in tumor immunosurveillance
Effective anti-tumor immune responses involve a series of stepwise events. Chen et al. summarized the complex anti-tumor immune process as the “Cancer-Immunity Cycle” (reviewed in (10)), which provides an important framework for understanding the overall picture of the anti-tumor immune process. Furthermore, DCs are pivotal in the overall anti-tumor immune response due to their key role in T cell activation and immune response initiation (Figure 1). Briefly, immature DCs that infiltrate the tumor tissue recognize and phagocytose apoptotic or necrotic tumor cells and thus tumor cell antigens. They subsequently enter an activation/maturation process triggered by an intrinsic program and migrate from the tumor tissue via the lymphatic vessels or blood circulation to tumor-draining lymph nodes (TDLNs). During migration, DCs mature and acquire new characteristics, including the upregulation of CC-chemokine receptor 7 (CCR7) for improved motility, the upregulation of major histocompatibility complex (MHC) class I and class II molecules for antigen presentation, upregulation of costimulatory molecules such as CD80, CD86, and CD40, and increased cytokine secretion for enhanced T-cell stimulation. Mature DCs load endo-processed antigenic peptides onto MHC class I or MHC class II molecules for presentation to naïve T-cells, and at the same time, the costimulatory molecules interact with the ligands on T cells, which synergistically stimulate the activation and differentiation of T-cells in TDLNs (3, 11–17). Tertiary lymphoid structures (TLS), which are crucial in the anti-tumor immune response, may also be the destination for the migration of mature DCs (18, 19). The TLS may represent a privileged site for the local presentation of neighboring tumor antigens to T-cells by DCs and the activation, proliferation, and differentiation of T-cells (19, 20). This is also supported by a single-cell analysis of human non-small cell lung cancer lesions, which showed that mature DCs enriched in immunoregulatory molecules (mregDCs) accumulated in the TLS in close proximity to T-cells (21). MregDCs are a new cluster of DCs identified by Maier et al. in human and mouse non-small cell cancers and are characterized by the expression of both maturation markers and regulatory molecules (22). MregDCs have also been described in various human cancers, including hepatocellular carcinoma (23), breast cancer (24), colon cancer (25), and gastric cancer (26). Li et al. summarized the basic characteristics of mregDCs and suggested that lysosomal-associated membrane protein 3 (LAMP3) may be a fundamental recognition marker for them (27). Ginhoux et al. proposed that mregDCs can refer to a distinct molecular state induced in cDC1s, cDC2s, and potentially inflammatory DC3s upon sensing or capturing cell-associated materials that have a distinct ability to interact with antigen-specific T-cells (28). Analysis of tumors and metastatic lymph nodes from patients with head and neck lymphoma revealed that mregDCs may contribute to the prognosis by balancing regulatory and effector T-cells (29).
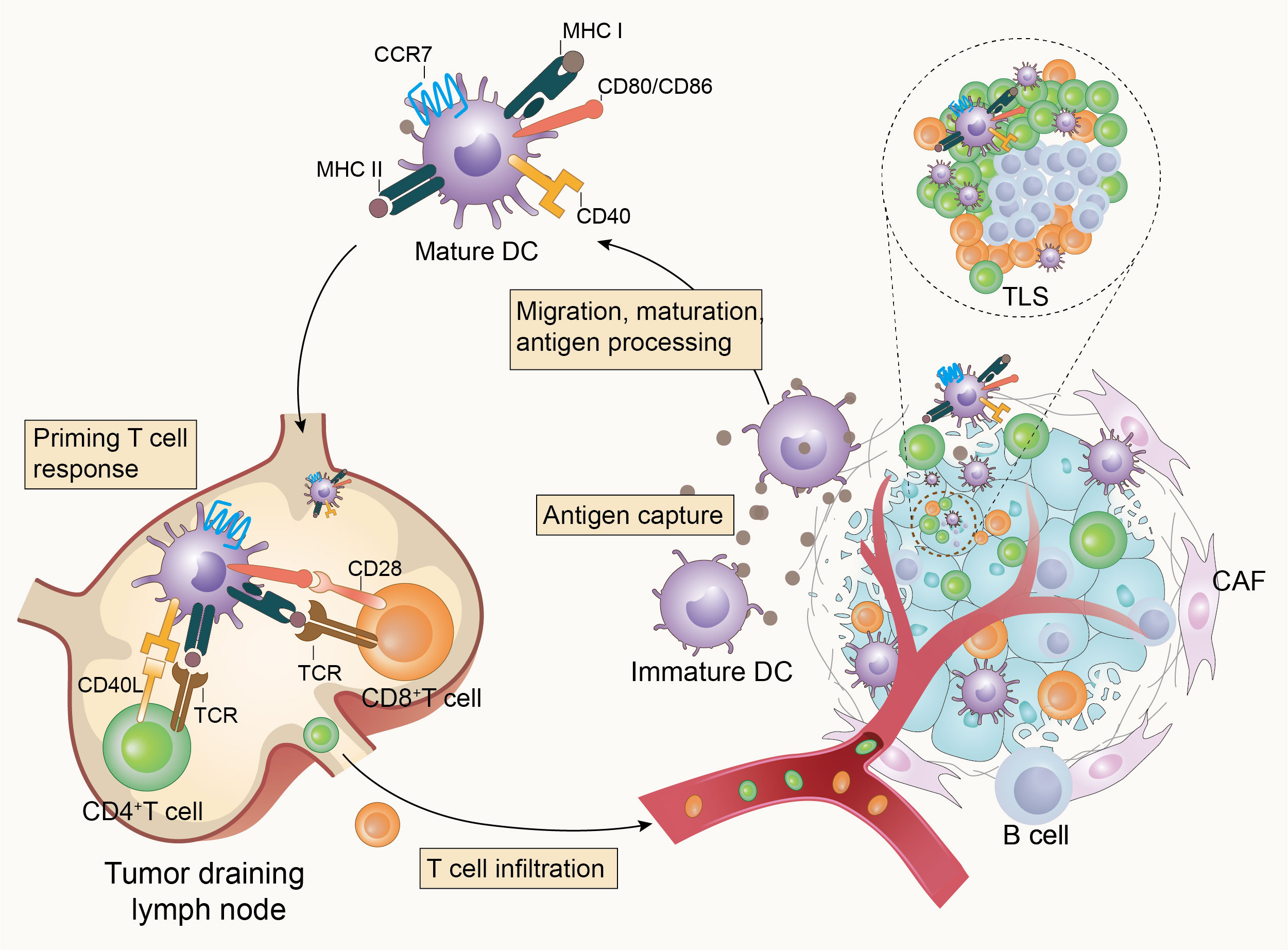
Figure 1 Dendritic cells initiate anti-tumor immunity. Tumor-infiltrating dendritic cells recognize and capture tumor-associated antigens, then become mature and homing to tumor-draining lymph nodes (TDLNs) or tertiary lymphoid structures (TLS) to activate T-cells and initiate anti-tumor immunity in response to the presence of tumors.
It is well established that DCs play a key role in stimulating cytotoxic T-cells and driving immune responses against cancer and that the levels of intratumoral stimulatory DCs in human tumors are associated with increased overall survival (30–32). Hegde et al. suggested that different scales of infiltration of cDCs would induce different levels of T-cell responses and that increased infiltration and activation of cDCs enhanced the activity of CD8+ T and TH1 cells in a pancreatic cancer mouse model (33). In addition, further evidence for the role of DCs in controlling tumor development is derived from the fact that the absence and dysfunction of DCs in tumor-bearing mouse models lead to poorer outcomes and insensitivity to anti-tumor treatment. Batf3-deficient mice (Batf3-/-) lack cross-presenting DCs and fail to trigger cytotoxic T lymphocyte-mediated immune responses to tumor-associated antigens (34–36), and Mittal et al. observed increased tumor metastasis and poorer survival in Batf3-/- mouse models of breast cancer and melanoma than in wild-type mice (37). Furthermore, it has been observed in several Batf3-/- mouse models that activated DCs are required to promote the anti-tumor efficacy of immunostimulatory antibodies, such as anti-PD-1, anti-PD-L1, and anti-CD137, and deficiencies in DCs limit the efficacy (35, 38). This suggests that the functional status of DCs is closely related to the efficacy of tumor immunotherapy. pDCs have a weak antigen-presenting capacity but can participate in the tumor immune response in other ways, such as by secreting IFN-I (39) and cross-priming naïve CD8+ T-cells by transferring antigens to cDCs via exosomes (40). However, the function of pDCs in TME remains controversial. In patients with colon cancer, an increased density of infiltrating pDCs was significantly correlated with increased progression-free and overall survival (41). In addition, a naturally occurring pDCs subset expressing high levels of OX40 with a unique immunostimulatory phenotype was identified in the TME of patients with head and neck squamous cell carcinoma, which, when synergized with cDCs, generated potent tumor antigen-specific CD8+ T-cell responses (42). However, as reported by Sisirak and partners, tumor-infiltrating pDCs in patients with breast and ovarian cancer are associated with poor outcomes (43, 44), and this may be linked to tumor cell-derived cytokines such as TGF-β and TNF-α, which limit the ability of pDCs to produce IFN-I and induce them to be tolerogenic (45, 46). The specific microenvironmental context and functional status of pDCs appear to determine their effects on cancer immunity and patient outcomes.
Overall, the evidence indicates that DCs, although representing a relatively rare subset of immune cells, are an essential part of anti-tumor immunogenesis. Moreover, when functionally activated, they are associated with stalled tumor progression and improved therapeutic responsiveness. However, the prognostic role of DCs in patients with cancer cannot be generalized and is largely dependent on the density, maturation, and activity of DCs. In general, tumor infiltration by activated, well-functioning DCs tends to predict a better prognosis, whereas DCs with impaired functional status in the TME may have the opposite effect on tumor progression (47–50). The TME causes the loss of antigen presentation and T-cell stimulatory capacity by inhibiting the maturation and migration of DCs, altering their ability to secrete cytokines. This can even induce tolerogenic or immunosuppressive DCs, allowing the tumor to escape surveillance and extermination by the immune system.
3 Immunosuppressive effects of the TME on dendritic cells
The conditions for tumor development, metastasis, and invasion are provided by the TME, a complex and dynamically evolving system composed of numerous components, including tumor cells, immune cells, the extracellular matrix, and soluble cytokines. Accumulating evidence indicates that immunosuppressive populations and stromal cells, as well as the unique metabolic environment of the TME, negatively regulate the maturation, migration, and effector functions of DCs (Figure 2).
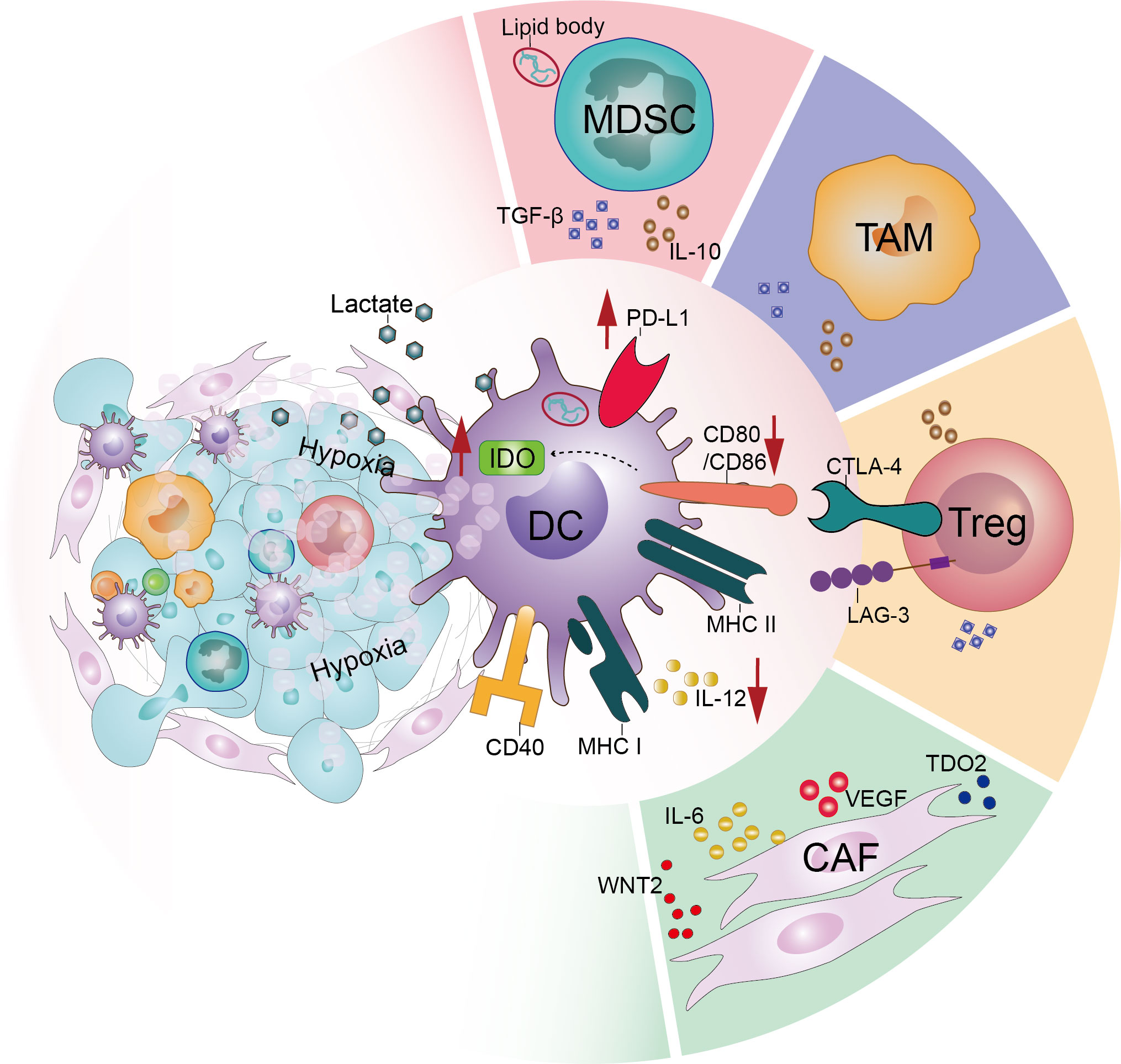
Figure 2 Tumor microenvironment acts on dendritic cells and downregulates their function. In the tumor microenvironment, various factors interact directly or indirectly with dendritic cells to dysfunction them. These include the large number of immunosuppressive populations such as regulatory T-cells (Tregs), tumor-associated macrophages (TAMs) and myeloid-derived suppressor cells (MDSCs) infiltrating the tumor microenvironment. In addition, the effects of stromal cells such as cancer-associated fibroblasts (CAFs) and the particular hypoxic and acidic microenvironment of the tumor microenvironment cannot be ignored.
3.1 Inhibition of dendritic cells by immunosuppressive populations
One of the most prominent features of the TME is the progressive accumulation of tumor-associated immunosuppressive cell populations, such as regulatory T-cells (Tregs), myeloid-derived suppressor cells (MDSCs), and tumor-associated macrophages (TAMs) (51, 52).
Aberrant chemokine alterations in the TME are important in the tumor recruitment of immunosuppressive cells (53). Tumor cells can induce the migration of Tregs to the TME by upregulating the expression of several chemokines, including the C-C motif chemokine ligand (CCL) 17/22 (54), CCL20 (55), and CCL28 (56, 57). Moreover, the ability of Tregs to use free fatty acids and lactate allows them to survive and maintain their suppressive identity, particularly in a harsh nutrient TME (58, 59). Tregs are a major suppressor group that induce DCs dysfunction and limit tumor immunogenesis (60). One important mechanism by which Tregs cause DCs dysfunction is through cytotoxic T-lymphocyte-associated antigen 4 (CTLA-4). Tregs expressing CTLA-4 compete with CD28 on conventional T-cells for the co-stimulatory molecules CD80 and CD86 on the surface of DCs, with CTLA-4 having a greater affinity and avidity than CD28 (61). In addition, Tregs are able to downregulate CD80/CD86 molecules expressed by DCs in a CTLA-4-dependent manner (62–65), and depletion of CD80/86 in mice was also found to cause upregulation of PD-L1 in DCs (66), resulting in multiple inhibitory effects on DC-mediated T-cell immune responses. Furthermore, the interaction of CTLA-4 with CD80/CD86 induces the production of indoleamine-2,3-dioxygenase (IDO) in DCs, which can induce tryptophan catabolism to pro-apoptotic metabolites, leading to the suppression of effector T-cell activation (67–69). In addition to CTLA-4, lymphocyte activation gene-3 (LAG-3), an immune checkpoint molecule that has recently received considerable attention, is constitutively expressed on Tregs and can limit the T cell stimulatory capacity of DCs by interacting with MHC class II molecules (70, 71). A number of other interactions, including the secretion of inhibitory cytokines such as IL-10 and TGF-β (72), delivery of miRNAs to DCs by secreted extracellular vesicles, thereby inducing a tolerogenic phenotype in DCs (73), expression of CD27 molecules that interfere with CD70/CD27 stimulatory signaling between DCs and effector T-cells (74), and direct induction of death through mutual contact with DCs (75), are also important means for Tregs to impede the onset of DCs-mediated tumor immunity. Consistently, enhanced anti-tumor immune responses induced by DCs have been observed after reducing the infiltration of tumor-associated Tregs and the secretion of their immunosuppressive molecules in various tumor-bearing mouse models (67, 76–78). Thus, Tregs appear to be an important cell subpopulation in the TME that acts directly on DCs and mediates their dysfunction, so the depletion of Tregs may be beneficial for DCs to mediate anti-tumor immunity.
MDSCs are a heterogeneous population of immature myeloid cells with immunosuppressive properties. Under the stimulation of the pathological conditions of cancer, the maturation and differentiation of bone marrow-derived progenitor cells are blocked, resulting in the accumulation of immunosuppressive MDSCs. MDSCs are recruited to the TME via multiple chemokine signals such as CCL2, CCL5, CCL26, C-X-C motif chemokine ligand (CXCL) 8, CXCL12, and other mediators such as granulocyte-macrophage colony-stimulating factor (GM-CSF), IL-6, or prostaglandin E2 (PGE2) that participate in expanding MDSCs (79). Previous studies have shown that activated MDSCs impede anti-tumor immunity and promote tumor progression through a series of actions, and that DCs are negatively affected (80). Hu et al. observed that upregulated MDSCs were associated with higher IL-10 expression, lower IL-12 production by DCs, and lower T-cell stimulatory activity in mice with hepatocellular carcinoma (81). Furthermore, it has been reported that tumor-associated DCs accumulate large amounts of lipid bodies (LB) containing oxidized lipids, impeding cross-presentation in DCs by covalently binding to heat shock protein 70 and preventing the translocation of peptide-MHC I complexes (pMHC) to the cell surface (82–84). Ugolini et al. found that in tumor-bearing mice, polymorphonuclear (PMN)-MDSCs are able to transfer lipid bodies to DCs, causing them to exhibit impaired antigen cross-presentation. Consistently, in MDSCs depleted or myeloperoxidase (MPO, a key enzyme for the production of oxidized lipids in MDSCs) deficient mice, DCs showed improved activity for tumor antigens cross-presentation (85). Thus, it appears that the abnormally large accumulation of lipids and impaired antigen cross-presentation in DCs are at least partially related to MDSCs and that selective depletion of MDSCs may be a potential option for restoring the function of DCs in tumor conditions.
In many solid tumor types, TAMs are among the most abundant populations of tumor-infiltrating immune cells in the TME (86). TAMs may localize to the TME either by traveling via chemotactic gradients regulated by factors such as CCL2, IL-1β, and macrophage colony-stimulating factor 1 (CSF1), differentiating from monocytes in the TME or by repolarization of tissue-resident macrophages (87). In addition, TAMs in the TME are more inclined to polarize into an anti-inflammatory phenotype due to the influence of cytokines such as PGE2 (88–90). TAMs are involved in multiple aspects of immunosuppression, and a high infiltration of TAMs into solid tumors is usually associated with a poor prognosis (86, 91–93). Unlike Tregs, which interact directly with DCs, TAMs mediate the recruitment of other immunosuppressive cells and secrete inhibitory cytokines that influence the maturation and function of DCs (94). Ruffell et al. described that in the TME of breast cancer mice, TAMs inhibit the production of IL-12 by DCs through the secretion of IL-10, attenuating the cytotoxic CD8+ T-cell response (95). Several preclinical studies have also suggested that TAM depletion in the TME can reshape the link between DCs and T-cells. For example, in a study based on a murine model of lung cancer, after targeting macrophages with a CSF1R inhibitor (CSF1Ri), the authors observed increased crosstalk between immunostimulatory populations, including DCs, NK cells, and T-cells, and increased levels of IL-12 expressed by DCs and T-cells, respectively (96). TAMs were consistently targeted by CSF1Ri (PLX3397) in a mouse model of mesothelioma. When combined with a DC-based vaccine, a robust and durable anti-tumor immune response was observed (97).
3.2 The function of dendritic cells is limited by stromal cells
Tumor progression and immune tolerance cannot be achieved without the involvement of tumor stromal components (98). Cancer-associated fibroblasts (CAFs), a complex and heterogeneous cell population, are the most abundant components of a tumor stroma. Tissue-resident fibroblasts are the major sources of CAFs (99), which can be activated by stimulation of various factors of TME such as TGF-β, TNF, fibroblast growth factor, and platelet-derived growth factor (100, 101). Additionally, mesenchymal stem cells, epithelial cells, and endothelial cells adjacent to cancer cells and fibroblasts recruited from the bone marrow are potential sources of CAFs (102, 103). The interaction of CAFs with immune cells has been identified as a key contributor to tumor progression. Several recent studies have revealed that CAFs can drive the immune escape of tumor cells by impeding the maturation, migration, and antigen presentation of DCs. Berzaghi et al. reported that the co-incubation of CAFs obtained from surgically resected fresh tumor tissue from lung cancer patients with mature DCs results in impaired migration and antigen uptake (104). In another study, it was proposed that human lung cancer cell-stimulated CAFs impair the differentiation and function of DCs by upregulating tryptophan-2,3-dioxygenase (TDO2) (105). Cheng et al. found that in vitro hepatocellular carcinoma patient-derived CAFs can recruit normal DCs and mediate STAT3 pathway activation by expressing IL-6, inducing their transformation into regulatory DCs (106). Furthermore, CAFs secrete abundant active factors such as vascular endothelial growth factor (VEGF), which promote angiogenesis while mediating damage to the migratory and T-cell stimulatory capacities of DCs (107, 108). Excellent work was reported by Huang et al., who found that CAF-secreted WNT2 was involved in the differentiation and immunostimulatory activity of DCs in vitro, and accordingly, anti-WNT2 was observed to increase the level of intratumoral activated DCs and significantly improve the anti-tumor responses of DC-mediated antigen-specific CD8+ T cells in murine tumor models (109). This suggests that in the TME, both stromal cells and immunosuppressive cells influence anti-tumor immunity. Therefore, for effective tumor therapy, it is essential to consider targeting stromal cells.
3.3 Environmental factors that regulate dendritic cell function in the TME
Compared with normal tissues, the TME exhibits a significantly hypoxic and acidic environment and is an important mediator of tumor progression.
Hypoxia is a central player in shaping the immune context of the TME, which results from an imbalance between increased oxygen consumption and inadequate oxygen supply owing to the rapid proliferation of tumor cells (110). Many physiological functions of DCs, including migration and maturation, are regulated by hypoxia. Hypoxic immature DCs exhibit upregulated motility/migration ability (111), while their antigen uptake ability is seemingly downregulated (112, 113). Consistently, Suthen et al. observed significant enrichment of Tregs and cDC2 in hypoxic regions of tumor samples from patients with HCC, as well as lower CD8+ T-cells, and found a significant downregulation of HLA-DR expression by cDC2 under hypoxic conditions, which may be related to the increased intercontact between Tregs and cDC2 during hypoxia (114). Besides, it is well known that hypoxia-inducible factor-1alpha (HIF-1α) plays a key role in the cellular response to hypoxia (115, 116), yet the effects of HIF-1α on DCs appear to be controversial. On the one hand, several scholars have demonstrated that the increase in HIF-1α in DCs under hypoxia is accompanied by an increase in the expression of HIF-1α target genes, including those involved in glycolysis, and that the increase in glycolysis will promote the maturation and migration of DCs (117–119). On the other hand, however, it has been proposed that constitutive expression of HIF-1α impairs the immunostimulatory capacity of DCs in vivo by inducing DCs to upregulate the expression of immunosuppressive mediators such as IL-10, iNOS, and VEGF (120, 121). Additionally, prolonged exposure to hypoxia induces cell death in DCs, which can be prevented by HIF-1α inhibition, suggesting that HIF-1α may be involved in this process (122). It was observed in human glioma cells that hypoxia induces PD-L1 upregulation in an HIF-1α-dependent manner, and it was further found in a murine glioma model that the combination of HIF-1α inhibitor and anti-PD-L1 antibody can improve the activation of DCs and CD8+ T-cells (123). Notably, hypoxic conditions recruit more immunosuppressive Tregs (56, 114) and TAMs (124), thereby indirectly curbing the function of DCs. Overall, hypoxia appears to facilitate the migration and maturation of DCs and compromise their normal functions. The exact changes in the behavior of DCs under hypoxic conditions need to be further elucidated.
Tumor cells exhibit altered metabolism, preferentially converting glucose to lactate through glycolysis even under oxygen-rich conditions. This results in a large accumulation of lactate and increases the acidity of the TME (125–127). Numerous studies have shown that lactate accumulation in the TME adversely affects the DC function. For example, tumor-derived lactate restricts the presentation of tumor-specific antigens by DCs to other immune cells (128). Lactate is also involved in regulating the phenotype of DCs, resulting in increased production of anti-inflammatory cytokines and decreased production of pro-inflammatory cytokines (129, 130). In patients with melanoma, the function of pDCs is impaired by lactic acidosis (131), with the same phenomenon observed in patients with breast cancer and murine models (132). Some researchers have suggested that in mice, the migratory capacity of DCs is significantly diminished in acidic environments and does not recover after removal of the acidic microenvironment, suggesting that extracellular acidosis may cause irreversible DCs dysfunction (133). In addition, exposure of mesothelioma cells to acidosis promotes the secretion of TGF-β2, which in turn leads to the accumulation of lipid droplets in DCs, resulting in a reduction in DC migratory capacity (134). These findings support the view that an acidic environment is not conducive to the proper functioning of DCs. However, Geffner et al. argued that extracellular acidosis stimulates antigen capture, promotes the expression of MHC class II molecules CD86 and CD40, and induces the maturation and secretion of IL-12 in mouse (135) and human DCs (136). Notably, the maintenance of an acidic environment and the accumulation of lactate in the TME complement each other. In tumors, an acidic environment can promote the accumulation of lactate and thus impair the function of DCs.
In general, owing to the combination of many factors in the TME, DCs are significantly dysfunctional. An accurate understanding of the role of each component in DC dysfunction will help to better understand the tumor state and to accurately explore ways to restore the activity of DCs. However, the TME is a complex and interconnected whole, and ultimately, all factors need to be linked for a systematic and comprehensive understanding of the causes and processes of the dysfunction of DCs.
4 Dendritic cell-based strategies for cancer immunotherapy
As the key activators of the immune response, the immune activation potential of DCs can be used to induce anti-tumor responses in patients with cancer, which is a promising development. Primary strategies based on DCs include the creation of immunoenhancers that promote the generation and activation of DCs, or the preparation of autologous DC-based vaccines for patient administration. Flt3L, GM-CSF, and Toll-like receptor (TLR) ligands are common immunoenhancers. The development and maintenance of DCs depend on the Flt/Flt3L axis (137), and attempts have been made in clinical studies to enhance the immune response induced by tumor vaccines by administering Flt3L (NCT02129075) (138). GM-CSF stimulates the differentiation, activation, and migration of DCs (139, 140), and consistently, administration of the CpG ODN/GM-CSF combination in melanoma patients results in enhanced mutation of all identifiable DC subpopulations and the recruitment of T-cell-stimulating and cross-presenting DCs to support protective melanoma immunity (141). When combined with TLRs in DCs, TLR ligands can activate signal transduction pathways and induce the expression of genes involved in the maturation of DCs (142). Therefore, some immunostimulatory ligands for TLRs, such as poly(I:C), are often used as immunoadjuvants in DC-based therapies and have shown promising results (143, 144). DEC205, also known as CD205 or LY75, is an endocytic receptor expressed at high levels by CD8+ DCs and is involved in antigen uptake and cross-presentation (145). The fusion of tumor antigens with targeted antibodies against DEC205 to enhance DC-induced immune responses has been well studied and explored in clinical trials (138, 146). Recently, a pioneering study provided new insights into the application of DEC205 as a therapeutic target. Martinek et al. analyzed the transcriptome of T-cells and macrophages in situ in melanoma patient samples using immunofluorescence-guided laser capture microdissection and observed that stromal macrophages contained a gene expression signature linked to antigen capture and presentation (CD14+LY75+). This can distinguish patients with significantly better long-term survival and includes a gene module of monocyte-derived DCs (147). This study provides valuable insights into the reprogramming of stromal macrophages to upregulate gene features related to antigen capture and presentation to acquire DCs function and could be a potential option for cancer therapy.
DC-based therapeutic cancer vaccines are a popular strategy for stimulating an effective tumor immune response as they return autologous activated DCs loaded with tumor-associated antigens to patients (148). In April 2010, the FDA approved the marketing of the first DCs vaccine, sipuleucel-T, for the treatment of prostate cancer (149). Furthermore, in the NCCN Clinical Practice Guidelines in Oncology (NCCN Guidelines®): Prostate Cancer (version 1.2023), sipuleucel-T is recommended for the treatment of metastatic castration-resistant prostate cancer (CRPC) and is a category 1 option for certain patients who have not received previous treatment with docetaxel or novel hormone therapy. Sipuleucel-T is also an option for patients with metastatic CRPC who have received prior treatment with docetaxel or a novel hormone therapy, but not for patients who have already received both (150). In recent years, DC-based vaccines have undergone extensive clinical trials for the treatment of various cancers, including liver cancer (151), melanoma (152), lung cancer (153), ovarian cancer (154), and pancreatic cancer (155). Although the safety of DC-based vaccines has been proven over the past few decades, their clinical efficacy requires improvement. Consequently, DC-based vaccines are undergoing a great deal of technical innovation, including the selection of DC subpopulations, methods of induction maturation, and choice of loading antigens (148, 156), with the aim of exploiting the anti-tumor potential of DCs more effectively.
The key to cancer immunotherapy is the manipulation of the immune system to achieve cancer control and the desired treatment. The efficacy of immune checkpoint inhibitors, which have shown some success, depends largely on the present baseline immune response, and DC-based vaccines are highly effective at rescuing the baseline anti-tumor immune response. Therefore, there has been considerable interest in combining DC-based vaccines with immune checkpoint inhibitors (ICIs), and several such studies have been conducted in recent years (Table 1). Recently, Guo et al. reported a case of a patient with metastatic gastric cancer whose tumor progressed after the first two months of receiving personalized neoantigen-loaded monocyte-derived dendritic cell (Neo-MoDC) vaccine alone, despite the observed T-cell response against the tumor neoantigen and the fact that upregulated PD-1 levels in T-cells were observed after Neo-MoDC vaccine administration. Subsequently, the patient received a combination treatment of the Neo-MoDC vaccine and nivolumab; promisingly, the combination triggered a stronger immune response and mediated complete regression of all tumors for over 25 months (157). Furthermore, anti-PD-1/PD-L1 antibodies in combination with DC-based vaccines have been extensively explored in a variety of murine tumor models (158–164) and, without exception, combination treatment has shown superior efficacy compared to monotherapy, with stronger anti-tumor-specific T-cell responses and lower immunosuppressive cell infiltration. Additionally, the combination of anti-CTLA-4 and DC-based vaccines could lead to more effective cancer treatments. For example, in a clinical trial (NCT01302496), researchers enrolled 39 patients with pretreated advanced melanoma who received a DC-based mRNA vaccination plus ipilimumab. The results showed that a strong tumor-associated antigen-specific immune response was observed in patients treated with the combination of a DC-based vaccine and ipilimumab, with an encouraging 6-month overall response rate of 38%. Subsequent long-term follow-up after more than 5 years indicates that 7/39 patients, who all achieved a complete response, were still disease-free (165). Similarly, in the exploration of multiple preclinical experimental models of pancreatic cancer (166), breast cancer (167), colorectal cancer (168), and melanoma (169), the silencing of CTLA-4 can induce a more effective anti-tumor immune response together with DC-based vaccines by reducing the infiltration of immunosuppressive cells and increasing the Teff/Treg ratio. In summary, combining DC-based vaccines with immune checkpoint inhibitors is a promising option for treating tumors.
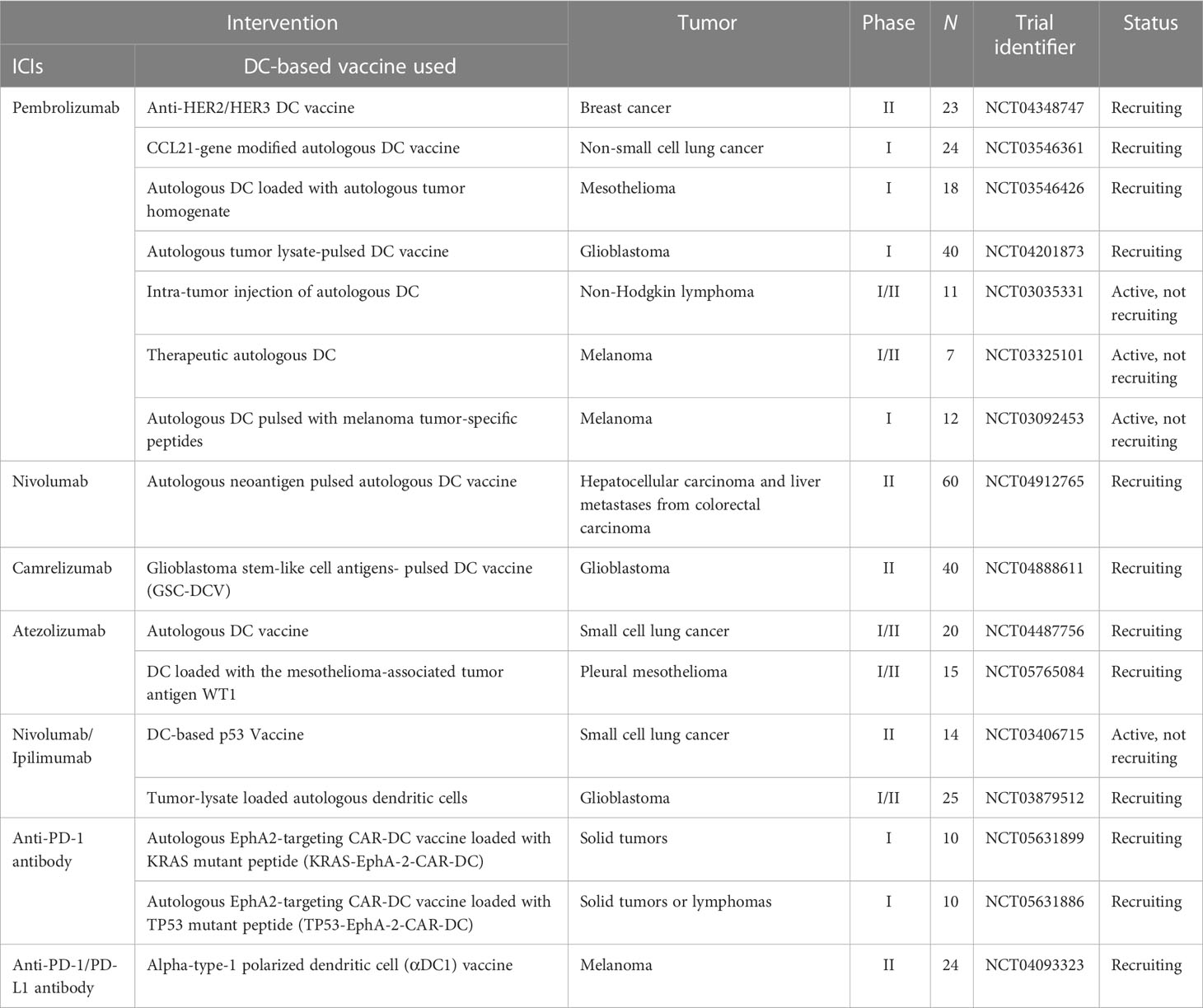
Table 1 Active clinical trials combining DC-based vaccine with immune checkpoint inhibitors (ICIs) therapy (clinicaltrials.gov, April 28, 2023).
Combining a personalized DC-based vaccine with chemotherapeutic agents and targeted drugs is also an effective way to improve the efficacy of tumor vaccines, and we have compiled active relevant clinical trials in Tables 2, 3. It is already clear that chemotherapy can enhance the efficacy of DC-based vaccines by enhancing antigen production and eliminating suppressive immune cells. Some specific chemotherapeutic drugs, such as cyclophosphamide (170), have been shown to directly deplete suppressive immune cells in patients with cancer at low doses. A phase I clinical study suggested that cyclophosphamide with a DC-based vaccine treatment downregulated tumor infiltration of immunosuppressed cells and demonstrated excellent anticancer effects (NCT01241682) (171). In glioblastoma, a combination of Temozolomide- and DC-based vaccines has been favored, and recently, the publication of the results of a phase III prospective externally controlled cohort trial has gained widespread attention (NCT00045968). The results show that the median overall survival for patients with newly diagnosed glioblastoma assigned to the DCVax-L cohort (232 patients, 222 of whom received autologous tumor lysate-loaded dendritic cell vaccine “DCVax-L” plus temozolomide) at enrollment was 19.3 months from the time of randomization compared with 16.5 months from randomization for the 1366-patient external control populations. In addition, in patients with recurrent glioblastoma, the combination of DCVax-L with standard treatment showed a survival benefit (172). Currently, chemotherapy remains the primary treatment for most cancers, and the combination of chemotherapy and DC-based vaccines has promising prospects owing to their cooperative effect. Furthermore, the combination of DC-based vaccines and targeted drugs has been explored. In a phase II clinical trial, Storkus et al. proposed that DC-based vaccines targeting tumor blood vessel antigens combined with dasatinib could induce therapeutic immune responses in patients with checkpoint-refractory advanced melanoma (NCT01876212) (173). Trastuzumab can enhance the uptake and cross-presentation of HER-2 derived peptides by DCs to improve the generation of peptide-specific CTLs (174), which provides a theoretical reference for the combination of Trastuzumab with a DC-based vaccine.
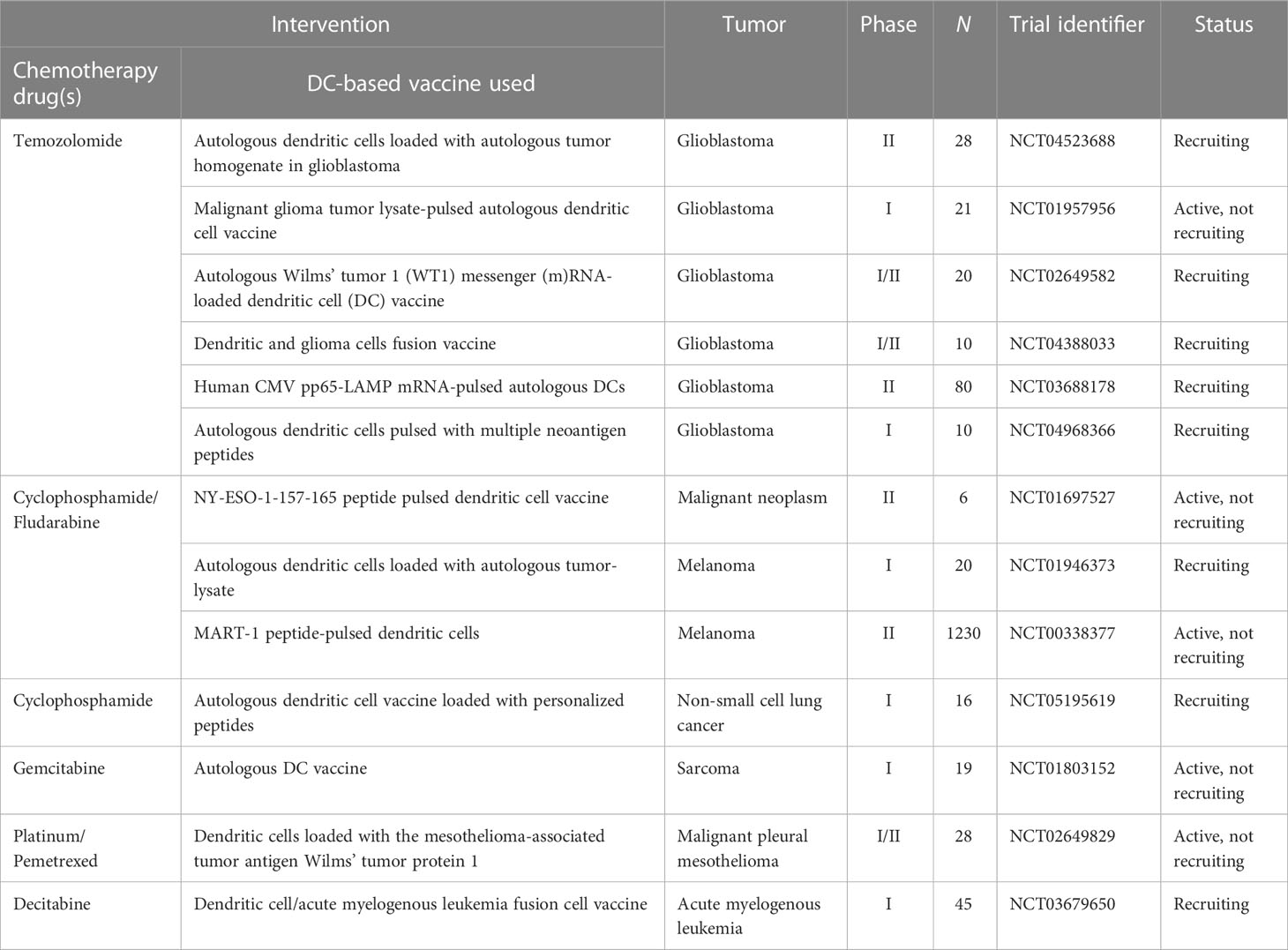
Table 2 Active clinical trials combining DC-based vaccine with chemotherapy drugs (clinicaltrials.gov, May 28, 2023).

Table 3 Active clinical trials combining DC-based vaccine with targeted drugs (clinicaltrials.gov, May 28, 2023).
5 Conclusion
DCs play an indispensable role in triggering anti-tumor immune responses. However, under tumor conditions, immunosuppressive TME weakens their function. The defective function of DCs is an important reason why tumors evade immune surveillance and is closely associated with the poor efficacy of some immunotherapies, such as immune checkpoint inhibitors. Based on the pivotal role of DCs in the immune response, which determines their importance in anti-tumor therapy, many studies have been undertaken to improve the function of DCs, and some protocols, such as DC-based vaccines, have become available options for the treatment of tumors. In addition, the use of DC-based vaccines in combination with ICIs has good application prospects because they can induce a more effective baseline immune response, which is necessary for ICIs to exert their anticancer effects. However, several issues remain unaddressed. The complex composition of the TME and the close and diverse interactions among its components ultimately result in the inhibition of the normal function of multiple immunostimulatory cells, including DCs, and the induction of immune escape. How to effectively and selectively target the immunosuppressive effects of the TME on DCs needs to be further explored.
Author contributions
ZZ designed the study. ZX and RW wrote the manuscript and generated the figures. XW and HY revised and reviewed the manuscript. JD, XH, YY, JG, and JC contributed to the conceptualization and critically edited the manuscript. All authors contributed to the article and approved the submitted version.
Funding
This work was supported by the Guangdong Provincial Basic and Applied Basic Research Fund Project Provincial Enterprise Joint Fund General Project (2022A1515220220), the Research Start Project of Zhuhai People’s Hospital (No.2020ycqd001), The Xiangshan Talented Scientific Research Foundation of Zhuhai People’s Hospital (2021XSYC-02 and 2021XSYC-06) and the Medical Scientific Research Foundation of Guangdong Province(B2022206).
Conflict of interest
The authors declare that the research was conducted in the absence of any commercial or financial relationships that could be construed as a potential conflict of interest.
Publisher’s note
All claims expressed in this article are solely those of the authors and do not necessarily represent those of their affiliated organizations, or those of the publisher, the editors and the reviewers. Any product that may be evaluated in this article, or claim that may be made by its manufacturer, is not guaranteed or endorsed by the publisher.
References
1. Steinman RM, Cohn ZA. Identification of a novel cell type in peripheral lymphoid organs of mice. i. morphology, quantitation, tissue distribution. J Exp Med (1973) 137(5):1142–62. doi: 10.1084/jem.137.5.1142
2. Guilliams M, Ginhoux F, Jakubzick C, Naik SH, Onai N, Schraml BU, et al. Dendritic cells, monocytes and macrophages: a unified nomenclature based on ontogeny. Nat Rev Immunol (2014) 14(8):571–8. doi: 10.1038/nri3712
3. Wculek SK, Cueto FJ, Mujal AM, Melero I, Krummel MF, Sancho D. Dendritic cells in cancer immunology and immunotherapy. Nat Rev Immunol (2020) 20(1):7–24. doi: 10.1038/s41577-019-0210-z
4. Kvedaraite E, Ginhoux F. Human dendritic cells in cancer. Sci Immunol (2022) 7(70):eabm9409. doi: 10.1126/sciimmunol.abm9409
5. Anderson NM, Simon MC. The tumor microenvironment. Curr Biol CB. (2020) 30(16):R921–r5. doi: 10.1016/j.cub.2020.06.081
6. Boedtkjer E, Pedersen SF. The acidic tumor microenvironment as a driver of cancer. Annu Rev Physiol (2020) 82:103–26. doi: 10.1146/annurev-physiol-021119-034627
7. Huber V, Camisaschi C, Berzi A, Ferro S, Lugini L, Triulzi T, et al. Cancer acidity: an ultimate frontier of tumor immune escape and a novel target of immunomodulation. Semin Cancer Biol (2017) 43:74–89. doi: 10.1016/j.semcancer.2017.03.001
8. Nakamura K, Smyth MJ. Myeloid immunosuppression and immune checkpoints in the tumor microenvironment. Cell Mol Immunol (2020) 17(1):1–12. doi: 10.1038/s41423-019-0306-1
9. Pitt JM, Marabelle A, Eggermont A, Soria JC, Kroemer G, Zitvogel L. Targeting the tumor microenvironment: removing obstruction to anticancer immune responses and immunotherapy. Ann Oncol Off J Eur Soc Med Oncol (2016) 27(8):1482–92. doi: 10.1093/annonc/mdw168
10. Chen DS, Mellman I. Oncology meets immunology: the cancer-immunity cycle. Immunity (2013) 39(1):1–10. doi: 10.1016/j.immuni.2013.07.012
11. Joffre OP, Segura E, Savina A, Amigorena S. Cross-presentation by dendritic cells. Nat Rev Immunol (2012) 12(8):557–69. doi: 10.1038/nri3254
12. Guermonprez P, Valladeau J, Zitvogel L, Théry C, Amigorena S. Antigen presentation and T cell stimulation by dendritic cells. Annu Rev Immunol (2002) 20:621–67. doi: 10.1146/annurev.immunol.20.100301.064828
13. Sánchez-Paulete AR, Teijeira A, Cueto FJ, Garasa S, Pérez-Gracia JL, Sánchez-Arráez A, et al. Antigen cross-presentation and T-cell cross-priming in cancer immunology and immunotherapy. Ann Oncol Off J Eur Soc Med Oncol (2017) 28(suppl_12):xii44–55. doi: 10.1093/annonc/mdx237
14. Dunn GP, Old LJ, Schreiber RD. The three Es of cancer immunoediting. Annu Rev Immunol (2004) 22:329–60. doi: 10.1146/annurev.immunol.22.012703.104803
15. Worbs T, Hammerschmidt SI, Förster R. Dendritic cell migration in health and disease. Nat Rev Immunol (2017) 17(1):30–48. doi: 10.1038/nri.2016.116
16. Veglia F, Gabrilovich DI. Dendritic cells in cancer: the role revisited. Curr Opin Immunol (2017) 45:43–51. doi: 10.1016/j.coi.2017.01.002
17. Borst J, Ahrends T, Bąbała N, Melief CJM, Kastenmüller W. CD4(+) T cell help in cancer immunology and immunotherapy. Nat Rev Immunol (2018) 18(10):635–47. doi: 10.1038/s41577-018-0044-0
18. Schumacher TN, Thommen DS. Tertiary lymphoid structures in cancer. Sci (New York NY) (2022) 375(6576):eabf9419. doi: 10.1126/science.abf9419
19. Sautès-Fridman C, Petitprez F, Calderaro J, Fridman WH. Tertiary lymphoid structures in the era of cancer immunotherapy. Nat Rev Cancer. (2019) 19(6):307–25. doi: 10.1038/s41568-019-0144-6
20. Goc J, Germain C, Vo-Bourgais TK, Lupo A, Klein C, Knockaert S, et al. Dendritic cells in tumor-associated tertiary lymphoid structures signal a Th1 cytotoxic immune contexture and license the positive prognostic value of infiltrating CD8+ T cells. Cancer Res (2014) 74(3):705–15. doi: 10.1158/0008-5472.Can-13-1342
21. Leader AM, Grout JA, Maier BB, Nabet BY, Park MD, Tabachnikova A, et al. Single-cell analysis of human non-small cell lung cancer lesions refines tumor classification and patient stratification. Cancer Cell (2021) 39(12):1594–609.e12. doi: 10.1016/j.ccell.2021.10.009
22. Maier B, Leader AM, Chen ST, Tung N, Chang C, LeBerichel J, et al. A conserved dendritic-cell regulatory program limits antitumour immunity. Nature (2020) 580(7802):257–62. doi: 10.1038/s41586-020-2134-y
23. Zhang Q, He Y, Luo N, Patel SJ, Han Y, Gao R, et al. Landscape and dynamics of single immune cells in hepatocellular carcinoma. Cell (2019) 179(4):829–45.e20. doi: 10.1016/j.cell.2019.10.003
24. Bassez A, Vos H, Van Dyck L, Floris G, Arijs I, Desmedt C, et al. A single-cell map of intratumoral changes during anti-PD1 treatment of patients with breast cancer. Nat Med (2021) 27(5):820–32. doi: 10.1038/s41591-021-01323-8
25. Zhang L, Li Z, Skrzypczynska KM, Fang Q, Zhang W, O'Brien SA, et al. Single-cell analyses inform mechanisms of myeloid-targeted therapies in colon cancer. Cell (2020) 181(2):442–59.e29. doi: 10.1016/j.cell.2020.03.048
26. Li Y, Hu X, Lin R, Zhou G, Zhao L, Zhao D, et al. Single-cell landscape reveals active cell subtypes and their interaction in the tumor microenvironment of gastric cancer. Theranostics (2022) 12(8):3818–33. doi: 10.7150/thno.71833
27. Li J, Zhou J, Huang H, Jiang J, Zhang T, Ni C. Mature dendritic cells enriched in immunoregulatory molecules (mregDCs): a novel population in the tumour microenvironment and immunotherapy target. Clin Trans Med (2023) 13(2):e1199. doi: 10.1002/ctm2.1199
28. Ginhoux F, Guilliams M, Merad M. Expanding dendritic cell nomenclature in the single-cell era. Nat Rev Immunol (2022) 22(2):67–8. doi: 10.1038/s41577-022-00675-7
29. Minohara K, Imai M, Matoba T, Wing JB, Shime H, Odanaka M, et al. Mature dendritic cells enriched in regulatory molecules may control regulatory T cells and the prognosis of head and neck cancer. Cancer Sci (2023) 114(4):1256–69. doi: 10.1111/cas.15698
30. Barry KC, Hsu J, Broz ML, Cueto FJ, Binnewies M, Combes AJ, et al. A natural killer-dendritic cell axis defines checkpoint therapy-responsive tumor microenvironments. Nat Med (2018) 24(8):1178–91. doi: 10.1038/s41591-018-0085-8
31. Böttcher JP, Bonavita E, Chakravarty P, Blees H, Cabeza-Cabrerizo M, Sammicheli S, et al. NK cells stimulate recruitment of cDC1 into the tumor microenvironment promoting cancer immune control. Cell (2018) 172(5):1022–37.e14. doi: 10.1016/j.cell.2018.01.004
32. Broz ML, Binnewies M, Boldajipour B, Nelson AE, Pollack JL, Erle DJ, et al. Dissecting the tumor myeloid compartment reveals rare activating antigen-presenting cells critical for T cell immunity. Cancer Cell (2014) 26(5):638–52. doi: 10.1016/j.ccell.2014.09.007
33. Hegde S, Krisnawan VE, Herzog BH, Zuo C, Breden MA, Knolhoff BL, et al. Dendritic cell paucity leads to dysfunctional immune surveillance in pancreatic cancer. Cancer Cell (2020) 37(3):289–307.e9. doi: 10.1016/j.ccell.2020.02.008
34. Tussiwand R, Lee WL, Murphy TL, Mashayekhi M, Kc W, Albring JC, et al. Compensatory dendritic cell development mediated by BATF-IRF interactions. Nature (2012) 490(7421):502–7. doi: 10.1038/nature11531
35. Sánchez-Paulete AR, Cueto FJ, Martínez-López M, Labiano S, Morales-Kastresana A, Rodríguez-Ruiz ME, et al. Cancer immunotherapy with immunomodulatory anti-CD137 and anti-PD-1 monoclonal antibodies requires BATF3-dependent dendritic cells. Cancer discovery. (2016) 6(1):71–9. doi: 10.1158/2159-8290.Cd-15-0510
36. Spranger S, Dai D, Horton B, Gajewski TF. Tumor-residing Batf3 dendritic cells are required for effector T cell trafficking and adoptive T cell therapy. Cancer Cell (2017) 31(5):711–23.e4. doi: 10.1016/j.ccell.2017.04.003
37. Mittal D, Vijayan D, Putz EM, Aguilera AR, Markey KA, Straube J, et al. Interleukin-12 from CD103(+) Batf3-dependent dendritic cells required for NK-cell suppression of metastasis. Cancer Immunol Res (2017) 5(12):1098–108. doi: 10.1158/2326-6066.Cir-17-0341
38. Salmon H, Idoyaga J, Rahman A, Leboeuf M, Remark R, Jordan S, et al. Expansion and activation of CD103(+) dendritic cell progenitors at the tumor site enhances tumor responses to therapeutic PD-L1 and BRAF inhibition. Immunity (2016) 44(4):924–38. doi: 10.1016/j.immuni.2016.03.012
39. Zitvogel L, Galluzzi L, Kepp O, Smyth MJ, Kroemer G. Type I interferons in anticancer immunity. Nat Rev Immunol (2015) 15(7):405–14. doi: 10.1038/nri3845
40. Fu C, Peng P, Loschko J, Feng L, Pham P, Cui W, et al. Plasmacytoid dendritic cells cross-prime naive CD8 T cells by transferring antigen to conventional dendritic cells through exosomes. Proc Natl Acad Sci United States America. (2020) 117(38):23730–41. doi: 10.1073/pnas.2002345117
41. Kießler M, Plesca I, Sommer U, Wehner R, Wilczkowski F, Müller L, et al. Tumor-infiltrating plasmacytoid dendritic cells are associated with survival in human colon cancer. J immunother Cancer (2021) 9(3):e001813. doi: 10.1136/jitc-2020-001813
42. Poropatich K, Dominguez D, Chan WC, Andrade J, Zha Y, Wray B, et al. OX40+ plasmacytoid dendritic cells in the tumor microenvironment promote antitumor immunity. J Clin Invest (2020) 130(7):3528–42. doi: 10.1172/jci131992
43. Labidi-Galy SI, Sisirak V, Meeus P, Gobert M, Treilleux I, Bajard A, et al. Quantitative and functional alterations of plasmacytoid dendritic cells contribute to immune tolerance in ovarian cancer. Cancer Res (2011) 71(16):5423–34. doi: 10.1158/0008-5472.Can-11-0367
44. Sisirak V, Faget J, Gobert M, Goutagny N, Vey N, Treilleux I, et al. Impaired IFN-α production by plasmacytoid dendritic cells favors regulatory T-cell expansion that may contribute to breast cancer progression. Cancer Res (2012) 72(20):5188–97. doi: 10.1158/0008-5472.Can-11-3468
45. Sisirak V, Vey N, Goutagny N, Renaudineau S, Malfroy M, Thys S, et al. Breast cancer-derived transforming growth factor-β and tumor necrosis factor-α compromise interferon-α production by tumor-associated plasmacytoid dendritic cells. Int J cancer. (2013) 133(3):771–8. doi: 10.1002/ijc.28072
46. Terra M, Oberkampf M, Fayolle C, Rosenbaum P, Guillerey C, Dadaglio G, et al. Tumor-derived TGFβ alters the ability of plasmacytoid dendritic cells to respond to innate immune signaling. Cancer Res (2018) 78(11):3014–26. doi: 10.1158/0008-5472.Can-17-2719
47. Meyer MA, Baer JM, Knolhoff BL, Nywening TM, Panni RZ, Su X, et al. Breast and pancreatic cancer interrupt IRF8-dependent dendritic cell development to overcome immune surveillance. Nat Commun (2018) 9(1):1250. doi: 10.1038/s41467-018-03600-6
48. Zhu S, Yang N, Wu J, Wang X, Wang W, Liu YJ, et al. Tumor microenvironment-related dendritic cell deficiency: a target to enhance tumor immunotherapy. Pharmacol Res (2020) 159:104980. doi: 10.1016/j.phrs.2020.104980
49. Laoui D, Keirsse J, Morias Y, Van Overmeire E, Geeraerts X, Elkrim Y, et al. The tumour microenvironment harbours ontogenically distinct dendritic cell populations with opposing effects on tumour immunity. Nat Commun (2016) 7:13720. doi: 10.1038/ncomms13720
50. Verneau J, Sautés-Fridman C, Sun CM. Dendritic cells in the tumor microenvironment: prognostic and theranostic impact. Semin Immunol (2020) 48:101410. doi: 10.1016/j.smim.2020.101410
51. Gajewski TF, Schreiber H, Fu YX. Innate and adaptive immune cells in the tumor microenvironment. Nat Immunol (2013) 14(10):1014–22. doi: 10.1038/ni.2703
52. Tie Y, Tang F, Wei YQ, Wei XW. Immunosuppressive cells in cancer: mechanisms and potential therapeutic targets. J Hematol Oncol (2022) 15(1):61. doi: 10.1186/s13045-022-01282-8
53. Bule P, Aguiar SI, Aires-Da-Silva F, Dias JNR. Chemokine-directed tumor microenvironment modulation in cancer immunotherapy. Int J Mol Sci (2021) 22(18):9804. doi: 10.3390/ijms22189804
54. Mizukami Y, Kono K, Kawaguchi Y, Akaike H, Kamimura K, Sugai H, et al. CCL17 and CCL22 chemokines within tumor microenvironment are related to accumulation of Foxp3+ regulatory T cells in gastric cancer. Int J cancer. (2008) 122(10):2286–93. doi: 10.1002/ijc.23392
55. Wang D, Yang L, Yu W, Wu Q, Lian J, Li F, et al. Colorectal cancer cell-derived CCL20 recruits regulatory T cells to promote chemoresistance via FOXO1/CEBPB/NF-κB signaling. J immunother cancer. (2019) 7(1):215. doi: 10.1186/s40425-019-0701-2
56. Facciabene A, Peng X, Hagemann IS, Balint K, Barchetti A, Wang LP, et al. Tumour hypoxia promotes tolerance and angiogenesis via CCL28 and t(reg) cells. Nature (2011) 475(7355):226–30. doi: 10.1038/nature10169
57. Ren L, Yu Y, Wang L, Zhu Z, Lu R, Yao Z. Hypoxia-induced CCL28 promotes recruitment of regulatory T cells and tumor growth in liver cancer. Oncotarget (2016) 7(46):75763–73. doi: 10.18632/oncotarget.12409
58. Watson MJ, Vignali PDA, Mullett SJ, Overacre-Delgoffe AE, Peralta RM, Grebinoski S, et al. Metabolic support of tumour-infiltrating regulatory T cells by lactic acid. Nature (2021) 591(7851):645–51. doi: 10.1038/s41586-020-03045-2
59. Angelin A, Gil-de-Gómez L, Dahiya S, Jiao J, Guo L, Levine MH, et al. Foxp3 reprograms T cell metabolism to function in low-glucose, high-lactate environments. Cell Metab (2017) 25(6):1282–93.e7. doi: 10.1016/j.cmet.2016.12.018
60. Togashi Y, Shitara K, Nishikawa H. Regulatory T cells in cancer immunosuppression - implications for anticancer therapy. Nat Rev Clin Oncol (2019) 16(6):356–71. doi: 10.1038/s41571-019-0175-7
61. Chen L, Flies DB. Molecular mechanisms of T cell co-stimulation and co-inhibition. Nat Rev Immunol (2013) 13(4):227–42. doi: 10.1038/nri3405
62. Qureshi OS, Zheng Y, Nakamura K, Attridge K, Manzotti C, Schmidt EM, et al. Trans-endocytosis of CD80 and CD86: a molecular basis for the cell-extrinsic function of CTLA-4. Sci (New York NY). (2011) 332(6029):600–3. doi: 10.1126/science.1202947
63. Hou TZ, Qureshi OS, Wang CJ, Baker J, Young SP, Walker LS, et al. A transendocytosis model of CTLA-4 function predicts its suppressive behavior on regulatory T cells. J Immunol (Baltimore Md 1950). (2015) 194(5):2148–59. doi: 10.4049/jimmunol.1401876
64. Ovcinnikovs V, Ross EM, Petersone L, Edner NM, Heuts F, Ntavli E, et al. CTLA-4-mediated transendocytosis of costimulatory molecules primarily targets migratory dendritic cells. Sci Immunol (2019) 4(35):eaaw0902. doi: 10.1126/sciimmunol.aaw0902
65. Gu P, Gao JF, D'Souza CA, Kowalczyk A, Chou KY, Zhang L. Trogocytosis of CD80 and CD86 by induced regulatory T cells. Cell Mol Immunol (2012) 9(2):136–46. doi: 10.1038/cmi.2011.62
66. Tekguc M, Wing JB, Osaki M, Long J, Sakaguchi S. Treg-expressed CTLA-4 depletes CD80/CD86 by trogocytosis, releasing free PD-L1 on antigen-presenting cells. Proc Natl Acad Sci United States America. (2021) 118(30):e2023739118. doi: 10.1073/pnas.2023739118
67. Liu Y, Xu P, Liu H, Fang C, Guo H, Chen X, et al. Silencing IDO2 in dendritic cells: a novel strategy to strengthen cancer immunotherapy in a murine lung cancer model. Int J Oncol (2020) 57(2):587–97. doi: 10.3892/ijo.2020.5073
68. Shevach EM. Mechanisms of foxp3+ T regulatory cell-mediated suppression. Immunity (2009) 30(5):636–45. doi: 10.1016/j.immuni.2009.04.010
69. Grohmann U, Orabona C, Fallarino F, Vacca C, Calcinaro F, Falorni A, et al. CTLA-4-Ig regulates tryptophan catabolism in vivo. Nat Immunol (2002) 3(11):1097–101. doi: 10.1038/ni846
70. Huang CT, Workman CJ, Flies D, Pan X, Marson AL, Zhou G, et al. Role of LAG-3 in regulatory T cells. Immunity (2004) 21(4):503–13. doi: 10.1016/j.immuni.2004.08.010
71. Liang B, Workman C, Lee J, Chew C, Dale BM, Colonna L, et al. Regulatory T cells inhibit dendritic cells by lymphocyte activation gene-3 engagement of MHC class II. J Immunol (Baltimore Md 1950). (2008) 180(9):5916–26. doi: 10.4049/jimmunol.180.9.5916
72. Li C, Jiang P, Wei S, Xu X, Wang J. Regulatory T cells in tumor microenvironment: new mechanisms, potential therapeutic strategies and future prospects. Mol Cancer. (2020) 19(1):116. doi: 10.1186/s12943-020-01234-1
73. Tung SL, Boardman DA, Sen M, Letizia M, Peng Q, Cianci N, et al. Regulatory T cell-derived extracellular vesicles modify dendritic cell function. Sci Rep (2018) 8(1):6065. doi: 10.1038/s41598-018-24531-8
74. Muth S, Klaric A, Radsak M, Schild H, Probst HC. CD27 expression on treg cells limits immune responses against tumors. J Mol Med (Berlin Germany). (2022) 100(3):439–49. doi: 10.1007/s00109-021-02116-9
75. Boissonnas A, Scholer-Dahirel A, Simon-Blancal V, Pace L, Valet F, Kissenpfennig A, et al. Foxp3+ T cells induce perforin-dependent dendritic cell death in tumor-draining lymph nodes. Immunity (2010) 32(2):266–78. doi: 10.1016/j.immuni.2009.11.015
76. Galvin KC, Dyck L, Marshall NA, Stefanska AM, Walsh KP, Moran B, et al. Blocking retinoic acid receptor-α enhances the efficacy of a dendritic cell vaccine against tumours by suppressing the induction of regulatory T cells. Cancer immunology immunother CII. (2013) 62(7):1273–82. doi: 10.1007/s00262-013-1432-8
77. Rossowska J, Anger N, Szczygieł A, Mierzejewska J, Pajtasz-Piasecka E. Intratumoral lentivector-mediated TGF-β1 gene downregulation as a potent strategy for enhancing the antitumor effect of therapy composed of cyclophosphamide and dendritic cells. Front Immunol (2017) 8:713. doi: 10.3389/fimmu.2017.00713
78. Conroy H, Galvin KC, Higgins SC, Mills KH. Gene silencing of TGF-β1 enhances antitumor immunity induced with a dendritic cell vaccine by reducing tumor-associated regulatory T cells. Cancer immunology immunother CII. (2012) 61(3):425–31. doi: 10.1007/s00262-011-1188-y
79. Groth C, Hu X, Weber R, Fleming V, Altevogt P, Utikal J, et al. Immunosuppression mediated by myeloid-derived suppressor cells (MDSCs) during tumour progression. Br J cancer. (2019) 120(1):16–25. doi: 10.1038/s41416-018-0333-1
80. Yaseen MM, Abuharfeil NM, Darmani H, Daoud A. Mechanisms of immune suppression by myeloid-derived suppressor cells: the role of interleukin-10 as a key immunoregulatory cytokine. Open Biol (2020) 10(9):200111. doi: 10.1098/rsob.200111
81. Hu CE, Gan J, Zhang RD, Cheng YR, Huang GJ. Up-regulated myeloid-derived suppressor cell contributes to hepatocellular carcinoma development by impairing dendritic cell function. Scandinavian J gastroenterology. (2011) 46(2):156–64. doi: 10.3109/00365521.2010.516450
82. Ramakrishnan R, Tyurin VA, Veglia F, Condamine T, Amoscato A, Mohammadyani D, et al. Oxidized lipids block antigen cross-presentation by dendritic cells in cancer. J Immunol (Baltimore Md 1950). (2014) 192(6):2920–31. doi: 10.4049/jimmunol.1302801
83. Herber DL, Cao W, Nefedova Y, Novitskiy SV, Nagaraj S, Tyurin VA, et al. Lipid accumulation and dendritic cell dysfunction in cancer. Nat Med (2010) 16(8):880–6. doi: 10.1038./nm.2172
84. Veglia F, Tyurin VA, Mohammadyani D, Blasi M, Duperret EK, Donthireddy L, et al. Lipid bodies containing oxidatively truncated lipids block antigen cross-presentation by dendritic cells in cancer. Nat Commun (2017) 8(1):2122. doi: 10.1038/s41467-017-02186-9
85. Ugolini A, Tyurin VA, Tyurina YY, Tcyganov EN, Donthireddy L, Kagan VE, et al. Polymor-phonuclear myeloid-derived suppressor cells limit antigen cross-presentation by dendritic cells in cancer. JCI Insight (2020) 5(15):e138581. doi: 10.1172/jci.insight.138581
86. Chen Y, Song Y, Du W, Gong L, Chang H, Zou Z. Tumor-associated macrophages: an accomplice in solid tumor progression. J Biomed Sci (2019) 26(1):78. doi: 10.1186/s12929-019-0568-z
87. Pathria P, Louis TL, Varner JA. Targeting tumor-associated macrophages in cancer. Trends Immunol (2019) 40(4):310–27. doi: 10.1016/j.it.2019.02.003
88. Mazzoni M, Mauro G, Erreni M, Romeo P, Minna E, Vizioli MG, et al. Senescent thyrocytes and thyroid tumor cells induce M2-like macrophage polarization of human monocytes via a PGE2-dependent mechanism. J Exp Clin Cancer Res (2019) 38(1):208. doi: 10.1186/s13046-019-1198-8
89. Boutilier AJ, Elsawa SF. Macrophage polarization states in the tumor microenvironment. Int J Mol Sci (2021) 22(13):6995. doi: 10.3390/ijms22136995
90. Mantovani A, Sozzani S, Locati M, Allavena P, Sica A. Macrophage polarization: tumor-associated macrophages as a paradigm for polarized M2 mononuclear phagocytes. Trends Immunol (2002) 23(11):549–55. doi: 10.1016/s1471-4906(02)02302-5
91. Pan Y, Yu Y, Wang X, Zhang T. Tumor-associated macrophages in tumor immunity. Front Immunol (2020) 11:583084. doi: 10.3389/fimmu.2020.583084
92. DeNardo DG, Ruffell B. Macrophages as regulators of tumour immunity and immunotherapy. Nat Rev Immunol (2019) 19(6):369–82. doi: 10.1038/s41577-019-0127-6
93. Hinshaw DC, Shevde LA. The tumor microenvironment innately modulates cancer progression. Cancer Res (2019) 79(18):4557–66. doi: 10.1158/0008-5472.Can-18-3962
94. Beury DW, Parker KH, Nyandjo M, Sinha P, Carter KA, Ostrand-Rosenberg S. Cross-talk among myeloid-derived suppressor cells, macrophages, and tumor cells impacts the inflammatory milieu of solid tumors. J Leukoc Biol (2014) 96(6):1109–18. doi: 10.1189/jlb.3A0414-210R
95. Ruffell B, Chang-Strachan D, Chan V, Rosenbusch A, Ho CM, Pryer N, et al. Macrophage IL-10 blocks CD8+ T cell-dependent responses to chemotherapy by suppressing IL-12 expression in intratumoral dendritic cells. Cancer Cell (2014) 26(5):623–37. doi: 10.1016/j.ccell.2014.09.006
96. Pfirschke C, Zilionis R, Engblom C, Messemaker M, Zou AE, Rickelt S, et al. Macrophage-targeted therapy unlocks antitumoral cross-talk between IFNγ-secreting lymphocytes and IL12-producing dendritic cells. Cancer Immunol Res (2022) 10(1):40–55. doi: 10.1158/2326-6066.Cir-21-0326
97. Dammeijer F, Lievense LA, Kaijen-Lambers ME, van Nimwegen M, Bezemer K, Hegmans JP, et al. Depletion of tumor-associated macrophages with a CSF-1R kinase inhibitor enhances antitumor immunity and survival induced by DC immunotherapy. Cancer Immunol Res (2017) 5(7):535–46. doi: 10.1158/2326-6066.Cir-16-0309
98. Turley SJ, Cremasco V, Astarita JL. Immunological hallmarks of stromal cells in the tumour microenvironment. Nat Rev Immunol (2015) 15(11):669–82. doi: 10.1038/nri3902
99. Arina A, Idel C, Hyjek EM, Alegre ML, Wang Y, Bindokas VP, et al. Tumor-associated fibroblasts predominantly come from local and not circulating precursors. Proc Natl Acad Sci United States America. (2016) 113(27):7551–6. doi: 10.1073/pnas.1600363113
100. Park D, Sahai E, Rullan A. SnapShot: cancer-associated fibroblasts. Cell (2020) 181(2):486–.e1. doi: 10.1016/j.cell.2020.03.013
101. Hawinkels LJ, Paauwe M, Verspaget HW, Wiercinska E, van der Zon JM, van der Ploeg K, et al. Interaction with colon cancer cells hyperactivates TGF-β signaling in cancer-associated fibroblasts. Oncogene (2014) 33(1):97–107. doi: 10.1038/onc.2012.536
102. Chen X, Song E. Turning foes to friends: targeting cancer-associated fibroblasts. Nat Rev Drug discovery. (2019) 18(2):99–115. doi: 10.1038/s41573-018-0004-1
103. Mao X, Xu J, Wang W, Liang C, Hua J, Liu J, et al. Crosstalk between cancer-associated fibroblasts and immune cells in the tumor microenvironment: new findings and future perspectives. Mol Cancer. (2021) 20(1):131. doi: 10.1186/s12943-021-01428-1
104. Berzaghi R, Tornaas S, Lode K, Hellevik T, Martinez-Zubiaurre I. Ionizing radiation curtails immunosuppressive effects from cancer-associated fibroblasts on dendritic cells. Front Immunol (2021) 12:662594. doi: 10.3389/fimmu.2021.662594
105. Hsu YL, Hung JY, Chiang SY, Jian SF, Wu CY, Lin YS, et al. Lung cancer-derived galectin-1 contributes to cancer associated fibroblast-mediated cancer progression and immune suppression through TDO2/kynurenine axis. Oncotarget (2016) 7(19):27584–98. doi: 10.18632/oncotarget.8488
106. Cheng JT, Deng YN, Yi HM, Wang GY, Fu BS, Chen WJ, et al. Hepatic carcinoma-associated fibroblasts induce IDO-producing regulatory dendritic cells through IL-6-mediated STAT3 activation. Oncogenesis (2016) 5(2):e198. doi: 10.1038/oncsis.2016.7
107. Long J, Hu Z, Xue H, Wang Y, Chen J, Tang F, et al. Vascular endothelial growth factor (VEGF) impairs the motility and immune function of human mature dendritic cells through the VEGF receptor 2-RhoA-cofilin1 pathway. Cancer Sci (2019) 110(8):2357–67. doi: 10.1111/cas.14091
108. Kugeratski FG, Atkinson SJ, Neilson LJ, Lilla S, Knight JRP, Serneels J, et al. Hypoxic cancer-associated fibroblasts increase NCBP2-AS2/HIAR to promote endothelial sprouting through enhanced VEGF signaling. Sci Signaling (2019) 12(567):eaan8247. doi: 10.1126/scisignal.aan8247
109. Huang TX, Tan XY, Huang HS, Li YT, Liu BL, Liu KS, et al. Targeting cancer-associated fibroblast-secreted WNT2 restores dendritic cell-mediated antitumour immunity. Gut (2022) 71(2):333–44. doi: 10.1136/gutjnl-2020-322924
110. Jing X, Yang F, Shao C, Wei K, Xie M, Shen H, et al. Role of hypoxia in cancer therapy by regulating the tumor microenvironment. Mol Cancer. (2019) 18(1):157. doi: 10.1186/s12943-019-1089-9
111. Ricciardi A, Elia AR, Cappello P, Puppo M, Vanni C, Fardin P, et al. Transcriptome of hypoxic immature dendritic cells: modulation of chemokine/receptor expression. Mol Cancer Res MCR. (2008) 6(2):175–85. doi: 10.1158/1541-7786.Mcr-07-0391
112. Elia AR, Cappello P, Puppo M, Fraone T, Vanni C, Eva A, et al. Human dendritic cells differentiated in hypoxia down-modulate antigen uptake and change their chemokine expression profile. J Leukoc Biol (2008) 84(6):1472–82. doi: 10.1189/jlb.0208082
113. Yang M, Ma C, Liu S, Sun J, Shao Q, Gao W, et al. Hypoxia skews dendritic cells to a T helper type 2-stimulating phenotype and promotes tumour cell migration by dendritic cell-derived osteopontin. Immunology (2009) 128(1 Suppl):e237–49. doi: 10.1111/j.1365-2567.2008.02954.x
114. Suthen S, Lim CJ, Nguyen PHD, Dutertre CA, Lai HLH, Wasser M, et al. Hypoxia-driven immunosuppression by treg and type-2 conventional dendritic cells in HCC. Hepatol (Baltimore Md). (2022) 76(5):1329–44. doi: 10.1002/hep.32419
115. McGettrick AF, O'Neill LAJ. The role of HIF in immunity and inflammation. Cell Metab (2020) 32(4):524–36. doi: 10.1016/j.cmet.2020.08.002
116. Kierans SJ, Taylor CT. Regulation of glycolysis by the hypoxia-inducible factor (HIF): implications for cellular physiology. J Physiol (2021) 599(1):23–37. doi: 10.1113/jp280572
117. Liu J, Zhang X, Chen K, Cheng Y, Liu S, Xia M, et al. CCR7 chemokine receptor-inducible lnc-Dpf3 restrains dendritic cell migration by inhibiting HIF-1α-Mediated glycolysis. Immunity (2019) 50(3):600–15.e15. doi: 10.1016/j.immuni.2019.01.021
118. Guak H, Al Habyan S, Ma EH, Aldossary H, Al-Masri M, Won SY, et al. Glycolytic metabolism is essential for CCR7 oligomerization and dendritic cell migration. Nat Commun (2018) 9(1):2463. doi: 10.1038/s41467-018-04804-6
119. Filippi I, Morena E, Aldinucci C, Carraro F, Sozzani S, Naldini A. Short-term hypoxia enhances the migratory capability of dendritic cell through HIF-1α and PI3K/Akt pathway. J Cell Physiol (2014) 229(12):2067–76. doi: 10.1002/jcp.24666
120. Han Z, Dong Y, Lu J, Yang F, Zheng Y, Yang H. Role of hypoxia in inhibiting dendritic cells by VEGF signaling in tumor microenvironments: mechanism and application. Am J Cancer Res (2021) 11(8):3777–93. Available at: https://pubmed.ncbi.nlm.nih.gov/34522449/.
121. Tran CW, Gold MJ, Garcia-Batres C, Tai K, Elford AR, Himmel ME, et al. Hypoxia-inducible factor 1 alpha limits dendritic cell stimulation of CD8 T cell immunity. PloS One (2020) 15(12):e0244366. doi: 10.1371/journal.pone.0244366
122. Naldini A, Morena E, Pucci A, Miglietta D, Riboldi E, Sozzani S, et al. Hypoxia affects dendritic cell survival: role of the hypoxia-inducible factor-1α and lipopolysaccharide. J Cell Physiol (2012) 227(2):587–95. doi: 10.1002/jcp.22761
123. Ding XC, Wang LL, Zhang XD, Xu JL, Li PF, Liang H, et al. The relationship between expression of PD-L1 and HIF-1α in glioma cells under hypoxia. J Hematol Oncol (2021) 14(1):92. doi: 10.1186/s13045-021-01102-5
124. Leblond MM, Gérault AN, Corroyer-Dulmont A, MacKenzie ET, Petit E, Bernaudin M, et al. Hypoxia induces macrophage polarization and re-education toward an M2 phenotype in U87 and U251 glioblastoma models. Oncoimmunology (2016) 5(1):e1056442. doi: 10.1080/2162402x.2015.1056442
125. Vander Heiden MG, Cantley LC, Thompson CB. Understanding the warburg effect: the metabolic requirements of cell proliferation. Sci (New York NY). (2009) 324(5930):1029–33. doi: 10.1126/science.1160809
126. Manoharan I, Prasad PD, Thangaraju M, Manicassamy S. Lactate-dependent regulation of immune responses by dendritic cells and macrophages. Front Immunol (2021) 12:691134. doi: 10.3389/fimmu.2021.691134
127. Hayes C, Donohoe CL, Davern M, Donlon NE. The oncogenic and clinical implications of lactate induced immunosuppression in the tumour microenvironment. Cancer letters. (2021) 500:75–86. doi: 10.1016/j.canlet.2020.12.021
128. Brown TP, Bhattacharjee P, Ramachandran S, Sivaprakasam S, Ristic B, Sikder MOF, et al. The lactate receptor GPR81 promotes breast cancer growth via a paracrine mechanism involving antigen-presenting cells in the tumor microenvironment. Oncogene (2020) 39(16):3292–304. doi: 10.1038/s41388-020-1216-5
129. Gottfried E, Kunz-Schughart LA, Ebner S, Mueller-Klieser W, Hoves S, Andreesen R, et al. Tumor-derived lactic acid modulates dendritic cell activation and antigen expression. Blood (2006) 107(5):2013–21. doi: 10.1182/blood-2005-05-1795
130. Nasi A, Fekete T, Krishnamurthy A, Snowden S, Rajnavölgyi E, Catrina AI, et al. Dendritic cell reprogramming by endogenously produced lactic acid. J Immunol (Baltimore Md 1950). (2013) 191(6):3090–9. doi: 10.4049/jimmunol.1300772
131. Monti M, Vescovi R, Consoli F, Farina D, Moratto D, Berruti A, et al. Plasmacytoid dendritic cell impairment in metastatic melanoma by lactic acidosis. Cancers (2020) 12(8):2085. doi: 10.3390/cancers12082085
132. Raychaudhuri D, Bhattacharya R, Sinha BP, Liu CSC, Ghosh AR, Rahaman O, et al. Lactate induces pro-tumor reprogramming in intratumoral plasmacytoid dendritic cells. Front Immunol (2019) 10:1878. doi: 10.3389/fimmu.2019.01878
133. Tong L, Yue P, Yang Y, Huang J, Zeng Z, Qiu W. Motility and mechanical properties of dendritic cells deteriorated by extracellular acidosis. Inflammation (2021) 44(2):737–45. doi: 10.1007/s10753-020-01373-z
134. Trempolec N, Degavre C, Doix B, Brusa D, Corbet C, Feron O. Acidosis-induced TGF-β2 production promotes lipid droplet formation in dendritic cells and alters their potential to support anti-mesothelioma T cell response. Cancers (2020) 12(5):1284. doi: 10.3390/cancers12051284
135. Vermeulen M, Giordano M, Trevani AS, Sedlik C, Gamberale R, Fernández-Calotti P, et al. Acidosis improves uptake of antigens and MHC class I-restricted presentation by dendritic cells. J Immunol (Baltimore Md 1950). (2004) 172(5):3196–204. doi: 10.4049/jimmunol.172.5.3196
136. Martínez D, Vermeulen M, von Euw E, Sabatté J, Maggíni J, Ceballos A, et al. Extracellular acidosis triggers the maturation of human dendritic cells and the production of IL-12. J Immunol (Baltimore Md 1950). (2007) 179(3):1950–9. doi: 10.4049/jimmunol.179.3.1950
137. Cueto FJ, Sancho D. The Flt3L/Flt3 axis in dendritic cell biology and cancer immunotherapy. Cancers (2021) 13(7):1525. doi: 10.3390/cancers13071525
138. Bhardwaj N, Friedlander PA, Pavlick AC, Ernstoff MS, Gastman BR, Hanks BA, et al. Flt3 ligand augments immune responses to anti-DEC-205-NY-ESO-1 vaccine through expansion of dendritic cell subsets. Nat cancer. (2020) 1(12):1204–17. doi: 10.1038/s43018-020-00143-y
139. van de Laar L, Coffer PJ, Woltman AM. Regulation of dendritic cell development by GM-CSF: molecular control and implications for immune homeostasis and therapy. Blood (2012) 119(15):3383–93. doi: 10.1182/blood-2011-11-370130
140. Oosterling SJ, Mels AK, Geijtenbeek TB, van der Bij GJ, Tuk CW, Vuylsteke RJ, et al. Preoperative granulocyte/macrophage colony-stimulating factor (GM-CSF) increases hepatic dendritic cell numbers and clustering with lymphocytes in colorectal cancer patients. Immunobiology (2006) 211(6-8):641–9. doi: 10.1016/j.imbio.2006.06.010
141. Sluijter BJ, van den Hout MF, Koster BD, van Leeuwen PA, Schneiders FL, van de Ven R, et al. Arming the melanoma sentinel lymph node through local administration of CpG-b and GM-CSF: recruitment and activation of BDCA3/CD141(+) dendritic cells and enhanced cross-presentation. Cancer Immunol Res (2015) 3(5):495–505. doi: 10.1158/2326-6066.Cir-14-0165
142. Duan T, Du Y, Xing C, Wang HY, Wang RF. Toll-like receptor signaling and its role in cell-mediated immunity. Front Immunol (2022) 13:812774. doi: 10.3389/fimmu.2022.812774
143. Mehrotra S, Britten CD, Chin S, Garrett-Mayer E, Cloud CA, Li M, et al. Vaccination with poly(IC:LC) and peptide-pulsed autologous dendritic cells in patients with pancreatic cancer. J Hematol Oncol (2017) 10(1):82. doi: 10.1186/s13045-017-0459-2
144. Anfray C, Mainini F, Digifico E, Maeda A, Sironi M, Erreni M, et al. Intratumoral combination therapy with poly(I:C) and resiquimod synergistically triggers tumor-associated macrophages for effective systemic antitumoral immunity. J immunother Cancer (2021) 9(9):e002408. doi: 10.1136/jitc-2021-002408
145. Shrimpton RE, Butler M, Morel AS, Eren E, Hue SS, Ritter MA. CD205 (DEC-205): a recognition receptor for apoptotic and necrotic self. Mol Immunol (2009) 46(6):1229–39. doi: 10.1016/j.molimm.2008.11.016
146. Dhodapkar MV, Sznol M, Zhao B, Wang D, Carvajal RD, Keohan ML, et al. Induction of antigen-specific immunity with a vaccine targeting NY-ESO-1 to the dendritic cell receptor DEC-205. Sci Trans Med (2014) 6(232):232ra51. doi: 10.1126/scitranslmed.3008068
147. Martinek J, Lin J, Kim KI, Wang VG, Wu TC, Chiorazzi M, et al. Transcriptional profiling of macrophages in situ in metastatic melanoma reveals localization-dependent phenotypes and function. Cell Rep Med (2022) 3(5):100621. doi: 10.1016/j.xcrm.2022.100621
148. van Willigen WW, Bloemendal M, Gerritsen WR, Schreibelt G, de Vries IJM, Bol KF. Dendritic cell cancer therapy: vaccinating the right patient at the right time. Front Immunol (2018) 9:2265. doi: 10.3389/fimmu.2018.02265
149. National Cancer Institute. Drugs approved for prostate cancer . Available at: https://www.cancer.gov/about-cancer/treatment/drugs/sipuleucel-t (Accessed 9 April 2023).
150. National Comprehensive Cancer Network. NCCN clinical practice guidelines in oncology: prostate cancer (Version 1.2023) . Available at: https://www.nccn.org/guidelines.
151. Peng S, Chen S, Hu W, Mei J, Zeng X, Su T, et al. Combination neoantigen-based dendritic cell vaccination and adoptive T-cell transfer induces antitumor responses against recurrence of hepatocellular carcinoma. Cancer Immunol Res (2022) 10(6):728–44. doi: 10.1158/2326-6066.Cir-21-0931
152. Carreno BM, Magrini V, Becker-Hapak M, Kaabinejadian S, Hundal J, Petti AA, et al. Cancer immunotherapy. a dendritic cell vaccine increases the breadth and diversity of melanoma neoantigen-specific T cells. Sci (New York NY). (2015) 348(6236):803–8. doi: 10.1126/science.aaa3828
153. Ding Z, Li Q, Zhang R, Xie L, Shu Y, Gao S, et al. Personalized neoantigen pulsed dendritic cell vaccine for advanced lung cancer. Signal transduction targeted Ther (2021) 6(1):26. doi: 10.1038/s41392-020-00448-5
154. Fucikova J, Hensler M, Kasikova L, Lanickova T, Pasulka J, Rakova J, et al. An autologous dendritic cell vaccine promotes anticancer immunity in patients with ovarian cancer with low mutational burden and cold tumors. Clin Cancer Res an Off J Am Assoc Cancer Res (2022) 28(14):3053–65. doi: 10.1158/1078-0432.Ccr-21-4413
155. Lau SP, Klaase L, Vink M, Dumas J, Bezemer K, van Krimpen A, et al. Autologous dendritic cells pulsed with allogeneic tumour cell lysate induce tumour-reactive T-cell responses in patients with pancreatic cancer: a phase I study. Eur J Cancer (Oxford Engl 1990). (2022) 169:20–31. doi: 10.1016/j.ejca.2022.03.015
156. Saxena M, Bhardwaj N. Re-emergence of dendritic cell vaccines for cancer treatment. Trends cancer. (2018) 4(2):119–37. doi: 10.1016/j.trecan.2017.12.007
157. Guo Z, Yuan Y, Chen C, Lin J, Ma Q, Liu G, et al. Durable complete response to neoantigen-loaded dendritic-cell vaccine following anti-PD-1 therapy in metastatic gastric cancer. NPJ Precis Oncol (2022) 6(1):34. doi: 10.1038/s41698-022-00279-3
158. Teng CF, Wang T, Shih FY, Shyu WC, Jeng LB. Therapeutic efficacy of dendritic cell vaccine combined with programmed death 1 inhibitor for hepatocellular carcinoma. J Gastroenterol hepatology. (2021) 36(7):1988–96. doi: 10.1111/jgh.15398
159. Barshidi A, Karpisheh V, Noukabadi FK, Kiani FK, Mohammadi M, Afsharimanesh N, et al. Dual blockade of PD-1 and LAG3 immune checkpoints increases dendritic cell vaccine mediated T cell responses in breast cancer model. Pharm Res (2022) 39(8):1851–66. doi: 10.1007/s11095-022-03297-9
160. Chu TH, Vo MC, Park HS, Lakshmi TJ, Jung SH, Kim HJ, et al. Potent anti-myeloma efficacy of dendritic cell therapy in combination with pomalidomide and programmed death-ligand 1 blockade in a preclinical model of multiple myeloma. Cancer immunology immunother CII. (2021) 70(1):31–45. doi: 10.1007/s00262-020-02654-0
161. Vo MC, Jung SH, Chu TH, Lee HJ, Lakshmi TJ, Park HS, et al. Lenalidomide and programmed death-1 blockade synergistically enhances the effects of dendritic cell vaccination in a model of murine myeloma. Front Immunol (2018) 9:1370. doi: 10.3389/fimmu.2018.01370
162. Shi W, Yang X, Xie S, Zhong D, Lin X, Ding Z, et al. A new PD-1-specific nanobody enhances the antitumor activity of T-cells in synergy with dendritic cell vaccine. Cancer letters. (2021) 522:184–97. doi: 10.1016/j.canlet.2021.09.028
163. Yazdani M, Gholizadeh Z, Nikpoor AR, Mohamadian Roshan N, Jaafari MR, Badiee A. Ex vivo dendritic cell-based (DC) vaccine pulsed with a low dose of liposomal antigen and CpG-ODN improved PD-1 blockade immunotherapy. Sci Rep (2021) 11(1):14661. doi: 10.1038/s41598-021-94250-0
164. Teng CF, Wang T, Wu TH, Lin JH, Shih FY, Shyu WC, et al. Combination therapy with dendritic cell vaccine and programmed death ligand 1 immune checkpoint inhibitor for hepatocellular carcinoma in an orthotopic mouse model. Ther Adv Med Oncol (2020) 12:1758835920922034. doi: 10.1177/1758835920922034
165. De Keersmaecker B, Claerhout S, Carrasco J, Bar I, Corthals J, Wilgenhof S, et al. TriMix and tumor antigen mRNA electroporated dendritic cell vaccination plus ipilimumab: link between T-cell activation and clinical responses in advanced melanoma. J immunother Cancer (2020) 8(1):e000329. doi: 10.1136/jitc-2019-000329
166. Zaidi N, Quezada SA, Kuroiwa JMY, Zhang L, Jaffee EM, Steinman RM, et al. Anti-CTLA-4 synergizes with dendritic cell-targeted vaccine to promote IL-3-dependent CD4(+) effector T cell infiltration into murine pancreatic tumors. Ann New York Acad Sci (2019) 1445(1):62–73. doi: 10.1111/nyas.14049
167. Liu L, Wang Y, Miao L, Liu Q, Musetti S, Li J, et al. Combination immunotherapy of MUC1 mRNA nano-vaccine and CTLA-4 blockade effectively inhibits growth of triple negative breast cancer. Mol Ther J Am Soc Gene Ther (2018) 26(1):45–55. doi: 10.1016/j.ymthe.2017.10.020
168. Esmaily M, Masjedi A, Hallaj S, Nabi Afjadi M, Malakotikhah F, Ghani S, et al. Blockade of CTLA-4 increases anti-tumor response inducing potential of dendritic cell vaccine. J Controlled release Off J Controlled Release Society. (2020) 326:63–74. doi: 10.1016/j.jconrel.2020.06.017
169. Sun NY, Chen YL, Lin HW, Chiang YC, Chang CF, Tai YJ, et al. Immune checkpoint ab enhances the antigen-specific anti-tumor effects by modulating both dendritic cells and regulatory T lymphocytes. Cancer letters. (2019) 444:20–34. doi: 10.1016/j.canlet.2018.11.039
170. Ghiringhelli F, Menard C, Puig PE, Ladoire S, Roux S, Martin F, et al. Metronomic cyclophosphamide regimen selectively depletes CD4+CD25+ regulatory T cells and restores T and NK effector functions in end stage cancer patients. Cancer immunology immunother CII. (2007) 56(5):641–8. doi: 10.1007/s00262-006-0225-8
171. Cornelissen R, Hegmans JP, Maat AP, Kaijen-Lambers ME, Bezemer K, Hendriks RW, et al. Extended tumor control after dendritic cell vaccination with low-dose cyclophosphamide as adjuvant treatment in patients with malignant pleural mesothelioma. Am J Respir Crit Care Med (2016) 193(9):1023–31. doi: 10.1164/rccm.201508-1573OC
172. Liau LM, Ashkan K, Brem S, Campian JL, Trusheim JE, Iwamoto FM, et al. Association of autologous tumor lysate-loaded dendritic cell vaccination with extension of survival among patients with newly diagnosed and recurrent glioblastoma: a phase 3 prospective externally controlled cohort trial. JAMA Oncol (2023) 9(1):112–21. doi: 10.1001/jamaoncol.2022.5370
173. Storkus WJ, Maurer D, Lin Y, Ding F, Bose A, Lowe D, et al. Dendritic cell vaccines targeting tumor blood vessel antigens in combination with dasatinib induce therapeutic immune responses in patients with checkpoint-refractory advanced melanoma. J immunother Cancer (2021) 9(11):e003675. doi: 10.1136/jitc-2021-003675
Keywords: dendritic cell, tumor microenvironment, immune tolerance, immunosuppressive populations, DC-based vaccine
Citation: Xiao Z, Wang R, Wang X, Yang H, Dong J, He X, Yang Y, Guo J, Cui J and Zhou Z (2023) Impaired function of dendritic cells within the tumor microenvironment. Front. Immunol. 14:1213629. doi: 10.3389/fimmu.2023.1213629
Received: 28 April 2023; Accepted: 13 June 2023;
Published: 27 June 2023.
Edited by:
Juana Serrano Lopez, Health Research Institute Foundation Jimenez Diaz (IIS-FJD), SpainReviewed by:
Joaquin Sanchez-Garcia, Hospital Universitario Reina Sofía, SpainDong-Ming Kuang, Sun Yat-sen University, China
Charles Antoine Dutertre, Institut National de la Santé et de la Recherche Médicale (INSERM), France
Lei Xue, Tongji University, China
Copyright © 2023 Xiao, Wang, Wang, Yang, Dong, He, Yang, Guo, Cui and Zhou. This is an open-access article distributed under the terms of the Creative Commons Attribution License (CC BY). The use, distribution or reproduction in other forums is permitted, provided the original author(s) and the copyright owner(s) are credited and that the original publication in this journal is cited, in accordance with accepted academic practice. No use, distribution or reproduction is permitted which does not comply with these terms.
*Correspondence: Zhiling Zhou, emhvdXpoaWxpbmdAZXh0LmpudS5lZHUuY24=
†These authors have contributed equally to this work and share first authorship